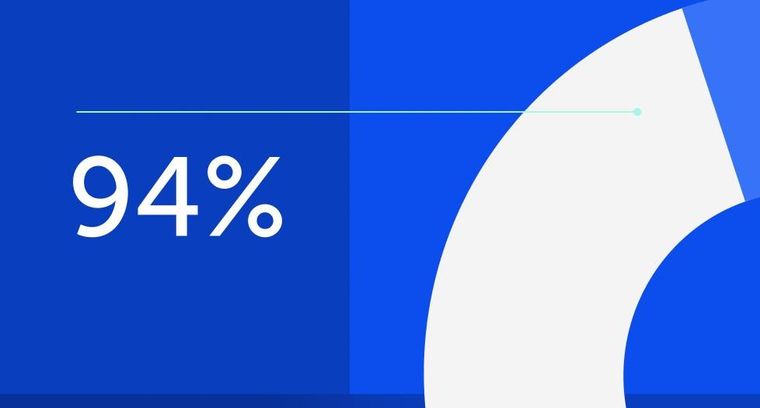
94% of researchers rate our articles as excellent or good
Learn more about the work of our research integrity team to safeguard the quality of each article we publish.
Find out more
ORIGINAL RESEARCH article
Front. Plant Sci., 28 March 2022
Sec. Plant Abiotic Stress
Volume 13 - 2022 | https://doi.org/10.3389/fpls.2022.848766
This article is part of the Research TopicDrought Stress in LegumesView all 9 articles
Drought is a major environmental constraint that causes substantial reductions in plant growth and yield. Expression of stress-related genes is largely regulated by transcription factors (TFs), including in soybean [Glycine max (L.) Merr.]. In this study, 301 GmAP2/ERF genes that encode TFs were identified in the soybean genome. The TFs were divided into five categories according to their homology. Results of previous studies were then used to select the target gene GmAP2/ERF144 from among those up-regulated by drought and salt stress in the transcriptome. According to respective tissue expression analysis and subcellular determination, the gene was highly expressed in leaves and encoded a nuclear-localized protein. To validate the function of GmAP2/ERF144, the gene was overexpressed in soybean using Agrobacterium-mediated transformation. Compared with wild-type soybean, drought resistance of overexpression lines increased significantly. Under drought treatment, leaf relative water content was significantly higher in overexpressed lines than in the wild-type genotype, whereas malondialdehyde content and electrical conductivity were significantly lower than those in the wild type. Thus, drought resistance of transgenic soybean increased with overexpression of GmAP2/ERF144. To understand overall function of the gene, network analysis was used to predict the genes that interacted with GmAP2/ERF144. Reverse-transcription quantitative PCR showed that expression of those interacting genes in two transgenic lines was 3 to 30 times higher than that in the wild type. Therefore, GmAP2/ERF144 likely interacted with those genes; however, that conclusion needs to be verified in further specific experiments.
Plants are regularly exposed to environmental stresses during their life cycle that include drought, low temperature, saline alkali, diseases, and insect pests (Bhat et al., 2020). Under pressure from environmental stresses, plants evolved and formed complex signal transduction networks (Li et al., 2020). In a signal transduction network, various stress signals are identified and then expression of downstream related-genes is stimulated, allowing plants to survive in complex environments. Under stress conditions, transcription factors (TFs) have key roles in expression and regulation of functional genes. Transcription factors, also known as trans-acting factors, are DNA proteins that specifically bind to cis-acting elements in the promoter region of eukaryotic genes and activate or inhibit expression of downstream genes. In the Arabidopsis genome, more than 1,500 genes encode TFs, including those involved in regulating plant stress responses, such as AP2/ERF, bZIP, NAC, WRKY, and MYB (Mizoi et al., 2012).
Jofuku et al. (1994) isolated the first AP2 gene from the model plant Arabidopsis thaliana, which is associated with flower development and contains two AP2/ERF (Apetala2/ethylene responsive factor) domains. Ohme-Takagi and Shinshi (1995) isolated ethylene response element binding proteins (ERF1, 2, 3, 4) from Nicotiana tabacum L., which contain a conserved ERF domain. Kagaya et al. (1999) isolated full-length cDNA sequences of RAV1 and RAV2 genes from Arabidopsis thaliana, which encode proteins that contain an AP2/ERF domain and a B3 domain. Subsequently, in-depth research has been conducted on the AP2/ERF gene family in many species (Hu et al., 2020). Faraji et al. (2020) used RNA-seq analysis to conduct a detailed study on tpap2s/ERFs in the genome of durum wheat (Triticum turgidum ssp. durum) and identified 271 genes. They further analyzed the potential of the genes to affect resistance to drought and salt stress (Faraji et al., 2020). Li et al. (2020) identified 178 AP2/ERF genes in eggplant. Eggplant RNA-seq data on anthocyanin biosynthesis were combined with yeast single-hybridization and double-luciferase analysis to determine that smap2/ERF genes (Smechr0902114.1 and Smechr1102075.1) were involved in regulation of anthocyanin biosynthesis (Li et al., 2021). Zhou and Yarra (2021) used genome-wide analysis to identify 172 EgAP2/ERFs (AP2, ERF, RAV, and Soloist subfamily members) in oil palm. Quantitative PCR analysis validated abiotic stress (salinity, cold, and drought)-responsive AP2/ERFs in the oil palm genome (Zhou and Yarra, 2021).
The AP2/ERF TFs are composed of four main functional domains: DNA binding domain, transcriptional regulatory domain, oligomerization site, and nuclear localization signal region. Members of this family contain one or more AP2/ERF domains (Jofuku et al., 1994). The domain is composed of 60 highly conserved amino acid sequences, which can recognize and bind DNA. Each AP2/ERF binding domain contains two conserved amino acid sequences, YRG and RAYD elements. An extremely basic element, YRG is composed of 19 to 22 amino acid residues, and it contains conserved YRG amino acid motifs. In addition, this region contains three β-foldings that are important in identifying various cis-acting elements, which is in the second β-folding. The difference between the 14th and 19th amino acid residues in the folding determines the specific binding of these TFs to different cis-acting elements. The RAYD element contains 42 to 43 amino acid residues, and the core sequence is composed of 18 highly conserved amino acid residues, which can form an amphiphilic α-helix that is involved in the interaction between AP2/ERF TFs and those of other genes (Yamasaki et al., 2008).
Soybean (Glycine max (L.) Merr.) provides edible protein and vegetable oil for human consumption (Holle and Damme, 2015) and is a crop with high economic value. As research on soybean genetics and genomics has increased, research on the AP2/ERF gene family has also gradually increased. For example, GmERF057 can improve soybean resistance to salt and pathogens (Zhang et al., 2008), and GmERF3 regulates soybean biological and abiotic stress responses (Zhang et al., 2009). The gene GmDREB2 participates in abscisic acid (ABA)-dependent and independent signaling pathways and can induce expression of downstream genes such as RD29A and COR15a to increase soybean resistance to drought and high-salt environments (Chen et al., 2007). However, few studies have conducted genome-wide analysis of the AP2/ERF gene family. Simultaneously with the continuous improvement in information on the soybean genome, predicted gene numbers and gene structures have also changed. Therefore, it is necessary to analyze and identify members of the soybean AP2/ERF gene family (Mizoi et al., 2012).
In this paper, members of the soybean AP2/ERF gene family were identified and classified by using an HMM profile (pf00847) database (Jin et al., 2014). Then, the genes were analyzed and annotated. Based on published transcriptome data on salt tolerance and drought resistance, target genes were screened by transcriptome analysis, and then, stable transformation of soybean was performed. The gene improved drought resistance of transgenic soybean under stress. Thus, this study provides an important theoretical basis to understand the functions of AP2/ERF genes in soybean. Because of the increasing negative effects of stress on crop growth and development, a stress-resistance gene from the soybean genome was cloned in this study in order to provide an important gene resource and theoretical basis to improve stress resistance through genetic engineering (Xie et al., 2019). However, mechanisms by which plant cells sense and transmit stress signals and regulate downstream genes under drought stress are not clear. Therefore, it is very important to study AP2/ERF encoded TFs under drought stress in order to better understand drought resistance response mechanisms and ultimately cultivate drought-resistant soybean varieties. Such investigations will help further studies on roles of members of this gene family in soybean growth and development, stress response, and production breeding (Mizoi et al., 2012).
