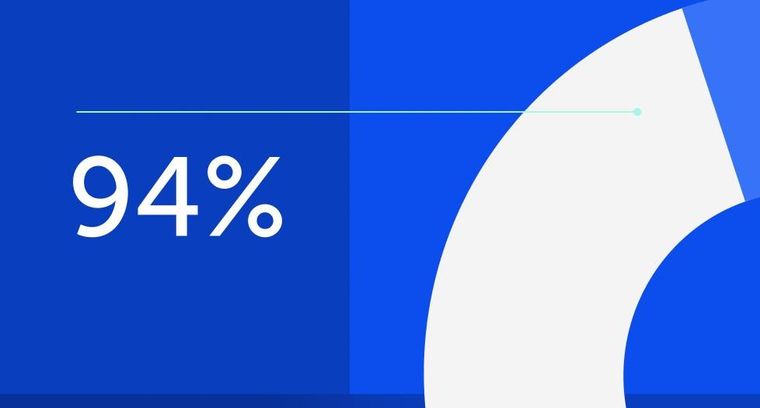
94% of researchers rate our articles as excellent or good
Learn more about the work of our research integrity team to safeguard the quality of each article we publish.
Find out more
SYSTEMATIC REVIEW article
Front. Plant Sci., 28 June 2022
Sec. Crop and Product Physiology
Volume 13 - 2022 | https://doi.org/10.3389/fpls.2022.878498
This article is part of the Research TopicPhysiological and Molecular Perspectives of Stress Tolerance in VegetablesView all 13 articles
Vegetables are a distinct collection of plant-based foods that vary in nutritional diversity and form an important part of the healthy diet of the human being. Besides providing basic nutrition, they have great potential for boosting human health. The balanced consumption of vegetables is highly recommended for supplementing the human body with better nutrition density, dietary fiber, minerals, vitamins, and bioactive compounds. However, the production and quality of fresh vegetables are influenced directly or indirectly by exposure to high temperatures or heat stress (HS). A decline in quality traits and harvestable yield are the most common effects of HS among vegetable crops. Heat-induced morphological damage, such as poor vegetative growth, leaf tip burning, and rib discoloration in leafy vegetables and sunburn, decreased fruit size, fruit/pod abortion, and unfilled fruit/pods in beans, are common, often rendering vegetable cultivation unprofitable. Further studies to trace down the possible physiological and biochemical effects associated with crop failure reveal that the key factors include membrane damage, photosynthetic inhibition, oxidative stress, and damage to reproductive tissues, which may be the key factors governing heat-induced crop failure. The reproductive stage of plants has extensively been studied for HS-induced abnormalities. Plant reproduction is more sensitive to HS than the vegetative stages, and affects various reproductive processes like pollen germination, pollen load, pollen tube growth, stigma receptivity, ovule fertility and, seed filling, resulting in poorer yields. Hence, sound and robust adaptation and mitigation strategies are needed to overcome the adverse impacts of HS at the morphological, physiological, and biochemical levels to ensure the productivity and quality of vegetable crops. Physiological traits such as the stay-green trait, canopy temperature depression, cell membrane thermostability, chlorophyll fluorescence, relative water content, increased reproductive fertility, fruit numbers, and fruit size are important for developing better yielding heat-tolerant varieties/cultivars. Moreover, various molecular approaches such as omics, molecular breeding, and transgenics, have been proved to be useful in enhancing/incorporating tolerance and can be potential tools for developing heat-tolerant varieties/cultivars. Further, these approaches will provide insights into the physiological and molecular mechanisms that govern thermotolerance and pave the way for engineering “designer” vegetable crops for better health and nutritional security. Besides these approaches, agronomic methods are also important for adaptation, escape and mitigation of HS protect and improve yields.
Vegetables are parts of plants cultivated worldwide for consumption as flowers (e.g., cauliflower, broccoli), fruits (e.g., okra, tomato, cucumber, capsicum), leaves (e.g., spinach, lettuce, brassica, cabbage), tubers (e.g., potato, sweet potato), pods and seeds (e.g., common bean, chickpea, broad bean, mungbean, peas) (Peet and Wolfe, 2000). Vegetables contain secondary metabolites with bioactive properties, including carotenoids (e.g., carrots, pepper, tomato, spinach), polyphenols (e.g., tomato, cabbage), glucosinolates (e.g., brassica), saponins (e.g., beans, pea), and terpenes (e.g., carrots, tomato) (Crozier et al., 2006). These bioactive compounds are metabolic intermediates of primary metabolic processes, which are not essential for plant growth but are used in plant defense responses and plant-insect interactions and can stimulate human health. Clearly, vegetables are an important part of the human diet as they replenish our body with various nutrients, including vitamins, dietary minerals, fibers, proteins, antioxidants, carbohydrates, small amounts of fat, and phytochemicals with anticarcinogenic, antiviral, antifungal, and antibacterial properties (Osagie and Eka, 1998; Teng et al., 2021). While not a major energy source, vegetables nourish our bodies with much-needed minerals and vitamins. According to Food and Agriculture Organization (FAO) statistics, vegetables are the source of dietary requirements about 60% of vitamin A and 90% of vitamin C (Gruda, 2005). Vegetables can earn extra income for farmers as they are seasonal plants with higher yields per hectare than staple crops (Abewoy, 2018). The market value of vegetables is assessed by their quality; FAO and WHO provide many quality attributes for grading vegetables, e.g., color, size, shape, texture, aroma, shelf life, and storability (Gruda, 2005). Vegetables are categorized into two groups according to their growing season; warm-season vegetables include capsicum, common bean, cucumber, cowpea, okra, tomato, and mungbean (Peet and Wolfe, 2000), while cool-season vegetables include brassica, broad bean, broccoli, cabbage, cauliflower, lettuce, radish, spinach, soybean, pea, and potato (Peet and Wolfe, 2000) (Table 1).
Like other crops, vegetables are also affected by environmental changes that can render vegetable cultivation unprofitable. Abiotic stresses, mainly the high temperature (heat stress. HS), severely limit crop quantity, quality, nutritional status, and production (Boote et al., 2005; Aleem et al., 2021). High temperatures affect the overall growth and development of vegetable crops by altering morphology, physiology, and enzymatic activities. Heat stress (HS) accelerates phenology, shortening the vegetative and reproductive stages. HS reduces vegetable quality, such as changing the color and texture of fruits (e.g., cucumber, pepper, and tomato) (Zipelevish et al., 2000). In general, HS affects morphological, physiological, and biochemical processes of the plant by hampering photosynthetic activity, source-sink relationship, and altered enzymatic activities (Bita and Gerats, 2013; Janni et al., 2020). The quality of vegetables is also impacted by HS, through a change in color and texture of fruit (e.g., cucumber, pepper, and tomato) (Zipelevish et al., 2000). HS also affects the nutritional status of vegetables; for instance, reducing lycopene in tomato (Gross, 1991) and β-carotene in spinach and lettuce (Oyama et al., 1999) and increasing nitrate levels to harmful levels for human consumption.
Due to climate change, in most regions of the world, rising temperatures will decrease quantity and quality of vegetables crops. Studies of Waithaka et al. (2013) suggested that changes in the climate (increased temperatures) will also provide avenues to grow crops in areas where they could not be grown previously. Climate change scenarios further suggest that development of crop and cultivar choice—especially for water-limited or high-temperature areas—will be an important strategy to have adequate yields under changing climate (Thomas et al., 2007). Hence, targeted studies are needed to assess the impact of high-temperature stress on the growth, yield, and quality (taste, flavor, color, nutritional content) of vegetable crops, with suitable agronomic strategies, developed to create heat-tolerant cultivars or mitigate HS.
High temperatures adversely impact plant growth and development (Hasanuzzaman et al., 2013). The constantly rising average surface temperature due to global warming is stressful for all plant growth and development phases, limiting metabolism and productivity, particularly in tropical and subtropical countries (Li et al., 2018). According to the newly released sixth assessment report of IPCC (2021), temperature during the twenty-first century is likely to increase by 1.5°C of warming within just the next two decades, and by 4.5°C, depending on the rate of greenhouse gas emissions. As plants are sedentary organisms, they acclimate to HS by using avoidance mechanisms or programmed cell death (Mittler et al., 2012; Singh, 2013; Zhang T. et al., 2020). Each vegetable crop has temperature threshold for its growth and development; HS will occur beyond the upper threshold for temperature (Wahid et al., 2007; Prasad et al., 2008, 2017). HS impedes photosynthesis through reduced carbon assimilation, ATP reduction, and oxidative damage to chloroplasts, with simultaneous reductions in dry matter accumulation and yield (Sharkey, 2005; Farooq et al., 2011). HS adversely affects vegetative and reproductive plant parts (Bita and Gerats, 2013); thus, the impact of HS varies depending on the developmental stage and crop species (Prasad et al., 2017; Li et al., 2018) (Table 2).
Moderate high temperatures stimulate early vegetative growth and accelerate physiological maturity (Nahar et al., 2015). During seed germination, HS reduces germination percentage and seedling emergence, reduces radical and plumule growth in germinated seedlings, and causes abnormal seedlings and poor seedling vigor (Hasanuzzaman et al., 2013). At later stages of vegetative growth, HS reduces plant height, leaf area, and leaf, stem, pod, root, and total biomass (Kumar et al., 2013). Leafy vegetables require proper growth and development of vegetative parts for realizing only the yield but also the quality. In 45-day-old cabbage plants exposed to 40°C for 6, 12, 24, 48, or 72 h, HS caused loosening or bolting of heads, smaller and tighter heads, and rougher leaf texture (Chang et al., 2016). Likewise, in 30-day-old cauliflower plants exposed to 40°C for 6, 12, 24, 48, 72, or 96 h, HS caused uneven heads, puffy buds, yellow eyes, narrow leaves, reduced leaf growth, and reduced petiole-to-blade ratio (Lin et al., 2015). HS (34.5°C) further delayed the curd induction stage and decreased the chlorophyll content in cauliflower plants; effects were more distinct in heat susceptible genotypes where they were unable to develop curd at high temperature and continued their vegetative growth until temperature fall below 30°C (Aleem et al., 2021). Exposing 4- to 5-leaved lettuce seedlings to 42/37°C for 3 days reduced seedling germination and caused tip burn, rib discoloration, and bolting (Jenni and Yan, 2009; Han et al., 2013). In spinach exposed to 35°C for 21 days, HS decreased seed germination (Chitwood et al., 2016). In potato, high temperature (30–40°C) inhibited tuber development and blocked the tuberization signal (Reynolds and Ewing, 1989). Potato plants exposed to 30/20°C (day/night) for 1 week had reduced yields by 16% compared to plants grown at 22/16°C due to decreased carbon transport to the sink organ (Hancock et al., 2014). Further, reduced yield has been reported in 50 potato cultivars when exposed to heat stressed conditions (35/28°C) than control conditions (22/18°C) (Zhang G. et al., 2020). Likewise, in 6–7-leaved radish seedlings exposed to 40°C for 12 and 24 h, HS affected fleshy taproot growth and development, reducing quality and yield (Zhang et al., 2013) (Figure 1).
Figure 1. A schematic representation of the effects of heat stress (HS) on vegetative and reproductive growth stages that reduce yield. Heat stress at the vegetative stage promotes leaf damage, rib discoloration in leafy vegetables, biomass reduction in food legumes, and secondary tuberization in potato. Heat stress at the reproductive stage negatively affects the overall route from Microspore Mother Cell (MMC) development to fruit setting/seed filling through pollination and fertilization. The male gametophyte is more prone to heat stress, leading to poor pollen germination, pollen load, and pollen tube growth inside the style and inability to fertilize the ovule at the required rate.
Reproductive stage is highly sensitive to HS; even a single degree increase for a few hours can be fatal for proper reproductive growth, contributing to poor yields (Prasad et al., 2017). However, studies on reproductive tissues are difficult to assess because gamete development and fertilization are major events that occur over short periods. Here, we categorize the effects of HS in vegetables during three stages of reproduction: pre-fertilization (flower bud initiation, flowering, male and female gametophyte development), fertilization (pollen dehiscence, pollination, pollen reception by stigma, pollen tube growth and fertilization), and post-fertilization events (fruit/pod set, seed development, seed filling) (Figure 2; Table 3).
Figure 2. Generalized overview of the effects of heat stress (HS) on the reproductive stage of plants, broadly categorized into three events: pre-fertilization, fertilization, and post-fertilization. Heat stress affects the flowering stage by promoting early flowering and flower bud/flower abortion. During male gametophyte development, heat stress disrupts meiosis and decreases tapetum growth, resulting in shriveled and non-viable pollen grains. During female gametophyte development, heat stress reduces style and ovary size and callose deposition, reduces stigma receptivity, and causes early embryo abortion. Moreover, immature dehiscence and malformed pollen grains result in poor pollination and fertilization. Heat stress during post-fertilization decreases the seed filling rate and disturb source–sink relations, potentially reducing yield manifold.
High-temperature stress causes flower bud abortion and abscission of reproductive organs in many crop species, including tomato (Levy et al., 1978; Pressman et al., 2002; Sato et al., 2002), common bean (Konsens et al., 1991), pea (Guilioni et al., 1997), brassica (Angadi et al., 2000), capsicum (Aloni et al., 2001; Erickson and Markhart, 2002), resulting in severe yield losses. Common bean grown at 32/27°C (from flowering to pod maturity) experienced greater abscission and drop of flower primordia (2–5 mm) and flower buds (>5 mm) than at 27/17°C (Konsens et al., 1991). In capsicum, high-temperature stress (33°C for 120 h) affected flower buds (<2.5 mm) and early pistil development less than stamen development, whereas buds (3–4 mm) during tetrad formation and dissolution were highly sensitive to elevated temperature, leading to pollen sterility (Erickson and Markhart, 2002). Flower and flower bud abscission also occurred in heat-stressed (35/15°C for 7 days at early stage) brassica species (Angadi et al., 2000). HS (32/28°C) severely affected flower initiation and development in tomato (Levy et al., 1978; Sato et al., 2002). HS (32/26°C for 8 days before anthesis) in capsicum reduced and altered sucrose mobilization and utilization by flower buds and flowers, resulting in fruit drop and abscission and thus reducing yield by 17% compared to normal sown (28/22°C) (Aloni et al., 2001).
HS during flowering reduces flower numbers by damaging flower organs, reducing yield (Morrison and Stewart, 2002). HS also decreases the number of flowering branches and thus flower numbers per plant (Harsant et al., 2013). Damage to flower organs has been reported in many crops, including chickpea (Tickoo et al., 1996), common bean (Suzuki et al., 2001; Omae et al., 2012), and mungbean (Kaur et al., 2015). Early flowering and flower abortion are other impacts of HS, as reported in pea (Guilioni et al., 1997), tomato (Sato et al., 2004), common bean (Omae et al., 2012), and mungbean (Sharma et al., 2016).
Threshold temperatures needed to impose damages in reproductive tissues are less than the one needed to cause injury to vegetative tissues. Male gametophytes are more sensitive to HS than female gametophytes, with lower threshold temperatures than vegetative tissues. HS damage can occur pre-pollination or post-pollination, impairing fertilization and ultimately reducing seed set (Sage et al., 2015). Pre-pollination events that are highly susceptible to high temperature are (1) meiosis I and meiosis II of the microspore mother cell (Young et al., 2004), (2) development and subsequent dissolution of the tapetum layer (Farooq et al., 2017), and (3) exine and intine formation (Nahar et al., 2016). Post-pollination events affected by HS are (1) pollen load, (2) pollen germination, (3) pollen tube growth, and (4) fertilization (Hedhly et al., 2009; Sita et al., 2017). The sensitivity of male gametophytes to HS varies according to plant species (Li et al., 2018).
HS reduced fertility of microgametophytes in brassica (Rao et al., 1992) and impaired meiosis in tomato, damaging pollen germination and pollen tube growth (Foolad, 2005). In soybean, HS reduced pollen production, germination, tube elongation, and impaired pollen development (no apertures and disturbed exile ornamentation) (Salem et al., 2007; Nahar et al., 2016; Djanaguiraman et al., 2019). In capsicum, HS produced shrunken and empty microspores without an exine layer (Erickson and Markhart, 2002). Shriveled pollen grains under HS may be due to decreased starch accumulation in anther walls and pollen grains reducing soluble sugars for their development (Pressman et al., 2002).
Female gametophytes are relatively more tolerant to HS than male gametophytes (Hedhly, 2011). HS impairs megaspore mother cell development by impeding meiosis, reducing pistil size, reducing stigma receptivity due to poor pollen adhesion, reducing stigmatic papillae for holding pollen grains, interrupting nutrient transport from style to pollen impeding pollen tube germination and growth, as noticed in chickpea (Kaushal et al., 2016), bean (Porch and Jahn, 2001) and cowpea (Ahmed et al., 1992). HS, reduced callose deposition in lentil styles (Bhandari et al., 2017), reduced the amount of attractants from ovule synergids cells that misguide the pollen tube (Saini et al., 1983) to severely affect the fertilization. Furthermore, HS damages the embryo sac and causes early embryo abortion, likely arresting fertilization; for instance, in tomato, HS exposure (40°C for 3 h) for 4 days before anthesis resulted in aborted embryos with degenerated eggs and synergids (Iwahori, 1965). Abnormalities in embryo sac development have also been observed in brassica, reducing seed set and yield (Polowick and Sawhney, 1988). HS also reduced ovule viability in common beans (Ormrod et al., 1967; Suzuki et al., 2001). Unlike, male gametophyte, detailed impacts of HS on female gametophyte organs are, however, barely known. This may be because of the reason that female gametophyte is protected inside the ovary and sheltered and difficult to reach and dissect.
High-temperature stress (>30°C) negatively impacts male and female gametophyte development, leading to poor development and deformities of reproductive tissues, limiting the fertilization process in many plant species (Saini and Aspinall, 1982; Prasad et al., 2017). HS also reported to affect the flower pollination rate in tomato resulting in low fruit set with reduced lycopene content and fruit quality (Alsamir et al., 2021) Indehiscent anthers, non-viable pollen, and poor stigma receptivity are possible causes for fertilization failure and sterility imposition in many crops, including chickpea (Kumar et al., 2013), soybean (Board and Kahlon, 2011), mung bean (Kaur et al., 2015), tomato (Pressman et al., 2002), common bean (Porch and Jahn, 2001), and capsicum (Erickson and Markhart, 2002).
High-temperature stress affects the proportion of flowers forming fruits (fruit set) (Prasad et al., 2000). HS (38/30°C) markedly decreased fruit weight (51.6%), fruit diameter (25%), fruit length (30%), and seed number per fruit (57%) in sweet pepper compared with normal temperature (33/21°C) (Thuy and Kenji, 2015). Peet et al. (1998) reported that high temperature (29°C) decreased fruit number (10%), total fruit weight/plant (6.4%) and seed number/fruit (16.4%) in male fertile tomatoes compared to optimum temperature (25°C). The high temperature impaired pollen development and release, leading to reduced fruit set in male-fertile tomatoes compared with male-sterile lines. Similarly, fruit set and fruit size in tomato plants declined at 29/23°C compared to 24/18°C (Saha et al., 2010). HS seriously damaged fruit set in tomatoes exposed to 40°C for 4 h before anthesis and reduced the pollen germination from 79.5% (at 30/17°C) to 30% and pod set from 63% (at 30/17°C) to 14.9% (Rudich et al., 1977). In Common bean, high temperature (32/27°C) reduced the pod set from 17 to 97%, seed set by 39–98%, and seeds/pod by 42 to 73% compared to control temperature (22/17°C) (Gross and Kigel, 1994). Similar finding on bean plants exposed to even higher temperatures (40/30°C) had fewer filled pods, parthenocarpic pod development, sickle-shaped pods, reduced seed size, and fewer seeds/pod and total seeds than control condition (Prasad et al., 2002; Soltani et al., 2019). In peas, high temperature (32°C for 6 h) at the reproductive stage increased the abortion rate of reproductive organs (flower buds and young pods) from 20 to 50% which reduce seed yield (Bueckert et al., 2015).
Seed formation and seed filling are the last phases of the life cycle of seed plants; and; HS drastically affects seed development and the seed-filling phase, increasing the fraction of abnormal and shriveled seeds (Sehgal et al., 2018). In common bean, a linear relationship between temperature and grain weight was recorded resulting in a significant decrease in seed weight, i.e., 0.07 g per °C when temperature was raised beyond 31/21°C (Prasad et al., 2002). Seed development starts from cell division and, when seed cells are fully formed, storage reserves start to accumulate (Egli, 1998). Direct effects of HS on division and size of endosperm cells are well-documented (Commuri and Jones, 2001). Reduced division and size of endosperm cells results in accumulation of fewer carbohydrates, proteins, lipids, and starch accumulate in developing seeds. HS also accelerates the rate and duration of seed filling, resulting in abnormal seeds and significant yield losses (Farooq et al., 2017). Not only yields, HS affects seed quality characteristics, reducing seed number and size, degrading nutrient composition, and decreasing seed viability, through impaired nutrient uptake, assimilate partitioning, and translocation (Prasad et al., 2008). Starch, proteins, and lipids are the principal reserves transferred from the main plant to developing seeds (Alencar et al., 2012), but HS limits their synthesis and translocation during seed filling, affecting grain quality (Farooq et al., 2017), and could be due to decreased enzyme activity. The activity of starch synthesizing enzymes, such as starch synthase, sucrose synthase, and invertase, decrease under HS, as reported in pea (Smith and Denyer, 1992) and chickpea (Kaushal et al., 2013). Similarly, HS disrupts seed storage proteins, such as β-glycocynin and globulin 11S in soybean (Hashizume and Watanabe, 1979; Iwabuchi and Yamauchi, 1984), and sucrose-synthesizing enzymes and proteins that aid in sucrose translocation. Reduced sucrose synthase activity affects the sucrose and starch ratio, decreasing the transfer of soluble carbohydrates to developing ovules, as reported in pea (Jeuffroy et al., 1990) and cowpea (Ismail and Hall, 1999). Reduced crop duration and seed filling has been correlated with an inefficient light capture ability (canopy growth rate) in small plants, decreasing the photosynthetic rate and thus seed size, as reported in soybean (Board and Kahlon, 2011). Prasad et al. (2002) reported a linear relationship between temperature and grain weight in common bean, with seed weight decreasing by 0.07 g per °C at temperatures above 31/2.
HS disrupts the organization of the plasma membrane by increasing unsaturated fatty acids, thus making the membrane more fluid (Hofmann, 2009), and influencing the cellular functions by initiating a signal cascade (Firmansyah and Argosubekti, 2020; Hassan et al., 2021). HS also accelerates the kinetic energy and movement of various molecules through the membrane. Further, protein denaturation and altered tertiary and quaternary structure of membrane proteins increase membrane fluidity (Savchenko et al., 2002). Thus, HS disturbs primary processes of plant-like photosynthesis and respiration due to increased permeability or solute leakage from cells (Figure 3). Therefore, cell membrane thermostability trait used to evaluate HS on plants and identify heat-tolerant and heat-sensitive genotypes; for example, in soybean (Martineau et al., 1979), potato (Chen et al., 1982), and cowpea (Ismail and Hall, 1999). The effectiveness of cell membrane thermostability assays depends on the tissue type and stress type used for plant adaptation. It is also unknown whether membrane thermostability is linked to other plant characteristics that confer heat tolerance, such as growth and yield.
