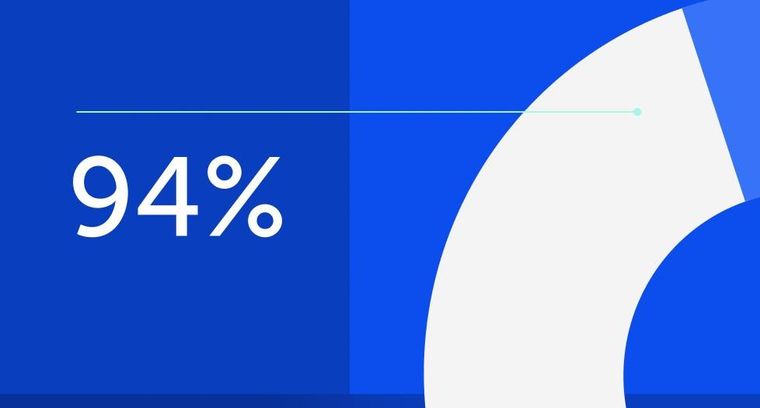
94% of researchers rate our articles as excellent or good
Learn more about the work of our research integrity team to safeguard the quality of each article we publish.
Find out more
REVIEW article
Front. Plant Sci., 29 April 2022
Sec. Plant Biotechnology
Volume 13 - 2022 | https://doi.org/10.3389/fpls.2022.879366
This article is part of the Research TopicInsights in Plant Biotechnology: 2021View all 22 articles
An increase in temperature and extreme heat stress is responsible for the global reduction in maize yield. Heat stress affects the integrity of the plasma membrane functioning of mitochondria and chloroplast, which further results in the over-accumulation of reactive oxygen species. The activation of a signal cascade subsequently induces the transcription of heat shock proteins. The denaturation and accumulation of misfolded or unfolded proteins generate cell toxicity, leading to death. Therefore, developing maize cultivars with significant heat tolerance is urgently required. Despite the explored molecular mechanism underlying heat stress response in some plant species, the precise genetic engineering of maize is required to develop high heat-tolerant varieties. Several agronomic management practices, such as soil and nutrient management, plantation rate, timing, crop rotation, and irrigation, are beneficial along with the advanced molecular strategies to counter the elevated heat stress experienced by maize. This review summarizes heat stress sensing, induction of signaling cascade, symptoms, heat stress-related genes, the molecular feature of maize response, and approaches used in developing heat-tolerant maize varieties.
Graphical Abstract. This review summarized heat stress-mediated morphological and physiological changes in maize and elucidated the molecular mechanisms responsible for maize response to heat stress. Furthermore, plausible approaches to dissecting the regulatory network associated with heat stress response and improving maize adaptation to global warming have been discussed. This figure was made using BioRender.
Heat stress is the most devastating abiotic stress factor influencing seasonal growth and spatial variations in various crops (Sallam et al., 2018; Magaña Ugarte et al., 2019). Global warming caused by the increasing growth of the population and the accompanying industrial development has become a concern that cannot be overlooked (Baus, 2017). Also, the average rise in global temperature between 1900 and 2020 was 1.13°C, and it is expected to increase by 1.4–5.8°C in 2100
(Figure 1; Houghton et al., 2001). This gradual increase in global warming and heat waves have become a serious threat to crop productivity (Hoegh-Guldberg et al., 2019). Data published by the Food and Agriculture Organization has revealed the annual relative yield loss in major cereal crops (Faostat, 2019). Also, recent studies have shown that effective heat stress tolerance via genetic improvement is the only possible remedy; otherwise, every 1°C temperature rise will cause a 6.0% yield loss of wheat, 3.2% of rice, 7.4% of maize, and 3.1% of soybean (Zhao et al., 2017; Kraus et al., 2022). However, due to increasing population growth, crop yield ought to increase by 70% for sustaining food security to meet the demand of a projected 9 billion population rise in 2050 (Popp et al., 2013; Dawson et al., 2016).
Figure 1. (A) Map of annual mean temperature change (°C) during 1900–1910 and 2010–2020. (B) The zonal means plot. Air temperature data of the land surface was retrieved from GHCNv4 (GISS analysis based on global historical climatology network v4), and sea surface temperature data was retrieved from ERSST_v5 (NOAA/NCEI’s extended reconstructed sea surface temperature v5). The number at the top right-hand corner of the map plot is an estimate (°C) of the global mean of the calculated area. The maps were made using GISS Surface Temperature Analysis software (https://data.giss.nasa.gov/gistemp/maps/index.html).
Maize (Zea mays) is an important cereal crop that belongs to the Poaceae family (Li et al., 2022) and has ensured global food security with a worldwide production ≥1 × 109 t (1012 kg) since 2013 (Faostat, 2017). Maize was initially cultivated in tropical areas under rainfed conditions (Li J. et al., 2021; Maitra et al., 2021). However, there is an increased demand for maize due to its utilization of carbohydrates as biomass for ethanol fuel production, leaves and stem as livestock fodder, grains as raw material in the baking industry, and food and feed crop in many countries (Rooney et al., 2007; Parmar et al., 2017; Dar et al., 2021). Maize is a rich source of starch and calcium in addition to numerous essential minerals, vitamins, and fiber. However, it labors to some nutrients, such as vitamins B12 and C (McKevith, 2004). Iron absorption, particularly the non-heme iron present in maize, can be inhibited by some components of the diet being consumed, such as vegetables, coffee (e.g., polyphenols), tea (e.g., oxalates), milk (e.g., calcium), and eggs (e.g., phosvitin) (Ranum et al., 2014).
Elevated temperature accelerates crop growth but shortens its growing season (Mo et al., 2016; Hu et al., 2017; Ahmed et al., 2018; Ihsan et al., 2019). Additionally, maize growth requires an optimum daytime temperature range of 28–32°C, comparatively higher than the optimum temperature necessary for other cereal crops, such as wheat (Triticum aestivum) and rice (Oryza sativa) (Sánchez et al., 2014).
The global change resulting from harsh climatic conditions has negatively affected maize crop yields (Lobell et al., 2011; Ahmed et al., 2018; El-Sappah and Rather(eds)., 2022). Also, increased temperature stimulates the over-accumulation of phenolic compounds, resulting in cell necrosis, consequently contributing to maize yield loss (Tebaldi and Lobell, 2018). Furthermore, heat stress (>32°C) causes the deterioration of several metabolic processes in maize plants, including a severe break in photosynthesis, increased surface transpiration rate (Crafts-Brandner and Salvucci, 2002; Sharma et al., 2020), pollen-sterilization at anthesis (flowering stage) (Gourdji et al., 2013), kernels shortening at grain-filling stage (Singletary et al., 1994; Rezaei et al., 2015), cumulatively resulting in a significant yield loss.
The approval of multiple agronomic and breeding alternatives along with advanced genomic tools is inevitable to cope with the deleterious effects of extreme temperatures (Waqas et al., 2021). Several agronomic management practices, such as the management of soil and nutrients, crop rotation, plantation rate, timing, and irrigation, are beneficial for the development of heat tolerance in maize (Sabagh et al., 2020). Genetically modified crops could also be a valuable resource for the development of novel traits that enhance the survival of plants under harsh conditions (Jha et al., 2020). In recent years, the rate of crop improvement has accelerated owing to the rapid progress in plant molecular biology. In several crops, different genetic approaches, including marker-assisted selection (MAS), map-based gene cloning, quantitative trait locus (QTL) mapping, and genome editing (such as RNA interference [RNAi] and CRISPR)/CRISPR-associated-9, Cas9), have been utilized for the selection and improvement of plant traits (Waqas et al., 2021).
This review summarized heat stress-mediated morphological and physiological changes in maize and elucidated the molecular mechanisms responsible for maize response to heat stress. We also discussed plausible approaches in dissecting the regulatory network associated with heat stress response and improving maize adaptation to global warming.
Technically, the growth of stems, leaves, and roots, usually referred to as vegetative growth, is also known as germination, leaf, and tasseling (Dolatabadian et al., 2010). Heat stress affects the abovementioned growth stages (Figure 2) significantly. Also, the optimum soil temperature for maize seeds germination is 21°C, whereas <13°C causes a severe stoppage in germination and <10°C causes a total cessation (Kaspar and Bland, 1992; Towil, 2010; Sánchez et al., 2014). The germination rate of spring sowing of maize seeds cultivated in higher altitudes, such as North Europe and North America, is comparatively low due to low soil temperature (Paul et al., 1996). Early seed germination may expose the crop to freezing temperature, and early flowering leads to short crop duration leading to severe yield loss (Jagadish et al., 2016). However, late cultivation for optimum temperature conditions caused a severe loss in yield due to pest attacks (Rosenzweig et al., 2001). Therefore, only the day-neutral spring maize is favorably cultivated in higher altitudes (Colasanti and Muszynski, 2009).
Figure 2. Morphological and physiological characteristics of maize under heat stress. This figure was made using BioRender.
Notably, the younger seedlings are less susceptible to high temperatures (Sánchez et al., 2014). The overall required temperature range for early maize seedling growth is 30–35°C, and the optimal temperature is around 20°C (Khaeim et al., 2022), 4–6°C higher than the suitable temperature for wheat and barley growth (Sánchez et al., 2014). Importantly, depending upon maize variety and below 20°C, every 0.5°C downfalls in daily temperature resulted in 10–20 days extended crop duration (Rahman et al., 2009). At an average daytime temperature of 15°C may take 200 days for the maturity of maize crop (Wilson et al., 1995).
Maize is susceptible to cold temperature but can recover from its effects if height is less than 15 cm when exposed to cold (Sakai and Larcher, 2012). Temperature below 10°C causes stunted root growth, whereas 17°C temperature results in 1.5 mm root growth per day, and temperature above 40°C inhibits root growth (Ryel et al., 2002). Maize seedlings can recover from constraints of drought stress because it is naturally resistant to drought (Daryanto et al., 2016). In conclusion, maize can recover from adverse climatic conditions if exposed at very early vegetative growth stages. The early cultivation of maize also facilitates the avoidance of pest attacks and the possible development of diseases (Bruns, 2003). So, early sowing of maize is highly recommended.
The fruit setting stage is the reproductive stage that begins with vegetative growth termination and flowering initiation. The stage is susceptible to unexpected fluctuation in temperature, i.e., >32°C temperature, or frost causing severe yield loss (Silim et al., 2006; Siebers et al., 2017). Also, hailstorm adversely affects outcomes at the jointing and silking stage (Chen K. et al., 2018). Similarly, soil moisture contents before, during, and after silking result in a severe reduction in yield by 25, 21, and 50%, respectively (Pandey et al., 2000). The optimum temperature at tasseling is between 21 and 30°C (Kiniry and Bonhomme, 1991). Additionally, elevated temperature encourages respiration (Guo et al., 2019) and shortens grain-filling duration, contributing to a significant yield loss (Sánchez et al., 2014). Conversely, low-temperature extends the length of the grain-filling period, the appropriate phase change of photosynthesis to dry matter, and grain filling, resulting in a higher yield (Dordas, 2009; Edreira et al., 2014; Chao et al., 2016). Overall, during pollination and grain filling, temperatures ≥35°C suppress fertilization in maize and decreases its yield by 101 kg/ha per day (Naveed et al., 2014; Dawood et al., 2020).
Heat stress causes cell physiological changes, such as inactivating the photosystem II (PSII) reaction center and the denaturation of the lipid bilayer and embedded proteins in the thylakoid membrane, resulting in the damaging of the cell membrane (Yang et al., 1996; Nijabat et al., 2020). The damaged cell membrane has caused severe retardation of ion exchange, leakage of electrolytes, viscous cytosol due to water loss, toxic compounds production, and homeostasis disruption (Stanley and Parkin, 1991; Demidchik, 2015). Also, these changes have resulted in plant growth cessation through leaf wilt, reduced leaf area, and leaf abscission (Bartels and Sunkar, 2005; Mafakheri et al., 2010). Furthermore, the cell membrane stability varies with plant tissue age, growth stage, growing season, plant species, and heat intensity (Nijabat et al., 2020). Therefore, the plant’s retention of its cell membrane stability and water contents under heat stress during the vegetative and reproductive growth period has generated higher yields (Khakwani et al., 2012).
Heat stress stimulates ROS biosynthesis that promotes membranous lipids peroxidation, leakage of cellular contents, protein degradation, enzymatic inactivation, bleaching of chlorophyll pigments, and DNA damage, consequently resulting in necrosis (He and Häder, 2002; Mujahid et al., 2007). Phospholipids-peroxidation causes the production of malondialdehyde (MDA) which causes damage to the cell membrane (Pamplona, 2008; Wadhwa et al., 2012). Additionally, ROS causes polyunsaturated fatty acid peroxidation, leading to chain breakage contributing to increased membrane permeability and fluidity (Catalá, 2009). Notably, the increased accumulation of H2O2 causes lipid peroxidation and membrane damage (Banerjee and Roychoudhury, 2018; Yadav et al., 2018). Heat stress-mediated genetic variations have been investigated in several cereal crops, including wheat, barley, rice, and maize (Kumari et al., 2009; Khajuria et al., 2016; Swapna and Shylaraj, 2017). Balanced redox reaction system activation via enzymatic antioxidants, such as superoxide dismutase, catalase, ascorbate peroxidase, glutathione reductase, and non-enzymatic antioxidants, such as NADH; NADPH; ascorbic acid, glutathione, and secondary metabolites play a crucial role in heat stress tolerance (Wahid et al., 2007; Foyer and Shigeoka, 2011).
Photosynthetic apparatus is highly vulnerable to damage when exposed to heat stress and intense light (Essemine et al., 2012; Li Y. T. et al., 2020). Therefore, heat stress causes a severe reduction in carbon assimilation, restricts electron transfer, aggravates oxidative damage and photoinhibition of PSII, resulting in significant yield loss (Elferjani and Soolanayakanahally, 2018; Li Y. T. et al., 2020). Heat stress also denatures vital enzymes associated with the Calvin cycle, such as rubisco, and reduces carbon assimilation in C3 plants (Dias and Brüggemann, 2010; Zhang et al., 2020a). However, C4 plants, such as maize, harbor the CO2 concentration mechanism (Dai et al., 1993; Majeran and van Wijk, 2009), reducing the restriction of photosynthetic carbon assimilation via the Calvin cycle (von Caemmerer and Furbank, 2016). Furthermore, Phosphoenol pyruvate carboxylase is the highly thermostable initial enzyme involved in the C4 cycle (O’Leary et al., 2011), suggesting that other photosynthesis pathways contribute to declining photosynthetic carbon assimilation under heat stress in maize (Li Y. T. et al., 2020). Notably, the photosynthetic apparatus acclimatizes to heat stress by improving its antioxidant capacity and changing leaf structure and metabolism (Li Y. T. et al., 2020). However, shock heat stress during flowering causes irreparable yield loss by damaging the leaves, rendering them unable to sprout again due to the completion of vegetative growth (Li Y. T. et al., 2020).
Respiration plays a crucial role in photosynthesis, whereas its inhibition suppresses CO2 fixation and photoinhibition (Gardeström and Igamberdiev, 2016). However, stomatal closure does not limit the exchange of gases like CO2 but limits the transpiration rate through leaves. The CO2 concentration mechanism of C4 plants, such as maize leaves, provides more robust resistance to stomatal restriction than in C3 plants (Markelz et al., 2011). Additionally, the blockage of respiratory electron transfer inhibits photorespiration resulting in PSII photoinhibition (Rochaix, 2011; Zhang et al., 2017). Transpiration through stomata is an important heat-dissipating mechanism, with their closure under heat stress resulting in severe loss in net photosynthetic rate (Pn) (Caine et al., 2019). The lower stomatal conductance (Gs) in maize leaves maintains water-use efficiency but damages photosynthetic apparatus under heat stress. Therefore, the lower Gs due to stomata closure indicates less heat dissipation via the transpiration mechanisms in the leaves of C4 plants, such as maize, compared to C3 plants (El-Sharkawy, 2007; Li Y. T. et al., 2020).
Photoinhibition of photosystems (PSI and PSII) in the chloroplast results from the degradation of the light receptors under heat stress contributing to the significant halt in photosynthesis (Zivcak et al., 2015). The oxygen-evolving complex (OEC) of PSII is highly sensitive to heat stress than of high-intensity light, whereas the D1 protein of PSII is more sensitive to high-intensity light instead of heat stress (Vass and Cser, 2009; Tóth et al., 2011). It is reported that heat stress significantly affects the acceptor site of PSII instead of PSI in maize leaves (Yan et al., 2013; Li Y. T. et al., 2020). Accordingly, OEC is the primary site in maize leaf cells affected by heat stress, whereas D1 is the primary site affected by high-intensity light. The over-accumulation of ROS is another cause of D1 protein denaturation (Kong et al., 2013). Therefore, overexpression of OEC and D1 protein and downregulation of ROS via genetic engineering and breeding techniques will improve heat tolerance in maize (Li and Howell, 2021).
