- 1Hubei Insect Resources Utilization and Sustainable Pest Management Key Laboratory, College of Plant Science and Technology, Huazhong Agricultural University, Wuhan, China
- 2Department of Entomology, Faculty of Agricultural Sciences and Technology, Bahauddin Zakariya University, Multan, Pakistan
- 3Department of Plant Protection, Faculty of Agriculture, Ordu University, Ordu, Turkey
- 4School of Biological Sciences, University of Nebraska–Lincoln, Lincoln, NE, United States
Functional responses are central to predator–prey dynamics and describe how predation varies with prey abundance. Functional responses often are measured without regard to prey size (i.e., body mass) or the temperature dependence of feeding rates. However, variation in prey size within populations is ubiquitous, and predation rates are often both size and temperature-dependent. Here, we assessed functional responses of larvae and adult Harmonia axyridis on the 1st, 2nd, and 3rd instars of the prey Spodoptera litura across a range of temperatures (i.e., 15, 20, 25, 30, and 35°C). The type and parameters of the functional responses were determined using logistic regression and fitted to the Roger's random predator equation. The magnitude of predation varied with the predator and prey stage, but prey predation increased with warming and predator age. Predation by the female and 4th instar of H. axyridis on the 1st instar of prey was greater, followed by the 2nd and 3rd instar of prey S. litura. No predation occurred on the larger prey for the 1st, 2nd, and 3rd instars of H. axyridis. The larvae and adult H. axyridis produced a type II (hyperbolic) functional response curve across all temperatures and the three prey types they consumed. Space clearance rates, handling time, and maximum predation rates of H. axyridis changed with temperature and prey size, increasing with temperature and decreasing with prey size, suggesting more predation will occur on younger prey. This study indicates an interactive role of temperature and prey/predator size in shaping functional responses, which might complicate the planning of effective biocontrol strategies against this serious pest.
Introduction
Trophic interactions are central to community ecology and ecosystem stability (Wang and Zou, 2017) by structuring food webs (Mcintosh et al., 2017), driving population dynamics (Noman et al., 2021), and shaping landscapes of fear (Fardell, 2022). These ecological functions, however, are regulated by species interaction strengths within food webs (Novak and Wootton, 2010). Many biotic and abiotic factors (King et al., 2021), including temperature, arena size, species identity, body mass, and offensive or defensive structures (DeLong, 2014; Li et al., 2018; Uiterwaal and DeLong, 2020), influence species interaction strengths. Measuring functional responses, i.e., the relationship between foraging rate and prey abundance, can thus help improve our understanding of predator–prey relationships and use them to generate biocontrol programs that are effective and sustainable (Holling, 1959; DeLong and Uiterwaal, 2021).
A fundamental factor influencing the biological rates of most ectotherms is the temperature (Kim et al., 2020). The impact of temperature on biological rates extends from biochemical reactions to individual physiology to nutrient cycling in ecosystems (Brown et al., 2004; Rall et al., 2012; Yvon-Durocher et al., 2012; Amarasekare, 2015; Islam et al., 2022). The temperature has the potential to modify species interaction strengths and alter energy distribution across food webs. With climate change becoming a major challenge worldwide, interest in determining the effect of temperature on ecological systems has grown (García et al., 2018). Insects are ectotherms, and hence their growth, maturation, reproduction, and trophic interactions can be modified by their thermal surroundings (Amarasekare, 2015; Govindan and Hutchison, 2020). These effects indicate that pest management strategies relying on insects as biocontrol agents against arthropod pests can be altered by temperature changes.
There are three typical types of functional responses (Holling, 1959; DeLong, 2021): a linear rate of predation up to a constant plateau (type I), hyperbolic (type II), or sigmoid (type III), based upon whether the functional response parameters, i.e., the coefficients of space clearance rate (a) and prey handling time (Th), vary with prey abundance. The space clearance rate corresponds to the area cleared of prey per predator per unit of time, whereas handling time measures the loss in search time associated with killing, consuming, and digesting the prey (Pervez, 2005). A high space clearance rate and low handling time are general characteristics of a successful biocontrol agent (DeLong and Uiterwaal, 2021).
Functional responses often are estimated without regard to body size or other traits (Jalali et al., 2010; DeLong et al., 2021). However, variation in prey size owing to ontogeny and individual variation is ubiquitous, and predation rates typically depend on the size of the predator, the prey, or both (Cogni et al., 2002; Mccoy et al., 2011). This variation stems from the effect of predator and prey body size on the functional response parameters (i.e., handling time and space clearance rate) (DeLong, 2014; Uiterwaal and DeLong, 2020; Buba et al., 2021). Generally, handling time increases as prey size increases and decreases as predator body size increases. Space clearance rate may increase with predator body size, while the effect of prey body size on space clearance rate is more variable (Uiterwaal and DeLong, 2020; Buba et al., 2021). Similarly, functional responses often are estimated at only one temperature, despite the widespread effect of temperature on functional response parameters. Across many systems and in several focused studies, the temperature has a unimodal effect on both space clearance rate and handling time (Uszko et al., 2017; Uiterwaal and DeLong, 2020). In smaller comparative studies, however, the temperature may have a monotonic effect on functional responses (Buba et al., 2021; DeLong and Uiterwaal, 2021). It is imperative to consider the temperature in biological control strategies, especially for biological control with non-indigenous natural enemies (Islam et al., 2020, 2021).
Spodoptera litura F. (Lepidoptera: Noctuidae) is a noxious polyphagous pest with a worldwide distribution, attacking many agricultural, horticultural, and ornamental plants (Atwal and Dhaliwal, 2009). It feeds on an extensive host range of 389 species in 109 plant families (Qin et al., 2006). The pest lays eggs in batches, yielding a large population of hatchlings that are gregarious and spread over nearby plants as they grow. The hatchlings develop through 5-7 larval instars (Rao et al., 1989), during which they can inflict huge losses on sensitive crops (Razaq et al., 2019; Shah et al., 2020). The potential for whole crop failures causes farmers to heavily apply pesticides to their crops (Saleem et al., 2016; Shah et al., 2017, 2019). As a result, the pest has developed resistance to synthetic pesticides, leading to control failures and outbreaks (Xie et al., 2010; Xu et al., 2012). With over 638 resistance reports against 39 active pesticide ingredients (Whalon et al., 2012), the management of this pest remains a challenge.
It is therefore necessary to consider alternative approaches, such as biological control with predators, to suppress this pest. About 131 species of natural enemies are reported to feed on S. litura (Rao et al., 1993). Harmonia axyridis (Pallas) (Coleoptera: Coccinellidae) is an important predator of several economically important hemipterans (Huang et al., 2019; Gao et al., 2020) and lepidopterans (Koch et al., 2003; Uiterwaal and DeLong, 2018, 2020; Islam et al., 2020). Recently, in companion studies, we showed temperature-dependent predation and functional responses of H. axyridis to eggs of S. litura, but there are no prior estimates of the functional response of H. axyridis to the larval stages of S. litura. This study estimated the functional responses of H. axyridis foraging on five larval stages of S. litura across a range of temperatures and the body size of the predators. We tested the hypothesis that the functional response interactively depends upon prey size, predator growth stage, and temperature.
Materials and Methods
Rearing of H. axyridis
In January 2019, colonies of H. axyridis and Acyrthosiphon pisum Harris (Hemiptera:Aphididae) were obtained from a collection of adults from a stock culture at the Key Laboratory of Hubei, Insect Resources Utilization and Sustainable Pest Management, located at Huazhong Agricultural University (HZAU), Wuhan, China. The predator was fed with A. pisum, and both cultures were maintained on Vicia faba (L.) Fabaceae (Leguminosae) bean plants inside a growth chamber at 26 ± 1°C, 60–70% RH, and 16:8 h (light:dark) photoperiod (Knapp and Nedvěd, 2013).
Thirty pairs of H. axyridis adults were mated inside 9 ×5 cm2 plastic cups (Yongxin and Plastic Products Guangzhou Co., Ltd: Model No 277), which had fine mesh covering the top for ventilation and crumpled filter papers at the bottom as an oviposition substrate. The adults of H. axyridis were kept inside plastic cups and fed with A. pisum. The oviposition was checked daily. Eggs were removed daily and kept in 6 cm Petri dishes with moistened cotton. Post-emergence hatchlings were subsequently maintained inside similar plastic cups containing aphids for prey that were refreshed daily. Upon reaching sexual maturity, the adults were sexed and the developed progeny was used to establish population culture. In this way, the whole population culture was maintained throughout the trial with individuals from each stage required for this study.
Rearing of Spodoptera litura
The S. litura egg masses were purchased from Henan Jiyuan Baiyun Industrial Co., Ltd, China, in February 2019, and incubated inside the growth chamber at 26°C and 70 ± 5 % RH under a 12:12 h (light: dark) photoperiod. Post-emergence hatchlings were fed with a standard artificial diet (Ul Haq et al., 2015) and cultured until they reached the final instar stage inside mesh-covered transparent circular glass jars (1 L). The artificial diet (semi-solid) consisted of ascorbic acid (2.35 g), distilled water (550 ml), kidney bean flour (150 g), agar (8.4 g), sorbic acid (0.75 g), yeast powder (24 g), methyl-4-hydroxy benzoate (1.5 g), formaldehyde solution (1 ml), and streptomycin (0.75 g). The final instars were isolated and subsequently cultured through maturity inside similar jars that contained moist cotton balls as a pupation media and a 15% glucose solution for food. A clean piece of paper completely covered the jar's bottom as an oviposition substratum (Chang et al., 2020). Oviposition was checked daily, and post-emergence hatchlings were reared together to obtain uniform sets of individuals of the same age. The population culture was established separately for the 1st, 2nd, and 3rd instars. The length of the 1st instar of prey varied from 1.1 to 1.7 mm, whereas that of the 2nd and 3rd instars varied from 2.4 to 3.9 mm and 7.6 to 8.5 mm, respectively (Ramaiah and Maheswari, 2018). The mean weights were 7.31 ×105 ± 2.99 ×10−5 g/larva for 1st instar of prey, 2.95 ×10−4 ± 1.20 ×10−4 g/larva for 2nd instar of prey, and 1.37 ×10−3 ± 5.58 ×10−4 g/larva for 3rd instar of prey S. litura.
Functional Response Experiment
The predators were fed with aphids during development. It was therefore necessary to remove maternal prey effects and acclimatize the predator to the new prey. This was approached by rearing H. axyridis on 1st instar of S. litura for at least two complete generations. The 1st, 2nd, 3rd, and 4th instars, and adult (male and female) individuals of H. axyridis used in this study were of the approximate same age (0-6 h) and were starved singly to stimulate hunger. The starvation period was 6 h for the 1st instar and 24 h for the subsequent instars/stages (Islam et al., 2020). During starvation, humidity was provided inside Petri dishes through moist cotton. The minimum time required between molts was always greater than 48 h for the tested temperatures. This suggests little chance for molting to interfere with functional response experiments over a 24-h period.
Experiments were conducted at five constant temperatures (i.e., 15, 20, 25, 30, and 35°C) under fixed settings of 65 ± 5 % RH and photoperiod of 16:8 (light: dark) inside a computer-controlled growth chamber (Shanghai Xinmiao Medical Device Manufacturing Co., Ltd: Model No QHX-250 BSH-III). The temperature regimes reflect thermal conditions experienced by H. axyridis in various protected plantations and field crops in tropical and temperate areas (Brown et al., 2011). Tomato, Solanum lycopersicum L. (Solanales: Solanaceae), is a suitable host plant for S. litura (Bano and Muqarab, 2017). The experiments were conducted on circular tomato leaflets, cut to fit inside a 9-cm diameter dish, and wrapped at petioles with moist cotton to prevent drying. Three independent prey/predator systems were established in this study. In the first system, predators in all growth stages were allowed to feed on the 1st instar of S. litura. The densities of 1st instar of prey offered to the 1st and 2nd instars of H. axyridis were 3, 6, 10, 15, 20, 25, 35, and 50 larvae/arena, and 5, 10, 20, 30, 40, 50, 60, and 70 larvae/arena, respectively, whereas 3rd and 4th instars, and adult male and female were offered the densities of 25, 50, 75, 100, 150, 200, 250, and 300 larvae/arena. In the second system, older predator instars/stages (i.e., 3rd and 4th instars, and both adults) were given only 2nd instar of S. litura at the densities of 5, 10, 20, 30, 50, 80, 100, and 120 prey larvae/arena. Here, 1st and 2nd instars of H. axyridis were excluded from the candidate list, as they were not found to be able to feed on 2nd instar of prey in the preliminary assessments. In the third system, the predators (i.e., 4th instar and both adults) were offered with the 3rd instar of S. litura at the densities of 2, 4, 8, 16, 32, 40, 45, and 50 prey larvae/arena. Here, 1st, 2nd, and 3rd instars of H. axyridis were excluded from the candidate list, as they could not consume the 3rd instar of prey. A control treatment that only contained prey but no predator was also established for each prey/predator system to account for natural mortality. Within each of these systems, the selection of prey densities offered to the predator was based on preliminary assessments. All experimental treatments were replicated 10 times, and the control treatment had five replicates. One predator was placed on the tomato leaflet inside the arena (9-cm diameter) using a fine camel hairbrush. Prey was pre-released about 30 min before the predator to settle over the substrate. The escape of predator and prey was prevented by wrapping parafilm around the edges of the arena. Larvae consumed by H. axyridis were not replaced during the entire experiment. After 24 h, the larval and adult H. axyridis were removed from the arenas, and the number of prey caterpillars, either damaged, killed, regurgitated, or consumed, was recorded for predation assessment.
Data Analyses
The control mortality of all stages of prey S. litura (1st, 2nd, and 3rd instars) was none to very low (<2%) across all five temperatures, and therefore data were not adjusted for control mortality. Predation of prey by H. axyridis was analyzed using generalized linear models (GLM) with a binomial distribution and a logit link function. Temperature, growth stage, prey size, density, and their interactions were considered as fixed effects. The dependent variable was Ne. As three prey sizes were used, and as prey densities could be confounded by prey size (Uszko et al., 2020), we used the mean weight of each prey in each replicate as a covariate. Non-normal distributions of the data (P > 0.05) were tested by Kolmogorov–Smirnov test. Factors and interaction effects were assessed using the likelihood ratio (LR) chi-square test with a 95 % percent confidence interval (CI). Following the detection of significant effects of prey size (main/interaction), we further tested the effects of temperature, growth stage, density, and their two-way or three-way interactions within each of the three prey sizes offered. Because several tests were carried out to determine the effects of temperature, predator stage, prey instar, and their interaction, we employed the Bonferroni correction to correct for significance, i.e., dividing the standard significance criterion (P <0.05) by the number of tests evaluated. Statistical analyses were performed by using R software (v4.1.2) (R Core Team et al., 2022) for GLM analysis and “car” (Fox and Weisberg, 2011) packages for the likelihood ratio (LR) chi-square test.
Functional Response Assays
We used the two-step procedure recommended by Juliano (2001) to determine the form and parameters of a functional response. The form of the functional response curve was first determined by fitting polynomial logistic regression on the proportion of larvae consumed across larvae densities, as described by:
where Ne and N0 represent the number of prey killed or consumed and initial prey density, respectively, and represents the proportion of prey that was consumed. P0, P1, P2, and P3 are the constant, linear, quadratic, and cubic coefficients of the regression, respectively. We started with a cubic model for fitting the polynomial logistic regression; however, since the parameters of this model are non-significant (P > 0.05), we then eliminated the cubic term and simplified polynomial logistic regression to fit with the quadratic coefficient. These coefficients were calculated using generalized linear models (GLM) with a binomial distribution and a logit link, and a maximum likelihood function with R software (v4.1.2) (R Core Team et al., 2022). Type II and type III functional responses can be distinguished based on the shape of the curves obtained by fitting Equation 1 to the proportional predation data. A type II response is described by a negative linear estimate (decreasing proportional predation with increasing prey density), and a type III functional response by a positive linear estimate followed by a negative quadratic term (increasing but then decreasing proportional predation with increasing prey density) (Juliano, 2001). The type II response indicates that predation declines monotonically with prey density, and a type III response shows that the proportional prey predation is positively density-dependent (Holling, 1959). The logistic regression indicated that the data fit a type II functional response (P1 <0) at all stages and tested temperatures (see Table 1). Thereafter, Roger's “random predator equation” (Equation 2) (Rogers, 1972) was applied, which is appropriate for our experiment without prey replacement (Juliano, 2001). This model is as follows:
where Ne and N0 again represent the number of prey consumed and offered, respectively, α is the space clearance rate, Th is the handling time, and T is the total time available for predators to forage (i.e., 24 h for this study), and it is solved using Lambert's transcendental equation (Bolker, 2008). We used the “frair” R software package to determine the coefficients of space clearance rate and handling time (Pritchard et al., 2017). The 95% confidence intervals (CIs) for space clearance rate and handling time were generated by nonparametric bootstrapping (n = 10,000) again by using “frair” R package. Then we compared fitted coefficients in which non-overlapping 95% CIs are considered significantly different (Pritchard et al., 2017).
