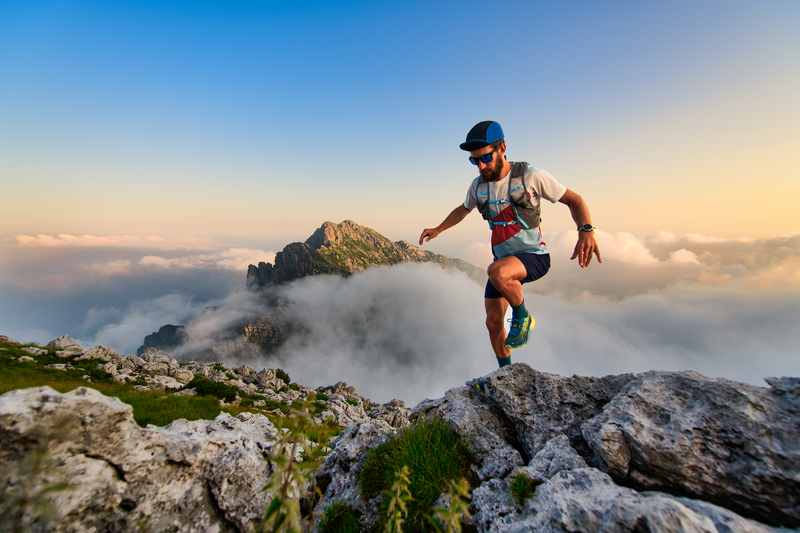
95% of researchers rate our articles as excellent or good
Learn more about the work of our research integrity team to safeguard the quality of each article we publish.
Find out more
ORIGINAL RESEARCH article
Front. Plant Sci. , 24 March 2022
Sec. Plant Abiotic Stress
Volume 13 - 2022 | https://doi.org/10.3389/fpls.2022.854899
This article is part of the Research Topic Hormonal Control of Plant Stress Responses: Brassinosteroids and Gibberellin View all 7 articles
Plant hormone brassinosteroids (BRs) play key roles in plant adaptation to biotic stresses, including various pathogen infections. As a core factor in BR signaling, the transcription factor BRI1-EMS-SUPPRESSOR 1 (BES1) activates BR responses via regulating the expression of target genes. However, the molecular mechanism of BRs in regulating plant immunity is unclear, and the key components are not identified. In this study, we found that BR biosynthesis and signaling transduction are essential for plant resistance to pathogen infection, and BR biosynthesis or BR signaling-deficient mutants displayed susceptibility to Pseudomonas syringae pv. tomato DC3000 (Pst DC3000) infection [including more serious symptoms and more photosystem II (PSII) photochemistry damage]. We identified a callose synthase gene GLUCAN SYNTHASE-LIKE 8 (GSL8) as a direct target of BES1, and its expression was induced by BRs/BES1. Meanwhile, BRs induced callose accumulation after Pst DC3000 infection. Moreover, BES1 gain-of-function mutant bes1-D showed promoted Pst DC3000 resistance. GSL8 T-DNA insertion mutant gsl8-1 was susceptible to DC3000, while brassinolide (BL) treatment partially rescued gsl8-1 susceptible phenotypes. Our study suggests that BR-induced pathogen resistance partly depends on the BR-induced BES1-GSL8 cascade to mediate callose accumulation.
One-upmanship competition between plants and pathogens has been going on for millions of years. Sessile plants have evolved a dynamic defense regulatory network to survive from pathogen attacks. The plant rapidly activates defense response after perceiving pathogen attack (Robert-Seilaniantz et al., 2011). There are two key interconnected branches in plant immunity (Jones and Dangl, 2006); at first, pathogen-associated molecular patterns (PAMPs) or host-derived damage-associated molecular patterns are perceived by pattern recognition receptors (PRRs) which can lead to pattern-triggered immunity (PTI) (Zipfel, 2014), which can resist most of the attacks. However, pathogens have evolved an ability that delivers effectors to suppress PTI (Chisholm et al., 2006; Jones and Dangl, 2006; Goehre and Robatzek, 2008). In response, plants have acquired resistance (R) genes that can recognize these attacker-specific effectors, resulting in effector-triggered immunity (ETI) (Chisholm et al., 2006; Jones and Dangl, 2006). Phytohormones play essential roles during pathogen infections. Salicylic acid (SA), jasmonic acid (JA), ethylene (ET), cytokinin (CK), abscisic acid (ABA), gibberellic acid (GA), auxins, and brassinosteroids (BRs) are known to primarily regulate the basal defense responses (Nemhauser et al., 2006), and the hormone signaling works synergistically or antagonistically in plant–microbe interactions (Verhage et al., 2010).
Brassinosteroids, a kind of plant steroid hormones, play essential roles during plant growth and development such as hypocotyl and petiole elongation, leaf senescence, vascular development, and stress response (Clouse, 1996; Yang et al., 2011; Nolan et al., 2017). BR signaling pathway is well understood. BRs are perceived by receptor kinase BR INSENSITIVE 1 (BRI1) and co-receptor BRI1-ASSOCIATED KINASE 1 (BAK1) (Li and Chory, 1997; Li et al., 2002; Nam and Li, 2002; Hothorn et al., 2011; She et al., 2011; Gou et al., 2012). Then, BR signaling is transmitted from the plasma membrane to nuclear by other components, including BRI1 SUPPRESSOR 1 (BSU1), BRASSINOSTEROID-INSENSITIVE 2 (BIN2), BRASSINAZOLE-RESISTANT 1/BRI1-EMS-SUPPRESSOR 1 (BES1/BZR1), MYB-LIKE 2 (MYBL2), HOMEODOMAIN-LEUCINE ZIPPER PROTEIN 1 (HAT1), UPBEAT 1 (UPB1), and GOLDEN2-LIKE 1 (GLK1) to regulate up to 4,000–5,000 gene expressions (Yin et al., 2002; He et al., 2005; Sun et al., 2010; Kim et al., 2011; Yu et al., 2011; Ye et al., 2012; Zhang et al., 2014, 2021; Li et al., 2020). Several studies have been indicated that BRs participate in the pathogen defense process (Heese et al., 2007; Albrecht et al., 2012; Belkhadir et al., 2012). A previous study demonstrated that the MITOGEN-ACTIVATED PROTEIN KINASE 6 (MEK6) phosphorylates BES1 to enhance plant immunity (Kang et al., 2015). BAK1 plays as a partner of FLAGELLIN SENSING 2 (FLS2) or PEPTIDE 1 RECEPTORS (PEPRs) to function in PTI (Chinchilla et al., 2007; Sun et al., 2013). RECEPTOR-LIKE CYTOPLASMIC KINASES (RLCK) group VII members, BR-SIGNALING KINASE 1 (BSK1) and BSK5, play essential roles in PTI (Shi et al., 2013; Majhi et al., 2019; Zhao et al., 2019; Wang et al., 2020). Recent studies have also found important roles of BRs in the plant antivirus process (Deng et al., 2015, 2016; Zhang et al., 2015). However, the molecular mechanism of BRs in regulating plant immunity is unclear, and the key component is not identified.
