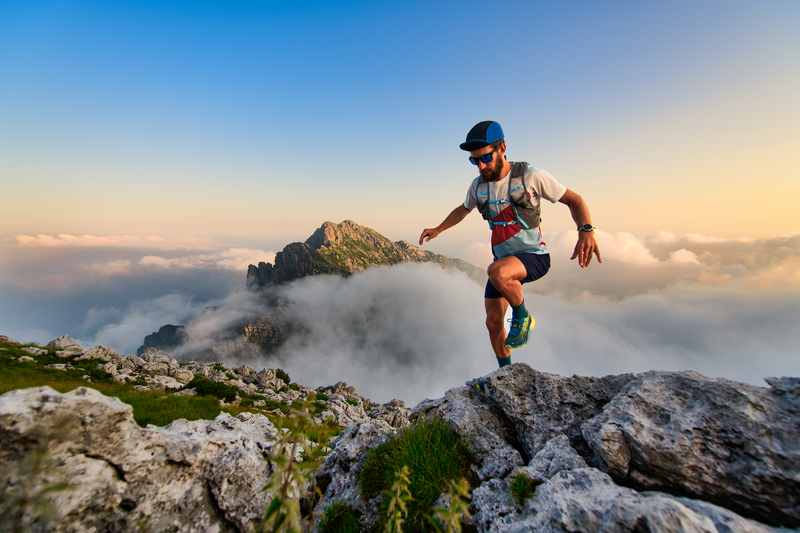
95% of researchers rate our articles as excellent or good
Learn more about the work of our research integrity team to safeguard the quality of each article we publish.
Find out more
REVIEW article
Front. Plant Sci. , 12 April 2022
Sec. Plant Physiology
Volume 13 - 2022 | https://doi.org/10.3389/fpls.2022.866034
This article is part of the Research Topic Molecular and Genetic Perspectives of Cold Tolerance in Plants View all 13 articles
Maize (Zea mays L.) is an annual grass that originated in tropical and subtropical regions of the New World. Maize is highly sensitive to cold stress during seed gemination and the seedling phase, which can lead to reductions in plant vigor and grain production. There are large differences in the morphological and physiological changes caused by cold stress among maize varieties. In general, cold tolerant varieties have a stronger ability to maintain such changes in traits related to seed germination, root phenotypes, and shoot photosynthesis. These morphological and physiological characteristics have been widely used to evaluate the cold tolerance of maize varieties in genetic analyses. In recent years, considerable progress has been made in elucidating the mechanisms of maize in response to cold tolerance. Several QTL, GWAS, and transcriptomic analyses have been conducted on various maize genotypes and populations that show large variations in cold tolerance, resulting in the discovery of hundreds of candidate cold regulation genes. Nevertheless, only a few candidate genes have been functionally characterized. In the present review, we summarize recent progress in molecular, physiological, genetic, and genomic analyses of cold tolerance in maize. We address the advantages of joint analyses that combine multiple genetic and genomic approaches to improve the accuracy of identifying cold regulated genes that can be further used in molecular breeding. We also discuss the involvement of long-distance signaling in plant cold tolerance. These novel insights will provide a better mechanistic understanding of cold tolerance in maize.
Maize (Zea mays L.) is one of the world's most important cereal crops for food, economy, and feed (Wang et al., 2020a). Maize is particularly susceptible to cold injury as it is a crop that requires high temperatures due to its tropical/subtropical origins (yyGreaves, 1996). Although studies have shown that maize is susceptible to the effects of low temperature during grain filling (Chen and Tang, 2012), cold stress mainly affects seed germination, seedling development, and growth at the seedling phase, eventually leading to reduced grain production (Li et al., 2019b). Cold damage in early spring is one of the main meteorological disasters that can befall maize production, especially in northern regions and high altitude areas in mountainous regions (Zhang, 2014).
Cold reduces both the seed germination rate and seedling vigor (Zhang et al., 2020). When maize seeds are exposed to low temperatures during the water absorption phase (imbibition), the permeability of cell membranes is impaired, resulting in the loss of cellular components (Hussain et al., 2018). Cold may also damage the ultrastructure of the embryonic root meristem cells and impair root development (Farooq et al., 2009). Maize seedling growth slows down considerably when the temperature falls below 10°C, and it ceases at temperatures between 6 and 8°C (Peng et al., 2016). The cells and tissues of the seedling can be irreversibly damaged at lower temperatures. Low temperatures during the seedling phase can reduce plant height, root length, the ability of the roots to absorb mineral nutrients, leaf chlorophyll content, and the net photosynthetic rate in seedlings. This will eventually result in growth inhibition, leaf yellowing, wilting, and necrosis, or even death of the seedlings (Chen and Tang, 2012; Yang et al., 2018). Cold stress can not only reduce the emergence rate and seedling vitality of maize directly, but can also affect plant health indirectly by increasing the chances of infection by soil bacteria (Juurakko et al., 2021). These direct and indirect impacts can both acutely reduce the yield and quality of the maize crop. Improving cold tolerance in maize will allow early sowing to withstand cold snaps that occur in early spring. Early sowing can result in an extended vegetative period, thus allowing the plants to accumulate additional biomass (Aydinoglu, 2020).
Detailed cold tolerance mechanisms have been investigated in other plant species, such as Arabidopsis [Arabidopsis thaliana (L.) Heynh.] and rice (Oryza sativa L.; Farhangi-Abriz and Torabian, 2017; Ding et al., 2019; Ritonga and Chen, 2020). In recent years, considerable progress has been made in elucidating the mechanisms by which maize responds to cold stress. Although three very recent reviews have discussed the efficacy of genetic and genomic approaches used to assess maize cold tolerance (Frascaroli and Revilla, 2018; Sowiński et al., 2020; Gillani et al., 2021), a comprehensive review of this subject is still lacking. Here, we have summarized the recent progress in molecular, physiological, genetic, and genomic analyses of cold tolerance in maize in order to provide a theoretical basis for molecular breeding of cold tolerance in maize.
The cold-responsive pathways of Arabidopsis have been the subject of intensive studies. Briefly, when Arabidopsis plants are exposed to cold stress, some cold-responsive inorganic substances activate transcription factors (TFs) through signal transduction pathways. Activated TFs bind to cis-elements present in downstream cold-responsive genes to activate their expression and induce cold tolerance in the plants (Li et al., 2019a). The signal pathway with CBF (C-repeat-binding factor) transcription factors as the core elements is mainly involved in regulation of the cold response (Rihan et al., 2017; shown in Figure 1A). There are three members of the CBF gene family in Arabidopsis: CBF1/DREB1B (dehydration responsive element binding factor 1B), CBF2/DREB1C, and CBF3/DREB1A (Liu et al., 2018). Overexpression of AtCBF1, AtCBF2, and AtCBF3 in Arabidopsis and other plant species is reported to significantly improve plant cold tolerance (Liu et al., 2018, 2020; Ding et al., 2019). The CBF genes have been cloned from other plant species such as rice, Brassica rapa, wheat and maize, indicating that the CBF genes show an important association with the cold response in plants (Liu et al., 2018, 2020; Ding et al., 2019).
Figure 1. Schematic diagram of the cold-responsive molecular networks in Arabidopsis (A) and maize (B). The eight green dashed arrows that point to (A) from (B) indicate that the maize genes were functionally verified in transgenic Arabidopsis plants. The orange dashed arrows in (B) indicate that the maize genes were functionally studied in tobacco. The blue dashed arrow in (B) indicates that the Arabidopsis gene AtICE1 was transformed into forage maize. The colored ellipses represent molecular elements that belong to the ICE-CBF-COR pathway. Small dots represent osmotic substances. Brackets encompass genes with the same induction level. Straight and dashed arrows represent positive regulation, whereas lines ending with a bar represent negative regulation. At, Arabidopsis thaliana L.; Zm, Zea mays L.; Zmm, Zea mays ssp. mexicana L.; Nt: Nicotiana tabacum L.; CAMTA, Calmodulin-binding transcription activator; ZAT, Zinc-finger transcription factor; HSFC, Heat shock transcription factor C; JAZ, Jasmonate ZIM-domain; DREB, Dehydration responsive element binding factor; LTI, Low temperature induced; KIN, Cold inducible; MPK, Mitogen-activated protein kinase; SEC14P, Sec14-like protein; RR, Response regulator; DBP, Dehydration responsive element binding protein; CesA, Cellulose synthase; MKK, Mitogen-activated protein kinase kinase; ERD, Early response to dehydration; JA, Jasmonic acid; ABA, abscisic acid; SA, Salicylic acid; BL, Brassinolide.
Overexpression of CBF genes significantly induces the expression of COR (Cold-regulated) genes in plants (light green ellipses in Figure 1A). COR refers to a class of genes regulated by cold stress, such as LTI (LOW TEMPERATURE INDUCED) and KIN (COLD INDUCIBLE). Some of these genes encode key enzymes involved in synthesizing osmotic substances, and are associated with the accumulation of cryoprotective proteins and soluble sugars to normalize cellular osmotic pressure and provide protection from freezing damage (Shi et al., 2018; Ding et al., 2019; small dots in Figure 1A). The CBF protein recognizes CRT/DRE (C-repeat/ Dehydration Responsive Element) motifs in the promoters of COR genes and induces their expression to enhance cold tolerance (Lu et al., 2017). The removal of the CBF-CRT/DRE regulatory module of AtCBF2 protein reduces the level of cold resistance in plants (Park et al., 2015).
The expression of CBF genes is regulated by both transcriptional activators and repressors (indicated by arrows and lines with a bar in Figure 1A). The positive regulatory genes include AtICE1 (inducer of CBF expression 1), AtICE2, and CAMTA (calmodulin-binding transcription activator; Chinnusamy et al., 2007; Ding et al., 2015). The transcriptional inhibitors which are directly involved in inhibiting cold-induced CBF activation include AtMYB15 and the AtPIFs (phytochrome interacting factors; Shi et al., 2018; red lines with a bar in Figure 1A). The AtICE1 protein interacts with the MYC-binding sequences present in the promoters of CBF genes to increase their expression during cold stress (Chinnusamy et al., 2003). Over-expression of AtICE2 has been shown to improve cold tolerance and the expression of AtCBFs in Arabidopsis (Fursova et al., 2009). The CAMTA3 protein binds to a CAMTA DNA regulatory motif, vCGCGb, located in a region of the promoter of AtCBF genes and functions together with CAMTA1 and CAMTA2 to promote the expression of CBF genes and cold tolerance in plants (Doherty et al., 2009; Kim et al., 2013). AtMYB15 and AtICE1 form a protein complex that binds to MYB recognition sites in the promoters of CBF genes. Overexpression of AtMYB15 results in reduced expression of CBF genes, whereas loss-of-function of AtMYB15 leads to increased expression of CBF genes (Agarwal et al., 2006). Furthermore, it has been recently discovered that redox changes mediated by cold stress can induce structural transformations and functional activation of CBF proteins (Lee et al., 2021).