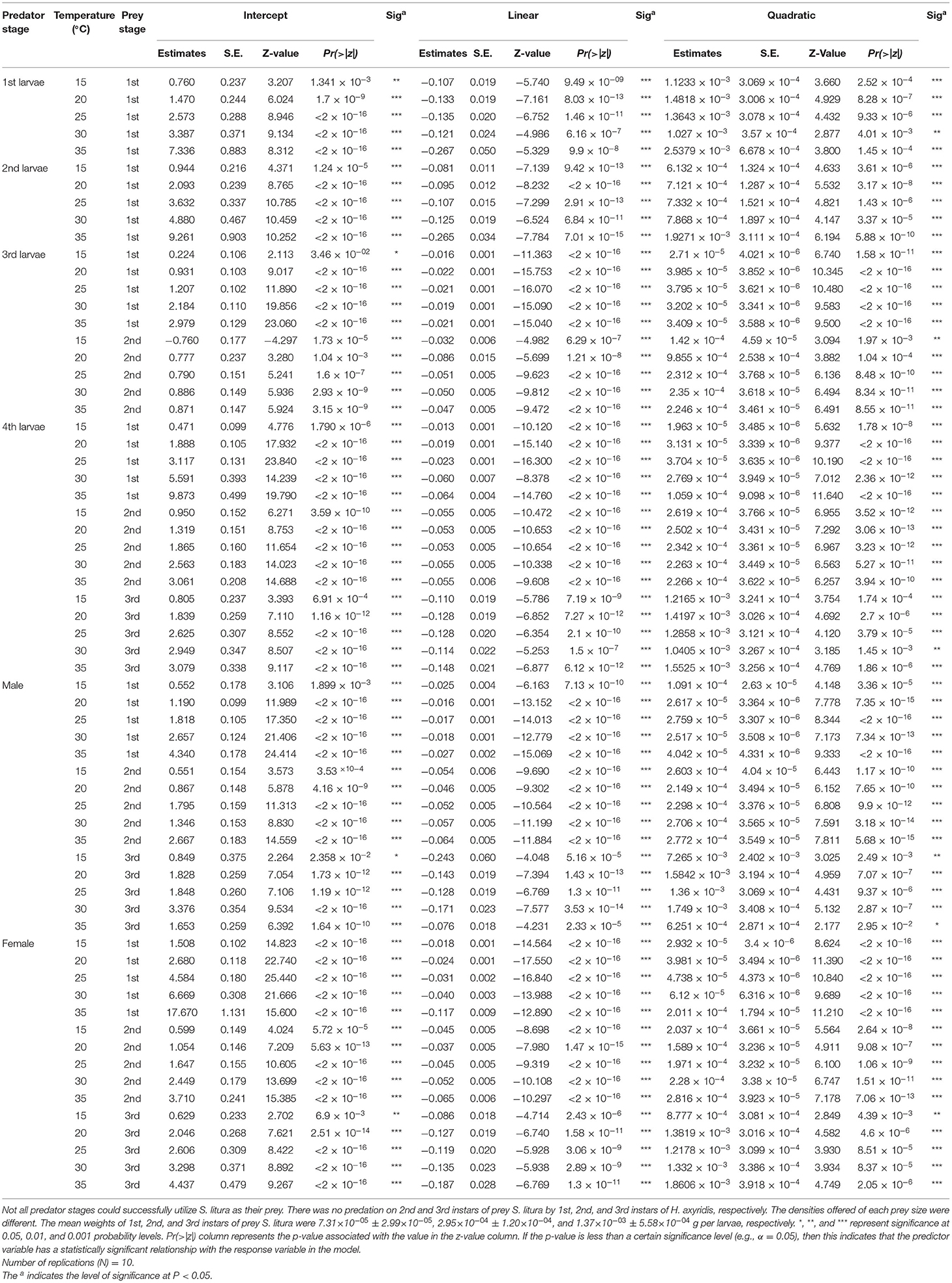
Table 1. Maximum likelihood estimates from logistic regression analyses of the proportion of prey eaten by different stages of Harmonia axyridis preying upon 1st, 2nd, and 3rd instars of prey Spodoptera litura at various temperatures.
Finally, we calculated the maximum predation rate from the estimates of Th. The ratio of 1/Th represents the maximum predation rate considering a predator that spends all its time handling (i.e., 24 h here). The CIs for maximum predation rate were obtained from a set of 20 simulated samples, and 50 datasets (i.e., 50 simulated replicates) were generated for this study by using “simaR” R software package (Benhadi-Marín et al., 2018). Again, significant differences in maximum predation rates among the temperatures were calculated by non-overlapping CIs.
Results
Predation of H. axyridis on S. litura
Predation of H. axyridis on the larvae of S. litura was dependent upon temperature and the size of both predator and prey (all P <0.05, Supplementary Table 1). The rate of predation increased with warming and with advances in the predator stage; however, predation decreased with advances in the prey stage (Figure 1).
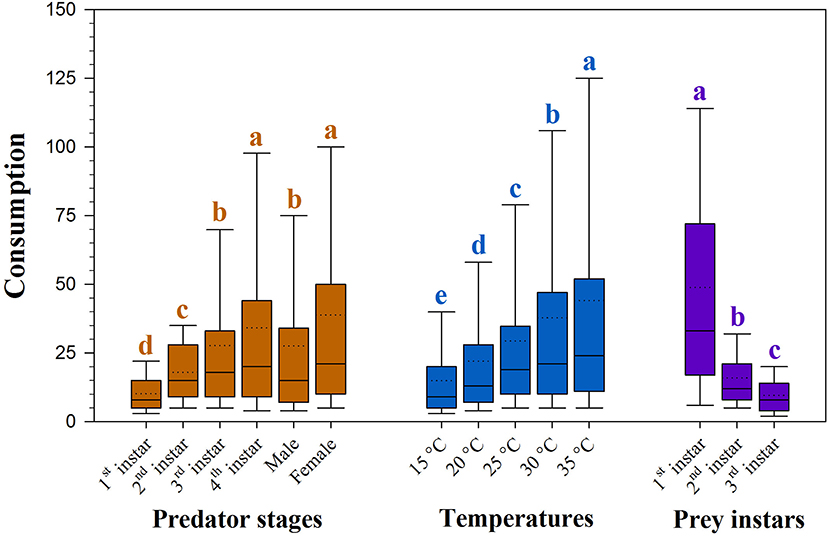
Figure 1. Consumption of prey Spodoptera litura by Harmonia axyridis at five temperatures and three prey instars. Box plots show the range of data (lower and upper quartiles), median (line), and mean (dotted line), and different lowercase letters above the whisker caps indicate significant differences among group mean values according to 95% CI. Number of replications (N) = 10.
Effects of temperature, predator stage, prey density, and their interactions were significant on the prey instars tested (P <0.05, Supplementary Table 2), except for predator stage and prey density interaction on 3rd prey instars (P = 0.1156).
The magnitude of predation was different for the same predator in the three prey systems across the temperatures tested (Figure 2). The mean predation rate for females decreased from 84.88 ± 2.47 larvae/arena to 10.83 ± 0.36 larvae/arena with advances in the prey size from the 1st to the 3rd instar (Figure 2A). The mean rate of prey predation increased from 35.05 ± 1.16 larvae/arena to 73.92 ± 2.58 larvae/arena on the 1st instar of prey S. litura, 8.50 ± 0.23 larvae/arena to 23.43 ± 0.88 larvae/arena on the 2nd instar of prey of S. litura, and 4.86 ± 0.17 larvae/arena to 12.53 ± 0.49 larvae/arena on the 3rd instar of prey S. litura as the temperature increased from 15 to 35°C. Predation was lowest at 15 and 20°C, intermediate at 25 and 30°C, and greatest at 35°C on the 1st and 2nd instars of prey S. litura, whereas on the 3rd instar of prey S. litura, predation was found to be the lowest at 15 and 20°C, intermediate at 30°C and 35°C, and greatest at 25°C (Figure 2B).
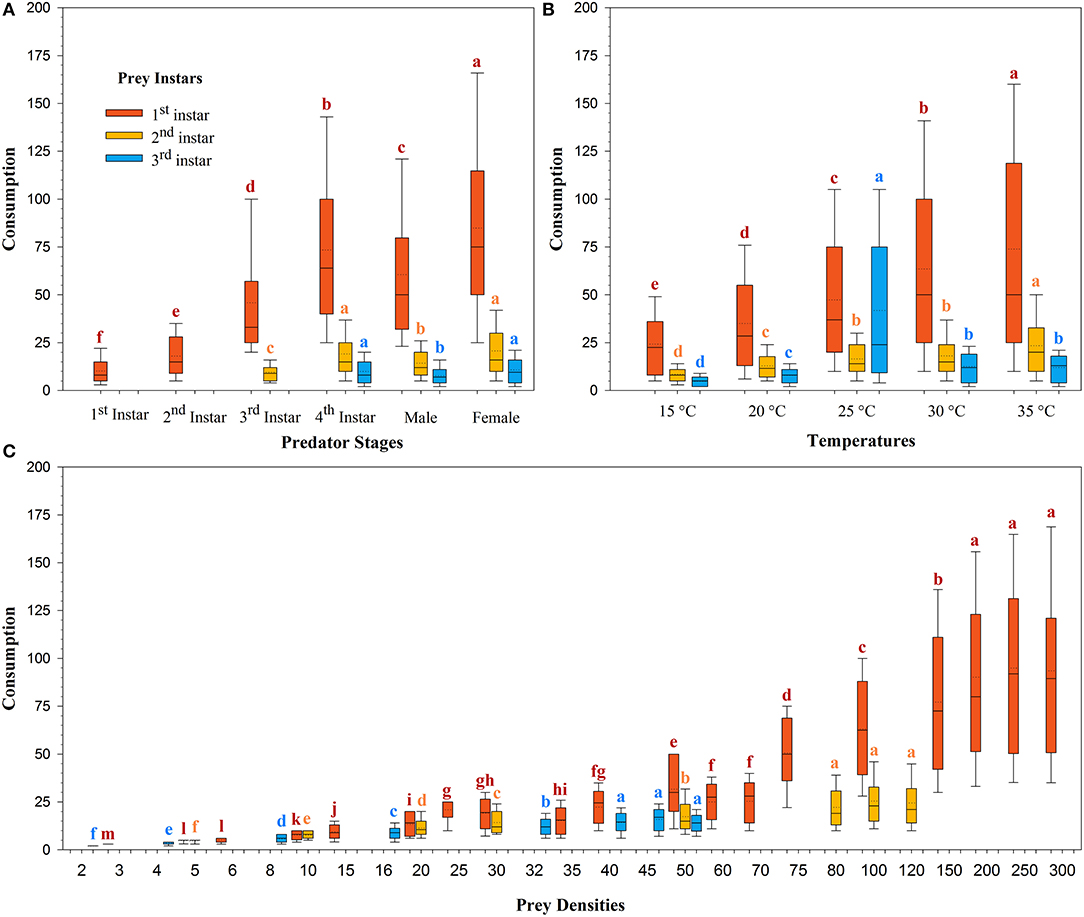
Figure 2. Consumption of prey Spodoptera litura by Harmonia axyridis at five temperatures, according to predator stage (A), temperatures (B), and prey densities offered (C). Box plots show the range of data (lower and upper quartiles), median (line), and mean (dotted line), and different lowercase letters above the whisker caps indicate significant differences among group mean values according to 95% CI. Number of replications (N) = 10.
