- 1Department of Agriculture Technology, Faculty of Agriculture, Universiti Putra Malaysia, Serdang, Malaysia
- 2Laboratory of Agronomy and Sustainable Crop Protection, Institute of Plantation Studies, Universiti Putra Malaysia, Serdang, Malaysia
- 3Department of Biochemistry, Faculty of Biotechnology and Biomolecular Sciences, Universiti Putra Malaysia, Serdang, Malaysia
Inflictions caused by cold stress can result in disastrous effects on the productivity and survival of plants. Cold stress response in plants requires crosstalk between multiple signaling pathways including cold, heat, and reactive oxygen species (ROS) signaling networks. CBF, MYB, bHLH, and WRKY families are among the TFs that function as key players in the regulation of cold stress response at the molecular level. This review discusses some of the latest understanding on the regulation of expression and the mechanistic actions of plant TFs to address cold stress response. It was shown that the plant response consists of early and late responses as well as memory reprogramming for long-term protection against cold stress. The regulatory network can be differentiated into CBF-dependent and independent pathways involving different sets of TFs. Post-transcriptional regulation by miRNAs, control during ribosomal translation process, and post-translational regulation involving 26S proteosomic degradation are processes that affect the cellular abundance of key regulatory TFs, which is an important aspect of the regulation for cold acclimation. Therefore, fine-tuning of the regulation by TFs for adjusting to the cold stress condition involving the dynamic action of protein kinases, membrane ion channels, adapters, and modifiers is emphasized in this review.
Introduction
Abiotic stresses such as low temperature, drought, and high salinity are complex quantitative traits where numerous stress-responsive genes including transcription factors (TFs) take part to ensure the survival of plants. The low temperature adversely affects plant growth and yield and significantly reduces crop efficiency. The TFs play a pivotal role in regulating eukaryotic gene expression, and several TFs families in plants are involved in regulating the expression of cold stress-responsive genes (Wang et al., 2020a). These TFs bind to the promoter regions of these genes to achieve a coordinated and effective response for cold acclimation. Depending on the types of TFs, different mechanisms are employed, thus affecting the selectivity and expression levels of the genes being regulated.
Plants respond differently for tolerance to low temperatures (0–15 degrees Celsius) and frost (below zero degrees Celsius). Adaptation to low temperatures is achieved by exposure to short-term cold, a process known as cold tolerance. Therefore, the cold tolerance can be defined as the ability of a plant to withstand low temperatures to prevent the stress from damaging its tissues (Ding et al., 2019). This process is associated with biochemical and physiological changes that will eventually lead to dramatic changes in gene expression, membrane lipid status, cell water uptake, membrane fluidity, and small molecules accumulation. Cold adaptation will increase the plant’s tolerance to physical and physicochemical changes when exposed to freezing temperatures. Plants in the tropical and subtropical regions however lack cold resistance mechanisms and they will suffer irreversible damage in the face of low temperatures (Knight and Knight, 2012). Plant resistance to low temperatures has a complex mechanism that involves different metabolic pathways in diverse cellular segments. Several genes have been identified to be involved in cold stress response, including genes that encode TFs, phosphatases, and kinases (Sun et al., 2018).
Plants show different reactions to cold stress where stress signaling pathways play a key role at the molecular and cellular levels. Signal detection, signal transmission, and stress response are three stages of signal transduction in plants when they receive stress stimuli. Possible mechanisms for coping with cold stress include binding of TFs to the cis-element in the promoter of cold stress-responsive genes, interactions of TFs with auxiliary proteins to facilitate their functions, regulation of reactive oxygen species (ROS), and signal transduction (Kumar et al., 2021). The stress response phase begins with phosphorylation and dephosphorylation events regulating the expression of these TF genes for protection at the cellular level. In addition, the expression of the TFs is regulated by microRNAs (miRNA) and their presence in the cells are subjected to translational and post-translational regulation. The different mechanisms will be described and discussed in the different sections of this review.
The TFs that are key players in plant response to cold stress including C-repeat Binding Factor (CBF)/Dehydration-responsive element-binding protein (DREB), Myeloblastosis (MYB), Basic helix–loop–helix (bHLH), NAC (NAM-ATAF1,2-CUC2), WRKY, and Basic leucine zipper (bZIP) gene families (Zhao et al., 2018) are shown in Table 1. The structures and activities of CBF/DREB, MYB, bHLH, and WRKY as well as their roles in regulating cold stress response are described in greater details as these TFs are mainly covered in this review.
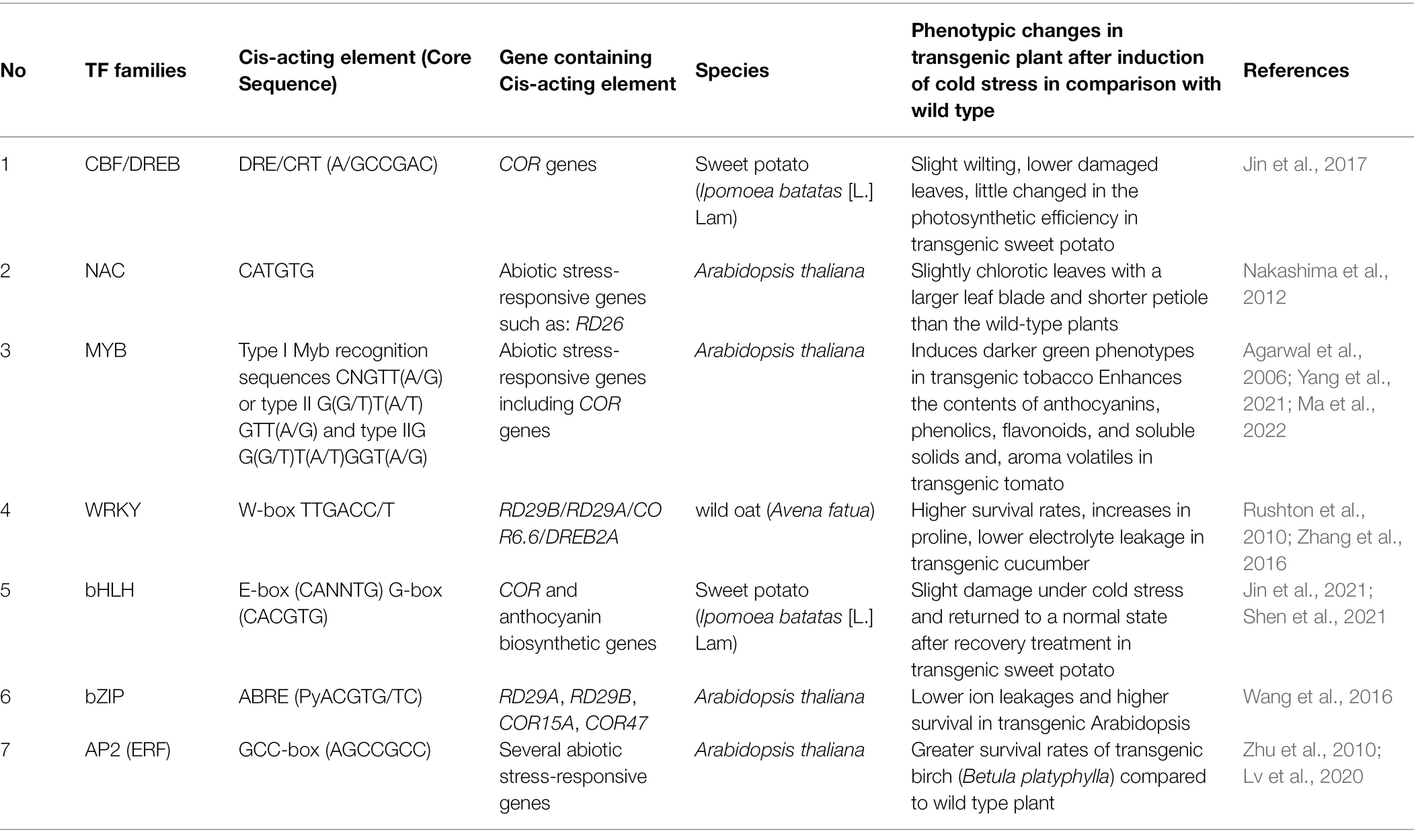
Table 1. Transcription factor, cis-acting elements, and the corresponding responsive genes that are involved in plant abiotic stress.
C-Repeat Binding Factor/Dehydration-Responsive Element-Binding Protein
Plant stress TFs can control a set of genes by binding specifically to the cis-regulatory elements in the target gene promoters. The products of these genes act as stress response proteins, thereby increasing the plant’s tolerance to stress. CBF/DREB TFs are a large subset of the AP2/ERF family that play a key role in the expression of stress-responsive genes in the ABA-independent pathway. This family of TFs plays a vital role in regulating plant growth and response to external environmental stresses (Chen et al., 2013). At low temperatures, CBF activates the expression of cold-regulated (COR) genes which encode key enzymes for osmolyte biosynthesis and other cold stress-responsive genes by binding to the DRE/CRT (A/GCCGAC) cis-regulatory element (Vazquez-Hernandez et al., 2017). This enhances freezing tolerance through the accumulation of cryoprotective proteins and soluble sugars that repair cold-hardened membranes and stabilize cellular osmotic potential (Shi et al., 2018b).
The most well-known pathways for understanding and responding to cold stress in plants are the ICE-CBF-COR pathway involving 12% of all cold-responsive genes. This pathway is stimulated by low temperature, and with the induction of multiple intermediates step by step, eventually increases the expression of downstream genes regulated by CBF (Wang et al., 2020a). ICE (Inducer of CBF Expression) genes are at the forefront of the cold adaptation process, which induce the expression of CBF genes. When plants experience non-freezing low temperatures, the CBF genes are activated rapidly, and subsequently, the expression of downstream target COR genes referred to as regulons of CBF is induced. Thus far, there are four CBFs found in plants, and they are known as CBF1, CBF2, CBF3, and CBF4 representing DREB1B, DREB1C, DREB1A, and DREB1D (Alves et al., 2017; Liu et al., 2019; Xie et al., 2021a). The CBF1, CBF2, and CBF3 involved in cold response are sequentially located on chromosome four of Arabidopsis (Shi et al., 2018b). Mutations made on the CBF genes through the CRISPR/Cas9 system demonstrated the triple CBF mutants were more at risk of freezing (Zhao et al., 2016). Thus, indicates CBF genes are critical for cold adaptation. Therefore, further studies on CBF/DREB TFs will increase our understanding of applying beneficial strategies to improve plant tolerance to cold stress.
Myeloblastosis
The MYB family is one of the most widespread plant TF families, containing above 100 members in Arabidopsis and rice involved in low temperature stress response. These TFs are divided into four different subtypes based on the structure of the DNA binding domain, which include 1R-MYB, R2R3-MYB, R1R2R3-MYB, and 4R-MYB (Su et al., 2014). This super family of proteins is involved in various processes such as cell cycle control, flower and seed development, primary and secondary metabolites regulation, hormonal signals, and biotic and abiotic stress responses (Li et al., 2015). Many studies have shown that the expression of MYB genes in response to cold and frost stress is dependent on the CBF/DREB pathway (Mehrotra et al., 2020). Transgenic plants that over-expressed MYB show enhanced expression of CBF genes with corresponding increase in tolerance to freezing stress before and after cold adaptation (Wang et al., 2014a).
MYB15 can be found in vegetative and reproductive organs of plants and plays a special role in regulating cold and salinity stress (Ding et al., 2009; Lindemose et al., 2013). MYB15 belongs to R2R3-MYB family of TFs in Arabidopsis that negatively regulates frost tolerance, which is brought about by its ability to repress the levels of expression of the CBF genes. MYB proteins are also involved in the interactions between cold, drought, and salinity stress responses (Li et al., 2015). Therefore, MYB proteins can be considered as an important regulator in plant responses to multiple abiotic stresses that can simultaneously control these abiotic stresses in higher plants. Current interest focuses on molecular mechanism associated with plant response to multiple stresses rather than focusing on a single type in isolation.
Basic Helix–Loop–Helix
One of the important families of TFs in eukaryotes is bHLH, which has completely different functions in animals and plants. In plants, this TF family is active in regulating the expression of genes involved in hormonal and optical signals, stomata development, flowering, flavonoid biosynthesis, and response to biotic and abiotic stresses, including cold stress (Mehrotra et al., 2020). The protein sequence of this TF shows the presence of two functional domains, the HLH and basic domains, which together contain 60 conserved amino acids. The HLH domain with two alpha helices separated by a loop located at the C-terminal of the TF allows the interaction with other subunits to form homodimer and heterodimer. The basic domain with 15 amino acids at the N-terminal facilitates in binding to the G-box and E-box in gene promoters (Jin et al., 2021).