Figure 3. Model representing morphological, physiological, biochemical, and molecular characteristics of plants under heat stress. Morphological damages at vegetative and reproductive stages can be visualized as direct measures of plant stress. At the physiological level, these damages are associated with leaky plasma membrane, altered transpiration, chlorophyll damage, reduced photosynthesis, respiration, and nodulation rate. Disturbed physiological processes can promote oxidative stress damage observed through stress indicators like increased malondialdehyde (MDA) and hydrogen peroxide (H2O2) content. Protein damage and impaired carbon and nitrogen metabolism due to impaired enzymatic activities further exaggerate stress levels at the biochemical level. Heat shock proteins (HSPs), heat shock factors (HSFs), and quantitative trait loci (QTLs) related to heat stress responses of plants may play a key role in the plant adaptation. HSPs and HSFs have a central role in regulating the activity of various genes that amplify the production of antioxidants and osmolytes and are helpful governing thermotolerance.
Photosynthesis is highly sensitive to HS and photosynthetic activity reduces drastically under HS. Studies have detailed the affected photosynthetic mechanisms that ultimately reduce the photosynthetic capacity of plants (Berry and Bjorkman, 1980; Sharkey, 2005). Thylakoid reactions, Rubisco activity, and photosynthetic pigments are generally disturbed by HS. HS primarily affects the physical state and structure of the thylakoid membrane by triggering thylakoid leakiness and unstacking thylakoids, damaging the D1 protein of PSII (Sharkey, 2005). To counterbalance these reactions, zeaxanthin synthesis increases, affecting the normal state of thylakoids (Havaux, 1996). HS disturbs the electron flow between the two photosystems (PSI and PSII) and reduces the photosynthetic efficiency of plants. HS also accelerates the phosphorylation of light-harvesting complex (LHCII) and disconnects it from PSII core complex, thus decreasing its turnover rate, but increasing the turnover rate of PSI (Wise et al., 2004). HS dephosphorylates core proteins (D1, D2, and CP43), deactivating PSII (Yamamoto et al., 2016). HS alters the fluorescence induction parameters, measured as the Fv/Fm ratio; this ratio helps to determine the quantum efficiency of PSII and indicates the rate of linear electron flow and overall photosynthetic performance of plants (Jamil et al., 2007). HS decreased chlorophyll a fluorescence, PII quantum yield, photochemical quenching, and increased respiration rate in soybean (Djanaguiraman et al., 2013a).
Along with thylakoid reactions, HS triggers the deactivation of Rubisco (Crafts-Brandner and Salvucci, 2000). Rubisco being dual enzyme catalyses the carboxylation of ribulose−1-5-bisphosphate in the photosynthetic Calvin cycle and oxygenation in the photorespiratory pathway; the ratio between two reactions governs the photosynthetic efficiency of plant. But the elevated temperature inhibits the CO2 fixation and increases the oxygenase activity and reduces photosynthetic rate (Crafts-Brandner and Salvucci, 2000). Rubisco activation is not only associated with pH and Mg2+ concentration of stroma but also with Rubisco activase (RA); an ATPase. RA induces the activation of the Rubisco by increasing the proportion of its active sites and brings conformational changes that allow CO2 and Mg2+ for activation and carbamylation. High temperature can disturb the pH and Mg2+ concentration of stroma, interfering with the carbamylation step of Rubisco activation (Weis, 1981a,b) and also caused RA dissociation because of its poor structural stability and heat labile nature (Demirevska-Kepova and Feller, 2004). Few reports have noticed that heat stress affects the photosynthesis through heat sensitivity of Rubisco and RA activity, for instance in tomato, heat stress (40°C for 8 h for 6 days to 3 weeks old plant) decreased the accumulation of Rubisco enzyme's isoforms (Parrotta et al., 2020), as in pea (Haldimann and Feller, 2005), potato (Cen and Sage, 2005) and spinach (Zhao Q. et al., 2018).
Pea plants exposed to HS reduced chlorophyll biosynthesis due to the destruction of various enzymes involved in biosynthetic pathways (Dutta et al., 2009; Aleem et al., 2021). HS decreased the activity of first enzyme of the biosynthetic pathway, 5-aminolevulinate dehydratase, in cucumber (Tewari and Tripathy, 1998). Decreased chlorophyll content, Chl a/b ratio, and chlorophyll/carotenoid ratio have been reported in many crops under HS (Aien et al., 2011) (Table 4). Similarly, HS stress causes pre-mature leaf senescence in soybean leaves which results in decreased photosynthesis primarily due to decreased chlorophyll content, higher reactive oxygen species, lower antioxidants, and increased thylakoid membrane damage (Djanaguiraman and Prasad, 2010). HS increased ethylene production in leaves which was one of the reasons of premature leaf senescence in soybean (Djanaguiraman and Prasad, 2010). Detailed anatomical studies showed that HT stress significantly increased the thicknesses of the palisade and spongy layers and the lower epidermis (Djanaguiraman et al., 2013a). In addition, HT stress damaged the plasma membrane, chloroplast membrane, thylakoid membranes; mitochondrial membranes, cristae, and matrix were distorted which led to decreased photosynthesis (Djanaguiraman et al., 2013a) (Figure 3).
Nitrogen is one of the main nutrients required by the plant for proper growth, development and productivity. It is the constituent of various important organic compounds like amino acids, proteins, nucleic acids, enzymes, and the chlorophyll molecule (Christophe et al., 2011). Nitrogen content in the plant measured as nitrate, ammonium ions, and proteins. Besides performing basic roles in plants, its metabolism is also very crucial for heat tolerance because it increases the osmolyte content and antioxidant enzyme activity (Ru et al., 2022). Studies have also shown their role in promoting the HSP production (Heckathorn et al., 1996). Osmolytes like proline and quaternary ammonium compounds, being nitrogen rich and accumulate in plants under heat stress conditions (Rivero et al., 2004). Ammonium ion and proline accumulation confer heat tolerance to tomato and promoting higher biomass production (Rivero et al., 2004). During the reproductive period, nitrogen concentration successively increases when temperatures rise for example in pea, when high temperature occurs during or after flowering seed N concentration is increased (Larmure et al., 2005). Similarly, in soybean, seed N concentration increases during the reproductive period at temperature 40/30°C (Thomas et al., 2003). Increases in the accumulation of proteins; level of globulin protein storage causing a reduction of the albumin/globulin content in mature seeds (Hurkman et al., 2009). In pea, the final level of vicilin storage proteins was higher under heat stress (Bourgeois et al., 2009). However, in tomato roots, it has been reported that HS disturbs enzymes involve in nitrogen metabolism (nitrate and ammonium assimilation) thereby decreasing total protein content and level of nutrient uptake and assimilation (Giri et al., 2017). Further, studies on the contrasting genotypes of brassica revealed that HS (40/30°C for 7 days) negatively affected the activities of nitrogen assimilation enzyme including Glutamate synthase (GOGAT), glutamine synthetase (GS), glutamate dehydrogenase (GDH), more in heat sensitive genotype (WS-6) as compared to heat tolerant genotype (WS-1). These enzymes help in possessing better photosynthetic nitrogen use efficiency (Yuan et al., 2017).
Symbiotic nitrogen fixation in leguminous crops depends on the presence of appropriate Rhizobium species in the vicinity of root zone, however, almost all processes starting from rhizobial survival to host infection and nitrogen fixation depend mainly on the environmental factors, such as soil temperature (Bordeleau and Prévost, 1994). High temperature interferes with almost all processes of symbiotic nitrogen fixation, directly as well as indirectly, soil temperature affects not only the rhizobial survival in the root zone but also the exchange of molecular signals between two symbiotic partners (Alexandre and Oliveira, 2013). Rhizobial strains have an optimum soil temperature (25–30°C) for their growth and nitrogen fixing ability and Rhizobia are greatly affected by high soil temperature. However, optimum temperature varies with the crop species, for instance, in soybean, weak rhizobia were formed at 40°C and no rhizobia were isolated at 45°C (Chen et al., 2002). HT interferes directly with nodule development as it hampers nodule development and increases nodule senescence (Aranjuelo et al., 2007). HS affects indirectly the nitrogen fixation by inhibiting the formation of root hairs, infection thread formation, reducing the nodulation sites, adherence between bacteria and root hair (bacterial infection), and bacteroid formation (Zahran, 1999; Hungria and Vargas, 2000; Alexandre and Oliveira, 2013).
Elevated temperature also affects nodule growth rate, nodule size, and nodule fixation ability, as reported for common bean exposed to HS (35 and 38°C/8 h/day) at the flowering stage (Hungria and Franco, 1993). Another study showed that at 47°C temperature no nodules were formed in common bean (Karanja and Wood, 1988). Studies have shown that nodulation ability varies inversely with temperature, and legume species differ in their temperature endurance; for instance, common bean is more sensitive to temperature stress than cowpea and soybean for nitrogen fixation (Piha and Munns, 1987). In cowpea, the optimum temperature for nodule growth and development is 30–36°C; temperatures above 40°C lead to fewer or no nodules (Day et al., 1978). In common bean, nodules that formed at high temperature (≥35°C) were inefficient and unable to fix nitrogen (Hungria and Franco, 1993). Piha and Munns (1987) noted that nodules formed at 35°C were small and had low nitrogenase activity. The optimum temperature for nodule growth is 20°C for pea and 25–30°C for soybean (Michiels et al., 1994). HS decreased nodulation ability in mungbean (Sharma et al., 2016). In common bean, HS affected nitrogen fixation due to decreased activity of enzymes involved in nitrogen metabolism, such as dinitrogenase complex, glutamine synthetase (GS), and glutamine synthase (GOGAT), decreasing the concentration of ureids-N in nodules and xylem sap (Hungria and Kaschuk, 2014). Prasad et al. (2000) observed that high soil temperatures (35°C) significantly decreased number of nodules and nodule dry weight per plant compared to optimum soil temperature (25°C) in peanut.
C.N ratio: Plant growth and defense are both fuelled by compounds synthesized from a common pool of carbon and nitrogen, implying the existence of a competition for carbon and nitrogen allocation to both metabolisms. The ratio of carbon to nitrogen (C: N) of an organ is often regarded as a convenient indicator of growth and quality. Almost a century ago, plant nutrition was considered a crucial factor in controlling flowering time. According to Klebs (1913), a high endogenous carbon: nitrogen ratio promotes flowering, while a low carbon: nitrogen ratio promotes vegetative growth. Inferred from the fact that (a) conditions favoring photosynthetic CO2 fixation generally accelerate flowering and (b) high nitrogen intake (fertilizers) might delay or reduce reproductive development in some plants (Bernier et al., 1981). The flowering percentage increased when NH4NO3 concentration decreased from 16.5 to 8 g l−1, in tomato plant (Dielen et al., 2001). Royer et al. (2013) revealed that C:N ratio in the pool of resources in the total plant, were correlated with the concentrations of diverse compounds of the primary and secondary metabolisms in young tomatoes. Under HS, Peet et al. (1997) found that in tomato plants, the carbon and nitrogen metabolism get imbalanced, and stem and petiole elongation consume too much nutrients, which in turn reduces the dry matter storage of the plant, affecting tomato quality and yield. Soil mixed with dry powder of Sesbania plant (leaves + tender stems; C: N ratio 15.4) plays effective role in enhancing resistance and resilience (stability) of soil microbial activity against heat stress (Kumar et al., 2014). Heat stress may accelerate leaf senescence and increase respiration rate which consequently decreases plant N and C availability for seeds and shorten the duration of seed filling period in soyabean (Egli and Wardlaw, 1980). Thus, balanced C:N ratio plays an important role in plant physiological process. Similarly, Larmure et al., 2005 demonstrated that the lower seed N concentration in pea plant at the average temperature range (13–23°C) can be explained by prolonged duration of the seed-filling associated with the lower seed N concentration, higher C availability for the seeds. Because the rate of seed N accumulation per degree-day mainly depends on N availability to seed filling, the rate of N accumulation was higher at 25/20°C than at lower temperature. HS reduces seed size and modifies the C:N ratio in the period of seed formation in pea (Guilioni et al., 2003).
Severe HS generates ROS, such as hydrogen peroxide (H2O2) and superoxide radical (), as byproducts of the aerobic metabolism, which adversely affect cellular metabolism, such as lipid membrane peroxidation, and damage nucleic acids and proteins (Bita and Gerats, 2013). Plants respond to ROS production by activating enzymatic and non-enzymatic ROS scavenging systems (Bita and Gerats, 2013). The main ROS scavenging enzymes are superoxide dismutase (SOD), catalase (CAT), peroxidase (POX), ascorbate peroxidase (APX) glutathione reductase (GR), whereas non-enzymatic chemical are ascorbic acid (ASC) and glutathione (GSH) (Suzuki et al., 2012). SOD helps scavenge whereas CAT and POX degrade H2O2. Elevated levels of these antioxidants are crucial in imparting thermotolerance in plants (Awasthi et al., 2014). In soybean, ROS accumulation (mainly H2O2 and ) due to HS is associated with decreased enzyme activities of various antioxidants (Djanaguiraman et al., 2005, 2013a). Similarly, GR and CAT activities decreased in common bean under oxidative stress (Babu and Devaraj, 2008). Likewise, decreased APX and GR expression occurred in mungbean exposed to HS (Sharma et al., 2016). However, relationship between antioxidant enzymes and HS is far more complex in tomato where activity of SOD, APX increased and CAT activity decreased (Zhou et al., 2014). This complexity was also evident in capsicum where, NADPH oxidase and CAT activity increased at high temperature (Gulen et al., 2012). In chickpea, tolerant genotypes had higher SOD, CAT, APX, and GR activity than sensitive genotypes under HS (40/30°C and 45/35°C) (Kumar et al., 2013). Moderate HS increases the expression of various enzymatic antioxidants, while severe HS suppresses it (Wilson et al., 2014).
In addition to antioxidants, plants endure HS by activating major defense mechanisms which are mainly comprised of increased production of heat shock proteins (HSPs) and compatible solutes (Sakamoto and Murata, 2002; Wahid et al., 2007; Mittler et al., 2012; Khan and Shahwar, 2020). HSPs are the molecular chaperones that protect the misfolded proteins from irreversible aggregation, sorting, translocation, and degradation, important for establishing cellular homeostasis in normal and stressed conditions (Vierling, 1991). There are five classes of HSPs categorized according to their molecular weight: HSP100, HSP90, HSP70, HSP60, and Small HSP (sHSP), and located in the cytoplasm as well as cellular orgenelles, nucleus, chloroplast, mitochondria, and endoplasmic reticulum (Wang et al., 2004). Different chaperone families though have a peculiar role but coordinate cellular homeostasis. Chaperones also maintain crosstalk with signaling molecules, antioxidants (acerbate peroxidase), and osmolytes (trehalose, proline, glycine betaine) (Wang et al., 2004; Kang et al., 2022). Various reports have confirmed accumulation of all HSP families in different vegetables and food legumes under HS, with greater accumulation of sHSPs than other HSPs, as reported for spinach (Guy and Li, 1998), tomato (Preczewski et al., 2000), soybean (Ortiz and Cardemil, 2001), common bean and cowpea (Simões-Araújo et al., 2003), potato (Ahn et al., 2004), cabbage (Park et al., 2013), pea (Talalaiev and Korduym, 2014), faba bean (Kumar et al., 2015), capsicum (Li et al., 2015), chickpea (Meena et al., 2017), and broccoli (Lin et al., 2019). Accumulation of these proteins helps plants to re-establish homeostasis under HS conditions. Hence, the expression level of HSPs and HSFs could be manipulated genetically to improve heat tolerance ability. Overexpression of HSPs facilitates transformed cells to endure HS better than non-transformed cells (Grover et al., 2013); for instance, overexpression of sHSP (HSP21) in transgenic tomato imparts stable PSII, shielding photosynthesis from temperature-dependent oxidative stress and accumulating more carotenoids under HS (Neta-Sharir et al., 2005). Furthermore, overexpression of HSFs facilitates the expression of HSPs; for example, overexpression of HSFA1 in transgenic soybean enhanced the expression of GmHSP70 leading to thermotolerance (45°C) (Zhu et al., 2006). Similarly, overexpression of transcription factor (CaWRKY40) enhanced thermotolerance in capsicum (Dang et al., 2013).
The role of various osmolytes, including proline and glycine betaine, in imparting heat tolerance is well-documented (Sakamoto and Murata, 2002). Osmolytes are low molecular weight compounds that can buffer cellular redox potential under HS. Proline is a well-studied osmolyte, concentration of which increases by several-fold under stress conditions. A heat-tolerant cabbage genotype accumulated more proline (and soluble sugars and antioxidants) than a sensitive genotype (Song et al., 2019). Similarly, Paul et al. (2014) even suggested using increased proline and soluble sugars in potato under HS can used as markers for selecting heat-tolerant genotypes. Increasing HS gradually increased proline and soluble sugar contents in lettuce seedlings, indicating heat tolerance (Han et al., 2013). The role of proline in thermotolerance was also confirmed using exogenous proline applications. Kaushal et al. (2011) noted that exogenous treatment of proline induced thermotolerance in chickpea by protecting the enzymes involved in carbon and antioxidant metabolism. Glycine betaine is another compound that confers heat tolerance; Aien et al. (2011) suggested that glycine betaine imparts heat tolerance in potato genotypes under HS conditions.
Heat avoidance through transpiration cooling is the best strategy adopted by plants to minimize the losses (Julia and Dingkuhn, 2013) Under moderately HS conditions, plants can accelerate growth to promote plant thermonastic responses and architectural changes to move susceptible parts away from soil heat flow or to improve evaporative cooling (Havko et al., 2020). In soybean, tomato, or cabbage, moderately high ambient temperature induces hypocotyl elongation, and tomato displays leaf hyponasty (Quint et al., 2016; Casal and Balasubramanian, 2019; Vu et al., 2019). Pea canopies architecture and leaf type as traits of heat resistance can avoid heat and maintain a lower canopy temperature as leafed cultivars have greater leaf surface area and likely greater transpirational cooling, assuming soil moisture availability and an adequate root system (Tafesse et al., 2019). Another study showed that the leaf movement capacity in beans was shown to function in direct sunlight avoidance and benefited the plant by protecting it against photoinhibition and by maintaining leaf temperatures lower than the air temperature (Pastenes et al., 2004). Thus, as novel donors with higher heat tolerance or escape provides, there is an ample evidence for systematic exploration of wild species and accessions (Prasad et al., 2017) for introducing these traits.
Heat tolerance is a polygenic trait greatly influenced by environmental changes (Blum, 2018). HS effects are stage-specific, with the response at one stage differing from the response at another. Breeders employ various techniques to minimize the impact of an unpredictable environment on crops. Conventional breeding is the oldest but most prevalent method, primarily based on selecting phenotypic plant characters (Acquaah, 2015). In recent decades, new techniques have emerged based on morpho-physiological plant characters merged with conventional breeding methods to screen superior varieties. These methods exploit inbuilt plant properties to cope with HS and assist in selecting heat-tolerant genotypes. Screening germplasm of various vegetable crops using various physiological traits linked to heat tolerance would be useful for breeding programs focused on developing HS tolerant genotypes. Although there are several methods or traits used for screening, some of the most common are discussed.
The stay-green character is the plant's ability to retain chlorophyll and remain green for longer to sustain photosynthesis, especially during seed filling (Thomas and Howarth, 2000). However, the adverse impacts of HS cause leaves structural changes and chlorophyll degradation and it ultimately induces premature, leaf senescence (Djanaguiraman and Prasad, 2010; Jha et al., 2014). Moreover, the onset of HS during seed filling affects various physiological processes, including increased leaf senescence (chlorophyll loss), altered source–sink relationship, and decreased assimilation of reserve food material in developing seeds, limiting plant yield (Luche et al., 2015). Therefore, delayed leaf senescence may be associated with heat tolerance, enabling plants to maintain their photosynthetic ability (Lim et al., 2007). High chlorophyll and carotenoid contents in leaves improve the photochemical efficiency of plants and reduces ROS concentration in plants such as tomato (Zhou et al., 2015) and pea (Tafesse, 2018).
In addition, the stay-green character positively correlates with canopy temperature depression. Stay-green genotypes have lower canopy temperatures due to transpirational cooling than non-stay-green genotypes (Kumari et al., 2013). In addition to these modifications, HS also causes plant morphological and architectural modifications like leaf hyponasty (measured through leaf angles), leaf petiole elongation, small and thin leaves, that are helpful for the plants to keep their canopies cool. For instance, the cucumber species have hyponastic leaves (Park et al., 2019) and reduced leaf size is found in potato (Tang et al., 2018) and capsicum species (Utami and Aryanti, 2021) under heat stress conditions. These processes involve various signaling cascades that mediate the developmental shaping for environment adaptation in plants (Gil and Park, 2019). This trait is also associated with grain yield and quality and abiotic stress tolerance (Kamal et al., 2019). Hence, the stay-green trait is essential for improving crop yield and useful for imparting heat tolerance (Joshi et al., 2007; Kusaba et al., 2013), and thus may be an important genetic trait for improving crop yield under HS.
Canopy temperature depression (CTD) is usually measured as the difference between air and canopy temperature, indicating the plant's ability to lower its foliar temperature by transpirational cooling, as measured by an infrared thermometer. CTD also reflects plant water status and is influenced by the plant's ability to extract water and the transpiration difference between air and plant. Accordingly, CTD has been used to select heat-tolerant and drought-tolerant genotypes. Plants that can maintain cooler canopies during seed filling can tolerate high-temperature stress (Munjal and Rana, 2003). Heat-tolerant varieties of capsicum (Gajanayake et al., 2011) have been selected based on the stay-green trait. In soybean, there is a direct relationship between CTD, canopy greenness, photosynthetic rate, and yield (Kumar et al., 2017). Thus, the CTD trait can be used as a critical genetic trait for crop improvement aimed at increased yields at the vegetative stage.
HS is amounts of sensed by cell membranes of leaf tissues, weakening cell membrane integrity/rigidity due to an increased degree of unsaturated fatty acids that increase membrane fluidity. This may change membrane permeability and disturb the selective transport of molecules across the membrane, affecting cellular homeostasis (Marcum, 1998). HS can directly affect membrane integrity through photochemical modifications during photosynthesis or ROS (Bita and Gerats, 2013). Cell membrane thermostability (CMT) can be evaluated with an electrolyte leakage test for screening crops for heat tolerance. The method is simple, quick, and inexpensive compared with whole-plant screening and can be used to assess plant tissue responses at the vegetative stage (Yeh and Lin, 2003). Electrolyte leakage is measured using a conductivity meter, with higher conductivity values indicating higher membrane damage (Nyarko et al., 2008). The CMT test has been used to screen heat-tolerant varieties of many crops, including soybean (Martineau et al., 1979), potato (Nagarajan and Bansal, 1986), cowpea (Ismail and Hall, 1999), cabbage (Nyarko et al., 2008), cauliflower (Aleem et al., 2021) chickpea (Kumar et al., 2013), mungbean (Sharma et al., 2016), and cucumber (Ali et al., 2019).
