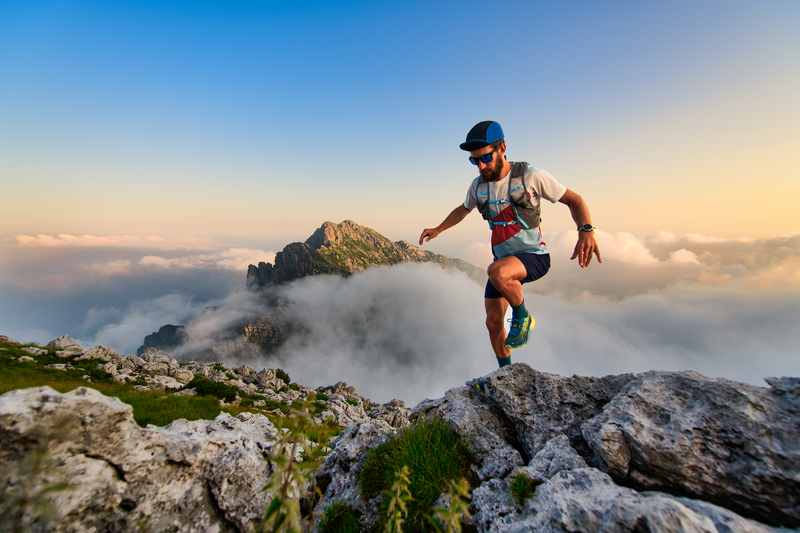
95% of researchers rate our articles as excellent or good
Learn more about the work of our research integrity team to safeguard the quality of each article we publish.
Find out more
REVIEW article
Front. Plant Sci. , 27 April 2022
Sec. Plant Physiology
Volume 13 - 2022 | https://doi.org/10.3389/fpls.2022.855559
This article is part of the Research Topic Molecular and Genetic Perspectives of Cold Tolerance in Plants View all 13 articles
Exposure of plants to low temperatures adversely affects plant growth, development, and productivity. Plant response to cold stress is an intricate process that involves the orchestration of various physiological, signaling, biochemical, and molecular pathways. Calcium (Ca2+) signaling plays a crucial role in the acquisition of several stress responses, including cold. Upon perception of cold stress, Ca2+ channels and/or Ca2+ pumps are activated, which induces the Ca2+ signatures in plant cells. The Ca2+ signatures spatially and temporally act inside a plant cell and are eventually decoded by specific Ca2+ sensors. This series of events results in the molecular regulation of several transcription factors (TFs), leading to downstream gene expression and withdrawal of an appropriate response by the plant. In this context, calmodulin binding transcription activators (CAMTAs) constitute a group of TFs that regulate plant cold stress responses in a Ca2+ dependent manner. The present review provides a catalog of the recent progress made in comprehending the Ca2+ mediated cold acclimation in plants.
Plants sense and respond to distinct environmental and developmental cues via intricate signal transduction pathways. The signal transduction pathways comprise various protein and non-protein elements. The protein elements encompass various enzymes, receptors, and TFs, while the non-protein elements include second messengers such as Ca2+, cyclic AMP, cyclic GMP, inositol triphosphate, diacylglycerol, lipids, and hydrogen ions. Amongst all the reported second messenger molecules, Ca2+ is considered central to several signal transduction pathways (Stael et al., 2012; Sarwat et al., 2013; Kudla et al., 2018). Ca2+ is an essential plant macro-nutrient that is pivotal for maintaining the structural integrity of cell walls, regulating stomatal guard cells movement, growth of pollen tubes, and elongation of root hairs (Sanders et al., 2002; White and Broadley, 2003; Dodd et al., 2010). Ca2+ signals are elicited when a plant experiences any environmental and developmental stimuli, leading to spatial and temporal changes in Ca2+ ion concentration in cells. Several reviews have extensively covered different aspects of plant Ca2+ signaling (Costa et al., 2018; Kudla et al., 2018; Thor, 2019; Tian et al., 2020; Iqbal et al., 2021a; Pirayesh et al., 2021). Briefly, under control conditions, the levels of Ca2+ ions in the cell are usually low (ranging from 100 to 200 nm), but upon receiving signals to respond, the Ca2+ channels are transiently opened, resulting in the rapid influx of Ca2+ ions inside the cell. This eventually leads to an increase in cytosolic Ca2+ ([Ca2+]cyt) levels. The levels of Ca2+ ion inside the cell fluctuates either due to Ca2+ influx via dedicated channels or Ca2+ efflux via specific pumps (Xiong et al., 2006; Tuteja and Mahajan, 2007). In Arabidopsis thaliana, plasma membrane-bound Ca2+-permeable channels are categorized into four main families, namely, cyclic nucleotide-gated channels (CNGCs), glutamate receptor-like channels (GLRs), stretch-activated Ca2+ channels (OSCAs), and the MID1-complementing activity (MCA) (Romola, 2002; Kurusu et al., 2013; Jha et al., 2016; Liu X. et al., 2018). Several other Ca2+ channels are localized in organelles, such as endoplasmic reticulum, mitochondria, golgi body, and plant vacuole (Costa et al., 2018; Thor, 2019; He et al., 2021; Pandey and Sanyal, 2021). These include autoinhibited Ca2+-ATPases (ACAs), ER-type Ca2+-ATPases (ECAs), mitochondrial Ca2+ uniporter (MCU), P1-ATPases (e.g., HMA1), Ca2+ exchangers (CAX), two-pore channel (TPC), 1,4,5-trisphosphate receptor-like channel (InsP3R), 1,4,5-trisphosphate (IP3), cyclic ADP-ribose (cADPR)-activator ryanodine receptor-like channel (RyR), slow-activating vacuolar channel (SV), and sodium–calcium exchanger (NCX).
The stimuli triggered by environmental or developmental signals generates discrete Ca2+ signatures that are sensed and recognized by specific Ca2+ sensors. This cascade of events eventually results in transcriptional and metabolic responses (Perochon et al., 2011). Ca2+ signals are recognized by most of the Ca2+ sensors via the elongation factor hand (EF-hand) motif. Multiple EF-hand containing proteins are present in plants, and Ca2+ sensors represent just one of the many that translate chemical signals into an appropriate biochemical response. The EF-hand motif is represented by a conserved helix–loop–helix structure that binds to one Ca2+ ion. They occur in pairs as distinct domain, hence, the majority of Ca2+ sensors harbor two, four, or six EF-hands (Gifford et al., 2007; Perochon et al., 2011). The pairing in certain cases is generally co-operative, consequently minimizing the required Ca2+ signal for protein saturation. Conformational changes occur upon binding of Ca2+ ion to appropriate Ca2+ sensor. These structural changes prompt the interaction between the sensor and its target protein (TP). Three major classes of Ca2+ sensor families have been recognized in plants, namely, (i) Calmodulins (CaMs) and calmodulin-like proteins (CMLs), (ii) calcineurin B-like proteins (CBLs), and (iii) Ca2+-dependent protein kinases (CDPKs) (Hrabak et al., 2003; Batistič and Kudla, 2012). CaMs are highly conserved in eukaryotes, while CMLs, CBLs, and CDPKs had only been reported in plants and protists (Day et al., 2002; Reddy and Reddy, 2004). CaMs, CMLs, and CBLs are small protein molecules possessing a Ca2+ sensing domain, thereby, acting as sensor relays. They tend to bind to the downstream effector molecules in a Ca2+ concentration-dependent manner (Luan et al., 2002). Different from the afore-mentioned Ca2+ sensors, CDPKs possess an effector domain (serine/threonine protein kinase catalytic domain) along with the Ca2+ sensing domain. Accordingly, CDPKs act as sensor responders to directly activate and regulate their TPs upon sensing Ca2+ signals (Hashimoto and Kudla, 2011). Thus, the series of events: perception of stress, the opening of Ca2+ channels, transient changes in Ca2+ levels, sensing of Ca2+ signals by appropriate Ca2+ sensor, and subsequent activation of TFs for downstream molecular and biochemical outputs generates specific responses by the plant to combat the cold stress condition. One such TF is CAMTA that regulates plant responses toward cold stress in a Ca2+ dependent manner (Iqbal et al., 2020b). The CAMTA protein is characterized by the presence of five functional domains: CG- DNA binding motif, TAD- transcriptional activation domain, TIG- for non-specific DNA interaction, Ankyrin repeats- protein–protein interaction, CAMBD- for CaM binding. Concisely, when a plant is exposed to cold stress, the Ca2+ channels are opened leading to a rapid and transient influx of Ca2+ inside the cell. This results in an increase in ([Ca2+]cyt), which is sensed by Ca2+ sensor—CaM. Eventually, CaM in a Ca2+ dependent manner regulates the transcriptional activity of the CAMTA gene, withdrawing an appropriate response by the plant against cold stress. The present review summarizes the progress made in the recent years to comprehend the involvement of Ca2+signaling in cold stress tolerance (Figure 1).
Figure 1. Cold stress signaling in a plant cell. The plasma membrane is considered as one of the primary target for cold sensing and eventual transmission of Ca2+ signals into the plant cell nuclei. Cyclic nucleotide-gated channels (CNGCs), glutamate receptor-like channels (GLRs), and the MID1-complementing activity (MCA) channels are the main plasma membrane Ca2+ channels that allow the entry of Ca2+ ions into the cytoplasm. Once the Ca2+ ion enters the plant cell, they are sensed by Calmodulins (CaMs) and Calmodulin-like proteins (CMLs), Calcineurin B-like proteins (CBLs), and Ca2+-dependent protein kinases (CDPKs). Upon cold exposure, plasma membrane associated cold sensor chilling-tolerance divergence 1 (COLD1) interacts with G protein a subunit (RGA). Ca2+/CaM regulated receptor-like kinase (CRLK) positively regulate cold triggered gene expression by inducing the MEKK1–MKK2–MPK4 pathway. CRLK suppress cold-induced activation of MPK3/6 and is necessary for inducer of CBF expression (ICE) accumulation. ICE proteins are stabilized by either phosphorylation (P) or sumolyation (S). Calmodulin binding transcription activators (CAMTAs) activate C-repeat binding factor (CBF) expression through the CM2 (CCGCGT) promoter motif. CBF proteins eventually activate the expression of various cold-responsive (COR) genes which confers cold tolerance in plants.
Low temperatures lead to intricate cellular and molecular mechanisms inside plant cells via key components of Ca2+ signaling (Yuan et al., 2018b). Ca2+ channels play critical roles in low-temperature acclimatization of chilling-tolerant A. thaliana and root hair development (Hong-Bo et al., 2008). It has been proposed that Ca2+-permeable mechanosensitive channels MCA1 and MCA2 regulate cold-induced [Ca2+]cyt increase, cold tolerance, and CBF/DREB1-independent cold signaling. The cold-induced [Ca2+]cyt was lower in mca1 and mca2 mutants than control plants. The mca1 mca2 double mutant compared to control were more sensitive to chilling and freezing stress (Mori et al., 2018). Additionally, vesicle membrane Ca2+/H+ antiporter, A. thaliana calcium exchanger 1 (AtCAX1) is implicated in an accurate development of the cold-acclimation response by regulating the induction of CBF/DREB1 and downstream genes (Catalá et al., 2003). Recently, Ca2+/cation antiporter (CaCA) superfamily proteins have been identified in Saccharum to play pivotal roles in environmental stresses, including cold (Su et al., 2021). Likewise, CNGC is a family of non-selective cation-conducting channels primarily localized to the plasma membrane (Zelman et al., 2012). They are implicated in thermal sensing and thermotolerance in Arabidopsis thaliana and mosses (Finka et al., 2012). CNGCs have been reported to play crucial roles in regulating cold tolerance in plants. Oryza sativa OsCNGC9 transcriptional activation and phosphorylation confers enhanced chilling tolerance in rice (Wang et al., 2021). OsCNGC9 overexpression provides increased cold tolerance, while its mutation leads to defects in cold-induced Ca2+ influx. Rice OsDREB1A TF is responsible for the activation of OsCNGC9 transcription. In crux, OsCNGC9 increases chilling tolerance by regulating cold-induced Ca2+ influx and [Ca2+]cyt elevation (Wang et al., 2021). Additionally, CNGC family has been characterized in Chinese jujube (Ziziphus jujuba Mill.), and ZjCNGC2 was reported to regulate signaling cascades in response to cold stress (Wang et al., 2020b). Further, it was shown that rice CNGC14 and CNGC16 are involved in promoting tolerance toward heat and chilling stresses, and are regulators of Ca2+ signals in response to temperature stress (Cui et al., 2020). Their homologs in A. thaliana (AtCNGC2 and AtCNGC4) are also implicated in tolerance toward low temperature (Cui et al., 2020). CNGCs had also been implicated in modulating cold stress responses along with other biological stresses via Ca2+ signals in Brassica oleracea (Kakar et al., 2017), O. sativa (Nawaz et al., 2014), and Nicotiana tabacum (Nawaz et al., 2019).
The endoplasmic reticulum and plasma-membrane localized G-protein regulator CHILLING TOLERANCE DIVERGENCE1 (COLD1) coupled with RICE G-PROTEIN α SUBUNIT1 (RGA1) was reported in cold stress signaling via Ca2+ signals and electrophysiological responses in O. sativa (Ma Y. et al., 2015). The COLD1-RGA1 complex regulates the cold stress-driven influx of intracellular Ca2+, eventually resulting in the activation of COR (cold regulated) genes. It remains a subject of further evaluation whether COLD1 plays a role as a Ca2+-permeable channel or as a mediator promoting Ca2+-permeable channel activity. Taking into account another plasma membrane-bound Ca2+ channel—GLR—mediate Ca2+ fluxes across membranes and is responsive to an array of exogenous and endogenous signals in plants. AtGLR3.4 localizes to the plasma membrane and is stimulated by cold stress in a Ca2+-dependent manner (Meyerhoff et al., 2005; Weiland et al., 2015). AtGLR1.2 and AtGLR1.3 were reported to positively regulate cold tolerance by modulating jasmonate signaling in A. thaliana (Zheng et al., 2018). The cold sensitivity of glr1.2 and glr1.3 mutants was attenuated by exogenous jasmonate treatment, while the over-expression of GLR1.2 or GLR1.3 led to elevated cold tolerance by enhancing endogenous jasmonate levels. Additionally, under cold stress, the expression of genes in the CBF/DREB1 signaling pathway were lowered in glr1.2 and glr1.3 mutants, whereas higher in GLR1.2 and GLR1.3 over-expression lines (Zheng et al., 2018). Similar to the above finding, tomato GLR3.3 and GLR3.5 were reported to regulate cold acclimation-induced chilling tolerance by modulating apoplastic H2O2 production and redox homeostasis (Li H. et al., 2019). Next, annexins are Ca2+ permeable transporters that mediate the accumulation of [Ca2+]cyt in responses to abiotic stresses (Lee et al., 2004; Laohavisit et al., 2012; Richards et al., 2014). Recently, ANNEXIN1 was reported to regulate cold-induced Ca2+ influx and freezing tolerance in A. thaliana (Liu et al., 2021). The mutation of AtANN1 decreased freeing tolerance, impaired cold triggered [Ca2+]cyt increase, and upregulated cold-responsive CBF and COR genes. The study revealed that AtANN1 acts downstream of OST1 in responses to cold stress (Liu et al., 2021). Furthermore, the organellar Ca2+ channel, GhCAX3 gene from Gossypium hirsutum was characterized under various abiotic stresses, including cold. Transgenics compared to control plants were more sensitive to cold stress during seed germination. Over-expression of GhCAX3 led to the transcript enrichment of some of the abscisic acid (ABA)-and cold-responsive genes. The study concluded that GhCAX3 plays an imperative part in the cross-talk of cold and ABA signal transduction (Xu et al., 2013). Likewise, IP3 was reported to mediate nitric oxide (NO) triggered chilling tolerance in postharvest peach fruit (Jiao et al., 2019).
Calmodulins and calmodulin-like protein are widely studied Ca2+ sensors that sense and decode rapid and transient fluctuations in the intracellular Ca2+ levels in response to environmental cues. In plants, CaMs and CMLs have been reported to play pivotal roles in developmental and stress biology (Zeng et al., 2015; Ranty et al., 2016; Aldon et al., 2018; Gao et al., 2019). CaMs and CMLs transcripts are induced or suppressed in response to a variety of abiotic stresses (Zeng et al., 2017; Li C. et al., 2019). Initial studies revealed that CaM3 overexpressing lines had reduced levels of COR transcripts, suggestive of the fact that CaM might act as a negative regulator of cold stress (Townley and Knight, 2002). In a similar vein, AtCaM4 had been reported to negatively regulate freezing tolerance in A. thaliana. The cam4 mutants exhibited increased tolerance to freezing stress. AtCaM4 might regulate freezing tolerance in a CBF-independent manner (Chu et al., 2018). In an interesting study, the germination of developing immature cml39 seeds in comparison to control seeds was not sensitive to cold-stratification. Hence, it was reported that CML39 has a role in stratification-dependant seed dormancy (Midhat et al., 2018). Lately, the effect of cold stress along with other abiotic stresses was assessed for the expression of CaMs and CMLs in wild-growing grapevine Vitis amurensis. VaCaM8 and VaCaM10 showed significant differential expression under cold stress (4°C). Incubation at 4°C or 10°C induced the expression of six CML genes (VaCML21, VaCML44, VaCML61, VaCML78, VaCML86, and VaCML89; while reduced the expression of eight CML genes (VaCML9a, VaCML48, VaCML57, VaCML75, VaCML82, VaCML85, VaCML92, and VaCML107) (Dubrovina et al., 2019). The same group reported four alternatively spliced mRNA forms of the grapevine CML21 gene (CML21v1, CML21v2, CML21v3, and CML21v4). All the four splice variants were highly induced under cold stress. Heterologous expression of CML21v2 and VaCML21v4 in A. thaliana increased the survival percentage of the transgenics upon freezing. Cold stress-responsive marker genes: dehydration-responsive element-binding, AtDREB1A and AtDREB2A were induced in VaCML21v2 overexpression lines, while AtCOR47, AtRD29A, AtRD29B, and AtKIN1 genes were induced in VaCML21v4 overexpression lines after freezing stress in the transgenic Arabidopsis plants. Thus, it was established that CML21 acts as a positive regulator of cold stress (Aleynova et al., 2020). Likewise, Medicago sativa, MsCML46 gene encoding calmodulin-like protein confers tolerance to cold and other abiotic stress in tobacco. The MsCML46 was upregulated in the leaves and roots after exposure to cold stress. The expression peaked after 1 h in leaves, while in roots, the expression peaked at 3 h (Du et al., 2021). In a similar vein, five Camellia sinensis- CsCML genes (CsCML16, CsCML18-1, CsCML18-2, CsCML38, and CsCML42) were functionally characterized under various environmental stresses. The transcript levels of CsCML16, 18-2, and 42 were significantly induced by low temperature and salt stress (Ma Q. et al., 2019). Previously, Solanum habrochaites (cold-tolerant wild tomato) ShCML44 gene was functionally characterized under a variety of environmental stresses, including cold stress. The ShCML44 overexpressed plants had higher antioxidant enzymes activity, better gas exchange and water retention capacity, lower malondialdehyde (MDA) accumulation and membrane damage, reduced reactive oxygen species (ROS), and higher relative water contents (Munir et al., 2016). Very recently, Solanum lycopersicum SlCML37 has been shown to interact with proteasome maturation factor SlUMP1 and has been reported in tomato fruit chilling stress tolerance (Tang et al., 2021). Additionally, Medicago truncatula MtCML42 has been reported to regulate cold tolerance and flowering time (Sun et al., 2021). Further, in rice, six new putative interacting partners of OsCML16 were identified (OsLRK5a, OsDCNL2, OsWD40-139, OsGDH1, OsCIP, and OsERD2). The in vitro peptide-binding assays suggested that OsERD2 could bind both OsCaM1 and OsCML16, while the other five TPs specifically binded to OsCML16. Moreover, Ca2+ and trifluoperazine (TFP)—CaM antagonist were involved in ABA-induced transcription of OsCML16 and its target genes. OsCML16 and its target genes were triggered by salt, drought, and low-temperature stress (Yang et al., 2020).
