- 1College of Forestry, Shenyang Agricultural University, Shenyang, China
- 2Key Laboratory of Forest Tree Genetics, Breeding and Cultivation of Liaoning Province, Shenyang, China
Chrysanthemum white rust (CWR), a disease caused by the fungus Puccinia horiana Henn., seriously impairs the production and ornamental value of chrysanthemums. We previously isolated the disease-resistance gene CmWRKY15-1 from the chrysanthemum and generated CmWRKY15-1 transgenic plants. Here, we determined that CmWRKY15-1-overexpressing lines of the susceptible cultivar ‘Jinba’ show higher defensive enzyme activity and lower H2O2 levels than a wild type after inoculation with P. horiana, indicating that CmWRKY15-1 positively regulates plant responses to P. horiana. To further explore the mechanism underlying this effect, we performed RNA sequencing using the leaves of wild-type and CmWRKY15-1-RNA interference lines of the resistant cultivar ‘C029’ after treatment with P. horiana. We identified seven differentially expressed genes in the salicylic acid (SA) pathway, including CmNPR1 (Non-expressor of pathogenesis-related genes 1), encoding an important regulator of this pathway. We isolated the CmNPR1 promoter by hiTAIL-PCR and predicted that it contains pathogen-induced W-box elements. The promoter region of CmNPR1 was activated by P. horiana in a β-glucuronidase activity assay. Yeast one-hybrid assays showed that CmWRKY15-1 binds to the CmNPR1 promoter region to regulate its expression. Finally, we confirmed the interaction between CmWRKY15-1 and CmNPR1 in a bimolecular fluorescence complementation assay. We propose that CmWRKY15-1 interacts with CmNPR1 to activate the expression of downstream pathogenesis-related genes that enhance resistance to P. horiana through the SA pathway. These findings shed light on the mechanism underlying resistance to CWR.
Introduction
Chrysanthemum (Chrysanthemum morifolium) originated in China and is one of 10 flowers traditionally grown there. This plant is an important ornamental flower with considerable economic value globally (Li et al., 2015; Xin et al., 2021). Chrysanthemum white rust (CWR) is an epidemic disease caused by the fungus Puccinia horiana. Spores of P. horiana spread easily in autumn and winter at temperatures of 4–24°C and quickly infect leaf surfaces during germination (Yoo and Roh, 2014; Keefe and Davis, 2015). This disease has become endemic to most chrysanthemum-growing areas in China, greatly hampering the sale of this crop to the international market (Zeng et al., 2013; Torres et al., 2017). Exploring the molecular mechanisms that result in resistant cultivars could help provide safer and more economical methods for controlling CWR (Alaei et al., 2009).
Plants must respond to numerous environmental stresses and have therefore evolved numerous defense mechanisms to protect themselves throughout their lifecycles (Chen et al., 2020). Plants utilize two strategies to detect pathogens: pathogen-associated molecular pattern (PAMP)-triggered immunity (PTI) and effector-triggered immunity (ETI) (Tsuda and Katagiri, 2010; Resjö et al., 2019). ETI is qualitatively stronger and faster than PTI and often involves a form of localized cell death called the hypersensitive response (HR) (Dodds and Rathjen, 2010). This regional cell necrosis in plants is accompanied by an increased generation of reactive oxygen species (ROS) to help the plant resist pathogen invasion (Yun and Chen, 2011). Following the HR, the plant rapidly produces salicylic acid (SA) and generates a signal in the primary infected tissue that induces the distal end of the uninfected plant to increase its resistance to secondary infection. This process is called systemic acquired resistance (SAR) (Deenamo et al., 2018; Zhang et al., 2018). ROS and SA are very important in preventing pathogen invasion, and ROS are indispensable components of plant responses to stress (Wang Y. J. et al., 2020; Wang Y. Q. et al., 2020). Plants stressed by pathogenic bacteria produce large amounts of ROS, and ROS levels are closely related to improvements in plant resistance. In response to many types of damage, defensive enzymes such as phenylalanine ammonia-lyase (PAL), peroxidase (POD), catalase (CAT), and superoxide dismutase (SOD) also eliminate ROS to reduce oxidative damage (Torun, 2019).
WRKY transcription factors are important regulators of the SA signaling pathway (Zhao N. et al., 2020). CsWRKY22, MdWRKY15, and LiWRKY enhance the resistance of orange (Citrus sinensis Osbeck), apple (Malus × domestica), and Easter lily (Lilium longiflorum) to Xanthomonas citri subsp. citri, Botryosphaeria dothidea, and Botrytis cinerea, respectively (Zhao X. Y. et al., 2020; Kumari et al., 2021; Long et al., 2021). Heterologous expression of PtrWRKY73 in Arabidopsis (Arabidopsis thaliana) activates the expression of other downstream genes in the SA pathway and enhances resistance to the pathogen Pseudomonas syringae pathovar tomato strain DC3000 (Pst DC3000) (Duan et al., 2015). We previously identified CmWRKY15-1, a gene whose expression is significantly induced upon infection of the disease-resistant chrysanthemum variety ‘C029’ with P. horiana, based on transcriptomic data. In addition, CmWRKY15-1-overexpressing (OE) lines showed higher SA content and greater resistance to P. horiana. This indicates that CmWRKY15-1 can participate in the regulation of plant disease via the SA pathway (Dong et al., 2018; Bi et al., 2021).
WRKY transcription factors regulate gene expression by specifically binding to W-box (TTGACC/T) cis-elements in the promoters of their target genes to regulate their transcription and enhance plant defense (Yu et al., 2001; He et al., 2016). NON-EXPRESSOR OF PATHOGENESIS-RELATED GENES 1 (NPR1) is a master regulator involved in SAR. The NPR1 promoter contains a W-box that binds WRKY transcription factors, suggesting that expression of NPR1 is induced by WRKY or other transcription factors (Li et al., 2018). NPR1 contains two domains associated with protein-protein interactions: the BTB/POZ (broad-complex, tramtrack, and bric-a-brac/pox virus and zinc finger) domain and the ankyrin-repeat (ANK) domain (Chern et al., 2005). Under stable conditions, NPR1 oligomers exist in the cytoplasm via intermolecular disulfide bonds. ETI signals facilitate the generation of SA in plants, which results in biphasic redox changes and the phosphorylation and subsequent monomerization of NPR1. This, in turn, allows NPR1 to translocate into the nucleus, where it regulates target gene expression by interacting with WRKY and TGA transcription factors and NIM1-interacting proteins (Mou et al., 2003). Hordeum vulgare NPR1 interacts with the HvWRKY6, HvWRKY40, and HvWRKY70 genes to increase resistance to P. triticina in barley (Gao et al., 2018).
Although we previously demonstrated that the overexpression of CmWRKY15-1 enhanced the resistance to pathogens and obtained the transgenic plants of CmWRKY15-1 (Bi et al., 2021), the mechanism of CmWRKY15-1 and its target gene in the SA pathway is not clear. Therefore, here, we sampled leaves of wild-type (WT) and CmWRKY15-1-RNA interference (RNAi) lines of ‘C029’ after inoculation with P. horiana and analyzed the samples by transcriptome sequencing (RNA-seq). This analysis identified the differentially expressed gene (DEG) CmNPR1. Our results showed that the expression of pathogenesis-related (PR) genes significantly decreased in CmNPR1 silenced lines after inoculation with P. horiana. Next, we showed that CmWRKY15-1 binds to the promoter region of CmNPR1 to regulate its expression. Finally, we determined that CmWRKY15-1 interacts with CmNPR1 to activate the expression of PR genes, thereby contributing to resistance to P. horiana. These findings shed light on the regulatory mechanisms of CmWRKY15-1 and CmNPR1 involved in plant-pathogen interactions via the SA signaling pathway. In addition, they provide a valuable reference to help fulfill the long-term goal of breeding disease-resistant chrysanthemum.
Materials and Methods
Plant Materials
Using RNAi, we previously generated CmWRKY15-1-silenced lines of ‘C029’ and CmWRKY15-1-OE lines of ‘Jinba.’ The white rust-resistant chrysanthemum cultivar ‘C029,’ the susceptible cultivar ‘Jinba,’ and various transgenic lines were used as experimental materials. These plant materials were provided by the Forestry College of Shenyang Agricultural University, Shenyang, China, and all experiments were conducted there in 2020 and 2021. Seedlings at the six- to eight-leaf stage were placed in a potting soil mixture and grown in a greenhouse under fluorescent lights for 2 weeks at 25 ± 3°C.
Puccinia horiana Inoculation and Sampling
Teliospores of P. horiana were collected from the abaxial sides of chrysanthemum leaves infected with white rust and placed in 1 ml sterile water. The concentration of the teliospores was adjusted to 40–60 per field of vision under a BA400 microscope (Motic, Xiamen). Teliospores were cultured according to the protocol by Takatsu (Takatsu et al., 2008). A drop of teliospore suspension was removed with a sterile straw and dropped onto a glass substrate. This was placed on a U-shaped rod in a culture dish covered with wet filter paper. The teliospores were allowed to germinate at 18–21°C for 24 h and resuspended in sterile water with 0.05% (w/v) Tween 20 (pH 4–6.5). The abaxial sides of leaves were sprayed evenly with the solution, and the plants were covered with plastic film and transferred to the dark and 90% relative humidity. After 16–24 h, infected plants were transferred to a growth chamber maintained at 17°C and 50% relative humidity. Leaves were harvested from WT, OE, and RNAi plants at 0 day (before inoculation), and at 24, 48, and 72 h after treatment with P. horiana. Leaves were wrapped in aluminum foil, immediately frozen in liquid nitrogen, and stored at –80°C for sequencing experiments and to measure physiological indices.
Diaminobenzidine Staining and H2O2 Measurements
H2O2 content in WT and CmWRKY15-1-OE lines of ‘Jinba’ leaves was measured at 0, 24, 48, and 72 h after inoculation with P. horiana. Leaves were immersed in diaminobenzidine (DAB) staining solution (1 mg/ml 3,3′-DAB, 10 mM Na2HPO4, and 0.05% Tween 20, pH 3.8) in the dark for 4–8 h and decolorized in 95% (w/v) ethanol. The intensity of brown coloration indicates H2O2 content. Quantitative H2O2 measurements were performed using an Amplex Red hydrogen peroxide/peroxidase assay kit (Solarbio Science and Technology, Beijing). Absorbance was measured using a Tecan infinite F200/M200 plate reader at 560 nm. H2O2 concentration is indicated in μmol/g fresh weight. The experiment was performed with at least three independent replicates.