To identify all AP2/ERF genes in soybean, the AP2/ERF HMM profile (PF00847) was used as a query (Chen et al., 2020; Faraji et al., 2020). After removal of redundant and incomplete ORF sequences, the SMART database1 was used to eliminate sequences that did not contain a complete AP2/ERF domain. Molecular weight (MW), isoelectric point (pI), and amino acid (aa) number of GmAP2/ERF proteins were obtained from ExPASy2 online tools. The software CELLO3 was used to predict GmAP2/ERF gene subcellular localization.
The software TBtools (v1.0692) was used to draw gene structures by comparing cDNA sequences with the corresponding genomic DNA sequences of their GmAP2/ERF transporter members (Wang et al., 2017; Huang et al., 2021).
The online motif-finding tool MEME 4.11.24 was used to identify conserved motifs in TFs of AP2/ERF genes. Parameters were as follow: 6–200, optimum width of amino acids; 25, maximum number of motifs; and 0 or 1 single motif in each sequence of the model.
All sequences of proteins encoded by GmAP2/ERF genes and those of 29 previously reported GmAP2/ERF transporters from Arabidopsis thaliana were used for multiple sequence alignments in MAFFT 7.05. An unrooted phylogenetic tree was constructed using the neighbor-joining method in MEGA 6 with the following parameters: bootstrap value of 1,000, Poisson correction, and pairwise deletion (He et al., 2016; Li et al., 2021).
According to the soybean genome annotation file, the 300-kb hereditary interval gene densities were obtained and then further transformed into a gradient-colored heat map on soybean chromosomes or scaffolds. Tbtools was used to display chromosomal locations of GmAP2/ERF genes (Tian et al., 2020). Gene duplication events were analyzed by MCScanX in Tbtools and visualized in CIRCOS using default parameters. To evaluate selection pressure, ratios of non-synonymous (Ka) substitutions to synonymous (Ks) substitutions of each duplicated GmAP2/ERF gene were calculated by the NG method in TBtools. Values of Ks > 2.0 were discarded to avoid saturation of substitutions. Occurrence time of duplicated GmAP2/ERF gene pairs was calculated as follows: T = Ks/(2λ × 10–6), where λ = 6.5 × 10–9 (Blanc and Wolfe, 2004; Zhou and Yarra, 2021).
Soybean cultivars Qihuang22 and Tianlong No.1 were used in different experiments. Qihuang22 was used to isolate the GmAP2/ERF144 gene, and Tianlong 1 (wild type, WT) was the recipient parent to develop overexpression (OE) lines. Plants were grown in a controlled-environment growth chamber at 26/23°C and 70% relative humidity under a 16-h light/8-h dark photoperiod. Fourteen-day-old soybean seedlings were subjected to drought stress by withholding water supply. Root, stem, and leaf samples were harvested at 0, 2, 4, 6, and 8 d following drought treatment of seedlings, with samples subsequently frozen quickly in liquid nitrogen (Borges et al., 2012; Yang C. et al., 2020; Wang et al., 2021).
To determine whether GmAP2/ERF144 could regulate the drought response of soybean, three groups of experiments were conducted. Two strains with good drought performance were selected for the test. Steps in the process of transgenic verification of GmAP2/ERF144 are presented in Supplementary Figure 4. Wild-type Tianlong 1 and GmAP2/ERF144 overexpression lines were planted under the same conditions, and drought tests were conducted when soybean compound leaves with three leaflets were fully unfolded (approximately 2 weeks). Three types of drought tests were conducted: (1) a single plant of each soybean type (WT, OE1, OE2) was dry for 18 d and then rehydrated for 7 d; (2) after 16 d of drought, two plants of each soybean type (WT, OE1, OE2) were rehydrated for 7 d; and (3) a single plant of each soybean type (WT, OE1, OE2) naturally dry for 28 d was rehydrated for 7 d.
Total RNA was isolated from soybean roots, stems, and leaves using the protocol of an RNAprep Pure Plant Kit (Tiangen, Beijing, China). Purity and concentration of total RNA were determined using a Nanodrop UV spectrophotometer and an RNA Nano chip on an Agilent Bioanalyzer 2100, respectively. The cDNA was synthesized using a Prime Script™ RT Reagent Kit (TaKaRa, Dalian, Japan) following a standard protocol. Reverse-transcription quantitative PCR (RT-qPCR) was performed for each cDNA template using AceQ qPCR SYBR Green Master Mix (Vazyme, Nanjing, China) according to a standard protocol. Amplification conditions for PCRs were 95°C for 3 min followed by 40 cycles of 95°C for 10 s and 60°C for 30 s. In RT-qPCR assays, three biological replications were used, and three measurements were performed on each replicate. Results from PCRs were normalized using the Ct value corresponding to the soybean actin gene GmActin11 (Glyma.18g290800) as the internal control. Specificity of reactions was verified by melting curve analysis, and relative mRNA level for each gene was calculated as follows: ratio = 2–ΔΔCt = 2–[Ct,t–Ct,r] (Ctcycle threshold: Ct, tCt of the target gene; Ct, rCt of the control gene) (Huang et al., 2019). The NCBI Primer BLAST was used to design all primers (Supplementary Table 2). Reverse-transcription qPCR was conducted on a Light Cycler 480 instrument (Bessire et al., 2011).
To determine subcellular location of the protein encoded by GmAP2/ERF144, first, cNLS online software6 was used to analyze the amino acid sequence and then predict the nuclear localization signal (NLS) of GmAP2/ERF144. To further verify localization of the protein encoded by GmAP2/ERF144 within plant cells, the conserved domain sequence of the GmAP2/ERF144 gene without the terminator was cloned into a pBIN-GFP4 vector to construct a fusion vector, following the manufacturer’s protocol (Supplementary Figure 2). When sequencing was successful, constructs and empty bodies were transiently transformed into tobacco leaves. Leaf fluorescence was observed under a Zeiss LSM 880 Upright Confocal Microscope (Carl Zeiss, Thornwood, NY, United States) at 48 to 60 h post-inoculation. Excitation wavelengths were 488 nm for green fluorescent protein (GFP) and 405 nm for 4′,6-diamidino-2-phenylindole (DAPI; Yang et al. 2020).
The full-length coding region of GmAP2/ERF144 was cloned into a pTF101.1 vector for overexpression of GmAP2/ERF144 under the control of CaMV 35S promoter (35S:GmAP2/ERF144). Primers are listed in Supplementary Table 2. The 35S:GmAP2/ERF144 construct and pTF101.1 empty vector control were individually transformed into A. tumefaciens strain EHA101 via electroporation. Tian Long No. 1 was used for tissue culture and transformation according to a previously reported protocol (Huang et al., 2019). The T3 homozygous lines were used for phenotypic investigation. To confirm the transgenic lines, PCR and RT-qPCR were used to verify GmAP2/ERF144 overexpression in transgenic plants. The basta was also used to screen soybean seedlings with three fully expanded compound leaves.
To predict the proteins interacting with GmAP2/ERF144, each protein was separately submitted to the STRING database7. Online prediction software displayed both experimentally demonstrated and hypothetical proteins interacting with GmAP2/ERF144 (Noman et al., 2019). Primers are listed in Supplementary Table 2.
To identify all AP2/ERF genes in soybean, the AP2/ERF HMM profile (PF00847) was used as a query. In the HMM search of the G. max genome, 301 GmAP2/ERF genes were identified. The GmAP2/ERF genes were named in sequence from GmAP2/ERF001 to GmAP2/ERF301 by following the classification principles/criteria of A. thaliana (Supplementary Table 1). Length of amino acid sequences of GmAP2/ERF proteins varied from 144 aa to 710 aa, with corresponding molecular weights ranging from 15,961.1 Da to 77,512.2 Da. Predicted theoretical points (pI) ranged from 4.26 to 11.66. To classify GmAP2/ERF proteins, full-length sequences of the 301 GmAP2/ERF proteins and those of 29 AtAP2/ERF proteins of Arabidopsis were aligned, and a phylogenetic tree was constructed using the neighbor-joining method (Figure 1).
Figure 1. Phylogenetic tree of GmAP2/ERF proteins in soybean and Arabidopsis. Five subfamilies are highlighted by different colors. Arabidopsis is marked with a blue pentagram.
According to the phylogenetic tree and homology, the 44 proteins containing two AP2 domains were closely related to the AP2 subfamily of proteins. Four proteins containing one AP2 domain and one B3 domain were classified in the RAV subfamily of proteins. However, 98 proteins were classified in the DREB subfamily of proteins and 153 proteins were classified in the ERF subfamily of proteins. GmAP2/ERF300 and GmAP2/ERF301 showed homology with AT4G13040 and therefore were classified as members of the Soloist subfamily of proteins. Additionally, according to the classification criteria of A. thaliana ERF and DREB subfamilies, the corresponding subfamilies in G. max could be divided into six groups (Figure 1). The DREB subfamily had 12, 11, 2, 36, 21, and 16 members in the A1, A2, A3, A4, A5, and A6 subgroups, respectively, and the ERF subfamily had 45, 9, 17, 19, 16, and 47 members in the B1, B2, B3, B4, B5, and B6 subgroups, respectively. Thus, DREB and ERF subfamilies of GmAP2/ERF encoded proteins were dominant in G. max.
To examine the structural diversity of GmAP2/ERF genes, number of exon–introns and distribution of conserved domains of GmAP2/ERF genes were investigated. Number of introns among different GmAP2/ERF subfamilies varied markedly. Most DREB and ERF genes did not have introns. All AP2 and Soloist genes had from three to nine introns, whereas in the RAV subfamily, genes did not contain introns. The GmAP2/ERF genes classified in the same subfamily showed similar gene structures.
To better illustrate conserved domain patterns of GmAP2/ERF gene members, MEME was used. The GmAP2/ERF genes within the same subfamily displayed similar motif compositions. Most AP2 subfamily members contained motif-10, motif-2, motif-3, and motif-5, whereas ERF subfamily members contained motif-6 and motif-5. Most DREB subfamily members contained motif-2 and motif-5, whereas RAV subfamily genes contained motif-7, moti-8, motif-1, and moif-4 (Supplementary Figures 1A–D).
The 301 GmAP2/ERF genes were mapped on the 20 chromosomes and scaffold_21 and scaffold_44 of the soybean genome. The highest number of genes was 25 on Chr13, whereas scaffold_21 and scaffold_44 each contained one gene. By contrast, Chr09 contained the fewest genes (only nine). There were 11 genes on Chr04 and Chr12 and 14 genes on Chr05, Chr06, Chr11, and Chr19. Coincidentally, there were 12 genes on adjacent chromosomes 14, 15, and 16. There were 17 genes distributed in the scaffolds of Chr01 and Chr08. The distribution of the 301 genes on the 20 chromosomes was relatively balanced (Figure 2A).
Figure 2. Chromosomal distributions of GmAP2/ERF genes and schematic of interchromosomal relations. (A) Chromosomal distributions of GmAP2/ERF genes. Chromosomal (Chr) names in yellow are on the left, and gene names are on the right. Left-side scale is in megabases (Mb). Gradient colors from red (high) to blue (low) indicate gene density in heat maps on soybean chromosomes, by setting the estimated hereditary interval as 300 kb. Red indicates high gene density, and blue indicates low gene density. (B) Schematic of interchromosomal relations of soybean GmAP2/ERF genes. Green lines indicate all syntenic blocks in the soybean genome. Heat maps represent the gene density.
Gene duplication is an important event leading to amplification of gene families. To elucidate the mechanism of expansion of GmAP2/ERF genes, gene duplication event analysis was performed, and details of duplicated gene pairs are presented in Figure 2B. A chromosomal region within 200 kb containing two or more genes is defined as a tandem duplication event. In this study, six tandem duplication events associated with four GmAP2/ERF genes (GmAP2/ERF209/GmAP2/ERF208, GmAP2/ERF210/GmAP2/ERF208, GmAP2/ERF208/GmAP2/ER F211, GmAP2/ERF210/GmAP2/ERF209, GmAP2/ERF211/GmAP2/ERF209, GmAP2/ERF211/GmAP2/ERF210) were detected on Chr14. By contrast, segmental duplications result in a large amount of duplicated chromosomal blocks in a genome and often occur during polyploidization events with chromosome rearrangements. In the soybean genome, 96 segmental duplication events associated with 180 GmAP2/ERF genes were identified (Figure 2B). Genes that undergo tandem and segmental duplication events are closely related genetically, and thus, these results provide a potential reference for functional prediction.
The cis-elements in promoter regions are important in regulating gene transcription and abiotic stress responses. Therefore, promoter region sequences (i.e., 2-kp upstream sequences from gene initiation codons) of GmAP2/ERF genes were subjected to cis-element analysis. Cis-elements included ABA-responsive elements (ABRE), drought-inducible elements (MBS, MYB binding site), low-temperature responsive elements (LTR), MEJA-responsive elements (CGTCA-motif), SA-responsive elements (TCA-element), and defensive and stress responsive elements (TC-rich repeats). Cis-elements are displayed by proportion in Supplementary Figure 2. Among the elements, ABRE, MBS, and CGTCA-motif were detected in almost every promoter region of the GmAP2/ERF genes. However, correlations between cis-elements and gene responses to abiotic stresses need further experimental validation to determine whether GmAP2/ERF genes respond abiotic stresses.
Genes in the AP2/ERF family can be induced by various abiotic stresses in some species. To determine expression of GmAP2/ERF genes in response to abiotic stress, GmAP2/ERF gene expression profiles were examined in soybean under saline stress and drought by using previously published RNA-seq data8. Based on RNA-seq results, 152 and 112 GmAP2/ERF genes responded to drought and saline stress, respectively. Of those genes, 67.1% (102/152) and 81.25% (91/112) were up-regulated under drought and saline stress, respectively. Therefore, 50 and 21 genes were down-regulated under drought and saline stress, respectively.
Thirty-nine genes were up-regulated under both abiotic stresses, of which six were significantly up-regulated, including GmAP2/ERF192, GmAP2/ERF272, GmAP2/ERF158, GmAP2/ERF167, GmAP2/ERF144, and GmAP2/ERF197. Tissue expression data of the 39 genes was downloaded from the SoyBase database (see text footnote 8). The six genes had relatively high expression in 14 tissues of soybean but particularly in roots, stems, and leaves (Figure 3 and Supplementary Figure 3).
Figure 3. Expression of drought-responsive differentially expressed genes and expression profiles of GmAP2/ERF genes in different soybean tissues. (A) Heat map with hierarchical cluster analysis of drought-responsive differentially expressed soybean GmAP2/ERF genes. Red indicates high relative transcript abundance, whereas green indicates low relative transcript abundance. (B) Heat map of expression profiles of soybean GmAP2/ERF genes in 14 soybean tissues. Hierarchical clustering of the 39 soybean GmAP2/ERF genes was constructed by average linkage with Euclidean distance in MeV 4.9 software. Different colors indicate relative transcript abundance of soybean GmAP2/ERF genes in 14 soybean tissues, with red indicating high abundance and blue indicating low abundance.