At present, the ICE-CBF-COR cold responsive pathway is a well-accepted defense mechanism to cope with cold stress, but only some of the COR genes are regulated by CBF (Park et al., 2015). Some other transcription factors, including HSFC1 (Heat shock transcription factor C1), AtZAT10, and AtZAT12, are also capable of inducing COR gene expression under cold stress, and may co-regulate cold signal transduction with CBF (Park et al., 2015). Plant hormone JA (jasmonic acid) also positively regulates the CBF signal by mediating the interaction between the JAZ1/4 protein and ICE1/2, thereby regulating the transcriptional activity of ICE proteins and the expression of CBF1-3 (Hu et al., 2013). Taken together, these findings indicate that the cold regulatory network is very complicated in its functioning and needs to be seen in a broader sense so that it can be better understood.
The ICE-CBF-COR pathway has also been investigated in maize, in order to understand its involvement in cold tolerance in maize (as shown by ellipses in Figure 1B). However, the functional verification of most of these maize genes, as well as other cold-responsive genes, were determined by expression in heterologous species such as Arabidopsis or tobacco, and not in maize (green and orange arrows in Figure 1B). Four maize CBF TF genes, includingZmDREB1A, ZmDREB2A, ZmDBP3, and ZmDBP4, are induced by cold stress in maize. Overexpression of ZmDREB1A, ZmDBP3, and ZmDBP4 in Arabidopsis induced the overexpression of cold-inducible genes, resulting in enhanced cold tolerance (Qin et al., 2004; Wang and Dong, 2009; Wang et al., 2011). The overexpression of ZmDREB2A was found to enhance thermotolerance, but not cold tolerance (Qin et al., 2007). A low temperature-associated gene, ZmmICE1, has been isolated from Zea mays ssp. mexicana L., a close relative of maize, and ectopic expression of ZmmICE1 in the Arabidopsis ice1-2 mutant was observed to be associated with enhancing plant cold tolerance (Lu et al., 2017). When transformed with the Arabidopsis AtCBF1 gene, plants of the forage maize line SAUMZ1 showed reduced relative electrolyte leakage compared to wild type plants, and this resulted in improved cold tolerance (Xiang et al., 2012; blue dashed arrow in Figure 1B). These studies indicate that ICE-CBF-COR is a conserved pathway in various plant species. Other than the ICE and CBF genes reviewed above, there are many other regulators, such as transcription factors and protein kinases related to cold tolerance, that have been functionally characterized (Weckwerth et al., 2015; Erpen et al., 2018; Kimotho et al., 2019; Wang and Fu, 2019).
The MYB family is one of the largest TF families in plants, and it was named for the conserved MYB domain that is present in all eukaryotic MYB TFs (Katiyar et al., 2012). MYB TFs play important roles in the tolerance to various stresses in plants, including cold stress (Wu et al., 2019). The Arabidopsis CBF gene promoters contain a MYB recognition sequence and can be activated by MYB TFs (Li et al., 2019a). Unlike AtMYB15 in Arabidopsis, which is a negative regular of CBF gene transcription, two characterized maize MYBs are positive regulators of CBF genes (Figure 1B). Expression of the maize ZmMYB31 gene, which encodes an R2R3-MYB transcription factor, is induced at low temperature. Overexpression of ZmMYB31 in Arabidopsis was found to upregulate the expression of Arabidopsis CBF genes, thereby enhancing the resistance of transgenic Arabidopsis plants to low temperature and oxidative stress (Li et al., 2019a). Meng and Sui (2019) isolated another nucleus-located R2R3-MYB transcription factor, ZmMYB-IF35. Low temperature induced the expression of ZmMYB-IF35 in the cold-tolerant maize inbred line M54. Transgenic Arabidopsis plants overexpressing ZmMYB-IF35 showed improved cold tolerance, higher antioxidant enzyme activity, reduced levels of reactive oxygen species (ROS), and less ion leakage. ZmMYB-IF35 expression also positively regulated the expression of stress-related genes such as AtCBF2, AtCBF3, AtCOR1, and AtCOR2. Similar to the functions of maize ZmMYB genes, overexpression of a rice R2R3-MYB gene, OsMYB2, was found to improve cold tolerance, indicating that OsMYB2 is also a positive regulator of cold tolerance in rice (Yang et al., 2012). Further analysis of the expression patterns of 46 ZmMYB genes under different abiotic stress showed that 22 of these genes respond to different stress treatments. Among them, there were six ZmMYB genes that responded to cold treatment, but only ZmMYB53 expression was exclusively induced by cold stress (Chen et al., 2018).
MAPK or MPK (mitogen-activated protein kinases) proteins, a family of serine/threonine protein kinases, are involved in many important processes including stress signal transduction and development (Kong et al., 2013). The MAPK cascade pathway is a ubiquitous signal transduction module in eukaryotes that transmits biological signals from receptors to target molecules through a variety of intracellular and extracellular routes (Moustafa, 2014). The MAPK cascade component involves a three-kinase module, namely MAPK, MAPKK (MAPK kinase), and MAPKKK (MAPKK kinase; Xiang et al., 2021). The MAP kinase cascade can phosphorylate AtICE1 to promote its degradation, and thus is involved in the regulation of cold tolerance in Arabidopsis (Zhao et al., 2017; Figure 1A). In maize leaves, ZmMPK5 was found to be involved in the recovery of plants from cold stress (Berberich et al., 1999). Kong et al. (2011) isolated ZmMKK4, a group C MAPKK gene, from the root system of maize variety “Zhengdan 958,” and expression of the ZmMKK4 transcript was found to be up-regulated by low temperature exposure. Overexpression of ZmMKK4 in Arabidopsis increased the plants' cold tolerance, which showed that ZmMKK4 is a positive regulator of cold tolerance in maize (indicated by the green arrow in Figure 1B). Pan et al. (2012) identified ZmMPK17, a group D MAPK gene, that was induced by cold stress. The overexpression of ZmMPK17 enhanced cold tolerance in tobacco (Nicotiana tabacum L.) plants by affecting the antioxidant defense system. Cai et al. (2014) isolated and identified ZmMKK1, a group A MAPKK gene, from “Zhengdan 958”. Ectopic expression of ZmMKK1 in tobacco enhanced its cold tolerance, suggesting that ZmMKK1 is also involved in the response of plants to low temperature.
A very recent study identified a new cold regulation pathway, ZmMPK8-ZmRR1-ZmDREB1.10/ZmCesA2, in maize (Zeng et al., 2021; Figure 1B). The ZmRR1 (type-A Response Regulator 1) transcript level is slightly decreased, whereas the ZmRR1 protein level is increased by cold treatment of maize seedlings at 4°C. Overexpression of ZmRR1 leads to enhanced cold tolerance by accumulating and inducing the expression of the downstream genes, ZmDREB1.10 and ZmCesA2 (Cellulose synthase 2), suggesting that ZmRR1 acts as a positive regulator of maize cold tolerance. The ZmMPK8 protein, a negative regulator of cold tolerance, phosphorylates ZmRR1 at Ser15. A natural variation of ZmRR1 with a 45-bp deletion that encompasses Ser15 prevents its phosphorylation by ZmMPK8. At present, the ZmMPK8-ZmRR1-ZmDREB1.10/ZmCesA2 pathway is the best characterized cold regulation pathway in maize, and it provides an in-depth understanding of the molecular mechanism underlying cold tolerance in maize.
To date, functional investigations of many cold responsive genes have been performed in maize, but the functions of many of these genes were studied by overexpression in model plant species. As shown by the green and orange dashed arrows in Figure 1B, a total of eight maize genes were transformed into Arabidopsis, and two maize genes were transformed into tobacco. Only one study investigated the molecular function of ZmRR1, ZmMPK8, and their downstream genes ZmDREB1.10 and ZmCesA2, by either mutating or over-expressing the corresponding genes in maize. Transformation of maize genes into maize plants not only provides stronger evidence for gene functions, but also enables the straightforward elucidation of molecular pathways to provide in-depth biological insights. Furthermore, such native transformation would provide potential genetic resources for improving cold tolerance in maize.
Cold stress can induce a series of physiological responses in maize, such as the adjustment of osmotic substances, accumulation of ROS, disruption of hormonal homeostasis, impaired uptake of mineral nutrients, and a decrease in photosynthesis. To survive under such unfavorable conditions, plants need to maintain cellular function and integrity by stabilizing the cell membranes and biologically active proteins in order to sustain basic physiological activities (Ritonga and Chen, 2020). Even though plants have developed mechanisms by which they can to acclimate to cold temperatures, several strategies have been developed to reduce the effects of cold stress on maize seedlings.
Osmotic adjustment is one of the most important physiological mechanisms employed by plants to cope with many types of stresses. Under adverse conditions, osmotic adjustment substances help to maintain cell turgor and the capacity of tissues to retain water, and the contents of osmotic adjustment substances are positively correlated with plant stress resistance (Farhangi-Abriz and Torabian, 2017). The accumulation of osmotic adjustment substances can reduce the water potential in plant cells, prevent water outflow, and at the same time protect the structure of macromolecules in the cell (Li et al., 2013). The main osmotic adjustment substances in plants include soluble sugars, soluble proteins, and free proline (Zhang, 2014).
Soluble sugars are strongly hydrophilic, and they can reduce the stress damage to plant cells by reducing the water potential and maintaining the activity of some biological macromolecules (Guan et al., 2009). Soluble proteins can scavenge ROS (reactive oxygen species) and stabilize cell membrane structure (Nadarajah, 2020). Proline is a hydrophilic amino acid, and free proline functions to stabilize the metabolic process in the cytoplasm (Guan et al., 2009). Ma et al. (2015a) found that cold stress induces the expression of ZmP5CS1, a key gene for proline synthesis in immature maize embryos. Some studies have reported that low temperature treatments increase the contents of soluble sugars, proline, and soluble proteins in the seedlings of many maize varieties. The relative increases were larger in the varieties with strong cold tolerance compared to the varieties with relatively weaker cold tolerance. The lower the temperature, the more significant the difference between varieties (Li and Fang, 2018; Zhao et al., 2020). In conclusion, cold tolerant maize can maintain the water absorption capacity of cells and can reduce cold damage by accumulating higher levels of osmotic adjustment substances in response to cold stress.
Cold stress can destroy cellular homeostasis and cause the accumulation of high levels of ROS in plant cells. Excessive levels of ROS can be harmful to the cell membrane system because of lipid peroxidation (Nadarajah, 2020). Plant cells can induce the expression of a protective system to resist the threat posed by ROS. The protective enzymes within a plant cell, such as SOD (superoxide dismutase), CAT (catalase), POD (peroxidase), and APX (ascorbate peroxidase), cooperate with one another to scavenge the reactive oxygen free radicals so as to maintain normal physiological metabolic activities as much as possible to avoid damage to cell components (Nadarajah, 2020). Maize cells can stabilize the membrane structures and reduce cell damage by constantly oxidizing the reactive oxygen free radicals. Many studies have shown that cold stress enhances the activities of SOD, POD, and CAT in maize. The activities of the three main antioxidant enzymes and the relative expression of related genes are positively correlated with the cold tolerance of inbred lines (Wei et al., 2014; Yang et al., 2016; Li and Fang, 2018). For example, an early study showed that low temperature induces the expression of the APX gene, and the APX activity in cold tolerant maize was found to be higher than in cold sensitive maize (Pinhero et al., 1997). One study showed that the activities of protective enzymes decreased in the three cold-tolerant and three cold-sensitive maize inbred lines under cold stress, but the relative decrease in the cold-tolerant inbred lines was less than that in the cold-sensitive inbred lines (Peng et al., 2016). These differences could be due to many reasons, but we can conclude that the antioxidant enzyme system in cold-resistant maize lines is generally stronger than that of cold-sensitive lines.