Chlorophyll fluorescence—expressed as the Fv/Fm ratio (Fv: variable fluorescence; Fm: maximum fluorescence)—is used to detect the state of PSII function in terms of the energy absorbed by PSII in chlorophyll and damage to photosynthetic apparatus by excess light in vivo (Maxwell and Johnson, 2000). Chlorophyll fluorescence is a rapid, reliable, and inexpensive procedure for predicting photosynthetic performance under HS. Reduced Fv/Fm values indicate damage to the light-harvesting complex (Moradpour et al., 2021). Chlorophyll fluorescence has been used to select heat-tolerant varieties of sweet pepper (Hanying et al., 2001), common bean (Stefanov et al., 2011), chickpea (Kaushal et al., 2013), mungbean (Kaur et al., 2015), tomato (Zhou et al., 2015; Poudyal et al., 2018), and okra (Hayamanesh, 2018). Makonya et al. (2019) showed that tolerant chickpea genotypes maintain higher Fv/Fm during HS than sensitive genotypes, and Fv/Fm positively correlates with grain yield in the field. Killi et al. (2020) reported the retention of PSII function at elevated temperature positively correlated with antioxidant activity, confirming the applicability of this trait for selecting heat-tolerant varieties.
Relative water content indicates the hydration status of plants and reflects the balance between leaf water supply and transpiration rate. Hence, it can measure leaf water deficit and the degree of damage under HS (Mullan and Pietragalla, 2012). High transpiration increases water loss, which can cause tissue dehydration and wilting (Mazorra et al., 2002). Therefore, genotypes that can maintain turgid leaves will minimize HS effects and have numerous physiological advantages. Gowda et al. (2011) suggested using RWC as selection criteria for improving yield under HS. High temperature (40–42°C) at the vegetative and reproductive stage gradually reduced the RWC of capsicum genotypes, more so at the reproductive stage (Puneeth, 2018). RWC has been used to select heat-tolerant genotypes of mungbean (Sharma et al., 2016), capsicum (Puneeth, 2018), common bean (Chavez-Arias et al., 2018), lentil (Sita et al., 2017), tomato (Zhou et al., 2018), cucumber (Ali et al., 2019), and potato (Handayani and Watanabe, 2020) where genotypes with high RWC under HS were rated as heat tolerant.
Stomatal conductance measures the rate of carbon dioxide entering or water vapor exiting stomata. This change in transpiration rate facilitates changes in leaf temperature and water potential (Farquhar and Sharkey, 1982). Leaf stomatal conductance is often recognized as an important trait for evaluating differences in response to changing environments. It can be used to determine trait such as photosynthetic CO2 uptake, leaf temperature, and water loss (Vialet-Chabrand and Lawson, 2019). Decreased stomatal activity under a changing environment can significantly affect plant growth and biomass (Way and Pearcy, 2012). In vivo stomatal conductance can be measured with a steady-state leaf porometer and gas exchange. HS increases in vivo adaxial stomatal conductance relative to the control (Sharma et al., 2016). Low stomatal responses under stress can limit photosynthetic rate and cause unnecessary transpiration, decreasing plant water use efficiency and productivity (Matthews et al., 2018). This phenomenon has been used to select heat-tolerant genotypes of sweet pepper (Hanying et al., 2001); tomato (Camejo et al., 2005; Abdelmageed and Gruda, 2009), chickpea (Kaushal et al., 2013), and mungbean (Kaur et al., 2015). While many studies have successfully used one of the traits above to select heat-tolerant genotypes, combining multiple traits would reflect heat tolerance better than relying on a single trait.
Fruit yield in vegetables crops is a function of fruit numbers and fruit size. There is a strong and positive correlation between fruit-set and gamete viability (Prasad et al., 2017). Gamete functions (pollen and ovule) is the most important factor for fruit-set under HS. In tomato, fruit-set has been shown to correlate with pollen viability (Firon et al., 2006). In general, heat tolerant genotypes maintain higher pollen viability compared to heat susceptible genotypes (Dane et al., 1991). Gamete functions depend on its viability, which can be evaluated by viability assays like staining, in-vitro and in-vivo germination of pollen, and ovule function. Genotypes are known to differ in gamete viability under HS stress. Singh et al. (2015) concluded from their research on tomato that traits like fruit-set and pollen viability could be used as a strategy to screen genotypes for HS. In general, the combination of gamete viability and fruit-set provide tolerance to HS (Paupière et al., 2017b; Pham et al., 2020). Similarly observations were also made on peppers (Aloni et al., 2001; Reddy and Kakani, 2007).
Cardinal temperatures (Tmin, Topt, and Tmax) for pollen grain germination can be used to screen germplasm for HT stress tolerance. Results from in-vitro studies showed that genotypes varied in response to temperature for cardinal temperatures, and the differences in cardinal temperatures were mainly responsible for tolerance/susceptibility of genotypes to HT stress in soybean (Djanaguiraman et al., 2019) and peanut (Kakani et al., 2002). The genotypes having higher ceiling temperature (Tmax) for pollen germination values tend to be HT tolerant in most cases. Cardinal temperature for pepper were different among susceptible and tolerant cultivars (Reddy and Kakani, 2007) and can be used to identify temperature tolerant or sustainable genotypes of pepper (Gajanayake et al., 2011). All the aforementioned traits based on leaf function are used collectively to select heat tolerant cultivars. Though many studies have successfully employed one trait for selection of heat tolerant genotypes, a combination of these traits reflects a better status of heat tolerance rather than relying on a single trait.
Various modern genome-based technologies can be used to introduce genetic variations for HS tolerance into plants. Under high-temperature stress, plants activate a complex chain of molecular responses, including heat-stress-responsive genes that control primary and secondary metabolism, transcription, translation, and lipid signaling, or protein modifications, including phosphorylation HS transcription factors (HSFs) that regulate differential expression of HSPs (Janni et al., 2020). HSPs and HSFs are key players in the acquisition of the HS response. HSFs are mainly involved in sensing and relaying the HS signal to activate the response (Mittler et al., 2012). Genome-wide associated studies (GWAS) have been conducted on a few vegetable crops to search for novel genes and transcription factors associated with heat tolerance. Genomic studies on cabbage (Brassica rapa ssp.) disclosed the role of differentially expressed long non-coding (lncRNAs), mRNAs, and microRNAs. Their expression is associated with phytohormones such as salicylic acid (SA) and brassinosteroids (BRs), possibly involved in heat tolerance. Of these, 25 lncRNAs were co-expressed with ten heat-responsive genes (Wang A. et al., 2019). NAC, a large family of transcription factors, was analyzed in cabbage; 188 genes were identified that play a major role in resistance to high-temperature stress (Ma et al., 2014). Analysis of the potato Hsp 20 gene family revealed 48 putative Hsp20 (StHsp20) that accumulated under heat treatment. Different levels of these transcripts were upregulated during different HS exposures. The transcription of HSPs are regulated by HSFs that play an important role in imparting thermotolerance in plants (Zhao P. et al., 2018). Guo et al. (2015) characterized 35 putative Hsp 20 genes (CaHsp20) located on 12 chromosomes in thermotolerant (R9) and thermosensitive (B6) lines of pepper in four tissues (roots, stem, leaves, and flowers). Under high temperature stress (40°C), most of the CaHsp20 genes had higher expression in both lines, more so in the thermosensitive line. Chidambaranathan et al. (2018) identified 22 Hsfs in the desi (ICC4958) and kabuli (CDC Frontier) genomes of chickpea (15-day-old seedlings; heat treatment of 35 ± 2°C). Field analysis was undertaken to compare the expression pattern at the podding stage. HS at the seedling and pod development stages upregulated the expression of CarHsfA2, A6a, A6c, and B2a, indicating their role in conferring HS tolerance in chickpea. Yang et al. (2016) recorded 26 HSF (Sly HSF) genes in tomato, with HS (38°C) increasing the expression of most, especially SlyHSF-05/07/13/18/20/23/24. Expression of the SlyHSF-18 gene increased manifold compared to the control, indicating its strong response and correlation to high temperature sensitivity. Moreover, SlyHSF-02 was the main regulator for activating the heat response and acquiring thermotolerance in tomato.
Transcriptomics refers to the study of the transcriptome [entire set of transcripts (mRNA, tRNA, and rRNA, miRNA, siRNA, snRNA, snoRNA, and lncRNA)] expressed in a cell, tissue, organ, or organism. It represents all RNA synthesized, including protein-coding, non-coding, spliced, polyadenylated, and RNA-edited transcripts (Imadi et al., 2015). Transcriptomics reveals the molecular mechanism underlying the phenotype and explains how genes are expressed and interconnected (Jha et al., 2017). High throughput methods (microarray, RNA sequencing, RT-PCR) are used to analyze the expression level of multiple transcripts in different conditions. Several transcriptome studies in vegetable crops under HS have revealed the molecular basis for heat tolerance.
Transcriptome analysis in heat-stressed spinach (42°C for 15 days) revealed the expression of 4,145 transcripts (2,420 upregulated and 1,725 downregulated) in heat-tolerant and heat-sensitive genotypes (Guo et al., 2020). An enrichment analysis showed that the major metabolic difference between tolerant and sensitive genotypes was carbohydrate metabolism (Guo et al., 2020). Similarly, transcriptome analysis revealed 23,000–30,000 expressed genes in soybean seeds and differentially expressed genes (DEGs; 5–44% of expressed genes) (Gillman et al., 2019). The DEGs were measured at high temperature in mature, imbibed, and germinated seeds in a heat-tolerant (PI 587982A) and conventional high-yielding variety (S 99-11986), with 7,789 DEGs common between genotypes, 11,833 common between mature and imbibed seeds, and 13,344 common between imbibed and germinated seedlings (Gillman et al., 2019). In capsicum, seedling transcriptomics revealed 3,799 DEGs in R597 (heat-tolerant genotype) and 4,010 DEGs in S590 (heat-sensitive genotype), related to hormones, HSPs, transcription factors, and calcium and kinase signaling (Li et al., 2015). Further, R597 had higher expression of transcription factors and hormone signaling genes than S590 (Li et al., 2015). Transcriptomic analysis of heat-tolerant PS-1 and heat-sensitive H-24 tomato genotypes under HS (40°C for 1 h) revealed upregulated genes associated with protease inhibitors, HSPs, and transcription factors, manifold higher in the tolerant genotype than the sensitive genotype (Sadder et al., 2014).
Proteomic analysis in heat-stressed radish leaves (advanced inbred line NAU-08Hr-10) revealed eleven deferentially expressed proteins, of which four belonged to HSPs, four to energy and metabolism, two to redox homeostasis, and one to signal transduction (Zhang et al., 2013). Comparative proteome analysis of heat-tolerant (JG 14) and heat-sensitive (ICC16374) chickpea genotypes under HS during anthesis revealed that 482 heat-responsive proteins (related to photosynthesis, energy metabolism, and signaling molecules) were synthesized in higher amounts in the heat tolerant genotype compared to the sensitive genotype (Parankusam et al., 2017). Proteomics of spinach (50-day-old) exposed to 37/32°C for 24, 48, or 72 h identified heat-stress-responsive proteins in heat-tolerant (Sp75) and heat-sensitive (Sp73) lines (Li et al., 2019). The abundance pattern indicated that HS inhibited photosynthesis, initiated ROS scavenging pathways, and sped up carbohydrate and amino acid metabolism. A comparative proteomic study showed that heat-sensitive genotypes have a lower ability for photosynthetic adaptation, osmotic homeostasis, and antioxidant enzyme activities than heat-tolerant genotypes (Li et al., 2018). Ahsan et al. (2010) used a proteomics approach to study the tissue-specific protein expression pattern in heat-stressed soybean seedlings (40 ± 2°C for 12 h), identifying 61, 54, and 35 differentially expressed proteins in roots, leaves, and stem, respectively. Many of the proteins related to HSPs and the antioxidant system were upregulated.
Recent metabolite profiling has focused on important metabolites that govern temperature stress tolerance (Guy et al., 2008). Wang J. et al. (2019) studied the metabolism of heat-tolerant (17CL30) and heat-sensitive (05S180) capsicum cultivars; the tolerant genotype accumulated 94 differentially accumulated metabolites (DEM) while the sensitive genotype accumulated 108 DEM. Both genotypes shared common metabolites, but they were more highly expressed in tolerant genotypes. Metabolite profiling of tomato anthers exposed to 38°C for 2 h revealed that flavonoids (alkaloids and flavonoids in young microspores) protect against HS (Paupière et al., 2017a,b). A metabolomics study on heat-stressed soybean seeds revealed 275 metabolites that comprised antioxidants, including ascorbate precursors, tocopherol, flavonoids, phenylpropanoids, which were more enriched in tolerant than sensitive genotypes (Chebrolu et al., 2016).
Of late, molecular breeding has emerged as one of the important tools to identify progeny plants possessing the targeted genes/QTLs including the presence of several genes or ascertain the amount of genome of recurrent parent in a plant. Molecular breeding relies on molecular markers and hence the outcome, unlike the phenotyping, is not influenced by environmental factors. The molecular breeding has been exploited successfully in crop breeding and has led to the development of crop varieties possessing resistance to diseases or varieties with resistance genes pyramids (Janni et al., 2020). Molecular breeding methods to improve heat tolerance include (i) transfer of quantitative trait loci, (ii) marker-assisted selection. Other methods include marker assisted recurrent selection, marker-assisted pyramiding, and single nucleotide polymorphism. These methods pave the way for breeding stress tolerance in plants (Collard and Mackill, 2007). These methods pave the way for breeding stress tolerance in plants (Collard and Mackill, 2007).
QTL is a stretch of genomic regions on a chromosome that is linked to a quantitative trait. Usually, this stretch contains several genes and each QTL contribute partially to the trait in question; and hence, several QTLs together govern a trait. In molecular breeding, whole QTL is transferred to the recurrent parent utilizing markers flanking to the QTLs and sometimes using markers present within the QTL region. The exploitation of molecular breeding for QTLs transfers in breeding programs, a QTL must be well-defined and demonstrated to be linked to a particular trait (Collard and Mackill, 2009). Heat tolerance is a polygenic trait governed by several genes (Golam et al., 2012) and several QTLs. Unprecedented advances in genomics, especially molecular marker development, have identified numerous QTLs contributing to HS tolerance by dissecting various traits ranging from phenological, physiological, biochemical, reproductive biology to yield and yield-related traits (Lucas et al., 2013; Wen et al., 2019; Song et al., 2020; Jha et al., 2021; Vargas et al., 2021) in various vegetable crops, including bottle gourd (Lagenaria siceraria), cowpea (Vigna unguiculata [L.] Walp.), common bean, chickpea, chili, and tomato (Table 5). In broccoli (Brassica oleracea var. italica), five QTLs were identified under HS—QHT_C02, QHT_C03, QHT_C05, and QHT_C07 from the heat-tolerant parent and QHT_C09 from the heat-sensitive parent, with a positive epistatic co-relation between QHT_C03 and QHT_C05 for heat tolerance and APX activity was co-located with QHT_C03 (Branham et al., 2017). Likewise, QTLs such as QHT_C02, QHT_C05, and QHT_C09 were co-located with the AP2 gene governing floral development under HS (Aukerman and Sakai, 2003). Similarly, the meristem identity gene (TFL) was associated with QHT_C02 (Duclos and Björkman, 2008). Subsequently, two novel QTLs contributing to heat tolerance were uncovered by phenotypic evaluation of double haploid-based mapping population for two consecutive summer seasons and by employing QTL-seq approach in broccoli (Branham and Farnham, 2019). Recently, subjecting genome wide association (GWAS) study of one hundred forty two lines unearthed a total of fifty seven significant marker trait associations for various physiological and yield related traits under heat stress in Brassica rapa (Chen et al., 2022). In tomato, Xu et al. (2017) mapped 13 QTLs for heat tolerance linked with reproductive traits, including pollen viability, pollen number, style protrusion, anther length, style length, flower per inflorescence, and inflorescence number. These QTLs showed additive effects and no epistatic interaction. Likewise, six QTLs linked to fruit set in tomato at high temperatures were identified (Grilli et al., 2007). Based on evaluating recombinant inbred lines and introgression lines developed from Solanum lycopersicum var. “MoneyMaker” × S. pimpinellifolium across multi environments under high temperature stress enabled in identification of 22 QTLs related to reproductive traits (flower number fruit number and fruit set proportion) on LG1, 2, 4, 6, 7, 10, and 11 explaining phenotypic variation from 4 to 13% (Gonzalo et al., 2020). In combination of phenotypic assessment of leaf cell membrane stability by applying heat stress in F2 derived mapping population with QTL-seq approach in F2 derived mapping population assisted in uncovering a total of seven QTLs qHT1. 1, qHT2. 1, qHT2. 2, qHT5. 1, qHT6. 1, qHT7. 1, and qHT8. 1 conferring heat tolerance in bottle gourd (Song et al., 2020). Likewise, employing conventional QTL mapping and QTL-seq analysis allowed in identifying a total of five major QTLs qHII-1-1, qHII-1-2, qHII-1-3, qHII-2-1, and qCC-1-5 (qREC-1-3) related to heat injury index under heat stress in tomato (Wen et al., 2019). The authors performed the functional validation of the underlying selected four potential candidate genes SlCathB2, SlGST, SlUBC5, and SlARG1. To decipher genetic basis of heat tolerance in cucumber, QTL analysis of mapping population developed from “99281” (heat-tolerant) × “931” (heat-sensitive) population phenotypically evaluated during summer 2018, 2019, and 2020 allowed to identify one major QTL qHT1.1 on LG1 (Liu et al., 2021). There were 98 genes underlying this QTL. Of these identified genes, expression of Csa1G004990 candidate gene was higher in “99281” than “931” genotype rendering it heat tolerant. In order to shed light into the functional role of HSP20 contributing to heat tolerance, in Cucurbita moschata, genome wide bioinformatic analysis enabled in unveiling 33 HSP20 genes across the genome (Hu et al., 2021). Functional validation of CmoHSP20-7, 13, 18, 22, 26 and 32 genes indicated their possible role in heat tolerance in Cucurbita moschata (Hu et al., 2021).
In cowpea, five QTLs governing pod set at high temperature, namely Cht-1, Cht-2, Cht-3, Cht-4, and Cht-5, with CB 27 line of cowpea donating alleles for four QTLs (Cht-1, Cht-2, Cht-3, Cht-4) and IT82E-18 contributing alleles for Cht-5 (Lucas et al., 2013). Combinations of any of the four QTLs with Cht-5 positively correlated with heat tolerance in cowpea. Further, the presence of all five QTLs in the same line had the strongest positive correlation with heat tolerance (Lucas et al., 2013). Recently, four QTLs were identified in chickpea that conferred heat tolerance for filled pods (qfpod03_6), grain yield (qgy03_6), total seed number (qvs05_6), and pod set (q% podset08_6) using recombinant inbred lines produced from ICC 4567 (heat-sensitive) × ICC 15614 (heat-tolerant) lines (Paul et al., 2018). One QTL (qTBP5.2) was detected in lettuce, governing the tip-burn resistance trait, therefore beneficial in breeding programs (Jenni et al., 2013). The information on genomes of crops is expanding rapidly. The sequencing coupled with resequencing will generate more information that will subsequently be used to gather detailed knowledge of QTLs and genomic bases of heat tolerance in crops. The closely-related crops share syntenic relationships and possess similar genomic regions with each other. In the forthcoming years, comparative genomic analysis and advancements in knowledge of molecular biology might allow us to transfer heat tolerant regions from one crop to another, thereby expanding the repository of cold tolerance in crop plants.
As mentioned earlier, phenotype-based selection is prone to environmental conditions sometimes leading to erroneous conclusions especially if trait is complex and conferred by polygenes or QTLs. Under such circumstances, genotype-based selection is more effective, precise and fast as compared to phenotypic selection. Genotype-based selection rather than phenotype-based selection is possible using markers linked to gene of interest. Genotype-based selection utilizes DNA markers that are linked tightly to the gene(s) of interest (Collard and Mackill, 2007). For MAS, first step is to identify markers linked to the gene or QTL using either mapping populations or association mapping where a panel of genotypes is used to identify liked markers. Subsequently, these markers are used to ascertain transfer of the gene to the progeny populations. Different types of markers, such as RFLP (restricted fragment length polymorphism), AFLP (amplified fragment length polymorphism), SSR (single sequence repeat), and SNPs (single nucleotide polymorphisms), can be detected, and the amount of variation in each marker can be determined. Using this approach, gene mapping and identifying gene associations with particular traits are useful for genetic crop improvement (Ruane and Sonnino, 2007).
Paul et al. (2018) identified SNP markers linked to QTLs for heat tolerance traits (50% flowering, podding behavior, total filled pods, % pod set, total seed number, grain yield, biomass, harvest index, 100-seed weight) in chickpea RILs (heat-tolerant ICC 15614 × heat-sensitive ICC 4567). Composite interval mapping analysis affirmed two genomic regions (CaLG05 and CaLG06) with four QTLs (grain yield, total seed number, total filled pods, % pod set). A GWAS used 16,877 SNPs to identify marker-trait associations (MTA) in 135 diverse pea lines exposed to >28°C in the field to understand the genetic basis for heat tolerance (Gali et al., 2019). The study identified 32 MTAs and 48 candidate genes associated with various traits, including chlorophyll concentration, photochemical reflectance index, canopy temperature, reproductive stem length, internode length, pod number, with the potential for developing heat-tolerant cultivars (Tafesse et al., 2020). Lin et al. (2006) identified 14 RAPD markers linked to heat tolerance traits (flower number, fruit number, fruit set, yield) in tomato RILs derived from CL5915 (heat-tolerant) and L4422 (heat-sensitive) under HS. Developing heat tolerant Capsicum annuum through transferring heat shock protein encoding gene Hsp70 and sHsp from AVPP0702 into Kulai an elite C. annuum cultivar by adopting marker assisted back crossing approach is notable illustration of marker assisted breeding for heat tolerance (Usman et al., 2018). Likewise, three non-synonymous SNPs identified in the qHT2.1 major effect QTL in bottle gourd (Song et al., 2020) and non-synonymous SNP identified in the QHT_C09.2 QTL regions in broccoli (Branham and Farnham, 2019) contributing to heat tolerance, which could be potentially used as candidate markers for screening heat tolerant bottle gourd and broccoli genotypes.
Altering the genetic makeup of vegetable crops is a possible solution for developing crops that can grow and reproduce well under increasing temperatures. Plants have an inherent ability to endure supra optimal temperatures (“basal thermotolerance” or “acquired tolerance to increasing temperature”) (Grover et al., 2013). The level of thermotolerance varies between plant species depending on their genetic makeup and specific expression of defense-related genes, however, levels of thermotolerance vary in different plant species again due to differences in genetic makeup of the plant species. Even within a species, genotypes differ for reaction (tolerance or sensitive) to HS owing to varying genetic makeup. Considerable number of genes/QTLs conferring tolerance to HS has been identified in vegetable crops and these genes/QTLs can be transferred from heat-tolerant genotypes to heat-sensitive genotypes using transgenic approaches to develop genetically modified heat tolerant crops. Genes expressed in heat-tolerant crops can be transferred to heat-sensitive crops using transgenic approaches to develop genetically modified heat-tolerant crops. Candidate genes for development of transgenics for heat tolerance are HSP, compatible osmolyte, and antioxidant levels, and detoxifying pathways (Parmar et al., 2017).