Calcium/Calmodulins-regulated receptor-like kinases 1 (CRLK1) encoding a plasma membrane-associated serine/threonine kinase has been reported to play a crucial role in cold stress responses (Yang et al., 2010a,b; Furuya et al., 2013, 2014). The crlk1 mutants compared to control plants are sensitive to freezing temperatures. The expression of cold-responsive genes, such as, CBF1, RD29A, and COR15a was suppressed in crlk1 mutants, making them more susceptible to cold stress than control plants. CRLK1 protein expression is induced upon low temperature (4°C) exposures and oxidative stress (H2O2). Thus, CRLK1 is considered a positive regulator of cold stress responses in A. thaliana. Additionally, the Ca2+/CaM complex is a requisite for triggering CRLK1 kinase. It has been reported previously that an increase in CaM levels in the presence of Ca2+ elevates the activity of CRLK1 kinase. On the contrary, chlorpromazine (CPZ)—CaM antagonist blocked the CaM mediated CRLK1 kinase activity (Yang et al., 2010a). Explicitly, the presence of CaM-binding domain at the C-termini of CRLK1 is essential for CaM-modulated kinase activity (Yang et al., 2010a). Besides, the inducer of CBF expression 1 (ICE1) is a transcription activator and a major component of the cold response pathway as it binds with the promoters of the C-repeat binding factor (CBF) and COR genes (Tang et al., 2020). CRLK1 and CRLK2 suppress cold-induced activation of MPK3/6 and are necessary for ICE1 accumulation (Zhao et al., 2017). Hence, there exists a Ca2+ signaling-mediated cold-responsive pathway which is regulated by CRLK1 (Yang et al., 2010a,b).
Calcineurin B-like proteins represent a major class of Ca2+ binding proteins and are considered imperative relays in plant Ca2+ signaling pathways. CBL and CBL-interacting protein kinase (CIPK) complex are central to Ca2+ signaling. This complex had been reported to be implicated in a plethora of external stress signals (Kolukisaoglu et al., 2004; Yu et al., 2014; Mohanta et al., 2015). In this context, CBL9 had been shown to negatively regulate cold tolerance via Ca2+ signaling in A. thaliana (Gao and Zhang, 2019). cbl9 mutants showed enhanced freezing tolerance under cold-acclimating and non-acclimating conditions. Exposure to cold stress increased [Ca2+]cyt in cbl9 mutants compared to wild type. Contrarily, ethylene glycol-bis(2-aminoethylether)-N,N,N′,N′-tetraacetic acid (EGTA)—Ca2+ chelator and lanthanum chloride—Ca2+ channel blocker significantly altered [Ca2+]cyt in cbl9 mutants (Gao and Zhang, 2019). Lately, in Camellia sinensis (tea plant), it was shown that CsCBL9 and CsCIPK4/6a/6b/7/11/14b/19/20 were upregulated in both mature leaves and young shoots upon cold stress. Results of yeast two-hybrid assay demonstrated that CsCBL1 potentially interacted with CsCIPK1/10b/12 but not with CsCIPK6a/7/11/14b/20. Similarly, CsCBL9 interacted with CsCIPK1/10b/12/14b but not with CsCIPK6a/7/11/20. Thus, the study proposed distinct responses to cold stress mediated by CBL–CIPK complexes (Wang et al., 2020a). In addition, CIPKs had also been functionally characterized in Triticum aestivum (Deng et al., 2013), Capsicum annuum (Ma X. et al., 2019), Manihot esculenta (Mo et al., 2018), Malus domestica (Wang et al., 2012; Niu et al., 2018), and Brachypodium distachyon (Luo et al., 2018) under different environmental cues, including cold stress. TaCIPK29 transcript increased after cold treatment (Deng et al., 2013), while CaCIPK1 expression changed in response to cold stress (Ma X. et al., 2019). The expression of MeCIPK7 significantly increased in roots upon cold treatment. The transcript levels of MeCIPK10 and 13 in roots, whereas transcript levels MeCIPK12 and 16 in leaves were also altered upon cold treatment (Mo et al., 2018). This study by Mo et al. (2018) suggested that cassava (Manihot esculenta) CBL–CIPK signal networks function in responses to abiotic stresses. MdCIPK6L ectopic expression significantly enhanced chilling tolerance in transgenic tomatoes (Wang et al., 2012), whereas the ectopic expression of BdCIPK31 renders increased low-temperature tolerance in transgenic tobacco (Luo et al., 2018). Likewise, CBLs had been molecularly characterized under a variety of environmental stresses, including cold in Brassica napus (Zhang H. et al., 2014), Brassica rapa (Jung et al., 2017), Stipa purpurea (Zhou et al., 2016), and Pyrus betulifolia Bunge (Xu Y. et al., 2015). For Brassica napus, BnaCBL1 transcripts significantly increased at 6 h of cold treatment; however, it was downregulated at 24 h. At 24 h of cold treatment, only BnaCBL10 was slightly upregulated, and transcripts of BnaCBL2, -3, -4 were downregulated (Zhang H. et al., 2014). For Brassica rapa, BrCBL1-1 transcript levels were highly elevated (∼30-fold upregulation) after 4 h of cold treatment in one of the in-bred lines of Brassica rapa (Chiifu) (Jung et al., 2017). Further, overexpression of SpCBL6 from Stipa purpurea increased cold tolerance and decreased drought tolerance in transgenic A. thaliana (Zhou et al., 2016). On similar grounds, PbCBL1 responded to alterations in the intracellular Ca2+ concentrations and was induced by cold stress (Xu Y. et al., 2015).
Calcium-dependent protein kinases comprise a multi-gene kinase family in plants and are major regulators of developmental and stress responses in plants (Cheng et al., 2002; Valmonte et al., 2014). As already stated, CDPKs function as direct sensor responders to decode the Ca2+ signals (Hashimoto and Kudla, 2011). Upon sensing Ca2+ signals, CDPKs activate and regulate the TPs directly. Several CDPK-encoding genes are differentially expressed upon cold stress; however, their underlying molecular mechanisms remain elusive. In rice, OsCPK17 targets the sucrose–phosphate synthase and plasma membrane intrinsic proteins and was reported in cold stress response (Almadanim et al., 2017). Additionally, OsCPK24 inhibits glutaredoxin (OsGrx10), thereby, sustaining higher glutathione levels and phosphorylation. OsCPK24 has been shown to positively regulate cold stress tolerance (Liu Y. et al., 2018). In yet another monocot plant—banana, MaCDPK7 was shown to regulate the fruit ripening process and chilling resistance induced by heat treatment (Wang et al., 2017). Later, the CDPK gene family was characterized in banana for their involvement in the development, fruit ripening, and abiotic stress responses, including cold (Li et al., 2020). Genome-wide identification of the CDPK gene family in Medicago truncatula also revealed that MtCDPK4, 8, 15, 16, and 22 transcripts were quickly elevated after 2 h of cold treatment (Zhao et al., 2021). Previously, the CDPK gene family had been identified and assessed for its involvement under abiotic stress conditions, including cold in Solanum lycopersicum (tomato; Hu et al., 2016), Cucumis melo (melon; Zhang et al., 2017), Cucumis sativus (cucumber; Xu X. et al., 2015), zea mays (maize; Kong et al., 2013). Moreover, in Populus euphratica, PeCPK10 confers cold and drought stress tolerance. Precisely, overexpression of PeCPK10 increased freezing tolerance in the transgenics. The expression of ABA and stress-responsive genes such as RD29B and COR15A were induced by constitutive expression of PeCPK10 (Chen et al., 2013). In an interesting study, the roles of VaCPK16, VaCPK25, VaCPK30, and VaCPK32 in secondary metabolites biosynthesis and stress resistance was studied in V. amurensis (grapevine) (Dubrovina et al., 2018). Overexpressing the VaCPK30 gene conferred enhanced resistance to cold and salt stress in transgenics, whereas overexpressing VaCPK16, VaCPK25, and VaCPK32 did not influence temperature and salt stress tolerance. Instead, the overexpression of VaCPK16 and VaCPK32 enhanced stilbene accumulation in V. amurensis cell cultures (Dubrovina et al., 2018). Earlier the same group had reported the involvement of VaCPK20 in cold and drought stress response pathways (Dubrovina et al., 2015). On similar lines in Zea mays, ZmCPK1 was reported as a negative regulator of cold stress signaling in maize (Weckwerth et al., 2015). ZmCPK1 displayed Ca2+-independent protein kinase activity. The expression of ZmCPK1 increased, while the expression of ZmCPK25 decreased upon cold stress (Weckwerth et al., 2015). Recently, Malus domestica (apple) MdCPK1a gene was reported to enhance tobacco cold resistance via scavenging ROS accumulation (Dong et al., 2020). The underlying mechanism of cold resistance through the involvement of MdCPK1a was further investigated. The MdCPK1a tobacco transgenics had a better survival ratio and root length when subjected to cold stress. The superoxide dismutase (SOD), peroxidase (POD), and catalase (CAT) activities were higher, while electrolyte leakages (EL), MDA content, and ROS were lower. This was suggestive of the fact that the transgenics underwent less chilling injury than control plants (Dong et al., 2020). Thus, Ca2+ signaling plays a pivotal part in cold acclimation in plants (Table 1).