MDA (malondialdehyde) is the main product of peroxidation of polyunsaturated cell membrane lipids and is a marker for oxidative stress. MDA is a highly reactive compound that can restrain the activity of cell protective enzymes and aggravate membrane peroxidation. When the cell membrane is damaged, a large number of electrolytes flow out of the cell, resulting in a surge of electrolytes (Wang et al., 2006). Therefore, relative conductivity and MDA levels are negatively correlated with cold tolerance in maize lines (Zhang, 2014). Several studies have reported that with increasing time of exposure to cold, the relative conductivity and MDA content increases. The cell membranes of cold tolerant varieties were less damaged, and the relative conductivity and MDA contents increased to a lesser extent in maize lines that show strong cold tolerance (Zhang, 2014; Peng et al., 2016).
Plants tightly regulate the levels of some hormones to cope with the changing environment (Lamers et al., 2020). ABA (abscisic acid) is an abiotic stress hormone that can improve the stress resistance of plants, and it participates in the regulatory response for a variety of abiotic stresses, including cold (Qin et al., 2021). The ABA contents in the roots of cold-tolerant lines were significantly higher than in the roots of cold-sensitive lines. Exogenous ABA treatment can promote the ability of maize seeds to germinate at low temperature (Zhang, 2011). GA (gibberellic acid) is also considered to play a role in plant cold resistance, but its effect is not as obvious as that of ABA (Eremina et al., 2016; Rihan et al., 2017). Studies have shown that the GA and IAA (indole-3-acetic acid; auxin) contents in the roots of maize seedlings decrease under cold stress, while the ABA content gradually increased (Janowiak et al., 2002; Zhang, 2011; Wei et al., 2014).
SA (salicylic acid) is another important plant hormone that is involved in cold tolerance in several plant species including maize, and its direct physiological effect is the change in the antioxidant enzyme activity of plants (Farooq et al., 2009). At low temperature, the increase in endogenous SA biosynthesis is closely related to the increase in antioxidant enzyme activity in maize seeds and during seedling growth (Wang et al., 2013). Cold stress in maize seedlings was not moderated by treating the seeds with SA (Gómez-Muñoz et al., 2018), but SA+H2O2 treatment at low temperature shortened seed germination time, improved seedling vigor, reduced cold damage to maize seeds, increased the activities of antioxidant enzymes and the expression levels of the corresponding genes, and thus improved cold tolerance (Li et al., 2017). Exogenous application of BL (brassinolide) also increased the germination rate, reduced cold damage to maize seedlings and caused an increase antioxidant enzyme activity, all of which resulted in an increase in plant biomass (Sun et al., 2020). Thus, manipulation of plant hormones appears to be an efficient way to alleviate the damage caused by cold temperature and to ensure the growth of seedlings at later stages of development.
Cold tolerance in plants is highly correlated with mineral nutrient levels (Waraich et al., 2012). The inhibition of growth in maize from cold stress is at least partly caused by indirect damage due to the decreased uptake of nutrients from the soil (Gómez-Muñoz et al., 2018). For example, the absorbance of K and Pi (orthophosphate) by maize roots is particularly affected by cold soil temperature (Bravo et al., 1981). Seed treatment with Mn/Zn can mitigate the negative effects of cold stress, and it resulted in increased biomass production in high-P soil but not in low-P soil, indicating that the absorbance of P is relieved by Mn/Zn treatment (Gómez-Muñoz et al., 2018). In addition, both Mn and Zn are key co-factors of several enzymes that are involved in the detoxification ROS caused by cold stress. Application of Si (silicon) is also a useful strategy to improve cold tolerance in maize seedlings during the early growth stage. The beneficial effects of Si seed treatments included the restoration of hormonal balances that were disrupted by cold stress and maintaining homeostasis of other micronutrients (Moradtalab et al., 2018). Therefore, appropriate plant nutrition is a useful strategy to alleviate the cold stress.
Cold stress significantly decreases photosynthesis in maize (Fracheboud et al., 2002). This decrease in photosynthesis may result from many factors, such as impaired chloroplast development, changes to the pigment composition, damage of the PSII reaction centers, and reduced activity of carbon cycle enzymes. The reduced photosynthetic ability at low temperatures also reflects the cold resistance of maize (Hund et al., 2008). Fracheboud et al. (2002) found that the chlorophyll a/b ratio in the cold tolerant lines was higher than in cold sensitive lines. Duran Garzon et al. (2020) showed that cold tolerance is related to higher chlorophyll content, higher G6PD (glucose-6-phosphate dehydrogenase) activity, and a higher sucrose-to-starch ratio. Many studies have used photosynthesis-related traits to quantify cold tolerance in maize. These studies are discussed below and are given in Tables 1, 2.
In general, cold tolerant maize may have stronger ability to accumulate more osmotic adjusting substances, stronger antioxidant enzyme system to stabilized ROS, stronger ability to maintain hormonal homeostasis, higher absorbance of mineral nutrient, higher photosynthetic ability, etc.
Cold tolerance in maize is a quantitative agronomic trait that is controlled by multiple genes (Turk et al., 2019). In the past few years, a number of genetic and genomic approaches have been applied to dissecting the components of this complex trait.
Quantitative genetic analyses of cold tolerance in maize have so far shown that heterosis, general and specific combining abilities, reciprocal maternal and non-maternal effects, additive effects, genotype, growth stage, and environmental factors are all involved in the expression of cold tolerance in maize. For example, Hodges et al. conducted two studies to explore the cold tolerance of maize at both germination and seedling stages using twelve hybrids derived from a complete diallel of four inbred lines (Hodges et al., 1997a,b). The results showed that the seed germination rate, the activities of CAT and APX in leaves at the 3-leaf stage, and the general and the special combining ability of the dry matter weight of leaves at the 4-leaf stage were significantly different. Revilla et al. (2000) proposed that cold tolerance has additive, dominant, and maternal effects. The experiment of Yan et al. (2017) showed that there was significant heterosis in maize cold tolerance at the seedling stage. Neta et al. (2020) used three cold-tolerant lines and three cold-sensitive lines to carry out partial diallel cross experiments to analyze the heterosis, general and specific combining abilities, reciprocal maternal and non-maternal effects, as well as the expression of CAT, APX, SOD and other genes. Results showed that there was heterosis and a reciprocal effect for germination under cold stress, and that the non-additive genes were more important. The genes that control cold tolerance depend on the particular materials used and the traits studied (Frascaroli and Revilla, 2018). Each growth stage of maize, including germination, emergence, and early seedling growth, is controlled by an independent genetic model; therefore, cold tolerance might also be regulated independently in the different growth stages (Frascaroli and Revilla, 2018). To make the situation more complicated, some studies have also reported that there are interactions between genes and the environment in the expression of cold tolerance in maize (Presterl et al., 2007).
The studies cited above show that the genetics of cold tolerance in maize is very complicated. However, with the broad application of genomic tools, considerable progress has been made in recent years in the identification of genetic loci and genes that are associated with maize cold tolerance. The information from these studies will facilitate a deeper understanding of the genetic mechanisms that control maize cold tolerance at the molecular level.
QTL (quantitative trait locus) mapping is a powerful tool for the identification and manipulation of loci underlying important and complex traits in agricultural crops. Recent studies have used different mapping populations, such as F2:3 families, RILs (recombinant inbred lines), and BC (back cross) populations, to study the QTLs that control cold tolerance in maize (Table 1). Most of these segregating populations were constructed by crossing cold-sensitive and cold-tolerant inbred lines in order to increase the relative degree of variation in the cold-response phenotypes. Several cold responsive physiological traits, such as seed germination rate, root phenotypes, and seedling photosynthesis, were measured under both normal and cold conditions (Table 1). These studies identified many reliable molecular marker loci associated with QTLs or genes that regulate cold tolerance and could be further used for breeding of cold tolerant inbred lines or hybrids.
Fracheboud et al. (2002) performed a QTL analysis of five traits related to the function of the photosynthetic apparatus at low temperature using a set of 233 maize RILs. They identified 18 QTLs that were significantly correlated with the target traits, of which the main QTL for leaf development at low temperature was the main QTL for pigment composition. Fracheboud et al. (2004) used an F2:3 population derived from the cross ETH-DH7×ETH-DL3 and detected 19 QTLs controlling chlorophyll fluorescence at low temperature. The major QTL for photosynthetic cold tolerance in maize seedlings is located on chromosome 6. Using the same population, Jompuk et al. (2005) detected a major QTL for cold tolerance located on chromosome 6 that corresponded to chlorophyll fluorescence and chlorophyll content. Hund et al. (2004) used an F2:3 population constructed from the cross Lo964×Lo1016 to map QTLs for root and shoot development in maize seedlings under cold conditions, and found a dominant QTL located on chromosome 5. Presterl et al. (2007) performed QTL mapping on 720 DH (doubled haploid) lines and found a total of 18 QTLs associated with leaf chlorosis, leaf purpling (anthocyanin), and frost damage at low temperature. Rodríguez et al. (2008) performed QTL analysis by using an IBM (intermated B73 × Mo17) population. None of the QTLs was identified under normal growth conditions, but two QTLs significantly correlated with leaf color at low temperature were located on chromosomes 3 and 6, and these two OTLs explained 14.2% of the phenotypic variation and 28.2% of the genetic variation. Guerra-Peraza et al. (2012) used the IBM302 population for QTL analysis and discovered a major QTL for photosynthesis-related traits on chromosome 5. The favorable allele of this QTL was contributed by Mo17 and appeared to be the major factor that explained the differential response of B73 and Mo17 to changes in the temperature at night. Rodríguez et al. (2013) used an F2:3 population derived from the cross of a cold susceptible (A661) with a cold tolerant (EP42) inbred line to detected genomic regions related to the cold-induced albino phenotype. A major QTL on chromosome 2 was identified that explained 14% of the phenotypic variation. Using the same F2:3 population, 10 QTLs related to photosystem traits were identified, with six of them at normal temperatures and four under cold conditions. A parallel meta-QTL analysis identified three genomic regions that regulate the development of maize seedlings at low temperatures (Rodríguez et al., 2014). Hu et al. (2016) performed a QTL analysis using 243 IBM Syn4 RILs and detected six QTLs associated with the low-temperature germination rate and six QTLs correlated with low-temperature primary root length. Of these, four pairs of QTLs were located in the same genomic regions. Yan et al. (2017) performed a QTL analysis with seedlings from an F2:3 population (207 lines) obtained by crossing a cold sensitive line (K932) with a cold tolerant (Mei C) inbred line. Their analysis resulted in the detection of seven QTLs controlling four cold-related traits, and one of the QTLs explained 10.55–25.29% of the phenotypic variation.