Many vegetable crops have been manipulated for increased expression of HSPs. For instance, in tomato, overexpression of trehalose-6-phosphate synthase/phosphatase (TPSP) gene derived from Escherichia coli increased the expression of HsfA1, HsfA2, and HsfB1, which was linked to escalating Hsp17.8, ER-sHsp and Mt-sHsp levels to impart heat tolerance (Lyu et al., 2018). Similarly, overexpression of small heat shock protein (CaHsp 25.9) improved thermotolerance in Capsicum transgenic lines (R9 and B6) under HS, decreasing MDA content and increasing proline and SOD content (Feng et al., 2019). In transgenic potato lines, overexpression of the A2 HSc70 (Heat-Shock Cognate) allele-maintained tuber yield at elevated temperature (Trapero-Mozos et al., 2018).
HS causes oxidative damage in plants; therefore, developing transgenics with enhanced antioxidative mechanisms may enhance thermotolerance in plants. Antioxidant mechanisms were manipulated in pea by incorporating heat shock factor gene (HsfA1d) from Arabidopsis thaliana. Under HS (42°C), transgenic pea plants had five-fold higher expression of HsfA1d than wild pea, decreasing H2O2 accumulation, and higher SOD and APX activities and proline content (Shah et al., 2020). Tang et al. (2006) developed transgenic potato plants (SSA plants) expressing Cu/Zn SOD and APX gene in chloroplasts under the control of a SWPA2. The transgenic plants had less damage induced by methyl viologen than non-transgenic plants. In the same study, photosynthetic activity decreased by 29% in non-transgenic plants but only 6% in transgenic plants under HS (42°C for 20 h). Overexpression of cytosolic APX (cAPX) in transgenic tomato (Lycopersicon esculentum cv. Zhongshu No. 5) under HS (40°C for 13 h) resulted in several-fold higher APX activity than wild plants, reducing electrolyte leakage (24% in A9 line and 52% in A16 line) compared with wild plants. Similarly, overexpression of cAPX in transgenic tomato increased tolerance HS (Wang et al., 2006).
Equilibrium between ROS generation and ROS scavenging is disturbed by the high temperature stress (Foyer and Noctor, 2005). One of the best strategies adopted by the plant cells is the production of HSPs on exposure to high temperature (Wang et al., 2004). HSPs positively affect thermotolerance by protecting ROS scavenging system and actively resulting in lower ROS concentration. HSPs also enable protein refolding, preventing aggregation of non-native proteins and stabilize polypeptides and membrane under stress conditions (Scarpeci et al., 2008). It is unclear whether there is specific interaction between HSPs and ROS scavenging machinery but ROS accumulation is reduced via HSP induced ROS scavenging activity. Hence the cross-talk between production of HSFs/HSPs and ROS scavenging activity play important role in acclimation (Kang et al., 2022). The communication between ROS and HSFs involve Mitogen Activated Protein Kinase (MAPK). ROS dependent phosphorylation can play vital role in HSF activation (Driedonks et al., 2015). MAPK3 and MAPK6 are the key players which are activated by H2O2 and further phosphorylate the HSFs, for instance in tomato, heat induced MAPK transduces the heat stress signal via HSFA3 (Link et al., 2002). Induction of heat shock transcription factors HsfA2 and HsfA4 is reported to be regulators of genes associated with ROS mitigation. HsfA4A is the principle candidate to function as H2O2 sensor (Scarpeci et al., 2008). At transcriptional level, HSPs are regulated by HSFs that bind to the conserved regulatory element of heat shock element (HSEs) and act as promoter for Hsp genes. Under stress conditions ROS mainly H2O2 functions as signal transduction molecule and cause HSF activation. ROS enhances the dissociation of HSP and HSF complex and promote the HSF trimerization and relocate the same to the nucleus leading to activation of the expression of HSPs and other heat responsive genes (Ul Haq et al., 2019) (Figure 4).
Figure 4. Cross talk between HSPs and redox reaction: -Heat stress imposes damages to plant like increased membrane fluidity, unfolding of proteins, ROS production and dissociation of HSP70/90-HsfA1 complex. To endure HS, Plants activate various mechanisms to preserve their adaptation. First such mechanism is the activation of cyclic nucleotide gated calcium (CNGC) channels that result in the movement of Ca2+ions in to cytoplasm and bind with Calmodulin Protein (CaM3) forming the Ca2+-CaM3 complex and help in the activation of Heat shock factors (HSFs). Second mechanism involves Phosphoinositol signaling pathway that also lead to the influx of more Ca2+in to the cytoplasm and merge with Ca2+-CaM3 pathway. Another mechanism during HS is the activation of ROS signaling network by Respiratory Burst Oxidase Homolog D (RBOHD) that produce O2− which is converted in to H2O2 that is involved in the induction of HSFs activation. ROS like H2O2 also activate the HSFs complex through mitogen activated protein kinase (MAPK). On activation, HSFs move to the nucleus and activate HSE and HSP target genes. HS also lead to the dissociation, of HSP70/90-HsfA1 complex; on dissociation HsfA1 undergoes trimerization that further activates the HSFs complex in the cytosol and Heat shock element (HSE) in the nucleus. Their activation has many positive effects on the cellular metabolism like transcriptional regulation, activation of antioxidant system and multi chaperone network (HSP60, HSP70, HSP90, HSP100, and sHSP) that may lower down the ROS levels in the cell and help in achieving thermotolerance.
By employing improved agronomic practices for different crops has improved crop yields. These practices include better soil, water, nutrient, weed, and pest management strategies, selection of varieties, and appropriate planting times and planting densities, and more and more (HanumanthaRao et al., 2016). Agronomic practices control soil temperature by minimizing the evaporation (Ferrante and Mariani, 2018) helping the cultivators with sustained water use, proper fertilizer use, and improved land maintenance, consequently improving crop quality and quantity. In addition, agronomic practice also helps with increased soil physical, chemical and microbial status. These help with water and nutrient availability and plant uptake. Agronomic practices for increasing vegetable crop yields that are efficient, cost-effective, and easily adaptable for HS management are described below.
Land preparation for planting involves tillage, seedbed shaping, and mulching. These practices depend on the soil type, physical and chemical properties. Sandy loam soils are best for raising vegetables such as potato, cauliflower, lettuce, cabbage, and tomato. Tillage includes breaking up/loosening the soil by plow, favoring seed germination, and proper seedling growth. Tillage also helps control weeds, aerate soil, and bury the previous crop's residues; the tillage method varies between crops (Kladivko, 2001). However, the same benefits can be obtained with no-till or minimum tillage practices that minimizes soil disturbance and helps with building of soil organic carbon over time. Mulching is a process of covering the soil with chopped residues; it has many benefits, including reduced soil erosion and water loss, which maintain soil temperature (Mulumba and Lal, 2008). Use of conservation agricultural practices with minimum soil disturbance, grass mulch cover and crop rotations not only significantly increased yield of green pepper but also decreased irrigation water use and runoff, while increasing percolated water in the root zone (Belay et al., 2020). Similarly, improved yields of tomato, cucumber and bitter guard were observed under conservation agriculture (Paudel et al., 2020). Conservation agricultural practices in vegetable production systems has shown to increase soil organic matter and nutrients (Belay et al., 2022). Irrigation increases soil moisture, decreasing soil temperature (by 2°) compared to non-irrigated soil (Lobell and Bonfils, 2008). Water quality and supply varies according to soil type, crop (warm- or cool-season), and weather conditions. Generally, vegetable crops are irrigated at 4–6-day intervals during summer and 14–15-day intervals during winter to reduce the high-temperature effects. Many modern technologies for irrigation are available that minimize water use, such as drip or trickle irrigation and overhead micro-sprinklers.
Variety selection is a successful agronomic approach for achieving high yields under high-temperature stress. Selection characteristics include high yield, disease resistance, maturity group, and grain quality (Pedersen, 2003). Suitable crop genotypes need to be early maturing and high yielding to escape heat by completing their life cycle early and thus perform better under HS (Sekhon et al., 2010). Furthermore, shifting the sowing time (early or late) is another strategy to avoid HS and avoid heat induced yield reduction as has been reported in mungbean (up to 50%) and soybean where yield declined tremendously by delay in the sowing date (Coventry et al., 1993; Miah et al., 2009). The goal of selection of crop duration and time of planting is to avoid HS during sensitive stages of reproductive development. In contrast, late sowing has been used to screen large populations of chickpea (Gaur et al., 2013), mungbean (Sharma et al., 2016), and lentil (Sita et al., 2017) genotypes for heat tolerance, some of which have been released (e.g., chickpea ICCV 92944) (Gaur et al., 2013). Heat-tolerant varieties of some vegetable crops are listed in Table 6. Hence, determining the ideal sowing time and selection of heat tolerant varieties is crucial for growth, development, and yield of crops.
HS can be alleviated by exogenous application of nutrients or thermo-protectants as a seed pretreatment, foliar spray, or by fertilizer application via broadcasting, pellet placement, or band placement (Waraich et al., 2012; HanumanthaRao et al., 2016). Macro-nutrients such as N, P, K, Ca, and Mg are required by plants (>10 mM) and help maintain structural and functional integrity (Waraich et al., 2011). Nutrient deficiencies alter the levels of tolerance to abiotic stresses. During HS, N deficient plants were associated with increased lipid peroxidation, while N supplemented plants tolerated photo-oxidative damage (Kato et al., 2003). Likewise, K deficient plants had reduced translocation of photo-assimilates to the sink organ, whereas K application improved the translocation and utilization of photo-assimilates, maintained cell turgidity, and upregulated enzymatic activity under HS (Mengel et al., 2001; Cakmak, 2005), increasing yield by 1.9-fold in Capsicum and 2.4-fold in tomato (Waraich et al., 2012). Similarly, exogenous application of calcium (2 L/ha) increased lettuce production under HS (Almeida et al., 2016).
Micronutrients such as B and Mn also provide heat tolerance of plants by increasing antioxidant activity and alleviating the damage induced by HS stress (Waraich et al., 2011). Other elements such as Se increased enzymatic activity and decreased membrane damage and ROS production in soybean (Djanaguiraman et al., 2005). Seed pretreatment and foliar application of thermoprotectant molecules such as proline, glycinebetaine, salicylic acid, spermidine, putrescine, GABA, ascorbic acid provides thermotolerance to crop plants (HanumanthaRao et al., 2016). For instance, exogenous application of proline mitigated HS effects in chickpea (Kaushal et al., 2011). Ascorbic acid application to mungbean seedlings under HS in a controlled environment improved seedling growth (Kumar et al., 2011). In cucumber, a 1 mM SA foliar spray provided heat tolerance by increasing CAT activity and thus reducing membrane damage and H2O2 levels (Shi et al., 2006). Similarly, Kaur et al. (2009) reported that exogenous application of SA (10 and 20 μM) to heat-stressed brassica seedlings (40–55°C) improved CAT and POX activities. Pretreatment of SA to mungbean seedlings decreased lipid peroxidation and enhanced antioxidant activity, improving membrane stability (Saleh et al., 2007). In chickpea, a 100 μM SA foliar spray to heat-stressed seedlings (46°C) increased proline content (Chakraborty and Tongden, 2005). Thus, exogenous SA application mitigates the harmful impacts of heat-induced damage by strengthening antioxidative pathways. Foliar spray of Se (8 μM) to cucumber plants exposed to 40/30°C during flower initiation (35–75 DAS) decreased oxidative damage by stabilizing the antioxidative mechanism and increasing ROS scavenging (Balal et al., 2016).
In addition to other factors, plant-associated microorganisms, including plant-growth-promoting rhizobacteria, endophytic bacteria, and symbiotic fungi, play a significant role in imparting thermotolerance in plants (Grover et al., 2011). Many agriculturally important microbes have been discovered that colonize and promote plant growth and aid in nutrient and disease control through various direct and indirect methods (Singh et al., 2016). The interaction between microorganisms and host plants imparting stress tolerance is a complex process and polygenic in nature. Ali et al. (2009) discovered a thermotolerant strain of Pseudomonas sp. AMK-P6 in sorghum that elicits HSPs synthesis under high-temperature stress, and improves biochemical activities by inducing the synthesis of osmolytes such as proline, sugars, amino acids, and chlorophyll. Pseudomonas putida NBRI0987, a thermotolerant strain (<40°C) was isolated from the chickpea rhizosphere (Srivastava et al., 2008). A recent study on different rhizobacterial strains of pigeon pea at high temperature (30, 40, 50°C) showed that S1p1 and S12p6 were the most promising strains for plant growth and development, stimulating auxin production, flavonoid production, and siderophore formation (Modi and Khanna, 2018). It would be worth evaluating the effectiveness of these microbes in vegetable crops for induction of thermotolerance.
Growing vegetables in protected environments on small-scale farms using modern technologies has gained considerable attention for their high yields and quality and regular vegetable supply in the off-season (Sabir and Singh, 2013). Protected cultivation involves manipulating environmental factors such as temperature, humidity, light, water, and soil by designing suitable structures and following appropriate practices (Wittwer and Castilla, 1995). The main practices for protected cultivation are row tunnels, polytunnels, and mulching, which are more beneficial than open-field cultivation with less demand for fertilizers, pesticides, and water (Choudhary et al., 2013). In tomato, using a fogging system for 20 min/h (between 10 a.m. and 4 p.m.) in a hot shade house (>37°C) obtained high fruit yields with fewer physiological disorders (Ro et al., 2021). A similar fogging system improved the antioxidant defense responses in tomato plants (Leyva et al., 2013). Related approaches have been used to cultivate cucumber, capsicum, and lettuce with high yields (Sabir and Singh, 2013).
Vegetables are a distinct collection of plant-based foods that vary in nutritional diversity and form an important part of healthy diets. They also have great potential for boosting human health. Exposure to high temperatures or HS can directly or indirectly influence the production and quality of fresh vegetables. Several heat-induced morphological damages, such as poor vegetative growth, leaf tip burning, rib discoloration in leafy vegetables, sun burned fruits, decreased fruit size; pod abortion, and unfilled pods are common, which can render vegetable cultivation unprofitable. Key physiological and biochemical effects associated with crop failure include membrane damage, photosynthetic inhibition, oxidative stress, and reproductive tissue damage. Reproductive stage has extensively been studied and found to be more sensitive to HS as it directly affects yields by reducing processes like pollen germination, pollen load, pollen tube growth, stigma receptivity, ovule fertility, and seed filling, resulting in poorer yields. Hence, sound and robust adaptation strategies are needed to mitigate the adverse impacts of HS to ensure the productivity and quality of vegetable crops.
Most important strategy to manage HS is deployment of heat tolerant cultivars (Figure 5). Physiological traits, such as stay-green trait, canopy temperature depression, cell membrane thermostability, chlorophyll fluorescence, relative water content, and stomatal conductance, are especially important in developing high-yielding heat-tolerant varieties/cultivars. Molecular approaches like omics, molecular breeding and transgenics have the potential to enhancing heat tolerance either by transferring heat tolerant genes/QTLs to elite cultivars with the help of molecular markers or elucidating mechanisms of tolerance leading to identification of heat tolerance genes and transferring those across genera or families via genetic modifications. Besides these approaches, simple agronomic methods are also important for mitigating HS effects at the grassroots level. Therefore, developing heat-tolerant plant types using physiological, molecular, and breeding-based techniques is essential for sustaining vegetable production systems and human health. Further, these approaches will offer insight into the physiological and molecular mechanisms that govern thermotolerance and pave the way for engineering ‘designer' vegetable crops for better health and nutritional security.
Figure 5. Heat stress has various negative impacts on the plant like reducing vegetative and reproductive growth, interfering with the physiological and cellular functions. To combat such impacts, plant activates multiple responses and heat avoidance mechanisms which can be used to identify heat resilient vegetable crops. Different approaches categorized in this article for this purpose are physiological based, omics based, molecular breeding based and agronomic based. Such possible options will pave the way for improving adaptation and mitigation of heat stress in vegetable crops.
The original contributions presented in the study are included in the article/supplementary material, further inquiries can be directed to the corresponding authors.
All authors listed have made a substantial, direct, and intellectual contribution to the work and approved it for publication.
The authors declare that the research was conducted in the absence of any commercial or financial relationships that could be construed as a potential conflict of interest.
All claims expressed in this article are solely those of the authors and do not necessarily represent those of their affiliated organizations, or those of the publisher, the editors and the reviewers. Any product that may be evaluated in this article, or claim that may be made by its manufacturer, is not guaranteed or endorsed by the publisher.
SC and PD thank CSIR-UGC, India for providing their doctoral research fellowship. The corresponding author (HN) is thankful to DST, UGC, DBT, CSIR, India, UWA (Australia), ICARDA (Morocco), IIPR (Kanpur, India), PAU (Ludhiana, India), and World Vegetable Center (at ICRISAT) for supporting the research work at various times.
Abdelmageed, A. H. A., and Gruda, N. (2009). Influence of high temperatures on gas exchange rate and growth of eight tomato cultivars under controlled heat stress conditions. Eur. J. Hortic. Sci. 74, 152–159.
Abdelmula, A. A., and Abuanja, I. K. (2007). “Genotypic responses, yield stability, and association between characters among some of Sudanese faba bean (Vicia faba L.) genotypes under heat stress,” in Conference on International Agricultural Research for Development, October 9-11, Tropentag 2007 (Göttingen: University of Kassel-Witzenhausen and University of Göttingen).
Abdul-Baki, A. A. (1991). Tolerance of tomato cultivars and selected germplasm to heat stress. J. Am. Soc. Hortic. Sci. 116, 1113–1116. doi: 10.21273/JASHS.116.6.1113
Abewoy, D (2018). Review on impacts of climate change on vegetable production and its management practices. Adv. Crop Sci. Tech. 6, 1–7. doi: 10.4172/2329-8863.1000330
Acquaah, G. (2015). “Conventional plant breeding principles and techniques,” in Advances in Plant Breeding Strategies: Breeding, Biotechnology and Molecular Tools, eds J. M. Al-khayri, S. M. Jain, and D. V. Johnson (Cham: Springer), 115–158.
Ahmed, F. E., Hall, A. E., and DeMason, D. A. (1992). Heat injury during floral development in cowpea (Vigna unguiculata, Fabaceae). Am. J. Bot. 79, 784–791. doi: 10.1002/j.1537-2197.1992.tb13655.x
Ahn, Y. J., Claussen, K., and Zimmerman, J. L. (2004). Genotypic differences in the heat-shock response and thermotolerance in four potato cultivars. Plant Sci. 166, 901–911. doi: 10.1016/j.plantsci.2003.11.027
Ahsan, N., Donnart, T., Nouri, M. Z., and Komatsu, S. (2010). Tissue-specific defense and thermo-adaptive mechanisms of soybean seedlings under heat stress revealed by proteomic approach. J. Proteome Res. 9, 4189–4204. doi: 10.1021/pr100504j
Aien, A., Khetarpal, S., and Pal, M. (2011). Photosynthetic characteristics of potato cultivars grown under high temperature. Am. Eurasian J. Agric. Environ. Sci. 11, 633–639. doi: 10.5829/idosi.wasj.2013.24.04.2311
Aleem, S., Sharif, I., Tahir, M., Najeebullah, M., Nawaz, A., Khan, M. I., et al. (2021). Impact of heat stress on cauliflower (Brassica oleracea var. Botrytis): a physiological assessment. Pak. J. Agric. Sci. 34, 479–486. doi: 10.17582/journal.pjar/2021/34.3.479.486
Alencar, N. L., Innecco, R., Gomes-Filho, E., Gallão, M. I., Alvarez-Pizarro, J. C., Prisco, J. T., et al. (2012). Seed reserve composition and mobilization during germination and early seedling establishment of Cereus jamacaru DC ssp. jamacaru (Cactaceae). An. Acad. Bras. Cienc. 84, 823–832. doi: 10.1590/S0001-37652012000300024
Alexandre, A., and Oliveira, S. (2013). Response to temperature stress in rhizobia. Crit. Rev. Microbiol. 39, 219–228 doi: 10.3109/1040841X.2012.702097
Ali, M., Ayyub, C. M., Amjad, M., and Ahmad, R. (2019). Evaluation of thermo-tolerance potential in cucumber genotypes under heat stress. Pak. J. Agric. Sci. 56, 53–61. doi: 10.21162/PAKJAS/19.7519
Ali, S. Z., Sandhya, V., Grover, M., Kishore, N., Rao, L. V., and Venkateswarlu, B. (2009). Pseudomonas sp. strain AKM-P6 enhances tolerance of sorghum seedlings to elevated temperatures. Biol. Fertil. Soils 46, 45–55. doi: 10.1007/s00374-009-0404-9
Almeida, P. H., Mógor, Á. F., Ribeiro, A. Z., Heinrichs, J., and Amano, E. (2016). Increase in lettuce (Lactuca sativa L.) production by foliar calcium application. Aust. J. Basic Appl. Sci. 10, 161–167.
Aloni, B., Peet, M., Pharr, M., and Karni, L. (2001). The effect of high temperature and high atmospheric CO2 on carbohydrate changes in bell pepper (Capsicum annuum) pollen in relation to its germination. Physiol. Plant. 112, 505–512. doi: 10.1034/j.1399-3054.2001.1120407.x
Alsajri, F., Singh, B., Wijewardana, C., Irby, J., Gao, W., and Reddy, K. (2019). Evaluating soybean cultivars for low-and high-temperature tolerance during the seedling growth stage. Agronomy. 9, 1–20. doi: 10.3390/agronomy9010013
Alsamir, M., Mahmood, T., Trethowan, R., and Ahmad, N. (2021). An overview of heat stress in tomato (Solanum lycopersicum L.). Saudi J. Biol. Sci. 28, 1654–1663. doi: 10.1016/j.sjbs.2020.11.088
Angadi, S. V., Cutforth, H. W., Miller, P. R., McConkey, B. G., Entz, M. H., Brandt, S. A., et al. (2000). Response of three Brassica species to high temperature stress during reproductive growth. Can. J. Plant Sci. 80, 693–701. doi: 10.4141/P99-152
Aranjuelo, I., Irigoyen, J. J., and Sánchez-Díaz, M. (2007). Effect of elevated temperature and water availability on CO2 exchange and nitrogen fixation of nodulated alfalfa plants. Environ. Exp. Bot. 59, 99–108. doi: 10.1016/j.envexpbot.2005.10.008
Aukerman, M. J., and Sakai, H. (2003). Regulation of flowering time and floral organ identity by a microRNA and its APETALA2-like target genes. Plant Cell 15, 2730–2741. doi: 10.1105/tpc.016238
Awasthi, R., Kaushal, N., Vadez, V., Turner, N. C., Berger, J., Siddique, K. H., et al. (2014). Individual and combined effects of transient drought and heat stress on carbon assimilation and seed filling in chickpea. Funct. Plant Biol. 41, 1148–1167. doi: 10.1071/FP13340
Babu, N. R., and Devaraj, V. R. (2008). High temperature and salt stress response in French bean (Phaseolus vulgaris). Aust. J. Crop Sci. 2, 40–48.
