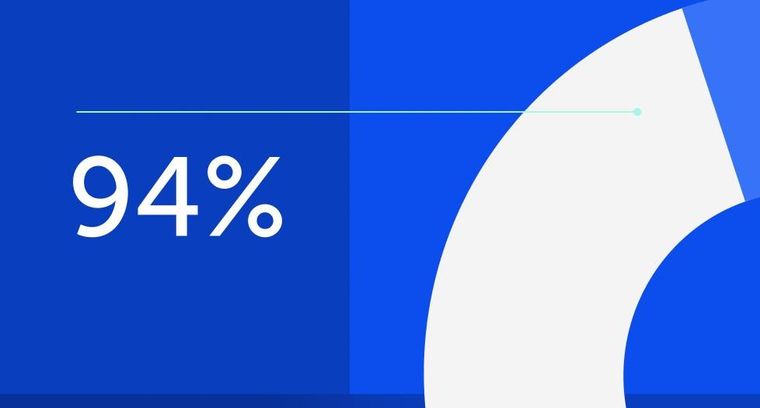
94% of researchers rate our articles as excellent or good
Learn more about the work of our research integrity team to safeguard the quality of each article we publish.
Find out more
ORIGINAL RESEARCH article
Front. Plant Sci., 31 May 2022
Sec. Plant Bioinformatics
Volume 13 - 2022 | https://doi.org/10.3389/fpls.2022.846697
MADS-box is an important transcription factor family that is involved in the regulation of various stages of plant growth and development, especially flowering regulation and flower development. Being a holoparasitic plant, the body structure of Balanophoraceae has changed dramatically over time, and its vegetative and reproductive organs have been extensively modified, with rudimentary flower organs. Meanwhile, extraordinary gene losses have been identified in holoparasitic plants compared with autotrophs. Our study reveals that the MADS-box gene family contracted sharply in Balanophora subcupularis and Balanophora fungosa var. globosa, and some subfamilies were lost, exhibiting reduced redundancy in both. The genes that functioned in the transition from the vegetative to floral production stages suffered a significant loss, but the ABCE model genes remained intact. We further investigated genes related to flowering regulation in B. subcupularis and B. fungosa var. globosa, vernalization and autonomous ways of regulating flowering time remained comparatively integrated, while genes in photoperiod and circadian clock pathways were almost lost. Convergent gene loss in flowering regulation occurred in Balanophora and another holoparasitic plant Sapria himalayana (Rafflesiaceae). The genome-wide analysis of the MADS-box gene family in Balanophora species provides valuable information for understanding the classification, gene loss pattern, and flowering regulation mechanism of MADS-box gene family in parasitic plants.
The MADS-box gene family is involved in all stages of plant development and is one of the most thoroughly investigated gene families in plants (Theißen et al., 2016; Schilling et al., 2018). Previous research elucidated that MADS-box family plays a vital role in many developmental processes, especially in the flower organ identity, control of flowering time, vegetative development, seed and fruit development, pollen, and embryo sac formation (Smaczniak et al., 2012; Theißen et al., 2016).
MADS-box genes contain a highly conserved MADS (M) domain at the N-terminus which has a length of 50–60 amino acids, binding to CArG boxes (CC-“Adenine rich”-GG) (Riechmann et al., 1996; Gramzow et al., 2010). Phylogenetically, MADS-box gene family is divided into two categories: type I (SRF-like) and type II (MEF2-like), based on their conserved domain (Alvarez-Buylla et al., 2000; Parenicová et al., 2003). In plants, the length of type I genes are generally shorter and have simple structures, having only the MADS domain, which can be further classified into three clades, Mα, Mβ, and Mγ. Compared to type I genes, the type II genes tend to be more complicated consisting of MADS (M) domain, Intervening (I) domain, Keratin (K) domain, and highly variable C-terminal (C) domain, also named as MIKC-type genes (Theißen et al., 1996; Parenicová et al., 2003; Kaufmann et al., 2005; Smaczniak et al., 2012). According to the different intervening regions, the MIKC-type can be divided into two subgroups, MIKCC and MIKC* (Henschel et al., 2002; Liu et al., 2018). Some reports about Arabidopsis thaliana showed that MIKCC type genes can be further subclassified into 12 groups based on their phylogenetic relationships (Parenicová et al., 2003). During the long-term evolution of this family of genes, varying degrees of duplication events have occurred followed by subfunctionalization, which resulted in the functional diversification of MADS-box genes (Theissen and Saedler, 2001).
Flowering is a critical process that requires the cooperation and interaction of many genes in order to detect developmental and environmental cues and make decisions. Numerous studies have manifested that MADS-box genes play crucial roles in the flowering process, not only participate in regulating floral transition, floral meristem specialization, floral organ formation, and pollen growth, but are also related to root, ovule, and seed development (Michaels and Amasino, 1999; Liljegren et al., 2000; Adamczyk and Fernandez, 2009; Moreno-Risueno et al., 2010; Garay-Arroyo et al., 2013; Xu et al., 2016). Extensive research on mutants with floral organ identity defects has led to the birth of the “ABCE model,” which explains how the class A, B, C, D, and E genes co-determine floral organ identities (Riechmann and Meyerowitz, 1997; Theissen and Saedler, 2001). In Arabidopsis, class A [APETALA1 (AP1)] and class E [SEPALLATA1/2/3/4 (SEP1/2/3/4)] protein complex are involved in sepal development. Class A, class B, and class E protein complex participate in petal development. Class B [APETALA3 (AP3) and PISTILLATA (PI)], class C [AGAMOUS (AG)] and class E protein complex are involved in stamen development, while class C and class E protein complex take part in carpel development. Ovule development is modulated by class D genes [SEEDSTICK (STK), SHATTERPROOF1 (SHP1), and SHP2] (Weigel and Meyerowitz, 1994; Ehlers et al., 2016). Class E proteins are involved in the formation of tetramer protein complexes and form complexes with type A, B, C proteins (Melzer and Theissen, 2009; Pan et al., 2014). In this model, most genes belong to the type-II MADS-box family in Arabidopsis (Kaufmann et al., 2005). In addition, there are some other type II genes of MADS-box that are also involved in the regulation of flowering time and flower initiation, including FLOWERING LOCUS C (FLC), SHORT VEGETATIVE PHASE (SVP), SUPPRESSOR OF CONSTANS1 (SOC1), AGAMOUS-LIKE 15 (AGL15), AGL18, AGL24, MADS AFFECTING FLOWERING (MAF1/FLM), etc. (Michaels and Amasino, 1999; Hartmann et al., 2000; Samach et al., 2000), which play key roles in regulating flowering time through photoperiod, vernalization, or functioning as an integrator of flowering signals.
Parasitic plants differ from free-living plants, as they have evolved a heterotrophic lifestyle, relying on haustoria that connect to the host’s vascular system to get resources for growth and development. Parasitic plants make up about 1% of angiosperms in the world, including about 4,500 species, representing at least 12 independent evolutionary events from autotrophs into parasitic plants (Westwood et al., 2010). Parasitic plants could be classified into hemiparasites and holoparasites, with the distinction being that the former can carry out partial or complete photosynthesis while the latter lacks the photosynthetic capacity. Some of these parasitic plants of Orobanchaceae, such as Phtheirospermum, Striga, are considered as agricultural weeds, which seriously jeopardize crops (Clarke et al., 2019; Kountche et al., 2019).
Santalales is one of the largest family of parasitic plants, comprising autotrophic, hemiparasitic and holoparasitic plants (Chen et al., 2020), among which the holoparasitic plants of Balanophoraceae exhibit special morphological structure and extreme manifestation of parasitism. The aboveground part of the Balanophoraceae plants resembles the appearance of basidiomycetes fungi, consisting of a thickened and fleshy inflorescence, with or without scaly leaves (bracts), monoecious or dioecious (Shivamurthy et al., 1981a; Eberwein et al., 2009). Due to the holoparasitic habit, the vegetative and reproductive systems of Balanophoraceae plants are severely reduced, with the tuber serving as the only remaining vegetative organ from which the floral organs eventually emerge (Shivamurthy et al., 1981b). Unusually, 5–10 hypodermal cell-layers of the tuber re-differentiated into inflorescence meristem, which lacks a morphologically distinguishable epidermis and characteristically aligned hypodermal cell layers, indicating the special origin of flowers in Balanophora (Shivamurthy et al., 1981b). The female flowers of Balanophoraceae plants are very simple, filamentous structures, without tepals, only a style and a brief ovary, and a spadicale that overlays the surface of inflorescence (Figure 1A). Male flowers are also simple and consist of perianth and pollen (Figure 1A; Eberwein et al., 2009). Moreover, the tuber contains two vascular systems, one derived from the parasitic plant and the other is a complex tissue containing the parasitic and host plant tissue (Hsiao et al., 1994, 1995).
Figure 1. Morphological traits of Balanophora plants and MADS-box gene number. (A) Morphological structure of Balanophora plants (B. fungosa var. globosa). (a,b) Extremely simplified male plant and the female plant. (c) Female flower structure was observed under an electron microscope. FF, female flower; MF, male flower; S, stem; BR, bract; T, tuber; SP, spadicale; P, pollen. Image courtesy of Runxian Yu (CC BY-SA 2.0). (B) The number of MADS-box genes in B. subcupularis, B. fungosa var. globosa, and reference species. Fourteen species were selected according to the degree of parasitism and genetic relationship. Gray, orange, and blue indicate autotrophic plants, hemiparasite, and holoparasite, respectively. The bar chart shows the number of MADS-box genes in each species. The iTAK (http://itak.feilab.net/cgi-bin/itak/online_itak.cgi) and plantTFdb (http://planttfdb.gao-lab.org/prediction.php) programs were used to identify the MADS-box genes of these species.
Previous studies indicated that there was a different degree of gene loss in hemiparasitic plant Striga asiatica, hemi-holoparasitic plant Cuscuta australis and holoparasitic plant Sapria himalayana according to the degree of host dependence, among which about 44% genes were lost in the genome of Sapria (Cai et al., 2021), we also found that gene redundancy was greatly reduced in two Balanophora species genomes, especially transcriptional factors, and extremely convergent gene loss was identified in two lineages of holoparasites, Balanophora and Sapria himalayana (Rafflesiaceae).
To explore the initiation, development and regulation of flowering in Balanophora, we identified the MADS-box genes in B. subcupularis (monoecious) and B. fungosa var. globosa (diocious). We analyzed the gene structures, conserved motifs, phylogeny, and tissue-specific expression of those genes. In addition, we also studied the gene loss pattern and further discussed the potential loss of the MADS-box genes related to the flowering regulation pathways in B. subcupularis and B. fungosa var. globosa and other holoparasitic plants. The results of our study can improve our understanding of the evolution and functions of MADS-box genes in B. subcupularis and B. fungosa var. globosa and would lay a foundation for further studies of the flowering development and regulation mechanisms in parasitic plants.
In this study, we selected fourteen species based on the degree of parasitism, comprising seven orders (Supplementary Table 1), including holoparasitic B. subcupularis, B. fungosa var. globosa and B. fungosa (Santalales) (Leebens-Mack et al., 2019), S. himalayana (Malpighiales) (Cai et al., 2021), C. australis (Solanales) (Sun et al., 2018), hemiparasitic S. asiatica (Lamiales) (Yoshida et al., 2019), S. parasitica var. graciliflora (Santalales), S. album (Santalales) (Dasgupta et al., 2019), M. oleifera (Santalales) (Xu et al., 2019) and their close relatives. The genomes of Balanophora plants were obtained from China National GeneBank DataBase (CNGBdb) (CNP0003054). We employed iTAK1 and TFplantdb2 to search the MADS-box genes from genomes analyzed in this study. Then, all obtained MADS-box gene sequences of B. subcupularis and B. fungosa var. globosa were further analyzed in SMART,3 Pfam,4 CDD5 databases to verify the existence of MADS domain.
A total of 829 MADS-box protein sequences from a total of 14 species and one MADS-box gene of Aquilegia coerulea as an outgroup were aligned using MAFFT software,6 and the phylogenetic tree was constructed by IQ-TREE7 program based on the maximum likelihood (ML) method. The parameters were set to −m GTR + R, −bb 1000, −alrt 1000, and the best alternative model is determined by model testing software. The tree was visualized by Evolview8 software.
According to the annotation information of B. subcupularis and B. fungosa var. globosa, the intron and exon composition of MADS-box gene were mapped by GSDS 2.0 (Gene Structure Display Server).9 The software MEME10 was used to search for the conservative motifs in the MADS-box genes of Balanophora. The parameter was as follows: the maximum number of motifs was 20, and the motifs width was set to 6–200 amino acids. The motifs were annotated by the Pfam database (see text footnote 4). The number of introns of species analyzed in this study was calculated according to the annotated information. The box plots of the intron length of B. subcupularis, B. fungosa var. globosa, and reference species were plotted using R packages.
We identified MADS-box gene loss primarily according to the phylogenetic tree at the ortholog level with Arabidopsis thaliana and Vitis vinifera. We divided the phylogenetic tree into ortholog groups and allowed the existence of paralogs of Arabidopsis in the same group, each ortholog group was assigned according to Supplementary Figure 2, and the primary identification of gene loss was identified based on it. For those absent genes in Balanohpora, orthologs from Arabidopsis and V. vinifera were used to predict against the assembly again (e-value threshold of 1e-5, coverage threshold of 0.25), the new annotations were checked manually including conserved domains, premature stop codons, and the transcriptome evidences. Then the manually confirmed gene models were used to construct a new phylogenetic tree, and gene loss was identified at the ortholog level again, and the absent genes in each orthologs group were considered as gene loss.
For those important flowering regulation pathways, we further identified the gene loss through orthologs modified from Sun et al. (2018). First, BLASTP was used for all-to-all proteins alignment with e-value of 1e-5, after that, we employed OrthoFinder (v.2.3.7) to cluster genes with a Markov inflation index of 1.5 and a maximum e-value of 1e-5; next, the absent genes in parasitic plants will be searched against their genomes with homologs from Arabidopsis and V. vinifera (e-value threshold of 1e-5, coverage threshold of 0.25), and new annotated gene models will be included for the next step; then, phylogenetic trees were constructed with IQ-TREE in each orthogroup generated in the last step; each tree was divided into subgroups, and each subgroup must be included at least one Arabidopsis gene; finally, the gene numbers for every species in each subgroup were counted as the ortholog gene number.
The expression profiles of flowering regulate genes in different tissues during the development of Balanophora were explored using transcriptome data downloaded from CNGBdb (CNP0003054). The expression data included three stages, stage 1: samples containing different developmental stages, LC21-YT1-3 (tuber sizes < 8 mm, duplicate: LC21-YT1, LC21-YT2, and LC21-YT3), LC21-YT4-6 (tuber sizes: 8∼15 mm, duplicate: LC21-YT4, LC21-YT5, and LC21-YT6), stage 3 samples without visible inflorescence tissues, tuber and host root were collected and named as LC24-YT1-3 (duplicate: LC24-YT1, LC24-YT2, and LC24-YT3), stage 3 samples with grown inflorescence were separated into different tissues, including tuber LC24-T3-32 (duplicate: LC24-T3 and LC24-T32), male inflorescence LC22-MF1-3 (duplicate: LC22-MF1, LC22-MF2, LC22-MF3), female inflorescence LC24-FF1-3 (LC24-FF1, LC24-FF2, LC24-FF3), inflorescence stem LC24-S1-2 (duplicate: LC24-S1, LC24-S2), and bracts (LC23-BR1). The heatmap was generated by taking the average of these duplicates.
Because the tuber of Balanophora also contains tissues from the host, we carefully classified the reads into two distinct species to avoid possible contaminations. First, the gene set of the Balanophora and its host were decontaminated (removal of genes potentially from fungi, bacteria), and then combined. Next, bowtie2 was used to map the high-quality reads from each tissue with the combined gene models of Balanophora and its host. Those reads which could be mapped into both Balanophora and its host were removed. Finally, the mapped reads were divided into two species.
Using the Cufflinks pipeline11 the FPKM (Fragments Per Kilobase of transcript per Million mapped reads) value of genes in each tissue was calculated, and low-expressed and non-expressed genes were filtered out (FPKM < 10). There were three replicates for each tissue, and we took the average FPKM of the three replicates for the expression analysis. The expression levels were visualized using heatmap tools with FPKM data in each tissue in Hiplot.12 DESeq2 was used to calculate modest estimates of folding changes and dispersion of RNA-seq data, and log2 (fold change) >1, FDR < 0.05 was considered to be a differentially expressed gene.
To study the evolution of MADS-box gene family in parasitic plants, we identified the MADS-box genes from parasitic plants and their autotrophic relatives (Figure 1B). Totally, 25 and 23 candidate genes were identified as MADS-box genes of B. subcupularis and B. fungosa var. globosa, respectively (Figure 1B). Then we named them BsubMADS1-25 and BgloMADS1-23 (Supplementary Table 2). Compared with Arabidopsis (108) and Vitis (81), we found that the gene number of this family in free-living plants was about twice that in hemiparasitic plants and three-five times that in holoparasitic plants, indicating that the number of MADS-box genes showed a stepwise loss trend with the increasing levels of parasitism. We found that the MADS-box gene family contracted sharply in holoparasitic B. subcupularis, B. fungosa var. globosa, S. himalayana, and their number of MADS-box genes is even fewer than Amborella trichopoda (36) (Albert et al., 2013). So, we inferred that the MADS-box genes of holoparasitic plants are drastically lost during the evolutionary process.
To investigate the evolutionary relationship of MADS-box genes between parasitic plants, we constructed a phylogenetic tree that included autotrophic and parasitic plants (Figure 2). They were categorized into two types: type I (Mα, Mβ, and Mγ) and type II (MIKCC and MIKC*) (Supplementary Table 3), which were further subdivided into sixteen subgroups following the previous classification (Smaczniak et al., 2012; Fatima et al., 2020). Based on the phylogenetic trees, ten and eight genes were classified as type I in B. subcupularis and B. fungosa var. globosa, respectively, and 15 genes were classified as type II in B. subcupularis and B. fungosa var. globosa, respectively. There are at least 21 MADS-box clades in the Most Recent Common Ancestor (MRCA) of extant angiosperms (Albert et al., 2013). While in our study we found that six clades were totally absent in Balanophora and seven clades were absent in Sapria, during which four clades were convergently lost (Supplementary Table 4).
Figure 2. Phylogenetic tree of MADS-box genes in B. subcupularis, B. fungosa var. globosa, and reference species. The phylogenetic tree was constructed using the maximum likelihood (ML) method of IQ-TREE program (see text footnote 7). And visualized and decorated the tree by Evolview (see text footnote 8). Taking Arabidopsis as a reference, the tree was divided into 16 subfamilies (Smaczniak et al., 2012; Fatima et al., 2020). Different species are represented in different colors. Orange, green, blue, and red dotted lines represent Arabidopsis, B. subcupularis, S. himalayana, B. fungosa var. globosa, respectively. The numbers show the number of MADS-box genes of parasitic plants and reference species in each subfamily. The Bootstraps are ≥ 50. See Supplementary Figure 2 for more details. The alignment information of these genes are available in Supplementary Table 9.
We noticed several losses of whole subfamilies in B. subcupularis and B. fungosa var. globosa, such as the AGL17, AGL15, AGL12, AGL6, and GNETUM GNEMON MADS13 (GGM13) clades, among which AGL6/12/15/17 function in the flowering transition, and TRANSPARENT TESTA16 (TT16) gene of GGM13 subfamily involved in seed pigmentation and embryo development (Erdmann et al., 2010). Most genes of AGL12 and AGL17 subfamilies are mainly involved in root development in addition to flowering (Tapia-López et al., 2008; Puig et al., 2013), which may be consistent with the lack of typical roots in Balanophora. It is worth noting that several genes classified in type II only contained the MADS domain confirmed by domain identification against Pfam database (Supplementary Figures 1, 2). Phylogenetic analysis showed that these non-K domain genes were more closely related to type II, suggesting that they may have lost their K domain during evolution. Further evidence in the following part such as intron number also supported the classification of these genes in type II.
To understand the structural diversity of MADS-box genes, we analyzed the intron and exon structure of these genes. We found that both Balanophora showed bimodal distribution between type I and II genes like other species (Wang et al., 2017, 2019; Fatima et al., 2020), significant differences in the number of introns were observed in the MADS-box genes of type I and type II in both Balanophora species (Figure 3). The number of the type II gene varied greatly, containing introns ranging from 0 to 8, among which 67% of the type II genes had at least five introns in both Balanophora. In addition, we also analyzed the number of introns of MADS-box genes of autotrophic plants and found that the intron number of type I genes was generally less than that of type II genes (Supplementary Table 5), suggesting that intron number in two types of the MADS-box gene family are conserved during evolution in flowering plants.
Figure 3. Gene structure and conserved motifs analysis of MADS-box genes in B. subcupularis and B. fungosa var. globosa. (A) The exon-intron structure of BsubMADS genes and BgloMADS genes were predicted by Gene Structure Display Server (GSDS) (see text footnote 9). The lines represent introns, and the red boxes indicate exons. The size of the introns and exons can be calculated using the bottom scale. (B) The motifs were identified by the Multiple EM for Motif Elicitation (MEME) program (http://meme-suite.org/tools/meme). Motifs 1–15 were represented by different colors, and the gene names and different groups are shown on the left side of the figure. The ruler at the bottom indicates the amino acid length of the sequences. The details of motifs are described in Supplementary Table 7.
Intriguingly, there were very long introns inserted into the MADS-box genes of Balanophora, for example, the first intron of FLC homologue genes BsubMADS15 and BgloMADS12 are 64 kb and 56 kb, respectively, which are longer than other species (Supplementary Figure 3A). Moreover, the maximum intron length of MADS-box gene in B. subcupularis, B. fungosa var. globosa, and S. himalayana were longer than in other species (Supplementary Figure 3B). Furthermore, we analyzed the eight non-K domain genes of B. subcupularis and B. fungosa var. globosa, which were classified into type II on the phylogenetic tree. Previous studies showed that the length of the 1–6 exons of type II genes are conserved (Johansen et al., 2002). The average length of the first intron of the eight non-K domain genes in two Balanophora (197bp) was highly similar to that of type II (188bp), which was markedly smaller than that of type I genes (647bp) (Supplementary Table 6). Therefore, the results further proved the reliability of the classification of MADS-box non-K domain genes in the phylogenetic tree.
Next, we verified the conserved motifs of MADS-box genes in B. subcupularis and B. fungosa var. globosa using MEME program, then annotated the obtained motifs employing Pfam database. As a result, a total of 15 conserved motifs were identified and named motifs 1–15 (Supplementary Table 7). As shown in Figure 3, genes in the same family tend to have common patterns of conserved motifs, especially the type II gene whose domains are more conserved than type I, such as subgroups SOC1, SEP, AP1/FUL, AP3/PI. The MADS-box genes of B. subcupularis and B. fungosa var. globosa both contained motifs 1, 2, and 3, which were the most typical MADS domain according to Pfam database search. Motif 4, 6, and 10 were verified to be K-box domain, which was another conserved domain, and all MIKCC type genes contained the K domain except BsubMADS14, BsubMADS16. We also observed that motif 7 represented the I domain that existed in all subgroups except for the subgroups AP3/PI, Mγ, Mβ of B. subcupularis and B. fungosa var. globosa. Besides, motif 8, 9, and 14 could be found in Arabidopsis, but absent in two Balanophora. Though the number and lengths of introns are variable in Balanophora, the motifs are conserved between Balanophora and Arabidopsis.
The MADS-box gene family showed stepwise contraction from hemiparasitic to holoparasitic plants (Figure 1B). We analyzed the gene loss of nine parasitic plants, most MADS-box genes of parasitic plants showed a decreasing trend in each subfamily compared with autotrophic plants (Figure 4A). In addition, there was convergent genes loss among different lineages of parasitic plants, during which the most remarkable is the loss of subfamilies AGL6, AGL12, AGL15, AGL17 in Balanophora and S. himalayana, AGL12 and AGL17 were also lost in C. australis. There was some species-specific gene loss, such as the loss of SVP in C. australis, and the loss of SOC1 in S. himalayana (Figure 4A). Hemiparasitic plants showed mild gene loss and most of them were also lost in holoparasites.
Figure 4. Gene loss of MADS-box genes in parasitic plants. (A) The gene loss and retention of 16 subgroups of MADS-box gene family in nine parasitic plants. The tree topology is modified from Nickrent (2020) with Aquilegia coerulea as outgroup, 14 species comprising seven orders (see Supplementary Table 1). The gray circle and square indicate WGD (whole genome duplication) and WGT (whole genome triplication) events, respectively, which were identified by 1KP (Leebens-Mack et al., 2019). The pink and white blocks indicate retain and loss of MADS-box genes in 16 subgroups, respectively. The green, blue, red branches represent autotrophic plants, hemiparasites, and holoparasites, respectively. (B) Gene loss of MADS-box genes in flowering transition. Genes marked in red represent loss and black represent retained genes in B. subcupularis and B. fungosa var. globosa. (C) A conventional flower structure of ABCE model genes. The numbers represent retained ABCE model genes in B. subcupularis, B. fungosa var. globosa, and reference species, while the numbers are obtained based on the phylogenetic tree.
Based on the gene function of their homologies in Arabidopsis, we speculated that AGL12 and AGL17 genes may be related to the degradation of root and photoperiod regulation of flower transition in Balanophora and S. himalayana (Han et al., 2008; Tapia-López et al., 2008; Puig et al., 2013; Shu et al., 2020), they also convergently lost AGL15 and AGL6 genes, among which AGL15 genes play roles in regulating flowering through photoperiod and somatic embryo development (Perry et al., 1999) and AGL6 genes are involved in floral meristem regulation, development of floral organs, and ovule and seed, and AGL6 genes also have possible roles in the development of male and female germline and gametophyte (Dreni and Zhang, 2016). Two Balanophora also lost AGL63 and TRANSPARENT TESTA16 (TT16) genes in GGM13 subfamily, which are involved in fruit development, seed formation, and embryo development (Erdmann et al., 2010). In conclusion, parasitic plants showed convergent and functional-biased gene loss in MADS-box gene families, which may be related to the parasitic lifestyle.
There are several genes related to floral meristem identity, including AP1, FUL (AGL 8), CAL (CAULIFLOWER), AGL24, SVP, SOC1, and LEAFY (LFY) (Gregis et al., 2009; Grandi et al., 2012). We observed that two Balanophora species only lost FUL gene, however, AP1/CAL, and SOC1 genes were lost in S. himalayana (Supplementary Table 8). FUL gene promotes the identity of early flower meristem in coordination with AP1 and appears to be partially redundant to the function of AP1 (Ferrándiz et al., 2000). AP1, AGL24, and SVP control floral meristem identity redundantly by inhibiting the expression of class B, C, and E genes (Gregis et al., 2009). SOC1 regulates the expression of LFY, and LFY is a non-MADS-box gene that links floral induction and floral development (Lee et al., 2008). These results showed that Balanophora and S. himalayana showed different levels of gene loss related to floral meristem, and they may lose those functional redundant genes which were unnecessary for them.
ABCE model genes coordinate together to determine floral organ identity (Soltis et al., 2007; Murai, 2013). Two Balanophora species retained all ABCE model genes (Figure 4C). While no AP1 and no SEP3 homologs were found in S. himalayana, suggesting that the A function does not rely on the function of AP1 homologs or that the perianth has an entirely different contribution. Another holoparasite C. australis also lost AP1 in class A, and PI in class B. The result suggested that holoparasites retained different levels of the ABCE genes, these may be a consequence of the highly modified floral structure or employing different flower identity genes in them.
Flowering is essential to plant reproduction and can be regulated by a variety of pathways, such as photoperiod, circadian clock, vernalization, temperature, and autonomous, etc. (Liu et al., 2015). Previous studies have illustrated that there were at least 16 MADS-box genes involved in the regulation of flowering transition in Arabidopsis, but in our study, only three flowering signal integrators SOC1, SVP, FLC were found to be retained in Balanophora, and most genes were lost, for example, AGL15, AGL17, AGL18, MAF1, MAF3/4/5 genes involved in photoperiod pathway (Adamczyk et al., 2007; Han et al., 2008; Kim and Sung, 2010), AGL19 and MAF2 genes associated with the vernalization pathway (Ratcliffe et al., 2003; Schönrock et al., 2006), AGL28 gene involved in autonomic pathway (Yoo et al., 2006), the SOC1-like genes AGL42, AGL71, AGL72 appeared to act through gibberellin-dependent pathway in Arabidopsis (Dorca-Fornell et al., 2011; Figure 4B and Supplementary Figure 4). We observed that the subfamilies AGL12, AGL15, AGL17, and AGL6 were convergently lost in B. subcupularis, B. fungosa var. globosa, and S. himalayana, while the subfamilies of SOC1 and FLC were specifically lost in S. himalayana, and GGM13 was specifically lost in Balanophora (Figure 4A). Among them, SOC1 gene is an integrator of flowering signals involved in photoperiod, temperature, age, and gibberellin regulatory pathways in Arabidopsis to promote flowering transition, while FLC gene is a repressor of flowering, in part, it retards flowering by inhibiting the expression of SOC1. Another integrator FLC are involved in the vernalization and autonomic pathways (Michaels and Amasino, 1999, 2001; Michaels et al., 2005).
Cuscuta australis is another holoparasitic plant without roots and leaves that also lost many MADS-box genes. We observed that the subfamily SVP was specifically absent in C. australis (Figure 4A), which are involved in flowering time regulation in response to temperature changes by controlling the expression of the FT gene (Lee et al., 2007). Previous research has manifested that C. australis eavesdrops FT signals of the host to control flowering (Shen et al., 2020), taken together, we predicted that Cuscuta may have totally lost this SVP-FT signal pathway. In addition, it also lost subfamilies such as AGL17, AGL12, FLC, and GGM13 (Figure 4A), which showed convergent gene loss with holoparasitic plants. These indicated that holoparasites dramatically lost the flowering transition genes in MADS-box, Balanophora and S. himalayana showed a more severe loss.
Furthermore, we found that the gene loss in semi-parasitic plants with relatively intact body structures was not as pronounced as in holoparasitic plants. For example, S. album only lost subfamilies AGL12 and GGM13, S. parasitica lost AGL17 and GGM13 (Figure 4A). Loss of function in these subfamilies may be compensated by other genes, such as AGL17, a gene associated with root development, was functionally redundant with AGL12 (Tapia-López et al., 2008; Puig et al., 2013).
We further explored the conserved subfamilies in B. subcupularis and B. fungosa var. globosa and discovered that the number of retained genes was decreased and only one gene was kept in some subgroups. For example, the subgroups SVP, SOC1, AP1/FUL, and FLC all retained only one gene in each Balanophora species, while there were more than two copies in Arabidopsis in each subgroup (Figure 4A). We concluded that the redundancy of MADS-box genes in holoparasitic plants was greatly reduced, and they would abandon sequence similar genes and retain the diversity of protein families.
We further investigated genes involved in flower regulation pathways, and found that two Balanophora plants and S. himalayana lost the circadian clock and photoperiod pathways (Figure 5A), comprising the important genes CONSTANS (CO), TOC1, LHY, CCA1, EARLY FLOWERING 4 (ELF4), Two-component response regulator-like APRR9 (PRR9) which are not MADS-box genes (Matsushika et al., 2000; Mizoguchi et al., 2002; McWatters et al., 2007; Nakamichi et al., 2010). However, S. himalayana also some species-specific loss, including FT/FD, SOC1, and FLC functioned as the floral integrator, the FRIGIDA (FRI) complex functioned in vernalization pathways (Choi et al., 2011; Figure 5A). Compared with holoparasitic plants, hemiparasitic plants kept all crucial flowering regulatory genes (Figure 5B). Based on these results, we inferred that Balanophora and Sapria may have lost the photoperiod and circadian clock pathways to control flowering.
Figure 5. Four simplified pathways controlling flowering in parasitic plants and expression profiles of MADS-box genes for Balanophora (Photoperiod, Circadian clock, Autonomous, Vernalization Pathways). Panels (A,B) represent holoparasitic plants B. subcupularis, B. fungosa var. globosa, Sapria and hemiparasitic plants S. parasitica, S. album, M. oleifera, respectively. Red circles represent all lost in holoparasities. Green circles represent all retained in hemiparasites. Yellow represents only lost in Balanophora. Blue represents the loss in Sapria. Purple represents the loss in S. parasitica. The arrow indicates promoting gene activation, the blunt line indicates gene inhibition, and the two endpoints indicate no interaction in known directions. Genes FT, LFY, and SOC1 integrate signals from multiple pathways. And in the pathways, SVP, FLC, SOC1, AGL24, AGL17, and AP1 belong to MADS-box gene family, while the rest genes correspond to non-MADS-box genes. (C) The red and blue colors indicate the expression levels of BgloMADS genes from high to low, and white indicates the median expression level in the heatmap. The tissues cover three stages, stage 1: samples containing different tuber sizes, LC21-YT1-3 (<8 mm, duplicate: LC21-YT1, LC21-YT2, and LC21-YT3), LC21-YT4-6 (8∼15 mm, duplicate: LC21-YT4, LC21-YT5, and LC21-YT6), stage 2 samples without visible inflorescence tissues, tuber and host root were collected and named as LC24-YT1-3 (duplicate: LC24-YT1, LC24-YT2, and LC24-YT3), stage 3 samples with inflorescence were separated into different tissues, including tuber LC24-T3-32 (duplicate: LC24-T3 and LC24-T32), male inflorescence LC22-MF1-3 (duplicate: LC22-MF1, LC22-MF2, LC22-MF3), female inflorescence LC24-FF1-3 (LC24-FF1, LC24-FF2, LC24-FF3), inflorescence stem LC24-S1-2 (duplicate: LC24-S1, LC24-S2), and bracts (LC23-BR1).
To further confirm the functions of MADS-box genes in Balanophora, the transcriptome data from eight different tissues at different developmental stages were analyzed. In the MADS-box genes of B. fungosa var. globosa, the expression of eleven genes could be detected. The other genes cannot be identified because their expression levels were too low, or they have specific expression patterns, which were not collected in this study, it is also possible that they have lost their functions as they adapt to parasitic life. Several genes showed specific expression patterns (Figure 5C), in B. fungosa var. globosa, genes AP1 (BgloMADS23), SEP1 (BgloMADS21), AG (BgloMADS19), STK (BgloMADS18) were highly expressed in the female inflorescence, and AG (BgloMADS19) may be involved in the development of carpel, and STK (BgloMADS18) in controlling the development of ovules of the female flower. Furthermore, PI (BgloMADS15), AP3 (BgloMADS14), AGL33 (BgloMADS11), and SVP (BgloMADS17) were highly expressed in the male inflorescence, and AG (BgloMADS19) was also expressed in male flower, suggesting that PI (BgloMADS15), AP3 (BgloMADS14), and AG (BgloMADS19) may be involved in the development of stamen. The expression pattern of these genes was consistent with the expression pattern of the ABCE model gene. In addition, we noticed that gene FLC (BgloMADS12) with extremely long introns showed high expression levels in the tuber and low expression levels in inflorescence (Figure 5C), which was similar to the expression pattern of FLC in Arabidopsis (highly expressed in the vegetative apex and root tissue). These findings revealed that the expression patterns of MADS-box genes in B. fungosa var. globosa were similar to other species, indicating that the function of these genes may be still conserved.
Because the vascular systems in the tuber of Balanophora contained both host and Balanophora tissues, the intimate connection may facilitate the usage of host signals, especially the long-distance mRNA movement between host and parasites (Kim et al., 2014). We investigated the expression levels of host genes related to regulating flowering in the tuber of Balanophora, and the results showed that several flowering regulation genes from the host were upregulated in the tuber compared to its own root, including (i) genes that can regulate FT or FLC levels transcriptionally or epigenetically, for example, AHL22, EBS, and bHLH63 function in the regulation of FT expression (Piñeiro et al., 2003; Yun et al., 2012; Liu et al., 2013); (ii) CO depression genes including MIP1A and CDF3 (Fornara et al., 2009; Graeff et al., 2016); (iii) Serine/threonine-protein kinase WNK1 regulates flowering time by modulating the photoperiod pathway (Wang et al., 2008; Supplementary Figure 5). Based on these lines of evidence, we speculated one possibility that Balanophora may take advantage of transcripts or proteins from the host plant to retain functionality in pathways of photoperiod regulation of flowering and has not lost associated regulatory pathways completely.
MADS-box transcription factors are important regulators, widely present in eukaryotes and highly conserved, and have been proven to play a crucial role in plant growth and development (Becker et al., 2000; Ng and Yanofsky, 2001; Parenicová et al., 2003). To the best of our knowledge, this is the first attempt to compare MADS-box genes among different parasitic plants. The number of MADS-box genes in holoparasitic plants was significantly reduced compared with that in autotrophic plants. Many MADS-box genes of parasitic plants have been lost during the process of adaption to parasitism.
A phylogenetic tree of the fourteen species was built and divided into sixteen subgroups based on the taxonomy of Arabidopsis (Smaczniak et al., 2012). Phylogenetic analysis revealed that there were eight non-K domain genes clustered into type II. This phenomenon was also found in MADS-box genes of rice, apple, and pear (Arora et al., 2007; Tian et al., 2015; Wang et al., 2017), indicating that it might be common in angiosperms. Studies have shown that MIKC genes are conserved in the length of the 1–6 exons (Johansen et al., 2002), so we analyzed the length of the first exon of these eight genes and found that the length was closer to that of type II genes but different from type I genes, among them, BgloMADS11 was highly expressed in inflorescence, indicated that this gene might be functional. This result illustrated that some MADS-box genes are probably experiencing K domain loss, and at least several genes without K domain are still functional.
Reports on the evolutionary mechanism of phenotypic adaptation indicated gene loss can be beneficial by providing an evolutionary mechanism for phenotypic adaptation (Albalat and Cañestro, 2016; Sahu et al., 2019; Wang et al., 2021; Li et al., 2022). Studies on the flowering mechanism of C. australis show that in order to ensure flowering, dodder eavesdropped on the flowering signal of the host and kept the synchronization of flowering (Sun et al., 2018; Shen et al., 2020). In this study, severe gene loss of flowering regulation genes was also found in B. subcupularis, B. fungosa var. globosa, and S. himalayana, especially the circadian clock and photoperiodic flowering pathways, but there were several photoperiodic flowering response genes from the host plant are upregulated in the chimeric tuber, speculating that Balanophora may take advantage of transcripts or proteins from the host to retain functionality in pathways of photoperiod regulation of flowering, instead of losing the regulatory pathway completely. It is probably a convergent strategy for parasitic plants to steal flowering signals from the host (Shen et al., 2020). However, in hemiparasitic plants, almost all the flowering regulation pathways were retained. Dramatic loss of flower regulation genes in holoparasites analyzed in this study indicated reduced robustness in parasites with extremely degraded structure and high levels of dependence on the host.
Redundant genes generally have a genetic compensation function (i.e., the loss of one gene can be compensated by another with overlapping functions and expression patterns), and probably establish a genetically robust system for adaption (Wagner, 2000; Dean et al., 2008; Rutter et al., 2017; Yang et al., 2022). Studies have indicated that the reduction of gene expression after replication promotes the long-term maintenance of duplicate genes and functional redundancy (Qian et al., 2010). Several subgroups showed genetic redundancy in MADS-box, for example, the AP1/FUL subgroup, including AP1/CAL, FUL, and AGL79, among which AP1/CAL and FUL played important roles in the transition to floral meristem, floral meristem development, perianth, or fruit development (Gu et al., 1998; Ferrándiz et al., 2000; Acri-Nunes-Miranda and Mondragón Palomino, 2014), only one member could be identified in Balanophora (retained AP1/CAL) and Sapria (retained AGL79), respectively (Figure 2). In FLC and SOC1 subgroups, six members were confined to Arabidopsis, respectively, and only one member in each subgroup was identified in Balanophora, with a complete absence in Sapria. The reduction of redundancy in holoparasites, together with the loss of other regulatory genes, indicated that holoparasites may employ a relatively simpler flowering regulation system compared to autotrophs. The simplicity may be due to the utilization of signals directly from the host, or the highly modified structure, or the reduced demand for additional robustness when hosts offer stable environments.
Identification of MADS-box gene family in parasitic plants showed that the MADS-box genes family was contracted step by step from hemiparasites to holoparasites compared to autotrophs. Plenty of MADS-box genes may have been lost in Balanophora and Sapria genomes, and, they seem to have reduced redundancy in conserved subfamilies. Gene loss and redundancy reduction in Balanophora and Sapria indicated that they may not require a complicated network to regulate flowering due to their degraded structure, or relatively stable environments supported by hosts, or they directly use the flowering signals from the host. Overall, this is the first genome-wide analysis of the MADS-box gene family in hemi- and holo-parasites, and it contributes to our understanding of the MADS-box gene family’s categorization, gene loss pattern, and flowering regulatory mechanism in parasitic plants.
The datasets generated for this study can be found in the Supplementary Material, and China National GeneBank DataBase (CNGBdb) database under the accession number: CNP0003054.
HL, XC, and SS led and designed this project. KD, HF, DF, and WZ analyzed the data. KD wrote the original draft manuscript. XC, SS, and KW revised the manuscript. All authors contributed to the article and approved the submitted version.
This study was supported by the Shenzhen Municipal Government of China (No. JCYJ20160331150739027). This study was part of the 10KP project (https://db.cngb.org/10kp/). This study was also supported by China National GeneBank (CNGB; https://www.cngb.org/).
The authors declare that the research was conducted in the absence of any commercial or financial relationships that could be construed as a potential conflict of interest.
All claims expressed in this article are solely those of the authors and do not necessarily represent those of their affiliated organizations, or those of the publisher, the editors and the reviewers. Any product that may be evaluated in this article, or claim that may be made by its manufacturer, is not guaranteed or endorsed by the publisher.
We thank Shuai Yang, Sisi Chen, Fang Wang, Sunhuan Xiang, Yinghui Wang, and Lin Xian for their technical assistance. We thank Runxian Yu for the species identification and photographs.
The Supplementary Material for this article can be found online at: https://www.frontiersin.org/articles/10.3389/fpls.2022.846697/full#supplementary-material
Acri-Nunes-Miranda, R., and Mondragón Palomino, M. (2014). Expression of paralogous SEP-, FUL-, AG- and STK-like MADS-box genes in wild-type and peloric Phalaenopsis flowers. Front. Plant Sci. 5:76. doi: 10.3389/fpls.2014.00076
Adamczyk, B. J., and Fernandez, D. E. (2009). MIKC* MADS Domain Heterodimers Are Required for Pollen Maturation and Tube Growth in Arabidopsis. Plant Physiol. 149, 1713–1723. doi: 10.1104/pp.109.135806
Adamczyk, B. J., Lehti-Shiu, M. D., and Fernandez, D. E. (2007). The MADS domain factors AGL15 and AGL18 act redundantly as repressors of the floral transition in Arabidopsis. Plant J. 50, 1007–1019. doi: 10.1111/j.1365-313X.2007.03105.x
Albalat, R., and Cañestro, C. (2016). Evolution by gene loss. Nat. Rev. Genet. 17, 379–391. doi: 10.1038/nrg.2016.39
Albert, V. A., Barbazuk, W. B., dePamphilis, C. W., Der, J. P., Leebens-Mack, J., Ma, H., et al. (2013). The Amborella Genome and the Evolution of Flowering Plants. Science 342:1241089. doi: 10.1126/science.1241089
Alvarez-Buylla, E. R., Pelaz, S., Liljegren, S. J., Gold, S. E., Burgeff, C., Ditta, G. S., et al. (2000). An ancestral MADS-box gene duplication occurred before the divergence of plants and animals. Proc. Natl. Acad. Sci. U S A. 97, 5328–5333. doi: 10.1073/pnas.97.10.5328
Arora, R., Agarwal, P., Ray, S., Singh, A. K., Singh, V. P., Tyagi, A. K., et al. (2007). MADS-box gene family in rice: genome-wide identification, organization and expression profiling during reproductive development and stress. BMC Genom. 8:242. doi: 10.1186/1471-2164-8-242
Becker, A., Winter, K.-U., Meyer, B., Saedler, H., and Theißen, G. (2000). MADS-Box Gene Diversity in Seed Plants 300 Million Years Ago. Mol. Biol. Evol. 17, 1425–1434. doi: 10.1093/oxfordjournals.molbev.a026243
Cai, L., Arnold, B. J., Xi, Z., Khost, D. E., Patel, N., Hartmann, C. B., et al. (2021). Deeply Altered Genome Architecture in the Endoparasitic Flowering Plant Sapria himalayana Griff. (Rafflesiaceae). Curr. Biol. 31, 1002–1011.e9. doi: 10.1016/j.cub.2020.12.045
Chen, X., Fang, D., Wu, C., Liu, B., Liu, Y., Sahu, S. K., et al. (2020). Comparative plastome analysis of root-and stem-feeding parasites of Santalales untangle the footprints of feeding mode and lifestyle transitions. Genom. Biol. Evol. 12, 3663–3676. doi: 10.1093/gbe/evz271
Choi, K., Kim, J., Hwang, H. J., Kim, S., Park, C., Kim, S. Y., et al. (2011). The FRIGIDA complex activates transcription of FLC, a strong flowering repressor in Arabidopsis, by recruiting chromatin modification factors. Plant Cell 23, 289–303. doi: 10.1105/tpc.110.075911
Clarke, C. R., Timko, M. P., Yoder, J. I., Axtell, M. J., and Westwood, J. H. (2019). Molecular Dialog Between Parasitic Plants and Their Hosts. Annu. Rev. Phytopathol. 57, 279–299. doi: 10.1146/annurev-phyto-082718-100043
Dasgupta, M. G., Ulaganathan, K., Dev, S. A., and Balakrishnan, S. (2019). Draft genome of Santalum album L. provides genomic resources for accelerated trait improvement. Tree Genet. Genomes 15:34. doi: 10.1007/s11295-019-1334-9
Dean, E. J., Davis, J. C., Davis, R. W., and Petrov, D. A. (2008). Pervasive and persistent redundancy among duplicated genes in Yeast. PLoS Genet. 4:e1000113. doi: 10.1371/journal.pgen.1000113
Dorca-Fornell, C., Gregis, V., Grandi, V., Coupland, G., Colombo, L., and Kater, M. M. (2011). The Arabidopsis SOC1-like genes AGL42, AGL71 and AGL72 promote flowering in the shoot apical and axillary meristems. Plant J. 67, 1006–1017. doi: 10.1111/j.1365-313X.2011.04653.x
Dreni, L., and Zhang, D. (2016). Flower development: the evolutionary history and functions of the AGL6 subfamily MADS-box genes. J. Exp. Bot. 67, 1625–1638. doi: 10.1093/jxb/erw046
Eberwein, R., Nickrent, D., and Weber, A. (2009). Development and morphology of flowers and inflorescences in Balanophora Papuana And B. Elongata (Balanophoraceae). Am. J. Bot. 96, 1055–1067. doi: 10.3732/ajb.0800289
Ehlers, K., Bhide, A. S., Tekleyohans, D. G., Wittkop, B., Snowdon, R. J., and Becker, A. (2016). The MADS Box Genes ABS, SHP1, and SHP2 Are Essential for the Coordination of Cell Divisions in Ovule and Seed Coat Development and for Endosperm Formation in Arabidopsis thaliana. PLoS One 11:e0165075. doi: 10.1371/journal.pone.0165075
Erdmann, R., Gramzow, L., Melzer, R., Theißen, G., and Becker, A. (2010). GORDITA (AGL63) is a young paralog of the Arabidopsis thaliana Bsister MADS box gene ABS (TT16) that has undergone neofunctionalization. Plant J. 63, 914–924. doi: 10.1111/j.1365-313X.2010.04290.x
Fatima, M., Zhang, X., Lin, J., Zhou, P., Zhou, D., and Ming, R. (2020). Expression profiling of MADS-box gene family revealed its role in vegetative development and stem ripening in S. spontaneum. Sci. Rep. 10:20536. doi: 10.1038/s41598-020-77375-6
Ferrándiz, C., Gu, Q., Martienssen, R., and Yanofsky, M. F. (2000). Redundant regulation of meristem identity and plant architecture by FRUITFULL, APETALA1 and CAULIFLOWER. Development 127, 725–734. doi: 10.1242/dev.127.4.725
Fornara, F., Panigrahi, K. C., Gissot, L., Sauerbrunn, N., Rühl, M., Jarillo, J. A., et al. (2009). Arabidopsis DOF transcription factors act redundantly to reduce CONSTANS expression and are essential for a photoperiodic flowering response. Dev. Cell 17, 75–86. doi: 10.1016/j.devcel.2009.06.015
Garay-Arroyo, A., Ortiz-Moreno, E., de la Paz Sánchez, M., Murphy, A. S., García-Ponce, B., Marsch-Martínez, N., et al. (2013). The MADS transcription factor XAL2/AGL14 modulates auxin transport during Arabidopsis root development by regulating PIN expression. EMBO J. 32, 2884–2895. doi: 10.1038/emboj.2013.216
Graeff, M., Straub, D., Eguen, T., Dolde, U., Rodrigues, V., Brandt, R., et al. (2016). MicroProtein-Mediated Recruitment of CONSTANS into a TOPLESS Trimeric Complex Represses Flowering in Arabidopsis. PLoS Genet. 12:e1005959. doi: 10.1371/journal.pgen.1005959
Gramzow, L., Ritz, M. S., and Theissen, G. (2010). On the origin of MADS-domain transcription factors. Trends Genet. 26, 149–153. doi: 10.1016/j.tig.2010.01.004
Grandi, V., Gregis, V., and Kater, M. M. (2012). Uncovering genetic and molecular interactions among floral meristem identity genes in Arabidopsis thaliana. Plant J. 69, 881–893. doi: 10.1111/j.1365-313X.2011.04840.x
Gregis, V., Sessa, A., Dorca-Fornell, C., and Kater, M. M. (2009). The Arabidopsis floral meristem identity genes AP1, AGL24 and SVP directly repress class B and C floral homeotic genes. Plant J. 60, 626–637. doi: 10.1111/j.1365-313X.2009.03985.x
Gu, Q., Ferrandiz, C., Yanofsky, M. F., and Martienssen, R. (1998). The FRUITFULL MADS-box gene mediates cell differentiation during Arabidopsis fruit development. Development 125, 1509–1517. doi: 10.1242/dev.125.8.1509
Han, P., García-Ponce, B., Fonseca-Salazar, G., Alvarez-Buylla, E. R., and Yu, H. (2008). AGAMOUS-LIKE 17, a novel flowering promoter, acts in a FT-independent photoperiod pathway. Plant J. 55, 253–265. doi: 10.1111/j.1365-313X.2008.03499.x
Hartmann, U., Höhmann, S., Nettesheim, K., Wisman, E., Saedler, H., and Huijser, P. (2000). Molecular cloning of SVP: a negative regulator of the floral transition in Arabidopsis. Plant J. 21, 351–360. doi: 10.1046/j.1365-313x.2000.00682.x
Henschel, K., Kofuji, R., Hasebe, M., Saedler, H., Münster, T., and Theissen, G. (2002). Two ancient classes of MIKC-type MADS-box genes are present in the moss Physcomitrella patens. Mol. Biol. Evol. 19, 801–814. doi: 10.1093/oxfordjournals.molbev.a004137
Hsiao, S.-C., Mauseth, J. D., and Gomez, L. D. (1994). Growth and Anatomy of the Vegetative Body of the Parasitic Angiosperm Langsdorffia hypogaea (Balanophoraceae). Bull. Torrey Bot. Club. 121, 24–39. doi: 10.2307/2996881
Hsiao, S.-C., Mauseth, J. D., and Peng, C.-I. (1995). Composite bundles, the host/parasite interface in the holoparasitic angiosperms Langsdorffia and Balanophora (Balanophoraceae). Am. J. Bot. 82, 81–91. doi: 10.1002/j.1537-2197.1995.tb15652.x
Johansen, B., Pedersen, L. B., Skipper, M., and Frederiksen, S. (2002). MADS-box gene evolution-structure and transcription patterns. Mol. Phylogenet. Evol. 23, 458–480. doi: 10.1016/s1055-7903(02)00032-5
Kaufmann, K., Melzer, R., and Theissen, G. (2005). MIKC-type MADS-domain proteins: structural modularity, protein interactions and network evolution in land plants. Gene 347, 183–198. doi: 10.1016/j.gene.2004.12.014
Kim, D. H., and Sung, S. (2010). The Plant Homeo Domain finger protein, VIN3-LIKE 2, is necessary for photoperiod-mediated epigenetic regulation of the floral repressor, MAF5. Proc. Natl. Acad. Sci. U S A. 107, 17029–17034. doi: 10.1073/pnas.1010834107
Kim, G., LeBlanc, M. L., Wafula, E. K., dePamphilis, C. W., and Westwood, J. H. (2014). Genomic-scale exchange of mRNA between a parasitic plant and its hosts. Science 345, 808–811. doi: 10.1126/science.1253122
Kountche, B. A., Jamil, M., Yonli, D., Nikiema, M. P., Blanco-Ania, D., Asami, T., et al. (2019). Suicidal germination as a control strategy for Striga hermonthica (Benth.) in smallholder farms of sub-Saharan Africa. Plants People Planet 1, 107–118. doi: 10.1002/ppp3.32
Lee, J., Oh, M., Park, H., and Lee, I. (2008). SOC1 translocated to the nucleus by interaction with AGL24 directly regulates leafy. Plant J. 55, 832–843. doi: 10.1111/j.1365-313X.2008.03552.x
Lee, J. H., Yoo, S. J., Park, S. H., Hwang, I., Lee, J. S., and Ahn, J. H. (2007). Role of SVP in the control of flowering time by ambient temperature in Arabidopsis. Genes Dev. 21, 397–402. doi: 10.1101/gad.1518407
Leebens-Mack, J. H., Barker, M. S., Carpenter, E. J., Deyholos, M. K., Gitzendanner, M. A., Graham, S. W., et al. (2019). One thousand plant transcriptomes and the phylogenomics of green plants. Nature 574, 679–685. doi: 10.1038/s41586-019-1693-2
Li, L., Chen, X., Fang, D., Dong, S., Guo, X., Li, N., et al. (2022). Genomes shed light on the evolution of Begonia, a mega-diverse genus. New Phytol. 234, 295–310. doi: 10.1111/nph.17949
Liljegren, S. J., Ditta, G. S., Eshed, Y., Savidge, B., Bowman, J. L., and Yanofsky, M. F. (2000). SHATTERPROOF MADS-box genes control seed dispersal in Arabidopsis. Nature 404, 766–770. doi: 10.1038/35008089
Liu, J., Fu, X., Dong, Y., Lu, J., Ren, M., Zhou, N., et al. (2018). MIKCC-type MADS-box genes in Rosa chinensis: the remarkable expansion of ABCDE model genes and their roles in floral organogenesis. Horticult. Res. 5:25. doi: 10.1038/s41438-018-0031-4
Liu, Y., Li, X., Li, K., Liu, H., and Lin, C. (2013). Multiple bHLH proteins form heterodimers to mediate CRY2-dependent regulation of flowering-time in Arabidopsis. PLoS Genet. 9:e1003861. doi: 10.1371/journal.pgen.1003861
Liu, Y., Yang, J., and Yang, M. (2015). [Pathways of flowering regulation in plants]. Sheng Wu Gong Cheng Xue Bao 31, 1553–1566.
Matsushika, A., Makino, S., Kojima, M., and Mizuno, T. (2000). Circadian waves of expression of the APRR1/TOC1 family of pseudo-response regulators in Arabidopsis thaliana: insight into the plant circadian clock. Plant Cell Physiol. 41, 1002–1012. doi: 10.1093/pcp/pcd043
McWatters, H. G., Kolmos, E., Hall, A., Doyle, M. R., Amasino, R. M., Gyula, P., et al. (2007). ELF4 is required for oscillatory properties of the circadian clock. Plant Physiol. 144, 391–401. doi: 10.1104/pp.107.096206
Melzer, R., and Theissen, G. (2009). Reconstitution of ‘floral quartets’ in vitro involving class B and class E floral homeotic proteins. Nucleic Acids Res. 37, 2723–2736. doi: 10.1093/nar/gkp129
Michaels, S. D., and Amasino, R. M. (1999). FLOWERING LOCUS C Encodes a Novel MADS Domain Protein That Acts as a Repressor of Flowering. Plant Cell 11, 949–956. doi: 10.1105/tpc.11.5.949
Michaels, S. D., and Amasino, R. M. (2001). Loss of FLOWERING LOCUS C activity eliminates the late-flowering phenotype of FRIGIDA and autonomous pathway mutations but not responsiveness to vernalization. Plant Cell 13, 935–941. doi: 10.1105/tpc.13.4.935
Michaels, S. D., Himelblau, E., Kim, S. Y., Schomburg, F. M., and Amasino, R. M. (2005). Integration of Flowering Signals in Winter-Annual Arabidopsis. Plant Physiol. 137, 149–156. doi: 10.1104/pp.104.052811
Mizoguchi, T., Wheatley, K., Hanzawa, Y., Wright, L., Mizoguchi, M., Song, H. R., et al. (2002). LHY and CCA1 are partially redundant genes required to maintain circadian rhythms in Arabidopsis. Dev. Cell 2, 629–641. doi: 10.1016/s1534-5807(02)00170-3
Moreno-Risueno, M. A., Norman, J. M. V., Moreno, A., Zhang, J., Ahnert, S. E., and Benfey, P. N. (2010). Oscillating Gene Expression Determines Competence for Periodic Arabidopsis Root Branching. Science 329, 1306–1311. doi: 10.1126/science.1191937
Murai, K. (2013). Homeotic Genes and the ABCDE Model for Floral Organ Formation in Wheat. Plants 2, 379–395. doi: 10.3390/plants2030379
Nakamichi, N., Kiba, T., Henriques, R., Mizuno, T., Chua, N. H., and Sakakibara, H. (2010). PSEUDO-RESPONSE REGULATORS 9, 7, and 5 are transcriptional repressors in the Arabidopsis circadian clock. Plant Cell 22, 594–605. doi: 10.1105/tpc.109.072892
Ng, M., and Yanofsky, M. F. (2001). Function and evolution of the plant MADS-box gene family. Nat. Rev. Genet. 2, 186–195. doi: 10.1038/35056041
Nickrent, D. L. (2020). Parasitic angiosperms: how often and how many? Taxon 69, 5–27. doi: 10.1002/tax.12195
Pan, Z. J., Chen, Y. Y., Du, J. S., Chen, Y. Y., Chung, M. C., Tsai, W. C., et al. (2014). Flower development of Phalaenopsis orchid involves functionally divergent SEPALLATA-like genes. New Phytol. 202, 1024–1042. doi: 10.1111/nph.12723
Parenicová, L., de Folter, S., Kieffer, M., Horner, D. S., Favalli, C., Busscher, J., et al. (2003). Molecular and phylogenetic analyses of the complete MADS-box transcription factor family in Arabidopsis: new openings to the MADS world. Plant Cell 15, 1538–1551. doi: 10.1105/tpc.011544
Perry, S. E., Lehti, M. D., and Fernandez, D. E. (1999). The MADS-domain protein AGAMOUS-like 15 accumulates in embryonic tissues with diverse origins. Plant Physiol. 120, 121–130. doi: 10.1104/pp.120.1.121
Piñeiro, M., Gómez-Mena, C., Schaffer, R., Martínez-Zapater, J. M., and Coupland, G. (2003). EARLY BOLTING IN SHORT DAYS is related to chromatin remodeling factors and regulates flowering in Arabidopsis by repressing FT. Plant Cell 15, 1552–1562. doi: 10.1105/tpc.012153
Puig, J., Meynard, D., Khong, G. N., Pauluzzi, G., Guiderdoni, E., and Gantet, P. (2013). Analysis of the expression of the AGL17-like clade of MADS-box transcription factors in rice. Gene. Expr. Patterns 13, 160–170. doi: 10.1016/j.gep.2013.02.004
Qian, W., Liao, B.-Y., Chang, A. Y.-F., and Zhang, J. (2010). Maintenance of duplicate genes and their functional redundancy by reduced expression. Trends Genet. 26, 425–430. doi: 10.1016/j.tig.2010.07.002
Ratcliffe, O. J., Kumimoto, R. W., Wong, B. J., and Riechmann, J. L. (2003). Analysis of the Arabidopsis MADS AFFECTING FLOWERING gene family: MAF2 prevents vernalization by short periods of cold. Plant Cell 15, 1159–1169. doi: 10.1105/tpc.009506
Riechmann, J. L., and Meyerowitz, E. M. (1997). Determination of floral organ identity by Arabidopsis MADS domain homeotic proteins AP1, AP3, PI, and AG is independent of their DNA-binding specificity. Mol. Bio. Cell 8, 1243–1259. doi: 10.1091/mbc.8.7.1243
Riechmann, J. L., Wang, M., and Meyerowitz, E. M. (1996). DNA-binding properties of Arabidopsis MADS domain homeotic proteins APETALA1, APETALA3, PISTILLATA and AGAMOUS. Nucleic Acids Res. 24, 3134–3141. doi: 10.1093/nar/24.16.3134
Rutter, M. T., Wieckowski, Y. M., Murren, C. J., and Strand, A. E. (2017). Fitness effects of mutation: testing genetic redundancy in Arabidopsis thaliana. J. Evol. Biol. 30, 1124–1135. doi: 10.1111/jeb.13081
Sahu, S. K., Liu, M., Yssel, A., Kariba, R., Muthemba, S., Jiang, S., et al. (2019). Draft Genomes of Two Artocarpus Plants, Jackfruit (A. heterophyllus) and Breadfruit (A. altilis). Genes 11:27. doi: 10.3390/genes11010027
Samach, A., Onouchi, H., Gold, S. E., Ditta, G. S., Schwarz-Sommer, Z., Yanofsky, M. F., et al. (2000). Distinct roles of CONSTANS target genes in reproductive development of Arabidopsis. Science 288, 1613–1616. doi: 10.1126/science.288.5471.1613
Schilling, S., Pan, S., Kennedy, A., and Melzer, R. (2018). MADS-box genes and crop domestication: the jack of all traits. J. Exp. Bot. 69, 1447–1469. doi: 10.1093/jxb/erx479
Schönrock, N., Bouveret, R., Leroy, O., Borghi, L., Köhler, C., Gruissem, W., et al. (2006). Polycomb-group proteins repress the floral activator AGL19 in the FLC-independent vernalization pathway. Genes Dev. 20, 1667–1678. doi: 10.1101/gad.377206
Shen, G., Liu, N., Zhang, J., Xu, Y., Baldwin, I. T., and Wu, J. (2020). Cuscuta australis (dodder) parasite eavesdrops on the host plants’ FT signals to flower. Proc. Natl. Acad. Sci. U S A. 117, 23125–23130. doi: 10.1073/pnas.2009445117
Shivamurthy, G. R., Arekal, G. D., and Swamy, B. G. L. (1981a). Establishment, Structure and Morphology of the Tuber of Balanophora. Ann. Bot. 47, 735–745. doi: 10.1093/oxfordjournals.aob.a086072
Shivamurthy, G. R., Swamy, B. G. L., and Arekal, G. D. (1981b). Ontogeny and Organization of the Inflorescence in Balanophora. Ann. Bot. 48, 853–859. doi: 10.1093/oxfordjournals.aob.a086192
Shu, J., Chen, C., Kohalmi, S. E., and Cui, Y. (2020). Evidence that AGL17 is a significant downstream target of CLF in floral transition control. Plant Signal Behav. 15:1766851. doi: 10.1080/15592324.2020.1766851
Smaczniak, C., Immink, R. G., Angenent, G. C., and Kaufmann, K. (2012). Developmental and evolutionary diversity of plant MADS-domain factors: insights from recent studies. Development 139, 3081–3098. doi: 10.1242/dev.074674
Soltis, D. E., Chanderbali, A. S., Kim, S., Buzgo, M., and Soltis, P. S. (2007). The ABC Model and its Applicability to Basal Angiosperms. Ann. Bot. 100, 155–163. doi: 10.1093/aob/mcm117
Sun, G., Xu, Y., Liu, H., Sun, T., Zhang, J., Hettenhausen, C., et al. (2018). Large-scale gene losses underlie the genome evolution of parasitic plant Cuscuta australis. Nat. Commun. 9:2683. doi: 10.1038/s41467-018-04721-8
Tapia-López, R., Garciía-Ponce, B., Dubrovsky, J. G., Garay-Arroyo, A., Pérez-Ruiíz, R. V., Kim, S.-H., et al. (2008). An AGAMOUS-Related MADS-Box Gene, XAL1 (AGL12), Regulates Root Meristem Cell Proliferation and Flowering Transition in Arabidopsis. Plant Physiol. 146, 1182–1192. doi: 10.1104/pp.107.108647
Theissen, G., and Saedler, H. (2001). Plant biology. Floral quartets. Nature 409, 469–471. doi: 10.1038/35054172
Theißen, G., Kim, J. T., and Saedler, H. (1996). Classification and phylogeny of the MADS-box multigene family suggest defined roles of MADS-box gene subfamilies in the morphological evolution of eukaryotes. J. Mol. Evol. 43, 484–516. doi: 10.1007/BF02337521
Theißen, G., Melzer, R., and Rümpler, F. (2016). MADS-domain transcription factors and the floral quartet model of flower development: linking plant development and evolution. Development 143, 3259–3271. doi: 10.1242/dev.134080
Tian, Y., Dong, Q., Ji, Z., Chi, F., Cong, P., and Zhou, Z. (2015). Genome-wide identification and analysis of the MADS-box gene family in apple. Gene 555, 277–290. doi: 10.1016/j.gene.2014.11.018
Wagner, A. (2000). Robustness against mutations in genetic networks of yeast. Nat. Genet. 24, 355–361. doi: 10.1038/74174
Wang, R., Ming, M., Li, J., Shi, D., Qiao, X., Li, L., et al. (2017). Genome-wide identification of the MADS-box transcription factor family in pear (Pyrus bretschneideri) reveals evolution and functional divergence. Peer J 5:e3776. doi: 10.7717/peerj.3776
Wang, S., Liang, H., Xu, Y., Li, L., Wang, H., Sahu, D. N., et al. (2021). Genome-wide analyses across Viridiplantae reveal the origin and diversification of small RNA pathway-related genes. Commun. Biol. 4:412. doi: 10.1038/s42003-021-01933-5
Wang, Y., Liu, K., Liao, H., Zhuang, C., Ma, H., and Yan, X. (2008). The plant WNK gene family and regulation of flowering time in Arabidopsis. Plant Biol. 10, 548–562. doi: 10.1111/j.1438-8677.2008.00072.x
Wang, Y., Zhang, J., Hu, Z., Guo, X., Tian, S., and Chen, G. (2019). Genome-Wide Analysis of the MADS-Box Transcription Factor Family in Solanum lycopersicum. Int. J. Mol. Sci. 20:2961. doi: 10.3390/ijms20122961
Weigel, D., and Meyerowitz, E. M. (1994). The ABCs of floral homeotic genes. Cell 78, 203–209. doi: 10.1016/0092-8674(94)90291-7
Westwood, J. H., Yoder, J. I., Timko, M. P., and Depamphilis, C. W. (2010). The evolution of parasitism in plants. