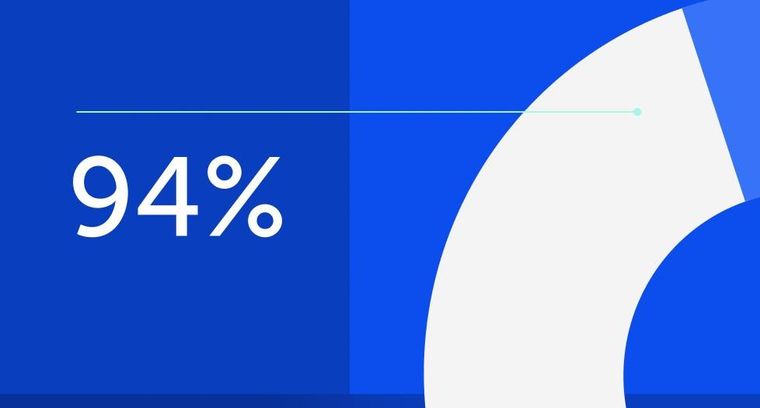
94% of researchers rate our articles as excellent or good
Learn more about the work of our research integrity team to safeguard the quality of each article we publish.
Find out more
MINI REVIEW article
Front. Plant Sci., 19 May 2022
Sec. Plant Biotechnology
Volume 13 - 2022 | https://doi.org/10.3389/fpls.2022.870626
This article is part of the Research TopicMechanisms of Abiotic Stress Responses and Tolerance in Plants: Physiological, Biochemical and Molecular Interventions, volume IIView all 37 articles
Plants defend themselves against ecological stresses including drought. Therefore, they adopt various strategies to cope with stress, such as seepage and drought tolerance mechanisms, which allow plant development under drought conditions. There is evidence that microbes play a role in plant drought tolerance. In this study, we presented a review of the literature describing the initiation of drought tolerance mediated by plant inoculation with fungi, bacteria, viruses, and several bacterial elements, as well as the plant transduction pathways identified via archetypal functional or morphological annotations and contemporary “omics” technologies. Overall, microbial associations play a potential role in mediating plant protection responses to drought, which is an important factor for agricultural manufacturing systems that are affected by fluctuating climate.
Despite several advances in agricultural technology, drought is a major environmental stress for plants. Interventions with microbes can mitigate drought stress (Hossain et al., 2016; Li and Liu, 2016; Salehi-Lisar and Bakhshayeshan-Agdam, 2016; Shinozaki et al., 2016). In this review, we summarized drought stress mitigation using microbes, current control tactics, and unconventional control approaches. We also discussed possible alternative strategies for more effective drought-stress management.
Drylands are used to evaluate drought stress in biomes because of their limited agricultural productivity. Given the importance of water as a macromolecule for the physiological growth and development of plants, water scarcity and deprivation are phenomena that commonly cause drought stress (Siddique et al., 2000; Bartels and Phillips, 2010; Farooq et al., 2012; Askari-Khorasgani et al., 2021). This is a major environmental constraint that limits crop production; indeed, drought stress affects physiological, biochemical, and molecular levels. In response to such stress, plants enter avoidance, tolerance, or adaptation phases (Palmer, 1965; Svoboda et al., 2002; Farooq et al., 2009; Basu et al., 2016). Lack of water affects the water-plant relationship, arrests the plant growth, and causes a reduction in the leaf size, stem extension, and root proliferation. Arrested plant growth leads to reduced carbon dioxide assimilation, which subsequently damages membrane potential. This damage leads to the accumulation of reactive oxygen species (ROS), with free radical accumulation leading to oxidative stress and disturbances in adenosine triphosphate synthesis (Anjum et al., 2011; Van Loon, 2015). Drought stress can be reduced through breeding, mass screening, and exogenous phytohormone production (Siddiqui et al., 2022). Plants can autoregulate to minimize stress by producing phytohormones [e.g., abscisic acid (ABA) and gibberellins] and low-molecular-weight osmolytes (e.g., amino acids and polyols) and by modifying succulent leaves to reduce transpiration loss (Seki et al., 2007; Farooq et al., 2009; Rahdari and Hoseini, 2012).
Microbes can promote plant growth both directly and indirectly (Figure 1). The indirect activation of plant growth involves a series of events by which microbes prevent the inhibition of plant growth and development induced by pathogens (Ahmad et al., 2021). During direct activation, microbes biosynthesize bacterial compounds that promote the uptake of nutrients from the soil and stimulate plant growth and development (Berg, 2009; Choudhary et al., 2016). Microbes induce local or systemic stress mitigation response mechanisms that help plants survive under abiotic stress conditions (Figure 2), such as drought stress, and help plants sustain growth and development through the fixation, mobilization, and/or production of nutrients, hormones, and organic phytostimulants (Martins et al., 2018; Yadav and Yadav, 2018; Kour and Yadav, 2020). In this study, we summarized the currently known implications of using microbes for drought tolerance, including their fundamental mechanisms of action (Figure 3). This review adds to previously published reviews by addressing the implementation of mitigation strategies to prevent adverse effects of drought stress.
Phytohormones such as gibberellins, ethylene, indole acetic acid (IAA), ABA, and cytokinin are chemical messengers that are organic in nature, synchronize cellular events in plants, and play key roles in plant progression and drought stress (Kefeli et al., 2003; Khan et al., 2012; Jogawat et al., 2021).
Phytohormones are produced by plants and, in some cases, by microorganisms that interact with plants. Auxin mitigates drought stress in a secondary manner via cumulative root growth and modification of root design and/or root hairs, which absorb water and nutrients from the soil. Auxins are produced via tryptophan-dependent pathways (Vanneste and Friml, 2009; Zhao, 2010). They produce indole-3-acetamide, which is converted into indole-3-acetaldoxime and tryptamine to give the final product, i.e., indole-3-pyruvic acid (Skubacz et al., 2016). Several studies have provided evidence to support the role of IAA in inducing drought-related signaling pathways when plants are exposed to osmotic stress (Fahad et al., 2015; Yu et al., 2020). These adaptations are interrelated with higher auxin amalgamation in microbe-treated plants; auxin has the capacity to elongate the stems and coleoptiles of plants under stress conditions (Raheem et al., 2018; Ullah et al., 2019).
The application of microbes also enhances the production of plant gibberellins, which are diterpeniods that cause hyperactive elongation of stems in response to stress. Gibberellins are associated with two bioactive components, namely, carotenes and isoprene (Davies, 1995; Alagoz et al., 2018). Carotenes can stimulate photosynthesis and protect plant cells from harmful photodynamic reactions, whereas isoprene maintains turgor pressure and stabilizes the cell membrane structure (Jeon et al., 2003; Mollah and Pratiwi, 2020).
In response to abiotic stress, cytokinin biosynthesis increases in plants in association with auxin and regulation of developmental responses. Cytokinin can transfer the phosphoryl group P+O32–. In addition, cytokinin helps in the phosphorylation of sugar molecules, which leads to their accumulation in cells and prevents reverse diffusion (Mok and Mok, 2001). Competitive phosphorylation helps plant cells combat stress, and cytokinin protects plant cells from oxidative stress (Werner and Schmülling, 2009; Wybouw and De Rybel, 2019).
Ethylene is an important hormone that regulates not only plant growth but also senescence of growth attributes. It frequently interacts with other hormones. Ethylene is a second messenger hormone that regulates various developmental processes, ranging from germination to the plant life cycle (Kanellis et al., 2012; Wen, 2014).
Abscisic acid is a particularly important hormone that is secreted when plants are subjected to stress; thus, it is known as a universal stress hormone. ABA plays an important role in mitigating stress because it causes stomatal closure and is a powerful inhibitor of stomatal opening (Bright et al., 2006). ABA also regulates genes responsible for desiccation tolerance (Sakata et al., 2014).
The ability of these rhizobacteria to synthesize and produce phytohormones is the first key factor in mitigating stress through a complex signaling network in plants to respond to environmental conditions and maintain the plant inner environment through regulation of these hormones, antioxidants, and osmolytes to enhance stress tolerance.
Aminocyclopropane-1-carboxylic acid (ACC) is a precursor of ethylene, the production of which increases in plants under ecological stress. Numerous microorganisms have been reported to produce ACC deaminase, which reduces ACC, thereby lowering the increased levels of ethylene in host plants (Campbell, 1995; Houda et al., 2013). Under drought stress, plants enhance their ethylene production, which inhibits plant growth by affecting seed germination and root enlargement. Higher ACC levels are induced to combat severe drought stress (Dikarev et al., 2016; Ustiatik et al., 2021).
Beneficial microbes can produce ACC deaminase. ACC regulates plant growth and development by sequestering and cleaving plant-produced ACC and lowering the level of ethylene in plants.
When plants are subjected to abiotic stress, a sudden loss of osmolytes occurs, which can shock the plant. However, microbial interactions lead to osmotic adjustments. Plant–microbe interactions are facilitated by secreted metabolites; plants upregulate the production of metabolites such as proline, sugars, glycine, organic acids, trehalose, betaine, potassium, calcium, and chloride ions as an adaptive response to drought stress. As one of the most important osmolytes, proline accumulates in plants under osmotic stress and facilitates the maintenance and osmotic adjustment of cellular components in plant cells to overcome stress. Some rhizosphere bacteria produce proline (Yamada et al., 2005; Mohammadkhani and Heidari, 2008; Dobra et al., 2010).