Balal, R. M., Shahid, M. A., Javaid, M. M., Iqbal, Z., Anjum, M. A., Garcia-Sanchez, F., et al. (2016). The role of selenium in amelioration of heat-induced oxidative damage in cucumber under high temperature stress. Acta Physiol. Plant. 38, 1–14. doi: 10.1007/s11738-016-2174-y
Belay, S. A., Assefa, T. T., Prasad, P. V., Schmitter, P., Worqlul, A. W., Steenhuis, T. S., et al. (2020). The response of water and nutrient dynamics and of crop yield to conservation agriculture in the Ethiopian highlands. Sustainability 12, 1–15. doi: 10.3390/su12155989
Belay, S. A., Assefa, T. T., Worqlul, A. W., Steenhuis, T. S., Schmitter, P., Reyes, M. R., et al. (2022). Conservation and conventional vegetable cultivation increase soil organic matter and nutrients in the ethiopian highlands. Water J. 14, 476. doi: 10.3390/w14030476
Bernier, G., Kinet, J.-M., and Sachs, R. M. (1981). The Physiology of Flowering, Vol. I. Boca Raton, FL: CRC Press.
Berry, J., and Bjorkman, O. (1980). Photosynthetic response and adaptation to temperature in higher plants. Annu. Rev. Plant Physiol. 31, 491–543. doi: 10.1146/annurev.pp.31.060180.002423
Bhandari, K., Sharma, K. D., Rao, B. H., Siddique, K. H., Gaur, P., Agrawal, S. K., et al. (2017). Temperature sensitivity of food legumes: a physiological insight. Acta Physiol. Plant. 39, 1–22. doi: 10.1007/s11738-017-2361-5
Bineau, E., Diouf, I., Carretero, Y., Duboscq, R., Bitton, F., Djari, A., et al. (2021). Genetic diversity of tomato response to heat stress at the QTL and transcriptome levels. Plant J. 107, 1213–1227. doi: 10.1111/tpj.15379
Bishop, J., Potts, S. G., and Jones, H. E. (2016). Susceptibility of faba bean (Vicia faba L.) to heat stress during floral development and anthesis. J. Agron. Crop Sci. 202, 508–517. doi: 10.1111/jac.12172
Bita, C. E., and Gerats, T. (2013). Plant tolerance to high temperature in a changing environment: scientific fundamentals and production of heat stress-tolerant crops. Front. Plant Sci. 4, 273. doi: 10.3389/fpls.2013.00273
Björkman, T., and Pearson, K. J. (1998). High temperature arrest of inflorescence development in broccoli (Brassica oleracea var. italica L.). J. Exp Bot. 49, 101–106. doi: 10.1093/jxb/49.318.101
Board, J. E., and Kahlon, C. S. (2011). “Soybean yield formation: what controls it and how it can be improved,” in Soybean Physiology and Biochemistry, ed A. El Shemy (Rijeka: Intech Publishers), 1–36.
Boote, K. J., Allen, L. H., Prasad, P. V., Baker, J. T., Gesch, R. W., Snyder, A. M., et al. (2005). Elevated temperature and CO2 impacts on pollination, reproductive growth, and yield of several globally important crops. J. Agric. Meteorol. 60, 469–474. doi: 10.2480/agrmet.469
Bordeleau, L. M., and Prévost, D. (1994). Nodulation and nitrogen fixation in extreme environments. Plant Soil 161, 115–125. doi: 10.1007/BF02183092
Bourgeois, M., Jacquin, F., Savois, V., Sommerer, N., Labas, V., Henry, C., et al. (2009). Dissecting the proteome of pea mature seeds reveals the phenotypic plasticity of seed protein composition. Proteomics 9, 254–271. doi: 10.1002/pmic.200700903
Branham, S. E., and Farnham, M. W. (2019). Identification of heat tolerance loci in broccoli through bulked segregant analysis using whole genome resequencing. Euphytica 215, 1–9. doi: 10.1007/s10681-018-2334-9
Branham, S. E., Stansell, Z. J., Couillard, D. M., and Farnham, M. W. (2017). Quantitative trait loci mapping of heat tolerance in broccoli (Brassica oleracea var. italica) using genotyping-by-sequencing. Theor. Appl. Genet. 130, 529–538. doi: 10.1007/s00122-016-2832-x
Bueckert, R. A., Wagenhoffer, S., Hnatowich, G., and Warkentin, T. D. (2015). Effect of heat and precipitation on pea yield and reproductive performance in the field. Can. J. Plant Sci. 95, 629–639. doi: 10.4141/cjps-2014-342
Cakmak, I. (2005). The role of potassium in alleviating detrimental effects of abiotic stresses in plants. J. Plant. Nutr. Soil Sci. 168, 521–530. doi: 10.1002/jpln.200420485
Camejo, D., Rodríguez, P., Morales, M. A., Dell'Amico, J. M., Torrecillas, A., and Alarcón, J. J. (2005). High temperature effects on photosynthetic activity of two tomato cultivars with different heat susceptibility. J. Plant Physiol. 162, 281–289. doi: 10.1016/j.jplph.2004.07.014
Casal, J. J., and Balasubramanian, S. (2019). Thermomorphogenesis. Annu Rev. Plant Biol. 70, 321–346. doi: 10.1146/annurev-arplant-050718-095919
Cen, Y. P., and Sage, R. F. (2005). The regulation of Rubisco activity in response to variation in temperature and atmospheric CO2 partial pressure in sweet potato. Plant Physiol. 139, 979–990. doi: 10.1104/pp.105.066233
Chakraborty, U., and Tongden, C. (2005). Evaluation of heat acclimation and salicylic acid treatments as potent inducers of thermotolerance in Cicer arietinum L. Curr. Sci. 89, 384–389.
Chang, K. Y., Lin, K. H., and Lo, H. F. (2016). Physiology and proteomics of cabbage under heat and flooding stress. Res. Rev.: J. Bot. Sci. 5, 44–53.
Chauhan, Y. S., and Senboku, T. (1996). Thermostabilities of cell-membrane and photosynthesis in cabbage cultivars differing in heat tolerance. J. Plant Physiol. 149, 729–734. doi: 10.1016/S0176-1617(96)80099-X
Chavez-Arias, C. C., Ligarreto-Moreno, G. A., and Restrepo-Díaz, H. (2018). Evaluation of heat stress period duration and the interaction of daytime temperature and cultivar on common bean. Environ. Exp. Bot. 155, 600–608. doi: 10.1016/j.envexpbot.2018.08.012
Chebrolu, K. K., Fritschi, F. B., Ye, S., Krishnan, H. B., Smith, J. R., and Gillman, J. D. (2016). Impact of heat stress during seed development on soybean seed metabolome. Metabolomics 12, 1–14. doi: 10.1007/s11306-015-0941-1
Chen, H. H., Shen, Z. Y., and Li, P. H. (1982). Adaptability of crop plants to high temperatures stress. Crop Sci. 22, 719–725. doi: 10.2135/cropsci1982.0011183X002200040006x
Chen, L., Figueredo, A., Villani, H., Michajluk, J., and Hungria, M. (2002). Diversity and symbiotic effectiveness of rhizobia isolated from field-grown soybean nodules in Paraguay. Biol. Fertil. Soils 35, 448–457. doi: 10.1007/s00374-002-0493-1
Chen, S., Hayward, A., Dey, S. S., Choudhary, M., Witt Hmon, K. P., Inturrisi, F. C., et al. (2022). Quantitative trait loci for heat stress tolerance in Brassica rapa L. are distributed across the genome and occur in diverse genetic groups, flowering phenologies and morphotypes. Genes 13, 296. doi: 10.3390/genes13020296
Chidambaranathan, P., Jagannadham, P. T. K., Satheesh, V., Kohli, D., Basavarajappa, S. H., Chellapilla, B., et al. (2018). Genome-wide analysis identifies chickpea (Cicer arietinum) heat stress transcription factors (Hsfs) responsive to heat stress at the pod development stage. J. Plant Res. 131, 525–542. doi: 10.1007/s10265-017-0948-y
Chitwood, J., Shi, A., Evans, M., Rom, C., Gbur, E. E., Motes, D., et al. (2016). Effect of temperature on seed germination in spinach (Spinacia oleracea). Hort. Sci. 51, 1475–1478. doi: 10.21273/HORTSCI11414-16
Choudhary, A. K., Thakur, S. K., and Suri, V. K. (2013). Technology transfer model on integrated nutrient management technology for sustainable crop production in high-value cash crops and vegetables in northwestern Himalayas. Commun. Soil Sci. Plant Anal. 44, 1684–1699. doi: 10.1080/00103624.2013.783058
Christophe, S., Jean-Christophe, A., Annabelle, L., Alain, O., Marion, P., and Anne-Sophie, V. (2011). “Plant N fluxes and modulation by nitrogen, heat and water stresses: a review based on comparison of legumes and non-legume plants,” in Abiotic Stress in Plants - Mechanisms and Adaptations, eds A. Shanker and B. Venkateswarlu (Croatia: Tech Publishers), 79–118.
Collard, B. C., and Mackill, D. J. (2007). Marker-assisted selection: an approach for precision plant breeding in the twenty-first century. Philos. Trans. R. Soc. Lond. B Biol. Sci. 363, 557–572. doi: 10.1098/rstb.2007.2170
Collard, B. C., and Mackill, D. J. (2009). Start codon targeted (SCoT) polymorphism: a simple, novel DNA marker technique for generating gene-targeted markers in plants. Plant Mol. Biol. Rep. 27, 86–93. doi: 10.1007/s11105-008-0060-5
Commuri, P. D., and Jones, R. J. (2001). High temperatures during endosperm cell division in maize. Crop Sci. 41, 1122–1130. doi: 10.2135/cropsci2001.4141122x
Costa, E. S., Bressan-Smith, R., Oliveira, J. G., and Campostrini, E. (2003). Chlorophyll a fluorescence analysis in response to excitation irradiance in bean plants (Phaseolus vulgaris L. and Vigna unguiculata L. Walp) submitted to high temperature stress. Photosynthetica 41, 77–82. doi: 10.1023/A:1025860429593
Coventry, D. R., Reeves, T. G., Brooke, H. D., and Cann, D. K. (1993). Influence of genotype, sowing date, and seeding rate on wheat development and yield. Aust. J. Exp. Agric. 33, 751–757. doi: 10.1071/EA9930751
Crafts-Brandner, S. J., and Salvucci, M. E. (2000). Rubisco activase constrains the photosynthetic potential of leaves at high temperature and CO2. Proc. Natl. Acad. Sci. U.S.A. 97, 13430–13435. doi: 10.1073/pnas.230451497
Craufurd, P. Q., Bojang, M., Wheeler, T. R., and Summerfield, R. J. (1998). Heat tolerance in cowpea: effect of timing and duration of heat stress. Ann. Appl. Biol. 133, 257–267. doi: 10.1111/j.1744-7348.1998.tb05826.x
Crozier, A., Yokota, T., Jaganath, I. B., Marks, S. C., Saltmarsh, M., and Clifford, M. N. (2006). “Secondary metabolites in fruits, vegetables, beverages and other plant based dietary components,” in Plant Secondary Metabolites: Occurrence, Structure and Role in the Human Diet, eds A. Crozier, H. Ashihara, and M. N. Clifford (United Kingdom: Wiley-Blackwell), 208–302. doi: 10.1002/9780470988558.ch7
Dahal, K. C., Sharma, M. D., Dhakal, D. D., and Shakya, S. M. (2006). Evaluation of heat tolerant chilli (Capsicum annuum L.) genotypes in Western Terai of Nepal. J. Inst. Agric. Anim. Sci. 27, 59–64. doi: 10.3126/jiaas.v27i0.696
Dane, F., Hunter, A. G., and Chambliss, O. L. (1991). Fruit set, pollen fertility, and combining ability of selected tomato genotypes under high temperature field conditions. J. Am. Soc. Hortic. Sci. 116, 906–910 doi: 10.21273/JASHS.116.5.906
Dang, F. F., Wang, Y. N., Yu, L., Eulgem, T., Lai, Y., Liu, Z. Q., et al. (2013). CaWRKY40, a WRKY protein of pepper, plays an important role in the regulation of tolerance to heat stress and resistance to Ralstonia solanacearum infection. Plant Cell Environ. 36, 757–774. doi: 10.1111/pce.12011
Day, J. M., Roughley, R. J., Eaglesham, A. R. J., Dye, M., and White, S. P. (1978). Effect of high soil temperatures on nodulation of cowpea, Vigna unguiculata. Ann. Appl. Biol. 88, 476–481. doi: 10.1111/j.1744-7348.1978.tb00747.x
Demirevska-Kepova, K., and Feller, U. (2004). Heat sensitivity of Rubisco, Rubisco activase and Rubisco binding protein in higher plants. Acta Physiol. Plant. 26, 103–114. doi: 10.1007/s11738-004-0050-7
Devasirvatham, V., Gaur, P. M., Mallikarjuna, N., Raju, T. N., Trethowan, R. M., and Tan, D. K. (2013). Reproductive biology of chickpea response to heat stress in the field is associated with the performance in controlled environments. Field Crops Res. 142, 9–19. doi: 10.1016/j.fcr.2012.11.011
Devi, A, P., Singh, S., and Das, S. P., Kabiraj, J. (2017). Effects of climate change on vegetables production-a review. Int. J. Curr. Microbiol. Appl. Sci. 6, 477–483. doi: 10.20546/ijcmas.2017.610.058
Dielen, V., Lecouvet, V., Dupont, S., and Kinet, J. M. (2001). In vitro control of floral transition in tomato (Lycopersicon esculentum Mill.), the model for autonomously flowering plants, using the late flowering uniflora mutant. J. Exp. Bot. 52, 715–723. doi: 10.1093/jexbot/52.357.715
Ding, X., Jiang, Y., Hao, T., Jin, H., Zhang, H., He, L., et al. (2016). Effects of heat shock on photosynthetic properties, antioxidant enzyme activity, and downy mildew of cucumber (Cucumis sativus L.). PLoS ONE 11, e152429. doi: 10.1371/journal.pone.0152429
Djanaguiraman, M., Devi, D. D., Shanker, A. K., Sheeba, J. A., and Bangarusamy, U. (2005). Selenium-an antioxidative protectant in soybean during senescence. Plant Soil 272, 77–86. doi: 10.1007/s11104-004-4039-1
Djanaguiraman, M., and Prasad, P. V. (2010). Ethylene production under high temperature stress causes premature leaf senescence in soybean. Funct. Plant Biol. 37, 1071–1084. doi: 10.1071/FP10089
Djanaguiraman, M., Prasad, P. V., Boyle, D. L., and Schapaugh, W. T. (2013b). Soybean pollen anatomy, viability and pod set under high temperature stress. J. Agron. Crop Sci. 199, 171–177. doi: 10.1111/jac.12005
Djanaguiraman, M., Prasad, P. V., and Schapaugh, W. T. (2013a). High day and night temperature alters leaf assimilation, reproductive success and phosphatidic acid of pollen grain in soybean (Glycine max L. Merr.). Crop Sci. 53, 1594–1604. doi: 10.2135/cropsci2012.07.0441
Djanaguiraman, M., Schapaugh, W., Fritschi, F., Nguyen, H., and Prasad, P. V. (2019). Reproductive success of soybean (Glycine max L. Merril) cultivars and exotic lines under high daytime temperature. Plant Cell Environ. 42, 321–336. doi: 10.1111/pce.13421
Driedonks, N., Xu, J., Peters, J. L., Park, S., and Rieu, I. (2015). Multi-level interactions between heat shock factors, heat shock proteins, and the redox system regulate acclimation to heat. Front. Plant Sci. 6, 999. doi: 10.3389/fpls.2015.00999
Duclos, D. V., and Björkman, T. (2008). Meristem identity gene expression during curd proliferation and flower initiation in Brassica oleracea. J. Exp. Bot. 59, 421–433. doi: 10.1093/jxb/erm327
Dutta, S., Mohanty, S., and Tripathy, B. C. (2009). Role of temperature stress on chloroplast biogenesis and protein import in pea. Plant Physiol. 150, 1050–1061. doi: 10.1104/pp.109.137265
Egli, D. B., and Wardlaw, I. F. (1980). Temperature response of seed growth characteristics of soybeans. J. Agron. 72, 560–564 doi: 10.2134/agronj1980.00021962007200030036x
Erickson, A. N., and Markhart, A. H. (2002). Flower developmental stage and organ sensitivity of bell pepper (Capsicum annuum L.) to elevated temperature. Plant Cell Environ. 25, 123–130. doi: 10.1046/j.0016-8025.2001.00807.x
Farnham, M. W., and Bjorkman, T. (2011). Breeding vegetables adapted to high temperatures: a case study with broccoli. Hort Sci. 46, 1093–1097. doi: 10.21273/HORTSCI.46.8.1093
Farooq, M., Bramley, H., Palta, J. A., and Siddique, K. H. (2011). Heat stress in wheat during reproductive and grain-filling phases. Crit. Rev. Plant Sci. 30, 491–507. doi: 10.1080/07352689.2011.615687
Farooq, M., Nadeem, F., Gogoi, N., Ullah, A., Alghamdi, S. S., Nayyar, H., et al. (2017). Heat stress in grain legumes during reproductive and grain-filling phases. Crop Pasture Sci. 68, 985–1005. doi: 10.1071/CP17012
Farquhar, G. D., and Sharkey, T. D. (1982). Stomatal conductance and photosynthesis. Annu. Rev. Plant Physiol. 33, 317–345. doi: 10.1146/annurev.pp.33.060182.001533
Feng, X. H., Zhang, H. X., Ali, M., Gai, W. X., Cheng, G. X., Yu, Q., et al. (2019). A small heat shock protein CaHsp25. 9 positively regulates heat, salt, and drought stress tolerance in pepper (Capsicum annuum L.). Plant Physiol. Biochem. 142, 151–162. doi: 10.1016/j.plaphy.2019.07.001
Ferrante, A., and Mariani, L. (2018). Agronomic management for enhancing plant tolerance to abiotic stresses: high and low values of temperature, light intensity, and relative humidity. Horticulturae 4, 1–20. doi: 10.3390/horticulturae4030021
Firmansyah, and Argosubekti, N. (2020). “A review of heat stress signaling in plants,” in IOP Conference Series: Earth and Environmental Science, 23-25 September 2019 (Makassar). Avaialble online at: https://iopscience.iop.org/issue/1755-1315/484/1
Firon, N., Shaked, R., Peet, M. M., Pharr, D. M., Zamski, E., Rosenfeld, K., et al. (2006). Pollen grains of heat tolerant tomato cultivars retain higher carbohydrate concentration under heat stress conditions. Sci. Hortic. 109, 212–217. doi: 10.1016/j.scienta.2006.03.007
Foolad, M. R. (2005). “Breeding for abiotic stress tolerances in tomato,” in Abiotic Stresses: Plant Resistance Through Breeding and Molecular Approaches, eds M. Ashraf and P. J. C. Harris (New York, NY: The Haworth Press Inc), 613–684.
Foyer, C. H., and Noctor, G. (2005). Redox homeostasis and antioxidant signaling: a metabolic interface between stress perception and physiological responses. Plant Cell 17, 1866–1875. doi: 10.1105/tpc.105.033589
Fu, I. M., Shennan, C., and Welbaum, G. E. (1993). “Evaluating Chinese cabbage cultivars for high temperature tolerance,” in Proceedings of the Second National Symposium New Crops: Exploration, Research and Commercialization, eds J. Janick and J. E. Simon (New York, NY: Wiley), 570–573.
Gajanayake, B., Trader, B. W., Reddy, K. R., and Harkess, R. L. (2011). Screening ornamental pepper cultivars for temperature tolerance using pollen and physiological parameters. Hort Sci. 46, 878–884. doi: 10.21273/HORTSCI.46.6.878
Gali, K. K., Sackville, A., Tafesse, E. G., Lachagari, V. B., McPhee, K., Hybl, M., et al. (2019). Genome-wide association mapping for agronomic and seed quality traits of field pea (Pisum sativum L.). Front. Plant Sci. 10, 1538. doi: 10.3389/fpls.2019.01538
Gaur, P. M., Jukanti, A. K., Samineni, S., Chaturvedi, S. K., Basu, P. S., Babbar, A., et al. (2013). “Climate change and heat stress tolerance in chickpea,” in Climate Change and Plant Abiotic Stress Tolerance, eds N. Tutuja and S. S. Gill (Weinheim: Wiley Blackwell), 837–856. doi: 10.1002/9783527675265.ch31
Georgieva, K., Tsonev, T., Velikova, V., and Yordanov, I. (2000). Photosynthetic activity during high temperature treatment of pea plants. J. Plant Physiol. 157, 169–176. doi: 10.1016/S0176-1617(00)80187-X
Gil, K. E., and Park, C. M. (2019). Thermal adaptation and plasticity of the plant circadian clock. New Phytol. 221, 1215–1229. doi: 10.1111/nph.15518
Gillman, J. D., Biever, J. J., Ye, S., Spollen, W. G., Givan, S. A., Lyu, Z., et al. (2019). A seed germination transcriptomic study contrasting two soybean genotypes that differ in terms of their tolerance to the deleterious impacts of elevated temperatures during seed fill. BMC Res. Notes 12, 1–8. doi: 10.1186/s13104-019-4559-7
Giri, A., Heckathorn, S., Mishra, S., and Krause, C. (2017). Heat stress decreases levels of nutrient-uptake and-assimilation proteins in tomato roots. Plants 6, 1–15. doi: 10.3390/plants6010006
Golam, F., Prodhan, Z. H., Nezhadahmadi, A., and Rahman, M. (2012). Heat tolerance in tomato. Life Sci. J. 9, 1936–1950.
Gonzalo, M. J., Li, Y. C., Chen, K. Y., Gil, D., Montoro, T., Nájera, I., et al. (2020). Genetic control of reproductive traits in tomatoes under high temperature. Front. Plant Sci. 11, 326. doi: 10.3389/fpls.2020.00326
Gowda, D. S., Singh, G. P., and Singh, A. M. (2011). Relationship between canopy temperature depression, membrane stability, relative water content and grain yield in bread wheat (Triticum aestivum) under heat-stress environments. Indian J. Agric. Sci. 81, 197–202.
Grilli, G. V. G., Braz, L. T., and Lemos, E. G. M. (2007). QTL identification for tolerance to fruit set in tomato by fAFLP markers. Crop. Breed. Appl. Biotechnol. 7, 234–241. doi: 10.12702/1984-7033.v07n03a02
Gross, J. (1991). Pigment in Vegetables: Chlorophylls and Carotenoids. New York, NY: Springer Science and Business Media.
Gross, Y., and Kigel, J. (1994). Differential sensitivity to high temperature of stages in the reproductive development of common bean (Phaseolus vulgaris L.). Field Crops Res. 36, 201–212. doi: 10.1016/0378-4290(94)90112-0
Grover, A., Mittal, D., Negi, M., and Lavania, D. (2013). Generating high temperature tolerant transgenic plants: achievements and challenges. Plant Sci. 205, 38–47. doi: 10.1016/j.plantsci.2013.01.005
Grover, M., Ali, S. Z., Sandhya, V., Rasul, A., and Venkateswarlu, B. (2011). Role of microorganisms in adaptation of agriculture crops to abiotic stresses. World J. Microbiol. Biotechnol. 27, 1231–1240. doi: 10.1007/s11274-010-0572-7
Gruda, N. (2005). Impact of environmental factors on product quality of greenhouse vegetables for fresh consumption. Crit. Rev. Plant Sci. 24, 227–247. doi: 10.1080/07352680591008628
Guilioni, L., We'ry, J., and Lecoeur, J. (2003). High temperature and water deficit may reduce seed number in field pea purely by decreasing plant growth rate. Funct. Plant Biol. 30, 1151–1164. doi: 10.1071/FP03105
Guilioni, L., Wery, J., and Tardieu, F. (1997). Heat stress-induced abortion of buds and flowers in pea: is sensitivity linked to organ age or to relations between reproductive organs?. Ann Bot. 80, 159–168. doi: 10.1006/anbo.1997.0425
Gulen, H., Ipek, A., and Turhan, E. (2012). “Effects of antioxidant enzymes on heat stress tolerance of pepper (Capsicum annuum L.) seedlings,” in International Symposium on Biotechnology and Other Omics in Vegetable Science (Antalya), 43–50.
