- 1Robert W. Holley Center for Agriculture and Health, USDA-Agricultural Research Service, Cornell University, Ithaca, NY, United States
- 2State Key Laboratory of Crop Genetics and Germplasm Enhancement, Nanjing Agricultural University, Nanjing, China
- 3Plant Breeding and Genetics Section, School of Integrative Plant Science, Cornell University, Ithaca, NY, United States
- 4ScreenSYS GmbH, Freiburg, Germany
Phytoene synthase (PSY) catalyzes the first committed step in the carotenoid biosynthesis pathway and is a major rate-limiting enzyme of carotenogenesis. PSY is highly regulated by various regulators and factors to modulate carotenoid biosynthesis in response to diverse developmental and environmental cues. Because of its critical role in controlling the total amount of synthesized carotenoids, PSY has been extensively investigated and engineered in plant species. However, much remains to be learned on its multifaceted regulatory control and its catalytic efficiency for carotenoid enrichment in crops. Here, we present current knowledge on the basic biology, the functional evolution, the dynamic regulation, and the metabolic engineering of PSY. We also discuss the open questions and gaps to stimulate additional research on this most studied gene/enzyme in the carotenogenic pathway.
Introduction
Carotenoids are a group of lipophilic isoprenoid metabolites. They play diverse roles in plants as essential photoprotective and light-harvesting pigments in photosynthesis, color agents, and precursors of phytohormones, aroma/flavor compounds, and signaling molecules. Carotenoids are also important to human nutrition and health as dietary precursors of vitamin A and antioxidants in preventing vitamin A deficiency and reducing the risk of various chronic diseases (Eggersdorfer and Wyss, 2018). The critical roles of carotenoids to plants and humans have provoked significant efforts to understand carotenoid metabolism in plants and to generate carotenoid enriched crops (Nisar et al., 2015; Giuliano, 2017; Rodriguez-Concepcion et al., 2018; Zheng et al., 2020; Sun et al., 2022).
Carotenoids are de novo synthesized in nearly all kinds of plastids and are abundant in chloroplasts and chromoplasts in plant cells (Sun et al., 2018). Carotenoid biosynthesis occurs primarily in dependence of plastid membrane association and involves a group of nuclear-encoded enzymes (Shumskaya and Wurtzel, 2013). Phytoene synthase (PSY) catalyzes the first committed step of carotenogenesis by condensation of two molecules of geranylgeranyl diphosphate (GGPP) derived from the methylerythritol phosphate (MEP) pathway to produce the C40 hydrocarbon 15-cis-phytoene (Figure 1). Consecutive modifications of 15-cis-phytoene by desaturases and isomerases form all-trans-lycopene, which is cyclized by two cyclases to convert into either β-carotene or α-carotene. The subsequent hydroxylation and epoxidation by hydroxylases and epoxidase produce xanthophylls (Hermanns et al., 2020; Sun et al., 2020). Being a major rate-limiting and highly regulated enzyme, PSY has been extensively investigated and engineered in plant species (Burkhardt et al., 1997; Shewmaker et al., 1999; Ye et al., 2000; Ducreux et al., 2005; Paine et al., 2005; Diretto et al., 2007; Fraser et al., 2007; Maass et al., 2009; Naqvi et al., 2009; Rodriguez-Villalon et al., 2009; Welsch et al., 2010, 2018; Zhou et al., 2015; Zhu et al., 2018; Cao et al., 2019; Sun et al., 2021).
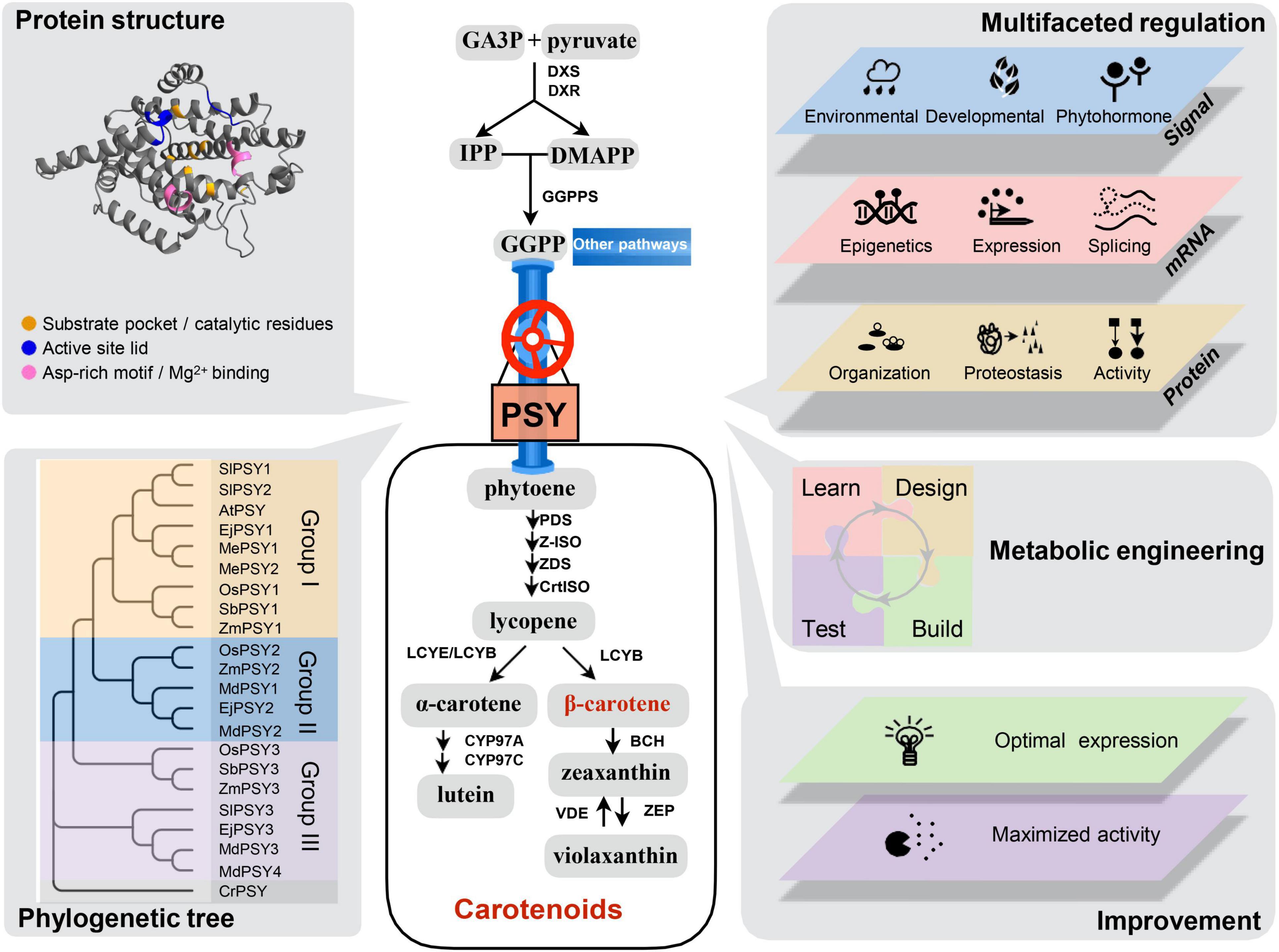
Figure 1. Overview of plant carotenoid biosynthesis pathway and the biology of plant phytoene synthase. Carotenoid biosynthesis starts with GA3P and pyruvate to produce the direct precursor of GGPP. PSY is the first committed and major-rate limiting enzyme in the carotenoid biosynthesis pathway. β-carotene is the most potent provitamin A carotenoid. Abbreviations for metabolites and pathway enzymes can be found in Sun et al. (2022). Protein structure: A high confident structure prediction of Arabidopsis PSY protein (https://www.alphafold.ebi.ac.uk/entry/P37271) with conserved motifs of enzymatic active site indicated. Phylogenetic tree: Land plant PSY proteins can be divided into three large groups (I, II, and III) and PSY genes in each group show tissue-specific expression pattern. Multifaceted regulation: PSY gene expression and activity are under multifaceted regulation by various signals and factors at epigenetic, transcriptional, post-transcriptional, and post-translational levels. Metabolic engineering: PSY is the main target gene of carotenoid metabolic engineering. The design of genetic manipulation of PSY for carotenoid enrichment benefits from the learning process. Improvement: Further understanding of the regulatory mechanisms of PSY and maximization of its activity will enable predictable engineering and breeding to achieve the targeted goals of carotenoid improvement in crops. Sl, Solanum lycopersicum; At, Arabidopsis thaliana; Ej, Eriobotrya japonica; Me, Manihot esculenta; Os, Oryza sativa; Sb, Sorghum bicolor; Zm, Zea mays; Md, Malus domestica; Cr, Chlamydomonas reinhardtii. The database accession numbers of sequences used are: AtPSY (AAA32836), EjPSY1 (KF922363), EjPSY2A (KF922364), EjPSY3 (KF922367), MdPSY1 (KT189149), MdPSY2 (KT189150), MdPSY3 (KT189151), MdPSY4 (KT189152), MePSY1 (ACY42666), MePSY2 (ACY42670), OsPSY1 (AAS18307), OsPSY2 (AK073290), OsPSY3 (DQ356431), SbPSY1 (AY705389), SbPSY3 (AAW28997), SlPSY1 (ABM45873), SlPSY2 (ABU40771), SlPSY3 (Solyc01g005940), ZmPSY1 (AX13806), ZmPSY2 (AAQ91837), ZmPSY3 (DQ356430), and CrPSY (Q6J214).