While the above-cited QTL analyses used “regular” populations, several studies have used “advanced” populations to improve the accuracy of the study. For example, Shi et al. (2016) constructed two connected RIL populations that shared one parental line and were able to identify 26 QTLs associated with seed vigor traits under low temperature conditions. Fourteen of these QTLs were further integrated into five mQTL regions through a meta-analysis. Two of the mQTL regions located on chromosomes 2 and 9 had R2 values >10% and were previously identified as QTLs for seed vigor traits. Using two cold-tolerant and two cold-susceptible inbred lines, Li et al. (2018) generated three connected F2:3 populations to detect QTLs related to seed germination ability at low temperature. A total of 43 QTLs and three mQTL regions were detected. Yi et al. (2020) used 406 lines from a multi-parent advanced generation intercross (MAGIC) population and found 858 SNPs grouped in 148 QTLs that were significantly associated with cold tolerance-related traits, and most of the QTLs were located in specific regions, particularly bin 10.04.
Several studies combined QTL analyses with other genetic or genomic approaches such as GWAS (genome-wide association study) or transcriptome analysis to efficiently uncover further biological insights. From the same population used by Hu et al. (2016); Goering et al. (2021) selected a panel of 97 lines for QTL analysis of traits including chlorophyll concentration, leaf color, and tissue damage at low temperature. Two cold-related QTLs with high additive impact were detected. These authors further verified the two QTLs using transcriptome data and identified 13 candidate genes likely to be involved in controlling the cold responses. Recently, Jin et al. (2021) studied cold responses in maize using a joint analyses that combined QTL and GWAS. They first performed QTL mapping for POD activity using an F2:3 population (210 lines) derived from cold-tolerant (W10) and cold-sensitive (W72) lines, and detected 12 QTLs significantly associated with POD activity and cold tolerance. They then conducted GWAS on a natural population consisting of 80 backbone inbred lines and found that four SNPs were significantly associated with POD activity at low temperature (Table 2). Using a joint analysis of the QTL and GWAS results, Zm00001d002729 was determined to be a potential cold tolerance gene. Overexpression of Zm00001d002729 increased the cold tolerance of maize seedlings by increasing POD activity and decreasing the MDA content and relative conductivity (ion leakage).
Table 1 shows that the genomic loci involved in the regulation of cold tolerance are distributed on almost all of the maize chromosomes, but most of these QTLs have not been further fine mapped or functionally characterized as cold tolerant genes, which prevents more detailed studies of cold tolerant mechanism in maize. One major reason for this may be the huge amount of work involved in gene mapping in large populations. In recent years, joint analysis using advanced populations or genomic tools has become increasingly popular to narrow down the search for candidate genes. Such an approach was recently used successfully to identify the major-effect cold regulating gene Zm00001d002729 (Jin et al., 2021), suggesting that it can be more widely applied in other studies.
Compared to biparental segregating populations, natural populations consisting of several hundred maize inbred lines have also been used for the genetic analyses of cold tolerance in GWAS (Frascaroli and Revilla, 2018). Although most of the cold-responsive physiological traits used in GWAS were similar to those in QTL analyses, including leaf color, root length, and seed germination-related traits (Tables 1, 2), GWAS offers increased mapping resolution and accuracy due to the higher level of genetic diversity in the mapping populations (Lipka et al., 2015).
Strigens et al. (2013) conducted the first GWAS in maize to dissect traits related to cold tolerance. Using a maize germplasm collection of 375 inbred genotypes with 56K SNPs (single nucleotide polymorphisms) GWAS identified 19 significant association signals that explained between 5.7 and 52.5% of the phenotypic variance for cold-related traits such as early growth and chlorophyll fluorescence. Huang et al. (2013) used 125 maize inbred lines to perform GWAS on 10 traits related to cold tolerance at the germination and seedling stages. A total of 43 SNPs were detected that were associated with cold tolerance and 40 candidate genes were predicted based on 31 of these SNPs. Yan et al. (2017) crossed the cytoplasmic male sterile parental line S-Mo17 with 338 different inbred lines to generate a test cross association mapping population. GWAS was performed on these 338 test crosses, and 19 significant SNPs associated with cold tolerance-related traits were detected. Hu et al. (2017) conducted GWAS on seed germination traits using 282 inbred lines of maize under normal and low temperature conditions. A total of 17 associated SNPs related to cold tolerance were identified, and seven of the SNPs were located in candidate genes. In a population of 222 maize inbred lines, Zhang et al. (2020) used GWAS to identify 30 SNPs related to cold tolerance during maize seed germination. Fourteen candidate genes directly related to the SNPs were found and further verified by gene expression analysis. Zhang et al. (2021) studied germination-related traits in 300 inbred lines under low temperature conditions. GWAS analysis revealed a total of 15 significant SNPs, and three genomic loci were repeatedly associated with multiple traits. Yi et al. (2021) evaluated a large panel of 836 maize inbreds, and GWAS analysis uncovered a total of 187 significant SNPs that could be integrated into 159 genomic regions that controlled seed emergence and traits related to early growth.
Despite its higher genetic mapping resolution, GWAS has not been without controversy. In particular, many of the cold-associated SNP markers identified in these studies were found to be located in the non-coding regions and generally thought to function in the regulation of gene expression. But which gene(s) do they regulate? There are large structural variations present in the genomes of different maize inbred lines, and the genetic loci predicted in the B73 genome may not represent all the genetic loci in other inbred lines. Hence, there may be some other unpredicted genes near or even located on cold-associated SNP markers, leading to inaccurate results.
Transcriptome analyses are also widely used to understand the molecular responses of maize to cold stress and to mine for cold tolerant genes. Li et al. (2016) used RNA-seq analyses to compare the transcriptomes of a freezing tolerant (KR701) and a freezing sensitive line (Hei8834) before and after cold treatment at the seedling stage and identified 948 DEGs (differentially expressed genes). GO (Gene Ontology) analysis revealed that the terms “binding functions,” “protein kinase,” and “peptidase activity” were over-represented in the DEGs. Li et al. (2019b) used RNA-seq to analyze the gene expression of cold tolerant (M54) and cold sensitive (753F) inbred lines under cold stress at the seedling stage. More DEGs were found in M54 than in 753F after both 4 and 24 h of cold treatment, indicating that the cold-responsive signaling networks were more active in the cold tolerant line. Li et al. (2020) analyzed the transcriptome of maize B73 seedlings under different low temperature conditions. In this study, 5,358, 5,485, and 5,312 DEGs were detected in response to cold stresses of 4, 10, and 16°C, respectively, and the expression of five genes including ZmDERB1 was significantly up regulated. Frey et al. (2020) selected 21 DH lines from a DH population (flint landrace “Petkuser Ferdinand rot”) based on their cold tolerance; 11 lines were cold resistant and 10 were cold sensitive. The transcriptomes of the 21 DH lines were analyzed after control and cold treatments. Here, 148, 3,254, and 563 DEGs were found to be related to cold treatment, cold tolerance, and growth rate at low temperature, respectively. Zhang et al. (2020) used RNA-seq to verify the correlation between the candidate genes and low-temperature tolerance and found 10 DEGs that were located in the linkage disequilibrium region of a GWAS analysis. Of these genes, two of them appear to regulate cold signal transduction and cell membrane fluidity. Li et al. (2021) analyzed transcriptomic changes in seeds of three sweet corn NILs and their parents under cold stress. A total of 20 DEGs were found to be highly related to low-temperature germination, and a gene encoding UDP-glucosyltransferase was hypothesized to be essential to cold germination in sweet corn. Waititu et al. (2021) conducted a comparative analysis of the transcriptomes of seedlings of 24 cold-tolerant and 22 cold-sensitive inbred lines under cold stress. A total of 2,237 DEGs were identified, which included 147 TFs belonging to 32 families such as MYB, ERF, NAC, WRKY, bHLH, MIKC MADS, and C2H2. Yu et al. (2021) studied the leaf transcriptomic response of two maize inbred lines with contrasting cold tolerance levels under a time series cold treatment. The results showed that cold tolerance in line B144 is due to active mediation of stomatal opening and protection of photosystem II from photooxidation by upregulating the expression of genes for D1 proteins, while the sensitive line Q319 was unable to close its stomata in response to cold.
In addition to RNA-seq analyses, other genomic tools have also been used to study the transcriptomic changes in maize to cold. Rodríguez et al. (2013) used microarray hybridization on the chlorophyll-less and chlorophyll-containing sections of leaves of maize inbred line A661 which shows a cold-induced albino phenotype. A total of 1,002 differentially expressed transcripts were identified between the two sections, and these DEGs were classified into 23 categories including genes in the tetrapyrrole biosynthesis pathway and photosynthesis. Di Fenza et al. (2017) conducted microarray analysis with four varieties, with two cold-tolerant and two less cold-tolerant lines, to identify genes that were differentially expressed under chilling conditions. A total number of 64 DEGs were identified in the two chilling-tolerant varieties, while no significant changes in expression were observed in less cold-tolerant lines. Another study used cDNA-AFLP to analyze the gene expression changes in response to cold stress and identified three maize genes, ZmMAPKKK, ZmCLC-D, and ZmRLK, that were possibly involved in the cold response (Yang et al., 2011).
To investigate the regulatory roles of miRNAs (microRNAs) in cold tolerance, Aydinoglu (2020) studied the miRNome (miRNA microarray) in seedlings of the maize hybrid ADA313 that were treated with cold temperature. In this study, 24, 6, and 20 miRNAs were specifically differentially regulated in the meristem, the elongation zone, and the mature zone by cold stress, respectively. This study highlighted the importance of miRNAs in the maize response to cold stress. Combined with large-scale bioinformatic analysis, Zhou et al. (2022) examined the transcriptome changes in seedlings of the inbred lines B73, Mo17, and W22 and the F1 hybrids with all three combinations (B73xMo17, W22xB73, and W22xMo17) in response to cold or heat stress. They identified many stress-related DEGs among the different maize genotypes and assigned these expression changes to cis-or trans-regulatory mechanisms using the F1 hybrid data. Their study answered the question of how sequence differences in cis-elements of different genotypes impact a gene's responsiveness to stress, and shed light on the prediction of a plant response to stress by implementing a more sophisticated model construction (convolutional neural networks).