Guo, M., Liu, J. H., Lu, J. P., Zhai, Y. F., Wang, H., Gong, Z. H., et al. (2015). Genome-wide analysis of the CaHsp20 gene family in pepper: comprehensive sequence and expression profile analysis under heat stress. Front. Plant Sci. 6, 806. doi: 10.3389/fpls.2015.00806
Guo, R., Wang, X., Han, X., Chen, X., and Wang-Pruski, G. (2020). Physiological and transcriptomic responses of water spinach (Ipomoea aquatica) to prolonged heat stress. BMC Genom. 21, 1–15. doi: 10.1186/s12864-020-06953-9
Guy, C., Kaplan, F., Kopka, J., Selbig, J., and Hincha, D. K. (2008). Metabolomics of temperature stress. Physiol. Plant. 132, 220–235. doi: 10.1111/j.1399-3054.2007.00999.x
Guy, C. L., and Li, Q. B. (1998). The organization and evolution of the spinach stress 70 molecular chaperone gene family. Plant Cell 10, 539–556. doi: 10.1105/tpc.10.4.539
Haldimann, P., and Feller, U. R. S. (2005). Growth at moderately elevated temperature alters the physiological response of the photosynthetic apparatus to heat stress in pea (Pisum sativum L.) leaves. Plant Cell Environ. 28, 302–317. doi: 10.1111/j.1365-3040.2005.01289.x
Hamada, A. M. (2001). Alteration in growth and some relevant metabolic processes of broad bean plants during extreme temperatures exposure. Acta Physiol. Plant. 23, 193–200. doi: 10.1007/s11738-001-0008-y
Han, Y., Fan, S., Zhang, Q., and Wang, Y. (2013). Effect of heat stress on the MDA, proline and soluble sugar content in leaf lettuce seedlings. Agric. Sci. 4, 112–115. doi: 10.4236/as.2013.45B021
Hancock, R. D., Morris, W. L., Ducreux, L. J., Morris, J. A., Usman, M., Verrall, S. R., et al. (2014). Physiological, biochemical and molecular responses of the potato (Solanum tuberosum L.) plant to moderately elevated temperature. Plant Cell Environ. 37, 439–450. doi: 10.1111/pce.12168
Handayani, T., and Watanabe, K. (2020). The combination of drought and heat stress has a greater effect on potato plants than single stresses. Plant Soil Environ. 66, 175–182. doi: 10.17221/126/2020-PSE
HanumanthaRao, B., Nair, R. M., and Nayyar, H. (2016). Salinity and high temperature tolerance in mungbean [Vigna radiata (L.) Wilczek] from a physiological perspective. Front. Plant Sci. 7, 957. doi: 10.3389/fpls.2016.00957
Hanying, W., Shenyan, S., Zhujun, Z., and Xinting, Y. (2001). Effects of high temperature stress on photosynthesis and chlorophyll fluorescence in sweet pepper (Capsicum fructescens L.). Acta Hortic. Sin. 28, 517–521.
Harsant, J., Pavlovic, L., Chiu, G., Sultmanis, S., and Sage, T. L. (2013). High temperature stress and its effect on pollen development and morphological components of harvest index in the C3 model grass Brachypodiumdistachyon. J. Exp. Bot. 64, 2971–2983. doi: 10.1093/jxb/ert142
Hasanuzzaman, M., Nahar, K., Alam, M., Roychowdhury, R., and Fujita, M. (2013). Physiological, biochemical, and molecular mechanisms of heat stress tolerance in plants. Int. J. Mol. Sci. 14, 9643–9684. doi: 10.3390/ijms14059643
Hashizume, K., and Watanabe, T. (1979). Influence of heating temperature on conformational changes of soybean proteins. Agr. Biol. Chem. 43, 683–690. doi: 10.1080/00021369.1979.10863529
Hassan, M. U., Chattha, M. U., Khan, I., Chattha, M. B., Barbanti, L., Aamer, M., et al. (2021). Heat stress in cultivated plants: nature, impact, mechanisms, and mitigation strategies-a review. Plant Biosyst. 155, 211–234. doi: 10.1080/11263504.2020.1727987
Hatfield, J. L., and Prueger, J. H. (2015). Temperature extremes: effect on plant growth and development. Weather. Clim. Extremes 10, 4–10. doi: 10.1016/j.wace.2015.08.001
Havaux, M. (1996). Short-term responses of photosystem I to heat stress. Photosynth. Res. 47, 85–97. doi: 10.1007/BF00017756
Havko, N. E., Das, M. R., McClain, A. M., Kapali, G., Sharkey, T. D., and Howe, G. A. (2020). Insect herbivory antagonizes leaf cooling responses to elevated temperature in tomato. Proc. Natl. Acad. Sci. U.S.A. 117, 2211–2217. doi: 10.1073/pnas.1913885117
Hayamanesh, S. (2018). The effect of high temperature on physiological and metabolic parameters and reproductive tissues of okra (Abelmoschus esculentus (L.) Moench) (Doctoral dissertation). The University of Sydney, Sydney, NSW, Australia.
Heckathorn, S. A., Poeller, G. J., Coleman, J. S., and Hallberg, R. L. (1996). Nitrogen availability alters patterns of accumulation of heat stress-induced proteins in plants. Oecologia 105, 413–418. doi: 10.1007/BF00328745
Hedhly, A. (2011). Sensitivity of flowering plant gametophytes to temperature fluctuations. Environ. Exp. Bot. 74, 9–16. doi: 10.1016/j.envexpbot.2011.03.016
Hedhly, A., Hormaza, J. I., and Herrero, M. (2009). Global warming and sexual plant reproduction. Trends Plant Sci. 14, 30–36. doi: 10.1016/j.tplants.2008.11.001
Hofmann, N. R. (2009). The plasma membrane as first responder to heat stress. Plant Cell 21, 2544–2544. doi: 10.1105/tpc.109.210912
Hu, Y., Zhang, T., Liu, Y., Li, Y., Wang, M., Zhu, B., et al. (2021). Pumpkin (Cucurbita moschata) HSP20 gene family identification and expression under heat stress. Front. Genet. 12:753953. doi: 10.3389/fgene.2021.753953
Hungria, M., and Franco, A. A. (1993). Effects of high temperature on nodulation and nitrogen fixation by Phaseolus vulgaris L. Plant Soil 149, 95–102. doi: 10.1007/BF00010766
Hungria, M., and Kaschuk, G. (2014). Regulation of N2 fixation and NO3–/NH4+ assimilation in nodulated and N-fertilized Phaseolus vulgaris L. exposed to high temperature stress. Environ. Exp. Bot. 98, 32–39. doi: 10.1016/j.envexpbot.2013.10.010
Hungria, M., and Vargas, M. A. (2000). Environmental factors affecting N2 fixation in grain legumes in the tropics, with an emphasis on Brazil. Field Crops Res. 65, 151–164. doi: 10.1016/S0378-4290(99)00084-2
Hurkman, W. J., Vensel, W. H., Tanaka, C. K., Whitehand, L., and Altenbach, S. B. (2009). Effect of high temperature on albumin and globulin accumulation in the endosperm proteome of the developing wheat grain. J. Cereal Sci. 49, 12–23. doi: 10.1016/j.jcs.2008.06.014
Imadi, S. R., Kazi, A. G., Ahanger, M. A., Gucel, S., and Ahmad, P. (2015). Plant transcriptomics and responses to environmental stress: an overview. J. Genet. 94, 525–537. doi: 10.1007/s12041-015-0545-6
IPCC (2021). The Physical Science Basis. Contribution of Working Group I to the Sixth Assessment Report of the Intergovernmental Panel on Climate Change. United Kingdom: Cambridge University Press.
Ismail, A. M., and Hall, A. E. (1999). Reproductive-stage heat tolerance, leaf membrane thermostability and plant morphology in cowpea. Crop Sci. 39, 1762–1768. doi: 10.2135/cropsci1999.3961762x
Iwabuchi, S., and Yamauchi, F. (1984). Effects of heat and ionic strength upon dissociation-association of soybean protein fractions. J. Food Sci. 49, 1289–1294. doi: 10.1111/j.1365-2621.1984.tb14971.x
Iwahori, S. (1965). High temperature injuries in tomato. IV. J. Jpn. Soc. Hortic. Sci. 34, 33–41. doi: 10.2503/jjshs.34.33
Jamil, M., Rehman, S., and Rha, E. S. (2007). Salinity effect on plant growth, PSII photochemistry and chlorophyll content in sugar beet (Beta vulgaris L.) and cabbage (Brassica oleracea capitata L.). Pak. J. Bot. 39, 753–760.
Janni, M., Gullì, M., Maestri, E., Marmiroli, M., Valliyodan, B., Nguyen, H. T., et al. (2020). Molecular and genetic bases of heat stress responses in crop plants and breeding for increased resilience and productivity. J. Exp. Bot. 71, 3780–3802. doi: 10.1093/jxb/eraa034
Jenni, S. (2005). Rib discoloration: a physiological disorder induced by heat stress in crisphead lettuce. Hort Sci. 40, 2031–2035. doi: 10.21273/HORTSCI.40.7.2031
Jenni, S., Truco, M. J., and Michelmore, R. W. (2013). Quantitative trait loci associated with tip burn, heat stress-induced physiological disorders, and maturity traits in crisp head lettuce. Theor. Appl. Genet. 126, 3065–3079. doi: 10.1007/s00122-013-2193-7
Jenni, S., and Yan, W. (2009). Genotype by environment interactions of heat stress disorder resistance in crisp head lettuce. Plant Breed. 128, 374–380. doi: 10.1111/j.1439-0523.2009.01657.x
Jeuffroy, M. H., Duthion, C., Meynard, J. M., and Pigeaire, A. (1990). Effect of a short period of high day temperatures during flowering on the seed number per pod of pea (Pisum sativum L). Agronomie 10, 139–145. doi: 10.1051/agro:19900207
Jha, U. C., Bohra, A., Parida, S. K., and Jha, R. (2017). Integrated “omics” approaches to sustain global productivity of major grain legumes under heat stress. Plant Breed. 136, 437–459. doi: 10.1111/pbr.12489
Jha, U. C., Bohra, A., and Singh, N. P. (2014). Heat stress in crop plants: its nature, impacts and integrated breeding strategies to improve heat tolerance. Plant Breed. 133, 679–701. doi: 10.1111/pbr.12217
Jha, U. C., Kole, P. C., and Singh, N. P. (2019). QTL mapping for heat stress tolerance in chickpea (Cicer arietinum L.). Legume Res. Int. J. 44, 382–387. doi: 10.18805/LR-4121
Jha, U. C., Palakurthi, R., Nayyar, H., Jha, R., Valluri, V. K., Bajaj, P., et al. (2021). Major QTLs and potential candidate genes for heat stress tolerance identified in chickpea (Cicer arietinum L.). Front. Plant Sci. 12, 1241. doi: 10.3389/fpls.2021.655103
Jiang, Y., Lahlali, R., Karunakaran, C., Kumar, S., Davis, A. R., and Bueckert, R. A. (2015). Seed set, pollen morphology and pollen surface composition response to heat stress in field pea. Plant Cell Environ. 38, 2387–2397. doi: 10.1111/pce.12589
Joshi, A. K., Kumari, M., Singh, V. P., Reddy, C. M., Kumar, S., Rane, J., et al. (2007). Stay green trait: variation, inheritance and its association with spot blotch resistance in spring wheat (Triticum aestivum L.). Euphytica 153, 59–71. doi: 10.1007/s10681-006-9235-z
Julia, C., and Dingkuhn, M. (2013). Predicting temperature induced sterility of rice spikelets requires simulation of crop-generated microclimate. Eur. J. Agron. 49, 50–60. doi: 10.1016/j.eja.2013.03.006
Kakani, V. G., Prasad, P. V. V., Craufurd, P. Q., and Wheeler, T. R. (2002). Response of in vitro pollen germination and pollen tube growth of groundnut (Arachis hypogaea L.) genotypes to temperature. Plant Cell Environ. 25, 1651–1661. doi: 10.1046/j.1365-3040.2002.00943.x
Kaloki, P., Devasirvatham, V., and Tan, D. K. (2019). “Chickpea abiotic stresses: combating drought, heat and cold,” in Abiotic and Biotic Stress in Plants, ed A. B. de Oliveira (London: IntechOpen), 139–162.
Kamal, N. M., Gorafi, Y. S. A., Abdelrahman, M., Abdellatef, E., and Tsujimoto, H. (2019). Stay-green trait: a prospective approach for yield potential, and drought and heat stress adaptation in globally important cereals. Int. J. Mol. Sci. 20, 1–16. doi: 10.3390/ijms20235837
Kang, Y., Lee, K., Hoshikawa, K., Kang, M., and Jang, S. (2022). Molecular bases of heat stress responses in vegetable crops with focusing on heat shock factors and heat shock proteins. Front. Plant Sci. 13, 837152. doi: 10.3389/fpls.2022.837152
Karanja, N. K., and Wood, M. (1988). Selecting Rhizobium phaseoli strains for use with beans (Phaseolus vulgaris L.) in Kenya: infectiveness and tolerance of acidity and aluminium. Plant Soil 112, 7–13. doi: 10.1007/BF02181746
Kato, M. C., Hikosaka, K., Hirotsu, N., Makin, A., and Hirose, T. (2003). The excess light energy that is neither utilized in photosynthesis nor dissipated by photoprotective mechanisms determines the rate of photoinactivation in photosystem II. Plant Cell Physiol. 44, 318–325. doi: 10.1093/pcp/pcg045
Kaur, P., Ghai, N., and Sangha, M. K. (2009). Induction of thermotolerance through heat acclimation and salicylic acid in Brassica species. Afr. J. Biotechnol. 8, 619–625.
Kaur, R., Bains, T. S., Bindumadhava, H., and Nayyar, H. (2015). Responses of mungbean (Vigna radiata L.) genotypes to heat stress: effects on reproductive biology, leaf function and yield traits. Sci. Hortic. 197, 527–541. doi: 10.1016/j.scienta.2015.10.015
Kaushal, N., Awasthi, R., Gupta, K., Gaur, P., Siddique, K. H. M., and Nayyar, H. (2013). Heat-stress-induced reproductive failures in chickpea (Cicer arietinum) are associated with impaired sucrose metabolism in leaves and anthers. Funct. Plant Biol. 40, 1334–1349. doi: 10.1071/FP13082
Kaushal, N., Bhandari, K., Siddique, K. H. M., and Nayyar, H. (2016). Food crops face rising temperatures: an overview of responses, adaptive mechanisms, and approaches to improve heat tolerance. Cogent Food Agric. 2, 1–42. doi: 10.1080/23311932.2015.1134380
Kaushal, N., Gupta, K., Bhandhari, K., Kumar, S., Thakur, P., and Nayyar, H. (2011). Proline induces heat tolerance in chickpea (Cicer arietinum L.) plants by protecting vital enzymes of carbon and antioxidative metabolism. Physiol. Mol. Biol. Plants 17, 203–213. doi: 10.1007/s12298-011-0078-2
Khan, Z., and Shahwar, D. (2020). “Role of heat shock proteins (HSPs) and heat stress tolerance in crop plants,” in Sustainable Agriculture in the Era of Climate Change, eds R. Roychowdhury, S. Choudhury, M. Hasanuzzaman, and S. Srivastava (Cham: Springer), 211–234.
Khattak, G. S. S., Saeed, I., and Muhammad, T. (2006). Breeding for heat tolerance in mungbean (Vigna radiata (L.) Wilczek). Pak. J. Bot. 38, 1539–1550.
Killi, D., Raschi, A., and Bussotti, F. (2020). Lipid peroxidation and chlorophyll fluorescence of photosystem II performance during drought and heat stress is associated with the antioxidant capacities of C3 sunflower and C4 maize varieties. Int. J. Mol. Sci. 21, 1–21. doi: 10.3390/ijms21144846
Kladivko, E. J. (2001). Tillage systems and soil ecology. Soil Tillage Res. 61, 61–76. doi: 10.1016/S0167-1987(01)00179-9
Klebs, G. S. B. A. W. H. S. B. (1913). Uber des Verhaltnis der Aubenwelt zur Entwicklung der Pflanze. Sitz-Ber. Akad. Wiss. Heidelberg Ser. B. 5, 3–47.
Konsens, I., Ofir, M., and Kigel, J. (1991). The effect of temperature on the production and abscission of flowers and pods in snap bean (Phaseolus vulgaris L.). Ann. Bot. 67, 391–399. doi: 10.1093/oxfordjournals.aob.a088173
Kumar, M., Govindasamy, V., Rane, J., Singh, A. K., Choudhary, R. L., Raina, S. K., et al. (2017). Canopy temperature depression (CTD) and canopy greenness associated with variation in seed yield of soybean genotypes grown in semi-arid environment. S. Afr. J. Bot. 113, 230–238. doi: 10.1016/j.sajb.2017.08.016
Kumar, R., Lavania, D., Singh, A. K., Negi, M., Siddiqui, M. H., Al-Whaibi, M. H., et al. (2015). Identification and characterization of a small heat shock protein 17.9-CII gene from faba bean (Vicia faba L.). Acta Physiol. Plant. 37, 1–13. doi: 10.1007/s11738-015-1943-3
Kumar, S., Kaur, R., Kaur, N., Bhandhari, K., Kaushal, N., Gupta, K., et al. (2011). Heat-stress induced inhibition in growth and chlorosis in mungbean (Phaseolus aureus Roxb.) is partly mitigated by ascorbic acid application and is related to reduction in oxidative stress. Acta Physiol. Plant. 33, 2091–2101. doi: 10.1007/s11738-011-0748-2
Kumar, S., Patra, A. K., Singh, D., and Purakayastha, T. J. (2014). Long-term chemical fertilization along with farmyard manure enhances resistance and resilience of soil microbial activity against heat stress. J. Agron. Crop Sci. 200, 156–162. doi: 10.1111/jac.12050
Kumar, S., Thakur, P., Kaushal, N., Malik, J. A., Gaur, P., and Nayyar, H. (2013). Effect of varying high temperatures during reproductive growth on reproductive function, oxidative stress and seed yield in chickpea genotypes differing in heat sensitivity. Arch. Agron. Soil Sci. 59, 823–843. doi: 10.1080/03650340.2012.683424
Kumari, M., Pudake, R. N., Singh, V. P., and Joshi, A. K. (2013). Association of staygreen trait with canopy temperature depression and yield traits under terminal heat stress in wheat (Triticum aestivum L.). Euphytica 190, 87–97. doi: 10.1007/s10681-012-0780-3
Kusaba, M., Tanaka, A., and Tanaka, R. (2013). Stay-green plants: what do they tell us about the molecular mechanism of leaf senescence. Photosynth. Res. 117, 221–234. doi: 10.1007/s11120-013-9862-x
Kushwah, A., Bhatia, D., Singh, I., Thudi, M., Singh, G., Bindra, S., et al. (2021). Identification of stable heat tolerance QTLs using inter-specific recombinant inbred line population derived from GPF 2 and ILWC 292. PLoS ONE 16, e0254957. doi: 10.1371/journal.pone.0254957
Larmure, A., Salon, C., and Munier-Jolain, N. G. (2005). How does temperature affect C and N allocation to the seeds during the seed-filling period in pea? Effect on seed nitrogen concentration. Funct. Plant Biol. 32, 1009–1017. doi: 10.1071/FP05154
Levy, A., Rabinowitch, H. D., and Kedar, N. (1978). Morphological and physiological characters affecting flower drop and fruit set of tomatoes at high temperatures. Euphytica 27, 211–218. doi: 10.1007/BF00039137
Leyva, R., Constán-Aguilar, C., Blasco, B., Sánchez-Rodríguez, E., Soriano, T., and Ruíz, J. M. (2013). A fogging system improves antioxidative defense responses and productivity in tomato. J. Am. Soc. Hortic. Sci. 138, 267–276. doi: 10.21273/JASHS.138.4.267
Li, B., Gao, K., Ren, H., and Tang, W. (2018). Molecular mechanisms governing plant responses to high temperatures. J. Integr. Plant Biol. 60, 757–779. doi: 10.1111/jipb.12701
Li, P., Cheng, L., Gao, H., Jiang, C., and Peng, T. (2009). Heterogeneous behavior of PSII in soybean (Glycine max) leaves with identical PSII photochemistry efficiency under different high temperature treatments. J. Plant Physiol. 166, 1607–1615. doi: 10.1016/j.jplph.2009.04.013
Li, S., Yu, J., Li, Y., Zhang, H., Bao, X., Bian, J., et al. (2019). Heat-responsive proteomics of a heat-sensitive spinach variety. Int. J. Mol. Sci. 20, 3872. doi: 10.3390/ijms20163872
Li, T., Xu, X., Li, Y., Wang, H., Li, Z., and Li, Z. (2015). Comparative transcriptome analysis reveals differential transcription in heat-susceptible and heat-tolerant pepper (Capsicum annum L.) cultivars under heat stress. J. Integr. Plant Biol. 58, 411–424. doi: 10.1007/s12374-015-0423-z
Lim, P. O., Kim, H. J., and Gil Nam, H. (2007). Leaf senescence. Annu. Rev. Plant Biol. 58, 115–136. doi: 10.1146/annurev.arplant.57.032905.105316
Lin, C. W., Fu, S. F., Liu, Y. J., Chen, C. C., Chang, C. H., Yang, Y. W., et al. (2019). Analysis of ambient temperature-responsive transcriptome in shoot apical meristem of heat-tolerant and heat-sensitive broccoli inbred lines during floral head formation. BMC Plant Biol. 19, 1–16. doi: 10.1186/s12870-018-1613-x
Lin, K. H., Chen, L. F. O., Li, S. D., and Lo, H. F. (2015). Comparative proteomic analysis of cauliflower under high temperature and flooding stresses. Sci. Hortic. 183, 118–129. doi: 10.1016/j.scienta.2014.12.013
Lin, K. H., Lo, H. F., Lee, S. P., George Kuo, C., Chen, J. T., and Yeh, W. L. (2006). RAPD markers for the identification of yield traits in tomatoes under heat stress via bulked segregant analysis. Hereditas. 143, 142–154. doi: 10.1111/j.2006.0018-0661.01938.x
Link, V., Sinha, A., Vashista, P., Hofmann, M., Proels, R., Ehness, R., et al. (2002). A heat-activated MAP kinase in tomato: a possible regulator of the heat stress response. FEBS Lett. 531, 179–183. doi: 10.1016/S0014-5793(02)03498-1
Liu, Y., Dong, S., Wei, S., Wang, W., Miao, H., Bo, K., et al. (2021). QTL mapping of heat tolerance in cucumber (Cucumis sativus L.) at adult stage. Plants 10, 324. doi: 10.3390/plants10020324
Lobell, D. B., and Bonfils, C. (2008). The effect of irrigation on regional temperatures: a spatial and temporal analysis of trends in California 1934–2002. J. Clim. 21, 2063–2071. doi: 10.1175/2007JCLI1755.1
Lucas, M. R., Ehlers, J. D., Huynh, B. L., Diop, N. N., Roberts, P. A., and Close, T. J. (2013). Markers for breeding heat-tolerant cowpea. Mol. Breed. 31, 529–536. doi: 10.1007/s11032-012-9810-z
Luche, H. D. S., Silva, J. A. G. D., Maia, L. C. D., and Oliveira, A. C. D. (2015). Stay-green: a potentiality in plant breeding. Ciência Rural. 45, 1755–1760. doi: 10.1590/0103-8478cr20140662
Lyu, J. I., Park, J. H., Kim, J. K., Bae, C. H., Jeong, W. J., Min, S. R., et al. (2018). Enhanced tolerance to heat stress in transgenic tomato seeds and seedlings overexpressing a trehalose-6-phosphate synthase/phosphatase fusion gene. Plant Biotechnol. Rep. 12, 399–408. doi: 10.1007/s11816-018-0505-8
Ma, J., Xu, Z. S., Wang, F., Tan, G. F., Li, M. Y., and Xiong, A. S. (2014). Genome-wide analysis of HSF family transcription factors and their responses to abiotic stresses in two Chinese cabbage varieties. Acta Physiol. Plant. 36, 513–523. doi: 10.1007/s11738-013-1432-5
Makonya, G. M., Ogola, J. B., Muasya, A. M., Crespo, O., Maseko, S., Valentine, A. J., et al. (2019). Chlorophyll fluorescence and carbohydrate concentration as field selection traits for heat tolerant chickpea genotypes. Plant Physiol. Biochem. 141, 172–182. doi: 10.1016/j.plaphy.2019.05.031
Marcum, K. B. (1998). Cell membrane thermostability and whole-plant heat tolerance of Kentucky bluegrass. Crop Sci. 38, 1214–1218. doi: 10.2135/cropsci1998.0011183X003800050017x
Martineau, J. R., Specht, J. E., Williams, J. H., and Sullivan, C. Y. (1979). Temperature tolerance in soybeans. I. Evaluation of a technique for assessing cellular membrane thermostability. Crop Sci. 19, 75–78. doi: 10.2135/cropsci1979.0011183X001900010017x
Matthews, J. S., Vialet-Chabrand, S., and Lawson, T. (2018). Acclimation to fluctuating light impacts the rapidity of response and diurnal rhythm of stomatal conductance. Plant Physiol. 176, 1939–1951. doi: 10.1104/pp.17.01809
Maxwell, K., and Johnson, G. N. (2000). Chlorophyll fluorescence-a practical guide. J. Exp Bot. 51, 659–668. doi: 10.1093/jexbot/51.345.659
Mazorra, L. M., Nunez, M., Hechavarria, M., Coll, F., and Sánchez-Blanco, M. J. (2002). Influence of brassinosteroids on antioxidant enzymes activity in tomato under different temperatures. Biol. Plant. 45, 593–596. doi: 10.1023/A:1022390917656
McDonald, G. K., and Paulsen, G. M. (1997). High temperature effects on photosynthesis and water relations of grain legumes. Plant Soil 196, 47–58. doi: 10.1023/A:1004249200050
Meena, N. L., Ali, K., Deshmukh, P., and Tyagi, A. (2017). Thermotolerance in chickpea: physio-biochemical analysis. Acta Sci. Agric. 1, 22–27.