The Basic Biology of Phytoene Synthase
The first gene encoding PSY from land plants was identified from tomato. A tomato fruit cDNA, pTOM5, which was previously shown to be highly upregulated during fruit ripening, was cloned (Slater et al., 1985). Sequencing of pTOM5 revealed homology to the bacterial crtB (Ray et al., 1987) and the pTOM5 antisense tomato plants showed strongly reduced carotenoid levels in the fruit (Bird et al., 1991). Further evidence that pTOM5 encodes a phyotene synthase came from complementation of carotenoid biosynthesis in the crtB mutant of Rhodobacter capsulatus by pTOM5, which finally confirmed its identify as fruit-specific PSY1 (Bartley et al., 1992).
Phytoene synthase enzyme (EC 2.5.1.32) was characterized as a bifunctional enzyme, which catalyzes two tightly coupled reactions of dimerization of GGPP into prephytoene diphosphate and its subsequent conversion into phytoene (Dogbo et al., 1988). Enzymatic activity of PSY is strictly dependent on Mn2+ cofactor, which is believed to regulate its competition with other GGPP-consuming enzymes in plastids (Dogbo et al., 1988; Fraser et al., 2000). PSY also has a specific galactolipid and membrane association requirement for its catalytic activity (Schledz et al., 1996; Welsch et al., 2000). In general, plant PSY proteins are unstable (Mukherjee and Mukhopadhyay, 2020). This is especially true in photosynthetically active tissues, where PSY protein amounts are extremely low and subjected to a relatively high protein turnover rate. Many PSYs are known to require chaperone proteins for maintaining their stability and carotenogenic functions within plastids (Zhou et al., 2015; Park et al., 2016; Chayut et al., 2017; D’andrea et al., 2018; Welsch et al., 2018; Ahrazem et al., 2020).
Phytoene synthase belongs to the class 1 superfamily of isoprenoid biosynthetic enzymes and shares a conserved prenyltransferase domain with squalene synthase (Summers et al., 1993). PSY proteins of land plants generally contain 380–450 amino acid residues (Han et al., 2015). The active site of PSY enzymes is comprised of six conserved motifs, i.e., substrate binding pocket, catalytic residues, active lid-residues, two aspartate-rich regions, and substrate Mg2+ binding site (GGPP is usually complexed with Mg2+) (Figure 1). Most residues of the active site are identical and conserved even with the bacterial and fungal orthologs (Mukherjee and Mukhopadhyay, 2020). The three-dimensional structure modeling shows an identical structure of the active site region of PSY enzymes from evolutionarily distant lineages, despite that their global structure vary (Cao et al., 2019; Mukherjee and Mukhopadhyay, 2020).
Functional Evolution of Phytoene Synthase
Gene duplication plays a prominent role in generating evolutionary novelty, facilitating acclimation and adaptation to adverse environments and contributing to the emergence of new agronomic traits (Conant and Wolfe, 2008; Van De Peer et al., 2017). Gene duplication events and a separate evolutionary history in land plants formed a small gene family and produced a various number of PSY genes in plant genomes.
In many plant species, there are two or more PSY paralogs belonging to three subgroups (Figure 1). Numbering of PSY gene nomination in tomato followed the chronological order of their identification and PSY paralogs identified subsequently in other plant species followed the same principle or are based on homologies to previously identified PSY genes. Thus, PSY numbering does not always expresses similarity of functional roles. Various PSY paralogs were recruited with overlapping functions in carotenogenesis during evolution. They acquired tissue-specific expression patterns and subfunctionalizations to fine-tune carotenoid biosynthesis in response to developmental and environmental cues (Li et al., 2008b). For instance, tomato, maize, and wheat genomes harbor three PSY genes, with PSY1 primarily responsible for carotenoid accumulation in fruit or grains, PSY2 functioning in green tissues for photosynthesis, and PSY3 in roots to regulate ABA biosynthesis under abiotic stress or mycorrhizal symbiosis (Fraser et al., 1999; Giorio et al., 2008; Li et al., 2008a; Dibari et al., 2012; Stauder et al., 2018). In rice, both OsPSY1 and OsPSY2 contain light responsive cis-acting elements and play predominant roles in carotenogenesis in green tissues, whereas OsPSY3 is induced in roots by high salt and/or drought stress (Welsch et al., 2008). The expression and functional evolution of PSY paralogs have been also observed in many other plant species. Interestingly, although Arabidopsis has experienced four gene duplication events (Bowers et al., 2003), there is only a single PSY gene. Perhaps the alternative splicing of PSY in Arabidopsis allows encoding multiple PSY isozymes to adapt to the changing environments (Alvarez et al., 2016).
While the key sites or motifs of plant PSYs are highly conserved during the enzyme evolution (Han et al., 2015; Cao et al., 2019), evolutionary processes with gene duplication, divergence, and allelic variations generated PSY enzymes with different activities among and even within the same plant species. An example is the finding that an alteration of evolutionarily conserved neighboring aromatic-aromatic amino acid combination in the PSY active site gives variable activities of PSY isoforms in tomato (Cao et al., 2019). These isoforms also evolved with different biochemical properties for the requirements of Mn2+ cofactor, optimal pH, and substrate affinity (Fraser et al., 2000). In cassava, a divergence of one amino acid residue in a highly conserved structure region of PSY2 altered its catalytic activity and is associated with root carotenoid content in cassava (Welsch et al., 2010). Allelic variations resulting in loss of PSY activity led to white-fleshed cultivars in loquat (Fu et al., 2014) and white petal varieties of the California poppy (Pollack et al., 2019). Moreover, allelic variation was found to give PSY isozymes distinct plastid suborganellar localization and presumably altered activity with different carotenoid sequestration structures in maize (Shumskaya et al., 2012). Uneven evolution of domains and regions of PSY was noticed in grass species (Fu et al., 2010). These examples underscore the profound impact of evolutionary processes on PSY activity and in shaping agronomic traits.
Multifaceted Regulation of Phytoene Synthase
Transcriptional Regulation
Phytoene synthase is a major rate-limiting enzyme in carotenoid biosynthesis and its activity effectively determines the metabolic flux to carotenoids. It is therefore not surprising that multiple mechanisms at various levels are utilized to regulate the spatiotemporal expression and activity of PSY in plants (Figure 1; Ruiz-Sola and Rodríguez-Concepción, 2012; Sun and Li, 2020; Sun et al., 2022). Transcriptional regulation is central to the control of PSY activity for carotenogenesis. Various signals and factors, such as development, phytohormone, retrograde, light, temperature, drought, salt, and circadian, as well as allelic variation, mutation, and feedback/feedforward are all known in the literature to regulate PSY gene expression. However, there is a large gap for the mechanistic understanding of their regulatory roles.