Callose is a β-(1,3)-D-glucan polymer, and callose deposition is a typical PTI response (Ellinger and Voigt, 2014; Voigt, 2016). Callose deposits on the site of pathogen infection to restrict the ingression of pathogen-secreted cell wall-degrading enzymes (Stone, 2009). Callose is involved in various plant developmental processes and stress responses, and its biosynthesis is regulated by the family of GLUCAN SYNTHASE-LIKE (GSL) genes (Ellinger and Voigt, 2014). After pathogens attack, callose is deposited between the plasma membrane and the cell wall (Nishimura et al., 2003). A recent study indicates that GSL6 and GSL4 are bona fide callose synthases required for SA-dependent and reactive oxygen species (ROS)-dependent plasmodesmata regulation, respectively (Cui and Lee, 2016). BR-enhanced plant immunity was accompanied by increased callose accumulation (Xiong et al., 2020). However, BR enhances plant immunity via inducing callose accumulation that lacks direct evidence. To gain more insight into BR-activated plant immunity, we investigated the effects of BR-induced callose accumulation on pathogen resistance. We identified a key component GLUCAN SYNTHASE-LIKE 8 (GSL8), which was a direct target of BES1, and its expression was promoted by BRs/BES1. BR-induced pathogen resistance correlated with callose enrichment, and GSL8 played key roles in BR-mediated resistance against Pseudomonas syringae pv. tomato DC3000 (Pst DC3000).
The Arabidopsis transgenic and mutant plants BRI1OX, DWF4OX, det2, bes1-D, BES1-RNAi, and gsl8-1 are in Col-0 background, and the DET2OX is in Wassilewskija (WS) background. GSL8 T-DNA insertion mutant gsl8-1 (SALK_111094) was obtained from Arabidopsis Biological Resource Center (ABRC), and the details were described previously (Chen et al., 2009). Arabidopsis plants used in the study were sterilized using 70% (v/v) ethanol and 0.1% (v/v) Triton X-100, plated on 1/2 Murashige and Skoog (1/2 MS) medium, vernalized at 4°C for 2 days in the dark, were incubated for 6 h in the light (150 μmol m–2 s–1) at 22°C for germination, and then grown under a long-day condition (22°C, 16-h light/8-h dark).
The Arabidopsis leaves were pretreated by BL or BRZ (1 μM with 0.02% Tween 20) at 12 h before infection. Pst DC3000 or Pst hrcC was cultured on the solid King’s B (KB) medium (peptone 20 g/L, glycerin 10 ml/L, K2HPO4 1.5 g/L, MgSO4⋅7H2O 1.5 g/L, and rifampicin 50 mg/L) at 28°C for 24–48 h. Bacteria were scraped off the plates and suspended in 10 mM MgCl2 to OD600 of 0.02, inoculating 10 mM MgCl2 without bacteria as mock (Choi et al., 2010), photographed at 3 and 5 days postinoculation (dpi). Pathogen growth analyzed at 1 dpi, and 0.8 cm2 leaf disks were taken by puncher, washed by 15% H2O2 for 3 min, and then washed with sterile distilled H2O. The leaf disks were then continuously diluted by water and plated onto the KB medium.
The analysis of chlorophyll fluorescence was described previously (Deng et al., 2015). In brief, chlorophyll fluorescence was determined with an imaging pulse amplitude-modulated fluorometer (IMAG-MINI, Heinz Walz, Germany). For the measurement of Fv/Fm, plants were dark-adapted for 30 min. Minimal fluorescence (Fo) was measured during the weak measuring pulses, and maximal fluorescence (Fm) was measured by a 0.8-s pulse of light at about 4,000 μmol m–2 s–1. An actinic light source was then applied to obtain steady-state fluorescence yield (Fs), after which a second saturation pulse was applied for 0.7 s to obtain light-adapted maximum fluorescence (Fm′). Fv/Fm and non-photochemical quenching (NPQ) were calculated as Fm−Fo/Fm and (Fm/Fm′)–1, respectively.
For superoxide staining, leaves were stained by nitroblue tetrazolium (NBT; 0.5 mg/ml) for 2 h and then soaked in boiling ethanol (95%) until the green color of the leaves faded. The Micro Superoxide Anion Assay Kit (BC1295, Solarbio, Beijing, China) was used to measure the superoxide content. The Catalase (CAT) Activity Assay Kit (BC0205, Solarbio), the Superoxide Dismutase (SOD) Activity Detection Kit (BC0170, Solarbio), the Peroxidase (POD) Activity Detection Kit (BC0090, Solarbio), and the Ascorbate Peroxidase (APX) Activity Assay Kit (BC0220, Solarbio) were used to detect the activity of CAT, SOD, POD, and APX, respectively.
Callose deposition staining was observed at 1 dpi; leaves were cleared by decolorizing solution (acetic acid:ethanol = 1:3) for 12 h, then washed by water, and stained by aniline blue (150 mM K2HPO4 (pH 9.5) supplemented with 0.01% aniline blue). Callose deposition was observed by fluorescence microscope with DAPI filter (MDG41, Leica). ImageJ software1 was used to count the number of callose depositions (Choi et al., 2010).
Total RNA was extracted by the Total RNA Extraction Kit (Solarbio). For quantitative reverse-transcription PCR (qRT-PCR), cDNA was prepared using PrimeScript™ RT Reagent Kit (Takara). Gene expression was performed using the SYBR Green PCR Master Mix (Invitrogen). The CFX Connect Real-Time System (Bio-Rad, Hercules, CA, United States) was used for the qRT-PCR analysis. For each sample, three replicates were performed, and the expression levels were normalized to those of ACTIN2. The primers used for qRT-PCR are listed in Supplementary Table 1.
Chromatin immunoprecipitation (ChIP) assays were performed as previously described (Zou et al., 2019). In brief, 4-week-old Col-0 was cross-linked with formaldehyde, and 125 mM glycine stopped the reaction. Chromatin was sonicated to produce approximately 0.3 kbp DNA fragments. The sonicated protein-DNA complexes were precipitated with an anti-BES1 antibody. After incubation with protein A beads, the beads were further washed with low salt and high salt buffer and reverse cross-linked with 200 mM NaCl. After removing proteins with proteinase K, DNA fragments were purified by phenol-chloroform extraction and ethanol precipitation. The DNA fragments were dissolved in TE buffer (10 mM Tris–HCl pH 8.0, 1 mM EDTA) and used as qPCR templates for the real-time system. TA3 fragment served as a normalization for the qPCR analysis.