Mengel, K., Kosegarten, H., Kirkby, E. A., and Appel, T. (2001). Principles of Plant Nutrition. Dordrecht: Springer.
Miah, M. A. K., Anwar, M. P., Begum, M., Juraimi, A. S., and Islam, M. A. (2009). Influence of sowing date on growth and yield of summer mungbean varieties. J. Agric. Soc. Sci. 5, 73–76.
Michiels, J., Verreth, C., and Vanderleyden, J. (1994). Effects of temperature stress on bean-nodulating Rhizobium strains. Appl. Environ. Microbiol. 60, 1206–1212. doi: 10.1128/aem.60.4.1206-1212.1994
Minhas, J. S., Singh, B., Kumar, D., Joseph, T. A., and Prasad, K. S. K. (2001). Selection of heat tolerant potato genotypes and their performance under heat stress. J. Indian Potato Assoc. 28, 132–134.
Mittler, R., Finka, A., and Goloubinoff, P. (2012). How do plants feel the heat? Trends Biochem. Sci. 37, 118–125. doi: 10.1016/j.tibs.2011.11.007
Modi, R., and Khanna, V. (2018). Evaluation of thermotolerant rhizobacteria for multiple plant growth promoting traits from pigeonpea rhizosphere. J. Appl. Nat. Sci. 10, 518–521. doi: 10.31018/jans.v10i1.1660
Moradpour, M., Abdullah, S. N. A., and Namasivayam, P. (2021). The impact of heat stress on morpho-physiological response and expression of specific genes in the heat stress-responsive transcriptional regulatory network in Brassica oleracea. Plants 10, 1–19. doi: 10.3390/plants10061064
Moretti, C. L., Mattos, L. M., Calbo, A. G., and Sargent, S. A. (2010). Climate changes and potential impacts on postharvest quality of fruit and vegetable crops: a review. Food Res. Int. 43, 1824–1832. doi: 10.1016/j.foodres.2009.10.013
Morrison, M. J., and Stewart, D. W. (2002). Heat stress during flowering in summer Brassica. Crop Sci. 42, 797–803. doi: 10.2135/cropsci2002.7970
Mullan, D., and Pietragalla, J. (2012). “Leaf relative water content,” in Physiological Breeding II: A Field Guide to Wheat Phenotyping, eds A. J. D. Pask, J. Pietragalla, D. M. Mullan, and M. P. Reynolds (Mexico: CIMMYT), 25–27.
Mulumba, L. N., and Lal, R. (2008). Mulching effects on selected soil physical properties. Soil Tillage Res. 98, 106–111. doi: 10.1016/j.still.2007.10.011
Munjal, R., and Rana, R. K. (2003). “Evaluation of physiological traits in wheat (Triticum aestivum L.) for terminal high temperature tolerance,” in Proceedings of the Tenth International Wheat Genetics Symposium (Poestum), 804–805.
Muralidharan, B., Sadhur Ram, A., Chandra, S., Tamilselvan, A., and Yuvasakthi, S. (2016). Effects of climate change on vegetable crops- a review. J. Agroecol. Nat. Resour. Manag. 3, 299–301. doi: 10.5958/j.2230-732X.7.1.020
Nagarajan, S., and Bansal, K. C. (1986). Measurement of cellular membrane thermostability to evaluate foliage heat tolerance of potato. Potato Res. 29, 163–167. doi: 10.1007/BF02361989
Nahar, K., Hasanuzzaman, M., Ahamed, K. U., Hakeem, K. R., Ozturk, M., and Fujita, M. (2015). “Plant responses and tolerance to high temperature stress: role of exogenous phytoprotectants,” in Crop Production and Global Environmental Issues, ed K. R. Hakeem (Cham: Springer), 385–435.
Nahar, K., Hasanuzzaman, M., and Fujita, M. (2016). “Heat stress responses and thermotolerance in soybean,” in Abiotic and Biotic Stresses in Soybean Production, ed M. Miransari (Cambridge, MA: Academic Press), 261–284.
Neta-Sharir, I., Isaacson, T., Lurie, S., and Weiss, D. (2005). Dual role for tomato heat shock protein 21: protecting photosystem II from oxidative stress and promoting color changes during fruit maturation. Plant Cell 17, 1829–1838. doi: 10.1105/tpc.105.031914
Nyarko, G., Alderson, P. G., Craigon, J., Murchie, E., and Sparkes, D. L. (2008). Comparison of cell membrane thermostability and chlorophyll fluorescence parameters for the determination of heat tolerance in ten cabbage lines. J. Hortic. Sci. Biotechnol. 83, 678–682. doi: 10.1080/14620316.2008.11512443
Omae, H., Kumar, A., and Shono, M. (2012). Adaptation to high temperature and water deficit in the common bean (Phaseolus vulgaris L.) during the reproductive period. J. Bot. 2012, 1–6. doi: 10.1155/2012/803413
Ormrod, D. P., Woolley, C. J., Eaton, G. W., and Stobbe, E. H. (1967). Effect of temperature on embryo sac development in Phaseolus vulgaris L. Can. J. Bot. 45, 948–950. doi: 10.1139/b67-097
Ortiz, C., and Cardemil, L. (2001). Heat-shock responses in two leguminous plants:a comparative study. J. Exp. Bot.1711–1719. doi: 10.1093/jxb/52.361.1711
Osagie, A. U., and Eka, O. U. (1998). Nutritional Quality of Plant Foods. Benin: University of Benin; Department of Biochemistry; Post-Harvest Research Unit.
Oyama, H., Shinohara, Y., and Ito, T. (1999). Effects of air temperature and light intensity on β-carotene concentration in spinach and lettuce. J. Jpn. Soc. Hortic. Sci. 68, 414–420. doi: 10.2503/jjshs.68.414
Parankusam, S., Bhatnagar-Mathur, P., and Sharma, K. K. (2017). Heat responsive proteome changes reveal molecular mechanisms underlying heat tolerance in chickpea. Environ. Exp. Bot. 141, 132–144. doi: 10.1016/j.envexpbot.2017.07.007
Park, H., Jung, W., Lee, S., Song, J., Kwon, S. Y., Kim, H., et al. (2013). Use of heat stress responsive gene expression levels for early selection of heat tolerant cabbage (Brassica oleracea L.). Int. J. Mol. Sci. 14, 11871–11894. doi: 10.3390/ijms140611871
Park, Y. J., Lee, H. J., Gil, K. E., Kim, J. Y., Lee, J. H., Lee, H., et al. (2019). Developmental programming of thermonastic leaf movement. Plant Physiol. 180, 1185–1197. doi: 10.1104/pp.19.00139
Parmar, N., Singh, K. H., Sharma, D., Singh, L., Kumar, P., Nanjundan, J., et al. (2017). Genetic engineering strategies for biotic and abiotic stress tolerance and quality enhancement in horticultural crops: a comprehensive review. Biotech. 7, 1–35. doi: 10.1007/s13205-017-0870-y
Parrotta, L., Aloisi, I., Faleri, C., Romi, M., Del Duca, S., and Cai, G. (2020). Chronic heat stress affects the photosynthetic apparatus of Solanum lycopersicum L. cv Micro-Tom. Plant Physiol. Biochem. 154, 463–475. doi: 10.1016/j.plaphy.2020.06.047
Pastenes, C., Porter, V., Baginsky, C., Horton, P., and González, J. (2004). Paraheliotropism can protect water-stressed bean (Phaseolus vulgaris L.) plants against photoinhibition. J. Plant Physiol. 161, 1315–1323. doi: 10.1016/j.jplph.2003.09.002
Paudel, S., Sah, L. P., Devkota, M., Poudyal, V., Prasad, P. V., and Reyes, M. R. (2020). Conservation agriculture and integrated pest management practices improve yield and income while reducing labor, pests, diseases and chemical pesticide use in smallholder vegetable farms in Nepal. Sustainability 12, 1–16. doi: 10.3390/su12166418
Paul, P. J., Samineni, S., Thudi, M., Sajja, S. B., Rathore, A., Das, R. R., et al. (2018). Molecular mapping of QTLs for heat tolerance in chickpea. Int. J. Mol. Sci. 19, E2166. doi: 10.3390/ijms19082166
Paul, S., Gogoi, N., Sarma, B., and Baroowa, B. (2014). Biochemical changes in potato under elevated temperature. Indian J. Plant Physiol. 19, 36–42. doi: 10.1007/s40502-014-0066-y
Paupière, M. J., Müller, F., Li, H., Rieu, I., Tikunov, Y. M., Visser, R. G., et al. (2017a). Untargeted metabolomic analysis of tomato pollen development and heat stress response. Plant Reprod. 30, 81–94. doi: 10.1007/s00497-017-0301-6
Paupière, M. J., van Haperen, P., Rieu, I., Visser, R. G., Tikunov, Y. M., and Bovy, A. G. (2017b). Screening for pollen tolerance to high temperatures in tomato. Euphytica 213, 1–8. doi: 10.1007/s10681-017-1927-z
Pedersen, P. (2003). “Variety selection is crucial to soybean yield and quality,” in Integrated Crop Management News (Ames, IA: Iowa State University), 19–20.
Peet, M. M., Sato, S., and Gardner, R. G. (1998). Comparing heat stress effects on male-fertile and male-sterile tomatoes. Plant Cell Environ. 21, 225–231. doi: 10.1046/j.1365-3040.1998.00281.x
Peet, M. M., Willits, D. H., and Gardner, R. (1997). Response of ovule development and post-pollen production processes in male-sterile tomatoes to chronic, sub-acute high temperature stress. J. Exp. Bot. 48, 101–111. doi: 10.1093/jxb/48.1.101
Peet, M. M., and Wolfe, D. W. (2000). “Crop ecosystem responses to climate change: vegetable crops,” in Climate Change and Global Crop Productivity, eds H. F. Hodges and K. R. Reddy (New York, NY; Wallingford: CABI Publishing), 213–243.
Petkova, V., Denev, I. D., Cholakov, D., and Porjazov, I. (2007). Field screening for heat tolerant common bean cultivars (Phaseolus vulgaris L.) by measuring of chlorophyll fluorescence induction parameters. Sci. Hortic. 111, 101–106. doi: 10.1016/j.scienta.2006.10.005
Pham, D., Hoshikawa, K., Fujita, S., Fukumoto, S., Hirai, T., Shinozaki, Y., et al. (2020). A tomato heat-tolerant mutant shows improved pollen fertility and fruit-setting under long-term ambient high temperature. Environ. Exp. Bot. 178, 104150. doi: 10.1016/j.envexpbot.2020.104150
Piha, M. I., and Munns, D. N. (1987). Sensitivity of the common bean (Phaseolus vulgaris L.) symbiosis to high soil temperature. Plant Soil 98, 183–194. doi: 10.1007/BF02374822
Polowick, P. L., and Sawhney, V. K. (1988). High temperature induced male and female sterility in canola (Brassica napus L.). Ann. Bot. 62, 83–86. doi: 10.1093/oxfordjournals.aob.a087639
Porch, T. G., and Jahn, M. (2001). Effects of high temperature stress on microsporogenesis in heat-sensitive and heat-tolerant genotypes of Phaseolus vulgaris. Plant Cell Environ. 24, 723–731. doi: 10.1046/j.1365-3040.2001.00716.x
Pottorff, M. O., Roberts, P. A., Close, T. J., Lonardi, S., Wanamaker, S., and Ehlers, J. D. (2014). Identification of candidate genes and molecular markers for heat-induced brown discoloration of seed coats in cowpea [Vigna unguiculata (L.) Walp]. BMC Genom. 15, 328. doi: 10.1186/1471-2164-15-328
Poudyal, D., Rosenqvist, E., and Ottosen, C. O. (2018). Phenotyping from lab to field-tomato lines screened for heat stress using Fv/Fm maintain high fruit yield during thermal stress in the field. Funct Plant Biol. 46, 44–55. doi: 10.1071/FP17317
Prasad, P. V., Bheemanahalli, R., and Jagadish, S. K. (2017). Field crops and the fear of heat stress-opportunities, challenges and future directions. Field Crops Res. 200, 114–121. doi: 10.1016/j.fcr.2016.09.024
Prasad, P. V. V., Boote, K. J., Allen, L. H. Jr., and Thomas, J. M. (2002). Effects of elevated temperature and carbon dioxide on seed set and yield of kidney bean (Phaseolus vulgaris L.). Glob. Chang Biol. 8, 710–721. doi: 10.1046/j.1365-2486.2002.00508.x
Prasad, P. V. V., Craufurd, P. Q., Summerfield, R. J., and Wheeler, T. R. (2000). Effects of short episodes of heat stress on flower production and fruit-set of groundnuts (Arachis hypogaea L.). J. Exp Bot. 51, 777–784. doi: 10.1093/jxb/51.345.777
Prasad, P. V. V., Pisipati, S. R., Mutava, R. N., and Tuinstra, M. R. (2008). Sensitivity of grain sorghum to high temperature stress during reproductive development. Crop Sci. 48, 1911–1917. doi: 10.2135/cropsci2008.01.0036
Preczewski, P. J., Heckathorn, S. A., Downs, C. A., and Coleman, J. S. (2000). Photosynthetic thermotolerance is quantitatively and positively correlated with production of specific heat-shock proteins among nine genotypes of Lycopersicon (tomato). Photosynthetica 38, 127–134 doi: 10.1023/A:1026760311255
Pressman, E., Peet, M. M., and Pharr, D. M. (2002). The effect of heat stress on tomato pollen characteristics is associated with changes in carbohydrate concentration in the developing anthers. Ann. Bot. 90, 631–636. doi: 10.1093/aob/mcf240
Pumphrey, F. V., and Ramig, R. E. (1990). Field response of peas to excess heat during the reproductive stage of growth. Am. Soc. Hortic. Sci. 115, 898–900. doi: 10.21273/JASHS.115.6.898
Puneeth, P. (2018). Evaluation of Hot pepper (Capsicum annuum L.) genotypes for heat stress tolerance (Doctoral dissertation). Division of Vegetable Science ICAR-Indian Agricultural Research Institute, New Delhi, India.
Quint, M., Delker, C., Franklin, K. A., Wigge, P. A., Halliday, K. J., and Van Zanten, M. (2016). Molecular and genetic control of plant thermomorphogenesis. Nat. Plants 2, 1–9. doi: 10.1038/nplants.2015.190
Rao, G. U., Jain, A., and Shivanna, K. R. (1992). Effects of high temperature stress on Brassica pollen: viability, germination and ability to set fruits and seeds. Ann. Bot. 69, 193–198. doi: 10.1093/oxfordjournals.aob.a088329
Reddy, K. R., and Kakani, V. G. (2007). Screening Capsicum species of different origins for high temperature tolerance by in vitro pollen germination and pollen tube length. Sci. Hort. 112, 130–135 doi: 10.1016/j.scienta.2006.12.014
Reynolds, M. P., and Ewing, E. E. (1989). Heat tolerance in tuber bearing Solanum species: a protocol for screening. Am. Potato J. 66, 63–74. doi: 10.1007/BF02854425
Rivero, R. M., Ruiz, J. M., and Romero, L. (2004). Oxidative metabolism in tomato plants subjected to heat stress. J. Hortic. Sci. Biotechnol. 79, 560–564. doi: 10.1080/14620316.2004.11511805
Ro, S., Chea, L., Ngoun, S., Stewart, Z. P., Roeurn, S., Theam, P., et al. (2021). Response of tomato genotypes under different high temperatures in field and greenhouse conditions. Plants 10, 1–13. doi: 10.3390/plants10030449
Royer, M., Larbat, R., Le Bot, J., Adamowicz, S., and Robin, C. (2013). Is the C: N ratio a reliable indicator of C allocation to primary and defence-related metabolisms in tomato?. Phytochemistry 88, 25–33. doi: 10.1016/j.phytochem.2012.12.003
Ru, C., Hu, x., Chen, D., Song, T., Wang, W., Lv, M., et al. (2022). Nitrogen modulates the effects of short-term heat, drought and combined stresses after anthesis on photosynthesis, nitrogen metabolism, yield, and water and nitrogen use efficiency of wheat. Water 14, 1–23. doi: 10.3390/w14091407
Ruane, J., and Sonnino, A. (2007). “Marker-assisted selection as a tool for genetic improvement of crops, livestock, forestry and fish in developing countries: an overview of the issues,” in Marker-Assisted Selection, eds E. P. Guimaraes, J. Ruane, B. D. Scherf, A. Sonnino, and J. D. Dargie (Rome: FAO), 3–13.
Rudich, J., Zamski, E., and Regev, Y. (1977). Genotypic variation for sensitivity to high temperature in the tomato: pollination and fruit set. Bot. Gaz. 138, 448–452. doi: 10.1086/336947
Sabir, N., and Singh, B. (2013). Protected cultivation of vegetables in global arena: a review. Indian J. Agric. Sci. 83, 123–135.
Sadder, M. T., Alsadon, A., and Wahb-Allah, M. (2014). Transcriptomic analysis of tomato lines reveals putative stress-specific biomarkers. Turk. J. Agric. For. 38, 700–715. doi: 10.3906/tar-1312-17
Sage, T. L., Bagha, S., Lundsgaard-Nielsen, V., Branch, H. A., Sultmanis, S., and Sage, R. F. (2015). The effect of high temperature stress on male and female reproduction in plants. Field Crops Res. 182, 30–42. doi: 10.1016/j.fcr.2015.06.011
Saha, S. R., Hossain, M. M., Rahman, M. M., Kuo, C. G., and Abdullah, S. (2010). Effect of high temperature stress on the performance of twelve sweet pepper genotypes. Bangladesh J. Agr. Res. 35, 525–534. doi: 10.3329/bjar.v35i3.6459
Saini, H. S., and Aspinall, D. (1982). Abnormal sporogenesis in wheat (Triticum aestivum L.) induced by short periods of high temperature. Ann. Bot. 49, 835–846. doi: 10.1093/oxfordjournals.aob.a086310
Saini, H. S., Sedgley, M., and Aspinall, D. (1983). Effect of heat stress during floral development on pollen tube growth and ovary anatomy in wheat (Triticum aestivum L.). Funct. Plant Biol. 10, 137–144. doi: 10.1071/PP9830137
Sakamoto, A., and Murata, N. (2002). The role of glycine betaine in the protection of plants from stress: clues from transgenic plants. Plant Cell Environ. 25, 163–171. doi: 10.1046/j.0016-8025.2001.00790.x
Saleh, A. A., Abdel-Kader, D. Z., and El Elish, A. M. (2007). Role of heat shock and salicylic acid in antioxidant homeostasis in Mungbean (Vigna radiata L.) plant subjected to heat stress. Am. J. Plant Physiol. 2, 344–355. doi: 10.3923/ajpp.2007.344.355
Salem, M. A., Kakani, V. G., Koti, S., and Reddy, K. R. (2007). Pollen-based screening of soybean genotypes for high temperatures. Crop Sci. 47, 219–231. doi: 10.2135/cropsci2006.07.0443
Sato, S., Peet, M. M., and Gardner, R. G. (2004). Altered flower retention and developmental patterns in nine tomato cultivars under elevated temperature. Sci. Hortic. 101, 95–101. doi: 10.1016/j.scienta.2003.10.008
Sato, S., Peet, M. M., and Thomas, J. F. (2002). Determining critical pre and post anthesis periods and physiological processes in Lycopersicon esculentum Mill. exposed to moderately elevated temperatures. J. Exp. Bot. 53, 1187–1195. doi: 10.1093/jexbot/53.371.1187
Savchenko, G. E., Stupak, A. P., and Klyuchareva, E. A. (2002). Study of dynamic properties of the lipid layer of plastid inner membranes using lipophilic fluorescent probes, Zh. Prikl. Spektr. 69, 497–501. doi: 10.1023/A:1020660300687
Scarpeci, T. E., Zanor, M. I., Carrillo, N., Mueller-Roeber, B., and Valle, E. M. (2008). Generation of superoxide anion in chloroplasts of Arabidopsis thaliana during active photosynthesis: a focus on rapidly induced genes. Plant Mol. Biol. 66, 361–378. doi: 10.1007/s11103-007-9274-4
Sehgal, A., Sita, K., Siddique, K. H. M., Kumar, R., Bhogireddy, S., Varshney, R. K., et al. (2018). Drought or/and heat-stress effects on seed filling in food crops: impacts on functional biochemistry, seed yields, and nutritional quality. Front. Plant Sci. 9, 1705. doi: 10.3389/fpls.2018.01705
Sekhon, H. S., Singh, G., Sharma, P., and Bains, T. S. (2010). “Water use efficiency under stress environments,” in Climate Change and Management of Cool Season Grain Legume Crops, eds S. S. Yadav, and R. Redden (Dordrecht: Springer), 207–227.