The rate of prey predation was much higher when feeding on the 1st instar of S. litura than on the 2nd and 3rd instars of S. litura (Figure 1). The highest predation was noted for females, followed by 4th instar and adult males against 1st instar of S. litura. The predation rate of the 4th instar and female H. axyridis was similar in the 2nd and 3rd instars of S. litura. Initial instars of H. axyridis (i.e., 1st and 2nd instar) showed the lowest predation against the 1st instar of S. litura. Likewise, 3rd instar of H. axyridis exhibited the lowest predation rate against the 2nd instar of S. litura (Figure 2A).
The predation rate increased with the initial density of prey offered. The maximum predation rate was noted at densities of 250 (i.e., 94.93 ± 3.37), 300 (i.e., 93.55 ± 3.42), and 200 (i.e., 90.12 ± 3.20) larvae/arena against 1st instar of S. litura. On the 2nd instar of S. litura, the predation was greatest at a density of 100 (i.e., 25.41 ± 0.93), 120 (i.e., 24.47 ± 0.94), and 80 (i.e., 22.12 ± 0.83) larvae/arena, whereas on 3rd instar of S. litura, the predation was greatest at a density of 45 (i.e., 15.84 ± 0.50), 40 (i.e., 14.27 ± 0.46), and 50 (i.e., 14.00 ± 0.44) (Figure 2C).
Functional Response
Logistic regression showed that all predator stages exhibited type II functional responses at all temperatures. This is because the linear estimates of Equation 1 were significantly negative for all stages of predator except for 1st instar and adult female at 35°C (Table 1). The curves show that initially at low prey densities, predation quickly increased across all predatory stages at all tested temperatures but leveled off with a further increase in prey density (Figures 3–5). The proportion of all stages of S. litura consumed by H. axyridis decreased monotonically with prey density offered (Supplementary Figures 1A–M).
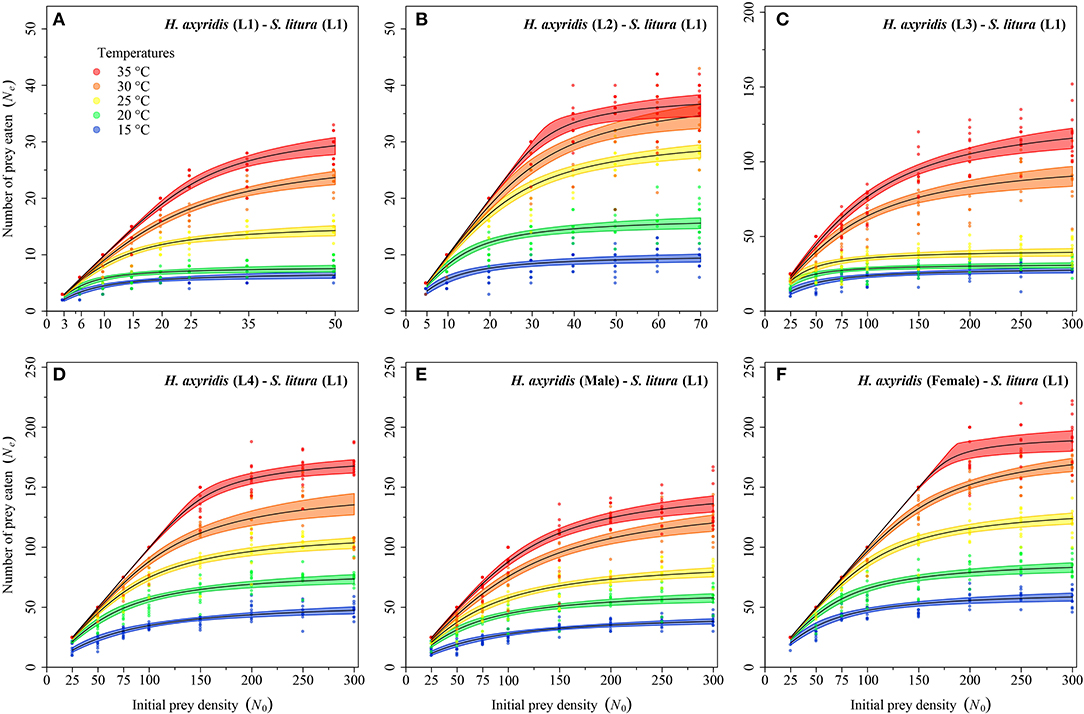
Figure 3. Functional response of different stages of Harmonia axyridis [L1-Female (A–F)] foraging on 1st instar of Spodoptera litura. Dots represent the observed numbers of prey consumed at each initial prey density, and black lines were predicted by the Roger's random predator equation, while the shaded areas represent the limits of the 95% confidence intervals. Number of replications (N) = 10.
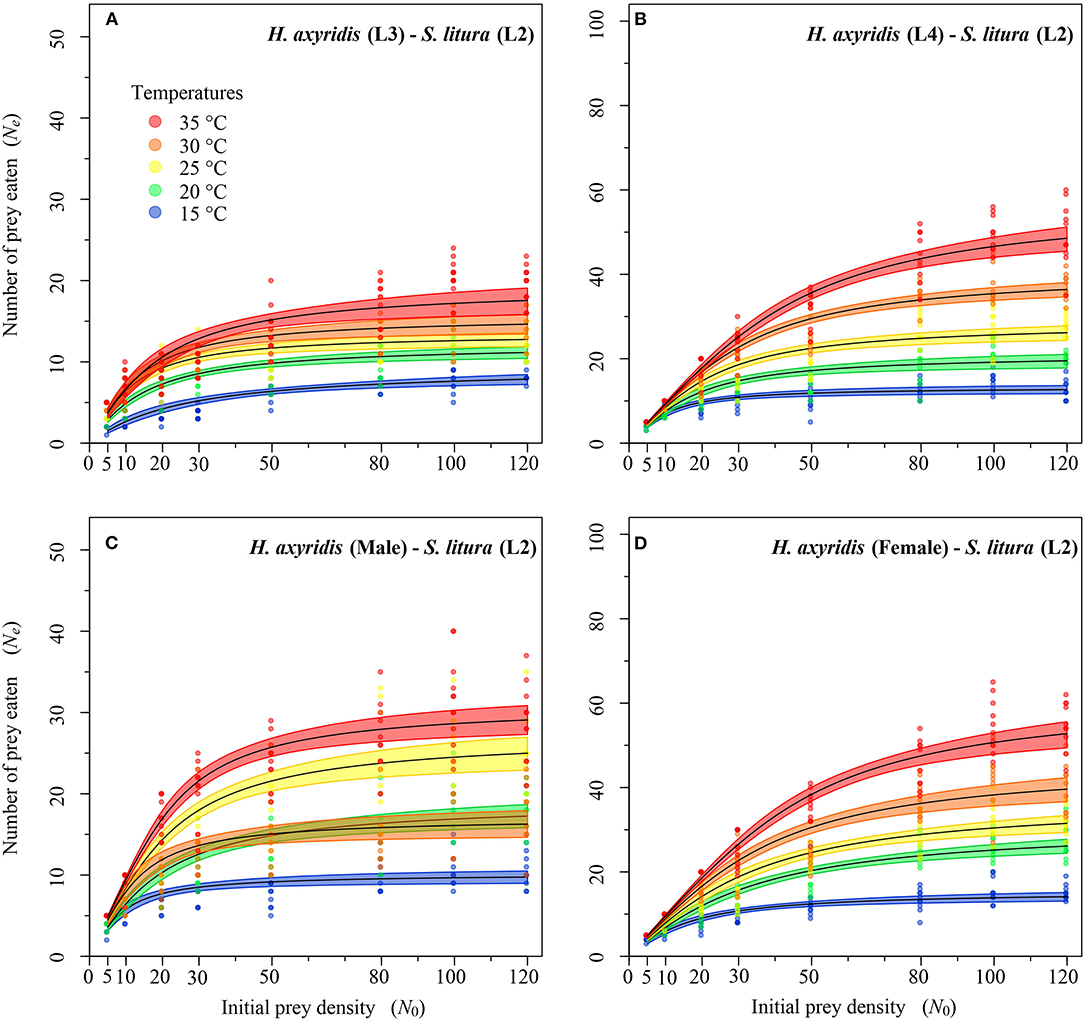
Figure 4. Functional response of different stages of Harmonia axyridis [L3-Female (A–D)] foraging on 2nd instar of Spodoptera litura. Dots represent the observed numbers of prey consumed at each initial prey density, and black lines were predicted by the Roger's random predator equation, while the shaded areas represent the limits of the 95% confidence intervals. Number of replications (N) = 10.