Above transcriptomic analyses indentified tens to thousands of DEGs in individual studies. This astonishing variaiton in the numbers of DEGs indicates that a vast majority of the DEGs can not overlap to each other, leaving an almost impossible task to mine the conserved overlaping genes. In deed, when Sowiński et al. (2020) surveyed 13 independent studies addressing the transcriptomic changes in response to cold stress in maize seedlings at the V2-V5 stages, they found <500 DEGs were reported in more than one study and the rest are specific to individual studies. Furthermore, among the 13 independent studies survied, four of these 13 studies used moderate low temperature stress and the other nine used severe cold stress. By comparing the DEGs, it was found that the transcriptomic changes that occur in response to moderate low tempreture and severe cold stress are fundamentally different (Sowiński et al., 2020). Taken together, the variation in gene expression in different studies can be caused by the genotypes, tissue, developmental stage, experimental design and the strength of cold. Although the results of individual studies varied considerably, the “common genes” shared by several independent projects after carefully eliminate the differences can be attractive candidates for further functional studies.
All of the above studies extensively investigated the “responses” of maize to cold, but how does maize actively perceive cold and transmit these signals to other parts of the plant? In rice, the OsCOLD1 protein was found to localize to the endoplasmic reticulum and plasma membrane and promote GTPase activity by interacting with a G-protein. In response to cold stress, COLD1 changes membrane fluidity to mediate extracellular Ca2+ influx and cytosolic Ca2+ concentration, and the altered Ca2+ concentration acts as a secondary messenger to regulate downstream genes (Ma et al., 2015b). Thus, COLD1 is thought to be the first cold sensor identified in plants (Shi and Yang, 2015). In maize, ZmSEC14p, a Sec14-like protein, was found to regulate the expression of phosphoinositide-specific phospholipase C in the phosphoinositide signal transduction pathway, which generates the second messengers inositol 1,4,5-trisphosphate and 1,2- diacylglycerol for downstream signal transduction (Wang et al., 2016). Over-expression of ZmSEC14p results in the up regulation of some cold-responsive genes such as CBF3, suggesting its important role in cold signal transduction.
The above studies, together with the findings in the ICE-CBF-COR pathways, lay important foundations for understanding the cold signal transduction pathway within a local cell; however, the way in which plants transmit cold signals to other parts of the plant is largely unknown. One of the possibilities involves the long-distance signaling system. The phloem sap contains plant hormones, small peptides, transcription factors, and various types of RNA molecules (mRNAs, miRNAs, and tRNAs) that can travel long distances to the other parts of the plant. These molecules, as potential signaling factors, regulate plant growth and development and the stress response (Xia et al., 2018). Wang et al. (2020b) developed a watermelon–bottle gourd heterografting system to identify the mobile mRNAs under both control and cold conditions. Their results showed that cold stress significantly enhances the mobility of mRNAs in the phloem (Figure 2). In particular, some of the scion-delivered mobile mRNAs in the rootstock are derived from some well-known genes related to osmotic adjustment and cold tolerance, while mRNA that moves in the opposite direction includes transcripts from genes related to ABA-activated signaling. In another study, Chen et al. (2020) found that the Arabidopsis clock component ELF4 (EARLY FLOWERING 4) protein showed long-distance movement from the shoot to the root through the vascular system to control the root clock in a temperature-dependent manner, and low temperatures favored ELF4 mobility (Figure 2). Hence, ELF4 has been suggested to a mobile long-distance cold signal that establishes a shoot-to-root communication for temperature information. In addition to ELF4 protein and mobile mRNAs, Ca2+ has also been implicated as a long-distance signal for water trafficking and defense signaling (Shkolnik et al., 2018; Toyota et al., 2018). A previous study showned that the AtMIZ1 (Mizu-Kussey 1) protein regulates AtECA1 (ER-type CA2+-ATPase 1) to generate the long-distance phloem-mobile Ca2+ signal for water trafficking (Shkolnik et al., 2018). Interestingly, both AtMIZ1 and AtECA1 were found to be responsive to cold treatment (He et al., 2016; Sharma et al., 2018). Despite the involvement of Ca2+ in local cold signaling, it is unknown whether Ca2+ also functions as a long-distance cold signal through the MIZ1-ECA1- Ca2+ pathway (Figure 2). These studies clearly show that long-distance signaling is involved in the response to cold. One very recent study shows that monocotyledonous plants are also capable of being grafted (Reeves et al., 2021), and discoveries related to cold-induced long-distance signaling in maize can be expected soon through use of this powerful method.
Figure 2. A proposed model for the long-distance regulation of cold signaling by the ELF4 protein and mobile mRNAs. Full and dashed arrows indicate known and potential long-distance signaling molecules, respectively. The thickness of the arrows indicates the relative strength of the signal. ELF4, Early flowering 4.
Cold tolerance is an important breeding objective in the pursuit of high productivity and better environmental compliance. Cold tolerance research in maize is of great significance to stabilize yield and enhance food security by broadening the geographical regions in which maize can be cultivated. Cold tolerance in maize is a complex trait and is a cumulative function of many physiological and molecular pathways. Significant achievements have made in studying the physiology of maize cold tolerance, but much remains to be done to solve the problems that result from cold damage to maize plants. The molecular analyses of maize cold tolerance mechanisms are still insufficient, and many of the current studies do not contribute much to maize biology compared to those in Arabidopsis and rice. This could be due in part to the lack of discovery of novel genes that may regulate cold tolerance in maize.
There are large differences in cold tolerance among various maize varieties. Cold tolerant varieties usually have a stronger ability to maintain osmotic pressure, the ROS balance, hormonal homeostasis, mineral nutrient absorbance, and photosynthesis. These physiological characteristics have been widely used to evaluate the cold tolerance of maize varieties in QTL and GWAS analyses. Many of the QTL and GWAS analyses were based on various maize genotypes and populations that express large variations in cold tolerance, but only a few candidate genes have been identified. Further fine mapping and map-based cloning of genes in the genomic regions identified via QTL and GWAS analysis is required, and more candidate genes will provide a basis for further understanding the molecular and genetic mechanism of cold tolerance in maize. Furthermore, using QTL mapping and GWAS, molecular markers closely linked to cold tolerance genes can also be identified as resources for MAS (marker assisted selection) of cold tolerant varieties. In addition, with the aid of data used for GWAS analysis, future work can also use GS (genomic selection) to predict cold tolerant varieties in large maize populations.
Transcriptome analysis is a powerful tool that has been used in many studies for the identification of cold-responsive genes. Comparisons of the DEGs detected in different studies have shown little overlap with each other. A few studies integrated QTL, GWAS, RNA-seq, and other methods, which significantly reduced the number of candidate genes for selection, partly because joint analyses in the same study can eliminate the differences that arise from the genotypes used or the experimental design. Future research that integrates more methods, such as phenomic, proteomic, metabolomic, and bioinformatic approaches, may greatly improve the accuracy of identifying cold-regulated genes and provide better candidates that can be further used in molecular breeding.
CX and HL conceived the work. XZ drafted the manuscript. HL, CX, and IM made comments and revisions. All authors have read and approved the manuscript.
This work was supported by the National Natural Science Foundation of China (NSFC) 32101673 and the Sichuan Science and Technology Program 2021YFYZ0017.
The authors declare that the research was conducted in the absence of any commercial or financial relationships that could be construed as a potential conflict of interest.
All claims expressed in this article are solely those of the authors and do not necessarily represent those of their affiliated organizations, or those of the publisher, the editors and the reviewers. Any product that may be evaluated in this article, or claim that may be made by its manufacturer, is not guaranteed or endorsed by the publisher.
Agarwal, M., Hao, Y., Kapoor, A., Dong, C., Fujii, H., Zheng, X., et al. (2006). A R2R3 type MYB transcription factor is involved in the cold regulation of CBF genes and in acquired freezing tolerance. J. Biol. Chem. 281, 37636–37645. doi: 10.1074/jbc.M605895200
Aydinoglu, F. (2020). Elucidating the regulatory roles of microRNAs in maize (Zea mays L.) leaf growth response to chilling stress. Planta 251:38. doi: 10.1007/s00425-019-03331-y
Berberich, T., Sano, H., and Kusano, T. (1999). Involvement of a MAP kinase, ZmMPK5, in senescence and recovery from low-temperature stress in maize. Mol. Gen. Genet. 262, 534–542. doi: 10.1007/s004380051115