Upon perception of cold stress, Ca2+ signals are elevated, which might direct Ca2+ to either repress or activate the activity of Ca2+ responding protein. Similarly, the interaction of Ca2+ with Ca2+ sensors either suppresses or enhances the binding to a TF. Depending upon whether the TF itself is a repressor or activator, the transcription of the target gene is repressed or activated. Ca2+/CaM dependent TFs relay cold-induced Ca2+ transients to transcriptional reprograming. CAMTAs are one such group of TFs that regulate plant cold stress responses in a Ca2+-dependent manner. CAMTA proteins have been stipulated to play a direct link between Ca2+ signals and cold acclimation (Eckardt, 2009). CAMTAs also known as signal responsive (SR) protein (Yang and Poovaiah, 2000) or EICBP (ethylene-induced CaM-binding proteins) (Reddy et al., 2000) is a well-characterized CaM dependent TF that regulates gene expression by binding to the signature “CGCG” DNA motif (Galon et al., 2008; Du et al., 2009; Yuan et al., 2018a). Furthermore, CBF cold response pathway plays a pivotal role in cold acclimation (Shi et al., 2018). It is characterized by rapid cold induction of genes encoding the CBF1-3 TFs, followed by the expression of the CBF gene regulon. The CRT/DRE cis-element is recognized by the CBF protein and is characterized by the presence of a conserved CCGAC sequence. The CCGAC sequence is present in the 1000 bp upstream region of a subset of COR genes (Stockinger et al., 1997; Gilmour et al., 1998; Shi et al., 2018; Liu et al., 2019). The cis and trans-acting factors implicated in the expression of CBF2 were studied by Doherty et al. (2009). Seven conserved DNA motifs (CM1 to 7) were identified in the promoters of CBF2 and ZAT12 (cold-induced genes). CM4 and CM6 have negative regulatory activity, while CM2 has both negative and positive activity. The study also revealed that CAMTA3 binds to the CM2 motif and is a positive regulator of CBF2 expression. Moreover, camta1 camta3 double mutant plants were impaired in freezing tolerance. This study exhibited a novel role of CAMTA in cold acclimation and provided a plausible link of low-temperature Ca2+ and CaM signaling with cold-regulated gene expression (Doherty et al., 2009). Later, CAMTA3 and CAMTA5 were reported to respond to a rapid decrease in temperature and induce the expression of DREB1s (Kidokoro et al., 2017). Additionally, contrary to circadian clock associated1 and late elongated hypocotyl genes that modulate DREB1 expression only during the day, CAMTA3 and CAMTA5 function both during the day and night (Kidokoro et al., 2017).
Salicylic acid (SA) has a central role in transcriptional machinery at low temperatures (Scott et al., 2004). However, accumulated SA did not influence cold tolerance in atsr1 (also referred as CAMTA3) (Kim et al., 2013). CAMTA1 and CAMTA2 in combination with CAMTA3 induced transcripts of CBF1, CBF2, and CBF3 at 2 h and enhanced plant freezing tolerance. Additionally, CAMTA1, CAMTA2, and CAMTA3 work simultaneously to inhibit SA biosynthesis at warm temperatures (22°C). However, the SA levels increased in plants exposed to low-temperatures for more than one week. The study revealed that the isochorismate synthase (ICS) pathway is involved in chilling-induced SA biosynthesis. The accumulation of ICS1, CBP60g, and SARD1 transcripts were suppressed at warm temperatures by these three CAMTAs, but not at low temperatures (Kim et al., 2013). The analysis of upstream regions to the transcription start site (TSS) in wound-induced genes indicated the presence of rapid stress response DNA element (RSRE), CGCGTT. Moreover, promoter activity assay depicted that luciferase activity level induced by cold stress was lower in camta3 mutants than control plants (Benn et al., 2014). The study revealed that CAMTA3 modulates cold tolerance in A. thaliana via the regulation of genes that harbor RSRE elements in their promoters (Benn et al., 2014). Another interesting study found that heptahelical protein 2 (HHP2) interacts with CBF upstream regulators, such as ICE1, ICE2, and CAMTA3 (Lee and Seo, 2015). At low-temperatures, MYB96 (R2R3-type MYB TF) induced the HHP genes (Lee and Seo, 2015). This suggests that a cross-wired mesh of pathways exist that incorporates Ca2+ signaling to regulate cold stress tolerance through CAMTA3. Kim et al. (2017) revealed that the IQ motifs in AtCAMTA3 (residues 850–875) are necessary for its activity (Kim et al., 2017). Post-translational modifications (phosphorylation or dephosphorylation) play imperative part in AtCAMTA3 mediated response to environmental cues. S454 and S964 were identified as two putative phosphorylation sites in AtCAMTA3 protein (Jones et al., 2009). The camta1 camta3 double mutants complemented with mutated AtCAMTA3 protein, S454A and S964A (phosphorylation sites of AtCAMTA3) were partially restored to control plants. Moreso, the suppression of SA biosynthesis in the mutants was compromised, suggestive of the fact that phosphorylation is necessary for the full functionality of AtCAMTA3 (Kim et al., 2017). It is well reported that CAMTA3 is a defense repressor. CAMTA3 is degraded to trigger SA-mediated immune response during pathogen incursion (Galon et al., 2008; Poovaiah et al., 2013; Zhang L. et al., 2014; Fromm and Finkler, 2015; Kim et al., 2017). Intriguingly, SA-mediated signaling pathways also cross-talk with pathways implicated in long-term cold treatments (4°C, 2 weeks) (Kurepin et al., 2013; Miura and Tada, 2014). Nonetheless, AtCAMTA3 protein is also accumulated at low temperatures (Kim et al., 2017). These observations suggest that a complex mesh of networks intersect with each other to overcome the AtCAMTA3 suppression of the SA signaling pathway. Very recently, evolution analyses of CAMTA genes in 112 plant species were performed to study its enhancing effect on cold tolerance (Xiao et al., 2021). Thus, CAMTAs via Ca2+/CaM signaling has an intersecting role in imparting cold tolerance to plants.
The underpinning mechanisms of cold signaling pathways and genes implicated in cold stress have been extensively studied in the past few years. Different signaling pathways converge to allow plants cope with cold stress. Perception of cold stress by the plant is contemplated to be the first event for the induction of Ca2+ transients (Ma Y. et al., 2015). The cold stress-triggered Ca2+ transients are generated via a number of Ca2+ channels and/or Ca2+ pumps. These Ca2+ transients are relayed and decoded by a variety of Ca2+ sensors to regulate gene expression and subsequently confer cold tolerance to plants (Ma Y. et al., 2015; Mori et al., 2018). Considerable advancements have been made to comprehend the underlying components of the Ca2+ signaling network, such as, Ca2+–CBL–CIPK, CDPK, and Ca2+–CaM–CAMTA (Weckwerth et al., 2015; Kidokoro et al., 2017; Wang et al., 2020a). Moreover, plant cold tolerance is an intricate process involving dissecting signal transduction pathways. It remains elusive how other signaling pathways intersect with Ca2+ signaling pathways to confer cold tolerance in plants. It is still a challenge to deeply decipher the role of Ca2+ signals in the cold stress tolerance mechanism and to ascertain whether cold stress-triggered Ca2+ transients exist in the cell nucleus. Cutting edge techniques such as multi-omics (Iqbal et al., 2021b), CRISPR/cas9 gene-editing systems (Iqbal et al., 2020a), and sensitive Ca2+ imaging (Grenzi et al., 2021) can prove to be potent tools to determine the un-discovered aspects of Ca2+ signaling pathways. Thus, future research should focus on deciphering the key converging and diverging pathways pivotal to Ca2+ mediated cold signaling. Further, gaining in-depth insights as to how Ca2+ signatures are induced and decoded in response to cold stress can help better comprehend the involvement of Ca2+ ion in cold stress signaling. Nonetheless, efforts should be made to identify low-temperature sensors using biological methods in combination with biochemical and biophysical approaches.
ZI drafted and wrote the manuscript. AGM and AA critically revised the manuscript for consistency and content. MSI conceptualized the idea and reviewed the manuscript. All authors reviewed and approved the final version of the manuscript.
The authors did not receive any external funding.
The authors declare that the research was conducted in the absence of any commercial or financial relationships that could be construed as a potential conflict of interest.
All claims expressed in this article are solely those of the authors and do not necessarily represent those of their affiliated organizations, or those of the publisher, the editors and the reviewers. Any product that may be evaluated in this article, or claim that may be made by its manufacturer, is not guaranteed or endorsed by the publisher.
The authors are thankful to all the contributors in the field of calcium signaling and cold stress biology.
