- 1Key Laboratory of State Forestry Administration on Biodiversity Conservation in Karst Mountain Area of Southwest of China, School of Life Science, Guizhou Normal University, Guiyang, China
- 2Pharmacy College, Guizhou University of Traditional Chinese Medicine, Guiyang, China
Flower color, largely determined by anthocyanin, is one of the most important ornamental values of Rhododendron delavayi. However, scant information of anthocyanin biosynthesis has been reported in R. delavayi. We found that anthocyanidin 3-O-glycosides were the predominant anthocyanins detected in R. delavayi flowers accounting for 93.68–96.31% of the total anthocyanins during its development, which indicated the key role of flavonoid 3-O-glycosyltransferase (3GT) on R. delavayi flower color formation. Subsequently, based on correlation analysis between anthocyanins accumulation and Rd3GTs expressions during flower development, Rd3GT1 and Rd3GT6 were preliminarily identified as the pivotal 3GT genes involved in the formation of color of R. delavayi flower. Tissue-specific expressions of Rd3GT1 and Rd3GT6 were examined, and their function as 3GT in vivo was confirmed through introducing into Arabidopsis UGT78D2 mutant and Nicotiana tabacum plants. Furthermore, biochemical characterizations showed that both Rd3GT1 and Rd3GT6 could catalyze the addition of UDP-sugar to the 3-OH of anthocyanidin, and preferred UDP-Gal as their sugar donor and cyanidin as the most efficient substrate. This study not only provides insights into the biosynthesis of anthocyanin in R. delavayi, but also makes contribution to understand the mechanisms of its flower color formation.
Introduction
In addition to proteins, fats, and carbohydrates, plants also produce a great deal of additional molecules, which are known as secondary metabolites, including alkaloids, terpenoids, and phenolics (Chuan et al., 2018). Flavonoids, a big cluster of polyphenolic products, occur widely in plants with various biological functions such as pigments, feeding deterrents, antimicrobial agents, chemical messengers, UV protectants, auxins transporters as well as cell cycle inhibitors (Galeotti et al., 2008; Ferreyra et al., 2012). Based on their basic skeleton, flavonoids can be classified into flavonols, flavones, flavanols, flavanones, isoflavones, proanthocyanidins, and anthocyanins (Charles et al., 2010). Among them, anthocyanins are major pigments responsible for the formation of orange, pink, red, purple, and blue colors of many flowers, fruits, and vegetables (Alan et al., 2021; Giuseppe et al., 2021). In addition to this, anthocyanins have also been recognized for their biological benefits on human health. For example, dietary intake of anthocyanin-rich food can reduce the risk of dementia in humans (Commenges et al., 2000). An experimental study on Alzheimer's disease suggests that anthocyanin derivatives may play positive effects on improving cognitive function and neurological resilience (Wang et al., 2008).
The biosynthetic pathway leading to anthocyanin has been well-characterized in many plant species such as Arabidopsis thaliana, Zea mays, and Petunia × hybrid (Winkel-Shirley, 2001; Mandeep et al., 2011; Tohge et al., 2016). Most of the enzymes, including chalcone synthase (CHS), chalcone isomerase (CHI), flavone 3-hydroxylase (F3H), flavonoid 3′-hydroxylase (F3′H), flavonol synthase (FLS), dihydroflavonol 4-reductase (DFR), and anthocyanidin synthase (ANS) related to the biosynthesis of anthocyanin, have been isolated and functionally identified (Sara et al., 2020). But the enzymes involved in the terminal modification (glycosylation, methylation, and acylation) of anthocyanins are less understood, although they are commercially significant for metabolic engineering of flavonoid production (Forkmann and Martens, 2001). Moreover, these modifications can enrich the variety of end products that possess diverse bioactive effects. For instance, over 500 different anthocyanins have been reported in the literature despite the fact that only six chromophore forms (aglycones) existed in anthocyanins. Related studies have also demonstrated that a great part of this diversity is owing to the attachment of different kinds and quantities of sugar moieties at different positions of anthocyanidin, which was called glycosylation (Sonia et al., 2010).
Glycosylation is one of the most extensive modifications that fulfill multifarious functions during plant metabolism, such as an increase in the solubility and stability of acceptor, form glycogen for energy storage, synthesize oligosaccharides at cell surface, detoxify xenobiotics, as well as participate in hormonal homeostasis (Coutinho et al., 2003; Jae et al., 2008). Glycosylation of anthocyanidin is catalyzed by uridine diphosphate glycosyltransferases (UGTs), which transfer a carbohydrate usually from UDP-sugar to a wide range of low-molecular-weight acceptors (Joe et al., 2001). PSPG (Plant Secondary Product Glycosyltransferase) box, a conserved 44-residue motif at C-terminal end, is known as the typical of all UGTs. Several conserved residues in this UGT-defining sequence are found to interact with sugar donor (Gachon et al., 2005; Le et al., 2016). In plant UGTs, UDP-glucose is regarded as the most favored sugar donor, while other similar UDP-sugars, including UDP-galactose, UDP-rhamnose, UDP-xylose, and UDP-arabinose, are also encountered (Yun et al., 2020). The sugar donor preference of UGTs is promiscuous, for example, UGTs from Vitis Vinifera and Fragaria×ananassa can only use UDP-glucose, UCGalT1 from Daucus carota L. accepts UDP-galactose only, but 3GT from Scutellaria baicalensis transfers at least four kinds of sugars to flavonoids (Christopher et al., 1998; Markus et al., 2008; Zhi-Sheng et al., 2016; Kai et al., 2018; Zilong et al., 2019). At the same time, crystal structures and specific mutagenesis analysis have proved that the last residue in the PSPG box, Arg350, as well as amino acid residues forming hydrogen bonds all play a decisive role on the sugar donor specificity. No conserved amino acid residues have been identified as the general determinants of sugar donor specificity of UGTs (Modolo et al., 2007; Yun et al., 2020).
In addition, glycosyltransferases also have selective substrate specificities as well as regiospecificity. For instance, flavonoid 3-O-glycosyltransferases from Arabidopsis and petunia can traffic sugar donors only to 3-position of flavonols and anthocyanidins, respectively (Mami et al., 2002; Takayuki et al., 2005). Meanwhile, based on the regiospecificity for substrates, glycosyltransferases can be divided into flavonoid 3-O-glycosyltransferases, flavonoid 5-O-glycosyltransferases, flavonoid 7-O-glycosyltransferases, and so on (Zhao et al., 2012). Of these glycosyltransferases, flavonoid 3-O-glycosyltransferases are the ones that are best-studied, as flavonoid 3-O-glycosides are the most popular phenolic compounds in plants. The gene encoding flavonoid 3-O-glycosyltransferase was first isolated in maize and later characterized at the molecular level in many other plant species (Ralston et al., 1988; Katayama-Ikegami et al., 2020). But for the catalytic mechanism of 3GT, there are still many unsolved problems. Therefore, characterization and mechanistic study of 3GTs from more different plants would help to resolve this complex task.
Rhododendron delavayi (R. delavayi) is one of the most famous flowering shrubs. Because of colorful flowers and high horticultural values, it has been widely used in landscape greening (Zhang et al., 2017). Nevertheless, the key enzyme, flavonoid 3-O-glycosyltransferase critical for flower color formation, has not been cloned and characterized from R. delavayi (Yuan et al., 2021). In the current study, anthocyanins were first identified and quantified at five flower developmental stages, and the anthocyanin biosynthetic pathway in R. delavayi flowers was drawn based on these results (Figure 1). Meanwhile, quantitative results showed that anthocyanidin 3-O-glycoside was the most abundant during flower development, which accounted for 93.68–96.31% of the total anthocyanins, indicating the importance of Rd3GTs for R. delavayi flower color formation. Thus, we analyzed the transcriptome data of R. delavayi to search for Rd3GTs, and six genes with complete open reading frames (ORFs) were obtained. Then, according to the correlation analysis between the anthocyanin accumulation and expression profiles of Rd3GTs at different flower developmental stages, two Rd3GT genes (Rd3GT1 and Rd3GT6), which may play a vital role in flower anthocyanin accumulation, were selected and further functionally characterized. Temporal and spatial expressions of Rd3GT1 and Rd3GT6 were detected and their potential roles in planta were examined via introducing into Arabidopsis UGT78D2 mutant and Nicotiana tabacum plants. Furthermore, biochemical properties of Rd3GT1 and Rd3GT6 proteins were also confirmed. The results displayed that both flavonoid 3-O-glycosyltransferases performed the crucial roles on flower color formation as well as anthocyanin biosynthesis in R. delavayi. To our knowledge, this is the first report of the characterization of flavonoid 3-O-glycosyltransferase in R. delavayi and the results will not only provide new insights into the biosynthesis of anthocyanin in R. delavayi but also contribute to the further study of UTGs sugar donor preference and structure.
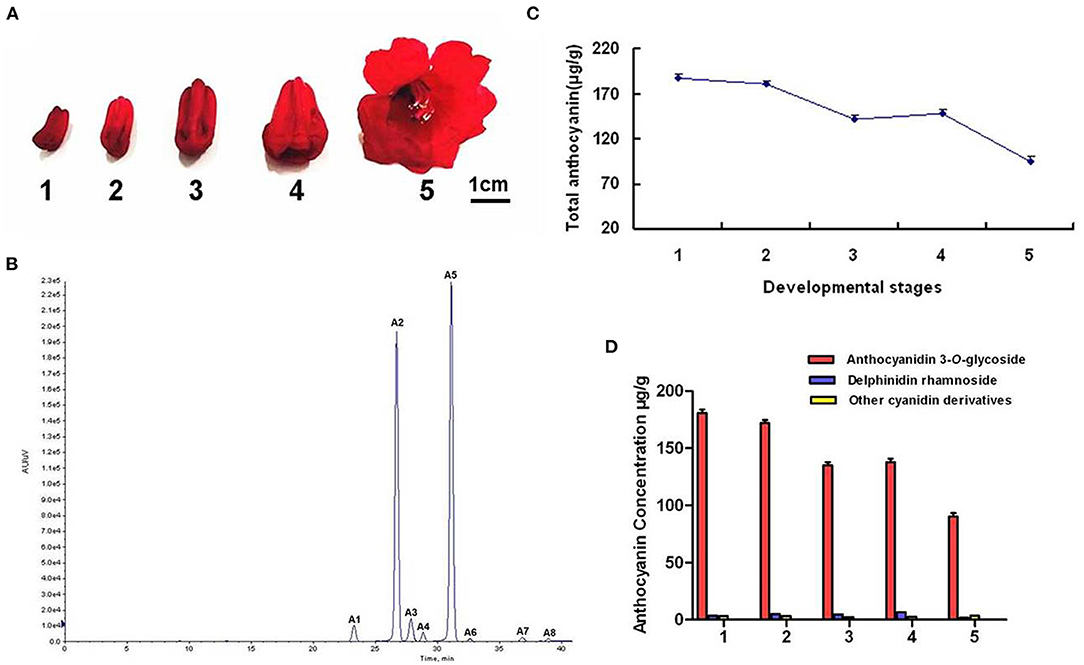
Figure 1. Anthocyanin component analyses in R. delavayi flower. (A) The phenotypes of different development stage. (B) Total anthocyanin profile at different developmental stages. (C) HPLC profiles of anthocyanin. A1, delphinidin 3-O-galacoside; A2, cyanidin 3-O-galacoside; A3, delphinidin rhamnoside; A4, cyanidin 3-O-glucoside; A5, cyanidin 3-O-arabinoside; A6/A7/A8, other cyanidin derivatives. (D) The contents of different anthocyanin at five developmental stages.