Bravo, -F P., and Uribe, E. G. (1981). Temperature dependence of the concentration kinetics of absorption of phosphate and potassium in corn roots. Plant Physiol. 67, 815–819. doi: 10.1104/pp.67.4.815
Cai, G., Wang, G., Wang, L., Pan, J., Liu, Y., and Li, D. (2014). ZmMKK1, a novel group A mitogen-activated protein kinase kinase gene in maize, conferred chilling stress tolerance and was involved in pathogen defense in transgenic tobacco. Plant Sci. 214, 57–73. doi: 10.1016/j.plantsci.2013.09.014
Chen, M., and Tang, Y. (2012). Effects of low temperature stress on chlorophyll fluorescence characteristics of com seedlings. J. Inn. Mong. Agric. Univ. Nat. Sci. Ed. 33, 20–24. doi: 10.16853/j.cnki.1009-3575.2012.03.006
Chen, W., Takahashi, N., Hirata, Y., Ronald, J., Porco, S., Davis, S. J., et al. (2020). A mobile ELF4 delivers circadian temperature information from shoots to roots. Nat. Plants. 6, 416–426. doi: 10.1038/s41477-020-0634-2
Chen, Y., Cao, Y., Wang, L., Li, L., Yang, J., and Zou, M. (2018). Identification of MYB transcription factor genes and their expression during abiotic stresses in maize. Biol. Plant. 62, 222–230. doi: 10.1007/s10535-017-0756-1
Chinnusamy, V., Ohta, M., Kanrar, S., Lee, B.-H., Hong, X., Agarwal, M., et al. (2003). ICE1: a regulator of cold-induced transcriptome and freezing tolerance in Arabidopsis. Genes Dev. 17, 1043–1054. doi: 10.1101/gad.1077503
Chinnusamy, V., Zhu, J., and Zhu, J. (2007). Cold stress regulation of gene expression in plants. Trends. Plant Sci. 12, 444–451. doi: 10.1016/j.tplants.2007.07.002
Di Fenza, M., Hogg, B., Grant, J., and Barth, S. (2017). Transcriptomic response of maize primary roots to low temperatures at seedling emergence. Peer J. 5:e2839. doi: 10.7717/peerj.2839
Ding, Y., Shi, Y., and Yang, S. (2015). Molecular mechanisms of plant responses to cold stress. Chinese Bullet. Life Sci. 27, 398–405. doi: 10.13376/j.cbls/2015052
Ding, Y., Shi, Y., and Yang, S. (2019). Advances and challenges in uncovering cold tolerance regulatory mechanisms in plants. New Phytol. 222, 1690–1704. doi: 10.1111/nph.15696
Doherty, C. J., Van Buskirk, H. A., Myers, S. J., and Thomashow, M. F. (2009). Roles for Arabidopsis CAMTA transcription factors in cold-regulated gene expression and freezing tolerance. Plant Cell. 21, 972–984. doi: 10.1105/tpc.108.063958
Duran Garzon, C., Lequart, M., Rautengarten, C., Bassard, S., Sellier-Richard, H., Baldet, P., et al. (2020). Regulation of carbon metabolism in two maize sister lines contrasted for chilling tolerance. J. Exp. Bot. 71, 356–369. doi: 10.1093/jxb/erz421
Eremina, M., Rozhon, W., and Poppenberger, B. (2016). Hormonal control of cold stress responses in plants. Cell. Mol. Life Sci. 73, 797–810. doi: 10.1007/s00018-015-2089-6
Erpen, L., Devi, H. S., Grosser, J. W., and Dutt, M. (2018). Potential use of the DREB/ERF, MYB, NAC and WRKY transcription factors to improve abiotic and biotic stress in transgenic plants. Plant Cell Tissue Organ Cult. 132, 1–25. doi: 10.1007/s11240-017-1320-6
Farhangi-Abriz, S., and Torabian, S. (2017). Antioxidant enzyme and osmotic adjustment changes in bean seedlings as affected by biochar under salt stress. Ecotoxicol. Environ. Saf. 137, 64–70. doi: 10.1016/j.ecoenv.2016.11.029
Farooq, M., Aziz, T., Wahid, A., Lee, D.-J., and Siddique, K. H. (2009). Chilling tolerance in maize: agronomic and physiological approaches. Crop Pasture Sci. 60, 501–516. doi: 10.1071/CP08427
Fracheboud, Y., Jompuk, C., Ribaut, J., Stamp, P., and Leipner, J. (2004). Genetic analysis of cold-tolerance of photosynthesis in maize. Plant Mol. Biol. 56, 241–253. doi: 10.1007/s11103-004-3353-6
Fracheboud, Y., Ribaut, J. M., Vargas, M., Messmer, R., and Stamp, P. (2002). Identification of quantitative trait loci for cold-tolerance of photosynthesis in maize (Zea mays L.). J. Exp. Bot. 53, 1967–1977. doi: 10.1093/jxb/erf040
Frascaroli, E., and Revilla, P. (2018). “Genomics of cold tolerance in maize.” in The Maize Genome. eds J. Bennetzen, S. Flint-Garcia, C. Hirsch and R. Tuberosa (Cham: Springer International Publishing), 287–303. doi: 10.1007/978-3-319-97427-9_17
Frey, F. P., Pitz, M., Schön, C. C., and Hochholdinger, F. (2020). Transcriptomic diversity in seedling roots of European flint maize in response to cold. BMC Genom. 21:300. doi: 10.1186/s12864-020-6682-1
Fursova, O. V., Pogorelko, G. V., and Tarasov, V. A. (2009). Identification of ICE2, a gene involved in cold acclimation which determines freezing tolerance in Arabidopsis thaliana. Gene 429, 98–103. doi: 10.1016/j.gene.2008.10.016
Gillani, S. F. A., Rasheed, A., Yuhong, G., Jian, W., Xia, W. Y., Tariq, H., et al. (2021). Assessment of cold stress tolerance in maize through quantitative trait locus, genome-wide association study and transcriptome analysis. Not. Bot. Horti Agrobo. 49:12525. doi: 10.15835/nbha494125
Goering, R., Larsen, S., Tan, J., Whelan, J., and Makarevitch, I. (2021). QTL mapping of seedling tolerance to exposure to low temperature in the maize IBM RIL population. PLoS ONE. 16:e0254437. doi: 10.1371/journal.pone.0254437
Gómez-Muñoz, B., Lekfeldt, J. D., Magid, J., Jensen, L. S., and de Neergaard, A. (2018). Seed treatment with penicillium sp. or Mn/Zn can alleviate the negative effects of cold stress in maize grown in soils dependent on soil fertility. J. Agro. Crop Sci. 204, 603–612. doi: 10.1111/jac.12288
Guan, Y., Hu, J., Wang, X., and Shao, C. (2009). Seed priming with chitosan improves maize germination and seedling growth in relation to physiological changes under low temperature stress. J. Zhejiang Univ Sci. B. 10, 427–433. doi: 10.1631/jzus.B0820373
Guerra-Peraza, O., Leipner, J., Reimer, R., Nguyen, H. T., and Fracheboud, Y. (2012). Temperature at night affects the genetic control of acclimation to cold in maize seedlings. Maydica 56, 367–377.
He, F., Arce, A. L., Schmitz, G., Koornneef, M., Novikova, P., et al. (2016). The footprint of polygenic adaptation on stress-responsive Cis-regulatory divergence in the Arabidopsis genus. Mol. Biol. Evol. 33, 2088–2101. doi: 10.1093/molbev/msw096
Hodges, D. M., Andrews, C. J., Johnson, D. A., and Hamilton, R. I. (1997a). Antioxidant enzyme and compound responses to chilling stress and their combining abilities in differentially sensitive maize hybrids. Crop Sci. 37, 857–863. doi: 10.2135/cropsci1997.0011183X003700030027x
Hodges, D. M., Andrews, C. J., Johnson, D. A., and Hamilton, R. I. (1997b). Sensitivity of maize hybrids to chilling and their combining abilities at two developmental stages. Crop Sci. 37, 850–856. doi: 10.2135/cropsci1997.0011183X003700030026x
Hu, G., Li, Z., Lu, Y., Li, C., Gong, S., Yan, S., et al. (2017). Genome-wide association study identified multiple genetic loci on chilling resistance during germination in maize. Maize. Sci. Rep. 7:10840. doi: 10.1038/s41598-017-11318-6
Hu, S., Lübberstedt, T., Zhao, G., and Lee, M. (2016). QTL mapping of low-temperature germination ability in the maize IBM Syn4 RIL population. PLoS ONE. 11:e0152795. doi: 10.1371/journal.pone.0152795
Hu, Y., Jiang, L., Wang, F., and Yu, D. (2013). Jasmonate regulates the inducer of CBF expression-C-repeat binding factor/DRE binding factor1 cascade and freezing tolerance in Arabidopsis. Plant Cell. 25, 2907–2924. doi: 10.1105/tpc.113.112631
Huang, J., Zhang, J., Li, W., Hu, W., Duan, L., Feng, Y., et al. (2013). Genome-wide association analysis of ten chilling tolerance indices at the germination and seedling stages in maize. J. Integr. Plant Biol. 55, 735–744. doi: 10.1111/jipb.12051
Hund, A., Fracheboud, Y., Soldati, A., Frascaroli, E., Salvi, S., and Stamp, P. (2004). QTL controlling root and shoot traits of maize seedlings under cold stress. Theor. Appl. Genet. 109, 618–629. doi: 10.1007/s00122-004-1665-1
Hund, A., Fracheboud, Y., Soldati, A., and Stamp, P. (2008). Cold tolerance of maize seedlings as determined by root morphology and photosynthetic traits. Eur. J. Agron. 28, 178–185. doi: 10.1016/j.eja.2007.07.003
Hussain, H. A., Hussain, S., Khaliq, A., Ashraf, U., Anjum, S. A., Men, S., et al. (2018). Chilling and drought stresses in crop plants: implications, cross talk, and potential management opportunities. Front. Plant Sci. 9:393. doi: 10.3389/fpls.2018.00393
Janowiak, F., Maas, B., and Dörffling, K. (2002). Importance of abscisic acid for chilling tolerance of maize seedlings. J. Plant Physiol. 159, 635–643. doi: 10.1078/0176-1617-0638
Jin, Y., Zhang, Z., Xi, Y., Yang, Z., Xiao, Z., Guan, S., et al. (2021). Identification and functional verification of cold tolerance genes in spring maize seedlings based on a genome-wide association study and quantitative trait locus mapping. Front. Plant Sci. 12:776972. doi: 10.3389/fpls.2021.776972
Jompuk, C., Fracheboud, Y., Stamp, P., and Leipner, J. (2005). Mapping of quantitative trait loci associated with chilling tolerance in maize (Zea mays L.) seedlings grown under field conditions. J. Exp. Bot. 56, 1153–1163. doi: 10.1093/jxb/eri108
Juurakko, C. L., DiCenzo, G. C., and Walker, V. K. (2021). Cold acclimation and prospects for cold-resilient crops. Plant Stress 2:100028. doi: 10.1016/j.stress.2021.100028
Katiyar, A., Smita, S., Lenka, S. K., Rajwanshi, R., Chinnusamy, V., and Bansal, K. C. (2012). Genome-wide classification and expression analysis of MYB transcription factor families in rice and Arabidopsis. BMC Genom. 13:544. doi: 10.1186/1471-2164-13-544
Kim, Y., Park, S., Gilmour, S. J., and Thomashow, M. F. (2013). Roles of CAMTA transcription factors and salicylic acid in configuring the low-temperature transcriptome and freezing tolerance of Arabidopsis. Plant J. 75, 364–376. doi: 10.1111/tpj.12205
Kimotho, R. N., Baillo, E. H., and Zhang, Z. (2019). Transcription factors involved in abiotic stress responses in maize (Zea mays L.) and their roles in enhanced productivity in the post genomics era. Peer J. 7:e7211. doi: 10.7717/peerj.7211