Physiological Indices
Frozen leaf samples of WT and CmWRKY15-1-OE lines of ‘Jinba’ were used to measure the activity of defense enzymes, namely, PAL, POD, CAT, and SOD. SOD and POD activities were measured following the protocol by Sun et al. (2013). CAT and PAL activities were measured following the protocol by Kwon and Liu (Kwon and Nguyen, 2003; Liu et al., 2005). All experiments were performed with three biological replicates.
RNA Extraction, cDNA Library Construction, and Sequencing
The Illumina HiSeq-2000 platform (Illumina Inc., San Diego, CA, United States) by Wuhan MetWare Biotechnology Co., Ltd., (1 Wuhan, China) was used for RNA-seq. A total of 12 high-quality RNA samples [six from before inoculation (0 h) and six from after inoculation] were prepared from the leaves of WT and CmWRKY15-1-RNAi lines of ‘C029.’ First, samples were obtained from uninoculated WT and CmWRKY15-1-RNAi lines of ‘C029’ (0 h). Three samples were collected per strain for a total of six samples (labeled W0 and R0). WT and CmWRKY15-1-RNAi lines were then sampled at 24, 48, and 72 h after inoculation with P. horiana. Samples at specific time points were combined to form six inoculated samples, labeled WJ and RJ. Because most eukaryotic mRNAs have poly-A tails, mRNAs were enriched using oligo(dT) magnetic beads. Examination of RNA quality, cDNA library construction, and Illumina sequencing library construction were performed as previously described (Ana et al., 2016; Chuang et al., 2020).
Identification and Functional Analysis of Differentially Expressed Genes
We assembled pathogen-sequence-free reads from the above RNA-seq data into contigs using the Trinity program. All contigs were analyzed with RNA-seq by Expectation-Maximization software to map the clean reads of each sample to the transcriptional reference sequence obtained via Trinity splicing (Michael et al., 2014). Next, we obtained the raw counts for each unigene and used the DESeq R package to identify DEGs between W0 vs. WJ, R0 vs. RJ, and WJ vs. RJ. The parameter standards included a cut-off of |log2(fold change)| > 1 and a false discovery rate < 0.05. Finally, we analyzed unigene sequences from the NCBI non-redundant (NR) database and euKaryotic Ortholog Groups (KOG) databases and annotated DEGs between W0 vs. WJ, R0 vs. RJ, and WJ vs. RJ using the Gene Ontology (GO) and Kyoto Encyclopedia of Genes and Genomes (KEGG) pathway databases.
Quantitative Reverse Transcription Polymerase Chain Reaction
To confirm the RNA-seq results, seven disease-resistance DEGs in the SA pathway were selected for verification by quantitative reverse transcription PCR (RT-qPCR). Primers used are shown in Supplementary Table 4. Leaves of the ‘C029’ WT and CmWRKY15-1-RNAi lines were harvested at 0 and 48 h after treatment with P. horiana. A PrimeScript™ RT reagent Kit with gDNA Eraser (Perfect Real Time), PrimeScript™ II 1st Strand cDNA Synthesis Kit, and SYBR® Premix ExTaq™ II were purchased from Takara. RT-qPCR was performed using an SYBR® Premix ExTaq™ II, following the protocols of the manufacturer. The 2–ΔΔCT method was used to calculate the expression levels of these disease resistance DEGs (Livak and Schmittgen, 2008). All experiments were performed with three biological replicates.
Isolation and Sequencing Analysis of CmNPR1
One DEG, CmNPR1, was identified by sequencing. The coding sequence (CDS) of CmNPR1 was cloned from ‘C029’ by PCR with CmNPR1 forward (F) and reverse (R) primers and was cloned into pEASY®-T1 using a Simple Cloning Kit (TransGen Biotech, Beijing). Primers are shown in Supplementary Table 4. This vector was transformed into Escherichia coli DH5α (Aidlab) and clones harboring the inserts were identified for subsequent experiments. The cDNA and deduced protein sequences from CmNPR1 were analyzed using DNAMAN software. The online tool ExPASy2 was used to predict the physicochemical properties of CmNPR1, and the SOPMA3 website and Phyre2 database were used to predict protein structure.4 Finally, MEGA 5.0 software was used for the phylogenetic analysis of CmNPR1 proteins.
Functional Analysis of CmNPR1
To investigate the potential function of CmNPR1, the silencing vector pTRV2-CmNPR1 was constructed, and expression of the gene was silenced using the virus-induced gene silencing (VIGS) technique in ‘C029.’ Leaves of WT and TRV-NPR1 lines were sampled at 0, 24, 48, and 72 h after inoculation with P. horiana. The relative expression levels of CmPR1, CmPR2, and CmPR10 were quantified by RT-qPCR. All experiments were performed with three biological replicates. Primers are shown in Supplementary Table 4.
Isolation of the CmNPR1 Promoter and Glucuronidase Staining
The primers NPR1SP1, NPR1SP2, and NPR1SP3 were synthesized by hiTAIL-PCR following the protocol of the manufacturer (Chen and Liu, 2007). Through genome walking, we obtained the 1,685-bp promoter region of CmNPR1 upstream of the ATG codon. Promoter elements of CmNPR1 were analyzed using the Plant CARE5 and PLACE6 websites. The CmNPR1 promoter was amplified by PCR with specific primers CmNPR1.1-F/R, CmNPR1.2-F/R, and CmNPR1.3-F/R (based on the predicted cis-acting elements in this promoter) using ‘C029’ DNA as a template. The 1,685-bp CmNPR1 promoter region was obtained, along with truncated sequences 850 and 450 bp long. Primers used are shown in Supplementary Table 4. These sequences were ligated into the pCAMBIA1301 vector, replacing the 35S promoter, to form the recombinant expression vectors pNPR1.1:GUS, pNPR1.2:GUS, and pNPR1.3:GUS, respectively. The vectors were introduced into Agrobacterium tumefaciens strain EHA105. Combination vectors were transformed into ‘C029’ via Agrobacterium-mediated transient transformation. Leaves of WT and transgenic plants at 0, 24, 48, and 72 h after treatment with P. horiana were subjected to β-glucuronidase (GUS) staining using a GUS staining kit (Real-Times Biotechnology Co., Ltd., Beijing, China). All experiments were performed with three biological replicates.
Yeast One-Hybrid Assay
Yeast one-hybrid (Y1H) assays were conducted using the Matchmaker™Gold Yeast One-Hybrid System (Clontech, Palo Alto, CA, United States) for experimental validation. The full-length 1,685-bp CmNPR1 promoter was ligated into the pAbAi vector, generating pNPR1-AbAi. The linearized vector was digested with a single endonuclease and transfected into yeast strain Y1HGold and used as bait. The CDS of CmWRKY15-1 was cloned into pGADT7 to generate the prey vector pGAD-CmWRKY15-1; the pGADT7 vector and bait constructs were transformed into yeast cells as a negative control. Subsequently, all constructs were transformed into the Y1HGold strain. Finally, the colonies were plated on synthetic defined (SD) medium without leucine (SD/–Leu) and SD/–Leu supplemented with 800 ng/ml aureobasidin A (AbA) and allowed to grow for 3 days at 28°C. The CmNPR1 promoter was divided into two segments based on analysis of the cis-acting elements PA1 and PA2. PA1 contains the critical regulatory W-box element of the CmNPR1 promoter. Two fragments were cloned into the pAbAi vector to produce new recombinant vectors pA1-AbAi and pA2-AbAi. These two bait vectors, and the prey vector pGAD-CmWRKY15-1, were transferred into Y1HGold. The transformants were grown on medium with 800 ng/ml SD/–Leu with AbA and cultivated at 28–30°C for 3 days. All experiments were performed with three biological replicates. Primers are shown in Supplementary Table 4.
Bimolecular Fluorescence Complementation Assay
Bimolecular fluorescence complementation (BiFC) vectors were constructed for the transient transformation of Nicotiana benthamiana. The CDSs of CmNPR1 and CmWRKY15-1 were cloned into the p2YC and p2YN vectors using the BiFC-NPR1-F1/BiFC-NPR1-R1 and BiFC-WRKY15-1-F1/BiFC-WRKY15-1-R1 primers via PacI/SpeI, generating p2YC-CmNPR1 and p2YN-CmWRKY15-1, respectively. Cells of A. tumefaciens strain GV3101 carrying the BiFC constructs were infiltrated into 4- to 5-week-old N. benthamiana leaves. Yellow fluorescent protein (YFP) signals in the N. benthamiana leaf cells were observed under a laser scanning confocal microscope. All experiments were performed with three biological replicates. Primers are shown in Supplementary Table 4.
Statistical Analysis
Data were analyzed using ANOVA and t-tests to determine significant differences with SPSS 24.0 software.
Results
CmWRKY15-1 Functions in Resistance to Puccinia horiana by Regulating Reactive Oxygen Species Levels
To determine whether ROS are involved in resistance to P. horiana, we performed DAB staining to measure the H2O2 content in leaves. More H2O2 accumulated in WT than in CmWRKY15-1-OE lines after inoculation with P. horiana (Figure 1A). We also quantified endogenous H2O2 content. The levels of H2O2 increased over time and peaked at 48 h in WT and CmWRKY15-1-OE lines after inoculation with P. horiana. Compared with the WT, CmWRKY15-1-OE lines had lower H2O2 levels after inoculation with P. horiana, which is consistent with the results of DAB staining (Figure 1B). This result indicated that OE CmWRKY15-1 enhanced plants’ resistance to P. horiana by inhibiting the accumulation of ROS.