Arabidopsis mesophyll cell protoplasts were prepared and transformed as described previously (Li et al., 2020). For luciferase (LUC) assays, we cloned the promoters of GSL8 into the pGreen II 0800 vector and the full-length coding sequence (CDS) of BES1 into the pCAMBIA1307. The constructs used in this study were mentioned in the previous studies (Li et al., 2020; Zhang et al., 2021). Plasmids were singly or co-transformed into Arabidopsis protoplasts. LUC activities were measured using a Luciferase Assay System (Promega) after 16 h, and the data were normalized to REN activity. The experiments were repeated three times with similar results.
Sequence data from this study can be found in the Arabidopsis Genome Initiative database under the following accession numbers: GSL8 (AT2G36850), BES1 (AT1G19350), BRI1 (AT4G39400), DWF4 (AT3G50660), and DET2 (AT2G38050).
The experimental data were statistically analyzed using three or more averages, using one-way ANOVA, and considered significant when P < 0.05.
To study the roles of BRs in plant–pathogen interactions, we examined the effect of brassinolide (BL; the most active BRs) or brassinazole (BRZ, a specific BR biosynthesis inhibitor) treatments on Pst DC3000 resistance in Arabidopsis. At 3 and 5 dpi, plants presented typical disease symptoms and chlorotic leaves (Ishiga et al., 2009). It spread more rapidly in treatment with 1 μM BRZ, while the application of 1 μM BL significantly enhanced Pst DC3000 resistance (Figures 1A,B). Then, we detected the pathogen accumulation in infected leaves at 1 dpi, and BL-treated plants showed less bacterial counts than mock and BRZ-treated plants (Figure 1C). Then, we used various BR biosynthesis genes and transgenic and mutant plants, including BR biosynthesis gene DWF4 overexpression transgenic line (DWF4OX), BR biosynthesis gene DET2 knock out mutant (det2), and DET2 overexpression transgenic line (DET2OX), to investigate the role of BR biosynthesis in plant immunity. BR biosynthesis-enhanced transgenic plants DWF4OX and DET2OX showed higher resistance to Pst DC3000 infection, while BR biosynthesis-deficient mutant det2 showed susceptibility to infection (Figure 1). It demonstrated that BRs enhance plant resistance to Pst DC3000.
Figure 1. Brassinosteroids (BRs) increase resistance to Pst DC3000. (A) Typical Pst DC3000 infection symptoms in Col-0, BL-treated, BRZ-treated, DWFOX, DET2OX, WS, and det2 plants. Pictures were taken at 3 and 5 dpi, respectively. Bar, 1.00 cm. (B) Total chlorophyll content in inoculated leaves was detected in planta at 3 dpi. (C) Bacterial growth in the inoculated leaves was detected in planta. Bacteria were isolated from plants at 1 dpi and quantified with gradient dilution assays. Bars represent mean ± SD obtained from three biological replicates per genotype and time point, chlorophyll content or bacterial growth measured from five leaves of each genotype and treatment were pooled for one replicate. Significant differences (P < 0.05) are denoted by different lowercase letters.
Two typical indicators of photosystem II (PSII) photochemistry activity, namely, Fv/Fm (the maximal quantum efficiency of PSII) and NPQ, were detected to test the degree of damage to the light system caused by bacterial inoculation. As shown in Figure 2, there were no significant differences in Fv/Fm (Figures 2A,B) and NPQ (Figures 2C,D) in unchallenged or Pst hrcC inoculated plants. On Pst DC3000 infection, compared with mock-treated wild-type plants, both Fv/Fm (Figures 2A,B) and NPQ (Figures 2C,D) decreased in all of the plants, but BRZ-treated and det2 plants decreased more. It indicated that BRs played a critical role in protecting plant photosystem against Pst DC3000 infection.
Figure 2. Brassinosteroids alleviate photosystem damage after Pst DC3000 infection. Images of the maximum photosystem II (PSII) quantum yield (Fv/Fm) (A) and non-photochemical quenching (NPQ)/4 (C) in the leaves infected by Pst DC3000 or Pst hrcC at 3 dpi. Bar, 1.00 cm. Average values of Fv/Fm (B) and NPQ/4 (D) for the respective chlorophyll fluorescence images. Fv/Fm or NPQ/4 measured from 8 to 10 leaves and three biological repeats. Significant differences (P < 0.05) are denoted by different lowercase letters.
Pathogen infection promotes the accumulation of ROS in plants (Deng et al., 2015). Then, we explored the effects of BRs on antioxidant systems when plants were incubated with Pst DC3000. We detected the accumulation of superoxide by NBT staining (Figure 3A) and quantified it by biochemical testing (Figure 3B). The accumulation of superoxide had no significant difference in unchallenged plants but increased after Pst DC3000 infection. The accumulation of superoxide was higher in BL-treated, DWF4OX, and DET2OX plants but lower in BRZ-treated and det2 plants. The enzyme activity of several antioxidative enzymes, such as SOD, POD, CAT, and APX, and the relative expression levels of defense-related genes (PR1 and PR2) were also detected. Pst DC3000 infection increased the activities of all these antioxidative enzymes (Figures 3C–F) and relative expression levels of defense-related genes (Figures 3G,H), and the increase was higher in BL-treated and BR biosynthesis-enhanced transgenic plants and lower in BRZ-treated and BR biosynthesis-deficient mutant plants. All these results illustrated that BRs-induced defense of Pst DC3000 was related to the antioxidant system.
Figure 3. Antioxidant system induced by BRs after infection. (A) Nitroblue tetrazolium (NBT) staining for observing superoxide accumulation at 1 dpi. (B) The superoxide content in the infected leaves at 1 dpi. Bar, 1.00 cm. The activities of the antioxidant enzymes superoxide dismutase (SOD) (C), peroxidase (POD) (D), catalase (CAT) (E), and ascorbate peroxidase (APX) (F). Bars represent mean ± SD obtained from three biological replicates per genotype and time point, superoxide content or the activities of the antioxidant enzymes measured from five leaves of each genotype and treatment were pooled for one replicate. (G,H) Relative expression levels of defense-related genes PR1 and PR2. The expression of ACTIN2 was used as an internal reference. Data presented are mean ± SD from three independent experiments. Significant differences (P < 0.05) are denoted by different lowercase letters.