Studies in Arabidopsis and rice demonstrated the presence of 167 and 177 bHLH genes, respectively (Mao et al., 2017). Although limited information is available on this protein family in plants, studies have shown the involvement of bHLH in responding to abiotic stresses, including cold tolerance. ICE1 and ICE2 encode MYC-type bHLH TFs consisting of 496 amino acid residues. ICE1 binds to the MYC cis-element (CANNTG) at the promoter of CBF1/2/3 to promote CBF genes transcription (Mehrotra et al., 2020; Sharma et al., 2020). NtbHLH123 transcription factor in tobacco is also a transcriptional activator that controls the expression of genes involved in ROS clearance, which led to the increase in cold tolerance (Zhao et al., 2018). In a study on sweet cherries, 66 bHLH genes were identified and most members of the PavbHLH family are associated with various processes in response to cold stress (Shen et al., 2021). The IbbHLH gene in sweet potato may also play a role in regulating cold stress, and IbbHLH79 was identified as a potential candidate for plant molecular breeding to increase cold tolerance in sweet potatoes (Jin et al., 2021). These findings show the importance of bHLH in cold stress response. However, more studies are needed to reveal cold and ROS signaling pathways regulated by bHLH in plants.
WRKY
The WRKY TF family in plants responds to a variety of abiotic stresses such as drought, salinity, and abnormal temperature. This family has undergone significant changes in higher plants during evolution, and the function of their genes has changed greatly from the original ancestral gene. Segmental and tandem duplications have also played a vital role in the development of this gene family. Previous reports indicate the essential role of WRKY TFs in managing low temperature stress. In tomatoes, for example, fragmentary duplication significantly contributed to the development of WRKY genes, and the expression of ten TFs from the WRKY family doubled during the period of cold stress (Chen et al., 2015). In a study on cucumber, over-expression of CsWRKY6 increased cold tolerance as well as sensitivity to ABA and proline accumulation (Zhang et al., 2016). Recently, transcriptomic analysis in Brassica napus identified several genes of the WRKY family that play a vital role in cold resilience (Ke et al., 2020). Studies involving cold-resistant and cold-sensitive peanuts suggested that members from the NAC, MYB, and WRKY TF families were jointly involved in cold tolerance, indicating the importance of crosstalk between signaling pathways involving different TF families in response to cold stress (Jiang et al., 2020). The findings show WRKY enhances plant defense response to cold stress through ABA signaling pathway. It would be valuable to delineate the mechanism for cooperative involvement of the different TFs in cold stress response.
Many of the TFs involved in regulating cold stress response also play a role in other abiotic stress response pathways such as heat and drought. This is expected as most of the stress conditions lead to similar cellular disturbance especially excessive accumulation of ROS with damaging effects on macromolecules including carbohydrates, proteins, lipids, and DNA. Each TF recognizes a specific regulatory motif found on the promoters of different stress-responsive genes enabling simultaneous alteration in their expression (either up- or downregulation). The detection of adverse environmental condition is conveyed through plant signaling cascades to the TFs in order to achieve the desired cellular responses to overcome the damaging effects from the external environment as elaborated in the following sections.
Transcriptional Reprogramming Involving Chromatin Modification and Heat Shock Factors
In response to cold stress, the production of ROS and NO mediates the alterations in chromatin structure and coordinates modification of histone and DNA methylation to activate the expression of stress-responsive genes (Kim, 2021; Tanpure et al., 2021). In eukaryotes, chromatin is organized as repeating subunits called nucleosome consisting of 147 bp DNA wrapped around a histone octamer containing four different histone proteins, H2A, H2B, H3, and H4 present in pairs. Loosening of the chromatin structure at a particular gene locus increases the accessibility of the transcriptional machinery and enhancing the transcriptional activity, which can be heritable and stably maintained between cell generations (Xie et al., 2021b). Histone acetyltransferase GENERAL CONTROL NON-REPRESSED PROTEIN5 (GCN5) catalyzes the acetylation of H3K9 and H3K14, which improves thermotolerance by activating heat stress-responsive genes, such as HEAT SHOCK TRANSCRIPTION FACTOR A3 (HSFA3), UV-HYPERSENSITIVE6 (UVH6), CHOLINE TRANSPORTER-LIKE 1 (CTL1), POLYGALACTURONASE INVOLVED IN EXPANSION3 (PGX3), and MYB54 (Hu et al., 2015; Zheng et al., 2019). The bivalent H3K4me3 and H3K27me3 marks found in active cold stress-responsive genes represent enhanced chromatin accessibility, which potentially allow access of proteins involved in transcriptional gene regulation (Zeng et al., 2019). The HIGH EXPRESSION OF OSMOTICALLY RESPONSIVE GENE15 (HOS15)-mediated chromatin modifications enable recruitment of CBF to COR gene promoters (Park et al., 2018).
The involvement of heat shock proteins (HSPs) in cold stress is still unclear compared to their action in heat stress. However, recent findings showed the role played by the HSPs (HSP90, HSP70, and smHSP) in controlling the intervention between heat and cold stresses in plants. Among HSPs, the HSP90 acts as a regulator of signal transfer to the nucleus, which is believed to be involved in reprogramming of transcriptional regulation of both stresses (Sohrabi et al., 2022). The regulation of HSPs expression is controlled by heat shock factors (HSFs). In plants, HSFs are categorized into three groups, the HSFA, HSFB, and HSFC. Among the three HSFs, the HSFA is involved in regulating HSPs expression and therefore activates multi-chaperone network. Under normal condition, the HSFA activity is negatively regulated by HSP90 while when responding to abiotic stress, the HSP90 is dissociated from the phospho-protein complex allowing interaction between HSFA and heat stress element (HSE) in the gene promoter region of many genes including those encoding antioxidants enzymes (Haq et al., 2019). The HSP90 was also reported to regulate cold stress response through resistance (R) proteins when pathogen attacks occurred under cold condition. This can be observed in Arabidopsis wherein HSP90 was found to be involved in the activation of RECOGNITION OF PERONOSPORA PARASITICA 4 (RPP4), one of the R genes in plants through SALICYLIC ACID GLUCOSYLTRANSFERASE 1-REQUIRED FOR Mla12 RESISTANCE 1-HEAT SHOCK PROTEIN 90 (SGT1-RAR1-HSP90) chaperone complex. The activity of SGT1-RAR1-HSP90 complex activated ENHANCED DISEASE SUSCEPTIBILITY 1 (EDSI)- and WRKY70-dependent cell death and defense response under cold condition (Bao et al., 2014). The findings show significant function of HSP90 in regulating cold signaling pathway even though under combined stresses of biotic and temperature stresses. In cold stress alone, increased HSP90 transcript was observed in Brassica napus (Krishna et al., 1995). However, in Glycine max, the downregulation of HSP90 was observed under cold condition compared to heat and salinity stress (Xu et al., 2013). This suggests the strong involvement of HSP90 in dehydration acclimation in G. max compared to cold stress. Recent findings in lentil further showed the involvement of other HSPs, the HSP70, HSP83, and HSP21 in mediating the cold response. Interestingly, these HSPs are also involved in heat stress response (Sohrabi et al., 2022). As cold and heat stress conditions do not occur concurrently in the plant, regulation of a specific HSP by HSF under cold and heat stress is potentially two independents events that assist plants to cope with abnormal temperature.
Stress memory programming at the transcriptional level is also important to enhance acclimation process toward cold stress in plants. This process involves the ability of primed plants to remember previous stress experience and therefore acquire them to enhance tolerance to similar or different stresses. It is a long-term effect that enables the plants to be protected from various stresses based on a previous encounter. In this process, the epigenetic modifications take place to mediate transcriptional memory, thus increasing plant adaptation to adverse temperatures (Xie et al., 2021b). As reported in Arabidopsis, the repetitive cold stress treatments reduced COR15A in resistance-improved plants. The reduction suggests the acclimation process, which could involve establishment of stress-induced H3K4me3 histone modification (Leuendorf et al., 2020). Increase in the expression of HISTONE DEACETYLASE 6 (HDA6) under cold stress in Arabidopsis had positively regulated freezing tolerance (Luo et al., 2017). Maize histone deacetylases (HDACs) may directly activate ZmDREB1 gene expression and histone hyperacetylation under cold stress (Yu et al., 2018; Ding et al., 2019). Meanwhile, the regulation of expression of COR genes (COR47 and COR15A) was reported to be regulated by HIGH EXPRESSION OF OSMOTICALLY RESPONSIVE GENE 15 (HOS15) and HISTONE DEACETYLASE 2C (HD2C) through direct binding to COR gene promoters (Park et al., 2018).
Early and Late Cold Stress Response by Transcription Factors
Modification of membrane proteins and activation of Ca2+ ion channels are plants primary responses when they sense cold stress from the environment. The chilling tolerance divergence 1 (COLD1) localized on plasma membrane and endoplasmic reticulum is the cold signal sensor that is responsible for receiving the cold signal from the environment (Figure 1). COLD1 gene encodes a regulator of G-protein signaling, which causes the G-protein α subunit to activate the guanosine triphosphatase (GTPase) activity of rice G-protein A subunit 1 (RGA1; Guo et al., 2018). Ma et al., 2015 showed that over-expression of japonica rice COLD1 enhanced chilling tolerance in rice compared to that of rice lines with downregulated expression of COLD 1. Once COLD1 receives the cold signal, elevation of cytosolic and nuclear Ca2+ occurs, and the plants activate calcium Ca2+ signaling pathway. Transportation of extracellular Ca2+ across plasma membrane through Ca2+ channels and/or Ca2+ pumps is triggered, increasing Ca2+ influx across plasma membrane (Yuan et al., 2018). The intracellular Ca2+ signal is then transduced through Ca2+ sensors, such as calmodulins (CaMs), CaM-like proteins (CMLs), Ca2+-dependent protein kinases (CPKs/CDPKs), and calcineurin B-like proteins (CBLs; Shi et al., 2018a). The Ca2+ sensors such as CaMs convey cold signal to TFs such as Calmodulin-Binding Transcription Activators (CAMTA). The promoter sequence of CAMTA genes possesses multiple cis-acting elements responsible for stress responses such as ABRE, SARE, G-box, W-box, AuXRE, DRE, and others (Noman et al., 2021), suggesting the potential interaction of cold-stressed responsive TFs with cis-acting elements of CAMTA. Upregulation of CBF2 in Arabidopsis was reported due to interaction of Arabidopsis AtCAMTA3 to CM2 motif located in the CBF2 promoter (Doherty et al., 2009). Kim et al., 2013 further reported the involvement of salicylic acid in inducing interaction between CAMTA1, CAMTA2, and CAMTA3 that further activated CBF1, CBF2, and CBF3 under cold stress. The decrement of salicylic acids was observed when the plants were exposed to warm temperature. Thus, CAMTAs play a critical role in the cold stress signaling cascade through binding to specific promoter motifs, which consequently influence the expression of CBF genes. However, based on the availability of multiple stress-responsive motifs located at CAMTAs promoters, multiples stress-responsive TFs can induce CAMTAs expression; therefore, accumulation of CAMTAs protein can enhance cold stress response through CBF-dependent signaling pathway. It further suggests the regulation of CBFs expression through CAMTAs other than that of ICE1 regulatory pathway.