Aldon, D., Mbengue, M., Mazars, C., and Galaud, J.-P. (2018). Calcium signalling in plant biotic interactions. Int. J. Mol. Sci. 19:665. doi: 10.3390/ijms19030665
Aleynova, O. A., Kiselev, K. V., Ogneva, Z. V., and Dubrovina, A. S. (2020). The grapevine calmodulin-like protein gene CML21 is regulated by alternative splicing and involved in abiotic stress response. Int. J. Mol. Sci. 21:7939. doi: 10.3390/ijms21217939
Almadanim, M. C., Alexandre, B. M., Rosa, M. T., Sapeta, H., Leitão, A. E., Ramalho, J. C., et al. (2017). Rice calcium-dependent protein kinase OsCPK17 targets plasma membrane intrinsic protein and sucrose-phosphate synthase and is required for a proper cold stress response. Plant Cell Environ. 40, 1197–1213. doi: 10.1111/pce.12916
Batistič, O., and Kudla, J. (2012). Analysis of calcium signaling pathways in plants. Biochim. Biophys. Acta 1820, 1283–1293. doi: 10.1016/j.bbagen.2011.10.012
Benn, G., Wang, C. Q., Hicks, D. R., Stein, J., Guthrie, C., and Dehesh, K. (2014). A key general stress response motif is regulated non-uniformly by CAMTA transcription factors. Plant J. 80, 82–92. doi: 10.1111/tpj.12620
Catalá, R., Santos, E., Alonso, J. M., Ecker, J. R., Martínez-Zapater, J. M., and Salinas, J. (2003). Mutations in the Ca2+/H+ transporter CAX1 increase CBF/DREB1 expression and the cold-acclimation response in Arabidopsis. Plant Cell 15, 2940–2951. doi: 10.1105/tpc.015248
Chen, J., Xue, B., Xia, X., and Yin, W. (2013). A novel calcium-dependent protein kinase gene from Populus euphratica, confers both drought and cold stress tolerance. Biochem. Biophys. Res. Commun. 441, 630–636. doi: 10.1016/j.bbrc.2013.10.103
Cheng, S.-H., Willmann, M. R., Chen, H.-C., and Sheen, J. (2002). Calcium signaling through protein kinases. The Arabidopsis calcium-dependent protein kinase gene family. Plant Physiol. 129, 469–485. doi: 10.1104/pp.005645
Chu, M., Li, J., Zhang, J., Shen, S., Li, C., Gao, Y., et al. (2018). AtCaM4 interacts with a Sec14-like protein, PATL1, to regulate freezing tolerance in Arabidopsis in a CBF-independent manner. J. Exp. Bot. 69, 5241–5253. doi: 10.1093/jxb/ery278
Costa, A., Navazio, L., and Szabo, I. (2018). The contribution of organelles to plant intracellular calcium signalling. J. Exp. Bot. 69, 4175–4193. doi: 10.1093/jxb/ery185
Cui, Y., Lu, S., Li, Z., Cheng, J., Hu, P., Zhu, T., et al. (2020). CYCLIC NUCLEOTIDE-GATED ION CHANNELs 14 and 16 promote tolerance to heat and chilling in rice. Plant Physiol. 183, 1794–1808. doi: 10.1104/pp.20.00591
Day, I. S., Reddy, V. S., Ali, G. S., and Reddy, A. (2002). Analysis of EF-hand-containing proteins in Arabidopsis. Genome Biol. 3, 1–24. doi: 10.1186/gb-2002-3-10-research0056
Deng, X., Hu, W., Wei, S., Zhou, S., Zhang, F., Han, J., et al. (2013). TaCIPK29, a CBL-interacting protein kinase gene from wheat, confers salt stress tolerance in transgenic tobacco. PLoS One 8:e69881. doi: 10.1371/journal.pone.0069881
Dodd, A. N., Kudla, J., and Sanders, D. (2010). The language of calcium signaling. Ann. Rev. Plant Biol. 61, 593–620. doi: 10.1146/annurev-arplant-070109-104628
Doherty, C. J., Van Buskirk, H. A., Myers, S. J., and Thomashow, M. F. (2009). Roles for Arabidopsis CAMTA transcription factors in cold-regulated gene expression and freezing tolerance. Plant Cell 21, 972–984. doi: 10.1105/tpc.108.063958
Dong, H., Wu, C., Luo, C., Wei, M., Qu, S., and Wang, S. (2020). Overexpression of MdCPK1a gene, a calcium dependent protein kinase in apple, increase tobacco cold tolerance via scavenging ROS accumulation. PLoS One 15:e0242139. doi: 10.1371/journal.pone.0242139
Du, B., Chen, N., Song, L., Wang, D., Cai, H., Yao, L., et al. (2021). Alfalfa (Medicago sativa L.) MsCML46 gene encoding calmodulin-like protein confers tolerance to abiotic stress in tobacco. Plant Cell Rep. 40, 1907–1922. doi: 10.1007/s00299-021-02757-7
Du, L., Ali, G. S., Simons, K. A., Hou, J., Yang, T., Reddy, A., et al. (2009). Ca 2+/calmodulin regulates salicylic-acid-mediated plant immunity. Nature 457, 1154–1158. doi: 10.1038/nature07612
Dubrovina, A. S., Aleynova, O. A., Ogneva, Z. V., Suprun, A. R., Ananev, A. A., and Kiselev, K. V. (2019). The effect of abiotic stress conditions on expression of calmodulin (CaM) and calmodulin-like (CML) genes in wild-growing grapevine Vitis amurensis. Plants 8:602. doi: 10.3390/plants8120602
Dubrovina, A. S., Kiselev, K. V., Khristenko, V. S., and Aleynova, O. A. (2015). VaCPK20, a calcium-dependent protein kinase gene of wild grapevine Vitis amurensis Rupr., mediates cold and drought stress tolerance. J. Plant Physiol. 185, 1–12. doi: 10.1016/j.jplph.2015.05.020
Dubrovina, A., Aleynova, O., Manyakhin, A., and Kiselev, K. (2018). The role of calcium-dependent protein kinase genes CPK16, CPK25, CPK30, and CPK32 in stilbene biosynthesis and the stress resistance of grapevine vitis amurensis rupr. Appl. Biochem. Microbiol. 54, 410–417. doi: 10.1134/s0003683818040051
Eckardt, N. A. (2009). CAMTA proteins: a direct link between calcium signals and cold acclimation? Plant Cell 21, 697–697. doi: 10.1105/tpc.109.210310
Finka, A., Cuendet, A. F. H., Maathuis, F. J., Saidi, Y., and Goloubinoff, P. (2012). Plasma membrane cyclic nucleotide gated calcium channels control land plant thermal sensing and acquired thermotolerance. Plant Cell 24, 3333–3348. doi: 10.1105/tpc.112.095844
Fromm, H., and Finkler, A. (2015). Repression and de-repression of gene expression in the plant immune response: the complexity of modulation by Ca2+ and calmodulin. Mol. Plant 8, 671–673. doi: 10.1016/j.molp.2015.01.019
Furuya, T., Matsuoka, D., and Nanmori, T. (2013). Phosphorylation of Arabidopsis thaliana MEKK1 via Ca 2+ signaling as a part of the cold stress response. J. Plant Res. 126, 833–840. doi: 10.1007/s10265-013-0576-0
Furuya, T., Matsuoka, D., and Nanmori, T. (2014). Membrane rigidification functions upstream of the MEKK1-MKK2-MPK4 cascade during cold acclimation in Arabidopsis thaliana. FEBS Lett. 588, 2025–2030. doi: 10.1016/j.febslet.2014.04.032
Galon, Y., Nave, R., Boyce, J. M., Nachmias, D., Knight, M. R., and Fromm, H. (2008). Calmodulin-binding transcription activator (CAMTA) 3 mediates biotic defense responses in Arabidopsis. FEBS Lett. 582, 943–948. doi: 10.1016/j.febslet.2008.02.037
Gao, Q., Xiong, T., Li, X., Chen, W., and Zhu, X. (2019). Calcium and calcium sensors in fruit development and ripening. Sci. Horticult. 253, 412–421. doi: 10.1016/j.scienta.2019.04.069
Gao, Y., and Zhang, G. (2019). A calcium sensor calcineurin B-like 9 negatively regulates cold tolerance via calcium signaling in Arabidopsis thaliana. Plant Signal. Behav. 14:e1573099. doi: 10.1080/15592324.2019.1573099
Gifford, J. L., Walsh, M. P., and Vogel, H. J. (2007). Structures and metal-ion-binding properties of the Ca2+–binding helix–loop–helix EF-hand motifs. Biochem. J. 405, 199–221. doi: 10.1042/BJ20070255
Gilmour, S. J., Zarka, D. G., Stockinger, E. J., Salazar, M. P., Houghton, J. M., and Thomashow, M. F. (1998). Low temperature regulation of the Arabidopsis CBF family of AP2 transcriptional activators as an early step in cold-induced COR gene expression. Plant J. 16, 433–442. doi: 10.1046/j.1365-313x.1998.00310.x
Grenzi, M., Resentini, F., Vanneste, S., Zottini, M., Bassi, A., and Costa, A. (2021). Illuminating the hidden world of calcium ions in plants with a universe of indicators. Plant Physiol. 187, 550–571. doi: 10.1093/plphys/kiab339
Hashimoto, K., and Kudla, J. (2011). Calcium decoding mechanisms in plants. Biochimie 93, 2054–2059. doi: 10.1016/j.biochi.2011.05.019
He, J., Rössner, N., Hoang, M. T., Alejandro, S., and Peiter, E. (2021). Transport, functions, and interaction of calcium and manganese in plant organellar compartments. Plant Physiol. 187:122. doi: 10.1093/plphys/kiab122