The induction of callose deposition indicates the activation of basal defenses. As Pst DC3000 suppressed callose deposition, to understand the nature of resistance induced by BL, callose deposition was observed at the Pst hrcC infection leaves. When infected with Pst hrcC, compared with wild-type plants, BRZ-treated and BR biosynthesis-deficient mutant showed significantly lower levels of callose deposition, while BL-treated and BR biosynthesis-enhanced transgenic plants accumulated a higher number of callose deposition in leaves (Figures 4A,B). It indicated that BRs induced callose accumulation against Pst DC3000 infection.
Figure 4. Brassinosteroids induce callose deposition after infection. (A) Callose deposition in infected leaves at 1 dpi. Callose deposition was visualized by fluorescence microscopy. Bar, 200 μm. (B) Number of callose deposition in the 5.5 mm2 microscopic fields. Callose deposition was counted in 8–12 microscopic fields of 5.5 mm2 from 8 to 12 different leaves and three biological repeats. Significant differences (P < 0.05) are denoted by different lowercase letters.
To further investigate the roles of BRs in plant disease resistance, we analyzed the effects of different BR signaling components and transgenic and mutant plants in Pst DC3000 resistance. BR receptor BRI1 overexpression transgenic line (BRI1OX), BES1 gain-of-function mutant (bes1-D), and BES1 RNA interference transgenic line (BES1-RNAi) were used in the future study. BRI1OX and bes1-D displayed increased Pst DC3000 resistance, including fewer disease symptoms (Figures 5A,B), less bacterial accumulation (Figure 5C), alleviated photosystem damage (Figures 6A–D), enhanced antioxidant system (Figures 7A–F), and increased defense-related gene expression (Figures 7G,H), while BES1-RNAi displayed the opposite. Then, we analyzed callose deposition in different transgenic and mutant plants after infection. As shown in Figures 8A,B, after infecting with Pst hrcC, BES1-RNAi showed significantly lower levels of callose deposition, while more callose accumulated in the leaves of BRI1OX and bes1-D. These results indicated that BR signaling positively regulated plant resistance to Pst DC3000.
Figure 5. Brassinosteroid signaling positively regulates plant defense. (A) Typical Pst DC3000 infection symptoms in Col-0, BRI1OX, bes1-D, and BES1-RNAi. Pictures were taken at 3 and 5 dpi, respectively. Bar, 1.00 cm. (B) Total chlorophyll content in inoculated leaves was detected in planta at 3 dpi. (C) Bacterial growth in the inoculated leaves was detected in planta. Bacteria were isolated from plants at 1 dpi and quantified with gradient dilution assays. Bars represent mean ± SD obtained from three biological replicates per genotype and time point, chlorophyll content or bacterial growth measured from five leaves of each genotype and treatment were pooled for one replicate. Significant differences (P < 0.05) are denoted by different lowercase letters.
Figure 6. Brassinosteroid signaling enhances plant defense by alleviating photosystem damage. Images of the maximum PSII quantum yield (Fv/Fm) (A) and NPQ/4 (C) in the leaves infected by Pst DC3000 or Pst hrcC at 3 dpi. Bar, 1.00 cm. Average values of Fv/Fm (B) and NPQ/4 (D) for the respective chlorophyll fluorescence images. Fv/Fm or NPQ/4 measured from 8 to 10 leaves and three biological repeats. Significant differences (P < 0.05) are denoted by different lowercase letters.
Figure 7. Brassinosteroid signaling enhances the antioxidant system to defend against pathogens. (A) NBT staining for observing superoxide accumulation at 1 dpi in Col-0, BRI1OX, bes1-D, and BES1-RNAi. Bar, 1.00 cm. (B) The superoxide content in the infected leaves at 1 dpi. The activities of the antioxidant enzymes SOD (C), POD (D), CAT (E), and APX (F). Bars represent mean ± SD obtained from three biological replicates per genotype and time point, superoxide content or the activities of the antioxidant enzymes measured from five leaves of each genotype and treatment were pooled for one replicate. (G,H) Relative expression levels of defense-related genes PR1 and PR2. The expression of ACTIN2 was used as an internal reference. Data presented are mean ± SD from three independent experiments. Significant differences (P < 0.05) are denoted by different lowercase letters.
Figure 8. Brassinosteroid signaling induces callose deposition after infection. (A) Callose deposition in Col-0, BRI1OX, bes1-D, and BES1-RNAi infected leaves at 1 dpi. Callose deposition was visualized by fluorescence microscopy. Bar, 200 μm. (B) Number of callose deposition in the 5.5 mm2 microscopic fields. Callose deposition was counted in 8–12 microscopic fields of 5.5 mm2 from 8 to 12 different leaves and three biological repeats. Significant differences (P < 0.05) are denoted by different lowercase letters.
Previous ChIP-chip studies have shown that GSL8 was a direct target of BES1 and induced by BRs (Yu et al., 2011) (Arabidopsis eFP Browser2), and GSL8-deficient mutants had short hypocotyls (Chen et al., 2009) which are typical BR-deficient phenotypes. Thus, we hypothesized that GSL8 was a direct target of BES1 in BR-induced plant immunity. To confirm the result, qRT-PCR experiments were performed. The expression of GSL8 was increased in Col-0 seedlings after BL treatment. In addition, the expression of GSL8 increased to 294% without exogenous BL in bes1-D and even more increased with BL treatment (Figure 9A). Then, the ChIP experiments were performed using an anti-BES1 antibody to confirm whether GSL8 is a direct target of BES1. TA3, a retrotransposable element, was used as the internal control. In the promoter of GSL8, there are two putative BES1 binding fragments at the promoter of GSL8 (Figure 9B). Results of ChIP-qPCR showed that BES1 was enriched significantly at the A1 and A2 regions of GSL8 which contain a typical E-box (CANNTG). We then expressed GSL8 promoter:LUC reporter gene in tobacco leaves. When co-expressed with BES1, GSL8 pro:LUC gene expression was induced (Figures 9D,E). Taken together, our results demonstrated that GSL8 was a direct target of BES1, and its expression was induced by BES1.
Figure 9. GLUCAN SYNTHASE-LIKE 8 is a direct target of BES1. (A) Detection of GSL8 expression in Col-0 or bes1-D with/without BL treatment. Quantitative reverse-transcription PCR (qRT-PCR) was performed using 4-week-old plants treated with or without 1 μM BL. Data presented are mean ± SD from three independent experiments. (B) Schematic representation of GSL8 promoter. (C) Chromatin immunoprecipitation (ChIP)-quantitative PCR (qPCR) assay using 4-week-old Col-0 seedlings. TA3 was used as an internal control. Data presented are mean ± SD from three independent experiments. (D) Schematic diagrams of the reporters and effectors used in the transient transactivation assays. (E) Transient dual-luciferase (LUC) reporter assays show that BES1 enhances the promoter activity of GSL8. The relative LUC activities were calculated by normalizing the LUC values against Renilla LUC (REN). Data presented are mean ± SD from three independent experiments. Significant differences (P < 0.05) are denoted by different lowercase letters.
