- 1Key Laboratory of Eco-environments in Three Gorges Reservoir Region (Ministry of Education), Chongqing Key Laboratory of Plant Ecology and Resources in Three Gorges Reservoir Region, School of Life Sciences, Southwest University, Chongqing, China
- 2School of Art and Design, Huanghuai University, Zhumadian, China
Global climate change has resulted in an increase in intensity and frequency of flooding, plants living in lowlands, and shore areas have to confront submergence caused by flooding, submergence-tolerant plants usually respond by adopting either escape or quiescence strategies. While certain plants exhibit a changeover from escape strategy upon partial submergence to quiescence strategy under complete shallow submergence, it remains unknown whether plants completely submerged at different water depths would adjust their strategies to cope with the change in submergence depth. Alternanthera philoxeroides is an ideal species to explore this adjustment as it is widely distributed in flood-disturbed habitats and exhibits an escape strategy when completely submerged in shallow waters. We investigated the responses of A. philoxeroides in terms of morphology, anatomy, and non-structural carbohydrate metabolism by conducting experiments using a series of submergence depths (0, 2, 5, and 9 m). During the submergence treatment, environmental factors such as light, dissolved oxygen, and temperature for submerged plants were kept constant. The results showed that A. philoxeroides plants submerged at depth of 2 m presented an escape strategy via fast stem elongation, extensive pith cavity development, and small biomass loss. However, the retarded stem elongation, reduced pith cavity transverse area, and increased biomass loss along the water depth gradient indicated that A. philoxeroides altered its growth response as water depth increased from 2 to 9 m. It is found that the changeover of response strategies occurred at higher submergence depths (5–9 m). Based on the results of our experiments, we demonstrated that water depth played an important role in driving the change in strategy. The water-depth-dependent growth performance of A. philoxeroides would benefit the species in habit exploration and exploitation. Further studies should focus on the performances of plants when submerged at varied water depths with different light climates and dissolved oxygen content, and how water depths drive the response behaviors of the submerged plants.
Introduction
Global climate change has increased the intensity and frequency of flood events, and this trend is predicted to continue in the future (Milly et al., 2002; Blöschl et al., 2019). River engineering has considerably altered the hydrological regimes of rivers worldwide (Dynesius and Nilsson, 1994; Huang et al., 2016). These changes mainly include the transition from shallow floods to deep floods (Belt, 1975; Brock et al., 1987; Sparks et al., 1998; Bertola et al., 2020). The construction of more than 50,000 dams higher than 15 m worldwide (Lehner et al., 2011) has resulted in huge drawdown zones. For example, the Three Gorges Reservoir in China has a drawdown zone of ~350 km2 with a maximum submergence depth of 30 m, the water level of which fluctuates annually between 145 and 175 m above sea level (Lei et al., 2017). Such deep submergence has detrimental effects on the growth and survival of plants (Lei et al., 2014; Bejarano et al., 2018; Huang et al., 2021), especially on those completely submerged (Vervuren et al., 2003; Fukao et al., 2019; Striker et al., 2019). As a consequence, plants have to strive to cope with submergence in flood-prone habitats.
One of the major problems that terrestrial plants face when submerged is the energy crisis, induced by low gas partial pressure and light intensity in water (Bailey-Serres et al., 2012; Huber et al., 2012; Pedersen et al., 2017). Gas (e.g., oxygen, carbon dioxide, and ethylene) exchange between completely submerged plants and water column significantly slows as gas diffusion is severely restricted underwater (Jackson, 1985; Voesenek et al., 2006; Voesenek and Bailey-Serres, 2015). Due to the low oxygen content in the water column, the aerobic respiration of completely submerged plants decreases, leading to reduced ATP production and even plant death (Voesenek et al., 2006; Bailey-Serres and Voesenek, 2008). Compared to the atmosphere, water bodies have relatively low levels of CO2, moreover, the photosynthetically active radiation (PAR) in water bodies sharply declines from the water surface downwards (Voesenek et al., 2006; Voesenek and Bailey-Serres, 2015). This further limits underwater photosynthesis and increases the energy crisis of plants when submerged.
The escape and quiescence strategies are two major strategies adopted by plants in flood disturbed habitats (van Veen et al., 2014). Generally, the escape strategy enables plants to cope with shallow but prolonged submergence, whereas the quiescence strategy is favored to withstand deeper and short-term submergence (Bailey-Serres and Voesenek, 2008; Manzur et al., 2009; Striker et al., 2017). The “escape strategy” syndrome includes fast elongation of shoot organs (Sauter et al., 1993; Müller et al., 2019) or leaf petioles (Groeneveld and Voesenek, 2003; Pierik et al., 2009) to restore air contact rapidly, enhanced adventitious root formation to improve oxygen uptake in water (Ayi et al., 2016; Zhang et al., 2017), and increased formation of internal aerenchyma tissue to efficiently transport gas (Manzur et al., 2009; Striker et al., 2019). These morph-physiological behaviors require carbohydrates for cell division and new cell production (Sauter, 2000; Voesenek et al., 2006; Luo et al., 2011). Therefore, one side effect of the “escape strategy” would be fast carbohydrate depletion (Bailey-Serres and Voesenek, 2008; Manzur et al., 2009; Akman et al., 2012), which is lethal for submerged terrestrial plants if they cannot protrude from the water surface. Under deep submergence conditions (where water depths are usually deeper than 2 m and light climate is poor), plants with a quiescence strategy will be more successful. They suppress energy consumption by only running basic metabolism with little or no organ elongation and new tissue formation, thus conserving carbohydrate reserves to prolong survival time in deep water while waiting for the water level to recede (Manzur et al., 2009; Pierik et al., 2009; Luo et al., 2011; Akman et al., 2012; Striker et al., 2017). Theoretically, it is likely that plants would survive and distribute from low to high elevations in the river riparian zones (or the reservoir drawdown zones and other flood disturbed habitats) if they are able to alter their response strategy at different submergence depths. It was reported that the wetland plant Lotus tenuis chose to escape from partial submergence by shoot elongation but adopted a non-elongating quiescent strategy when completely immersed in shallow water (Manzur et al., 2009). Nevertheless, the literature on whether terrestrial plants can alter their response strategies along a gradient of submergence depths is still scarce.
Besides gas and light, which induce plant responses to submergence, submergence depth—especially extremely deep submergence—may strongly affect plant performance (Vervuren et al., 2003). Submergence depth is a primary physical factor that varies along elevational gradients in many riparian regions (Howard and Mendelssohn, 1995). The effects of submergence depth on plant metabolism and growth can be direct or indirect (increasing hydrostatic pressure, increasing soil oxygen consumption, and changing temperature, which indirectly affects plant performance in water) (Grace, 1989; Howard and Mendelssohn, 1995; Casanova and Brock, 2000; Bejarano et al., 2018; Meng et al., 2022). Some studies have demonstrated the negative effect of increased submergence depth on plant survival; the median lethal time (LT50) of Rhine riparian plants at a depth of 1.6 m was approximately half of that at 0.4 m (Vervuren et al., 2003), similar to the effects observed in rice cultivars (Adkins et al., 1990). So far, the published literatures primarily reported the studies focused on the influences of submergence depths <2 m on terrestrial plants. The absence of studies investigating how deeper water affects plant performance might limit our understanding of plant distribution patterns in flood-prone habitats.
Alternanthera philoxeroides (Mart.) Griseb., a terrestrial perennial herbaceous plant belonging to the Amaranthaceae family, originates from South America and has spread to many parts of the world. It is considered an invasive species in the United States, Australia, New Zealand, Thailand, and China, and able to survive nicely in flood-disturbed habitats (Zhang et al., 2015a; Dong et al., 2018). A. philoxeroides exhibits an escape strategy in shallow submergence (Luo et al., 2009, 2011; Ayi et al., 2016), with quickly elongated shoots, increased adventitious roots formation, and widened aerenchyma channels conducive to enhance gas transport (Ayi et al., 2019). In addition, the species lowers its metabolic rate to substantially reduce carbohydrate consumption in water (Ye et al., 2016). A. philoxeroides not only distributes in shallow wetlands but also well exists in areas experiencing submergence with a maximum depth of 20 m for up to 4 months (Zheng et al., 2021). Therefore, this species is an ideal species for investigating how plants respond to submergence depth gradient. Considering the side effects of escape strategy and the wide distribution of A. philoxeroides in areas with varied submergence depths, we hypothesize that A. philoxeroides is likely to change its growth strategy when submergence depth differs.