Glycine betaine is a secondary metabolite that helps plants maintain growth and development. Trehalose is a safe, non-reducing sugar that contains two glucose molecules and stores energy for use under stress conditions. Microbes can accelerate trehalose biosynthesis through the TPS/trehalose-6-phosphate phosphatases (TPS/TPP) pathway, maintain osmolytes concentrations, and stabilize turgor pressure in plant cells (Iordachescu and Imai, 2011; Kahraman et al., 2019). The application of microbes to plant cells increases the secretion of organic acids (e.g., oxalic acid, citric acid, and malic acid) and minerals (e.g., chlorine, sodium, and potassium), which are important for nutrient availability, metabolic reactions, and the maintenance of osmoregulation in plant cells (Kirst, 1996; Chen and Jiang, 2010).
The ability to regulate osmotic amendment of plant-growth-promoting rhizobacteria (PGPR) in plant cells is due to generating a low water gradient potential in the cytosol. They maintain the turgor pressure and osmotic adjustment and improve stress tolerance.
Exopolysaccharides are macromolecules composed of long-chain polymers of repeating sugar units such as glucose, galactose, and rhannose in different ratios (Ilyas et al., 2020; Bhagat et al., 2021). Exopolysaccharides form hydrophilic biofilms that offer protection against aridness during osmotic stress by enhancing the water-retaining potential of the soil and regulating the distribution of biological carbon sources. Microbes form sheaths to protect roots from dehydration and maintain the moisture content (Sandhya et al., 2009; Nadeem et al., 2021). Exopolysaccharides can be released into soil as slime ingredients comprising cation hydrogen bridges, van der Waals linkages, and anion adsorption interactions, which act as defensive capsules around the soil and improve the biological properties of the soil by increasing aggregation and macroporosity (Ilyas et al., 2020). Overall, exopolysaccharide production helps plants cope with abiotic stress (Naseem et al., 2018).
The vital interaction of the plant and microbes facilitates the production of a biofilm that allows microbes to attach with plant roots, provides a shield to the roots, and imparts a strong root adhering capability.
The application of beneficial microbes produces volatile compounds that can increase growth, development, photosynthesis, iron uptake, and crop productivity, while reducing the incidence of plant diseases and death. For example, stress-induced volatile compounds such as 3-hydroxy-2-butanone (acetoin), 2,3-butanediol, and 2-pentylfuran play a role in plant growth and development (Seco et al., 2015; Ali et al., 2017). These volatile compounds are also insect repellents owing to their strong odor and help boost plant growth. Under stress conditions, these compounds cause stomatal closure and impart systemic stress resistance to plants, highlighting their role in plant growth and development under stress conditions (Liu and Zhang, 2015; Mohammadipanah and Zamanzadeh, 2019).
Plant–microbial interactions emit volatile oil compounds (VOCs), which mitigate stress tolerance through the expression of genes and scavenge free radicals.
Osmotic stress induces the production of ROS in plants, including superoxide, radicals, singlet oxygen, hydrogen peroxide (H2O2), superoxide anion radicals, and alkoxy radicals. ROS react with lipids, proteins, and DNA, thereby causing oxidative damage and affecting redox regulation in plants (Tambussi et al., 2000; Ahmad et al., 2014). To protect against oxidative damage during osmotic stress, plants induce antioxidant defense systems involving enzymatic and non-enzymatic pathways (Chai et al., 2005). Enzymatic antioxidant pathways involve enzymes such as glutathione reductase, superoxide dismutase (SOD), catalase, and ascorbate peroxidase, whereas non-enzymatic components include ABA, cysteine, and glutathione (Chai et al., 2005; Ahmad et al., 2014; Li B. et al., 2020). The presence and exogenous inoculation of microbes in the soil can confer drought tolerance by inducing antioxidant systems. Various microbes, such as Actinomycetes, fungi, and algae, contain phenolic components that are secreted when plants are subjected to stress (Kaushal and Wani, 2016; Ilyas et al., 2021).
The ROS scavenging ability of PGPR regulates the antioxidant enzymes and may provide a solid barrier against abiotic stress. The plant–microbial interaction ensures that there is always a homeostatic balance between ROS and removal machinery, which mitigates stress tolerance in plants.
Stress tolerance can be enhanced by treating plants with microbes because this induces stress-response genes that regulate plant stress. The expression of such genes is modulated in plants under drought stress, which helps optimize plant growth and development. Stress-related genes and proteins involved in plant–microbe interactions include CaPR-10, sHSP, Δ1-pyrroline-5-carboxylate reductase (P5CR), dehydrin-like protein (Cadhn), vacuolar ATPase (VA), and pyrroline-5-carboxylate dehydrogenase (P5CDH) (Shinozaki and Yamaguchi-Shinozaki, 1998, 2007). Microarray studies have suggested that gene expression can be categorized into functional or regulatory proteins depending on the role of the encoded protein. Regulatory proteins include transcription factors, protein phosphate kinases, and ABA biosynthetic factors. Functional proteins include detoxification enzymes, water channel transporters, proteases, osmolyte biosynthesis enzymes, and macromolecule protection factors. Genes and transcription factors can encode sustainable agricultural products (Shinozaki and Yamaguchi-Shinozaki, 1998, 2007; Kavar et al., 2008).
The expression and upregulation of stress-related genes in response to drought stress can be used as a powerful tool for mitigating and enhancing drought tolerance in plants.
Drought stress causes an imbalance between the mineral and ion exchange. It disturbs the levels of Na+, Cl–, and K+, which are responsible for plant growth. Plant–microbial interactions are able to maintain the ionic balance by scavenging the NHXI protein in the plant cytosol (Mukerji et al., 2006; Banfield and Nealson, 2018).
Beneficial microbial populations produce large numbers of organic components that can be used as signaling molecules and nutritional components for plants under drought stress. Various microbiota, such as fungi, cyanobacteria, and plant growth-promoting rhizobacteria, play important roles in mineral uptake and rehabilitation of the nutritional status (Teotia et al., 2016; Zhu et al., 2016). They mediate the solubilization of phosphosulfate compounds, nitrogen fixation, denitrification, and siderophore production. There are two pathways for mineral uptake, namely, direct and indirect (Zhu et al., 2016; Kapadia et al., 2021). Phosphate is present in the ecosystem mostly in the form of inositol phosphate; therefore, microbes solubilize phosphorus into the most soluble form by synthesizing low-molecular-weight components such as citric acid and gluconic acid. This is an example of an indirect pathway: in direct pathways, phosphorus is solubilized by lowering the pH of the external environment and turns into low-molecular-weight organic compounds (Hupfauf et al., 2016; Sharma et al., 2020).
Therefore, a microbial population that interacts with plants in a beneficial environment can provide hemostasis for ionic exchange in the cytosol by maintaining the nutritional status of plants under drought stress.
Endophytes are microscopic organisms (i.e., bacteria, fungi, and viruses) that exhibit symbiotic relationships with plants (Figure 4). They have been comprehensively investigated because of their beneficial effects on environmental stress (Cheng et al., 2021; Meena et al., 2021; Verma et al., 2021). Endophytes can amplify biomass accumulation (fresh and dry) in host plants under stressful conditions (Zhang et al., 2006; Barman et al., 2016). The stress mitigation effects of the endophytes were comparable among different plant species. Under drought conditions in the presence of endophytes, eudicots and C4 plant species showed increased biomass compared with monocots and C3 species. Evidence of endophyte-mediated plant stress attenuation has been established in recent decades (Strobel, 2003; Rodriguez et al., 2009).
Microbes are beneficial for reducing stress, which would otherwise limit the crop yield. When plants are under stress, they experience difficulty in sustaining their normal growth. In a previous study (Singh et al., 2020), rice crops were subjected to drought stress, and various characteristics, such as growth limitation and metabolite content, were recorded. These plants were inoculated with a combination of Trichoderma T42 and Pseudomonas, which significantly improved their metabolic ability during drought stress. Microbial induction increases the total polyphenolic content and activates antioxidant enzymes to reduce oxidative stress. Inoculation also led to overexpression of PAL, a gene responsible for combating drought stress. These results were significant compared with those of uninoculated plants (Singh et al., 2020).
In a comparative analysis, two groups were experimentally compared, namely, untreated red rice seedlings and red rice seedlings inoculated with Gluconacetobacter diazotrophicus strain Pal5 (Filgueiras et al., 2020). Both seedling types were grown under water constraints of 30–35, 50–55, 70–75, or 100% field capacity for 15 days. Physical, chemical, and molecular evaluations of the plants were subsequently conducted. Leaves were found to contain increased levels of osmoprotectants, such as osmolytes (e.g., proline and glycine betaine), which help combat drought stress. Omics analysis showed the relative gene expression of gor, cat, P5CR, sod, and BADH. Overall, this study suggests that G. diazotrophicus is effective at combating the effects of drought stress.
Currently, water scarcity is a major challenge; however, rhizobacteria can facilitate plant growth under water stress. A study conducted in India involved the inoculation of great millet plants with strains of Streptomyces laurentii (EU-LWT3-69) and Penicillium sp. (EU-DSF-10), which was followed by exposure to drought stress (Kour et al., 2020a). Under drought stress, plants experience various issues, including reduced bioavailability of phosphorus, which is an important component of the soil present in its active form in microbes that are precursors to the initiation of the phosphorus availability cycle. Both the tested bacterial strains, namely, EU-DSF-10 and EU-LWT3-69, tended to solubilize phosphorus, which increased its availability to plants. These two bacterial strains are also known to solubilize phosphorus. Phosphorus-solubilizing microbes also increased the chlorophyll content, proline levels, and glycine betaine levels while reducing lipid peroxidation. Overall, the results of this study suggest that inoculated plants show better growth and defense under drought conditions than those of uninoculated plants (Kour et al., 2020a). In a study (Bilal et al., 2020), the combined effects of LHL10 and LHL06 inoculation on Glycine max were investigated. The microbes produced a significant synergistic effect, with observable increases in the dry biomass, roots, shoot length, and leaf area. The genomic analysis revealed an increase in HSP90 expression levels, while the biochemical analysis revealed that lipid peroxidation increased by 24.8–141.6%. Additionally, increased calcium levels and phosphate-solubilizing potential were observed. In conclusion, the synergistic effects were remarkably effective against combined stresses, as shown in Table 1 (Bilal et al., 2020).
