- College of Horticulture, Gansu Agricultural University, Lanzhou, China
Cruciferous vegetable crops are grown widely around the world, which supply a multitude of health-related micronutrients, phytochemicals, and antioxidant compounds. Glucosinolates (GSLs) are specialized metabolites found widely in cruciferous vegetables, which are not only related to flavor formation but also have anti-cancer, disease-resistance, and insect-resistance properties. The content and components of GSLs in the Cruciferae are not only related to genotypes and environmental factors but also are influenced by hormones, plant growth regulators, and mineral elements. This review discusses the effects of different exogenous substances on the GSL content and composition, and analyzes the molecular mechanism by which these substances regulate the biosynthesis of GSLs. Based on the current research status, future research directions are also proposed.
Introduction
As one of the most important leafy vegetables, cruciferous plants are cultivated and consumed throughout the world. Most cruciferous vegetables can be eaten fresh or cooked; meanwhile, the leaves can also be used as animal feed, and the seeds can be used to produce edible oil. Cruciferae supply a multitude of health-related micronutrients and phytochemicals (Nilsson et al., 2006; Hanschen and Rohn, 2021). With the pursuit of healthy lifestyles, the consumption of the Cruciferae is increasing, and the planting area is expanding, according to the statistics of the Food and Agriculture Organization of the United Nations (FAOSTA, 2019). Studies have shown that the Cruciferae are enriched for antioxidant compounds, especially glucosinolate.
Glucosinolate (GSL) is a type of anion hydrophilic specialized metabolite containing nitrogen and sulfur; over 15 types of GSLs were detected in the Cruciferae, and the GSL contents are relatively high in the Cruciferae compared with other species (Hwang et al., 2019). The GSL biosynthesis pathway is complex and mainly consists of three stages: side chain extension, core structure formation, and secondary modification. The side chain extension includes deamination, condensation, isomerization, and oxidative decarboxylation, involving BCAT, MAM, and other gene families. Biosynthesis of core structures consists of five biochemical steps, including oxidation, conjugated oxidation, C-S cleavage, glycosylation, and sulfidation, mainly involving CYP79 and CYP83 gene families. Side chain modifications lead to different types of GSLs; FMOGS-OX and AOP gene families are involved in side chain modification of aliphatic GSL; CYP81F and IGMT gene families are involved in indole GSL, respectively (Harus et al., 2020). Studies have shown that R2R3-MYB transcription factors play important roles in regulating the biosynthesis of aliphatic and indole GSL (Sonderby et al., 2010a; Kumari et al., 2019). MYB28, MYB29, MYB34, MYB51, MYB76, and MYB122 participate in the regulation of GSL biosynthesis, among which MYB28, MYB29, and MYB76 regulate the biosynthesis of aliphatic GSL (Gigolashvili et al., 2008; Cai et al., 2018). MYB34, MYB51, and MYB122 regulate the biosynthesis of indole GSL (Frerigmann et al., 2014; Mitreiter and Gigolashvili, 2021). Naturally occurring GSL have a common chemical structure: the structures are generally composed of β-D-glucosaminyl, a sulfide oxime group, and side-chain R groups derived from amino acids; furthermore, GSL exist generally in the form of potassium or sodium salts (Fahey et al., 2001). Based on the amino-acid side chain R groups, GSLs can be divided into three categories, including aliphatic GSL (side chains are mainly derived from methionine, alanine, valine, leucine, or isopropyl leucine), indole GSL (side chain derived from tryptophan), and aromatic GSL (side chains derived from phenylalanine or tyrosine) (Sonderby et al., 2010b; Bekaert et al., 2012).
The GSL are generally stable in the cytoplasm of cells, while the myrosinase that hydrolyzes these compounds is located in vacuoles (Bhat and Vyas, 2019). When plant tissue is damaged, GSL produce a large number of different hydrolysates under the catalysis action of myrosinase. The initial enzymolysis products are unstable glycoside ligands and D-glucose, and the glycoside ligands are hydrolyzed or rearranged to produce different products. Under acidic conditions, the products are mainly nitriles, while these products mainly form thiocyanates through loosened rearrangement under neutral conditions. In the presence of the EPS (extracellular polymer) enzyme, Fe2+ and with unsaturated bonds at the end of the R group, the degradation products were mainly cyclic thionitrile. When R is indole or benzene and its derivatives, thiocyanates will be rearranged, and the degradation products spontaneously cyclize to form azolidinone when R contains the hydroxyl group. The varieties of the GSLs hydrolysis products lead to a wide range of biological activity. GSL are not only related to the flavor of cruciferous vegetables (Anthony et al., 2020; Connolly et al., 2021; Shin et al., 2021) but also play important roles in protecting plants from insect and microbial pathogens (Kunierczyk et al., 2008; Robert et al., 2019; Yao et al., 2019). Some GSL, such as glucoraphanin, play a significant role in the prevention of certain chronic diseases, including hypertension, diabetes, neurodegenerative diseases (Parkinson’s and Alzheimer’s diseases), some types of cancers, and certain cardiovascular diseases (Folmer et al., 2014; Lee et al., 2019). However, the degradation of 2-hydroxyl-3-butenyl GSL adversely affects animal growth, reproduction, and also causes goiter and abnormalities in internal organs of animals (Angelino and Jeffery, 2021). Because the GSL have great influence on plants, animals, and humans, the GSL biosynthesis and functional studies are one of the hot topics in international research at present. Medical specialists focus on the therapeutic effects of GSL, while the breeders are more concerned with reducing harmful GSL and increasing GSL content, which are beneficial to humans and have pest-control effects. Therefore, studying the change mechanism of GSL content and components induced by different factors is of great significance to increase the quality of cruciferous vegetables, enhance the resistance to disease and insect pests, and extract beneficial GSL components.
Studies have shown that the main factor affecting the content and composition of GSL is genotype, and the content and composition of GSL were different among different species and varieties (SzydłOwska-Czerniak et al., 2011; Wiesner et al., 2013b; Rhee et al., 2020; Jeon et al., 2022). Temperature, light quality, mechanical injury, and insect feeding can affect GSL biosynthesis and component content (Pedreno et al., 2017; Kim D. et al., 2018; Lin et al., 2022). Moreover, biosynthesis of GSL is also influenced by plant hormones, plant growth regulators, chemical fertilizers, pesticides, metal ions, gas, etc. (Barickman et al., 2013; Bakhtiari et al., 2018; Li et al., 2018; Mao et al., 2018; Teng et al., 2021). Although there are many reports about the effects of these chemicals on GSL biosynthesis, there is no review of the effects of these substances. This review explores and summarizes the effects of plant hormones, plant growth regulators, mineral elements, heavy metals, antibiotics, and other chemicals substances on GSLs biosynthesis. The present review could provide a theoretical basis for the application of GSLs in regulating the content of certain GSLs and high-quality production of cruciferous vegetables.
Plant Growth-Regulating Substances
Jasmonic Acid and Methyl Jasmonate
As an endogenous growth regulator in higher plants, jasmonic acid (JA) can inhibit plant germination, growth, and improve plant resistance (Pangesti et al., 2016; Zhou and Johan, 2017; Mao et al., 2018). In the regulation of GSL biosynthesis, Traw et al. (2003) found that JA increased the total GSLs in Arabidopsis thaliana (Traw et al., 2003). After JA treatment, 1-methoxy-indol-3-ylmethyl in the culture medium cultivated Pak Choi (Brassica rapa L.) hairy roots and the glucobrassicin, indole-3-carbinol, 1-methoxy-indole-3-carbinol, and total GSLs contents in the broccoli (Brassica oleracea L. var. italica) suspension-cultured cells increased (Kastell et al., 2013; Wiesner et al., 2013a). Besides, the JA increased sinigrin, gluconapin, progoitrin, glucoiberin, and total GSLs concentrations consistently in cabbage (B. oleracea L. var. capitata L.) (Bruinsma et al., 2007; Fritz et al., 2010). Accumulation of 4-hydroxyglucobrassicin, 4-methoxyglucobrassicin, glucobrassicin, neoglucobrassicin, and total aliphatic GSLs increased significantly after application of JA in turnip (B. rapa L. subsp. rapa) and broccoli (B. oleracea L. var. italica) sprouts (Thiruvengadam et al., 2016b; Guo et al., 2017; Zhu et al., 2019). Except for cabbage (B. oleracea L. var. capitata L.) and turnip (B. rapa L. subsp. rapa), the total GSLs, glucoraphanin, glucoraphenin, glucobrassicin, glucotropeolin, gluconasturtiin, and glucobrassicanapin contents of rapeseed (B. napus L.), red radish (Raphanus sativus L.), and Cardamine hirsuta also increased after treatment with JA (Bae et al., 2014; Thiruvengadam and Chung, 2015; Thiruvengadam et al., 2016a; Bakhtiari et al., 2018). Transcriptome profiling and gene expression analysis indicated that the expression level of MYB34, MYB51, MYB122, and the gene expression of the indole core biosynthesis genes, including CYP79B2, CYP83B1, CYP79F1, UGT74B1, and SOT16 increased in broccoli (B. oleracea L. var. italica) (Ku et al., 2016; Thiruvengadam et al., 2016b). JA also induced aliphatic GSL biosynthesis in rapeseed (Brassica napus L.), the levels of AP2/ERF, bHLH, WRKY, and MYB, which regulate the biosynthesis of total GSLs and aliphatic GSLs were also upregulated (Zhou and Memelink, 2016; Li et al., 2018). Proteins related to GSL biosynthesis and degradation were mediated by JA, leading to the accumulation of GSLs and sulforaphane in broccoli (B. oleracea L. var. italica) sprouts and the biosynthesis of indole GSLs in A. thaliana (Guo et al., 2017; Castillo et al., 2019).
Methyl jasmonate (MeJA), a kind of cyclopentanone derivative signal substance that exists widely in plants, can regulate plant growth, development, secondary metabolism, and disease resistance (Vahid and Soheil, 2019; Kurowska et al., 2020). MeJA application leads two and fourfold increases of 2-phenylethyl GSL and indole GSLs contents in turnip (B. rapa L. subsp. rapa) roots and leaves. Meanwhile, the 4-methoxy-3-indolylmethyl, 1-methoxy-3-indolylmethyl, 3-indolylmethyl, 2-phenylethyl, and indole GSLs contents in the root increased (Schreiner et al., 2011). Same with Schreiner et al. (2011) and Zang et al. (2015a) also concluded that roots accumulated much more GSLs and were more sensitive and rapidly responsive than leaves; indole GSLs (glucobrassicin, 4-methoxy glucobrassicin, and neoglucobrassicin) were the major component of total GSLs that accumulated rapidly in both roots and leaves (Zang et al., 2015a). Meanwhile, preharvest MeJA treatment increased 4- and 12-fold of GSL concentration in pak choi (B. rapa L.) in the soil and hydroponics-growing conditions, respectively (Baek et al., 2021). MeJA also significantly increased the concentrations of glucobrassicin, neoglucobrassicin, and indole GSLs in Chinese kale (Brassica alboglabra), and leaf-spraying MeJA induced greater accumulation of indole GSL (Sun et al., 2012b). Similar with turnip (B. rapa L. subsp. rapa) and kale (B. oleracea var. acephala DC), the glucoraphanin, glucoraphanin, and glucobrassicin contents in MeJA-treated China rose radish (R. sativus L. cv. China rose), red radish (R. sativus L. cv. Rambo), and A. thaliana was also enhanced (Bae et al., 2014; Chen et al., 2017). The contents of glucoraphenin, 4-hydroxyglucobrassicin, and glucobrassicin in radish (R. sativus L.) seedlings increased with increasing concentrations of MeJA (Al-Dhabi et al., 2015). After MeJA treatment, the glucobrassicin, 4-methoxyglucobrassicin, neoglucobrassicin, gluconasturtiin, and total GSLs concentrations in broccoli (B. oleracea L. var. italica) floret increased (Kang and Juvik, 2011; Ku and Juvik, 2012; Kang et al., 2013; Hassini et al., 2017a,b; Chiu et al., 2019, 2020). The accumulation of glucobrassicin, neoglucobrassicin, and gluconasturtiin in broccoli (B. oleracea L. var. italica) flower bulb (Liu et al., 2014; Ku et al., 2016), indole GSL content in broccoli (B. oleracea L. var. italica) suspension-cultured cells also enhanced (Sánchez-Pujante et al., 2020). Moreover, foliar application of MeJA + NaCl provoked higher GSL content than NaCl application alone (Rios et al., 2021). These results indicated that MeJA has different effects on aboveground leaves and roots, and the MeJA and NaCl may synergistically participate in the regulation of GSL biosynthesis.
The JA and MeJA are the most commonly used elicitors for induction of GSL. JA plays an important role in sensing and transmitting signals (Kim et al., 2021). JA regulation of GSL depends on the jasmonoyl-L-isoleucine-mediated COI1/JAZ/MYC2 pathways. When endogenous JA is below the threshold concentration, JAZ binds to MYC and prevents its transcription, thus inhibiting the expression of JA-responsive genes (Major et al., 2017). Under stress, JA content increases and isomerizes into JA-ILE, which promotes the interaction between COI1 and JAZ, leading to degradation of JAZ protease and release of MYC, and promotes the expression of JA-responsive genes. After MYC release, regulation of R2R3-MYB transcription factor expression ultimately activates the GSL synthesis gene (Wasternack and Strnad, 2019; Kim et al., 2021). JA can be converted to the more volatile MeJA; MeJA also can be converted to JA by esterases; both JA and MeJA have been reported to function as transported signals that induce systemic wound responses (Stratmann, 2003; Sun and Zhang, 2021). MeJA can act as an elicitor to enhance GSL biosynthesis in leaves and roots by upregulating the MYB, CYP79, CYP83, AOP2, FMOGS-OX5, and other GSL biosynthesis-related genes (Kang et al., 2013; Chen et al., 2015; Yi et al., 2016; Yu-Chun et al., 2018). Thus, long-distance transport of GSLs between the roots and leaves through phloem and xylem vascular tissues could be possible depending on the type of GSLs (Zang et al., 2015b).
Salicylic Acid
Salicylic acid (SA) is a small phenolic substance that exists widely in higher plants. It is a common endogenous signaling molecule in plants and plays important roles in physiological processes, such as disease, drought, cold, and salt resistance (Henschel et al., 2020; Xiao et al., 2020; Zhang et al., 2020). By examining the effects of SA and SA + JA in A. thaliana, Traw et al. (2003) and Barickman et al. (2013) found that SA could enhance the biosynthesis of GSLs but attenuated the induction of GSLs by JA, suggesting cross-effects between SA and JA (Traw et al., 2003; Barickman et al., 2013). SA also led to the enhancement of indole GSLs (glucobrassicin, 4-methoxy glucobrassicin, and neoglucobrassicin), aliphatic GSLs, and total GSLs in Chinese kale (B. alboglabra) roots and leaves. Compared with irrigation, leaf spraying produced higher amounts of indole GSLs (Sun et al., 2012b; Guo et al., 2013a; Zang et al., 2015a). Similar with Chinese kale, SA also led to the accumulation of the aromatic 2-phenylethyl, and different GSL contents in cabbage (B. oleracea L. var. capitata L.) and turnip (B. rapa L. subsp. rapa) (Schreiner et al., 2011; Thiruvengadam et al., 2016a; Yi et al., 2016).
Under abiotic stress, SA has a complex effect on the regulation of GSL and interacts with JA in the regulation of GSL biosynthesis (Guo et al., 2013a). ROS formation has a dual function, toxic to cells at high levels, and activate local and systemic defense responses to stress at low concentrations (You and Chan, 2015; Liu et al., 2021). ROS produces and amplified signals and then transmitted to SA, which regulates the expression of R2R3-MYB transcription factors through the MAPK cascade pathway (Liu et al., 2021). By enhancing the expression levels of MYBs, CYP79F1 and CYP83B1, the GSL contents increased (Schreiner et al., 2011; Thiruvengadam et al., 2016a; Yi et al., 2016).
Brassinosteroids and Ethylene
As a newly discovered plant endogenous hormone, brassinosteroids (BR) is internationally recognized as the most effective, broad-spectrum, non-toxic plant growth hormone, which cannot only regulate the photosynthesis, respiration, transpiration of plants but also improves stress resistance (Guo J. et al., 2018; Maghsoudi et al., 2019). Two studies on BR regulation of GSLs both focused on alleviating NaCl stress. Treated with different concentrations of BR, Guo et al. (2014) found that the GSL content in broccoli (B. oleracea L. var. italica) sprouts varied with the concentrations of BR. The contents of total GSLs and glucoraphanin increased by 86 and 85% after treatment with 2 nM BR under 40-mM NaCl stress (Guo et al., 2014). Similar to broccoli (B. oleracea L. var. italica), the 100-nM BR enhanced 1.23 ∼ 5.30-fold of glucoiberin, glucoraphanin, glucoerucin, gluconapin, progoitrin, sinigrin, total aliphatic GSLs, and total GSLs in Chinese kale (B. alboglabra) sprouts under 160-mM NaCl stress. Meanwhile, the expression levels of indole GSLs biosynthesis genes MYB51 increased by fourfold. However, under normal cultivation conditions, the total GSLs content reduced 70.1% after 100-nM BR treatment, which indicates that BR may synergistically participate in GSL biosynthesis with NaCl (Wang et al., 2020).