Traditionally, metabolites are divided into primary and secondary/specialized metabolites. Primary metabolites reinforce cell and secondary/specialized metabolites are concerned with an organism’s interaction with its environment. Primary metabolism produces precursors for secondary metabolite biosynthesis and plays a direct and central role in plant growth, development, and reproduction. It also produces precursors for secondary metabolite biosynthesis (Medeiros et al., 2021). Secondary metabolites possess functional and chemical diversity (Erb and Kliebenstein, 2020). Thousands of metabolites serve as mediators for the various interactions between plant and the environment (Medeiros et al., 2021). During a stress response, plants fine-tune their metabolic production accordingly; however, the mechanisms, reasons, and regulations for this process are only partially understood.
Leaf metabolites were most affected by long-duration salt, heat, or drought stress treatments compared with the rest of the maize organs. The raffinose pathway metabolites (raffinose and galactinol) and some amino acids such as threonine, tryptophan, and histidine also stood out in the heat stress metabolome profile (Joshi et al., 2021). In the metabolic studies of Joshi et al. (2021), 2,549 genes were upregulated including galactinol synthase (Zm00001d028931), stachyose synthase (Zm00001d039685), and a putative inositol transporter (Zm00001d018803), while 2,587 genes were downregulated as a result of heat stress. Two stress-induced arginine decarboxylase paralogs exhibited a similar dichotomy with drought and heat, inducing Zm00001d051194. However, the responses from pairing drought and heat stressors contrasts with the pattern exhibited by the raffinose pathway genes described above where the effects of heat and salt were correlated (Joshi et al., 2021).
Heat stress adversely affects carbohydrate catabolism by denaturing relevant enzymes resulting in the over-accumulation of starch and sucrose (Ruan et al., 2010; Xalxo et al., 2020). Varied expression patterns of genes and proteins involved in carbohydrate metabolism were observed in Arabidopsis exposed to heat stress (Kaplan et al., 2004). In addition, heat stress causes over-accumulation of maltose, sucrose, and cell wall-specific monosaccharides (Lima et al., 2013; Sengupta et al., 2015). Additionally, the metabolic profiling of plants exposed to two abiotic stress factors, such as drought and heat, showed over-accumulation of glucose, fructose, sucrose, trehalose, maltose responsible for maintaining cell turgor pressure, stabilizing cell membranes and proteins (Rodziewicz et al., 2014; Kumar et al., 2021). During unfavorable conditions, plants digest starch molecules to get energy as a substitute for glucose; however, extended heat stress causes depletion of all carbohydrate reservoirs and causes plants starvation (Kaplan et al., 2004; Djanaguiraman et al., 2010).
Temperature significantly affects starch biosynthesis in maize kernels, which contributes to the total dry weight of grains (Keeling et al., 1994). Heat stress stimulates the production of osmolytes including fructose, mannose, sucrose, and proline, which plays a vital role in heat stress tolerance (Slama et al., 2015; Sharma et al., 2019). The grain-filling rate and duration are determined by the sucrose contents available in kernels and enzyme activity level (Singletary et al., 1994; Alam et al., 2021). Short interval time series analysis revealed that the “tipping point” for maize metabolome perturbation is lengthened at a >1 day of drought stress, including a combined effect of drought and heat stress (Bechtold et al., 2016). Generally, heat stress causes mechanical changes, whereas drought stress results in the disequilibrium of osmosis in plants cell (Haswell and Verslues, 2015). Therefore, abiotic stress-mediated changes in metabolic responses are probably attributed to adaptations to drought and heat stresses (Kaplan et al., 2004; Khan et al., 2015).
Osmolytes also contribute a crucial role in maintaining membrane structure (Sharma et al., 2019), alleviating proteins degradation, reducing ionic toxicity, protecting cell organelles, scavenging ROS, protecting antioxidant compounds, and maintaining redox equilibrium (Hasanuzzaman et al., 2020). Osmolytes, such as sucrose, fructose, and mannose, are resources of energy, nutrition, structural materials, signaling molecules, and crucially contribute to seed germination and the growth of plantlets (Osuna et al., 2015). Maize (Zea mays L.) seedlings exposed to heat stress displayed sudden degradation of glycan contents and upregulated fructose and mannose metabolism (Lieu et al., 2021). The expression of genes involved in fructose, mannose, and sucrose biosynthesis was also upregulated in 21-day-old maize seedlings exposed to heat stress (Stavridou et al., 2021).
The mitochondria and nuclear membrane structure were also disrupted by heat stress, more severe in the heat-sensitive hybrid (Török et al., 2014; Li Y. T. et al., 2020). Also, disruption of mitochondrial membrane structure decreases the efficiency of oxidative phosphorylation, requiring increased consumption of carbohydrates to supply sufficient ATP and further reducing light energy utilization (Li Y. T. et al., 2020). Additionally, many chloroplast proteins are encoded by the nuclear genome; hence, destruction of the nuclear envelope may inhibit the upregulation of photo-protection mechanisms, aggravating the photosynthetic mechanism damage and delaying photo inhibition repair and structural damage (Kumar and Kaushik, 2021). The less grouped PSII units are more sensitive to light, partly explaining the more severe PSII under heat stress (Strasser et al., 2004).
Phytohormones, such as auxin/indole acetic acid (IAA), gibberellic acid (GA), abscisic acid (ABA), cytokinin (CTK), ethylene (ET), salicylic acid (SA), brassinosteroids (BRs), strigolactone (SL), and jasmonic acid (JA) importantly regulates cellular processes which are ubiquitous to plant growth under abiotic stress factors (Sharma et al., 2019). Heat stress causes over-accumulation of ABA and the downregulation of CTK, resulting in the improper development of maize kernels (Cheikh and Jones, 1994; Niu et al., 2021). The application of benzyladenine on maize seedlings maintains a proper balance between ABA and CTK, causing an increased heat tolerance (Cheikh and Jones, 1994). Similarly, the treatment of maize seedlings with Ca2+ ions solution and ABA improves the antioxidant enzyme activity, reduces lipid peroxidation, and improves heat tolerance (Hossain et al., 2015; Yang et al., 2021). Similarly, SA, GA, and H2S stimulate the biosynthesis of proline, betaine, and trehalose, contributing to the enhanced antioxidant activity in maize (Li, 2015; Li Z. G. et al., 2015; Zhou et al., 2018). Overexpression of ZmbZIP4 induces longer primary roots, more lateral roots, and enhanced biosynthesis of ABA, which cumulatively results in enhanced abiotic stress tolerance (Ma et al., 2018).
Plant cells and organelles harbor an efficient heat sensing mechanism that subsequently stimulates a signaling cascade for rapid adaptive modifications (Figure 3; Nievola et al., 2017; Niu and Xiang, 2018). For example, calcium ions (Ca2+) flow through their conducting channels, acting as messengers in a signaling cascade to sense and respond to heat stimuli (Jammes et al., 2011). The plasma membrane is also a primary heat-sensing organelle that contains three types of Ca2+ conducting channels, including voltage-dependent Ca2+-permeable channels (VDCCs), voltage-independent Ca2+-permeable channels (VICCs), depolarization-activated Ca2+-permeable channels (DACCs), and hyperpolarization-activated Ca2+-permeable channels (HACCs) (Horváth et al., 2012; Liu et al., 2018). These channels are also known as cyclic nucleotide-gated ion channels (CNGCs), naturally tetrameric cationic, and comprise six transmembrane domains (Urquhart et al., 2011). Notably these CNGCs can be genetically modified as homotetrameric or heterotetrameric to improve their ability to respond to diverse and variable intensities (Ketehouli et al., 2019; Tan et al., 2020).
Figure 3. A heat stresses responsive regulatory network in maize. Heat stress damages the cell membrane when exposed to heat stress and promotes the release of apoplastic Ca2+. Heat stress disturbs the plasma membrane’s fluidity and permeability, resulting in a loss in function of chloroplasts and mitochondria, higher cytosolic Ca2+, ROS, NO, and over-accumulation of misfolded or unfolded proteins. Increased cytosolic Ca2+, ROS, and NO are secondary messengers and stimulate downstream regulatory networks. Heat stress disrupts protein homeostasis, inducing unfolded-protein response (UPR) and signaling pathways mediated by IRE1-ZmZIP60 and ZmZIP28. The ZmZIP60 activates the expression of a type-A HSF and HSFTF13, which upregulates the expression of HSP genes, i.e., Hsp90. The Ca2+ signaling is essential in heat tolerance of seed-set in maize under field conditions, where calcium, a critical secondary messenger, converges signals transmitted from high temperature, membrane fluidity, calcium efflux, and ABA (among others), amplifying them through the activation downstream of genes, such as HSFA6b, ABF1, CYCD5;1, MutS2, and HSPs during reproductive stage via the MAPK pathway, and eventually enhance maize tolerance to heat stress for seed-set. This figure was made using BioRender.
In maize, 11 plasma membrane-localized CNGC genes were identified, contributing a major role in heat tolerance (Hao and Qiao, 2018). The downregulation of AtCNGC2, AtCNGC6, PpCNGCb, and PpCNGC resulted in an increased accumulation of the following heat shock proteins; Hsp18.2, Hsp25.3, and Hsp70 (Gao et al., 2012; Finka and Goloubinoff, 2014). Glutamate heat receptor-like channels also stimulated the Ca2+ signaling cascade on exposure to heat stress, and the exogenous application of glutamate resulted in improved heat tolerance in maize (Li et al., 2019). Other calcium channel families have been identified as responsible for the heat tolerance capability in maize, such as downregulation of synaptotagmin A that caused the downregulation of HSPs (Yan et al., 2017; Bourgine and Guihur, 2021). Under heat stress, maize annexin, such as AnxZm33 and AnxZm35 expression stimulated HACCs in the roots and cytosol (Bassani et al., 2004; Nichols, 2005; Mortimer et al., 2008; He et al., 2019). Phosphoinositide-specific phospholipases C (PLCs) genes, such as PLC3 and PLC9 are plasma membrane-localized heat sensors that stimulate phosphoinositide-signaling mediated Ca2+ channels (Rupwate and Rajasekharan, 2012; Hayes et al., 2021). For example, ZmPLC1 encodes a PI-PLC, which plays a major role in maize roots during drought stress (Zhai et al., 2013).
Additionally, heat stress alters the normal working of the chloroplasts and mitochondria membranes, resulting in the over-accumulation of ROS simultaneously stimulating the Ca2+ signaling pathway (Li B. et al., 2018; Navarro et al., 2021). ROS, including NADPH-oxidase (NOX) and respiratory burst oxidase homolog, also stimulate signaling cascade for heat tolerance (Sagi and Fluhr, 2006; Takemoto et al., 2007; Chapman et al., 2019). However, the over-accumulation of ROS stimulates a Ca2+ based signaling cascade in the cytosol, which then stimulates phosphorylation mediated calcium-dependent protein kinases (CDPKs), causing a direct activation of the respiratory burst oxidase homolog D (RBOHD) (Gao et al., 2014; Marcec et al., 2019). RTH5 family proteins comprise four transmembrane functional domains responsible for membrane embedding and two EF motifs, FAD and NAD, required for Ca2+transport (Lin et al., 2009; Nestler et al., 2014). In maize, RTH5 protein encodes NOX, distributed among all eukaryotic species (Bedard et al., 2007).
Heat-sensitive CNGC gene families comprise the cyclic nucleotide-binding domain and calmodulin-binding domain (CaMBD), facing toward cytosol (Gao et al., 2012; Duszyn et al., 2019). Ca2+ sensor-dependent transcription regulation depends upon calcineurin b-like protein (CBL), CDPK, and calmodulin (CaM) (Reddy et al., 2004, 2011; Hashimoto and Kudla, 2011). CDPKs can sense Ca2+ to assist their EF-hand domain and transduce Ca2+ signals via their protein kinase domain (Shi et al., 2018). In maize, 35 CDPKs were identified (Ma et al., 2013), and ZmCDPK1 has been characterized in cold-stressed roots and leaves (Weckwerth et al., 2015). CaMs bind with the C-terminal of CNGC family genes to activate the heat shock signaling pathway (Hao and Qiao, 2018), as mitogen-activated protein kinase 6 and calmodulin-binding protein kinase 3 (CBK3) (Yan et al., 2017). In maize, the Ca2+–CaM contributes to the activation of ABA-induced antioxidants and nitric oxide (NO) production (Hu et al., 2007; Sang et al., 2008).
Many TFs, such as bZIP, CAMTA, MYB, and WRKY, bind with CaM proteins due to various abiotic and biotic stresses effects (Table 1; Yang et al., 2013). Among all, the CAMTA-mediated transcriptional regulation network is dominant, contributing against the diverse environmental stresses, including heat stress, salinity, drought, heavy metals, and exogenous application of hormones (Pandey et al., 2013; Yang et al., 2013; Yue et al., 2015). Additionally, CAMTA genes also play a key role in the mutual induction of regulation in expressing different stress-responsive genes and hormones (Reddy et al., 2000; Yang and Poovaiah, 2002). For example, heat stress induces upregulation of multiple ZmCAMTA genes in maize plants (Atkinson et al., 2013). In maize, ZmCAMTA1, ZmCAMTA2, and ZmCAMTA3 have been identified, and their expression was upregulated during heat stress (Yue et al., 2015).
Heat stress affects plasma membrane, mitochondria, endoplasmic reticulum, and chloroplasts, resulting in ROS over-accumulation, a critical secondary signaling messenger (Sewelam et al., 2014; Czarnocka and Karpińskiski, 2018).
When maize was exposed to high temperature, the related genes for protein processing in the endoplasmic reticulum (ER) pathway were significantly enriched, which mainly induced heat shock proteins expressions, such as Hsp40, Hsp70, Hsp90, Hsp100 (Table 2), and small HSP (Qian et al., 2019). In response, heat stress response (HSR) genes, such as MYB, AP2/EREBP, NAC, BRs, HSPs, Rubisco, antioxidants (APX and Glutathione S-transferase), and kinases are activated to respond to ROS (Khan et al., 2019; Jagtap et al., 2020). ROS, such as H2O2 produced by RBOHD, acted as a signaling molecule that directly stimulates mitogen-activated protein kinases, such as MAPK3 and MAPK6, which activate Ca2+ or CDPK-mediated phosphorylation HSFA2 and HSFA4a (Luna et al., 2011; Frederickson Matika and Loake, 2014). H2O2 also directly activates HSFA1a, HSFA4a, and HSFA8 transcription factors, and NO signaling cascade, inducing the binding of heat shock element (HSE) with promoters of HSPs (Miller and Mittler, 2006; Li B. et al., 2018). Phytohormones, such as IAA, CKs, ABA, ET, GA, SA, BRs, and JA, contribute to the signal transduction pathways during heat stress (Eyidogan et al., 2012; Li N. et al., 2021). Several studies indicated calcineurin b-like protein-interacting protein kinase (CIPK) and named sucrose non-fermenting 1-related kinase (SnRK) family members as key players in pollen tube growth seed-set and abiotic stress by perceiving and mediating Ca2 + signaling (Yang et al., 2008; Zhou et al., 2015). The Ca2+ signaling plays an essential role in the heat tolerance of seed-set in maize under field conditions. In this, calcium, as the critical secondary messenger converges signals transmitted from high temperature, membrane fluidity, calcium efflux, and ABA (among others), amplifies them through activation downstream of genes, such as HSFA6b, ABF1, CYCD5;1, MutS2, and HSPs during reproductive stage via the MAPK pathway (Figure 3 and Tables 1, 2), and eventually enhancing maize tolerance to heat stress for seed-set (Gao et al., 2019).
Heat stress stimulates transcription of heat stress factors (HSFs) (Table 1) which subsequently results in overexpression of HSPs to mitigate the effect of heat stress (El-Sappah et al., 2012, 2017). However, only HSF or HSP overexpression has no significant role in heat tolerance, indicating that both gene families act synergistically (Wang et al., 2004).
Maize contains 25 HSFs, further divided into A, B, and C subclasses (Lin et al., 2011). Class A HSFs contribute to transcriptional activation, whereas the rest two classes have no specific role in transcriptional activation due to the absence of specific protein motifs (Reddy et al., 2014; Haider et al., 2021). A master transcription activator HSFA1 stimulates immediate expression of different heat shock responsive transcription factors (TFs), including dehydration responsive element binding protein 2A (DREB2A), HSFA2, HSFA7, HSFBs, and multiprotein-bridging factor 1C (MBF1C) (Yan et al., 2020; Zhao J. et al., 2021). Additionally, heat stress stimulates the transactivation of HSFA1 upon the interaction between Hsp70 and Hsp90 (Ohama et al., 2017).
HSFA1 is comprised two alleles; HSFA1a and HSFA1b (El-Shershaby et al., 2019). HSFA1 stimulates transcription of ERF/AP2 and DREB2A (Mizoi et al., 2012), HSFA2 acts as a heat-inducible trans-activator of different genes (Chauhan et al., 2013), and HSFA3 regulates the expression of DREB2A and DREB2C (Chen et al., 2010). ZmHsf-6 belongs to class A1, ZmHsf-1, ZmHsf-4, ZmHsf-5, and ZmHsf-17 belong to subclass A2, ZmHsf-3, ZmHsf-11, and ZmHsf-25 belong to class B, all contributing key roles in heat tolerance in maize (Table 1; Lin et al., 2011; Zhang et al., 2020b; Jiang et al., 2021). The expression of ZmHsf-6 was localized in pollens, and its expression was upregulated under heat stress (Jiang et al., 2021). Furthermore, Hsp70-2 and Hsp70-4 are downstream targets of ZmHsf-6 and contribute significantly to abiotic stress response (Li H.-C. et al., 2015). The highest expression of ZmHsf-1, ZmHsf-3, and ZmHsf-23 was observed in maize plants exposed to heat stress proving their significant role in maize during heat stress (Tables 1, 2; Lin et al., 2011).
