- 1School of Public Administration, Hohai University, Nanjing, China
- 2Cell and Molecular Biology Program, University of Arkansas, Fayetteville, NC, United States
- 3Botany and Microbiology Department, College of Science, King Saud University, Riyadh, Saudi Arabia
- 4Engineering Research Center of Ministry of Education for Mine Ecological Restoration, China University of Mining and Technology, Xuzhou, China
- 5Department of Environmental Science and Engineering, Government College University Faisalabad, Faisalabad, Pakistan
- 6Department of Environmental Sciences, The University of Lahore, Lahore, Pakistan
- 7Department of Environmental Sciences, University of Jhang, Jhang, Pakistan
The continuous increase in the heavy metals concentration in the soil due to anthropogenic activities has become a global issue. The chromium, especially hexavalent chromium, is highly toxic for living organisms due to high mobility, solubility, and carcinogenic properties. Considering the beneficial role of nanoparticles and bacteria in alleviating the metal stress in plants, a study was carried out to evaluate the role of cerium dioxide (CeO2) nanoparticles (NPs) and Staphylococcus aureus in alleviating the chromium toxicity in sunflower plants. Sunflower plants grown in chromium (Cr) contaminated soil (0, 25, and 50 mg kg−1) were treated with CeO2 nanoparticles (0, 25, and 50 mg L−1) and S. aureus. The application of Cerium Dioxide Nanoparticles (CeO2 NPs) significantly improved plant growth and biomass production, reduced oxidative stress, and enhanced the enzymatic activities in the sunflower plant grown under chromium stress. The application of S. aureus further enhanced the beneficial role of nanoparticles in alleviating metal-induced toxicity. The maximum improvement was noted in plants treated with both nanoparticles and S. aureus. The augmented application of CeO2 NPs (50 mg l−1) at Cr 50 mg kg−1 increased the chl a contents from 1.2 to 2.0, chl b contents 0.5 to 0.8 and mg g−1 FW, and decreased the leakage of the electrolyte from 121 to 104%. The findings proved that the application of CeO2 nanoparticles and S. aureus could significantly ameliorate the metal-induced stress in sunflower plants. The findings from this study can provide new horizons for research in the application of nanoparticles in phytoremediation and bioremediation.
Introduction
Chromium is naturally found in the soil, air, and water. The trivalent chromium (Cr+3) and hexavalent chromium (Cr+6) are two forms of chromium (Yoshinaga et al., 2018). The hexavalent chromium is considered the most toxic form that mainly exits as chromate or dichromate. Human activities and industrialization have increased the addition of chromium in the environment, and it has become a significant concern for plants, animals, and humans due to its lethal effects. The primary source of chromium pollution is the leather industry, followed by the electroplating industry textile and chromate mining (Belay, 2010; Tumolo et al., 2020). Chromium is a toxic and non-essential element for plants, and plants do not have a specific mechanism for its uptake (Shanker et al., 2005). Chromium is highly toxic for plants due to its detrimental effect on plants’ growth and development. The most common effect of chromium on plants is reduced leaf and root growth, reduced yield, inhibition of enzymatic activity, and mutagenesis (Singh et al., 2013).
Sunflower (Helianthus annuus L.) is one of the essential vegetable oil sources, with an annual production of about 9 million tonnes. The sunflower oil is highly healthy due to its high concentration of mono-saturated and poly-saturated fatty acid and high contents of vitamin E (Kaya et al., 2012). Sunflower has been widely studied due to its unique ability to uptake and accumulate various heavy metals (Alaboudi et al., 2018; Chen et al., 2020). Sunflower can uptake and accumulate metals such as Pb, Cd, and Cr in its roots and shoots from contaminated soil (Chen et al., 2020). Due to its high biomass, sunflower is recommended to remediation soil contaminated with heavy metals. However, the high concentration of chromium has a detrimental effect on sunflower productivity and enzymatic activity (Fozia et al., 2008).
Phytoremediation is a green technology in which plants remove toxic pollutants and heavy metals from contaminated soil and water. In this technology, the potential of plants is applied to uptake, accumulate, and degrade pollutants from the contaminated medium (Hauptvogl et al., 2019). The ability of plants to remediate the pollutants depends upon the type of pollutants, concentration of pollutant and plant biomass production, and ability to accumulate metals (Ashraf et al., 2019). Hyperaccumulator plants have tremendous ability to accumulate particular metals and metalloids, hundred or thousand times greater than typical plants in the same environmental condition (Peer et al., 2005). These plants are most tolerant to high concentrations of heavy metals, can uptake these pollutants through their roots system, and accumulate high levels of these metals in their living tissue such as roots, stems, and leaves (Peer et al., 2005; Baker et al., 2020). Plants also have different metabolic processes to alleviate the metal stress, such as producing organic acids that can chelate the heavy metals and covert these metals into not toxic form and prevent the uptake of heavy metal by plants (Shahid et al., 2017, 2019). Other methods used by the plant to minimize the metal toxification include extracellular complexation and cytoplasmic complexation. The metal stress in the plant causes severe damage to the plant’s metabolic process. To tolerate the metals stress, the plant should activate the antioxidant enzymes system and repair the oxidative stress and damage caused by metal stress (Sharma et al., 2016).
The microorganism can be applied as a biological tool to remove the heavy metals from the polluted medium due to their ability to concentrate and recover heavy metals (Devi et al., 2021). Bioremediation is the application of microorganisms to remediate polluted sites contaminated with heavy metals and other organic/inorganic pollutants (Shahid et al., 2020). Bacteria have evolved efficient mechanisms to bio-remediate heavy metals. These mechanisms include detoxification, adsorption, oxidation, and reduction of heavy metals (Kang et al., 2016; Naik and Kumar, 2020). The versatility of bacteria to degrade various pollutants and heavy metals has emphasized their application in the remediation of different types of soils. The bioremediation has shown promising results in the remediation of heavy metals such as lead, chromium, and cadmium, even in deficient concentrations in soil and water where other processes failed (Tiquia-Arashiro, 2018; Pushkar et al., 2021). The phytoremediation process can be boosted by applying selective bacteria resistant to specific metals. It has been widely proven that the application of bacteria in phytoremediation increases heavy metals such as lead, chromium, and iron uptake and removal from the contaminated soils (Kong et al., 2019; Ma et al., 2019).
Nanotechnology has grown remarkably in the last two decades with its wide application in agriculture and environmental remediation (Song et al., 2019; Zand et al., 2020). Nanoparticle-assisted phytoremediation has emerged as a reliable technology for removing contaminated soil with metals and metalloids. The nanoparticle has been widely applied from remediation of lead, cadmium, and arsenic from contaminated soil (Song et al., 2019; Zhu et al., 2019). The nanoparticle may improve the phytoremediation by a direct effect such as directly removing the pollutant from the soil by adsorption and immobilizing the metals, and ultimately reducing the concentration of metals in the soil (Omara et al., 2019). The nanoparticle can also improve phytoremediation by promoting plant growth, such as carbon nanotubes, Ag nanoparticles, and ZnO nanoparticles (Sabir et al., 2014). The nanoparticle may also increase the plant tolerance to abiotic stress by regulating the gene expression of enzymes, such as application of silicon nanoparticles improved the phytoextraction capacity of pea plant for chromium (VI; Tripathi et al., 2015). The applied nanoparticle may also improve plant growth by increasing the availability and absorption of nutrients and water (Kale and Gawade, 2016).
Considering the influential role of nanoparticles and bacteria, a field study was conducted to analyze the potential of Cerium Dioxide Nanoparticles (CeO2 NPs) and a chromium-resistant bacteria Staphylococcus aureus in remediating the chromium from contaminated soil through sunflower plants. It was assumed that combined application of CeO2 NPs and chromium-resistant bacteria will alleviate the chromium toxicity by their mutual role and enhance plant tolerance to metal toxicity. The augmented and individual role of CeO2 NPs and S. aureus on the performance of chromium-stressed plants was evaluated by analyzing the changes in growth parameters, enzymatic activity, and oxidative stress. The finding from this research will provide valuable information about the application of nanoparticles and bacteria in remediating heavy metals from the contaminated medium.
Materials and Methods
Soil Sampling and Analysis
The soil for this experiment was collected from the field of Govt. College University, Faisalabad from various points and depths from 0 to 20 cm to make a homogeneous mixture as recommended by previous researchers (Rehman et al., 2018; Rizwan et al., 2019). The collected soil sample was stored at 4°C in cooler box to protect from sunlight. All collected soil samples were air-dried, and the plant debris and large soil particles were removed by sieving the soil through a 2 mm sieve. The pH of the soil was analyzed by making the soil water paste and noted by calibrated pH meter. Similarly, the electrical conductivity (EC) of the soil was noted by calibrated EC meter. The collected soil was analyzed for metal contents by treating ammonium bicarbonate diethylene triamine penta acetic acid (AB-DTPA) at pH 6.7 (Soltanpour, 1991). The soil was artificially spiked with K2Cr2O7 to make the chromium-contaminated soil just like the natural agricultural soil of district Qasur and Sialkot highly contaminated with chromium due to irrigation with tannery effluent.
Bacterial Inoculation of Seeds
The soil was collected, ground, and sieved through a 2 mm sieve after drying at 70°C. The sieved soil was autoclaved at 121°C for 20 min to remove bacterial contamination. Nutrient broth and Cr-resistant bacteria, S. aureus, were used to make the bacterial inoculum. The bacterial inoculum was shaken at 2000 rpm for 48 h at 30°C, then centrifuged for 10 min at 6000 rpm. The supernatant was collected and diluted with distilled water (Zulaika and Sembiring, 2014). The density of the bacterial isolate was measured using a hemocytometer. The population size of the centrifuged bacterial cells was set at 2.8 × 108 wet weight. Sunflower seeds were disinfected by inoculating them with a 10% sugar solution. The seeds were adequately coated with clay and an equal amount of peat moss (1:1) and placed overnight.