Ethylene (ET) is a gaseous metabolite found in plants, which can inhibit plants growth and promotes leaf loss and fruit ripening (Bleecker and Kende, 2000). Sun et al. (2012a) concluded that there were no significant changes in the content of individual and total contents of aliphatic and indole GSLs in Chinese kale (B. alboglabra) after Ethrel vapor fumigation (Sun et al., 2012b). However, Thiruvengadam et al. (2015b) found that the ET significantly increased the contents of indole, aliphatic, and aromatic GSLs in rapeseed (B. napus L.) (Thiruvengadam et al., 2015b). As an inhibitor of ethylene, 1-methylcyclopropene (1-MCP) inhibited the decrease of total GSLs, sulforaphane, glucoraphanin, glucobrassicin in Chinese kale (B. alboglabra), broccoli (B. oleracea L. var. italica), and cabbage (B. oleracea L. var. capitata L.) (Yuan et al., 2010; Sun et al., 2012a; Kang et al., 2013; Xu et al., 2013).
The BRs function through a complex signal transduction pathway involving the BR receptor BRI1 and its co-receptor BAK1. In addition, the BR signaling components BZR1 and BES1 are important transcription factors involved in the BR-signaling pathway that participates in the regulation of GSL biosynthesis by BR. BR signaling affects GSL metabolism via transcriptional and/or post-translational modifications of compounds in the GSL catabolic pathways (Lee et al., 2018; Wang et al., 2020). Plant responses are coordinated by several signaling systems. One of these, the oxylipin-signaling pathway includes the JA and related compounds, and has been shown to influence the production of various metabolic defenses, including GSL (Howe and Jander, 2008; Erb et al., 2012). ET pathways, which are one of the JA-signaling pathways, cross-communicate with other hormonal pathways, and the impact of ET signaling may involve in GSL biosynthesis mechanisms (Erb et al., 2012; Kang et al., 2013).
Auxins and Gibberellic Acid
Auxins are the first discovered growth-promoting hormone in plants. Using different concentrations of indole−3−acetic acid (IAA) to treat hairy root culture of broccoli (Brassica oleracea L. var. capitata L.), Kim et al. (2013) concluded the accumulation of total GSLs, glucoraphanin, gluconapin, 4-hydroxyglucobrassicin, glucoerucin, glucobrassicin, 4-methoxyglucobrassicin, gluconasturtiin, and neoglucobrassicin, increased after 0.1-mg/L IAA treatment (Kim et al., 2013). Similar to IAA, 0.1-mg/L indolebutyric acid (IBA) and naphthylacetic acid (NAA) also resulted the highest accumulation of the total GSLs and the contents of eight individual GSLs (glucoraphanin, gluconapin, 4-hydroxyglucobrassicin, glucoerucin, glucobrassicin, 4-methoxyglucobrassicin, gluconasturtiin, and neoglucobrassicin) (Kim et al., 2013).
As a widely used hormone in plant production, gibberellic acid (GA3) can improve the growth, germination, flowering, and yield, as well as enhance plant-disease resistance (Maurya et al., 2019; Abbasi et al., 2020). Miao et al. (2017) found the GA3 treatment did not exert a remarkable influence on the aliphatic and total GSLs contents but increased the glucobrassicin Chinese kale (B. alboglabra) sprouts. However, combined treatment of GA3 and glucose increased the total GSLs content, indicating that GA3 and glucose synergistically regulate the biosynthesis of GSL (Miao et al., 2017).
It has recently been shown that the cytochromes CYP79B2 and CYP79B3 metabolize tryptophan to indole-3-acetaldoxime. This metabolite is often suggested to be the precursor of indole-3-acetonitrile (IAN) in IAA biosynthesis as well as the precursor of thiohydroximates in GSL biosynthesis. CYP83B1 is a regulator of auxin production by controlling the flux of indole-3-acetaldoxime into IAA and indole GSL biosynthesis (Bak et al., 2001; Grubb et al., 2004; Malka and Cheng, 2017; Salehin et al., 2019). In addition, CYP83B1 catalyzes the first committed step in indole GSL biosynthesis by metabolizing indole-3-acetaldoxime to its corresponding aci-nitro compound. Furthermore, the phylogenetic relationship between CYP83B1 and CYP71E1, the cytochrome P450 involved in the oxime-metabolizing step in cyanogenic glucoside biosynthesis, argues for an evolutionary relationship between IAA, GSL, and cyanogenic glucoside biosynthesis (Bak et al., 2001; Grubb et al., 2004; Salehin et al., 2019).
Abscisic Acid
Unlike other plant hormones, abscisic acid (ABA) could inhibit plant growth by promoting leaf shedding, causing bud dormancy and inhibiting cell elongation (Xie et al., 2020). Keling and Zhu (2013) concluded that 10-mg/L ABA decreased the proportion of aliphatic GSLs but increased the relative percentages of indole and aromatic GSLs in pak choi (B. rapa L.) shoots (Keling and Zhu, 2013). Meanwhile, the expression levels of the MYB28, MYB51, and MYB122, which are involved in indole and aliphatic GSL biosynthesis, were also negatively regulated in A. thaliana (Peskan-Berghofer et al., 2015). However, by application of different concentrations of ABA on cabbage (B. oleracea L. var. capitata L.) sprouts, the total GSLs content increased 72.7% under 50 μM (Wang et al., 2015). And indole GSLs (glucobrassicin, 4-methoxyglucobrassicin, neoglucobrassicin, and 4-hydroxyglucobrassicin) and aromatic GSL (gluconasturtiin) contents were significantly increased in turnip (B. rapa L. subsp. rapa) (Thiruvengadam et al., 2015b,2016a). The reasons for the discrepancies among the results of these studies might be the differences in the concentration of ABA treatments and the experimental species.
As a stress hormone, ABA content increases rapidly under drought, cold, high temperature, salt, and waterlogging. These abiotic stresses increase ABA delivery to guard cells, and the hydrolysis of GSLs catalyzed by myrosinases is also induced in some manner by ABA (Zhi et al., 2008). Meanwhile, ABA enhanced the expression levels of MYB, CYP79F1, and CYP83B1, and several sulfotransferase homologs in steps of the GSL core formation (Thiruvengadam et al., 2015b,2016a; Miret et al., 2017).
Other Plant Growth-Regulating Substances
Studies have shown that, in addition to JA, MeJA, SA, EBR, and melatonin, certain other substances also can affect the synthesis of GSLs. Melatonin (MT) is an indole substance found in many organisms that, in plants, promotes seed germination, plant growth, and adventitious root formation (Chen et al., 2020; He and He, 2020). About 100-μM MT increased the total GSLs, sulforaphane, and glucoraphanin contents in postharvest broccoli (B. oleracea L. var. italica) (Wei et al., 2019); 1-mM MT also sustained higher content of GSLs and glucoraphanin in broccoli (B. oleracea L. var. italica) florets (Miao et al., 2020). Transcriptomics analysis showed the expression levels of glucoraphanin biosynthesis-related genes increased under MT treatment in broccoli (B. oleracea L. var. italica) hairy roots (Tian et al., 2021). Melatonin also enhanced the biosynthesis of cabbage (B. oleracea L. var. capitata L.) GSL and increased the GSL contents (Teng et al., 2021). These results showed that the MT could enhance the glucoraphanin content, and the different organs had different melatonin response concentrations.
6-benzylaminopurine (6-BAP) inhibited the decrease rate of total GSLs content in harvested broccoli (B. oleracea L. var. italica) florets (Xu et al., 2011), and 200 mg/L6-BA + 2.5 μL/L1-MCP enhanced the biosynthesis of GSLs and the formation of the sulforaphane (Xu et al., 2013). 5-aminolevulinic acid (ALA) increased the aliphatic, aromatic, and total GSLs contents, while the indole GSLs content (glucobrassicin, 4-methoxyglucobrassicin, and 1-methoxyglucobrassicin) decreased by regulating the expression level of the UGT79B1, MYB12, and MYB28 in rapeseed (B. napus L.) seedlings (Maodzeka et al., 2019). Folic acid and coronatine effectively increase the level of GSLs in the post-harvested broccoli (B. oleracea L. var. italica) and suspension-cultured cells (Sánchez-Pujante et al., 2020). After liquiritin treatment, the total GSLs, 4-hydroxyglucobrassicin, 4-methoxyglucobrassicin, glucobrassicin, progoitrin, glucoraphanin, glucoiberin, neoglucobrassicin, and sinigrin contents significantly increased by enhancing the expression levels of genes involved in cabbage (B. oleracea L. var. capitata L.) GSL biosynthesis (Akram et al., 2020). Due to the lack of research reports on these substances, the mechanism of their regulation of GSL remains unclear.
Metal Ions
Selenium
As a rare element, selenium (Se) plays an important role in maintaining plant growth and development, promoting stress tolerance, enhancing resistance, and improving the quality index of cruciferous vegetables (Barickman et al., 2013; Azizi et al., 2020; Yin et al., 2020). SeO2 increased the amount of gluconasturtiin, glucobrassicanapin, glucoallysin, glucobrassicin, 4-methoxyglucobrassicin, and 4-hydroxyglucobrassicin rapeseed (B. napus L.) (Thiruvengadam and Chung, 2015). Na2SeO3 increased the formation of cabbage (B. oleracea L. var. capitata L.) indole-3-carbinol and indole-3-acetonitrile (Penas et al., 2012); the total GSLs and the sinigrin contents also increased exposure to Na2SeO3 alone or in combination with NaCl (Sun et al., 2018). Besides, the glucoraphanin, glucobrassicin, 4-methoxy-glucobrassicin, and total indole GSLs contents were significantly affected (Gao et al., 2021), while the aliphatic GSLs (glucoraphanin and glucoerucin) were not affected by Na2SeO3 in broccoli (B. oleracea L. var. italica) sprouts (He et al., 2020). Same with SeO2 and Na2SeO3, Na2SeO4 also significantly increased the expression of GSL biosynthesis genes in broccoli (B. oleracea L. var. italica) florets (Kim and Juvik, 2011; Rao et al., 2021). However, total GSLs contents in Eruca sativa Mill and Diplotaxis tenuifolia decreased after Na2SeO4 treatment (Dall’Acqua et al., 2019). Meanwhile, the glucoraphanin, glucocheirolin, glucoerucin, dimeric-4-mercaptobutyl, glucosativin, and neoglucobrassicin contents of Eruca S. Mill and D. tenuifolia (Dall’Acqua et al., 2019), and total GSL in radish (R. sativus L.) (Mckenzie et al., 2019) did not increase significantly. Total GSLs and sulforaphane levels were also not significantly influenced in broccoli (B. oleracea L. var. italica) sprouts between Na2SeO3 and Na2SeO4 treatments (Tian et al., 2016; Mahn, 2017). These studies indicated that different plant species, ages, and anions could lead to different biosynthesis modes of GSL.
Because of the similar structure of Se and sulfur (S), the absorption and transport modes of these two mineral elements in plants are basically the same, which leads to an antagonistic relationship between Se and S. Se is often serves as a substitute for S in physiological and metabolic processes in plants (Barickman et al., 2013). Se increases S uptake by preventing its downregulation at the plant’s roots; increasing Se helps S uptake into the plants more than increasing S fertilizer concentrations alone (Toler et al., 2007), and then affects the biosynthesis of GSLs by inducing the expression level of MYBs, CYP79F1, and CYP83B1 (Sepuveda et al., 2013; Thiruvengadam and Chung, 2015; Mckenzie et al., 2019).
Calcium and Zinc
Calcium is an essential nutrient element in plants and plays a central role in plant growth, development, and the response to environmental stress (Gao et al., 2020; Yoshioka and Moeder, 2020). Environmental stress enhances the Ca2+ content in plant tissues and mitigates adverse effect of plant (Sarker and Oba, 2018e; Sarker et al., 2018). Aliphatic GSLs (glucoerucin, glucoiberin, glucoiberverin, glucoraphanin, pentyl-GSL, and hexyl-GSL), indole GSLs (glucobrassicin, neoglucobrassicin, and 4-hydroxyglucobrassicin) (Sun et al., 2015), and sulforaphane contents in broccoli (B. oleracea L. var. italica) sprouts also increased significantly after the CaCl2 treatment (Yang et al., 2015b). And with an increase in the CaCl2 concentration, the total GSLs content increased first and then decreased (Yang et al., 2016). Similar to CaCl2, after preharvest CaSO4 treatment, the total GSLs, glucoraphanin, glucoerucin, glucobrassicin, and 4-hydroxyglucobrassicin contents increased significantly (Guo L. et al., 2018).
As one of the essential trace elements in plants, Zinc (Zn) is not only involved in the formation of auxin but also is a component and an activator of many enzymes (Atsushi et al., 2020). Zn has extensive effects on carbon and nitrogen metabolism, photosynthesis, and stress resistance of plants. In addition, Zn affects protein synthesis; therefore, in agricultural production, Zn supplementation is becoming increasingly popular. Low concentration of ZnSO4 and ZnCl2 increased the GSL contents in white cabbage (B. oleracea var. capitata f. alba) (Kusznierewicz et al., 2012), broccoli (B. oleracea L. var. italica) sprouts (Yang et al., 2015a; Rivera-Martin et al., 2021), and Noccaea caerulescens (Fones et al., 2019), turnip (B. rapa L. subsp. rapa) (Aghajanzadeh et al., 2020), and pak choi (B. rapa L.) (Fatemi et al., 2020).
Calcium plays an important role in regulation plant growth and signal transduction as well as the accumulation of secondary metabolites in plants under either natural or stress conditions (Medvedev, 2018; Iqbal et al., 2020). In the present study, Ca2+ is important in enhancement of GSL content during plant growth and storage (Yang et al., 2016). Ca2+ increased the formation of ITCs and GSL through indirect effects instead of acting on MYR activity during hydrolysis (Guo L. et al., 2018). In the tolerance range of Zn stress, Zn increased the accumulation of indole GSL and enhanced the antioxidant capacity and defense capacity of plants (Aghajanzadeh et al., 2020). Meanwhile, the biosynthetic precursor of GSL was increased by promoting the formation of cysteine (Cys) through the sulfur assimilation pathway (Aghajanzadeh et al., 2020), and the expression levels of indole GSL biosynthesis-related genes (MYB34, CYP79B3, and CYP83B1) were also significantly increased (Yang et al., 2015a).
Cadmium and Arsenic
Cadmium (Cd), as one of the most harmful heavy metals, causing serious soil pollution, is not an essential element for plant growth. Cd can cause harm to plants at low concentrations, such as reducing the activity of enzymes and photosynthetic intensity, causing metabolic disorders, changing membrane permeability, preventing root growth, inhibiting root absorption of water and nutrients, and decreasing crop yield and quality (Hayashi et al., 2020; Jocsak et al., 2020). Therefore, it is of great scientific significance to study the effects of Cd on plant growth and development, and the tolerance mechanism of plants. CdCl2 and CdSO4 significantly decreased the total GSLs concentration in the A. thaliana, Thlaspi praecox, Thlaspi arvense, cabbage (B. oleracea L. var. capitata L.), and kale (B. oleracea var. acephala DC) (Roser et al., 2006; Sun et al., 2009; Kusznierewicz et al., 2012; Jakovljevic et al., 2013). Glucoibervirin and 4-methoxyglucobrassicin levels decreased significantly in the CdCl2-treated leaves, while the glucobrassicin, neoglucobrassicin, and 4-methoxyglucobrassicin levels all showed significant decreases in the A. thaliana roots (Sun et al., 2009). Similarly, a decrease in both aliphatic and indole GSLs contents associated with an increase in CdCl2 accumulation was observed in the roots and shoots of rapeseed (B. napus L.) plantlets under in vitro sterile conditions, demonstrating that Cd stress has a highly significant effect on roots’ and shoots’ GSL biosynthesis (Durenne et al., 2018). Different from A. thaliana and rapeseed (B. napus L.), CdCl2 had considerably enhanced gluconasturtiin and 4-hydroxyglucobrassicin levels in turnip (B. rapa L. subsp. rapa). Moreover, genes related to GSLs showed significant induction (Thiruvengadam and Chung, 2015).
As plants grow, they absorb arsenic from the environment, either passively or actively, causing damage to plants (Samanta et al., 2021). By examining two mustard (Brassica juncea) cultivars under different concentrations of Arsenic (As) stress, Pandey et al. (2016) concluded the As stress reduced the amount of total GSLs, and the GSLs content decreased with the increase of As concentration. Meanwhile, the overall contents of total aliphatic and indole GSLs decreased (Pandey et al., 2016).
Cadmium stress inhibits organic sulfur from entering the GSL synthesis pathway, promotes the biosynthesis of GSH and phytochelatins (PC) to detoxification, and enhances the tolerance mechanism of plants (Jakovljevic et al., 2013; Durenne et al., 2018). Under cadmium stress, plants can reduce the content of indolyl methyl 3-GSL, promote the generation of IAA, and regulate root growth (Jakovljevic et al., 2013; Durenne et al., 2018). After Cd2+ stress, cells can activate mitogen-activated protein kinase (MAPK), Ca2+/calmodulin system (Ca2+/CaM), JA, SA, and other stress response signal molecules. Then, these signal molecules fuse to regulate the family of transcription factors such as MYB in the nucleus (Xu et al., 2015).