According to previous studies, the six stress-related genes selected from the ERF family analysis were subjected to drought treatment in soybean seedlings. The RNA was extracted from soybean leaves at 0, 2, 4, 6, and 8 d of drought treatment. Expression patterns of the candidate genes under drought stress were investigated by fluorescence quantitative PCR, with actin used as the internal reference gene. Genes viz., GmAP2/ERF197, GmAP2/ERF192, and GmAP2/ERF144 was up-regulated four to seven times after 8 d of drought treatment (Figure 4A). With an increase in exposure to drought, the expression of GmAP2/ERF144 also increased, ultimately increasing by more than four times (Figure 4A). Because expression of GmAP2/ERF144 was more responsive to drought than that of other genes, it was selected for stable transformation of soybean.
Figure 4. Reverse-transcription quantitative PCR analyses of expression of six representative soybean GmAP2/ERF144 genes during increasing time of drought and gene expression pattern in different soybean tissues. (A) Reverse-transcription qPCR analyses of the expression of six representative genes in the GmAP2/ERF family during drought stress treatment. Leaves of two-week-old soybean plants were collected for analysis when watering was stopped for 0, 2, 4, 6, and 8 d. (B) Tissue expression pattern of gene GmAP2/ERF144. Values are the mean ± SE. *p < 0.05; **p < 0.01 (Student’s t-test).
To analyze expression of GmAP2/ERF144 in different organs, fluorescence quantitative PCR technology was used. The RNA extracted from roots, stems, and leaves of soybean seedlings was used as a template, and actin was the internal reference gene. Expression of GmAP2/ERF144 mRNA was the lowest in roots, with expression approximately 1.5 times higher in stems and approximately 2.5 times higher in leaves (Figure 4B).
To determine subcellular localization of the GmAP2/ERF144 protein, first, the amino acid sequence was analyzed, and protein domain and nuclear localization signal (NLS) were predicted using online software9. The coding region of the GmAP2/ERF144 gene was 939 bp in length, and it encoded a protein containing 312 amino acids. Amino acid sequence analysis showed that the GmAP2/ERF144 protein contained an AP2 domain located at 150–213 amino acid residues (Supplementary Figure 4A) and an NLS (lcriykkha) at 248–258 amino acid residues (Supplementary Figure 4B). In the subcellular localization analysis, the GmAP2/ERF144 full-length CD without stop codon was cloned, and expression vectors 35S:GmAP2/ERF144:GFP (containing GmAP2/ERF144) and 35S:GFP (without GmAP2/ERF144) were constructed. The two carriers were transformed into tobacco leaves, and a laser scanning confocal microscope was used to detect fluorescence signals. The 35S:GFP green fluorescence was observed in cell membrane, nucleus, and endoplasmic reticulum, whereas the 35S:GmAP2/ERF144 fusion protein was only concentrated in the nucleus (Figure 5). Therefore, the GmAP2/ERF144 protein was a nuclear localized protein.
Figure 5. Subcellular localization of GmAP2/ERF144. (A) 35S::PIP2;1-mCherry fusion protein. (B) 35S::EGFP fusion protein. (C) Bright-field. (D) Merged image of panels (A–C). (E) 35S::PIP2;1-mCherry fusion protein. (F) 35S::GmAP2/ERF144-EGFP fusion protein. (G) Bright-field. (H) Merged image of panels (E–G). Bar = 0.02 mm.
To determine whether GmAP2/ERF144 could regulate the drought response of soybean, three groups of experiments were conducted. In the first single-plant drought rehydration test, survival of overexpressed lines was 100%, whereas growth of the wild type was weak and only 13.3% (4/30 plants) survived after rehydration (Figure 6A). With two soybeans in a small flowerpot, plants did not grow as well as a single plant in a flowerpot. After 16 d of drought, leaves of overexpressed lines turned yellow, whereas those of the wild type withered. Degree of wilting of overexpressed lines was significantly lower than that of the wild type. After 1 week of rehydration, all overexpression lines survived, whereas almost all wild-type plants died (29/30) (Figure 6B). After 28 d of natural drought, wild type plants did not survive (0/30), whereas survival of transgenic lines was 53% (16/30) (Figure 6C). Thus, expression of GmAP2/ERF144 improved drought tolerance of transgenic plants.
Figure 6. The GmAP2/ERF144 acts as a positive regulator in response to drought stress. (A) Performance of wild-type (WT), and two GmAP2/ERF144 overexpressing lines (OE1 and OE2) under drought stress. Two-week-old plants were incubated at 18 days drought stress, and rehydration after drought. (B) Performance of wild-type (WT), and two GmAP2/ERF144 overexpressing lines under drought stress. Two-week-old plants were incubated at 16 days drought stress, and rehydration after drought. (C) Performance of wild-type (WT), and two GmAP2/ERF144 overexpressing lines under drought stress. Two-week-old plants were incubated at 28 days drought stress, and rehydration after drought. (D) The leaf relative water contents in different lines under drought stress. (E) Leaf relative conductivity in different lines under drought stress. (F) MDA content in different lines under drought stress (n = 3). Data represent the mean ± SE. *, P < 0.05 (Student’s t-test). **, P < 0.01 (Student’s t-test).
Under normal watering, there was no morphological difference between leaves of the wild type and those of transgenic soybean, and there was no significant difference in leaf relative water content (Figure 6D). However, after 2 weeks of drought, wild-type plants suffered from the serious water shortage, and leaf relative water content decreased significantly compared with that of the two transgenic lines (Figure 6D).
To evaluate physiological changes in transgenic plants, malondialdehyde (MDA) content and relative conductivity of leaves in wild-type and transgenic soybeans were measured before and after drought treatment (Figures 6E,F). After 14 days of drought stress, MDA content in the wild type increased significantly by approximately five times that under normal conditions. In addition, MDA content of the wild type was significantly higher than that of transgenic lines by 2.5 times. There was no significant difference in leaf relative conductivity between the wild type and transgenic lines before treatment. After 2 weeks of drought, leaf relative conductivity of both the wild type and transgenic lines increased, but that of the wild type was significantly higher than that of overexpression lines. Therefore, drought resistance of soybean increased with overexpression of GmAP2/ERF144.
Overexpression lines of GmAP2/ERF144 indicated the GmAP2/ERF144 gene was associated with drought resistance in soybean. Therefore, to fully understand possible effects of the GmAP2/ERF144 gene, the String database was used to predict its regulatory network. Ten genes were predicted to interact with GmAP2/ERF144 (Figure 7A). Because there was no information on gene Glyma14g34070, it was not considered further. Total RNA from drought-treated WT and OE plants was isolated and reverse transcribed to cDNA, and gene-specific primers (Supplementary Table 2) were used to perform qPCR. Differential expression patterns of the nine genes in WT and OE plants are shown in Figure 7B.
Figure 7. Expression of interacting genes in the regulatory network of GmAP2/ERF144. (A) STRING-predicted regulatory network of genes interacting with GmAP2/ERF144 in soybean. (B) Expression profiles of nine genes (of 10) that interacted with GmAP2/ERF144 in wild-type (WT) and overexpressing (OE) lines of soybean. Protein–protein interaction network of GmAP2/ERF144 was detected in silico using the STRING database (https://string-db.org/). Values are the mean ± SE, n = 3. *p < 0.05; **p < 0.01 (Student’s t-test).
Whereas Glyma.10g013300 (GmbZIP) increases salt and freezing tolerance, most other ABA-induced bZIP genes increase drought tolerance. This divergence suggests functional specificity of each bZIP gene in improving plant stress tolerance. Alternatively, each group of bZIP genes may have specific functions. For example, group A bZIP genes are involved in ABA signaling and drought tolerance (Liao et al., 2008).
The gene Glyma.01g198100 encodes a transcription factor bhlh18. Basic helix–loop–helix (bHLH) TFs are encoded by one of the largest gene families in plants, and they participate in various physiological processes. The bHLHs function in transcriptional regulation networks by binding to E-box elements within the promoter region of their target genes (Huang et al., 2013).