Materials and Methods
Plant Materials
R. delavayi plants were cultivated in the experimental field of National Forest Garden of GuiZhou Province, China. The roots, leaves, petals, pistils, stamens, toruses, scapes, and developing flowers (stages 1–5) were collected. Wild-type Arabidopsis (Arabidopsis thaliana) and T-DNA insertion mutant (UGT78D2) were purchased from the Nottingham Arabidopsis Stock Center (NASC) and maintained in a long day condition (16 h light/8 h dark photoperiod). For RT-PCR and anthocyanin analysis, Arabidopsis seedlings were harvested at 7 days after germination on 1/2 MS medium containing 3% sucrose (anthocyanin induction medium). Nicotiana tabacum plants used for transformation were grown in a glasshouse at 22°C with a 12 h light/12 h dark photoperiod, and full-blooming flowers of T1 transgenic Nicotiana tabacum were sampled for further analysis. All samples mentioned above were frozen immediately in liquid nitrogen, and kept at −80°C until further use.
Chemicals
UDP-glucose, UDP-galactose, UDP-rhamnose, UDP-arabinose, cyanidin, delphinidin, and malvidin were purchased from Sigma-Aldrich (USA), and pelargonidin, petunidin, peonidin, cyanidin 3-O-glucoside, delphinidin 3-O-glucoside, pelargonidin 3-O-glucoside, malvidin 3-O-glucoside, petunidin 3-O-glucoside, and peonidin 3-O-glucoside were obtained from Phytolab (Germany).
Detection of Anthocyanins
The extraction and detection of anthocyanins were conducted as previously described (Sun et al., 2016). Briefly, 0.3 g freeze-dried flowers of R. delavayi were pulverized in liquid nitrogen and extracted with H2O: MeOH: HCl (75/24/1v/v/v) overnight at 4°C in darkness. After centrifugation, the extracts were filtered (with 0.22 μm microporous membrane) and separated by an HPLC system (Shimadzu) equipped with an ACCHROM XUnion C18 column (250 mm × 4.6 mm, 5 μm). The absorbance of anthocyanin pigments was monitored at 520 nm, and the flow rate was 1 ml/min. Then, the mobile phase A (5% formic acid in H2O) and B (methanol) worked as follows: 0–10 min, 14–17% B; 10–35 min, 17–23% B; 35–60 min, 23–47% B; 60–67 min, 47–14% B; 67–70 min, 14% B. According to the procedures depicted by Sun et al. (2015) mass spectrometer was selected for qualitative analysis (Sun et al., 2015). The contents of anthocyanin in each sample were calculated via the external standard calibration of cyanidin 3-O-glucoside standards (Fanali et al., 2011). The calibration curves used were linear (R2 > 0.99) and the concentration ranges were 5–1,000 μg/ml. Mean values were obtained from three biological replicates per sample.
Expression Profiles of Rd3GTs Using Real-Time PCR
Total RNA for real-time PCR was extracted from flowers and other vegetative tissues of R. delavayi using RNA pure Plant Kit (CWBIO, China). Synthesis of first strand cDNA from 1 μg total RNA was performed using oligo (dT) and M-MLV reverse transcriptase (Takara, Japan). Then, real-time detection was conducted by using BioRad CFX96 Real-Time PCR System (BIO-RAD, Hercules, CA, USA) and TransStart® Green qPCR SuperMix (TRANSGEN, China) with primers designed based on sequence information from transcriptome analysis performed previously (Supplementary Table 1). Amplification of RdActin under the identical conditions was carried out to normalize the levels of Rd3GTs as follows: 60 s at 95°C, followed by 40 cycles of 5 s at 95°C and 60 s at 60°C. Each sample was carried out in three biological replicates, and the relative expression levels of target genes were calculated by formula 2−ΔΔCt. Meanwhile, the specific amplification of each gene was confirmed by melting curve analysis and agarose gel electrophoresis.
Cloning of Rd3GT Candidate Genes
According to the methods mentioned above, cDNAs synthesized from flower of R. delavayi were used as PCR templates. Specific primers obtained from the assembled transcriptomic information (Supplementary Table 1) were used to clone the full-length coding sequence of Rd3GT1 and Rd3GT6. After PCR amplification, the products were sub-cloned into the T/A cloning vector pMD18-T (Takara, Japan), and transformed into Escherichia coli JM109 competent cells. After positive screening, the correct recombinant clone was verified by sequencing.
Sequence Alignment and Phylogenetic Analysis
Alignment of amino acid sequences was carried out by using the DNAMAN 6.0 software. The multiple sequence alignment was performed through using Clustal Omega. Based on this alignment, a phylogenetic tree was drawn using MEGA5.1 with neighbor-joining method and 1,000 bootstrap replicates.
Plant Transformation
For ectopic expression of Rd3GTs in Arabidopsis and Nicotiana tabacum, their full-length CDS were amplified from the pMD18-T vector and inserted into the binary vector pBI121 digested with Xba I and BamH I, generating the pBI121-Rd3GTs overexpression constructs. Then, the resulting constructs were confirmed by sequencing and introduced into Agrobacterium tumefaciens strain GV3101 for Arabidopsis and Nicotiana tabacum transformation. Subsequently, inflorescences of Arabidopsis mutant (UGT78D2) were transformed via floral dip method, as described by Clough (Clough and Bent, 1998). The harvested seeds were selected on 1/2 MS medium supplemented with 50 mg/l kanamycin to set T1 seeds. After growing the seeds on anthocyanin induction media (1/2 MS medium containing 3% sucrose) for 7 days, the T2 transgenic seedlings were collected and used for molecular and anthocyanin analysis. At the same time, Nicotiana tabacum transformation was also performed according to the method previously depicted by Sparkes (Sparkes et al., 2006). Through resistant selection, T1 transgenic Nicotiana tabacum seedlings were obtained and cultured in green house, and after flowering, their full-blooming flowers were used for sample collection and later on analysis. For confirming the expressions of Rd3GTs, RT-PCR analysis was conducted both in Arabidopsis and Nicotiana tabacum. Furthermore, total anthocyanin concentration in T2 transgenic Arabidopsis seedlings and T1 transgenic Nicotiana tabacum flowers was determined using the method described above with three biological replicates.
Expression and Purification of Rd3GTs Proteins
The full-length CDS of Rd3GT1 and Rd3GT6 was introduced into the EcoR I/EcoR I and BamH I/Hind III site of pET32a (+) expression vector, respectively, and transformed into E. coli strain BL21 (DE3). On the second day, transformed E. coli expressing the recombinant Rd3GTs protein was cultured in liquid Luria-Bertani (LB) medium at 37°C till the value of OD600 of 0.6 was reached; after that, the cultures were induced several times for 36 and 48 h at 15°C through adding 0.2 mM of isopropyl-β-d-thiogalactopyranoside (IPTG). The cells were then collected by centrifugation (5,000 rpm, 10 min, 4°C) and suspended in phosphate-buffered saline without protein inhibitor (PBS, pH 7.4). After sonication in ice, the cell debris was removed and filtered with a 0.45 μm filter (Millipore). Next, the supernatant which contained soluble recombinant protein was purified via Ni-NTA pre-packed column (TransGen, China) and its purity was checked by sodium dodecyl sulfate polyacrylamide gel electrophoresis (SDS-PAGE). The concentration of recombinant Rd3GTs protein was measured by NanoDrop 1,000 Spectrophotometer (Thermo scientific, Waltham, MA, USA).
Enzyme Assays
In vitro enzymatic activity of Rd3GT1 and Rd3GT6 was tested by using cyanidin and UDP-Glu as substrates. The assay reaction was carried out at 30°C for 5 min in a final volume of 200 μl containing 20–30 μl purified Rd3GT1 and Rd3GT6 protein, 100 mM potassium phosphate buffer (pH 8.0), 10 mM UDP-glucose, and 100 μM cyanidin. After quenching by adding 5% HCl (50 μl), the reaction mixture was centrifuged (12,000 rpm, 5 min, 4°C) and analyzed by HPLC using the method described above in “Detection of Anthocyanins”; then, the corresponding glucosylation product was confirmed by comparing it with the standard. Meanwhile, the protein extracted from BL21 (DE3) cells that express empty pET-32a (+) vectors was always used as a negative control. For determination, the substrate specificity of recombinant Rd3GT1 and Rd3GT6, delphinidin, pelargonidin, petunidin, peonidin, and malvidin was selected as acceptors using UDP-glucose as a donor. In addition, 10 mM UDP-galactose, UDP-rhamnose, and UDP-arabinose were also used to examine the specificity of sugar donors.
Statistical Analysis
Correlation analysis was carried out via calculating pairwise Pearson correlations between each Rd3GT gene and anthocyanin. p < 0.05 was taken as statistically significant by Student's t-test.
Results
Anthocyanin Profiling in Developing Flower
To uncover the detailed biochemical basis of the red color of R. delavayi flower, its anthocyanin profiles were identified and quantified during flower development by using HPLC (Figure 1A). The results showed that a total of eight peaks (A1–A8) were identified in flowers (Figure 1B). These eight peaks were then confirmed as delphinidin 3-O-galacoside, cyanidin 3-O-galacoside, delphinidin rhamnoside, cyanidin 3-O-glucoside, cyanidin 3-O-arabinoside, and cyanidin derivatives according to the MS analysis (Table 1). But pelargonidin glycosides, one kind of the basic anthocyanin, were not detected in R. delavayi flower. To correlate anthocyanin accumulation with gene expression, anthocyanin contents in five developmental stages of R. delavayi flower were determined. During flower development, anthocyanin accumulations declined gradually from stage 1 to stage 3 and raised at stage 4 with the minimum levels at stage 5 (Figure 1C). Interestingly, among different anthocyanins, anthocyanidin 3-O-glycoside was used most of the times, which accounted for 93.68–96.31% of the total anthocyanins (Figure 1D).