In maize, ZmAP2/ERF is the most prominent TFs family comprised of 292 potential members, out of which 153 belong to the ERF subfamily (Zhou et al., 2012). Also, ZmDREB2A plays an essential role in heat tolerance and during drought tolerance in maize plants (Qin et al., 2007) when subjected to heat stress, DREB2A regulates transcription of HSFA3 by stimulating coactivation complex comprised of NUCLEAR FACTOR Y, SUBUNIT A2 (NF-YA2), DNA POLYMERASE II SUBUNIT B3-1 (DPB3-1)/NF-YC10, and NF-YB3 (Schramm et al., 2008). Additionally, heat stress causes the over-accumulation of secondary heat stress-responsive ROS, with HSFA4a and HSFA8 acting as sensors (Cimini et al., 2019; Xu et al., 2021). The maize genome contains 72 MYB TFs, with only 46 playing a key role in abiotic stress response (Du et al., 2012; Chen Y. et al., 2018). Maize plants exposed to abiotic stress factors including heat, salinity, drought, cold, and ABA resulted in overexpression of ZmMYB-R (Table 2; Liu et al., 2012; Kimotho et al., 2019).
Heat stress interrupts the molecular mechanism of proper protein folding in the ER, which is toxic to ER (Howell, 2013; Fragkostefanakis et al., 2016). Unfolded-protein response (UPR) is an adaptive change in ER that avoids the toxic effect of misfolded proteins (Figure 3; Vitale and Boston, 2008); however, prolonged toxicity resulted in programmed cell death (Iurlaro and Muñoz-Pinedo, 2016). UPR also stimulates the signaling cascade to send an ER message to the nucleus to initiate the expression of toxicity-responsive genes (Neill et al., 2019). ER stress activates UPR via splicing of ZmbZIP60 transcripts with the help of kinase (IRE1) and membrane-localized TFs, such as ZmbZIP17 and a type II membrane protein ZmbZIP28 (Figure 3; Nawkar et al., 2018; Pastor-Cantizano et al., 2020). Both signaling factors bind, producing heterodimers resulting from the upregulation of stress-responsive genes (Gayral et al., 2020). N-terminal domain of bZIP28 transcription factor face toward cytosol, whereas C-terminal domain face toward the lumen of ER (Liu et al., 2007). From ER, bZIP28 was first associated with Sar1 GTPase for packaging inside COPII vesicles and then exported to Golgi bodies for modifications by the Golgi site-1 and site-2 proteases (S1P and S2P) (Chung et al., 2018; Pastor-Cantizano et al., 2020). Under heat stress, the N-terminus of bZIP28 is cleaved by S2P, released inside the cytosol, and finally transported to the nucleus. Similarly, IRE1 activates the bZIP60 transcription factor by splicing and transporting to the nucleus (Reimold et al., 2000; Huang et al., 2017).
The second abiotic stress signaling pathway initiated from ER is comprised of IRE1, a splicing protein, namely kinase/ribonuclease, which activates the bZIP60 transcription factor via proteolysis (Kørner et al., 2015; Pastor-Cantizano et al., 2020). When maize seedlings are exposed to heat stress, the transcript of ZmbZIP60 is activated by splicing and transferred to the nucleus to induce the expression of HSPs (Li Z. et al., 2018). Another ER-localized ZmbZIP17 transcription factor is activated under heat and ABA stress and subsequently transported into the nucleus to transactivate HSPs with the help of UPR (Cacas, 2015). HSPs maintain cell metabolites stability under heat stress (Efeoǧlu, 2009). Major HSPs which play a key role during heat tolerance in maize are ZmHSP16.9, ZmsHSP17, ZmsHSP17.8, ZmsHSP26, ZmHSP68, ZmHSP70, ZmHSP90, and ZmHSP101 (Tables 1, 2; Sun et al., 2012; Klein et al., 2014; Kumar et al., 2019; Zhao Y. et al., 2021). For example, when maize plants are exposed to heat stress at the reproductive stage, ZmHSP101 is overexpressed in pollens to prevent their mortality, keep them viable and result in more grains (Gurley, 2000). Generally, transcriptome studies of four heat-tolerant and four heat-susceptible inbred lines, 607 heat-responsive genes, and 39 heat-tolerance genes were identified (Frey et al., 2015).
Several agronomic management practices, such as soil and nutrients management, crop rotation, plantation rate, timing, and irrigation, are beneficial in heat tolerance in maize (Sabagh et al., 2020). For example, early sowing of longer season varieties can overcome heat stress in spring maize (Liu et al., 2013). Similarly, nighttime subsurface drip irrigation reduces the root-zone causes in soil temperature, resulting in improved growth and yield in maize (Dong et al., 2016). Additionally, optimized irrigation enhances water use efficiency and aids heat tolerance (Tao and Zhang, 2010). Maize crops exposed to drought and heat stresses during vegetative growth are likely to have shortened reproductive growth stage, resulting in yield loss; however, they can be managed by maintaining soil moisture contents at 65% via drip irrigation (Yuan et al., 2004).
Heat stress negatively affects the absorption of adequate concentrations of minerals and nutrients required for normal metabolic and physiological processes (Fahad et al., 2017). For example, nitrogen (N) and magnesium (Mg) are structural parts of chlorophyll, phosphorus (P) is a structural part of nucleic acids (DNA and RNA), and potassium is required for osmotic regulation and activation of enzymes (Waraich et al., 2012; Meena et al., 2020). Additionally, nitrogen plays a key role in utilizing absorbed light, carbon assimilation, and heat tolerance (Meena et al., 2019). Thus, nutrient management can mitigate physiological disorders of maize plants exposed to heat stress, such as applying potassium (K), improving membranes’ stability, and maintaining turgor pressure in maize (Tao and Zhang, 2010). Specifically, nutrient management at the grain-filling stage contributes significantly to increased yield. Additionally, applying bioregulators, such as Putrescine and Thiourea, improved heat tolerance in maize seedlings (Yadav et al., 2017).
Exogenous application of plant growth regulators, such as ABA and CaCl2, play a key role in heat tolerance in maize by improving the capability of PSII and stopping the ROS, respectively (Gong et al., 1997; Tao and Zhang, 2010). Artificial application of auxin also improves abiotic stresses, including drought, salinity, waterlogging, heat and cold stress, UV irradiation, and heavy metals tolerance (Vardhini and Anjum, 2015). Similarly, the CK application helps mitigate the denaturation of proteins metabolites due to over-accumulation of ROS and improves the rate of photosynthesis in maize (Zulfiqar and Ashraf, 2021). Additionally, the application of SA and ABA ameliorate the effects of abiotic stress factors, improve seedling growth, mitigate ROS, stimulate the cell-signaling pathway via biosynthesis of NO, resulting in enhanced plant growth and crop yield (Meena et al., 2015). Exogenous application of GA improves the growth and development of plants via mitigating adverse effects of abiotic stresses (Yamaguchi, 2008). The BRs are a newly discovered group of plant hormones with promising potential in abiotic stress tolerance, ROS tolerance, and heat stress tolerance (Arif et al., 2020).
Availability of genetic variations in a population and relationship among traits is base for any successful plant breeding program (Lorenz et al., 2011; Aruah et al., 2012). The exact knowledge of genetic parameters, including population structure, heritability, and genotypic variance among the traits under selection pressure, helps develop efficient breeding lines (Farshadfar et al., 2013). In traditional breeding, selection procedures have been developed to identify and subsequently multiply maize verities with improved heat tolerance (Gong et al., 2015; Gedil and Menkir, 2019). Breeding heat-tolerant varieties is an effective strategy for improving heat tolerance in the spring maize grain-filling stage (Mishra et al., 2021). Many maize cultivars have been screened for canopy structure, flag leaf stomata, and rate of photosynthesis to obtain maximum yield and heat tolerance (Sah et al., 2020). The application of genetic markers accompanied by next-generation sequencing (NGS) has accelerated various development in breeding techniques (Le Nguyen et al., 2019).
Conventional breeding has significantly improved the selection of heat-tolerant crop varieties (Fu et al., 2012; Bai et al., 2018). During heat stress at the reproductive stage, quantitative trait locus (QTLs) play a major role in pollen production and preservation, receptivity and pollen tube development, proper grain-filling, and post-anthesis leaf senescence (Tiwari and Yadav, 2019). Therefore, the number and origin of QTLs are pivotal to mitigating heat stress (Sharma et al., 2017). Also, the number of QTLs and their roles studied in heat stress-exposed maize seedlings were six during pollen heat tolerance (Tiwari and Yadav, 2019), 11 at two different loci (HSIDY and HSIDYA) during grain-filling located on chromosomes 2, 3, 5, and 9 (Frey et al., 2016) and six during heat susceptibility index (Van Inghelandt et al., 2019). Moreover, 6 and 5 QTLs have been detected associated with pollen tube growth and pollen germination, respectively, using a recombinant inbred population with 45 materials under abiotic stresses, including high temperature (Frova and Sari-Gorla, 1994; Frova et al., 1998). Therefore, these QTLs can be employed in conventional breeding to improve heat tolerance in maize cultivars (Frey et al., 2015). Previously explored maize QTLs can be assessed by exploring the following datasets; http://www.maizegdb.org and http://www.plantstress.com.
Quantitative trait locus are being widely employed in the introgression of favorable alleles in elite maize cultivars via backcrossing and confirmation in F1 (Frey et al., 2016; Cerrudo et al., 2018). Molecular markers including simple sequence repeats (SSR), single nucleotide polymorphisms (SNPs), random amplified polymorphic DNA (RAPD), and amplified fragment length polymorphism associated with heat tolerance are also employed in MAS (El-Sappah et al., 2019; Younis et al., 2020). The SNP and SSR are vast in identifying genotypes with maximum heat tolerance (Sabagh et al., 2020). Genome-wide association study (GWAS) is also a valuable tool in identifying novel QTLs responsible for heat tolerance to improve the genetic pool in maize breeding (Wen et al., 2014; Lafarge et al., 2017; Lin et al., 2020). GWAS is also helpful in revealing the linkage between SNPs and specific traits that confers heat tolerance at the flowering stage (Lafarge et al., 2017). GWAS was performed in sub-tropical maize, identifying significant SNPs and haplotype blocks associated with yield contributing traits that help select donor lines with favorable alleles for multiple characteristics, providing insights into heat stress tolerance genetics (Longmei et al., 2021; Seetharam et al., 2021).
Recently, several gene families have been identified and subsequently characterized in maize involved in heat stress response, such as heat shock protein-70 and heat shock factor (Casaretto et al., 2016; Jagtap et al., 2020; Jiang et al., 2021). Additionally, transcriptomic profiling of maize seedlings exposed to heat stress showed several differentially expressed genes employed in developing improved heat-tolerant maize varieties using robust genome editing techniques, such as RNAi and CRISPR/Cas9 (El-Sappah et al., 2021; Razzaq et al., 2021; Singh et al., 2021). Integration of robust genetic engineering techniques has accelerated conventional breeding of maize by reducing the time of variety development with the application of genetic markers in the early detection of desired traits in F1 (Ahmar et al., 2020). Furthermore, fast growth in NGS has enabled high throughput sequencing of desired traits which is cost-effective, time-saving, reproducible, impossible to achieve via conventional breeding (Kulski, 2016).
In maize, several genes have been identified to develop genetically modified (GMO) or transgenic verities with improved heat tolerance (Tiwari and Yadav, 2019; Malenica et al., 2021). For example, overexpression of ZmVPP1 and OsMYB55 resulted in increased heat and drought tolerance in maize (Casaretto et al., 2016; Wang et al., 2016). Furthermore, the HSFs gene family plays a pivotal role during heat stress (Haider et al., 2021). Up to 25 HSFs have been reported in several cereal crops, and their key role is confirmed in regulating Hsp genes (Guo et al., 2008). This discovery of identifying and characterizing HSFs and their role in regulating Hsp genes has provided a fundamental basis for the development of GM maize with the highest heat stress tolerance (Ahuja et al., 2010). Furthermore, the overexpression of chloroplast localized 6-phosphogluconate dehydrogenase (6PGDH) PGD3 displayed an over-accumulation of starch in maize endosperm under heat stress improved grain size and weight, whereas, WPGD1 and WPGD2 transgenes can increase the number of kernels to mitigate losses in high nighttime temperature conditions (Ribeiro et al., 2020). In the metabolic studies of Joshi et al. (2021), a total of 5,136 genes expression were affected in response to heat stress.
Plant growth, development, and productivity are significantly affected by abiotic or biotic stresses because the plants, as sessile organisms, cannot move to favorable environments. Globally, high temperature has become a significant stressor that has accelerated the increase in the air temperature in the recent decades. Maize is a C4 crop species that belongs to the Poaceae family and is moderately sensitive to abiotic stresses, such as temperature. Maize plants are considered to be heat tolerant, but an extended duration of a temperature >35°C is deemed to be unfavorable for the development and growth of crops. In comparison, temperatures above 40°C, mainly during flowering and grain-filling season, will negatively affect the grain productivity of grain in these plants.
Heat stress may alter several physiological processes, namely membrane fluidity, net photosynthesis, respiration rate, hormone levels, osmolytes accumulation, and so on. High temperatures are related to several metabolic events at cellular and sub-cellular levels, leading to the production of ROS and oxidative stress. The anti-oxidative defense system is a prospective mechanism to protect them from ROS damage in plants. Finally, several agronomic management practices, such as the management of soil and nutrients, crop rotation, plantation rate, timing, and irrigation, are beneficial in developing heat tolerance in maize, along with advanced genomic tools. This review summarizes heat stress sensing, the induction of signaling cascade, symptoms, heat stress-related genes, molecular feature of maize response, and approaches to establishing heat-tolerant maize varieties.
Environmental factors affecting maize growth and development include rainfall, light intensity, temperature (heat and cold), relative humidity, heavy metal stress, and wind speed. Drought and heat stress have severe implications for sustainable crop yield. Therefore, it is necessary to develop maize verities having maximum tolerance against drought and heat stress with breeding and genetic engineering. Although substantial efforts had been made to develop heat-tolerant maize verities via conventional breeding, it has limitations, such as being laborious, time-consuming, and the possibility of only intra-species gene transfer. However, modern genetic approaches, such as GWAS and genotyping by sequencing, have facilitated inter-species gene transfer to develop maize verities with the highest heat tolerance. Additionally, the complementation of conventional breeding with the development of modern and robust genetic engineering techniques, such as RNAi, CRISPR/Cas9, and TILLING, has accelerated the process of variety development.
AE-S, KE-T, JL, SAR, RRM, and MA: conceptualization. AE-S: writing original draft and collecting the data. AE-S and ASE: draw the figures. AE-S, KY, SHW, MB, QH, ZAD, MMAE, MK, RRM, JL, and KE-T: review, and editing of the manuscript. AE-S, KE-T, and MA: writing final copy. All authors contributed to the article and approved the submitted version.
The authors declare that the research was conducted in the absence of any commercial or financial relationships that could be construed as a potential conflict of interest.
All claims expressed in this article are solely those of the authors and do not necessarily represent those of their affiliated organizations, or those of the publisher, the editors and the reviewers. Any product that may be evaluated in this article, or claim that may be made by its manufacturer, is not guaranteed or endorsed by the publisher.
Many thanks to Prof. Dr. Wang Ling, the President of Yibin University, for her support. At the same time, thanks to Zhou Lei and Jiang Qianwen, the International Office Team, for their continuous help to achieve a suitable environment for research. KE-T would like to thank the library at Murdoch University, Australia, for the valuable online resources and comprehensive databases.
Abou-Deif, M. H., Rashed, M. A.-S., Khalil, K. M., and Mahmoud, F. E.-S. (2019). Proteomic analysis of heat shock proteins in maize (Zea mays L.). Bull. Natl. Res. Cent. 43:199.
Ahmar, S., Gill, R. A., Jung, K.-H., Faheem, A., Qasim, M. U., Mubeen, M., et al. (2020). Conventional and molecular techniques from simple breeding to speed breeding in crop plants: recent advances and future outlook. Int. J. Mol. Sci. 21:2590. doi: 10.3390/ijms21072590
Ahmed, I., Ur Rahman, M. H., Ahmed, S., Hussain, J., Ullah, A., and Judge, J. (2018). Assessing the impact of climate variability on maize using simulation modeling under semi-arid environment of Punjab, Pakistan. Environ. Sci. Pollut. Res. 25, 28413–28430. doi: 10.1007/s11356-018-2884-3
Ahuja, I., De Vos, R. C., Bones, A. M., and Hall, R. D. (2010). Plant molecular stress responses face climate change. Trends Plant Sci. 15, 664–674. doi: 10.1016/j.tplants.2010.08.002
Alam, M. R., Nakasathien, S., Molla, M. S. H., Islam, M. A., Maniruzzaman, M., Ali, M. A., et al. (2021). Kernel water relations and kernel filling traits in maize (Zea mays L.) are influenced by water-deficit condition in a tropical environment. Front. Plant Sci. 12:717178. doi: 10.3389/fpls.2021.717178
Amara, I., Capellades, M., Ludevid, M. D., Pagès, M., and Goday, A. (2013). Enhanced water stress tolerance of transgenic maize plants over-expressing LEA Rab28 gene. J. Plant Physiol. 170, 864–873. doi: 10.1016/j.jplph.2013.01.004
Arif, Y., Singh, P., Siddiqui, H., Bajguz, A., and Hayat, S. (2020). Salinity induced physiological and biochemical changes in plants: an omic approach towards salt stress tolerance. Plant Physiol. Biochem. 156, 64–77. doi: 10.1016/j.plaphy.2020.08.042
Aruah, B. C., Uguru, M. I., and Oyiga, B. C. (2012). Genetic variability and inter-relationship among some Nigerian pumpkin accessions (Cucurbita spp.). Int. J. Plant Breed. 6, 34–41.