In another study conducted by Kang et al. (2021), two Enterobacter ludwigii strains, namely, AFFR02 and Mj1212, were isolated and inoculated into alfalfa plants; subsequently, their growth attributes, hormones, and mineral concentrations were assessed. The data showed that bacterial inoculation significantly rescued plants from drought stress. The stalk diameter, fresh and dry biomass, and root/shoot elongation were all significantly higher in the treated plants than in the untreated plants. In addition, treated plants have higher levels of ABA, flavonoids, and minerals than those of untreated plants (Kang et al., 2021).
Silambarasan et al. (2019) mixed the Rhodotorula mucilaginosa strain CAM4 with sawdust at a ratio of 1–5%, and the formulation was applied to Lactuca sativa. The inoculated microbial formulations displayed tolerance to drought stress at various developmental stages. An increase in the content of chlorophyll (48–65%), carotenoids (12–54%), and proline was observed. Proline is an important precursor for maintaining the cell structure and clearing oxidative stress. Plant growth and development were observed for 24 weeks, and growth, dry biomass, root proliferation, and stem elongation were all significantly improved in the treated group compared with those in the untreated group. Furthermore, malondialdehyde (MDA) levels decreased, indicating lipid peroxidation. Overall, the formulation was suitable for reducing aluminum, drought, and salinity stress and reduced oxidative stress by activating antioxidant enzymes (Silambarasan et al., 2019).
Desert plants use fungal endophytes to mitigate salinity and drought stresses in arid environments. In a study conducted by Jain et al. (2020), tomato and cucumber seedlings were exposed to fungal endophytes for 96 days. These include halotolerant microbes, such as Neocamarosporium chichastianum, Neocamarosporium goegapense, and Periconia macrospinosa, which mitigate stress by increasing the levels of proline, antioxidant enzymes, and chlorophyll, as well as enhancing growth attributes. Among these, P. macrospinosa significantly reduced drought and salinity stress. Thus, some endophytes can adapt to particular habitats and act as biofertilizers and ecofriendly protectors against stress (Jain et al., 2020).
When the bacterial strain YNA59 was inoculated into a broccoli plant (Kim et al., 2020), a remarkable increase in hormone levels (e.g., ABA, jasmonate, and salicylic acid) was observed. Inoculated plants also showed significant enhancement in antioxidant enzyme levels, including SOD, catalase, and ascorbate peroxidase levels (Kim et al., 2020). Another study conducted to determine the role of the fungal endophyte Neotyphodium coenophialum when inoculated into Lolium arundinaceum under drought stress revealed that the mechanism of drought tolerance was dependent on the osmotic balance and increased water uptake efficiency, which enhanced photosynthesis and altered gene expression. In addition, endophytes isolated from a Nicotiana plant and inoculated into Nicotiana benthamiana increased the ability of the plant to tolerate drought stress (Dastogeer et al., 2020; Table 2).
Under low-water conditions, endophytic microorganisms associated with plants have the potential to enhance resistance by maintaining water status, ion homeostasis, and nutrient uptake to induce oxidative stress and enhance stress tolerance.
Phyllosphere microbes are present above the ground surface of plants. These bacterial strains can promote plant growth (Figure 4). In one study, rice plant seedlings were selected and subjected to drought stress, and the plants were inoculated with the phyllosphere bacterial strains PB50, PB46, and PB3 based on their significant plant-growth-promoting activities in PEG 6000 plants subjected to drought (Arun et al., 2020). Growth attributes, such as root and shoot length, leaf area, and stem extension, were measured, and the biochemical analysis of the plants revealed their remarkable potential to solubilize important nutrients, including potassium, phosphorus, and zinc. Nutrient availability enables plant hydration under drought stress. In addition, the biochemical analysis revealed an increase in the levels of exopolysaccharides, phytohormones, soluble sugars, chlorophyll, total protein, and sugars, which improved plant survival under conditions of water constraints (Arun et al., 2020). In another study (Devarajan et al., 2021), rice phyllosphere bacterial strains (e.g., Bacillus endophyticus PB3, Bacillus altitudinis PB46, and Bacillus megaterium PB50) were inoculated into Oryza sativa pots in a greenhouse experiment. Drought tolerance was moderately minimized in strain PB50, resulting in significant tolerance to drought. Additionally, biochemical and morphological traits were observed and compared; inoculated plants showed higher expression of LEA, SNAC1, HSP70, RAB16B, and bZIP23 as well as osmolyte accumulation, which helped combat drought (Devarajan et al., 2021; Table 3).
These beneficial microorganisms reside on the ground surface of plants. Phyllosphere microbiota enhance drought tolerance by promoting plant growth and protecting plants.
Plant-growth-promoting rhizobacteria can ameliorate drought stress and improve agronomic sustainability (Kanwal et al., 2017; Vanamala et al., 2021; Aslam et al., 2022). PGPR alleviate drought stress via rhizobacteria-induced drought endurance and resilience (RIDER), which induces biochemical changes (Rubin et al., 2017; Khan et al., 2018; Ansari and Ahmad, 2019). Various RIDER mechanisms include optimization of the antioxidant defense system, bacterial exopolysaccharides, phytohormone production, and cyclic metabolic pathway conventions that are involved in the deposition of several carbon-based components (Figure 1), such as sugars, amino acids, and polyamines, as well as the production of heat-shock proteins (Figueiredo et al., 2010; Monteiro et al., 2021; Sinha et al., 2021).
Plant-growth-promoting rhizobacteria can effectively mitigate drought stress in wheat and Zea mays grass. In one study (Jochum et al., 2019), two microbial strains, namely, Bacillus sp. 12D6 and Enterobacter sp. 16i, were used to combat drought stress. Plant growth attributes, hormones, and metabolite contents were analyzed. The results suggest that Bacillus sp. 12D6 was more effective in mitigating drought stress by increasing the root length, surface area, and plant productivity. The phytohormones IAA and salicylic acid were also found to be partly responsible for drought stress tolerance (Jochum et al., 2019). Bano et al. (2013) inoculated Azospirillum (GQ255950) into Z. mays plants. Microbes were isolated from water-restricted environments and inoculated into plants during the vegetative phase. After inoculation, the plants were subjected to drought conditions, and their growth attributes were recorded. Inoculation increased the root length, fresh biomass, and shoot length by 2.69–9.70%. Inoculation also increased the levels of proline, soluble sugars (63.15%), amino acids (54.54%), and osmoticum. Overall, inoculated plants can cope better with drought stress than uninoculated plants (Bano et al., 2013).
Drought is an important biotic stress factor that negatively affects crop yield. In a previous study, wheat plants were inoculated with Pseudomonas libanensis EU-LWNA-33, and the measurement of growth attributes revealed that the root length and biomass increased following inoculation. Furthermore, the biochemical analysis showed that proline levels increased by up to twofold, whereas glycine betaine levels increased by up to 1.2-fold at 75% stress. Proline and glycine betaine levels affect osmoregulation, as well as the solubilization and uptake of phosphorus, which is an important macronutrient with limited availability in plants. The inoculated strains effectively solubilized phosphorus (Kour et al., 2020b).
Drought stress can alter microbial interactions; for example, in a greenhouse experiment, three types of soil obtained from California valley rice fields were subjected to drought stress (Santos-Medellín et al., 2017). Water restriction altered the microbial interactions in all soil types. The altered patterns comprised enriched Actinobacteria and Chloroflexi and loss of Acidobacteria and Deltaproteobacteria. Compartment-specific restructuring results in plant survival under drought conditions (Santos-Medellín et al., 2017).
Plant-growth-promoting bacteria (PGPB) can help mitigate drought stress in various ways. Phytohormone production, 1-aminocyclopropane-1-carboxylate deaminase exopolysaccharide activation, solute production, chlorophyll synthesis, and increased mineral solubilization can help reduce stress. Such bacteria are safe, eco-friendly, and help sustain drought-tolerant crops (Priyanka et al., 2019). In a previous study (Chiappero et al., 2019), PGPR were isolated from soil, inoculated on Lysogeny broth (LB) medium plates, and cultured for 24 h in an incubator. After 1 day, the plate media contained colonies of Pseudomonas fluorescens WCS417r and Bacillus amyloliquefaciens GB03, both of which were evaluated for their effects on drought stress. Peppermint was selected, drought stress was induced, and plants were inoculated with microbes. The results demonstrated that drought stress was significantly reduced by the upregulation of the antioxidant defense system. Bioassay results revealed the hallmarks of phenolic components (Chiappero et al., 2019). In addition, the roots of Acacia arabica plants have been shown to be enriched with rhizosphere bacteria.
Plant-growth-promoting rhizobacteria such as Bacillus, Enterobacter, Moraxella, and Pseudomonas have been isolated and inoculated into drought-stressed wheat plants. The analysis of physiological and biochemical markers in these plants revealed increased levels of auxin, which help plants mitigate stress and enhance drought tolerance, thereby overcoming drought-related crop loss. Bacillus species can significantly improve auxin production to 25.9 μg ml–1, which, in turn, improves field capacity by 10% and crop yield by 34% (Raheem et al., 2018). PGPR strains such as Pseudomonas putida (i.e., NBRIRA) and B. amyloliquefaciens (i.e., NBRISN13) have been isolated from alkaline soils in Uttar Pradesh, India (Kumar et al., 2016). These two strains were simultaneously inoculated into chickpea plants to observe their combined effects in in vivo greenhouse and in vitro experiments. Their combination in plants led to an increased chlorophyll content, photosynthesis, osmolyte content, and biomass compared with those in single-strain treatments. Thus, PGPB are ecofriendly biofertilizers that can alleviate drought stress (Kumar et al., 2016). Various studies on several microbes have provided a basis for studies on microbial interactions. These studies have investigated the dynamics of microbial interactions to reveal their combined effects on stress tolerance in plants. In a field study, Azospirillum brasilense Sp245 was inoculated into wheat seedlings, and comparisons were made between inoculated and uninoculated seedlings (Creus et al., 2004). The inoculated seeds contained a higher mineral content, with increased levels of Mg, K, and Ca, and showed improved water regulation. Overall, a 12.4% improvement in grain yield was observed after inoculation.
Actinomycetes are Gram-positive anaerobic unicellular microbes that mitigate drought stress. However, research on this topic is scarce. In a previous study involving Actinomycetes, Streptomyces pactum Act12 was inoculated into the wheat plant cultivar Xinong 979 (Li H. et al., 2020). This inoculation significantly increased the overexpression of EXPA6, P5CS, EXPA2, and SnRK2 and increased the root length by 13.6%, shoot length by 10.3%, and fresh biomass by 21.3%. Chemical assays also revealed that the exposed seedlings showed stress reduction through an increase in the levels of sugars and antioxidant enzymes.
Water shortages can be a major challenge in agricultural systems because they negatively affect the quality and quantity of crops. An experiment was conducted on foxtail millet crops that were subjected to drought stress, followed by inoculation with Acinetobacter calcoaceticus EU-LRNA-72 and Penicillium sp. EU-FTF-6 (Kour et al., 2020c). Plant growth attributes were measured, which showed mitigation of drought stress and physiological growth due to inoculation. In this study, drought stress was mitigated by the accumulation of osmolytes, proline, and glycine betaine, as well as by increased levels of chlorophyll a and b. An increased chlorophyll content resulted in plant growth and development, whereas increased proline and glycine betaine levels improved osmotic adjustment and membrane integrity, respectively. These results demonstrated that inoculation with microbes efficiently combats drought stress (Kour et al., 2020c). In a randomized controlled trial (Martins et al., 2018), soybean was inoculated with Bacillus subtilis (i.e., UFGS1), Bacillus thuringiensis (i.e., UFGS2 and UFGRB2), and Bacillus cereus (i.e., UFGRB3) strains. The strains were selected based on their growth under water stress on media plates. B. thuringiensis UFGRB2 maintained the quantum efficiency of PSII; B. thuringiensis and B. cereus maintained the photosynthetic rates of the plants, while B. thuringiensis sustained the transpiration rate and stomatal conductance. These physiological features demonstrate the improved growth observed in inoculated plants compared with that of uninoculated plants. Additionally, the genomic analysis showed that overexpression of Gmdreb1a contributed to drought stress tolerance (Martins et al., 2018; Table 4).
Plant-growth-promoting rhizobacteria enhance the growth, development, and disease resistance of plants through a wide range of mechanisms. These are the most common microbes that mitigate drought stress. They secrete compounds of valuable potential biostimulants and play a pivotal role in plant stress responses.
To sustain biofertilization in India, an attempt was made to isolate and inoculate a methylotrophic bacterial community (e.g., Methylarcula, Methyloferula, Methylobacterium, Methylotenera, Methylobacillus, Methylophilus, Methylocapsa, Methylocella, Methylohalomonas, Methylomonas, Methylopila, Methylosinus, and Methylovirgula) (Kumar et al., 2019). Bacteria were isolated from the phyllospheres of cotton, maize, earthworms, and rhizosphere soil in Tamil Nadu, India. Methylotrophs increased the levels of auxin and cytokines, and the increased levels of phytohormones promoted germination and growth. In addition to increasing the ACC deaminase activity, these bacteria enhance nitrogen fixation and mineral solubilization. The novelty of this microbial community is that it is composed of type 1 and type 2 methanotrophs that have the potential to tolerate stress (Kumar et al., 2019). In another study (Danish et al., 2020), rhizosphere bacteria were collected from Z. mays and reinoculated into plants; data (submitted to National Center for Biotechnology Information (NCBI)) indicated the presence of Pseudomonas aeruginosa, Enterobacter cloacae, Achromobacter xylosoxidans, and Leclercia adecarboxylata. Additionally, bioassays demonstrated the significant involvement of ACC deaminase. Among these microbes, E. cloacae significantly increased grain yield by up to 73% owing to the ACC deaminase activity (Table 5).
Methanotrophs metabolize methane from carbon molecules and produce sulfate, nitrate, and oxidation energy. They require only a single carbon atom for survival; therefore, their survival rate is higher.
Mycorrhizae are microbial symbiotes that maintain a symbiotic association with plants. They are host-specific and have interspecific functionality (Sheteiwy et al., 2021). Arbuscular mycorrhizae facilitate host plant survival by increasing water and nutrient absorption from the rhizosphere (Agapitos et al., 2008), whereas endophytes do so by providing phytohormones and inducing defense-related secondary metabolism within the plants (Barman et al., 2016; Cheng et al., 2021; Kozjek et al., 2021).
In a study on soybean, which is highly sensitive to abiotic stress, plants were inoculated with arbuscular mycorrhizal fungi (AMF); the isolates were obtained from Argentina (Grümberg et al., 2015). After inoculation, the plants were subjected to drought conditions, and their physiological, biochemical, and molecular characteristics were evaluated. The quantitative analysis showed increased levels of proline, soluble sugars, and glycine betaine and reduced levels of MDA. MDA is a product of polyunsaturated fatty acids in cells and serves as the gold standard for identifying oxidative stress. The fungal strains Septoglomus constrictum, Glomus sp., and Glomus aggregatum effectively increase the levels of osmoprotectants in plant leaves and provide potential protection against drought stress (Grümberg et al., 2015).
Apart from abiotic stress, drought stress is the leading cause of plant diseases and limits crop production. In a randomized trial conducted by Cheng et al. (2021), Funneliformis mosseae was used to colonize trifoliate orange. After treatment for 8 weeks, a dramatic increase was observed in the leaf number, leaf area, stem elongation, and root microenvironment of the plants. Additionally, the qualitative analysis revealed increased phenolic, coumarin, and terpene contents in the root exudates. Phenolic components are key elements of drought stress tolerance, as they reduce oxidative stress (Cheng et al., 2021). In another study, two cultivars of wheat bread and durum wheat were selected and inoculated with Glomus mosseae, a strain of mycorrhizal fungi, and then subjected to water restriction (Bernardo et al., 2017). In a randomized controlled trial, analyses of growth parameters and proteomics were conducted to determine the mechanisms of drought tolerance. The results suggest that inoculation increased the accumulation of aboveground dry biomass. Furthermore, an increased genotype diversity is related to stress tolerance. Various genes modulate the signaling pathways of sulfur and oxylipin metabolism, which inhibits ethylene formation. Ethylene causes plant stress and damages cell integrity and osmotic pressure. Downregulation of 6-SFT expression was also noted. The upregulation of osmolytes in the cellular components of plants was significant, indicating that oxidation–reduction mechanism is associated with drought tolerance (Bernardo et al., 2017).
Microbes can be ecofriendly and aid in stress tolerance in plants. Drought stress involves a cascade of events that cause oxidative damage. Thus, the plant antioxidant defense system is activated by the induction of gene expression to combat ROS. For example, inoculation of two AMF strains of Gigaspora margarita and Glomus intraradices in host plants resulted in the expression of GmarCuZnSOD, GintPDX1, GintMT1, and GintSOD. In addition, the mechanism for tolerating drought stress involves the reduction of cytoplasmic protein levels and regulation of redox status via pyridoxamine synthesis (Zou et al., 2021).
Drought stress negatively affects the crop quality and quantity. However, this can be mitigated through the inoculation of three species of AMF, namely, G. mosseae, Glomus etunicatum, and G. intraradices. Al-Arjani et al. (2020) isolated these three species from the rhizosphere of Acacia gerrardii and applied them to Ephedra foliata Boiss plants. Consequently, plants were preserved under restricted water conditions (used to induce drought stress). Specifically, compared with control plants, the inoculated plants were more likely to show photoassimilation, which increased the chlorophyll and carotenoid contents. Additionally, increased levels of sucrose-phosphate synthase were observed in the carbon pool. Furthermore, increased osmolytes are among other factors attributed to drought stress tolerance (Al-Arjani et al., 2020). In another study, AMF and silicon were inoculated with 1-week-old strawberry seedlings to observe their synergistic effects against drought stress (Moradtalab et al., 2019). Following a randomized trial over 4 weeks, AMF and silicone were found to increase the biomass, water uptake, and mineral content and promote the antioxidant defense system. Thus, the two are beneficial for combating drought stress when combined (Moradtalab et al., 2019; Table 6).
Mycorrhiza form a symbiotic relationship between fungi and plants. The term mycorrhiza refers to the role of the fungus in the plant axis; mycorrhizae play a significant role in plant growth, nutrition, soil biochemistry, and stress tolerance.