Sunflower Sowing and Harvesting
The experiment was performed in the pots at the botanical garden of Government College University, Faisalabad, at temperatures 20–25°C with 70% humidity. Each plastic container was filled with 5 kg of sieved soil mixed with three chromium concentrations (0, 25, and 50 mg kg−1). Six uniform healthy sunflower seeds were rinsed with hydrogen peroxide solution (15% v/v) followed by tap and distilled water. Six sunflowers’ seeds are sown in each pot, and after germination, only three healthy and uniform seedlings were kept to grow for further proceedings. A mixture of nitrogen, phosphorus, and potassium was applied at the rate of 120:50:25 kg ha−1 for the healthy growth of the plants. After 2 weeks of germination, the nanoparticle CeO2 NPs were applied as a foliar spray in three concentrations (0, 25, and 50 mg L-1), while controls were treated with distilled water. Cerium (IV) oxide nano-powder (CeO2-NPs) was of Alfa Aesar with size, purity, and surface area of 15–30 nm, 99.5%, and 30–50 m2/g, respectively. The experiment was run for 4 months and when plants reached at maturity, the harvesting was done. The plants were harvested about 1 cm high from the soil, and plants were separated into different parts such as root, shoot, and leaves for further analysis. Fresh plant’s samples were kept in a cooler box during transportation to the laboratory for analysis to prevent changes in biochemical properties. The plants shoot and roots were appropriately washed with distilled water and roots were washed with 1% HCl followed by filtered water to remove the soil, contamination, and acid. The samples were air-dried and then oven dried at 72°C for 72 h.
Plant Growth Parameter and Chlorophyll Contents
After harvesting, the plant height (cm) was measured by measuring tape; then, the shoot dry weight (g pot−1) and roots dry weight (g pot−1) were measured by weight balance. The number of leaves per plant was counted, and leaf area (cm2) was measured. The chlorophyll a, b, total chlorophyll, and carotenoid contents were measured by extracting 0.2 g of fresh leaves in 0.5 ml of acetone (3% v/v). The supernatant was obtained through centrifugation for 10 min at 10,000 rpm, and absorption was noted through spectrophotometer for chlorophyll a (chl a) at 663 nm, chlorophyll b (chl b) 645 nm, and carotenoid at 470 nm (Lichtenthaler, 1987; Gohari et al., 2020).
Estimation of Antioxidant Enzymes and Reactive Oxygen Species Contents
The antioxidant activities of the enzymes (CAT, APX, POD, and SOD) in sunflower plants were determined using methods developed by Aebi (1984) for catalase (CAT), Nakano and Physiology (1981) for ascorbate oxidase (APX), and Zhang (1992) for peroxidases (POD) and superoxide dismutase (SOD; Nakano and Physiology, 1981; Aebi, 1984; Zhang, 1992).
The reactive oxygen species (ROS) were measured by analyzing the electrolyte leakage (EL), malondialdehyde (MDA) contents, and H2O2 in sunflower plants. The initial EC1 from the leaves was checked by extracting the supernatant by autoclaving the plants at 32°C for 2 h, then heating the sample at 121°C. The final EL contents were noted using the Dionisio-Sese and Tobita (1998) method (Dionisio-Sese and Tobita, 1998). The MDA and H2O2 contents were determined by preparing a supernatant solution by crushing a 0.1 g plant sample in liquid nitrogen and then in a 0.05 M phosphate buffer. This supernatant solution was prepared with TCA (0.1%) and TBA (0.5%) for MDA content, and absorbance was measured at 532 nm (Zhang and Kirkham, 1994). The H2O2 content was determined by mixing the supernatant with phosphate buffer and measuring the absorbance at 410 nm (Jana and Choudhuri, 1981).
Metal Contents in Shoot and Roots
The concentration of Cr+6 and Cr+3 in plants roots and shoots sample was measured by acid digestion of root and shoot samples by the standard method (Apha, 2012). The roots and shoot samples of 1 g each were ground and acid digested using HNO3 and HCLO4 (3:1) ratio. The metals contents in digested samples were determined by atomic absorption spectrophotometer.
Statistical Analysis
The data from the experimental research work are expressed as a mean value with a standard deviation. The replicated data were statistically analyzed using two-way variance (ANOVA) with Statistix 10.0 version software to recognize significant variance, and means were compared using Tukey’s post-hoc test. At p < 0.05, values were considered significant for all treatments.
Results
Effect on Growth Parameters
It is evident from Figures 1A–F that increasing the concentration of chromium from 0 to 50 mg kg−1 significantly decreased all plant growth parameters (plant height, shoot dry weight, root dry weight, number of leaves per plant, leaf area, and number of flowers per plant). At the highest concentration of chromium (Cr 50), the plant height decreased from 75 to 37 cm, and shoot dry weight decreased 19 to 9 g pot−1. A similar reduction was observed at a concentration of Cr50 for other growth attributes. However, S. aureus and CeO2 NPs significantly improved all plant growth parameters in sunflower plants under chromium stress. In plants with stress Cr50, the treatment with S. aureus increased the plant height up to 47 cm as compared to 37 in treatment without bacterial application. On the other hand, the application of CeO2 NPs further boosted the role of bacteria in plants under chromium stress. At stress level Cr50, the combined application of S. aureus and CeO2 NPs improved the plant height from 46 to 55 cm and shoot dry weight 11 to 13 g pot−1.
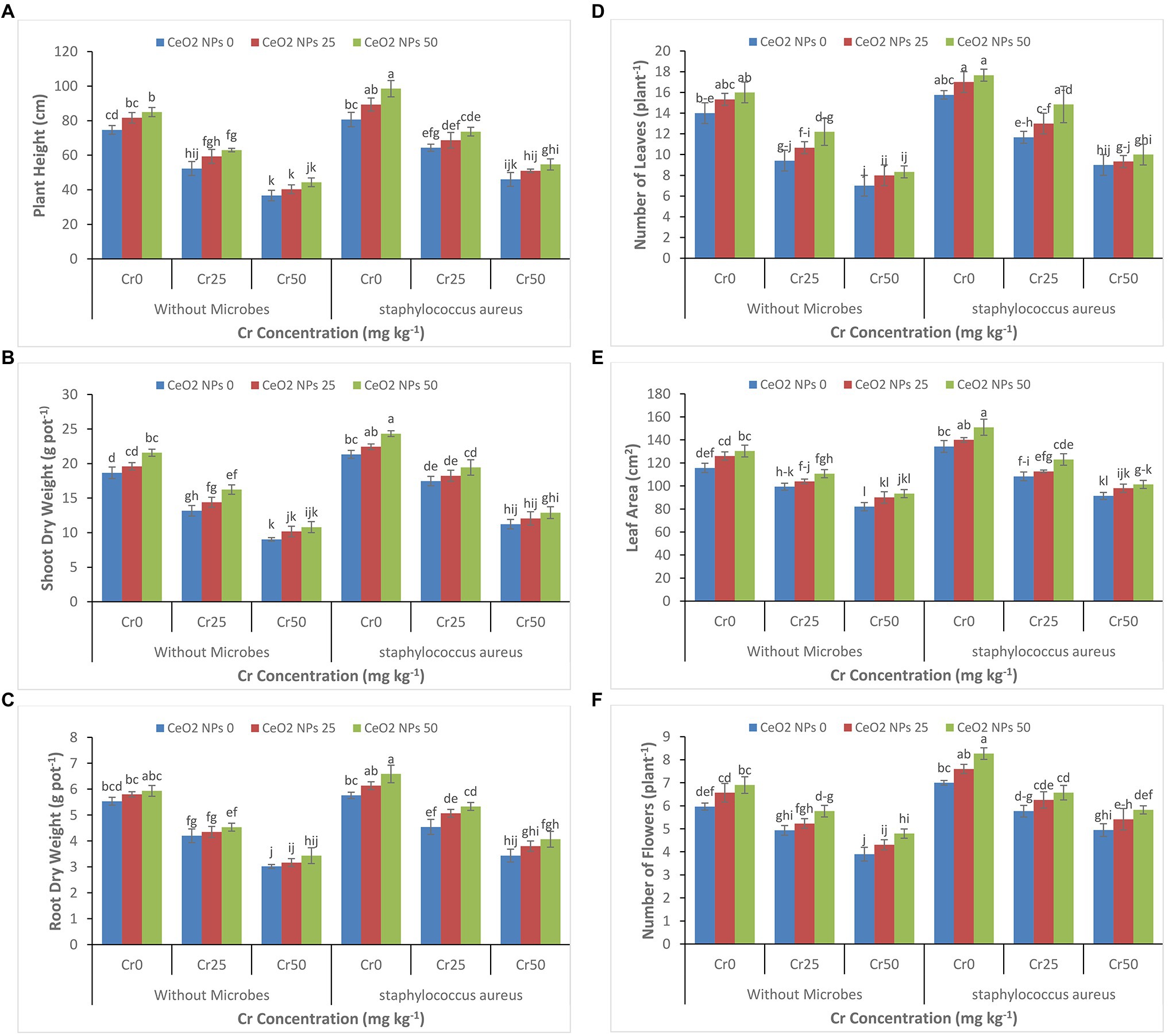
Figure 1. (A–F): Effect of CeO2 NPs and Staphylococcus aureus on plant height (A), shoot dry weight (B), root dry weight (C), number of leaves (D), leaf area (E), and number of flowers (F) in sunflower plant under Cr stress. The given values are means of three replications with standard deviation. The alphabets represent the significant/non-significant difference at p ≤ 0.05.