Cuprum and Ag NPs
Cuprum (Cu) is not only a component of various enzymes but is also closely related to carbon assimilation, nitrogen metabolism, absorption, and redox processes in plants (Letchumanan et al., 2021; Yusuf et al., 2021). At the same time, it is beneficial to the growth and development of crops and can affect the photosynthetic capacity and drought- and cold-resistance ability. Drought stress augmented the Cu content in plant tissues and mitigated the adverse effect of plants (Sarker and Oba, 2018d). High concentrations (50 ∼ 500 mM) CuSO4 decreased the turnip (B. rapa L. subsp. rapa) GSL content (Jahangir et al., 2008). The GSL content of Nasturtium officinale also decreased under 0.1 ∼ 1-mM CuCl2 (Kim J. et al., 2018). Under CuCl2 stress, the content of total GSLs, and indole and aromatic GSLs were only elevated in the Chinese cabbage [Brassica pekinensis (Lour.) Rupr.] roots. By enhancing the transcript levels of CYP79B2, CYP83B1, and MYB51 in GSL biosynthesis, the indole GSLs and glucobrassicin levels increased by two and fourfold under treatment with 5- and 10-μM CuCl2, respectively. In addition, the indole GSLs in the roots could be considered as an index to judge effects of the CuCl2 concentrations (Aghajanzadeh et al., 2019).
Currently, research on the effect of Argentum (Ag) on the synthesis of GSLs has mainly focused on silver nanoparticles (AgNPs). In turnip (B. rapa L. subsp. rapa), glucoallysin, glucobrassicanapin, sinigrin, progoitrin, gluconapin, glucobrassicin, 4-methoxyglucobrassicin, 4-hydroxyglucobrassicin, neoglucobrassicin, and gluconasturtiin contents, and the levels of their associated transcription factors (MYB28, MYB29, MYB34, and MYB51) enhanced significantly after biologically synthesized AgNP treatment (Thiruvengadam et al., 2015a; Chung et al., 2018). The AgNPs also enhanced the total GSL content in A. thaliana (Kruszka et al., 2019; Zhang et al., 2019). Similar with AgNP, low concentration of AgNO3 (10 μM) enhanced the accumulation of glucosiberin and glucohirsutinin N. officinale (Kim J. et al., 2018).
Similar to Zinc, Cu can also enhance the accumulation of indole GSL within the tolerance range, and then enhance the antioxidant capacity and defense capacity of plants (Aghajanzadeh et al., 2019). The distribution of organic sulfur to GSL in plants under Cu stress was higher than that of organic sulfur compounds such as GSH, thus promoting the accumulation of GSL (Aghajanzadeh et al., 2019). Moreover, Cu stress promoted indole GSL biosynthesis by enhancing the expression of MYB51, CYP79B2, and CYP83B1 (Kolbert et al., 2012; Aghajanzadeh et al., 2019). Ag can induce ROS production and subsequently increase the expression levels of MYB transcription factor, SUR1, and ST5C, thus inducing the signal transduction pathway of GSL accumulation (Thiruvengadam et al., 2015a; Chung et al., 2018).
Salinity Stress
Salinity stress causes many physiological and molecular changes, such as imbalance in Na+ and K+ (Sarker and Oba, 2020), creation of reactive oxygen species (ROS) and oxidative stress (Sarker and Oba, 2018b), osmotic stress (Sarker and Oba, 2018c), which eventually interrupt the growth and productivity of crops (Sarker and Oba, 2018a,2019). Saline stress is mainly NaCl stress. In general, the GSL content increased under low and moderate salt stress; 40 ∼ 160-mM NaCl increased total GSL, glucoerucin, glucobrassicin, sulforaphane, neoglucobrassicin, and 4-hydroxy glucobrassicin contents in broccoli (B. oleracea L. var. italica) (Carmen et al., 2008; Gioia et al., 2018; Rios et al., 2020). Glucoalyssin, gluconapin, glucobrassicin, and neglucobrassicin contents in pak choi (B. rapa L.) enhanced under 50-mM NaCl (Hu and Zhu, 2010). In cabbage (B. oleracea L. var. capitata L.), the total GSLs increased (Sun et al., 2018); the aliphatic and indole GSLs increased 1.29- and 1.42-fold, respectively (Wang et al., 2020). And the GSL contents of Chinese cabbage [Brassica pekinensis (Lour.) Rupr.], cabbage (B. oleracea L. var. capitata L.), kale (B. oleracea var. acephala DC), and E. sativa were elicited by NaCl in a dose-dependent manner (Cocetta et al., 2018; Linic et al., 2019; Samec et al., 2021), and different rapeseed (B. napus L.) genotypes also showed varying levels of GSL accumulation (Gyawali et al., 2019). However, the GSL content decreased under high NaCl concentrations in red radish (R. sativus L.) (<500 mM) (Chen et al., 2018), and D. tenuifolia (130 mM) (Petretto et al., 2019). KCl, Na2SO4, K2SO4, and other salts can also affect the biosynthesis of GSL. About 50 ∼ 100-mM KCl did not affect the total GSLs, the GSL composition in the roots, whereas it resulted in an up to 60% decrease in total GSLs content of the shoot in turnip (B. rapa L. subsp. rapa) (Aghajanzadeh et al., 2018). The total and individual GSLs in the root increased 1.8 ∼ 4.5-fold in the 50-mM Na2SO4, while 50-mM K2SO4 significantly increased the contents of indole and aromatic GSL in the shoots. Phosphate-sufficient plants exhibited lower GSL concentrations, phosphite increased the pak choi (B. rapa L.) 1-methoxyindol-3-ylmethyl content compared with low concentration of phosphite, while high phosphite levels increased the but-3-enyl-GSL concentration compared with the medium levels of phosphite in mustard (B. juncea) (Trejo-Tellez et al., 2019).
When salinity stress is within the tolerance level of plants, the increase of GSL content will participate in osmotic regulation to maintain water balance (Carmen et al., 2008), enhance plant-defense response, and maintain plant growth (Aghajanzadeh et al., 2018; Fatemi et al., 2020). However, high concentration of salinity stress can increase the activity of GSL degradation enzyme DtTMT, resulting in the decrease of GSL content. Plants will distribute more sulfur to the primary assimilation process, promote the glutathione (GSH) biosynthesis, thus limiting the GSL biosynthesis (Reich et al., 2017; Aghajanzadeh et al., 2018). At the same time, the enhanced ROS signal in chloroplast of plants under salt stress will further promote the transcription level of transcription factor gene MYBs and GSL biosynthetic genes (Sewelam et al., 2014; Pilarska et al., 2016; Aghajanzadeh et al., 2018; Wang et al., 2020).
Glucose and Sucrose
In the process of plant growth, glucose (Glu) is generated by photosynthesis. When subjected to the stress of an adverse environment, the Glu generated cannot meet the growth and reproduction needs of the plants. Exogenous supplementation of Glu could increase plant-stress resistance (e.g., cold resistance and drought resistance), and promote growth and propagation. By exogenous Glu treatment, the glucoraphasatin, 4-OH-glucobrassicin, and 4-methoxyglucobrassicin contents dramatically reduced in Chinese kale (B. alboglabra) and radish (R. sativus L.) sprouts, while the content of gluconapin and glucobrassicanapin increased markedly (Wei et al., 2011). Total GSLs, glucoraphanin, glucoraphenin, and glucobrassicin contents of cabbage (B. oleracea L. var. capitata L.), rapeseed (B. napus L.), turnip (B. rapa L. subsp. rapa), and mustard (R. sativus) increased after treatment with 277-mM Glu (Bae et al., 2014). However, Glu did not influence the individual and total GSLs of the Chinese kale sprouts significantly (Miao et al., 2017). Glu + JA enhanced the A. thaliana GSL content significantly, whereas the synergistic effect of SA + Glu was less obvious (Guo et al., 2013a; Miao et al., 2013, 2016). The induction of indole and aliphatic GSLs was inhibited after treatment with JA and Glu. In addition, in the Glu-insensitive mutants, the effects of JA and Glu on the GSL content reduced significantly, suggesting that JA and Glu signaling is involved in cross-talk in regulating GSL biosynthesis. Moreover, Glu upregulates GSLs via the ABA signaling pathway and decreased accumulation of 4-methylthiobutyl (Li et al., 2021). Same with Glu, the contents of total GSLs, glucoiberin, glucoraphanin, glucobrassicin, sulforaphane, glucobrassicin, and neoglucobrassicin in cabbage (B. oleracea L. var. capitata L.), rapeseed (B. napus L.), turnip (B. rapa L. subsp. rapa), and radish (R. sativus) sprouts, and broccoli (B. oleracea L. var. italica) florets increased significantly and maintained a higher level after sucrose treatment (Guo et al., 2011; Bae et al., 2014; Xu et al., 2016), while the level of GSLs in fructooligosaccharides (FOS)-treated turnip (B. rapa L. subsp. rapa) decreased markedly (Thiruvengadam et al., 2016b).
Sugars play important roles in plant growth and development as a carbon and energy source. They can also act as effective signaling molecules throughout plant life (Bolouri-Moghaddam et al., 2010; Smeekens et al., 2010). Hexokinases (HXKs) are one of the most conserved sugar sensors together with other sugar kinases, and carry out diverse and distinct functions in glucose metabolism and signaling (Rolland et al., 2002; Miao et al., 2013). Among them, HXK1-dependent glucose signaling can affect plant growth, which relies on the endogenous glucose level and the sensitivity to glucose. By regulating the HXK1-mediated signaling, the MYB transcription factors expression levels, which participated in the regulation of GSL biosynthesis, changed (Fritz et al., 2010; Guo et al., 2013a; Miao et al., 2013).
Environmental Pollution
Gaseous Contamination
Gaseous contamination (SO2, H2S, and O3) is a very serious environmental problem affecting crop growth, which can lead to changes in the biosynthesis of bioactive molecules (Mukherjee et al., 2019). By determining the shoots and roots, GSL contents treated with different sulfur sources (i.e., sulfate, sulfite, and sulfide), SO2, and H2S. Aghajanzadeh et al. (2014, 2015) concluded that sulfate deprivation resulted in a strong decrease in the content and an altered composition of the mustard (B. juncea) and turnip (B. rapa L. subsp. rapa) GSLs. H2S and SO2 did not affect the total content but slightly affected the GSL composition in the shoots and roots of turnip (B. rapa L. subsp. rapa). Meanwhile, SO2 and H2S exposure largely alleviated the decrease in C4-aliphatic GSLs (glucoerucin, gluconapin, and progoitrin) in roots of turnip (B. rapa L. subsp. rapa) and mustard (B. juncea) (Aghajanzadeh et al., 2014, 2015). Different from SO2 and H2S, O3 pollution can inhibit the accumulation of GSL. The total GSLs contents in rapeseed (B. napus L.) (Gielen et al., 2006; Himanen et al., 2008), black mustard (B. nigra) (Khaling et al., 2015), turnip (B. rapa L. subsp. rapa) (Han et al., 2020, 2021) decreased, while the accumulation of aromatic GSL increased (Han et al., 2020, 2021).
Greenhouse effect leads to the increase of CO2 content in the air, which then affects GSL on plants. High CO2 concentration significantly increased the contents of aromatic GSL in cabbage (B. oleracea L. var. capitata L.) (Reddy et al., 2004), and rapeseed (B. napus L.) leaves (Himanen et al., 2008), but had no significant effect on the total amount of GSL. However, as CO2 treatment enhanced primary production, the total GSLs contents of broccoli (B. oleracea L. var. italica) (Schonhof et al., 2007; Rodriguez-Hernandez et al., 2014; Almuhayawi et al., 2020), Chinese kale (B. alboglabra) (La et al., 2009), Brussels sprout (B. oleracea var. gemmifera) (Klaiber et al., 2013), A. thaliana (Paudel et al., 2016), kale (B. oleracea var. acephala DC) (Chowdhury et al., 2021), and the accumulation of glucoraphanin and sulforaphane also increased in broccoli (B. oleracea L. var. italica) sprouts (Almuhayawi et al., 2020). Thus, it is conceivable to recycle excess CO2 by using it as supplement greenhouse gas to produce high-GSL cruciferous plants (Wiesner-Reinhold et al., 2021).
Accumulation of aromatic and indole GSL under O3 stress can enhance the mechanism of antioxidant damage and physical damage resistance in plants, and then induce the accumulation of JA and SA, which promote the biosynthesis of indole GSL and aromatic GSL (Han et al., 2021). Therefore, O3 regulates the biosynthesis of GSL by inducing the production of ROS and activating the JA and SA signal transduction pathways (Zhang et al., 2017). However, the influence of O3 stress on the transcription levels of GSL transcription factors and biosynthetic genes remains to be further studied. The pathway of GSL response under H2S stress has not been reported. CO2 induces the formation of amino acids and the precursors of GSL biosynthesis through different pathways. High concentrations of CO2 not only promote the biosynthesis of Cys (the precursor of Met) (Rodriguez-Hernandez et al., 2014) but also promote the accumulation of amino acids and other compounds (Rodriguez-Hernandez et al., 2014; Almuhayawi et al., 2020).
Chemical Pollution
Pesticides are indispensable chemical agents in modern agricultural production to control diseases, insect pests, and weeds, and also play an important role in the yield and quality of crops (Huang et al., 2021; Zhang and Yang, 2021). However, the excessive use of pesticides causes great harm to the natural environment and induces pesticide stress, which affects the normal physiological and metabolic activities of crops. Imidacloprid, pyramezone, beta-cypermethrin, and acephate treatments significantly increased the contents of Pak Choi (B. rapa L.) total GSLs content and all individual GSL contents, especially the aliphatic group (Zhu et al., 2015). In fluridon (Flu)-treated sprouts, the cabbage (B. oleracea L. var. capitata L.) GSL content and isothiocyanate formation reduced by 46.5 and 38%, respectively (Wang et al., 2015). By increasing the content of amino acids of the precursor of GSL biosynthesis, pesticides can increase the content of GSLs in Pak Choi (B. rapa L.) (Zhu et al., 2015).
There are many inorganic and organic toxic substances in the environment that potentially affect plants (Thompson and Darwish, 2019). The contents of total GSLs and sulforaphane increased significantly after treatment with mannitol in broccoli (B. oleracea L. var. italica) (Guo et al., 2011). Putrescine treatment enhanced 4-hydroxyglucobrassicin and gluconasturtiin contents considerably in turnip (B. rapa L. subsp. rapa) (Thiruvengadam and Chung, 2015). Adenosine monophosphate (AMP) and gamma-aminobutyric acid (GABA) increased the amount of GSLs significantly in turnip (B. rapa L. subsp. rapa) (Thiruvengadam et al., 2016b). Using an RNA sequencing analysis, Gudio et al. (2018) detected the CYP79B3 was misregulated and decreased the amount of A. thaliana indole GSLs in the presence of carbenicillin (Gudio et al., 2018).
Future Work
As more biological functions of GSLs and their metabolites are gradually revealed in plant defense, human anti-cancer antibacterial, biological control, the regulation of GSL biosynthesis has become one of the hot topics in research into specialized metabolism and the stress response of cruciferous plants (Vo et al., 2018; Mitreiter and Gigolashvili, 2021; Sun and Zhang, 2021). Various researchers have carried out a large number of studies on the regulation mechanism of GSL synthesis. These studies have shown that there are many factors affecting the GSL biosynthesis and component content, including genotype, development stage, organs, and environmental conditions (Rhee et al., 2020; Chowdhury et al., 2021). Plant hormones, plant growth regulators, mineral elements, heavy metal, antibiotics, and other chemicals can also affect GSL biosynthesis (Figure 1) (Yuan et al., 2010; Kim et al., 2013; Zhou and Johan, 2017; Jocsak et al., 2020). Meanwhile, the content and composition of GSLs are closely related to the plant materials, plant organ/tissue, and the concentration of these chemicals (Bruinsma et al., 2007). Same concentration of a biological agent leads to different GSL compositions and response mechanisms in different plants, and the response mechanism of same chemicals in different periods is also different (Wang et al., 2015; Miret et al., 2017). Moreover, there are cross-talk effects among these chemicals (Figure 2). These studies provide data support and theoretical guidance for the use of exogenous chemicals to regulate the content of GSLs in the Cruciferae in subsequent production (Table 1). However, most of these studies focused on the changes in the GSL content induced by exogenous chemicals, and regulation at the transcriptional level, the specific upstream action elements, and regulation modes of transcription factors in the process of responding to exogenous chemicals require further research (Ku et al., 2014; Zhou and Memelink, 2016). Moreover, many studies have confirmed that GSL synthesis is regulated cooperatively by different hormones, chemicals, and environments; however, the interaction mechanisms among these substances are still unclear (Traw et al., 2003; Cipollini et al., 2010; Zang et al., 2015a) (Figure 2); that is, how the crop perceives the stimulation of exogenous hormones, growth regulators, or chemical substances, which pathways subsequently act on GSL synthesis, and how feedback regulates GSL synthesis after the stimulation disappears. Elucidation of this interaction mechanism will not only enrich the regulation network of GSL biosynthesis but also provide new ideas and methods to regulate the GSL biosynthesis by using chemical substances.