There is an increasing trend in publications related to this topic, which will likely continue to increase owing to the need to identify adaptive solutions that can determine the effects of climate change on crops. Notably, several studies have used a mixture of diverse microbial strains, and it has been speculated that a consortium would be more archetypal of the original microbiome, which would include numerous strains that can provide distinctive synergistic benefits compared with individual strains (Figure-5).
Plants have evolved in a sophisticated manner with microbes to overcome drought stress and its adverse effects within cells. Microbes strengthen the intrinsic stress tolerance mechanisms of plants by producing exopolysaccharides, phytohormones, ACC deaminase, osmolytes, and volatile compounds. In addition, cells synthesize diverse molecules that regulate redox damage and overcome antioxidant damage.
This review highlights a variety of adverse effects of drought stress on plant growth and reveals how such effects can be mitigated using microbes. Previous reviews have focused on drought mitigation by using a group of microbes. However, this review discusses a variety of microbes that mitigate drought stress, their fundamental mechanisms of action, and the changes that they produce at the molecular level. Microbial intervention has led to substantial progress in drought tolerance. Future investigations should evaluate whether acute and chronic effects are beneficial to plant phenotypes. Although known microbial interventions are promising, their dose, frequency, and timing warrant practical validation.
SS and MAK contributed to the original draft writing. MI and S-MK helped with review and graphical representation. Y-SP and SHW helped in formatting the manuscript. I-JL helped with funding. All authors contributed to the article and approved the submitted version.
This work was supported by the National Research Foundation of Korea (NRF) grant funded by the Korean government (MSIT) (No. 2022R1A2C1008993).
The authors declare that the research was conducted in the absence of any commercial or financial relationships that could be construed as a potential conflict of interest.
All claims expressed in this article are solely those of the authors and do not necessarily represent those of their affiliated organizations, or those of the publisher, the editors and the reviewers. Any product that may be evaluated in this article, or claim that may be made by its manufacturer, is not guaranteed or endorsed by the publisher.
AMF, Arbuscular mycorrhizal fungi; MDA, Malondialdehyde; LB, Lysogeny broth; PGPR, Plant-growth-promoting rhizobacteria; PGPB, Plant-growth-promoting bacteria; RIDER, Rhizobacteria-induced drought endurance and resilience; SOD, Superoxide dismutase; ROS, Reactive oxygen species; TPP, Trehalose-6-phosphate phosphatases; ABA, Abscisic acid; IAA, Indole acetic acid; ACC, Aminocyclopropane-1-carboxylic acid; VOC, Volatile oil compounds.
Agapitos, A., Togelius, J., Lucas, S. M., Schmidhuber, J., and Konstantinidis, A. (2008). “Generating diverse opponents with multiobjective evolution,” in Proceedings of the IEEE Symposium On Computational Intelligence and Games, 2008. CIG’08, (Piscataway, NJ: IEEE), 135–142.
Ahmad, A., Aslam, Z., Naz, M., Hussain, S., Javed, T., Aslam, S., et al. (2021). Exogenous salicylic acid-induced drought stress tolerance in wheat (Triticum aestivum L.) grown under hydroponic culture. PLoS One 16:e0260556. doi: 10.1371/journal.pone.0260556
Ahmad, P., Jamsheed, S., Hameed, A., Rasool, S., Sharma, I., Azooz, M., et al. (2014). “Drought stress induced oxidative damage and antioxidants in plants,” in Oxidative Damage to Plants, ed. P. Ahmad (New York, NY: Elsevier), 345–367.
Alagoz, Y., Nayak, P., Dhami, N., and Cazzonelli, C. I. (2018). Cis-carotene biosynthesis, evolution and regulation in plants: the emergence of novel signaling metabolites. Arch. Biochem. Biophys. 654, 172–184. doi: 10.1016/j.abb.2018.07.014
Al-Arjani, A.-B. F., Hashem, A., and Abd_Allah, E. F. (2020). Arbuscular mycorrhizal fungi modulates dynamics tolerance expression to mitigate drought stress in Ephedra foliata Boiss. Saudi J. Biol. Sci. 27, 380–394. doi: 10.1016/j.sjbs.2019.10.008
Ali, F., Bano, A., and Fazal, A. (2017). Recent methods of drought stress tolerance in plants. Plant Growth Regul. 82, 363–375. doi: 10.1007/s10725-017-0267-2
Anjum, S. A., Xie, X.-Y., Wang, L.-C., Saleem, M. F., Man, C., and Lei, W. (2011). Morphological, physiological and biochemical responses of plants to drought stress. Afr. J. Agricult. Res. 6, 2026–2032.
Ansari, F. A., and Ahmad, I. (2019). “Alleviating drought stress of crops through PGPR: mechanism and application,” in Microbial Interventions in Agriculture and Environment, eds D. P. Singh, V. K. Gupta, and R. Prabha (Singapore: Springer), 341–358. doi: 10.1007/978-981-13-8383-0_11
Arun, K. D., Sabarinathan, K. G., Gomathy, M., Kannan, R., and Balachandar, D. (2020). Mitigation of drought stress in rice crop with plant growth-promoting abiotic stress-tolerant rice phyllosphere bacteria. J. Basic Microbiol. 60, 768–786. doi: 10.1002/jobm.202000011
Askari-Khorasgani, O., Pardo, F. B. F., and Pessarakli, M. (2021). “Drought stress sensing-signaling in plants,” in Handbook of Plant and Crop Physiology, ed. M. Pessarakli (Boca Raton, FL: CRC Press), 465–481. doi: 10.1201/9781003093640-30
Aslam, M. M., Idris, A. L., Zhang, Q., Weifeng, X., Karanja, J. K., and Wei, Y. (2022). Rhizosphere microbiomes can regulate plant drought tolerance. Pedosphere 32, 61–74. doi: 10.1016/s1002-0160(21)60061-9
Banfield, J. F., and Nealson, K. H. (2018). Geomicrobiology: Interactions between Microbes and Minerals. Berlin: Walter de Gruyter GmbH & Co KG.
Bano, Q., Ilyas, N., Bano, A., Zafar, N., Akram, A., and Hassan, F. (2013). Effect of Azospirillum inoculation on maize (Zea mays L.) under drought stress. Pak. J. Bot. 45, 13–20.
Bartels, D., and Phillips, J. (2010). “Drought stress tolerance,” in Genetic Modification of Plants, eds F. Kempken and C. Jung (Berlin: Springer), 139–157.
Basu, S., Ramegowda, V., Kumar, A., and Pereira, A. (2016). Plant adaptation to drought stress. F1000Research 5:1554. doi: 10.12688/f1000research.7678.1
Berg, G. (2009). Plant–microbe interactions promoting plant growth and health: perspectives for controlled use of microorganisms in agriculture. Appl. Microbiol. Biotechnol. 84, 11–18. doi: 10.1007/s00253-009-2092-7
Bernardo, L., Morcia, C., Carletti, P., Ghizzoni, R., Badeck, F. W., Rizza, F., et al. (2017). Proteomic insight into the mitigation of wheat root drought stress by arbuscular mycorrhizae. J. Proteomics 169, 21–32. doi: 10.1016/j.jprot.2017.03.024
Bhagat, N., Raghav, M., Dubey, S., and Bedi, N. (2021). Bacterial exopolysaccharides: insight into their role in plant abiotic stress tolerance. J. Microbiol. Biotechnol. 31, 1045–1059. doi: 10.4014/jmb.2105.05009
Bilal, S., Shahzad, R., Imran, M., Jan, R., Kim, K. M., and Lee, I.-J. (2020). Synergistic association of endophytic fungi enhances Glycine max L. resilience to combined abiotic stresses: heavy metals, high temperature and drought stress. Industr. Crops Prod. 143:111931. doi: 10.1016/j.indcrop.2019.111931
Bright, J., Desikan, R., Hancock, J. T., Weir, I. S., and Neill, S. J. (2006). ABA-induced NO generation and stomatal closure in Arabidopsis are dependent on H2O2 synthesis. Plant J. 45, 113–122. doi: 10.1111/j.1365-313X.2005.02615.x
Campbell, B. G. (1995). Isolation and Molecular Characterisation of Two Pseudomonas sp. ACC Deaminase Genes. Cape Town: University of Cape Town.
Chai, T.-T., Fadzillah, N., Kusnan, M., and Mahmood, M. (2005). Water stress-induced oxidative damage and antioxidant responses in micropropagated banana plantlets. Biol. Plant. 49, 153–156. doi: 10.1007/s00000-005-3156-9
Chen, H., and Jiang, J.-G. (2010). Osmotic adjustment and plant adaptation to environmental changes related to drought and salinity. Environ. Rev. 18, 309–319. doi: 10.1139/a10-014
Cheng, H.-Q., Giri, B., Wu, Q.-S., Zou, Y.-N., and Kuèa, K. (2021). Arbuscular mycorrhizal fungi mitigate drought stress in citrus by modulating root microenvironment. Arch. Agron. Soil Sci. Online ahead of Print,
Chiappero, J., Del Rosario Cappellari, L., Alderete, L. G. S., Palermo, T. B., and Banchio, E. (2019). Plant growth promoting rhizobacteria improve the antioxidant status in Mentha piperita grown under drought stress leading to an enhancement of plant growth and total phenolic content. Industr. Crops Prod. 139:111553. doi: 10.1016/j.indcrop.2019.111553
Choudhary, D. K., Varma, A., and Tuteja, N. (2016). Plant-Microbe Interaction: An Approach to Sustainable Agriculture. Berlin: Springer.