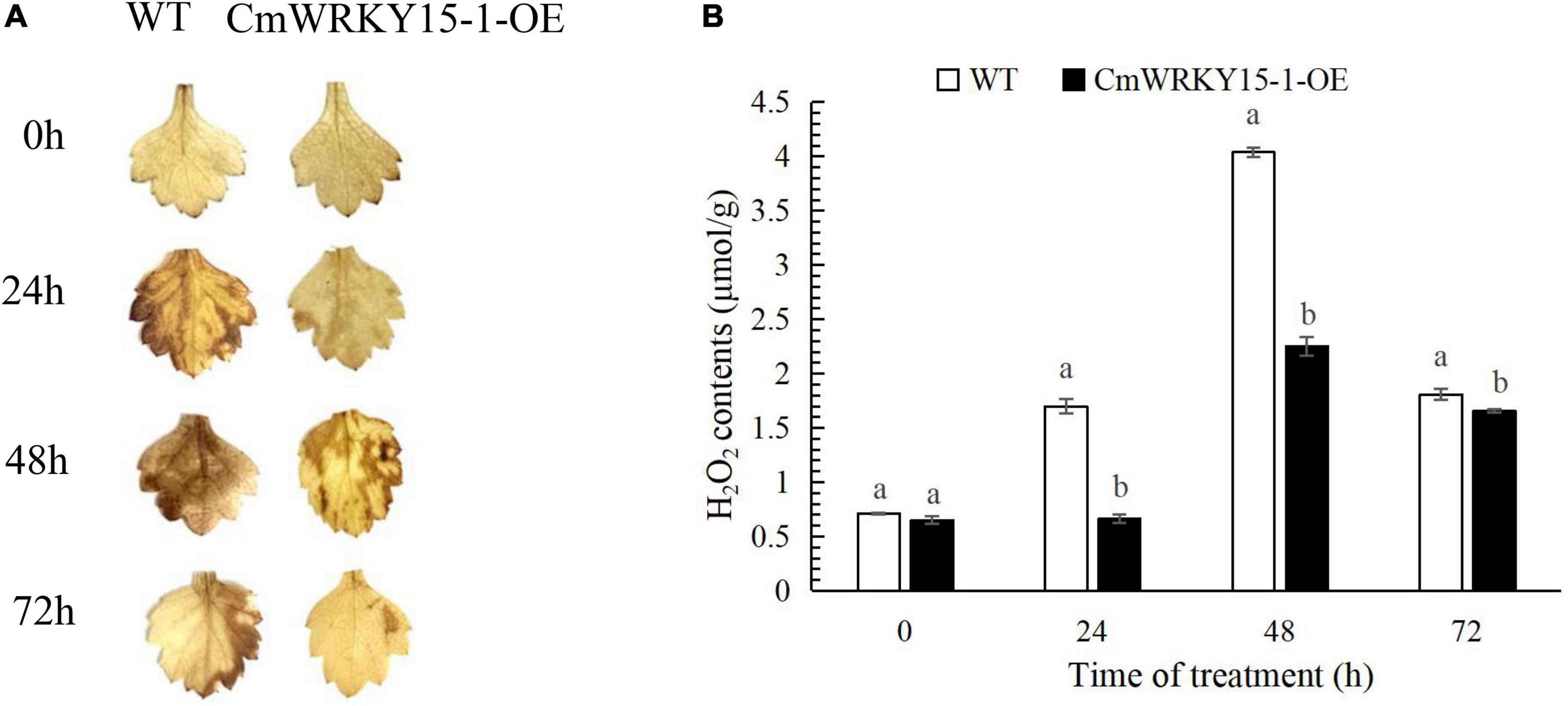
Figure 1. Changes in the levels of H2O2 in wild-type (WT) and CmWRKY15-1-overexpressing (OE) lines of ‘Jinba.’ (A) DAB staining of detached leaves to observe H2O2 levels. (B) Measurement of H2O2 content in leaves. Different letters demonstrate significantly different by Duncan’s multiple range test at p < 0.05.
Overexpression of CmWRKY15-1 Resulted in Increased Enzyme Activities
We measured PAL, POD, SOD, and CAT activities in leaf samples leaves from WT and CmWRKY15-1-OE lines of ‘Jinba.’ The activities of all four enzymes showed an upward trend and then a decrease at 72 h both in WT and CmWRKY15-1-OE lines following inoculation with P. horiana. The activities of these enzymes were significantly higher in CmWRKY15-1-OE lines than in the WT. SOD and POD activities reached their highest levels at 48 h in both WT and CmWRKY15-1-OE lines. CAT and PAL activities peaked at 24 h in WT and CmWRKY15-1-OE lines (Figure 2). Our results showed that the overexpression of CmWRKY15-1 helps to activate defense systems to respond to infection with P. horiana.
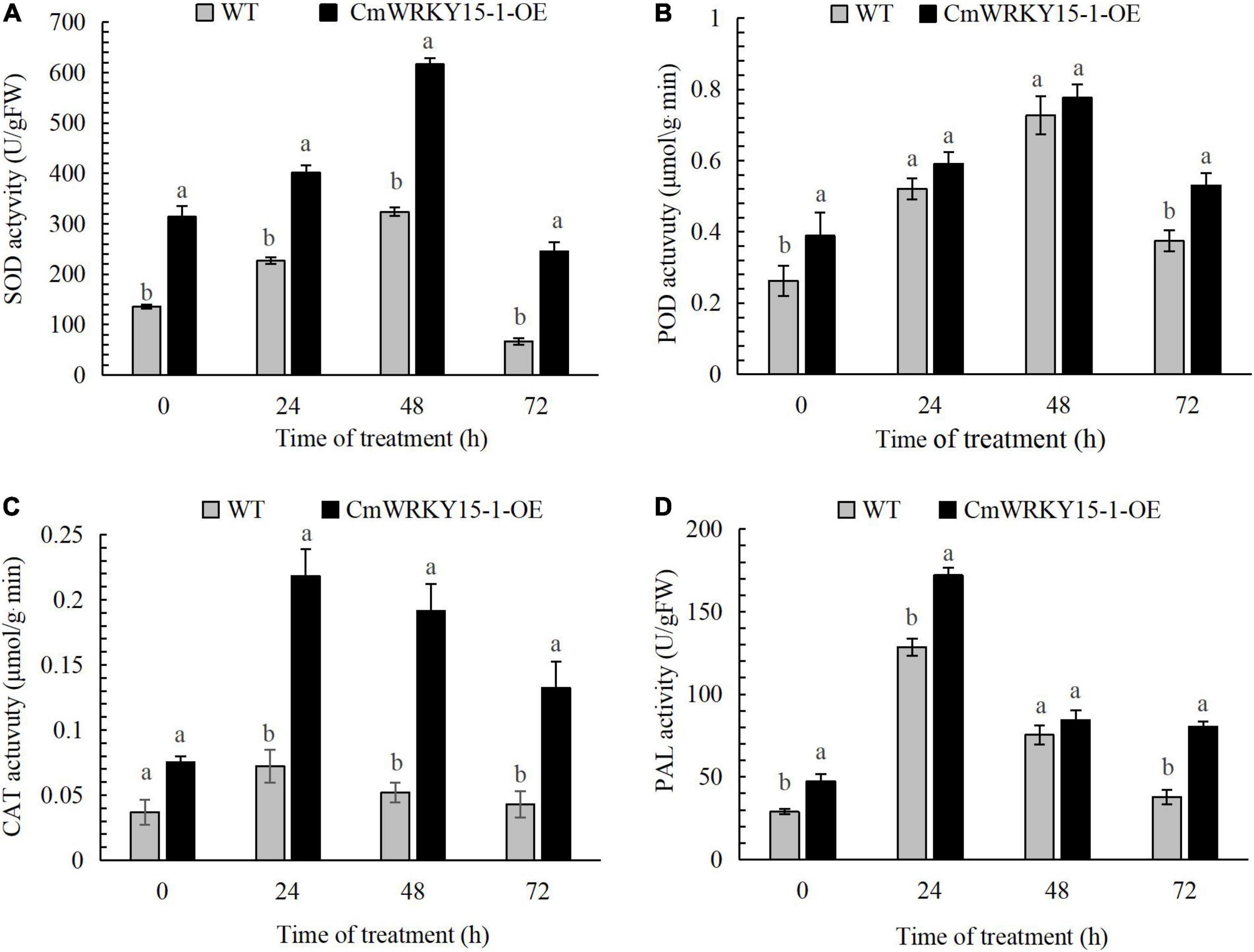
Figure 2. Activity of defense enzymes in WT and CmWRKY15-1-OE lines of ‘Jinba.’ (A) SOD activity. (B) POD activity. (C) CAT activity. (D) PAL activity. Error bars show SD of three replicates. Different letters demonstrate significantly different by Duncan’s multiple range test at p < 0.05.
RNA-Seq Data Analysis
Approximately 100.8 GB of clean reads were acquired from 12 samples. The average Q20 and Q30 values of the clean reads were 95.56% and 89.94%, respectively, showing that all clean reads were of high quality (Supplementary Table 1). We successfully annotated 272,669 unigenes via comparisons with databases, namely, KOG, NR, Swiss-Prot, GO, KEGG, Pfam, and TrEMBL. Results are shown in Supplementary Table 2. We performed a statistical analysis of the unigenes with homologs in the NR database to determine the species distribution of homologous sequences. The top three species with the most annotated homologous genes were sunflower (Helianthus annuus), lettuce (Lactuca sativa), and cardoon (Cynara cardunculus), accounting for 35.56, 19.09, and 16.63% of the total, respectively (Supplementary Figure 1). We also annotated the 53,463 unigenes in the KOG database. The highest number of genes (11,233) belonged to the R category: General Function Prediction Only (Supplementary Figure 2).
Differentially Expressed Genes Were Analyzed Among Three Comparison Pairs
To achieve a comprehensive insight into the functions and mechanisms of DEGs in ‘C029’ after inoculation with P. horiana, we analyzed the DEGs among three comparison pairs: W0 vs. WJ, R0 vs. RJ, and WJ vs. RJ. The expression levels of 5,449 and 6,128 genes significantly differed in W0 vs. WJ and in R0 vs. RJ, respectively. In addition, we also found 1,684 DEGs in WJ vs. RJ. To explore the functions of these DEGs, they were annotated using GOseq. The annotations were grouped into three categories: cellular components, molecular functions, and biological processes. In the cellular components category, the “cell” and “cell part” subclasses were the most enriched in W0 vs. WJ, R0 vs. RJ, and WJ vs. RJ. In the molecular functions category, the most highly enriched GO terms were “catalytic activity” and “binding” in W0 vs. WJ, R0 vs. RJ, and WJ vs. RJ (Supplementary Figure 3). Analysis of the biological process category showed that the “cellular process” and “metabolic process” subclasses were the most abundant in W0 vs. WJ, R0 vs. RJ, and WJ vs. RJ.