Compared with the wild type, Glyma.16g151500 was up-regulated by 25 times in overexpression lines (Figure 7B), which was the greatest increase in expression among the predicted interaction genes. The gene encodes a protein containing a NAC domain and that has DNA binding characteristics. Overall, expression of the nine interacting genes was significantly up-regulated in the two lines overexpressing GmAP2/ERF144 by 3 to 30 times compared with that in the wild type. Thus, the nine genes likely interacted with GmAP2/ERF144, although specific experiments are needed to verify that conclusion.
Plant growth and development under different environmental stresses are regulated by genetic elements. Genetic improvement of drought resistance has become an important focus of research in soybean breeding, particularly as drought increases. Transcription factors contain one or more specific DNA-binding domains and are essential in regulating gene expression throughout the life cycles of higher plants (Hao et al., 1998). The AP2/ERF (APETALA2/ethylene-responsive element binding factors) TFs are a large group that is mainly found in plants (Yang S. U. et al., 2020). The TFs of this family are important regulators of many biological and physiological processes, including plant morphogenesis, responses to various stresses, hormone signal transduction, and biosynthesis of metabolites (Feng et al., 2020).
Transcription factors in the AP2/ERF family are mainly classified into four major subfamilies, including DREB (dehydration-responsive element-binding), ERF (ethylene-responsive element-binding), AP2 (APETALA2), and RAV (related to ABI3/VP), with some also classified in the Soloist subfamily (a few unclassified factors) (Riechmann and Meyerowitz, 1998; Magnani et al., 2004). Transcription factors of the AP2/ERF superfamily regulate diverse processes involved in plant development and are also important in hormonal regulation and stress responses. Based on genome-wide sequence analyses of those TFs, 147 and 163 have been reported in Arabidopsis thaliana and rice, respectively (Nakano et al., 2006; Sharoni et al., 2011). Based on the EST database, 112, 167, 53, and 117 TFs in the AP2/ERF family have also been reported in tomato (Sharma et al., 2010), maize, barley, and common wheat (Jing et al., 2011), respectively. In this study, the AP2/ERF HMM profile (PF00847) was used as a query to identify the genes in the AP2/ERF family in soybean. The HMM search of the G. max genome resulted in the identification of 301 GmAP2/ERF genes. Based on the phylogenetic tree and homology analysis, 44 proteins that contained two AP2 domains were closely related to the AP2 subfamily of proteins, and four proteins that contained one AP2 domain and one B3 domain were classified in the RAV subfamily of proteins. However, 98 proteins were identified in the DREB subfamily of proteins, and 153 proteins were identified in the ERF subfamily of proteins (Supplementary Figure 1). The genes GmAP2/ERF300 and GmAP2/ERF301 showed homology with AT4G13040, and therefore, the proteins of those genes were classified as members of the Soloist subfamily of proteins.
Gene duplication might be the primary reason for expansion of the soybean AP2/ERF family (Figure 2B). Duplicate genes are an important feature of genome structure, and evolutionary processes such as duplication events, especially segmental and tandem repeats, have expanded members of gene families in plants (Abdullah et al., 2021; Musavizadeh et al., 2021). In addition, mutations in gene structure and at the promoter site cause diversity in functions of members of a gene family (Faraji et al., 2020). Overall, the AP2/ERF family genes in soybean may have been relatively highly influenced by evolutionary pressure (Musavizadeh et al., 2021).
In AP2/ERF TFs that contain the AP2 DNA-binding domain (approximately 60 amino acids in length), it directly interacts with cis-acting elements such as dehydration responsive elements (DRE)/C-repeat elements (CRT) and GCC-box at the promoter of target genes (Allen et al., 1998; Riechmann and Meyerowitz, 1998; Magnani et al., 2004; Mizoi et al., 2012; Yang S. U. et al., 2020). In this study, previously collected transcriptome data were used to screened six of the 39 genes up-regulated under drought and salt stress for drought-induction treatment (Figure 4 and Supplementary Figure 2). The genes GmAP2/ERF197, GmAP2/ERF192, and GmAP2/ERF144 were up-regulated four- to seven-fold after 8 d of drought treatment (Figure 4). In this quantitative analysis, GmAP2/ERF144 expression increased as the time of drought increased. Hence, although results are preliminary, GmAP2/ERF144 likely responds to drought stress. Therefore, GmAP2/ERF144 was overexpressed in soybean using an Agrobacterium-mediated method. In three types of drought tests, drought resistance of overexpression lines increased compared with that of wild-type soybean (Figure 6).
Water deficient conditions caused by drought limit plant growth and crop yields. The dehydration-responsive element/C-repeat (DRE/CRT) is recognized by the DREB subfamily of the AP2/ERF family. Members of the DREB subfamily function in regulating plant resistance to abiotic stress, including drought (Yamaguchi-Shinozakiaib and Shinozaki, 1994; Kole et al., 2015). According to Park et al. (2021) genes DREB2A and DREB2B are induced by both dehydration and high-salt stress, although they do not respond to low temperature in Arabidopsis (Park et al., 2021). Haake et al. (2002) isolated the CBF4 gene, also named DREB1D, from Arabidopsis. The gene is important in drought adaptation and is induced by drought stress but not by low temperatures. Similarly, TaERF3 from tomato directly interacts with the GCC-box in the promoters of stress-related genes, including BG3, Chit1, RAB18, LEA3, TIP2, POX2, and GST6, to promote resistance to salt and drought stress in wheat (Min et al., 2014; Rong et al., 2014). The gene GmDREB2 encodes a DRE-binding transcription factor in soybeans and is induced under drought and salinity stresses. Overexpression of GmDREB2 in transgenic plants increases expression of downstream gene transcripts and content of free proline to increase tolerance to salinity and drought stresses (Chen et al., 2007). In this study, the GmAP2/ERF144 protein targeted the nucleus (Figure 5) and increased drought resistance of overexpressed plants following stable genetic transformation of soybean. The results in this study are consistent with those of Yamaguchi-Shinozakiaib and Shinozaki (1994).
Leaf water content is a major physiological indicator that reflects a plant’s ability to withstand adversity in stressful environments (Jin et al., 2017; Zhang et al., 2019). Heat and drought stress decrease plant water uptake and increase transpiration, which in turn significantly decrease efficiency of water utilization and moisture retention in leaves (Alhaithloul, 2019; Cohen et al., 2020). In the present study, physiological indices of the wild type and two overexpression lines were measured under natural culture conditions and drought treatment for 14 d. After 14 d of drought, relative water content of leaves of overexpression plants was significantly higher than that of leaves of the wild type (Figure 6D). Increased ability of OsERF115/AP2EREBP110-OE transgenic lines to utilize water efficiently may contribute to conserve plant water content and maintain a lower leaf temperature than that in WT plants under heat–drought combined stress (Park et al., 2021). Drought stress negatively affects many aspects of cellular physiology. The major responses to water deficit stress are ROS accumulation, membrane damage, and altered antioxidant enzymatic activity, which subsequently leads to the loss of membrane integrity. Electrical conductivity is negatively correlated with membrane integrity and reflects the extent of membrane injury. In the present study, under drought treatment, MDA content and EC were significantly lower than those in the wild type (Figures 6E,F). Therefore, the results suggested there was less production of ROS in response to water deficit in overexpression lines, which helped to maintain membrane integrity (Ying et al., 2015). The results of this study and others suggest that overexpression of GmAP2/ERF144 contributes to improved drought stress tolerance by increasing water retention capability.
To fully understand the function of GmAP2/ERF144, network analysis was used to predict those genes that interacted with GmAP2/ERF144 (Figure 7A). Interaction gene Glyma.01g198100 encodes transcription factor bhlh18. Basic helix–loop–helix (bHLH) TFs are members of one of the largest gene families in plants, and they participate in various physiological processes. The bHLHs can function in a transcriptional regulation network by binding to E-box elements within the prompter region of their target genes (Huang et al., 2013). Expression of Glyma.16g151500, which encodes a NAC transcription factor, was significantly up-regulated during drought. The TF is a nuclear localized TF that regulates many important processes via gene regulation.