In this study, we aimed to explore how A. philoxeroides plants respond to a gradient of submergence depths in terms of morphology, anatomy, biomass, and carbohydrate metabolism by conducting submergence experiments with varied water depths. Physical conditions of the water body including light, dissolved oxygen, pH, and temperature were kept constant in the experiments. The study may help understand the mechanisms of plant tolerance to extreme flooding and explain why A. philoxeroides remains highly invasive in regions where submergence depths are varied (e.g., the drawdown zones of large reservoirs). The findings may also provide insight into the effective management of this species.
Materials and Methods
Plant Material and Cultivation
Alternanthera philoxeroides is a herbaceous perennial plant, under normal conditions, it can spread quickly via clonal growth (Luo et al., 2009; Ayi et al., 2016), growing to a height of 50–120 cm, with a long single or sparsely branched stem. The stem has several internodes of different lengths, and its maturity degree gradually decreases from the base to the top, making it an ideal organ type to study the adaptation and response of A. philoxeroides to different submergence depths.
To reduce the influence of environmental conditions on mother plants, A. philoxeroides plants used in this experiment were cultivated from cuttings obtained from plants naturally growing on the banks of the Jialing River in Chongqing, Southwest China (29°49′N, 106°25′E). In May 2020, unbranched plants with a stem length of ~30 cm were selected and cut at the stem base. The cuttings were transported to the laboratory immediately, and healthy and vigorous cuttings were selected for subsequent treatments, all trimmed to have five internodes and six leaves. Each selected cutting was planted in a plastic pot (diameter and depth were both 13 cm) containing riparian soil from the Jialing River banks, two stem nodes of the cutting (hereafter referred to as “plant”) were buried in soil for rooting. All plants were cultivated under the same conditions and placed in an open field of the experimental garden affiliated to the Key Laboratory of Eco-environments in Three Gorges Reservoir Region (Ministry of Education) at Southwest University, Chongqing. The temperature, relative humidity, daily maximum light (PAR) intensity, and water provision were maintained at 10–15°C, 75–85%, 600–800 μmol m−2 s−1, and ~80–90% of soil water-holding capacity, respectively. Plants were watered daily. Lateral buds, if produced, were removed to ensure that the main stem of plants grew without branching. Plants were kept growing upright by the support of thin bamboo sticks. After ~1 month of cultivation, plants with ~45 cm in height and 12 internodes were selected for submergence treatments.
Submergence Treatments
Four submergence treatments were applied in a fully randomized design using selected plants (20 replicates per treatment). Submergence depth of 0 m was set as control: unsubmerged plants were placed under dark conditions and watered normally to keep soil water at field capacity. Additionally, three groups of plants were submerged in a water-filled concrete reservoir (length × width × depth = 2.3 × 1.9 × 10 m), with the top of plants 2, 5, and 9 m beneath the water surface. The plants in pots were suspended at planned water depths (Figure 1A). Pilot experiments showed that the stem tips of plants started to die (characterized by becoming flaccid) on the 7th day following submergence at depth of 9 m. Therefore, the treatment duration was set at six days for all submergence depths to ensure tested plants kept vigorous during this period.
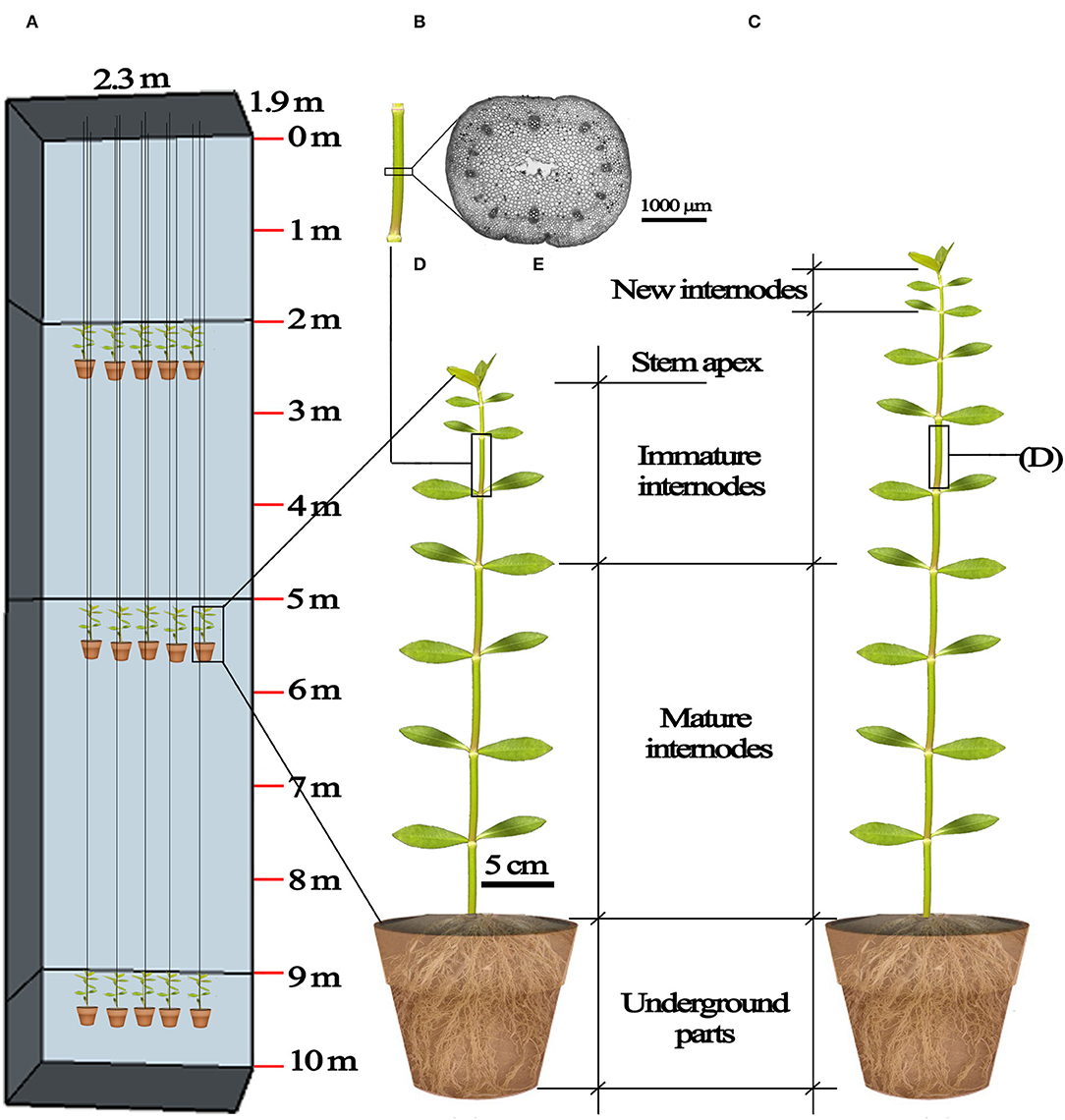
Figure 1. Diagrammatic representation of the submergence design and plant material. (A) The frame of the 10 m deep concrete reservoir (length × width × depth= 2.3 m ×1.9 m ×10 m) and the 2, 5, and 9 m submergence treatments; (B) underground parts, mature internodes, immature internodes and stem apex of A. philoxeroides before submergence treatments; (C) underground parts, mature internodes, immature internodes, new internodes, of A. philoxeroides after submergence; (D) the middle part of an internode, marked before treatments and used for making transverse sections at the end of submergence; (E) a transverse section of the internode.
Water Environment Management
To investigate the effects of submergence depth on plants, the physico-chemical status of water (light, dissolved oxygen (DO), pH, and temperature) in the concrete reservoir were kept constant at any depths. The water was air-saturated by pumping air every day through an air pipe with vent holes installed at the bottom of the reservoir, ensuring the adequate and uniform supply of oxygen and carbon dioxide at various water depths. The temperature of the whole water body was kept constant by applying an electric heating system equipped at the bottom of the reservoir. The reservoir was covered with black sun-shading nets to eliminate the influence of light. The control treatment was conducted in darkness under the same temperature as the submergence treatments. DO concentration, photosynthetically active radiation (PAR) intensity, temperature, and pH of the water column at different depths in the reservoir were checked twice per day (morning and evening) using a multi-parameter water quality analyzer (Hydrolab DS5, Hach, United States). During the experiment, no significant difference in these factors was found among different water depths (Table 1).