We used KEGG enrichment pathway analysis to explore the molecular mechanisms of these DEGs. Most of the DEGs in W0 vs. WJ, R0 vs. RJ, and WJ vs. RJ belonged to the KEGG pathways “metabolic pathway” and “biosynthesis of secondary metabolites” (Supplementary Figure 5). A Venn diagram created to visualize the number of DEGs between W0 vs. WJ, R0 vs. RJ, and WJ vs. RJ revealed an overlap of 65 DEGs (Supplementary Figure 4).
Changes in Candidate Differentially Expressed Genes Expression Are Closely Related to the RNA-Seq Data
To validate the reliability of DEGs identified by RNA-seq analysis, we analyzed the expression levels of seven candidate genes in the SA signaling pathway from the 65 DEGs between W0 vs. WJ, R0 vs. RJ, and WJ vs. RJ via RT-qPCR (Supplementary Table 3). These genes included two SA synthetic signaling pathway genes (CmPAL1 and CmPAL2), three SA-response-related genes (CmPR1, CmPR5, and CmNPR1), and two TGA transcription factor genes (CmTGA1 and CmTGA3). Our results showed that the RT-qPCR data were closely related to the RNA-seq data. In the silenced lines, expression of DEGs was inhibited, as their expression levels were always lower in these lines than in the WT (Figure 3). One DEG, CmNPR1, is an important regulator and a core point in the downstream signal transduction network of plant disease resistance. Expression of this gene significantly increased in both the WT and CmWRKY15-1-RNAi lines after treatment with P. horiana.
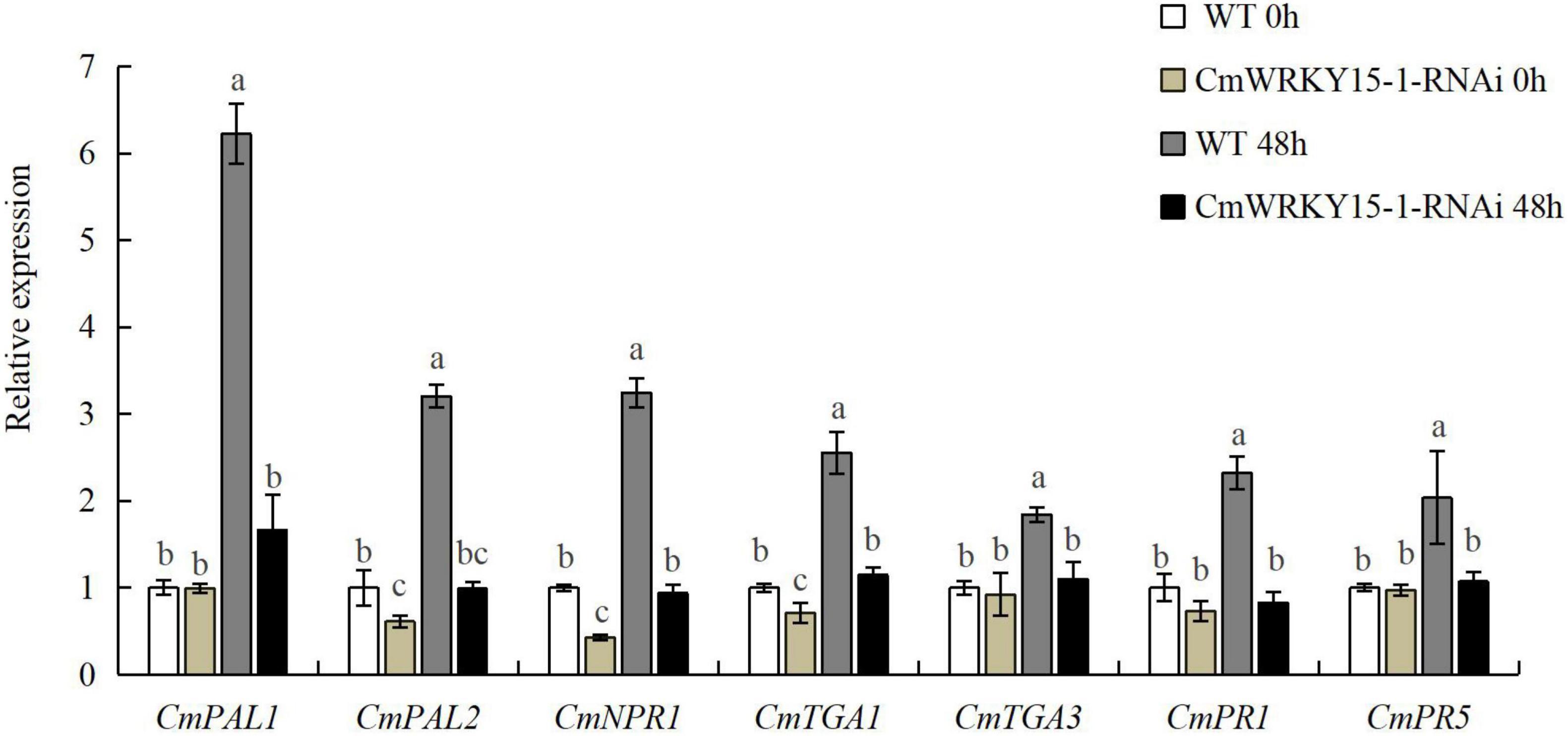
Figure 3. Expression of differentially expressed genes (DEGs) in wild-type (WT) and CmWRKY15-1-RNA interference (RNAi) lines of ‘C029.’ Error bars show standard deviation of three replicates. Different letters demonstrate significantly different by Duncan’s multiple range test at p < 0.05.
Isolation and Sequence Analysis of CmNPR1
We cloned the CDS of CmNPR1 from ‘C029,’ showing that it is 1,670 bp long and encodes a 558-amino-acid protein (Figure 4A). Analysis of the CmNPR1 protein sequence revealed that it has 97, 77, and 78% sequence similarity to the NPR protein sequences from sweet wormwood (Artemisia annua), H. annuus, and C. cardunculus, respectively (Figure 4B), confirming that this gene is CmNPR1. According to the predicted results, the CmNPR1 protein N-terminal region includes an evident BTB/POZ domain between amino acids 137 and 552; this domain is a potential target of the Cullin3-E3 ligase ubiquitin degradation pathway. An ANK domain was present in the middle region of this protein at amino acids 802–1,059. This domain is responsible for mediating the interaction between NPR1 and WRKY or TGA. The C-terminal NPR1/NIM1 region (between amino acids 1,102 and 1,632) and the N-terminal BTB/POZ domain participate in the binding of NPR1 to components of the SA signaling pathway.
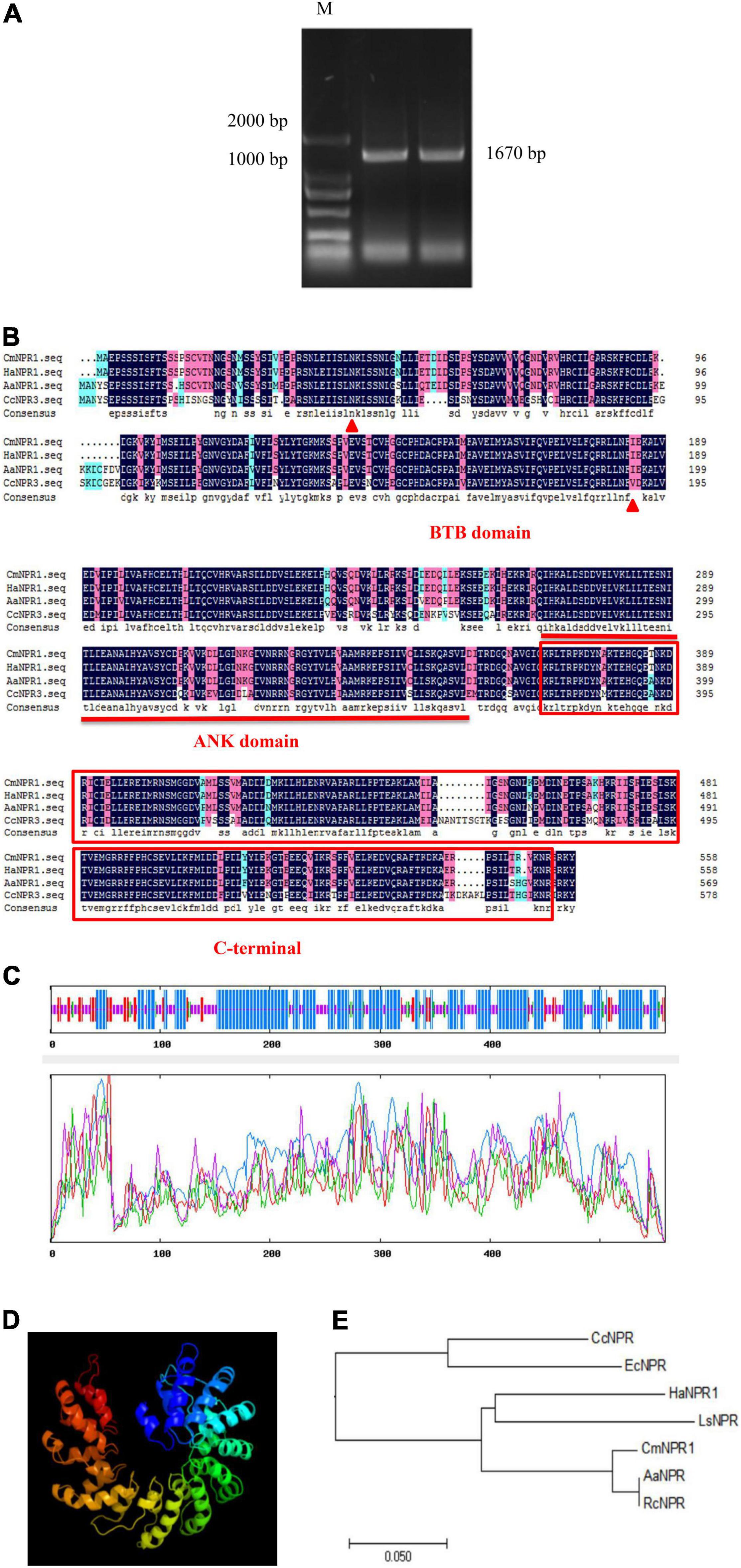
Figure 4. (A) PCR amplification product of CmNPR1: M, Marker. (B) Sequence alignment analysis between CmNPR1 and other several related NPR1 proteins from other Asteraceae. Triangle, N-terminal BTB/POZ domain; straight line, ANK domain; box, C-terminal NPR1/NIM1 region. Cm, Chrysanthemum morifolium; Ha, Helianthus annuus L.; Aa, Artemisia annua; Cc, Cynara cardunculus L. (C) The predicted secondary structure of CmNPR1. Blue, alpha helix; red, folding and extending chain; green, beta turn; yellow, random coil. (D) The predicted three-dimensional structure of CmNPR1. (E) Phylogenetic analysis of CmNPR1. Rc, Ricinus communis; Ls, Lactuca sativa; Ec, Erigeron canadensis.