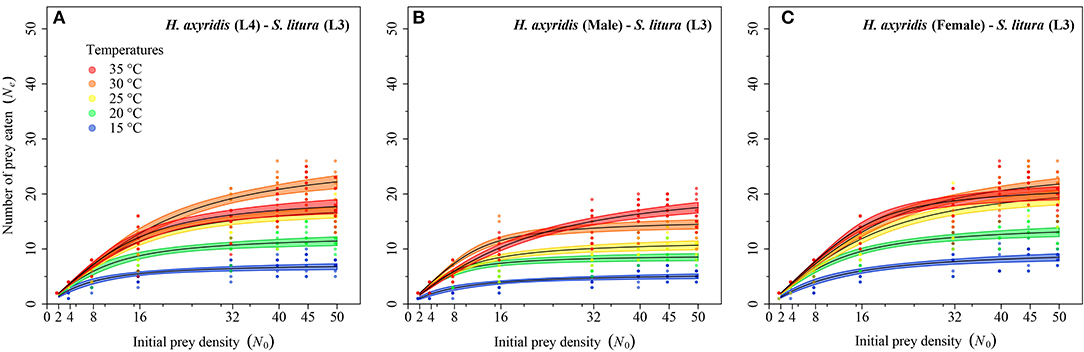
Figure 5. Functional response of different stages of Harmonia axyridis [L4, Female (A–C)] foraging on 3rd instar Spodoptera litura. Dots represent the observed numbers of prey consumed at each initial prey density, and black lines were predicted by the Roger's random predator equation, while the shaded areas represent the limits of the 95% confidence intervals. Number of replications (N) = 10.
The magnitude of the functional response of H. axyridis was considerably different between temperatures and prey instars offered (Figure 6). The space clearance rate decreased (Figures 6A–C) and handling time increased (Figures 6D–F) as the prey size increased. For 1st instar of S. litura, the highest space clearance rate (α) and lowest handling time (Th) was found for females at 35°C. The lowest space clearance rate was shown by the 3rd instar of predator (i.e., 0.018 ± 0.003 h−1) at 15°C against the 2nd instar of prey larvae. The highest handling time against the 1st instar of S. litura was exhibited by the 1st instar of H. axyridis (i.e., 3.457 ± 0.309 h) at 15°C. The highest space clearance rate (i.e., 0.173 ± 0.017 h−1) and lowest handling time (i.e., 0.386 ± 0.013 h) against the 2nd instar of prey S. litura were noted for males and females, respectively, at 35°C. The highest space clearance rate (i.e., 0.239 ± 0.035 h−1) and the lowest handling time (i.e., 0.871 ± 0.047 h) on the 3rd instar of S. litura were exhibited by female and 4th instar of H. axyridis at 35 and 30°C, respectively. The handling time of the 4th instar of H. axyridis was lower than that of the female at the 3rd instar of prey at 30°C.
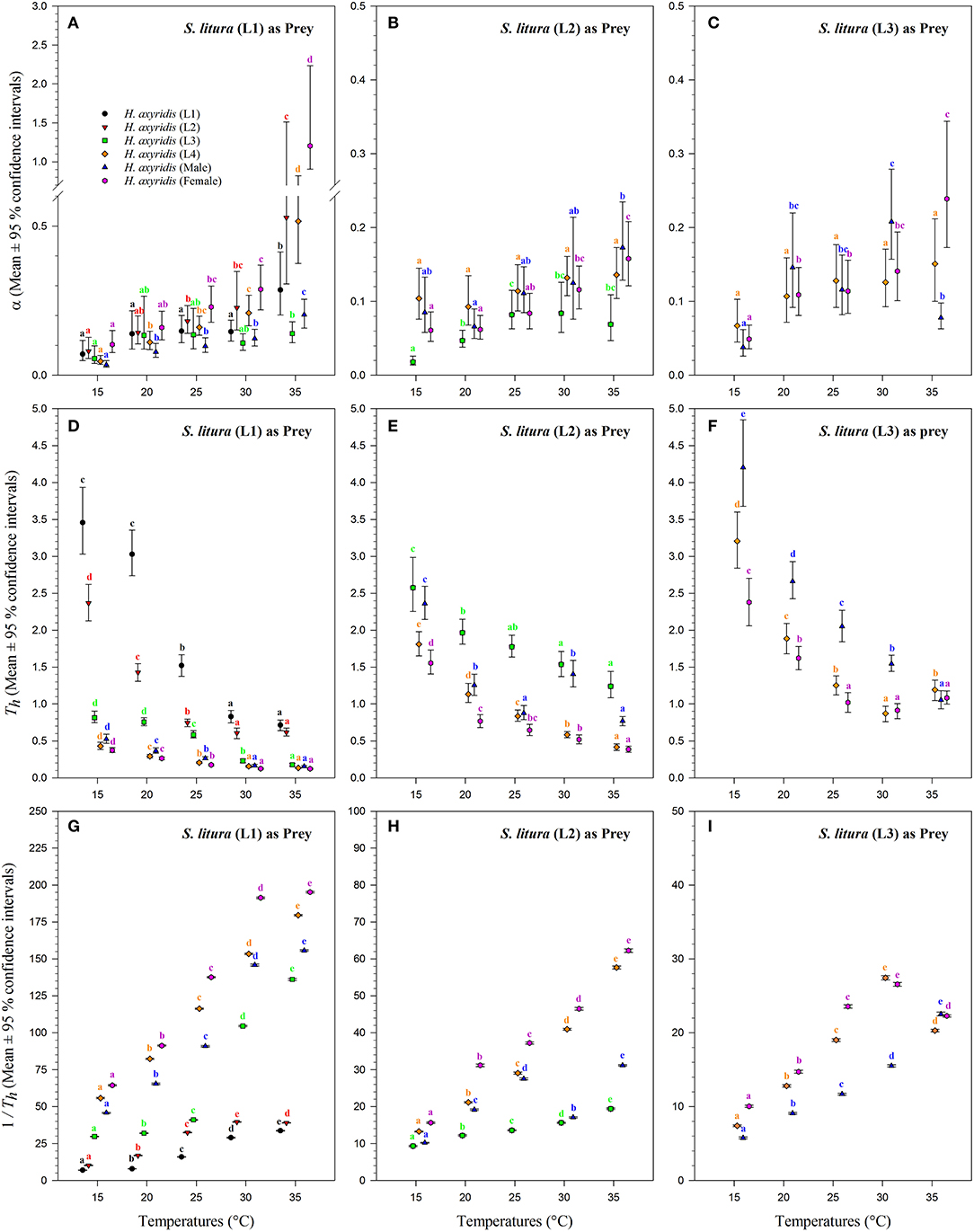
Figure 6. Functional response coefficients of Harmonia axyridis foraging on 1st, 2nd, and 3rd instars of Spodoptera litura under different temperatures. Mean ± 95% confidence intervals of space clearance rate [a (A–C)], handling time [Th (D–F)], and maximum predation rate [1/Th (G–I)] were estimated from bootstrapped data (n = 10,000). Different lowercase letters above the caps indicate significant differences in H. axyridis stages among the temperatures according to 95% CI. Number of replications (N) = 10.
The space clearance rate of 4th instar larvae was higher on the 1st instar of prey (i.e., 0.517 ± 0.039 h−1) than on the 2nd (i.e., 0.136 ± 0.009 h−1) and 3rd instars of S. litura (i.e., 0.150 ± 0.02 h−1) at 35°C. Similarly, the handling time of 4th instar larvae was lower on the 1st instar (i.e., 0.134 ± 0.002 h) than on the 2nd (i.e., 0.416 ± 0.015 h) and 3rd instar larvae of S. litura (i.e., 1.192 ± 0.060 h) at 35°C. At 15°C, the space clearance rates of the 4th instar and adult males were higher than that of the 2nd instar and 1st instar of S. litura.
The maximum predation rate (1/Th) increased with warming and H. axyridis age but decreased with prey age (Figures 6G–I). Overall, the maximum rate was noted for females, followed by 4th instar and adult males against all stages of the prey. For the 1st instar of prey S. litura, the maximum predation rate of females increased from 64.370 ± 0.184 to 195.209 ± 0.238 as the temperature increased from 15 to 35°C. As the prey size increased from the 2nd to the 3rd instar, the maximum predation rate of females and males decreased. The maximum predation rate for both 4th instars also was dependent on the prey size-, and it decreased as the prey size increased. For the 1st instar of prey S. litura, the maximum predation rates were calculated for the 4th instar, males, and females (i.e., 179.535 ± 0.255, 155.651 ± 0.272, and 195.209 ± 0.238, respectively), which were also greater at 35°C.
Discussion
The quantification of biotic interaction strengths is fundamental for understanding population processes (Shaver et al., 2000) and the trophic interactions structuring the food webs (Anderson et al., 2001). H. axyridis is among a group of predators with a worldwide distribution and depends on trophic interactions with agricultural pests. This study demonstrated the broad dependence of the foraging rates of H. axyridis on temperature, prey densities, and both prey and predator body masses (stages). H. axyridis in all growth stages readily preyed upon 1st instar of prey S. litura, but 1st, 2nd, and 3rd instar predators were unable to consume the larger 2nd and 3rd instars of prey, suggesting that predators at initial stages lack the ability to capture or handle bigger prey that is generally more aggressive over their smaller counterparts. Adults (male and female) and the 4th instar of H. axyridis could feed upon all prey sizes, but predation substantially declined with increasing prey size. The 4th instar and adult female H. axyridis consumed more than their male counterparts. Both larval and adult predators foraged more with increasing temperature. This may be explained by the acceleration of biological rates (i.e., metabolism) under warm conditions, which increases both the capacity to do biological work and the demand for resources. Our results are consistent with previous reports (Islam et al., 2020, 2021) that showed heightened predation with warming by the 4th instar and female H. axyridis against S. litura eggs and Acyrthosiphon pisum (Hemiptera: Aphididae) (Harris) and also that they foraged relatively more than males and younger predators. Similar results were shown for Harmonia dimidiata (F.) (Coleoptera: Coccinellidae) preying on Aphis gossypii Glover (Hemiptera: Aphididae) (Yu et al., 2013) and for Podisus maculiventris (Say) and Podisus nigrispinus (Dallas) (Heteroptera: Pentatomidae) preying upon immature Spodoptera exigua (Hubner) (Lepidoptera: Noctuidae) (Mohaghegh et al., 2001).