A number of transcription factors (TFs) were found to directly bind to the promoters of PSY and regulate its transcript levels in photosynthetic and non-photosynthetic tissues. During seedling de-etiolation, PSY transcript level is greatly induced by light (Von Lintig et al., 1997; Welsch et al., 2000). Two key photomorphogenetic regulators, Phytochrome-Interacting Factors (PIFs, repressors) and LONG HYPOCOTYL5 (HY5, activator) form a dynamic repression-activation module. They directly bind to the same G-box motif in the PSY promoter in dark or light to regulate PSY transcription in response to light, temperature, and circadian cues (Toledo-Ortiz et al., 2010, 2014). A different repression-activation module involving PIF1 and Phytochrome Rapidly Regulated 1 (PAR1) is recruited to regulate PSY expression in response to shade (Bou-Torrent et al., 2015). In addition, RAP2.2 binds to the ATCTA element in the PSY promoter with modest regulatory activity, suggesting RAP2.2 being one element of the complex regulatory network (Welsch et al., 2007).
Many fruit-specific PSYs are transcriptionally upregulated during fruit ripening (Lu P. et al., 2018). Several TFs including Ripening Inhibitor (RIN), Tomato AGAMOUS-LIKE1 (TAGL1), FRUITFULL1 (FUL1), SlBBX20, SlWRKY, SlMYB72, and SlPIF1 directly bind to the SlPSY1 promoter to positively or negatively regulate its expression in tomato fruit (Itkin et al., 2009; Vrebalov et al., 2009; Fujisawa et al., 2013, 2014; Llorente et al., 2016; Xiong et al., 2019; Wu et al., 2020). In citrus, both CsMADS5 and CsMADS6 bind and regulate CsPSY transcription and these two TFs physically interact with each other, possibly forming an enhancer complex to promote carotenogenic activity (Lu S. et al., 2018; Lu et al., 2021). While multiple TFs are shown to directly activate PSY gene expression in non-photosynthetic tissues, it remains unclear whether they are the primary regulators, function across plant species, form regulatory modules with others, and/or represent bona fide regulators of PSY.
Modulation of PSY expression has also been documented by other mechanisms. Uncharacterized carotenoids or degraded products were found to negatively regulate SlPSY1 in tomato or PSY protein level in carrot root, constituting a negative feedback regulation of PSY activity (Kachanovsky et al., 2012; Arango et al., 2014; Enfissi et al., 2017). Perturbation of carotenoid biosynthesis has also been observed to activate PSY2 activity in tomato and pepper fruits when PSY1 is not functional (Jang et al., 2020; Gupta et al., 2022; Karniel et al., 2022). Epigenetic regulation such as histone modification as well as DNA methylation and demethylation modifies SlPSY1 mRNA levels during tomato fruit ripening (Liang et al., 2017; Liu et al., 2020; Sun et al., 2022).
Post-transcriptional and Post-translational Regulation
Additionally, PSY expression and enzymatic activity are also regulated at post-transcriptional and post-translational levels (Figure 1). Mechanisms comprising of alternative splicing, protein-protein interactions, and multi-enzyme complexes constitute efficient, rapid, and dynamic regulation to fine-tune PSY activity and carotenogenesis (Ruiz-Sola and Rodríguez-Concepción, 2012; Nisar et al., 2015; Sun and Li, 2020; Sun et al., 2022).
Alternative splicing changes PSY transcript sequence length, producing variants with different translation efficiency and/or distinct enzyme activity to control the functional PSY in Arabidopsis leaves (Alvarez et al., 2016), tomato fruit (Chen et al., 2019), saffron stigmata (Ahrazem et al., 2019), and bread wheat endosperm (Howitt et al., 2009). Such post-transcriptional regulatory mechanisms may also provide an alternative toward employing multiple copies of a gene. Moreover, regulation of translational activity via the 5’UTR of PSY transcripts might represent a regulatory mode that allows a fast adaptation of PSY protein abundance and thus biosynthetic activity toward carotenoid requirement. Considering that PSY translation takes place in the cytoplasm while (lipophilic) carotenoids are present in plastids, an attractive hypothesis includes shuttling of regulatory, hydrophilic carotenoid degradation products via plastid membranes (Alvarez et al., 2016).
Protein-protein interaction is fundamentally important to maintain and fine-tune metabolic processes in plant cells (Struk et al., 2019). The interactions of PSY protein with OR chaperones and Clp proteases exemplify the crucial role of protein-protein interactions in regulating carotenogenic enzyme activity, proteostasis, and carotenoid biosynthesis. PSY physically interacts with OR proteins to maintain its activity through OR chaperone activity (Yuan et al., 2015; Zhou et al., 2015; Park et al., 2016; Chayut et al., 2017; Welsch et al., 2020). PSY directly interacts with Clp protease to mediate its degradation (D’andrea et al., 2018; Welsch et al., 2018). Through the protein-protein interactions, OR and Clp proteins counterbalance each other to adjust the functional form and proteostasis of PSY in plant cells. In addition, PSY is associated with GGPPS to facilitate channeling of precursor for carotenoid biosynthesis in Arabidopsis, pepper and tomato (Ruiz-Sola et al., 2016; Wang et al., 2018; Barja et al., 2021) SGR was found to physically interact with CsPSY protein to regulate carotenogenesis in citrus (Zhu et al., 2021). Post-translational modification via ubiquitination-mediated turnover of non-imported PSY precursors outside of plastids was noted via direct interaction between SlPSY1 and a E3 ubiquitin ligase (Wang et al., 2020).
Enzyme complexes and metabolons facilitate metabolic flux. As lipophilic carotenoids are synthesized from small hydrophilic precursors (IPP, DMAPP), but include amphiphilic intermediates like GGPP, free substrate diffusion can be excluded and metabolite channeling between different enzymes of the pathway plays an essential role. Importantly, the PSY substrate GGPP is shared with other pathways (Figure 1), such as chlorophyll and tocopherol biosynthesis in chloroplasts. However, there are mainly one or two GGPPS isoenzymes that serve all GGPP-consuming pathways (Ruiz-Sola et al., 2016; Barja et al., 2021). This situation raises the question whether competitive interaction between enzymes of off-branching pathways regulates flux into various pathways. Interestingly, a synthetic GGPPS-PSY metabolon has been demonstrated with increased efficiency in channelizing GGPP into carotenogenesis (Camagna et al., 2019). An early study also suggests that PSY is associated with a large enzyme complex containing IPI and GGPPS in chloroplasts of tomato plants for active carotenogenesis (Fraser et al., 2000).
Genetic Engineering of Phytoene Synthase
Since carotenoids, as important nutrients in human diets, are deficient or low in many food crops, enormous endeavors have been made to engineer crops with enriched carotenoid content (Giuliano, 2017; Zheng et al., 2020). The learning process of multifaceted regulation provides constitutive input to design, build, and test metabolic engineering module of carotenoids (Figure 1). PSY is the main target for manipulation as it is the major rate-limiting and key enzyme driving the flux into carotenogenesis. Because PSY genes are not expressed in rice endosperm in all rice germplasms, the use of genetic engineering to obtain the capacity for carotenoid biosynthesis is required for Golden Rice (Beyer et al., 2002). Endosperm specific expression of a highly efficient ZmPSY along with a bacterial phytoene desaturase (crtI) generated β-carotene enriched Golden Rice (Paine et al., 2005) and CRISPR golden rice (Dong et al., 2020), as well as orange maize (Naqvi et al., 2009) and astaxanthin rice with two additional genes (Zhu et al., 2018). Tissue-specific expression of plant or bacteria PSY also dramatically increases α-carotene and β-carotene in many tissues such as wheat grain (Wang et al., 2014), canola seeds (Shewmaker et al., 1999), cotton seed (Yao et al., 2018), soybean seed (Schmidt et al., 2015), eggplant fruit (Mishiba et al., 2020), Arabidopsis seed (Lindgren et al., 2003; Sun et al., 2021), and potato tuber (Ducreux et al., 2005). Constitutive expression of PSY either under CaMV35S or other promoters such as maize polyubiquitin shows enhanced carotenoid levels in tomato fruit (Fray et al., 1995; Fraser et al., 2007), banana (Paul et al., 2017), cassava root (Welsch et al., 2010), and carrot root (Maass et al., 2009), although undesirable or pleiotropic phenotypes by constitutive PSY expression are observed in some cases.