Analysis using ExPASy revealed that NPR1 protein primarily comprises leucine, serine, valine, glutamic acid, aspartic acid, and lysine residues, which account for 12.2, 9.1, 8.2, 7.5, 7.2, and 7.0% of the amino acid residues, respectively. The molecular formula of the protein is C2774H4501N773O847S29. Based on an analysis of the predicted protein structure, we submitted the CmNPR1 protein sequences to SOPMA to predict its secondary structure. Of the 310 alpha-helices present in CmNPR1, 176 were part of a random coil, 52 were part of an extension strand, and 21 were part of a beta turn, accounting for 55.46, 31.48, 9.30, and 3.76% of the protein, respectively (Figure 4C). We predicted its tertiary structure using the Phyre2 database (Figure 4D). In addition, a comparison of CmNPR1 with other proteins from various species indicated that it is most similar to AaNPR from A. annua and RcNPR1 from the castor bean (Ricinus communis) (Figure 4E).
CmNPR1 Silencing Resulted in Decreased Expression of Pathogenesis-Related Genes
To verify the function of CmNPR1, we obtained CmNPR1-silenced lines (TRV-NPR1) of ‘C029’ generated by VIGS. We then performed RT-qPCR to identify any changes in the expression of these downstream PR genes associated with plant defense in WT and TRV-NPR1 lines. Three genes, CmPR1, CmPR2, and CmPR10, showed lower expression in the silenced plants than in the WT controls at 0, 24, 48, and 72 h. Transcript levels of all three genes initially increased, peaked at 48 h, and then decreased in both WT and TRV-NPR1 lines (Figure 5). The results showed that CmNPR1 can respond to infection with P. horiana and induce the expression of PR genes.
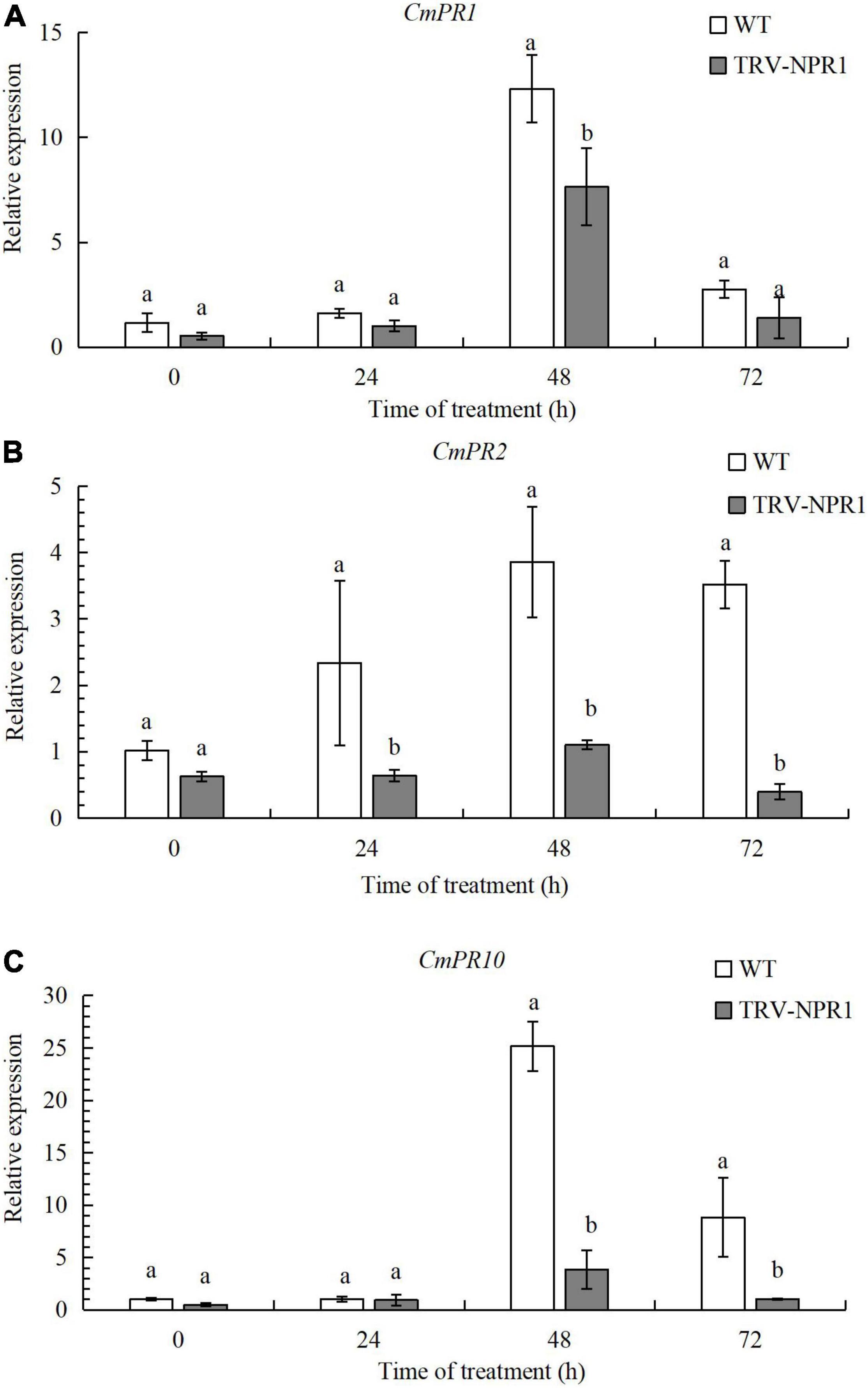
Figure 5. Transcript levels of CmPR1 (A), CmPR2 (B), and CmPR10 (C) genes determined by RT-qPCR. Error bars show SD of three replicates. Different letters demonstrate significantly different by Duncan’s multiple range test at p < 0.05.
The CmNPR1 Promoter Can Respond to Puccinia horiana Infection
We cloned the 1,685-bp promoter of CmNPR1 by hiTAIL-PCR (Figure 6A). We analyzed cis-acting elements in the CmNPR1 promoter using the Plant CARE and PLACE databases. Numerous motifs were discovered in the CmNPR1 promoter, including core cis-acting elements: 13 transcription start site TATA-boxes; 19 CAAT-boxes; one MYB element (GA-responsive); one SARE sequence; seven as-1 (SA-responsive) elements; one TGACG motif [methyljasmonate (MeJA)-responsive]; one AT-rich sequence (stress-responsive); three GT1 motifs (light responsive); and five W-boxes (Figure 6B). The W-box is a binding site for WRKY transcription factors, suggesting that the promoter of CmNPR1 may be regulated by the transcription factor CmWRKY15-1. Based on analysis of cis-acting elements, using ‘C029’ DNA as a template, we constructed three expression vectors with the GUS gene driven by three promoter sequences and obtained by PCR (in place of the Cauliflower mosaic virus 35S promoter): the full-length CmNPR1 promoter sequence and two truncated sequences (Figure 6C). To explore the mechanism of CmNPR1 promoter fragments on P. horiana response, we transiently transformed three promoter fragments into ‘C029.’ We sampled leaves at different time points after inoculation with P. horiana and evaluated GUS activity. Little GUS staining was observed in any of the transgenic leaves at 0 h. Compared with the mock control, the three transgenic leaves (harboring pNPR1.1:GUS, pNPR1.2:GUS, and pNPR1.3:GUS) gradually turned blue with increasing time after inoculation, with the deepest staining observed at 48 h, after which staining decreased again. GUS activity driven by the CmNPR1 promoter was significantly upregulated after treatment with P. horiana, and GUS activity driven by the CmNPR1.1 promoter (pNPR1.1: GUS) was significantly higher than that of pNPR1.2:GUS and pNPR1.3:GUS (Figure 6D). We propose that CmNPR1 has a pathogen-inducible promoter and can be induced by P. horiana. In addition, the change in CmNPR1 expression levels in ‘C029’ leaves after treatment with P. horiana is closely related to its change in promoter activity.
