- 1College of Plant Protection, Shenyang Agricultural University, Shenyang, China
- 2College of Bioscience and Biotechnology, Shenyang Agricultural University, Shenyang, China
- 3Liaoning Academy of Agricultural Sciences, Shenyang, China
- 4Key Laboratory of Protected Horticulture of Ministry of Education, Shenyang Agricultural University, Shenyang, China
Powdery mildew (PM) caused by Podosphaera xanthii poses a continuous threat to the performance and yield of the cucumber (Cucumis sativus L.). Control in the initial stages of infection is particularly important. Here, we studied the differential physiological and transcriptomic changes between PM-resistant strain B21-a-2-1-2 and PM-susceptible strain B21-a-2-2-2 at the early stage of P. xanthii attack. When challenged with P. xanthii, the tolerant line can postpone the formation of the pathogen primary germ. Comparative transcriptomic analysis suggested that DEGs related to the cell wall and to pathogen and hormone responses were similar enriched in both cucumber lines under P. xanthii infection. Notably, the number of DEGs triggered by P. xanthii in B21-a-2-1-2 was quintuple that in B21-a-2-2-2, revealing that the success of defense of resistant cucumber is due to rapidly mobilizing multiple responses. The unique responses detected were genes related to SA signaling, MAPK signaling, and Dof and WRKY transcription factors. Furthermore, 5 P. xanthii -inducible hub genes were identified, including GLPK, ILK1, EIN2, BCDHβ1, and RGGA, which are considered to be key candidate genes for disease control. This study combined multiple analytical approaches to capture potential molecular players and will provide key resources for developing cucumber cultivars resistant to pathogen stress.
Introduction
Cucumber (Cucumis sativus), a species of plant of the Cucurbitaceae family, is a key economic crop cultivated in protected horticultural areas, and it plays an indispensable role in the vegetable industry. However, with increasing planting density and continuous cropping, cucumbers are becoming more susceptible to various pathogens, especially powdery mildew (PM). PM is widely distributed, has a short incubation period, and is transmitted rapidly, leading to frequent epidemics and making PM an important global disease (Chen et al., 2021).
PM in cucumbers is mainly caused by Golovinomyces chicoracearum (formerly Erysiphe cichoracearum) and Podosphaera xanthii (formerly Sphaerotheca fuliginea), of which P. xanthii is more common (Gao et al., 2020). Both pathogens belong to the subphylum Ascomycota and are generally distinguished by the types of conidia and their germination methods (Ren, 2011). When the conidia contact a surface on the host, PM fungi adsorb to the host and penetrate into their stratum corneum and cell walls. Every PM fungus produces 1 or 2 germination tubes, which swell at the top to form an appressorium, which is the main organ mediating host invasion. PM fungi can also invade the cell walls of the host, mainly relying on enzymes and mechanical power. After invading the cell walls, the PM fungi continue to produce haustoria to invade the host cell membranes, to obtain nutrients, and then to enter the host cells themselves (Hückelhoven, 2005).
These PM pathogens are living vegetative fungi that are transmitted by air and that mainly infect cucumber leaves. When the fungi encounter cucumber leaves, they initially affect respiration and photosynthesis by forming a layer of white powder on the surface, invasiveness into the plant cells which results in the weakening of growth and even the death of the plant (Bi et al., 2016). Even this initial surface-level infestation, then, reduces yields and can cause substantial economic losses, critically impacting the cucumber industry. Hence, control of PM at its initial stages is particularly important. Understanding the process of infection by PM fungi in cucumbers and the molecular mechanisms of interaction between cucumbers and PM fungi can provide a scientific basis for controlling PM. These efforts will contribute to increasing cucumber performance and yield.
Cucumber plants have multiple mechanisms to resist fungal infections, and the interactions between cucumbers and PM fungi are strongly influenced by resistance genes. While previous studies have confirmed that cucumber PM resistance is controlled by multiple resistance loci, most of these studies have been limited to quantitative trait locus mapping (Shen, 2009; Yu, 2015; Wang et al., 2020; Liu et al., 2021). At present, only a few recessive resistance genes, mainly including Mildew Resistance Locus O (MLO) and NBS-LRR-type resistance gene families, have been successfully cloned and validated (Nie et al., 2015; Shi, 2016; Wang et al., 2021). Therefore, mining for additional PM resistance-related genes is of great significance for controlling the occurrence of PM and increasing cucumber yield.
In this study, RNA-sequencing (RNA-seq) analysis was performed on the cotyledons of a PM-resistant cucumber strain (B21-a-2-1-2) and a PM-susceptible cucumber strain (B21-a-2-2-2) that had been infected with P. xanthii, so as to identify differentially expressed genes (DEGs). We investigated biology processes and pathways that are enriched in these DEGs and that potentially contribute to PM resistance. Additionally, based on weighted gene co-expression correlation network analysis (WGCNA), 5 P. xanthii-inducible hub genes were detected. This study was designed to lay a theoretical foundation for further research on the regulatory mechanisms of cucumber PM resistance.
Materials and Methods
Plant Growth and Inocula
The cucumber tested in this study were the two sister lines, PM-resistant strain B21-a-2-1-2 and PM-susceptible strain B21-a-2-2-2, which are provided by Liaoning Academic of Agricultural Science. The two lines were selected from a segregated population originated from four generation selfing of a South Korea cultivar. They are different in resistance to PM, but alike in the plant type, commodity characteristics, tolerance to other stresses, and so on.
Cucumbers were cultivated in a 26°C greenhouse with 16 h light/8 h dark cycles. 9-day-old cotyledons of cucumber lines were inoculated with P. xanthii (105 conidia mL–1) by uniform spray as described previously (Ren, 2011). The cotyledons were harvested at seven time points [0, 6, 12, 24, 48, and 96 h post-inoculation (hpi) and 7 days post-inoculation (dpi)] and the euphylla were harvested 7 dpi. The tissues were quick-frozen in liquid nitrogen and stored at –80°C for later use.
Investigation of Coomassie Brilliant Blue Staining and Disease Index
Coomassie brilliant blue staining was used to detect the changes of P. xanthii in inoculated cucumber cotyledons. Firstly, cucumber cotyledons were soaked in decolorizing solution containing trichloroacetic acid, absolute ethanol and chloroform (0.225:150:50, w/v/v), and incubated at 70°C for more than 30 min until the leaves were white and transparent. Then, they were placed in staining solution containing trichloroacetic acid, Coomassie brilliant blue, methanol and ddH2O (0.225:0.9:150:150, w/w/v/v) for 5 min. The stained tissues were rinsed with distilled water to remove the dye liquor and observed under an optical microscope.
The disease index was determined to quantify the incidence of P. xanthii on inoculated cucumber cotyledons and euphylla. The disease index survey procedure and the grading criteria were used as described by Meng et al. (2018).
RNA Isolation and Sequencing
RNA was extracted according to the method described in the RNAprep Pure Plant Kit (Tiangen, Beijing, China). The purity and concentration of RNA were detected with a BioDrop μLite spectrophotometer (BioDrop in Cambridge CB4 OFJ England). The integrity of the RNA was assessed by 1% agarose gel electrophoresis. RNA samples extracted from B21-a-2-1-2 and B21-a-2-2-2 cucumber cotyledons harvested at 0 and 6 hpi were sequenced on the Illumina Hiseq platform (Personalgene, Nanjing, China). Equal volumes of the RNA from the three biological replicate samples at each time point were mixed prior to sequencing.
Clean data was obtained by filtering out the joints and low-quality reads of raw data. Clean data was mapped to the cucumber genome database1 via HISAT2 (an updated version of TopHat2).2 The mapping was considered successful when the mismatches between the default reads and the reference genome sequences were within 2. Htseq was used to calculate the read count value mapped to each gene, and this value was considered the original level of expression of the gene. The expression was then standardized as fragments per kilobase million (FPKM). DESeq was used for differential analysis of gene expression. The screening conditions of differentially expressed genes (DEGs) were | log2FoldChange| > 1 and p-value < 0.05.
Functional Annotation
For systematic analysis of gene functions, all DEGs were mapped according to the Gene Ontology (GO)3 and Kyoto Encyclopedia of Genes and Genomes (KEGG)4 internet utilities. Following GO and KEGG analysis, Mapman 3.6.0RC15 was used to trace the transcriptome changes regarding cucumber- P. xanthii interactions (Thimm et al., 2004). A p-value < 0.05 was considered as the threshold for significant enrichment.
Module Construction and Identification of Hub Genes
The WGCNA package 1.70-3 in R software 4.04 was used to screen for key modules related to cucumber defense against P. xanthii infection (Langfelder and Horvath, 2008). After removing the outliers (FPKM values > 0), 18,035 genes were selected for further analysis. We set a weighted correlation threshold of > 0.85, the optimal power at 22, the minimal module size at 30 and the branch merge cut height at 0.25. Other parameters were maintained at their default settings. For exploring the key module gene functions, GO and KEGG enrichment analyses were also constructed. Subsequently, hub genes were identified on the basis of Module Membership (MM) > 0.98 and edge weight value > 0.5.
qRT-PCR Validation
To verify the reproducibility of RNA-seq results, we selected 16 DEGs to analyze by qRT-PCR. RNA extraction and quality detection were carried out as described above. The first strand of cDNA was synthesized with the FastQuant RT Kit (Tiangen, Beijing, China). A LightCycler 480 (Roche Molecular Systems, CA, United States) was used to perform qRT-PCR using the SuperReal PreMix Plus Kit (Tiangen, Beijing, China). CsActin was used as an internal reference to normalize the data. The relative expression levels of genes were computed with the 2–ΔΔCt quantitative analysis method. The primers used in this validation are shown in Supplementary Table 1.
Accession Code
All raw sequencing reads were deposited to the NCBI Sequence Read Archive under the project ID PRJNA816625.
Results
Podosphaera xanthii Inoculation and Plant Responses
We inoculated the cotyledons and euphylla of PM-resistant (B21-a-2-1-2) and PM-susceptible (B21-a-2-2-2) cucumbers with P. xanthii to test varieties in phenotypes and lesions. At 7 d after inoculation, the P. xanthii infection on B21-a-2-2-2 leaves was clearly more serious than on B21-a-2-1-2, and investigation using the disease index (DI) yielded results that were compatible with this conclusion (Supplementary Figure 1). Furthermore, we also detected the expression level of P. xanthii by qRT-PCR and found that its expression level in B21-a-2-1-2 was remarkably lower than that in B21-a-2-2-2. These results supported the reliability of the experimental materials.
We also inoculated the cotyledons of B21-a-2-1-2 and B21-a-2-2-2 cucumbers with P. xanthii, and carried out Coomassie brilliant blue staining at 0, 6, 12, 24, 48, and 96 hpi to observe the process of P. xanthii infection. As shown in Figure 1, for B21-a-2-2-2 cucumbers, the primary germ tube (PGT), appressorium (App), penetration peg (Pp), fungal colony (FC), conidiophore (Cdp) were successively observed at 6, 12, 24, 48, and 96 hpi, and spores were found to be able to complete their asexual growth cycle.
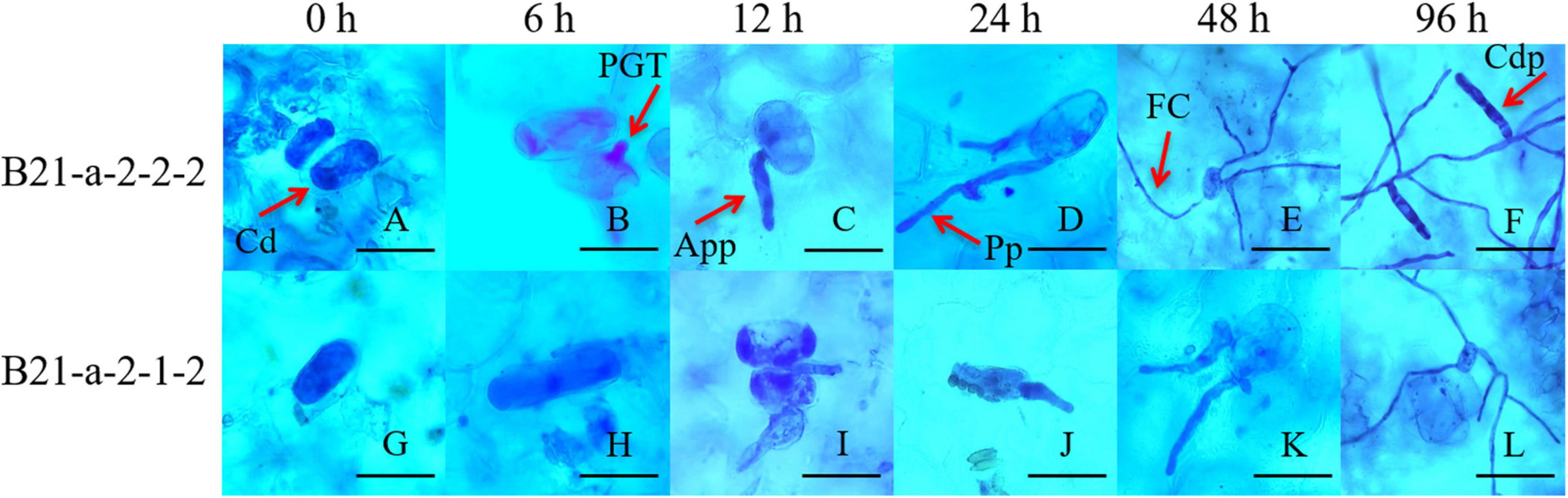
Figure 1. Process of infection of P. xanthii of the PM-susceptible cucumber strain B21-a-2-2-2 and the PM-resistant cucumber strain B21-a-2-1-2. Cd, conidia; PGT, primary germ tube; App, appressorium; Pp, penetration peg; FC, fungal colony; Cdp, conidiophore. The bar in panels A through D and G through K is 20 μm, and the bar in panels E through F and L is 60 μm.
However, for B21-a-2-1-2 cucumbers, only spores could be observed at 6 hpi, and spores began to germinate and to form a small amount of PGT at 12 hpi. A few App were formed, and most of the spores remained in the PGT stage at 24 hpi. Most of the spores stopped germinating and remained in the App stage, and only a few spores formed Pp at 48 hpi. FC was formed at 96 hpi, but Cdp was not seen in the visual field. Together, these observations indicated that the spores of P. xanthii could not complete their asexual growth cycle in the resistant strain B21-a-2-1-2. These results showed that the process of infection of P. xanthii in the B21-a-2-1-2 strain showed a substantial lag compared with the B21-a-2-2-2 strain, and the difference in the process was apparent by 6 hpi. Therefore, we used 0 and 6 hpi samples for the following studies.
RNA-Seq Analysis and Mapping to the Cucumis sativus Reference Genome
To characterize the transcriptional response of cucumbers to P. xanthii inoculation, high-throughput sequencing was performed using RNA isolated from resistant (B21-a-2-1-2) and susceptible (B21-a-2-2-2) tissues at 0 and 6 hpi. Three biological replicates were performed for each sample. A total of 41.6–66.0 million raw reads were yielded from the 12 transcriptome libraries (Table 1). After trimming of adapter and low-quality sequences, the numbers of clean reads ranged from 39.8 to 66.0 million, and the proportions of clean reads of samples (Q30) were 93.9–94.5%. The correlation coefficients of the expression levels of each sample are shown in Supplementary Figure 2, and principal components analysis is exhibited in Supplementary Figure 3 and Supplementary Table 2. These parameters indicated that the sequences used in the following analyses were of high quality. Of the clean reads, 95.2–96.1% were uniquely aligned to the C. sativus Gy14 genome v2.
Using the criteria of | log2 FC| > 1 and q-value < 0.05, we obtained a total of 4,099 DEGs by comparing RNA expression in the susceptible to the resistant strains at 0 hpi (S0h vs. R0h), the susceptible and resistant strains at 6 hpi (S6h vs. R6h), the resistant strain at 0 hpi to the resistant strain at 6 hpi (R0h vs. R6h), and the sensitive strain at 0 hpi to the sensitive strain at 6 hpi (S0h vs. S6h) (Figure 2 and Supplementary Figure 4). In total, 568 DEGs were identified in the S0h vs. R0h comparison, of which 236 were up-regulated and 332 were down-regulated (Supplementary Table 3). Upon P. xanthii inoculation, 472 DEGs (193 up-regulated and 279 down-regulated) were identified in the susceptible line (Supplementary Table 4), while 2,473 DEGs (1,242 up-regulated and 1,231 down-regulated) were identified in the resistant line (Supplementary Table 5). Among these DEGs, 313 transcripts were shared between both lines, while 2160 and 159 genes were specifically expressed in the resistant and susceptible line, respectively (Figure 2B). These data indicated that the resistant line mobilized more unique responses to fight against P. xanthii.
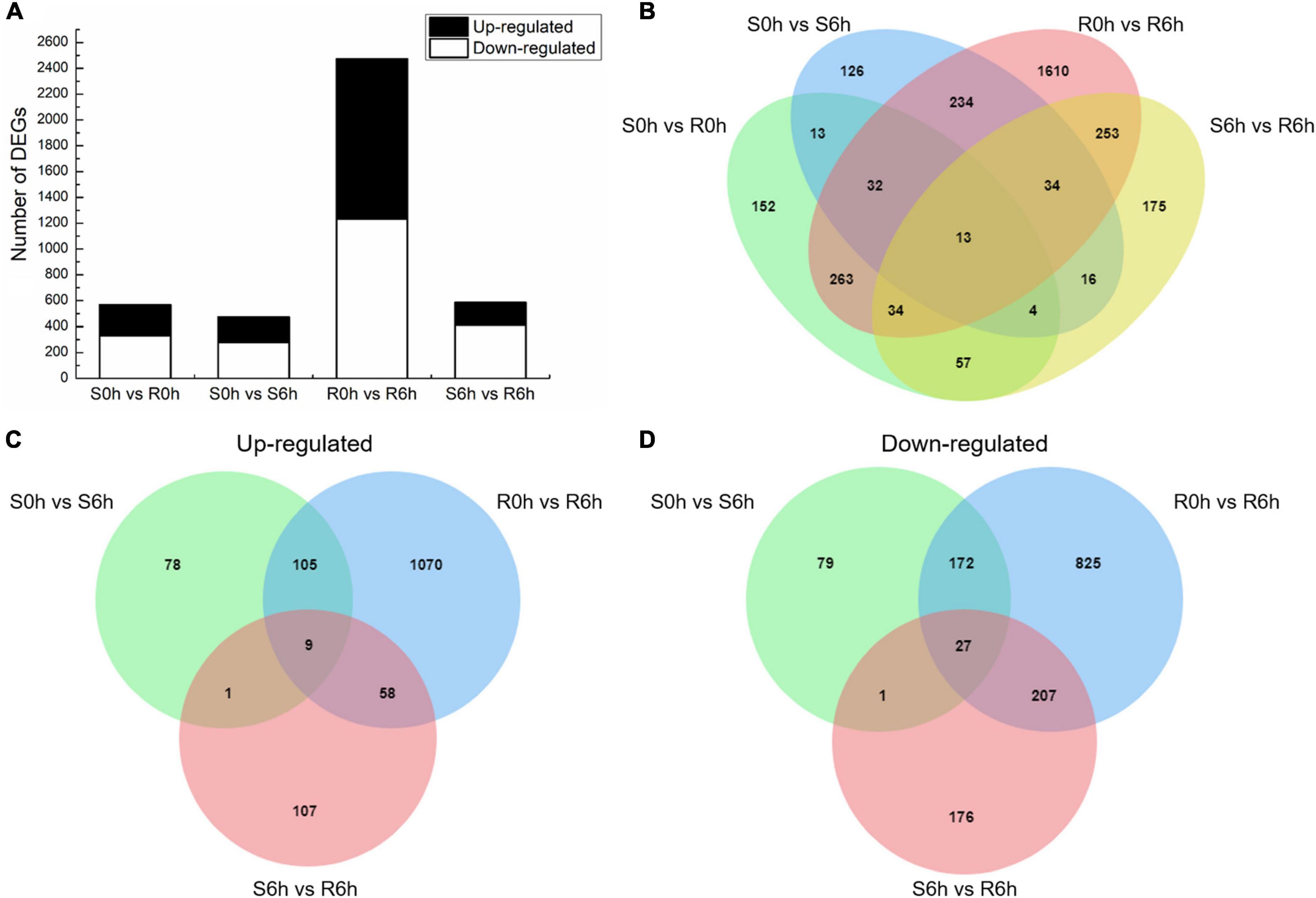
Figure 2. DEGs between the PM-susceptible strain B21-a-2-2-2 (S) and PM-resistant strain B21-a-2-1-2 (R) of cucumber in response to P. xanthii. (A) Numbers of DEGs obtained in each comparison. (B) Overlap of DEGs in each comparison. (C) Venn diagram of genes up-regulated in S0h vs. S6h, R0h vs. R6h, and S6h vs. R6h comparisons. (D) Venn diagram of genes down-regulated in S0h vs. S6h, R0h vs. R6h, and S6h vs. R6h comparisons.
By comparing S0h vs. S6h, R0h vs. R6h and S6h vs. R6h, 36 transcripts were identified as the same expression trend DEGs, of which 9 were up-regulated and 27 were down-regulated (Figures 2C,D). The up-regulated transcripts included E3 ubiquitin-protein ligase (RNF115), caffeoyl shikimate esterase (CSE), peroxidase 2-like (POX 2-like), glycine-rich cell wall structural protein 1.8 (GRP 1.8), calcium-dependent protein kinase 8-like (CDPK 8-like), major pollen allergen Ole e 6, and beta-amylase, and two genes encoding uncharacterized protein (Supplementary Table 6). Among these, CSE and POX both code for key enzymes in the lignin biosynthesis pathway, and GRP codes for a plant cell wall structural protein (Mceldoon et al., 2012; Vanholme et al., 2013; Zhao et al., 2020). Lignin is component of the plant secondary cell wall, therefore indicating that resistant plants perform more cell wall component alterations.
Functional Category Enrichment of Differentially Expressed Genes
GO, KEGG and Mapman analyses were performed to capture key biology processes or pathways involved in defense against P. xanthii in the resistant and susceptible cucumber. Through analysis of GO terms, 343 and 1,886 DEGs were functionally annotated in S0h vs. S6h and R0h vs. R6h, respectively (Figure 3 and Supplementary Figures 5–10). Biology processes such as regulation of RNA metabolic and biosynthetic process, transcription and nucleobase-containing compound metabolic process were obviously enriched in S0h vs. S6h. In R0h vs. R6h, carbohydrate metabolic process was the largest biology process gene ontology. P. xanthii clearly affected cell extracellular region, especially the cell wall, in both lines, as evidenced by GO terms in the cellular component category. Additionally, GO terms in molecular function for each comparison were quite different. Transcription regulator activity, cation binding and metal ion binding are the three most enriched GO terms in S0h vs. S6h, while catalytic activity comprised the majority of terms in R0h vs. R6h.
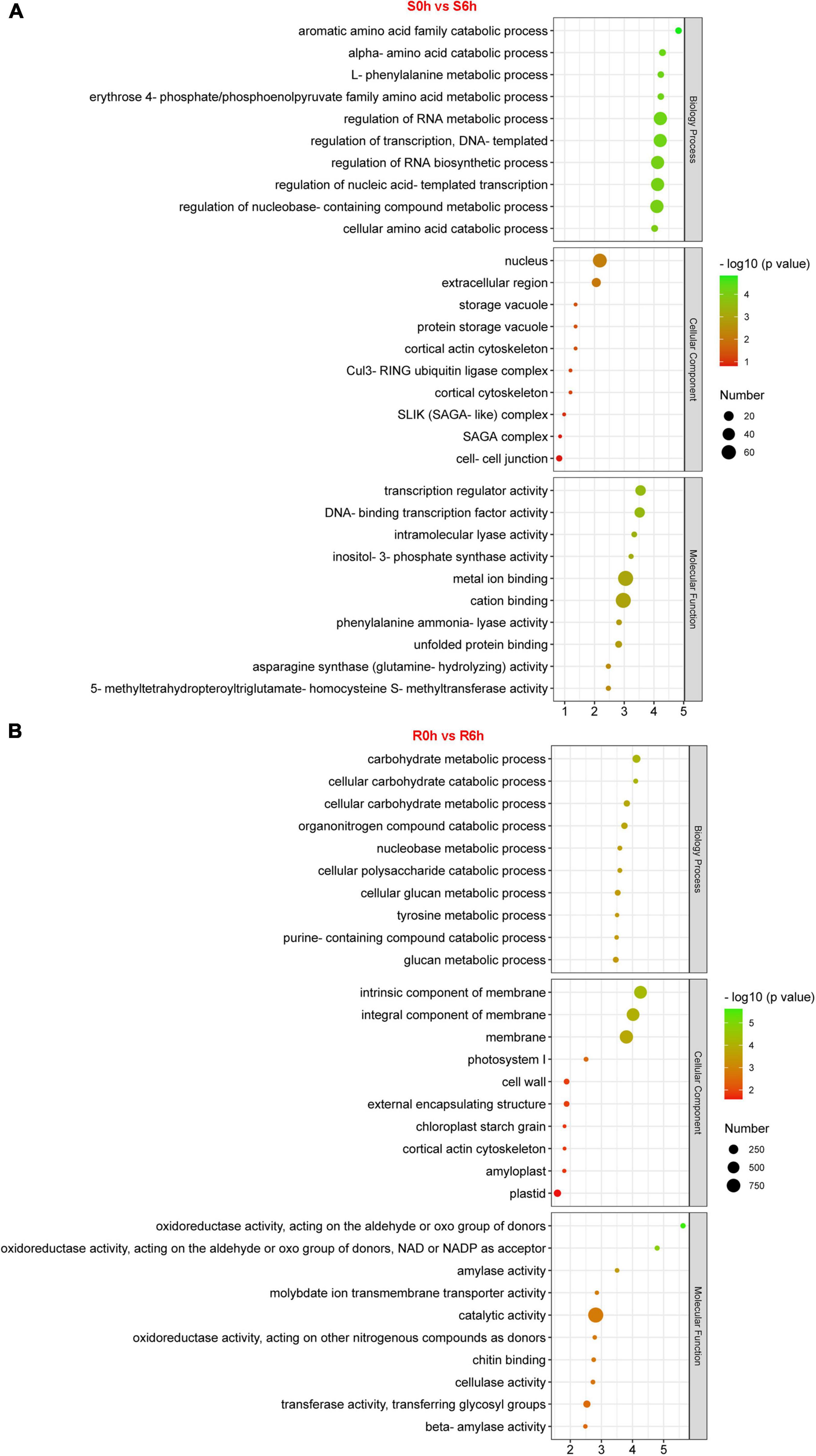
Figure 3. GO enrichment analysis of DEGs in PM-susceptible cucumber B21-a-2-2-2 (A) and PM-resistant cucumber B21-a-2-1-2 (B) subjected to P. xanthii. The results from the biological process, cellular component and molecular function categories are summarized.
Considering KEGG classifications, the P. xanthii-resistance mechanism of the resistant line was different from that of susceptible line. In S0h vs. S6h, pathways such as plant hormone signal transduction, protein processing in endoplasmic reticulum, phenylpropanoid biosynthesis and plant-pathogen interaction were significantly enriched. In R0h vs. R6h, the function classes of plant hormone signal transduction, starch and sucrose metabolism, MAPK signaling pathway and plant-pathogen interaction were obviously enriched (Figure 4).
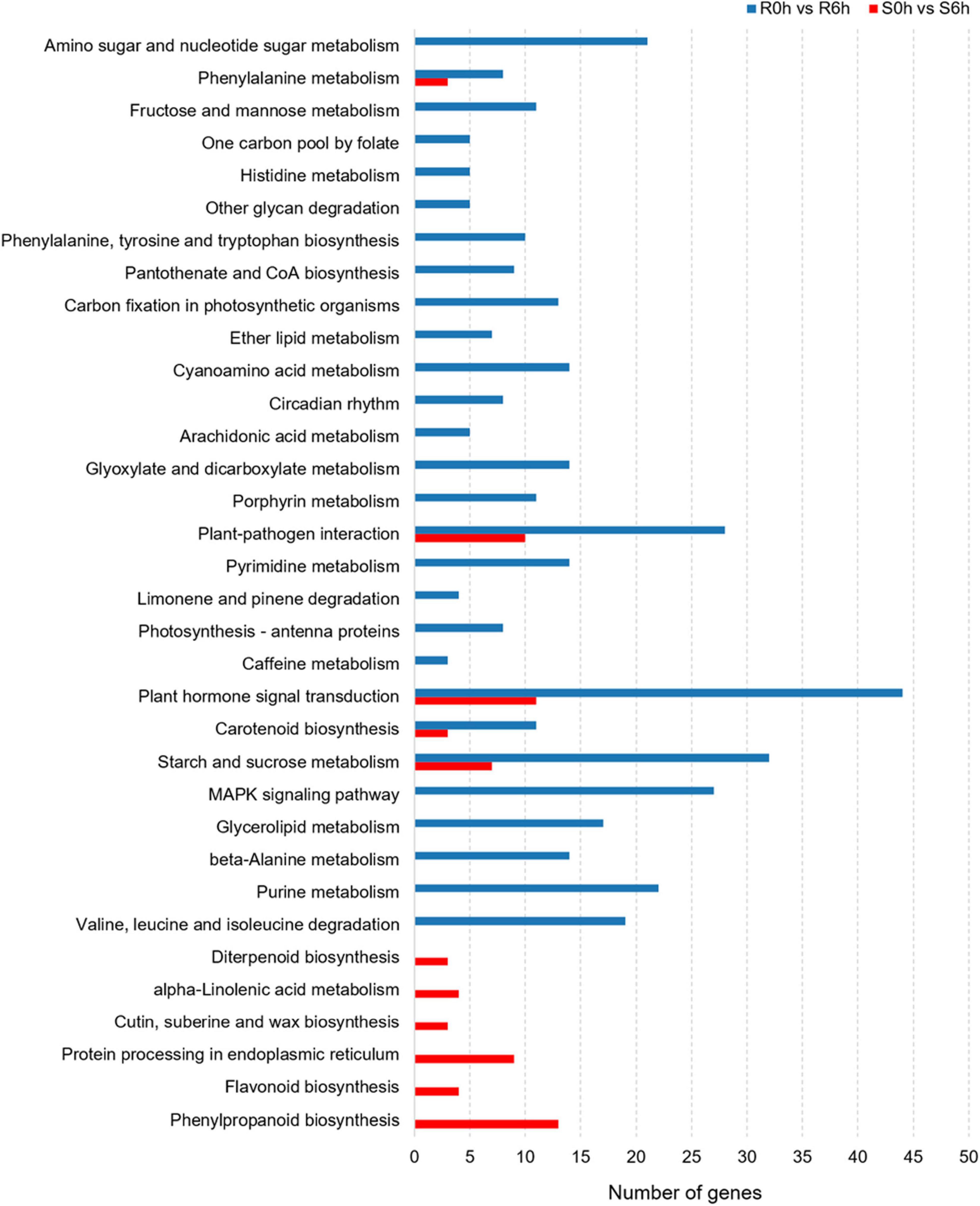
Figure 4. KEGG pathway analysis of DEGs in PM-susceptible cucumber strain B21-a-2-2-2 and PM-resistant cucumber strain B21-a-2-1-2 subjected to infection with P. xanthii.
By using Mapman analysis, we found a number of pathways involved in the regulation of defense against P. xanthii in the resistant cucumber that were absent in the susceptible line (Figure 5). Most defense-related DEGs were up-regulated in R0h vs. R6h, while these DEGs were totally absent in S0h vs. S6h. DEGs related to salicylic acid (SA) signaling, MAPK signaling and gene transcription regulated by Dof and WRKY transcription factors were detected in R0h vs. R6h, but not present in S0h vs. S6h, revealing that these pathways likely affect cucumber resistance to P. xanthii. In addition, the resistant cucumber also exhibited obvious changes to transcripts related to cell wall, proteolysis, signaling and secondary metabolites. These pathways have been reported to be involved in responses to abiotic and biotic stresses in plants. Taken together, DEGs related to cell wall component, signaling and gene transcription regulation are collectively involved in regulating the defense response in cucumber, resulting in different degrees of resistance in different plant materials.