Creus, C. M., Sueldo, R. J., and Barassi, C. A. (2004). Water relations and yield in Azospirillum-inoculated wheat exposed to drought in the field. Can. J. Bot. 82, 273–281. doi: 10.1139/b03-119
Danish, S., Zafar-Ul-Hye, M., Mohsin, F., and Hussain, M. (2020). ACC-deaminase producing plant growth promoting rhizobacteria and biochar mitigate adverse effects of drought stress on maize growth. PLoS One 15:e0230615. doi: 10.1371/journal.pone.0230615
Dastogeer, K. M. G., Chakraborty, A., Sarker, M. S. A., and Akter, M. A. (2020). Roles of fungal endophytes and viruses in mediating drought stress tolerance in plants. Int. J. Agricult. Biol. 24, 1497–1512.
Davies, P. J. (1995). “The plant hormone concept: concentration, sensitivity and transport,” in Plant Hormones Davies, ed. P. J. Davies (Dordrecht: Springer), 13–38. doi: 10.1007/978-94-011-0473-9_2
Devarajan, A. K., Muthukrishanan, G., Truu, J., Truu, M., Ostonen, I., Panneerselvam, P., et al. (2021). The foliar application of rice phyllosphere bacteria induces drought-stress tolerance in Oryza sativa (L.). Plants 10:387. doi: 10.3390/plants10020387
Dikarev, A., Dikarev, V., Geras’kin, S. A., and Dikareva, N. (2016). Plant tolerance. Biochem. Physiol. Traits 51, 89–99.
Dobra, J., Motyka, V., Dobrev, P., Malbeck, J., Prasil, I. T., Haisel, D., et al. (2010). Comparison of hormonal responses to heat, drought and combined stress in tobacco plants with elevated proline content. J. Plant Physiol. 167, 1360–1370.
Fahad, S., Nie, L., Chen, Y., Wu, C., Xiong, D., Saud, S., et al. (2015). “Crop plant hormones and environmental stress,” in Sustainable Agriculture Reviews, ed. E. Lichtfouse (Cham: Springer), 371–400. doi: 10.1007/978-3-319-09132-7_10
Farooq, M., Hussain, M., Wahid, A., and Siddique, K. (2012). “Drought stress in plants: an overview,” in Plant Responses to Drought Stress, ed. R. Aroca (Berlin: Springer), 1–33.
Farooq, M., Wahid, A., Kobayashi, N., Fujita, D., and Basra, S. (2009). Plant drought stress: effects, mechanisms and management. Sustain. Agricult. 29, 153–188.
Figueiredo, M. D. V. B., Seldin, L., De Araujo, F. F., and Mariano, R. D. L. R. (2010). “Plant growth promoting rhizobacteria: fundamentals and applications,” in Plant Growth and Health Promoting Bacteria, ed. D. Maheshwari (Berlin: Springer), 21–43. doi: 10.1007/978-3-642-13612-2_2
Filgueiras, L., Silva, R., Almeida, I., Vidal, M., Baldani, J. I., and Meneses, C. H. S. G. (2020). Gluconacetobacter diazotrophicus mitigates drought stress in Oryza sativa L. Plant Soil 451, 57–73. doi: 10.1007/s11104-019-04163-1
Grümberg, B. C., Urcelay, C., Shroeder, M. A., Vargas-Gil, S., and Luna, C. M. (2015). The role of inoculum identity in drought stress mitigation by arbuscular mycorrhizal fungi in soybean. Biol. Fertil. Soils 51, 1–10. doi: 10.1007/s00374-014-0942-7
Hossain, M. A., Wani, S. H., Bhattacharjee, S., Burritt, D. J., and Tran, L.-S. P. (2016). Drought Stress Tolerance in Plants, Vol 2: Molecular and Genetic Perspectives. Berlin: Springer.
Houda, T., Issam, B. S., and Naïma, K. B. (2013). Effectiveness of the plant growth-promoting rhizobacterium Pantoea sp. Soil Sci. 158, 442–453.
Hupfauf, S., Bachmann, S., Juárez, M. F.-D., Insam, H., and Eichler-Löbermann, B. (2016). Biogas digestates affect crop P uptake and soil microbial community composition. Sci. Total Environ. 542, 1144–1154. doi: 10.1016/j.scitotenv.2015.09.025
Ilyas, M., Nisar, M., Khan, N., Hazrat, A., Khan, A. H., Hayat, K., et al. (2021). Drought tolerance strategies in plants: a mechanistic approach. J. Plant Growth Regul. 40, 926–944.
Ilyas, N., Mumtaz, K., Akhtar, N., Yasmin, H., Sayyed, R., Khan, W., et al. (2020). Exopolysaccharides producing bacteria for the amelioration of drought stress in wheat. Sustainability 12:8876. doi: 10.3390/su12218876
Iordachescu, M., and Imai, R. (2011). “Trehalose and abiotic stress in biological systems,” in Abiotic Stress in Plants-Mechanisms and Adaptations, eds A. K. Shanker and B. Venkateswarlu (Croatia: Intech), 215–234.
Jain, D., Phurailatpam, L., and Mishra, S. (2020). “Microbes-mediated mitigation of drought stress in plants: recent trends and future challenges,” in Advances in Plant Microbiome and Sustainable Agriculture, eds A. Yadav, A. Rastegari, N. Yadav, and D. Kour (Singapore: Springer), 199–218. doi: 10.1007/978-981-15-3204-7_9
Jeon, J.-S., Lee, S.-S., Kim, H.-Y., Ahn, T.-S., and Song, H.-G. (2003). Plant growth promotion in soil by some inoculated microorganisms. J. Microbiol. 41, 271–276.
Jochum, M. D., Mcwilliams, K. L., Borrego, E. J., Kolomiets, M. V., Niu, G., Pierson, E. A., et al. (2019). Bioprospecting plant growth-promoting rhizobacteria that mitigate drought stress in grasses. Front. Microbiol. 10:2106. doi: 10.3389/fmicb.2019.02106
Jogawat, A., Yadav, B., Lakra, N., Singh, A. K., and Narayan, O. P. (2021). Crosstalk between phytohormones and secondary metabolites in the drought stress tolerance of crop plants: a review. Physiol. Plant. 172, 1106–1132. doi: 10.1111/ppl.13328
Kahraman, M., Sevim, G., and Bor, M. (2019). “The role of proline, glycinebetaine, and trehalose in stress-responsive gene expression,” in Osmoprotectant-Mediated Abiotic Stress Tolerance in Plants, eds M. Hossain, V. Kumar, D. Burritt, M. Fujita, and P. Mäkelä (Cham: Springer), 241–256. doi: 10.1007/978-3-030-27423-8_11
Kanellis, A., Chang, C., Klee, H., Bleecker, A., Pech, J., and Grierson, D. (2012). Biology and Biotechnology of the Plant Hormone Ethylene II. Berlin: Springer Science & Business Media.
Kang, S.-M., Khan, M.-A., Hamayun, M., Kim, L.-R., Kwon, E.-H., Kang, Y.-S., et al. (2021). Phosphate-Solubilizing Enterobacter ludwigii AFFR02 and Bacillus megaterium Mj1212 rescues Alfalfa’s growth under post-drought stress. Agriculture 11:485. doi: 10.3390/agriculture11060485
Kanwal, S., Ilyas, N., Batool, N., and Arshad, M. (2017). Amelioration of drought stress in wheat by combined application of PGPR, compost, and mineral fertilizer. J. Plant Nutr. 40, 1250–1260. doi: 10.1080/01904167.2016.1263322
Kapadia, C., Sayyed, R., El Enshasy, H. A., Vaidya, H., Sharma, D., Patel, N., et al. (2021). Halotolerant microbial consortia for sustainable mitigation of salinity stress, growth promotion, and mineral uptake in tomato plants and soil nutrient enrichment. Sustainability 13:8369. doi: 10.3390/su13158369
Kaushal, M., and Wani, S. P. (2016). Plant-growth-promoting rhizobacteria: drought stress alleviators to ameliorate crop production in drylands. Ann. Microbiol. 66, 35–42. doi: 10.1007/s13213-015-1112-3
Kavar, T., Maras, M., Kidriè, M., Šuštar-Vozliè, J., and Megliè, V. (2008). Identification of genes involved in the response of leaves of Phaseolus vulgaris to drought stress. Mol. Breed. 21, 159–172. doi: 10.1007/s11032-007-9116-8
Kefeli, V. I., Kefeli, V., Kalevitch, M., and Borsari, B. (2003). Natural Growth Inhibitors and Phytohormones in Plants and Environment. Berlin: Springer Science & Business Media.
Khan, N. A., Nazar, R., Iqbal, N., and Anjum, N. A. (2012). Phytohormones and Abiotic Stress Tolerance in Plants. Berlin: Springer Science & Business Media.
Khan, N., Bano, A., Shahid, M. A., Nasim, W., and Babar, M. A. (2018). Interaction between PGPR and PGR for water conservation and plant growth attributes under drought condition. Biologia 73, 1083–1098. doi: 10.2478/s11756-018-0127-1
Kim, Y.-N., Khan, M. A., Kang, S.-M., Hamayun, M., and Lee, I.-J. (2020). Enhancement of drought-stress tolerance of Brassica oleracea var. italica L. by newly isolated Variovorax sp. YNA59. J. Microbiol. Biotechnol. 30, 1500–1509. doi: 10.4014/jmb.2006.06010
Kirst, G. (1996). “Osmotic adjustment in phytoplankton and macroalgae,” in Biological and Environmental Chemistry of DMSP and Related Sulfonium Compounds, eds R. P. Kiene, P. T. Visscher, M. D. Keller, and G. O. Kirst (New York, NY: Springer), 121–129. doi: 10.1007/978-1-4613-0377-0_11
Kour, D., and Yadav, A. N. (2020). Microbe mediated mitigation of drought stress in crops. Agric. Lett. 1, 79–82.