Growth Measurements
Each plant had ~12 stem internodes at the start of treatments. From the stem base upwards, the 1st to 6th internodes were mature and the 7th to 12th internodes were immature (Figure 1B). We marked non-destructively immature internodes so as to distinguish between the mature, immature internodes formed before treatment and the new internodes produced during treatment. At the beginning of treatments, for each plant, 26 leaves were retained on the upper stem by trimming other leaves from the stem base upwards. The length of all internodes of each plant was measured twice (at the beginning and the end of treatments). We compared the elongation of mature, immature, and newly produced internodes at the end of the treatments to assess the effects of submergence depths on the growth of internodes of varying maturity degrees. The elongation of mature internodes, immature internodes, and newly produced internodes was, respectively, calculated as the sum of elongation from the 1st to 6th internodes, the sum of elongation from the 7th to 12th internodes, and the length sum of all newly produced internodes (Figure 1C). The number of newly produced internodes was also counted. Afterward, the leaves, stem (viz. the aboveground stem part), and underground part (including the underground stem part which can be regarded as rhizome, and roots) of each plant were oven-dried to constant weights at 75°C, and their dry mass was determined (BSA124S, Sartorius, Germany).
No adventitious roots were formed on the aboveground stem nodes of A. philoxeroides before the submergence treatments. Any formation of aquatic adventitious roots on the aboveground stem nodes was recorded at the end of the treatments.
Transverse Section and Pith Cavity of Internodes
Because the pilot experiment showed that the 10th stem internode had relatively large elongation during treatments (Supplementary Figure S1), and its pith cavity had not been formed at the start of the treatments, we marked the 10th internode before treatments to investigate the effects of submergence depths on the development of internodal pith cavity. Transverse sections were made at the middle of the internodes immediately when treatments terminated (Figures 1D,E). Sections were observed and photographed using a stereomicroscope (SMZ25, Nikon, Japan). The pith cavity cross-area of each section was measured using the NIS-elements imaging software (version 4.30).
Non-Structural Carbohydrate Analysis
Soluble sugars and starch of the dried stems and underground parts of plants were measured by using fine powder samples prepared with a ball mill (WS-MM200, Retsch, Haan, Germany). Soluble sugars and starch were extracted and determined using a modified method based on the traditional anthrone-sulfuric acid method (Zhang, 2003; Lei et al., 2014). Ethanol-soluble sugars of 0.01 g powder sample soaked in 80% (v/v) ethanol solution were extracted in the water bath at 80°C for 40 min. The extraction of each sample was repeated twice and the extracts were mixed and diluted with ultrapure water to 50 ml. Subsequently, the residue of each sample was soaked in 5 ml ultrapure water and extracted for soluble but ethanol-insoluble sugars in the water bath at 80°C for 40 min, the extraction of each sample was also repeated twice and the extracts were mixed and diluted with ultrapure water to 50 ml. The starch of the residue was hydrolyzed to soluble sugars using 6 mol L−1 HCl, the hydrolyte solution was filtered into a flask and diluted with ultrapure water to 100 ml. A total of 1 ml of the above-mentioned 50, 50, and 100 ml diluted extracts was respectively added with 5 ml anthrone-sulfuric acid reagent (0.1 g anthrone dissolved in 100 ml 75% (v/v) sulfuric acid solution) and heated for 10 min at 100°C, and the light absorbance at 625 nm was measured (UV-2700, Shimadzu, Japan) to determine the concentration of ethanol-soluble sugars, soluble but ethanol-insoluble sugars, and starch based on a glucose calibration curve. The concentration of total soluble sugars in a plant sample was the concentration sum of ethanol-soluble sugars plus soluble but ethanol-insoluble sugars. Since the starch was hydrolyzed to soluble sugars, starch concentration was therefore calculated by multiplying the concentration of hydrolytic soluble sugars with a hydrolysis coefficient (0.9). The sum of ethanol-soluble sugars, soluble but ethanol-insoluble sugars, and starch was regarded as non-structural carbohydrates in plant samples.
Data Analyses
The effects of treatments (control and submergence at different depths) on stem and internode elongation, plant and organ biomass, number and length of newly produced internodes, internodal pith cavity cross area, number of nodes forming adventitious roots, and contents of non-structural carbohydrates (soluble sugars and starch) were checked using one-way ANOVA. Logarithm transformation of data was performed to equalize variance if necessary. Differences between treatments were detected using Duncan's multiple range test, and the significance level was set at p = 0.05. All the analyses were conducted using SPSS 22 (SPSS Inc., Chicago).
Results
Stem Elongation and new Internode Production
A. philoxeroides plants subjected to four treatments [water depths of 0 m (control), 2, 5, and 9 m] all elongated their stems during the experiment; however, stem elongation significantly decreased with increasing submergence depth after 6 days of treatment (Figure 2A). The stem elongation of plants subjected to submergence at a water depth of 0 (control), 2, 5, and 9 was 19.79, 28.56, 23.21, and 11.99 cm, respectively. Plants submerged at water depths of 2 and 5 m presented significantly larger stem elongation than control plants, but plants submerged at water depth of 9 m presented much less stem elongation than control plants (Figure 2A). The contribution of mature and immature internodes produced before treatments and new internodes produced during treatment to the stem elongation differed significantly, immature internodes comparatively made the largest contribution to plant stem elongation (Figure 2B).
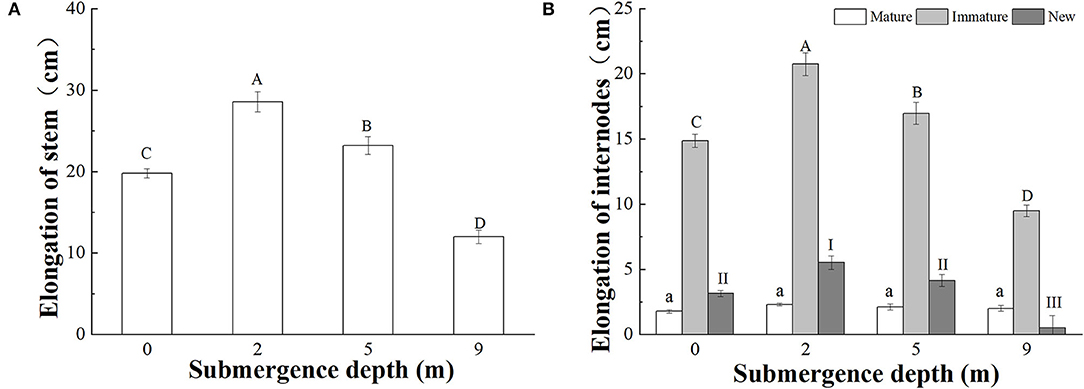
Figure 2. Stem elongation of (A) the whole stem; (B) mature and immature internodes before the treatments, and new internodes produced during the treatments, of A. philoxeroides at the end of treatments at different submergence depths (mean ± S.E; n = 20). Different lower-case letters indicate a significant difference (p < 0.05) in the elongation of mature internodes between treatments. Different upper-case letters indicate significant differences (p < 0.05) in elongation of the whole stem (A) and immature internodes (B) between treatments. Different roman letters indicate significant differences (p < 0.05) in the elongation of new internodes between treatments.
The elongation responses to submergence depths differed between mature, immature, and newly produced internodes. The total elongation of mature internodes was not affected by submergence depths, but the total elongation of immature internodes and newly produced internodes differed significantly between submergence depths (Figure 2B). The total elongation of immature internodes were 14.86, 20.75, 16.95, and 9.48 cm in plants submerged at water depths of 0 (control), 2, 5, and 9 m, respectively (Figure 2B).
Very few new internodes were produced during submergence at water depth of 9 m, and 1.85, 2.40, and 1.85 new internodes on average were produced at water depths of 0, 2, and 5 m, respectively (Table 2). The total length of new internodes was significantly different between the treatments, with the largest length realized in plants submerged at depth of 2 m (Figure 2B).
Based on the results of this study, it was found that the stem elongation and internode production of submerged A. philoxeroides decreased with increasing submergence depths, with the largest stem elongation and new internode production showing under the shallowest submergence (2 m depth) and the smallest stem elongation and nearly no new internode production under the deepest submergence (9 m depth). However, in comparison with plants unsubmerged, the stem elongation and internode production of plants were enhanced by submergence at depths of 2 and 5 m.