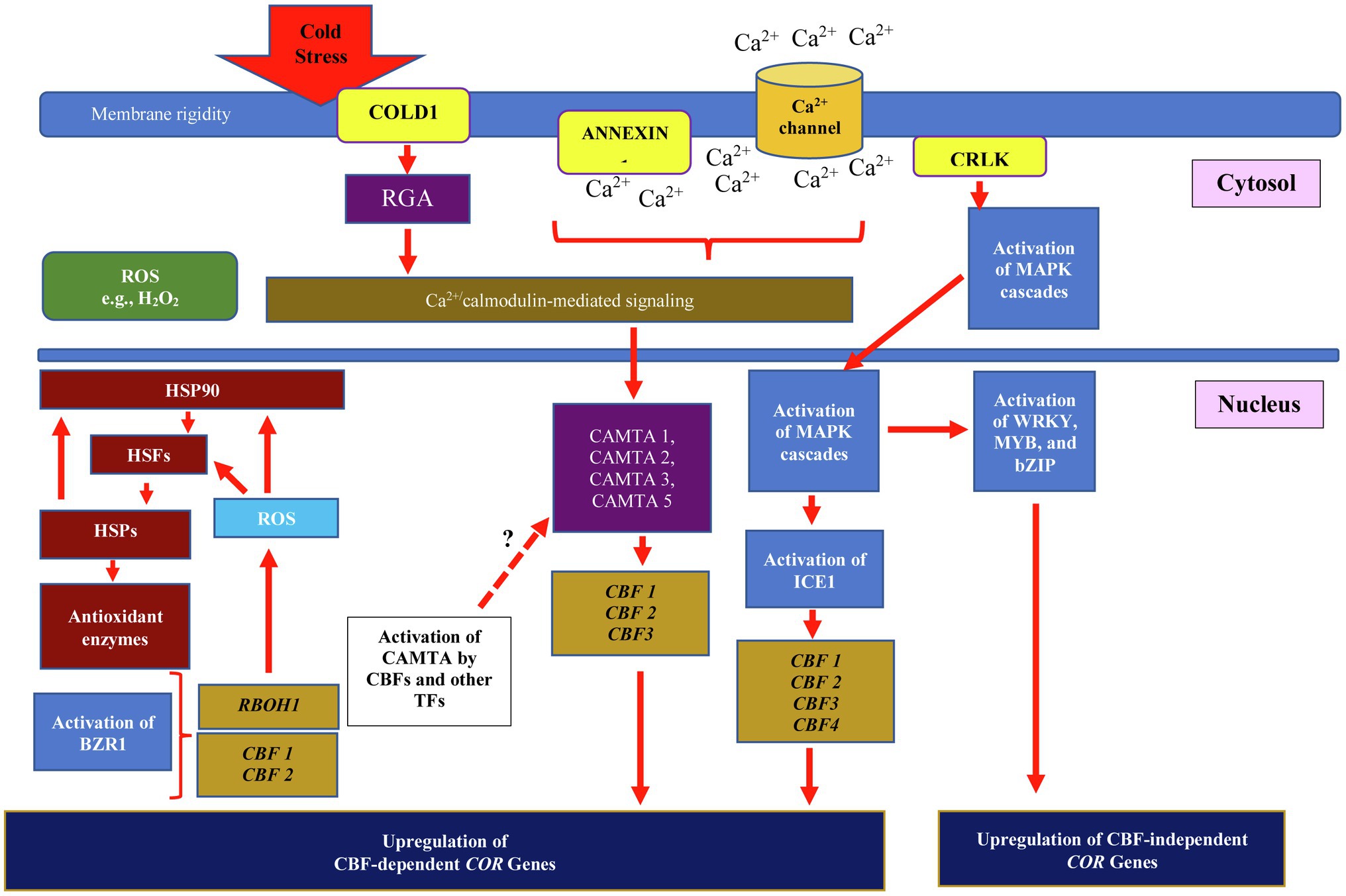
Figure 1. Integration of cold, heat, and ROS signaling in CBF-dependent and independent pathways for regulating plant cold stress response. Cold stress triggers changes in membrane fluidity and rigidity, and activates the expression of cold-regulated genes (CORs) through CBF-dependent and independent pathways. The chilling tolerance divergence 1 (COLD1) receives external cold stress signal and stimulates rice G-protein A subunit 1 (RGA1) activity. This further activates ANNEXIN1, the Ca2+ channels that transport Ca2+ into the cell. The COLD1/RGA activity and Ca2+ transmit cold stress signal to Ca2+/calmodulin-mediated signaling and Ca2+-dependent protein kinases (CDPKs) located in the cytosol. The CDPKs activities further convey cold stress signal through cytosolic and nuclear MAPKs signaling pathways, which leads to induction of ICE1 activity. ICE1 binds DRE/CRT motif in the promoter of CBF 1, 2, 3, and 4 and upregulates their expression. Integration of cold signal through Ca2+/calmodulin-mediated signaling activates interaction between CAMTAs and CM2 motif located in CBF promoter, which further upregulates COR genes expression. The presence of ABRE, SARE, G-box, W-box, AuXRE, and DRE motifs in CAMTAs promoters suggests the potential regulation of CAMTA by CBFs and other stress-responsive transcription factors (TFs). Accumulation of brassinosteroids promotes binding of BZR1 to E-box found in CBF1 or CBF2 genes and activates the expression of COR genes. Through CBF-independent pathway, the expression of CBF-independent COR genes is regulated through interaction of other TFs such as WRKY, MYB, and bZIP. The expression of CBF-independent COR genes could also be regulated through ROS signaling pathway, involving ROS produced by RESPIRATORY BURST OXIDASE HOMOLOG 1 (RBOH1). The ROS further regulate heat shock factors (HSFs) and heat shock protein 90 (HSP90) and hence develop multi-chaperone network that controls production of antioxidant enzymes. The multi-chaperone network is also involved in controlling HSP90 activity.
Recently, Ca2+-permeable transporter ANNEXIN1 (AtANN1) localized in plasma membrane was reported to be involved in mediating the accumulation of cytosolic Ca2+ in response to accumulation of ROS. In this study, the research group found that the activated protein kinase, Open Stomatal 1 (OST1), phosphorylated AtANN1 at Ser289, and therefore enhances the Ca2+ transport activity and amplifies the Ca2+ signaling to activate the expression of CBFs and CORs in the nucleus (Liu et al., 2021). Moreover, the Ca2+ signal transduction through calcium/calmodulin-regulated receptor-like kinases (CRLK) activates the perception of downstream regulatory and signaling pathway through phosphorylation and activation of mitogen-activated protein kinases (MAPKs; Shi et al., 2018a). Two MAPKs are recognized in Arabidopsis, the MEKK1-MKK2-MPK4-CBFs and MKK5-MPK3/6-CBFs. Initiation of MEKK1 activity activates signal transmission to MPK4 through MKK1/2 by phosphorylation. Further, the activated MKK2 phosphorylates MPK4 and MPK6, and consequently activates physio-biochemical responses in cold-stressed plants (Chen et al., 2022). The MAPKs regulate the expression of cold-responsive genes including TFs, where their involvement in cold stress response can be distinguished as early (e.g., changes of membrane proteins and activation of ion channels) and late responses (i.e., cold acclimation; Wang et al., 2020d). Recently, studies by Shu et al. (2022) showed the involvement of ferulic acid in enhancing cold stress response in tomato. The exogenous application of ferulic acid decreased the severity of cold injury and upregulated the expression of SlMAPK3, SlCBF1, and SlICE1. Knockout mutant of SlMAPK3 showed decrement of CBF expression in cold-stressed tomato suggesting the role of MAPK3 in CBF regulation.
The CBF coordinates cold signaling pathway through CBF-dependent regulatory pathway and CBF-independent signaling pathway (Park et al., 2018; Liu et al., 2019). In response to cold stress, the abscisic acid (ABA), jasmonic acid (JA), and indole acetic acid (IAA) are among the phytohormones involved in activating CBF-dependent signaling pathway (Wang et al., 2021; Zhou et al., 2021; Fu et al., 2022). The interaction between CBF and CRT/DRE motif regulates the downstream expression of an array of COR genes, which belong to KIN (cold induced), RD (responsive to desiccation), LTI (low temperature induced), and ERD (early dehydration inducible; Wani et al., 2021). The regulated products of COR genes include osmo-protectants biosynthetic enzymes, late embryogenesis abundant (LEA) proteins, TFs, protein kinases, proteins associated with lipid metabolism, proteins for hormone responses, cell wall modifiers, and chloroplast proteins (Liu et al., 2019). Discovery of an array of COR genes in Arabidopsis such as COR6.6 (Gilmour et al., 1996), COR15 (Lin and Thomashow, 1992), COR47 (Gilmour et al., 1992), COR78 (Horvath et al., 1993), and COR413 (Breton et al., 2003) shows the important function of these genes in plant growth, development, and response to abiotic stresses.
In the early response to cold stress, the regulation of membrane fluidity of chloroplast is important for photosynthesis. The COR413 was reported to be involved in the regulation process. The COR413 TFs have been categorized into two groups, the Cor413-plasma membrane (COR413pm) and COR413-inner membrane (COR413im) proteins. The COR413im plays a role in stabilizing chloroplast membrane under cold stress. Meanwhile, over-expression of Phlox subulata PsCor413pm2 in Arabidopsis showed increased influx of Ca2+ in transgenic Arabidopsis root responding to cold shock. In addition, under cold stress, the increment of cytosolic Ca2+ in PsCor413pm2 transgenic Arabidopsis increased the expression of five AtCOR (AtCor6.6/AtKIN2, AtCor15A, AtCor15B, AtCor47, and AtCor78/AtRD29) and two AtCBF (AtCBF2 and AtCBF3; Zhou et al., 2018). Studies by Su et al. (2018) further revealed the involvement of COR413PM1 in regulating the expression of COR regulons in response to cold stress including the fatty acid biosynthesis 1 (FAB1), fructokinase 3 (FRK3), sucrose phosphate synthase A1 (SPSA1), and GLN phosphoribosyl pyrophosphate amidotransferase 2 (ASE2) in Arabidopsis leaves. Our previous studies showed that over-expression of oil palm CBF1, the EgDREB1 enhanced tolerance to cold stress in lowland tomato. The EgDREB1 was suggested to control the expression of COR regulons such as LePOD, LeAPX, LeCAT, LeGP, LeHSP70, LeLEA, and LeMET2. Promoter analysis showed the presence of DRE/CRT motif in the promoter regions of these genes. EgDREB1 is also involved in drought stress signaling pathway as its expression was observed in transgenic tomato seedlings exposed to PEG treatment (Azzeme et al., 2017). These findings were similar with the observation carried out in oil palm, which we found that EgDREB1 and COR regulons were also differentially upregulated in the drought-stressed oil palm (Azzeme et al., 2016). These findings, therefore, further suggest the involvement of EgDREB1 in both cold and drought signaling of oil palm. Similar findings were observed in Arabidopsis, where CBF/DREB1 that was known to be involved only in cold signaling was also induced under drought, ABA treatment, and salinity (Wang et al., 2014c). Lowland tomato over-expressing EgDREB1 exhibited reduction in seed number, development of parthenocarpic fruits, alteration of leaves morphology, and increment of root biomass (Azzeme et al., 2020). Meanwhile, over-expression of EgCBF3 increased the expression of antifreeze proteins, the SlCHI3, SlPR1, SlPR-P2, and SlLAP2 in the lowland tomato. Delayed leaf senescence and flowering, increased chlorophyll content, and abnormal flowering were also recorded (Ebrahimi et al., 2016). These show involvements of CBF1 and CBF3 in plant growth and development, apart from their function as master regulators of abiotic stress.
Differentiating CBF-Dependent and CBF-Independent Signaling Pathways
The expression of COR genes and their regulation either through CBF-dependent regulatory pathway or CBF-independent signaling pathway is still controversial. In Arabidopsis, the different alleles of the CBF gene were found to be controlling different regulatory mechanisms involving COR genes, and therefore resulting in different degrees of freezing tolerance. However, interestingly most of the COR genes found in this study were co-regulated by CBF-dependent and CBF-independent pathways (Park et al., 2018). It shows that multiple allelism could control different metabolic pathways in cold-stressed plants due to the presence or absence of CRT/DRE motif in the COR gene promoters. The absence of CRT/DRE motif indicates the activation of CBF-independent signaling pathway through other TF proteins like WRKY, MYB, and bZIP families to modulate plant responses to cold stress. For instance, Arabidopsis BRASSINAZOLE-RESISTANT 1 (BZR1) was reported to be involved in regulating the expression of CBF1 and CBF2 and other COR genes via CBF-independent pathway to modulate plant response to cold stress (Li et al., 2017). The regulation of BZR1 under cold stress is controlled by brassinosteroids accumulation. In tomato, BZR1 was found to interact with E-box/BRRE motifs located in CBF1, CBF3, and RESPIRATORY BURST OXIDASE HOMOLOG 1 (RBOH1) promoters and upregulated their expression. The activation of tomato ROS signaling pathway was achieved through the expression of RBOH1, in which the gene was found responsible in triggering H2O2. The H2O2 modulated the redox status in cold-stressed plants and increased BZR1 production and CBF genes transcription (Fang et al., 2021). Further, Ca2+ influx is also reported to be involved in the activation of RBOH1, hence enhancing the production of ROS (Kour et al., 2021). Apart from that, the expression of CBF could also be controlled by other TFs like ICE1 that activates CBF in response to cold stress. The mutation of ICE1 in Arabidopsis showed inhibition in expression of CBF1, 2, and 3 and reduced resistance to cold stress (Chinnusamy et al., 2003). Conversely, the over-expression of SlICE1 enhanced the expression of SlCBF1 and increased the cold tolerance of tomato (Suzuki et al., 2012). The two-hour of cold exposure in transgenic potato over-expressing SaMKK2 showed significant increase in the expression of CBF1, 2, and 3 (Chen et al., 2022). In addition, a report by Jin et al., 2021 showed the involvement of bHLH (IbbHLH79) in activating sweet potato IbCBF3, through recognition of E-box (5′-CANNTG-3′) and G-box (5′-CACGTG-3′) located in the IbCBF3 promoter, therefore enhancing tolerance to cold stress.
The crosstalk between CBF-dependent and -independent signaling pathways in plant response to cold stress enables a more coordinated regulation by the large network of TFs. Fine-tuning of transcriptional regulation by these TFs is achieved through regulating their cellular abundance and their activities as discussed further in the following sections.
Regulated Expression of Transcription Factors by miRNA for Modulating Cold Stress Response
MicroRNAs (miRNAs) are recognized as a major gene family with an important role in gene regulation acting at the post-transcriptional level. First discovered in 1993, miRNAs are short endogenous single-stranded RNA of 20 to 24 nucleotides in length. These non-coding RNA molecules are derived from the typical stem loop precursors of 70 to 80 nucleotides long through the action of Dicer-like family of enzymes. Suppression of gene expression by miRNAs is achieved through two main mechanisms, which are mRNA cleavage and translation inhibition or combination of both (Megha et al., 2018). These mechanisms require binding of miRNAs to the target sites in mRNAs through perfect or near perfect base-pairing and there can be multiple mRNA targets for each miRNA. In plants, the target sites are mostly in the open-reading frame (ORFs) but some are found in the 5′-untranslated regions (UTRs) and 3’-UTRs of the mRNAs (German et al., 2008). Many of the miRNAs target genes encode TFs involved in transcriptional regulation of different plant developmental processes and stress responses. This constitutes an effective fine-tuning measure as the effects are extended to the various downstream genes regulated by these TFs.