Kour, D., Rana, K. L., Kaur, T., Sheikh, I., Yadav, A. N., Kumar, V., et al. (2020a). Microbe-mediated alleviation of drought stress and acquisition of phosphorus in great millet (Sorghum bicolour L.) by drought-adaptive and phosphorus-solubilizing microbes. Biocatal. Agricult. Biotechnol. 23:101501.
Kour, D., Rana, K. L., Sheikh, I., Kumar, V., Yadav, A. N., Dhaliwal, H. S., et al. (2020b). Alleviation of drought stress and plant growth promotion by Pseudomonas libanensis EU-LWNA-33, a drought-adaptive phosphorus-solubilizing bacterium. Proc. Natl. Acad. Sci. India B Biol. Sci. 90, 785–795. doi: 10.1007/s40011-019-01151-4
Kour, D., Rana, K. L., Yadav, A. N., Sheikh, I., Kumar, V., Dhaliwal, H. S., et al. (2020c). Amelioration of drought stress in Foxtail millet (Setaria italica L.) by P-solubilizing drought-tolerant microbes with multifarious plant growth promoting attributes. Environ. Sustainability 3, 23–34. doi: 10.1007/s42398-020-00094-1
Kozjek, K., Kundel, D., Kushwaha, S. K., Olsson, P. A., Ahrén, D., Fliessbach, A., et al. (2021). Long-term agricultural management impacts arbuscular mycorrhizal fungi more than short-term experimental drought. Appl. Soil Ecol. 168:104140. doi: 10.1016/j.apsoil.2021.104140
Kumar, M., Kour, D., Yadav, A. N., Saxena, R., Rai, P. K., Jyoti, A., et al. (2019). Biodiversity of methylotrophic microbial communities and their potential role in mitigation of abiotic stresses in plants. Biologia 74, 287–308. doi: 10.2478/s11756-019-00190-6
Kumar, M., Mishra, S., Dixit, V., Kumar, M., Agarwal, L., Chauhan, P. S., et al. (2016). Synergistic effect of Pseudomonas putida and Bacillus amyloliquefaciens ameliorates drought stress in chickpea (Cicer arietinum L.). Plant Signal. Behav. 11:e1071004. doi: 10.1080/15592324.2015.1071004
Li, B., Feng, Y., Zong, Y., Zhang, D., Hao, X., and Li, P. (2020). Elevated CO2-induced changes in photosynthesis, antioxidant enzymes and signal transduction enzyme of soybean under drought stress. Plant Physiol. Biochem. 154, 105–114. doi: 10.1016/j.plaphy.2020.05.039
Li, H., Guo, Q., Jing, Y., Liu, Z., Zheng, Z., Sun, Y., et al. (2020). Application of Streptomyces pactum Act12 enhances drought resistance in wheat. J. Plant Growth Regul. 39, 122–132. doi: 10.1007/s00344-019-09968-z
Li, X., and Liu, F. (2016). “Drought stress memory and drought stress tolerance in plants: biochemical and molecular basis,” in Drought Stress Tolerance in Plants, Vol 1, eds I. M. A. Hossain, S. H. Wani, S. Bhattacharjee, D. J. Burritt, and L.-S. P. Tran (Berlin: Springer), 17–44. doi: 10.1007/978-3-319-28899-4_2
Liu, X.-M., and Zhang, H. (2015). The effects of bacterial volatile emissions on plant abiotic stress tolerance. Front. Plant Sci. 6:774. doi: 10.3389/fpls.2015.00774
Martins, S. J., Rocha, G. A., De Melo, H. C., De Castro Georg, R., Ulhôa, C. J., De Campos Dianese, É, et al. (2018). Plant-associated bacteria mitigate drought stress in soybean. Environ. Sci. Pollut. Res. 25, 13676–13686. doi: 10.1007/s11356-018-1610-5
Meena, K. K., Bitla, U., and Sorty, A. M. (2021). “Root-endophytes and their contribution to plant abiotic stress tolerance,” in Microbial Management of Plant Stresses, eds A. Kumar and S. Droby (Elsevier), 119–129. doi: 10.1016/b978-0-323-85193-0.00002-4
Mohammadipanah, F., and Zamanzadeh, M. (2019). “Bacterial mechanisms promoting the tolerance to drought stress in plants,” in Secondary Metabolites of Plant Growth Promoting Rhizomicroorganisms, eds H. B. Singh, C. Keswani, M. S. Reddy, E. Sansinenea, and C. García-Estrada (Singapore: Springer), 185–224. doi: 10.1007/978-981-13-5862-3_10
Mohammadkhani, N., and Heidari, R. (2008). Drought-induced accumulation of soluble sugars and proline in two maize varieties. World Appl. Sci. J. 3, 448–453.
Mok, D. W., and Mok, M. C. (2001). Cytokinin metabolism and action. Annu. Rev. Plant Biol. 52, 89–118. doi: 10.1146/annurev.arplant.52.1.89
Mollah, A., and Pratiwi, E. (2020). Application of various types of local microorganisms to the growth and production of two rice varieties. IOP Conf. Ser. Earth Environ. Sci. 575:012148. doi: 10.1088/1755-1315/575/1/012148
Monteiro, G., Nogueira, G., Neto, C., Nascimento, V., and Freitas, J. (2021). “Promotion of nitrogen assimilation by plant growth-promoting rhizobacteria,” in Nitrogen in Agriculture-Physiological, Agricultural and Ecological Aspects, eds T. Ohyama and K. Inubushi (London: IntechOpen).
Moradtalab, N., Hajiboland, R., Aliasgharzad, N., Hartmann, T. E., and Neumann, G. (2019). Silicon and the association with an arbuscular-mycorrhizal fungus (Rhizophagus clarus) mitigate the adverse effects of drought stress on strawberry. Agronomy 9:41. doi: 10.3390/agronomy9010041
Mukerji, K. G., Manoharachary, C., and Singh, J. (2006). Microbial Activity in the Rhizosphere. Berlin: Springer Science & Business Media.
Nadeem, S. M., Ahmad, M., Tufail, M. A., Asghar, H. N., Nazli, F., and Zahir, Z. A. (2021). Appraising the potential of EPS-producing rhizobacteria with ACC-deaminase activity to improve growth and physiology of maize under drought stress. Physiol. Plant. 172, 463–476. doi: 10.1111/ppl.13212
Naseem, H., Ahsan, M., Shahid, M. A., and Khan, N. (2018). Exopolysaccharides producing rhizobacteria and their role in plant growth and drought tolerance. J. Basic microbiol. 58, 1009–1022. doi: 10.1002/jobm.201800309
Palmer, W. C. (1965). Meteorological Drought. Washington, DC: US Department of Commerce Weather Bureau.
Priyanka, J. P., Goral, R. T., Rupal, K. S., and Saraf, M. (2019). “Rhizospheric microflora: a natural alleviator of drought stress in agricultural crops,” in Plant Growth Promoting Rhizobacteria for Sustainable Stress Management, ed. R. Z. Sayyed (Singapore: Springer), 103–115. doi: 10.1007/978-981-13-6536-2_6
Rahdari, P., and Hoseini, S. (2012). Drought stress: a review. Int. J. Agron. Plant Production 3, 443–446.
Raheem, A., Shaposhnikov, A., Belimov, A. A., Dodd, I. C., and Ali, B. (2018). Auxin production by rhizobacteria was associated with improved yield of wheat (Triticum aestivum L.) under drought stress. Arch. Agron. Soil Sci. 64, 574–587. doi: 10.1080/03650340.2017.1362105
Rodriguez, R., White, J. Jr., Arnold, A., and Redman, A. R. A. (2009). Fungal endophytes: diversity and functional roles. N. Phytol. 182, 314–330. doi: 10.1111/j.1469-8137.2009.02773.x
Rubin, R. L., Van Groenigen, K. J., and Hungate, B. A. (2017). Plant growth promoting rhizobacteria are more effective under drought: a meta-analysis. Plant Soil 416, 309–323. doi: 10.1007/s11104-017-3199-8
Sakata, Y., Komatsu, K., and Takezawa, D. (2014). “ABA as a universal plant hormone,” in Progress in Botany, eds U. Lüttge, W. Beyschlag, and J. Cushman (Berlin: Springer), 57–96. doi: 10.1007/978-3-642-38797-5_2
Salehi-Lisar, S. Y., and Bakhshayeshan-Agdam, H. (2016). “Drought stress in plants: causes, consequences, and tolerance,” in Drought Stress Tolerance in Plants, Vol. 1, eds M. Hossain, S. Wani, S. Bhattacharjee, D. Burritt, and L. S. Tran (Cham: Springer), 1–16. doi: 10.1007/978-3-319-28899-4_1
Sandhya, V., Sk, Z. A., Grover, M., Reddy, G., and Venkateswarlu, B. (2009). Alleviation of drought stress effects in sunflower seedlings by the exopolysaccharides producing Pseudomonas putida strain GAP-P45. Biol. Fertil. Soils 46, 17–26. doi: 10.1007/s00374-009-0401-z
Santos-Medellín, C., Edwards, J., Liechty, Z., Nguyen, B., and Sundaresan, V. (2017). Drought stress results in a compartment-specific restructuring of the rice root-associated microbiomes. MBio 8:e00764-17. doi: 10.1128/mBio.00764-17
Seco, R., Karl, T., Guenther, A., Hosman, K. P., Pallardy, S. G., Gu, L., et al. (2015). Ecosystem-scale volatile organic compound fluxes during an extreme drought in a broadleaf temperate forest of the Missouri Ozarks (central USA). Glob. Change Biol. 21, 3657–3674. doi: 10.1111/gcb.12980
Seki, M., Umezawa, T., Urano, K., and Shinozaki, K. (2007). Regulatory metabolic networks in drought stress responses. Curr. Opin. Plant Biol. 10, 296–302. doi: 10.1016/j.pbi.2007.04.014
Sharma, V., Salwan, R., and Tawfeeq, L. (2020). Molecular Aspects of Plant Beneficial Microbes in Agriculture. Cambridge, MA: Academic Press.