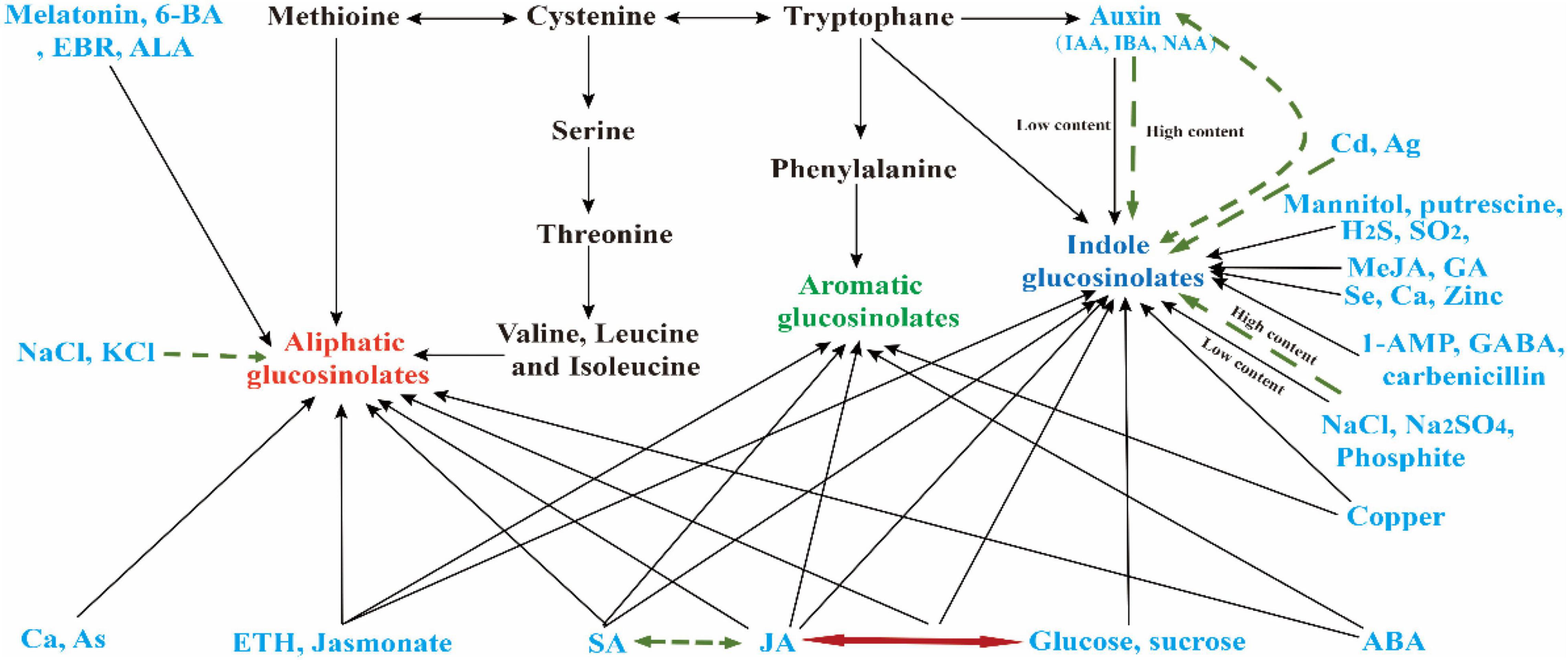
Figure 2. Relationship between different chemicals in GSLs biosynthesis. Figures in a blue font represent different exogenous chemicals; black and green single arrows indicate chemicals that can promote and inhibit the synthesis of GSL, respectively. Green-dotted-line double arrows represent the two substances showing antagonism in the synthesis of GSL, while the red double arrow indicates that the two substances coordinate to regulate GSL synthesis in Cruciferous crops.
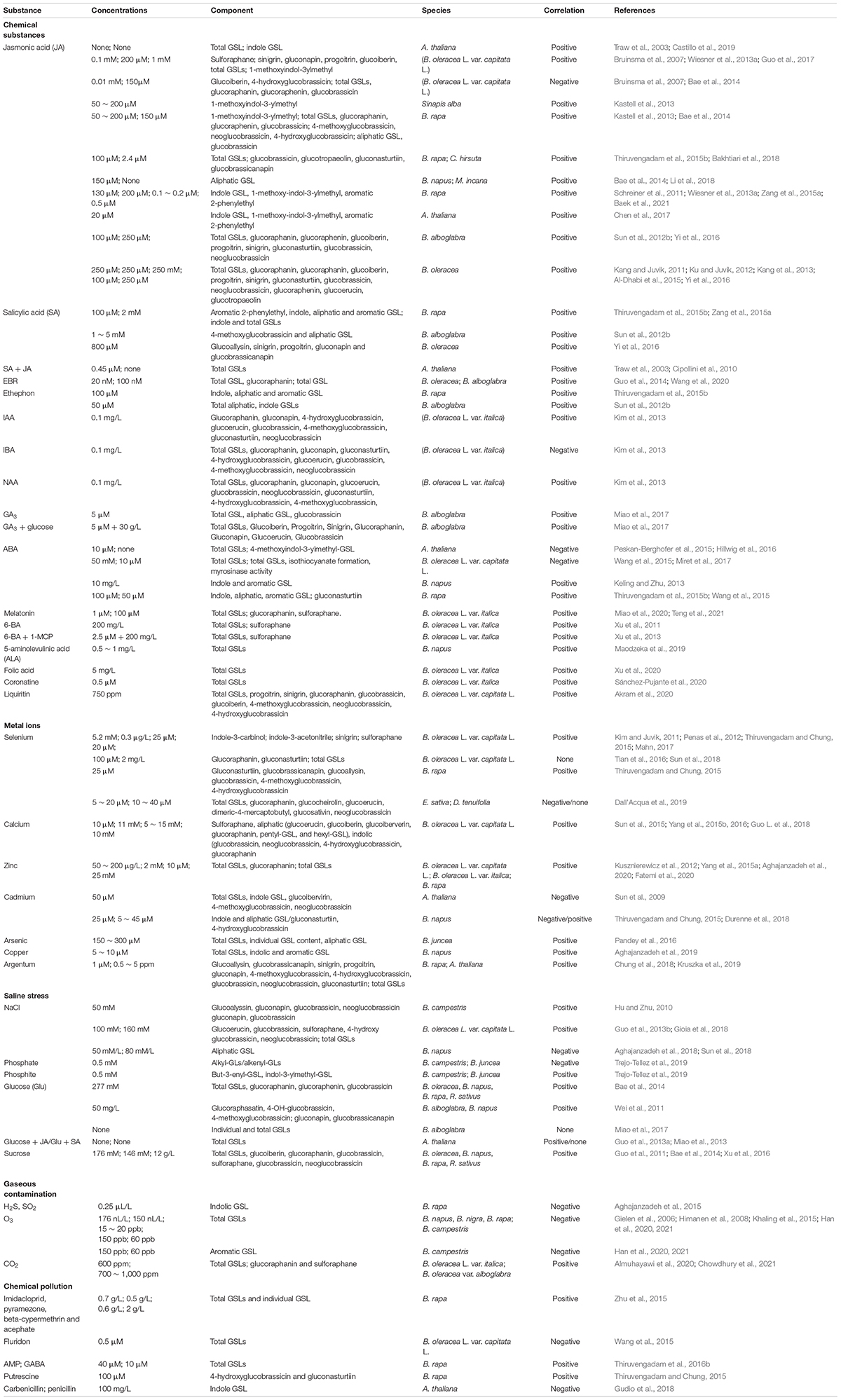
Table 1. Effects of different exogenous chemical substances on glucosinolates (GSL) synthesis in Cruciferous crops.
With the development of molecular biology technology, the molecular biological mechanism of GSL biosynthesis, accumulation, and transport have made great progress recently, especially in A. thaliana (Barco and Clay, 2019; Kim et al., 2020). A large number of studies have shown that GSL biosynthesis is a complex process, which is regulated by multiple layers comprising genetic sites, developmental processes, transcription, and synthetic products (Barco and Clay, 2019; Meier et al., 2019). At present, the biosynthetic framework roadmap of GSL is basically clear in A. thaliana, and most regulatory genes in related pathways are known, but the specific functions of these regulatory genes are not fully elucidated (Ashari et al., 2018; Vo et al., 2018). In this process, MYB transcription factors play an important role in the regulation of GSL formation. The regulation pathway can be summarized as: MYB transcription factors are stimulated; MYB further induces or inhibits the expression levels of BCAT4, MAM1, MAM3, and other key genes of GSL biosynthesis in response to exogenous stimuli, thus achieving positive or negative regulation of GSL biosynthesis (Peskan-Berghofer et al., 2015; Zhou and Memelink, 2016; Harus et al., 2020). Therefore, a study of the regulation of MYB transcription factors and other GSL biosynthesis genes could provide a theoretical basis for molecular breeding and high-quality cultivation of cruciferous vegetable crops. Different from A. thaliana, the biosynthesis and regulation MYB genes of GSL in most cruciferous crops have multiple copies (Yang et al., 2021); there may be differences in the function of the same genes among different species of plants and resulting in much more complex studies. Through this gene redundancy, vegetable crops can rapidly induce the synthesis of GSLs when subjected to stress and improve their defense ability through high content and multi-component GSLs (Yang et al., 2021). However, this also leads to a more complex regulatory network for GSL biosynthesis in these crops, requiring a lot of work to explore their regulatory mechanisms in terms of upstream and downstream transcription factors. Besides, it is also not clear whether transcription factors, combined with the downstream target genes encoding interaction proteins, work together, nor whether, after the addition or diminution of exogenous chemicals, the resultant high content of GSLs is involved in feedback to regulate the biosynthesis of GSLs, which require further a in-depth study to reveal the complex GSL biosynthetic regulation network (Figure 3). Through the study of GSL biosynthesis and regulatory networks, we could elucidate the exogenous stimulus response mechanism of transcription factors and the mechanism regulating GSL synthesis induction, further enriching our knowledge of the regulation network of GSL synthesis, and providing a theoretical basis for molecular breeding, high-quality cultivation, and biological control of diseases and insect pests of cruciferous vegetables with high GSL contents.
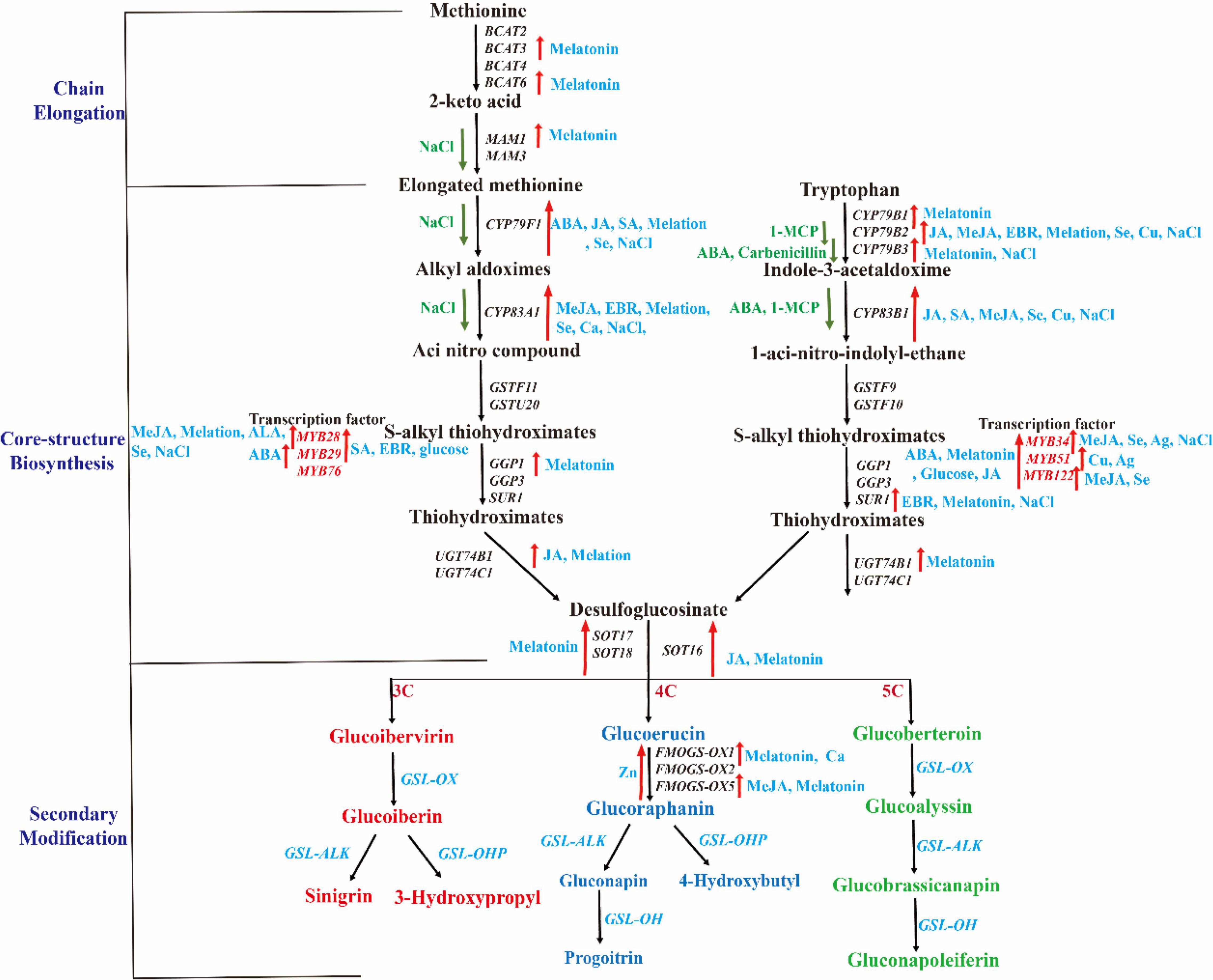
Figure 3. Regulation of different exogenous substances in Cruciferous crops GSL synthesis. The black italics and red texts represent related biosynthetic genes and transcription factors, respectively. The red and green arrows represent the gene expression increased or decreased after treatment with related chemicals.
The well-researched GSL biosynthesis regulation genes provide a direction for future Cruciferae molecular breeding. It is well-known that traditional breeding is time-consuming and difficult to change plant traits. Through molecular breeding, we can improve the content of beneficial GSL and reduce the content of harmful GSL in Cruciferae. For example, in the aliphatic GSL biosynthesis pathway, the anticancer glucoraphanin is first synthesized, and then the downstream 3-butenyl GSL is biosynthesized using glucoraphanin as raw material, which is then used to biosynthesize the harmful DL-GOITRIN (2-hydroxy-3-butenyl GSL). Through genetic engineering (e.g., RNA interference technology and gene editing technology), by knocking out the related genes that were involved in the subsequent biosynthesis after glucoraphanin, we can stop the process at the biosynthesis stage of sulforaphanin or making a very small amount of downstream GSL. In addition, because the biosynthesis of GSL has tissue specificity, the GSL can be transported after biosynthesis, so harmful GSL can be transported to non-edible organs and beneficial GSL can be transported to edible organs. By this method, high beneficial GSL plant products can be obtained while improving plant resistance to pests and diseases. Although there are a few reports on this aspect, the effect is not obvious and needs further research, especially the new gene editing technology has not been widely used in this research area (Abuyusuf et al., 2018; Petersen et al., 2019; Neequaye et al., 2021; He et al., 2022). It is believed that, in the near future, with the development of omics and gene editing technology, there will be a new understanding of the function and interaction network of GSL biosynthesis genes. Moreover, we can regulate GSL content and composition by functional editing of GSLs biosynthesis genes as needed.
Author Contributions
ZL, HW, and JY designed the research. JL, SL, JW, and LL prepared the manuscript. LH, GZ, and JX revised the manuscript. All authors have read and agreed to the published version of the manuscript.
Funding
This research was funded by Natural Science Foundation of Gansu Province, Grant No. 20JR10RA537; Special Project of Central Government Guiding Local Science and Technology Development, Grant No. ZCYD-2020-5; Gansu Provincial Key Laboratory of Arid land Crop Science, Gansu Agricultural University, Grant No. GSCS-2020-11; and Gansu Agricultural University Doctoral Research Initiation Fund, Grant No. GAU-KYQD-2019-30.
Conflict of Interest
The authors declare that the research was conducted in the absence of any commercial or financial relationships that could be construed as a potential conflict of interest.
Publisher’s Note
All claims expressed in this article are solely those of the authors and do not necessarily represent those of their affiliated organizations, or those of the publisher, the editors and the reviewers. Any product that may be evaluated in this article, or claim that may be made by its manufacturer, is not guaranteed or endorsed by the publisher.