Glyma.16g151500 was up-regulated 25 times in overexpression lines, compared with expression in the wild type, which was the greatest difference in expression among the predicted interaction genes (Figure 7B). The gene encodes a protein that contains a NAC domain and has DNA binding characteristics. The ERF proteins regulate biotic and abiotic stress responses by directly or indirectly regulating gene expression by binding with the GCC-box motif or by interacting with other TFs (Fujimoto et al., 2000; Zhu et al., 2003; Min et al., 2014). The tomato ERF protein TSRF1 binds to the cis-acting element GCC-box in the promoter of a pathogenesis-related gene and positively regulates pathogen resistance in tomato and tobacco (Fujimoto et al., 2000). The gene also increases expression of MYB, MYC, and proline synthesis-related genes, which contain the GCC-box in their promoter region, leading to improved osmotic and drought tolerance (Quan et al., 2010). Therefore, cis-elements and their associated genes may be important in regulating the response of soybean to drought stress (Liu et al., 1998).
In this study, 301 GmAP2/ERF genes encoding TFs were identified in the soybean genome, which were unevenly distributed on 20 chromosomes and two chromosome scaffolds. The TFs were divided into five categories according to their homology. Then, according to results of previous studies, the target gene GmAP2/ERF144 was selected from the genes up-regulated by drought and salt stress in the transcriptome. According to tissue expression analysis and subcellular determination, the gene was highly expressed in leaves, and its protein was localized in the nucleus. To validate gene function, GmAP2/ERF144 was overexpressed in soybean using an Agrobacterium-mediated method. Compared with wild-type soybean, drought resistance in overexpression lines increased significantly. Network analysis was used to predict genes that interacted with GmAP2/ERF144. To conclude, this work provides a foundation to increase understanding of mechanisms of drought resistance in soybean and ultimately cultivation of drought-resistant varieties.
The original contributions presented in the study are included in the article/Supplementary Material, further inquiries can be directed to the corresponding author/s.
HW, JZ, and HXi designed the experiments and wrote the manuscript. HW, DN, JS, SD, CW, and HXu executed the experiments and prepared the figures. HW, JX, LZ, and NG analyzed the data. All authors read and approved the final manuscript.
This work was supported by Genetically Modified Organisms Breeding Major Projects (2016ZX08004002), the China Agriculture Research System of MOF and MARA (CARS-04-PS12), the National Natural Science Foundation of China (32072082), the Jiangsu Agriculture Science and Technology Innovation Fund (CX(20)2015), the Program for Changjiang Scholars and Innovative Research Team in University (PCSIRT_17R55), the Jiangsu Collaborative Innovation Center for Modern Crop Production, and the Cyrus Tang Innovation Center for Seed Industry.
The authors declare that the research was conducted in the absence of any commercial or financial relationships that could be construed as a potential conflict of interest.
All claims expressed in this article are solely those of the authors and do not necessarily represent those of their affiliated organizations, or those of the publisher, the editors and the reviewers. Any product that may be evaluated in this article, or claim that may be made by its manufacturer, is not guaranteed or endorsed by the publisher.
The Supplementary Material for this article can be found online at: https://www.frontiersin.org/articles/10.3389/fpls.2022.848766/full#supplementary-material
Supplementary Figure 1 | Phylogenetic relations, gene structures, and motif patterns of GmAP2/ERF members. Phylogenetic relations of the (A) AP2 subfamily, (B) ERF subfamily, (C) DREB subfamily, and (D) RAV subfamily. Orange boxes, blue boxes, and black lines indicate UTRs, exons, and introns, respectively. Bottom scales indicate exon and intron lengths.
Supplementary Figure 2 | Cis-Element Analyses of Soybean GmAP2/ERF Genes. Therefore, the promoter region sequences (the 2-kp upstream sequences from gene initiation codons) of GmAP2/ERF genes were extracted for cis-element analyses. Cis-elements in the 2,000-bp upstream regions of soybean GmAP2/ERF genes were predicted using the PlantCare database, and colored boxes contain their names and positions (relative to the start codon).
Supplementary Figure 3 | Salt-responsive differentially expressed genes and expression profiles of soybean GmAP2/ERF genes. Heat map with hierarchical cluster analysis of salt-responsive differentially expressed genes (DEGs) among soybean GmAP2/ERF genes. Different colors indicate relative transcript abundance of salt-responsive soybean GmAP2/ERF genes, with red indicating high abundance and green indicating low abundance.
Supplementary Figure 4 | GmAP2/ERF144 protein structure analysis.
Supplementary Figure 5 | Identification of GmAP2/ERF144 transgenic lines. (A) Cloning of GmAP2/ERF144. (B) Quantitative real-time (RT)-PCR analysis of four independent transgenic soybean plants overexpressing GmAP2/ERF144. (C) Herbicide screening transgenic lines. (D) The transgenic positive vaccine was detected by PCR. (E) Detection of overexpression soybean herbicide bar marker. Data represent the mean ± SE. *, P < 0.05 (Student’s t-test). **, P < 0.01 (Student’s t-test).
Abdullah, Faraji, S., Mehmood, F., Malik, H. M. T., Ahmed, I., Heidari, P., et al. (2021). The GASA gene family in Cacao (Theobroma cacao, Malvaceae): genome wide identification and expression analysis. Agronomy 11:1425. doi: 10.3390/agronomy11071425
Alhaithloul, H. A. S. (2019). Impact of combined heat and drought stress on the potential growth responses of the desert grass artemisia sieberi alba: relation to biochemical and molecular adaptation. Plants 8:416. doi: 10.3390/plants8100416
Allen, M. D., Yamasaki, K., Ohme-Takagi, M., Tateno, M., and Suzuki, M. (1998). A novel mode of DNA recognition by a beta-sheet revealed by the solution structure of the GCC-box binding domain in complex with DNA. EMBO J. 17, 5484–5496. doi: 10.1093/emboj/17.18.5484
Bessire, M., Borel, S., Fabre, G., Carraca, L., Efremova, N., and Yephremov, A. (2011). A member of the pleiotropic drug resistance Family of ATP binding cassette transporters is required for the formation of a functional cuticle in Arabidopsis. Plant Cell 23, 1958–1970. doi: 10.1105/tpc.111.083121
Bhat, J. A., Deshmukh, R., Zhao, T., Patil, G., Deokar, A., Shinde, S., et al. (2020). Harnessing high-throughput phenotyping and genotyping for enhanced drought tolerance in crop plants. J. Biotechnol. 324, 248–260. doi: 10.1016/j.jbiotec.2020.11.010