To further investigate the connection of BR-induced disease resistance and GSL8, a GSL8 T-DNA insertion mutant gsl8-1 was used for the follow-up experiments. We analyzed the effects of BL on Pst DC3000 resistance in Col-0, BL, gsl8-1, and BL + gsl8-1. As shown in Figures 10A,B, gsl8-1 mutant showed more obvious disease symptoms, after being treated with BL, the symptoms were relieved but still severer than BL-treated wild-type plants. Then, we detected bacterial growth in infected leaves. As the same as symptoms, bacterial counts in gsl8-1 were higher than wild-type. After being treated with BL, bacterial counts in gsl8-1 were obviously relieved but still higher than BL-treated wild-type plants (Figure 10C). We found BR-induced callose accumulation against Pst DC3000, and GSL8 is one of the callose synthases, whether the susceptibility of gsl8-1 is due to callose synthesis blocked? Thus, we detected the callose accumulation in Col-0 and gsl8-1 with/without BL treatment after being infected with Pst hrcC. Compared with Col-0, callose accumulation in gsl8-1 was decreased 38%, although increased 28% in BL + gsl8-1, and increased 58% in BL-treated wild-type plants (Figures 10D,E). Then, we detected the superoxide contents, antioxidative enzyme activities, and defense-related gene expressions in Col-0 and gsl8-1 with/without BL treatment after infection. Compared with Col-0, superoxide contents in gsl8-1 were significantly decreased to 19% (Figure 10F). Also, the antioxidative enzyme activities (Figures 10G–J) and defense-related gene expressions (Figures 10K,L) showed similar trends. These results suggested that BR-induced disease resistance partially depends on GSL8.
Figure 10. Brassinosteroid-induced disease resistance partially depends on GSL8. (A) Typical Pst DC3000 infection symptoms in Col-0, BL, gsl8-1, and BL + gsl8-1. Pictures were taken at 3 and 5 dpi, respectively. Bar, 1.00 cm. (B) Total chlorophyll content in inoculated leaves was detected in planta at 3 dpi. (C) Bacterial growth in the inoculated leaves was detected in planta. Bacteria were isolated from plants at 1 dpi and quantified with gradient dilution assays. (D) Callose deposition in infected leaves at 1 dpi. Callose deposition was visualized by fluorescence microscopy. Bar, 200 μm. (E) Number of callose deposition in the 5.5 mm2 microscopic fields. Callose deposition was counted in 8–12 microscopic fields of 5.5 mm2 from 8 to 12 different leaves and three biological repeats. (F) The superoxide content in the infected leaves at 1 dpi. The activities of the antioxidant enzymes SOD (G), POD (H), CAT (I), and APX (J). (K,L) Relative expression levels of defense-related genes PR1 and PR2. Data presented are mean ± SD from three independent experiments. The expression of ACTIN2 was used as an internal reference. Significant differences (P < 0.05) are denoted by different lowercase letters.
Plant immunity is regulated by a powerful and efficient phytohormone regulatory network (Pieterse et al., 2009), ET, JA, SA, ABA, CK, auxin, and BRs have been reported to positively or negatively regulate plant immunity (Pieterse et al., 2014). Previous studies have demonstrated that BAK1 leads to the initiation of innate immunity (Chinchilla et al., 2007; Heese et al., 2007), and BSK1 promotes disease resistance by phosphorylating a site in the N terminus of MAPK5 (Yan et al., 2018; Zhou and Zhang, 2020). Meanwhile, as a direct substrate of MEK6, BES1 plays a critical role in plant immunity (Kang et al., 2015). However, systematic research about the molecular mechanism of BRs in regulating plant immunity from BR biosynthesis to signaling perception and response is poor, and the downstream potential component still needs to be identified. In this study, we used various BR biosynthesis and signaling transgenic and mutant plants to study the mechanism of how BRs work in plant immunity. BL-treated and BR biosynthesis-enhanced transgenic plants DWF4OX and DET2OX displayed significantly enhanced plant resistance to Pst DC3000, and BRI1 overexpression transgenic plant BRI1OX and BES1 gain of function mutant bes1-D increased Pst DC3000 resistance, while BRZ-treated, det2, and BES1-RNAi showed reduced resistance (Figures 1, 5). All the data indicate that BRs are comprehensively involved in plant immunity comprehensively, from biosynthesis to signaling perception and response.
Biotic and abiotic stress often accompanies the production of ROS, which plays a critical role in stress responses. Recent studies indicate that BR-induced ROS accumulation enhances plant tolerance to abiotic stress, and BRs enhance virus resistance through MEK2-salicylic acid-induced protein kinase (SIPK) cascade and respiratory burstoxidase homolog B (RBOHB)-dependent ROS burst (Deng et al., 2015, 2016). In this study, we found that BRI1OX, DWF4OX, DET2OX, and bes1-D performed higher ROS accumulation after Pst DC3000 inoculation (Figures 3, 7), suggesting BRs induced Pst DC3000 resistance partially by stimulating the production of ROS.
As a core transcription factor in BR signaling, BES1 regulates plant growth and development by influencing BR-regulated gene expression (Nolan et al., 2020). Previous studies have revealed that BR antagonizes JA responses (He et al., 2020; Liao et al., 2020; Song et al., 2021). BES1 suppresses JA-induced transcription of PDF1.2s and indole-GS biosynthesis genes during pathogen infection and herbivore feeding (Liao et al., 2020), while Pst bacteria are able to synthesize the JA mimic coronatine, and our results reinforce the concept that BR antagonizes the JA responses. Callose is involved in various plant developmental processes and stress responses, and its biosynthesis is regulated by the family of GSL genes (Ellinger and Voigt, 2014), and several GSL genes are induced during plant immunity. BR-induced plant resistance to Pst DC3000 accompanied with callose accumulation (Figures 4, 8), whether BR directly regulates callose synthesis to enhance plant defense remains unknown. Our research found that GSL8 was a direct target of BES1 and its expression was induced by BRs/BES1 (Figure 9), GSL8-deficient mutant gsl8-1 showed a susceptible phenotype, and BR-induced callose accumulation in gsl8-1 was blocked (Figures 10A–E), indicating that GSL8-induced callose accumulation was important to BR-induced plant defense. Plants treated with BL rescued susceptible phenotype and callose deposition deficient in gsl8-1 but still lower than BL-treated wild-type plants (Figures 10A–E), suggesting that there may be other components take part in BR-induced plant defense. These results suggest that BR-induced pathogen resistance partly depends on the GSL8-mediated callose accumulation.