References
Abbasi, B., Ullah, M., Nadeem, M., Tungmunnithum, D., and Hano, C. (2020). Exogenous application of salicylic acid and gibberellic acid on biomass accumulation, antioxidant and anti-inflammatory secondary metabolites production in multiple shoot culture of Ajuga integrifolia Buch. Ham. ex D.Don. Ind. Crops Prod. 145:112098. doi: 10.1016/j.indcrop.2020.112098
Abuyusuf, M., Robin, A., Kim, H., Islam, M., Park, J., and Nou, I. (2018). Altered glucosinolate profiles and expression of glucosinolate biosynthesis genes in ringspot-resistant and susceptible cabbage lines. Int. J. Mol. Sci. 19:2833. doi: 10.3390/ijms19092833
Aghajanzadeh, T., Hawkesford, M., and De Kok, L. (2014). The significance of glucosinolates for sulfur storage in Brassicaceae seedlings. Front. Plant Sci. 5:704. doi: 10.3389/fpls.2014.00704
Aghajanzadeh, T., Kopriva, S., Hawkesford, M., Koprivova, A., and De Kok, L. J. (2015). Atmospheric H2S and SO2 as sulfur source for Brassica juncea and Brassica rapa: impact on the glucosinolate composition. Front. Plant Sci. 6:924. doi: 10.3389/fpls.2015.00924
Aghajanzadeh, T., Prajapati, D., and Burow, M. (2019). Copper toxicity affects indolic glucosinolates and gene expression of key enzymes for their biosynthesis in Chinese cabbage. Arch. Agron. Soil Sci. 66, 1288–1301. doi: 10.1080/03650340.2019.1666208
Aghajanzadeh, T., Prajapati, D., and Burow, M. (2020). Differential partitioning of thiols and glucosinolates between shoot and root in Chinese cabbage upon excess zinc exposure. J. Plant Physiol. 244:153088. doi: 10.1016/j.jplph.2019.153088
Aghajanzadeh, T., Reich, M., Kopriva, S., and Kok, L. (2018). Impact of chloride (NaCl, KCl) and sulphate (Na2SO4, K2SO4) salinity on glucosinolate metabolism in Brassica rapa. J. Agron. Crop Sci. 204, 137–146. doi: 10.1111/jac.12243
Akram, W., Saeed, T., Ahmad, A., Yasin, N., Akbar, M., Khan, W., et al. (2020). Liquiritin elicitation can increase the content of medicinally important glucosinolates and phenolic compounds in Chinese kale plants. J. Sci. Food Agric. 100, 1616–1624. doi: 10.1002/jsfa.10170
Al-Dhabi, N., Arasu, M., Kim, S., Romijuddin, M., Park, W., Lee, S., et al. (2015). Methyl Jasmonate- and Light-Induced Glucosinolate and Anthocyanin Biosynthesis in Radish Seedlings. Nat. Prod. Commun. 10, 1211–1214.
Almuhayawi, M., Abdelgawad, H., Jaouni, S., Selim, S., and Khamis, G. (2020). Elevated CO2 improves glucosinolate metabolism and stimulates anticancer and anti-inflammatory properties of broccoli sprouts. Food Chem. 328:127102. doi: 10.1016/j.foodchem.2020.127102
Angelino, D., and Jeffery, E. (2021). Editorial: glucosinolate metabolites: bioavailability, bioactivity and clinical variability. Front. Nutr. 8:823203. doi: 10.3389/fnut.2021.823203
Anthony, M., Celenza, J., Armstrong, A., and Frey, S. (2020). Indolic glucosinolate pathway provides resistance to mycorrhizal fungal colonization in a non-host Brassicaceae. Ecosphere 11:e03100.
Ashari, K., Abdullah-Zawawi, M., Harun, S., and Mohamed-Hussein, Z. (2018). Reconstruction of the transcriptional regulatory network in Arabidopsis thaliana Aliphatic Glucosinolate biosynthetic pathway. Sains Malays. 47, 2993–3002. doi: 10.17576/jsm-2018-4712-08
Atsushi, O., Ikumi, K., and Kyoko, T. (2020). Establishment of a cultivation method for leaf lettuce (Lactuca sativa var. crispa) and komatsuna (Brassica rapa var. perviridis) with high zinc content for patients with zinc deficiency and evaluation of its effectiveness. J. Sci. Food Agric. 101, 3202–3207. doi: 10.1002/jsfa.10949
Azizi, I., Esmaielpour, B., and Fatemi, H. (2020). Effect of foliar application of selenium on morphological and physiological indices of savory (Satureja hortensis) under cadmium stress. Food Sci. Nutr. 8, 6539–6549. doi: 10.1002/fsn3.1943
Bae, N., Garciaviguera, C., and Moreno, D. (2014). Biotic elicitors effectively increase the glucosinolates content in Brassicaceae sprouts. J. Agric. Food Chem. 62, 1881–1889. doi: 10.1021/jf404876z
Baek, M., Choi, H., Solomon, T., Jeong, C., Lee, O., and Tilahun, S. (2021). Preharvest Methyl Jasmonate treatment increased the antioxidant activity and glucosinolate contents of hydroponically grown Pak Choi. Antioxidants 10:131. doi: 10.3390/antiox10010131
Bak, S., Tax, F., Feldmann, K., Galbraith, D., and Feyereisen, R. (2001). CYP83B1, a cytochrome P450 at the metabolic branch point in auxin and indole glucosinolate biosynthesis in Arabidopsis. Plant Cell 13, 101–111. doi: 10.1105/tpc.13.1.101
Bakhtiari, M., Bakhtiari, M., and Rasmann, S. (2018). Root JA Induction Modifies Glucosinolate Profiles and Increases Subsequent Aboveground Resistance to Herbivore Attack in Cardamine hirsuta. Front. Plant Sci. 9:1230. doi: 10.3389/fpls.2018.01230
Barco, B., and Clay, N. (2019). Evolution of glucosinolate diversity via whole-genome duplications, gene rearrangements, and substrate promiscuity. Annu. Rev. Plant Biol. 70, 585–604. doi: 10.1146/annurev-arplant-050718-100152
Barickman, T., Kopsell, D., and Sams, C. (2013). Selenium influences glucosinolate and isothiocyanates and increases sulfur uptake in arabidopsis thaliana and rapid-cycling Brassica oleracea. J. Agric. Food Chem. 61, 202–209. doi: 10.1021/jf3037227
Bekaert, M., Edger, P., Hudson, C., Pires, J., and Conant, G. (2012). Metabolic and evolutionary costs of herbivory defense: systems biology of glucosinolate synthesis. New Phytol. 196, 596–605. doi: 10.1111/j.1469-8137.2012.04302.x
Bhat, R., and Vyas, D. (2019). Myrosinase: insights on structural, catalytic, regulatory, and environmental interactions. Crit. Rev. Biotechnol. 39, 508–523. doi: 10.1080/07388551.2019.1576024
Bleecker, A., and Kende, H. (2000). Ethylene: a gaseous signal molecule in plants. Annu. Rev. Cell Dev. Biol. 16, 1–18. doi: 10.1146/annurev.cellbio.16.1.1
Bolouri-Moghaddam, M., Le, R., Xiang, L., Rolland, F., and Van, E. (2010). Sugar signalling and antioxidant network connections in plant cells. FEBS J. 277, 2022–2037. doi: 10.1111/j.1742-4658.2010.07633.x
Bruinsma, M., Da, M., Loon, J., and Dicke, M. (2007). Jasmonic acid-induced changes in Brassica oleracea affect oviposition preference of two specialist herbivores. J. Chem. Ecol. 33, 655–668. doi: 10.1007/s10886-006-9245-2
Cai, C., Yuan, W., Miao, H., Deng, M., Wang, M., Lin, J., et al. (2018). Functional Characterization of BoaMYB51s as central regulators of indole glucosinolate biosynthesis in Brassica oleracea var. alboglabra Bailey. Front. Plant Sci. 9:1599. doi: 10.3389/fpls.2018.01599
Carmen, L., Carmen, M., Cristina, G., and Micaela, C. (2008). Leaf water balance mediated by aquaporins under salt stress and associated glucosinolate synthesis in broccoli. Plant Sci. 174, 321–328. doi: 10.1016/j.plantsci.2007.11.012
Castillo, N., Pastor, V., Chávez, N., Arró, M., and Altabella, T. (2019). Inactivation of UDP-glucose sterol glucosyltransferases enhances Arabidopsis resistance to Botrytis cinerea. Front. Plant Sci. 10:1162. doi: 10.3389/fpls.2019.01162
Chen, L., Liu, L., Lu, B., Ma, T., and Li, C. (2020). Exogenous melatonin promotes seed germination and osmotic regulation under salt stress in cotton (Gossypium hirsutum L.). PLoS One 15:e0228241. doi: 10.1371/journal.pone.0228241
Chen, W., Karangwa, E., Yu, J., Xia, S., Feng, B., and Zhang, X. (2018). Effect of sodium chloride concentration on off-flavor removal correlated to glucosinolate degradation and red radish anthocyanin stability. J. Food Sci. Technol. 56, 937–950. doi: 10.1007/s13197-018-03559-8
Chen, Y., Fei, M., Wang, Y., Chen, S., and Yan, X. (2015). Proteomic investigation of glucosinolate systematically changes in Arabidopsis Rosette leaves to exogenous methyl jasmonate. Plant Biosyst. 149, 346–353. doi: 10.1080/11263504.2013.819044
Chen, Y., Wang, Y., Huang, J., Zheng, C., Cai, C., Wang, Q., et al. (2017). Salt and methyl jasmonate aggravate growth inhibition and senescence in Arabidopsis seedlings via the JA signaling pathway. Plant Sci. 261, 1–9. doi: 10.1016/j.plantsci.2017.05.005
Chiu, Y., Matak, K., and Ku, K. (2019). Methyl jasmonate treated broccoli: Impact on the production of glucosinolates and consumer preferences. Food Chem. 299:125099. doi: 10.1016/j.foodchem.2019.125099
Chiu, Y., Matak, K., and Ku, K. (2020). Methyl Jasmonate treatment of broccoli enhanced glucosinolate concentration, which was retained after boiling, steaming, or microwaving. Foods 9:758. doi: 10.3390/foods9060758
Chowdhury, M., Kiraga, S., Islam, M., Ali, M., Reza, M., Lee, W., et al. (2021). Effects of temperature, relative humidity, and carbon dioxide concentration on growth and glucosinolate content of kale grown in a plant factory. Foods 10:1524. doi: 10.3390/foods10071524
Chung, I., Rekha, K., Rajakumar, G., and Thiruvengadam, M. (2018). Influence of silver nanoparticles on the enhancement and transcriptional changes of glucosinolates and phenolic compounds in genetically transformed root cultures of Brassica rapa ssp. rapa. Bioprocess Biosyst. Eng. 41, 1665–1677. doi: 10.1007/s00449-018-1991-3
Cipollini, D., Enright, S., Traw, M., and Bergelson, J. (2010). Salicylic acid inhibits jasmonic acid-induced resistance of Arabidopsis thaliana to Spodoptera exigua. Mol. Ecol. 13, 1643–4653. doi: 10.1111/j.1365-294X.2004.02161.x
Cocetta, G., Mishra, S., Raffaelli, A., and Ferrante, A. (2018). Effect of heat root stress and high salinity on glucosinolates metabolism in wild rocket. J. Plant Physiol. 231, 261–270. doi: 10.1016/j.jplph.2018.10.003
Connolly, E., Sim, M., Travica, N., Marx, W., Beasy, G., Lynch, G., et al. (2021). Glucosinolates from cruciferous vegetables and their potential role in chronic disease: investigating the preclinical and clinical evidence. Front. Pharmacol. 12:767975. doi: 10.3389/fphar.2021.767975
Dall’Acqua, S., Ertani, A., Pilon-Smits, E., Fabrega-Prats, M., and Schiavon, M. (2019). Selenium biofortification differentially affects sulfur metabolism and accumulation of phytochemicals in Two Rocket Species (Eruca Sativa Mill. and Diplotaxis Tenuifolia) Grown in Hydroponics. Plants 8:68. doi: 10.3390/plants8030068
Durenne, B., Druart, P., Blondel, A., and Fauconnier, M. (2018). How cadmium affects the fitness and the glucosinolate content of oilseed rape plantlets. Environ. Exp. Bot. 155, 185–194. doi: 10.1016/j.envexpbot.2018.06.008
Erb, M., Meldau, S., and Howe, G. (2012). Role of phytohormones in insect-specific plant reactions. Trends Plant Sci. 17, 250–259. doi: 10.1016/j.tplants.2012.01.003
Fahey, J., Zalcmann, A., and Talalay, P. (2001). The chemical diversity and distribution of glucosinolates and isothiocyanates among plants. Phytochemistry 56, 5–51. doi: 10.1016/s0031-9422(00)00316-2
Fatemi, H., Carvajal, M., and Rios, J. (2020). Foliar Application of Zn Alleviates Salt Stress Symptoms of Pak Choi Plants by Activating Water Relations and Glucosinolate Synthesis. Agronomy 10:1528. doi: 10.3390/agronomy10101528
Folmer, F., Basavaraju, U., Jaspars, M., Hold, G., El-Omar, E., Dicato, M., et al. (2014). Anticancer effects of bioactive berry compounds. Phytochem. Rev. 13, 295–322. doi: 10.1007/s11101-013-9319-z
Fones, H., Preston, G., and Smith, J. (2019). Variation in defence strategies in the metal hyperaccumulator plant Noccaea caerulescens is indicative of synergies and trade-offs between forms of defence. R. Soc. Open Sci. 6:172418. doi: 10.1098/rsos.172418
Frerigmann, H., Berger, B., and Gigolashvili, T. (2014). bHLH05 is an interaction partner of MYB51 and a novel regulator of glucosinolate biosynthesis in Arabidopsis. Plant Physiol. 166, 349–369. doi: 10.1104/pp.114.240887
Fritz, V., Justen, V., Bode, A., Schuster, T., and Wang, M. (2010). Glucosinolate Enhancement in Cabbage Induced by Jasmonic Acid Application. Hortscience 45, 1188–1191. doi: 10.21273/hortsci.45.8.1188
Gao, H., Wu, X., Zorrilla, C., Vega, S., and Palta, J. (2020). Fractionating of calcium in tuber and leaf tissues explains the calcium deficiency symptoms in potato plant overexpressing CAX1. Front. Plant Sci. 10:1793. doi: 10.3389/fpls.2019.01793
Gao, M., He, R., Shi, R., Li, Y., Song, S., Zhang, Y., et al. (2021). Combination of Selenium and UVA radiation affects growth and phytochemicals of broccoli microgreens. Molecules 26:4646. doi: 10.3390/molecules26154646
Gielen, B., Vandermeiren, K., Horemans, N., D’Haese, D., Serneels, R., and Valcke, R. (2006). Chlorophyll a fluorescence imaging of ozone-stressed Brassica napus L. plants differing in glucosinolate concentrations. Plant Biol. 8, 698–705. doi: 10.1055/s-2006-924150
Gigolashvili, T., Engqvist, M., Yatusevich, R., Müller, C., and Flügge, U. (2008). HAG2/MYB76 and HAG3/MYB29 exert a specific and coordinated control on the regulation of aliphatic glucosinolate biosynthesis in Arabidopsis thaliana. New Phytol. 177, 627–642. doi: 10.1111/j.1469-8137.2007.02295.x
Gioia, F., Rosskof, E., Leonardi, C., and Giuffrida, F. (2018). Effects of application timing of saline irrigation water on broccoli production and quality. Agric. Water Manage. 203, 97–104. doi: 10.1002/jsfa.7900
Grubb, C., Zipp, B., Ludwig-Muller, J., Masuno, M., Molinski, T., and Abel, S. (2004). Arabidopsis glucosyltransferase UGT74B1 functions in glucosinolate biosynthesis and auxin homeostasis. Plant J. 40, 893–908. doi: 10.1111/j.1365-313X.2004.02261.x
Gudio, M., Blanco-Tourián, N., Arbona, V., Gómez-Cadenas, A., and Navarro-Garcia, F. (2018). Beta-lactam antibiotics Modify root architecture and indole glucosinolate metabolism in Arabidopsis thaliana. Plant Cell Physiol. 59, 2086–2098. doi: 10.1093/pcp/pcy128
Guo, J., Zhou, R., Ren, X., Jia, H., Hua, L., Xu, H., et al. (2018). Effects of salicylic acid, Epi-brassinolide and calcium on stress alleviation and Cd accumulation in tomato plants. Ecotoxicol. Environ. Saf. 157, 491–496. doi: 10.1016/j.ecoenv.2018.04.010
Guo, L., Zhu, Y., and Wang, F. (2018). Calcium sulfate treatment enhances bioactive compounds and antioxidant capacity in broccoli sprouts during growth and storage. Postharvest Biol. Technol. 139, 12–19. doi: 10.1016/j.postharvbio.2018.01.010
Guo, L., Wang, P., Gu, Z., Jin, X., and Yang, R. (2017). Proteomic analysis of broccoli sprouts by iTRAQ in response to jasmonic acid. J. Plant Physiol. 218, 16–25. doi: 10.1016/j.jplph.2017.07.003
Guo, R., Hou, Q., Yuan, G., Wang, Q., and Zhao, Y. (2014). Effect of 2, 4-epibrassinolide on main health-promoting compounds in broccoli sprouts. Food Sci. Technol. 58, 287–292. doi: 10.1016/j.lwt.2014.02.047
Guo, R., Shen, W., Qian, H., Zhang, M., Liu, L., and Wang, Q. (2013a). Jasmonic acid and glucose synergistically modulate the accumulation of glucosinolates in Arabidopsis thaliana. J. Exp. Bot. 64, 5707–5719. doi: 10.1093/jxb/ert348
Guo, R., Yuan, G., and Wang, Q. (2013b). Effect of NaCl treatments on glucosinolate metabolism in broccoli sprouts. J. Zhejiang Univ. Sci. B 14, 124–131. doi: 10.1631/jzus.B1200096
Guo, R., Yuan, G., and Wang, Q. (2011). Effect of sucrose and mannitol on the accumulation of health-promoting compounds and the activity of metabolic enzymes in broccoli sprouts. Sci. Hortic. 128, 159–165. doi: 10.1016/j.scienta.2011.01.014
Gyawali, S., Parkin, I., Steppuhn, H., Buchwaldt, M., Adhikari, B., Wood, R., et al. (2019). Seedling, early vegetative, and adult plant growth of oilseed rapes (Brassica napus L.) under saline stress. Can. J. Plant Sci. 99, 927–941. doi: 10.1139/cjps-2019-0023
Han, Y., Gharibeshghi, A., Mewis, I., Forster, N., Beck, W., and Ulrichs, C. (2020). Plant responses to ozone: Effects of different ozone exposure durations on plant growth and biochemical quality of Brassica campestris L. ssp. chinensis. Sci. Hortic. 262:108921. doi: 10.1016/j.scienta.2019.108921
Han, Y. J., Gharibeshghi, A., Mewis, I., Forster, N., Beck, W., and Ulrichs, C. (2021). Effect of different durations of moderate ozone exposure on secondary metabolites of Brassica campestris L. ssp. chinensis. J. Hortic. Sci. Biotechnol. 96, 110–120. doi: 10.1080/14620316.2020.1793692
Hanschen, F., and Rohn, S. (2021). Advanced research on glucosinolates in food products. Foods 10:3148. doi: 10.3390/foods10123148
Harus, S., Abdullah-Zawawi, M., Goh, H., and Mohamed-Hussein, Z. (2020). A comprehensive gene inventory for glucosinolate biosynthetic pathway in Arabidopsis thaliana. Food Chem. 68, 7281–7297. doi: 10.1021/acs.jafc.0c01916
Hassini, I., Baenas, N., Alcaraz, M., Boughanmi, N., and María, C. (2017a). Effects of seed priming, salinity and methyl jasmonate treatment on bioactive composition of Brassica oleracea var. capitata (white and red varieties) sprouts. J. Sci. Food Agric. 97, 2291–2299. doi: 10.1002/jsfa.8037
Hassini, I., Martinez-Ballesta, M., Boughanmi, N., Moreno, D., and Carvajal, M. (2017b). Improvement of broccoli sprouts (Brassica oleracea L. var. italica) growth and quality by KCl seed priming and methyl jasmonate under salinity stress. Sci. Hortic. 226, 141–151. doi: 10.1016/j.scienta.2017.08.030
Hayashi, S., Tanikawa, H., Kuramata, M., Abe, T., and Ishikawa, S. (2020). Domain exchange between Oryza sativa phytochelatin synthases reveals a region that determines responsiveness to arsenic and heavy metals. Biochem. Biophys. Res. Commun. 523, 548–553. doi: 10.1016/j.bbrc.2019.12.093
He, H., and He, L. (2020). Crosstalk between melatonin and nitric oxide in plant development and stress responses. Physiol. Plant. 170, 218–226. doi: 10.1111/ppl.13143
He, R., Gao, M., Shi, R., Song, S., and Liu, H. (2020). The Combination of Selenium and LED light quality affects growth and nutritional properties of broccoli sprouts. Molecules 25:4788. doi: 10.3390/molecules25204788
He, Y., Yang, Z., Tang, M., Yang, Q., Zhang, Y., and Liu, S. (2022). Enhancing canola breeding by editing a glucosinolate transporter gene lacking natural variation. Plant Physiol. [Epub ahead of print]. doi: 10.1093/plphys/kiac021
Henschel, J., Resende, J., Zeist, A., Paladinimoreira, A., and Gonalves, L. (2020). Salicylic acid treatments induce resistance to Tuta absoluta and Tetranychus urticae on tomato plants. Hortic. Bras. 38, 288–294. doi: 10.1590/s0102-053620200308
Hillwig, M., Chiozza, M., Casteel, C., Lau, S., Hohenstein, J., Hernandez, E., et al. (2016). Abscisic acid deficiency increases defence responses against Myzus persicae in Arabidopsis. Mol. Plant Pathol. 17, 225–235. doi: 10.1111/mpp.12274
Himanen, S., Nissinen, A., Auriola, S., Poppy, G., Stewart, C., Holopainen, J., et al. (2008). Constitutive and herbivore-inducible glucosinolate concentrations in oilseed rape (Brassica napus) leaves are not affected by Bt Cry1Ac insertion but change under elevated atmospheric CO2 and O3. Planta 227, 427–437. doi: 10.1007/s00425-007-0629-5
Howe, G., and Jander, G. (2008). Plant immunity to insect herbivores. Annu. Rev. Plant Biol. 59, 41–66. doi: 10.1146/annurev.arplant.59.032607.092825
Hu, K., and Zhu, Z. (2010). Effects of different concentrations of sodium chloride on plant growth and glucosinolate content and composition in pakchoi. Afr. J. Biotechnol. 9, 4428–4433.
Huang, W., Lu, Y., Chen, L., Sun, D., and An, Y. (2021). Impact of pesticide/fertilizer mixtures on the rhizosphere microbial community of field-grown sugarcane. 3 Biotech 11:210. doi: 10.1007/s13205-021-02770-3
Hwang, I., Park, B., Dang, Y., Kim, S., and Seo, H. (2019). Simultaneous direct determination of 15 glucosinolates in eight Brassica species by UHPLC-Q-Orbitrap-MS. Food Chem. 282, 127–133. doi: 10.1016/j.foodchem.2018.12.036
Iqbal, Z., Iqbal, M., Singh, S., and Buaboocha, T. (2020). Ca2+/Calmodulin Complex Triggers CAMTA transcriptional machinery under stress in plants: signaling cascade and molecular regulation. Front. Plant Sci. 11:598327. doi: 10.3389/fpls.2020.598327
Jahangir, M., Abdel-Farid, I., Choi, Y., and Verpoorte, R. (2008). Metal ion-inducing metabolite accumulation in Brassica rapa. J. Plant Physiol. 165, 1429–1437. doi: 10.1016/j.jplph.2008.04.011
Jakovljevic, T., Cvjetko, M., Sedak, M., Dokic, M., Bilandzic, N., Vorkapic-Furac, J., et al. (2013). Balance of glucosinolates content under Cd stress in two Brassica species. Plant Physiol. Biochem. 63, 99–106. doi: 10.1016/j.plaphy.2012.10.019
Jeon, B., Oh, M., Kim, H., Kim, E., and Chae, W. (2022). Glucosinolate variation among organs, growth stages and seasons suggests its dominant accumulation in sexual over asexual-reproductive organs in white radish. Sci. Hortic. 291:110617. doi: 10.1016/j.scienta.2021.110617
Jocsak, I., Malgwi, I., Rabnecz, G., Szeg, A., Varga-Visi, E., Vegvari, G., et al. (2020). Effect of cadmium stress on certain physiological parameters, antioxidative enzyme activities and biophoton emission of leaves in barley (Hordeum vulgare L.) seedlings. PLoS One 15:e0240470. doi: 10.1371/journal.pone.0240470
Kang, M., Choi, J., Kim, H., Kushad, M., Jeffery, E., and Juvik, J. (2013). Methyl Jasmonate and 1-methylcyclopropene treatment effects on quinone reductase inducing activity and post-harvest quality of Broccoli. PLoS One 8:e77127. doi: 10.1371/journal.pone.0077127
Kang, M., and Juvik, J. (2011). The effect of field Methyl Jasmonate treatments on glucosinolate and phenolic concentrations in broccoli florets. Hortscience S339–S339.
Kastell, A., Smetanska, I., Ulrichs, C., Cai, Z., and Mewis, I. (2013). Effects of Phytohormones and Jasmonic Acid on glucosinolate content in hairy root cultures of Sinapis alba and Brassica rapa. Appl. Biochem. Biotechnol. 169, 624–635. doi: 10.1007/s12010-012-0017-x
Keling, H., and Zhu, Z. (2013). Effects of different concentrations of naphthylacetic acid on glucosinolates contents in pakchoi (Brassica campestris l. ssp. chinensis makino var. communis). Plant Physiol. J. 48, 1221–1227.
Khaling, E., Papazian, S., Poelman, E., Holopainen, J., Albrectsen, B., and Blande, J. (2015). Ozone affects growth and development of Pieris brassicae on the wild host plant Brassica nigra. Envion. Pollut. 199, 119–129. doi: 10.1016/j.envpol.2015.01.019
Kim, D., Shim, J., Ko, M., Chung, S., Chowdhury, M., and Lee, W. (2018). Statistical modeling for estimating glucosinolate content in Chinese cabbage by growth conditions. J. Sci. Food Agric. 98, 3580–3587. doi: 10.1002/jsfa.8874
Kim, J., Bong, S., and Park, S. (2018). Effect of heavy metal treatment on glucosinolate biosynthesis in hairy root culture of watercress (Nasturtium officinale). Biosci. Res. 15, 303–309.
Kim, H., and Juvik, J. (2011). Effect of selenium fertilization and methyl jasmonate treatment on glucosinolate accumulation in broccoli florets. J. Am. Soc. Hortic. Sci. 136, 239–246. doi: 10.21273/jashs.136.4.239
Kim, H., Kwon, D., Bae, H., Kim, S., and Sang, U. (2013). Influence of auxins on glucosinolate biosynthesis in hairy root cultures of broccoli (Brassica oleracea var. italica). Asian J. Chem. 25, 6099–6101. doi: 10.14233/ajchem.2013.14266
Kim, H., Seomun, S., Yoon, Y., and Jang, G. (2021). Jasmonic acid in plant abiotic stress tolerance and interaction with abscisic acid. Agronomy 11:1886. doi: 10.3390/agronomy11091886
Kim, J., Zhang, X., Pascuzzi, P., Liu, C., and Chapple, C. (2020). Glucosinolate and phenylpropanoid biosynthesis are linked by proteasome-dependent degradation of PAL. New Phytol. 225, 154–168. doi: 10.1111/nph.16108
Klaiber, J., Dorn, S., and Najar-Rodriguez, A. (2013). Acclimation to Elevated CO2 increases constitutive glucosinolate levels of brassica plants and affects the performance of specialized herbivores from contrasting feeding guilds. J. Chem. Ecol. 39, 653–665. doi: 10.1007/s10886-013-0282-3
Kolbert, Z., Pet, A., Lehotai, N., Feigl, G., and Erdei, L. (2012). Long-term copper (Cu2+) exposure impacts on auxin, nitric oxide (NO) metabolism and morphology of Arabidopsis thaliana. Plant Growth Regul. 68, 151–159. doi: 10.1007/s10725-012-9701-7
Kruszka, D., Sawikowska, A., Selvakesavan, R., Krajewski, P., and Franklin, G. (2019). Silver nanoparticles affect phenolic and phytoalexin composition of Arabidopsis thaliana. Sci. Total Environ. 716:135361. doi: 10.1016/j.scitotenv.2019.135361
Ku, K., Becker, T., and Juvik, J. (2016). Transcriptome and metabolome analyses of glucosinolates in two broccoli cultivars following jasmonate treatment for the induction of Glucosinolate Defense to Trichoplusia ni (Hübner). Int. J. Mol. Sci. 17:1135. doi: 10.3390/ijms17071135
Ku, K., Jeffery, E., Juvik, J., and Ashida, H. (2014). Exogenous Methyl Jasmonate Treatment Increases Glucosinolate Biosynthesis and Quinone Reductase Activity in Kale Leaf Tissue. PLoS One 9:e103407. doi: 10.1371/journal.pone.0103407
Ku, K., and Juvik, J. (2012). Optimum methyl jasmonate application to enhance glucosinolate concentration in Broccoli Florets. Hortscience 47, S311–S312.
Kumari, S., Jo, J., Choi, H., Lee, J., Lee, S., Jeong, M., et al. (2019). Molecular characterization and expression analysis of MYB transcription factors involved in the glucosinolate pathway in Chinese cabbage (Brassica rapa ssp. pekinensis). Agronomy 9:807. doi: 10.3390/agronomy9120807
Kunierczyk, A., Winge, P., Jrstad, T., Troczyska, J., Rossiter, J., and Bones, A. (2008). Towards global understanding of plant defence against aphids – timing and dynamics of early Arabidopsis defence responses to cabbage aphid (Brevicoryne brassicae) attack. Plant Cell Environ. 31, 1097–1105. doi: 10.1111/j.1365-3040.2008.01823.x
Kurowska, M., Daszkowska-Golec, A., Gajecka, M., Koscielniak, P., Bierza, W., and Szarejko, I. (2020). Methyl jasmonate affects photosynthesis efficiency, expression of HvTIP genes and nitrogen homeostasis in barley. Int. J. Mol. Sci. 21:4335. doi: 10.3390/ijms21124335
Kusznierewicz, B., Baczek-Kwinta, R., Bartoszek, A., Piekarska, A., Huk, A., Manikowska, A., et al. (2012). The dose-dependent influence of zinc and cadmium contamination of soil on their uptake and glucosinolate content in white cabbage (Brassica oleracea var. capitata f. alba). Environ. Toxicol. Chem. 31, 2482–2489. doi: 10.1002/etc.1977
La, G., Fang, P., Teng, Y., Li, Y., and Lin, X. (2009). Effect of CO2 enrichment on the glucosinolate contents under different nitrogen levels in bolting stem of Chinese kale (Brassica alboglabra L.). J. Zhejiang Univ. 10, 454–464. doi: 10.1631/jzus.B0820354
Lee, J., Lee, J., Kim, H., Chae, W., Kim, S., Lim, Y., et al. (2018). Brassinosteroids regulate glucosinolate biosynthesis in Arabidopsis thaliana. Physiol. Plant. 163, 450–458. doi: 10.1111/ppl.12691
Lee, Y., Chen, M., Lee, J., Zhang, J., Lin, S., Fu, T., et al. (2019). Reactivation of PTEN tumor suppressor for cancer treatment through inhibition of a MYC-WWP1 inhibitory pathway. Science 364:eaau0159. doi: 10.1126/science.aau0159
Letchumanan, D., Sok, S., Ibrahim, S., Nagoor, N., and Arshad, N. (2021). Plant-Based Biosynthesis of Copper/copper oxide nanoparticles: an update on their applications in biomedicine, mechanisms, and toxicity. Biomolecules 11:564. doi: 10.3390/biom11040564
Li, X., Yang, H., Li, R., Chen, W., Liu, L., Liu, F., et al. (2018). Jasmonic Acid-Mediated Aliphatic Glucosinolate metabolism is involved in clubroot disease development in Brassica napus L. Front. Plant Sci. 9:750. doi: 10.3389/fpls.2018.00750
Li, Y., Li, R., Sawada, Y., Boerzhijin, S., and Hirai, M. (2021). Abscisic acid-mediated induction of FLAVIN-CONTAINING MONOOXYGENASE 2 leads to reduced accumulation of methylthioalkyl glucosinolates in Arabidopsis thaliana. Plant Sci. 303:110764. doi: 10.1016/j.plantsci.2020.110764
Lin, P., Di, H., Li, Z., Wang, Y., Zhou, W., Huang, S., et al. (2022). Light irradiation maintains the sensory quality, health-promoting phytochemicals, and antioxidant capacity of post-harvest baby mustard. J. Food Sci. 87, 112–123. doi: 10.1111/1750-3841.15980
Linic, I., Samec, D., Gruz, J., Bok, V., Strnad, M., and Salopek-Sondi, B. (2019). Involvement of phenolic acids in short-term adaptation to salinity stress is species-specific among Brassicaceae. Plants 8:155. doi: 10.3390/plants8060155
Liu, A., Juvik, J., Jeffery, E., Berman-Booty, L., Clinton, S., and Erdman, J. (2014). Enhancement of Broccoli Indole Glucosinolates by Methyl Jasmonate Treatment and Effects on Prostate Carcinogenesis. J. Med. Food 17, 1177–1182. doi: 10.1089/jmf.2013.0145
Liu, J., Fu, C., Li, G., Khan, M., and Wu, H. (2021). ROS homeostasis and plant salt tolerance: plant nanobiotechnology updates. Sustainability 13:3552. doi: 10.3390/su13063552
Maghsoudi, K., Arvin, M., and Ashraf, M. (2019). Mitigation of arsenic toxicity in wheat by the exogenously applied salicylic Acid, 24-Epi-Brassinolide and Silicon. J. Soil Sci. Plant Nutr. 20, 577–588. doi: 10.1007/s42729-019-00147-3
Mahn, A. (2017). Modelling of the effect of selenium fertilization on the content of bioactive compounds in broccoli heads. Food Chem. 233, 492–499. doi: 10.1016/j.foodchem.2017.04.144
Major, I., Yoshida, Y., Campos, M., Kapali, G., Xin, X. F., Sugimoto, K., et al. (2017). Regulation of growth-defense balance by the JASMONATE ZIM-DOMAIN (JAZ)-MYC transcriptional module. New Phytol. 215, 1533–1547. doi: 10.1111/nph.14638
Malka, S., and Cheng, Y. (2017). Possible interactions between the biosynthetic pathways of indole glucosinolate and auxin. Front. Plant Sci. 8:2131. doi: 10.3389/fpls.2017.02131
Mao, Y., Liu, Y., Chen, D., Chen, F., Fang, X., Hong, G., et al. (2018). Jasmonate response decay and defense metabolite accumulation contributes to age-regulated dynamics of plant insect resistance. Nat. Commun. 8:13925. doi: 10.1038/ncomms13925
Maodzeka, A., Wang, Q., Chen, X., Hussain, N., and Jiang, L. (2019). Effects of 5-aminolevulinic Acid on the Bioactive Compounds and Seedling Growth of Oilseed Rape (Brassica napus L.). J. Plant Biol. 62, 181–194. doi: 10.1007/s12374-018-0299-9
Maurya, V., Ranjan, V., Gothandam, K., and Pareek, S. (2019). Exogenous gibberellic acid treatment extends green chili shelf life and maintain quality under modified atmosphere packaging. Sci. Hortic. 269:108934. doi: 10.1016/j.scienta.2019.108934
Mckenzie, M., Matich, A., Hunter, D., Esfandiari, A., and Trolove, S. (2019). Selenium Application During Radish (Raphanus sativus) Plant Development Alters Glucosinolate Metabolic Gene Expression and Results in the Production of 4-(methylseleno)but-3-enyl glucosinolate. Plants 8:427.