Atkinson, N. J., Lilley, C. J., and Urwin, P. E. (2013). Identification of genes involved in the response of Arabidopsis to simultaneous biotic and abiotic stresses. Plant Physiol. 162, 2028–2041. doi: 10.1104/pp.113.222372
Bai, S., Yu, H., Wang, B., and Li, J. (2018). Retrospective and perspective of rice breeding in China. J. Genet. Genomics 45, 603–612. doi: 10.1016/j.jgg.2018.10.002
Banerjee, A., and Roychoudhury, A. (2018). “Abiotic stress, generation of reactive oxygen species, and their consequences: an overview,” in Reactive Oxygen Species in Plants: Boon or Bane? Revisiting the Role of ROS, eds V. P. Singh, S. Singh, D. Tripathi, S. Mohan Prasad, and D. K. Chauhan (Hoboken, NJ: John Wiley & Sons Ltd), 23–50. doi: 10.1002/9781119324928.ch2
Bartels, D., and Sunkar, R. (2005). Drought and salt tolerance in plants. CRC Crit. Rev. Plant Sci. 24, 1446–1452.
Bassani, M., Neumann, P. M., and Gepstein, S. (2004). Differential expression profiles of growth-related genes in the elongation zone of maize primary roots. Plant Mol. Biol. 56, 367–380. doi: 10.1007/s11103-004-3474-y
Baus, D. (2017). Overpopulation and the Impact on the Environment. Master thesis. New York, NY: City University of New York.
Bechtold, U., Penfold, C. A., Jenkins, D. J., Legaie, R., Moore, J. D., Lawson, T., et al. (2016). Time-series transcriptomics reveals that AGAMOUS-LIKE22 affects primary metabolism and developmental processes in drought-stressed Arabidopsis. Plant Cell 28, 345–366. doi: 10.1105/tpc.15.00910
Bedard, K., Lardy, B., and Krause, K. H. (2007). NOX family NADPH oxidases: not just in mammals. Biochimie 89, 1107–1112. doi: 10.1016/j.biochi.2007.01.012
Borghi, L. (2010). Inducible gene expression systems for plants. Plant Dev. Biol. 655, 65–75. doi: 10.1007/978-1-60761-765-5_5
Bourgine, B., and Guihur, A. (2021). Heat shock signaling in land plants: from plasma membrane sensing to the transcription of small heat shock proteins. Front. Plant Sci. 12:710801. doi: 10.3389/fpls.2021.710801
Bruns, H. A. (2003). Controlling aflatoxin and fumonisin in maize by crop management. J. Toxicol. Toxin Rev. 22, 153–173. doi: 10.1081/txr-120024090
Brusamarello-Santos, L., Pacheco, F., Aljanabi, S., Monteiro, R., Cruz, L., Baura, V., et al. (2012). Differential gene expression of rice roots inoculated with the diazotroph Herbaspirillum seropedicae. Plant Soil 356, 113–125. doi: 10.1007/s11104-011-1044-z
Cacas, J.-L. (2015). “Out for a walk along the secretory pathway during programmed cell death,” in Plant Programmed Cell Death, eds A. H. Gunawardena and P. F. McCabe (Powell, WY: Springer), 123–161. doi: 10.1007/978-3-319-21033-9_6
Caine, R. S., Yin, X., Sloan, J., Harrison, E. L., Mohammed, U., Fulton, T., et al. (2019). Rice with reduced stomatal density conserves water and has improved drought tolerance under future climate conditions. New Phytol. 221, 371–384. doi: 10.1111/nph.15344
Casaretto, J. A., El-Kereamy, A., Zeng, B., Stiegelmeyer, S. M., Chen, X., Bi, Y.-M., et al. (2016). Expression of OsMYB55 in maize activates stress-responsive genes and enhances heat and drought tolerance. BMC Genomics 17:312. doi: 10.1186/s12864-016-2659-5
Catalá, A. (2009). Lipid peroxidation of membrane phospholipids generates hydroxy-alkenals and oxidized phospholipids active in physiological and/or pathological conditions. Chem. Phys. Lipids 157, 1–11. doi: 10.1016/j.chemphyslip.2008.09.004
Cerrudo, D., Cao, S., Yuan, Y., Martinez, C., Suarez, E. A., Babu, R., et al. (2018). Genomic selection outperforms marker assisted selection for grain yield and physiological traits in a maize doubled haploid population across water treatments. Front. Plant Sci. 9:366. doi: 10.3389/fpls.2018.00366
Chao, L., Zou, J.-X., Peng, S., Peng, Y., Yuan, S.-F., Xia, W., et al. (2016). causes and impacts for heat stress in spring maize during grain filling in the North China plain â a review. J. Integr. Agric. 15, 2677–2687. doi: 10.1016/s2095-3119(16)61409-0
Chapman, J. M., Muhlemann, J. K., Gayomba, S. R., and Muday, G. K. (2019). RBOH-dependent ROS synthesis and ROS scavenging by plant specialized metabolites to modulate plant development and stress responses. Chem. Res. Toxicol. 32, 370–396. doi: 10.1021/acs.chemrestox.9b00028
Chauhan, H., Khurana, N., Agarwal, P., Khurana, J. P., and Khurana, P. (2013). A seed preferential heat shock transcription factor from wheat provides abiotic stress tolerance and yield enhancement in transgenic Arabidopsis under heat stress environment. PLoS One 8:e79577. doi: 10.1371/journal.pone.0079577
Cheikh, N., and Jones, R. J. (1994). Disruption of maize kernel growth and development by heat stress (role of cytokinin/abscisic acid balance). Plant Physiol. 106, 45–51. doi: 10.1104/pp.106.1.45
Chen, H., Hwang, J. E., Lim, C. J., Kim, D. Y., Lee, S. Y., and Lim, C. O. (2010). Arabidopsis DREB2C functions as a transcriptional activator of HsfA3 during the heat stress response. Biochem. Biophys. Res. Commun. 401, 238–244. doi: 10.1016/j.bbrc.2010.09.038
Chen, K., Ali, S., Chen, Y., Sohail, A., Jan, A., and Fahad, S. (2018). Effect of ridge-covering mulching materials on hormonal changes, antioxidative enzyme activities and production of maize in semi-arid regions of China. Agric. Water Manag. 204, 281–291. doi: 10.1016/j.agwat.2018.03.023
Chen, Y., Cao, Y., Wang, L., Li, L., Yang, J., and Zou, M. (2018). Identification of MYB transcription factor genes and their expression during abiotic stresses in maize. Biol. Plant. 62, 222–230. doi: 10.1007/s10535-017-0756-1
Chung, K. P., Zeng, Y., Li, Y., Ji, C., Xia, Y., and Jiang, L. (2018). Signal motif-dependent ER export of the Qc-SNARE BET12 interacts with MEMB12 and affects PR1 trafficking in Arabidopsis. J. Cell Sci. 131:jcs202838. doi: 10.1242/jcs.202838
Cimini, S., Gualtieri, C., Macovei, A., Balestrazzi, A., De Gara, L., and Locato, V. (2019). Redox balance-DDR-miRNA triangle: relevance in genome stability and stress responses in plants. Front. Plant Sci. 10:989. doi: 10.3389/fpls.2019.00989
Colasanti, J., and Muszynski, M. (2009). “The maize floral transition,” in Handbook of Maize: its Biology, Vol. 1, eds S. C. Hake and J. L. Bennetzen (New York, NY: Springer Science), 41–55. doi: 10.1111/nph.16772
Crafts-Brandner, S. J., and Salvucci, M. E. (2002). Sensitivity of photosynthesis in a C4 plant, maize, to heat stress. Plant Physiol. 129, 1773–1780. doi: 10.1104/pp.002170
Czarnocka, W., and Karpińskiski, S. (2018). Friend or foe? Reactive oxygen species production, scavenging and signaling in plant response to environmental stresses. Free Radic. Biol. Med. 122, 4–20. doi: 10.1016/j.freeradbiomed.2018.01.011
Dai, Z., Ku, M. S., and Edwards, G. E. (1993). C4 photosynthesis (the CO2-concentrating mechanism and photorespiration). Plant Physiol. 103, 83–90. doi: 10.1104/pp.103.1.83
Dar, Z. A., Dar, S. A., Khan, J. A., Lone, A. A., Langyan, S., Lone, B. A., et al. (2021). Identification for surrogate drought tolerance in maize inbred lines utilizing high-throughput phenomics approach. PLoS One 16:e0254318. doi: 10.1371/journal.pone.0254318
Daryanto, S., Wang, L., and Jacinthe, P.-A. (2016). Global synthesis of drought effects on maize and wheat production. PLoS One 11:e0156362. doi: 10.1371/journal.pone.0156362
Dawood, M., Moursi, Y., Amro, A., Baenziger, P., and Sallam, A. (2020). Investigation of heat-induced changes in the grain yield and grains metabolites, with molecular insights on the candidate genes in barley. Agronomy 10:1730. doi: 10.3390/agronomy10111730
Dawson, T. P., Perryman, A. H., and Osborne, T. M. (2016). Modelling impacts of climate change on global food security. Clim. Change 134, 429–440. doi: 10.1007/s10584-014-1277-y
Demidchik, V. (2015). Mechanisms of oxidative stress in plants: from classical chemistry to cell biology. Environ. Exp. Bot. 109, 212–228. doi: 10.1016/j.envexpbot.2014.06.021
Dhaubhadel, S., Chaudhary, S., Dobinson, K. F., and Krishna, P. (1999). Treatment with 24-epibrassinolide, a brassinosteroid, increases the basic thermotolerance of Brassica napus and tomato seedlings. Plant Mol. Biol. 40, 333–342. doi: 10.1023/a:1006283015582
Dias, M., and Brüggemann, W. (2010). Limitations of photosynthesis in Phaseolus vulgaris under drought stress: gas exchange, chlorophyll fluorescence and Calvin cycle enzymes. Photosynthetica 48, 96–102. doi: 10.1007/s11099-010-0013-8
Djanaguiraman, M., Prasad, P. V., and Seppanen, M. (2010). Selenium protects sorghum leaves from oxidative damage under high temperature stress by enhancing antioxidant defense system. Plant Physiol. Biochem. 48, 999–1007. doi: 10.1016/j.plaphy.2010.09.009
Dolatabadian, A., Sanavy, S. A. M. M., and Asilan, K. S. (2010). Effect of ascorbic acid foliar application on yield, yield component and several morphological traits of grain corn under water deficit stress conditions. Not. Sci. Biol. 2, 45–50. doi: 10.15835/nsb234717
Dong, X., Xu, W., Zhang, Y., and Leskovar, D. I. (2016). Effect of irrigation timing on root zone soil temperature, root growth and grain yield and chemical composition in corn. Agronomy 6:34. doi: 10.3390/agronomy6020034
Dordas, C. (2009). Dry matter, nitrogen and phosphorus accumulation, partitioning and remobilization as affected by N and P fertilization and source–sink relations. Eur. J. Agron. 30, 129–139. doi: 10.1016/j.eja.2008.09.001
Du, H., Feng, B.-R., Yang, S.-S., Huang, Y.-B., and Tang, Y.-X. (2012). The R2R3-MYB transcription factor gene family in maize. PLoS One 7:e37463. doi: 10.1371/journal.pone.0037463
Duszyn, M., świeżawska, B., Szmidt-Jaworska, A., and Jaworski, K. (2019). Cyclic nucleotide gated channels (CNGCs) in plant signalling—current knowledge and perspectives. J. Plant Physiol. 241:153035. doi: 10.1016/j.jplph.2019.153035
Edreira, J. I. R., Mayer, L. I., and Otegui, M. E. (2014). Heat stress in temperate and tropical maize hybrids: kernel growth, water relations and assimilate availability for grain filling. Field Crops Res. 166, 162–172. doi: 10.1016/j.fcr.2014.06.018
Efeoǧlu, B. (2009). Heat shock proteins and heat shock response in plants. Gazi Univ. J. Sci. 22, 67–75.
Elferjani, R., and Soolanayakanahally, R. (2018). Canola responses to drought, heat, and combined stress: shared and specific effects on carbon assimilation, seed yield, and oil composition. Front. Plant Sci. 9:1224. doi: 10.3389/fpls.2018.01224
El-Sappah, A., Shawky, A., Sayed-Ahmad, M., and Youssef, M. (2012). Nile tilapia as bio indicator to estimate the contamination of water using SDS-PAGE and RAPDPCR techniques. Egypt. J. Genet. Cytol. 41, 209–227. doi: 10.21608/ejgc.2012.10536
El-Sappah, A. H., Mm, H. I, El-Awady, H., Yan, S., Qi, S., Liu, J., et al. (2019). Tomato natural resistance genes in controlling the root-knot nematode. Genes 10:925. doi: 10.3390/genes10110925
El-Sappah, A. H., and Rather, S. A. (eds). (2022). “Genomics approaches to study abiotic stress tolerance in plants,” in Plant Abiotic Stress Physiology, Vol. 2, (Burlington: Apple Academic Press), 25. doi: 10.1201/9781003180579-2
El-Sappah, A. H., Shawky, A., Sayed-Ahmad, M. S., and Youssef, M. (2017). Estimation of heat shock protein 70 (hsp 70) gene expression in nile tilapia (Oreochromis niloticus) using quantitative real-time PCR. Zagazig J. Agric. Res. 44, 1003–1015. doi: 10.21608/zjar.2017.52300
El-Sappah, A. H., Yan, K., Huang, Q., Islam, M. M., Li, Q., Wang, Y., et al. (2021). Comprehensive mechanism of gene silencing and its role in plant growth and development. Front. Plant Sci. 12:705249. doi: 10.3389/fpls.2021.705249
El-Sharkawy, M. A. (2007). Physiological characteristics of cassava tolerance to prolonged drought in the tropics: implications for breeding cultivars adapted to seasonally dry and semiarid environments. Braz. J. Plant Physiol. 19, 257–286. doi: 10.1590/s1677-04202007000400003
El-Shershaby, A., Ullrich, S., Simm, S., Scharf, K.-D., Schleiff, E., and Fragkostefanakis, S. (2019). Functional diversification of tomato HsfA1 factors is based on DNA binding domain properties. Gene 714:143985. doi: 10.1016/j.gene.2019.143985
Erb, M., and Kliebenstein, D. J. (2020). Plant secondary metabolites as defenses, regulators, and primary metabolites: the blurred functional trichotomy. Plant Physiol. 184, 39–52. doi: 10.1104/pp.20.00433
Essemine, J., Govindachary, S., Ammar, S., Bouzid, S., and Carpentier, R. (2012). Enhanced sensitivity of the photosynthetic apparatus to heat stress in digalactosyl-diacylglycerol deficient Arabidopsis. Environ. Exp. Bot. 80, 16–26. doi: 10.1016/j.envexpbot.2011.12.022
Eyidogan, F., Oz, M., Yucel, M., and Oktem, H. (2012). “Signal transduction of phytohormones under abiotic stresses,” in Phytohormones and Abiotic Stress Tolerance in Plants, eds N. A. Khan, R. Nazar, N. Iqbal, and N. A. Anjum (Berlin: Springer), 1–48. doi: 10.1007/978-3-642-25829-9_1
Fahad, S., Bajwa, A. A., Nazir, U., Anjum, S. A., Farooq, A., Zohaib, A., et al. (2017). Crop production under drought and heat stress: plant responses and management options. Front. Plant Sci. 8:1147. doi: 10.3389/fpls.2017.01147
Faostat, F. (2017). Available Online at: http://www.fao.org/faostat/en/#data.QC [accessed January 2018].
Faostat, F. (2019). Food and Agriculture Organization of the United Nations-Statistic Division. Available Online at: https://www.fao.org/faostat/en/#data.QC
Farshadfar, E., Romena, H., and Safari, H. (2013). Evaluation of variability and genetic parameters in agro-physiological traits of wheat under rain-fed condition. Int. J. Agric. Crop Sci. 5, 1015–1021.