Manipulation of PSY with or without other carotenogenic genes often results in increased carotenoid levels. However, the synthesized carotenoids are subjected to oxidative degradation, particularly during grain seed maturation and post-harvest storage such as in Golden Rice, sorghum, and maize (Farre et al., 2013; Che et al., 2016; Schaub et al., 2017). Co-expression of PSY1 with barley homogentisate geranylgeranyl transferase (HGGT) to increase antioxidant vitamin E provides a strategy to improve β-carotene content and stability as shown in sorghum grain (Che et al., 2016). In addition, co-expression of PSY with ORHis, a nature variant of OR that induces chromoplast biogenesis, offers an additional strategy to significantly boost carotenoid accumulation and stability during seed maturation and storage as documented in Arabidopsis seed (Sun et al., 2021). Expression of an OR mutant variant has also shown to enhance carotenoid levels and stability in potato and sweetpotato (Lu et al., 2006; Li et al., 2012; Kim et al., 2021). No other regulators were tested for carotenoid enrichments in starch-rich organs despite of their reported roles in mediating carotenogenesis.
The intrinsic properties of PSY determine its efficiency. Numerous examples exemplify a simple change in PSY amino acid sequences that profoundly alters its activity (Welsch et al., 2010; Shumskaya et al., 2012; Cao et al., 2019), which provides the potential to rationally design PSY with high enzyme activity. Various approaches are available to facilitate rapid screening of PSY variants with improved efficiency, such as via heterologous expression in bacterial test system and in callus system, which seems transferable to various plant systems (Paine et al., 2005; Bai et al., 2014; Schaub et al., 2018; Camagna et al., 2019; Cao et al., 2019; Camagna and Welsch, 2020). Application of improved PSY variants is expected to effectively develop carotenoid enriched crops.
PSY is known to be the key rate-limiting enzyme of carotenogenesis. However, the availability of precursors and products following altering pathway enzyme expression and activities can create new metabolic bottlenecks in the pathway (Bai et al., 2016, 2017). Indeed, metabolic control analysis reveals that the flux control coefficient of PSY1 is predominant in the wild type fruit but reduces in the PSY transgenic tomato, and consequently the flux control is likely shared by several enzymes in the carotenoid pathway (Rios-Estepa and Lange, 2007). Thus, effective engineering of carotenoid metabolic pathway may benefit from multistep manipulation in some cases.
Conclusion and Future Research
Because of its critical role in carotenogenesis, PSY is the most well characterized and studied gene/enzyme in the carotenoid biosynthesis pathway. The accelerated sequencing of numerous plant genomes and transcriptomes makes it straightforward to identify PSY gene family members and to reveal their tissue-specific expression patterns in plant species. Extensive studies of PSY in carotenogenesis have also established various regulatory tiers acting at transcriptional, post-transcriptional, post-translational, and epigenetic levels. At the same time, great success has been made in engineering PSY expression to enhance crop nutritional quality. However, considerably more efforts are still required to delineate the mechanistic details of various regulatory controls and to develop/select highly efficient PSY enzyme (Figure 1). Open questions and gaps for the most studied enzyme in the pathway remain, including the followings.
What are the additional regulators that govern PSY gene expression, protein stability, and activity? The crucial role of PSY in carotenogenesis and multiple signals/factors affecting its expression suggest a complex regulatory control machinery and network. Although a number of TFs have been shown to bind directly to the cis-motifs in the promoters of PSY, additional regulators that may have dominant or additive roles in transcriptionally regulating PSY remain uncovered. In addition to a few post-translational regulators identified, many more are expected to be identified for rapid regulation of PSY activity and fine-tune of carotenogenesis.
What are the key amino acid residues that give high PSY activity? The intrinsic enzymatic efficiency of PSY enzymes from various plant species varies greatly to give different biosynthetic capacity as exemplified with daffodil and maize PSY used for Golden Rice 1 and 2, respectively. Single amino acid sequence changes can profoundly alter PSY activity. Therefore, identification and optimization of the key amino acid residues will not only unravel the intrinsic features of PSY activity, but also facilitate the design of highly efficient PSY for development of carotenoid enriched crops.
How does PSY work with other enzymes in the pathway to drive the metabolic flux and what are the mechanisms controlling metabolon assembly? It has long been hypothesized that carotenogenic enzymes form enzyme complexes although solid evidence is still lacking. GGPS-PSY metabolon can efficiently drive GGPP substrate into the carotenogenic pathway, supporting an important role of the complexes for carotenogenesis.
One interesting phenomenon observed in many studies is that overexpression of PSY is often associated with increased β-carotene accumulation in many non-photosynthetic tissues or organs. What are the regulatory mechanisms for this favorable phenomenon? Identification of the mechanisms involved might further help to develop additional approaches for crop nutritional quality improvement.
Phytoene synthase activity needs to be precisely coordinated with carotenoid end products. Of special interest for future research also includes how the rate-limiting function of PSY is dynamically regulated with the total carotenoid content and the relative levels of individual carotenoids.
The fast advance in techniques and multi-omics information from many plant species will be promising in elucidating the unknown regulators of PSY and unraveling the intrinsic features underlying its activity and function. The information obtained would greatly enrich our current knowledge of the regulatory mechanisms and lay down novel strategies for predictable carotenoid improvement in crops. Integrating both key regulators and targeted carotenogenic genes into a synthetic biology framework will allow us to achieve the targeted biofortification goals.
Author Contributions
XZ wrote the Sections “Functional Evolution of Phytoene Synthase” and “Genetic Engineering of Phytoene Synthase” as well as formatted the references. SR wrote the Sections “Basic Biology of Phytoene Synthase” and “Multifaceted Regulation of Phytoene Synthase.” EW wrote the Section “Introduction.” TS designed Figure 1. TS, AL, and RW critically revised the manuscript. LL prepared the final version with input of all authors. All authors contributed to the article and approved the submitted version.
Funding
This work was supported by Agriculture and Food Research Initiative competitive award grant no. 2019-67013-29162 (to LL) from the USDA National Institute of Food and Agriculture and USDA-ARS base fund. XZ acknowledges the support of the China Scholarship Council (CSC).
Conflict of Interest
RW was employed by ScreenSYS GmbH.
The remaining authors declare that the research was conducted in the absence of any commercial or financial relationships that could be construed as a potential conflict of interest.
Publisher’s Note
All claims expressed in this article are solely those of the authors and do not necessarily represent those of their affiliated organizations, or those of the publisher, the editors and the reviewers. Any product that may be evaluated in this article, or claim that may be made by its manufacturer, is not guaranteed or endorsed by the publisher.