Selection of Candidate Rd3GTs From Flowers of R. delavayi
On the basis of transcriptome data of different tissues of R. delavayi, a total of six potential 3GT genes were identified through blastn alignment with reference genes from proximal species and Arabidopsis. To confirm the key 3GT genes related to the biosynthesis of anthocyanins in R. delavayi flowers, we performed the expression analysis of these genes during flower development. As shown in Figure 2, transcript abundance of Rd3GT1 (R2 = 0.8785) decreased from stage 1 to stage 2, followed by a raised transcript level to stage 5, and thus exhibited a negative correlation to the accumulation patterns of anthocyanin in R. delavayi flowers. For Rd3GT6, its transcripts (R2 = 0.9526) declined from stage 1 to stage 3, and significantly upregulated at stage 4 with minimum transcript levels noted at stages 5, which perfectly correlated with the anthocyanin accumulation profiles in R. delavayi flowers. But for Rd3GT7 (R2 = 0.0297), Rd3GT9 (R2 = 0.0765), Rd3GT11 (R2 = 0.1002), and Rd3GT12 (R2 = 0.0003), there was a weak or nearly no correlation between their expressions and anthocyanin accumulations. Therefore, Rd3GT1 and Rd3GT6 were preliminarily identified as the key 3GT genes involved in the formation of R. delavayi flower color and selected for further functional characterization.
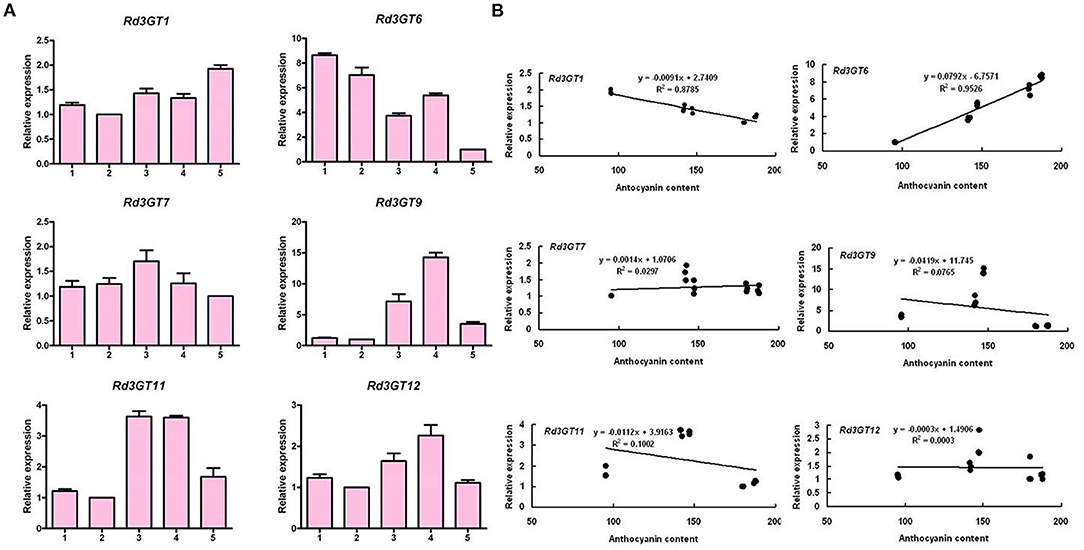
Figure 2. Expression profiles of Rd3GTs during flower development (A) and Pearson's correlation coefficient between Rd3GTs transcript levels and anthocyanin contents in R. delavayi flower (B). 1–5 represent the flowers of different developmental stages.
Sequence and Phylogenetic Analyses
Based on sequence information of transcriptome data, the coding region sequences of Rd3GT1 and Rd3GT6 were successfully cloned from flower of R. delavayi. The ORFs of Rd3GT1 and Rd3GT6 were 1,395 and 1,365 bp long encoding 464 and 454 amino acids residues, respectively. Multiple sequence alignment of Rd3GT1 and Rd3GT6 with UGT78G1 (flavonoid 3-O-glucosyltransferase from Medicago truncatula, A6XNC6) and UGT78D2 (flavonoid 3-O-glucosyltransferase from Arabidopsis thaliana, NM_121711.5) revealed that both Rd3GTs carried the conserved 44-residue C terminal PSPG signature motif, and the last residue within this motif was histidine (Figure 3A). Next, a neighbor-joining phylogenetic tree of plant flavonoid UGTs was constructed, and three major clusters, which exhibited activities specific toward 3-OH, 5-OH, and 7-OH glycosylation, were recognized. Rd3GT1 and Rd3GT6 were grouped with 3-OH cluster implying that these two glucosyltransferases might belong to flavonoid 3-O-glycosyltransferase and glycosylate at the 3-OH of flavonoid substrates (Figure 3B).
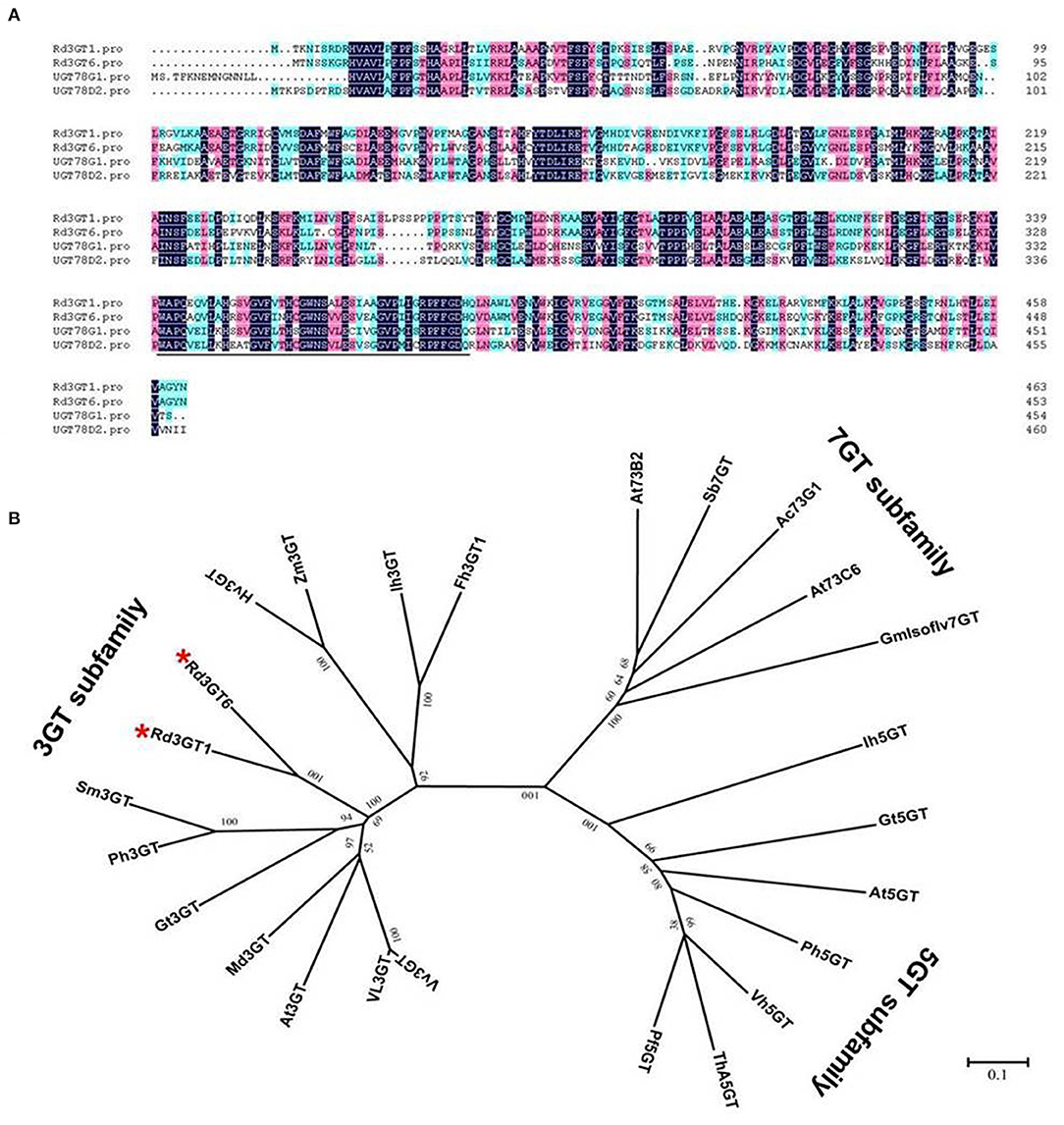
Figure 3. Sequence alignment and phylogenetic analyses of Rd3GT1 and Rd3GT6. (A) Sequence alignment of Rd3GT1 and Rd3GT6 with UGT78D2 (Arabidopsis thaliana, NM_121711.5) and UGT78G1 (Medicago sativa, A6XNC6). The PSPG motif that interacts with the sugar donor is underlined. (B) Phylogenetic analyses of the deduced amino acids of Rd3GT1 and Rd3GT6 and UFGTs from different plant species. GenBank accession numbers are as follows: VvFd3GT (Vitis vinifera AAB81683), Vl3GT (Vitis labrusca ABR24135), Mt3GT (Medicago truncatula XP_003610163), At3GT (Arabidopsis thaliana, NM_121711.5), Gt3GT (Gentiana triflora Q96493.1), Ph3GT (Petunia hybrida AB027454), Sm3GT (Solanum melongena Q43641), Zm3GT (Zea mays CAA31856), Hv3GT (Hordeum vulgare CAA33729), Ih3GT (Iris hollandica BAD83701), Fh3GT1 (Fressia hybrida ADK75021.1), Ih5GT (Iris hollandica BAD06874), Gt5GT (Gentiana triflora BAG32255), At5GT (Arabidopsis thaliana NP_193146), Pf5GT (Perilla frutescens BAA36421), Th5GT (Torenia hybrid BAC54093), Ph5GT (Petunia hybrida AB027455), Vh5GT (Verbena hybrida AB076698), Sb7GT (Scutellaria baicalensis BAA83484), At73B2 (Arabidopsis thaliana NM_179161.2), At73C6 (Arabidopsis thaliana NM_129234.2), Ac73G1 (Allium cepa AAP88406), GmIsoflv7GT (Glycine max ABB85236.1). Rd3GT1 and Rd3GT6 are highlighted in red stars.
Expression Analysis of Rd3GTs
Transcript levels of Rd3GT1 and Rd3GT6 were also determined in different R. delavayi organs by real-time qRT-PCR. As shown in Figure 4, Rd3GT1 and Rd3GT6 transcripts were detected in all organs tested, and their expressions were tissue specific. Accordingly, both Rd3GT1 and Rd3GT6 were most highly expressed in leaves where anthocyanins were barely detected (Supplementary Figure 4), and exhibited relatively lower expressions in pistil. Nevertheless, expression analysis during flower development displayed that the mRNA levels of Rd3GT1 and Rd3GT6 were dependent on flower development, and significantly correlated with the accumulation of anthocyanin. Taken together, this integrative expression analysis suggests that Rd3GT1 and Rd3GT6 may participate in not only the anthocyanin but also other flavonoid glycoside biosynthesis in R. delavayi.
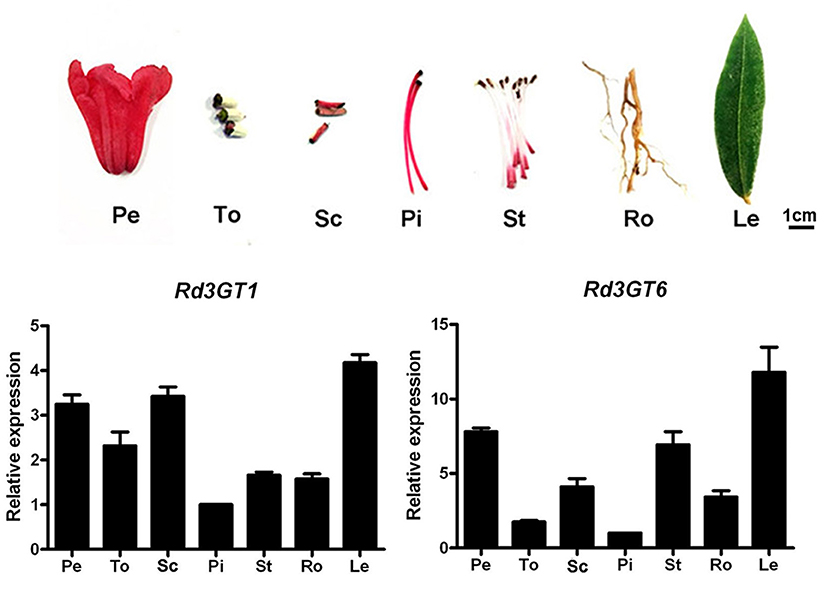
Figure 4. Expression profiles of Rd3GT1 and Rd3GT6 in different tissues of R. delavayi. Pe, petals; To, toruses; Sc, scapes; Pi, pistils; St, stamens; Ro, roots; Le, leaves.
Functional Identification of Rd3GTs in Planta
To investigate the function of Rd3GT1 and Rd3GT6 in vivo, these two genes were overexpressed in the Arabidopsis mutant (UGT78D2), which failed to generate anthocyanin pigments in their cotyledon and hypocotyls. After resistance selection, seeds of the wild-type, UGT78D2 mutant, and T2 transgenic plants were germinated and inductively cultured on 1/2 MS medium with 3% sucrose. As present in Figure 5A, the seedlings of UGT78D2 mutant exhibited purple coloration while overexpressing Rd3GT1 and Rd3GT6, although the mutant transformed with empty vector was still green (Supplementary Figure 5). Meanwhile, RT-PCR analysis was conducted for further confirming the expression of Rd3GTs in transgenic lines, and amplicons of expected size, which were absent in wild type and mutant were observed in transgenic plants (Figure 5C). In addition, extracted anthocyanin metabolites from the abovementioned seedlings were also analyzed by HPLC and HPLC-MS to determine the contents and kinds of individual anthocyanin (Supplementary Table 2). The results revealed that both Rd3GT1 and Rd3GT6 could restore the anthocyanin peaks (peak 1, 3, and 4) that lacked UGT78D2 mutant (Figure 5D), and showed higher anthocyanin contents than that in wild-type as well as UGT78D2 mutant (Figure 5B). Overall, these results confirmed that both Rd3GT1 and Rd3GT6 encode functional UF3GT protein that participated in the biosynthesis of anthocyanin in Arabidopsis.
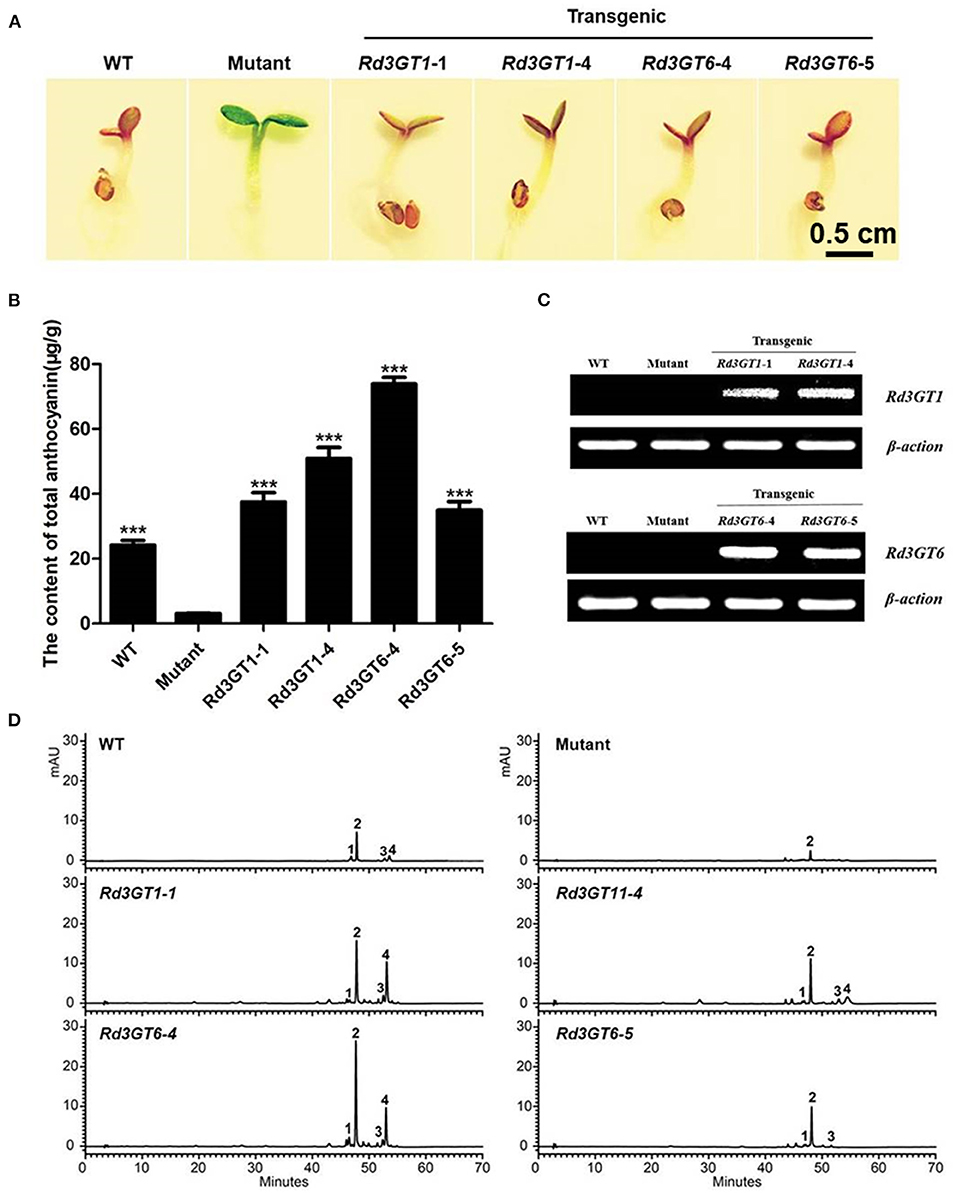
Figure 5. Complementation of the pigmentation of UGT78D2 mutant seedlings with Rd3GT1 and Rd3GT6. (A) Phenotypes of wild-type, mutant, and transgenic Arabidopsis seedlings. (B) Contents of anthocyanins in Arabidopsis seedlings. (C) Expressional analysis of Rd3GT1 and Rd3GT6 by reverse transcription polymerase chain reaction. (D) HPLC analyses of anthocyanins in Arabidopsis seedlings. Data correspond to means of three biological replicates. Asterisks indicate significant differences between means of mutant and wild-type as well as transgenic plants calculated by Student's t-test (***p < 0.001).
To further check the potential effect of Rd3GT1and Rd3GT6 on plant flowers, a total of 24 transgenic tobacco plants overexpressing Rd3GT1 and Rd3GT6 were generated. Then, two independent transgenic lines were analyzed for each gene, and RT-PCR analysis was used to confirm the overexpression of Rd3GT1 and Rd3GT6 (Figure 6C). As shown in Figure 6A, flower color of transgenic plants was darker than the control. Next, for examining this color change in detail, anthocyanin was extracted from the corollas of transgenic lines and quantified using HPLC (Figure 6D). The results showed that anthocyanin levels in flowers of transgenic tobacco were all higher than those in wild type (Figure 6B). Simultaneously, the kinds of anthocyanin in transgenic flower were unexpectedly increased from 2 to 5 (Figure 6D), and these anthocyanins were subsequently identified as cyanidin 3-O-rutinoside 5-O-glucoside, cyanidin 3-O-arabinoside, cyanidin 3-O-rutinoside, cyanidin 3-O-xyloside, and cyanidin 3-O-(6-O-malonyl-beta-D-glucoside), respectively (Table 2). Thus, combining all these results, it becomes clear that the enzymes encoded by Rd3GT1 and Rd3GT6 can effectively increase the contents and kinds of anthocyanin in transgenic tobacco flower.