Finka, A., and Goloubinoff, P. (2014). The CNGCb and CNGCd genes from Physcomitrella patens moss encode for thermosensory calcium channels responding to fluidity changes in the plasma membrane. Cell Stress Chaperones 19, 83–90. doi: 10.1007/s12192-013-0436-9
Foyer, C. H., and Shigeoka, S. (2011). Understanding oxidative stress and antioxidant functions to enhance photosynthesis. Plant Physiol. 155, 93–100. doi: 10.1104/pp.110.166181
Fragkostefanakis, S., Mesihovic, A., Hu, Y., and Schleiff, E. (2016). Unfolded protein response in pollen development and heat stress tolerance. Plant Reprod. 29, 81–91. doi: 10.1007/s00497-016-0276-8
Frederickson Matika, D. E., and Loake, G. J. (2014). Redox regulation in plant immune function. Antioxid. Redox Signal. 21, 1373–1388. doi: 10.1089/ars.2013.5679
Frey, F. P., Presterl, T., Lecoq, P., Orlik, A., and Stich, B. (2016). First steps to understand heat tolerance of temperate maize at adult stage: identification of QTL across multiple environments with connected segregating populations. Theor. Appl. Genet. 129, 945–961. doi: 10.1007/s00122-016-2674-6
Frey, F. P., Urbany, C., Hüttel, B., Reinhardt, R., and Stich, B. (2015). Genome-wide expression profiling and phenotypic evaluation of European maize inbreds at seedling stage in response to heat stress. BMC Genomics 16:123. doi: 10.1186/s12864-015-1282-1
Frova, C., Caffulli, A., and Pallavera, E. (1998). Mapping quantitative trait loci for tolerance to abiotic stresses in maize. J. Exp. Zool. 282, 164–170. doi: 10.1002/(sici)1097-010x(199809/10)282:1/2<164::aid-jez18>3.0.co;2-u
Frova, C., and Sari-Gorla, M. (1994). Quantitative trait loci (QTLs) for pollen thermotolerance detected in maize. Mol. Gen. Genet. 245, 424–430. doi: 10.1007/BF00302254
Fu, J., Momcilovic, I., Prasad, P., Josipovic, S., and Ludwig, E. (2012). “Molecular bases and improvement of heat tolerance in crop plants,” in Heat Stress: Causes, Prevention and Treatments, eds S. Josipovic and E. Ludwig (Hauppauge, NY: Nova Science Publishers), 185–214.
Gao, F., Han, X., Wu, J., Zheng, S., Shang, Z., Sun, D., et al. (2012). A heat-activated calcium-permeable channel–Arabidopsis cyclic nucleotide-gated ion channel 6–is involved in heat shock responses. Plant J. 70, 1056–1069. doi: 10.1111/j.1365-313X.2012.04969.x
Gao, H., Brandizzi, F., Benning, C., and Larkin, R. M. (2008). A membrane-tethered transcription factor defines a branch of the heat stress response in Arabidopsis thaliana. Proc. Natl. Acad. Sci. U.S.A. 105, 16398–16403. doi: 10.1073/pnas.0808463105
Gao, J., Wang, S., Zhou, Z., Wang, S., Dong, C., Mu, C., et al. (2019). Linkage mapping and genome-wide association reveal candidate genes conferring thermotolerance of seed-set in maize. J. Exp. Bot. 70, 4849–4864. doi: 10.1093/jxb/erz171
Gao, X., Cox, K. L. Jr., and He, P. (2014). Functions of calcium-dependent protein kinases in plant innate immunity. Plants 3, 160–176. doi: 10.3390/plants3010160
Gardeström, P., and Igamberdiev, A. U. (2016). The origin of cytosolic ATP in photosynthetic cells. Physiol. Plant. 157, 367–379. doi: 10.1111/ppl.12455
Gayral, M., Arias Gaguancela, O., Vasquez, E., Herath, V., Flores, F. J., Dickman, M. B., et al. (2020). Multiple ER-to-nucleus stress signaling pathways are activated during Plantago asiatica mosaic virus and Turnip mosaic virus infection in Arabidopsis thaliana. Plant J. 103, 1233–1245. doi: 10.1111/tpj.14798
Gedil, M., and Menkir, A. (2019). An integrated molecular and conventional breeding scheme for enhancing genetic gain in maize in Africa. Front. Plant Sci. 10:1430. doi: 10.3389/fpls.2019.01430
Gong, F., Wu, X., Zhang, H., Chen, Y., and Wang, W. (2015). Making better maize plants for sustainable grain production in a changing climate. Front. Plant Sci. 6:835. doi: 10.3389/fpls.2015.00835
Gong, M., Li, Y.-J., Dai, X., Tian, M., and Li, Z.-G. (1997). Involvement of calcium and calmodulin in the acquisition of heat-shock induced thermotolerance in maize seedlings. J. Plant Physiol. 150, 615–621. doi: 10.1016/s0176-1617(97)80328-8
Gourdji, S. M., Sibley, A. M., and Lobell, D. B. (2013). Global crop exposure to critical high temperatures in the reproductive period: historical trends and future projections. Environ. Res. Lett. 8:024041. doi: 10.1088/1748-9326/8/2/024041
Guo, H., Li, S., Kang, S., Du, T., Tong, L., and Ding, R. (2019). Annual ecosystem respiration of maize was primarily driven by crop growth and soil water conditions. Agric. Ecosyst. Environ. 272, 254–265. doi: 10.1016/j.agee.2018.11.026
Guo, J., Wu, J., Ji, Q., Wang, C., Luo, L., Yuan, Y., et al. (2008). Genome-wide analysis of heat shock transcription factor families in rice and Arabidopsis. J. Genet. Genomics 35, 105–118. doi: 10.1016/S1673-8527(08)60016-8
Gurley, W. B. (2000). HSP101: a key component for the acquisition of thermotolerance in plants. Plant Cell 12, 457–460. doi: 10.1105/tpc.12.4.457
Haider, S., Rehman, S., Ahmad, Y., Raza, A., Tabassum, J., Javed, T., et al. (2021). In silico characterization and expression profiles of heat shock transcription factors (HSFs) in maize (Zea mays L.). Agronomy 11:2335. doi: 10.3390/agronomy11112335
Hao, L., and Qiao, X. (2018). Genome-wide identification and analysis of the CNGC gene family in maize. PeerJ 6:e5816. doi: 10.7717/peerj.5816
Hasanuzzaman, M., Bhuyan, M., Zulfiqar, F., Raza, A., Mohsin, S. M., Mahmud, J. A., et al. (2020). Reactive oxygen species and antioxidant defense in plants under abiotic stress: revisiting the crucial role of a universal defense regulator. Antioxidants 9:681. doi: 10.3390/antiox9080681
Hashimoto, K., and Kudla, J. (2011). Calcium decoding mechanisms in plants. Biochimie 93, 2054–2059. doi: 10.1016/j.biochi.2011.05.019
Haswell, E. S., and Verslues, P. E. (2015). The ongoing search for the molecular basis of plant osmosensing. J. Gen. Physiol. 145, 389–394. doi: 10.1085/jgp.201411295
Hayes, S., Schachtschabel, J., Mishkind, M., Munnik, T., and Arisz, S. A. (2021). Hot topic: thermosensing in plants. Plant Cell Environ. 44, 2018–2033. doi: 10.1111/pce.13979
He, F., Gao, C., Guo, G., Liu, J., Gao, Y., Pan, R., et al. (2019). Maize annexin genes ZmANN33 and ZmANN35 encode proteins that function in cell membrane recovery during seed germination. J. Exp. Bot. 70, 1183–1195. doi: 10.1093/jxb/ery452
He, Y.-Y., and Häder, D.-P. (2002). UV-B-induced formation of reactive oxygen species and oxidative damage of the cyanobacterium Anabaena sp.: protective effects of ascorbic acid and N-acetyl-L-cysteine. J. Photochem. Photobiol. B Biol. 66, 115–124. doi: 10.1016/s1011-1344(02)00231-2
Hoegh-Guldberg, O., Jacob, D., Taylor, M., Bolaños, T. G., Bindi, M., Brown, S., et al. (2019). The human imperative of stabilizing global climate change at 1.5°C. Science 365:eaaw6974. doi: 10.1126/science.aaw6974
Horváth, I., Glatz, A., Nakamoto, H., Mishkind, M. L., Munnik, T., Saidi, Y., et al. (2012). Heat shock response in photosynthetic organisms: membrane and lipid connections. Prog. Lipid Res. 51, 208–220. doi: 10.1016/j.plipres.2012.02.002
Hossain, M. A., Bhattacharjee, S., Armin, S. M., Qian, P., Xin, W., Li, H. Y., et al. (2015). Hydrogen peroxide priming modulates abiotic oxidative stress tolerance: insights from ROS detoxification and scavenging. Front. Plant Sci. 6:420. doi: 10.3389/fpls.2015.00420
Houghton, J. T., Ding, Y., Griggs, D. J., Noguer, M., Van Der Linden, P. J., Dai, X., et al. (2001). Climate Change 2001: the Scientific Basis: Contribution of Working Group I to the Third Assessment Report of the Intergovernmental Panel on Climate Change. Cambridge: Cambridge university press.
Howell, S. H. (2013). Endoplasmic reticulum stress responses in plants. Annu. Rev. Plant Biol. 64, 477–499. doi: 10.1146/annurev-arplant-050312-120053
Hu, X., Huang, Y., Sun, W., and Yu, L. (2017). Shifts in cultivar and planting date have regulated rice growth duration under climate warming in China since the early 1980s. Agric. For. Meteorol. 247, 34–41. doi: 10.1016/j.agrformet.2017.07.014
Hu, X., Jiang, M., Zhang, J., Zhang, A., Lin, F., and Tan, M. (2007). Calcium/calmodulin is required for abscisic acid-induced antioxidant defense and functions both upstream and downstream of H2O2 production in leaves of maize plants. New Phytol. 173, 27–38. doi: 10.1111/j.1469-8137.2006.01888.x
Hu, X., Yang, Y., Gong, F., Zhang, D., Zhang, L., Wu, L., et al. (2015). Protein sHSP26 improves chloroplast performance under heat stress by interacting with specific chloroplast proteins in maize (Zea mays). J. Proteom. 115, 81–92. doi: 10.1016/j.jprot.2014.12.009
Huang, H.-W., Zeng, X., Rhim, T., Ron, D., and Ryoo, H. D. (2017). The requirement of IRE1 and XBP1 in resolving physiological stress during Drosophila development. J. Cell Sci. 130, 3040–3049. doi: 10.1242/jcs.203612
Huang, J., Chen, F., Del Casino, C., Autino, A., Shen, M., Yuan, S., et al. (2006). An ankyrin repeat-containing protein, characterized as a ubiquitin ligase, is closely associated with membrane-enclosed organelles and required for pollen germination and pollen tube growth in lily. Plant Physiol. 140, 1374–1383. doi: 10.1104/pp.105.074922
Huang, Y.-C., Niu, C.-Y., Yang, C.-R., and Jinn, T.-L. (2016). The heat stress factor HSFA6b connects ABA signaling and ABA-mediated heat responses. Plant Physiol. 172, 1182–1199. doi: 10.1104/pp.16.00860
Ihsan, M. Z., Daur, I., Alghabari, F., Alzamanan, S., Rizwan, S., Ahmad, M., et al. (2019). Heat stress and plant development: role of sulphur metabolites and management strategies. Acta Agric. Scand. B Soil Plant Sci. 69, 332–342. doi: 10.1080/09064710.2019.1569715
Iurlaro, R., and Muñoz-Pinedo, C. (2016). Cell death induced by endoplasmic reticulum stress. FEBS J. 283, 2640–2652.
Jagadish, S., Bahuguna, R. N., Djanaguiraman, M., Gamuyao, R., Prasad, P., and Craufurd, P. Q. (2016). Implications of high temperature and elevated CO2 on flowering time in plants. Front. Plant Sci. 7:913. doi: 10.3389/fpls.2016.00913
Jagtap, A. B., Vikal, Y., and Johal, G. S. (2020). Genome-wide development and validation of cost-effective KASP marker assays for genetic dissection of heat stress tolerance in maize. Int. J. Mol. Sci. 21:7386. doi: 10.3390/ijms21197386
Jammes, F., Hu, H. C., Villiers, F., Bouten, R., and Kwak, J. M. (2011). Calcium-permeable channels in plant cells. FEBS J. 278, 4262–4276. doi: 10.1111/j.1742-4658.2011.08369.x
Jha, A. K., Chakraborty, S., Kumari, K., and Bauddh, K. (2020). “Ecological consequences of genetically modified crops on soil biodiversity,” in Ecological and Practical Applications for Sustainable Agriculture, eds K. Bauddh, S. Kumar, R. Singh, and J. Korstad (Singapore: Springer), 89–106. doi: 10.1007/978-981-15-3372-3_5
Jia, Z., Lian, Y., Zhu, Y., He, J., Cao, Z., and Wang, G. (2009). Cloning and characterization of a putative transcription factor induced by abiotic stress in Zea mays. Afr. J. Biotechnol. 8, 6764–6771.
Jiang, L., Hu, W., Qian, Y., Ren, Q., and Zhang, J. (2021). Genome-wide identification, classification and expression analysis of the Hsf and Hsp70 gene families in maize. Gene 770:145348. doi: 10.1016/j.gene.2020.145348
Jiang, C., Zu, C., Lu, D., Zheng, Q., Shen, J., Wang, H., et al. (2017). Effect of exogenous selenium supply on photosynthesis, Na+ accumulation and antioxidative capacity of maize (Zea mays L.) under salinity stress. Sci. Rep. 7:42039. doi: 10.1038/srep42039
Joshi, J., Hasnain, G., Logue, T., Lynch, M., Wu, S., Guan, J.-C., et al. (2021). A core metabolome response of maize leaves subjected to long-duration abiotic stresses. Metabolites 11:797. doi: 10.3390/metabo11110797
Kaplan, F., Kopka, J., Haskell, D. W., Zhao, W., Schiller, K. C., Gatzke, N., et al. (2004). Exploring the temperature-stress metabolome of Arabidopsis. Plant Physiol. 136, 4159–4168. doi: 10.1104/pp.104.052142
Kaspar, T., and Bland, W. L. (1992). Soil temperature and root growth. Soil Sci. 154, 290–299. doi: 10.1097/00010694-199210000-00005
Keeling, P., Banisadr, R., Barone, L., Wasserman, B., and Singletary, G. (1994). Effect of temperature on enzymes in the pathway of starch biosynthesis in developing wheat and maize grain. Funct. Plant Biol. 21, 807–827. doi: 10.1071/pp9940807
Ketehouli, T., Idrice Carther, K. F., Noman, M., Wang, F.-W., Li, X.-W., and Li, H.-Y. (2019). Adaptation of plants to salt stress: characterization of Na+ and K+ transporters and role of CBL gene family in regulating salt stress response. Agronomy 9:687. doi: 10.3390/agronomy9110687
Khaeim, H., Kende, Z., Jolánkai, M., Kovács, G. P., Gyuricza, C., and Tarnawa, Á. (2022). Impact of temperature and water on seed germination and seedling growth of maize (Zea mays L.). Agronomy 12:397. doi: 10.3390/agronomy12020397
Khajuria, P., Singh, A. K., and Singh, R. (2016). Identification of heat stress tolerant genotypes in bread wheat. Electron. J. Plant Breed. 7, 1446–1452.
Khakwani, A. A., Dennett, M. D., Munir, M., and Baloch, M. S. (2012). Wheat yield response to physiological limitations under water stress condition. J. Anim. Plant Sci. 22, 773–780.
Khan, A., Ali, M., Khattak, A. M., Gai, W.-X., Zhang, H.-X., Wei, A.-M., et al. (2019). Heat shock proteins: dynamic biomolecules to counter plant biotic and abiotic stresses. Int. J. Mol. Sci. 20:5321. doi: 10.3390/ijms20215321
Khan, M. I. R., Fatma, M., Per, T. S., Anjum, N. A., and Khan, N. A. (2015). Salicylic acid-induced abiotic stress tolerance and underlying mechanisms in plants. Front. Plant Sci. 6:462. doi: 10.3389/fpls.2015.00462
Kim, J.-M., To, T. K., Ishida, J., Matsui, A., Kimura, H., and Seki, M. (2012). Transition of chromatin status during the process of recovery from drought stress in Arabidopsis thaliana. Plant Cell Physiol. 53, 847–856. doi: 10.1093/pcp/pcs053
Kimotho, R. N., Baillo, E. H., and Zhang, Z. (2019). Transcription factors involved in abiotic stress responses in maize (Zea mays L.) and their roles in enhanced productivity in the post genomics era. PeerJ 7:e7211. doi: 10.7717/peerj.7211
Klein, R. D., Chidawanyika, T., Tims, H. S., Meulia, T., Bouchard, R. A., and Pett, V. B. (2014). Chaperone function of two small heat shock proteins from maize. Plant Sci. 221-222, 48–58. doi: 10.1016/j.plantsci.2014.01.012
Kong, L., Wang, F., Si, J., Feng, B., Zhang, B., Li, S., et al. (2013). Increasing in ROS levels and callose deposition in peduncle vascular bundles of wheat (Triticum aestivum L.) grown under nitrogen deficiency. J. Plant Interact. 8, 109–116. doi: 10.1080/17429145.2012.712723
Kørner, C. J., Du, X., Vollmer, M. E., and Pajerowska-Mukhtar, K. M. (2015). Endoplasmic reticulum stress signaling in plant immunity—at the crossroad of life and death. Int. J. Mol. Sci. 16, 26582–26598. doi: 10.3390/ijms161125964
Kraus, K., Hnilickova, H., Pecka, J., Lhotska, M., Bezdickova, A., Martinek, P., et al. (2022). The effect of the application of stimulants on the photosynthetic apparatus and the yield of winter wheat. Agronomy 12:78. doi: 10.3390/agronomy12010078
Kulski, J. K. (ed.) (2016). “Next-generation sequencing—an overview of the history, tools, and “Omic” applications,” in Next Generation Sequencing–Advances, Applications and Challenges, (London: InTech), 3–60.