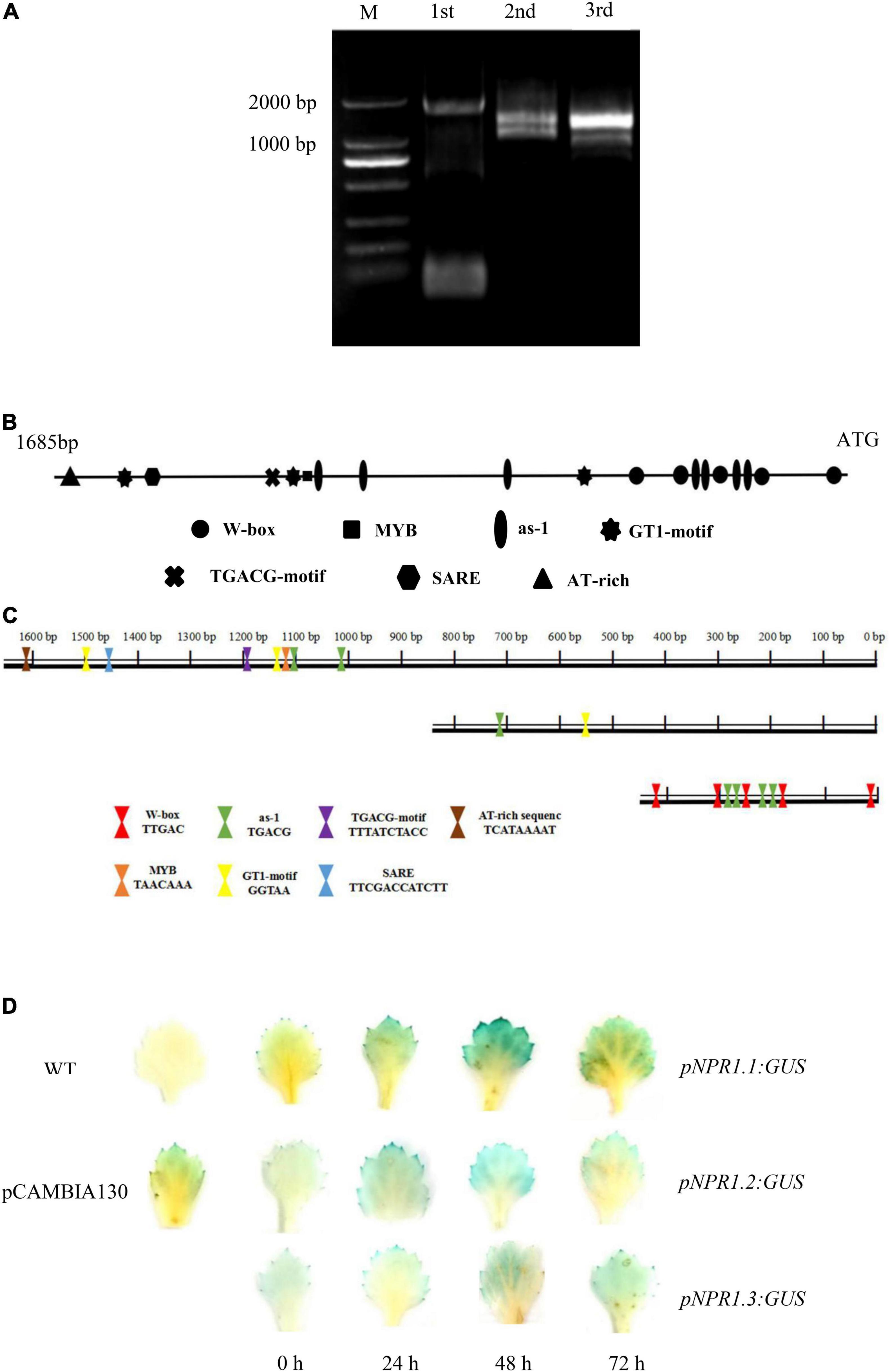
Figure 6. Analysis of the CmNPR1 promoter. (A) Amplification products of the CmNPR1 promoter obtained by hiTAIL-PCR: M, Marker; 1st: First round PCR; 2nd: Second round PCR; 3rd: Third round PCR. (B) Analysis of cis-elements in the CmNPR1 promoter. (C) Diagram of the pNPR1.1, pNPR1.2, and pNPR1.3 vectors. (D) GUS histochemical staining to analyze expression of the CmNPR1 promoter in response to the pathogen. WT, wild-type negative control; pCAMBIA1301, positive control.
CmNPR1 Expression Can Be Regulated by CmWRKY15-1
The interaction between the promoter of full-length CmNPR1 and the CmWRKY15-1 protein was verified with a Y1H assay. We transferred the bait (pNPR1-AbAi.) and prey (pGAD-CmWRKY15-1) vectors into Y1HGold yeast cells; pGADT7 and p53 were used as a positive control. The strain was able to grow on plates of SD/-Leu medium supplemented with 800 ng/ml AbA when pNPR1-AbAi was coexpressed with pGAD-CmWRKY15-1 in yeast (Figure 7B). The CmNPR1 promoter was divided into two fragments, PA1 and PA2, and the promoter region PA1 contains W-box elements (Figure 7A). We transformed Y1HGold cells with the pPA1-AbAi or pPA2-AbAi bait vector and the pGAD-CmWRKY15-1 prey vector and plated the cells on SD/-Leu medium containing 800 ng/ml AbA. Y1H assays showed that CmWRKY15-1 interacted with fragment PA1 but not PA2 (Figure 7A). Therefore, CmWRKY15-1 can bind to the W-box elements in the promoter of its target gene CmNPR1. These results show that CmWRKY15-1 has DNA binding activity and specifically binds to the CmNPR1 promoter.
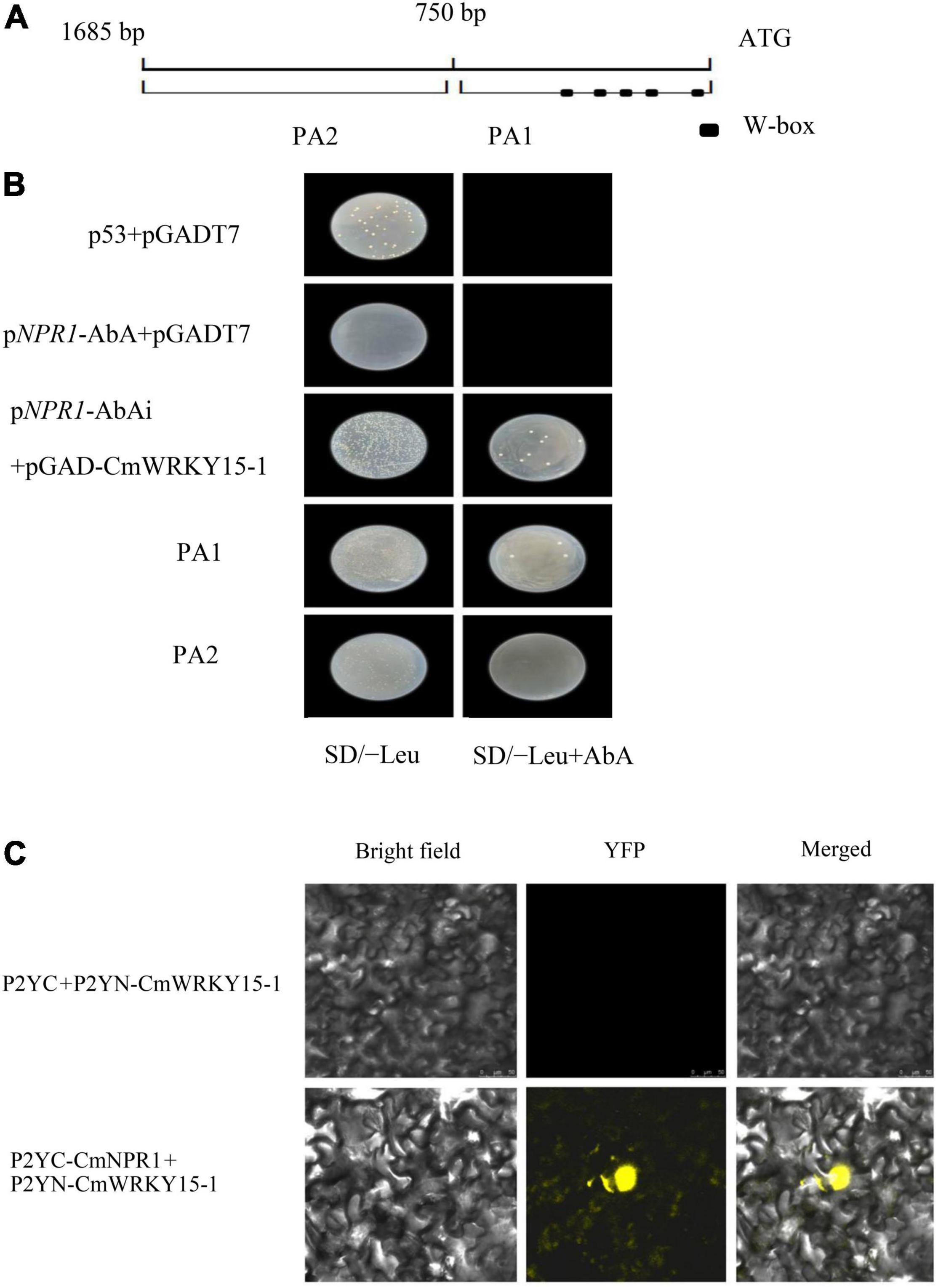
Figure 7. Regulatory relationship between CmWRKY15-1 and CmNPR1. (A) Schematic diagram of the 1,600-bp CmNPR1 promoter. PA1, 0 to –750 bp; PA2, –750 to –1,685 bp; squares indicate W-box elements. (B) Yeast one-hybrid (Y1H) analysis of CmWRKY15-1 binding to the CmNPR1 promoter. (C) Fluorescence confocal detection of Nicotiana benthamiana mesophyll cells. Bright field, visible images; YFP, yellow fluorescent protein images; Merged, merged fluorescence, and visible images.
CmWRKY15-1 Can Interact With CmNPR1
Finally, to examine the interaction between CmWRKY15-1 and CmNPR1, we performed a BiFC analysis. We cloned the CDS of CmWRKY15-1 and CmNPR1 into vectors containing the N-terminal of p2YN and C-terminal regions of p2YC and then cotransfected N. benthamiana leaves with these constructs (p2YC-CmNPR1 and p2YN-CmWRKY15-1). The p2YC vector coexpressed with p2YN-CmWRKY15-1 vectors served as the negative controls. YFP signal was observed only in N. benthamiana leaves coexpressing p2YC-CmNPR1 and p2YN-CmWRKY15-1, but not in cells coexpressing the negative control constructs (Figure 7C). This indicates that CmWRKY15-1 and CmNPR1 interact in vivo.
Discussion
Chrysanthemum is an important ornamental flower in China and around the world. ‘C029’ is a chrysanthemum cultivar with broad-spectrum resistance to pathogenic bacteria and fungi, including CWR due to the fungus P. horiana, which causes serious losses in chrysanthemum. Via RNA-seq analysis, we had previously isolated the disease resistance gene CmWRKY15-1 from chrysanthemum following P. horiana infection and determined that SA content increased significantly after pathogen infection in CmWRKY15-1-OE lines. In addition, CmWRKY15-1-OE lines showed higher resistance to P. horiana. These results show that CmWRKY15-1 positively regulates resistance to P. horiana by regulating the levels of SA.
Plant defense responses involve complex signal transduction mechanisms. The activation of SAR is accompanied by the production of several signaling molecules. Pathogens induce SA biosynthesis and downstream defense responses by activating R-gene-mediated defense signaling (Chen et al., 1993; Gonzalez et al., 2015). In addition, activation of the R gene induces ROS production and HR at the site of pathogen infection (Ji et al., 2021). In this study, we observed that more ROS accumulated in WT than in CmWRKY15-1-OE lines of ‘Jinba’ after P. horiana inoculation. We also quantified endogenous H2O2 content and found that, as expected, it was higher in WT than in CmWRKY15-1-OE lines of ‘Jinba’ after P. horiana inoculation, which is consistent with the above findings. Our results suggest that CmWRKY15-1 participates in plant resistance to P. horiana by regulating ROS levels.