Regulation by miRNA is critical for achieving the complex temporal and spatial expression profiles of each regulatory TF and the downstream genes being regulated by the TF (Samad et al., 2017; Wang et al., 2019a). The predicted target genes of miRNA under cold stress in winter turnip rape mainly encode for TFs, such as MYB, GAMYB, Teosinte branched 1/Cycloidea/Proliferating cell factor (TCP), bHLH, and SQUAMOSA promoter binding proteins (SBP; Zeng et al., 2018). The differential regulation of the TFs by the miRNA leads to cellular outcome essential for plant to cope with the cold stress condition. For example, integrated small RNA and transcriptome analysis of Populus simonii × P. nigra subjected to cold stress identified differential expression of miR319, miR159, miR167, miR172, miR395, miR393, miR390, and novel_63 and TFs including MYB, SBP, bZIP, Auxin Response Factors (ARF), and LONESOME HIGHWAY (LHW, atypical bHLH). These miRNAs and TFs direct or indirectly regulate the expression of Leucine-Rich Repeats (LRR) receptor kinase, DnaJ-related photosystem II, ARF, and SPLs associated with chilling injury (Zhou et al., 2019). In the construction of plant cold-responsive Gene Regulatory Network (GRN), the number of affected targets increased with the inclusion of TFs, which behave as central nodes for relaying information from miRNAs downstream to the TF regulons (Tiwari et al., 2020). Gene ontology enrichment analyses showed over-representation of distinct functional modules such as cold stress, transcription and translation, transport and pentatricopeptide repeat (PPR), cell wall and lignin synthesis, and signaling and protein degradation in the GRN.
Key miRNAs Involved in Regulating Transcription Factors for Cold Acclimation
MiRNAs are conserved among plant species and their expression varies depending on environmental biotic and abiotic conditions. The regulation of expression of targeted TFs by miRNAs is dependent on the miRNAs’ tissue-specific expression and the differential effects of the cleavage on the target transcripts (Megha et al., 2018). miR166 and miR319 showed altered expression in the roots of winter turnip rape under cold stress compared to normal condition suggesting their pivotal roles in cold stress response. The strong downregulated expression of miR319 (miR319e-1, miR319a, and miR319–2) and miR166 (miR166e-3p) in the roots of cold tolerant compared to cold-sensitive cultivars has important implication as root tolerance is critical during winter for plants’ survival (Zeng et al., 2018). It was found that the miR319 from winter turnip rape targets TCP4-like which controls secondary cell wall formation (Sun et al., 2017) while the miRNA166 regulates HD-ZIP III with a key role in cell wall and cellulose synthesis (Zhang et al., 2018). Significantly higher expression of both TFs demonstrated in the cold-tolerant cultivar compared to cold-sensitive cultivar showed that improvement in cell wall thickness and strength is important for cold acclimation.
Significantly higher expression of CORs including DREB1A/B/C, DREB2A, and TPP1/2 was observed in rice plants over-expressing Osa-miR319b, which targets GAMYB and TCP TFs. The increase in cold tolerance was evident based on the increase in proline content and survival rate of transgenic plants. It was suggested that miR319 negatively regulated OsPCF6 and OsTCP21 expression in rice, which partially increased the ROS scavenging capacity, therefore facilitating rice response under cold stress (Wang et al., 2014b). Over-expression of sha-miR319d from wild tomato silenced GAMYB-like1 and conferred chilling tolerance in cultivated tomato (Shi et al., 2019). Differential expression of key regulatory genes involved in chilling (CBF1 and MYB83), heat stress response (HSFA1a, HSFA1b, and HSP90), and ROS signaling [zinc-finger proteins (ZAT12 and ZAT10) and scavenging, superoxide dismutase (SOD), and catalase (CAT)] was observed in the transgenic compared to WT plants. This suggests that sha-miR319d regulates temperature stress in tomato via interaction of cold stress, heat stress, and ROS signaling pathways.
In rice, OsmiR156 plays an important role in enhancing tolerance to cold stress. Enhancement in cell viability and growth rate under cold stress was observed in Arabidopsis, pine, and rice over-expressing rice miRNA156 that targets OsSPL3 involved in upregulating the expression of OsWRKY71 (Zhou and Tang, 2019). Suppression of OsWRKY71 enhanced the expression of OsMYB2 and OsMYB3R-2. OsMYB2 is involved in regulating multiple stress responses including cold stress (Yang et al., 2012). OsMYB3R-2 transgenic rice showed enhanced tolerance to chilling stress due to the increase in expression of stress-responsive genes and the alteration in cell cycle (Ma et al., 2009).
However, a contrasting result was reported with OsmiR156k, probably due to the production of a different mature miRNA. OsmiR156k is a precursor miRNA which is differentially processed from the other precursor miRNAs (OsmiR156a-j; Moreal et al., 2016) and this may contribute to its differential accumulation and distinct function in plant tissues. Phenotypic analysis found that transgenic rice lines over-expressing OsmiR156k showed seedling growth inhibition at the very early seedling stage under cold stress. Lower survival rates, as well as reduction in chlorophyll and proline contents resulting from the ectopic expression of OsmiR156k, were observed. Downregulated expression of the CORs and SPL3, SPL14, and SPL17, the targets of OsmiR156k, was also detected (Cui et al., 2015).
Clearly, the comparison of miRNA expression between cold-tolerant and cold-sensitive cultivars and the functional studies in transgenic plants provided useful insights on regulatory mechanisms for cold acclimation involving miRNA156, miRNA166, and miRNA319 that play key roles in cold stress response in plants. The findings are summarized in Table 2.
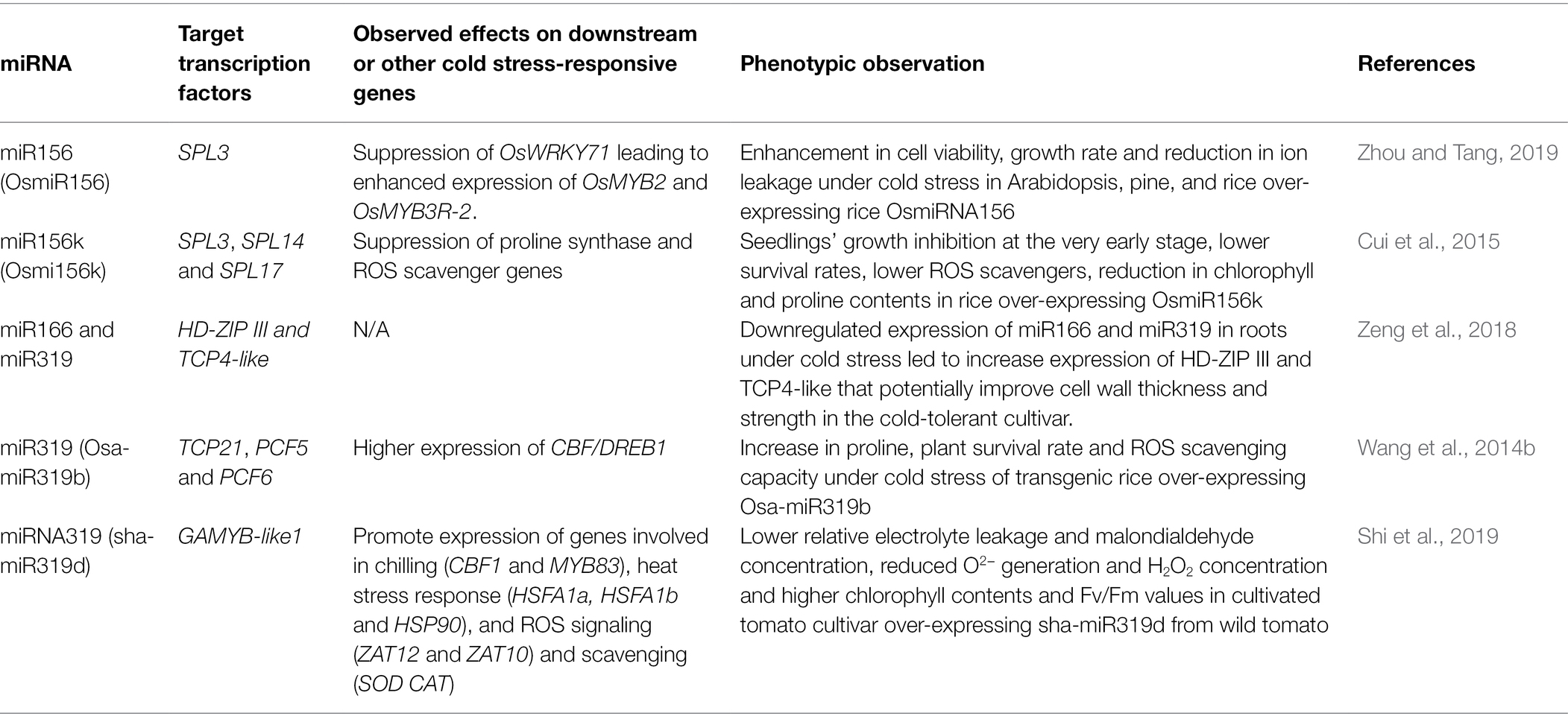
Table 2. Functional studies involving miRNA156, miR166, and miR319. The target transcription factors of the miRNAs, effects on the expression of downstream or cold stress-responsive genes regulated by the transcription factors are provided. The observed phenotypic effects of the miRNA regulation under cold stress are also included.
Controlled of Transcription Factor Levels Through Differential Protein Synthesis and Degradation During Cold Stress
Ribosome Biogenesis Factors in Accelerating de novo Protein Synthesis in Cold Acclimation
An effective cold stress response is dependent on controlled cellular levels of specific TFs along the cold signaling pathway through mechanisms that regulate their synthesis and degradation. This influences the cascade of events through the cold signaling pathway enabling fine-tuning of the cold stress responses. Protein translation which occurs at the ribosome is an important step in the production of a functional cellular proteins in living organisms. Ribosome assembly is a complex process which requires coordination of the activities of three RNA polymerases and more than 200 transiently associated ribosome biogenesis factors (RBFs; Sáez-Vásquez and Delseny, 2019). Arabidopsis zinc-finger proteins, REILs are cytosolic ribosomal 60S-biogenesis factors with potential role in accelerating ribosome de novo synthesis (Wang et al., 2017; Beine-Golovchuk et al., 2018). Studies utilizing Arabidopsis REIL mutants provided valuable insights on the factors influencing ribosome biogenesis and function under cold stress. Findings of the study by Cheong et al., 2021 suggested that biosynthesis of specialized ribosomes is required for cold acclimation.
It was demonstrated that STCH4/REIL2 helps in maintaining rRNA processing and promotes translation of CBF for regulating cold stress response (Yu et al., 2020). Over-expression of STCH4/REIL2 in Arabidopsis can confer chilling and freezing tolerance possibly through modified association of STCH4 with multiple ribosomal proteins. The reduction in rRNA processing ability exhibited by stch4 Arabidopsis mutants was further worsened by cold stress treatment. The key observations of the mutants include reduction in the level of CBF and delayed induction of the CBF regulons. Clearly, REILs play a role in enhancing cold stress tolerance through altering ribosomal composition and functions, which promotes translation of proteins essential for growth and survival of plants under the adverse effects of cold stress (Yu et al., 2020; Cheong et al., 2021). Studies involving Arabidopsis double mutants of REIL complemented by systems analyses of transcriptome and metabolome of the ribosomal complexes (Wang et al., 2017; Beine-Golovchuk et al., 2018) suggest that REILs likely serve as kinetic modulators of ribosome biogenesis or recycling that may assist in overcoming the initial cold-induced inhibition of translation.
Post-translational Regulation for Selective Degradation of Transcription Factors for Fine-Tuning Cold Stress Response
The 26S proteasome degrades TFs that have been covalently linked to a polyubiquitin chain to enable selective reduction in the abundance of a specific TF. Polyubiquitin chain is covalently attached to the target proteins through three sequential steps involving three enzymes; Ub-activating enzyme (E1), Ub-conjugating enzyme (E2), and Ub ligase (E3). The degradation of selective TFs is controlled strictly by numerous E3 Ub ligases responsible for substrate recognition. CULLIN-REALLY INTERESTING NEW GENE (RING) E3 ligases (CRLs) are the largest group of E3 ligase in plants. The multisubunit CRL regulates numerous biological processes through targeted ubiquitylation of signaling proteins. The four components of CRL core are the cullin scaffold protein, a RING finger protein that binds to an E2 ubiquitin conjugating enzyme, a receptor that recognizes the target protein, and adaptor proteins linking the receptor to the cullin. Different targets are recruited through binding of a large pool of distinct substrate-receptor modules to the N-termini of cullins (Lydeard et al., 2013; Wang et al., 2020c).