Kumar, A., and Kaushik, P. (2021). Heat stress and its impact on plant function: an update. Preprints 2021:2021080489.
Kumar, K., Singh, I., Aggarwal, C., Tewari, I., Jha, A. K., Yadava, P., et al. (2019). Expression profiling of heat shock protein genes in two contrasting maize inbred lines. Int. J. Curr. Microbiol. Appl. Sci. 8, 347–358. doi: 10.20546/ijcmas.2019.806.039
Kumar, M., Kumar Patel, M., Kumar, N., Bajpai, A. B., and Siddique, K. H. M. (2021). Metabolomics and molecular approaches reveal drought stress tolerance in plants. Int. J. Mol. Sci. 22:9108. doi: 10.3390/ijms22179108
Kumari, S., Sabharwal, V. P., Kushwaha, H. R., Sopory, S. K., Singla-Pareek, S. L., and Pareek, A. (2009). Transcriptome map for seedling stage specific salinity stress response indicates a specific set of genes as candidate for saline tolerance in Oryza sativa L. Funct. Integr. Genomics 9, 109–123. doi: 10.1007/s10142-008-0088-5
Lafarge, T., Bueno, C., Frouin, J., Jacquin, L., Courtois, B., and Ahmadi, N. (2017). Genome-wide association analysis for heat tolerance at flowering detected a large set of genes involved in adaptation to thermal and other stresses. PLoS One 12:e0171254. doi: 10.1371/journal.pone.0171254
Le Nguyen, K., Grondin, A., Courtois, B., and Gantet, P. (2019). Next-generation sequencing accelerates crop gene discovery. Trends Plant Sci. 24, 263–274. doi: 10.1016/j.tplants.2018.11.008
Leng, P., and Zhao, J. (2020). Transcription factors as molecular switches to regulate drought adaptation in maize. Theor. Appl. Genet. 133, 1455–1465. doi: 10.1007/s00122-019-03494-y
Li, B., Gao, K., Ren, H., and Tang, W. (2018). Molecular mechanisms governing plant responses to high temperatures. J. Integr. Plant Biol. 60, 757–779. doi: 10.1111/jipb.12701
Li, Z., Srivastava, R., Tang, J., Zheng, Z., and Howell, S. H. (2018). Cis-effects condition the induction of a major unfolded protein response factor, ZmbZIP60, in response to heat stress in maize. Front. Plant Sci. 9:833. doi: 10.3389/fpls.2018.00833
Li, H.-C., Zhang, H.-N., Li, G.-L., Liu, Z.-H., Zhang, Y.-M., Zhang, H.-M., et al. (2015). Expression of maize heat shock transcription factor gene ZmHsf06 enhances the thermotolerance and drought-stress tolerance of transgenic Arabidopsis. Funct. Plant Biol. 42, 1080–1091. doi: 10.1071/FP15080
Li, Z. G., Xie, L. R., and Li, X. J. (2015). Hydrogen sulfide acts as a downstream signal molecule in salicylic acid-induced heat tolerance in maize (Zea mays L.) seedlings. J. Plant Physiol. 177, 121–127. doi: 10.1016/j.jplph.2014.12.018
Li, J., Zhang, L., Elbaiomy, R. G., Chen, L., Wang, Z., Jiao, J., et al. (2022). Evolution analysis of FRIZZY PANICLE (FZP) orthologs explored the mutations in DNA coding sequences in the grass family (Poaceae). PeerJ 10:e12880. doi: 10.7717/peerj.12880
Li, J., Zhang, L., Yuan, Y., Wang, Q., Elbaiomy, R., Zhou, W., et al. (2021). In silico functional prediction and expression analysis of C2H2 zinc-finger family transcription factor revealed regulatory role of ZmZFP126 in maize growth. Front. Genet. 12:770427. doi: 10.3389/fgene.2021.770427
Li, N., Euring, D., Cha, J. Y., Lin, Z., Lu, M., Huang, L.-J., et al. (2021). Plant hormone-mediated regulation of heat tolerance in response to global climate change. Front. Plant Sci. 11:627969. doi: 10.3389/fpls.2020.627969
Li, Y. T., Xu, W. W., Ren, B. Z., Zhao, B., Zhang, J., Liu, P., et al. (2020). High temperature reduces photosynthesis in maize leaves by damaging chloroplast ultrastructure and photosystem II. J. Agron. Crop Sci. 206, 548–564. doi: 10.1111/jac.12401
Li, Z., Tang, J., Srivastava, R., Bassham, D. C., and Howell, S. H. (2020). The transcription factor bZIP60 links the unfolded protein response to the heat stress response in maize. Plant Cell 32, 3559–3575. doi: 10.1105/tpc.20.00260
Li, Z., and Howell, S. H. (2021). Heat stress responses and thermotolerance in maize. Int. J. Mol. Sci. 22:948. doi: 10.3390/ijms22020948
Li, Z. G. (2015). Synergistic effect of antioxidant system and osmolyte in hydrogen sulfide and salicylic acid crosstalk-induced heat tolerance in maize (Zea mays L.) seedlings. Plant Signal. Behav. 10:e1051278. doi: 10.1080/15592324.2015.1051278
Li, Z.-G., Ye, X.-Y., and Qiu, X.-M. (2019). Glutamate signaling enhances the heat tolerance of maize seedlings by plant glutamate receptor-like channels-mediated calcium signaling. Protoplasma 256, 1165–1169. doi: 10.1007/s00709-019-01351-9
Lieu, E. L., Kelekar, N., Bhalla, P., and Kim, J. (2021). Fructose and mannose in inborn errors of metabolism and cancer. Metabolites 11:479. doi: 10.3390/metabo11080479
Lima, R. B., Dos Santos, T. B., Vieira, L. G. E., Ferrarese, M. D. L. L., Ferrarese-Filho, O., Donatti, L., et al. (2013). Heat stress causes alterations in the cell-wall polymers and anatomy of coffee leaves (Coffea arabica L.). Carbohydr. Polym. 93, 135–143. doi: 10.1016/j.carbpol.2012.05.015
Lin, F., Ding, H., Wang, J., Zhang, H., Zhang, A., Zhang, Y., et al. (2009). Positive feedback regulation of maize NADPH oxidase by mitogen-activated protein kinase cascade in abscisic acid signalling. J. Exp. Bot. 60, 3221–3238. doi: 10.1093/jxb/erp157
Lin, F., Wani, S. H., Collins, P. J., Wen, Z., Li, W., Zhang, N., et al. (2020). QTL mapping and GWAS for identification of loci conferring partial resistance to Pythium sylvaticum in soybean(Glycine max (L.) Merr). Mol. Breed. 40:54.
Lin, Y.-X., Jiang, H.-Y., Chu, Z.-X., Tang, X.-L., Zhu, S.-W., and Cheng, B.-J. (2011). Genome-wide identification, classification and analysis of heat shock transcription factor family in maize. BMC Genomics 12:76. doi: 10.1186/1471-2164-12-76
Liu, J., Niu, Y., Zhang, J., Zhou, Y., Ma, Z., and Huang, X. (2018). Ca2+ channels and Ca2+ signals involved in abiotic stress responses in plant cells: recent advances. Plant Cell Tissue Organ Cult. 132, 413–424. doi: 10.1007/s11240-017-1350-0
Liu, J.-X., Srivastava, R., Che, P., and Howell, S. H. (2007). An endoplasmic reticulum stress response in Arabidopsis is mediated by proteolytic processing and nuclear relocation of a membrane-associated transcription factor, bZIP28. Plant Cell 19, 4111–4119. doi: 10.1105/tpc.106.050021
Liu, L., Hao, Z., Weng, J., Li, M., Zhang, D., Bai, L., et al. (2012). Identification of drought-responsive genes by cDNA-amplified fragment length polymorphism in maize. Ann. Appl. Biol. 161, 203–213. doi: 10.1111/j.1744-7348.2012.00565.x
Liu, Z., Hubbard, K. G., Lin, X., and Yang, X. (2013). Negative effects of climate warming on maize yield are reversed by the changing of sowing date and cultivar selection in Northeast China. Glob. Change Biol. 19, 3481–3492. doi: 10.1111/gcb.12324
Lobell, D. B., Bänziger, M., Magorokosho, C., and Vivek, B. (2011). Nonlinear heat effects on African maize as evidenced by historical yield trials. Nat. Clim. Change 1, 42–45. doi: 10.1038/nclimate1043
Longmei, N., Gill, G. K., Zaidi, P. H., Kumar, R., Nair, S. K., Hindu, V., et al. (2021). Genome wide association mapping for heat tolerance in sub-tropical maize. BMC Genomics 22:154. doi: 10.1186/s12864-021-07463-y
Lorenz, A. J., Chao, S., Asoro, F. G., Heffner, E. L., Hayashi, T., Iwata, H., et al. (2011). Genomic selection in plant breeding: knowledge and prospects. Adv. Agron. 110, 77–123.
Ludwig-Müller, J. (2011). Auxin conjugates: their role for plant development and in the evolution of land plants. J. Exp. Bot. 62, 1757–1773. doi: 10.1093/jxb/erq412
Luna, E., Pastor, V., Robert, J., Flors, V., Mauch-Mani, B., and Ton, J. (2011). Callose deposition: a multifaceted plant defense response. Mol. Plant Microbe Interact. 24, 183–193. doi: 10.1094/MPMI-07-10-0149
Ma, H., Liu, C., Li, Z., Ran, Q., Xie, G., Wang, B., et al. (2018). ZmbZIP4 contributes to stress resistance in maize by regulating ABA synthesis and root development. Plant Physiol. 178, 753–770. doi: 10.1104/pp.18.00436
Ma, P., Liu, J., Yang, X., and Ma, R. (2013). Genome-wide identification of the maize calcium-dependent protein kinase gene family. Appl. Biochem. Biotechnol. 169, 2111–2125. doi: 10.1007/s12010-013-0125-2
Mafakheri, A., Siosemardeh, A., Bahramnejad, B., Struik, P. C., and Sohrabi, Y. (2010). Effect of drought stress on yield, proline and chlorophyll contents in three chickpea cultivars. Aust. J. Crop Sci. 4, 580–585.
Magaña Ugarte, R., Escudero, A., and Gavilán, R. G. (2019). Metabolic and physiological responses of Mediterranean high-mountain and alpine plants to combined abiotic stresses. Physiol. Plant. 165, 403–412. doi: 10.1111/ppl.12898
Maitra, P., Al-Rashid, J., Barman, N. C., Khan, M., Morshed, N., Mandal, D., et al. (2021). Sand particle size and phosphorus amount affect Rhizophagus irregularis spore production using in vitro propagated spore as a starter inoculum in rhizosphere of maize (Zea mays) plantlets. J. Fungi 7:846. doi: 10.3390/jof7100846
Majeran, W., and van Wijk, K. J. (2009). Cell-type-specific differentiation of chloroplasts in C4 plants. Trends Plant Sci. 14, 100–109. doi: 10.1016/j.tplants.2008.11.006
Malenica, N., Duniæ, J. A., Vukadinoviæ, L., Cesar, V., and Šimiæ, D. (2021). Genetic approaches to enhance multiple stress tolerance in maize. Genes 12:1760. doi: 10.3390/genes12111760
Marcec, M. J., Gilroy, S., Poovaiah, B. W., and Tanaka, K. (2019). Mutual interplay of Ca2+ and ROS signaling in plant immune response. Plant Sci. 283, 343–354. doi: 10.1016/j.plantsci.2019.03.004
Markelz, R. C., Strellner, R. S., and Leakey, A. D. (2011). Impairment of C4 photosynthesis by drought is exacerbated by limiting nitrogen and ameliorated by elevated [CO2] in maize. J. Exp. Bot. 62, 3235–3246. doi: 10.1093/jxb/err056
Marrs, K. A., Casey, E. S., Capitant, S. A., Bouchard, R. A., Dietrich, P. S., Mettler, I. J., et al. (1993). Characterization of two maize HSP90 heat shock protein genes: expression during heat shock, embryogenesis, and pollen development. Dev. Genet. 14, 27–41. doi: 10.1002/dvg.1020140105
McKevith, B. (2004). Nutritional aspects of cereals. Nutr. Bull. 29, 111–142. doi: 10.1111/j.1467-3010.2004.00418.x
Medeiros, D. B., Brotman, Y., and Fernie, A. R. (2021). The utility of metabolomics as a tool to inform maize biology. Plant Commun. 2:100187. doi: 10.1016/j.xplc.2021.100187
Meena, A. K., Singh, D. K., Pandey, P. C., and Nanda, G. (2019). Dynamics of dry matter and nitrogen distribution in transplanted rice on mollisols. J. Plant Nutr. 42, 749–758. doi: 10.1080/01904167.2019.1567777
Meena, M. K., Ghawana, S., Dwivedi, V., Roy, A., and Chattopadhyay, D. (2015). Expression of chickpea CIPK25 enhances root growth and tolerance to dehydration and salt stress in transgenic tobacco. Front. Plant Sci. 6:683. doi: 10.3389/fpls.2015.00683
Meena, R. S., Lal, R., and Yadav, G. S. (2020). Long-term impact of topsoil depth and amendments on carbon and nitrogen budgets in the surface layer of an Alfisol in Central Ohio. Catena 194:104752. doi: 10.1016/j.catena.2020.104752
Miller, G., and Mittler, R. (2006). Could heat shock transcription factors function as hydrogen peroxide sensors in plants? Ann. Bot. 98, 279–288. doi: 10.1093/aob/mcl107
Mishra, V., Cruise, J. F., and Mecikalski, J. R. (2021). Assimilation of coupled microwave/thermal infrared soil moisture profiles into a crop model for robust maize yield estimates over Southeast United States. Eur. J. Agron. 123:126208. doi: 10.1016/j.eja.2020.126208
Mizoi, J., Shinozaki, K., and Yamaguchi-Shinozaki, K. (2012). AP2/ERF family transcription factors in plant abiotic stress responses. Biochim. Biophys. Acta 1819, 86–96. doi: 10.1016/j.bbagrm.2011.08.004
Mo, F., Sun, M., Liu, X.-Y., Wang, J.-Y., Zhang, X.-C., Ma, B., et al. (2016). Phenological responses of spring wheat and maize to changes in crop management and rising temperatures from 1992 to 2013 across the Loess Plateau. Field Crops Res. 196, 337–347. doi: 10.1016/j.fcr.2016.06.024
Mortimer, J. C., Laohavisit, A., Macpherson, N., Webb, A., Brownlee, C., Battey, N. H., et al. (2008). Annexins: multifunctional components of growth and adaptation. J. Exp. Bot. 59, 533–544. doi: 10.1093/jxb/erm344
Mujahid, A., Pumford, N. R., Bottje, W., Nakagawa, K., Miyazawa, T., Akiba, Y., et al. (2007). Mitochondrial oxidative damage in chicken skeletal muscle induced by acute heat stress. J. Poult. Sci. 44, 439–445. doi: 10.2141/jpsa.44.439
Navarro, J. A., Saiz-Bonilla, M., Sanchez-Navarro, J. A., and Pallas, V. (2021). The mitochondrial and chloroplast dual targeting of a multifunctional plant viral protein modulates chloroplast-to-nucleus communication, RNA silencing suppressor activity, encapsidation, pathogenesis and tissue tropism. Plant J. 108, 197–218. doi: 10.1111/tpj.15435
Naveed, S., Aslam, M., Maqbool, M., Bano, S., Zaman, Q., and Ahmad, R. (2014). Physiology of high temperature stress tolerance at reproductive stages in maize. J. Anim. Plant Sci. 24, 1141–1145.
Nawkar, G. M., Lee, E. S., Shelake, R. M., Park, J. H., Ryu, S. W., Kang, C. H., et al. (2018). Activation of the transducers of unfolded protein response in plants. Front. Plant Sci. 9:214. doi: 10.3389/fpls.2018.00214
Neill, E. M., Byrd, M. C., Billman, T., Brandizzi, F., and Stapleton, A. E. (2019). Plant growth regulators interact with elevated temperature to alter heat stress signaling via the Unfolded Protein Response in maize. Sci. Rep. 9:10392. doi: 10.1038/s41598-019-46839-9
Nestler, J., Liu, S., Wen, T.-J., Paschold, A., Marcon, C., Tang, H. M., et al. (2014). Roothairless5, which functions in maize (Zea mays L.) root hair initiation and elongation encodes a monocot-specific NADPH oxidase. Plant J. 79, 729–740. doi: 10.1111/tpj.12578
Nichols, C. (2005). Functional Characterisation of Plant Annexins. Ph.D. thesis. Cambridge: University of Cambridge.