Effect on Photosynthetic Parameters
Just like the effect of chromium stress on growth parameters, the high concentration of chromium severely reduced the photosynthetic rate, transpiration rate, stomatal conductance, and water use efficiency in sunflower plants (Figures 2A–D). The increasing chromium concentration from 0 to 50 mg kg−1 reduced the photosynthetic rate from 25.9 to 11.0 μmol CO2 m−2 s−1 and water use efficiency from 0.4 to 0.1%. The application of S. aureus and CeO2 NPs significantly alleviated the damage to photosynthetic parameters by increasing the photosynthetic rate from 15.4 to 19.0 μmol CO2 m−2 s−1 and water use efficiency from 0.2 to 0.3% with increasing concentration of CeO2 NPs from 0 to 50 mg L−1.
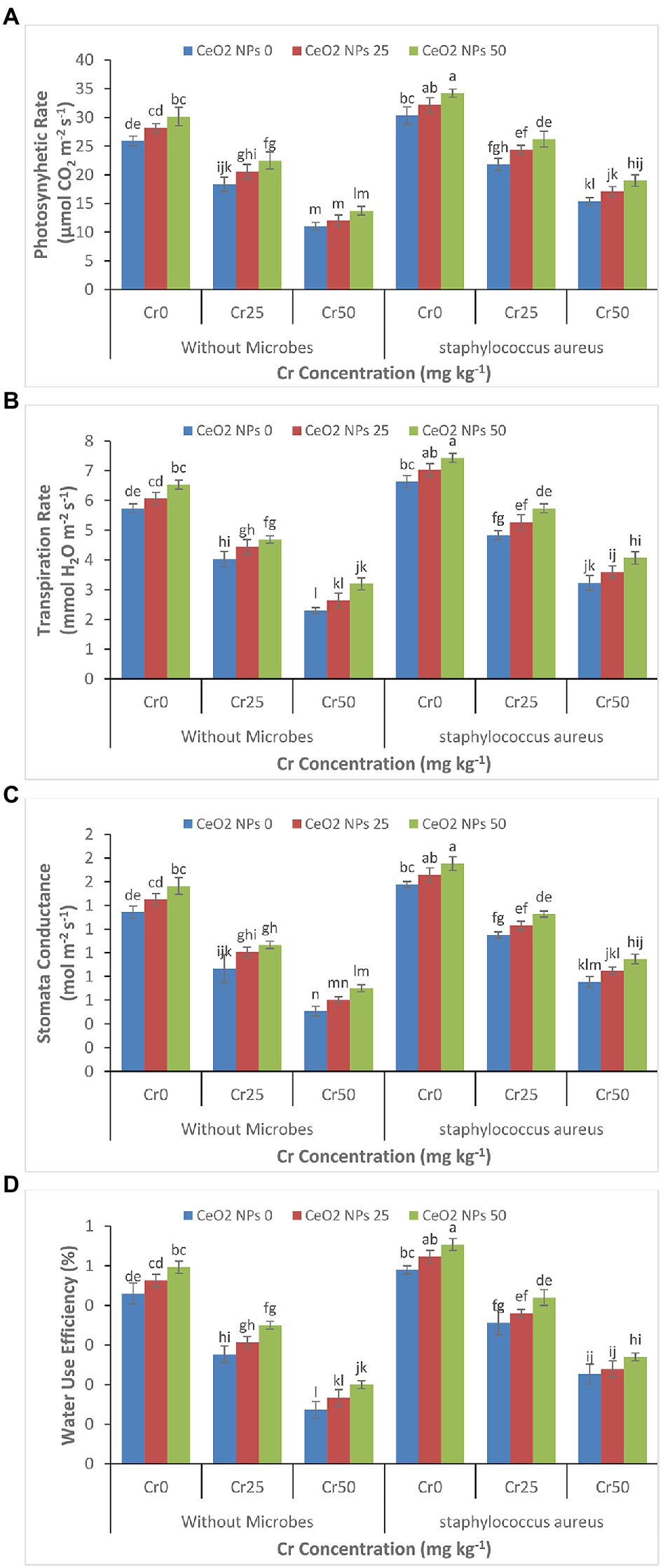
Figure 2. (A–D): Effect of CeO2 NPs and Staphylococcus aureus on photosynthetic rate (A), transpiration rate (B), stomatal conductance (C), and water use efficiency (D) in sunflower plant under Cr stress. The given values are means of three replications with standard deviation. The alphabets represent the significant/non-significant difference at p ≤ 0.05.
Effect on Chlorophyll Contents
Under Cr stress, chlorophyll contents such as Chlorophyll a, chlorophyll b, total chlorophyll, and carotenoids continued to decrease gradually with increasing concentration of chromium (Figures 3A–D). Whereas, the successful application of CeO2 NPs and S. aureus individually and collectively enhanced the chlorophyll contents in the sunflower plants facing chromium stress. The maximum increase was observed by the combined application of CeO2 NPs and S. aureus. In plants under chromium stress Cr 50, without S. aureus, CeO2 NPs from 0 to 50 improved the chl a content from 0.3 to 1.2 mg g−1 FW. The combined application of S. aureus and CeO2 NPs from 0 to 50 improved the chl a contents from 1.2 to 2.0 mg g−1 FW.
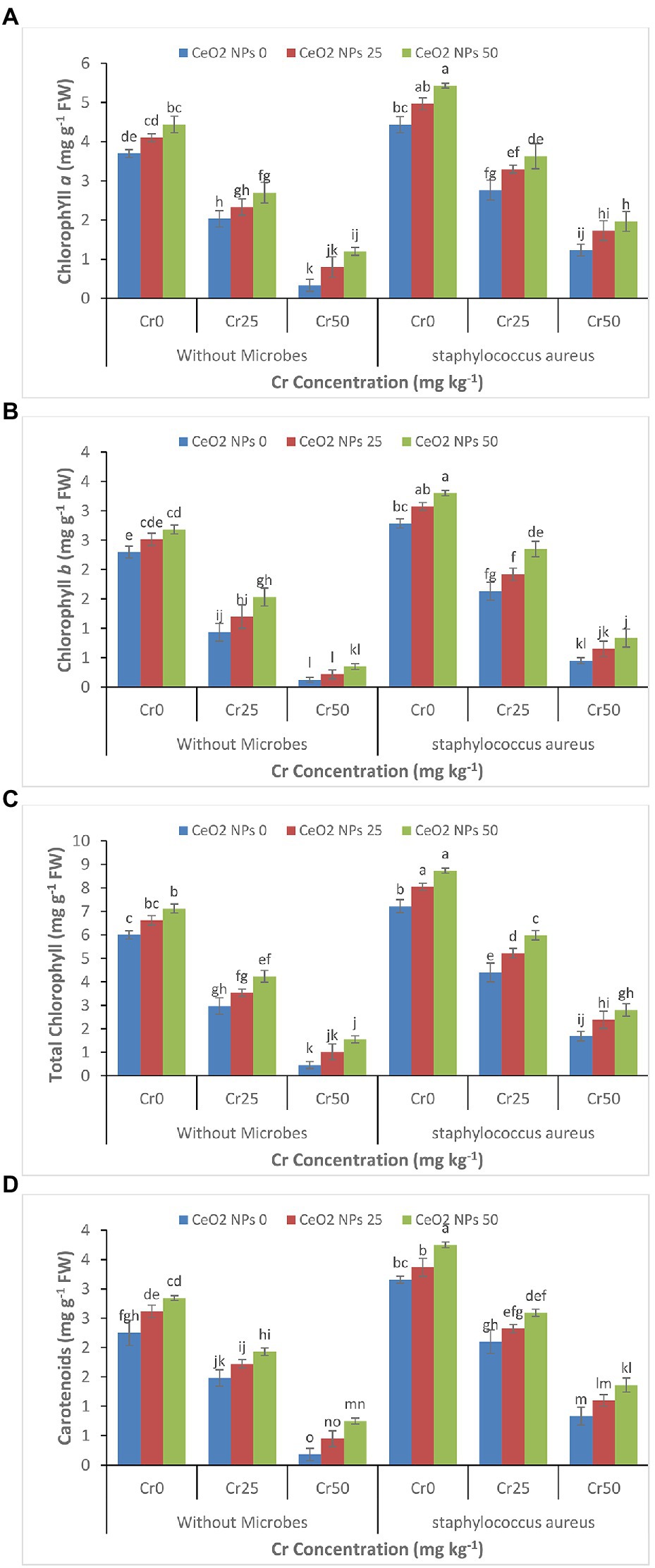
Figure 3. (A–D): Effect of CeO2 NPs and Staphylococcus aureus on chlorophyll a (A), chlorophyll b (B), total chlorophyll (C), and carotenoid content (D) in sunflower plant under Cr stress. The given values are means of three replications with standard deviation. The alphabets represent the significant/non-significant difference at p ≤ 0.05.
Effect on Enzymes Activity
The gradual decrease was depicted in enzymes activity (SOD, POD, CAT, and APX) in the sunflower plant with a gradual increase in the chromium concentration (0 to 50 mg kg−1; Figures 4A–D). The treatment of plants with CeO2 NPs alleviated the chromium stress and improved the plant’s enzyme activity under chromium stress. For instance, the SOD activity in plants under chromium stress Cr50 improved from 35 to 48 with increasing CeO2 NPs from 0 to 50 mg L−1. The maximum improvement in the enzyme’s activity in plants under the highest level of chromium stress (Cr50) was observed in plants treated with the combined application of S. aureus and CeO2 NPs. POD contents were increased from 657 to 853 Units g−1 FW.