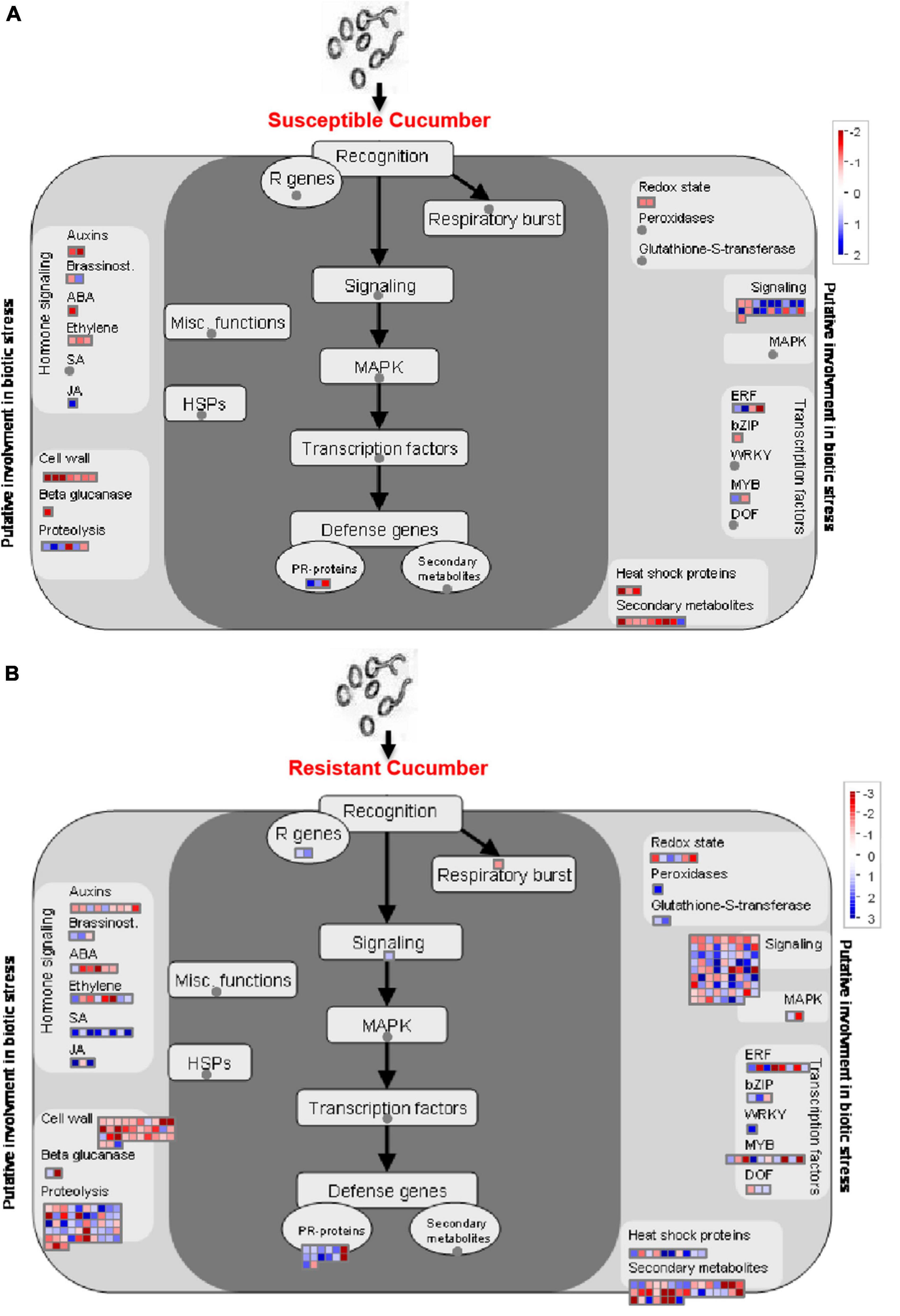
Figure 5. Mapman analysis of DEGs in PM-susceptible cucumber strain B21-a-2-2-2 (A) and PM-susceptible cucumber strain B21-a-2-2-2 (B) subjected to infection with P. xanthii. The blue and red dots represent up- and down-regulated genes.
Screening for Key Modules Related to Podosphaera xanthii Defense in Cucumber
To capture cohorts of genes associated with cucumber defenses against P. xanthii, a total of 18,035 genes were selected to conduct WGCNA after filtration of the outliers. By using the WGCNA platform, we obtained 15 different modules (decorated with different colors) based on similarities of expression patterns (Figure 6A). Among them, the largest module (turquoise) comprised 8,007 genes, whereas the smallest module (cyan) comprised only 66 genes (Figure 6B). These modules were clustered into two clades according to module eigengenes (Figure 6C). The correlation coefficients between each sample and module eigengenes ranged from –0.78 to 0.78 (Figure 6D). It is worth noting that the turquoise module was obviously positive in the resistant strain at 6 hpi (r = 0.78, p-value = 0.003) and negative in the susceptible strain at 6 hpi (r = –0.64, p-value = 0.02); the blue module was positive in the susceptible strain at 6 hpi (r = 0.71, p-value = 0.01), while the salmon module was negative in the susceptible strain at 6 hpi (r = –0.68, p-value = 0.01); the red module was negative in the resistant strain at 0 hpi (r = –0.78, p-value = 0.003) and positive in the susceptible strain at 6 hpi (r = 0.68, p-value = 0.02) (Figure 6C). These data indicated that the turquoise module was most closely correlated with P. xanthii resistance.
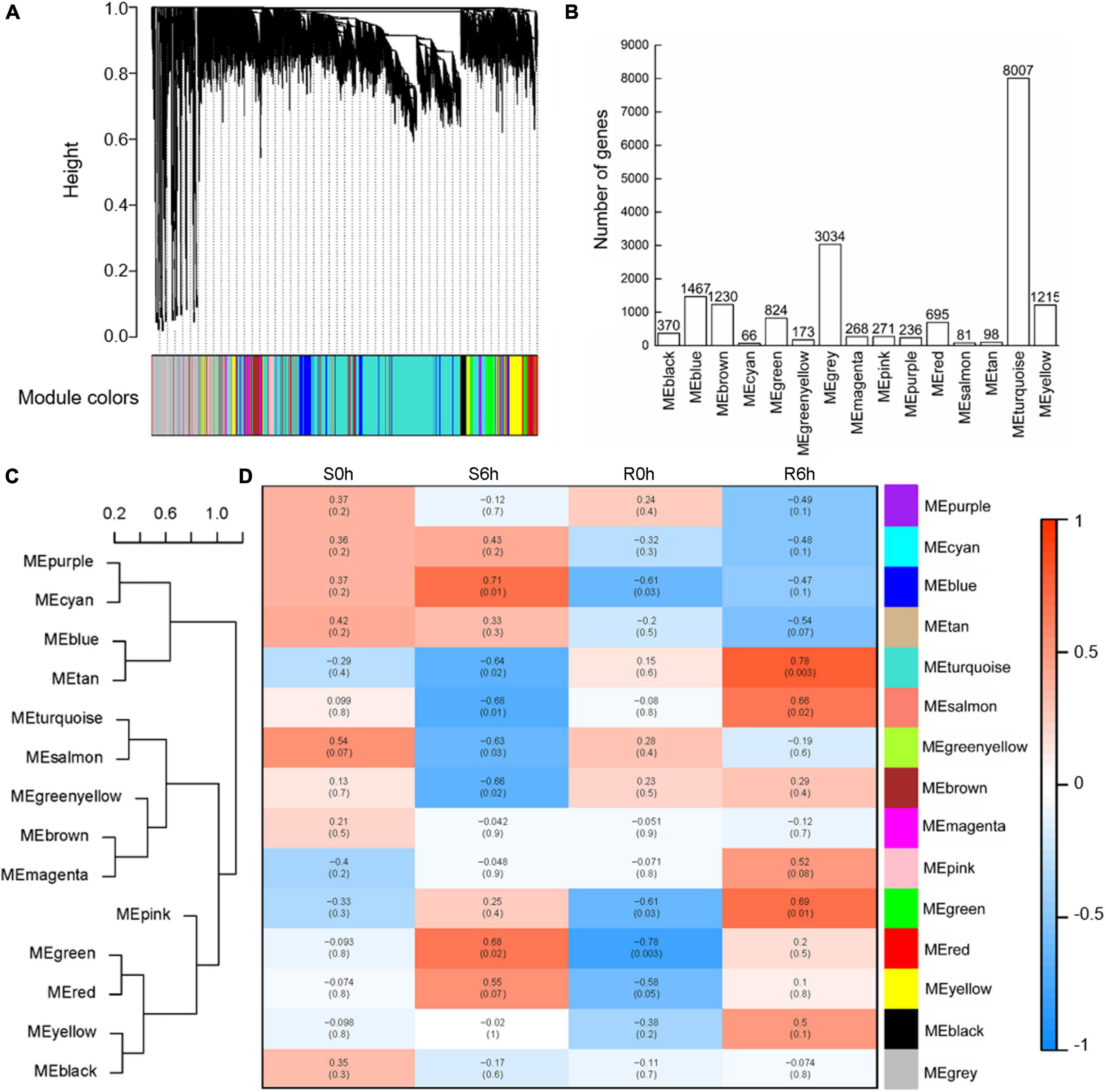
Figure 6. WGCNA analysis of key modules related to P. xanthii defense in cucumber. (A) Clustering dendrogram of co-expression modules. (B) The number of genes detected in each module. (C) Heat maps of gene expression in each module. Cluster tree (C) and heat maps (D) of gene expression in each module.
Upon GO enrichment analysis, 564 significantly enriched GO terms were identified in the turquoise module, with 401 of them in biological process, 72 in cellular component and 91 in molecular function. The most prevalent biological process function GO terms were catabolic process (417 genes), organic substance catabolic process (379 genes) and cellular catabolic process (362 genes). Of the cellular component group, catalytic complex (277 genes), nucleoplasm (231 genes) and transferase complex (172 genes) represented the three largest GO terms. Under the classification of molecular function group, hydrolase activity (379 genes), nuclease activity (246 genes) and endonuclease activity (216 genes) were the mostly abundant (Figure 7A). Based on KEGG pathway analysis, the turquoise module was found to mainly participate in microbial metabolism in diverse environments, carbon metabolism, biosynthesis of amino acids, spliceosome and purine metabolism (Figure 7B).
Identification of Hub Genes Within Key Modules
In order to find hub genes in the response to P. xanthii stress, we constructed a gene correlation network from the turquoise module. Based on the criteria of MM > 0.98 and edge weight value > 0.5, 32 genes were regarded as hub genes (Table 2). Of the 32 hub genes, 5 genes were found to participate in responses to stimuli. These genes included CsGy7G018660 (glycerol kinase, GLPK), CsGy5G023580 (integrin-linked protein kinase 1, ILK1), CsGy1G028370 (2-oxoisovalerate dehydrogenase subunit beta 1, BCDHβ1), CsGy6G023850 (ethylene-insensitive protein 2, EIN2), and CsGy6G008390 (RGG repeats nuclear RNA binding protein A, RGGA).
Four genes were found to participate in transcriptional regulation, including CsGy1G020430 (transcription factor bHLH35), CsGy3G002200 (GATA transcription factor 26), CsGy3G030790 (DNA-binding protein S1FA), and CsGy6G034530 (transcription factor VOZ1). Four genes were found to participate in molecular transport, including CsGy4G008900 (D-xylose-proton symporter-like 2, sugar transporter), CsGy4G007480 (cationic amino acid transporter 8, amino acid transporter), CsGy5G012190 (protein NRT1/PTR FAMILY 8.3, peptide, high affinity, low capacity, and histidine transporter), and CsGy7G020980 (probable phospholipid-transporting ATPase 4, phospholipids transporter). Two of the hub genes were found to participate in lipid metabolic process, including CsGy2G008200 (phospholipase A(1) LCAT3) and CsGy3G026520 (GDSL esterase/lipase 5). In addition, CsGy3G008770 (ubiquitin-conjugating enzyme E2) was annotated to participate in protein ubiquitination and CsGy7G011040 (calcium-dependent protein kinase 11, CDPK11) in abscisic acid (ABA) signaling pathways. Function annotation thus demonstrated that these hub genes were mainly related to defense responses, transcriptional regulation, molecular transport and lipid metabolic process.
Validation of Gene Expression Profiles by qRT-PCR
To validate the reliability of the RNA-seq data and to analyze the expression levels of stress-responsive genes, 16 DEGs were selected for qRT-PCR assays. We conducted experiments at six time points (0, 6, 12, 24, 48, and 96 hpi) using the B21-a-2-1-2 and B21-a-2-2-2 strains (Figure 8). Most DEGs expression levels were consistent with the RNA-seq results, indicating the reproducibility of the data. Notably, the expression level of more DEGs underwent a striking change in B21-a-2-1-2 than in B21-a-2-2-2 in the early stages of infection.