The activities of defensive enzymes such as SOD, CAT, POD, and PAL are generally an indicator of plant resistance to stress (Choudhury et al., 2017). Given that CmWRKY15-1-OE lines of ‘Jinba’ accumulated less H2O2, we measured the activities of these four defense enzymes in WT and CmWRKY15-1-OE lines after pathogen infection. The activities of all four enzymes were higher in CmWRKY15-1-OE lines than in WT after inoculation with P. horiana. In particular, PAL activity increased significantly after the inoculation and was significantly higher in CmWRKY15-1-OE lines than in WT. In addition, we showed that the CmPAL1 and CmPAL2 expression was upregulated after treatment with P. horiana. This suggests that SA may be synthesized through the PAL pathway to help plants resist P. horiana infection.
To further explore the resistance mechanism, we performed RNA-seq and identified one DEG, CmNPR1, between WT lines of the P. horiana-resistant cultivar ‘C029’ and ‘C029’ lines with CmWRKY15-1 silenced by RNAi. NPR1 is a key regulator of the SAR signal transduction pathway downstream of SA (Ding et al., 2018). Change in NPR1 expression affects the generation of SAR in plants; in the absence of NPR1, plants cannot respond to various SAR inducers, and PR genes are rarely expressed. In addition, NPR1 induces various defensive reactions, causing plants to exhibit a broad spectrum of resistance responses (Fu and Dong, 2013; Pajerowska-Mukhtar et al., 2013). AtNPR1 and its orthologs in various plants enhance resistance to biotrophic fungal and bacterial pathogens (Le et al., 2009; Nic-Matos et al., 2017). NPR1 improves disease resistance in Arabidopsis, rye (Secale cereale), and Cape periwinkle (Catharanthus roseus) by regulating the expression of PR genes (Hui et al., 1994; Yu et al., 2017; Sung et al., 2019). In this study, when we cloned the CmNPR1 gene from chrysanthemum ‘C029’ and obtained CmNPR1-silenced ‘C029’ lines, they showed decreased levels of PR gene expression after infection with P. horiana. These results indicate that CmNPR1 participates in the response of chrysanthemum to white rust by regulating the expression of PR genes. Furthermore, we isolated the CmNPR1 promoter and demonstrated that it responds to P. horiana, as revealed by GUS staining. We also showed that CmWRKY15-1 can bind to the CmNPR1 promoter region to activate its expression. Finally, the interaction between CmNPR1 and CmWRKY15-1 was verified by BiFC.
We propose a model for the mechanism underlying the defense response against P. horiana in ‘C029.’ When the plant is infected by P. horiana, ETI is triggered to modulate the level of ROS to resist P. horiana invasion. At the same time, SA is synthesized via the PAL pathway and promotes CmNPR1 interaction with CmWRKY15-1, thereby regulating a change in the expression of downstream PR genes to improve disease resistance. In addition, CmWRKY15-1 might also bind to the W-box in the CmNPR1 promoter to activate its expression. This could result in a change in PR gene expression, thereby enhancing resistance to P. horiana (Figure 8). Our future research will focus on screening and identification of upstream genes of the SA pathway. Our results convey new insights into the mechanism of plant defense responses and provide evidence for the suitability of ‘C029’ as an important resource for chrysanthemum breeding.
Data Availability Statement
The datasets presented in this study can be found in online repositories. The names of the repository/repositories and accession number(s) can be found below: National Center for Biotechnology Information (NCBI) BioProject database under accession number PRJNA797165.
Author Contributions
HM and GG designed the study and wrote the manuscript. GG and RJ performed most of the experiments. DL, XZ, XS, and PZ analyzed the data and discussed the article. All authors revised and approved the manuscript.
Funding
This work was supported jointly by the National Natural Science Foundation of China (31972447).
Conflict of Interest
The authors declare that the research was conducted in the absence of any commercial or financial relationships that could be construed as a potential conflict of interest.
Publisher’s Note
All claims expressed in this article are solely those of the authors and do not necessarily represent those of their affiliated organizations, or those of the publisher, the editors and the reviewers. Any product that may be evaluated in this article, or claim that may be made by its manufacturer, is not guaranteed or endorsed by the publisher.
Supplementary Material
The Supplementary Material for this article can be found online at: https://www.frontiersin.org/articles/10.3389/fpls.2022.865607/full#supplementary-material
Footnotes
- ^ www.metware.cn
- ^ https://prosite.expasy.org/
- ^ https://npsa-prabi.ibcp.fr/
- ^ http://www.sbg.bio.ic.ac.uk/phyre2/html/
- ^ http://bioinformatics.psb.ugent.be/webtools/plantcare/html/
- ^ https://www.dna.affrc.go.jp/PLACE/
References
Alaei, H., Baeyen, S., Maes, M., Höfte, M., and Heungens, K. (2009). Molecular detection of Puccinia horiana in Chrysanthemum x morifolium through conventional and real-time PCR. J. Microbiol. Methods 76, 136–145. doi: 10.1016/j.mimet.2008.10.001
Ana, C., Pedro, M., Sonia, T., David, G. C., Alejandra, C., Andrew, M., et al. (2016). A survey of best practices for RNA-seq data analysis. Genome Biol. 17:13. doi: 10.1186/s13059-016-0881-8
Bi, M., Li, X., Yan, X., Liu, D., Gao, G., Zhu, P. F., et al. (2021). Chrysanthemum WRKY15-1 promotes resistance to Puccinia horiana Henn. Via the salicylic acid signaling pathway. Hortic. Res. 8:6. doi: 10.1038/s41438-020-00436-4
Chen, L., Wu, Q., He, T., Lan, J., Ding, L., Liu, T. F., et al. (2020). Transcriptomic and metabolomic changes triggered by Fusarium solani in common bean (Phaseolus vulgaris L.). Genes 11:177. doi: 10.3390/genes11020177
Chen, Y., and Liu, Y. L. (2007). High-efficiency thermal asymmetric interlaced PCR for amplification of unknown flanking sequences. Biotechniques 43, 649–656. doi: 10.2144/000112601
Chen, Z., Silva, H., and Klessig, D. F. (1993). Active oxygen species in the induction of plant systemic acquired resistance by salicylic acid. Science 262, 1883–1886. doi: 10.1126/science.8266079
Chern, M., Fitzgerald, H. A., Canlas, P. E., Navarre, D. A., and Ronald, P. C. (2005). Overexpression of a rice NPR1 homolog leads to constitutive activation of defense response and hypersensitivity to light. Mol. Plant Microbe Interact. 18, 511–520. doi: 10.1094/MPMI-18-0511
Choudhury, F. K., Rivero, R. M., Blumwald, E., and Mittler, R. (2017). Reactive oxygen species, abiotic stress and stress combination. Plant J. 90, 856–867. doi: 10.1111/tpj.13299
Chuang, M., Jie, Y., Peng, Y., Ning, L., Kai, M., Liqun, H., et al. (2020). Full-length transcriptome and targeted metabolome analyses provide insights into defense mechanisms of Malus sieversii against Agrilus mali. PeerJ 8:e8992. doi: 10.7717/peerj.8992
Deenamo, N., Kuyyogsuy, A., Khompatara, K., Chanwun, T., Ekchaweng, K., and Churngchow, N. (2018). Salicylic acid induces resistance in rubber tree against Phytophthora palmivora. Int. J. Mol. Sci. 19:1883. doi: 10.3390/ijms19071883
Ding, Y., Sun, T., Ao, K., Peng, Y., Zhang, Y., Li, X., et al. (2018). Opposite roles of salicylic acid receptors NPR1 and NPR3/NPR4 in transcriptional regulation of plant immunity. Cell 173, 1454–1467. doi: 10.1016/j.cell.2018.03.044
Dodds, P. N., and Rathjen, J. P. (2010). Plant immunity: towards an integrated view of plant–pathogen interactions. Nat. Rev. Genet. 11, 539–548. doi: 10.1038/nrg2812
Dong, L., Huang, Z. Q., Liu, Di, Zhu, P. F., Lv, S. J., Na, L., et al. (2018). Transcriptome analysis of chrysanthemum in responses to white rust. Sci. Hortic. 233, 421–430. doi: 10.1016/j.scienta.2018.01.016
Duan, Y., Jiang, Y., Ye, S., Karim, A., Ling, Z., He, Y. Q., et al. (2015). PtrWRKY73, a salicylic acid-inducible poplar WRKY transcription factor, is involved in disease resistance in Arabidopsis thaliana. Plant Cell Rep. 34, 831–841. doi: 10.1007/s00299-015-1745-5
Fu, Z. Q., and Dong, X. (2013). Systemic acquired resistance: turning local infection into global defense. Annu. Rev. Plant Biol. 64, 839–863. doi: 10.1146/annurev-arplant-042811-105606
Gao, J., Bi, W. S., Li, H. P., Wu, J. J., Yu, X. M., Liu, D. Q., et al. (2018). WRKY transcription factors associated with NPR1-mediated acquired resistance in barley are potential resources to improve wheat resistance to Puccinia triticina. Front. Plant Sci. 9:1486. doi: 10.3389/fpls.2018.01486
Gonzalez, T. L., Liang, Y., Nguyen, B. N., Staskawicz, B. J., Loqué, D., Hammond, M. C., et al. (2015). Tight regulation of plant immune responses by combining promoter and suicide exon elements. Nucleic Acids Res. 43, 7152–7161. doi: 10.1093/nar/gkv655
He, G. H., Xu, J. Y., Wang, Y. X., Liu, J. M., Li, P. S., Chen, M., et al. (2016). Drought-responsive WRKY transcription factor genes TaWRKY1 and TaWRKY33 from wheat confer drought and/or heat resistance in Arabidopsis. BMC Plant Biol. 16:116. doi: 10.1186/s12870-016-0806-4
Hui, C., Scott, A. B., Susan, G., and Dong, X. N. (1994). Characterization of an Arabidopsis mutant that is nonresponsive to inducers of systemic acquired resistance. Plant Cell 6, 1583–1592. doi: 10.1105/tpc.6.11.1583
Ji, Z., Yu, M. H., Ding, Y. Y., Li, J., Zhu, F., He, J. X., et al. (2021). Coiled-Coil N21 of Hpa1 in Xanthomonas oryzae pv. Oryzae promotes plant growth, disease resistance and drought tolerance in non-hosts via eliciting HR and regulation of multiple defense response genes. Int. J. Mol. Sci. 22:203. doi: 10.3390/ijms22010203
Keefe, O. G., and Davis, D. D. (2015). Morphology of Puccinia horiana, causal agent of chrysanthemum white rust, sampled from naturally infected plants. Plant Dis. 99, 1738–1743. doi: 10.1094/PDIS-02-15-0239-RE
Kumari, S., Kanth, B. K., Ahn, J. Y., Kim, J. H., and Lee, G. J. (2021). Genome-wide transcriptomic identification and functional insight of lily WRKY genes responding to Botrytis fungal disease. Plants 10:776.