Hong-Bo, S., Li-Ye, C., Ming-An, S., Shi-Qing, L., and Ji-Cheng, Y. (2008). Bioengineering plant resistance to abiotic stresses by the global calcium signal system. Biotechnol. Adv. 26, 503–510. doi: 10.1016/j.biotechadv.2008.04.004
Hrabak, E. M., Chan, C. W., Gribskov, M., Harper, J. F., Choi, J. H., Halford, N., et al. (2003). The Arabidopsis CDPK-SnRK superfamily of protein kinases. Plant Physiol. 132, 666–680. doi: 10.1104/pp.102.011999
Hu, Z., Lv, X., Xia, X., Zhou, J., Shi, K., Yu, J., et al. (2016). Genome-wide identification and expression analysis of calcium-dependent protein kinase in tomato. Front. Plant Sci. 7:469. doi: 10.3389/fpls.2016.00469
Iqbal, Z., Iqbal, M. S., Ahmad, A., Memon, A. G., and Ansari, M. I. (2020a). New prospects on the horizon: genome editing to engineer plants for desirable traits. Curr. Plant Biol. 2020:100171. doi: 10.1016/j.cpb.2020.100171
Iqbal, Z., Shariq Iqbal, M., Singh, S. P., and Buaboocha, T. (2020b). Ca2+/calmodulin complex triggers CAMTA transcriptional machinery under stress in plants: signaling cascade and molecular regulation. Front. Plant Sci. 11:1829. doi: 10.3389/fpls.2020.598327
Iqbal, Z., Iqbal, M. S., Hashem, A., AbdAllah, E. F., and Ansari, M. I (2021a). Plant defense responses to biotic stress and its interplay with fluctuating dark/light conditions. Front. Plant Sci. 12:297. doi: 10.3389/fpls.2021.631810
Iqbal, Z., Iqbal, M. S., Khan, M. I. R., and Ansari, M. I. (2021b). Toward integrated multi-omics intervention: rice trait improvement and stress management. Front. Plant Sci. 2021:12. doi: 10.3389/fpls.2021.741419
Jha, K., Sharma, M., and Pandey, G. (2016). Role of cyclic nucleotide gated channels in stress management in plants. Curr. Genom. 17, 315–329. doi: 10.2174/1389202917666160331202125
Jiao, C., Chai, Y., and Duan, Y. (2019). Inositol 1, 4, 5-trisphosphate mediates nitric-oxide-induced chilling tolerance and defense response in postharvest peach fruit. J. Agricult. Food Chem. 67, 4764–4773. doi: 10.1021/acs.jafc.9b00153
Jones, A. M., Maclean, D., Studholme, D. J., Serna-Sanz, A., Andreasson, E., Rathjen, J. P., et al. (2009). Phosphoproteomic analysis of nuclei-enriched fractions from Arabidopsis thaliana. J. Proteom. 72, 439–451. doi: 10.1016/j.jprot.2009.02.004
Jung, H.-J., Kayum, M. A., Thamilarasan, S. K., Nath, U. K., Park, J.-I., Chung, M.-Y., et al. (2017). Molecular characterisation and expression profiling of calcineurin B-like (CBL) genes in Chinese cabbage under abiotic stresses. Funct. Plant Biol. 44, 739–750. doi: 10.1071/FP16437
Kakar, K. U., Nawaz, Z., Kakar, K., Ali, E., Almoneafy, A. A., Ullah, R., et al. (2017). Comprehensive genomic analysis of the CNGC gene family in Brassica oleracea: novel insights into synteny, structures, and transcript profiles. BMC Genom. 18:1–18. doi: 10.1186/s12864-017-4244-y
Kidokoro, S., Yoneda, K., Takasaki, H., Takahashi, F., Shinozaki, K., and Yamaguchi-Shinozaki, K. (2017). Different cold-signaling pathways function in the responses to rapid and gradual decreases in temperature. Plant Cell 29, 760–774. doi: 10.1105/tpc.16.00669
Kim, Y. S., An, C., Park, S., Gilmour, S. J., Wang, L., Renna, L., et al. (2017). CAMTA-mediated regulation of salicylic acid immunity pathway genes in Arabidopsis exposed to low temperature and pathogen infection. Plant Cell 29, 2465–2477. doi: 10.1105/tpc.16.00865
Kim, Y., Park, S., Gilmour, S. J., and Thomashow, M. F. (2013). Roles of CAMTA transcription factors and salicylic acid in configuring the low-temperature transcriptome and freezing tolerance of A rabidopsis. Plant J. 75, 364–376. doi: 10.1111/tpj.12205
Kolukisaoglu, U., Weinl, S., Blazevic, D., Batistic, O., and Kudla, J. (2004). Calcium sensors and their interacting protein kinases: genomics of the Arabidopsis and rice CBL-CIPK signaling networks. Plant Physiol. 134, 43–58. doi: 10.1104/pp.103.033068
Kong, X., Lv, W., Jiang, S., Zhang, D., Cai, G., Pan, J., et al. (2013). Genome-wide identification and expression analysis of calcium-dependent protein kinase in maize. BMC Genom. 14:1–15. doi: 10.1186/1471-2164-14-433
Kudla, J., Becker, D., Grill, E., Hedrich, R., Hippler, M., Kummer, U., et al. (2018). Advances and current challenges in calcium signaling. New Phytol. 218, 414–431. doi: 10.1111/nph.14966
Kurepin, L. V., Dahal, K. P., Savitch, L. V., Singh, J., Bode, R., Ivanov, A. G., et al. (2013). Role of CBFs as integrators of chloroplast redox, phytochrome and plant hormone signaling during cold acclimation. Int. J. Mol. Sci. 14, 12729–12763. doi: 10.3390/ijms140612729
Kurusu, T., Kuchitsu, K., Nakano, M., Nakayama, Y., and Iida, H. (2013). Plant mechanosensing and Ca2+ transport. Trends Plant Sci. 18, 227–233. doi: 10.1016/j.tplants.2012.12.002
Laohavisit, A., Shang, Z., Rubio, L., Cuin, T. A., Véry, A.-A., Wang, A., et al. (2012). Arabidopsis annexin1 mediates the radical-activated plasma membrane Ca2+-and K+-permeable conductance in root cells. Plant Cell 24, 1522–1533. doi: 10.1105/tpc.112.097881
Lee, H. G., and Seo, P. J. (2015). The MYB 96–HHP module integrates cold and abscisic acid signaling to activate the CBF–COR pathway in Arabidopsis. Plant J. 82, 962–977. doi: 10.1111/tpj.12866
Lee, S., Lee, E. J., Yang, E. J., Lee, J. E., Park, A. R., Song, W. H., et al. (2004). Proteomic identification of annexins, calcium-dependent membrane binding proteins that mediate osmotic stress and abscisic acid signal transduction in Arabidopsis. Plant Cell 16, 1378–1391. doi: 10.1105/tpc.021683
Li, C., Meng, D., Zhang, J., and Cheng, L. (2019). Genome-wide identification and expression analysis of calmodulin and calmodulin-like genes in apple (Malus× domestica). Plant Physiol. Biochem. 139, 600–612. doi: 10.1016/j.plaphy.2019.04.014
Li, H., Jiang, X., Lv, X., Ahammed, G. J., Guo, Z., Qi, Z., et al. (2019). Tomato GLR3. 3 and GLR3. 5 mediate cold acclimation-induced chilling tolerance by regulating apoplastic H2O2 production and redox homeostasis. Plant Cell Environ. 42, 3326–3339. doi: 10.1111/pce.13623
Li, M., Hu, W., Ren, L., Jia, C., Liu, J., Miao, H., et al. (2020). Identification, expression, and interaction network analyses of the CDPK gene family reveal their involvement in the development, ripening, and abiotic stress response in Banana. Biochem. Genet. 58, 40–62. doi: 10.1007/s10528-019-09916-2
Liu, Q., Ding, Y., Shi, Y., Ma, L., Wang, Y., Song, C., et al. (2021). The calcium transporter ANNEXIN1 mediates cold-induced calcium signaling and freezing tolerance in plants. EMBO J. 40:e104559. doi: 10.15252/embj.2020104559
Liu, X., Wang, J., and Sun, L. (2018). Structure of the hyperosmolality-gated calcium-permeable channel OSCA1. 2. Nat. Commun. 9, 1–9. doi: 10.1038/s41467-018-07564-5
Liu, Y., Dang, P., Liu, L., and He, C. (2019). Cold acclimation by the CBF–COR pathway in a changing climate: lessons from arabidopsis thaliana. Plant Cell Rep. 38, 511–519. doi: 10.1007/s00299-019-02376-3
Liu, Y., Xu, C., Zhu, Y., Zhang, L., Chen, T., Zhou, F., et al. (2018). The calcium-dependent kinase OsCPK24 functions in cold stress responses in rice. J. Integrat. Plant Biol. 60, 173–188. doi: 10.1111/jipb.12614
Luan, S., Kudla, J. R., Rodriguez-Concepcion, M., Yalovsky, S., and Gruissem, W. (2002). Calmodulins and calcineurin B–like proteins: calcium sensors for specific signal response coupling in plants. Plant Cell 14, S389–S400. doi: 10.1105/tpc.001115
Luo, Q., Wei, Q., Wang, R., Zhang, Y., Zhang, F., He, Y., et al. (2018). Ectopic expression of BdCIPK31 confers enhanced low-temperature tolerance in transgenic tobacco plants. Acta Biochim. Biophys. Sinica 50, 199–208. doi: 10.1093/abbs/gmx140
Ma, Q., Zhou, Q., Chen, C., Cui, Q., Zhao, Y., Wang, K., et al. (2019). Isolation and expression analysis of CsCML genes in response to abiotic stresses in the tea plant (Camellia sinensis). Sci. Rep. 9, 1–9. doi: 10.1038/s41598-019-44681-7