The ability to bind to the respective TFs that serve as substrates is crucial for the E3 Ub ligases activity and it is affected by other post-translational modifications including phosphorylation and SUMOylation (Buetow and Huang, 2016; Ding et al., 2019). Regulating the expression of CBF through controlling 26S proteasomic degradation of upstream TFs that act as regulators in the CBF-dependent signaling appeared as one of the key mechanisms for fine-tuning cold stress responses (Shi et al., 2018b). The increase or decrease in cellular abundance of a TF which acts as a positive or negative regulator influences the outcome of the cold signaling pathway.
In Arabidopsis, it was found that cold activation of ICE1 TF, the positive regulator of CBF3/DRB1A, is negatively affected by ubiquitination by RING E3 ubiquitin ligase HIGH EXPRESSION OF OSMOTICALLY RESPONSIVE GENE 1 (HOS1) which leads to its degradation through the 26S proteosomic degradation pathway (Ishitani et al., 1998). Similarly, cold tolerance is negatively regulated in banana by MaSINA1 which is an E3 ubiquitin ligase that targets MaICE1 (Fan et al., 2017). OPEN STOMATA 1 (OST1) is an important protein kinase involved in the regulation of plant response to cold stress (Ding et al., 2015). Under cold stress, it interacts with and phosphorylates ICE1, preventing it from interacting with HOS1. Stabilization of ICE1 promotes the transcriptional activity of CBF for COR induction. While under prolonged cold stress, activation of BRASSINOSTEROID-INSENSITIVE 2 (BIN2) kinase leads to phosphorylation of ICE1 which promotes ICE1 interaction with HOS1 and its subsequent degradation. This suppresses CBF activity and expression of COR genes (Ye et al., 2019). Thus, both kinases which act at different phases following cold stress encounter serve as partial regulators of CBF indirectly through ICE1. It is also believed that fine-tuning of CBF expression by BIN2 is important for balancing cold tolerance and plant growth (Ye et al., 2019). Thus, the regulation of the cellular abundance and activities of the CBF is important for plant adaptation and survival.
U-box E3 ligases (PUB25 and PUB26) from Arabidopsis target the upstream negative regulator of CBF/DREB1, MYB15. Phosphorylation of PUB25 and PUB26 by OST1 enhances their activities and the reduction in MYB15 level leads to increase expression of CBF genes which enhances the plant cold stress response (Wang et al., 2019b). Anthocyanin is essential for the cold acclimation response. Increase in anthocyanin improves antioxidant capability resulting in an increase in plant tolerance to low temperature (Naing et al., 2018). MYB TFs interact with bHLH TFs in regulating plant growth and development (Wang et al., 2020b). Apple MdbHLH33 activates the expression of MdCBF2 and for regulating cold tolerance and anthocyanin accumulation. An et al. (2020) reported on MdMYB308L interaction with MdbHLH33 for enhancing binding to MdCBF2 and MdDFR promoters. However, MYB30-INTERACTING E3 LIGASE 1 (MdMIEL1), an apple RING E3 ubiquitin ligase, was found to be interacting with MdMYB308L. The interaction which promotes degradation of MdMYB308L suppresses cold-tolerant response and anthocyanin accumulation in apple.
Other Mechanisms Modulating 26S Proteosomic Degradation of Cold Stress-Responsive Transcription Factors
SUMOylation can modulate the activity of TFs through regulating the localization and abundance of TFs as well as by influencing their interaction with chromatin. SUMOylation protects conjugated protein from degradation by blocking the lysine residues that can be ubiquitinated (Roy and Sadanandom, 2021). Thus, SUMOylation of ICE1 by SAP AND MIZ1 DOMAIN-CONTAINING LIGASE 1 (SIZ1; Jmii and Cappadocia, 2021) protects ICE1 from degradation through the 26S proteosomic degradation pathway. Expression of CBF/DREB1 and the downstream COR genes was increased while the expression of MYB15 was suppressed due to SIZ1 conjugation of ICE1, enhancing the tolerance to low temperature (Miura et al., 2007). The fine-tuning of the CBF/DREB1 signaling pathway through post-translational SUMOylation and ubiquitination events involving the positive regulator ICE1-like was also observed in apple. The apple MdCIbHLH1 which encodes an ICE1-like protein showed induced expression under cold stress. It can be modified through ubiquitination and SUMOylation pathways and binds to MdCBF2 for enhancing cold tolerance via the CBF signaling pathway. The ability of MdCIbHLH1 in maintaining its functionality in distantly related species, Nicotiana tabacum (tobacco; Feng et al., 2012), may suggest a universal mechanism for cold stress response in plants.
Long Hypocotyl 5 (HY5), a bZIP TF which acts as a positive regulator of cold acclimation, has been shown to activate about 10% of all cold-induced transcripts including anthocyanin biosynthetic genes, facilitating in the complete development of cold acclimation in Arabidopsis. Recent report showed that HY5 can directly regulate the expression of CBF 1, 2, and 3, or indirectly via MYB15 as it was shown to bind to MYB15 promoter region (Zhang et al., 2020). It has been reported that HY5 is subjected to post-translational control by COP1 E3 ubiquitin ligase (Catalá et al., 2011). Chaperonins are molecular chaperons involved in protein assembly, folding, trafficking, and degradation, with critical role in cellular development (Hartl et al., 2011). Prefoldin is a hexameric molecular chaperone belonging to group II chaperonins (Cao, 2016). Perea-Resa et al. (2017)demonstrated negative regulation of cold stress response in Arabidopsis by prefoldin which destabilizes HY5. Interaction of prefoldin, which accumulates in the nucleus with HY5, triggers ubiquitination and HY5 subsequent degradation through 26S proteosomic degradation pathway. This attenuated anthocyanin biosynthesis, which helped ensure accurate development of cold acclimation. Interestingly, the study showed that degradation of HY5 occurred in COP1-independent manner (Perea-Resa et al., 2017). Based on the findings, it is anticipated that prefoldin contributes indirectly through participation in cellular proteostasis to regulate the stability of TFs or complexes involved in different stages of gene expression (Blanco-Touriñán et al., 2021). Figure 2 provides a schematic diagram on translational and post-translational modifications affecting CBF-dependent signaling for cold acclimation that has been discussed.
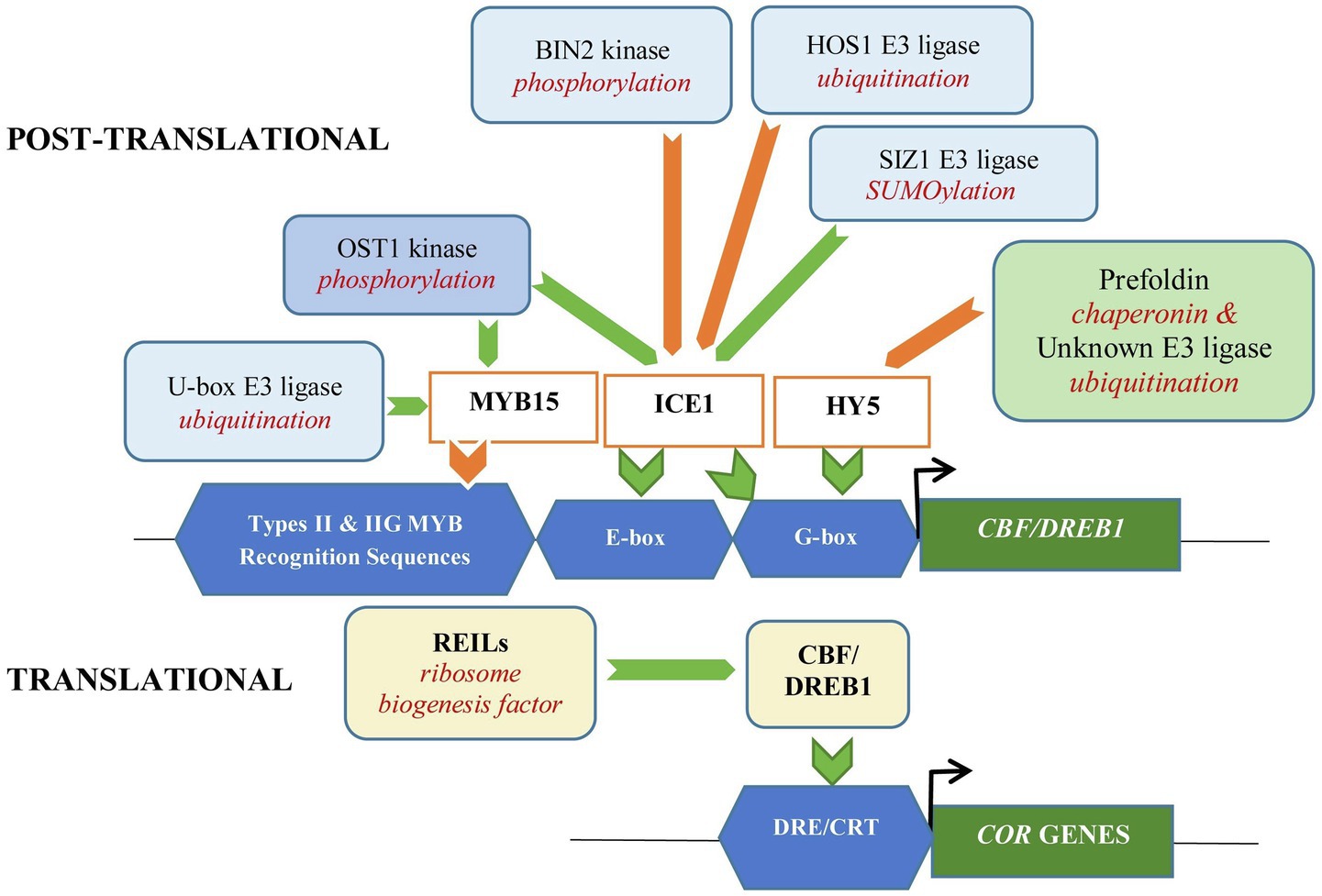
Figure 2. Translational and post-translational modifications of transcription factors affecting CBF-dependent signaling for cold acclimation in Arabidopsis thaliana. Translational regulation by ribosome biogenesis factor, REILs increase rRNA processing and CBF levels, positively affecting cold acclimation. Post-translational regulation through ubiquitination for proteosomic degradation of ICE1 (positive regulator of CBF) is suppressed by SUMOylation involving SIZ1 E3 ligase. Phosphorylation of ICE1 by OST1 disrupts its interaction with HOS1 E3 ligase preventing degradation of ICE1 and enhancing cold tolerance. While under prolonged cold stress, phosphorylation of ICE1 by BIN2 kinase facilitates interaction of ICE1 and HOS1 and subsequent degradation of ICE1. Phosphorylation of U-box E3 ligase (PUB25 and PUB26) by OST1 increases ubiquitination and degradation of the negative regulator MYB15 in Arabidopsis, enhancing the expression of CBF and its regulons, COR GENES. In contrast, promotion of ubiquitination of the positive regulator, HY5 through interaction with prefoldin which acts as a molecular chaperon, suppresses CBF expression. Green and orange arrows represent reactions/interactions that provide positive and negative effects, respectively, on the signaling pathway and expression of COR GENES.
Summary and Future Perspective
The crosstalk between multiple signaling pathways in plant response to cold stress enables access to a large network of TFs as transcriptional regulators. Fine-tuning through regulation of the cellular abundance and activities of the TFs in the GRN is important for plant adaptation and survival. The expression of TFs is affected by intracellular accumulation of secondary messengers like Ca2+, ROS, NO, and phytohormones. Activities of the secondary messengers that regulate rearrangement of chromatin and coordination of histone modification and DNA methylation allow plants to express specific TFs during cold stress. The spatial and temporal regulation by highly conserved miRNAs such as miRNA156, miRNA166, and miRNA319 that modulates the expression of target TFs is important for fine-tuning the cold stress response across plant species. In Arabidopsis, the production of CBF, the key TF for regulating the expression of COR genes is enhanced during cold stress through interaction of REILs, the ribosome biogenesis factors with multiple ribosomal proteins that influence ribosome composition and RNA processing. Other TFs like ICE, HY5, and MYB15 that serve as negative or positive regulators of ICE-CBF-COR signaling cascades are selectively degraded through the proteosomic degradation pathway. This provides the essential cellular metabolic balance for a proper cold stress response. It also helps overcome retardation in plant growth associated with CBF expression. Post-translational modifications of these TFs including through phosphorylation and SUMOylation events or attachment of molecular chaperons, which block or promote binding of the respective E3 ubiquitin ligases, regulate their degradation, with major consequence on the expression of COR genes.
The CBF-independent BZR1 signaling cascades involving other TF families such as WRKY regulate the induced production of specific sets of COR genes independent of the DRE/CRT motif, the recognition site for CBF. BZR1 and HSFA play a central role in linking the cold stress signaling with ROS signaling and heat stress signaling pathways, respectively, through a complex mechanism involving other TFs, HSPs, and ROS scavenger genes. While CAMTAs which possess multiple cis-elements recognized by a variety of stress-responsive TFs serve central position in the cold stress signaling cascades. CAMTAs integrate inputs from these TFs and convey them downstream for a coordinated expression of the different COR genes. Together, the CBF- and CBF-independent pathways allow plants to adjust to the varying severity of cold stress. The plants could also enhance their defense response by memory reprogramming at the transcriptional level based on previous cold stress encounters.