References
Ahrazem, O., Diretto, G., Argandoña Picazo, J., Fiore, A., Rubio-Moraga, A., Rial, C., et al. (2019). The specialized roles in carotenogenesis and apocarotenogenesis of the phytoene synthase gene family in saffron. Front. Plant Sci. 10:249. doi: 10.3389/fpls.2019.00249
Ahrazem, O., Lopez, A. J., Argandona, J., Castillo, R., Rubio-Moraga, A., and Gomez-Gomez, L. (2020). Differential interaction of Or proteins with the PSY enzymes in saffron. Sci. Rep. 10:552. doi: 10.1038/s41598-020-57480-2
Alvarez, D., Voss, B., Maass, D., Wust, F., Schaub, P., Beyer, P., et al. (2016). Carotenogenesis is regulated by 5’UTR-mediated translation of phytoene synthase splice variants. Plant Physiol. 172, 2314–2326. doi: 10.1104/pp.16.01262
Arango, J., Jourdan, M., Geoffriau, E., Beyer, P., and Welsch, R. (2014). Carotene hydroxylase activity determines the levels of both α-carotene and total carotenoids in orange carrots. Plant Cell 26, 2223–2233. doi: 10.1105/tpc.113.122127
Bai, C., Berman, J., Farre, G., Capell, T., Sandmann, G., Christou, P., et al. (2017). Reconstruction of the astaxanthin biosynthesis pathway in rice endosperm reveals a metabolic bottleneck at the level of endogenous beta-carotene hydroxylase activity. Transgenic Res. 26, 13–23. doi: 10.1007/s11248-016-9977-x
Bai, C., Capell, T., Berman, J., Medina, V., Sandmann, G., Christou, P., et al. (2016). Bottlenecks in carotenoid biosynthesis and accumulation in rice endosperm are influenced by the precursor-product balance. Plant Biotechnol. J. 14, 195–205. doi: 10.1111/pbi.12373
Bai, C., Rivera, S. M., Medina, V., Alves, R., Vilaprinyo, E., Sorribas, A., et al. (2014). An in vitro system for the rapid functional characterization of genes involved in carotenoid biosynthesis and accumulation. Plant J. 77, 464–475. doi: 10.1111/tpj.12384
Barja, M. V., Ezquerro, M., Beretta, S., Diretto, G., Florez-Sarasa, I., Feixes, E., et al. (2021). Several geranylgeranyl diphosphate synthase isoforms supply metabolic substrates for carotenoid biosynthesis in tomato. New Phytol. 231, 255–272. doi: 10.1111/nph.17283
Bartley, G. E., Viitanen, P. V., Bacot, K. O., and Scolnik, P. A. (1992). A tomato gene expressed during fruit ripening encodes an enzyme of the carotenoid biosynthesis pathway. J. Biol. Chem. 267, 5036–5039. (18)42724-x doi: 10.1016/s0021-9258
Beyer, P., Al-Babili, S., Ye, X., Lucca, P., Schaub, P., Welsch, R., et al. (2002). Golden Rice: introducing the beta-carotene biosynthesis pathway into rice endosperm by genetic engineering to defeat vitamin A deficiency. J. Nutr. 132, 506S–510S. doi: 10.1093/jn/132.3.506S
Bird, C. R., Ray, J. A., Fletcher, J. D., Boniwell, J. M., Bird, A. S., Teulieres, C., et al. (1991). Using antisense RNA to study gene function: Inhibition of carotenoid biosynthesis in transgenic tomatoes. Nat. Biotechnol. 9, 635–639. doi: 10.1038/nbt0791-635
Bou-Torrent, J., Toledo-Ortiz, G., Ortiz-Alcaide, M., Cifuentes-Esquivel, N., Halliday, K. J., Martinez-García, J. F., et al. (2015). Regulation of carotenoid biosynthesis by shade relies on specific subsets of antagonistic transcription factors and cofactors. Plant Physiol. 169, 1584–1594. doi: 10.1104/pp.15.00552
Bowers, J. E., Chapman, B. A., Rong, J., and Paterson, A. H. (2003). Unravelling angiosperm genome evolution by phylogenetic analysis of chromosomal duplication events. Nature 422, 433–438. doi: 10.1038/nature01521
Burkhardt, P. K., Beyer, P., Wunn, J., Kloti, A., Armstrong, G. A., Schledz, M., et al. (1997). Transgenic rice (Oryza sativa) endosperm expressing daffodil (Narcissus pseudonarcissus) phytoene synthase accumulates phytoene, a key intermediate of provitamin A biosynthesis. Plant J. 11, 1071–1078. doi: 10.1046/j.1365-313x.1997.11051071.x
Camagna, M., Grundmann, A., Bar, C., Koschmieder, J., Beyer, P., and Welsch, R. (2019). Enzyme fusion removes competition for geranylgeranyl diphosphate in carotenogenesis. Plant Physiol. 179, 1013–1027. doi: 10.1104/pp.18.01026
Camagna, M., and Welsch, R. (2020). Expression, Purification, and enzyme activity assay of phytoene synthase in vitro. Methods Mol. Biol. 2083, 39–52. doi: 10.1007/978-1-4939-9952-1_3
Cao, H., Luo, H., Yuan, H., Eissa, M. A., Thannhauser, T. W., Welsch, R., et al. (2019). A neighboring aromatic-aromatic amino acid combination governs activity divergence between tomato phytoene synthases. Plant Physiol. 180, 1988–2003. doi: 10.1104/pp.19.00384
Chayut, N., Yuan, H., Ohali, S., Meir, A., Sa’ar, U., Tzuri, G., et al. (2017). Distinct mechanisms of the ORANGE protein in controlling carotenoid flux. Plant Physiol. 173, 376–389. doi: 10.1104/pp.16.01256
Che, P., Zhao, Z. Y., Glassman, K., Dolde, D., Hu, T. X., and Jones, T. J. (2016). Elevated vitamin E content improves all-trans beta-carotene accumulation and stability in biofortified sorghum. Proc. Natl. Acad. Sci. U. S. A 113:E8209. doi: 10.1073/pnas.1605689113
Chen, L., Li, W., Li, Y., Feng, X., Du, K., Wang, G., et al. (2019). Identified trans-splicing of YELLOW-FRUITED TOMATO 2 encoding the PHYTOENE SYNTHASE 1 protein alters fruit color by map-based cloning, functional complementation and RACE. Plant Mol. Biol. 100, 647–658. doi: 10.1007/s11103-019-00886-y
Conant, G. C., and Wolfe, K. H. (2008). Turning a hobby into a job: how duplicated genes find new functions. Nat. Rev. Genet. 9, 938–950. doi: 10.1038/nrg2482
D’andrea, L., Simon-Moya, M., Llorente, B., Llamas, E., Marro, M., Loza-Alvarez, P., et al. (2018). Interference with Clp protease impairs carotenoid accumulation during tomato fruit ripening. J. Exp. Bot. 69, 1557–1568. doi: 10.1093/jxb/erx491
Dibari, B., Murat, F., Chosson, A., Gautier, V., Poncet, C., Lecomte, P., et al. (2012). Deciphering the genomic structure, function and evolution of carotenogenesis related phytoene synthases in grasses. BMC Genom. 13:221. doi: 10.1186/1471-2164-13-221
Diretto, G., Al-Babili, S., Tavazza, R., Papacchioli, V., Beyer, P., and Giuliano, G. (2007). Metabolic engineering of potato carotenoid content through tuber-specific overexpression of a bacterial mini-pathway. PLoS One 2:e350. doi: 10.1371/journal.pone.0000350
Dogbo, O., Laferriére, A., D’harlingue, A., and Camara, B. (1988). Carotenoid biosynthesis: Isolation and characterization of a bifunctional enzyme catalyzing the synthesis of phytoene. Proc. Natl. Acad. Sci. U. S. A. 85, 7054–7058. doi: 10.1073/pnas.85.19.7054
Dong, O. X., Yu, S., Jain, R., Zhang, N., Duong, P. Q., Butler, C., et al. (2020). Marker-free carotenoid-enriched rice generated through targeted gene insertion using CRISPR-Cas9. Nat. Commun. 11:1178. doi: 10.1038/s41467-020-14981-y
Ducreux, L. J., Morris, W. L., Hedley, P. E., Shepherd, T., Davies, H. V., Millam, S., et al. (2005). Metabolic engineering of high carotenoid potato tubers containing enhanced levels of beta-carotene and lutein. J. Exp. Bot. 56, 81–89. doi: 10.1093/jxb/eri016
Eggersdorfer, M., and Wyss, A. (2018). Carotenoids in human nutrition and health. Arch. Biochem. Biophys. 652, 18–26. doi: 10.1016/j.abb.2018.06.001
Enfissi, E. M., Nogueira, M., Bramley, P. M., and Fraser, P. D. (2017). The regulation of carotenoid formation in tomato fruit. Plant J. 89, 774–788. doi: 10.1111/tpj.13428