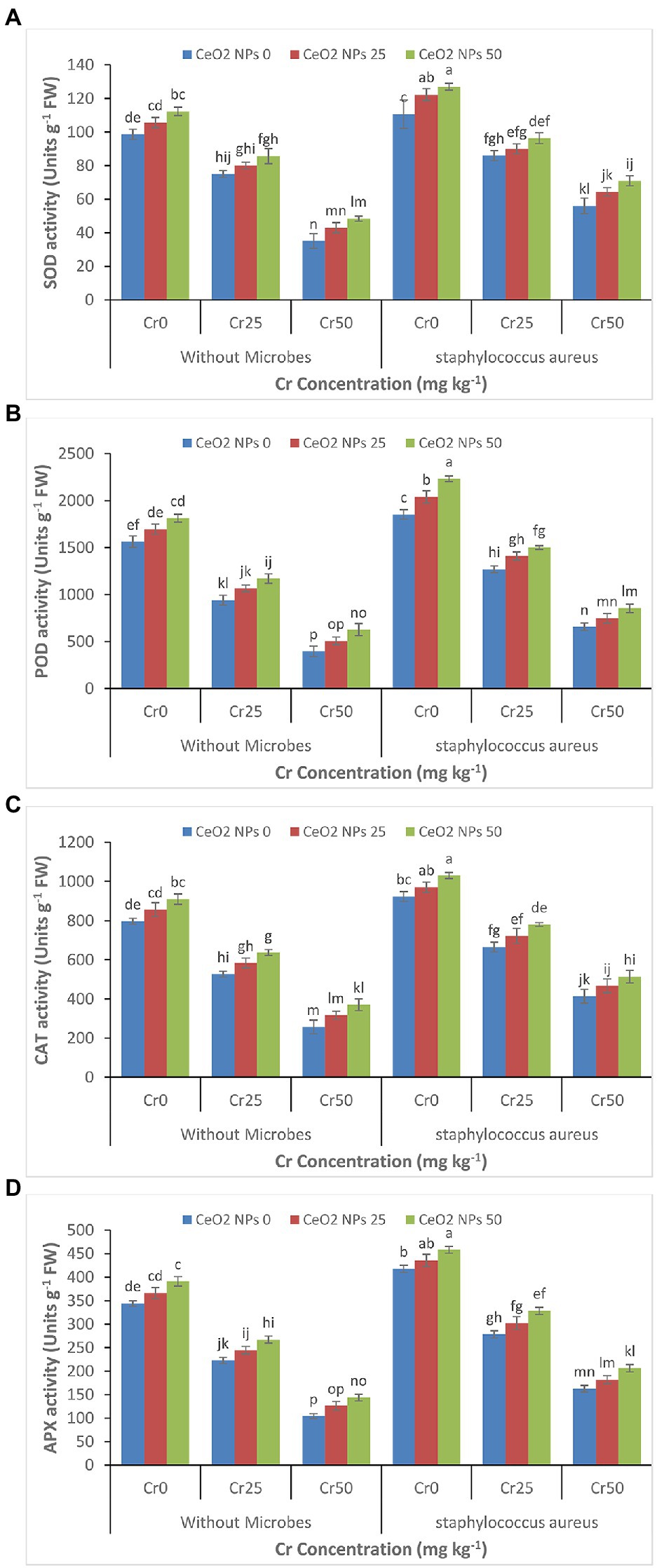
Figure 4. (A–D): Effect of CeO2 NPs and Staphylococcus aureus on SOD activity (A), peroxidases activity (B), catalase activity (C), and ascorbate oxidase activity (D) in sunflower plant under Cr stress. The given values are means of three replications with standard deviation. The alphabets represent the significant/non-significant difference at p ≤ 0.05.
Effect on Oxidative Stress
The increasing concentration of chromium increased the oxidative stress, which was evident from the increasing level of electrolyte leakage, malondialdehyde contents, and H2O2 contents in the sunflower plants (Figures 5A–C). The increasing chromium concentration from 0 to 50 increased the EL contents by 78 to 137%, MDA contents of 11 to 20 μmol g−1 FW, and H2O2 contents of 105 to 189 μmol g−1 FW in treatments without application of S. aureus and CeO2 NPs. In contrast, CeO2 NPs significantly decreased oxidative stress by reducing the EL contents 137 to 116% in treatments with chromium stress Cr50 and without S. aureus.
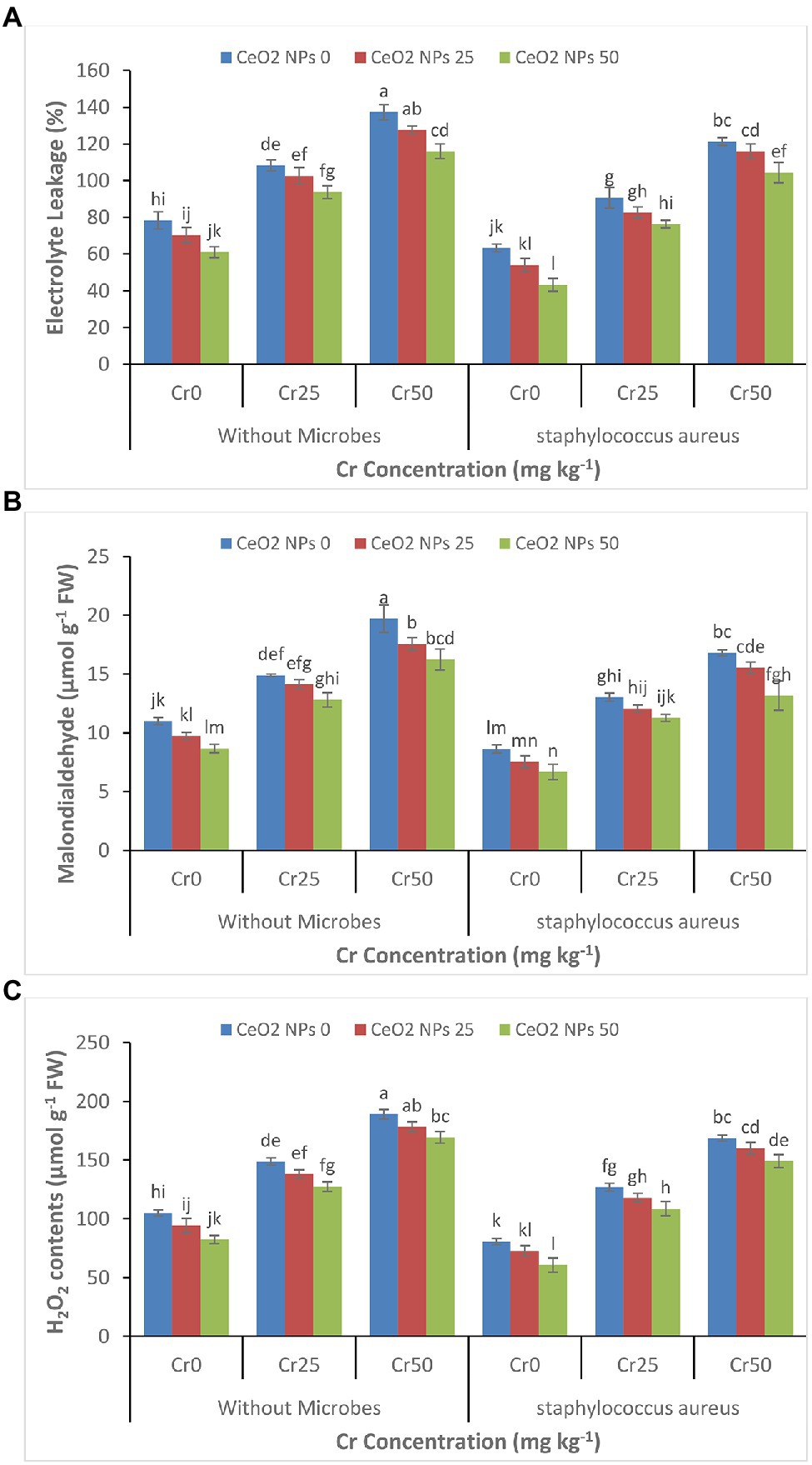
Figure 5. (A–C): Effect of CeO2 NPs and Staphylococcus aureus on electrolyte leakage (A), malondialdehyde contents (B) and H2O2 contents in sunflower plant under Cr stress. The given values are means of three replications with standard deviation. The alphabets represent the significant/non-significant difference at p ≤ 0.05.
Effect on Chromium Uptake and Accumulation
The increasing concentration of chromium (0–50 mg kg−1) increased the accumulation of chromium Cr+6 and Cr+3 in plant roots and shoots (Figures 6A–D). The Cr+6 concentration increased from 0.4 to 31 and 0.2 to 16.2 mg kg−1 DW in the plant’s roots and shoots. A similar trend was observed for Cr+3 in treatments without the application of S. aureus and CeO2 NPs. The application of CeO2 NPs decreased the accumulation of Cr+6 and Cr+3 in plant root, and shoots such as Cr+6 concentration in plant root decreased from 31 to 20.6 mg kg−1 DW and Cr+3 concentration decreased from 22 to 15.3 mg kg−1 DW in plant under stress level Cr50. However, the application of S. aureus brought a dramatic change in the accumulation of chromium by facilitating the more accumulation of Cr+3 than Cr+6 in plant roots and shoot. In plants treated with S. aureus, the Cr+3 accumulation in roots was 8.0 mg kg−1 FW compared to Cr+6 concentration, which was 6.8 mg kg−1 FW and 7.9 Cr+3 as compared to 3.3 mg kg−1 DW Cr+6 in the shoot at stress level Cr50.