Ma, X., Gai, W.-X., Qiao, Y.-M., Ali, M., Wei, A.-M., Luo, D.-X., et al. (2019). Identification of CBL and CIPK gene families and functional characterization of CaCIPK1 under Phytophthora capsici in pepper (Capsicum annuum L.). BMC Genom. 20:1–18. doi: 10.1186/s12864-019-6125-z
Ma, Y., Dai, X., Xu, Y., Luo, W., Zheng, X., Zeng, D., et al. (2015). COLD1 confers chilling tolerance in rice. Cell 160, 1209–1221. doi: 10.1016/j.cell.2015.01.046
Meyerhoff, O., Müller, K., Roelfsema, M. R. G., Latz, A., Lacombe, B., Hedrich, R., et al. (2005). AtGLR3. 4, a glutamate receptor channel-like gene is sensitive to touch and cold. Planta 222, 418–427. doi: 10.1007/s00425-005-1551-3
Midhat, U., Ting, M. K., Teresinski, H. J., and Snedden, W. A. (2018). The calmodulin-like protein, CML39, is involved in regulating seed development, germination, and fruit development in Arabidopsis. Plant Mol. Biol. 96, 375–392. doi: 10.1007/s11103-018-0703-3
Miura, K., and Tada, Y. (2014). Regulation of water, salinity, and cold stress responses by salicylic acid. Front. Plant Sci. 5:4. doi: 10.3389/fpls.2014.00004
Mo, C., Wan, S., Xia, Y., Ren, N., Zhou, Y., and Jiang, X. (2018). Expression patterns and identified protein-protein interactions suggest that cassava CBL-CIPK signal networks function in responses to abiotic stresses. Front. Plant Sci. 9:269. doi: 10.3389/fpls.2018.00269
Mohanta, T. K., Mohanta, N., Mohanta, Y. K., Parida, P., and Bae, H. (2015). Genome-wide identification of Calcineurin B-Like (CBL) gene family of plants reveals novel conserved motifs and evolutionary aspects in calcium signaling events. BMC Plant Biol. 15:1–15. doi: 10.1186/s12870-015-0543-0
Mori, K., Renhu, N., Naito, M., Nakamura, A., Shiba, H., Yamamoto, T., et al. (2018). Ca 2+-permeable mechanosensitive channels MCA1 and MCA2 mediate cold-induced cytosolic Ca 2+ increase and cold tolerance in Arabidopsis. Sci. Rep. 8, 1–10. doi: 10.1038/s41598-017-17483-y
Munir, S., Liu, H., Xing, Y., Hussain, S., Ouyang, B., Zhang, Y., et al. (2016). Overexpression of calmodulin-like (ShCML44) stress-responsive gene from Solanum habrochaites enhances tolerance to multiple abiotic stresses. Sci. Rep. 6, 1–20. doi: 10.1038/srep31772
Nawaz, Z., Kakar, K. U., Saand, M. A., and Shu, Q.-Y. (2014). Cyclic nucleotide-gated ion channel gene family in rice, identification, characterization and experimental analysis of expression response to plant hormones, biotic and abiotic stresses. BMC Genom. 15:1–18. doi: 10.1186/1471-2164-15-853
Nawaz, Z., Kakar, K. U., Ullah, R., Yu, S., Zhang, J., Shu, Q.-Y., et al. (2019). Genome-wide identification, evolution and expression analysis of cyclic nucleotide-gated channels in tobacco (Nicotiana tabacum L.). Genomics 111, 142–158. doi: 10.1016/j.ygeno.2018.01.010
Niu, L., Dong, B., Song, Z., Meng, D., and Fu, Y. (2018). Genome-wide identification and characterization of CIPK family and analysis responses to various stresses in apple (Malus domestica). Int. J. Mol. Sci. 19:2131. doi: 10.3390/ijms19072131
Pandey, G. K., and Sanyal, S. K. (2021). Ca 2+-ATPase and Ca 2+/cation antiporters in Functional Dissection of Calcium Homeostasis and Transport Machinery in Plants. Berlin: Springer, 89–104.
Perochon, A., Aldon, D., Galaud, J.-P., and Ranty, B. (2011). Calmodulin and calmodulin-like proteins in plant calcium signaling. Biochimie 93, 2048–2053. doi: 10.1016/j.biochi.2011.07.012
Pirayesh, N., Giridhar, M., Khedher, A. B., Vothknecht, U. C., and Chigri, F. (2021). Organellar calcium signaling in plants: an update. Mol. Cell Res. 2021:118948. doi: 10.1016/j.bbamcr.2021.118948
Poovaiah, B., Du, L., Wang, H., and Yang, T. (2013). Recent advances in calcium/calmodulin-mediated signaling with an emphasis on plant-microbe interactions. Plant Physiol. 163, 531–542. doi: 10.1104/pp.113.220780
Ranty, B., Aldon, D., Cotelle, V., Galaud, J.-P., Thuleau, P., and Mazars, C. (2016). Calcium sensors as key hubs in plant responses to biotic and abiotic stresses. Front. Plant Sci. 7:327. doi: 10.3389/fpls.2016.00327
Reddy, A., Reddy, V. S., and Golovkin, M. (2000). A calmodulin binding protein from Arabidopsis is induced by ethylene and contains a DNA-binding motif. Biochem. Biophys. Res. Commun. 279, 762–769. doi: 10.1006/bbrc.2000.4032
Reddy, V. S., and Reddy, A. S. (2004). Proteomics of calcium-signaling components in plants. Phytochemistry 65, 1745–1776. doi: 10.1016/j.phytochem.2004.04.033
Richards, S. L., Laohavisit, A., Mortimer, J. C., Shabala, L., Swarbreck, S. M., Shabala, S., et al. (2014). Annexin 1 regulates the H 2 O 2-induced calcium signature in A rabidopsis thaliana roots. Plant J. 77, 136–145. doi: 10.1111/tpj.12372
Sanders, D., Pelloux, J., Brownlee, C., and Harper, J. F. (2002). Calcium at the crossroads of signaling. Plant Cell 14, S401–S417.
Sarwat, M., Ahmad, P., Nabi, G., and Hu, X. (2013). Ca2+ signals: the versatile decoders of environmental cues. Crit. Rev. Biotechnol. 33, 97–109. doi: 10.3109/07388551.2012.672398
Scott, I. M., Clarke, S. M., Wood, J. E., and Mur, L. A. (2004). Salicylate accumulation inhibits growth at chilling temperature in Arabidopsis. Plant Physiol. 135, 1040–1049. doi: 10.1104/pp.104.041293
Shi, Y., Ding, Y., and Yang, S. (2018). Molecular regulation of CBF signaling in cold acclimation. Trends Plant Sci. 23, 623–637. doi: 10.1016/j.tplants.2018.04.002
Stael, S., Wurzinger, B., Mair, A., Mehlmer, N., Vothknecht, U. C., and Teige, M. (2012). Plant organellar calcium signalling: an emerging field. J. Exp. Bot. 63, 1525–1542. doi: 10.1093/jxb/err394
Stockinger, E. J., Gilmour, S. J., and Thomashow, M. F. (1997). Arabidopsis thaliana CBF1 encodes an AP2 domain-containing transcriptional activator that binds to the C-repeat/DRE, a cis-acting DNA regulatory element that stimulates transcription in response to low temperature and water deficit. Proc. Nat. Acad. Sci. 94, 1035–1040. doi: 10.1073/pnas.94.3.1035
Su, W., Zhang, C., Wang, D., Ren, Y., Sun, T., Feng, J., et al. (2021). The CaCA superfamily genes in Saccharum: Comparative analysis and their functional implications in response to biotic and abiotic stress. BMC Genom. 22:1–19. doi: 10.1186/s12864-021-07828-3
Sun, Q., Huang, R., Zhu, H., Sun, Y., and Guo, Z. (2021). A novel Medicago truncatula calmodulin-like protein (MtCML42) regulates cold tolerance and flowering time. Plant J. 108, 1069–1082. doi: 10.1111/tpj.15494
Tang, K., Zhao, L., Ren, Y., Yang, S., Zhu, J. K., and Zhao, C. (2020). The transcription factor ICE1 functions in cold stress response by binding to the promoters of CBF and COR genes. J. Integ. Plant Biol. 62, 258–263. doi: 10.1111/jipb.12918
Tang, M., Xu, C., Cao, H., Shi, Y., Chen, J., Chai, Y., et al. (2021). Tomato calmodulin-like protein SlCML37 is a calcium (Ca2+) sensor that interacts with proteasome maturation factor SlUMP1 and plays a role in tomato fruit chilling stress tolerance. J. Plant Physiol. 258:153373. doi: 10.1016/j.jplph.2021.153373
Thor, K. (2019). Calcium—Nutrient and messenger. Front. Plant Sci. 10:440. doi: 10.3389/fpls.2019.00440
Tian, W., Wang, C., Gao, Q., Li, L., and Luan, S. (2020). Calcium spikes, waves and oscillations in plant development and biotic interactions. Nat. Plants 6, 750–759. doi: 10.1038/s41477-020-0667-6
Townley, H. E., and Knight, M. R. (2002). Calmodulin as a potential negative regulator of ArabidopsisCOR gene expression. Plant Physiol. 128, 1169–1172. doi: 10.1104/pp.010814
Tuteja, N., and Mahajan, S. (2007). Calcium signaling network in plants: an overview. Plant Signal. Behav. 2, 79–85. doi: 10.4161/psb.2.2.4176
Valmonte, G. R., Arthur, K., Higgins, C. M., and Macdiarmid, R. M. (2014). Calcium-dependent protein kinases in plants: evolution, expression and function. Plant Cell Physiol. 55, 551–569. doi: 10.1093/pcp/pct200
Wang, H., Gong, J., Su, X., Li, L., Pang, X., and Zhang, Z. (2017). MaCDPK7, a calcium-dependent protein kinase gene from banana is involved in fruit ripening and temperature stress responses. J. Horticult. Sci. Biotechnol. 92, 240–250. doi: 10.1080/14620316.2016.1265902