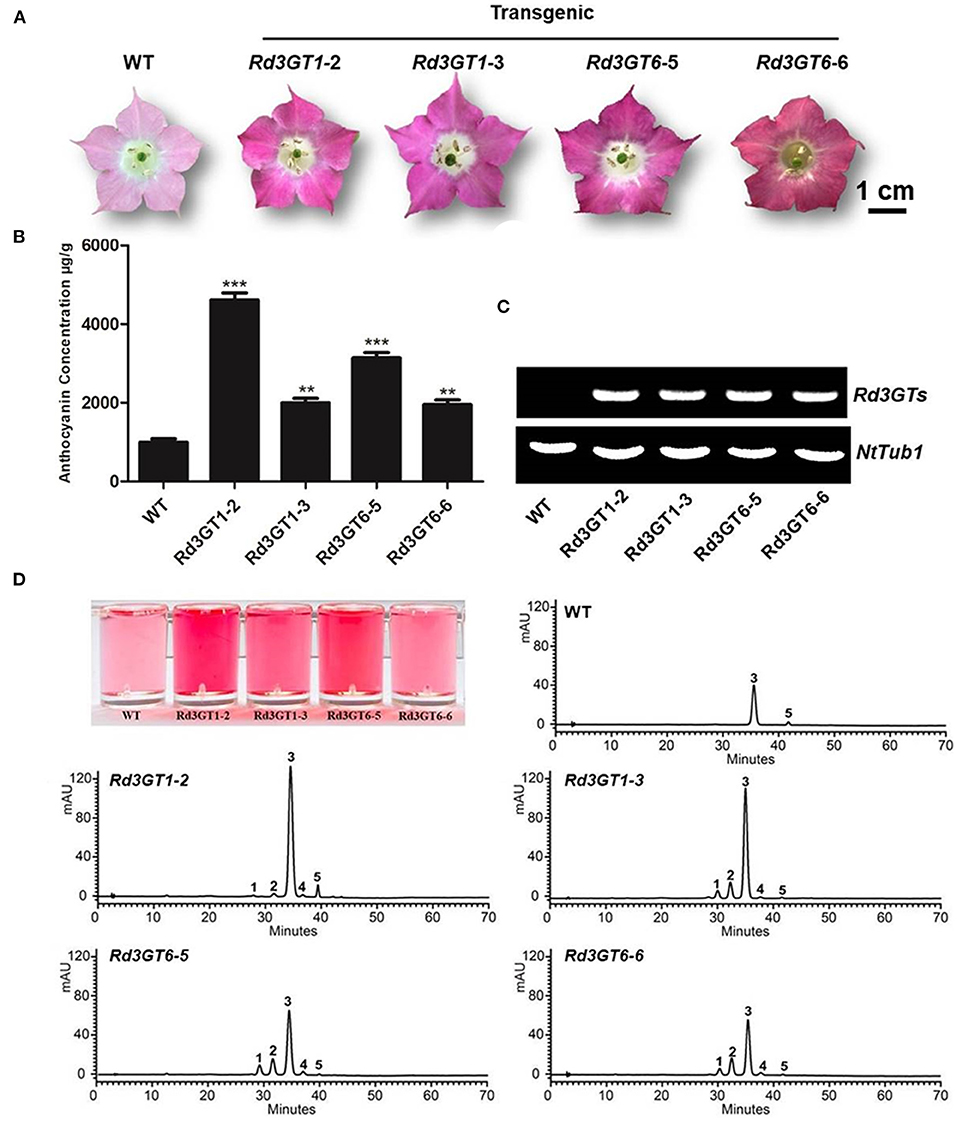
Figure 6. Effect of Rd3GT1 and Rd3GT6 on anthocyanin accumulation in transgenic tobacco flowers. (A) Tobacco flowers of wild-type and transgenic lines. (B) Contents of anthocyanin accumulation in transgenic tobacco flowers with HPLC. (C) Expression confirmation of Rd3GT1 and Rd3GT6 in flowers of transgenic tobacco. (D) HPLC analyses of anthocyanins in transgenic tobacco flowers. 1, Cyanidin 3-O-rutinoside 5-O-glucoside; 2, Cyanidin 3-O-arabinoside; 3, Cyanidin 3-O-rutinoside; 4, Cyanidin 3-O-xyloside; 5, Cyanidin 3-O-(6-O-malonyl-beta-D-glucoside). Results correspond to means from three biological replicates. Asterisks indicate significant differences between means of wild-type and transgenic plants calculated by Student's t-test (**p < 0.01); ***p < 0.001.
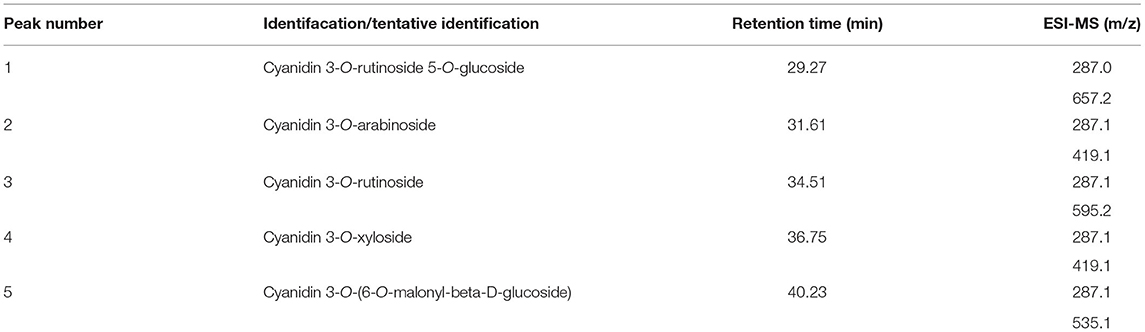
Table 2. HPLC-ESI-MS analysis of anthocyanin extracts of Rd3GTs over-expressing transgenic tobacco flowers.
Biochemical Characterization of Rd3GTs
The coding regions of Rd3GT1and Rd3GT6 were cloned into pET-32a (+) vector, and introduced into an E. coli BL21 (DE3) strain. The recombinant soluble proteins of Rd3GTs were purified by Ni-NTA pre-packed column and analyzed by SDS-PAGE (Supplementary Figure 1). The function of Rd3GT1 and Rd3GT6 was characterized using cyanidin as the substrate and UDP-glucose as the sugar donor. HPLC analysis identified that both Rd3GTs could catalyze the transfer of glucose to the 3-OH of cyanidin via comparing it with the reference standard. The recombinant protein extracted from E. coli BL21 expressing the empty vector could not glucosylate cyanidin (Figure 7A). Then, the reaction conditions for Rd3GTs were optimized, and both Rd3GTs exhibited their maximum activity at pH 8.0 and 30°C, which has been applied in the analysis of most plant UFGTs (Masayuki et al., 2000; Kim et al., 2006).
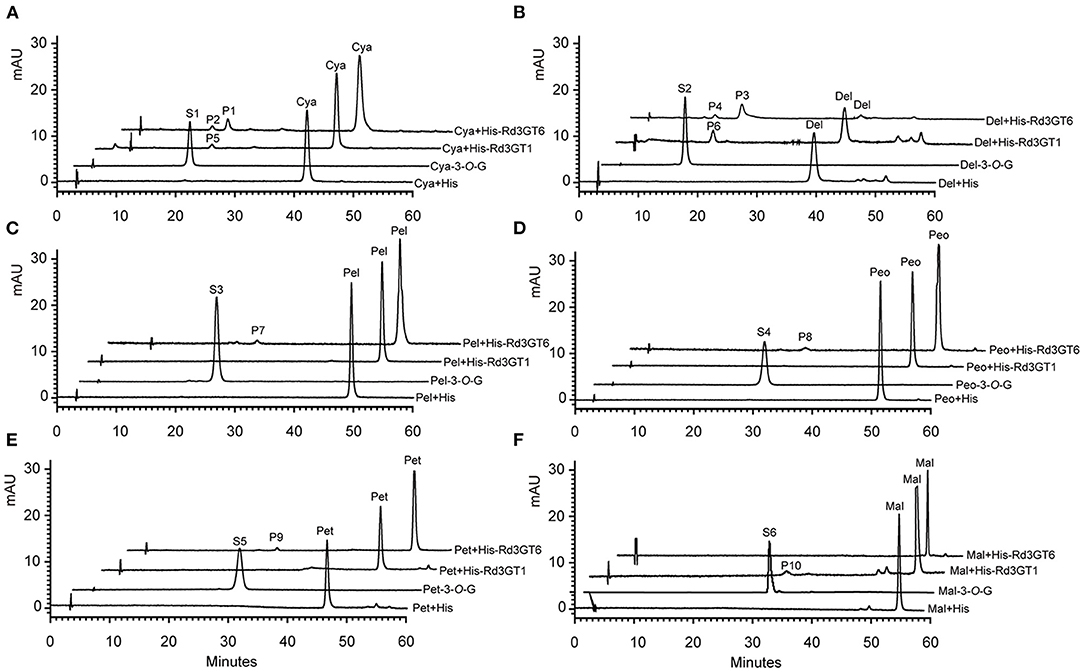
Figure 7. HPLC profiles of Rd3GT1 and Rd3GT6 reaction products with UDP-Glu and different anthocyanidin. (A) Cyanidin. P1/P5/S1, cyanidin 3-O-glucoside; P2, cyanidin 4'-O-glucoside, (B) Delphinidin. P3/P6/S2, delphinidin 3-O-glucoside; P4, delphinidin 4'-O-glucoside, (C) Pelargonidin. P7/S3, pelargonidin 3-O-glucoside, (D) Peonidin. P8/S4, peonidin 3-O-glucoside, (E) Petunidin. P9/S5, petunidin 3-O-glucoside, and (F) Malvinidin. P10/S6, malvinidin 3-O-glucoside. Cya-3-O-G, cyanidin 3-O-glucoside; Del-3-O-G, delphinidin 3-O-glucoside; Pel-3-O-G, pelargonidin 3-O-glucoside; Peo-3-O-G, peonidin 3-O-glucoside; Pet-3-O-G, petunidin 3-O-glucoside; Mal-3-O-G, malvinidin 3-O-glucoside.
To explore the substrate specificity of Rd3GTs, six anthocyanidin compounds were tested using UDP-glucose (UDP-Glu) as the sugar donor. Rd3GT1 showed the highest conversion rates for delphinidin, whereas pelargonidin, peonidin, and petunidin could not be accepted by it. However, Rd3GT6 was able to glucosylate five anthocyanidins except for malvinidin, and cyanidin exhibited the highest glucosylation rate, which was higher than that of Rd3GT1 toward delphinidin (Supplementary Table 3). Furthermore, HPLC analysis of Rd3GT6 reaction products showed that additional products (P2 and P4) were generated in the reaction of cyanidin and delphinidin (Figures 7A,B). The retention time of P1 and P3 was similar to the authentic cyanidin 3-O-glucoside and delphinidin 3-O-glucoside. Evidently, they were cyanidin 3-O-glucoside and delphinidin 3-O-glucoside, respectively. Then, HPLC-ESI-MS results demonstrated that the molecular weights of P1 and P2 were indistinguishable, indicating that only one UDP-glucose had been transferred to P2. It was reported that hypsochromic shift of UV spectra between Rd3GT6 products and substrates could be used to confirm the regiospecificity of Rd3GT6. Glycosylation at both 3-OH and 4'-OH would produce a hypsochromic shift, while at 7-OH, it does not display any effect (Thomas et al., 1997; Kramer et al., 2003). Therefore, the results suggested that P2 was likely to be cyanidin 4'-O-glucoside, and P4 was likely to be delphinidin 4'-O-glucoside based on hypsochromic shift (Supplementary Table 4).