Farre, G., Maiam Rivera, S., Alves, R., Vilaprinyo, E., Sorribas, A., Canela, R., et al. (2013). Targeted transcriptomic and metabolic profiling reveals temporal bottlenecks in the maize carotenoid pathway that may be addressed by multigene engineering. Plant J. 75, 441–455. doi: 10.1111/tpj.12214
Fraser, P. D., Enfissi, E. M., Halket, J. M., Truesdale, M. R., Yu, D., Gerrish, C., et al. (2007). Manipulation of phytoene levels in tomato fruit: effects on isoprenoids, plastids, and intermediary metabolism. Plant Cell 19, 3194–3211. doi: 10.1105/tpc.106.049817
Fraser, P. D., Kiano, J. W., Truesdale, M. R., Schuch, W., and Bramley, P. M. (1999). Phytoene synthase-2 enzyme activity in tomato does not contribute to carotenoid synthesis in ripening fruit. Plant Mol. Biol. 40, 687–698. doi: 10.1023/a:1006256302570
Fraser, P. D., Schuch, W., and Bramley, P. M. (2000). Phytoene synthase from tomato (Lycopersicon esculentum) chloroplasts–partial purification and biochemical properties. Planta 211, 361–369. doi: 10.1007/s004250000293
Fray, R. G., Wallace, A., Fraser, P. D., Valero, D., Hedden, P., Bramley, P. M., et al. (1995). Constitutive expression of a fruit phytoene synthase gene in transgenic tomatoes causes dwarfism by redirecting metabolites from the gibberellin pathway. Plant J. 8, 693–701. doi: 10.1046/j.1365-313X.1995.08050693.x
Fu, X., Feng, C., Wang, C., Yin, X., Lu, P., Grierson, D., et al. (2014). Involvement of multiple phytoene synthase genes in tissue- and cultivar-specific accumulation of carotenoids in loquat. J. Exp. Bot. 65, 4679–4689. doi: 10.1093/jxb/eru257
Fu, Z., Yan, J., Zheng, Y., Warburton, M. L., Crouch, J. H., and Li, J. S. (2010). Nucleotide diversity and molecular evolution of the PSY1 gene in Zea mays compared to some other grass species. Theor. Appl. Genet. 120, 709–720. doi: 10.1007/s00122-009-1188-x
Fujisawa, M., Nakano, T., Shima, Y., and Ito, Y. (2013). A large-scale identification of direct targets of the tomato MADS box transcription factor RIPENING INHIBITOR reveals the regulation of fruit ripening. Plant Cell 25, 371–386. doi: 10.1105/tpc.112.108118
Fujisawa, M., Shima, Y., Nakagawa, H., Kitagawa, M., Kimbara, J., Nakano, T., et al. (2014). Transcriptional regulation of fruit ripening by tomato FRUITFULL homologs and associated MADS box proteins. Plant Cell 26, 89–101. doi: 10.1105/tpc.113.119453
Giorio, G., Stigliani, A. L., and D’ambrosio, C. (2008). Phytoene synthase genes in tomato (Solanum lycopersicum L.) - new data on the structures, the deduced amino acid sequences and the expression patterns. FEBS J. 275, 527–535. doi: 10.1111/j.1742-4658.2007.06219.x
Giuliano, G. (2017). Provitamin A biofortification of crop plants: a gold rush with many miners. Curr. Opin. Biotechnol. 44, 169–180. doi: 10.1016/j.copbio.2017.02.001
Gupta, P., Rodriguez-Franco, M., Bodanapu, R., Sreelakshmi, Y., and Sharma, R. (2022). Phytoene synthase 2 in tomato fruits remains functional and contributes to abscisic acid formation. Plant Sci. 316:111177. doi: 10.1016/j.plantsci.2022.111177
Han, Y., Zheng, Q. S., Wei, Y. P., Chen, J., Liu, R., and Wan, H. J. (2015). In silico identification and analysis of phytoene synthase genes in plants. Genet. Mol. Res. 14, 9412–9422. doi: 10.4238/2015.August.14.5
Hermanns, A. S., Zhou, X., Xu, Q., Tadmor, Y., and Li, L. (2020). Carotenoid pigment accumulation in horticultural plants. Hortic. Plant J. 6, 343–360. doi: 10.1016/j.hpj.2020.10.002
Howitt, C. A., Cavanagh, C. R., Bowerman, A. F., Cazzonelli, C., Rampling, L., Mimica, J. L., et al. (2009). Alternative splicing, activation of cryptic exons and amino acid substitutions in carotenoid biosynthetic genes are associated with lutein accumulation in wheat endosperm. Funct. Integr. Genomics. 9, 363–376. doi: 10.1007/s10142-009-0121-3
Itkin, M., Seybold, H., Breitel, D., Rogachev, I., Meir, S., and Aharoni, A. (2009). TOMATO AGAMOUS-LIKE 1 is a component of the fruit ripening regulatory network. Plant J. 60, 1081–1095. doi: 10.1111/j.1365-313X.2009.04064.x
Jang, S. J., Jeong, H. B., Jung, A., Kang, M. Y., Kim, S., Ha, S. H., et al. (2020). Phytoene synthase 2 can compensate for the absence of Psy1 in capsicum fruit. J. Exp. Bot. 71, 3417–3427. doi: 10.1093/jxb/eraa155
Kachanovsky, D. E., Filler, S., Isaacson, T., and Hirschberg, J. (2012). Epistasis in tomato color mutations involves regulation of phytoene synthase 1 expression by cis-carotenoids. Proc. Natl. Acad. Sci. U. S. A. 109, 19021–19026. doi: 10.1073/pnas.1214808109
Karniel, U., Adler Berke, N., Mann, V., and Hirschberg, J. (2022). Perturbations in the carotenoid biosynthesis pathway in tomato fruit reactivate the leaf-specific phytoene synthase 2. Front. Plant Sci. 13:844748. doi: 10.3389/fpls.2022.8447
Kim, S. E., Lee, C. J., Park, S. U., Lim, Y. H., Park, W. S., Kim, H. J., et al. (2021). Overexpression of the golden SNP-carrying orange gene enhances carotenoid accumulation and heat stress tolerance in sweetpotato plants. Antioxidants 2021:10. doi: 10.3390/antiox10010051
Li, F., Vallabhaneni, R., and Wurtzel, E. T. (2008a). PSY3, a new member of the phytoene synthase gene family conserved in the Poaceae and regulator of abiotic stress-induced root carotenogenesis. Plant Physiol. 146, 1333–1345. doi: 10.1104/pp.107.111120
Li, F., Vallabhaneni, R., Yu, J., Rocheford, T., and Wurtzel, E. T. (2008b). The maize phytoene synthase gene family: overlapping roles for carotenogenesis in endosperm, photomorphogenesis, and thermal stress tolerance. Plant Physiol. 147, 1334–1346. doi: 10.1104/pp.108.122119
Li, L., Yang, Y., Xu, Q., Owsiany, K., Welsch, R., Chitchumroonchokchai, C., et al. (2012). The Or gene enhances carotenoid accumulation and stability during post-harvest storage of potato tubers. Mol. Plant 5, 339–352. doi: 10.1093/mp/ssr099
Liang, X.-H., Sun, H., Shen, W., Wang, S., Yao, J., Migawa, M. T., et al. (2017). Antisense oligonucleotides targeting translation inhibitory elements in 5’ UTRs can selectively increase protein levels. Nucleic Acids Res. 45, 9528–9546. doi: 10.1093/nar/gkx632
Lindgren, L. O., Stalberg, K. G., and Hoglund, A. S. (2003). Seed-specific overexpression of an endogenous Arabidopsis phytoene synthase gene results in delayed germination and increased levels of carotenoids, chlorophyll, and abscisic acid. Plant Physiol. 132, 779–785. doi: 10.1104/pp.102.017053
Liu, G., Li, C., Yu, H., Tao, P., Yuan, L., Ye, J., et al. (2020). GREEN STRIPE, encoding methylated TOMATO AGAMOUS-LIKE 1, regulates chloroplast development and Chl synthesis in fruit. New Phytol. 228, 302–317. doi: 10.1111/nph.16705
Llorente, B., D’andrea, L., Ruiz-Sola, M. A., Botterweg, E., Pulido, P., Andilla, J., et al. (2016). Tomato fruit carotenoid biosynthesis is adjusted to actual ripening progression by a light-dependent mechanism. Plant J. 85, 107–119. doi: 10.1111/tpj.13094
Lu, P., Yu, S., Zhu, N., Chen, Y. R., Zhou, B., Pan, Y., et al. (2018). Genome encode analyses reveal the basis of convergent evolution of fleshy fruit ripening. Nat. Plants 4, 784–791. doi: 10.1038/s41477-018-0249-z
Lu, S., Van Eck, J., Zhou, X., Lopez, A. B., O’halloran, D. M., Cosman, K. M., et al. (2006). The cauliflower Or gene encodes a DnaJ cysteine-rich domain-containing protein that mediates high levels of beta-carotene accumulation. Plant Cell 18, 3594–3605. doi: 10.1105/tpc.106.046417