In summary, our research demonstrated a plant defense pathway mediated by BR signaling, and BR signaling is involved in plant immunity comprehensive, from biosynthesis to signaling perception and response. The core transcription factor BES1 positively regulates pathogen-induced callose accumulation via a glucan synthase gene GSL8. BR-induced pathogen resistance partly depends on the BR-induced BES1-GSL8 cascade to mediate callose accumulation.
The original contributions presented in the study are included in the article/Supplementary Material, further inquiries can be directed to the corresponding author.
JX and FY designed the research and wrote the manuscript. JX and XW performed most of the experiments with the assistance of LC. XX and MR contributed to the analytical tools. FY analyzed the data. MR and XX undertook most of the manuscript revisions. All authors contributed to the article and approved the submitted version.
This study was supported by grants from the Open Project Funding of the Key Laboratory of Southwest China Wildlife Resources Conservation (Ministry of Education) (XNYB18-01), the Open Project Funding of the State Key Laboratory of Crop Stress Adaptation and Improvement, the Open Project Funding of Sichuan Huitai Agriculture Technology Co. Ltd., (Huitaitech-001), and the Sichuan Science and Technology Program (2020YJ0468).
LC is employed by the Sichuan Huitai Agriculture Technology Co. Ltd.
The remaining authors declare that the research was conducted in the absence of any commercial or financial relationships that could be construed as a potential conflict of interest.
All claims expressed in this article are solely those of the authors and do not necessarily represent those of their affiliated organizations, or those of the publisher, the editors and the reviewers. Any product that may be evaluated in this article, or claim that may be made by its manufacturer, is not guaranteed or endorsed by the publisher.
We thank Wenqiang Tang (Hebei Normal University) for the BRI1OX and DWF4OX seeds and Jia Li (Lanzhou University) for the DET2OX seeds.
The Supplementary Material for this article can be found online at: https://www.frontiersin.org/articles/10.3389/fpls.2022.854899/full#supplementary-material
BRs, brassinosteroids; BL, brassinolide; BRZ, brassinazole; Pst, Pseudomonas syringae pv. tomato; GSL8, GLUCAN SYNTHASE-LIKE 8; BRI1, BR INSENSITIVE 1; BES1, BRI1-EMS-SUPPRESSOR 1; DWF4, DWARF 4; DET2, DEETIOLATED 2; PSII, photosystem II; NPQ, non-photochemical quenching; ROS, reactive oxygen species; NBT, nitroblue tetrazolium; SOD, superoxide dismutase; POD, peroxidase; CAT, catalase; APX, ascorbate peroxidase; qRT-PCR, quantitative reverse-transcription PCR; ChIP, chromatin immunoprecipitation.
Albrecht, C., Boutrot, F., Segonzac, C., Schwessinger, B., Gimenez-Ibanez, S., Chinchilla, D., et al. (2012). Brassinosteroids inhibit pathogen-associated molecular pattern-triggered immune signaling independent of the receptor kinase BAK1. Proc. Natl. Acad. Sci. U.S.A. 109, 303–308. doi: 10.1073/pnas.1109921108
Belkhadir, Y., Jaillais, Y., Epple, P., Balsemao-Pires, E., Dangl, J. L., and Chory, J. (2012). Brassinosteroids modulate the efficiency of plant immune responses to microbe-associated molecular patterns. Proc. Natl. Acad. Sci. U.S.A. 109, 297–302. doi: 10.1073/pnas.1112840108
Chen, X. Y., Liu, L., Lee, E., Han, X., Rim, Y., Chu, H., et al. (2009). The Arabidopsis callose synthase gene GSL8 is required for cytokinesis and cell patterning. Plant Physiol. 150, 105–113. doi: 10.1104/pp.108.133918
Chinchilla, D., Zipfel, C., Robatzek, S., Kemmerling, B., Nuernberger, T., Jones, J. D. G., et al. (2007). A flagellin-induced complex of the receptor FLS2 and BAK1 initiates plant defence. Nature 448, 497–500. doi: 10.1038/nature05999
Chisholm, S. T., Coaker, G., Day, B., and Staskawicz, B. J. (2006). Host-microbe interactions: shaping the evolution of the plant immune response. Cell 124, 803–814. doi: 10.1016/j.cell.2006.02.008
Choi, J., Huh, S. U., Kojima, M., Sakakibara, H., Paek, K. H., and Hwang, I. (2010). The Cytokinin-Activated Transcription Factor ARR2 Promotes Plant Immunity via TGA3/NPR1-Dependent Salicylic Acid Signaling in Arabidopsis. Dev. Cell 19, 284–295. doi: 10.1016/j.devcel.2010.07.011
Clouse, S. D. (1996). Molecular genetic studies confirm the role of brassinosteroids in plant growth and development. Plant J. 10, 1–8. doi: 10.1046/j.1365-313x.1996.10010001.x
Cui, W., and Lee, J. Y. (2016). Arabidopsis callose synthases CalS1/8 regulate plasmodesmal permeability during stress. Nat. Plants 2:16034. doi: 10.1038/nplants.2016.34
Deng, X. G., Zhu, T., Zhang, D. W., and Lin, H. H. (2015). The alternative respiratory pathway is involved in brassinosteroid-induced environmental stress tolerance in Nicotiana benthamiana. J. Exp. Bot. 66, 6219–6232. doi: 10.1093/jxb/erv328
Deng, X. G., Zhu, T., Zou, L. J., Han, X. Y., Zhou, X., Xi, D. H., et al. (2016). Orchestration of hydrogen peroxide and nitric oxide in brassinosteroid-mediated systemic virus resistance in Nicotiana benthamiana. Plant J. 85, 478–493. doi: 10.1111/tpj.13120