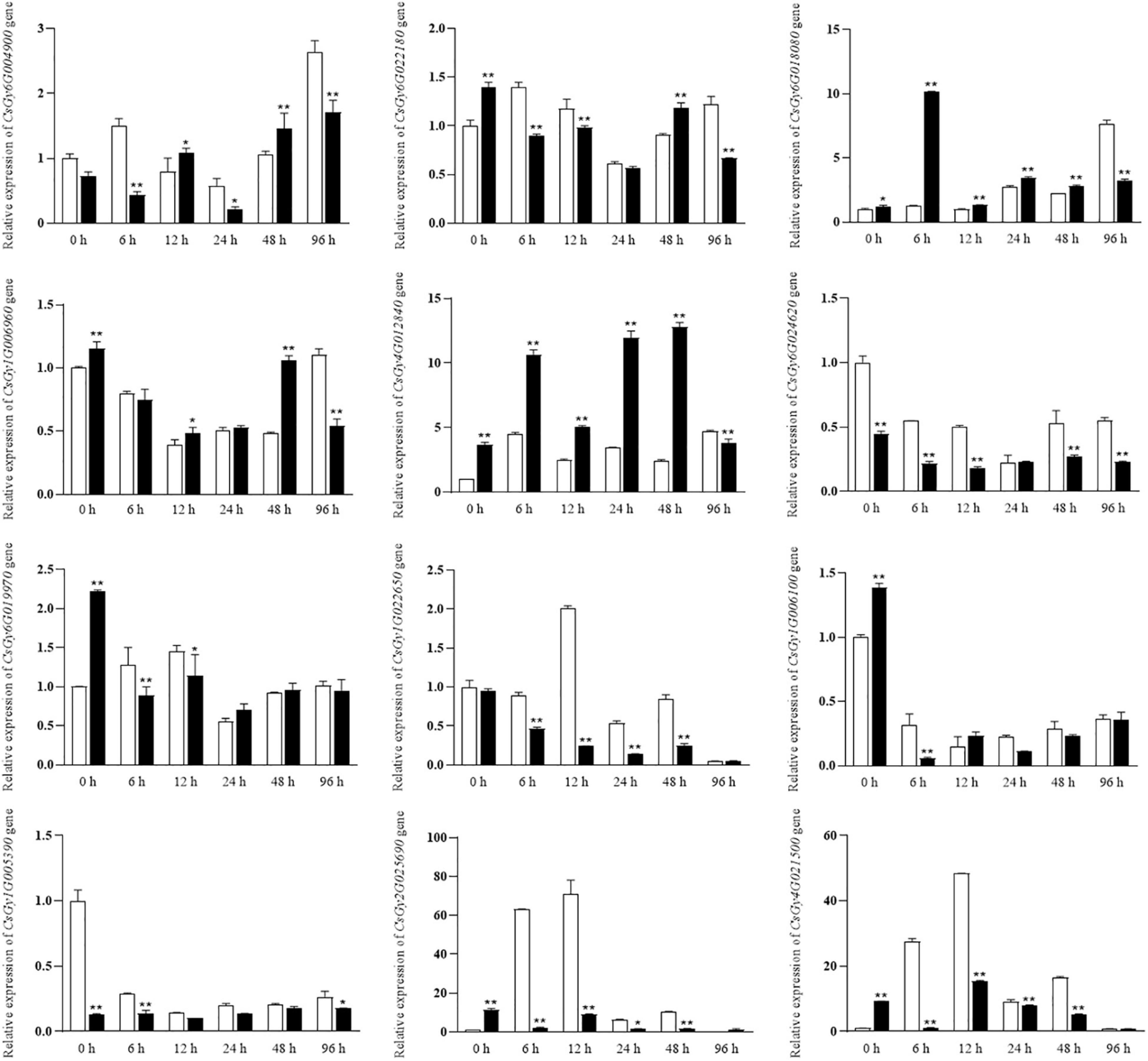
Figure 8. Expression analysis of select DEGs at 0, 6, 12, 24, 48, and 96 hpi using the 2– ΔΔCt method. Data are means ± SD of three biological replicates per variety. Significance was determined by Duncan’s multiple range test, and is represented by *P ≤ 0.05 and **P ≤ 0.01.
Discussion
This study was designed to capture key genome-related parameters related to the resistance of cucumber to P. xanthii. P. xanthii does not infect cucumber fruit directly. Instead, they weaken growth and impact fruit yield by invading cucumber leaves, reducing photosynthesis and increasing respiration and transpiration. Hence, control at the initial stages of infection is clearly optimal in order to reduce yield loss. Therefore, we selected 6 hpi for RNA-seq analysis, because that is a critical point when spores germinate on resistant and susceptible cucumber lines. In this study, the resistant line had comparatively more DEGs than did the susceptible line under P. xanthii invasion. This finding was similar to those found in previous transcriptomic studies in sorghum, sugarcane and wheat, in which resistant lines induced expression of more DEGs than did susceptible lines under biotic stress (Kiani and Szczepaniec, 2018; De Mello et al., 2020; Kiani et al., 2021).
Plants have evolved complex defense mechanisms to ward off invading pathogens. The cell wall, which covers the outermost surfaces of the plant, offers a frontline barrier against pathogen invasion. The plant cell wall is a complex and dynamic network that mainly consists of phenolic compounds (lignin), matrix polysaccharides (hemi-cellulose and pectin), structural polysaccharides (cellulose) and protein (Sandhu et al., 2009). Numerous studies have focused on ways in which the cell wall differs in resistant and susceptible plant hosts (Meng et al., 2016; Otulak-Kozieł et al., 2020; Kozieł et al., 2021). In our dataset, cell wall-related genes, such as CSL (cellulose synthase, CsGy6G024620, and CsGy2G018180), FLA (fasciclin-like arabinogalactan protein, CsGy7G007790), GH (glycosyl hydrolase, CsGy6G021790), EXP (expansin, CsGy5G023420, CsGy4G014170, and CsGy5G028430), PGX (polygalacturonase involved in expansion, CsGy2G017040, and CsGy5G022100), XTH (xyloglucan endotransglucosylase/hydrolase, CsGy6G022130, CsGy3G038470, CsGy1G019960, and CsGy1G019970), PE (pectinesterase, CsGy1G026850, CsGy2G012280, CsGy3G000030, CsGy3G026590, and CsGy4G005120), PAE (pectin acetylesterase, CsGy1G025090), AP (aspartyl protease, CsGy5G027100) and BGAL (beta-galactosidase, CsGy6G030970) tended to be down-regulated in the resistant line under P. xanthii stress.
It would seem reasonable to predict that cell wall-related genes confer resistance by augmenting wall strength; conversely, our data suggest that down-regulation of these cell wall-related genes confers resistance against P. xanthii in cucumber, perhaps owing to an activation of the plant immune response. This suggestion is in line with a previously published test of cell wall mutants in Arabidopsis, in which 81.6% of cell wall mutants were found to enhance resistance to necrotrophic and vascular pathogens (Molina et al., 2021). Lignin has been previously reported to provide a protective barrier upon infection and resistance against pathogens (Miedes et al., 2014; Wang X. et al., 2018). Infection by P. xanthii also markedly induced expression of lignin biosynthetic genes CSE (CsGy1G010260) and POX (CsGy4G012840) in the resistant line. Hence, these cell wall-related genes may either negatively or positively impact the resistance against pathogen infection, depending on the specific defense mechanism activated.
Plant hormone-mediated signaling pathways play an important role in plant defense responses to biotic stress. For example, JA- and ET- mediated signaling pathways contribute to plant resistance to necrotrophic pathogens, while SA-mediated signaling pathway is involved in plant resistance to biotrophic pathogens (Chen et al., 2010). Indeed, application of exogenous SA can induce cucumber resistance to P. xanthii (Mo, 2005). In our experiment, two salicylic acid glucosyltransferase 1 genes (SGT1, CsGy3G025850, and CsGy3G025860) were detected up-expressed only in the resistant cucumber in response to P. xanthii attack. SGT also known as uridine diphosphate (UDP)-glucosyltransferase (UGT), which converts SA into SA 2-O-β-D-glucoside (SAG) and the glucose ester of SA (SGE) (Vlot et al., 2009; Kobayashi et al., 2020). Several studies expanded the scope of functioning of SGT1 in plants to include pathogen resistance and mediation of SA production (Song et al., 2007; Hu et al., 2021). The SGT1 gene identified here may therefore serve as crucial elements for the bioengineering of enhanced disease resistance.
Plant innate immunity mainly consists of two layers of defense, pathogen-associated molecular pattern-triggered immunity (PTI) and effector-triggered immunity (ETI), which help host to fight against pathogens during early attack. Mitogen-activated protein kinase (MAPK) cascades play vital roles in both PTI and ETI signaling pathways (Thomma et al., 2011; Meng and Zhang, 2013; Bigeard et al., 2015). The canonical MAPK cascade consists of MAPKs (MPKs), MAPK kinases (MAPKKs/MAP2Ks/MKKs/MEKs), and MAPK kinase kinases (MAPKKKs/MAP3Ks/MEKKs) (Zhang et al., 2006). The Arabidopsis genome contains approximately 20 MAPKs, 10 MAPKKs, and 80 MAPKKKs (Colcombet and Hirt, 2008). Of these, MEKK1-MKK4/5-MPK3/6, MAPKKK3/5-MKK4/5-MAPK3/6, and MEKK1-MKK1/2-MPK4 are generally reported to confer resistance against pathogens (Qiu et al., 2008; Galletti et al., 2011; Xu et al., 2016; Su et al., 2017; Sun et al., 2018). In cucumber, there have been 14 MAPK, 6 MAPKK, and 59 MAPKKK genes identified based on sequence (Wang et al., 2015). To date, only TIPK (a homolog of Arabidopsis MPK3) was been discovered to participate in the defense of cucumber against pathogens (Shoresh et al., 2006). In our study, MPK9 (CsGy1G006960), and MPK20 (CsGy6G022180) were found to be up-regulated in the resistant line, with no changes observed in the susceptible strain upon inoculation with P. xanthii. MPK9 plays a pivotal role in regulating ABA and methyl jasmonate (MeJA) signaling pathways in Arabidopsis guard cells (Jammes et al., 2009; Khokon et al., 2015). ABA and MeJA are important defense signals that impart resistance against pathogen attack (Niu et al., 2010; Cao et al., 2011), so the altered expression of these genes suggests that MPK9 might also work in response to pathogen infection. An MPK20 homolog found in cucumber was previously reported to participate in the auxin signaling pathway and primary cell wall formation (Persson et al., 2005; Lalonde et al., 2008). In addition, this gene has been demonstrated to mediate resistance to Fusarium oxysporum in cotton (Wang C. et al., 2018).
Likewise, many genes related to MAPK cascades, such as VIP1 (CsGy6G004900), CAT3 (CsGy6G018080), and PYL4 (CsGy4G015470 and CsGy5G008430) were induced in the resistant cucumber strain during P. xanthii attack. VIP1, which encodes a bZip transcription factor, has been identified as the downstream target of MPK3 in Arabidopsis (Djamei et al., 2007). Under pathogen stress, when phosphorylated by MPK3, the VIP1 protein directly induces the MYB44 stress-response gene (Pitzschke et al., 2009). CAT (catalase) enzymes are H2O2 scavengers that maintains ROS homeostasis in various stress responses (Du et al., 2008). Arabidopsis ABA-induced CAT3 expression has been shown to be impaired in a MEK1 mutant (Xing et al., 2007). PYL, which encodes a core component of the ABA signaling pathway, has previously been reported to activate a MAPK cascade (Danquah et al., 2015). PYL mediates multiple biological processes, including leaf senescence, lateral root growth, dormancy, and stress responses (Zhao et al., 2014, 2016; Lee et al., 2015; Du et al., 2017). Alteration of the expression of these genes in the cucumber plant, therefore, may be important molecular events in the defense against P. xanthii.