Lu, S., Ye, J., Zhu, K., Zhang, Y., Zhang, M., Xu, Q., et al. (2021). A fruit ripening-associated transcription factor CsMADS5 positively regulates carotenoid biosynthesis in citrus. J. Exp. Bot. 72, 3028–3043. doi: 10.1093/jxb/erab045
Lu, S., Zhang, Y., Zhu, K., Yang, W., Ye, J., Chai, L., et al. (2018). The citrus transcription factor CsMADS6 modulates carotenoid metabolism by directly regulating carotenogenic genes. Plant Physiol. 176, 2657–2676. doi: 10.1104/pp.17.01830
Maass, D., Arango, J., Wust, F., Beyer, P., and Welsch, R. (2009). Carotenoid crystal formation in Arabidopsis and carrot roots caused by increased phytoene synthase protein levels. PLoS One 4:e6373. doi: 10.1371/journal.pone.0006373
Mishiba, K. I., Nishida, K., Inoue, N., Fujiwara, T., Teranishi, S., Iwata, Y., et al. (2020). Genetic engineering of eggplant accumulating beta-carotene in fruit. Plant Cell Rep. 39, 1029–1039. doi: 10.1007/s00299-020-02546-8
Mukherjee, T., and Mukhopadhyay, S. K. (2020). Comparative analysis of structural and functional aspects of phytoene synthase from Meiothermus taiwanensis strain RP. Ann. Microbiol. 70:9. doi: 10.1186/s13213-020-01558-9
Naqvi, S., Zhu, C. F., Farre, G., Ramessar, K., Bassie, L., Breitenbach, J., et al. (2009). Transgenic multivitamin corn through biofortification of endosperm with three vitamins representing three distinct metabolic pathways. Proc. Natl. Acad. Sci. U. S. A. 106, 7762–7767. doi: 10.1073/pnas.0901412106
Nisar, N., Li, L., Lu, S., Khin, N. C., and Pogson, B. J. (2015). Carotenoid metabolism in plants. Mol. Plant 8, 68–82. doi: 10.1016/j.molp.2014.12.007
Paine, J. A., Shipton, C. A., Chaggar, S., Howells, R. M., Kennedy, M. J., Vernon, G., et al. (2005). Improving the nutritional value of Golden Rice through increased pro-vitamin A content. Nat. Biotechnol. 23, 482–487. doi: 10.1038/nbt1082
Park, S., Kim, H. S., Jung, Y. J., Kim, S. H., Ji, C. Y., Wang, Z., et al. (2016). Orange protein has a role in phytoene synthase stabilization in sweetpotato. Sci. Rep. 6:33563. doi: 10.1038/srep33563
Paul, J. Y., Khanna, H., Kleidon, J., Hoang, P., Geijskes, J., Daniells, J., et al. (2017). Golden bananas in the field: elevated fruit pro-vitamin A from the expression of a single banana transgene. Plant Biotechnol. J. 15, 520–532. doi: 10.1111/pbi.12650
Pollack, A. J., Gong, X., and Pollack, J. R. (2019). A common phytoene synthase mutation underlies white petal varieties of the California poppy. Sci. Rep. 9:11615. doi: 10.1038/s41598-019-48122-3
Ray, J., Bird, C., Maunders, M., Grierson, D., and Schuch, W. (1987). Sequence of pTOM5, a ripening related cDNA from tomato. Nucleic Acids Res. 15:10587. doi: 10.1093/nar/15.24.10587
Rios-Estepa, R., and Lange, B. M. (2007). Experimental and mathematical approaches to modeling plant metabolic networks. Phytochemistry 68, 2351–2374. doi: 10.1016/j.phytochem.2007.04.021
Rodriguez-Concepcion, M., Avalos, J., Bonet, M. L., Boronat, A., Gomez-Gomez, L., Hornero-Mendez, D., et al. (2018). A global perspective on carotenoids: Metabolism, biotechnology, and benefits for nutrition and health. Prog. Lipid Res. 70, 62–93. doi: 10.1016/j.plipres.2018.04.004
Rodriguez-Villalon, A., Gas, E., and Rodriguez-Concepcion, M. (2009). Phytoene synthase activity controls the biosynthesis of carotenoids and the supply of their metabolic precursors in dark-grown Arabidopsis seedlings. Plant J. 60, 424–435. doi: 10.1111/j.1365-313X.2009.03966.x
Ruiz-Sola, M. A., Coman, D., Beck, G., Barja, M. V., Colinas, M., Graf, A., et al. (2016). Arabidopsis GERANYLGERANYL DIPHOSPHATE SYNTHASE 11 is a hub isozyme required for the production of most photosynthesis-related isoprenoids. New Phytol. 209, 252–264. doi: 10.1111/nph.13580
Ruiz-Sola, M. A., and Rodríguez-Concepción, M. (2012). Carotenoid biosynthesis in Arabidopsis: a colorful pathway. Arabid. Book 10:e0158. doi: 10.1199/tab.0158
Schaub, P., Rodriguez-Franco, M., Cazzonelli, C. I., Alvarez, D., Wust, F., and Welsch, R. (2018). Establishment of an Arabidopsis callus system to study the interrelations of biosynthesis, degradation and accumulation of carotenoids. PLoS One 13:e0192158. doi: 10.1371/journal.pone.0192158
Schaub, P., Wust, F., Koschmieder, J., Yu, Q., Virk, P., Tohme, J., et al. (2017). Nonenzymatic β-carotene degradation in provitamin A-biofortified crop plants. J. Agric. Food Chem. 65, 6588–6598. doi: 10.1021/acs.jafc.7b01693
Schledz, M., Al-Babili, S., Lintig, J. V., Haubruck, H., Rabbani, S., Kleinig, H., et al. (1996). Phytoene synthase from Narcissus pseudonarcissus: functional expression, galactolipid requirement, topological distribution in chromoplasts and induction during flowering. Plant J. 10, 781–792. doi: 10.1046/j.1365-313X.1996.10050781.x
Schmidt, M. A., Parrott, W. A., Hildebrand, D. F., Berg, R. H., Cooksey, A., Pendarvis, K., et al. (2015). Transgenic soya bean seeds accumulating beta-carotene exhibit the collateral enhancements of oleate and protein content traits. Plant Biotechnol. J. 13, 590–600. doi: 10.1111/pbi.12286
Shewmaker, C. K., Sheehy, J. A., Daley, M., Colburn, S., and Ke, D. Y. (1999). Seed-specific overexpression of phytoene synthase: increase in carotenoids and other metabolic effects. Plant J. 20, 401X–412X. doi: 10.1046/j.1365-313x.1999.00611.x
Shumskaya, M., Bradbury, L. M., Monaco, R. R., and Wurtzel, E. T. (2012). Plastid localization of the key carotenoid enzyme phytoene synthase is altered by isozyme, allelic variation, and activity. Plant Cell 24, 3725–3741. doi: 10.1105/tpc.112.104174
Shumskaya, M., and Wurtzel, E. T. (2013). The carotenoid biosynthetic pathway: thinking in all dimensions. Plant Sci. 208, 58–63. doi: 10.1016/j.plantsci.2013.03.012
Slater, A., Maunders, M. J., Edwards, K., Schuch, W., and Grierson, D. (1985). Isolation and characterisation of cDNA clones for tomato polygalacturonase and other ripening-related proteins. Plant Mol. Biol. 5, 137–147. doi: 10.1007/BF00015677
Stauder, R., Welsch, R., Camagna, M., Kohlen, W., Balcke, G. U., Tissier, A., et al. (2018). Strigolactone levels in dicot roots are determined by an ancestral symbiosis-regulated clade of the PHYTOENE SYNTHASE gene family. Front. Plant. Sci. 9:255. doi: 10.3389/fpls.2018.00255
Struk, S., Jacobs, A., Sanchez Martin-Fontecha, E., Gevaert, K., Cubas, P., and Goormachtig, S. (2019). Exploring the protein-protein interaction landscape in plants. Plant Cell Environ. 42, 387–409. doi: 10.1111/pce.13433
Summers, C., Karst, F., and Charles, A. D. (1993). Cloning, expression and characterisation of the cDNA encoding human hepatic squalene synthase, and its relationship to phytoene synthase. Gene 136, 185–192. (93)90462-c doi: 10.1016/0378-1119
Sun, T., and Li, L. (2020). Toward the ‘golden’era: the status in uncovering the regulatory control of carotenoid accumulation in plants. Plant Sci. 290:110331. doi: 10.1016/j.plantsci.2019.110331
Sun, T., Rao, S., Zhou, X., and Li, L. (2022). Plant carotenoids: recent advances and future perspectives. Mol. Hortic. 2022:2. doi: 10.1186/s43897-022-00023-2
Sun, T., Tadmor, Y., and Li, L. (2020). Pathways for carotenoid biosynthesis, degradation, and storage. Methods Mol. Biol. 2083, 3–23. doi: 10.1007/978-1-4939-9952-1_1