Our results indicate that the functional response of H. axyridis on S. litura larvae was type II across prey and predator body size (stages) and temperature. Our results are in line with some previous studies with coccinellids where warming did not change the type of functional response, including Scymnus levaillanti Mulsant, Adalia bipunctata L., and Cycloneda sanguinea (L.) (Coleoptera: Coccinellidae) feeding on Aphis gossypii Glover and Myzus persicae (Sulzer) (Hemiptera: Aphididae) (Işikber, 2005; Jalali et al., 2010). However, our results are different than other reports, where Eupeodes corollae (Fabricius) (Diptera: Syrphidae) preying on Spodoptera frugiperda (J. E. Smith) (Lepidoptera: Noctuidae) larvae (Li et al., 2021) showed a type III functional response (density-dependent predation rate) to initial instars of S. frugiperda on fresh maize leaves. The authors suggested the proportion of prey consumed by predators accelerated initially owing to better prey searching by predators in low-density patches. Other mechanisms generating type III functional responses include predator learning, prey switching, predator–prey encounter rates and movements, prey detectability, and intraguild predation (Verdy and Amarasekare, 2010; DeLong, 2021; Bruzzone et al., 2022). These mechanisms, however, do not seem to operate in our predator–prey system, suggesting the need to test for individual predator–prey systems for a better understanding of how temperature drives food webs.
The parameters space clearance rate and handling time determine the height and shape of functional responses (Pervez, 2005; DeLong, 2021). The space clearance rate describes the ability of a predator to capture prey and determines how steeply the functional response curve rises with the increasing prey density. The handling time includes all-time costs that interrupt further searching, including evaluating, catching, pursuit, and predation (Pervez, 2005; DeLong, 2021). Thus, the response of these two parameters to temperature is critical for understanding predator–prey interactions in different locations and in response to climate change. Our findings demonstrate that H. axyridis predators have increased space clearance rates at higher temperatures, indicating that low-density prey populations in warmer environments can be reduced more successfully. Further increases in temperature, however, might be expected to reduce space clearance rate, since predators of many species have their highest feeding rates at intermediate temperatures (Englund et al., 2011; Uszko et al., 2017; Uiterwaal and DeLong, 2020). Typically, predators spend more time handling prey at lower temperatures than at higher temperatures (Mohaghegh et al., 2001; DeLong and Uiterwaal, 2021), and this is consistent with our findings. In our findings, linear estimates of 1st instar and female H. axyridis also exhibited a type II response at 35°C, although the parameters were not significant. The temperature was found to impact changes in the linear estimates, driving higher magnitude functional response by decreasing handling times. Our results are consistent with previous findings where 4th instar and female H. axyridis showed a type II functional response against A. pisum at 30°C even though the linear estimates were not significant (Islam et al., 2021). This temperature effect suggests increased predator effectiveness at warmer temperatures at high prey densities as well. Overall, warming appears to likely improve the effectiveness of H. axyridis on S. litura, but the temperature at which foraging rates begin to decline is currently not known.
Variation in functional response parameters across prey stages suggests that H. axyridis will be effective at limiting only a portion of the S. litura population. Specifically, the space clearance rate of H. axyridis decreased with the larval sizes of S. litura, and handling time increased as prey size increased from the 1st to 3rd instar, indicating that H. axyridis is most effective at limiting smaller and younger S. litura individuals. It is likely more difficult and time consuming for the predator to capture large size prey, possibly due to physical feeding limitations (i.e., gape limitation), while predators may be unwilling to consume prey that is too small due to their poor nutritional value. A possible explanation is that larger prey (2nd and 3rd instars) are more aggressive and difficult to capture. Although observations of the predator's feeding behavior in this study showed that prey of all sizes was swallowed completely, in some cases, 4th instar and adult H. axyridis regurgitated large S. litura larvae several times before finally ingesting them. This behavior could explain the shorter handling times for small prey (Kreuzinger-Janik et al., 2019). The success of any natural enemy, however, cannot be traced solely to its functional response to the target insect in laboratory settings. Natural enemy behavior and efficiency can be affected by the limited size of the arena used in laboratory experiments compared to field circumstances, and also with respect to spatial complexity in nature, host features, biotic and abiotic influences, and numerical response. All these variables must be explored for the species under study for further development of successful biocontrol programs.
In conclusion, the present study found that the functional response of H. axyridis foraging on S. litura larvae was temperature-dependent and type II at all temperatures and stages of the prey. Variation in functional responses suggests that the most effective use of these predators is early in the season to cope with early pest infestation. In the tropics where the infestations of S. litura start in the hot season, this predator may provide effective control due to the steeper and higher functional response in warmer temperatures. Where prey size is large and thus difficult to control for H. axyridis, the use of botanicals that can physiologically stress the pest and possibly reduce their defense could be a viable method for increasing susceptibility to predators. More generally, this strong temperature and body size influence on functional response parameters can lead to important changes in predator–prey relationship, population dynamics, and food web interactions (Sentis et al., 2012). However, the nature of these ecological interactions can be very complex and difficult to predict from laboratory studies. It should be expected that warmer temperatures may favor the development of both the prey and predator but with impacts and concerns that should vary widely. S. litura can develop at a much faster rate under warmer temperatures, but aphids have a weaker developmental response to temperature (Fand et al., 2015; Islam et al., 2021). The development of H. axyridis may be fostered with warmer temperatures, but the life cycle may be disturbed, as the temperatures beyond 35°C did not allow the hatching of H. axyridis eggs (preliminary experiment results). Furthermore, increased development with warmer temperatures reduces the time of the developmental stages, resulting in the predator consuming more prey during a single unit of time compared to its entire life stage. As global warming represents a global challenge, and as parts of the tropics and Asia often experience temperatures above 35°C during summer months (Ma et al., 2021), the release of this predator when temperatures have gone beyond 35°C may affect biocontrol sustainability through effects on predator development and biology. We therefore suggest that current findings should be followed with great caution for sustained control of S. litura, particularly in protected crops and plantations, and when temperatures are between 25 and 35°C. Further studies should address the development and biology of H. axyridis foraging on the eggs and larvae of prey S. litura for developing a more effective biocontrol strategy to manage this serious pest.
Data Availability Statement
The original contributions presented in the study are included in the article/Supplementary Material, further inquiries can be directed to the corresponding author/s.
Author Contributions
FS conceived the idea. XZ supervised the laboratory trials. FS and XZ provided the necessary guidance to conduct this experiment. YI performed the experiments, analyzed the functional response data, and wrote the manuscript partially. FS and AG analyzed the predation and functional response data, prepared figure visuals, and wrote the final manuscript. JD reviewed and edited the manuscript. All authors critically reviewed, discussed, and approved the manuscript for publication.
Funding
This research was funded by the National Key R and D Program of China (2017YFD0201000), the National Natural Science Foundation of China (Grant No. 31872023), and the Key Research Program of Hubei Tobacco Company (027Y2018-008).
Conflict of Interest
The authors declare that the research was conducted in the absence of any commercial or financial relationships that could be construed as a potential conflict of interest.
Publisher's Note
All claims expressed in this article are solely those of the authors and do not necessarily represent those of their affiliated organizations, or those of the publisher, the editors and the reviewers. Any product that may be evaluated in this article, or claim that may be made by its manufacturer, is not guaranteed or endorsed by the publisher.
Acknowledgments
YI thanks Dr. M. Abas Shah (ICAR-Central Potato Research Institute-Regional Station, Jalandhar Punjab, India) for his kind help with data analysis in the R program and Muhammad Asim Rasheed for his help with insect collection and rearing.
Supplementary Material
The Supplementary Material for this article can be found online at: https://www.frontiersin.org/articles/10.3389/fpls.2022.849574/full#supplementary-material
References
Amarasekare, P.. (2015). Effects of temperature on consumer-resource interactions. J. Anim. Ecol. 84, 665–679. doi: 10.1111/1365-2656.12320
Anderson, M. T., Kiesecker, J. M., Chivers, D. P., and Blaustein, A. R. (2001). The direct and indirect effects of temperature on a predator prey relationship. Can. J. Zool. 79, 1834–1841. doi: 10.1139/z01-158
Atwal, A., and Dhaliwal, G. (2009). Agricultural Pests of South East Asia and Their Management. New Delhi: Kalyani Publishers.