Shah, Z., Iqbal, A., Khan, F. U., Khan, H. U., Durrani, F., and Ahmad, M. Z. (2020). Genetic manipulation of pea (Pisum sativum L.) with Arabidopsis's heat shock factor HsfA1d improves ROS scavenging system to confront thermal stress. Genet. Resour. Crop Evol. 67, 2119–2127. doi: 10.1007/s10722-020-00966-9
Sharkey, T. D. (2005). Effects of moderate heat stress on photosynthesis: importance of thylakoid reactions, rubisco deactivation, reactive oxygen species, and thermotolerance provided by isoprene. Plant Cell Environ. 28, 269–277. doi: 10.1111/j.1365-3040.2005.01324.x
Sharma, L., Priya, M., Bindumadhava, H., Nair, R. M., and Nayyar, H. (2016). Influence of high temperature stress on growth, phenology and yield performance of mungbean [Vigna radiata (L.) Wilczek] under managed growth conditions. Sci. Hortic. 213, 379–391. doi: 10.1016/j.scienta.2016.10.033
Shi, Q., Bao, Z., Zhu, Z., Ying, Q., and Qian, Q. (2006). Effects of different treatments of salicylic acid on heat tolerance, chlorophyll fluorescence, and antioxidant enzyme activity in seedlings of Cucumis sativa L. Plant Growth Regul. 48, 127–135. doi: 10.1007/s10725-005-5482-6
Simões-Araújo, J. L., Rumjanek, N. G., and Margis-Pinheiro, M. (2003). Small heat shock proteins genes are differentially expressed in distinct varieties of common bean. Braz. J. Plant Physiol. 15, 33–41. doi: 10.1590/S1677-04202003000100005
Singh, J. S., Abhilash, P. C., and Gupta, V. K. (2016). Agriculturally important microbes in sustainable food production. Trends Biotechnol. 34, 773–775. doi: 10.1016/j.tibtech.2016.06.002
Singh, S. (2013). Thermal requirements for flowering and fruit yield attainment in advance lines of okra. J. Agro meteorol. 15, 39–42. doi: 10.54386/jam.v15i1.1436
Singh, U., Patel, P. K., Singh, A. K., Tiwari, V., Kumar, R., Rai, N., et al. (2015). Screening of tomato genotypes under high temperature stress for reproductive traits. Veg. Sci. 42, 52–55.
Sita, K., Sehgal, A., Kumar, J., Kumar, S., Singh, S., Siddique, K. H., et al. (2017). Identification of high-temperature tolerant lentil (Lens culinaris Medik.) genotypes through leaf and pollen traits. Front. Plant Sci. 8, 744. doi: 10.3389/fpls.2017.00744
Smith, A. M., and Denyer, K. A. Y. (1992). Starch synthesis in developing pea embryos. New Phytol. 122, 21–33. doi: 10.1111/j.1469-8137.1992.tb00049.x
Soltani, A., Weraduwage, S. M., Sharkey, T. D., and Lowry, D. B. (2019). Elevated temperatures cause loss of seed set in common bean (Phaseolus vulgaris L.) potentially through the disruption of source-sink relationships. BMC Genom. 20, 312. doi: 10.1186/s12864-019-5669-2
Song, H., Huang, Y., and Gu, B. (2020). QTL-Seq identifies quantitative trait loci of relative electrical conductivity associated with heat tolerance in bottle gourd (Lagenaria siceraria). PLoS ONE 15, e0227663. doi: 10.1371/journal.pone.0227663
Song, Q., Yang, F., Cui, B., Li, J., Zhang, Y., Li, H., et al. (2019). Physiological and molecular responses of two Chinese cabbage genotypes to heat stress. Biol. Plant. 63, 548–555. doi: 10.32615/bp.2019.097
Srivastava, A., Singh, R. P., Srivastava, A. K., Saxena, A. K., and Arora, D. K. (2008). Growth promotion and charcoal rot management in chickpea by Trichoderma harzianum. J. Plant Prot. Res. doi: 10.2478/v10045-008-0009-6
Stefanov, D., Petkova, V., and Denev, I. D. (2011). Screening for heat tolerance in common bean (Phaseolus vulgaris L.) lines and cultivars using JIP-test. Sci. Hortic. 128, 1–6. doi: 10.1016/j.scienta.2010.12.003
Summerfield, R. J., and Wien, H. C. (1980). “Effects of photoperiod and air temperature on growth and yield of economic legumes,” in Advances in Legumes Sciences. Proceedings of the International Legumes Conference, eds R. J. Summerfield, A. H. Bunting (Kew: Royal Botanic Gardens), 17–36.
Suzuki, K., Takeda, H., Tsukaguchi, T., and Egawa, Y. (2001). Ultrastructural study on degeneration of tapetum in anther of snap bean (Phaseolus vulgaris L.) under heat stress. Sex. Plant Reprod. 13, 293–299. doi: 10.1007/s004970100071
Suzuki, N., Koussevitzky, S. H. A. I., Mittler, R. O. N., and Miller, G. A. D. (2012). ROS and redox signalling in the response of plants to abiotic stress. Plant Cell Environ. 35, 259–270. doi: 10.1111/j.1365-3040.2011.02336.x
Tafesse, E. G. (2018). Heat stress resistance in pea (Pisum sativum L.) based on canopy and leaf traits (Doctoral dissertation). University of Saskatchewan, Saskatoon, SK, Canada.
Tafesse, E. G., Gali, K. K., Lachagari, V. B., Bueckert, R., and Warkentin, T. D. (2020). Genome-wide association mapping for heat stress responsive traits in field pea. Int. J. Mol. Sci. 21, 1–26. doi: 10.3390/ijms21062043
Tafesse, E. G., Warkentin, T. D., and Bueckert, R. A. (2019). Canopy architecture and leaf type as traits of heat resistance in pea. Field Crops Res. 241, 107561. doi: 10.1016/j.fcr.2019.107561
Talalaiev, O., and Korduym, E. (2014). Expression of small heat shock protein (sHSP) genes in the garden pea (Pisum sativum) under slow horizontal clinorotation. Plant Signal Behav. 9, e29035-1–e29035-2. doi: 10.4161/psb.29035
Tang, L., Kwon, S. Y., Kim, S. H., Kim, J. S., Choi, J. S., Cho, K. Y., et al. (2006). Enhanced tolerance of transgenic potato plants expressing both superoxide dismutase and ascorbate peroxidase in chloroplasts against oxidative stress and high temperature. Plant Cell Rep. 25, 1380–1386. doi: 10.1007/s00299-006-0199-1
Tang, R., Niu, S., Zhang, G., Chen, G., Haroon, M., Yang, Q., et al. (2018). Physiological and growth responses of potato cultivars to heat stress. Botany 96, 897–912. doi: 10.1139/cjb-2018-0125
Teng, J., Liao, P., and Wang, M. (2021). The role of emerging micro-scale vegetables in human diet and health benefits-An updated review based on microgreens. Food Funct. 12, 1914–1932. doi: 10.1039/D0FO03299A
Tewari, A. K., and Tripathy, B. C. (1998). Temperature-stress-induced impairment of chlorophyll biosynthetic reactions in cucumber and wheat. Plant Physiol. 117, 851–858. doi: 10.1104/pp.117.3.851
Thomas, D. S. G., Twyman, C., Osbahr, H., and Hewitson, B. (2007). Adaptation to climate change and variability: farmer responses to intra-seasonal precipitation trends in South Africa. Clim. Change 83, 301–322. doi: 10.1007/s10584-006-9205-4
Thomas, H., and Howarth, C. J. (2000). Five ways to stay green. J. Exp. Bot. 51, 329–337. doi: 10.1093/jexbot/51.suppl_1.329
Thomas, J. M. G., Boote, K. J., Allen, L. H., Gallo-Meagher, M., and Davis, J. M. (2003). Elevated temperature and carbon dioxide effects on soybean seed composition and transcript abundance. Crop Sci. 43, 1548–1557. doi: 10.2135/cropsci2003.1548
Thuy, T. L., and Kenji, M. (2015). Effect of high temperature on fruit productivity and seed-set of sweet pepper (Capsicum annuum L.) in the field condition. J. Agric. Sci. Technol. 5, 515–520. doi: 10.17265/2161-6256/2015.12.010
Tickoo, J. L., Matho, G. R., and Manji, C. (1996). “Plant type in mungbean (Vigna radiata (L.) Wilczek),” in Proceedings of Recent Advances in Mungbean Research, eds A. N. Asthana and D. H. Kim (Kanpur: Indian Society of Pulses Research and Development; Indian Institute of Pulses Research), 197–213.
Timko, M. P., and Singh, B. B. (2008). “Cowpea, a multifunctional legume,” in Genomics of Tropical Crop Plants, eds P. H. Moore and R. Ming (New York, NY: Springer), 227–258.
Trapero-Mozos, A., Morris, W. L., Ducreux, L. J., McLean, K., Stephens, J., Torrance, L., et al. (2018). Engineering heat tolerance in potato by temperature-dependent expression of a specific allele of HEAT-SHOCK COGNATE 70. Plant Biotechnol. J. 16, 197–207. doi: 10.1111/pbi.12760
Ul Haq, S., Khan, A., Ali, M., Khattak, A. M., Gai, W. X., Zhang, H. X., et al. (2019). Heat shock proteins: dynamic biomolecules to counter plant biotic and abiotic stresses. Int J. Mol. Sci. 20, 1–31. doi: 10.3390/ijms20215321
Usman, M. G., Rafii, M. Y., Martini, M. Y., Yusuff, O. A., Ismail, M. R., and Miah, G. (2018). Introgression of heat shock protein (Hsp70 and sHsp) genes into the Malaysian elite chilli variety Kulai (Capsicum annuum L.) through the application of marker-assisted backcrossing (MAB). Cell Stress Chaperones 23, 223–234. doi: 10.1007/s12192-017-0836-3
Utami, D., and Aryanti, E. (2021). “Impact of heat stress on germination and seedling growth of chili pepper (Capsicum annuum L.),” in IOP Conference Series: Earth and Environmental Science, Vol. 637 (Vancouver, BC: IOP Publishing), 012032.
Vargas, Y., Mayor-Duran, V. M., Buendia, H. F., Ruiz-Guzman, H., and Raatz, B. (2021). Physiological and genetic characterization of heat stress effects in a common bean RIL population. PLoS ONE 16, e0249859. doi: 10.1371/journal.pone.0249859
Vialet-Chabrand, S., and Lawson, T. (2019). Dynamic leaf energy balance: deriving stomatal conductance from thermal imaging in a dynamic environment. J. Exp. Bot. 70, 2839–2855. doi: 10.1093/jxb/erz068
Vierling, E. (1991). The roles of heat shock proteins in plants. Annu. Rev. Plant Biol. 42, 579–620. doi: 10.1146/annurev.pp.42.060191.003051
Vu, L. D., Xu, X., Gevaert, K., and De Smet, I. (2019). Developmental plasticity at high temperature. Plant Physiol. 181, 399–411. doi: 10.1104/pp.19.00652
Wahid, A., Gelani, S., Ashraf, M., and Foolad, M. R. (2007). Heat tolerance in plants: an overview. Environ. Exp. Bot. 61, 199–223. doi: 10.1016/j.envexpbot.2007.05.011
Waithaka, M., Nelson, G. C., Thomas, T. S., and Kyotalimye, M. (2013). East African Agriculture and Climate Change: A Comprehensive Analysis. Washington, DC: IFPRI, 387p.
Wang, A., Hu, J., Gao, C., Chen, G., Wang, B., Lin, C., et al. (2019). Genome-wide analysis of long non-coding RNAs unveils the regulatory roles in the heat tolerance of Chinese cabbage (Brassica rapa ssp. chinensis). Sci. Rep. 9, 1–14. doi: 10.1038/s41598-019-41428-2
Wang, J., Lv, J., Liu, Z., Liu, Y., Song, J., Ma, Y., et al. (2019). Integration of transcriptomics and metabolomics for pepper (Capsicum annuum L.) in response to heat stress. Int. J. Mol. Sci. 20, 1–18. doi: 10.3390/ijms20205042
Wang, W., Vinocur, B., Shoseyov, O., and Altman, A. (2004). Role of plant heat-shock proteins and molecular chaperones in the abiotic stress response. Trends Plant Sci. 9, 244–252. doi: 10.1016/j.tplants.2004.03.006
Wang, Y., Wisniewski, M., Meilan, R., Cui, M., and Fuchigami, L. (2006). Transgenic tomato (Lycopersicon esculentum) overexpressing cAPX exhibits enhanced tolerance to UV-B and heat stress. J. Appl. Hortic. 8, 87–90. doi: 10.37855/jah.2006.v08i02.21
Waraich, E. A., Ahmad, R., Ashraf, M. Y., and Saifullah, Ahmad, M. (2011). Improving agricultural water use efficiency by nutrient management in crop plants. Acta Agric. Scand. B. Soil Plant Sci. 61, 291–304. doi: 10.1080/09064710.2010.491954
Waraich, E. A., Ahmad, R., Halim, A., and Aziz, T. (2012). Alleviation of temperature stress by nutrient management in crop plants: a review. Soil Sci. Plant Nutr. 12, 221–244. doi: 10.4067/S0718-95162012000200003
Warland, J., McKeown, A. W., and McDonald, M. R. (2006). Impact of high air temperatures on Brassicacae crops in southern Ontario. Can. J. Plant Sci. 86, 1209–1215. doi: 10.4141/P05-067
Way, D. A., and Pearcy, R. W. (2012). Sunflecks in trees and forests: from photosynthetic physiology to global change biology. Tree Physiol. 32, 1066–1081. doi: 10.1093/treephys/tps064
Weis, E. (1981a). Reversible heat-inactivation of the Calvin cycle: a possible mechanism of the temperature regulation of photosynthesis. Planta 151, 33–39. doi: 10.1007/BF00384234
Weis, E. (1981b). The temperature-sensitivity of dark-inactivation and light-activation of the ribulose-1, 5-bisphosphate carboxylase in spinach chloroplasts. FEBS Lett. 129, 197–200. doi: 10.1016/0014-5793(81)80164-0
Wen, J., Jiang, F., Weng, Y., Sun, M., Shi, X., Zhou, Y., et al. (2019). Identification of heat-tolerance QTLs and high-temperature stress-responsive genes through conventional QTL mapping, QTL-seq and RNA-seq in tomato. BMC Plant Biol. 19, 1–17. doi: 10.1186/s12870-019-2008-3
Wilson, R. A., Sangha, M. K., Banga, S. S., Atwal, A. K., and Gupta, S. (2014). Heat stress tolerance in relation to oxidative stress and antioxidants in Brassica juncea. Environ. Biol. 35, 383–387.
Wise, R. R., Olson, A. J., Schrader, S. M., and Sharkey, T. D. (2004). Electron transport is the functional limitation of photosynthesis in field-grown Pima cotton plants at high temperature. Plant Cell Environ. 27, 717–724. doi: 10.1111/j.1365-3040.2004.01171.x
Wittwer, S. H., and Castilla, N. (1995). Protected cultivation of horticultural crops worldwide. Hort Technol. 5, 6–23. doi: 10.21273/HORTTECH.5.1.6
Xu, J., Driedonks, N., Rutten, M. J., Vriezen, W. H., de Boer, G. J., and Rieu, I. (2017). Mapping quantitative trait loci for heat tolerance of reproductive traits in tomato (Solanum lycopersicum). Mol. Breed. 37, 58. doi: 10.1007/s11032-017-0664-2
Yamamoto, H., Takahashi, S., Badger, M. R., and Shikanai, T. (2016). Artificial remodelling of alternative electron flow by flavodiiron proteins in Arabidopsis. Nat. Plants 2, 1–7. doi: 10.1038/nplants.2016.12
Yang, X., Zhu, W., Zhang, H., Liu, N., and Tian, S. (2016). Heat shock factors in tomatoes: genome-wide identification, phylogenetic analysis and expression profiling under development and heat stress. Peer J. 4, 1–16. doi: 10.7717/peerj.1961
Yeh, D. M., and Lin, H. F. (2003). Thermostability of cell membranes as a measure of heat tolerance and relationship to flowering delay in chrysanthemum. J. Am. Soc. Hortic. Sci. 128, 656–660. doi: 10.21273/JASHS.128.5.0656
Yoshioka, M., Uchida, S., Mori, H., Komayama, K., Ohira, S., Morita, N., et al. (2006). Quality control of photosystem II cleavage of reaction center D1 protein in spinach thylakoids by FtsH protease under moderate heat stress. Biol. Chem. 281, 21660–21669. doi: 10.1074/jbc.M602896200
Young, L. W., Wilen, R. W., and Bonham-Smith, P. C. (2004). High temperature stress of Brassica napus during flowering reduces micro-and megagametophyte fertility, induces fruit abortion, and disrupts seed production. J. Exp. Bot. 55, 485–495. doi: 10.1093/jxb/erh038
Yu, B., Ming, F., Liang, Y., Wang, Y., Gan, Y., Qiu, Z., et al. (2022). Heat stress resistance mechanisms of two cucumber varieties from different regions. Int. J. Mol. Sci. 23, 1–23. doi: 10.3390/ijms23031817
Yuan, L., Tang, L., Zhu, S., Hou, J., Chen, G., Liu, F., et al. (2017). Influence of heat stress on leaf morphology and nitrogen-carbohydrate metabolisms in two wucai (Brassica campestris L.) genotypes. Acta Soc. Bot. Pol. 86, 1–16. doi: 10.5586/asbp.3554
Zahran, H. H. (1999). Rhizobium-legume symbiosis and nitrogen fixation under severe conditions and in an arid climate. Microbiol. Mol. Biol. Rev. 63, 968–989. doi: 10.1128/MMBR.63.4.968-989.1999
Zhang, G., Tang, R., Niu, S., Si, H., Yang, Q., Bizimungu, B., et al. (2020). Effects of earliness on heat stress tolerance in fifty potato cultivars. Am. J. Potato Res. 97, 23–32. doi: 10.1007/s12230-019-09740-9
Zhang, T., Li, Z., Li, D., Li, C., Wei, D., Li, S., et al. (2020). Comparative effects of glycinebetaine on the thermotolerance in codA- and BADH-transgenic tomato plants under high temperature stress. Plant Cell Rep. 39, 1525–1538. doi: 10.1007/s00299-020-02581-5
Zhang, Y., Xu, L., Zhu, X., Gong, Y., Xiang, F., Sun, X., et al. (2013). Proteomic analysis of heat stress response in leaves of radish (Raphanus sativus L.). Plant Mol. Biol. Rep. 31, 195–203. doi: 10.1007/s11105-012-0486-7
Zhao, P., Wang, D., Wang, R., Kong, N., Zhang, C., Yang, C., et al. (2018). Genome-wide analysis of the potato Hsp20 gene family: identification, genomic organization and expression profiles in response to heat stress. BMC Genom. 19, 1–13. doi: 10.1186/s12864-018-4443-1
Zhao, Q., Chen, W., Bian, J., Xie, H., Li, Y., Xu, C., et al. (2018). Proteomics and phosphoproteomics of heat stress-responsive mechanisms in spinach. Front. Plant Sci. 9, 800. doi: 10.3389/fpls.2018.00800
Zhou, J., Wang, J., Li, X., Xia, X. J., Zhou, Y. H., Shi, K., et al. (2014). H2O2 mediates the crosstalk of brassinosteroid and abscisic acid in tomato responses to heat and oxidative stresses. J. Exp. Bot. 65, 4371–4383. doi: 10.1093/jxb/eru217
Zhou, R., Wu, Z., Wang, X., Rosenqvist, E., Wang, Y., Zhao, T., et al. (2018). Evaluation of temperature stress tolerance in cultivated and wild tomatoes using photosynthesis and chlorophyll fluorescence. Hortic. Environ. Biotech. 59, 499–509. doi: 10.1007/s13580-018-0050-y
Zhou, R., Yu, X., Kjær, K. H., Rosenqvist, E., Ottosen, C. O., and Wu, Z. (2015). Screening and validation of tomato genotypes under heat stress using Fv/Fm to reveal the physiological mechanism of heat tolerance. Environ. Exp. Bot. 118, 1–11. doi: 10.1016/j.envexpbot.2015.05.006
Zhou, R., Yu, X., Ottosen, C. O., Rosenqvist, E., Zhao, L., Wang, Y., et al. (2017). Drought stress had a predominant effect over heat stress on three tomato cultivars subjected to combined stress. BMC Plant Biol. 17, 1–13. doi: 10.1186/s12870-017-0974-x
Zhu, B., Ye, C., Lü, H., Chen, X., Chai, G., Chen, J., et al. (2006). Identification and characterization of a novel heat shock transcription factor gene, GmHsfA1, in soybeans (Glycine max). J. Plant Res. 119, 247–256.doi: 10.1007/s10265-006-0267-1
Keywords: high temperature, vegetables, heat, environment, climate change
Citation: Chaudhary S, Devi P, HanumanthaRao B, Jha UC, Sharma KD, Prasad PVV, Kumar S, Siddique KHM and Nayyar H (2022) Physiological and Molecular Approaches for Developing Thermotolerance in Vegetable Crops: A Growth, Yield and Sustenance Perspective. Front. Plant Sci. 13:878498. doi: 10.3389/fpls.2022.878498
Received: 18 February 2022; Accepted: 17 May 2022;
Published: 28 June 2022.
Edited by:
Mostafa Abdelwahed Abdelrahman, Aswan University, EgyptReviewed by:
Pasala Ratnakumar, Indian Institute of Oilseeds Research (ICAR), IndiaCopyright © 2022 Chaudhary, Devi, HanumanthaRao, Jha, Sharma, Prasad, Kumar, Siddique and Nayyar. This is an open-access article distributed under the terms of the Creative Commons Attribution License (CC BY). The use, distribution or reproduction in other forums is permitted, provided the original author(s) and the copyright owner(s) are credited and that the original publication in this journal is cited, in accordance with accepted academic practice. No use, distribution or reproduction is permitted which does not comply with these terms.
*Correspondence: Harsh Nayyar, aGFyc2huYXl5YXJAaG90bWFpbC5jb20=; Kadambot H. M. Siddique, a2FkYW1ib3Quc2lkZGlxdWVAdXdhLmVkdS5hdQ==
Disclaimer: All claims expressed in this article are solely those of the authors and do not necessarily represent those of their affiliated organizations, or those of the publisher, the editors and the reviewers. Any product that may be evaluated in this article or claim that may be made by its manufacturer is not guaranteed or endorsed by the publisher.
Research integrity at Frontiers
Learn more about the work of our research integrity team to safeguard the quality of each article we publish.