Medvedev, S. (2018). Principles of calcium signal generation and transduction in plant cells. Russ. J. Plant Physiol. 65, 771–783. doi: 10.1146/annurev-arplant-042110-103745
Meier, K., Ehbrecht, M., and Wittstock, U. (2019). Glucosinolate content in dormant and germinating Arabidopsis thaliana seeds is affected by non-functional alleles of classical Myrosinase and Nitrile-Specifier Protein Genes. Front. Plant Sci. 10:1549. doi: 10.3389/fpls.2019.01549
Miao, H., Cai, C., Wei, J., Huang, J., Chang, J., Qian, H., et al. (2016). Glucose enhances indolic glucosinolate biosynthesis without reducing primary sulfur assimilation. Sci. Rep. 6:31854. doi: 10.1038/srep31854
Miao, H., Wang, M., Chang, J., Tao, H., Sun, B., and Wang, Q. (2017). Effects of glucose and gibberellic acid on glucosinolate content and antioxidant properties of Chinese kale sprouts. J. Zhejiang Univ. Sci. B 18, 1093–1100. doi: 10.1631/jzus.B1700308
Miao, H., Wei, J., Zhao, Y., Yan, H., Sun, B., Huang, J., et al. (2013). Glucose signalling positively regulates aliphatic glucosinolate biosynthesis. J. Exp. Bot. 64, 1097–1109. doi: 10.1093/jxb/ers399
Miao, H., Zeng, W., Zhao, M., Wang, J., and Wang, Q. (2020). Effect of melatonin treatment on visual quality and health-promoting properties of broccoli florets under room temperature. Food Chem. 319:126498. doi: 10.1016/j.foodchem.2020.126498
Miret, J., Munné-Bosch, S., and Dijkwel, P. (2017). ABA signalling manipulation suppresses senescence of a leafy vegetable stored at room temperature. Plant Biotechnol. J. 16, 530–544. doi: 10.1111/pbi.12793
Mitreiter, S., and Gigolashvili, T. (2021). Regulation of glucosinolate biosynthesis. J. Exp. Bot. 72, 70–91. doi: 10.1093/jxb/eraa479
Mukherjee, S., Chakraborty, A., Mondal, S., Saha, S., Haque, A., and Paul, S. (2019). Assessment of common plant parameters as biomarkers of air pollution. Environ. Monit. Assess. 191:400. doi: 10.1007/s10661-019-7540-y
Neequaye, M., Stavnstrup, S., Harwood, W., Lawrenson, T., Hundleby, P., Irwin, J., et al. (2021). CRISPR-Cas9-Mediated Gene Editing of MYB28 Genes Impair Glucorarphanin Accumulation of Brassica oleracea in the Field. CRISPR J. 4, 416–426. doi: 10.1089/crispr.2021.0007
Nilsson, J., Olsson, K., Engqvist, G., Ekvall, M., and Nyman, A. (2006). Variation in the content of glucosinolates, hydroxycinnamic acids, carotenoids, total antioxidant capacity and low-molecular-weight carbohydrates in Brassica vegetables. J. Sci. Food Agric. 86, 528–538. doi: 10.1002/jsfa.2355
Pandey, C., Augustine, R., Panthri, M., Zia, I., and Gupta, M. (2016). Arsenic affects the production of glucosinolate, thiol and phytochemical compounds: a comparison of two Brassica cultivars. Plant Physiol. Biochem. 111, 144–154. doi: 10.1016/j.plaphy.2016.11.026
Pangesti, N., Reichelt, M., Mortel, J., Kapsomenou, E., Gershenzon, J., Joop, J., et al. (2016). Jasmonic acid and ethylene signaling pathways regulate glucosinolate levels in plants during rhizobacteria-induced systemic resistance against a leaf-chewing herbivore. J. Chem. Ecol. 42, 1212–1225. doi: 10.1007/s10886-016-0787-7
Paudel, J., Amirizian, A., Krosse, S., Giddings, J., Ismail, S., Xia, J., et al. (2016). Effect of atmospheric carbon dioxide levels and nitrate fertilization on glucosinolate biosynthesis in mechanically damaged Arabidopsis plants. BMC Plant Biol. 16:68. doi: 10.1186/s12870-016-0752-1
Pedreno, A., Sanchez-Pujante, J., and Borja-Martinez, A. (2017). Biosynthesis and bioactivity of glucosinolates and their production in plant in vitro cultures. Planta 246, 19–32. doi: 10.1007/s00425-017-2705-9
Penas, E., Martinez-Villaluenga, C., Frias, J., Sanchez-Martinez, M., Perez-Corona, M., Madrid, Y., et al. (2012). Se improves indole glucosinolate hydrolysis products content, Se-methylselenocysteine content, antioxidant capacity and potential anti-inflammatory properties of sauerkraut. Food Chem. 132, 907–914. doi: 10.1016/j.foodchem.2011.11.064
Peskan-Berghofer, T., Vilches-Barro, A., Muller, T., Glawischnig, E., Reichelt, M., Gershenzon, J., et al. (2015). Sustained exposure to abscisic acid enhances the colonization potential of the mutualist fungus Piriformospora indica on Arabidopsis thaliana roots. New Phytol. 208, 873–886. doi: 10.1111/nph.13504
Petersen, A., Hansen, L., Mirza, N., Crocoll, C., Mirza, O., and Halkier, B. (2019). Changing substrate specificity and iteration of amino acid chain elongation in glucosinolate biosynthesis through targeted mutagenesis of Arabidopsis methylthioalkylmalate synthase 1. Biosci. Rep. 39:BSR20190446. doi: 10.1042/BSR20190446
Petretto, G., Urgeghe, P., Massa, D., and Melito, S. (2019). Effect of salinity (NaCl) on plant growth, nutrient content, and glucosinolate hydrolysis products trends in rocket genotypes. Plant Physiol. Biochem. 141, 30–39. doi: 10.1016/j.plaphy.2019.05.012
Pilarska, M., Wiciarz, M., Jajic, I., Kozieradzka-Kiszkurno, M., Dobrev, P., Vankova, R., et al. (2016). A Different Pattern of Production and Scavenging of Reactive Oxygen Species in Halophytic Eutrema salsugineum (Thellungiella salsuginea) Plants in Comparison to Arabidopsis thaliana and Its Relation to Salt Stress Signaling. Front. Plant Sci. 7:1179. doi: 10.3389/fpls.2016.01179
Rao, S., Gou, Y., Yu, T., Cong, X., and Xu, F. (2021). Effects of selenate on Se, flavonoid, and glucosinolate in broccoli florets by combined transcriptome and metabolome analyses. Food Res. Int. 146:110463. doi: 10.1016/j.foodres.2021.110463
Reddy, G., Tossavainen, P., Nerg, A., and Holopainen, J. (2004). Elevated Atmospheric CO2 affects the chemical quality of Brassica plants and the growth rate of the specialist, Plutella xylostella, but Not the Generalist, Spodoptera littoralis. J. Agric. Food Chem. 52, 4185–4191. doi: 10.1021/jf049358v
Reich, M., Aghajanzadeh, T., Helm, J., Parmar, S., Hawkesford, M., and De Kok, L. (2017). Chloride and sulfate salinity differently affect biomass, mineral nutrient composition and expression of sulfate transport and assimilation genes in Brassica rapa. Plant Soil 411, 319–332. doi: 10.1007/s11104-016-3026-7
Rhee, J., Choi, S., Lee, J., Hur, O., and Assefa, A. (2020). Glucosinolate content in Brassica genetic resources and their distribution pattern within and between inner, middle, and outer leaves. Plants 9:1421. doi: 10.3390/plants9111421
Rios, J., Agudelo, A., Moreno, D., and Carvajal, M. (2020). Growing broccoli under salinity: the influence of cultivar and season on glucosinolates content. Sci. Agric. 77:e20190028.
Rios, J., Pascual, J., Guillen, M., Lopez-Martinez, A., and Carvajal, M. (2021). Influence of foliar Methyl-jasmonate biostimulation on exudation of glucosinolates and their effect on root pathogens of broccoli plants under salinity condition. Sci. Hortic. 282:110027. doi: 10.1016/j.scienta.2021.110027
Rivera-Martin, A., Reynolds-Marzal, D., Martin, A., Velazquez, R., and Poblaciones, M. (2021). Combined Foliar Zinc and Nitrogen Application in Broccoli (Brassica oleracea var. italica L.): effects on Growth, Nutrient Bioaccumulation, and Bioactive Compounds. Agronomy 11:548. doi: 10.3390/agronomy11030548
Robert, C., Pellissier, L., Moreira, X., Defossez, E., and Rasmann, S. (2019). Correlated induction of phytohormones and glucosinolates shapes insect herbivore resistance of cardamine species along elevational gradients. J. Chem. Ecol. 45, 638–648. doi: 10.1007/s10886-019-01084-2
Rodriguez-Hernandez, M., Moreno, D., Carvajal, M., and Martinez-Ballesta, M. (2014). Genotype Influences Sulfur Metabolism in Broccoli (Brassica oleracea L.) Under Elevated CO2 and NaCl Stress. Plant Cell Physiol. 55, 2047–2059. doi: 10.1093/pcp/pcu130
Rolland, F., Moore, B., and Sheen, J. (2002). Sugar sensing and signaling in plants. Plant Cell 14(Suppl.), S185–S205.
Roser, T., Pongrac, P., Poschenrieder, C., Katarina, V., Regvar, M., and Juan, B. (2006). Distinctive effects of cadmium on glucosinolate profiles in Cd hyperaccumulator Thlaspi praecox and non-hyperaccumulator Thlaspi arvense. Plant Soil 288, 333–341. doi: 10.1007/s11104-006-9124-1
Salehin, M., Li, B., Tang, M., Katz, E., Song, L., Ecker, J., et al. (2019). Auxin-sensitive Aux/IAA proteins mediate drought tolerance in Arabidopsis by regulating glucosinolate levels. Nat. Commun. 10:4021. doi: 10.1038/s41467-019-12002-1
Samanta, S., Banerjee, A., and Roychoudhury, A. (2021). Exogenous melatonin regulates endogenous phytohormone homeostasis and thiol-mediated detoxification in two indica rice cultivars under arsenic stress. Plant Cell Rep. 40, 1585–1602. doi: 10.1007/s00299-021-02711-7
Samec, D., Lini, I., and Salopek-Sondi, B. (2021). Salinity stress as an elicitor for phytochemicals and minerals accumulation in selected leafy vegetables of Brassicaceae. Agronomy 11:361. doi: 10.3390/agronomy11020361
Sánchez-Pujante, P., Gionfriddo, M., Sabater-Jara, A., Almagro, L., and Diaz-Vivancos, P. (2020). Enhanced bioactive compound production in broccoli cells due to coronatine and methyl jasmonate is linked to antioxidative metabolism. J. Plant Physiol. 248:153136. doi: 10.1016/j.jplph.2020.153136
Sarker, U., Islam, M., and Oba, S. (2018). Salinity stress accelerates nutrients, dietary fiber, minerals, phytochemicals and antioxidant activity in Amaranthus tricolor leaves. PLoS One 13:e0206388. doi: 10.1371/journal.pone.0206388
Sarker, U., and Oba, S. (2018e). Response of nutrients, minerals, antioxidant leaf pigments, vitamins, polyphenol, flavonoid and antioxidant activity in selected vegetable amaranth under four soil water content. Food Chem. 252, 72–83. doi: 10.1016/j.foodchem.2018.01.097
Sarker, U., and Oba, S. (2018d). Drought stress enhances nutritional and bioactive compounds, phenolic acids and antioxidant capacity of Amaranthus leafy vegetable. BMC Plant Biol. 18:258. doi: 10.1186/s12870-018-1484-1
Sarker, U., and Oba, S. (2018b). Catalase, superoxide dismutase and ascorbate-glutathione cycle enzymes confer drought tolerance of Amaranthus tricolor. Sci. Rep. 8:16496. doi: 10.1038/s41598-018-34944-0
Sarker, U., and Oba, S. (2018c). Drought stress effects on growth, ros markers, compatible solutes, phenolics, flavonoids, and antioxidant activity in Amaranthus tricolor. Appl. Biochem. Biotechnol. 186, 999–1016. doi: 10.1007/s12010-018-2784-5
Sarker, U., and Oba, S. (2018a). Augmentation of leaf color parameters, pigments, vitamins, phenolic acids, flavonoids antioxidant activity in selected Amaranthus tricolor under salinity stress. Sci. Rep. 8:12349. doi: 10.1038/s41598-018-30897-6
Sarker, U., and Oba, S. (2019). Salinity stress enhances color parameters, bioactive leaf pigments, vitamins, polyphenols, flavonoids and antioxidant activity in selected Amaranthus leafy vegetables. J. Sci. Food Agric. 99, 2275–2284. doi: 10.1002/jsfa.9423
Sarker, U., and Oba, S. (2020). The response of salinity stress- induced A. tricolor to growth, anatomy, physiology, non-enzymatic and enzymatic antioxidants. Front. Plant Sci. 11:559876. doi: 10.3389/fpls.2020.559876
Schonhof, I., Klaring, H., Krumbein, A., and Schreiner, M. (2007). Interaction between atmospheric CO2 and glucosinolates in broccoli. J. Chem. Ecol. 33, 105–114. doi: 10.1007/s10886-006-9202-0
Schreiner, M., Krumbein, A., Knorr, D., and Smetanska, I. (2011). Enhanced glucosinolates in root exudates of Brassica rapa ssp. rapa mediated by salicylic acid and methyl jasmonate. J. Agric. Food Chem. 59, 1400–1405. doi: 10.1021/jf103585s
Sepuveda, I., Barrientos, H., Mahn, A., and Moenne, A. (2013). Changes in SeMSC, glucosinolates and sulforaphane levels, and in proteome profile in Broccoli (Brassica oleracea var. Italica) Fertilized with Sodium Selenate. Molecules 18, 5221–5234. doi: 10.3390/molecules18055221
Sewelam, N., Jaspert, N., Katrien, V., Tognetti, V., Schmitz, J., Frerigmann, H., et al. (2014). Spatial H2O2 signaling specificity: H2O2 from chloroplasts and peroxisomes modulates the plant transcriptome differentially. Mol. Ecol. 7, 1191–1210. doi: 10.1093/mp/ssu070
Shin, J., Lim, E., Cho, Y., and Nho, C. (2021). Cancer-preventive effect of phenethyl isothiocyanate through tumor microenvironment regulation in a colorectal cancer stem cell xenograft model. Phytomedicine 84:153493. doi: 10.1016/j.phymed.2021.153493
Smeekens, S., Ma, J., Hanson, J., and Rolland, F. (2010). Sugar signals and molecular networks controlling plant growth. Curr. Opin. Plant Biol. 13, 274–279. doi: 10.1016/j.pbi.2009.12.002
Sonderby, I., Burow, M., Rowe, H., Kliebenstein, D., and Halkier, B. (2010a). A complex interplay of Three R2R3 MYB transcription factors determines the profile of aliphatic glucosinolates in Arabidopsis. Plant Physiol. 153, 348–363. doi: 10.1104/pp.109.149286
Sonderby, I., Geu-Flores, F., and Halkier, B. (2010b). Biosynthesis of glucosinolates gene discovery and beyond. Trends Plant Sci. 15, 283–290. doi: 10.1016/j.tplants.2010.02.005
Stratmann, J. (2003). Long distance run in the wound response–jasmonic acid is pulling ahead. Trends Plant Sci. 8, 247–250. doi: 10.1016/S1360-1385(03)00106-7
Sun, B., Yan, H., Zhang, F., and Wang, Q. (2012b). Effects of plant hormones on main health-promoting compounds and antioxidant capacity of Chinese kale. Food Res. Int. 48, 359–366. doi: 10.1016/j.foodres.2012.04.021
Sun, B., Yan, H., Liu, N., Wei, J., and Wang, Q. (2012a). Effect of 1-MCP treatment on postharvest quality characters, antioxidants and glucosinolates of Chinese kale. Food Chem. 131, 519–526. doi: 10.1016/j.foodchem.2011.09.016
Sun, J., Kou, L., Geng, P., Huang, H., Yang, T., Luo, Y., et al. (2015). Metabolomic assessment reveals an elevated level of glucosinolate content in CaCl2 Treated Broccoli Microgreens. J. Agric. Food Chem. 63, 1863–1868. doi: 10.1021/jf504710r
Sun, T., and Zhang, Y. (2021). Short- and long-distance signaling in plant defense. Plant J. 105, 505–517. doi: 10.1111/tpj.15068
Sun, X., Zhang, J., Zhang, H., Zhang, Q., Ni, Y., Chen, J., et al. (2009). Glucosinolate profiles of Arabidopsis thaliana in response to cadmium exposure. Water Air Soil Pollut. 200, 109–117. doi: 10.1007/s11270-008-9897-3