Kwon, T. H., and Nguyen, H. L. (2003). Comparing constitutive promoters using CAT activity in transgenic tobacco plants. Mol. Cells 16, 117–122. doi: 10.1016/S0166-6851(03)00172-5
Le, H. G., Heitz, T., Mestre, P., Mutterer, J., Walter, B., and Chong, J. L. (2009). Characterization of Vitis vinifera NPR1 homologs involved in the regulation of pathogenesis-related gene expression. BMC Plant Biol. 9:54. doi: 10.1186/1471-2229-9-54
Li, M., Chen, H., Chen, J., Chang, M., Palmer, A., Gassmann, W., et al. (2018). TCP transcription factors interact with NPR1 and contribute redundantly to systemic acquired resistances. Front. Plant Sci. 9:1153. doi: 10.3389/fpls.2018.01153
Li, P., Song, A., Gao, C., Jiang, J., Chen, S., Fang, W., et al. (2015). The over-expression of a chrysanthemum WRKY transcription factor enhances aphid resistance. Plant Physiol. Biochem. 95, 26–34. doi: 10.1016/j.plaphy.2015.07.002
Liu, H. X., Jiang, W. B., Bi, Y., and Luo, Y. (2005). Postharvest BTH treatment induces resistance of peach (Prunus persica L. Cv. Jiubao) fruit to infection by Penicillium expansum and enhances activity of fruit defense mechanisms. Postharvest Biol. Technol. 35, 263–269. doi: 10.1016/j.postharvbio.2004.08.006
Livak, J. K., and Schmittgen, T. H. (2008). Analyzing real-time PCR data by the comparative CT method. Nat. Protoc. 3, 1101–1108. doi: 10.1038/nprot.2008.73
Long, Q., Du, M. X., Long, J. H., Xie, Y., Zhang, J. X., Xu, L. Z., et al. (2021). Transcription factor WRKY22 regulates canker susceptibility in sweet orange (Citrus sinensis Osbeck) by enhancing cell enlargement and CsLOB1 expression. Hortic. Res. 8:50. doi: 10.1038/S41438-021-00486-2
Michael, I. L., Wolfgang, H., and Simon, A. (2014). Moderated estimation of fold change and dispersion for RNA-seq data with DESeq2. Genome Biol. 15:550. doi: 10.1186/s13059-014-0550-8
Mou, Z., Fan, W., and Dong, X. (2003). Inducers of plant systemic acquired resistance regulate NPR1 function through redox changes. Cell 113, 935–944. doi: 10.1016/S0092-8674(03)00429-X
Nic-Matos, G., Narváez, M., Peraza-Echeverría, S., Sáenz, L., and Oropeza, C. (2017). Molecular cloning of two novel NPR1 homologue genes in coconut palm and analysis of their expression in response to the plant defense hormone salicylic acid. Genes Genomics 39, 1007–1019. doi: 10.1007/s13258-017-0566-z
Pajerowska-Mukhtar, K. M., Emerine, D. K., and Mukhtar, M. S. (2013). Tell me more: roles of NPRs in plant immunity. Trends Plant Sci. 18, 402–411. doi: 10.1016/j.tplants.2013.04.004
Resjö, S., Zahid, M. A., Burra, D. D., Lenman, M., Levander, F., and Andreassin, E. (2019). Proteomics of PTI and two ETI immune reactions in potato leaves. Int. J. Mol. Sci. 20:4726. doi: 10.3390/ijms20194726
Sun, J., Gu, J., Zeng, J., Han, S., Song, A., Chen, F. D., et al. (2013). Changes in leaf morphology, antioxidant activity and photosynthesis capacity in two different drought-tolerant cultivars of chrysanthemum during and after water stress. Sci. Hortic. 161, 249–258. doi: 10.1016/j.scienta.2013.07.015
Sung, Y., Lin, C., Hsu, H., Chen, Y., and Chen, J. (2019). Silencing of CrNPR1 and CrNPR3 alters plant susceptibility to periwinkle leaf yellowing phytoplasma. Front. Plant Sci. 10:1183. doi: 10.3389/fpls.2019.01183
Takatsu, Y., Ohishi, K., Tomita, Y., Hayashi, M., Nakajima, M., and Atsuy, K. (2008). Use of chrysanthemum plantlets grown in vitro to test cultivar susceptibility to white rust, Puccinia horiana P. Hennings. Plant Breed. 119, 528–530. doi: 10.1046/j.1439-0523.2000.00540.x
Torres, D. E., Rojas-Martínez, R. I., Zavaleta-Mejía, E., Guevara-Fefer, P., Márquez-Guzmán, G. J., and Caiolia, P. M. (2017). Cladosporium cladosporioides and Cladosporium pseudocladosporioides as potential new fungal antagonists of Puccinia horiana Henn., the causal agent of chrysanthemum white rust. PLoS One 12:e170782. doi: 10.1371/journal.pone.0170782
Torun, H. (2019). Time-course analysis of salicylic acid effects on ROS regulation and antioxidant defense in roots of hulled and hulless barley under combined stress of drought, heat and salinity. Physiol. Plant 165, 169–182. doi: 10.1111/ppl.12798
Tsuda, K., and Katagiri, F. (2010). Understanding the plant immune system. Mol. Plant Microbe Interact. 23, 1531–1536. doi: 10.1094/mpmi-04-10-0099
Wang, Y. J., Zeng, J., Xia, X. L., Xu, Y., Sun, J., Gun, L., et al. (2020). Comparative analysis of leaf trichomes, epidermal wax and defense enzymes activities in response to puccinia horiana in chrysanthemum and ajania species. Hortic. Plant J. 6, 191–198. doi: 10.1016/j.hpj.2020.03.006
Wang, Y. Q., Cui, X., Yang, B., Xu, S., Wei, X., Zhao, P. Y., et al. (2020). WRKY55 transcription factor positively regulates leaf senescence and defense response through modulating the transcription of genes implicated in ROS and SA biosynthesis in Arabidopsis. Development 147:dev189647. doi: 10.1242/dev.189647
Xin, J., Liu, Y., Li, H., Chen, S., Jiang, J., Song, A. P., et al. (2021). CmMLO17 and its partner CmKIC potentially support Alternaria alternata growth in Chrysanthemum morifolium. Hortic. Res. 8:101. doi: 10.1038/s41438-021-00534-x
Yoo, Y. K., and Roh, Y. S. (2014). Occurrence of white rust and growth of chrysanthemum ‘Baekma’ under various relative humidity and temperature conditions in the greenhouse. Korean J. Hortic. Sci. Technol. 32, 803–811. doi: 10.7235/hort.2014.14138
Yu, D., Chen, C., and Chen, Z. (2001). Evidence for an important role of WRKY DNA binding proteins in the regulation of NPR1 gene expression. Plant Cell 13, 1527–1540. doi: 10.1105/tpc.010115
Yu, G., Zhang, X., Yao, J., Zhou, M., and Ma, H. (2017). Resistance against fusarium head blight in transgenic wheat plants expressing the ScNPR1 gene. J. Phytopathol. 165, 223–231. doi: 10.1111/jph.12553
Yun, L. J., and Chen, W. L. (2011). SA and ROS are involved in methyl salicylate-induced programmed cell death in Arabidopsis thaliana. Plant Cell Rep. 30, 1231–1239. doi: 10.1007/s00299-011-1031-0
Zeng, J., Sun, J., Xu, Y., Chen, F. D., Jiang, J. F., Fang, W., et al. (2013). Variation for resistance to white rust (Puccinia horiana) among ajania and chrysanthemum species. HortScience 48, 1231–1234. doi: 10.21273/HORTSCI.48.10.1231
Zhang, W., Zhao, F., Jiang, L., Chen, C., Wu, L., and Liu, Z. B. (2018). Different pathogen defense strategies in arabidopsis: more than pathogen recognition. Cells 7:252. doi: 10.3390/cells7120252
Zhao, N., He, M., Li, L., Cui, S., Hou, M., Wang, L., et al. (2020). Identification and expression analysis of WRKY gene family under drought stress in peanut (Arachis hypogaea L.). Plos One 15:e231396. doi: 10.1371/journal.pone.0231396
Keywords: chrysanthemum white rust (CWR), resistance, CmWRKY15-1, regulation mechanism, CmNPR1
Citation: Gao G, Jin R, Liu D, Zhang X, Sun X, Zhu P and Mao H (2022) CmWRKY15-1 Promotes Resistance to Chrysanthemum White Rust by Regulating CmNPR1 Expression. Front. Plant Sci. 13:865607. doi: 10.3389/fpls.2022.865607
Received: 30 January 2022; Accepted: 16 March 2022;
Published: 27 April 2022.
Edited by:
Baoshan Chen, Guangxi University, ChinaReviewed by:
Youxiong Que, Fujian Agriculture and Forestry University, ChinaAdil Hussain, Abdul Wali Khan University Mardan, Pakistan
Copyright © 2022 Gao, Jin, Liu, Zhang, Sun, Zhu and Mao. This is an open-access article distributed under the terms of the Creative Commons Attribution License (CC BY). The use, distribution or reproduction in other forums is permitted, provided the original author(s) and the copyright owner(s) are credited and that the original publication in this journal is cited, in accordance with accepted academic practice. No use, distribution or reproduction is permitted which does not comply with these terms.
*Correspondence: Hongyu Mao, maohongyu@syau.edu.cn
†These authors have contributed equally to this work