Blanc, G., and Wolfe, K. H. (2004). Widespread paleopolyploidy in model plant species inferred from age distributions of duplicate Genes. Plant Cell 16, 1667–1678. doi: 10.1016/j.livsci.2009.01.009
Borges, A., Tsai, S. M., and Caldas, D. G. G. (2012). Validation of reference genes for RT-qPCR normalization in common bean during biotic and abiotic stresses. Plant Cell Rep. 31, 827–838. doi: 10.1007/s00299-011-1204-x
Chen, C., Chen, H., Zhang, Y., Thomas, H. R., Frank, M. H., He, Y., et al. (2020). TBtools: an integrative toolkit developed for interactive analyses of big biological data. Mol. Plant 13, 1194–1202. doi: 10.1016/j.molp.2020.06.009
Chen, M., Wang, Q., Cheng, X., Xu, Z., Li, L., and Ye, X. (2007). GmDREB2, a soybean DRE-binding transcription factor, conferred drought and high-salt tolerance in transgenic plants. Biochem. Biophys. Res. Commun. 353, 299–305. doi: 10.1016/j.bbrc.2006.12.027
Cohen, I., Zandalinas, S. I, Fritschi, F. B., Sengupta, S., and Mittler, R. (2020). The impact of water deficit and heat stress combination on the molecular response, physiology, and seed production of soybean. Physiol. Plant. 172, 41–52. doi: 10.1111/ppl.13269
Faraji, S., Filiz, E., Kazemitabar, S. K., Vannozzi, A., Palumbo, F., Barcacci, G., et al. (2020). The AP2/ERF Gene Family in Triticum durum: genome-wide identification and expression analysis under drought and salinity stresses. Genes 11:1464. doi: 10.3390/genes11121464
Feng, K., Hou, X., Xing, G., Liu, J., Duan, A., Xu, Z., et al. (2020). Advances in AP2/ERF super-family transcriptionfactors in plant. Crit. Rev. Biothechnol. 40, 750–776. doi: 10.1080/07388551.2020.1768509
Fujimoto, S. Y., Ohta, M., Usui, A., Shinshi, H., and Ohme-Takagi, M. (2000). Arabidopsis ethylene-responsive element binding factors act as transcriptional activators or repressors of GCC box-mediated gene expression. Plant Cell 12, 393–404. doi: 10.2307/3870944
Haake, V., Cook, D., Riechmann, J., Pineda, O., Thomashow, M. F., and Zhang, J. Z. (2002). Transcription Factor CBF4 Is a regulator of drought adaptation in Arabidopsis. Plant Physiol. 130, 639–648. doi: 10.1104/pp.006478
Hao, D., Ohme-Takagi, M., and Sarai, A. (1998). Unique mode of GCC box recognition by the DNA-binding domain of ethylene-responsive element-binding factor (ERF domain) in plant. J. Biol. Chem. 273, 26857–26861. doi: 10.1074/jbc.273.41.26857
He, Z., Zhang, H., Gao, S., Lercher, M. J., Chen, W., and Hu, S. (2016). Evolview v2: an online visualization and management tool for customized and annotated phylogenetic trees. Nucleic Acids Res. 44, 236–241. doi: 10.1093/nar/gkw370
Holle, S. V., and Damme, E. V. (2015). Distribution and evolution of the lectin family in soybean (Glycine max). Molecules 20, 2868–2891. doi: 10.3390/molecules20022868
Hu, Y., Xu, Z., Tian, Y., Gao, R., Ji, A., Pu, Y., et al. (2020). Genome-wide identification and analysis of AP2/ERF transcription factors related to camptothecin biosynthesis in Camptotheca acuminata. Chin. J. Nat. Med. 18, 582–593. doi: 10.1016/S1875-5364(20)30070-4
Huang, J., Li, X., Chen, X., Guo, Y., and Wang, H. (2021). Genome-wide identification of soybean ABC transporters relate to aluminum toxicity. Int. J. Mol. Sci. 22:6556. doi: 10.3390/ijms22126556
Huang, X., Wang, W., Zhang, Q., and Liu, J. (2013). A basic helix-loop-helix transcription factor, PtrbHLH of Poncirus trifoliata confers cold tolerance and modulates peroxidase-mediated scavenging of hydrogen peroxide. Plant Physiol. 162, 1178–1194. doi: 10.1104/pp.112.210740
Huang, Y., Xuan, H., Yang, C., Guo, N., Wang, H., and Zhao, J. (2019). GmHsp90A2 is involved in soybean heat stress as a positive regulator. Plant Sci. 285, 26–33. doi: 10.1016/j.plantsci.2019.04.016
Jin, J., Zhang, H., Kong, L., Gao, G., and Luo, J. (2014). Plant TFDB 3.0: a portal for the functional and evolutionary study of plant transcription factors. Nucleic Acids Res. 42, 1182–1187. doi: 10.1093/nar/gkt1016
Jin, X., Shi, C., Yu, C., Toshihiko, Y., and Sacks, E. J. (2017). Determination of leaf water content by visible and near-infrared spectrometry and multivariate calibration in Miscanthus. Front. Plant Sci. 8:721. doi: 10.3389/fpls.2017.00721
Jing, Z., Chen, J., Yao, Q., Xiong, F., Sun, C., and Zhou, X. (2011). Discovery and expression profile analysis of AP2/ERF family genes from Triticum aestivum. Mol. Biol. Rep. 38, 745–753. doi: 10.1007/s11033-010-0162-7
Jofuku, K. D., Boer, B. G. W. D., and Okamuro, M. J. K. (1994). Control of Arabidopsis flower and seed development by the homeotic gene APETALA2. Plant Cell 6, 1211–1225. doi: 10.2307/3869820
Kagaya, Y., Ohmiya, K., and Hattori, T. (1999). RAV1, a novel DNA-binding protein, binds to bipartite recognition sequence through two distinct DNA-binding domains uniquely found in higher plants. Nucleic Acids Res. 27, 470–478. doi: 10.1093/nar/27.2.470
Kole, C., Muthamilarasan, M., Henry, R., Edwards, D., Sharma, R., Abberton, M., et al. (2015). Application of genomics-assisted breeding for generation of climate resilient crops: progress and prospects. Front. Plant Sci. 6:563. doi: 10.3389/fpls.2015.00563
Li, B., Cheng, J., Wang, T., Min, D., and Ma, Y. (2020). Expression analyses of soybean VOZ transcription factors and the role of GmVOZ1G in drought and salt stress tolerance. Int. J. Mol. Sci. 21:2177. doi: 10.3390/ijms21062177
Li, D., He, Y., Li, S., Shi, S., Li, L., Liu, Y., et al. (2021). Genome-wide characterization and expression analysis of AP2/ERF genes in eggplant (Solanum melongena L.). Plant Physiol. Biochem. 167, 492–503. doi: 10.1016/j.plaphy.2021.08.006
Liao, Y., Zou, H., Wei, W., Hao, Y., Tian, A., Huang, J., et al. (2008). Soybean GmbZIP44, GmbZIP62 and GmbZIP78 genes function as negative regulator of ABA signaling and confer salt and freezing tolerance in transgenic Arabidopsis. Planta 228, 225–240. doi: 10.1007/s00425-008-0731-3
Liu, Q., Kasuga, M., Sakuma, Y., Abe, H., Miura, S., Yamaguchi-Shinozaki, K., et al. (1998). Two transcription factors, DREB1 and DREB2, with an EREBP/AP2 DNA binding domain separate two cellular signal transduction pathways in drought- and low-temperature-responsive gene expression, respectively, in Arabidopsis. Plant Cell 10, 1391–1406. doi: 10.1105/tpc.10.8.1391
Magnani, E., Sjo⋅lander, K., and Hake, S. (2004). From endonucleases to transcription factors: evolution of the AP2 DNA binding domain in Plants. Plant Cell 16, 2265–2277. doi: 10.1105/tpc.104.023135
Min, J. K., Ruzicka, D., Shin, R., and Schachtman, D. P. (2014). The Arabidopsis AP2/ERF Transcription Factor RAP2.11 modulates plant response to low-potassium conditions. Mol. Plant 5, 1042–1057. doi: 10.1093/mp/sss003
Mizoi, J., Shinozaki, K., and Yamaguchi-Shinozaki, K. (2012). AP2/ERF family transcription factors in plant abiotic stress responses. Biochim. Biophys. Acta 1819, 86–96. doi: 10.1016/j.bbagrm.2011.08.004
Musavizadeh, Z., Najafi-Zarrini, H., Kazemitabar, S. K., Hashemi, S. H., Faraji, S., Barcaccia, G., et al. (2021). Genome-wide analysis of potassium channel genes in rice: expression of the OsAKT and OsKAT genes under salt stress. Genes 12, 784. doi: 10.3390/genes12050784