Ellinger, D., and Voigt, C. A. (2014). Callose biosynthesis in arabidopsis with a focus on pathogen response: what we have learned within the last decade. Ann. Bot. 114, 1349–1358. doi: 10.1093/aob/mcu120
Goehre, V., and Robatzek, S. (2008). Breaking the barriers: microbial effector molecules subvert plant immunity. Ann. Rev. Phytopathol. 46, 189–215. doi: 10.1146/annurev.phyto.46.120407.110050
Gou, X., Yin, H., He, K., Du, J., Yi, J., Xu, S., et al. (2012). Genetic Evidence for an Indispensable Role of Somatic Embryogenesis Receptor Kinases in Brassinosteroid Signaling. PLoS Genet. 8:e1002452. doi: 10.1371/journal.pgen.1002452
He, J. X., Gendron, J. M., Sun, Y., Gampala, S. S. L., Gendron, N., Sun, C. Q., et al. (2005). BZR1 is a transcriptional repressor with dual roles in brassinosteroid homeostasis and growth responses. Science 307, 1634–1638. doi: 10.1126/science.1107580
He, Y., Hong, G., Zhang, H., Tan, X., Li, L., Kong, Y., et al. (2020). The OsGSK2 Kinase Integrates Brassinosteroid and Jasmonic Acid Signaling by Interacting with OsJAZ4. Plant Cell 32, 2806–2822. doi: 10.1105/tpc.19.00499
Heese, A., Hann, D. R., Gimenez-Ibanez, S., Jones, A. M. E., He, K., Li, J., et al. (2007). The receptor-like kinase SERK3/BAK1 is a central regulator of innate immunity in plants. Proc. Natl. Acad. Sci. U.S.A. 104, 12217–12222. doi: 10.1073/pnas.0705306104
Hothorn, M., Belkhadir, Y., Dreux, M., Dabi, T., Noel, J. P., Wilson, I. A., et al. (2011). Structural basis of steroid hormone perception by the receptor kinase BRI1. Nature 474, 467–471. doi: 10.1038/nature10153
Ishiga, Y., Uppalapati, S. R., Ishiga, T., Elavarthi, S., Martin, B., and Bender, C. L. (2009). The phytotoxin coronatine induces light-dependent reactive oxygen species in tomato seedlings. New Phytol. 181, 147–160. doi: 10.1111/j.1469-8137.2008.02639.x
Kang, S., Yang, F., Li, L., Chen, H., Chen, S., and Zhang, J. (2015). The Arabidopsis Transcription Factor brassinosteroid insensitive1-ethyl methanesulfonate-suppressor1 Is a Direct Substrate of MITOGEN-ACTIVATED PROTEIN KINASE6 and Regulates Immunity. Plant Physiol. 167, 1076–1086. doi: 10.1104/pp.114.250985
Kim, T. W., Guan, S., Burlingame, A. L., and Wang, Z. Y. (2011). The CDG1 Kinase Mediates Brassinosteroid Signal Transduction from BRI1 Receptor Kinase to BSU1 Phosphatase and GSK3-like Kinase BIN2. Mol. Cell 43, 561–571. doi: 10.1016/j.molcel.2011.05.037
Li, J., Wen, J. Q., Lease, K. A., Doke, J. T., Tax, F. E., and Walker, J. C. (2002). BAK1, an Arabidopsis LRR receptor-like protein kinase, interacts with BRI1 and modulates brassinosteroid signaling. Cell 110, 213–222. doi: 10.1016/s0092-8674(02)00812-7
Li, J. M., and Chory, J. (1997). A putative leucine-rich repeat receptor kinase involved in brassinosteroid signal transduction. Cell 90, 929–938. doi: 10.1016/s0092-8674(00)80357-8
Li, T., Lei, W., He, R., Tang, X., Han, J., Zou, L., et al. (2020). Brassinosteroids regulate root meristem development by mediating BIN2-UPB1 module in Arabidopsis. PLoS Genet. 16:e1008883. doi: 10.1371/journal.pgen.1008883
Liao, K., Peng, Y. J., Yuan, L. B., Dai, Y. S., Chen, Q. F., Yu, L. J., et al. (2020). Brassinosteroids Antagonize Jasmonate-Activated Plant Defense Responses through BRI1-EMS-SUPPRESSOR1 (BES1). Plant Physiol. 182, 1066–1082. doi: 10.1104/pp.19.01220
Majhi, B. B., Sreeramulu, S., and Sessa, G. (2019). BRASSINOSTEROID-SIGNALING KINASE5 Associates with Immune Receptors and Is Required for Immune Responses. Plant Physiol. 180, 1166–1184. doi: 10.1104/pp.18.01492
Nam, K. H., and Li, J. M. (2002). BRI1/BAK1, a receptor kinase pair mediating brassinosteroid signaling. Cell 110, 203–212. doi: 10.1016/s0092-8674(02)00814-0
Nemhauser, J. L., Hong, F., and Chory, J. (2006). Different plant hormones regulate similar processes through largely nonoverlapping transcriptional responses. Cell 126, 467–475. doi: 10.1016/j.cell.2006.05.050
Nishimura, M. T., Stein, M., Hou, B. H., Vogel, J. P., Edwards, H., and Somerville, S. C. (2003). Loss of a callose synthase results in salicylic acid-dependent disease resistance. Science 301, 969–972. doi: 10.1126/science.1086716
Nolan, T. M., Brennan, B., Yang, M., Chen, J., Zhang, M., Li, Z., et al. (2017). Selective Autophagy of BES1 Mediated by DSK2 Balances Plant Growth and Survival. Dev. Cell 41, 33–46. doi: 10.1016/j.devcel.2017.03.013
Nolan, T. M., Vukašinović, N., Liu, D., Russinova, E., and Yin, Y. (2020). Brassinosteroids: multidimensional Regulators of Plant Growth, Development, and Stress Responses. Plant Cell 32, 295–318. doi: 10.1105/tpc.19.00335
Pieterse, C. M. J., Leon-Reyes, A., Van Der Ent, S., and Van Wees, S. C. M. (2009). Networking by small-molecule hormones in plant immunity. Nat. Chem. Biol. 5, 308–316. doi: 10.1038/nchembio.164
Pieterse, C. M. J., Zamioudis, C., Berendsen, R. L., Weller, D. M., Van Wees, S. C. M., and Bakker, P. A. H. M. (2014). Induced Systemic Resistance by Beneficial Microbes. Ann. Rev. Phytopathol. 52, 347–375.
Robert-Seilaniantz, A., Grant, M., and Jones, J. D. G. (2011). Hormone Crosstalk in Plant Disease and Defense: more Than Just jasmonate-salicylate Antagonism. Ann. Rev. Phytopathol. 49, 317–343.