Bano, A., and Muqarab, R. (2017). Plant defence induced by PGPR against Spodoptera litura in tomato (Solanum lycopersicum L.). Plant Biol. 19, 406–412. doi: 10.1111/plb.12535
Benhadi-Marín, J., Pereira, J. A., Barreales, D., Sousa, J. P., and Santos, S. A. (2018). A simulation-based method to compare the pest suppression potential of predators: a case study with spiders. Biol. Control 123, 87–96. doi: 10.1016/j.biocontrol.2018.05.007
Bolker, B. M.. (2008). “Ecological models and data in R,” in Ecological Models and Data in R. (New Jersey, USA: Princeton University Press). doi: 10.1515/9781400840908
Brown, J. H., Gillooly, J. F., Allen, A. P., Savage, V. M., and West, G. B. (2004). Toward a metabolic theory of ecology. Ecology 85, 1771–1789. doi: 10.1890/03-9000
Brown, P. M., Thomas, C. E., Lombaert, E., Jeffries, D. L., Estoup, A., and Lawson Handley, L.-J. (2011). The global spread of Harmonia axyridis (Coleoptera: Coccinellidae): distribution, dispersal and routes of invasion. BioControl 56, 623–641. doi: 10.1007/s10526-011-9379-1
Bruzzone, O. A., Aguirre, M. B., Hill, J. G., Virla, E. G., and Logarzo, G. (2022). Revisiting the influence of learning in predator functional response, how it can lead to shapes different from type III. Ecol. Evol. 12, e8593. doi: 10.1002/ece3.8593
Buba, Y., DeLong, J. P., and Belmaker, J. (2021). Synthesizing drivers of fish functional responses across species. Fish Fish. 23, 376–391. doi: 10.1111/faf.12622
Chang, Y., Tang, C.-K., Lin, Y.-H., Tsai, C.-H., Lu, Y.-H., and Wu, Y.-L. (2020). Snellenius manilae bracovirus suppresses the host immune system by regulating extracellular adenosine levels in spodoptera litura. Sci. Rep. 10, 1–11. doi: 10.1038/s41598-020-58375-y
Cogni, R., Freitas, A., and Amaral Filho, B. (2002). Influence of prey size on predation success by Zelus longipes L. (Het., Reduviidae). J. Appl. Entomol. 126, 74–78. doi: 10.1046/j.1439-0418.2002.00593.x
DeLong, J. P.. (2014). The body-size dependence of mutual interference. Biol. Lett. 10, 20140261. doi: 10.1098/rsbl.2014.0261
DeLong, J. P.. (2021). Predator Ecology: Evolutionary Ecology of the Functional Response. Oxford, UK: Oxford University Press.
DeLong, J. P., and Uiterwaal, S. F. (2021). Predator functional responses and the biocontrol of aphids and mites. BioControl. 2022, 1–22. doi: 10.1007/s10526-021-10127-1
DeLong, J. P., Uiterwaal, S. F., and Dell, A. I. (2021). Trait-based variation in the foraging performance of individuals. Front. Ecol. Evol. 9, 394. doi: 10.3389/fevo.2021.649542
Englund, G., Öhlund, G., Hein, C. L., and Diehl, S. (2011). Temperature dependence of the functional response. Ecol. Lett. 14, 914–921. doi: 10.1111/j.1461-0248.2011.01661.x
Fand, B. B., Sul, N. T., Bal, S. K., and Minhas, P. (2015). Temperature impacts the development and survival of common cutworm (Spodoptera litura): simulation and visualization of potential population growth in India under warmer temperatures through life cycle modelling and spatial mapping. PLoS ONE 10, e0124682. doi: 10.1371/journal.pone.0124682
Fardell, L.. (2022). Fear and Stressing in the Urban Environment: Landscape of Fear Effects on Small Prey Animals From Predators and Human Activity. Sydney: University of Sydney.
Fox, J., and Weisberg, S. (2011). An R Companion to Applied Regression, 2nd edition. Thousand Oaks: Sage.
Gao, G., Liu, S., Feng, L., Wang, Y., and Lu, Z. (2020). Effect of temperature on predation by Harmonia axyridis (Pall.) (Coleoptera: Coccinellidae) on the walnut aphids Chromaphis juglandicola Kalt. and Panaphis juglandis (Goeze). Egypt. J. Biol. Pest Control 30, 1–6. doi: 10.1186/s41938-020-00337-7
García, F. C., Bestion, E., Warfield, R., and Yvon-Durocher, G. (2018). Changes in temperature alter the relationship between biodiversity and ecosystem functioning. Proc. Natl. Acad. Sci. 115, 10989–10994. doi: 10.1073/pnas.1805518115
Govindan, B. N., and Hutchison, W. D. (2020). Influence of temperature on age-stage, two-sex life tables for a Minnesota-acclimated population of the brown marmorated stink bug (Halyomorpha halys). Insects 11, 108. doi: 10.3390/insects11020108
Holling, C. S.. (1959). Some characteristics of simple types of predation and parasitism. Can. Entomol. 91, 385–398. doi: 10.4039/Ent91385-7
Huang, Z., Qureshi, J., Zhou, X., Pu, Z., Chen, G., Yu, J., et al. (2019). Predation and functional response of the multi-coloured Asian ladybeetle Harmonia axyridis on the adult Asian citrus psyllid Diaphorina citri. Biocontrol Sci. Technol. 29, 293–307. doi: 10.1080/09583157.2018.1553028
Işikber, A. A.. (2005). Functional response of two coccinellid predators, Scymnus levaillanti and Cycloneda sanguinea, to the cotton aphid, Aphis gossypii. Turk. J. Agric. For. 29, 347–355.
Islam, Y., Güncan, A., Fan, Y., Zhou, X., Naeem, A., and Shah, F. M. (2022). Age-stage, two-sex life table and predation parameters of Harmonia axyridis Pallas (Coleoptera: Coccinellidae), reared on Acyrthosiphon pisum (Harris) (Hemiptera: Aphididae), at four different temperatures. Crop Prot. 2022:106029. doi: 10.1016/j.cropro.2022.106029
Islam, Y., Shah, F. M., Rubing, X., Razaq, M., Yabo, M., Xihong, L., et al. (2021). Functional response of Harmonia axyridis preying on Acyrthosiphon pisum nymphs: the effect of temperature. Sci. Rep. 11, 1–13. doi: 10.1038/s41598-021-92954-x
Islam, Y., Shah, F. M., Shah, M. A., Musa Khan, M., Rasheed, M. A., Ur Rehman, S., et al. (2020). Temperature-dependent functional response of Harmonia axyridis (Coleoptera: Coccinellidae) on the eggs of Spodoptera litura (Lepidoptera: Noctuidae) in laboratory. Insects 11, 583. doi: 10.3390/insects11090583
Jalali, M. A., Tirry, L., and De Clercq, P. (2010). Effect of temperature on the functional response of Adalia bipunctata to Myzus persicae. BioControl 55, 261–269. doi: 10.1007/s10526-009-9237-6
Juliano, S. A.. (2001). “Nonlinear curve fitting: predation and functional response curves,” in Design and Analysis of Ecological Experiments, 2nd ed, eds S. M. Cheiner and J. Gurven. (Oxford: Oxford UniversityPress), p. 178–196.
Kim, K. E., Jang, T., and Lee, K. P. (2020). Combined effects of temperature and macronutrient balance on life-history traits in Drosophila melanogaster: implications for life-history trade-offs and fundamental niche. Oecologia 193, 299–309. doi: 10.1007/s00442-020-04666-0
King, T. W., Vynne, C., Miller, D., Fisher, S., Fitkin, S., Rohrer, J., et al. (2021). The influence of spatial and temporal scale on the relative importance of biotic vs. abiotic factors for species distributions. Divers. Distrib. 27, 327–343. doi: 10.1111/ddi.13182
Knapp, M., and Nedvěd, O. (2013). Gender and timing during ontogeny matter: effects of a temporary high temperature on survival, body size and colouration in Harmonia axyridis. PLoS ONE 8, 74984. doi: 10.1371/journal.pone.0074984
Koch, R. L., Hutchison, W. D., Venette, R., and Heimpel, G. E. (2003). Susceptibility of immature monarch butterfly, Danaus plexippus (Lepidoptera: Nymphalidae: Danainae), to predation by Harmonia axyridis (Coleoptera: Coccinellidae). Biol. Control 28, 265–270. doi: 10.1016/S1049-9644(03)00102-6
Kreuzinger-Janik, B., Brüchner-Hüttemann, H., and Traunspurger, W. (2019). Effect of prey size and structural complexity on the functional response in a nematode-nematode system. Sci. Rep. 9, 1–8. doi: 10.1038/s41598-019-42213-x
Li, H., Jiang, S.-S., Zhang, H.-W., Ting, G., Wyckhuys, K. A., and Wu, K.-M. (2021). Two-way predation between immature stages of the hoverfly Eupeodes corollae and the invasive fall armyworm (Spodoptera frugiperda JE Smith). J. Integr. Agr. 20, 829–839. doi: 10.1016/S2095-3119(20)63291-9
Li, Y., Rall, B. C., and Kalinkat, G. (2018). Experimental duration and predator satiation levels systematically affect functional response parameters. Oikos 127, 590–598. doi: 10.1111/oik.04479
Ma, X., Zhu, J., Yan, W., and Zhao, C. (2021). Projections of desertification trends in Central Asia under global warming scenarios. Sci. Total Environ. 781, 146777. doi: 10.1016/j.scitotenv.2021.146777
Mccoy, M. W., Bolker, B. M., Warkentin, K. M., and Vonesh, J. R. (2011). Predicting predation through prey ontogeny using size-dependent functional response models. Am. Nat. 177, 752–766. doi: 10.1086/659950
Mcintosh, A. R., Leigh, C., Boersma, K. S., Mchugh, P. A., Febria, C., and García-Berthou, E. (2017). “Food webs and trophic interactions in intermittent rivers and ephemeral streams,” in Intermittent Rivers and Ephemeral Streams (Amsterdam: Elsevier), p. 323–347. doi: 10.1016/B978-0-12-803835-2.00012-7
Mohaghegh, J., De Clercq, P., and Tirry, L. (2001). Functional response of the predators Podisus maculiventris (Say) and Podisus nigrispinus (Dallas) (Het., Pentatomidae) to the beet armyworm, Spodoptera exigua (Hübner) (Lep., Noctuidae): effect of temperature. J. Appl. Entomol. 125, 131–134. doi: 10.1046/j.1439-0418.2001.00519.x
Noman, Q. M., Shah, F. M., Mahmood, K., and Razaq, M. (2021). Population dynamics of Tephritid fruit flies in citrus and mango orchards of Multan, Southern Punjab, Pakistan. Pak. J. Zool. 54, 325–330. doi: 10.17582/journal.pjz/20191021181023
Novak, M., and Wootton, J. T. (2010). Using experimental indices to quantify the strength of species interactions. Oikos 119, 1057–1063. doi: 10.1111/j.1600-0706.2009.18147.x
Pervez, A.. (2005). Functional responses of coccinellid predators: an illustration of a logistic approach. J. Insect Sci. 5, 5. doi: 10.1673/031.005.0501
Pritchard, D. W., Paterson, R., Bovy, H. C., and Barrios-O'neill, D. (2017). Frair: an R package for fitting and comparing consumer functional responses. Methods Ecol. Evol. 8, 1528–1534. doi: 10.1111/2041-210X.12784
Qin, H., Wang, D., Ding, J., Huang, R., and Ye, Z. (2006). Host plants of Spodoptera litura. Acta. Agri. Jiangxi. 18, 51–58. doi: 10.19386/j.cnki.jxnyxb.2006.05.017
R Core Team, Bivand, R., Carey, V. J., Debroy, S., Eglen, S., Guha, R., Herbrandt, S., et al. (2022). “R: A Language and Environment for Statistical Computing,” in R Foundation for Statistical Computing (Vienna: Rcore Team).