After activation of defense mechanisms, plants transfer a series of signals to transcription factors (TFs). Then, TF activate or repress the expression of target genes to resist pathogen damage. Our Mapman analysis revealed that the Dof and WRKY TFs are affected by P. xanthii in the resistant cucumber but that these TFs were absent in the susceptible line. Dof transcription factors are unique to plants, with 36 members present in cucumber (Wen et al., 2016). Multiple studies have reported participation of Dofs in pathogen resistance pathways via the mediating of the expression of target resistance genes, including Sar8.2b, ACBP3, and cystatin (Song and Goodman, 2002; Martinez et al., 2005; Zheng et al., 2012). In grape, Dof3 has been described to be positively related to PM resistance (Yu et al., 2019). The up-regulation of Dofs was also documented when cucumber plants were exposed to the pathogen Pseudoperonospora cubensis. Thus, Dofs tend to be regarded as positive regulators in plant defenses against biotic stresses. Here, we found 3 Dof-coding genes (CsGy5G000240 and CsGy6G034890) to be weakly up-regulated and 3 (CsGy3G036390, CsGy6G014450, and CsGy1G005390) significantly down-regulated at 6 hpi in the resistant cucumber. Hence, the link between Dofs and host defense responses needs to be further examined.
The other relevant type of TF, the WRKY, forms a large family that is well-characterized in plants (Ulker and Somssich, 2004). Numerous studies have proposed a crucial role of WRKY in plant defense (Eulgem and Somssich, 2007; Amorim et al., 2017; Wani et al., 2021). Examples of WRKY that play roles in pathogen resistance response include Triticum aestivum WRKY19 in the defense against Puccinia striiformis, Fragaria × ananassa WRKY50 in the defense against Botrytis cinerea, Oryza sativa WRKY6 in the defense against Xanthomonas oryzae, and Hordeum vulgare WRKY6 in the defense against Pyrenophora teres (Ma et al., 2021; Tamang et al., 2021; Im et al., 2022; Nobori, 2022). Cucumber contains 61 WRKY that are classified into 3 main groups (I, II, and III) (Chen et al., 2020). Our data showed that the resistant cucumber exposed to P. xanthii stress obviously up-regulated the expression of WRKY31 (CsGy7G003250). Cucumber WRKY31 belongs to Group IIb gene that showed orthologous relationship with Arabidopsis WRKY6. The protein coded by WRKY6 was reported to participate in pathogen defense by modulating pathogen defense-associated PR1 promoter activity (Robatzek and Somssich, 2002).
Stress-responsive genes have also been found to have indispensable roles in plant defenses. According to our WGCNA analysis, GLPK, ILK1, EIN2, BCDHβ1, and RGGA were found to be highly associated with the P. xanthii stress response. Glycerol kinase phosphorylates glycerol to glycerol-3-phosphate (G3P), which is well known as a critical regulator of plant systemic immunity (Chanda et al., 2011). An Arabidopsis glycerol kinase gene (GLI1) was found to act as a positive modulator of defense toward bacterial and fungal diseases (Kang et al., 2003; Yang et al., 2013). In addition, GLI1 was found to be induced following PM infections in Triticum aestivum (Li et al., 2020). Similarly, we found that the cucumber orthologs of GLI1 was up-regulated in the resistant strain after P. xanthii treatment. ILK1 encodes a Raf-like MAPKKKs, as it is the major regulator of cell proliferation growth and immune responses in animals (Hannigan et al., 2011). In terms of molecular function in plants, ILK1 has been identified to control pathogen resistance (Brauer et al., 2016). EIN2 is also essential for plant immune response, as ein2 mutants confer sensitivity toward the pathogen fungus (Thomma et al., 1999; Sun et al., 2017). Information regarding RGGA and BCDH β1 have linked these genes to tolerance of osmotic and nutrient stresses, respectively (Ambrosone et al., 2015). However, there has been little work on these two genes in the context of plant defense against biotic stresses.
To summarize, this paper showcases key molecular players in plant defense against fungal infection by comparing physiological and gene expression responses in the PM-resistant cucumber strain B21-a-2-1-2 to those of the PM-susceptible strain B21-a-2-2-2 in early stages of infection with P. xanthii. After 6 h of P. xanthii stress, 472 DEGs (193 up-regulated and 279 down-regulated) were identified in the susceptible line, while 2,473 DEGs (1,242 up-regulated and 1,231 down-regulated) were identified in the resistant line, indicating that the resistant line can rapidly instigate resistance events to fight against pathogen attack. These DEGs were grouped by their functional annotation through GO, KEGG, and Mapman analyses. Genes related to the cell wall and to responses to pathogen and hormone were commonly enriched in both lines under P. xanthii stress. Importantly, DEGs related to SA signaling, MAPK signaling and WRKY and Dof transcription factors were found to be involved in the regulation of defense against P. xanthii in the resistant cucumber, but these genes were not identified as DEGs in the susceptible line. Meanwhile, 5 hub genes, including GLPK, ILK1, EIN2, BCDHβ1, and RGGA, were found to be highly associated with the plant immune response by WGCNA analysis. Our study provides valuable information on the early immune response and aids the understanding of the resistance mechanisms of cucumber to P. xanthii stress.
Data Availability Statement
The data presented in the study are deposited in the NCBI SRA repository, accession number PRJNA816625 (https://www.ncbi.nlm.nih.gov/sra/PRJNA816625).
Author Contributions
LC and HF conceived and designed the research. TS provided plant materials. YBY and ZM conducted the experiments. XM analyzed the data and wrote the manuscript. YY and NC revised the manuscript. All authors read and approved the manuscript.
Funding
This study was funded by the National Natural Science Foundation of China (32102366), the Natural Science Foundation of Liaoning Province of China (2020-BS-138), and the Initiative Grant of Shenyang Agricultural University (880419022).
Conflict of Interest
The authors declare that the research was conducted in the absence of any commercial or financial relationships that could be construed as a potential conflict of interest.
Publisher’s Note
All claims expressed in this article are solely those of the authors and do not necessarily represent those of their affiliated organizations, or those of the publisher, the editors and the reviewers. Any product that may be evaluated in this article, or claim that may be made by its manufacturer, is not guaranteed or endorsed by the publisher.
Acknowledgments
We thank the Liaoning Academy of Agricultural Sciences for the breeding technology support.
Supplementary Material
The Supplementary Material for this article can be found online at: https://www.frontiersin.org/articles/10.3389/fpls.2022.872218/full#supplementary-material
Supplementary Figure 1 | Morphological changes and qRT-PCR detection of P. xanthii infection of susceptible strain B21-a-2-2-2 and resistant strain B21-a-2-1-2 for 7 days.
Supplementary Figure 2 | A correlation coefficient analysis of samples.
Supplementary Figure 3 | Principal components analysis of samples.
Supplementary Figure 4 | Volcano diagram analysis of DEGs.
Supplementary Figure 5 | Directed acyclic graph for GO terms of biology process in S0h vs. S6h.
Supplementary Figure 6 | Directed acyclic graph for GO terms of cellular component in S0h vs. S6h.
Supplementary Figure 7 | Directed acyclic graph for GO terms of molecular function in S0h v. S6h.
Supplementary Figure 8 | Directed acyclic graph for GO terms of biology process in R0h vs. R6h.
Supplementary Figure 9 | Directed acyclic graph for GO terms of cellular component in R0h vs. R6h.
Supplementary Figure 10 | Directed acyclic graph for GO terms of molecular function in R0h vs. R6h.
Supplementary Table 1 | List of primers used in this study.
Supplementary Table 2 | Principal component analysis of samples.
Supplementary Table 3 | List of DEGs in S0h vs. R0h comparison.
Supplementary Table 4 | List of DEGs in S0h vs. S6h comparison.
Supplementary Table 5 | List of DEGs in R0h vs. R6h comparison.
Supplementary Table 6 | List of DEGs identified as the same expression trend in S0h vs. S6h, R0h vs. R6h, and S6h vs. R6h comparisons.
Footnotes
- ^ http://www.cucurbitgenomics.org/organism/16
- ^ https://daehwankimlab.github.io/hisat2/
- ^ http://www.geneontology.org/
- ^ http://www.genome.jp/kegg/
- ^ https://mapman.gabipd.org/
References
Ambrosone, A., Batelli, G., Nurcato, R., Aurilia, V., Punzo, P., Bangarusamy, D. K., et al. (2015). The Arabidopsis RNA-binding protein AtRGGA regulates tolerance to salt and drought stress. Plant Physiol. 168, 292–306. doi: 10.1104/pp.114.255802
Amorim, L. L. B., dos Santos, R. F., Neto, J. P. B., Guida-Santos, M., Crovella, S., and Benko-Iseppon, A. M. B. (2017). Transcription factors involved in plant resistance to pathogens. Curr. Protein Pept. Sc. 18, 1–17. doi: 10.2174/1389203717666160619185308
Bi, Y., Zhang, Y. J., Guo, W., Zhang, T., Li, H. Y., Han, X., et al. (2016). Screening of biological agents for controlling powdery mildew of Zucchini and cucumber. Plant Prot. 42, 234–241.