In general, plants have developed fine-tuning mechanisms for understanding temperature fluctuations to increase their chances of survival. Comprehensive understanding of the fine-tuning of spatial and temporal expression of genes and their translational and post-translational regulation associated with cold stress is critical to produce stress-resistant plants through genetic engineering or genome-assisted breeding strategies for increasing crop yield. It is also of critical importance for extending geographical distribution of crops and for survival under extreme seasonal conditions due to the effects of climate change. Studies on REILs, which are still confined to the model plant, Arabidopsis thaliana, can be expanded to economically important crops through powerful multi-omics strategy and functional studies utilizing gene editing technology. Functional analysis of the different TFs could benefit from CRISPR/dCas9 activation and suppression systems to unravel the complex GRN in endogenous system which could provide valuable additional information compared to utilizing model plant systems.
Author Contributions
SA produced the overall concept of the review, led the manuscript preparation, carried out the overall editing of the content, and she finalized the write-up. SA, AA, and KY contributed in writing and producing the tables and figures. All authors contributed to the article and approved the submitted version.
Funding
Funding for the research was provided by the Ministry of Higher Education Malaysia under the Long-Term Research Grant Scheme (LRGS) LRGS/1/2020/UPM/01/2/2.
Conflict of Interest
The authors declare that the research was conducted in the absence of any commercial or financial relationships that could be construed as a potential conflict of interest.
Publisher’s Note
All claims expressed in this article are solely those of the authors and do not necessarily represent those of their affiliated organizations, or those of the publisher, the editors and the reviewers. Any product that may be evaluated in this article, or claim that may be made by its manufacturer, is not guaranteed or endorsed by the publisher.
References
Agarwal, M., Hao, Y., Kapoor, A., Dong, C. H., Fujii, H., Zheng, X., et al. (2006). 5A R2R3 type MYB transcription factor is involved in the cold regulation of CBF genes and in acquired freezing tolerance. J. Biol. Chem. 281, 37636–37645. doi: 10.1074/jbc.M605895200
Alves, G. S. C., Torres, L. F., Déchamp, E., Breitler, J.-C., Joët, T., Gatineau, F., et al. (2017). Differential fine-tuning of gene expression regulation in coffee leaves by CcDREB1D promoter haplotypes under water deficit. J. Exp. Bot. 68, 3017–3031. doi: 10.1093/jxb/erx166
An, J. P., Wang, X. F., Zhang, X. W., Xu, H. F., Bi, S. Q., You, C. X., et al. (2020). An apple MYB transcription factor regulates cold tolerance and anthocyanin accumulation and undergoes MIEL1-mediated degradation. Plant Biotechnol. J. 18, 337–353. doi: 10.1111/pbi.13201
Azzeme, A. M., Abdullah, S. N. A., Aziz, M. A., and Wahab, P. E. M. (2016). Oil palm leaves and roots differ in physiological response, antioxidant enzyme activities and expression of stress-responsive genes upon exposure to drought stress. Acta Physiol. Plant. 38:52. doi: 10.1007/s11738-016-2073-2
Azzeme, A. M., Abdullah, S., Aziz, M. A., and Wahab, P. E. M. (2017). Oil palm drought inducible DREB1 induced expression of DRE/CRT- and non-DRE/CRT-containing genes in lowland transgenic tomato under cold and PEG treatments. Plant Physiol. Biochem. 112, 129–151. doi: 10.1016/j.plaphy.2016.12.025
Azzeme, A. M., Abdullah, S. N. A., Aziz, M. A., and Wahab, P. E. M. (2020). Overexpression of oil palm EgDREB1 in tomato decreased fruit size and produced parthenocarpic fruits. Biol. Plant. 64, 58–67. doi: 10.32615/bp.2019.084
Bao, F., Huang, X., Zhu, C., Zhang, X., Li, X., and Yang, S. (2014). Arabidopsis HSP90 protein modulates RPP4-mediated temperature-dependent cell death and defense responses. New Phytol. 202, 1320–1334. doi: 10.1111/nph.12760
Beine-Golovchuk, O., Firmino, A., Dąbrowska, A., Schmidt, S., Erban, A., Walther, D., et al. (2018). Plant temperature acclimation and growth rely on cytosolic ribosome biogenesis factor homologs. Plant Physiol. 176, 2251–2276. doi: 10.1104/pp.17.01448
Blanco-Touriñán, N., Esteve-Bruna, D., Serrano-Mislata, A., Esquinas-Ariza, R. M., Resentini, F., and Forment, J. (2021). A genetic approach reveals different modes of action of prefoldins. Plant Physiol. 187, 1534–1550. doi: 10.1093/plphys/kiab348
Breton, G., Danyluk, J., Charron, J. B., and Sarhan, F. (2003). Expression profiling and bioinformatic analyses of a novel stress-regulated multispanning transmembrane protein family from cereals and Arabidopsis. Plant Physiol. 132, 64–74. doi: 10.1104/pp.102.015255
Buetow, L., and Huang, D. (2016). Structural insights into the catalysis and regulation of E3 ubiquitin ligases. Nat. Rev. Mol. Cell Biol. 17, 626–642. doi: 10.1038/nrm.2016.91
Cao, J. (2016). Analysis of the prefolding gene family in 14 plant species. Front. Plant Sci. 7:317. doi: 10.3389/fpls.2016.00317
Catalá, R., Medina, J., and Salinas, J. (2011). Integration of low temperature and light signaling during cold acclimation response in Arabidopsis. Proc. Natl. Acad. Sci. U. S. A. 108, 16475–16480. doi: 10.1073/pnas.1107161108
Chen, Y., Chen, L., Sun, X., Kou, S., Liu, T., Dong, K., et al. (2022). The mitogen-activated protein kinase kinase MKK2 positively regulates constitutive cold resistance in the potato. Environ. Exp. Bot. 194:104702. doi: 10.1016/j.envexpbot.2021.104702
Chen, L., Yang, Y., Liu, C., Zheng, Y., Xu, M., Wu, N., et al. (2015). Characterization of WRKY transcription factors in Solanum lycopersicum reveals collinearity and their expression patterns under cold treatment. Biochem. Biophys. Res. Commun. 464, 962–968. doi: 10.1016/j.bbrc.2015.07.085
Chen, Y., Yang, J., Wang, Z., Zhang, H., Mao, X., and Li, C. (2013). Gene structures, classification, and expression models of the DREB transcription factor subfamily in Populus trichocarpa. Sci. World J. 2013:954640. doi: 10.1155/2013/954640
Cheong, B. E., Beine-Golovchuk, O., Gorka, M., Ho, W. W., Martinez-Seidel, F., Firmino, A. A. P., et al. (2021). Arabidopsis REI-LIKE proteins activate ribosome biogenesis during cold acclimation. Sci. Rep. 11:2410. doi: 10.1038/s41598-021-81610-z
Chinnusamy, V., Ohta, M., Kanrar, S., Lee, B. H., Hong, X. H., Agarwal, M., et al. (2003). ICE1: a regulator of cold-induced transcriptome and freezing tolerance in Arabidopsis. Gene 17, 1043–1054. doi: 10.1101/gad.1077503
Cui, N., Sun, X., Sun, M., Jia, B., Duanmu, H., Lv, D., et al. (2015). Overexpression of OsmiR156k leads to reduced tolerance to cold stress in rice (Oryza sativa). Mol. Breed. 35:214. doi: 10.1007/s11032-015-0402-6
Ding, Z., Li, S., An, X., Liu, X., Qin, H., and Wang, D. (2009). Transgenic expression of MYB15 confers enhanced sensitivity to abscisic acid and improved drought tolerance in Arabidopsis thaliana. J. Genet. Genom. 36, 17–29. doi: 10.1016/S1673-8527(09)60003-5
Ding, Y., Li, H., Zhang, X., Xie, Q., Gong, Z., and Yang, S. (2015). OST1 kinase modulates freezing tolerance by enhancing ICE1 stability in Arabidopsis. Dev. Cell 32, 278–289. doi: 10.1016/j.devcel.2014.12.023
Ding, Y., Shi, Y., and Yang, S. (2019). Advances and challenges in uncovering cold tolerance regulatory mechanisms in plants. New Phytol. 222, 1690–1704. doi: 10.1111/nph.15696
Doherty, C. J., Van Buskirk, H. A., Myers, S. J., and Thomashow, M. F. (2009). Roles for Arabidopsis CAMTA transcription factors in cold-regulated gene expression and freezing tolerance. Plant Cell 21, 972–984. doi: 10.1105/tpc.108.063958
Ebrahimi, M., Abdullah, S. N. A., Aziz, M. A., and Namasivayam, P. (2016). Oil palm EgCBF3 conferred stress tolerance in transgenic tomato plants through modulation of the ethylene signaling pathway. J. Plant Physiol. 202, 107–120. doi: 10.1016/j.jplph.2016.07.001
Fan, Z. Q., Chen, J. Y., Kuang, J. F., Lu, W. J., and Shan, W. (2017). The banana fruit SINA ubiquitin ligase MaSINA1 regulates the stability of MaICE1 to be negatively involved in cold stress response. Front. Plant Sci. 8:995. doi: 10.3389/fpls.2017.00995
Fang, P., Wang, Y., Wang, M., Wang, F., Chi, C., Zhou, Y., et al. (2021). Crosstalk between brassinosteroid and redox signaling contributes to the activation of CBF expression during cold responses in tomato. Antioxidants 10:509. doi: 10.3390/antiox10040509
Feng, X. M., Zhao, Q., Zhao, L. L., Qiao, Y., Xie, X. B., Li, H. F., et al. (2012). The cold-induced basic helix-loop-helix transcription factor gene MdCIbHLH1 encodes an ICE-like protein in apple. BMC Plant Biol. 12:22. doi: 10.1186/1471-2229-12-22
Fu, A., Zheng, Y., Yunhao Lv, Y., Watkins, C. B., Bai, C., Ma, L., et al. (2022). Multi-omics analysis reveals specific modifications associated with reduced chilling injury in bell pepper fruit by methyl jasmonate. Postharvest Biol. Technol. 185:111799. doi: 10.1016/j.postharvbio.2021.111799
German, M. A., Pillay, M., Jeong, D.-H., Hetawal, A., Luo, S., Janardhanan, P., et al. (2008). Global identification of microRNA-target RNA pairs by parallel analysis of RNA ends. Nat. Biotechnol. 26, 941–946. doi: 10.1038/nbt1417
Gilmour, S. J., Artus, N. N., and Thomashow, M. F. (1992). cDNA sequence analysis and expression of two cold-regulated genes of Arabidopsis thaliana. Plant Mol. Biol. 18, 13–21. doi: 10.1007/BF00018452
Gilmour, S. J., Lin, C., and Thomashow, M. F. (1996). Purification and properties of Arabidopsis thaliana COR (cold-regulated) gene polypeptides CORl5am and COR6.6 expressed in Escherichia coli. Plant Physiol. 111, 293–299. doi: 10.1104/pp.111.1.293
Guo, X., Liu, D., and Chong, K. (2018). Cold signaling in plants: insights into mechanisms and regulation. J. Integr. Plant Biol. 60, 745–756. doi: 10.1111/jipb.12706
Haq, S., Khan, A., Ali, M., Khattak, A. M., Gai, W.-X., Zhang, H.-X., et al. (2019). Heat shock proteins: dynamic biomolecules to counter plant biotic and abiotic stresses. Int. J. Mol. Sci. 20:5321. doi: 10.3390/ijms20215321
Hartl, F., Bracher, A., and Hayer-Hart, M. (2011). Molecular chaperones in protein folding and proteostasis. Nature 475, 324–332. doi: 10.1038/nature10317
Horvath, D. P., McLarney, B. K., and Thomashow, M. F. (1993). Regulation of Arabidopsis thaliana L. (Heyn) cor 78 in response to low temperature. Plant Physiol. 103, 1047–1053. doi: 10.1104/pp.103.4.1047
Hu, Z., Song, N., Zheng, M., Liu, X., Liu, Z., Xing, J., et al. (2015). Histone acetyltransferase GCN5 is essential for heat stress-responsive gene activation and thermotolerance in Arabidopsis. Plant J. 84, 1178–1191. doi: 10.1111/tpj.13076
Ishitani, M., Xiong, L., Lee, H., Stevenson, B., and Zhu, J. K. (1998). HOS1, a genetic locus involved in cold-responsive gene expression in Arabidopsis. Plant Cell 10, 1151–1161. doi: 10.1105/tpc.10.7.1151
Jiang, C., Zhang, H., Ren, J., Dong, J., Zhao, X., Wang, X., et al. (2020). Comparative transcriptome-based mining and expression profiling of transcription factors related to cold tolerance in peanut. Int. J. Mol. Sci. 21:6. doi: 10.3390/ijms21061921
Jin, R., Kim, B. H., Ji, C. Y., Kim, H. S., Li, H. M., Ma, D. F., et al. (2017). Overexpressing IbCBF3 increases low temperature and drought stress tolerance in transgenic sweet potato. Plant Physiol. Biochem. 118, 45–54. doi: 10.1016/j.plaphy.2017.06.002
Jin, R., Kim, H. S., Yu, T., Zhang, A., Yang, Y., Liu, M., et al. (2021). Identification and function analysis of bHLH genes in response to cold stress in sweet potato. Plant Physiol. Biochem. 169, 224–235. doi: 10.1016/j.plaphy.2021.11.027
Jmii, S., and Cappadocia, L. (2021). Plant SUMO E3 ligases: function, structural organization, and connection with DNA. Front. Plant Sci. 12:652170. doi: 10.3389/fpls.2021.652170
Ke, L., Lei, W., Yang, W., Wang, J., Gao, J., Cheng, J., et al. (2020). Genome-wide identification of cold responsive transcription factors in Brassica napus L. BMC Plant Biol. 20:62. doi: 10.1186/s12870-020-2253-5
Kim, J.-K. (2021). Multifaceted chromatin structure and transcription changes in plant stress response. Int. J. Mol. Sci. 22:22. doi: 10.3390/ijms22042013
Kim, Y., Park, S., Gilmour, S. J., and Thomashow, M. F. (2013). Roles of CAMTA transcription factors and salicylic acid in configuring the low-temperature transcriptome and freezing tolerance of Arabidopsis. Plant J. 75, 364–376. doi: 10.1111/tpj.12205
Knight, M. R., and Knight, H. (2012). Low-temperature perception leading to gene expression and cold tolerance in higher plants. New Phytol. 195, 737–751. doi: 10.1111/j.1469-8137.2012.04239.x
Kour, J., Kohli, S. K., Khanna, K., Bakshi, P., Sharma, P., Singh, A. D., et al. (2021). Brassinosteroid signaling, crosstalk and, physiological functions in plants Under heavy metal stress. Front. Plant Sci. 12:608061. doi: 10.3389/fpls.2021.608061
Krishna, P., Sacco, M., Cherutti, J. F., and Hill, S. (1995). Cold-lnduced accumulation of hsp 90 transcripts in Brassica napus. Plant Physiol. 107, 915–923. doi: 10.1104/pp.107.3.915
Kumar, A., Changwal, C., Thapa, B., Tanpure, R. S., Hada, A., Singh, P. K., et al. (2021). “Transcription factors: a tool box for countering the effect of abiotic stresses,” in Stress Tolerance in Horticultural Crop. eds. A. Kumar, A. C. Rai, A. Rai, K. K. Rai, and V. P. Rai (Amsterdam: Elsevier), 169–192.