Sun, Y., Park, J., Kim, E., Lim, S., and Chu, W. (2018). Exposure of kale root to NaCl and Na2SeO3 increases isothiocyanate levels and Nrf2 signalling without reducing plant root growth. Sci. Rep. 8:3999. doi: 10.1038/s41598-018-22411-9
SzydłOwska-Czerniak, A., Bartkowiak-Broda, I., Karlovi, I., Karlovits, G., and SzłYk, E. (2011). Antioxidant capacity, total phenolics, glucosinolates and colour parameters of rapeseed cultivars. Food Chem. 127, 556–563. doi: 10.1016/j.foodchem.2011.01.040
Teng, Z., Yu, Y., Zhu, Z., Hong, S., Yang, B., and Zang, Y. (2021). Melatonin elevated Sclerotinia sclerotiorum resistance via modulation of ATP and glucosinolate biosynthesis in Brassica rapa ssp. pekinensis. J. Proteomics 243:104264. doi: 10.1016/j.jprot.2021.104264
Thiruvengadam, M., Kim, S., and Chung, I. (2016b). Influence of amphetamine, γ-aminobutyric acid, and fosmidomycin on metabolic, transcriptional variations and determination of their biological activities in turnip (Brassica rapa ssp. rapa). S. Afr. J. Bot. 103, 181–192. doi: 10.1016/j.sajb.2015.08.021
Thiruvengadam, M., Baskar, V., Kim, S., and Chung, I. (2016a). Effects of abscisic acid, jasmonic acid and salicylic acid on the content of phytochemicals and their gene expression profiles and biological activity in turnip (Brassica rapa ssp. rapa). Plant Growth Regul. 80, 377–390. doi: 10.1007/s10725-016-0178-7
Thiruvengadam, M., and Chung, I. (2015). Selenium, putrescine, and cadmium influence health-promoting phytochemicals and molecular-level effects on turnip (Brassica rapa ssp rapa). Food Chem. 173, 185–193. doi: 10.1016/j.foodchem.2014.10.012
Thiruvengadam, M., Kim, S., and Chung, I. (2015b). Exogenous phytohormones increase the accumulation of health-promoting metabolites, and influence the expression patterns of biosynthesis related genes and biological activity in Chinese cabbage (Brassica rapa spp. pekinensis). Sci. Hortic. 193, 136–146. doi: 10.1016/j.scienta.2015.07.007
Thiruvengadam, M., Gurunathan, S., and Chung, I. (2015a). Physiological, metabolic, and transcriptional effects of biologically-synthesized silver nanoparticles in turnip (Brassica rapa ssp. rapa L.). Protoplasma 252, 1031–1046. doi: 10.1007/s00709-014-0738-5
Thompson, L., and Darwish, W. (2019). Environmental chemical contaminants in food: review of a global problem. J. Toxicol. 2019:2345283. doi: 10.1155/2019/2345283
Tian, M., Xu, X., Liu, Y., Xie, L., and Pan, S. (2016). Effect of Se treatment on glucosinolate metabolism and health-promoting compounds in the broccoli sprouts of three cultivars. Food Chem. 190, 374–380. doi: 10.1016/j.foodchem.2015.05.098
Tian, P., Lu, X., Bao, J., Zhang, X., Lu, Y., Zhang, X., et al. (2021). Transcriptomics analysis of genes induced by melatonin related to glucosinolates synthesis in broccoli hairy roots. Plant Singal. Behav. 16:1952742. doi: 10.1080/15592324.2021.1952742
Toler, H., Charron, C., Sams, C., and Randle, W. (2007). Selenium increases sulfur uptake and regulates glucosinolate metabolism in rapid-cycling Brassica oleracea. J. Am. Soc. Hortic. Sci. 132, 14–19. doi: 10.21273/jashs.132.1.14
Traw, M., Kim, J., Enright, S., Cipollini, D., and Bergelson, J. (2003). Negative cross-talk between salicylate- and jasmonate-mediated pathways in the Wassilewskija ecotype of Arabidopsis thaliana. Mol. Ecol. 12, 1125–1135. doi: 10.1046/j.1365-294x.2003.01815.x
Trejo-Tellez, L., Estrada-Ortiz, E., Gomez-Merino, F., Becker, C., Krumbein, A., and Schwarz, D. (2019). Flavonoid, nitrate and glucosinolate concentrations in Brassica species are differentially affected by photosynthetically active radiation, phosphate and phosphite. Front. Plant Sci. 10:371. doi: 10.3389/fpls.2019.00371
Vahid, T., and Soheil, K. (2019). Methyl jasmonate enhances salt tolerance of almond rootstocks by regulating endogenous phytohormones, antioxidant activity and gas-exchange. J. Plant Physiol. 234, 98–105. doi: 10.1016/j.jplph.2019.02.001
Vo, Q., Rochfort, S., Nam, P., Nguyen, T., Nguyen, T., and Mechler, A. (2018). Synthesis of aromatic and indole alpha-glucosinolates. Carbohydr. Res. 455, 45–53. doi: 10.1016/j.carres.2017.11.004
Wang, M., Cai, C., Lin, J., Tao, H., and Wang, Q. (2020). Combined treatment of epi-brassinolide and NaCl enhances the main phytochemicals in Chinese kale sprouts. Food Chem. 315:126275. doi: 10.1016/j.foodchem.2020.126275
Wang, Z., Yang, R., Guo, L., Fang, M., Zhou, Y., and Gu, Z. (2015). Effects of abscisic acid on glucosinolate content, isothiocyanate formation and myrosinase activity in cabbage sprouts. Int. J. Food Sci. Technol. 50, 1839–1846. doi: 10.1111/ijfs.12848
Wasternack, C., and Strnad, M. (2019). Jasmonates are signals in the biosynthesis of secondary metabolites - Pathways, transcription factors and applied aspects - A brief review. N. Biotechnol. 48, 1–11. doi: 10.1016/j.nbt.2017.09.007
Wei, A., Liu, C., Zheng, H., and Zheng, L. (2019). Melatonin treatment affects the glucoraphanin-sulforaphane system in postharvest fresh-cut broccoli (Brassica oleracea L.). Food Chem. 307:125562. doi: 10.1016/j.foodchem.2019.125562
Wei, J., Miao, H., and Wang, Q. (2011). Effect of glucose on glucosinolates, antioxidants and metabolic enzymes in Brassica sprouts. Sci. Hortic. 129, 535–540. doi: 10.1016/j.scienta.2011.04.026
Wiesner, M., Zrenner, R., Krumbein, A., Glatt, H., and Schreiner, M. (2013b). Genotypic Variation of the Glucosinolate Profile in Pak Choi (Brassica rapa ssp chinensis). J. Agric. Food Chem. 61, 1943–1953. doi: 10.1021/jf303970k
Wiesner, M., Hanschen, F., Schreiner, M., Glatt, H., and Zrenner, R. (2013a). Induced Production of 1-Methoxy-indol-3-ylmethyl Glucosinolate by Jasmonic Acid and Methyl Jasmonate in Sprouts and Leaves of Pak Choi (Brassica rapa ssp. chinensis). Int. J. Mol. Sci. 14, 14996–15016. doi: 10.3390/ijms140714996
Wiesner-Reinhold, M., Nickel, M., Graefe, J., Schreiner, M., and Hanschen, F. (2021). CO2 treatment increases glucosinolate hydrolysis products in two Arabidopsis thaliana accessions. J. Appl. Bot. Food Qual. 94, 16–25.
Xiao, Y., Yang, L., and Li, J. (2020). Progress on the biosynthesis and signal transduction of phytohormone salicylic acid. Hereditas 42, 858–869. doi: 10.16288/j.yczz.20-173
Xie, Q., Essemine, J., Pang, X., Chen, H., and Cai, W. (2020). Exogenous application of abscisic acid to shoots promotes primary root cell division and elongation. Plant Sci. 292:110385. doi: 10.1016/j.plantsci.2019.110385
Xu, D., Zuo, J., Fang, Y., Yan, Z., and Jiang, A. (2020). Effect of folic acid on the postharvest physiology of broccoli during storage. Food Chem. 339:127981. doi: 10.1016/j.foodchem.2020.127981
Xu, F., Chen, X., Yang, Z., Jin, P., Wang, K., Shang, H., et al. (2013). Maintaining quality and bioactive compounds of broccoli by combined treatment with 1-methylcyclopropene and 6-benzylaminopurine. J. Sci. Food Agric. 93, 1156–1161. doi: 10.1002/jsfa.5867
Xu, F., Tang, Y., Dong, S., Shao, X., Wang, H., Zheng, Y., et al. (2016). Reducing yellowing and enhancing antioxidant capacity of broccoli in storage by sucrose treatment. Postharvest Biol. Technol. 112, 39–45. doi: 10.1016/j.postharvbio.2015.09.038
Xu, F., Yang, Z., Chen, X., Jin, P., Wang, X., and Zheng, Y. (2011). 6-Benzylaminopurine delays senescence and enhances health-promoting compounds of Harvested Broccoli. J. Agric. Food Chem. 60, 234–240. doi: 10.1021/jf2040884
Xu, L., Wang, Y., Liu, W., Wang, J., Zhu, X., Zhang, K., et al. (2015). De novo sequencing of root transcriptome reveals complex cadmium-responsive regulatory networks in radish (Raphanus sativus L.). Plant Sci. 236, 313–323. doi: 10.1016/j.plantsci.2015.04.015
Yang, J., Wang, J., Li, Z., Li, X., He, Z., Zhang, L., et al. (2021). Genomic signatures of vegetable and oilseed allopolyploid Brassica juncea and genetic loci controlling the accumulation of glucosinolates. Plant Biotechnol. J. 19, 2619–2628. doi: 10.1111/pbi.13687
Yang, R., Guo, L., Zhou, Y., Shen, C., and Gu, Z. (2015b). Calcium mitigates the stress caused by ZnSO4 as a sulphur fertilizer and enhances the sulforaphane formation of broccoli sprouts. RSC Adv. 5, 12563–12570. doi: 10.1039/c4ra11371c
Yang, R., Guo, L., Jin, X., Shen, C., Zhou, Y., and Gu, Z. (2015a). Enhancement of glucosinolate and sulforaphane formation of broccoli sprouts by zinc sulphate via its stress effect. J. Funct. Foods 13, 345–349. doi: 10.1016/j.jff.2015.01.007
Yang, R., Hui, Q., Gu, Z., Zhou, Y., Guo, L., Shen, C., et al. (2016). Effects of CaCl2 on the metabolism of glucosinolates and the formation of isothiocyanates as well as the antioxidant capacity of broccoli sprouts. J. Funct. Foods 24, 156–163. doi: 10.1016/j.jff.2016.04.007
Yao, Q., Peng, Z., Tong, H., Yang, F., and Su, Q. (2019). Tomato plant flavonoids increase whitefly resistance and reduce spread of Tomato yellow leaf curl virus. J. Econ. Entomol. 112, 2790–2796. doi: 10.1093/jee/toz199
Yi, G., Arif, R., Yang, K., Jong-In, P., Byung, H., and Ill-Sup, N. (2016). Exogenous Methyl Jasmonate and Salicylic Acid Induce Subspecies-Specific Patterns of Glucosinolate accumulation and gene expression in Brassica oleracea L. Molecules 21:1417. doi: 10.3390/molecules21101417
Yin, N., Mu, L., Liang, Y., Hao, W., and An, X. (2020). Effects of foliar selenium fertilizer on fruit yield, quality and selenium content of three varieties of Vitis vinifera. J. Appl. Ecol. 31, 953–958. doi: 10.13287/j.1001-9332.202003.007
Yoshioka, K., and Moeder, W. (2020). Calcium channel in plants helps shut the door on intruders. Nature 585, 507–508. doi: 10.1038/d41586-020-02504-0
You, J., and Chan, Z. (2015). ROS regulation during abiotic stress responses in crop plants. Front. Plant Sci. 6:1092. doi: 10.3389/fpls.2015.01092
Yuan, G., Bo, S., Yuan, J., and Wang, Q. (2010). Effect of 1-methylcyclopropene on shelf life, visual quality, antioxidant enzymes and health-promoting compounds in broccoli florets. Food Chem. 118, 774–781. doi: 10.1016/j.foodchem.2009.05.062
Yu-Chun, C., John, J., and Kang-Mo, K. (2018). Targeted Metabolomic and Transcriptomic Analyses of “Red Russian” Kale (Brassicae napus var. pabularia) Following Methyl Jasmonate Treatment and Larval Infestation by the Cabbage Looper (Trichoplusia ni Hübner). Int. J. Mol. Sci. 19:1058. doi: 10.3390/ijms19041058
Yusuf, M., Almehrzi, A., Alnajjar, A., Alam, P., Elsayed, N., Khalil, R., et al. (2021). Glucose modulates copper induced changes in photosynthesis, ion uptake, antioxidants and proline in Cucumis sativus plants. Carbohydr. Res. 501:108271. doi: 10.1016/j.carres.2021.108271
Zang, Y., Ge, J., Huang, L., Gao, F., Lv, X., Zheng, W., et al. (2015a). Leaf and root glucosinolate profiles of Chinese cabbage (Brassica rapa ssp. pekinensis) as a systemic response to methyl jasmonate and salicylic acid elicitation. J. Zhejiang Univ. 16, 696–708. doi: 10.1631/jzus.B1400370
Zang, Y., Zhang, H., Huang, L., Wang, F., Gao, F., Lv, X., et al. (2015b). Glucosinolate enhancement in leaves and roots of pak choi (Brassica rapa ssp chinensis) by methyl jasmonate. Hortic. Environ. Biotechnol. 56, 830–840. doi: 10.1007/s13580-015-0079-0
Zhang, C., Jiang, H., Gu, S., and Zhou, X. (2019). Combination analysis of the physiology and transcriptome provides insights into the mechanism of silver nanoparticles phytotoxicity. Environ. Pollut. 252, 1539–1549. doi: 10.1016/j.envpol.2019.06.032
Zhang, J., and Yang, H. (2021). Metabolism and detoxification of pesticides in plants. Sci. Total Environ. 790:148034. doi: 10.1016/j.scitotenv.2021.148034
Zhang, L., Xu, B., Wu, T., Wen, X., Fan, L., Feng, Z., et al. (2017). Transcriptomic analysis of Pak Choi under acute ozone exposure revealed regulatory mechanism against ozone stress. BMC Plant Biol. 17:236. doi: 10.1186/s12870-017-1202-4
Zhang, L., Yang, S., Xu, J., Liu, T., and Shao, M. (2020). Application of exogenous salicylic acid on improving high temperature resistance of Nannochloropsis oceanica. Aquac. Int. 28, 2235–2246. doi: 10.1007/s10499-020-00592-3
Zhi, X., Wei, Z., Stanley, B. A., and Assmanna, S. M. (2008). Functional proteomics of Arabidopsis thaliana guard cells uncovers new stomatal signaling pathways. Plant Cell 20, 3210–3226. doi: 10.1105/tpc.108.063263
Zhou, M., and Johan, M. (2017). Regulation of growth-defense balance by the JASMONATE ZIM-DOMAIN (JAZ)-MYC transcriptional module. New Phytol. 215, 1533–1547.
Zhou, M., and Memelink, J. (2016). Jasmonate-responsive transcription factors regulating plant secondary metabolism. Biotechnol. Adv. 34, 441–449. doi: 10.1016/j.biotechadv.2016.02.004
Zhu, B., Yang, J., Yong, H., Zang, Y., and Zhu, Z. (2015). Glucosinolate accumulation and related gene expression in Pak Choi (Brassica rapa L. ssp. chinensis var. communis [N. Tsen & S.H. Lee] Hanelt) in Response to Insecticide Application. J. Agric. Food Chem. 63, 9683–9689. doi: 10.1021/acs.jafc.5b03894
Keywords: glucosinolate, cruciferous plant, secondary metabolite, hormone, exogenous substance
Citation: Liu Z, Wang H, Lv J, Luo S, Hu L, Wang J, Li L, Zhang G, Xie J and Yu J (2022) Effects of Plant Hormones, Metal Ions, Salinity, Sugar, and Chemicals Pollution on Glucosinolate Biosynthesis in Cruciferous Plant. Front. Plant Sci. 13:856442. doi: 10.3389/fpls.2022.856442
Received: 17 January 2022; Accepted: 17 March 2022;
Published: 28 April 2022.
Edited by:
Sezai Ercisli, Atatürk University, TurkeyReviewed by:
Umakanta Sarker, Bangabandhu Sheikh Mujibur Rahman Agricultural University, BangladeshShrawan Singh, Indian Agricultural Research Institute (ICAR), India
Copyright © 2022 Liu, Wang, Lv, Luo, Hu, Wang, Li, Zhang, Xie and Yu. This is an open-access article distributed under the terms of the Creative Commons Attribution License (CC BY). The use, distribution or reproduction in other forums is permitted, provided the original author(s) and the copyright owner(s) are credited and that the original publication in this journal is cited, in accordance with accepted academic practice. No use, distribution or reproduction is permitted which does not comply with these terms.
*Correspondence: Jihua Yu, eXVqaWh1YUBnc2F1LmVkdS5jbg==
†These authors have contributed equally to this work