Internodal Pith Cavity and Adventitious Root Formation
The internodal pith cavity developed during the experiment varied among four treatments (Figure 3). Plants submerged at depths of 2 and 9 m had the broadest and the narrowest internodal pith cavity, respectively. Plants submerged at depth of 5 m did not differ from unsubmerged plants in size of internodal pith cavity (Figure 3).
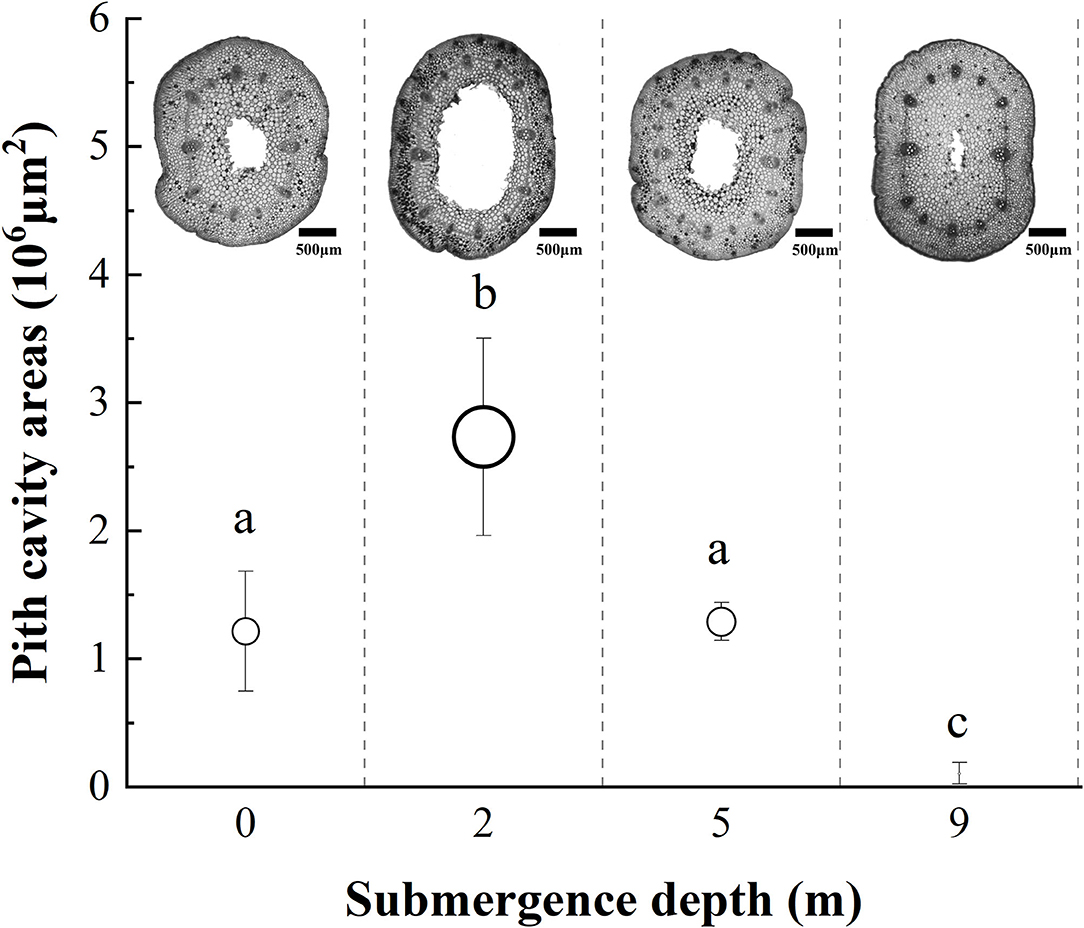
Figure 3. The pith cavity transverse areas of immature internodes of A. philoxeroides at the end of treatment at different submergence depths (mean ± S.E; n = 3). Sections were made at the middle position of internodes. Different lower-case letters indicate significant differences (p < 0.05 using one-way ANOVA) between treatments.
The number of stem nodes forming adventitious roots upon submergence decreased significantly with increasing submergence depth (Figure 4). Approximately 4–9 nodes (an average of 6.5) on plants submerged at depth of 2 m formed adventitious roots, and 0–5 nodes (an average of 1) on plants at a submergence depth of 5 m formed adventitious roots, only 1 node of 1 plant (20 plants in total) formed adventitious roots when submerged at depth of 9 m (Figure 4).
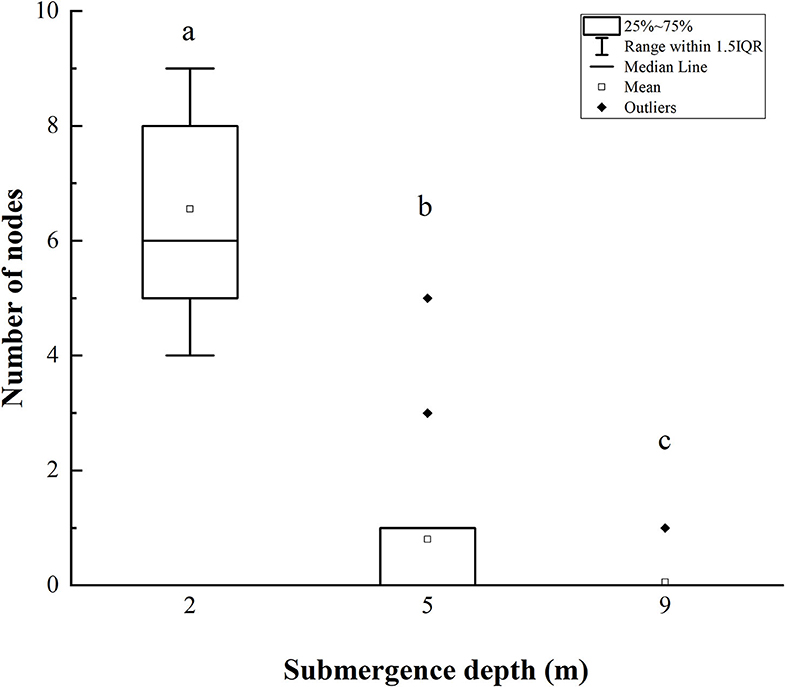
Figure 4. The number of stem nodes forming adventitious roots of A. philoxeroides at the end of submergence treatments (mean ± S.E.; n = 20). Different lower-case letters indicate significant differences (p < 0.05 using one-way ANOVA) between treatments.
It was obvious that increasing water depth impeded the pith cavity development and adventitious root formation of submerged A. philoxeroides plants.
Biomass and Carbohydrates
At the end of the experiment, the total biomass of submerged A. philoxeroides plants decreased gradually with increasing submergence depth, however, unsubmerged plants and plants submerged at a depth 2 m did not differ in total biomass (Figure 5A). The total leaf mass of plants submerged at a depth 9 m was the smallest among all treatments, but the total leaf mass did not differ between plants submerged at depths of 2 and 5 m, and plants unsubmerged. The largest and the smallest stem mass presented respectively in plants submerged at depths of 2 and 9 m, and plants submerged at depth of 5 m had similar stem mass to control plants (Figure 5B). Unlike the responses of stem and total leaf mass to treatments, the underground mass of plants declined with increasing submergence depths, plants unsubmerged tended to achieve the largest underground mass, and plants submerged at a depth 9 m had the smallest underground mass (Figure 5B).