Wang, J., Ren, Y., Liu, X., Luo, S., Zhang, X., Liu, X., et al. (2021). Transcriptional activation and phosphorylation of OsCNGC9 confer enhanced chilling tolerance in rice. Mol. Plant 14, 315–329. doi: 10.1016/j.molp.2020.11.022
Wang, L., Feng, X., Yao, L., Ding, C., Lei, L., Hao, X., et al. (2020a). Characterization of CBL–CIPK signaling complexes and their involvement in cold response in tea plant. Plant Physiol. Biochem. 154, 195–203. doi: 10.1016/j.plaphy.2020.06.005
Wang, L., Li, M., Liu, Z., Dai, L., Zhang, M., Wang, L., et al. (2020b). Genome-wide identification of CNGC genes in Chinese jujube (Ziziphus jujuba Mill.) and ZjCNGC2 mediated signalling cascades in response to cold stress. BMC Genom. 21:1–16. doi: 10.1186/s12864-020-6601-5
Wang, R.-K., Li, L.-L., Cao, Z.-H., Zhao, Q., Li, M., Zhang, L.-Y., et al. (2012). Molecular cloning and functional characterization of a novel apple MdCIPK6L gene reveals its involvement in multiple abiotic stress tolerance in transgenic plants. Plant Mol. Biol. 79, 123–135. doi: 10.1007/s11103-012-9899-9
Weckwerth, P., Ehlert, B., and Romeis, T. (2015). Zm CPK 1, a calcium-independent kinase member of the Z ea mays CDPK gene family, functions as a negative regulator in cold stress signalling. Plant Cell Environ. 38, 544–558. doi: 10.1111/pce.12414
Weiland, M., Mancuso, S., and Baluska, F. (2015). Signalling via glutamate and GLRs in Arabidopsis thaliana. Funct. Plant Biol. 43, 1–25. doi: 10.1071/FP15109
Xiao, P., Feng, J.-W., Zhu, X.-T., and Gao, J. (2021). Evolution analyses of CAMTA transcription factor in plants and its enhancing effect on cold-tolerance. Front. Plant Sci. 2021:12. doi: 10.3389/fpls.2021.758187
Xiong, T.-C., Bourque, S., Lecourieux, D., Amelot, N., Grat, S., Bričre, C., et al. (2006). Calcium signaling in plant cell organelles delimited by a double membrane. Biochim. Biophys. Acta 1763, 1209–1215. doi: 10.1016/j.bbamcr.2006.09.024
Xu, L., Zahid, K. R., He, L., Zhang, W., He, X., Zhang, X., et al. (2013). GhCAX3 gene, a novel Ca2+/H+ exchanger from cotton, confers regulation of cold response and ABA induced signal transduction. PLoS One 8:e66303. doi: 10.1371/journal.pone.0066303
Xu, X., Liu, M., Lu, L., He, M., Qu, W., Xu, Q., et al. (2015). Genome-wide analysis and expression of the calcium-dependent protein kinase gene family in cucumber. Mol. Genet. Genom. 290, 1403–1414. doi: 10.1007/s00438-015-1002-1
Xu, Y., Li, H., Lin, J., Li, X., and Chang, Y. (2015). Isolation and characterization of Calcineurin B-like gene (PbCBL1) and its promoter in birch-leaf pear (Pyrus betulifolia Bunge). Genet. Mol. Res. 14, 16756–16770. doi: 10.4238/2015.December.14.3
Yang, J., Liu, S., Ji, L., Tang, X., Zhu, Y., and Xie, G. (2020). Identification of novel OsCML16 target proteins and differential expression analysis under abiotic stresses in rice. J. Plant Physiol. 249:153165. doi: 10.1016/j.jplph.2020.153165
Yang, T., and Poovaiah, B. (2000). An early ethylene up-regulated gene encoding a calmodulin-binding protein involved in plant senescence and death. J. Biol. Chem. 275, 38467–38473. doi: 10.1074/jbc.M003566200
Yang, T., Chaudhuri, S., Yang, L., Du, L., and Poovaiah, B. (2010a). A calcium/calmodulin-regulated member of the receptor-like kinase family confers cold tolerance in plants. J. Biol. Chem. 285, 7119–7126. doi: 10.1074/jbc.M109.035659
Yang, T., Shad Ali, G., Yang, L., Du, L., Reddy, A., and Poovaiah, B. (2010b). Calcium/calmodulin-regulated receptor-like kinase CRLK1 interacts with MEKK1 in plants. Plant Signal. Behav. 5, 991–994. doi: 10.4161/psb.5.8.12225
Yu, Q., An, L., and Li, W. (2014). The CBL–CIPK network mediates different signaling pathways in plants. Plant Cell Rep. 33, 203–214. doi: 10.1007/s00299-013-1507-1
Yuan, P., Tanaka, K., Du, L., and Poovaiah, B. (2018a). Calcium signaling in plant autoimmunity: a guard model for AtSR1/CAMTA3-mediated immune response. Mol. Plant 11, 637–639. doi: 10.1016/j.molp.2018.02.014
Yuan, P., Yang, T., and Poovaiah, B. (2018b). Calcium signaling-mediated plant response to cold stress. Int. J. Mol. Sci. 19:3896. doi: 10.3390/ijms19123896
Zelman, A. K., Dawe, A., Berkowitz, G. A., and Gehring, C. (2012). Evolutionary and structural perspectives of plant cyclic nucleotide-gated cation channels. Front. Plant Sci. 3:95. doi: 10.3389/fpls.2012.00095
Zeng, H., Xu, L., Singh, A., Wang, H., Du, L., and Poovaiah, B. (2015). Involvement of calmodulin and calmodulin-like proteins in plant responses to abiotic stresses. Front. Plant Sci. 6:600. doi: 10.3389/fpls.2015.00600
Zeng, H., Zhang, Y., Zhang, X., Pi, E., and Zhu, Y. (2017). Analysis of EF-hand proteins in soybean genome suggests their potential roles in environmental and nutritional stress signaling. Front. Plant Sci. 8:877. doi: 10.3389/fpls.2017.00877
Zhang, H., Wei, C., Yang, X., Chen, H., Yang, Y., Mo, Y., et al. (2017). Genome-wide identification and expression analysis of calcium−dependent protein kinase and its related kinase gene families in melon (Cucumis melo L.). PLoS One 12:e0176352. doi: 10.1371/journal.pone.0176352
Zhang, H., Yang, B., Liu, W.-Z., Li, H., Wang, L., Wang, B., et al. (2014). Identification and characterization of CBL and CIPK gene families in canola (Brassica napus L.). BMC Plant Biol. 14:1–24. doi: 10.1186/1471-2229-14-8
Zhang, L., Du, L., Shen, C., Yang, Y., and Poovaiah, B. (2014). Regulation of plant immunity through ubiquitin-mediated modulation of Ca2+–calmodulin–A t SR 1/CAMTA 3 signaling. Plant J. 78, 269–281. doi: 10.1111/tpj.12473
Zhao, C., Wang, P., Si, T., Hsu, C.-C., Wang, L., Zayed, O., et al. (2017). MAP kinase cascades regulate the cold response by modulating ICE1 protein stability. Dev. Cell 43, 618–629. doi: 10.1016/j.devcel.2017.09.024
Zhao, P., Liu, Y., Kong, W., Ji, J., Cai, T., and Guo, Z. (2021). Genome-wide identification and characterization of calcium-dependent protein kinase (CDPK) and CDPK-Related Kinase (CRK) Gene Families in Medicago truncatula. Int. J. Mol. Sci. 22:1044. doi: 10.3390/ijms22031044
Zheng, Y., Luo, L., Wei, J., Chen, Q., Yang, Y., Hu, X., et al. (2018). The glutamate receptors AtGLR1. 2 and AtGLR1. 3 increase cold tolerance by regulating jasmonate signaling in Arabidopsis thaliana. Biochem. Biophys. Res. Commun. 506, 895–900. doi: 10.1016/j.bbrc.2018.10.153
Keywords: calcium, calmodulin, CAMTA, cold stress, transcription factor
Citation: Iqbal Z, Memon AG, Ahmad A and Iqbal MS (2022) Calcium Mediated Cold Acclimation in Plants: Underlying Signaling and Molecular Mechanisms. Front. Plant Sci. 13:855559. doi: 10.3389/fpls.2022.855559
Received: 15 January 2022; Accepted: 31 March 2022;
Published: 27 April 2022.
Edited by:
Yingfang Zhu, Henan University, ChinaReviewed by:
Takuya Furuichi, Hagoromo International University, JapanCopyright © 2022 Iqbal, Memon, Ahmad and Iqbal. This is an open-access article distributed under the terms of the Creative Commons Attribution License (CC BY). The use, distribution or reproduction in other forums is permitted, provided the original author(s) and the copyright owner(s) are credited and that the original publication in this journal is cited, in accordance with accepted academic practice. No use, distribution or reproduction is permitted which does not comply with these terms.
*Correspondence: Mohammed Shariq Iqbal, c2hhcmlxMDkwM0BnbWFpbC5jb20=
Disclaimer: All claims expressed in this article are solely those of the authors and do not necessarily represent those of their affiliated organizations, or those of the publisher, the editors and the reviewers. Any product that may be evaluated in this article or claim that may be made by its manufacturer is not guaranteed or endorsed by the publisher.
Research integrity at Frontiers
Learn more about the work of our research integrity team to safeguard the quality of each article we publish.