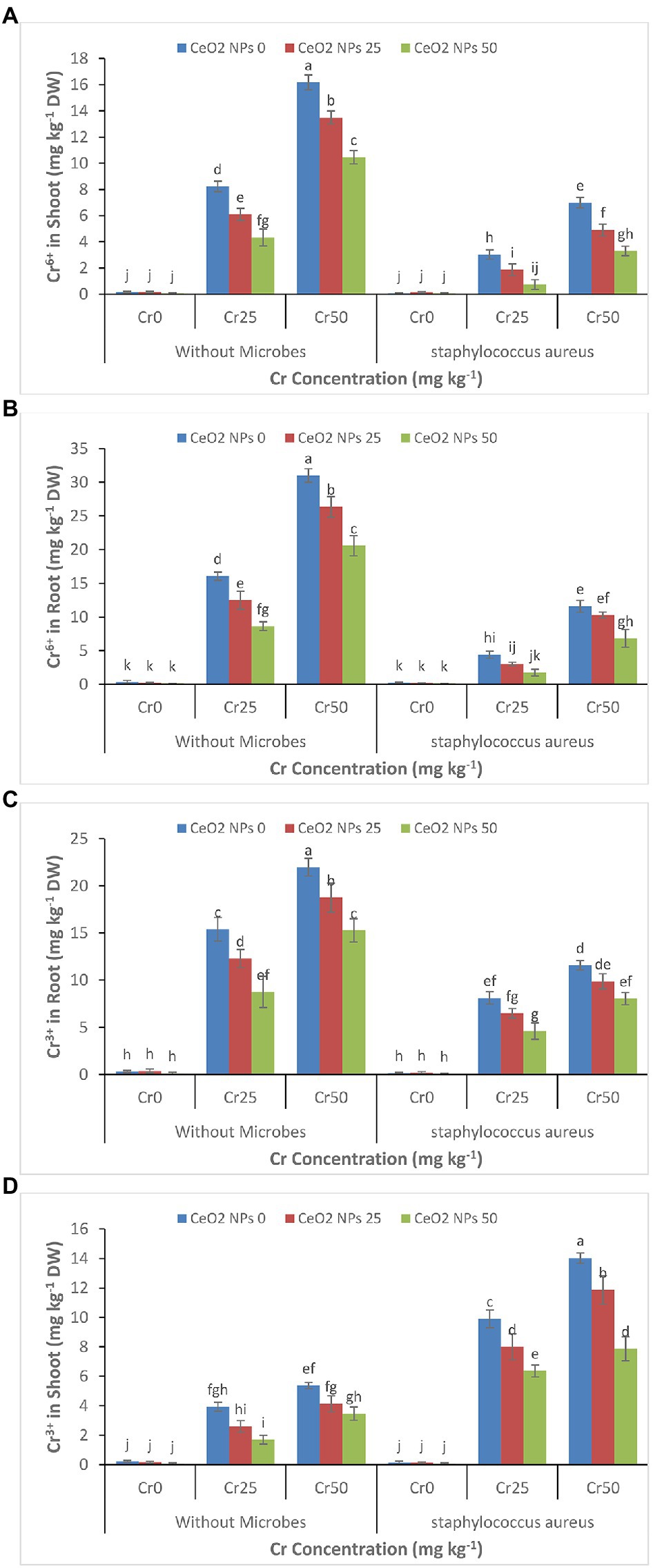
Figure 6. (A–D): Effect of CeO2 NPs and Staphylococcus aureus on bioaccumulation of Cr+6 in the shoot (A), Cr+6 in roots (B), Cr+3 in the shoot (C), and Cr+3 in roots (D) of sunflower plant under Cr stress. The given values are means of three replications with standard deviation. The alphabets represent the significant/non-significant difference at p ≤ 0.05.
Discussion
In this study, for the first time, we showed that the application of CeO2 NPs and bacteria S. aureus could alleviate chromium-induced toxicity in the sunflower plants at an optimal concentration of chromium (Cr50). The combined application of CeO2 nanoparticles and S. aureus efficiently ameliorated Cr-induced oxidative stress by promoting antioxidant enzymes activities and improved the photosynthetic activity by promoting chlorophyll contents in plants. In addition, CeO2 NPs and S. aureus decreased the Cr accumulation in roots and shoots in plants tolerating the highest chromium concentration (Cr50). The high concentration of chromium negatively affects morphological and agronomical parameters of plants through altering the water use efficiency, stomatal conductance, and photosynthesis process (Figures 1A–F, 2A–D; Ertani et al., 2017). In the present study, we evaluated that the application of CeO2 NPs alleviated the adverse impacts of chromium and improved plant agronomic characteristics (Figures 1A–F). Similar findings were reported by Gohari et al. (2020), where TiO2 NPs improved the agronomic traits in Moldavian balm under stress (Gohari et al., 2020). The large surface area and small size of the nanoparticles allow nanoparticles to penetrate in plant cells and then alleviate the negative effect of metal stress by improving plant growth (Singh et al., 2019). This increase in plant growth might be attributed by increases in water use efficiency, transpiration rate, stomatal conductance, and photosynthetic rate (Figures 2A–D; Hezaveh et al., 2019). The combined application of S. aureus and CeO2 NPs further improved the plant morphological and agronomic parameters as compared to the only application of NPs. It is well established that bacteria can increase essential nutrient availability to the plant (Ren et al., 2019). The bacteria also can degrade and transform the metals into less toxic forms and decrease the stress on the plant due to metal toxicity. Similarly, in this study, the application of S. aureus improved plant growth and biomass production due to its ability to detoxify the high concentration of chromium. The bacteria can improve plant nutrition through phosphorus solubilization, nitrogen fixation, and secretion of hormones and enzymes essential for plant growth and ultimately enhance the phytoremediation potential of plants (Marciano Marra et al., 2012; Matse et al., 2020).
The increased metal stress in various plant species resulted in a decrease in chlorophyll a, chlorophyll b, total chlorophyll, and carotenoid content, ultimately disrupting of whole photosynthesis process (Chandra and Kang, 2016; Sytar et al., 2019). The reduction in photosynthesis under metal stress is considered a sign of oxidative stress due to the rapid decrease in photosynthetic pigments and reduction in light absorbance capacity (Ashfaque et al., 2017). It was evident that the application of CeO2 NP improved the chlorophyll a, chlorophyll b, and total carotenoid content in plants under chromium stress and reduced the oxidative stress (Figures 3A–D). The beneficial role of many nanoparticles in enhancing the chlorophyll contents and improving the plant photosynthetic activity has been reported by many researchers. Such as application of CeO2 NPs acted as a catalyst in the production of Chl a and Chl b contents and increased the production of carotenoid contents in plant (Kataria et al., 2019; Etesami et al., 2021). Similarly, the foliar application of cerium oxide nanoparticles improved the photosynthesis pigment in Calendula officinalis (Jahani et al., 2019). The decline in antioxidant enzyme activity plays a crucial role in producing oxidative stress in plants suffering from chromium stress (Etesami, 2018). The significant increase in the chlorophyll contents and photosynthesis activity due to combined application of S. aureus and CeO2 NPs might be attributed to the ability of bacteria to increase the availability of micronutrients, decrease in bioavailability of chromium, and increase the ability of the plant to tolerate metal stress (Rizwan et al., 2019; Etesami et al., 2021). Similar to our findings, the application of bacteria improved the chlorophyll a, chlorophyll b, and carotenoid contents in the sunflower plant under Pb stress (Saleem et al., 2018). In another study, the TiO2 NPs improved the photosynthetic activity in soybean plants under Cd stress by entering the chloroplast and enhancing light adaption and electron transfer (Singh and Lee, 2016).
The increasing concentration of chromium declined the enzyme activity in the plants (Figures 4A–D). At the highest concentration of Cr50, the SOD activity, POD activity, CAT activity, and APX activity reduced sharply due to damage to the antioxidant enzymes metabolism induced by chromium toxicity. It is well reported that metal toxicity decreases the catalytic activities in the plant which ultimately decrease the activity of the antioxidant enzymes (Bhaduri and Fulekar, 2012). The production of ROS attacks antioxidants enzymes, consumes a huge concentration of antioxidant enzymes, and generates oxidative stress by disturbing the redox homeostasis in plants (He et al., 2017; Ahmad et al., 2019). This oxidative stress can cause severe damage to specific proteins and destruction to the cell structure and function (Ahmad et al., 2019). The application of nanoparticles has been reported to improve SOD, CAT, and POD activity without producing the hydroxyl radicals and improving the plants’ overall growth.
The chromium stress in plants leads to the production and accumulation of toxic substances such as reactive oxygen species (ROS) that initiate the lipid peroxidation and damage to cell structure (Figures 5A–C; Ghori et al., 2019; Jahani et al., 2019). The production of ROS initiates the death of plant cells by damaging specific proteins, lipids, and nucleic acids and decreases the chlorophyll contents (Sytar et al., 2019). This damage can be identified by increased production and accumulation of MDA, and increased concentration of electrolytes leakage (EL) and high content of H2O2 (Mallhi et al., 2019). Under metals stress, the concentrations of MDA, EL, and H2O2 increased in the plants (Berni et al., 2019; Ghori et al., 2019). The nanoparticle has been found to reduce ROS production in plants under metal stress. TiO2 NPs and Fe NPs have been found effective in reducing the abiotic stress in plants by reducing ROS production in Zea mays and grape, respectively (Mozafari and Ghaderi, 2018; Singh et al., 2021). In the current study, the increased production of antioxidants enzymes due to the positive effect of CeO2 NPs ultimately decreased the production of EL, MDA, and H2O2 contents in plants under chromium stress. In the plants, ROS production is initiated in response to stress, and antioxidants act as a defense mechanism against stress condition and act as a front line to cope with and protect the plant cell from free radicals and minimize the damage initiated by ROS in response to stress (Kohli et al., 2017). Antioxidant enzymes scavenge the H2O2 contents APX and SOD cope with ROS and neutralize the superoxidase radicals. Our study showed that CeO2 NPs increased the production of antioxidants enzymes, which resulted in decreased activity of EL, MDA, and H2O2 and alleviated the metal stress in the plant. Our findings agree with the aforementioned studies where the application of nanoparticles enhanced antioxidant enzyme activities in response to oxidative stress (Hussain et al., 2019b; Sun et al., 2019). The increased level of antioxidant enzyme activity might be linked to the antioxidant ability of nanoparticles, which enhances the plants’ activities (Jahani et al., 2019). It was found that the increasing concentration of Ag NPs increased the catalase and peroxidase activity in the Lycopersicon esculentum (Karami Mehrian et al., 2016). Similarly, wheat plants treated with silver and gold NPs significantly improved the plant dry biomass and improved the SOD, CAT, and APX activity in plants under abiotic stress (Manaf et al., 2021). The increase in antioxidant enzymes activity and decrease in ROS contents with the application of S. aureus may be attributed to bacteria’s ability to increase mRNA/gene expression of antioxidants in inoculated plants compared to non-inoculated plants (Gururani et al., 2013; Khan et al., 2022). The inoculation of bacteria enhanced the expression level of various ROS scavenging enzymes and increased the proline contents in potatoes plants under stress (Gururani et al., 2013).
The application of CeO2 nanoparticles reduces the uptake and accumulation of Cr+6 and Cr+3 in the sunflower root and shoots despite increasing chromium concentration (Figures 6A–D). Many studies reported that exogenous application of nanoparticles could reduce the uptake of toxic metals by plants (Hussain et al., 2019b; Rizwan et al., 2019). The use of TiO2 NPs in rice plants grown hydroponically reduced the concentration of Pb in roots and shoots and improved plant growth (Cai et al., 2017). Similarly, ZnO NPs decreased the arsenic concentration in the rice plat’s roots and leaves suffering from arsenic stress (Wu et al., 2020). It is well reported that nanoparticles can absorb and transform the heavy metals in the soils by reducing their mobility and bioavailability such as Fe3O4 NPs reduced the mobility of Cd in the soil and reduced its bioavailability to the plants (Chen et al., 2018; Hussain et al., 2019a). Thus, the decreased accumulation of chromium in the sunflower plant can be attributed to the ability of the nanoparticle to decrease the bioavailability of chromium. Further, most of the NPs accumulate in the cell wall, bind with the heavy metals, make them unavailable, and hinder the migration of heavy metals in the plant (Molnár et al., 2020). The bacteria can tolerate metal toxicity and break down and remove the heavy metal through their metabolic process. Bacteria can reduce the bioavailability of heavy metals through biosorption, bioaccumulation, biotransformation, bio-precipitation, and bio-crystallization in contaminated soil (Medfu Tarekegn et al., 2020). In this study, the application of bacteria transformed the Cr+6 into Cr+3 through their metabolic process. It reduced the bioavailability of the chromium to the plant, which ultimately reduced its bioaccumulation in plant roots and shoots. Similarly, the application of S. aureus in wheat plants reduced the uptake and accumulation of chromium in plant roots and shoots grown in chromium-contaminated soil (Zeng et al., 2020). The nanoparticles can improve bacterial growth when applied in small amounts; for example, the use of TiO2 NPs improved the performance of plant growth-promoting rhizospheric bacteria in plants under stress (Timmusk et al., 2018).
Conclusion
The application of nanoparticles is a promising approach that has the tremendous potential to protect the plant from metal-induced stress. The CeO2 NPs improved the morphological, physiological, and biochemical properties and overall growth and biomass production of sunflower plants grown in the high chromium concentration. The CeO2 NPs reduce the H2O2 stress, enhance the antioxidant enzymatic activities, and ultimately alleviate plants’ oxidative stress due to chromium toxicity. Along with nanoparticles, bacteria also have a prominent role in alleviating the metal-induced toxicity through their metabolic process and reducing the bioavailability of Cr to the plant. The combined application of S. aureus and CeO2 nanoparticles improved the metabolic process, triggered the activation of the enzymatic defense system, and thus enhanced the plant performance in sunflower plants under chromium stress. The use of nanoparticles and bacteria in combination could be a novel way to clean up contaminated soil and strengthen plants to withstand metal-induced stress. The novel application of nanoparticles in agriculture may aid in meeting rising food demand while also ensuring environmental sustainability. However, there is still needed to further explore about action mechanism of nanoparticles, permissible limit and ecotoxicity in edible crops.
Data Availability Statement
The raw data supporting the conclusions of this article will be made available by the authors, without undue reservation.
Author Contributions
JM: conceptualization and project administration. HA: data curtain and investigation. MO: funding and resources allocation. YA: formal analysis and software. FC: methodology and data curtain. MA: formal analysis and project administration. AH: review and editing and formal analysis. SH: investigation and formal analysis. MS: writing an original draft, methodology, and review and editing. All authors contributed to the article and approved the submitted version.
Funding
This work was supported by the National Natural Science Foundation of China (no. 51974313) and the key project of Jiangsu Key Laboratory of Coal-Based Greenhouse Gas Control and Utilization (2020ZDZZ03). The authors extend their appreciation to the Researchers Supporting Project number (RSP-2021/374) King Saud University, Riyadh, Saudi Arabia.
Conflict of Interest
The authors declare that the research was conducted in the absence of any commercial or financial relationships that could be construed as a potential conflict of interest.
Publisher’s Note
All claims expressed in this article are solely those of the authors and do not necessarily represent those of their affiliated organizations, or those of the publisher, the editors and the reviewers. Any product that may be evaluated in this article, or claim that may be made by its manufacturer, is not guaranteed or endorsed by the publisher.
References
Aebi, H. (1984). Catalase in vitro. Methods Enzymol. 105, 121–126. doi: 10.1016/S0076-6879(84)05016-3
Ahmad, R., Hussain, S., Anjum, M. A., Khalid, M. F., Saqib, M., Zakir, I., et al. (2019). Oxidative stress and antioxidant defense mechanisms in plants under salt stress. Plant Abiotic Str. Tol., 191–205. doi: 10.1007/978-3-030-06118-0_8
Alaboudi, K. A., Ahmed, B., and Brodie, G. (2018). Phytoremediation of Pb and cd contaminated soils by using sunflower (Helianthus annuus) plant. Ann. Agric. Sci. 63, 123–127. doi: 10.1016/j.aoas.2018.05.007
Ashfaque, F., Inam, A., Iqbal, S., and Sahay, S. (2017). Response of silicon on metal accumulation, photosynthetic inhibition and oxidative stress in chromium-induced mustard (Brassica juncea L.). South African J. Bot. 111, 153–160. doi: 10.1016/j.sajb.2017.03.002
Ashraf, S., Ali, Q., Zahir, Z. A., Ashraf, S., and Asghar, H. N. (2019). Phytoremediation: environmentally sustainable way for reclamation of heavy metal polluted soils. Ecotoxicol. Environ. Saf. 174, 714–727. doi: 10.1016/j.ecoenv.2019.02.068
Baker, A. J. M., McGrath, S. P., Reeves, R. D., and Smith, J. A. C. (2020). Metal hyperaccumulator plants: a review of the ecology and physiology of a biological resource for phytoremediation of metal-polluted soils. Phytorem. Contam. Soil Wat., 85–107. doi: 10.1201/9780367803148-5
Belay, A. A. (2010). Impacts of chromium from tannery effluent and evaluation of alternative treatment options. J. Environ. Prot. 01, 53–58. doi: 10.4236/jep.2010.11007
Berni, R., Luyckx, M., Xu, X., Legay, S., Sergeant, K., Hausman, J. F., et al. (2019). Reactive oxygen species and heavy metal stress in plants: impact on the cell wall and secondary metabolism. Environ. Exp. Bot. 161, 98–106. doi: 10.1016/j.envexpbot.2018.10.017
Bhaduri, A. M., and Fulekar, M. H. (2012). Antioxidant enzyme responses of plants to heavy metal stress. Rev. Environ. Sci. Bio/Technology 11, 55–69. doi: 10.1007/s11157-011-9251-x
Cai, F., Wu, X., Zhang, H., Shen, X., Zhang, M., Chen, W., et al. (2017). Impact of TiO2 nanoparticles on lead uptake and bioaccumulation in rice (Oryza sativa L.). NanoImpact 5, 101–108. doi: 10.1016/j.impact.2017.01.006
Chandra, R., and Kang, H. (2016). Mixed heavy metal stress on photosynthesis, transpiration rate, and chlorophyll content in poplar hybrids. Forest Sci. Technol. 12, 55–61. doi: 10.1080/21580103.2015.1044024
Chen, L., Yang, J., and Wang, D. (2020). Phytoremediation of uranium and cadmium contaminated soils by sunflower (Helianthus annuus L.) enhanced with biodegradable chelating agents. J. Clean. Prod. 263:121491. doi: 10.1016/j.jclepro.2020.121491
Chen, T., Zou, H., Wu, X., Liu, C., Situ, B., Zheng, L., et al. (2018). Nanozymatic antioxidant system based on MoS2 nanosheets. ACS Appl. Mater. Interfaces 10, 12453–12462. doi: 10.1021/acsami.8b01245
Devi, R., Behera, B., Raza, M. B., Mangal, V., Altaf, M. A., Kumar, R., et al. (2021). An insight into microbes mediated heavy metal detoxification in plants: a review. J. Soil Sci. Plant Nutr. 22, 914–936. doi: 10.1007/s42729-021-00702-x
Dionisio-Sese, M. L., and Tobita, S. (1998). Antioxidant responses of rice seedlings to salinity stress. Plant Sci. 135, 1–9. doi: 10.1016/S0168-9452(98)00025-9
Ertani, A., Mietto, A., Borin, M., and Nardi, S. (2017). Chromium in agricultural soils and crops: a review. Water Air Soil Pollut. 228, 1–12. doi: 10.1007/s11270-017-3356-y