To further explore the sugar donor specificity of Rd3GTs, we tested three other donors, that is, UDP-galactose (UDP-Gal), UDP-rhamnose (UDP-Rha), and UDP-arabinose (UDP-Ara). Surprisingly, Rd3GT6 exhibited high sugar donor promiscuity when catalyzing cyanidin, whereas Rd3GT1 did not, because it could not transfer UDP-Rha and UDP-Ara (Supplementary Tables 5, 6). In the presence of UDP-Gal, both Rd3GT1 and Rd3GT6 were able to efficiently glycosylate all the anthocyanidin substrates (Figure 8A). And with regard to UDP-Rha, Rd3GT6 could catalyze the glycosylation of cyanidin as well as delphinidin and showed relatively higher glycosylation rates toward delphinidin (Supplementary Table 6). For the catalytic reaction of UDP-Rha, Rd3GT6 produced four products (P1–P4), and all these products were identified as mono-O-glycosides through HPLC-ESI-MS analysis; thus, P1, P3 and P2, P4 were tentatively characterized as 3-O-rhamnoside and 4'-O-rhamnoside by the hypsochromic shift (Figure 8B; Supplementary Table 7). As shown in Supplementary Figure 3, only cyanidin could accept UDP-Ara at conversion rates of 5.96%.
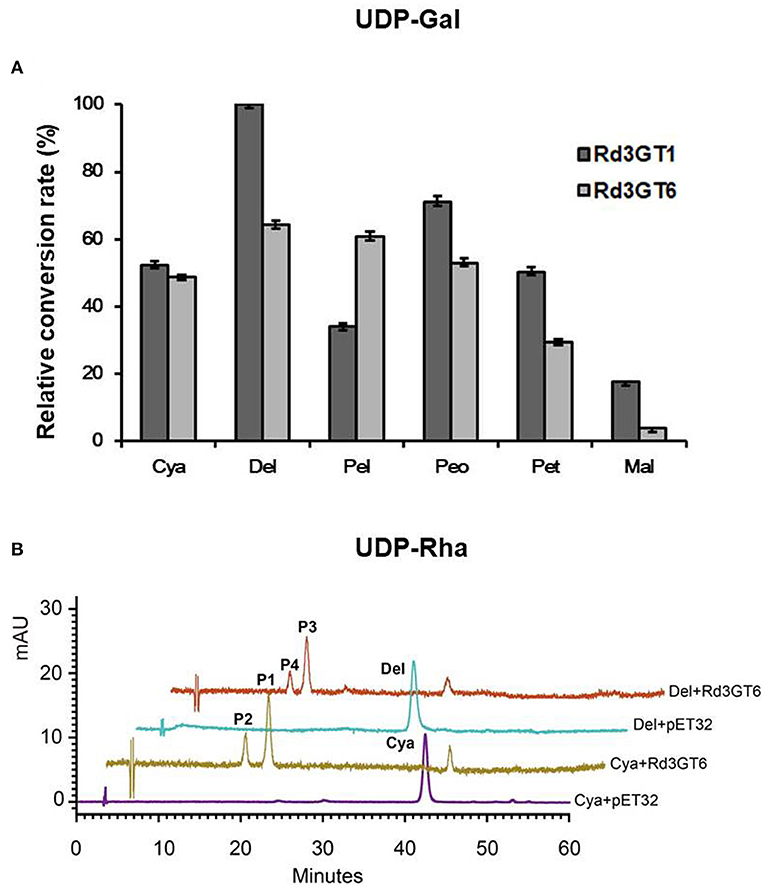
Figure 8. Relative activity of Rd3GT1 and Rd3GT6 toward several substrates and sugar donor. (A) Relative activity of Rd3GT1 and Rd3GT6 toward UDP- Gal and six anthocyanidins. The relative activity was calculated by Rd3GT1 activity toward delphinidin as 100%. Cya, cyanidin; Del, delphinidin; Pel, pelargonidin; Peo, peonidin; Pet, petunidin; Mal, malvinidin. (B) HPLC profiles of Rd3GT6 reaction products with UDP-Rha and cyanidin/delphinidin. P1, cyanidin 3-O-rhamnoside; P2, cyanidin 4'-O-rhamnoside; P3, delphinidin 3-O-rhamnoside; P4, delphinidin 4'-O-rhamnoside.
Discussion
Combining tissue-specific expressions with an analysis of metabolites has been specifically proven as an efficient method to identify the function of gene. In this study, we first conducted the analysis of anthocyanin in developing flowers of R. delavayi, and according to the anthocyanins detected in flowers, biosynthetic pathway of anthocyanin in R. delavayi was proposed (Figure 9). As summarized in Table 1, only cyanidin and delphinidin aglycons accumulated in R. delavayi, while pelargonidin derivatives were undetectable. This result was in accordance with the study of grape hyacinth and Cymbidium (Johnson et al., 2010; Hongli et al., 2019), where absence of Pg-type anthocyanin was due to the DFR inability to reduce dihydrokaempferol (DHK). In support of this, we recently demonstrated that DFR1 of R. delavayi also could not catalyze DHK to form pelargonidin (Wei et al., 2021). On the contrary, quantitative analysis of anthocyanins showed that the level of anthocyanin gradually decreased during flower development, and anthocyanidin 3-O-glycoside always accounted for more than 93% at any developmental stage (Figure 1D). Thus, the occurrence of the large proportions of anthocyanidin 3-O-glycosides implies that flavonoid 3-O-glycosyltransferase must be crucial for R. delavayi flower color formation.
After correlation analysis between anthocyanin accumulations and Rd3GTs expressions, Rd3GT1 and Rd3GT6 were preliminarily defined as the pivotal 3GT genes for anthocyanin formation in R. delavayi flowers (Figure 2B). Hence, their CDSs were successfully isolated from R. delavayi flowers, and phylogenetic analysis revealed that they were grouped into flavonoid 3-O-glycosyltransferase clade, which hinted the involvement of Rd3GT1 and Rd3GT6 in the 3-O-glycosylation of anthocyanidin in flowers of R. delavayi (Figure 3B). At the same time, multiple sequence alignment showed that these two Rd3GTs carried the typical PSPG sequence motif at the C terminal end, and their final amino acid residue within this motif was histidine (Figure 3A), which means that Rd3GT1 and Rd3GT6 are more likely to be galactosyltransferases (Akiko et al., 2004). Transcript analysis displayed that expressions of Rd3GT1 and Rd3GT6 were developmentally regulated. And among all Rd3GTs, Rd3GT6 exhibited the strongest correlation with the accumulation of anthocyanins (Figure 2B), indicating that Rd3GT6 might play a key role in anthocyanin biosynthesis during flower development. In addition, both Rd3GT1 and Rd3GT6 were also expressed in all the examined tissues, and not just the samples having anthocyanin (Figure 4). This expression profile is similar to ANL1 of Arabidopsis (Kubo et al., 2004) and suggests that Rd3GT1 and Rd3GT6 perhaps glycosylate not only anthocyanidin but also other flavonoids.
Transfer of Rd3GT1 and Rd3GT6 into Arabidopsis UGT78D2 mutant successfully restored the biosynthesis of anthocyanins in their cotyledons and hypocotyls (Figure 5A), which confirmed the functions of Rd3GT1 and Rd3GT6 as 3GT in planta. Similarly, 3GT genes from black soybean and Freesia overexpressed in UGT78D2 mutants also obtained the same results, hinting that 3GT proteins that take part in the biosynthesis of anthocynins are functionally exchangeable among diverse plants (Kovinich et al., 2010; Sun et al., 2016). Moreover, as shown in Figure 5D, pelargonidin derivatives (corresponding peak 3, Supplementary Table 2) which were absent in R. delavayi were detected in transgenic Arabidopsis; this observation suggests that both Rd3GT1 and Rd3GT6 could utilize pelargonidin as a substrate in Arabidopsis. For further investigating the potential role of Rd3GT1 and Rd3GT6 that participated in flower color development, they were introduced into tobacco plants. Comparing them to the wild type, transgenic tobacco plants expressing Rd3GT1 and Rd3GT6 generated deeper pink flowers (Figure 6A), and this alike phenomenon had also been observed in Litchi chinensis (Xiao et al., 2016). But surprisingly, introduction of Rd3GT1 and Rd3GT6 in tobacco synchronously led to the production of three novel anthocyanins in flowers, including cyanidin 3-O-rutinoside 5-O-glucoside, cyanidin 3-O-arabinoside, and cyanidin 3-O-xyloside (Table 2). Therefore, the abovementioned results apparently demonstrate that Rd3GT1 and Rd3GT6 are necessary for R. delavayi flower color development and can serve as useful molecular tools to improve the kinds of anthocyanin in plants.
Previously, it was reported that UGTs could recognize several different sugar donors, including UDP-Glu, UDP-Gal, UDP-Rha, UDP-Ara, UDP-Xyl, UDP-GlcUA, and so on. Of these donors, UDP-Glu is regarded as the most common sugar donor in plants (Sarah et al., 2008). Here, based on the kinds of anthocyanins detected in R. delavayi (Table 1), UDP-Glu, UDP-Gal, UDP-Rha, and UDP-Ara were selected to examine the UDP-sugar donor specificity of Rd3GT1 and Rd3GT6. As shown in Supplementary Tables 5, 6, both Rd3GT1 and Rd3GT6 could catalyze the addition of UDP-Glu and UDP-Gal to anthocyanidin, and the glycosylation rates toward UDP-Gal are much higher than that toward UDP-Glu. These results demonstrate that Rd3GT1 and Rd3GT6 prefer UDP-Gal as their sugar donors, which are congruent with the discovery that the last amino acid of their PSPG box is histidine (Akiko et al., 2004). But at the same time, the use of UDP-Glu as a sugar donor implies that sugar donor recognition is very complex, and both multiple amino acids and other structural features are responsible for the determination of sugar specificity (Yonekura-Sakakibara et al., 2007). In addition, Rd3GT6 also showed glycosylation activity to cyanidin and delphinidin when UDP-Rha and UDP-Ara act as sugar donors, but Rd3GT1 could not. This suggests that Rd3GT6 fulfills a much important role on determining the diversity of anthocyanins in R. delavayi flowers, which is consistent with its expression analysis. Meanwhile, it is interesting to note that when we use cyanidin and delphinidin as substrates, UDP-Glu and UDP-Rha act as sugar donors; apart from anthocyanidin 3-O-glycoside, anthocyanidin 4'-O-glycoside was also produced (Figures 7, 8). It has been reported that the presence of 3'-OH in acceptor molecules will influence the regiospecificity of glycosylation. If 3'-OH (e.g., cyanidin and delphinidin) is present, 4'-O-glycoside is preferentially generated. If it is missing (e.g., pelargonidin), then 7-O-glycoside is produced (Isayenkova et al., 2006). Perhaps, the production of 4'-O-glycoside for Rd3GT6 toward cyanidin and delphinidin is due to this fact.
To further biochemically characterize Rd3GT1 and Rd3GT6, different anthocyanidins were used as substrates to test their activity. As presented in Table 1, cyanidin glycoside and cyanidin derivatives were the predominant anthocyanins detected in R. delavayi flowers, suggesting that cyanidin might be the most efficient substrate for Rd3GT1 or Rd3GT6. Indeed, on the one hand, Rd3GT6 had a clear preference for cyanidin and could catalyze the transfer of all selected UDP-sugars to cyanidin (Supplementary Table 6). But, on the other hand, when UDP-Gal act as a sugar donor, the glycosylation efficiency of delphinidin was higher than that of cyanidin, which was conflicting to the relatively low levels of delphinidin glycoside in R. delavayi flowers (Supplementary Table 6). This similar phenomenon has also been observed from 3GT of Medicago truncatula, and revealed that UFGTs that take part in the biosynthesis of natural product are promiscuous, and also, the relative concentrations of substrates might be an important factor to determine their activity in planta (Modolo et al., 2007; Peel et al., 2009). Moreover, the substrate specificity analysis indicated that Rd3GT6 could catalyze the addition of UDP-Glu and UDP-Gal to the 3-OH of pelargonidin in vitro, while pelargonidin 3-O-glycoside was undetected in R. delavayi flowers. This implies that Rd3GT6 shows narrower substrate recognition in vivo, and biosynthesis of pelargonidin is stopped before Rd3GT6. Additionally, further research on UGTs should be conducted to explore how narrow vs. broad substrate recognition is regulated.
In summary, we first investigated the composition and contents of anthocyanins in R. delavayi flowers and proposed a pathway for its biosynthesis. Meanwhile, quantitative analysis of anthocyanins indicated that anthocyanidin 3-O-glycosides were the most prevalent at any flower development stage, suggesting that flavonoid 3-O-glycosyltransferase must play a vital role in flower color formation. Then, using a combined anthocyanin analysis and tissue-specific expressions approach, Rd3GT1 and Rd3GT6 were preliminarily confirmed as the key 3GT genes for R. delavayi anthocyanin formation. Indeed, subsequent biochemical characterizations together with in vivo data demonstrated their significance on the biosynthesis of anthocyanins in R. delavayi flower. Moreover, Rd3GT6 displayed great potential in sugar donor promiscuity and low regiospecificity, implying that it would be an attractive enzyme to engineer the diversity of anthocyanins for altering the color of plants and producing desired compounds. In conclusion, our findings make an important step forward in understanding the biosynthesis of anthocyanin in R. delavayi and will help to develop a useful method toward diversifying certain flavonoids in plants.
Data Availability Statement
The original contributions presented in the study are included in the article/Supplementary Materials, further inquiries can be directed to the corresponding author/s.
Author Contributions
ZJ and YY conceived and designed the study. WS, SS, HX, YC, and YW performed experiments, analyzed and interpreted the data, and wrote the manuscript. WS, XX, ZJ, and YY revised the manuscript critically. All authors read and approved the final manuscript.
Funding
This work was supported by the National Natural Science Foundation of China (Grant No. 31760076), the Joint Fund of the National Natural Science Foundation of China and the Karst Science Research Center of Guizhou province (U1812401), grant from department of education of Guizhou Province (KY [2021]059), and grants from Guizhou Science and Technology project ([2017]5726 and [2019]1019).
Conflict of Interest
The authors declare that the research was conducted in the absence of any commercial or financial relationships that could be construed as a potential conflict of interest.
Publisher's Note
All claims expressed in this article are solely those of the authors and do not necessarily represent those of their affiliated organizations, or those of the publisher, the editors and the reviewers. Any product that may be evaluated in this article, or claim that may be made by its manufacturer, is not guaranteed or endorsed by the publisher.
Supplementary Material
The Supplementary Material for this article can be found online at: https://www.frontiersin.org/articles/10.3389/fpls.2022.863482/full#supplementary-material
Abbreviations
HPLC, High-performance liquid chromatography; HPLC-MS, High-performance liquid chromatography-tandem mass spectrometry; IPTG, isopropyl-β-d-thiogalactopyranoside; SDS-PAGE, sodium dodecyl sulfate polyacrylamide gel electrophoresis; UDP-glucose, UDP-Glu; UDP-galactose, UDP-Gal; UDP-rhamnose, UDP-Rha; UDP-arabinose, UDP-Ara.
References
Akiko, K., Yuka, A., Shigeyuki, N., and Takafumi, Y. (2004). Alteration of sugar donor specificities of plant glycosyltransferases by a single point mutation. Arch. Biochem. Biophys. 429, 198–203. doi: 10.1016/j.abb.2004.06.021
Alan, H., Ingo, A., and Cathie, M. (2021). Natural blues: structure meets function in anthocyanins. Plants 10, 726. doi: 10.3390/plants10040726
Charles, S. B., Nijat, I., and Michael, A. D. (2010). flavonoids: new roles for old molecules. J. Integr. Plant Biol. 52, 98–111. doi: 10.1111/j.1744-7909.2010.00905.x
Christopher, M. F., Paul, K. B., and Høj, P. B. (1998). Cloning and characterization of Vitis vinifera UDP-glucose: flavonoid 3-O-glucosyltransferase, a homologue of the enzyme encoded by the maize bronze-1 locus that may primarily serve to glucosylate anthocyanidins in vivo. J. Biol. Chem. 273, 9224–9233. doi: 10.1074/jbc.273.15.9224
Chuan, K. S., Katja, H. K. M., Thomas, H., and Wilfried, S. (2018). Attractive but toxic: emerging roles of glycosidically bound volatiles and glycosyltransferases involved in their formation. Mol. Plant 11, 1225–1236. doi: 10.1016/j.molp.2018.09.001
Clough, S. J., and Bent, A. F. (1998). Floral dip: a simplified method for agrobacterium mediated transformation of Arabidopsis thaliana. Plant J. 16, 735–743. doi: 10.1046/j.1365-313x.1998.00343.x
Commenges, D., Scotet, V., Renaud, S., Jacqmin-Gadda, H., BarbergerGateau, P., and Dartigues, J.-F. (2000). Intake of flavonoids and risk of dementia. Eur. J. Epidemiol. 16, 357–363. doi: 10.1023/A:1007614613771
Coutinho, P. M., Deleury, E., Davies, G. J., and Henrissat, M. (2003). An evolving hierarchical family classification for glucosyltransferases. J. Mol. Biol. 328, 307–317. doi: 10.1016/S0022-2836(03)00307-3
Fanali, C., Dugo, L., D'Orazio, G., Lirangi, M., Dacha, M., and Dugo, P. (2011). Analysis of anthocyanins in commercial fruit juices by using nano-liquid chromatography-electrospray-mass spectrometry and high-performance liquid chromatography with UV-visdetector. J. Sep. Sci. 34, 150–159. doi: 10.1002/jssc.201000665
Ferreyra, M. L. F., Rius, S. P., and Casati, P. (2012). Flavonoids: biosynthesis, biological functions, and biotechnological applications. Front. Plant Sci. 3, 222. doi: 10.3389/fpls.2012.00222
Forkmann, G., and Martens, S. (2001). Metabolic engineering and application of flavonoid. Curr. Opin. Biotechnol. 12, 155–160. doi: 10.1016/S0958-1669(00)00192-0
Gachon, C. M., Langlois, M. M., and Saindrenan, P. (2005). Plant secondary metabolism glycosyltransferases: the emerging functional analysis. Trends Plant Sci. 10, 542–549. doi: 10.1016/j.tplants.2005.09.007
Galeotti, F., Barile, E., Curir, P., Dolci, M., and Lanzotti, V. (2008). Flavonoids from carnation (Dianthus caryophyllus) and their antifungal activity. Phytochem. Lett. 1, 44–48. doi: 10.1016/j.phytol.2007.10.001
Giuseppe, M., Carla, G., Andrea, E., Graziella, S., and Cinzia, M. B. (2021). Anthocyanins: biosynthesis, distribution, ecological role, and use of biostimulants to increase their content in plant foods. Agriculture 11, 212. doi: 10.3390/agriculture11030212
Hongli, L., Qian, L., Junren, M., Beibei, S., Zhuang, G., and Yali, L. (2019). Cloning and functional characterization of dihydroflavonol 4-reductase gene involved in anthocyanidin biosynthesis of grape hyacinth. Int. J. Mol. Sci. 20, 4743. doi: 10.3390/ijms20194743
Isayenkova, J., Wray, V., Nimtz, M., Strack, D., and Vogt, T. (2006). Cloning and functional characterisation of two regioselective flavonoid glucosyltransferases from Beta vulgaris. Phytochemistry 67, 1598–1612. doi: 10.1016/j.phytochem.2006.06.026
Jae, H. K., Bong, G. K., Jeong, H. K., Hojung, K., Chae, E. L., Jun, L., et al. (2008). Four glucosyltransferases from rice: cDNA cloning, expression, and characterization. J Plant Physiol. 165, 435–444. doi: 10.1016/j.jplph.2007.01.006
Joe, R., Yi, L., Eng-Kiat, L., and Dianna, J. B. (2001). Higher plant glycosyltransferases. Genome Biol. 2, 3004. doi: 10.1186/gb-2001-2-2-reviews3004
Johnson, E. T., Yi, H., Shin, B., Oh, B. J., Cheong, H., and Choi, G. (2010). Cymbidium hybrida dihydroflavonol 4-reductase does not efficiently reduce dihydrokaempferol to produce orange pelargonidin-type anthocyanins. Plant J. 19, 81–85. doi: 10.1046/j.1365-313X.1999.00502.x
Kai, F., Zhi-Sheng, X., Jie-Xia, L., Jing-Wen, L.i., Feng, W., and Ai-Sheng, X. (2018). Isolation, purifcation, and characterization of AgUCGalT1, a galactosyltransferase involved in anthocyanin galactosylation in purple celery (Apium graveolens L.). Planta 247, 1363–1375. doi: 10.1007/s00425-018-2870-5
Katayama-Ikegami, A., Byun, Z., Okada, S., Miyashita, M., and Kanzaki, S. (2020). Characterization of the recombinant udp:flavonoid 3-O-galactosyltransferase from mangifera indica 'irwin' (miufgalt3) involved in skin coloring. Horticult. J. 89, 1–9. doi: 10.2503/hortj.UTD-201
Kim, J. H., Kim, B. G., Park, Y., Ko, J. H., Lim, C. E., and Lim, J. (2006). Characterization of flavonoid 7-O-glucosyltransferase from Arabidopsis thaliana. Biosci. Biotechnol. Biochem. 70, 1471–1477. doi: 10.1271/bbb.60006
Kovinich, N., Saleem, A., Arnason, J. T., and Miki, B. (2010). Functional characterization of a UDP-glucose:flavonoid 3-O-glucosyltransferase from the seed coat of black soybean (Glycine max (L.) Merr.). Phytochemistry 71, 1253–1263. doi: 10.1016/j.phytochem.2010.05.009
Kramer, C. M., Prata, R. T. N., Willits, M. G., DeLuca, V., Steffens, J. C., and Graser, G. (2003). Cloning and regiospecificity studies of two flavonoid glucosyltransferases from Allium cepa. Phytochemistry 64, 1069–1076. doi: 10.1016/S0031-9422(03)00507-7
Kubo, A., Arai, Y., Nagashima, S., and Yoshikawa, T. (2004). Alteration of sugar donor specificities of plant glycosyltransferases by a single point mutation. Arch. Biochem. Biophys. 429, 198–203.
Le, R. J., Huss, B., Creach, A., Hawkins, S., and Neutelings, G. (2016). Glycosylation is a major regulator of phenylpropanoid availability and biological activity in plants. Front. Plant Sci. 7, 735. doi: 10.3389/fpls.2016.00735
Mami, Y., Emiko, Y., Gong, Z., Masako, F. M., Yuko, F., and Yoshikazu, T. (2002). Two flavonoid glucosyltransferases from Petunia hybrida: molecular cloning, biochemical properties and developmentally regulated expression. Plant Mol. Biol. 48, 401–411. doi: 10.1023/A:1014043214943
Mandeep, S., Moises, C., Kevin, R. A., Michael, M., Thomas, P. B., and Surinder, C (2011). Identification of the Pr1 gene product completes the anthocyanin biosynthesis pathway of maize. Genetics 188, 69–79. doi: 10.1534/genetics.110.126136
Markus, G., Florian, V., Barbara, F., Mari, L. B., Constanze, R., Juan, M.-B., et al. (2008). Multi-substrate flavonol O-glucosyltransferases from strawberry (Fragaria × ananassa) achene and receptacle. J. Exp. Bot. 59, 2611–2625. doi: 10.1093/jxb/ern117
Masayuki, K., Yutaka, H., Takaya, M., Tomoko, E. I., Ryoji, M., and Shin, H. (2000). Molecular cloning and characterization of a novel gene encoding limonoid UDP-glucosyltransferase in Citrus. FEBS Lett. 469, 173–178. doi: 10.1016/S0014-5793(00)01275-8
Modolo, L. V., Blount, J. W., Achnine, L., Naoumkina, M. A., Wang, X., and Dixon, R. A. (2007). A functional genomics approach to (iso)flavonoid glycosylation in the model legume Medicago truncatula. Plant Mol. Biol. 64, 499–518. doi: 10.1007/s11103-007-9167-6
Peel, G. J., Pang, Y., Modolo, L. V., and Dixon, R. A. (2009). The LAP1 MYB transcription factor orchestrates anthocyanidin biosynthesis and glycosylation in Medicago. Plant J. 59, 136–149. doi: 10.1111/j.1365-313X.2009.03885.x
Ralston, E. J., English, J. J., and Dooner, H. K. (1988). Sequence of three bronze alleles of maize and correlation with the genetic fine structure. Genetics 119, 185–197. doi: 10.1093/genetics/119.1.185
Sara, C., Pierdomenico, P., and Silvia, G. (2020). What's behind purple tomatoes? Insight into the mechanisms of anthocyanin synthesis in tomato fruits. Plant Physiol. 182, 1841–1853. doi: 10.1104/pp.19.01530
Sarah, A., Osmani, S. B., Anne, I., Carl, E, O., and Bandirger, L. M. (2008). Catalytic key amino acids and UDP-sugar donor specificity of a plant glucuronosyltransferase, UGT94B1: molecular modeling substantiated by site-specific mutagenesis and biochemical analyses. Plant Physiol. 148, 1295–1308. doi: 10.1104/pp.108.128256
Sonia, P.-T., Diego, A. M., and Cristina, G.-V. (2010). Flavanols and anthocyanins in cardiovascular health: a review of current evidence. Int. J. Mol. Sci. 11, 1679–1703. doi: 10.3390/ijms11041679
Sparkes, I. A., Runions, J., Kearns, A., and Hawes, C. (2006). Rapid, transient expression of fluorescent fusionproteins in tobacco plants and generation of stably transformed plants. Nat. Protoc. 4, 2019–2025. doi: 10.1038/nprot.2006.286
Sun, W., Liang, L., Meng, X., Li, Y., Gao, F., Liu, X., et al. (2016). Biochemical and molecular characterization of a flavonoid 3-O-glycosyltransferase responsible for anthocyanins and flavonols biosynthesis in Freesia hybrida. Front. Plant Sci. 7, 410. doi: 10.3389/fpls.2016.00410
Sun, W., Meng, X., Liang, L., Jiang, W., Huang, Y., and He, J. (2015). Molecular and biochemical analysis of chalcone synthase from Freesia hybrid in flavonoid biosynthetic pathway. PLoS ONE 10, e0119054. doi: 10.1371/journal.pone.0119054
Takayuki, T., Yasutaka, N., Masami, Y. H., Mitsuru, Y., Jun-ichiro, N., and Motoko, A. (2005). Functional genomics by integrated analysis of metabolome and transcriptome of Arabidopsis plants over-expressing an myb transcription factor. Plant J. 42, 218–235. doi: 10.1111/j.1365-313X.2005.02371.x
Thomas, V., Elke, Z., Rudi, G., Michael, M., and Dieter, S. (1997). Are the characteristics of betanidin glucosyltransferases from cell-suspension cultures of Dorotheanthus bellidiformis indicative of their phylogenetic relationship with flavonoid glucosyltransferases. Planta 203, 349–361. doi: 10.1007/s004250050201
Tohge, T., Wendenburg, R., Ishihara, H., Nakabayashi, R., Watanabe, M., Sulpice, R., et al. (2016). Characterization of a recently evolved flavonolphenylacyltransferase gene provides signatures of natural light selection in Brassicaceae. Nat. Commun. 7, 12399. doi: 10.1038/ncomms12399
Wang, J., Ho, L., Zhao, W., Ono, K., Rosensweig, C., Chen, L., et al. (2008). Grape-derived polyphenolics prevent Ab oligomerization and attenuate cognitive deterioration in a mouse model of Alzheimer's disease. J Neurosci. 28, 6388–6392. doi: 10.1523/JNEUROSCI.0364-08.2008
Wei, S., Nana, Z., Yuhan, W., Shiyu, S., Yan, Z., Zhigang, J., et al. (2021). Characterization and functional analysis of RdDFR1 regulation on flower color formation in Rhododendron delavayi. Plant Physiol Biochem. 169, 203–210. doi: 10.1016/j.plaphy.2021.11.016
Winkel-Shirley, B. (2001). Flavonoid biosynthesis. A colorful model for genetics, biochemistry, cell biology, and biotechnology. Plant Physiol. 126, 485–493. doi: 10.1104/pp.126.2.485
Xiao, J. L., Jie, Q. Z., Zi, C. W., Biao, L., Xu, M. H., Yong, H. Q., et al. (2016). Functional characterization of a glucosyltransferase gene, LcUFGT1, involved in the formation of cyanidin glucoside in the pericarp of Litchi chinensis. Physiol. Plant. 156, 139–149. doi: 10.1111/ppl.12391
Yonekura-Sakakibara, K., Tohge, T., Niida, R., and Saito, K. (2007). Identification of a flavonol 7-O-rhamnosyltransferase gene determining flavonoid pattern in Arabidopsis by transcriptome coexpression analysis and reverse genetics. J. Biol. Chem. 282, 14932–14941. doi: 10.1074/jbc.M611498200
Yuan, J. C., Xiong, R. L., Zhu, T. T., Ni, R., Fu, J., Lou, H. X., et al. (2021). Cloning and functional characterization of three flavonoid O-glucosyltransferase genes from the liverworts Marchantia emarginata and Marchantia paleacea. Plant Physiol. Biochem. 166, 495–504. doi: 10.1016/j.plaphy.2021.06.009
Yun, L., Shike, L., Song, G., and Jingwen, Z. (2020). Identification and characterization of three flavonoid 3-O-glycosyltransferases from Epimedium koreanum Nakai. Biochem. Eng. J. 163, 107759. doi: 10.1016/j.bej.2020.107759
Zhang, L., Xu, P. W., Cai, Y. F., Ma, L. L., Li, S. F., Xie, W. L., et al. (2017). The draft genome assembly of Rhododendron delavayi Franch var. delavayi. GigaScience 6, 1–11. doi: 10.1093/gigascience/gix076
Zhao, Z. C., Hu, G. B., Hu, F. C., Wang, H. C., Yang, Z. Y., and Lai, B. (2012). The UDP glucose: flavonoid-3-O-glucosyltransferase (UFGT) gene regulates anthocyanin biosynthesis in litchi (Litchi chinesis Sonn.) during fruit coloration. Mol. Biol. Rep. 39, 6409–6415. doi: 10.1007/s11033-011-1303-3
Zhi-Sheng, X., Jing, M., Feng, W., Hong-Yu, M., Qiu-Xia, W., and Ai-Sheng, X. (2016). Identification and characterization of DcUCGalT1, a galactosyltransferase responsible for anthocyanin galactosylation in purple carrot (Daucus carota L.) taproots. Sci. Rep. 6, 27356. doi: 10.1038/srep27356
Keywords: flower color, anthocyanin, flavonoid 3-O-glycosyltransferases, enzyme activity, Rhododendron delavayi
Citation: Sun W, Sun S, Xu H, Wang Y, Chen Y, Xu X, Yi Y and Ju Z (2022) Characterization of Two Key Flavonoid 3-O-Glycosyltransferases Involved in the Formation of Flower Color in Rhododendron Delavayi. Front. Plant Sci. 13:863482. doi: 10.3389/fpls.2022.863482
Received: 27 January 2022; Accepted: 11 April 2022;
Published: 16 May 2022.
Edited by:
Pan Liao, Purdue University, United StatesReviewed by:
Sarma Rajeev Kumar, String Bio Pvt Ltd, IndiaDinakaran Elango, Iowa State University, United States
Fuai Sun, University of California, Davis, United States
Copyright © 2022 Sun, Sun, Xu, Wang, Chen, Xu, Yi and Ju. This is an open-access article distributed under the terms of the Creative Commons Attribution License (CC BY). The use, distribution or reproduction in other forums is permitted, provided the original author(s) and the copyright owner(s) are credited and that the original publication in this journal is cited, in accordance with accepted academic practice. No use, distribution or reproduction is permitted which does not comply with these terms.
*Correspondence: Zhigang Ju, anV6aGlnYW5nekAxNjMuY29t; Yin Yi, eWl5aW5AZ3pudS5lZHUuY24=; Xiaorong Xu, NTEyNzQyOTExQHFxLmNvbQ==
†These authors have contributed equally to this work and share first authorship