She, J., Han, Z., Kim, T. W., Wang, J., Cheng, W., Chang, J., et al. (2011). Structural insight into brassinosteroid perception by BRI1. Nature 474, 471–472. doi: 10.1038/nature10178
Shi, H., Shen, Q., Qi, Y., Yan, H., Nie, H., Chen, Y., et al. (2013). BR-SIGNALING KINASE1 Physically Associates with FLAGELLIN SENSING2 and Regulates Plant Innate Immunity in Arabidopsis. Plant Cell 25, 1143–1157. doi: 10.1105/tpc.112.107904
Song, Y., Zhai, Y., Li, L., Yang, Z., Ge, X., Yang, Z., et al. (2021). BIN2 negatively regulates plant defence against Verticillium dahliae in Arabidopsis and cotton. Plant Biotechnol. J. 19, 2097–2112. doi: 10.1111/pbi.13640
Stone, B. A. (2009). “Chemistry of β-glucans,” in Chemistry, Biochemistry, and Biology of 1-3 beta glucans and Related Polysaccharides, (Eds) B. Antony, B. F. Geoffrey, A. S. Bruce. (Amsterdam: Elsevier), 5–46. doi: 10.1016/b978-0-12-373971-1.00002-9
Sun, Y., Fan, X. Y., Cao, D. M., Tang, W., He, K., Zhu, J. Y., et al. (2010). Integration of Brassinosteroid Signal Transduction with the Transcription Network for Plant Growth Regulation in Arabidopsis. Dev. Cell 19, 765–777. doi: 10.1016/j.devcel.2010.10.010
Sun, Y., Li, L., Macho, A. P., Han, Z., Hu, Z., Zipfel, C., et al. (2013). Structural Basis for flg22-Induced Activation of the Arabidopsis FLS2-BAK1 Immune Complex. Science 342, 624–628. doi: 10.1126/science.1243825
Verhage, A., Van Wees, S. C. M., and Pieterse, C. M. J. (2010). Plant Immunity: it’s the Hormones Talking, But What Do They Say? Plant Physiol. 154, 536–540. doi: 10.1104/pp.110.161570
Voigt, C. A. (2016). Cellulose/callose glucan networks: the key to powdery mildew resistance in plants? New Phytol. 212, 303–305. doi: 10.1111/nph.14198
Wang, W., Feng, B., Zhou, J. M., and Tang, D. (2020). Plant immune signaling: advancing on two frontiers. J. Integr. Plant Biol. 62, 2–24. doi: 10.1111/jipb.12898
Xiong, J., He, R., Yang, F., Zou, L., Yi, K., Lin, H., et al. (2020). Brassinosteroids are involved in ethylene-induced Pst DC3000 resistance in Nicotiana benthamiana. Plant Biol. 22, 309–316. doi: 10.1111/plb.13074
Yan, H., Zhao, Y., Shi, H., Li, J., Wang, Y., and Tang, D. (2018). BRASSINOSTEROID-SIGNALING KINASE1 Phosphorylates MAPKKK5 to Regulate Immunity in Arabidopsis. Plant Physiol. 176, 2991–3002. doi: 10.1104/pp.17.01757
Yang, C. J., Zhang, C., Lu, Y. N., Jin, J. Q., and Wang, X. L. (2011). The Mechanisms of Brassinosteroids’ Action: from Signal Transduction to Plant Development. Mol. Plant 4, 588–600. doi: 10.1093/mp/ssr020
Ye, H., Li, L., Guo, H., and Yin, Y. (2012). MYBL2 is a substrate of GSK3-like kinase BIN2 and acts as a corepressor of BES1 in brassinosteroid signaling pathway in Arabidopsis. Proc. Natl. Acad. Sci. U.S.A. 109, 20142–20147. doi: 10.1073/pnas.1205232109
Yin, Y. H., Wang, Z. Y., Mora-Garcia, S., Li, J. M., Yoshida, S., Asami, T., et al. (2002). BES1 accumulates in the nucleus in response to brassinosteroids to regulate gene expression and promote stem elongation. Cell 109, 181–191. doi: 10.1016/s0092-8674(02)00721-3
Yu, X., Li, L., Zola, J., Aluru, M., Ye, H., Foudree, A., et al. (2011). A brassinosteroid transcriptional network revealed by genome-wide identification of BESI target genes in Arabidopsis thaliana. Plant J. 65, 634–646. doi: 10.1111/j.1365-313X.2010.04449.x
Zhang, D., Tan, W., Yang, F., Han, Q., Deng, X., Guo, H., et al. (2021). A BIN2-GLK1 Signaling Module Integrates Brassinosteroid and Light Signaling to Repress Chloroplast Development in the Dark. Dev. Cell 56, 310–324. doi: 10.1016/j.devcel.2020.12.001
Zhang, D., Ye, H., Guo, H., Johnson, A., Zhang, M., Lin, H., et al. (2014). Transcription factor HAT1 is phosphorylated by BIN2 kinase and mediates brassinosteroid repressed gene expression in Arabidopsis. Plant J. 77, 59–70. doi: 10.1111/tpj.12368
Zhang, D. W., Deng, X. G., Fu, F. Q., and Lin, H. H. (2015). Induction of plant virus defense response by brassinosteroids and brassinosteroid signaling in Arabidopsis thaliana. Planta 241, 875–885. doi: 10.1007/s00425-014-2218-8
Zhao, Y., Wu, G., Shi, H., and Tang, D. (2019). RECEPTOR-LIKE KINASE 902 Associates with and Phosphorylates brassinosteroid-signaling kinase1 to Regulate Plant Immunity. Mol. Plant 12, 59–70. doi: 10.1016/j.molp.2018.10.008
Zhou, J. M., and Zhang, Y. (2020). Plant Immunity: danger Perception and Signaling. Cell 181, 978–989. doi: 10.1016/j.cell.2020.04.028
Zipfel, C. (2014). Plant pattern-recognition receptors. Trends Immunol. 35, 345–351. doi: 10.1016/j.it.2014.05.004
Keywords: brassinosteroids, Pst DC3000, Arabidopsis thaliana, plant immunity, callose deposition
Citation: Xiong J, Wan X, Ran M, Xu X, Chen L and Yang F (2022) Brassinosteroids Positively Regulate Plant Immunity via BRI1-EMS-SUPPRESSOR 1-Mediated GLUCAN SYNTHASE-LIKE 8 Transcription. Front. Plant Sci. 13:854899. doi: 10.3389/fpls.2022.854899
Received: 14 January 2022; Accepted: 14 February 2022;
Published: 24 March 2022.
Edited by:
Junbo Du, Sichuan Agricultural University, ChinaReviewed by:
Kai Shu, Northwestern Polytechnical University, ChinaCopyright © 2022 Xiong, Wan, Ran, Xu, Chen and Yang. This is an open-access article distributed under the terms of the Creative Commons Attribution License (CC BY). The use, distribution or reproduction in other forums is permitted, provided the original author(s) and the copyright owner(s) are credited and that the original publication in this journal is cited, in accordance with accepted academic practice. No use, distribution or reproduction is permitted which does not comply with these terms.
*Correspondence: Feng Yang, eWZlbmcxOTg3QDEyNi5jb20=
†These authors have contributed equally to this work
Disclaimer: All claims expressed in this article are solely those of the authors and do not necessarily represent those of their affiliated organizations, or those of the publisher, the editors and the reviewers. Any product that may be evaluated in this article or claim that may be made by its manufacturer is not guaranteed or endorsed by the publisher.
Research integrity at Frontiers
Learn more about the work of our research integrity team to safeguard the quality of each article we publish.