Etesami, H. (2018). Bacterial mediated alleviation of heavy metal stress and decreased accumulation of metals in plant tissues: mechanisms and future prospects. Ecotoxicol. Environ. Saf. 147, 175–191. doi: 10.1016/j.ecoenv.2017.08.032
Etesami, H., Fatemi, H., and Rizwan, M. (2021). Interactions of nanoparticles and salinity stress at physiological, biochemical and molecular levels in plants: A review. Ecotoxicol. Environ. Saf. 225:112769. doi: 10.1016/j.ecoenv.2021.112769
Fozia, A., Muhammad, A. Z., Muhammad, A., and Zafar, M. K. (2008). Effect of chromium on growth attributes in sunflower (Helianthus annuus L.). J. Environ. Sci. 20, 1475–1480. doi: 10.1016/S1001-0742(08)62552-8
Ghori, N. H., Ghori, T., Hayat, M. Q., Imadi, S. R., Gul, A., Altay, V., et al. (2019). Heavy metal stress and responses in plants. Int. J. Environ. Sci. Technol. 16, 1807–1828. doi: 10.1007/s13762-019-02215-8
Gohari, G., Mohammadi, A., Akbari, A., Panahirad, S., Dadpour, M. R., Fotopoulos, V., et al. (2020). Titanium dioxide nanoparticles (TiO2 NPs) promote growth and ameliorate salinity stress effects on essential oil profile and biochemical attributes of Dracocephalum moldavica. Sci. Rep. 10, 1–14. doi: 10.1038/s41598-020-57794-1
Gururani, M. A., Upadhyaya, C. P., Baskar, V., Venkatesh, J., Nookaraju, A., and Park, S. W. (2013). Plant growth-promoting rhizobacteria enhance abiotic stress tolerance in Solanum tuberosum through inducing changes in the expression of ROS-scavenging enzymes and improved photosynthetic performance. J. Plant Growth Regul. 32, 245–258. doi: 10.1007/s00344-012-9292-6
Hauptvogl, M., Kotrla, M., Prčík, M., Pauková, Ž., Kováčik, M., and Lošák, T. (2019). Phytoremediation potential of fast-growing energy plants: challenges and perspectives–A review. Polish J. Environ. Stud. 29, 505–516. doi: 10.15244/pjoes/101621
He, L., He, T., Farrar, S., Ji, L., Liu, T., and Ma, X. (2017). Antioxidants maintain cellular redox homeostasis by elimination of reactive oxygen species. Cell. Physiol. Biochem. 44, 532–553. doi: 10.1159/000485089
Hezaveh, T. A., Pourakbar, L., Rahmani, F., and Alipour, H. (2019). Interactive effects of salinity and ZnO nanoparticles on physiological and molecular parameters of rapeseed (Brassica napus L.). Commun. Soil Sci. Plant Anal. 50, 698–715. doi: 10.1080/00103624.2019.1589481
Hussain, A., Ali, S., Rizwan, M., Rehman, M. Z., Qayyum, M. F., Wang, H., et al. (2019a). Responses of wheat (Triticum aestivum) plants grown in a cd contaminated soil to the application of iron oxide nanoparticles. Ecotoxicol. Environ. Saf. 173, 156–164. doi: 10.1016/j.ecoenv.2019.01.118
Hussain, A., Rizwan, M., Ali, Q., and Ali, S. (2019b). Seed priming with silicon nanoparticles improved the biomass and yield while reduced the oxidative stress and cadmium concentration in wheat grains. Environ. Sci. Pollut. Res. 26, 7579–7588. doi: 10.1007/s11356-019-04210-5
Jahani, S., Saadatmand, S., Mahmoodzadeh, H., and Khavari-Nejad, R. A. (2019). Effect of foliar application of cerium oxide nanoparticles on growth, photosynthetic pigments, electrolyte leakage, compatible osmolytes and antioxidant enzymes activities of Calendula officinalis L. Biologia 74, 1063–1075. doi: 10.2478/s11756-019-00239-6
Jana, S., and Choudhuri, M. A. (1981). Glycolate metabolism of three submersed aquatic angiosperms: effect of heavy metals. Aquat. Bot. 11, 67–77. doi: 10.1016/0304-3770(81)90047-4
Kale, A. P., and Gawade, S. N. (2016). Studies on nanoparticle induced nutrient use efficiency of fertilizer and crop productivity. Green Chem. Tech. Lett. 2, 88–92. doi: 10.18510/gctl.2016.226
Kang, C.-H., Kwon, Y.-J., and So, J.-S. (2016). Bioremediation of heavy metals by using bacterial mixtures. Ecol. Eng. 89, 64–69. doi: 10.1016/j.ecoleng.2016.01.023
Karami Mehrian, S., Heidari, R., Rahmani, F., and Najafi, S. (2016). Effect of chemical synthesis silver nanoparticles on germination indices and seedlings growth in seven varieties of Lycopersicon esculentum mill (tomato) plants. J. Clust. Sci. 27, 327–340. doi: 10.1007/s10876-015-0932-4
Kataria, S., Jain, M., Rastogi, A., Živčák, M., Brestic, M., Liu, S., et al. (2019). “Role of nanoparticles on photosynthesis: avenues and applications,” in Nanomaterials in Plants, Algae and Microorganisms (United States: Elsevier), 103–127.
Kaya, Y., Jocic, S., and Miladinovic, D. (2012). “Sunflower,” in Technological Innovations in Major World Oil Crops. Vol 1 (United States: Springer), 85–129.
Khan, A. L., Numan, M., Bilal, S., Asaf, S., Crafword, K., Imran, M., et al. (2022). Mangrove’s rhizospheric engineering with bacterial inoculation improve degradation of diesel contamination. J. Haz. Mat. 423:127046. doi: 10.1016/j.jhazmat.2021.127046
Kohli, S. K., Handa, N., Gautam, V., Bali, S., Sharma, A., Khanna, K., et al. (2017). “ROS signaling in plants under heavy metal stress” in Reactive Oxygen Species and Antioxidant Systems in Plants: Role and Regulation Under Abiotic Stress (United States: Springer), 185–214.
Kong, Z., Wu, Z., Glick, B. R., He, S., Huang, C., and Wu, L. (2019). Co-occurrence patterns of microbial communities affected by inoculants of plant growth-promoting bacteria during phytoremediation of heavy metal-contaminated soils. Ecotoxicol. Environ. Saf. 183:109504. doi: 10.1016/j.ecoenv.2019.109504
Lichtenthaler, H. K. (1987). [34] chlorophylls and carotenoids: pigments of photosynthetic biomembranes. Methods Enzymol. 148, 350–382. doi: 10.1016/0076-6879(87)48036-1
Ma, Y., Rajkumar, M., Oliveira, R. S., Zhang, C., and Freitas, H. (2019). Potential of plant beneficial bacteria and arbuscular mycorrhizal fungi in phytoremediation of metal-contaminated saline soils. J. Hazard. Mater. 379:120813. doi: 10.1016/j.jhazmat.2019.120813
Mallhi, Z. I., Rizwan, M., Mansha, A., Ali, Q., Asim, S., Ali, S., et al. (2019). Citric acid enhances plant growth, photosynthesis, and phytoextraction of lead by alleviating the oxidative stress in castor beans. Plan. Theory 8:525. doi: 10.3390/plants8110525
Manaf, A., Wang, X., Tariq, F., Jhanzab, H. M., Bibi, Y., Sher, A., et al. (2021). Antioxidant enzyme activities correlated with growth parameters of wheat sprayed with silver and gold nanoparticle suspensions. Agronomy 11:1494. doi: 10.3390/agronomy11081494
Marciano Marra, L., Fonsêca Sousa Soares, C. R., de Oliveira, S. M., Avelar Ferreira, P. A., Lima Soares, B., de Fráguas Carvalho, R., et al. (2012). Biological nitrogen fixation and phosphate solubilization by bacteria isolated from tropical soils. Plant Soil 357, 289–307. doi: 10.1007/s11104-012-1157-z
Matse, D. T., Huang, C.-H., Huang, Y.-M., and Yen, M.-Y. (2020). Effects of coinoculation of rhizobium with plant growth promoting rhizobacteria on the nitrogen fixation and nutrient uptake of Trifolium repens in low phosphorus soil. J. Plant Nutr. 43, 739–752. doi: 10.1080/01904167.2019.1702205
Medfu Tarekegn, M., Zewdu Salilih, F., and Ishetu, A. I. (2020). Microbes used as a tool for bioremediation of heavy metal from the environment. Cogent Food Agric. 6:1783174. doi: 10.1080/23311932.2020.1783174
Molnár, Á., Rónavári, A., Bélteky, P., Szőllősi, R., Valyon, E., Oláh, D., et al. (2020). ZnO nanoparticles induce cell wall remodeling and modify ROS/RNS signalling in roots of brassica seedlings. Ecotoxicol. Environ. Saf. 206:111158. doi: 10.1016/j.ecoenv.2020.111158
Mozafari, A., and Ghaderi, N. (2018). Grape response to salinity stress and role of iron nanoparticle and potassium silicate to mitigate salt induced damage under in vitro conditions. Physiol. Mol. Biol. Plants 24, 25–35. doi: 10.1007/s12298-017-0488-x
Naik, M., and Kumar, P. (2020). Role of growth regulators and microbes for metal detoxification in plants and soil. Plant Arch. 20, 2820–2824.
Nakano, Y., and Physiology, K. A.-P. (1981). Hydrogen peroxide is scavenged by Ascorbate-specific peroxidase in spinach chloroplasts. Plant Cell Physiol. 22, 867–880. doi: 10.1093/oxfordjournals.pcp.a076232
Omara, A. E.-D., Elsakhawy, T., Alshaal, T., El-Ramady, H., Kovács, Z., and Fári, M. (2019). Nanoparticles: a novel approach for sustainable agro-productivity. Environ. Biodivers. Soil Secur. 3, 30–40. doi: 10.21608/jenvbs.2019.7478.1050
Peer, W. A., Baxter, I. R., Richards, E. L., Freeman, J. L., and Murphy, A. S. (2005). “Phytoremediation and hyperaccumulator plants,” in Molecular Biology of Metal Homeostasis and Detoxification (United States: Springer), 299–340.
Pushkar, B., Sevak, P., Parab, S., and Nilkanth, N. (2021). Chromium pollution and its bioremediation mechanisms in bacteria: A review. J. Environ. Manag. 287:112279. doi: 10.1016/j.jenvman.2021.112279
Rehman, M. Z., Rizwan, M., Khalid, H., Ali, S., Naeem, A., Yousaf, B., et al. (2018). Farmyard manure alone and combined with immobilizing amendments reduced cadmium accumulation in wheat and rice grains grown in field irrigated with raw effluents. Chemosphere 199, 468–476. doi: 10.1016/j.chemosphere.2018.02.030
Ren, X.-M., Guo, S.-J., Tian, W., Chen, Y., Han, H., Chen, E., et al. (2019). Effects of plant growth-promoting bacteria (PGPB) inoculation on the growth, antioxidant activity, cu uptake, and bacterial community structure of rape (Brassica napus L.) grown in cu-contaminated agricultural soil. Front. Microbiol. 10:1455. doi: 10.3389/fmicb.2019.01455
Rizwan, M., Ali, S., Ali, B., Adrees, M., Arshad, M., Hussain, A., et al. (2019). Zinc and iron oxide nanoparticles improved the plant growth and reduced the oxidative stress and cadmium concentration in wheat. Chemosphere 214, 269–277. doi: 10.1016/j.chemosphere.2018.09.120
Sabir, S., Arshad, M., and Chaudhari, S. K. (2014). Zinc oxide nanoparticles for revolutionizing agriculture: synthesis and applications. Sci. World J. 2014:925494. doi: 10.1155/2014/925494
Saleem, M., Asghar, H. N., Zahir, Z. A., and Shahid, M. (2018). Impact of lead tolerant plant growth promoting rhizobacteria on growth, physiology, antioxidant activities, yield and lead content in sunflower in lead contaminated soil. Chemosphere 195, 606–614. doi: 10.1016/j.chemosphere.2017.12.117
Shahid, M. J., al-surhanee, A. A., Kouadri, F., Ali, S., Nawaz, N., Afzal, M., et al. (2020). Role of microorganisms in the remediation of wastewater in floating treatmentwetlands: A review. Sustainability 12:559. doi: 10.3390/su12145559
Shahid, M. J., Arslan, M., Siddique, M., Ali, S., Tahseen, R., and Afzal, M. (2019). Potentialities of floating wetlands for the treatment of polluted water of river Ravi. Pakistan. Ecol. Eng. 133, 167–176. doi: 10.1016/j.ecoleng.2019.04.022
Shahid, M., Dumat, C., Khalid, S., Schreck, E., Xiong, T., and Niazi, N. K. (2017). Foliar heavy metal uptake, toxicity and detoxification in plants: A comparison of foliar and root metal uptake. J. Hazard. Mater. 325, 36–58. doi: 10.1016/j.jhazmat.2016.11.063
Shanker, A. K., Cervantes, C., Loza-Tavera, H., and Avudainayagam, S. (2005). Chromium toxicity in plants. Environ. Int. 31, 739–753. doi: 10.1016/j.envint.2005.02.003
Sharma, S. S., Dietz, K., and Mimura, T. (2016). Vacuolar compartmentalization as indispensable component of heavy metal detoxification in plants. Plant Cell Environ. 39, 1112–1126. doi: 10.1111/pce.12706
Singh, A., Hussain, I., Singh, N. B., and Singh, H. (2019). Uptake, translocation and impact of green synthesized nanoceria on growth and antioxidant enzymes activity of Solanum lycopersicum L. Ecotoxicol. Environ. Saf. 182:109410. doi: 10.1016/j.ecoenv.2019.109410
Singh, J., and Lee, B.-K. (2016). Influence of nano-TiO2 particles on the bioaccumulation of cd in soybean plants (Glycine max): A possible mechanism for the removal of cd from the contaminated soil. J. Environ. Manag. 170, 88–96. doi: 10.1016/j.jenvman.2016.01.015
Singh, H. P., Mahajan, P., Kaur, S., Batish, D. R., and Kohli, R. K. (2013). Chromium toxicity and tolerance in plants. Environ. Chem. Lett. 11, 229–254. doi: 10.1007/s10311-013-0407-5
Singh, A., Tiwari, S., Pandey, J., Lata, C., and Singh, I. K. (2021). Role of nanoparticles in crop improvement and abiotic stress management. J. Biotechnol. 337, 57–70. doi: 10.1016/j.jbiotec.2021.06.022
Soltanpour, P. N. (1991). “Determination of nutrient availability and elemental toxicity by AB-DTPA soil test and ICPS,” in Advances in Soil Science (United States: Springer), 165–190.
Song, B., Xu, P., Chen, M., Tang, W., Zeng, G., Gong, J., et al. (2019). Using nanomaterials to facilitate the phytoremediation of contaminated soil. Crit. Rev. Environ. Sci. Technol. 49, 791–824. doi: 10.1080/10643389.2018.1558891
Sun, S., Li, X., Sun, C., Cao, W., Hu, C., Zhao, Y., et al. (2019). Effects of ZnO nanoparticles on the toxicity of cadmium to duckweed Lemna minor. Sci. Total Environ. 662, 697–702. doi: 10.1016/j.scitotenv.2019.01.275
Sytar, O., Kumari, P., Yadav, S., Brestic, M., and Rastogi, A. (2019). Phytohormone priming: regulator for heavy metal stress in plants. J. Plant Growth Regul. 38, 739–752. doi: 10.1007/s00344-018-9886-8
Timmusk, S., Seisenbaeva, G., and Behers, L. (2018). Titania (TiO2) nanoparticles enhance the performance of growth-promoting rhizobacteria. Sci. Rep. 8, 1–13. doi: 10.1038/s41598-017-18939-x
Tiquia-Arashiro, S. M. (2018). Lead absorption mechanisms in bacteria as strategies for lead bioremediation. Appl. Microbiol. Biotechnol. 102, 5437–5444. doi: 10.1007/s00253-018-8969-6
Tripathi, D. K., Singh, V. P., Prasad, S. M., Chauhan, D. K., and Dubey, N. K. (2015). Silicon nanoparticles (SiNp) alleviate chromium (VI) phytotoxicity in Pisum sativum (L.) seedlings. Plant Physiol. Biochem. 96, 189–198. doi: 10.1016/j.plaphy.2015.07.026
Tumolo, M., Ancona, V., De Paola, D., Losacco, D., Campanale, C., Massarelli, C., et al. (2020). Chromium pollution in European water, sources, health risk, and remediation strategies: an overview. Int. J. Environ. Res. Public Health 17:5438. doi: 10.3390/ijerph17155438
Wu, F., Fang, Q., Yan, S., Pan, L., Tang, X., and Ye, W. (2020). Effects of zinc oxide nanoparticles on arsenic stress in rice (Oryza sativa L.): germination, early growth, and arsenic uptake. Environ. Sci. Pollut. Res. 27, 26974–26981. doi: 10.1007/s11356-020-08965-0
Yoshinaga, M., Ninomiya, H., Al Hossain, M. M. A., Sudo, M., Akhand, A. A., Ahsan, N., et al. (2018). A comprehensive study including monitoring, assessment of health effects and development of a remediation method for chromium pollution. Chemosphere 201, 667–675. doi: 10.1016/j.chemosphere.2018.03.026
Zand, A. D., Mikaeili Tabrizi, A., and Vaezi Heir, A. (2020). Application of titanium dioxide nanoparticles to promote phytoremediation of cd-polluted soil: contribution of PGPR inoculation. Biorem. J. 24, 171–189. doi: 10.1080/10889868.2020.1799929
Zeng, F., Zahoor, M., Waseem, M., Anayat, A., Rizwan, M., Ahmad, A., et al. (2020). Influence of metal-resistant Staphylococcus aureus strain K1 on the alleviation of chromium stress in wheat. Agronomy 10:1354. doi: 10.3390/agronomy10091354
Zhang, X. Z. (1992). The measurement and mechanism of lipid peroxidation and SOD, POD and CAT activities in biological system. Res. Methodol. Crop Physiol. Agric. Press., 208–211.
Zhang, J., and Kirkham, M. B. (1994). Drought-stress-induced changes in activities of superoxide dismutase, catalase, and peroxidase in wheat species. Plant Cell Physiol. 35, 785–791. doi: 10.1093/oxfordjournals.pcp.a078658
Zhu, Y., Xu, F., Liu, Q., Chen, M., Liu, X., Wang, Y., et al. (2019). Nanomaterials and plants: positive effects, toxicity and the remediation of metal and metalloid pollution in soil. Sci. Total Environ. 662, 414–421. doi: 10.1016/j.scitotenv.2019.01.234
Keywords: bacteria, chromium, nanoparticles, phytoremediation, sunflower
Citation: Ma J, Alshaya H, Okla MK, Alwasel YA, Chen F, Adrees M, Hussain A, Hameed S and Shahid MJ (2022) Application of Cerium Dioxide Nanoparticles and Chromium-Resistant Bacteria Reduced Chromium Toxicity in Sunflower Plants. Front. Plant Sci. 13:876119. doi: 10.3389/fpls.2022.876119
Edited by:
Rafaqat Ali Gill, Oil Crops Research Institute (CAAS), ChinaReviewed by:
Theodore Mulembo Mwamba, University of Lubumbashi, Democratic Republic of CongoSyed Tahir Ata-Ul-Karim, The University of Tokyo, Japan
Copyright © 2022 Ma, Alshaya, Okla, Alwasel, Chen, Adrees, Hussain, Hameed and Shahid. This is an open-access article distributed under the terms of the Creative Commons Attribution License (CC BY). The use, distribution or reproduction in other forums is permitted, provided the original author(s) and the copyright owner(s) are credited and that the original publication in this journal is cited, in accordance with accepted academic practice. No use, distribution or reproduction is permitted which does not comply with these terms.
*Correspondence: Muhammad Adrees, bWFkcmVlc0BnY3VmLmVkdS5waw==