Sun, T., Yuan, H., Cao, H., Yazdani, M., Tadmor, Y., and Li, L. (2018). Carotenoid metabolism in plants: The role of plastids. Mol. Plant 11, 58–74. doi: 10.1016/j.molp.2017.09.010
Sun, T., Zhu, Q., Wei, Z., Owens, L. A., Fish, T., Kim, H., et al. (2021). Multi-strategy engineering greatly enhances provitamin A carotenoid accumulation and stability in Arabidopsis seeds. aBIOTECH 2, 191–214. doi: 10.1007/s42994-021-00046-1
Toledo-Ortiz, G., Huq, E., and Rodríguez-Concepción, M. (2010). Direct regulation of phytoene synthase gene expression and carotenoid biosynthesis by phytochrome-interacting factors. Proc. Natl. Acad. Sci. U. S. A. 107, 11626–11631. doi: 10.1073/pnas.0914428107
Toledo-Ortiz, G., Johansson, H., Lee, K. P., Bou-Torrent, J., Stewart, K., Steel, G., et al. (2014). The HY5-PIF regulatory module coordinates light and temperature control of photosynthetic gene transcription. PLos Genet. 10:e1004416. doi: 10.1371/journal.pgen.1004416
Van De Peer, Y., Mizrachi, E., and Marchal, K. (2017). The evolutionary significance of polyploidy. Nat. Rev. Genet. 18, 411–424. doi: 10.1038/nrg.2017.26
Von Lintig, J., Welsch, R., Bonk, M., Giuliano, G., Batschauer, A., and Kleinig, H. (1997). Light-dependent regulation of carotenoid biosynthesis occurs at the level of phytoene synthase expression and is mediated by phytochrome in Sinapis alba and Arabidopsis thaliana seedlings. Plant J. 12, 625–634. doi: 10.1046/j.1365-313x.1997.00625.x
Vrebalov, J., Pan, I. L., Arroyo, A. J. M., Mcquinn, R., Chung, M., Poole, M., et al. (2009). Fleshy fruit expansion and ripening are regulated by the tomato SHATTERPROOF gene TAGL1. Plant Cell 21, 3041–3062. doi: 10.1105/tpc.109.066936
Wang, C., Zeng, J., Li, Y., Hu, W., Chen, L., Miao, Y., et al. (2014). Enrichment of provitamin A content in wheat (Triticum aestivum L.) by introduction of the bacterial carotenoid biosynthetic genes CrtB and CrtI. J. Exp. Bot. 65, 2545–2556. doi: 10.1093/jxb/eru138
Wang, P., Wang, Y., Wang, W., Chen, T., Tian, S., and Qin, G. (2020). Ubiquitination of phytoene synthase 1 precursor modulates carotenoid biosynthesis in tomato. Commun. Biol. 3, 1–14. doi: 10.1038/s42003-020-01474-3
Wang, Q., Huang, X. Q., Cao, T. J., Zhuang, Z., Wang, R., and Lu, S. (2018). Heteromeric geranylgeranyl diphosphate synthase contributes to carotenoid biosynthesis in ripening fruits of red pepper (Capsicum annuum var. conoides). J. Agric. Food Chem. 66, 11691–11700. doi: 10.1021/acs.jafc.8b04052
Welsch, R., Arango, J., Bar, C., Salazar, B., Al-Babili, S., Beltran, J., et al. (2010). Provitamin A accumulation in cassava (Manihot esculenta) roots driven by a single nucleotide polymorphism in a phytoene synthase gene. Plant Cell 22, 3348–3356. doi: 10.1105/tpc.110.077560
Welsch, R., Beyer, P., Hugueney, P., Kleinig, H., and Von Lintig, J. (2000). Regulation and activation of phytoene synthase, a key enzyme in carotenoid biosynthesis, during photomorphogenesis. Planta 211, 846–854. doi: 10.1007/s004250000352
Welsch, R., Maass, D., Voegel, T., Dellapenna, D., and Beyer, P. (2007). Transcription factor RAP2.2 and its interacting partner SINAT2: stable elements in the carotenogenesis of Arabidopsis leaves. Plant Physiol. 145, 1073–1085. doi: 10.1104/pp.107.104828
Welsch, R., Wust, F., Bar, C., Al-Babili, S., and Beyer, P. (2008). A third phytoene synthase is devoted to abiotic stress-induced abscisic acid formation in rice and defines functional diversification of phytoene synthase genes. Plant Physiol. 147, 367–380. doi: 10.1104/pp.108.117028
Welsch, R., Zhou, X., Koschmieder, J., Schlossarek, T., Yuan, H., Sun, T., et al. (2020). Characterization of cauliflower OR mutant variants. Front. Plant Sci. 10:1716. doi: 10.3389/fpls.2019.01716
Welsch, R., Zhou, X., Yuan, H., Álvarez, D., Sun, T., Schlossarek, D., et al. (2018). Clp protease and OR directly control the proteostasis of phytoene synthase, the crucial enzyme for carotenoid biosynthesis in Arabidopsis. Mol. Plant 11, 149–162. doi: 10.1016/j.molp.2017.11.003
Wu, M., Xu, X., Hu, X., Liu, Y., Cao, H., Chan, H., et al. (2020). SlMYB72 regulates the metabolism of chlorophylls, carotenoids, and flavonoids in tomato fruit. Plant Physiol. 183, 854–868. doi: 10.1104/pp.20.00156
Xiong, C., Luo, D., Lin, A., Zhang, C., Shan, L., He, P., et al. (2019). A tomato B-box protein SlBBX20 modulates carotenoid biosynthesis by directly activating PHYTOENE SYNTHASE 1, and is targeted for 26S proteasome-mediated degradation. New Phytol. 221, 279–294. doi: 10.1111/nph.15373
Yao, D., Wang, Y., Li, Q., Ouyang, X., Li, Y., Wang, C., et al. (2018). Specific upregulation of a cotton phytoene synthase gene produces golden cottonseeds with enhanced provitamin A. Sci. Rep. 8:1348. doi: 10.1038/s41598-018-19866-1
Ye, X. D., Al-Babili, S., Kloti, A., Zhang, J., Lucca, P., Beyer, P., et al. (2000). Engineering the provitamin A (beta-carotene) biosynthetic pathway into (carotenoid-free) rice endosperm. Science 287, 303–305. doi: 10.1126/science.287.5451.303
Yuan, H., Owsiany, K., Sheeja, T. E., Zhou, X., Rodriguez, C., Li, Y., et al. (2015). A single amino acid substitution in an ORANGE protein promotes carotenoid overaccumulation in Arabidopsis. Plant Physiol. 169, 421–431. doi: 10.1104/pp.15.00971
Zheng, X., Giuliano, G., and Al-Babili, S. (2020). Carotenoid biofortification in crop plants: citius, altius, fortius. Biochim. Biophys. Acta Mol. Cell Biol. Lipids 1865:158664. doi: 10.1016/j.bbalip.2020.158664
Zhou, X., Welsch, R., Yang, Y., Alvarez, D., Riediger, M., Yuan, H., et al. (2015). Arabidopsis OR proteins are the major posttranscriptional regulators of phytoene synthase in controlling carotenoid biosynthesis. Proc. Natl. Acad. Sci. U. S. A. 112, 3558–3563. doi: 10.1073/pnas.1420831112
Zhu, K., Zheng, X., Ye, J., Huang, Y., Chen, H., Mei, X., et al. (2021). Regulation of carotenoid and chlorophyll pools in hesperidia, anatomically unique fruits found only in Citrus. Plant Physiol. 187, 829–845. doi: 10.1093/plphys/kiab291
Keywords: carotenoid, PSY, functional evolution, regulation, metabolic engineering
Citation: Zhou X, Rao S, Wrightstone E, Sun T, Lui ACW, Welsch R and Li L (2022) Phytoene Synthase: The Key Rate-Limiting Enzyme of Carotenoid Biosynthesis in Plants. Front. Plant Sci. 13:884720. doi: 10.3389/fpls.2022.884720
Received: 26 February 2022; Accepted: 16 March 2022;
Published: 12 April 2022.
Edited by:
Peng Zhang, Institute of Plant Physiology and Ecology, Shanghai Institutes for Biological Sciences (CAS), ChinaReviewed by:
Shan Lu, Nanjing University, ChinaCopyright © 2022 Zhou, Rao, Wrightstone, Sun, Lui, Welsch and Li. This is an open-access article distributed under the terms of the Creative Commons Attribution License (CC BY). The use, distribution or reproduction in other forums is permitted, provided the original author(s) and the copyright owner(s) are credited and that the original publication in this journal is cited, in accordance with accepted academic practice. No use, distribution or reproduction is permitted which does not comply with these terms.
*Correspondence: Li Li, bGwzN0Bjb3JuZWxsLmVkdQ==; orcid.org/0000-0002-4352-4061
†These authors have contributed equally to this work