Kong, X., Pan, J., Zhang, D., Jiang, S., Cai, G., Wang, L., et al. (2013). Identification of mitogen-activated protein kinase kinase gene family and MKK-MAPK interaction network in maize. Biochem. Biophys. Res. Commun. 441, 964–969. doi: 10.1016/j.bbrc.2013.11.008
Kong, X., Pan, J., Zhang, M., Xing, X., Zhou, Y., Liu, Y., et al. (2011). ZmMKK4, a novel group C mitogen-activated protein kinase kinase in maize (Zea mays), confers salt and cold tolerance in transgenic Arabidopsis. Plant Cell Environ. 34, 1291–1303. doi: 10.1111/j.1365-3040.2011.02329.x
Lamers, J., van der Meer, T., and Testerink, C. (2020). How plants sense and respond to stressful environments. Plant Physiol. 182, 1624–1635. doi: 10.1104/pp.19.01464
Lee, E. S., Park, J. H., Wi, S. D., Kang, C. H., Chi, Y. H., Chae, H. B., et al. (2021). Redox-dependent structural switch and CBF activation confer freezing tolerance in plants. Nat. Plants. 7, 914–922. doi: 10.1038/s41477-021-00944-8
Li, B., and Fang, Z. (2018). Screening of low-temperature tolerance maize inbred lines and analysis of leaf physiological characteristics and cellular structure changes. J. Henan Agric. Sci. 47, 31–37. doi: 10.15933/j.cnki.1004-3268.2018.10.006
Li, M., Lin, L., Zhang, Y., and Sui, N. (2019a). ZmMYB31, a R2R3-MYB transcription factor in maize, positively regulates the expression of CBF genes and enhances resistance to chilling and oxidative stress. Mol. Biol. Rep. 46, 3937–3944. doi: 10.1007/s11033-019-04840-5
Li, M., Sui, N., Lin, L., Yang, Z., and Zhang, Y. (2019b). Transcriptomic profiling revealed genes involved in response to cold stress in maize. Funct. Plant Biol. 46, 830–844. doi: 10.1071/FP19065
Li, W., Li, G., Xiao, Y., Hu, J., Li, C., Li, Y., et al. (2021). Analysis of genes related to low-temperature germination ability in sweet corn based on transcriptome sequencing. Guangdong Agri. Sci. 48, 11–18. doi: 10.16768/j.issn.1004-874X.2021.03.002
Li, X., Wang, G., Fu, J., Li, L., Jia, G., Ren, L., et al. (2018). QTL mapping in three connected populations reveals a set of consensus genomic regions for low temperature germination ability in Zea mays L. Front. Plant Sci. 9:65. doi: 10.3389/fpls.2018.00065
Li, Y., Wang, X., Li, Y., Zhang, Y., Gou, Z., Qi, X., et al. (2020). Transcriptomic analysis revealed the common and divergent responses of maize seedling leaves to cold and heat stresses. Genes 11:881. doi: 10.3390/genes11080881
Li, Z., Hu, G., Liu, X., Zhou, Y., Li, Y., Zhang, X., et al. (2016). Transcriptome sequencing identified genes and Gene Ontologies associated with early freezing tolerance in maize. Front. Plant Sci. 7:1477. doi: 10.3389/fpls.2016.01477
Li, Z., Peng, Y., and Su, X. (2013). Physiological responses of white clover by different leaf types associated with anti-oxidative enzyme protection and osmotic adjustment under drought stress. Acta Pratacult. Sin. 22, 257–263. doi: 10.11686/cyxb20130233
Li, Z., Xu, J., Gao, Y., Wang, C., Guo, G., Luo, Y., et al. (2017). The synergistic priming effect of exogenous salicylic acid and H2O2 on chilling tolerance enhancement during maize (Zea mays L.) seed germination. Front. Plant Sci. 8:1153. doi: 10.3389/fpls.2017.01153
Lipka, A. E., Kandianis, C. B., Hudson, M. E., Yu, J., Drnevich, J., et al. (2015). From association to prediction: statistical methods for the dissection and selection of complex traits in plants. Curr. Opin. Plant Biol. 24, 110–118. doi: 10.1016/j.pbi.2015.02.010
Liu, J., Shi, Y., and Yang, S. (2018). Insights into the regulation of C-repeat binding factors in plant cold signaling. J. Integr. Plant Biol. 60, 780–795. doi: 10.1111/jipb.12657
Liu, J., Yan, S., Zhang, R., Su, J., Sun, Y., and Sun, L. (2020). Low temperature tolerance of rice: a review. Chinese Agri. Sci. Bullet. 36, 1–5. doi: 10.11924/j.issn.1000-6850.casb20190800542
Lu, X., Yang, L., Yu, M., Lai, J., Wang, C., McNeil, D., et al. (2017). A novel Zea mays ssp. mexicana L. MYC-type ICE-like transcription factor gene ZmmICE1, enhances freezing tolerance in transgenic Arabidopsis thaliana. Plant Physiol. Biochem. 113, 78–88. doi: 10.1016/j.plaphy.2017.02.002
Ma, J., Xing, G., Yang, X., Wang, Y., and Du, H. (2015a). Effects of exogenous EBR and NO signal on antioxidant system and low response gene expression under cold stress on maize embryo. Chin. J. Appl. Ecol. 26, 1411–1418. doi: 10.13287/j.1001-9332.20150319.006
Ma, Y., Dai, X., Xu, Y., Luo, W., Zheng, X., Zeng, D., et al. (2015b). COLD1 confers chilling tolerance in rice. Cell 160, 1209–1221. doi: 10.1016/j.cell.2015.01.046
Meng, C., and Sui, N. (2019). Overexpression of maize MYB-IF35 increases chilling tolerance in Arabidopsis. Plant Physiol. Biochem. 135, 167–173. doi: 10.1016/j.plaphy.2018.11.038
Moradtalab, N., Weinmann, M., Walker, F., Höglinger, B., Ludewig, U., and Neumann, G. (2018). Silicon improves chilling tolerance during early growth of maize by effects on micronutrient homeostasis and hormonal balances. Front. Plant Sci. 9:420. doi: 10.3389/fpls.2018.00420
Moustafa, K. (2014). MAPK transgenic circuit to improve plant stress-tolerance? Plant Signal. Behav. 9:e970101. doi: 10.4161/15592316.2014.970101
Nadarajah, K. K. (2020). ROS homeostasis in abiotic stress tolerance in plants. Int. J. Mol Sci. 21:5208. doi: 10.3390/ijms21155208
Neta, I. C. S., Von Pinho, É. V. d. R., de Abreu, V. M., Vilela, D. R., Santos, M. C., Dos Santos, H. O., et al. (2020). Gene expression and genetic control to cold tolerance during maize seed germination. BMC. Plant Biol. 20:188. doi: 10.1186/s12870-020-02387-3
Pan, J., Zhang, M., Kong, X., Xing, X., Liu, Y., Zhou, Y., et al. (2012). ZmMPK17, a novel maize group D MAP kinase gene, is involved in multiple stress responses. Planta 235, 661–676. doi: 10.1007/s00425-011-1510-0
Park, S., Lee, C. M., Doherty, C. J., Gilmour, S. J., Kim, Y., and Thomashow, M. F. (2015). Regulation of the Arabidopsis CBF regulon by a complex low-temperature regulatory network. Plant J. 82, 193–207. doi: 10.1111/tpj.12796
Peng, Y., Wang, Y., Zhao, X., and Lv, Y. (2016). Evaluation of chilling tolerance in different maize inbred lines. Agri. Res. Arid Areas 34, 267–280. doi: 10.7606/j.issn.1000-7601.2016.03.42
Pinhero, R. G., Rao, M. V., Paliyath, G., Murr, D. P., and Fletcher, R. A. (1997). Changes in activities of antioxidant enzymes and their relationship to genetic and paclobutrazol-induced chilling tolerance of maize seedlings. Plant Physiol. 114, 695–704. doi: 10.1104/pp.114.2.695
Presterl, T., Ouzunova, M., Schmidt, W., Möller, E. M., Röber, F. K., Knaak, C., et al. (2007). Quantitative trait loci for early plant vigour of maize grown in chilly environments. Theor. Appl. Genet. 114, 1059–1070. doi: 10.1007/s00122-006-0499-4
Qin, F., Kakimoto, M., Sakuma, Y., Maruyama, K., Osakabe, Y., Tran, L. S., et al. (2007). Regulation and functional analysis of ZmDREB2A in response to drought and heat stresses in Zea mays L. Plant J. 50, 54–69. doi: 10.1111/j.1365-313X.2007.03034.x
Qin, F., Sakuma, Y., Li, J., Liu, Q., Li, Y., Shinozaki, K., et al. (2004). Cloning and functional analysis of a novel DREB1/CBF transcription factor involved in cold-responsive gene expression in Zea mays L. Plant Cell Physiol. 45, 1042–1052. doi: 10.1093/pcp/pch118
Qin, P., Zhang, G., Hu, B., Wu, J., Chen, W., Ren, Z., et al. (2021). Leaf-derived ABA regulates rice seed development via a transporter-mediated and temperature-sensitive mechanism. Sci. Adv. 7:eabc8873. doi: 10.1126/sciadv.abc8873
Reeves, G., Tripathi, A., Singh, P., Jones, M., Nanda, A. K., Musseau, C., et al. (2021). Monocotyledonous plants graft at the embryonic root-shoot interface. Nature 2021, 1–7. doi: 10.1038/s41586-021-04247-y
Revilla, P., Malvar, R., Cartea, M., Butrón, A., and Ordás, A. (2000). Inheritance of cold tolerance at emergence and during early season growth in maize. Crop Sci. 40, 1579–1585. doi: 10.2135/cropsci2000.4061579x
Rihan, H. Z., Al-Issawi, M., and Fuller, M. P. (2017). Advances in physiological and molecular aspects of plant cold tolerance. J. Plant Interact. 12, 143–157. doi: 10.1080/17429145.2017.1308568
Ritonga, F. N., and Chen, S. (2020). Physiological and molecular mechanism involved in cold stress tolerance in plants. Plants 9:560. doi: 10.3390/plants9050560
Rodríguez, V., Butrón, A., Malvar, R. A., Ordás, A., and Revilla, P. (2008). Quantitative trait loci for cold tolerance in the maize IBM population. Int. J. Plant Sci. 169, 551–556. doi: 10.1086/528755
Rodríguez, V., Butrón, A., Rady, M. O., Soengas, P., and Revilla, P. (2014). Identification of quantitative trait loci involved in the response to cold stress in maize (Zea mays L.). Mol. Breed. 33, 363–371. doi: 10.1007/s11032-013-9955-4
Rodríguez, V., Velasco, P., Garrido, J. L., Revilla, P., Ordás, A., and Butrón, A. (2013). Genetic regulation of cold-induced albinism in the maize inbred line A661. J. Exp. Bot. 64, 3657–3667. doi: 10.1093/jxb/ert189
Sharma, R., Singh, G., Bhattacharya, S., and Singh, A. (2018). Comparative transcriptome meta-analysis of Arabidopsis thaliana under drought and cold stress. PLoS ONE. 13:e0203266. doi: 10.1371/journal.pone.0203266
Shi, Y., Ding, Y., and Yang, S. (2018). Molecular regulation of CBF signaling in cold acclimation. Trends. Plant Sci. 23, 623–637. doi: 10.1016/j.tplants.2018.04.002
Shi, Y., Li, G., Tian, Z., Wang, Z., Wang, X., Zhu, Y., et al. (2016). Genetic dissection of seed vigour traits in maize (Zea mays L.) under low-temperature conditions. J. Genet. 95, 1017–1022. doi: 10.1007/s12041-016-0714-2
Shi, Y., and Yang, S. (2015). COLD1: a cold sensor in rice. Sci. China. Life Sci. 58, 409–410. doi: 10.1007/s11427-015-4831-6
Shkolnik, D., Nuriel, R., Bonza, M. C., Costa, A., and Fromm, H. (2018). MIZ1 regulates ECA1 to generate a slow, long-distance phloem-transmitted Ca2+ signal essential for root water tracking in Arabidopsis. Proc. Natl. Acad. Sci. USA. 115:8031–8036. doi: 10.1073/pnas.1804130115
Sowiński, P., Fronk, J., Jończyk, M., Grzybowski, M., Kowalec, P., and Sobkowiak, A. (2020). Maize response to low temperatures at the gene expression level: a critical survey of transcriptomic studies. Front. Plant Sci. 11:576941. doi: 10.3389/fpls.2020.576941