Sheteiwy, M. S., Ali, D. F. I., Xiong, Y.-C., Brestic, M., Skalicky, M., Hamoud, Y. A., et al. (2021). Physiological and biochemical responses of soybean plants inoculated with Arbuscular mycorrhizal fungi and Bradyrhizobium under drought stress. BMC Plant Biol. 21:195. doi: 10.1186/s12870-021-02949-z
Shinozaki, K., and Yamaguchi-Shinozaki, K. (1998). Molecular Responses to Drought Stress. Amsterdam: Elsevier Science.
Shinozaki, K., and Yamaguchi-Shinozaki, K. (2007). Gene networks involved in drought stress response and tolerance. J. Exp. Bot. 58, 221–227. doi: 10.1093/jxb/erl164
Shinozaki, K., Urano, K., Maruyama, K., and Takahashi, F. (2016). Drought stress. Plant Physiol. Dev. 1, 8–15.
Siddique, M., Hamid, A., and Islam, M. (2000). Drought stress effects on water relations of wheat. Bot. Bull. Acad. Sin. 41, 35–39.
Siddiqui, M. H., Khan, M. N., and Singh, V. P. (2022). Hot and Dry: How Plants can Thrive in Future Climates. Berlin: Springer.
Silambarasan, S., Logeswari, P., Cornejo, P., Abraham, J., and Valentine, A. (2019). Simultaneous mitigation of aluminum, salinity and drought stress in Lactuca sativa growth via formulated plant growth promoting Rhodotorula mucilaginosa CAM4. Ecotoxicol. Environ. Saf. 180, 63–72. doi: 10.1016/j.ecoenv.2019.05.006
Singh, D. P., Singh, V., Gupta, V. K., Shukla, R., Prabha, R., Sarma, B. K., et al. (2020). Microbial inoculation in rice regulates antioxidative reactions and defense related genes to mitigate drought stress. Sci. Rep. 10:4818. doi: 10.1038/s41598-020-61140-w
Sinha, D., Mukherjee, S., and Mahapatra, D. (2021). “Multifaceted potential of Plant Growth Promoting Rhizobacteria (PGPR): an overview,” in Handbook of Research on Microbial Remediation and Microbial Biotechnology for Sustainable Soil, ed. J. Ahmad Malik (Pennsylvania: IGI Global), 205–268. doi: 10.4018/978-1-7998-7062-3.ch008
Skubacz, A., Daszkowska-Golec, A., and Szarejko, I. (2016). The role and regulation of ABI5 (ABA-Insensitive 5) in plant development, abiotic stress responses and phytohormone crosstalk. Front. Plant Sci. 7:1884. doi: 10.3389/fpls.2016.01884
Strobel, G. A. (2003). Endophytes as sources of bioactive products. Microbes Infect. 5, 535–544. doi: 10.1016/s1286-4579(03)00073-x
Svoboda, M., Lecomte, D., Hayes, M., Heim, R., Gleason, K., Angel, J., et al. (2002). The drought monitor. Bull. Am. Meteorol. Soc. 83, 1181–1190.
Tambussi, E. A., Bartoli, C. G., Beltrano, J., Guiamet, J. J., and Araus, J. L. (2000). Oxidative damage to thylakoid proteins in water-stressed leaves of wheat (Triticum aestivum). Physiol. Plant. 108, 398–404. doi: 10.1034/j.1399-3054.2000.108004398.x
Teotia, P., Kumar, V., Kumar, M., Shrivastava, N., and Varma, A. (2016). “Rhizosphere microbes: potassium solubilization and crop productivity–present and future aspects,” in Potassium Solubilizing Microorganisms for Sustainable Agriculture, eds V. S. Meena, B. R. Maurya, J. P. Verma, and R. S. Meena (Berlin: Springer), 315–325. doi: 10.1007/978-81-322-2776-2_22
Ullah, A., Nisar, M., Ali, H., Hazrat, A., Hayat, K., Keerio, A. A., et al. (2019). Drought tolerance improvement in plants: an endophytic bacterial approach. Appl. Microbiol. Biotechnol. 103, 7385–7397. doi: 10.1007/s00253-019-10045-4
Ustiatik, R., Nuraini, Y., Suharjono, S., Jeyakumar, P., Anderson, C. W. N., and Handayanto, E. (2021). Mercury resistance and plant growth promoting traits of endophytic bacteria isolated from mercury-contaminated soil. Bioremediat. J. 1–20. doi: 10.1080/10889868.2021.1973950
Van Loon, A. F. (2015). Hydrological drought explained. Wiley Interdiscip. Rev. 2, 359–392. doi: 10.1002/wat2.1085
Vanamala, P., Sultana, U., Sindhura, P., and Gul, M. Z. (2021). “Plant Growth-Promoting Rhizobacteria (PGPR): a unique strategy for sustainable agriculture,” in Handbook of Research on Microbial Remediation and Microbial Biotechnology for Sustainable Soil, ed. J. Ahmad Malik (Pennsylvania: IGI Global), 332–357. doi: 10.4018/978-1-7998-7062-3.ch012
Vanneste, S., and Friml, J. (2009). Auxin: a trigger for change in plant development. Cell 136, 1005–1016. doi: 10.1016/j.cell.2009.03.001
Verma, H., Kumar, D., Kumar, V., Kumari, M., Singh, S. K., Sharma, V. K., et al. (2021). The potential application of endophytes in management of stress from drought and salinity in crop plants. Microorganisms 9:1729. doi: 10.3390/microorganisms9081729
Werner, T., and Schmülling, T. (2009). Cytokinin action in plant development. Curr. Opin. Plant Biol. 12, 527–538. doi: 10.1016/j.pbi.2009.07.002
Wybouw, B., and De Rybel, B. (2019). Cytokinin–a developing story. Trends Plant Sci. 24, 177–185. doi: 10.1016/j.tplants.2018.10.012
Yadav, A. N., and Yadav, N. (2018). Stress-adaptive microbes for plant growth promotion and alleviation of drought stress in plants. Acta Sci. Agric. 2, 85–88.
Yamada, M., Morishita, H., Urano, K., Shiozaki, N., Yamaguchi-Shinozaki, K., Shinozaki, K., et al. (2005). Effects of free proline accumulation in petunias under drought stress. J. Exp. Bot. 56, 1975–1981. doi: 10.1093/jxb/eri195
Yu, Z., Duan, X., Luo, L., Dai, S., Ding, Z., and Xia, G. (2020). How plant hormones mediate salt stress responses. Trends Plant Sci. 25, 1117–1130. doi: 10.1016/j.tplants.2020.06.008
Zhang, H. W., Song, Y. C., and Tan, R. X. (2006). Biology and chemistry of endophytes. Nat. Prod. Rep. 23, 753–771. doi: 10.1039/b609472b
Zhao, Y. (2010). Auxin biosynthesis and its role in plant development. Annu. Rev. Plant Biol. 61, 49–64. doi: 10.1146/annurev-arplant-042809-112308
Zhu, Q., Riley, W. J., Tang, J., and Koven, C. D. (2016). Multiple soil nutrient competition between plants, microbes, and mineral surfaces: model development, parameterization, and example applications in several tropical forests. Biogeosciences 13, 341–363. doi: 10.5194/bg-13-341-2016
Keywords: microbes, mitigate, drought, stress, plants
Citation: Shaffique S, Khan MA, Imran M, Kang S-M, Park Y-S, Wani SH and Lee I-J (2022) Research Progress in the Field of Microbial Mitigation of Drought Stress in Plants. Front. Plant Sci. 13:870626. doi: 10.3389/fpls.2022.870626
Received: 07 February 2022; Accepted: 14 April 2022;
Published: 19 May 2022.
Edited by:
David W. M. Leung, University of Canterbury, New ZealandReviewed by:
Manzer H. Siddiqui, King Saud University, Saudi ArabiaCopyright © 2022 Shaffique, Khan, Imran, Kang, Park, Wani and Lee. This is an open-access article distributed under the terms of the Creative Commons Attribution License (CC BY). The use, distribution or reproduction in other forums is permitted, provided the original author(s) and the copyright owner(s) are credited and that the original publication in this journal is cited, in accordance with accepted academic practice. No use, distribution or reproduction is permitted which does not comply with these terms.
*Correspondence: In-Jung Lee, aWpsZWVAa251LmFjLmty
†These authors have contributed equally to this work
Disclaimer: All claims expressed in this article are solely those of the authors and do not necessarily represent those of their affiliated organizations, or those of the publisher, the editors and the reviewers. Any product that may be evaluated in this article or claim that may be made by its manufacturer is not guaranteed or endorsed by the publisher.
Research integrity at Frontiers
Learn more about the work of our research integrity team to safeguard the quality of each article we publish.