Nakano, T., Suzuki, K., Fujimura, T., and Shinshi, H. (2006). Genome-wide analysis of the ERF Gene family in Arabidopsis and rice. Plant Physiol. 140, 411–432. doi: 10.1104/pp.105.073783
Noman, M., Jameel, A., Qiang, W., Ahmad, N., and Li, H. (2019). Overexpression of GmCAMTA12 enhanced drought tolerance in Arabidopsis and Soybean. Int. J. Mol. Sci. 20:4849. doi: 10.3390/ijms20194849
Ohme-Takagi, M., and Shinshi, H. (1995). Ethylene-inducible DNA binding proteins that interact with an ethylene-responsive element. Plant Cell 7, 173–182. doi: 10.2307/3869993
Park, S. I., Kwon, H. J., Cho, M. H., Song, J. S., Kim, B. G., Baek, J., et al. (2021). The OsERF115/AP2EREBP110 transcription factor is involved in the multiple stress tolerance to heat and drought in rice plants. Int. J. Mol. Sci. 22:7181. doi: 10.3390/ijms22137181
Quan, R., Hu, S., Zhang, Z., Zhang, H., Zhang, Z., and Huang, R. (2010). Overexpression of an ERF transcription factor TSRF1 improves rice drought tolerance. Plant Biotechnol. J. 8, 476–488. doi: 10.1111/j.1467-7652.2009.00492.x
Riechmann, J. L., and Meyerowitz, E. M. (1998). The AP2/EREBP family of plant transcription factors. Biol. Chem. 379, 633–646. doi: 10.1515/bchm.1998.379.6.633
Rong, W., Qi, L., Wang, A., Ye, X., Du, L., Liang, H., et al. (2014). The ERF transcription factor TaERF3 promotes tolerance to salt and drought stresses in wheat. Plant Biotechnol. J. 12, 468–479. doi: 10.1111/pbi.12153
Sharma, M., Kumar, R., Solanke, A., Sharma, R., Tyagi, A., and Sharma, A. (2010). Identification, phylogeny, and transcript profiling of ERF family genes during development and abiotic stress treatments in tomato. Mol. Genet. Genomics 284, 455–475. doi: 10.1007/s00438-010-0580-1
Sharoni, A., Nuruzzaman, M., Satoh, K., Shimizu, T., Kondoh, H., and Sasaya, T. (2011). Gene structures, classification and expression models of the AP2/EREBP transcription factor family in Rice. Plant Cell Physiol. 52, 344–360. doi: 10.1093/pcp/pcq196
Tian, R., Yang, Y., and Chen, M. (2020). Genome-wide survey of the amino acid transporter gene family in wheat (Triticum aestivum L.): identification, expression analysis and response to abiotic stress. Int. J. Biol. Macromol. 162, 1372–1387. doi: 10.1016/j.ijbiomac.2020.07.302
Wang, K., Bu, T., Cheng, Q., Dong, L., Su, T., Chen, Z., et al. (2021). Two homologous LHY pairs negatively control soybean drought tolerance by repressing the abscisic acid responses. New Phytol. 229, 2660–2675. doi: 10.1111/nph.17019
Wang, Y., Liu, H., Wang, S., and Li, H. (2017). Genome-wide identification and expression analysis of the YUCCA gene family in soybean (Glycine max L.). Plant Growth Regul. 81, 265–275. doi: 10.1007/s10725-016-0203-x
Xie, Z., Nolan, T., Jiang, H., Tang, B., and Yin, Y. (2019). The AP2/ERF transcription Factor TINY modulates brassinosteroid-regulated plant growth and drought responses in Arabidopsis. Plant Cell 31, 1788–1806. doi: 10.1105/tpc.18.00918
Yamaguchi-Shinozakiaib, Y., and Shinozaki, K. (1994). A nove1 cis-acting element in an Arabidopsis gene 1s involved in responsiveness to drought, low qemperature, or high-salt stress. Plant Cell 6, 251–264. doi: 10.1105/tpc.6.2.251
Yamasaki, K., Kigawa, T., Inoue, M., Watanabe, S., Tateno, M., Seki, M., et al. (2008). Structures and evolutionary origins of plant-specific transcription factor DNA-binding domains. Plant Physiol. Biochem. 46, 394–401. doi: 10.1016/j.plaphy.2007.12.015
Yang, C., Huang, Y., Lv, W., Zhang, Y., and Zhao, T. (2020). GmNAC8 acts as a positive regulator in soybean drought stress. Plant Sci. 293:110442. doi: 10.1016/j.plantsci.2020.110442
Yang, S. U., Kim, H., Kim, R. J., Kim, J., and Suh, M. C. (2020). AP2/DREB Transcription Factor RAP2.4 activates cuticular wax biosynthesis in Arabidopsis leaves under drought. Front. Plant Sci. 11:895. doi: 10.3389/fpls.2020.00895
Ying, Y., Song, L., Jacobs, D. F., Mei, L., Liu, P., Jin, S., et al. (2015). Physiological response to drought stress in Camptotheca acuminata seedlings from two provenances. Front. Plant Sci. 6:361. doi: 10.3389/fpls.2015.00361
Zhang, G., Chen, M., Chen, X., Xu, Z., Guan, S., Li, L., et al. (2008). Phylogeny, gene structures, and expression patterns of the ERF gene family in soybean (Glycine max L.). J. Exp. Bot. 59, 4095–4107. doi: 10.1093/jxb/ern248
Zhang, G., Ming, C., Li, L., Xu, Z., Chen, X., Guo, J., et al. (2009). Overexpression of the soybean GmERF3 gene, an AP2/ERF type transcription factor for increased tolerances to salt, drought, and diseases in transgenic tobacco. J. Exp. Bot. 60, 3781–3796. doi: 10.1093/jxb/erp214
Zhang, R., Zhou, Y., Yue, Z., Chen, X., and Cao, X. (2019). The leaf-air temperature difference reflects the variation in water status and photosynthesis of sorghum under waterlogged conditions. PLoS One 14:e0219209. doi: 10.1371/journal.pone.0219209
Zhou, L., and Yarra, R. (2021). Genome-wide identification and characterization of AP2/ERF transcription factor family genes in oil palm under abiotic stress conditions. Int. J. Mol. Sci. 22, 2821. doi: 10.3390/ijms22062821
Keywords: transcription factors (TFs), drought, soybean, overexpression, expression analysis, AP2/ERF gene family
Citation: Wang H, Ni D, Shen J, Deng S, Xuan H, Wang C, Xu J, Zhou L, Guo N, Zhao J and Xing H (2022) Genome-Wide Identification of the AP2/ERF Gene Family and Functional Analysis of GmAP2/ERF144 for Drought Tolerance in Soybean. Front. Plant Sci. 13:848766. doi: 10.3389/fpls.2022.848766
Received: 10 January 2022; Accepted: 08 February 2022;
Published: 28 March 2022.
Edited by:
Esther M. Gonzalez, Public University of Navarre, SpainReviewed by:
Parviz Heidari, Shahrood University of Technology, IranCopyright © 2022 Wang, Ni, Shen, Deng, Xuan, Wang, Xu, Zhou, Guo, Zhao and Xing. This is an open-access article distributed under the terms of the Creative Commons Attribution License (CC BY). The use, distribution or reproduction in other forums is permitted, provided the original author(s) and the copyright owner(s) are credited and that the original publication in this journal is cited, in accordance with accepted academic practice. No use, distribution or reproduction is permitted which does not comply with these terms.
*Correspondence: Jinming Zhao, am16MzAwMEAxMjYuY29t; Han Xing, aGFueEBuamF1LmVkdS5jbg==
Disclaimer: All claims expressed in this article are solely those of the authors and do not necessarily represent those of their affiliated organizations, or those of the publisher, the editors and the reviewers. Any product that may be evaluated in this article or claim that may be made by its manufacturer is not guaranteed or endorsed by the publisher.
Research integrity at Frontiers
Learn more about the work of our research integrity team to safeguard the quality of each article we publish.