Bigeard, J., Colcombet, J., and Hirt, H. (2015). Signaling mechanisms in pattern-triggered immunity (PTI). Mol. Plant 8, 521–539. doi: 10.1016/j.molp.2014.12.022
Brauer, E. K., Ahsan, N., Dale, R., Kato, N., Coluccio, A. E., Piñeros, M. A., et al. (2016). The Raf-like kinase ILK1 and the high affinity K+ transporter HAK5 are required for innate immunity and abiotic stress response. Plant Physiol. 171, 1470–1484. doi: 10.1104/pp.16.00035
Cao, F. Y., Yoshioka, K., and Desveaux, D. (2011). The roles of ABA in plant-pathogen interactions. J. Plant Res. 124, 489–499. doi: 10.1007/s10265-011-0409-y
Chanda, B., Xia, Y., Mandal, M. K., Yu, K., Sekine, K. T., Gao, Q. M., et al. (2011). Glycerol-3-phosphate is a critical mobile inducer of systemic immunity in plants. Nat. Genet. 43, 421–427. doi: 10.1038/ng.798
Chen, C., Chen, X., Han, J., Lu, W., and Ren, Z. (2020). Genome-wide analysis of the WRKY gene family in the cucumber genome and transcriptome-wide identification of WRKY transcription factors that respond to biotic and abiotic stresses. BMC Plant Biol. 20:443. doi: 10.1186/s12870-020-02625-8
Chen, L., Zhang, L., and Yu, D. (2010). Wounding-induced WRKY8 is involved in basal defense in Arabidopsis. Mol. Plant Microbe Interact. 23, 558–565. doi: 10.1094/MPMI-23-5-0558
Chen, Q., Yu, G., Wang, X., Meng, X., and Lv, C. (2021). Genetics and resistance mechanism of the cucumber (Cucumis sativus L.) against powdery mildew. J. Plant Growth Regul. 40, 147–153. doi: 10.1007/s00344-020-10075-7
Colcombet, J., and Hirt, H. (2008). Arabidopsis MAPKs: a complex signaling network involved in multiple biological processes. Biochem. J. 413, 217–226. doi: 10.1042/BJ20080625
Danquah, A., de Zélicourt, A., Boudsocq, M., Neubauer, J., Freidit Frey, N., Leonhardt, N., et al. (2015). Identification and characterization of an ABA-activated MAP kinase cascade in Arabidopsis thaliana. Plant J. 82, 232–244. doi: 10.1111/tpj.12808
De Mello, U. S., Vidigal, P. M. P., Vital, C. E., Tomaz, A. C., de Figueiredo, M., Peternelli, L. A., et al. (2020). An overview of the transcriptional responses of two tolerant and susceptible sugarcane cultivars to borer (Diatraea saccharalis) infestation. Funct. Integr. Genomics 20, 839–855. doi: 10.1007/s10142-020-00755-8
Djamei, A., Pitzschke, A., Nakagami, H., Rajh, I., and Hirt, H. (2007). Trojan horse strategy in agrobacterium transformation: abusing MAPK defense signaling. Science 318, 453–456. doi: 10.1126/science.1148110
Du, H., Shi, Y., Li, D., Fan, W., Wang, G., and Wang, C. (2017). Screening and identification of key genes regulating fall dormancy in alfalfa leaves. PLoS One. 12:e0188964. doi: 10.1371/journal.pone.0188964
Du, Y. Y., Wang, P. C., Chen, J., and Song, C. P. (2008). Comprehensive functional analysis of the catalase gene family in Arabidopsis thaliana. J. Integr. Plant Biol. 50, 1318–1326. doi: 10.1111/j.1744-7909.2008.00741.x
Eulgem, T., and Somssich, I. E. (2007). Networks of WRKY transcription factors in defense signaling. Curr. Opin. Plant Biol. 10, 366–371. doi: 10.1016/j.pbi.2007.04.020
Galletti, R., Ferrari, S., and De Lorenzo, G. (2011). Arabidopsis MPK3 and MPK6 play different roles in basal and oligogalacturonide- or flagellin-induced resistance against Botrytis cinerea. Plant Physiol. 157, 804–814. doi: 10.1104/pp.111.174003
Gao, Y. J., Fu, W. J., Liu, J., Chen, Y. J., and Dai, G. H. (2020). Morphological changes of Podosphaera xanthii and induced biochemical defenses of cucumber after treated by (+)-(S)-ar-turmerone. Physiol. Mol. Plant Pathol. 112:101524. doi: 10.1016/j.pmpp.2020.101524
Hannigan, G. E., McDonald, P. C., Walsh, M. P., and Dedhars, S. (2011). Intergrin-linked kinase: not so ‘pseudo’ after all. Oncogene 30, 4375–4385. doi: 10.1038/onc.2011.177
Hu, Y., Zhang, M., Lu, M., Wu, Y., Jing, T., Zhao, M., et al. (2021). Salicylic acid carboxyl glucosyltransferase UGT87E7 regulates disease resistance in Camellia sinensis. Plant Physiol. 188, 1507–1520. doi: 10.1093/plphys/kiab569
Hückelhoven, R. (2005). Powdery mildew susceptibility and biotrophic infection strategies. FEMS Microbiol. Lett. 245, 9–17. doi: 10.1016/j.femsle.2005.03.001
Im, J. H., Choi, C., Park, S. R., and Hwang, D. J. (2022). The OsWRKY6 transcriptional cascade functions in basal defense and Xa1-mediated defense of rice against Xanthomonas oryzae pv. Oryzae. Planta 255:47. doi: 10.1007/s00425-022-03830-5
Jammes, F., Song, C., Shin, D., Munemasa, S., Takeda, K., Gu, D., et al. (2009). MAP kinases MPK9 and MPK12 are preferentially expressed in guard cells and positively regulate ROS-mediated ABA signaling. Proc. Natl. Acad. Sci. U.S.A 106, 20520–20525. doi: 10.1073/pnas.0907205106
Kang, L., Li, J., Zhao, T., Xiao, F., Tang, X., Thilmony, R., et al. (2003). Interplay of the Arabidopsis nonhost resistance gene NHO1 with bacterial virulence. Proc. Natl. Acad. Sci. U.S.A 100, 3519–3524. doi: 10.1073/pnas.0637377100
Khokon, M. A. R., Salam, M. A., Jammes, F., Ye, W., Hossain, M. A., Uraji, M., et al. (2015). Two guard cell mitogen-activated protein kinases, MPK9 and MPK12, function in methyl jasmonate-induced stomatal closure in Arabidopsis thaliana. Plant Biol. 17, 946–952. doi: 10.1111/plb.12321
Kiani, M., Bryan, B., Rush, C., and Szczepaniec, A. (2021). Transcriptional responses of resistant and susceptible wheat exposed to wheat curl mite. Int. J. Mol. Sci. 22:2703. doi: 10.3390/ijms22052703
Kiani, M., and Szczepaniec, A. (2018). Effects of sugarcane aphid herbivory on transcriptional responses of resistant and susceptible sorghum. BMC Genomics 19:774. doi: 10.1186/s12864-018-5095-x
Kobayashi, Y., Fukuzawa, N., Hyodo, A., Kim, H., Mashiyama, S., Ogihara, T., et al. (2020). Role of salicylic acid glucosyltransferase in balancing growth and defence for optimum plant fitness. Mol. Plant Pathol. 21, 429–442. doi: 10.1111/mpp.12906
Kozieł, E., Otulak-Kozieł, K., and Bujarski, J. J. (2021). Plant cell wall as a key player during resistant and susceptible plant-virus interactions. Front. Microbiol. 12:656809. doi: 10.3389/fmicb.2021.656809
Lalonde, S., Ehrhardt, D. W., Loqué, D., Chen, J., Rhee, S. Y., and Frommer, W. B. (2008). Molecular and cellular approaches for the detection of protein-protein interactions: latest techniques and current limitations. Plant J. 53, 610–635. doi: 10.1111/j.1365-313X.2007.03332.x
Langfelder, P., and Horvath, S. (2008). WGCNA: an R package for weighted correlation network analysis. BMC Bioinformatics 9:559. doi: 10.1186/1471-2105-9-559
Lee, H. N., Lee, K. H., and Kim, C. S. (2015). Abscisic acid receptor PYRABACTIN RESISTANCE-LIKE 8, PYL8, is involved in glucose response and dark-induced leaf senescence in Arabidopsis. Biochem. Biophys. Res. Commun. 463, 24–28. doi: 10.1016/j.bbrc.2015.05.010
Li, Y., Qiu, L., Liu, X., Zhang, Q., Zhuansun, X., Fahima, T., et al. (2020). Glycerol-induced powdery mildew resistance in wheat by regulating plant fatty acid metabolism, plant hormones cross-talk, and pathogenesis related genes. Int. J. Mol. Sci. 21:673. doi: 10.3390/ijms21020673
Liu, X., Gu, X., Lu, H., Liu, P., Miao, H., Bai, H., et al. (2021). Identification of novel loci and candidate genes for resistance to powdery mildew in a resequenced cucumber germplasm. Genes 12:584. doi: 10.3390/genes12040584
Ma, C., Xiong, J., Liang, M., Liu, X., Lai, X., and Bai, Y. (2021). Strawberry WRKY transcription factor WRKY50 is required for resistance to necrotrophic fungal pathogen Botrytis cinerea. Agronmy 11:2377. doi: 10.3390/agronomy11122377
Martinez, M., Somoza, I. R., Fuentes, R., Lara, P., Carbonero, P., and Diaz, I. (2005). The barley cystatin gene (Icy) is regulated by DOF transcription factors in aleurone cells upon germination. J. EXP. Bot. 56, 547–556. doi: 10.1093/jxb/eri033
Mceldoon, J. P., Pokora, A. R., and Dordick, J. S. (2012). Lignin peroxidase-type activity of soybean peroxidase. Enzyme Microb. Tech. 17, 359–369. doi: 10.1016/0141-0229(94)00060-3
Meng, X., Song, T., Fan, H., Yu, Y., Cui, N., Zhao, J., et al. (2016). A comparative cell wall proteomic analysis of cucumber leaves under Sphaerotheca fuliginea stress. Acta Physiol. Plant 38:260.
Meng, X., and Zhang, S. (2013). MAPK cascades in plant disease resistance signaling. Annu. Rev. Phytopathol. 51, 245–266. doi: 10.1146/annurev-phyto-082712-102314
Meng, X. N., Yang, Y., Zhao, J. Y., Cui, N., Song, T. F., Yang, Y., et al. (2018). The two translationally controlled tumor protein genes, CsTCTP1 and CsTCTP2, are negative modulators in the Cucumis sativus defense response to Sphaerotheca fuliginea. Front. Plant Sci. 9:544. doi: 10.3389/fpls.2018.00544
Miedes, E., Vanholme, R., Boerjan, W., and Molina, A. (2014). The role of the secondary cell wall in plant resistance to pathogens. Front. Plant Sci. 5:358. doi: 10.3389/fpls.2014.00358
Mo, L. N. (2005). Primary Research on the Application and Effect on Cucumber Powdery Mildew (Sphaerotheca fuliginea) by using salicylic acid. [dissertation/master’s thesis]. Yangling (SX): Northwest A&F University.
Molina, A., Miedesa, E., Bacetea, L., Rodríguezc, T., Mélidaa, H., Denancé, N., et al. (2021). Arabidopsis cell wall composition determines disease resistance specificity and fitness. Proc. Natl. Acad. Sci. U.S.A 118:e2010243118. doi: 10.1073/pnas.2010243118
Nie, J. T., Wang, Y. L., He, H. L., Guo, C. L., Zhu, W. Y., Pan, J., et al. (2015). Loss-of-function mutations in CsMLO1 confer durable powdery mildew resistance in cucumber (Cucumis sativus L.). Front. Plant Sci. 6:1155. doi: 10.3389/fpls.2015.01155
Niu, J., Ni, Y., Liu, J., Wang, Z., and Yin, J. (2010). Inducing effect of JA on wheat powdery mildew resistance. Chin. Agric. Sci. Bull. 26, 254–257.
Nobori, T. (2022). A qWRKY regulator of ROS: TaWRKY19 suppresses TaNOX10 and causes pathogen susceptibility in wheat. Plant Cell. doi: 10.1093/plcell/koac016
Otulak-Kozieł, K., Kozieł, E., Lockhart, B. E. L., and Bujarski, J. J. (2020). The expression of potato expansin A3 (StEXPA3) and extensin4 (StEXT4) genes with distribution of StEXPAs and HRGPs-extensin changes as an effect of cell wall rebuilding in two types of PVYNTN-Solanum tuberosum interactions. Viruses 12:66. doi: 10.3390/v12010066
Persson, S., Wei, H., Milne, J., Page, G. P., and Somerville, C. R. (2005). Identification of genes required for cellulose synthesis by regression analysis of public microarray data sets. Proc. Natl. Acad. Sci. U.S.A 102, 8633–8638. doi: 10.1073/pnas.0503392102
Pitzschke, A., Djamei, A., Teige, M., and Hirt, H. (2009). VIP1 response elements mediate mitogen-activated protein kinase 3-induced stress gene expression. Proc. Natl. Acad. Sci. U.S.A 106, 18414–18419. doi: 10.1073/pnas.0905599106
Qiu, J. L., Zhou, L., Yun, B. W., Nielsen, H. B., Fiil, B. K., Petersen, K., et al. (2008). Arabidopsis mitogen-activated protein kinase kinases MKK1 and MKK2 have overlapping functions in defense signaling mediated by MEKK1, MPK4, and MKS1. Plant Physiol. 148, 212–222. doi: 10.1104/pp.108.120006
Ren, H. M. (2011). Inhibitive mechanisms of chrysophanol against Sphaerotheca fuliginea. [dissertation/doctor’s thesis]. Baoding (HB): Hebei Agricultural University.