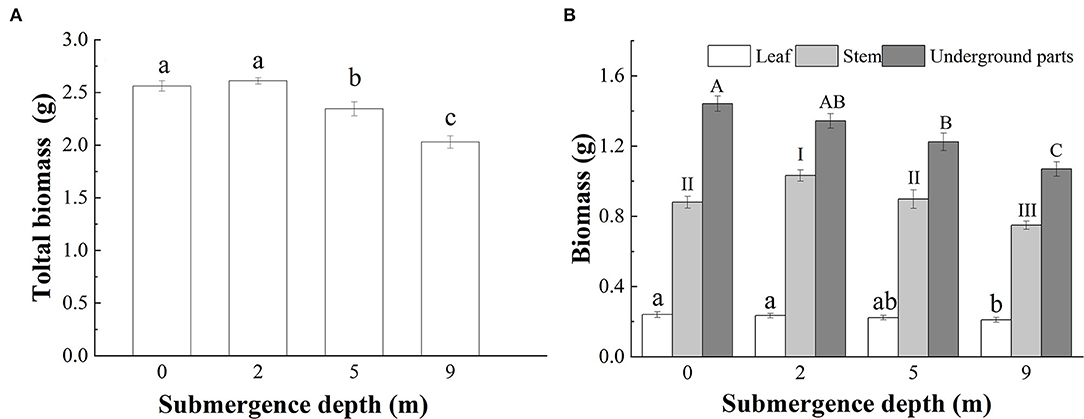
Figure 5. Biomass of (A) whole plant; and (B) leaves, stems, and underground parts of A. philoxeroides at the end of submergence treatments (Mean ± S.E, n = 20). Different lower-case letters indicate significant differences (p < 0.05) in the total plant mass (A) and the leaf mass (B) between treatments, different roman letters, and upper-case letters, respectively indicate significant differences (p < 0.05) in stem mass and underground plant mass between treatments.
The non-structural carbohydrates of A. philoxeroides plants were mainly composed of soluble sugars. At the end of the experiment, the concentration of soluble sugars in both stems and underground parts of submerged plants decreased with increasing submergence depth (Figures 6A,B). As to the concentration of soluble sugars in stems, unsubmerged plants were not different from plants submerged at depth of 5 m but were lower than plants submerged at depth of 2 m and higher than plants submerged at depth of 9 m (Figure 6A). Unsubmerged plants were similar to plants submerged at depth of 2 m in the concentration of soluble sugars in the underground plant parts but had a higher concentration of soluble sugars than plants submerged at depth of 5 and 9 m (Figure 6B).
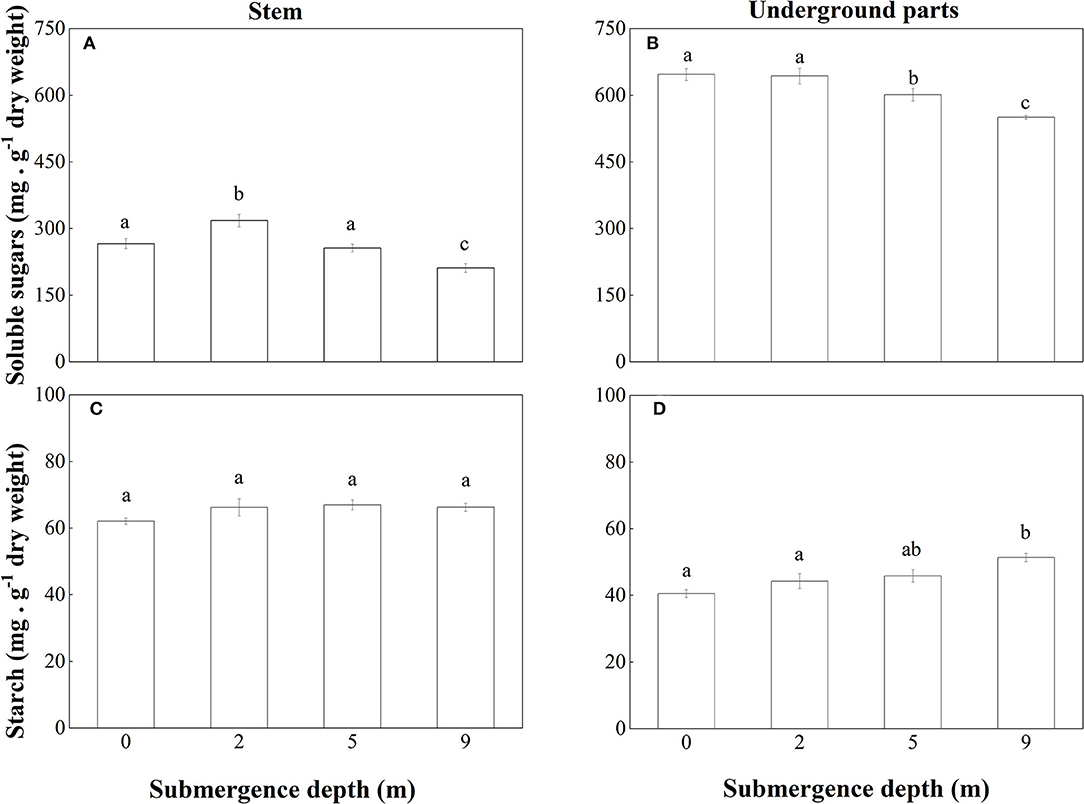
Figure 6. Concentrations of soluble sugars in (A) stems and (B) underground parts; starch in (C) stems and (D) underground parts of A. philoxeroides at the end of the submergence treatments (Mean ± S.E; n = 5). Different lower-case letters indicate significant differences (p < 0.05 using one-way ANOVA) between treatments.
No significant difference was found between treatments in starch concentration in stems of A. philoxeroides (Figure 6C). But starch concentration in underground plant parts tended to increase with increasing submergence depth, plants submerged at depth of 9 m had the highest concentration of starch (Figure 6D).
Discussion
This study aimed to investigate the responses of terrestrial plants to alteration in submergence depth. In some previous studies, the escape or quiescence strategies selected by submerged plants were extensively discussed (Bailey-Serres and Voesenek, 2008; Voesenek and Bailey-Serres, 2015). The strategy selection by plant species was directly linked to where the species is able to distribute in habitats prone to flooding at various depths (Parolin, 2002). However, whether species having wide distribution in flood-prone habitats can alter their response strategy to cope with various submergence depths is rarely discussed. Using A. philoxeroides as a model species, this study demonstrated that strategy change as a response to submergence depths alteration was possible in flood-tolerant plants.
Escape Strategy at Submergence Depth of 2 m
A. philoxeroides exhibited a typical “escape strategy” when submerged at depth of 2 m via fast shoot elongation by producing new internodes and elongating its immature internodes (Figure 2, Supplementary Figure S1, Table 2). Although previous studies showed that escape strategy was mainly presented in plants submerged partially or in waters shallower than 2 m depth (Bailey-Serres and Voesenek, 2008; Manzur et al., 2009; Akman et al., 2012), our present work indicated that escape strategy can also be selected by A. philoxeroides under submergence depths equal or larger than 2 m, which implies that A. philoxeroides can escape from the complete submergence with a water depth of 2 m or even deeper. Theoretically, the fast elongation of stems under submergence at depth of 2 m might be lethal to plants because of the substantial energy consumption and consequent energy crisis. However, our experimental results showed that the biomass and carbohydrate consumption of plants at 2 m submergence depth did not differ from those of unsubmerged plants at the end of the experiment (Figures 5, 6). This might be due to the morpho-physiological behaviors the plant took to help mitigate possible damages, especially energy crisis. First, adventitious root formation was enhanced in A. philoxeroides plants submerged at depth of 2 m (Figure 4), which was beneficial for plants to improve the supply of oxygen and mineral nutrients under submergence stress, because adventitious roots are able to absorb oxygen and mineral nutrients from water (Ayi et al., 2016, 2019). Second, the plants submerged at depth of 2 m developed the widest internodal pith cavity (Figure 3), which surely facilitated plants' internal gas transport (Jackson and Armstrong, 1999; Pedersen et al., 2021). Therefore, our results indicated that A. philoxeroides was able to resist submergence of 2 m depth by adopting an escape strategy via stem elongation, pith cavity development, and adventitious roots formation.
Quiescence Strategy at Submergence Depth of 9 m
When submerged at depth of 9 m, A. philoxeroides adopted a quiescence strategy by minimizing its growth, including almost no new internode production (Table 2) and new internode elongation (Figure 2), suppressed pith cavity development (Figure 3) and adventitious root formation (Figure 4). This quiescence had a “two-edged sword” effect. On one hand, the weakened growth of new tissues and organs led to low consumption of substances including carbohydrates; on the other hand, suppressed pith cavity development and adventitious roots formation decreased the utilization efficiency of carbohydrates under deep submergence conditions. The former would reduce the carbohydrate requirement of submerged plants and enhance their submergence tolerance; the latter may lead to fast carbohydrate consumption, which would be lethal under prolonged submergence. Our results showed that the loss of total biomass in plants submerged at depth of 9 m was significantly higher than that in unsubmerged plants (Figure 5), and the concentration of soluble sugars was lower in plants submerged at depth of 9 m than in unsubmerged plants (Figures 6A,B). Nevertheless, all plants submerged at depth of 9 m survived with no tissue corruption, indicating the high submergence tolerance of A. philoxeroides.
Strategy Shift at Intermediate Submergence Depth
The responses of A. philoxeroides to submergence at depth of 5 m indicated that the plant was probably in the process of strategy shift from escape to quiescence. Plants submerged at depth of 5 m presented larger stem elongation than unsubmerged controls (Figure 2), which was an obvious sign of escape strategy. However, the reduced adventitious root formation, decreased total plant biomass, and lowered soluble sugars concentration in the underground parts of plants submerged at depth of 5 m (Figures 4–6), as compared to those of unsubmerged controls, revealed quiescence strategy was also adopted by plants submerged at depth of 5 m. Thus, we inferred that A. philoxeroides may gradually switch its strategy from “escape” to “quiescence” as a response to submergence with increasing depth. It was reported that Lotus tenuis could quickly switch from an escape strategy under partial submergence to a quiescence strategy when confronted with complete shallow submergence (Manzur et al., 2009). Obviously, A. philoxeroides did not take this quick strategy switchover but performed gradual change in strategy when submergence depth altered.
Previous studies have pointed out that A. philoxeroides is a representative species that adopts an escape strategy in response to submergence (Luo et al., 2009, 2011; Ayi et al., 2016). This study supported this argument. However, it was also found in this study that A. philoxeroides was able to change its strategy from “escape” to “quiescence” under deep submergence (deeper than 5 m in this study), an intriguing phenomenon that was not observed in previous studies. Based on our experimental results, two questions need to be more focused on in the following studies are raised: (1) do all species that typically adopt escape strategy automatically alter their strategy with increasing submergence depth and (2) what role does the submergence depth play in the strategy switchover?
Role of Hydrostatic Pressure
Low light, whether in terrestrial or in aquatic habitats, is an inducing factor for plants to adopt an escape strategy (via fast shoot and petiole elongation) due to shade avoidance (Mommer et al., 2005; Pierik et al., 2011; Sasidharan et al., 2014). In this study, the stem elongation of A. philoxeroides submerged at depths of 0, 2, and 5 m in darkness substantiated this statement (Table 2; Figure 2); however, the retarded growth of A. philoxeroides submerged at depth of 9 m in the same darkness (Table 2; Figure 2) suggested that the light availability can not sufficiently explain the strategy change along a gradient of submergence depths. If the stem elongation upon submergence were induced by low light or darkness due to phototaxis of plants, plants submerged at any depths in darkness should present similar stem elongation.
Generally, the escape and quiescence strategies represent the syndrome that plants exhibit as a response to low oxygen levels in water (Voesenek and Bailey-Serres, 2013, 2015). Oxygen shortage might induce the production of reactive oxygen species (Paradiso et al., 2016; Sasidharan et al., 2018), nitric oxide (Mugnai et al., 2012; Paradiso et al., 2016), ethylene (Fukao and Bailey-Serres, 2008; Sasidharan et al., 2018), and other signaling molecules (Sasidharan et al., 2018), as well as activate a series of phytohormone-synthesizing molecules (Cox et al., 2004) to induce different strategies among species. In plant species adopting an escape strategy, the low oxygen level not only stimulates the elongation of shoots and petioles, but also induces aerenchyma formation, via increasing porosity in the roots or stem (Parlanti et al., 2011; Pedersen et al., 2021), widening the pith cavity (Steffens et al., 2011), and forming adventitious roots (Zhang et al., 2015b; Ayi et al., 2016). However, in a water body with dissolved oxygen saturated (like the water environment A. philoxeroides experienced in this study), it is hard to attribute the strategy shift of plants submerged at different water depths to oxygen availability.
In our experimental system, all factors except water depth were kept constant (Table 1). Therefore, it is logical to infer that water depth was linked to the strategy changeover of A. philoxeroides. It was found in previous studies that water depth affected plant distribution via hydrostatic pressure (Dale, 1981; Makarov, 2011). Some studies suggested that low hydrostatic pressure could accelerate physiological activities and stimulate plant growth, whereas high hydrostatic pressure may affect the stabilization of cells or enzymes, depress physiological activities, and impede cell division or elongation (Adkins et al., 1990; Vervuren et al., 2003; Yi et al., 2016; Bejarano et al., 2018). The strategy changeover of A. philoxeroides along submergence depth gradient in this study was very probably caused by the change in hydrostatic pressure, but how hydrostatic pressure induces strategy changeover needs to be clarified.
Conclusion
This study revealed that submerged A. philoxeroides can gradually change its response strategy from “escape strategy” to “quiescence strategy” when water depth was increasing. Notably, this changeover took place under deep submergence (deeper than 5 m), which implies that water depth plays an important role in the strategy selection of plants in response to submergence. According to our observations, we found the morphological responses of A. philoxeroides to submergence of different depths were chiefly the outcome of acclimatization, because the plants presenting strong stem elongation under shallow submergence did not exhibit stem elongation when transferred to deep submergence, and plants presenting no stem elongation under deep submergence can still show strong stem elongation upon shallow submergence. Undoubtedly, this acclimatization enhances greatly the capability of A. philoxeroides in coping with floods with unpredictable depths. Further studies should focus on understanding the driving mechanism of strategy changeover of plants submerged at different water depths, especially the driving effects of hydrostatic pressure.
Data Availability Statement
The original contributions presented in the study are included in the article/Supplementary Material, further inquiries can be directed to the corresponding author/s.
Author Contributions
BZ and XZ conceived the original research plan, designed, and supervised the experiments. SJ and HN performed most of the experiments. BW, XR, XS, and SS provided assistance for some experiments. SJ, XZ, QA, and BZ analyzed the data and wrote the article with contributions from SL and FL. All authors contributed to the article and approved the submitted version.
Funding
This work was supported by the National Natural Science Foundation of China (Grant Numbers 31400480, 31800331, and 31770465) and Chongqing Talents Program (Grant Number cstc2021ycjh-bgzxm0316).
Conflict of Interest
The authors declare that the research was conducted in the absence of any commercial or financial relationships that could be construed as a potential conflict of interest.
Publisher's Note
All claims expressed in this article are solely those of the authors and do not necessarily represent those of their affiliated organizations, or those of the publisher, the editors and the reviewers. Any product that may be evaluated in this article, or claim that may be made by its manufacturer, is not guaranteed or endorsed by the publisher.
Acknowledgments
The authors thank Xingrong Wu, Senrui Zhang, Dongdong Ji, Meng Wang, Jine Huang, Zhao Zhang, and all the people who helped with the fieldwork over the course of the experiments. We thank Xiaoqi Ye for helping amend the article.
Supplementary Material
The Supplementary Material for this article can be found online at: https://www.frontiersin.org/articles/10.3389/fpls.2022.883800/full#supplementary-material
References
Adkins, S. W., Shiraishi, T., and McComh, J. A. (1990). Submergence tolerance of rice-a new glasshouse method for the experimental submergence of plants. Physiol. Plantarum 80, 642–646. doi: 10.1111/j.1399-3054.1990.tb05691.x
Akman, M., Bhikharie, A. V., McLean, E. H., Boonman, A., Visser, E. J. W., Schranz, M. E., et al. (2012). Wait or escape? contrasting submergence tolerance strategies of rorippa amphibia, rorippa sylvestris and their hybrid. Ann. Bot. 109, 1263–1276. doi: 10.1093/aob/mcs059
Ayi, Q., Zeng, B., Liu, J., Li, S., van Bodegom, P. M., and Cornelissen, J. H. C. (2016). Oxygen absorption by adventitious roots promotes the survival of completely submerged terrestrial plants. Ann. Bot. 118, 675–683. doi: 10.1093/aob/mcw051
Ayi, Q., Zeng, B., Yang, K., Lin, F., Zhang, X., van Bodegom, P. M., et al. (2019). Similar growth performance but contrasting biomass allocation of root-flooded terrestrial plant Alternanthera philoxeroides (Mart.) Griseb. in response to nutrient versus dissolved oxygen stress. Front. Plant Sci. 10, 111. doi: 10.3389/fpls.2019.00111
Bailey-Serres, J., Fukao, T., Gibbs, D. J., Holdsworth, M. J., Lee, S. C., Licausi, F., et al. (2012). Making sense of low oxygen sensing. Trends Plant Sci. 17, 129–138. doi: 10.1016/j.tplants.2011.12.004
Bailey-Serres, J., and Voesenek, L. A. C. J. (2008). Flooding stress: acclimations and genetic diversity. Annu. Rev. Plant Biol. 59, 313–339. doi: 10.1146/annurev.arplant.59.032607.092752
Bejarano, M. D., Jansson, R., and Nilsson, C. (2018). The effects of hydropeaking on riverine plants: a review. Biol. Rev. 93, 658–673. doi: 10.1111/brv.12362
Belt, C. B. J. (1975). The 1973 flood and man's constriction of the Mississippi river. Science 189, 681–684. doi: 10.1126/science.189.4204.681
Bertola, M., Viglione, A., Lun, D., Hall, J., and Blöschl, G. (2020). Flood trends in Europe: are changes in small and big floods different? Hydrol. Earth Syst. Sc. 24, 1805–1822. doi: 10.5194/hess-24-1805-2020
Blöschl, G., Hall, J., Viglione, A., Perdigão, R. A. P., Parajka, J., Merz, B., et al. (2019). Changing climate both increases and decreases European river floods. Nature 573, 108–111. doi: 10.1038/s41586-019-1495-6
Brock, T. C. M., van der Velde, G., and van de Steeg, H. M. (1987). The effects of extreme water level fluctuations on the wetland vegetation of a nymphaeid-dominated oxbow lake in the Netherlands. Arch. für Hydrobiologie 27, 57–73.