Leuendorf, J. K., Frank, M., and Schmülling, T. (2020). Acclimation, priming and memory in the response of Arabidopsis thaliana seedlings to cold stress. Sci. Rep. 10:689. doi: 10.1038/s41598-019-56797-x
Li, C., Ng, C. K. Y., and Fan, L.-M. (2015). MYB transcription factors, active players in abiotic stress signaling. Environ. Exp. Bot. 114, 80–91. doi: 10.1016/j.envexpbot.2014.06.014
Li, H., Yi, K., Shi, Y., Cheng, J., Zhang, X., and Yang, S. (2017). BZR1 positively regulates freezing tolerance via CBF-dependent and CBF-independent pathways in Arabidopsis. Mol. Plant 10, 545–559. doi: 10.1016/j.molp.2017.01.004
Lin, C., and Thomashow, M. F. (1992). DNA sequence analysis of a complementary DNA for cold-regulated Arabidopsis gene cor15 and characterization of the COR 15 polypeptide. Plant Physiol. 99, 519–525. doi: 10.1104/pp.99.2.519
Lindemose, S., O’Shea, C., Jensen, M. K., and Skriver, K. (2013). Structure, function and networks of transcription factors involved in abiotic stress responses. Int. J. Mol. Sci. 14, 5842–5878. doi: 10.3390/ijms14035842
Liu, Y., Dang, P., Liu, L., and He, C. (2019). Cold acclimation by the CBF–COR pathway in a changing climate: lessons from Arabidopsis thaliana. Plant Cell Rep. 38, 511–519. doi: 10.1007/s00299-019-02376-3
Liu, Q., Ding, Y., Shi, Y., Ma, L., Wang, Y., Song, C., et al. (2021). The calcium transporter ANNEXIN1mediates cold-induced calcium signaling and freezing tolerance in plants. EMBO J. 40:e104559. doi: 10.15252/embj.2020104559
Luo, M., Cheng, K., Xu, Y., Yang, S., and Wu, K. (2017). Plant responses to abiotic stress regulated by histone deacetylases. Front. Plant Sci. 8:2147. doi: 10.3389/fpls.2017.02147
Lv, K., Li, J., Zhao, K., Chen, S., Nie, J., Zhang, W., et al. (2020). Overexpression of an AP2/ERF family gene, BpERF13, in birch enhances cold tolerance through upregulating CBF genes and mitigating reactive oxygen species. Plant Sci. 292:110375. doi: 10.1016/j.plantsci.2019.110375
Lydeard, J. R., Schulman, B. A., and Harper, J. W. (2013). Building and remodelling Cullin-RING E3 ubiquitin ligases. EMBO Rep. 14, 1050–1061. doi: 10.1038/embor.2013.173
Ma, Q., Dai, X., Xu, Y., Guo, J., Liu, Y., Chen, N., et al. (2009). Enhanced tolerance to chilling stress in OsMYB3R-2 transgenic rice is mediated by alteration in cell cycle and ectopic expression of stress genes. Plant Physiol. 150, 244–256. doi: 10.1104/pp.108.133454
Ma, Y., Dai, X., Xu, Y., Luo, W., Zheng, X., Zeng, D., et al. (2015). COLD1 conferschilling tolerance in rice. Cell 160, 1209–1221. doi: 10.1016/j.cell.2015.01.046
Ma, X., Yu, Y.-N., Jia, J.-H., Li, Q.-H., and Gong, Z.-H. (2022). The pepper MYB transcription factor CaMYB306 accelerates fruit coloration and negatively regulates cold resistance. Sci. Hortic. 295:110892. doi: 10.1016/j.scienta.2022.110892
Mao, K., Dong, Q., Li, C., Liu, C., and Ma, F. (2017). Genome wide identification and characterization of apple bHLH transcription factors and expression analysis in response to drought and salt stress. Front. Plant Sci. 8:480. doi: 10.3389/fpls.2017.00480
Megha, S., Urmila Basu, U., and Kav, N. N. V. (2018). Regulation of low temperature stress in plants by microRNAs. Plant Cell Environ. 41, 1–15. doi: 10.1111/pce.12956
Mehrotra, S., Verma, S., Kumar, S., Kumari, S., and Mishra, B. (2020). Transcriptional regulation and signalling of cold stress response in plants: An overview of current understanding. Environ. J. Exp. Bot. 180:104243. doi: 10.1016/j.envexpbot.2020.104243
Miura, K., Jin, J. B., Lee, J., Yoo, C. Y., Stirm, V., Miura, T., et al. (2007). SIZ1-mediated sumoylation of ICE1 controls CBF3/DREB1A expression and freezing tolerance in Arabidopsis. Plant Cell 19, 1403–1414. doi: 10.1105/tpc.106.048397
Moreal, E. G. O., da Silva, E. M., Silva, G. F. F., Valente, G. T., Rojas, C. H. B., Vincentz, M., et al. (2016). Functional and evolutionary analyses of the miR156 and miR529 families in land plants. BMC Plant Biol. 16:40. doi: 10.1186/s12870-016-0716-5
Naing, A. H., Ai, T. N., Lim, K. B., Lee, I. J., and Kim, C. K. (2018). Overexpression of Rosea1 from snapdragon enhances anthocyanin accumulation and abiotic stress tolerance in transgenic tobacco. Front. Plant Sci. 9:1070. doi: 10.3389/fpls.2018.01070
Nakashima, K., Takasaki, H., Mizoi, J., Shinozaki, K., and Yamaguchi-Shinozaki, K. (2012). NAC transcription factors in plant abiotic stress responses. Biochim. Biophys. Acta 1819, 97–103. doi: 10.1016/j.bbagrm.2011.10.005
Noman, M., Aysha, J., Ketehouli, T., Yang, J., Du, L., Wang, F., et al. (2021). Calmodulin binding transcription activators: An interplay between calcium signalling and plant stress tolerance. J. Plant Physiol. 256:153327. doi: 10.1016/j.jplph.2020.153327
Park, J., Lim, C. J., Shen, M. Z., Park, H. J., Cha, J. Y., Iniesto, E., et al. (2018). Epigenetic switch from repressive to permissive chromatin in response to cold stress. Proc. Natl. Acad. Sci. U. S. A. 115, E5400–E5409. doi: 10.1073/pnas.1721241115
Perea-Resa, C., Rodríguez-Milla, M. A., Iniesto, E., Rubio, V., and Julio Salinas, J. (2017). Prefoldins negatively regulate cold acclimation in Arabidopsis thaliana by promoting nuclear proteasome-mediated HY5 degradation. Mol. Plant 10, 791–804. doi: 10.1016/j.molp.2017.03.012
Roy, D., and Sadanandom, A. (2021). SUMO mediated regulation of transcription factors as a mechanism for transducing environmental cues into cellular signaling in plants. Cell. Mol. Life Sci. 78, 2641–2664. doi: 10.1007/s00018-020-03723-4
Rushton, P. J., Somssich, I. E., Ringler, P., and Shen, Q. J. (2010). WRKY transcription factors. Trends Plant Sci. 15, 247–258. doi: 10.1016/j.tplants.2010.02.006
Sáez-Vásquez, J., and Delseny, M. (2019). Ribosome biogenesis in plants: From functional 45S ribosomal DNA organization to ribosome assembly factors. Plant Cell 31, 1945–1967. doi: 10.1105/tpc.18.00874
Samad, A. F. A., Sajad, M., Nazaruddin, N., Fauzi, I. A., Murad, A. M. A., Zainal, Z., et al. (2017). MicroRNA and transcription factor: key players in plant regulatory network. Front. Plant Sci. 8:565. doi: 10.3389/fpls.2017.00565
Sharma, P., Sharma, M. M. M., Patra, A., Vashisth, M., Mehta, S., Singh, B., et al. (2020). “The role of key transcription factors for cold tolerance in plants,” in Transcription Factors for Abiotic Stress Tolerance in Plants. ed. S. H. Wani (London: Academic Press), 123–152.
Shen, T., Wen, X., Wen, Z., Qiu, Z., Hou, Q., Li, Z., et al. (2021). Genome-wide identification and expression analysis of bHLH transcription factor family in response to cold stress in sweet cherry (Prunus avium L.). Sci. Hortic. 279:109905. doi: 10.1016/j.scienta.2021.109905
Shi, Y., Ding, Y., and Yang, S. (2018b). Molecular regulation of CBF signaling in cold acclimation. Trends Plant Sci. 23, 623–637. doi: 10.1016/j.tplants.2018.04.002
Shi, X., Jiang, F., Wen, J., and Wu, Z. (2019). Overexpression of Solanum habrochaites microRNA319d (sha-miR319d) confers chilling and heat stress tolerance in tomato (S. lycopersicum). BMC Plant Biol. 19:214. doi: 10.1186/s12870-019-1823-x
Shi, S., Li, S., Asim, M., Mao, J., Xu, D., Ullah, Z., et al. (2018a). The Arabidopsis calcium-dependent protein kinases (CDPKs) and their roles in plant growth regulation and abiotic stress responses. Int. J. Mol. Sci. 19:1900. doi: 10.3390/ijms19071900
Shu, P., Li, Y., Li, Z., Xiang, L., Sheng, J., and Shen, L. (2022). Ferulic acid enhances chilling tolerance in tomato fruit by up-regulating the gene expression of CBF transcriptional pathway in MAPK3-dependent manner. Postharvest Biol. Technol. 185:111775. doi: 10.1016/j.postharvbio.2021.111775
Sohrabi, S. S., Ismaili, A., Nazarian-Firouzabadi, F., Fallahi, H., and Hosseini, S. Z. (2022). Identification of key genes and molecular mechanisms associated with temperature stress in lentil. Gene 807:145952. doi: 10.1016/j.gene.2021.145952
Su, C., Chen, K., Ding, Q., Mou, Y., Yang, R., Zhao, M., et al. (2018). Proteomic analysis of the function of a novel cold-regulated multispanning transmembrane protein COR413-PM1 in Arabidopsis. Int. J. Mol. Sci. 19:2572. doi: 10.3390/ijms19092572
Su, L. T., Li, J. W., Liu, D. Q., Zhai, Y., Zhang, H. J., Li, X. W., et al. (2014). A novel MYB transcription factor, GmMYBJ1, from soybean confers drought and cold tolerance in Arabidopsis thaliana. Gene 538, 46–55. doi: 10.1016/j.gene.2014.01.024
Sun, X., Wang, Y., and Sui, N. (2018). Transcriptional regulation of bHLH during plant response to stress. Biochem. Biophys. Res. Commun. 503, 397–401. doi: 10.1016/j.bbrc.2018.07.123
Sun, X., Wang, C., Xiang, N., Li, X., Yang, S., Du, J. C., et al. (2017). Activation of secondary cell wall biosynthesis by miR319-targeted TCP4 transcription factor. Plant Biotechnol. J. 15, 1284–1294. doi: 10.1111/pbi.12715
Suzuki, N., Koussevitzky, S., Mittler, R., and Miller, G. (2012). ROS and redox signalling in the response of plants to abiotic stress. Plant Cell Environ. 35, 259–270. doi: 10.1111/j.1365-3040.2011.02336.x
Tanpure, R. S., Ghuge, S. A., Dawkar, V. V., and Kumar, A. (2021). “Signaling responses and their role in the mitigation of abiotic stresses,” in Stress Tolerance in Horticultural Crops. eds. R. A. Chandra, A. Rai, R. K. Kumar, V. P. Rai, and A. Kumar (Sawston: Woodhead Publishing), 327–346.
Tiwari, B., Habermann, K., Arif, M. A., Weil, H. L., Garcia-Molina, A., Kleine, T., et al. (2020). Identification of small RNAs during cold acclimation in Arabidopsis thaliana. BMC Plant Biol. 20:298. doi: 10.1186/s12870-020-02511-3
Vazquez-Hernandez, M., Romero, I., Escribano, M. I., Merodio, C., and Sanchez-Ballesta, M. T. (2017). Deciphering the role of CBF/DREB transcription factors and dehydrins in maintaining the quality of Table grapes cv. Autumn royal treated with high CO2 levels and stored at 0°C. Front. Plant Sci. 8:1591. doi: 10.3389/fpls.2017.01591
Wang, R.-K., Cao, Z.-H., and Hao, Y.-J. (2014a). Overexpression of a R2R3 MYB gene MdSIMYB1 increases tolerance to multiple stresses in transgenic tobacco and apples. Physiol. Plant. 150, 76–87. doi: 10.1111/ppl.12069
Wang, F., Chen, S., Liang, D., Qu, G.-Z., Chen, S., and Zhao, X. (2020a). Transcriptomic analyses of Pinus koraiensis under different cold stresses. BMC Genomics 21:10. doi: 10.1186/s12864-019-6401-y
Wang, K., Deshaies, R. J., and Liu, X. (2020c). “Assembly and regulation of CRL ubiquitin ligases,” in Cullin-RING Ligases and Protein Neddylation. eds. Y. Sun, W. Wei, and J. Jin (Singapore: Springer Nature), 33–46.
Wang, X., Ding, Y., Li, Z., Shi, Y., Wang, J., Hua, J., et al. (2019b). PUB25 and PUB26 promote plant freezing tolerance by degrading the cold signaling negative regulator MYB15. Dev. Cell 51, 222–235. doi: 10.1016/j.devcel.2019.08.008
Wang, P., Hsu, C.-C., Dua, Y., Zhu, P., Zhao, C., Fu, X., et al. (2020d). Mapping proteome-wide targets of protein kinases in plant stress responses. Proc. Natl. Acad. Sci. U. S. A. 117, 3270–3280. doi: 10.1073/pnas.1919901117
Wang, J., Li, Q., Mao, X., Li, A., and Jing, R. (2016). Wheat transcription factor TaAREB3 participates in drought and freezing tolerances in Arabidopsis. Int. J. Biol. Sci. 12, 257–269. doi: 10.7150/ijbs.13538
Wang, L., Li, H., Zhao, C., Li, S., Kong, L., Wu, W., et al. (2017). The inhibition of protein translation mediated by AtGCN1 is essential for cold tolerance in Arabidopsis thaliana. Plant Cell Environ. 40, 56–68. doi: 10.1111/pce.12826
Wang, Z., Liu, J., Guo, H., He, X., Wu, W., Du, J., et al. (2014c). Characterization of two highly similar CBF/DREB1-like genes, PhCBF4a and PhCBF4b, in Populus hopeiensis. Plant Physiol. Biochem. 83, 107–116. doi: 10.1016/j.plaphy.2014.07.012
Wang, X., Liu, W.-C., Zeng, X. W., Yan, S., Qiu, Y.-M., Wang, J.-B., et al. (2021). HbSnRK2.6 functions in ABA-regulated cold stress response by promoting HbICE2 transcriptional activity in Hevea brasiliensis. Int. J. Mol. Sci. 22:12707. doi: 10.3390/ijms222312707
Wang, J., Mei, J., and Ren, G. (2019a). Plant microRNAs: biogenesis, homeostasis, and degradation. Front. Plant Sci. 10:360. doi: 10.3389/fpls.2019.00360
Wang, S.-T., Sun, X.-L., Hoshino, Y., Yu, Y., Jia, B., Sun, Z.-W., et al. (2014b). MicroRNA319 positively regulates cold tolerance by targeting OsPCF6 and OsTCP21 in rice (Oryza sativa L.). PLOS One 9:e91357. doi: 10.1371/journal.pone.0091357
Wang, H., Wang, X., Yu, C., Wang, C., Jin, Y., and Zhang, H. (2020b). MYB transcription factor PdMYB118 directly interacts with bHLH transcription factor PdTT8 to regulate wound-induced anthocyanin biosynthesis in poplar. BMC Plant Biol. 20:173. doi: 10.1186/s12870-020-02389-1
Wani, U. M., Majeed, S. T., Raja, V., Wani, Z. A., Jan, N., Andrabi, K. I., et al. (2021). Ectopic expression of a novel cold-resistance protein 1 from Brassica oleracea promotes tolerance to chilling stress in transgenic tomato. Sci. Rep. 11:16574. doi: 10.1038/s41598-021-96102-3
Xie, P., Liu, W., Kang, Y., Hua, W., Qian, L.-W., Guan, C.-Y., et al. (2021a). Identification and relative expression analysis of CBF gene family in Brassica napus L. Acta Agron. Sin. 47, 2394–2406. doi: 10.3724/SP.J.1006.2021.04259
Xie, W., Tang, Q., Yan, F., and Tao, Z. (2021b). Transcriptional memory and response to adverse temperatures in plants. J Zhejiang Univ Sci B 22, 791–804. doi: 10.1631/jzus.B2100287
Xu, J., Xue, C., Xue, D., Zhao, J., Gai, J., Guo, N., et al. (2013). Overexpression of GmHsp90s, a heat shock protein 90 (Hsp 90) gene family cloning from soybean, decrease damage of abiotic stresses in Arabidopsis thaliana. PLoS One 8:e69810. doi: 10.1371/journal.pone.0069810
Yang, A., Dai, X., and Zhang, W. H. (2012). A R2R3-type MYB gene, OsMYB2, is involved in salt, cold, and dehydration tolerance in rice. J. Exp. Bot. 63, 2541–2556. doi: 10.1093/jxb/err431
Yang, X., Guo, T., Li, J., Chen, Z., Guo, B., and An, X. (2021). Genome-wide analysis of the MYB-related transcription factor family and associated responses to abiotic stressors in Populus. Int. J. Biol. Macromol. 191, 359–376. doi: 10.1016/j.ijbiomac.2021.09.042
Ye, K., Li, H., Ding, Y., Shi, Y., Song, C., Gong, Z., et al. (2019). BRASSINOSTEROID-INSENSITIVE2 negatively regulates the stability of transcription factor ICE1 in response to cold stress in Arabidopsis. Plant Cell 31, 2682–2696. doi: 10.1105/tpc.19.00058
Yu, H., Kong, X., Huang, H., Wu, W., Park, J., Yun, D.-J., et al. (2020). STCH4/REIL2 confers cold stress tolerance in Arabidopsis by promoting rRNA processing and CBF protein translation. Cell Rep. 30, 229–242. doi: 10.1016/j.celrep.2019.12.012
Yu, X., Meng, X., Liu, Y., Li, N., Zhang, A., Wang, T. J., et al. (2018). The chromatin remodeler ZmCHB101 impacts expression of osmotic stress-responsive genes in maize. Plant Mol. Biol. 97, 451–465. doi: 10.1007/s11103-018-0751-8
Yuan, P., Yang, T., and Poovaiah, B. W. (2018). Calcium signaling-mediated plant response to cold stress. Int. J. Mol. Sci. 19:3896. doi: 10.3390/ijms19123896
Zeng, X., Xu, Y., Jiang, J., Zhang, F., Ma, L., Wu, D., et al. (2018). Identification of cold stress responsive microRNAs in two winter turnip rape (Brassica rapa L.) by high throughput sequencing. BMC Plant Biol. 18:52. doi: 10.1186/s12870-018-1242-4
Zeng, Z., Zhang, W., Marand, A. P., Zhu, B., Buell, C. R., and Jiang, J. (2019). Cold stress induces enhanced chromatin accessibility and bivalent histone modifications H3K4me3 and H3K27me3 of active genes in potato. Genome Biol. 20:123. doi: 10.1186/s13059-019-1731-2
Zhang, L., Jiang, X., Liu, Q., Ahammed, G. J., Lin, R., Wang, L., et al. (2020). The HY5 and MYB15 transcription factors positively regulate cold tolerance in tomato via the CBF pathway. Plant Cell Environ. 43, 2712–2726. doi: 10.1111/pce.13868
Zhang, J., Xie, M., Tuskan, G. A., Muchero, W., and Chen, J.-G. (2018). Recent advances in the transcriptional regulation of secondary cell wall biosynthesis in the woody plants. Front. Plant Sci. 9:1535. doi: 10.3389/fpls.2018.01535
Zhang, Y., Yu, H., Yang, X., Li, Q., Ling, J., Wang, H., et al. (2016). CsWRKY46, a WRKY transcription factor from cucumber, confers cold resistance in transgenic-plant by regulating a set of cold-stress responsive genes in an ABA-dependent manner. Plant Physiol. Biochem. 108, 478–487. doi: 10.1016/j.plaphy.2016.08.013
Zhao, Q., Xiang, X., Liu, D., Yang, A., and Wang, Y. (2018). Tobacco transcription factor NtbHLH123 confers tolerance to cold stress by regulating the NtCBF pathway and reactive oxygen species homeostasis. Front. Plant Sci. 9:381. doi: 10.3389/fpls.2018.00381
Zhao, C., Zhang, Z., Xie, S., Si, T., Li, Y., and Zhu, J. K. (2016). Mutational evidence for the critical role of CBF transcription factors in cold acclimation in Arabidopsis. Plant Physiol. 171, 2744–2759. doi: 10.1104/pp.16.00533
Zheng, M., Liu, X., Lin, J., Liu, X., Wang, Z., Xin, M., et al. (2019). Histone acetyltransferase GCN5 contributes to cell wall integrity and salt stress tolerance by altering the expression of cellulose synthesis genes. Plant J. 97, 587–602. doi: 10.1111/tpj.14144
Zhou, B., Kang, Y., Leng, J., and Xu, Q. (2019). Genome-wide analysis of the miRNA-mRNAs network involved in cold tolerance in Populus simonii × P. nigra. Genes 10:430. doi: 10.3390/genes10060430
Zhou, P., Li, X., Liu, X., Wen, X., Zhang, Y., and Zhang, D. (2021). Transcriptome profiling of Malus sieversii under freezing stress after being cold acclimated. BMC Genomics 22:681. doi: 10.1186/s12864-021-07998-0
Zhou, A., Liu, E., Li, H., Li, Y., Feng, S., Gong, S., et al. (2018). PsCor413pm2, a plasma membrane-localized, cold-regulated protein from Phlox subulata, confers low temperature tolerance in Arabidopsis. Int. J. Mol. Sci. 19:2579. doi: 10.3390/ijms19092579
Zhou, M., and Tang, W. (2019). MicroRNA156 amplifies transcription factor-associated cold stress tolerance in plant cells. Mol. Gen. Genomics. 294, 379–393. doi: 10.1007/s00438-018-1516-4
Keywords: cold stress, transcription factors, CBF-dependent pathway, ICE1, chromatin modification, miRNA, REIL, ubiquitin proteosomic degradation
Citation: Abdullah SNA, Azzeme AM and Yousefi K (2022) Fine-Tuning Cold Stress Response Through Regulated Cellular Abundance and Mechanistic Actions of Transcription Factors. Front. Plant Sci. 13:850216. doi: 10.3389/fpls.2022.850216
Edited by:
Cankui Zhang, Purdue University, United StatesCopyright © 2022 Abdullah, Azzeme and Yousefi. This is an open-access article distributed under the terms of the Creative Commons Attribution License (CC BY). The use, distribution or reproduction in other forums is permitted, provided the original author(s) and the copyright owner(s) are credited and that the original publication in this journal is cited, in accordance with accepted academic practice. No use, distribution or reproduction is permitted which does not comply with these terms.
*Correspondence: Siti Nor Akmar Abdullah, c25hYUB1cG0uZWR1Lm15