Robatzek, S., and Somssich, I. E. (2002). Targets of AtWRKY6 regulation during plant senescence and pathogen defense. Genes Dev. 16, 1139–1140. doi: 10.1101/gad.222702
Sandhu, A. P. S., Randhawa, G. S., and Dhugga, K. S. (2009). Plant cell wall matrix polysaccharide biosynthesis. Mol. Plant 2, 840–850. doi: 10.1093/mp/ssp056
Shen, L. P. (2009). Genetic analysis and preliminary mapping of QTL associated with powdery mildew resistance in cucumber. [dissertation/master’s thesis]. Yangzhou (JS): Yangzhou University.
Shi, Y. (2016). To identify candidate genes with LRR domain governing powdery mildew resistance based on whole genome re-sequencing in cucumber (Cucumis sativus L.). [dissertation/master’s thesis]. Yangzhou (JS): Yangzhou University.
Shoresh, M., Gal-On, A., Leibman, D., and Chet, I. (2006). Characterization of a mitogen-activated protein kinase gene from cucumber required for Trichoderma-conferred plant resistance. Plant Physiol. 142, 1169–1179. doi: 10.1104/pp.106.082107
Song, F., and Goodman, R. M. (2002). Cloning and identification of the promoter of the tobacco Sar8.2b gene, a gene involved in systemic acquired resistance. Gene 290, 115–124. doi: 10.1016/s0378-1119(02)00584-x
Song, J. T., Koo, Y. J., Seo, H. S., Kim, M. C., Chio, Y. D., and Kim, J. H. (2007). Overexpression of AtSGT1, an Arabidopsis salicylic acid glucosyltransferase, leads to increased susceptibility to Pseudomonas gringae. Phytochemistry 69, 1128–1134. doi: 10.1016/j.phytochem.2007.12.010
Su, J., Zhang, M., Zhang, L., Sun, T., Liu, Y., Lukowitz, W., et al. (2017). Regulation of stomatal immunity by interdependent functions of a pathogen-responsive MPK3/MPK6 cascade and abscisic acid. Plant Cell 29, 526–542. doi: 10.1105/tpc.16.00577
Sun, H., Song, N., Ma, L., Li, J., Ma, L., Wu, J., et al. (2017). Ethylene signalling is essential for the resistance of Nicotiana attenuata against Alternaria alternata and phytoalexin scopoletin biosynthesis. Plant Pathol. 66, 277–284. doi: 10.1111/ppa.12568
Sun, T., Nitta, Y., Zhang, Q., Wu, D., Tian, H., Lee, J. S., et al. (2018). Antagonistic interactions between two MAP kinase cascades in plant development and immune signaling. EMBO Rep. 19:e45324. doi: 10.15252/embr.201745324
Tamang, P., Richards, J. K., Solanki, S., Ameen, G., Poudel, R. S., Deka, P., et al. (2021). The barley HvWRKY6 transcription factor is required for resistance against Pyrenophora teres f. teres. Front. Genet. 11:601500. doi: 10.3389/fgene.2020.601500
Thimm, O., Bläsing, O., Gibon, Y., Nagel, A., Meyer, S., Krüger, P., et al. (2004). MAPMAN: a user-driven tool to display genomics data sets onto diagrams of metabolic pathways and other biological processes. Plant J. 37, 914–939. doi: 10.1111/j.1365-313x.2004.02016.x
Thomma, B. P. H. J., Eggermont, K., Tierens, K. F. M. J., and Broekaert, W. F. (1999). Requirement of functional Ethylene-Insensitive 2 gene for efficient resistance of Arabidopsis to infection by Botrytis cinerea. Plant Physiol. 121, 1093–1101. doi: 10.1104/pp.121.4.1093
Thomma, B. P. H. J., Nürnberger, T., and Joosten, M. H. A. J. (2011). Of PAMPs and effectors: the blurred PTI-ETI dichotomy. Plant Cell 23, 4–15. doi: 10.1105/tpc.110.082602
Ulker, B., and Somssich, I. E. (2004). WRKY transcription factors: from DNA binding towards biological function. Curr. Opin. Plant Biol. 7, 491–498. doi: 10.1016/j.pbi.2004.07.012
Vanholme, R., Cesarino, I., Rataj, K., Xiao, Y., Sundin, L., Goeminne, G., et al. (2013). Caffeoyl shikimate esterase (CSE) is an enzyme in the lignin biosynthetic pathway in Arabidopsis. Science 341, 1103–1106. doi: 10.1126/science.1241602
Vlot, A. C., Dempsey, D. A., and Klessig, D. F. (2009). Salicylic acid, a multifaceted hormone to combat disease. Annu. Rev. Phytopathol. 47, 177–206. doi: 10.1146/annurev.phyto.050908.135202
Wang, C., He, X., Li, Y., Wang, L., Guo, X., and Guo, X. (2018). The cotton MAPK kinase GhMPK20 negatively regulates resistance to Fusarium oxysporum by mediating the MKK4-MPK20-WRKY40 cascade. Mol. Plant Pathol. 19, 1624–1638. doi: 10.1111/mpp.12635
Wang, J., Pan, C., Wang, Y., Ye, L., Wu, J., Chen, L., et al. (2015). Genome-wide identification of MAPK, MAPKK, and MAPKKK gene families and transcriptional profiling analysis during development and stress response in cucumber. BMC Genomics 16:368. doi: 10.1186/s12864-015-1621-2
Wang, X., Chen, Q. M., Huang, J. N., Meng, X. N., Cui, N., Yu, Y., et al. (2021). Nucleotide-binding leucine-rich repeat genes CsRSF1 and CsRSF2 are positive modulators in the Cucumis sativus defense response to Sphaerotheca fuliginea. Int. J. Mol. Sci. 22:3986. doi: 10.3390/ijms22083986
Wang, X., Zhang, D., Cui, N., Yu, Y., Yu, G., and Fan, H. (2018). Transcriptome and miRNA analyses of the response to Corynespora cassiicola in cucumber. Sci. Rep. 8:7798. doi: 10.1038/s41598-018-26080-6
Wang, Y., Bo, K., Gu, X., Pan, J., Li, Y., Chen, J., et al. (2020). Molecularly tagged genes and quantitative trait loci in cucumber with recommendations for QTL nomenclature. Hortic. Res. 7:3. doi: 10.1038/s41438-019-0226-3
Wani, S. H., Anand, S., Singh, B., Bohra, A., and Joshi, R. (2021). WRKY transcription factors and plant defense responses: latest discoveries and future prospects. Plant Cell Rep. 40, 1071–1085. doi: 10.1007/s00299-021-02691-8
Wen, C., Cheng, Q., Zhao, L., Mao, A., Yang, J., Yu, S., et al. (2016). Identification and characterisation of Dof transcription factors in the cucumber genome. Sci. Rep. 6:23072. doi: 10.1038/srep23072
Xing, Y., Jia, W., and Zhang, J. (2007). AtMEK1 mediates stress-induced gene expression of CAT1 catalase by triggering H2O2 production in Arabidopsis. J. Exp. Bot. 58, 2969–2981. doi: 10.1093/jxb/erm144
Xu, J., Meng, J., Meng, X., Zhao, Y., Liu, J., Sun, T., et al. (2016). Pathogen-responsive MPK3 and MPK6 reprogram the biosynthesis of indole glucosinolates and their derivatives in Arabidopsis immunity. Plant Cell 28, 1144–1162. doi: 10.1105/tpc.15.00871
Yang, Y., Zhao, J., Liu, P., Xing, H., Li, C., Wei, G., et al. (2013). Glycerol-3-phosphate metabolism in wheat contributes to systemic acquired resistance against Puccinia striiformis f. sp. tritici. PLoS One. 8:e81756. doi: 10.1371/journal.pone.0081756
Yu, T. (2015). Identification of cucumber powdery mildew resistance of single segment substitution lines based on SNP markers and analysis of resistance gene function. [dissertation/master’s thesis]. Yangzhou (JS): Yangzhou University.
Yu, Y. H., Bian, L., Wan, Y. T., Jiao, Z. L., Yu, K. K., Zhang, G. H., et al. (2019). Grape (Vitis vinifera) VvDOF3 functions as a transcription activator and enhances powdery mildew resistance. Plant Physiol. Bioch. 143, 183–189. doi: 10.1016/j.plaphy.2019.09.010
Zhang, T., Liu, Y., Yang, T., Zhang, L., Xu, S., Xue, L., et al. (2006). Diverse signals converge at MAPK cascades in plant. Plant Physiol. Biochem. 44, 274–283. doi: 10.1016/j.plaphy.2006.06.004
Zhao, X., Qiu, T., Feng, H., and Yin, C. (2020). A novel glycine-rich domain protein, OsGRDP1, functions as a critical feedback regulator for controlling cell death and disease resistance in rice. J. EXP. Bot. 72, 608–622. doi: 10.1093/jxb/eraa450
Zhao, Y., Chan, Z., Gao, J., Xing, L., Cao, M., Yu, C., et al. (2016). ABA receptor PYL9 promotes drought resistance and leaf senescence. Proc. Natl. Acad. Sci. U.S.A 113, 1949–1954. doi: 10.1073/pnas.1522840113
Zhao, Y., Xing, L., Wang, X., Hou, Y. J., Gao, J., Wang, P., et al. (2014). The ABA receptor PYL8 promotes lateral root growth by enhancing MYB77-dependent transcription of auxin-responsive genes. Sci. Signal. 7:ra53. doi: 10.1126/scisignal.2005051
Keywords: Cucumis sativus L., Podosphaera xanthii, transcriptome, resistance gene, initial stage control
Citation: Meng X, Yu Y, Song T, Yu Y, Cui N, Ma Z, Chen L and Fan H (2022) Transcriptome Sequence Analysis of the Defense Responses of Resistant and Susceptible Cucumber Strains to Podosphaera xanthii. Front. Plant Sci. 13:872218. doi: 10.3389/fpls.2022.872218
Received: 17 February 2022; Accepted: 01 April 2022;
Published: 12 May 2022.
Edited by:
Zhiyong Liu, Institute of Genetics and Developmental Biology (CAS), ChinaReviewed by:
Yuhui Wang, Nanjing Agricultural University, ChinaLingli Dong, State Key Laboratory of Plant Cell and Chromosome Engineering, Institute of Genetics and Developmental Biology (CAS), China
Copyright © 2022 Meng, Yu, Song, Yu, Cui, Ma, Chen and Fan. This is an open-access article distributed under the terms of the Creative Commons Attribution License (CC BY). The use, distribution or reproduction in other forums is permitted, provided the original author(s) and the copyright owner(s) are credited and that the original publication in this journal is cited, in accordance with accepted academic practice. No use, distribution or reproduction is permitted which does not comply with these terms.
*Correspondence: Lijie Chen, Y2hlbmxqLTAyMTBAc3lhdS5lZHUuY24=; Haiyan Fan, aHlmYW43NEBzeWF1LmVkdS5jbg==
†These authors have contributed equally to this work