Nieto-Sotelo, J., Martínez, L. M., Ponce, G., Cassab, G. I., Alagón, A., Meeley, R. B., et al. (2002). Maize HSP101 plays important roles in both induced and basal thermotolerance and primary root growth. Plant Cell 14, 1621–1633. doi: 10.1105/tpc.010487
Nieto-Sotelo, J., Vierling, E., and Ho, T.-H. D. (1990). Cloning, sequence analysis, and expression of a cDNA encoding a plastid-localized heat shock protein in maize. Plant Physiol. 93, 1321–1328. doi: 10.1104/pp.93.4.1321
Nievola, C. C., Carvalho, C. P., Carvalho, V., and Rodrigues, E. (2017). Rapid responses of plants to temperature changes. Temperature 4, 371–405. doi: 10.1080/23328940.2017.1377812
Nijabat, A., Bolton, A., Mahmood-Ur-Rehman, M., Shah, A. I., Hussain, R., Naveed, N. H., et al. (2020). Cell membrane stability and relative cell injury in response to heat stress during early and late seedling stages of diverse carrot (Daucus carota L.) germplasm. Hortscience 55, 1446–1452. doi: 10.21273/hortsci15058-20
Niu, S., Du, X., Wei, D., Liu, S., Tang, Q., Bian, D., et al. (2021). Heat stress after pollination reduces kernel number in maize by insufficient assimilates. Front. Genet. 12:728166. doi: 10.3389/fgene.2021.728166
Niu, Y., and Xiang, Y. (2018). An overview of biomembrane functions in plant responses to high-temperature stress. Front. Plant Sci. 9:915. doi: 10.3389/fpls.2018.00915
Ohama, N., Sato, H., Shinozaki, K., and Yamaguchi-Shinozaki, K. (2017). Transcriptional regulatory network of plant heat stress response. Trends Plant Sci. 22, 53–65. doi: 10.1016/j.tplants.2016.08.015
O’Leary, B., Park, J., and Plaxton, W. C. (2011). The remarkable diversity of plant PEPC (phosphoenolpyruvate carboxylase): recent insights into the physiological functions and post-translational controls of non-photosynthetic PEPCs. Biochem. J. 436, 15–34. doi: 10.1042/BJ20110078
Osuna, D., Prieto, P., and Aguilar, M. (2015). Control of seed germination and plant development by carbon and nitrogen availability. Front. Plant Sci. 6:1023. doi: 10.3389/fpls.2015.01023
Pamplona, R. (2008). Membrane phospholipids, lipoxidative damage and molecular integrity: a causal role in aging and longevity. Biochim. Biophys. Acta 1777, 1249–1262. doi: 10.1016/j.bbabio.2008.07.003
Pandey, N., Ranjan, A., Pant, P., Tripathi, R. K., Ateek, F., Pandey, H. P., et al. (2013). CAMTA 1 regulates drought responses in Arabidopsis thaliana. BMC Genomics 14:216. doi: 10.1186/1471-2164-14-216
Pandey, R., Maranville, J., and Admou, A. (2000). Deficit irrigation and nitrogen effects on maize in a Sahelian environment: I. Grain yield and yield components. Agric. Water Manag. 46, 15–27. doi: 10.1016/s0378-3774(00)00074-3
Parmar, A., Sturm, B., and Hensel, O. (2017). Crops that feed the world: production and improvement of cassava for food, feed, and industrial uses. Food Secur. 9, 907–927. doi: 10.1007/s12571-017-0717-8
Pastor-Cantizano, N., Ko, D. K., Angelos, E., Pu, Y., and Brandizzi, F. (2020). Functional diversification of ER stress responses in Arabidopsis. Trends Biochem. Sci. 45, 123–136. doi: 10.1016/j.tibs.2019.10.008
Paul, E. A., Paustian, K. H., Elliott, E., and Cole, C. V. (1996). Soil Organic Matter in Temperate AgroecosystemsLong Term Experiments in North America. Boca Raton, FL: CRC Press.
Pegoraro, C., Mertz, L. M., Da Maia, L. C., Rombaldi, C. V., and De Oliveira, A. C. (2011). Importance of heat shock proteins in maize. J. Crop Sci. Biotechnol. 14, 85–95.
Popp, J., Petö, K., and Nagy, J. (2013). Pesticide productivity and food security. A review. Agron. Sustain. Dev. 33, 243–255. doi: 10.1007/s13593-012-0105-x
Prasad, T., Hack, E., and Hallberg, R. (1990). Function of the maize mitochondrial chaperonin hsp60: specific association between hsp60 and newly synthesized F1-ATPase alpha subunits. Mol. Cell. Biol. 10, 3979–3986. doi: 10.1128/mcb.10.8.3979-3986.1990
Qian, Y., Ren, Q., Zhang, J., and Chen, L. (2019). Transcriptomic analysis of the maize (Zea mays L.) inbred line B73 response to heat stress at the seedling stage. Gene 692, 68–78. doi: 10.1016/j.gene.2018.12.062
Qin, F., Kakimoto, M., Sakuma, Y., Maruyama, K., Osakabe, Y., Tran, L. S. P., et al. (2007). Regulation and functional analysis of ZmDREB2A in response to drought and heat stresses in Zea mays L. Plant J. 50, 54–69. doi: 10.1111/j.1365-313X.2007.03034.x
Rahman, M. A., Chikushi, J., Yoshida, S., and Karim, A. (2009). Growth and yield components of wheat genotypes exposed to high temperature stress under control environment. Bangladesh J. Agric. Res. 34, 360–372. doi: 10.3329/bjar.v34i3.3961
Ranum, P., Peña-Rosas, J. P., and Garcia-Casal, M. N. (2014). Global maize production, utilization, and consumption. Ann. N. Y. Acad. Sci. 1312, 105–112. doi: 10.1111/nyas.12396
Rashed, M. A.-S., Abou-Deif, M. H., Khalil, K. M., and Mahmoud, F. E.-S. (2021). Expression levels of heat shock proteins through western blot and real-time polymerase chain reaction in maize. Jordan J. Biol. Sci. 14, 671–676. doi: 10.1007/s00726-010-0538-y
Razzaq, A., Kaur, P., Akhter, N., Wani, S. H., and Saleem, F. (2021). Next-generation breeding strategies for climate-ready crops. Front. Plant Sci. 12:620420. doi: 10.3389/fpls.2021.620420
Reddy, A., Reddy, V. S., and Golovkin, M. (2000). A calmodulin binding protein from Arabidopsis is induced by ethylene and contains a DNA-binding motif. Biochem. Biophys. Res. Commun. 279, 762–769. doi: 10.1006/bbrc.2000.4032
Reddy, A. S., Ben-Hur, A., and Day, I. S. (2011). Experimental and computational approaches for the study of calmodulin interactions. Phytochemistry 72, 1007–1019. doi: 10.1016/j.phytochem.2010.12.022
Reddy, P. S., Kavi Kishor, P. B., Seiler, C., Kuhlmann, M., Eschen-Lippold, L., Lee, J., et al. (2014). Unraveling regulation of the small heat shock proteins by the heat shock factor HvHsfB2c in barley: its implications in drought stress response and seed development. PLoS One 9:e89125. doi: 10.1371/journal.pone.0089125
Reddy, V. S., Day, I. S., Thomas, T., and Reddy, A. S. N. (2004). KIC, a novel Ca2+ binding protein with one EF-hand motif, interacts with a microtubule motor protein and regulates trichome morphogenesis. Plant Cell 16, 185–200. doi: 10.1105/tpc.016600
Reimold, A. M., Etkin, A., Clauss, I., Perkins, A., Friend, D. S., Zhang, J., et al. (2000). An essential role in liver development for transcription factor XBP-1. Genes Dev. 14, 152–157. doi: 10.1101/gad.14.2.152
Rezaei, E. E., Webber, H., Gaiser, T., Naab, J., and Ewert, F. (2015). Heat stress in cereals: mechanisms and modelling. Eur. J. Agron. 64, 98–113. doi: 10.1016/j.eja.2014.10.003
Ribeiro, C., Hennen-Bierwagen, T. A., Myers, A. M., Cline, K., and Settles, A. M. (2020). Engineering 6-phosphogluconate dehydrogenase to improve heat tolerance in maize seed development. bioRxiv [Preprint] doi: 10.1101/2020.05.21.108985
Rochaix, J.-D. (2011). Regulation of photosynthetic electron transport. Biochim. Biophys. Acta 1807, 375–383.
Rodziewicz, P., Swarcewicz, B., Chmielewska, K., Wojakowska, A., and Stobiecki, M. (2014). Influence of abiotic stresses on plant proteome and metabolome changes. Acta Physiol. Plant. 36, 1–19. doi: 10.1007/s11738-013-1402-y
Rooney, W. L., Blumenthal, J., Bean, B., and Mullet, J. E. (2007). Designing sorghum as a dedicated bioenergy feedstock. Biofuels Bioprod. Biorefin. 1, 147–157. doi: 10.1186/s13068-017-0892-z
Rosenzweig, C., Iglesius, A., Yang, X.-B., Epstein, P. R., and Chivian, E. (2001). Climate change and extreme weather events: implications for food production, plant diseases, and pests. Glob. Change Hum. Health 2, 90–104.
Ruan, Y.-L., Jin, Y., Yang, Y.-J., Li, G.-J., and Boyer, J. S. (2010). Sugar input, metabolism, and signaling mediated by invertase: roles in development, yield potential, and response to drought and heat. Mol. Plant 3, 942–955. doi: 10.1093/mp/ssq044
Rupwate, S. D., and Rajasekharan, R. (2012). Plant phosphoinositide-specific phospholipase C: an insight. Plant Signal. Behav. 7, 1281–1283. doi: 10.4161/psb.21436
Ryel, R., Caldwell, M., Yoder, C., Or, D., and Leffler, A. (2002). Hydraulic redistribution in a stand of Artemisia tridentata: evaluation of benefits to transpiration assessed with a simulation model. Oecologia 130, 173–184. doi: 10.1007/s004420100794
Sabagh, A. E., Hossain, A., Iqbal, M. A., Barutçular, C., Islam, M. S., Çiǧ, F., et al. (2020). “Maize adaptability to heat stress under changing climate,” in Plant Stress Physiology, ed. A. Hossain (London: IntechOpen).
Sachs, M. M., Subbaiah, C. C., and Saab, I. N. (1996). Anaerobic gene expression and flooding tolerance in maize. J. Exp. Bot. 47, 1–15. doi: 10.1104/pp.109.2.433
Sagi, M., and Fluhr, R. (2006). Production of reactive oxygen species by plant NADPH oxidases. Plant Physiol. 141, 336–340. doi: 10.1104/pp.106.078089
Sah, R., Chakraborty, M., Prasad, K., Pandit, M., Tudu, V., Chakravarty, M., et al. (2020). Impact of water deficit stress in maize: phenology and yield components. Sci. Rep. 10:2944. doi: 10.1038/s41598-020-59689-7
Sakai, A., and Larcher, W. (2012). Frost Survival of Plants: Responses and Adaptation to Freezing Stress. Berlin: Springer Science & Business Media.
Sallam, A., Amro, A., El-Akhdar, A., Dawood, M. F. A., Kumamaru, T., and Stephen Baenziger, P. (2018). Genetic diversity and genetic variation in morpho-physiological traits to improve heat tolerance in Spring barley. Mol. Biol. Rep. 45, 2441–2453. doi: 10.1007/s11033-018-4410-6
Sánchez, B., Rasmussen, A., and Porter, J. R. (2014). Temperatures and the growth and development of maize and rice: a review. Glob. Change Biol. 20, 408–417. doi: 10.1111/gcb.12389
Sang, J., Zhang, A., Lin, F., Tan, M., and Jiang, M. (2008). Cross-talk between calcium-calmodulin and nitric oxide in abscisic acid signaling in leaves of maize plants. Cell Res. 18, 577–588. doi: 10.1038/cr.2008.39
Schramm, F., Larkindale, J., Kiehlmann, E., Ganguli, A., Englich, G., Vierling, E., et al. (2008). A cascade of transcription factor DREB2A and heat stress transcription factor HsfA3 regulates the heat stress response of Arabidopsis. Plant J. 53, 264–274. doi: 10.1111/j.1365-313X.2007.03334.x
Seetharam, K., Kuchanur, P. H., Koirala, K., Tripathi, M. P., Patil, A., Sudarsanam, V., et al. (2021). Genomic regions associated with heat stress tolerance in tropical maize (Zea mays L.). Sci. Rep. 11:13730. doi: 10.1038/s41598-021-93061-7
Sengupta, S., Mukherjee, S., Basak, P., and Majumder, A. L. (2015). Significance of galactinol and raffinose family oligosaccharide synthesis in plants. Front. Plant Sci. 6:656. doi: 10.3389/fpls.2015.00656
Sewelam, N., Jaspert, N., Van Der Kelen, K., Tognetti, V. B., Schmitz, J., Frerigmann, H., et al. (2014). Spatial H2O2 signaling specificity: H2O2 from chloroplasts and peroxisomes modulates the plant transcriptome differentially. Mol. Plant 7, 1191–1210. doi: 10.1093/mp/ssu070
Sharma, A., Kumar, V., Shahzad, B., Ramakrishnan, M., Singh Sidhu, G. P., Bali, A. S., et al. (2020). Photosynthetic response of plants under different abiotic stresses: a review. J. Plant Growth Regul. 39, 509–531.
Sharma, A., Shahzad, B., Kumar, V., Kohli, S. K., Sidhu, G. P. S., Bali, A. S., et al. (2019). Phytohormones regulate accumulation of osmolytes under abiotic stress. Biomolecules 9:285. doi: 10.3390/biom9070285
Sharma, D. K., Torp, A. M., Rosenqvist, E., Ottosen, C.-O., and Andersen, S. B. (2017). QTLs and potential candidate genes for heat stress tolerance identified from the mapping populations specifically segregating for Fv/Fm in wheat. Front. Plant Sci. 8:1668. doi: 10.3389/fpls.2017.01668
Shi, S., Li, S., Asim, M., Mao, J., Xu, D., Ullah, Z., et al. (2018). The Arabidopsis calcium-dependent protein kinases (CDPKs) and their roles in plant growth regulation and abiotic stress responses. Int. J. Mol. Sci. 19:1900. doi: 10.3390/ijms19071900
Siebers, M. H., Slattery, R. A., Yendrek, C. R., Locke, A. M., Drag, D., Ainsworth, E. A., et al. (2017). Simulated heat waves during maize reproductive stages alter reproductive growth but have no lasting effect when applied during vegetative stages. Agric. Ecosyst. Environ. 240, 162–170. doi: 10.1016/j.agee.2016.11.008
Silim, S., Coe, R., Omanga, P., and Gwata, E. (2006). The response of pigeonpea genotypes of different duration types to variation in temperature and photoperiod under field conditions in Kenya. J. Food Agric. Environ. 4, 209–214.
Singh, R., Gupta, R., Bhardwaj, R., and Singh, R. (2021). “CRISPR/CAS9 technologies to enhance tolerance to abiotic stress in crop plants,” in Environmental Stress Physiology of Plants and Crop Productivity, eds T. Kaur and S. Arora (New York, NY: Bantam Books), 206. doi: 10.2174/9781681087900121010016
Singletary, G. W., Banisadr, R., and Keeling, P. L. (1994). Heat stress during grain filling in maize: effects on carbohydrate storage and metabolism. Funct. Plant Biol. 21, 829–841. doi: 10.1071/pp9940829
Slama, I., Abdelly, C., Bouchereau, A., Flowers, T., and Savouré, A. (2015). Diversity, distribution and roles of osmoprotective compounds accumulated in halophytes under abiotic stress. Ann. Bot. 115, 433–447. doi: 10.1093/aob/mcu239
Song, J., Weng, Q., Ma, H., Yuan, J., Wang, L., and Liu, Y. (2016). Cloning and expression analysis of the Hsp70 gene ZmERD2 in Zea mays. Biotechnol. Biotechnol. Equip. 30, 219–226. doi: 10.1080/13102818.2015.1131625
Song, X., Weng, Q., Zhao, Y., Ma, H., Song, J., Su, L., et al. (2018). Cloning and expression analysis of ZmERD3 gene from Zea mays. Iran J. Biotechnol. 16:e1593. doi: 10.21859/ijb.1593
Stanley, D. W., and Parkin, K. L. (1991). Biological membrane deterioration and associated quality losses in food tissues. Crit. Rev. Food Sci. Nutr. 30, 487–553. doi: 10.1080/10408399109527554
Stavridou, E., Voulgari, G., Michailidis, M., Kostas, S., Chronopoulou, E. G., Labrou, N. E., et al. (2021). Overexpression of a biotic stress-inducible Pvgstu gene activates early protective responses in tobacco under combined heat and drought. Int. J. Mol. Sci. 22:2352. doi: 10.3390/ijms22052352
Strasser, R. J., Tsimilli-Michael, M., and Srivastava, A. (2004). “Analysis of the chlorophyll a fluorescence transient,” in Chlorophyll a Fluorescence, eds G. C. Papageorgiou Govindjee (Dordrecht: Springer), 321–362. doi: 10.1007/978-1-4020-3218-9_12
Su, H., Cao, Y., Ku, L., Yao, W., Cao, Y., Ren, Z., et al. (2018). Dual functions of ZmNF-YA3 in photoperiod-dependent flowering and abiotic stress responses in maize. J. Exp. Bot. 69, 5177–5189. doi: 10.1093/jxb/ery299
Sun, L., Liu, Y., Kong, X., Zhang, D., Pan, J., Zhou, Y., et al. (2012). ZmHSP16.9, a cytosolic class I small heat shock protein in maize (Zea mays), confers heat tolerance in transgenic tobacco. Plant Cell Rep. 31, 1473–1484. doi: 10.1007/s00299-012-1262-8
Swapna, S., and Shylaraj, K. S. (2017). Screening for osmotic stress responses in rice varieties under drought condition. Rice Sci. 24, 1446–1452.