Rall, B. C., Brose, U., Hartvig, M., Kalinkat, G., Schwarzmüller, F., Vucic-Pestic, O., et al. (2012). Universal temperature and body-mass scaling of feeding rates. Philos. Trans. R. Soc. B. 367, 2923–2934. doi: 10.1098/rstb.2012.0242
Ramaiah, M., and Maheswari, T. U. (2018). Biology studies of tobacco caterpillar, Spodoptera litura Fabricius. J. Entomol. Zool. Stud. 6, 2284–2289.
Rao, G. R., Wightman, J., and Rao, D. R. (1989). Threshold temperatures and thermal requirements for the development of Spodoptera litura (Lepidoptera: Noctuidae). Environ. Entomol. 18, 548–551. doi: 10.1093/ee/18.4.548
Rao, G. R., Wightman, J., and Rao, D. R. (1993). World review of the natural enemies and diseases of Spodoptera litura (F.)(Lepidoptera: Noctuidae). Int. J. Trop. Insect Sci. 14, 273–284. doi: 10.1017/S1742758400014764
Razaq, M., Shah, F. M., Ahmad, S., and Afzal, M. (2019). “Pest management for agronomic crops,” in Agronomic Crops, ed. Hasanuzzaman, M. (Singapore: Springer), p. 365–384. doi: 10.1007/978-981-32-9783-8_18
Rogers, D.. (1972). Random search and insect population models. J. Anim. Ecol. 41, 369–383. doi: 10.2307/3474
Saleem, M., Hussain, D., Ghouse, G., Abbas, M., and Fisher, S. W. (2016). Monitoring of insecticide resistance in Spodoptera litura (Lepidoptera: Noctuidae) from four districts of Punjab, Pakistan to conventional and new chemistry insecticides. Crop Prot. 79, 177–184. doi: 10.1016/j.cropro.2015.08.024
Sentis, A., Hemptinne, J.-L., and Brodeur, J. (2012). Using functional response modeling to investigate the effect of temperature on predator feeding rate and energetic efficiency. Oecologia 169, 1117–1125. doi: 10.1007/s00442-012-2255-6
Shah, F. M., Razaq, M., Ali, A., Han, P., and Chen, J. (2017). Comparative role of neem seed extract, moringa leaf extract and imidacloprid in the management of wheat aphids in relation to yield losses in Pakistan. PloS ONE 12, e0184639. doi: 10.1371/journal.pone.0184639
Shah, F. M., Razaq, M., Ali, Q., Ali, A., Shad, S. A., Aslam, M., et al. (2020). Action threshold development in cabbage pest management using synthetic and botanical insecticides. Entomol. Gene. 40, 157–172. doi: 10.1127/entomologia/2020/0904
Shah, F. M., Razaq, M., Ali, Q., Shad, S. A., Aslam, M., and Hardy, I. C. (2019). Field evaluation of synthetic and neem-derived alternative insecticides in developing action thresholds against cauliflower pests. Sci. Rep. 9, 1–13. doi: 10.1038/s41598-019-44080-y
Shaver, G. R., Canadell, J., Chapin, F. S., Gurevitch, J., Harte, J., Henry, G., et al. (2000). Global warming and terrestrial ecosystems: A conceptual framework for analysis: Ecosystem responses to global warming will be complex and varied. Ecosystem warming experiments hold great potential for providing insights on ways terrestrial ecosystems will respond to upcoming decades of climate change. Documentation of initial conditions provides the context for understanding and predicting ecosystem responses. Bioscience 50, 871–882. doi: 10.1641/0006-3568(2000)050[0871:GWATEA]2.0.CO;2
Uiterwaal, S. F., and DeLong, J. P. (2018). Multiple factors, including arena size, shape the functional responses of ladybird beetles. J. Appl. Ecol. 55, 2429–2438. doi: 10.1111/1365-2664.13159
Uiterwaal, S. F., and DeLong, J. P. (2020). Functional responses are maximized at intermediate temperatures. Ecology 101, e02975. doi: 10.1002/ecy.2975
Ul Haq, R., Khan, J., and Ali, G. (2015). Rearing of Spodoptera litura (Fabricius) on different artificial diets and its parasitization with Trichogramma chilonis (Ishii). Pak. J. Zool. 47, 169–175.
Uszko, W., Diehl, S., Englund, G., and Amarasekare, P. (2017). Effects of warming on predator-prey interactions-a resource-based approach and a theoretical synthesis. Ecol. Lett. 20, 513–523. doi: 10.1111/ele.12755
Uszko, W., Diehl, S., and Wickman, J. (2020). Fitting functional response surfaces to data: a best practice guide. Ecosphere 11, e03051. doi: 10.1002/ecs2.3051
Verdy, A., and Amarasekare, P. (2010). Alternative stable states in communities with intraguild predation. J. Theor. Biol. 262, 116–128. doi: 10.1016/j.jtbi.2009.09.011
Wang, X., and Zou, X. (2017). Modeling the fear effect in predator-prey interactions with adaptive avoidance of predators. Bull. Math. Biol. 79, 1325–1359. doi: 10.1007/s11538-017-0287-0
Whalon, M., Mota-Sanchez, D., Hollingworth, R., and Duynslager, L. (2012). “Arthropod pesticide resistance database,” in Leptinotarsa decemlineata. Available online at: http://www.pesticideresistance.org/display.php
Xie, S., Yanpo, L., and Zhufeng, L. (2010). The toxicity and control efficiency of 9 insecticides to Spodoptera litura. Plant Prot. 36, 175–177. doi: 10.3969/j.issn.0529-1542.2010.04.042
Xu, J., Wang, S., Ma, L., Luo, H., Chang, S., Zhou, X., et al. (2012). Efficacy trials of different types of biological insecticide against Prodenia litura (Fabrwius). Southw. China. J. Agric. Sci. 25, 912–916. doi: 10.16213/j.cnki.scjas.2012.03.018
Yu, J.-Z., Chi, H., and Chen, B.-H. (2013). Comparison of the life tables and predation rates of Harmonia dimidiata (F.) (Coleoptera: Coccinellidae) fed on Aphis gossypii Glover (Hemiptera: Aphididae) at different temperatures. Biol. Control 64, 1–9. doi: 10.1016/j.biocontrol.2012.10.002
Keywords: biocontrol, space clearance rate, handling time, lepidoptera, coccinellids, warming, predation
Citation: Islam Y, Shah FM, Güncan A, DeLong JP and Zhou X (2022) Functional Response of Harmonia axyridis to the Larvae of Spodoptera litura: The Combined Effect of Temperatures and Prey Instars. Front. Plant Sci. 13:849574. doi: 10.3389/fpls.2022.849574
Received: 14 January 2022; Accepted: 03 June 2022;
Published: 01 July 2022.
Edited by:
Xiao Ming Zhang, Yunnan Agricultural University, ChinaReviewed by:
Stéphane Dupas, Institut de Recherche Pour le Développement (IRD), FranceDeyu Zou, Tianjin Academy of Agricultural Sciences, China
Zdenka Svobodová, Biology Centre CAS, Czechia
Gaurav Kumar Taggar, Punjab Agricultural University, India
Copyright © 2022 Islam, Shah, Güncan, DeLong and Zhou. This is an open-access article distributed under the terms of the Creative Commons Attribution License (CC BY). The use, distribution or reproduction in other forums is permitted, provided the original author(s) and the copyright owner(s) are credited and that the original publication in this journal is cited, in accordance with accepted academic practice. No use, distribution or reproduction is permitted which does not comply with these terms.
*Correspondence: Farhan Mahmood Shah, ZmFyaGFuc2hhaDAwMDlAeWFob28uY29t; ZnNoYWhAb2xlbWlzcy5lZHU=; Xingmiao Zhou, eG16aG91QG1haWwuaHphdS5lZHUuY24=
†ORCID: Farhan Mahmood Shah orcid.org/0000-0002-6123-1860
Ali Güncan orcid.org/0000-0003-1765-648X
John Paul DeLong orcid.org/0000-0003-0558-8213
‡Present address: Farhan Mahmood Shah, National Center for Natural Products Research, University of Mississippi, University, Oxford, MS, United States