Casanova, M. T., and Brock, M. A. (2000). How do depth, duration and frequency of flooding influence the establishment of wetland plant communities? Plant Ecol. 147, 237–250. doi: 10.1023/A:1009875226637
Cox, M. C. H., Benschop, J. J., Vreeburg, R., Wagemakers, C., Moritz, T., Peeters, A., et al. (2004). The roles of ethylene, auxin, abscisic acid, and gibberellin in the hyponastic growth of submerged Rumex palustris petioles. Plant Physiol. 136, 2948–2960. doi: 10.1104/pp.104.049197
Dale, H. M. (1981). Hydrostatic pressure as the controlling factor in the depth distribution of Eurasian watermilfoil, Myriophyllum spicatum L. Hydrobiologia 79, 239–244. doi: 10.1007/BF00006319
Dong, B., van Kleunen, M., and Yu, F. (2018). Context-dependent parental effects on clonal offspring performance. Front. Plant Sci. 9, :1824. doi: 10.3389/fpls.2018.01824
Dynesius, M., and Nilsson, C. (1994). Fragmentation and flow regulation of river systems in the Northern Third of the World. Science 266, 753–762. doi: 10.1126/science.266.5186.753
Fukao, T., and Bailey-Serres, J. (2008). Ethylene-a key regulator of submergence responses in rice. Plant Sci. 175, 43–51. doi: 10.1016/j.plantsci.2007.12.002
Fukao, T., Barrera-Figueroa, B. E., Juntawong, P., and Peña-Castro, J. M. (2019). Submergence and waterlogging stress in plants: a review highlighting research opportunities and understudied aspects. Front. Plant Sci. 10, 340. doi: 10.3389/fpls.2019.00340
Grace, J. B. (1989). Effects of water depth on Typha latifolia and Typha domingensis. Am. J. Bot. 76, 762–768. doi: 10.1002/j.1537-2197.1989.tb11371.x
Groeneveld, H. W., and Voesenek, L. A. C. J. (2003). Submergence-induced petiole elongation in Rumex palustris is controlled by developmental stage and storage compounds. Plant Soil 253, 115–123. doi: 10.1023/A:1024511232626
Howard, R. J., and Mendelssohn, I. A. (1995). Effect of increased water depth on growth of a common perennial freshwater-intermediate marsh species in Coastal Louisiana. Wetlands 15, 82–91. doi: 10.1007/BF03160683
Huang, F., Zhang, N., Ma, X., Zhao, D., Guo, L., Ren, L., et al. (2016). Multiple changes in the hydrologic regime of the Yangtze River and the possible impact of reservoirs. Water 8, 408. doi: 10.3390/w8090408
Huang, Y., Chen, X., Li, F., Hou, Z., Li, X., Zeng, J., et al. (2021). Community trait responses of three dominant macrophytes to variations in flooding during 2011–2019 in a Yangtze River-connected floodplain wetland (Dongting Lake, China). Front. Plant Sci. 12, 604677. doi: 10.3389/fpls.2021.604677
Huber, H., Chen, X., Hendriks, M., Keijsers, D., Voesenek, L. A. C. J., Pierik, R., et al. (2012). Plasticity as a plastic response: how submergence-induced leaf elongation in Rumex palustris depends on light and nutrient availability in its early life stage. New Phytol. 194, 572–582. doi: 10.1111/j.1469-8137.2012.04075.x
Jackson, M. B. (1985). Ethylene and responses of plants to soil waterlogging and submergence. Annu. Rev. Plant Biol. 36, 145–174. doi: 10.1146/annurev.pp.36.060185.001045
Jackson, M. B., and Armstrong, W. (1999). Formation of aerenchyma and the processes of plant ventilation in relation to soil flooding and submergence. Plant Biol. 1, 274–287. doi: 10.1111/j.1438-8677.1999.tb00253.x
Lehner, B., Liermann, C. R., Revenga, C., Vörösmarty, C., Fekete, B., Crouzet, P., et al. (2011). High-resolution mapping of the world's reservoirs and dams for sustainable river-flow management. Front. Ecol. Environ. 9, 494–502. doi: 10.1890/100125
Lei, S., Zeng, B., Xu, S., and Zhang, X. (2017). Response of basal metabolic rate to complete submergence of riparian species Salix variegata in the Three Gorges Reservoir region. Sci. Rep. 7, 13885. doi: 10.1038/s41598-017-13467-0
Lei, S., Zeng, B., Yuan, Z., and Su, X. (2014). Changes in carbohydrate content and membrane stability of two ecotypes of Calamagrostis arundinacea growing at different elevations in the drawdown zone of the Three Gorges Reservoir. Plos One 9, e91394. doi: 10.1371/journal.pone.0091394
Luo, F., Nagel, K. A., Scharr, H., Zeng, B., Schurr, U., and Matsubara, S. (2011). Recovery dynamics of growth, photosynthesis and carbohydrate accumulation after de-submergence: a comparison between two wetland plants showing escape and quiescence strategies. Ann. Bot. 107, 49–63. doi: 10.1093/aob/mcq212
Luo, F., Nagel, K. A., Zeng, B., Schurr, U., and Matsubara, S. (2009). Photosynthetic acclimation is important for post-submergence recovery of photosynthesis and growth in two riparian species. Ann. Bot. 104, 1435–1444. doi: 10.1093/aob/mcp257
Makarov, M. V. (2011). Effect of the hydrostatic pressure on the vertical distribution of Laminaria saccharina (L.) Lamouroux in the Barents Sea. Oceanology 51, 457–464. doi: 10.1134/S0001437011030155
Manzur, M. E., Grimoldi, A. A., Insausti, P., and Striker, G. G. (2009). Escape from water or remain quiescent? lotus tenuis changes its strategy depending on depth of submergence. Ann. Bot. 104, 1163–1169. doi: 10.1093/aob/mcp203
Meng, Y., Yu, S., Yu, Y., and Jiang, L. (2022). Flooding depth and duration concomitantly influence the growth traits and yield of rice. Irrig. Drain. 71, 94–107. doi: 10.1002/ird.2632
Milly, P. C. D., Wetherald, R. T., Dunne, K. A., and Delworth, T. L. (2002). Increasing risk of great floods in a changing climate. Nature 514–517. doi: 10.1038/415514a
Mommer, L., De Kroon, H., Pierik, R., Bögemann, G. M., and Visser, E. J. W. (2005). A functional comparison of acclimation to shade and submergence in two terrestrial plant species. New Phytol. 167, 197–206. doi: 10.1111/j.1469-8137.2005.01404.x
Mugnai, S., Azzarello, E., Baluska, F., and Mancuso, S. (2012). Local root apex hypoxia induces NO-mediated hypoxic acclimation of the entire root. Plant Cell Physiol. 53, 912–920. doi: 10.1093/pcp/pcs034
Müller, J. T., van Veen, H., Bartylla, M. M., Akman, M., Pedersen, O., Sun, P., et al. (2019). Keeping the shoot above water-submergence triggers antithetical growth responses in stems and petioles of watercress (Nasturtium officinale). New Phytol. 229, 140–155. doi: 10.1111/nph.16350
Paradiso, A., Caretto, S., Leone, A., Bove, A., Nisi, R., and De Gara, L. (2016). ROS production and scavenging under anoxia and re-oxygenation in Arabidopsis cells: a balance between redox signaling and impairment. Front. Plant Sci. 7, 1803. doi: 10.3389/fpls.2016.01803
Parlanti, S., Kudahettige, N. P., Lombardi, L., Mensuali-Sodi, A., Alpi, A., Perata, P., et al. (2011). Distinct mechanisms for aerenchyma formation in leaf sheaths of rice genotypes displaying a quiescence or escape strategy for flooding tolerance. Ann. Bot. 107, 1335–1343. doi: 10.1093/aob/mcr086
Parolin, P. (2002). Submergence tolerance vs. escape from submergence: two strategies of seedling establishment in Amazonian floodplains. Environ. Exp. Bot. 48, 177–186. doi: 10.1016/S0098-8472(02)00036-9
Pedersen, O., Perata, P., and Voesenek, L. A. C. J. (2017). Flooding and low oxygen responses in plants. Funct. Plant Biol. 44, iii–vi. doi: 10.1071/FPv44n9_FO
Pedersen, O., Sauter, M., Colmer, T. D., and Nakazono, M. (2021). Regulation of root adaptive anatomical and morphological traits during low soil oxygen. New Phytol. 229, 42–49. doi: 10.1111/nph.16375
Pierik, R., De Wit, M., and Voesenek, L. A. C. J. (2011). Growth-mediated stress escape: convergence of signal transduction pathways activated upon exposure to two different environmental stresses. New Phytol. 189, 122–134. doi: 10.1111/j.1469-8137.2010.03458.x
Pierik, R., van Aken, J. M., and Voesenek, L. A. C. J. (2009). Is elongation-induced leaf emergence beneficial for submerged Rumex species? Ann. Bot. 103, 353–357. doi: 10.1093/aob/mcn143
Sasidharan, R., Hartman, S., Liu, Z., Martopawiro, S., Sajeev, N., van Veen, H., et al. (2018). Signal dynamics and interactions during flooding stress. Plant Physiol. 176, 1106–1117. doi: 10.1104/pp.17.01232
Sasidharan, R., Keuskamp, D. H., Kooke, R., Voesenek, L. A. C. J., Pierik, R., and Huq, E. (2014). Interactions between auxin, microtubules and XTHs mediate green shade-induced petiole elongation in Arabidopsis. Plos One 9, e90587. doi: 10.1371/journal.pone.0090587
Sauter, M. (2000). Rice in deep water: “How to take heed against a sea of troubles”. Naturwissenschaften 87, 289–303. doi: 10.1007/s001140050725
Sauter, M., Seagull, R. W., and Kende, H. (1993). Internodal elongation and orientation of cellulose microfibrils and microtubules in deepwater rice. Planta 190, 354–362. doi: 10.1007/BF00196964
Sparks, R. E., Nelson, J. C., and Yin, Y. (1998). Naturalization of the flood regime in regulated rivers-the case of the upper Mississippi River. Bioscience 48, 706–720. doi: 10.2307/1313334
Steffens, B., Geske, T., and Sauter, M. (2011). Aerenchyma formation in the rice stem and its promotion by H2O2. New Phytol. 190, 369–378. doi: 10.1111/j.1469-8137.2010.03496.x
Striker, G. G., Casas, C., Kuang, X., and Grimoldi, A. N. A. (2017). No escape? costs and benefits of leaf de-submergence in the pasture grass chloris gayana under different flooding regimes. Funct. Plant Biol. 44, 899. doi: 10.1071/FP17128
Striker, G. G., Kotula, L., and Colmer, T. D. (2019). Tolerance to partial and complete submergence in the forage legume Melilotus siculus: an evaluation of 15 accessions for petiole hyponastic response and gas-filled spaces, leaf hydrophobicity and gas films, and root phellem. Ann. Bot. 123, 169–180. doi: 10.1093/aob/mcy153
van Veen, H., Vashisht, D., Voesenek, L. A. C. J., and Sasidharan, R. (2014). “Different survival strategies amongst plants to cope with underwater conditions,” in Low-oxygen stress in plants: oxygen sensing and adaptive responses to hypoxia, eds van Dongen, JT, and Licausi, F, (Vienna: Springer), p. 329–349.
Vervuren, P. J., Blom, C. W., and de Kroon, H. (2003). Extreme flooding events on the Rhine and the survival and distribution of riparian plant species. J. Ecol. 91, 135–146. doi: 10.1046/j.1365-2745.2003.00749.x
Voesenek, L. A. C. J., and Bailey-Serres, J. (2013). Flooding tolerance: O2 sensing and survival strategies. Curr. Opin. Plant Biol. 16, 647–653. doi: 10.1016/j.pbi.2013.06.008
Voesenek, L. A. C. J., and Bailey-Serres, J. (2015). Flood adaptive traits and processes: an overview. New Phytol. 206, 57–73. doi: 10.1111/nph.13209
Voesenek, L. A. C. J., Colmer, T. D., Pierik, R., Millenaar, F. F., and Peeters, A. J. M. (2006). How plants cope with complete submergence. New Phytol. 170, 213–226. doi: 10.1111/j.1469-8137.2006.01692.x
Ye, X. Q., Meng, J. L., Zeng, B., Wu, M., Zhang, Y. Y., and Zhang, X. P. (2016). Submergence causes similar carbohydrate starvation but faster post-stress recovery than darkness in Alternanthera philoxeroides plants. Plos One 11, e165193. doi: 10.1371/journal.pone.0165193
Yi, J., Feng, H., Bi, J., Zhou, M., Zhou, L., Cao, J., et al. (2016). High hydrostatic pressure induced physiological changes and physical damages in Asparagus spears. Postharvest Biol. Tec. 118, 1–10. doi: 10.1016/j.postharvbio.2016.03.015
Zhang, H., Wang, R., Wang, X., Du, N., Ge, X., Du, Y., et al. (2015a). Recurrent water level fluctuation alleviates the effects of submergence stress on the invasive riparian plant Alternanthera philoxeroides. Plos One 10, e129549. doi: 10.1371/journal.pone.0129549
Zhang, Q., Huber, H., Beljaars, S. J. M., Birnbaum, D., de Best, S., de Kroon, H., et al. (2017). Benefits of flooding-induced aquatic adventitious roots depend on the duration of submergence: linking plant performance to root functioning. Ann. Bot. 120, 171–180. doi: 10.1093/aob/mcx049
Zhang, Q., Visser, E. J. W., de Kroon, H., and Huber, H. (2015b). Life cycle stage and water depth affect flooding-induced adventitious root formation in the terrestrial species Solanum dulcamara. Ann. Bot. 116, 279–290. doi: 10.1093/aob/mcv095
Keywords: adaptive strategy, submergence-tolerant plants, hydrostatic pressure, flood-prone habitats, submergence depths
Citation: Jing S, Zhang X, Niu H, Lin F, Ayi Q, Wan B, Ren X, Su X, Shi S, Liu S and Zeng B (2022) Differential Growth Responses of Alternanthera philoxeroides as Affected by Submergence Depths. Front. Plant Sci. 13:883800. doi: 10.3389/fpls.2022.883800
Received: 25 February 2022; Accepted: 27 April 2022;
Published: 02 June 2022.
Edited by:
Bi-Cheng Dong, Beijing Forestry University, ChinaCopyright © 2022 Jing, Zhang, Niu, Lin, Ayi, Wan, Ren, Su, Shi, Liu and Zeng. This is an open-access article distributed under the terms of the Creative Commons Attribution License (CC BY). The use, distribution or reproduction in other forums is permitted, provided the original author(s) and the copyright owner(s) are credited and that the original publication in this journal is cited, in accordance with accepted academic practice. No use, distribution or reproduction is permitted which does not comply with these terms.
*Correspondence: Xiaoping Zhang, enhwaW1tdW5Ac3d1LmVkdS5jbg==; Bo Zeng, YnplbmdAc3d1LmVkdS5jbg==
†These authors have contributed equally to this work