Takemoto, D., Tanaka, A., and Scott, B. (2007). NADPH oxidases in fungi: diverse roles of reactive oxygen species in fungal cellular differentiation. Fungal Genet. Biol. 44, 1065–1076. doi: 10.1016/j.fgb.2007.04.011
Tan, Y.-Q., Yang, Y., Zhang, A., Fei, C.-F., Gu, L.-L., Sun, S.-J., et al. (2020). Three CNGC family members, CNGC5, CNGC6, and CNGC9, are required for constitutive growth of Arabidopsis root hairs as Ca2+-permeable channels. Plant Commun. 1:100001. doi: 10.1016/j.xplc.2019.100001
Tao, F., and Zhang, Z. (2010). Adaptation of maize production to climate change in North China Plain: quantify the relative contributions of adaptation options. Eur. J. Agron. 33, 103–116. doi: 10.1016/j.eja.2010.04.002
Tebaldi, C., and Lobell, D. (2018). Estimated impacts of emission reductions on wheat and maize crops. Clim. Change 146, 533–545. doi: 10.1007/s10584-015-1537-5
Tiwari, Y. K., and Yadav, S. K. (2019). High temperature stress tolerance in maize (Zea mays L.): physiological and molecular mechanisms. J. Plant Biol. 62, 93–102. doi: 10.1007/s12374-018-0350-x
Török, Z., Crul, T., Maresca, B., Schütz, G. J., Viana, F., Dindia, L., et al. (2014). Plasma membranes as heat stress sensors: from lipid-controlled molecular switches to therapeutic applications. Biochim. Biophys. Acta 1838, 1594–1618. doi: 10.1016/j.bbamem.2013.12.015
Tóth, S. Z., Nagy, V., Puthur, J. T., Kovács, L., and Garab, G. (2011). The physiological role of ascorbate as photosystem ii electron donor: protection against photoinactivation in heat-stressed leaves. Plant Physiol. 156, 382–392. doi: 10.1104/pp.110.171918
Towil, L. E. (2010). Long-term pollen storage. Plant Breed. Rev. 13, 179–207. doi: 10.1002/9780470650059.ch5
Urquhart, W., Chin, K., Ung, H., Moeder, W., and Yoshioka, K. (2011). The cyclic nucleotide-gated channels AtCNGC11 and 12 are involved in multiple Ca2+-dependent physiological responses and act in a synergistic manner. J. Exp. Bot. 62, 3671–3682. doi: 10.1093/jxb/err074
Van Inghelandt, D., Frey, F. P., Ries, D., and Stich, B. (2019). QTL mapping and genome-wide prediction of heat tolerance in multiple connected populations of temperate maize. Sci. Rep. 9:14418. doi: 10.1038/s41598-019-50853-2
Vardhini, B. V., and Anjum, N. A. (2015). Brassinosteroids make plant life easier under abiotic stresses mainly by modulating major components of antioxidant defense system. Front. Environ. Sci. 2:67. doi: 10.3389/fenvs.2014.00067
Vass, I., and Cser, K. (2009). Janus-faced charge recombinations in photosystem II photoinhibition. Trends Plant Sci. 14, 200–205. doi: 10.1016/j.tplants.2009.01.009
Vitale, A., and Boston, R. S. (2008). Endoplasmic reticulum quality control and the unfolded protein response: insights from plants. Traffic 9, 1581–1588. doi: 10.1111/j.1600-0854.2008.00780.x
von Caemmerer, S., and Furbank, R. T. (2016). Strategies for improving C4 photosynthesis. Curr. Opin. Plant Biol. 31, 125–134. doi: 10.1016/j.pbi.2016.04.003
Wadhwa, N., Mathew, B. B., Jatawa, S., and Tiwari, A. (2012). Lipid peroxidation: mechanism, models and significance. Int. J. Curr. Sci. 3, 29–38.
Wahid, A., Gelani, S., Asharf, M., and Foolad, M. R. (2007). Heat tolerance in plants: an overview. Environ. Exp. Bot. 61, 199–223. doi: 10.1016/j.envexpbot.2007.05.011
Wang, C.-T., Ru, J.-N., Liu, Y.-W., Li, M., Zhao, D., Yang, J.-F., et al. (2018a). Maize WRKY transcription factor ZmWRKY106 confers drought and heat tolerance in transgenic plants. Int. J. Mol. Sci. 19:3046. doi: 10.3390/ijms19103046
Wang, C.-T., Ru, J.-N., Liu, Y.-W., Yang, J.-F., Li, M., Xu, Z.-S., et al. (2018b). The maize WRKY transcription factor ZmWRKY40 confers drought resistance in transgenic Arabidopsis. Int. J. Mol. Sci. 19:2580. doi: 10.3390/ijms19092580
Wang, W., Vinocur, B., Shoseyov, O., and Altman, A. (2004). Role of plant heat-shock proteins and molecular chaperones in the abiotic stress response. Trends Plant Sci. 9, 244–252. doi: 10.1016/j.tplants.2004.03.006
Wang, X., Wang, H., Liu, S., Ferjani, A., Li, J., Yan, J., et al. (2016). Genetic variation in ZmVPP1 contributes to drought tolerance in maize seedlings. Nat. Genet. 48, 1233–1241. doi: 10.1038/ng.3636
Waqas, M. A., Wang, X., Zafar, S. A., Noor, M. A., Hussain, H. A., Azher Nawaz, M., et al. (2021). Thermal stresses in maize: effects and management strategies. Plants 10:293. doi: 10.3390/plants10020293
Waraich, E., Ahmad, R., Halim, A., and Aziz, T. (2012). Alleviation of temperature stress by nutrient management in crop plants: a review. J. Soil Sci. Plant Nutr. 12, 221–244. doi: 10.4014/jmb.2105.05009
Weckwerth, P., Ehlert, B., and Romeis, T. (2015). ZmCPK1, a calcium-independent kinase member of the Zea mays CDPK gene family, functions as a negative regulator in cold stress signalling. Plant Cell Environ. 38, 544–558. doi: 10.1111/pce.12414
Wen, W., Li, D., Li, X., Gao, Y., Li, W., Li, H., et al. (2014). Metabolome-based genome-wide association study of maize kernel leads to novel biochemical insights. Nat. Commun. 5:3438. doi: 10.1038/ncomms4438
Wilson, D., Muchow, R., and Murgatroyd, C. (1995). Model analysis of temperature and solar radiation limitations to maize potential productivity in a cool climate. Field Crops Res. 43, 1–18. doi: 10.1016/0378-4290(95)00037-q
Xalxo, R., Yadu, B., Chandra, J., Chandrakar, V., and Keshavkant, S. (2020). “Alteration in carbohydrate metabolism modulates thermotolerance of plant under heat stress,” in Heat Stress Tolerance in Plants: Physiological, Molecular and Genetic Perspectives, eds S. H. Wani and V. Kumar (Hoboken, NJ: Wiley), 77–115. doi: 10.1016/j.jprot.2020.103968
Xu, Y., Chu, C., and Yao, S. (2021). The impact of high-temperature stress on rice: challenges and solutions. Crop J. 9, 963–976. doi: 10.3390/plants10010043
Yadav, S., Tiwari, Y. K., Singh, V., Patil, A. A., Shanker, A., Lakshmi, N. J., et al. (2018). Physiological and biochemical basis of extended and sudden heat stress tolerance in maize. Proc. Natl. Acad. Sci. India Sect. B Biol. Sci. 88, 249–263. doi: 10.1007/s40011-016-0752-9
Yadav, S., Vanaja, M., and Maheswari, M. (2017). Exogenous application of bio-regulators for alleviation of heat stress in seedlings of maize. J. Agric. Res. 2:000137.
Yamaguchi, S. (2008). Gibberellin metabolism and its regulation. Annu. Rev. Plant Biol. 59, 225–251. doi: 10.1146/annurev.arplant.59.032607.092804
Yan, K., Chen, P., Shao, H., Shao, C., Zhao, S., and Brestic, M. (2013). Dissection of photosynthetic electron transport process in sweet sorghum under heat stress. PLoS One 8:e62100. doi: 10.1371/journal.pone.0062100
Yan, Q., Huang, Q., Chen, J., Li, J., Liu, Z., Yang, Y., et al. (2017). SYTA has positive effects on the heat resistance of Arabidopsis. Plant Growth Regul. 81, 467–476. doi: 10.1007/s10725-016-0224-5
Yan, Q., Zong, X., Wu, F., Li, J., Ma, T., Zhao, Y., et al. (2020). Integrated analysis of co-expression, conserved genes and gene families reveal core regulatory network of heat stress response in Cleistogenes songorica, a xerophyte perennial desert plant. BMC Genomics 21:715. doi: 10.1186/s12864-020-07122-8
Yang, G., Rhodes, D., and Joly, R. J. (1996). Effects of high temperature on membrane stability and chlorophyll fluorescence in glycinebetaine-deficient and glycinebetaine-containing maize lines. Funct. Plant Biol. 23, 437–443. doi: 10.1071/pp9960437
Yang, L., Wang, Y., and Yang, K. (2021). Klebsiella variicola improves the antioxidant ability of maize seedlings under saline-alkali stress. PeerJ 9:e11963. doi: 10.7717/peerj.11963
Yang, T., Peng, H., Whitaker, B. D., and Jurick, W. M. (2013). Differential expression of calcium/calmodulin-regulated SlSRs in response to abiotic and biotic stresses in tomato fruit. Physiol. Plant. 148, 445–455. doi: 10.1111/ppl.12027
Yang, T., and Poovaiah, B. (2002). A calmodulin-binding/CGCG box DNA-binding protein family involved in multiple signaling pathways in plants. J. Biol. Chem. 277, 45049–45058. doi: 10.1074/jbc.M207941200
Yang, W., Kong, Z., Omo-Ikerodah, E., Xu, W., Li, Q., and Xue, Y. (2008). Calcineurin B-like interacting protein kinase OsCIPK23 functions in pollination and drought stress responses in rice (Oryza sativa L.). J. Genet. Genomics 35, 531–543, S1–S2. doi: 10.1016/S1673-8527(08)60073-9
Yeh, S.-Y., Lin, H.-H., Chang, Y.-M., Chang, Y.-L., Chang, C.-K., Huang, Y.-C., et al. (2021). Maize Golden2-like transcription factors boost rice chloroplast development, photosynthesis, and grain yield. Plant Physiol. 188, 442–459. doi: 10.1093/plphys/kiab511
Younis, A., Ramzan, F., Ramzan, Y., Zulfiqar, F., Ahsan, M., and Lim, K. B. (2020). Molecular markers improve abiotic stress tolerance in crops: a review. Plants 9:1374. doi: 10.3390/plants9101374
Yuan, B. Z., Sun, J., and Nishiyama, S. (2004). Effect of drip irrigation on strawberry growth and yield inside a plastic greenhouse. Biosyst. Eng. 87, 237–245. doi: 10.1016/j.biosystemseng.2003.10.014
Yue, R., Lu, C., Sun, T., Peng, T., Han, X., Qi, J., et al. (2015). Identification and expression profiling analysis of calmodulin-binding transcription activator genes in maize (Zea mays L.) under abiotic and biotic stresses. Front. Plant Sci. 6:576. doi: 10.3389/fpls.2015.00576
Zhai, S., Gao, Q., Liu, X., Sui, Z., and Zhang, J. (2013). Overexpression of a Zea mays phospholipase C1 gene enhances drought tolerance in tobacco in part by maintaining stability in the membrane lipid composition. Plant Cell Tissue Organ Cult. 115, 253–262. doi: 10.1007/s11240-013-0358-3
Zhang, D., Li, Q., Yamori, W., and Wei, M. (2020a). The Rate-Limiting Step for Photosynthetic CO2 Utilization Under Varying Atmospheric Evaporative Demand in Solanum lycopersicum (Tomato). Durham, NC: Research Square Company.
Zhang, H., Li, G., Fu, C., Duan, S., Hu, D., and Guo, X. (2020b). Genome-wide identification, transcriptome analysis and alternative splicing events of Hsf family genes in maize. Sci. Rep. 10:8073. doi: 10.1038/s41598-020-65068-z
Zhang, H., Li, G., Hu, D., Zhang, Y., Zhang, Y., Shao, H., et al. (2020c). Functional characterization of maize heat shock transcription factor gene ZmHsf01 in thermotolerance. PeerJ 8:e8926. doi: 10.7717/peerj.8926
Zhang, S.-S., Yang, H., Ding, L., Song, Z.-T., Ma, H., Chang, F., et al. (2017). Tissue-specific transcriptomics reveals an important role of the unfolded protein response in maintaining fertility upon heat stress in Arabidopsis. Plant Cell 29, 1007–1023. doi: 10.1105/tpc.16.00916
Zhao, C., Liu, B., Piao, S., Wang, X., Lobell, D. B., Huang, Y., et al. (2017). Temperature increase reduces global yields of major crops in four independent estimates. Proc. Natl. Acad. Sci. U.S.A. 114, 9326–9331. doi: 10.1073/pnas.1701762114
Zhao, J., Lu, Z., Wang, L., and Jin, B. (2021). Plant responses to heat stress: physiology, transcription, noncoding RNAs, and epigenetics. Int. J. Mol. Sci. 22:117. doi: 10.3390/ijms22010117
Zhao, Y., Du, H., Wang, Y., Wang, H., Yang, S., Li, C., et al. (2021). The calcium-dependent protein kinase ZmCDPK7 functions in heat-stress tolerance in maize. J. Integr. Plant Biol. 63, 510–527. doi: 10.1111/jipb.13056
Zhou, L., Lan, W., Chen, B., Fang, W., and Luan, S. (2015). A calcium sensor-regulated protein kinase, CALCINEURIN B-LIKE PROTEIN-INTERACTING PROTEIN KINASE19, is required for pollen tube growth and polarity. Plant Physiol. 167, 1351–1360. doi: 10.1104/pp.114.256065
Zhou, M.-L., Tang, Y.-X., and Wu, Y.-M. (2012). Genome-wide analysis of AP2/ERF transcription factor family in Zea mays. Curr. Bioinformatics 7, 324–332. doi: 10.2174/157489312802460776
Zhou, Z. H., Wang, Y., Ye, X. Y., and Li, Z. G. (2018). Signaling molecule hydrogen sulfide improves seed germination and seedling growth of maize (Zea mays L.) under high temperature by inducing antioxidant system and osmolyte biosynthesis. Front. Plant Sci. 9:1288. doi: 10.3389/fpls.2018.01288
Zivcak, M., Brestic, M., Kunderlikova, K., Sytar, O., and Allakhverdiev, S. I. (2015). Repetitive light pulse-induced photoinhibition of photosystem I severely affects CO 2 assimilation and photoprotection in wheat leaves. Photosynth. Res. 126, 449–463. doi: 10.1007/s11120-015-0121-1
Keywords: abiotic stress, gene signaling cascade, heat stress, molecular response, Zea mays
Citation: El-Sappah AH, Rather SA, Wani SH, Elrys AS, Bilal M, Huang Q, Dar ZA, Elashtokhy MMA, Soaud N, Koul M, Mir RR, Yan K, Li J, El-Tarabily KA and Abbas M (2022) Heat Stress-Mediated Constraints in Maize (Zea mays) Production: Challenges and Solutions. Front. Plant Sci. 13:879366. doi: 10.3389/fpls.2022.879366
Received: 19 February 2022; Accepted: 30 March 2022;
Published: 29 April 2022.
Edited by:
Ahmad M. Alqudah, Aarhus University, DenmarkReviewed by:
Mona F. A. Dawood, Assiut University, EgyptCopyright © 2022 El-Sappah, Rather, Wani, Elrys, Bilal, Huang, Dar, Elashtokhy, Soaud, Koul, Mir, Yan, Li, El-Tarabily and Abbas. This is an open-access article distributed under the terms of the Creative Commons Attribution License (CC BY). The use, distribution or reproduction in other forums is permitted, provided the original author(s) and the copyright owner(s) are credited and that the original publication in this journal is cited, in accordance with accepted academic practice. No use, distribution or reproduction is permitted which does not comply with these terms.
*Correspondence: Khaled A. El-Tarabily, a3RhcmFpbHlAdWFldS5hYy5hZQ==; Manzar Abbas, YWJiYXMyNDcyQGhvdG1haWwuY29t
†These authors have contributed equally to this work
Disclaimer: All claims expressed in this article are solely those of the authors and do not necessarily represent those of their affiliated organizations, or those of the publisher, the editors and the reviewers. Any product that may be evaluated in this article or claim that may be made by its manufacturer is not guaranteed or endorsed by the publisher.
Research integrity at Frontiers
Learn more about the work of our research integrity team to safeguard the quality of each article we publish.