Strigens, A., Freitag, N. M., Gilbert, X., Grieder, C., Riedelsheimer, C., Schrag, T. A., et al. (2013). Association mapping for chilling tolerance in elite flint and dent maize inbred lines evaluated in growth chamber and field experiments. Plant Cell Environ. 36, 1871–1887. doi: 10.1111/pce.12096
Sun, Y., He, Y., Irfan, A. R., Liu, X., Yu, Q., Zhang, Q., et al. (2020). Exogenous brassinolide enhances the growth and cold resistance of maize (Zea mays L.) seedlings under chilling stress. Agronomy 10:488. doi: 10.3390/agronomy10040488
Toyota, M., Spencer, D., Sawai-Toyota, S., Jiaqi, W., Zhang, T., Koo, A. J., et al. (2018). Glutamate triggers long-distance, calcium-based plant defense signaling. Science. 361, 1112–1115. doi: 10.1126/science.aat7744
Turk, H., Erdal, S., and Dumlupinar, R. (2019). Carnitine-induced physio-biochemical and molecular alterations in maize seedlings in response to cold stress. Archiv. Agron. Soil Sci. 66, 925–941. doi: 10.1080/03650340.2019.1647336
Waititu, J. K., Cai, Q., Sun, Y., Sun, Y., Li, C., Zhang, C., et al. (2021). Transcriptome profiling of maize (Zea mays L.) leaves reveals key cold-responsive genes, transcription factors, and metabolic pathways regulating cold stress tolerance at the seedling stage. Genes 12:1638. doi: 10.3390/genes12101638
Wang, C., and Dong, Y. (2009). Overexpression of maize ZmDBP3 enhances tolerance to drought and cold stress in transgenic Arabidopsis plants. Biologia 64, 1108–1114. doi: 10.2478/s11756-009-0198-0
Wang, C., Yang, Q., and Yang, Y. (2011). Characterization of the ZmDBP4 gene encoding a CRT/DRE-binding protein responsive to drought and cold stress in maize. Acta. Physiol. Plant. 33, 575–583. doi: 10.1007/s11738-010-0582-y
Wang, W., and Fu, J. (2019). Regulation of Ca2+ in plant response mechanisms under cold stress. Hans J. Agri. Sci. 9, 1173–1179. doi: 10.12677/HJAS.2019.912167
Wang, X., Feng, J., White, P. J., Shen, J., and Cheng, L. (2020a). Heterogeneous phosphate supply influences maize lateral root proliferation by regulating auxin redistribution. Ann. Bot. 125, 119–130. doi: 10.1093/aob/mcz154
Wang, X., Shan, X., Xue, C., Wu, Y., Su, S., Li, S., et al. (2016). Isolation and functional characterization of a cold responsive phosphatidylinositol transfer-associated protein, ZmSEC14p, from maize (Zea may L.). Plant Cell. Rep. 35, 1671–1686. doi: 10.1007/s00299-016-1980-4
Wang, Y., Chu, J., Sun, Z., and Wang, D. (2006). Study on physiological response of maize to low temperature and comparison of frost hardiness varieties. Chinese Agri. Sci. Bullet. 9, 210–212. doi: 10.3969/j.issn.1000-6850.2006.09.052
Wang, Y., Wang, L., Xing, N., Wu, X., Wu, X., Wang, B., et al. (2020b). A universal pipeline for mobile mRNA detection and insights into heterografting advantages under chilling stress. Hortic. Res. 7:13. doi: 10.1038/s41438-019-0236-1
Wang, Y., Wen, T., Hu, J., Han, R., Zhu, Y., Guan, Y., et al. (2013). Relationship between endogenous salicylic acid and antioxidant enzyme activities in maize seedlings under chilling stress. Expl. Agric. 49, 295–308. doi: 10.1017/S0014479712001329
Waraich, E. A., Ahmad, R., Halim, A., and Aziz, T. (2012). Alleviation of temperature stress by nutrient management in crop plants: a review. J. Soil Sci. Plant Nutr. 12, 221–244. doi: 10.4067/S0718-95162012000200003
Weckwerth, P., Ehlert, B., and Romeis, T. (2015). ZmCPK1, a calcium-independent kinase member of the Zea mays CDPK gene family, functions as a negative regulator in cold stress signalling. Plant Cell Environ. 38, 544–558. doi: 10.1111/pce.12414
Wei, S., Luo, N., Jing, L. I., Wanrong, G. U., Chen, G., Lijie, L. I., et al. (2014). Change of the root protective enzyme activities and endogenous hormones of maize seedling under low-temperature stress. J. Northeast Agric. Univ. 45, 1–8. doi: 10.19720/j.cnki.issn.1005-9369.2014.09.001
Wu, J., Jiang, Y., Liang, Y., Chen, L., Chen, W., and Cheng, B. (2019). Expression of the maize MYB transcription factor ZmMYB3R enhances drought and salt stress tolerance in transgenic plants. Plant Physiol. Biochem. 137, 179–188. doi: 10.1016/j.plaphy.2019.02.010
Xia, C., Zheng, Y., Huang, J., Zhou, X., Li, R., Zha, M., et al. (2018). Elucidation of the mechanisms of long-distance mrna movement in a Nicotiana benthamiana/tomato heterograft system. Plant Physiol. 177, 745–758. doi: 10.1104/pp.17.01836
Xiang, B., Li, C., Zhang, J., Luo, Y., and Jiang, A. (2012). Transformation of CBF1 gene to maize grass SAUMZ1 improves its ability of cold resistance. Pratac. Sci. 29, 1374–1378.
Xiang, Y., Bian, X., Wei, T., Yan, J., Sun, X., Han, T., et al. (2021). ZmMPK5 phosphorylates ZmNAC49 to enhance oxidative stress tolerance in maize. New Phytol. 232, 2400–2417. doi: 10.1111/nph.17761
Yan, J., Wu, Y., Li, W., Qin, X., Wang, Y., and Yue, B. (2017). Genetic mapping with testcrossing associations and F2:3 populations reveals the importance of heterosis in chilling tolerance at maize seedling stage. Sci Rep. 7:3232. doi: 10.1038/s41598-017-03585-0
Yang, A., Dai, X., and Zhang, W. (2012). A R2R3-type MYB gene, OsMYB2, is involved in salt, cold, and dehydration tolerance in rice. J. Exp. Bot. 63, 2541–2556. doi: 10.1093/jxb/err431
Yang, D., Sun, Y., Irfan, A. R., Liu, X., Jinying, L. V., Juping, Y. U., et al. (2018). Effect of low temperature stress on germination and physiological of maize seedling. J. Northeast Agric. Univ. 49, 8–44. doi: 10.19720/j.cnki.issn.1005-9369.2018.05.001
Yang, G., Zou, H., Wu, Y., Liu, H., and Yuan, Y. (2011). Identification and characterization of candidate genes involved in chilling responses in maize (Zea mays L.). Plant Cell Tissue Organ. Cult. 106, 127–141. doi: 10.1007/s11240-010-9900-8
Yang, J., Mao, J., Yu, Y., Li, C., Wang, Y., and Hu, J. (2016). Effects of chilling on antioxidant enzyme activity and related gene expression levels during seed germination. J. Nucl. Agric. Sci. 30, 1840–1847. doi: 10.11869/j.issn.100-8551.2016.09.1840
Yi, Q., Alvarez-Iglesias, L., Malvar, R. A., Romay, M. C., and Revilla, P. (2021). A worldwide maize panel revealed new genetic variation for cold tolerance. Theor. Appl. Genet. 134, 1083–1094. doi: 10.1007/s00122-020-03753-3
Yi, Q., Malvar, R. A., Alvarez-Iglesias, L., Ordas, B., and Revilla, P. (2020). Dissecting the genetics of cold tolerance in a multiparental maize population. Theor. Appl. Genet. 133, 503–516. doi: 10.1007/s00122-019-03482-2
Yu, T., Zhang, J., Cao, J., Cai, Q., Li, X., Sun, Y., et al. (2021). Leaf transcriptomic response mediated by cold stress in two maize inbred lines with contrasting tolerance levels. Genomics 113, 782–794. doi: 10.1016/j.ygeno.2021.01.018
yyGreaves, J. A. (1996). Improving suboptimal temperature tolerance in maize- the search for variation. J. Exp. Bot. 47, 307–323.
Zeng, R., Li, Z., Shi, Y., Fu, D., Yin, P., Cheng, J., et al. (2021). Natural variation in a type-A response regulator confers maize chilling tolerance. Nat. Commun. 12:4713. doi: 10.1038/s41467-021-25001-y
Zhang, H., Zhang, J., Xu, Q., Wang, D., Di, H., Huang, J., et al. (2020). Identification of candidate tolerance genes to low-temperature during maize germination by GWAS and RNA-seq approaches. BMC. Plant Biol. 20:333. doi: 10.1186/s12870-020-02543-9
Zhang, X. (2011). Effect of endogenous hormones content changing on the chilling tolerance in maize seed germination. J. Shenyang Agri. Univ. 42, 147–151. doi: 10.3969/j.issn.1000-1700.2011.02.004
Zhang, X. (2014). Effect of low temperature on cold resistance of maize in seed germination. Hortic. Seed. 9, 23–29. doi: 10.3969/j.issn.2095-0896.2014.03.009
Zhang, Y., Liu, P., Wang, C., Zhang, N., Zhu, Y., Zou, C., et al. (2021). Genome-wide association study uncovers new genetic loci and candidate genes underlying seed chilling-germination in maize. Peer J. 9:e11707. doi: 10.7717/peerj.11707
Zhao, C., Wang, P., Si, T., Hsu, C., Wang, L., Zayed, O., et al. (2017). MAP kinase cascades regulate the cold response by modulating ICE1 protein stability. Dev. Cell. 43, 618–629. doi: 10.1016/j.devcel.2017.09.024
Zhao, X., Ge, S., Wei, Y., Xu, X., Ding, D., Liu, M., et al. (2020). Analysis of root physiology and related gene expression in maize (Zea mays) under low temperature stress. J. Agric. Biotechnol. 28, 32–41. doi: 10.3969/j.issn.1674-7968.2020.01.004
Keywords: maize, cold tolerance, QTL, GWAS, transcriptome, long-distance signaling
Citation: Zhou X, Muhammad I, Lan H and Xia C (2022) Recent Advances in the Analysis of Cold Tolerance in Maize. Front. Plant Sci. 13:866034. doi: 10.3389/fpls.2022.866034
Received: 30 January 2022; Accepted: 21 March 2022;
Published: 12 April 2022.
Edited by:
Cankui Zhang, Purdue University, United StatesReviewed by:
Kewei Zhang, Zhejiang Normal University, ChinaCopyright © 2022 Zhou, Muhammad, Lan and Xia. This is an open-access article distributed under the terms of the Creative Commons Attribution License (CC BY). The use, distribution or reproduction in other forums is permitted, provided the original author(s) and the copyright owner(s) are credited and that the original publication in this journal is cited, in accordance with accepted academic practice. No use, distribution or reproduction is permitted which does not comply with these terms.
*Correspondence: Chao Xia, Y2hhb3hpYUBzaWNhdS5lZHUuY24=; Hai Lan, bGFuaGFpX21haXplQDE2My5jb20=
Disclaimer: All claims expressed in this article are solely those of the authors and do not necessarily represent those of their affiliated organizations, or those of the publisher, the editors and the reviewers. Any product that may be evaluated in this article or claim that may be made by its manufacturer is not guaranteed or endorsed by the publisher.
Research integrity at Frontiers
Learn more about the work of our research integrity team to safeguard the quality of each article we publish.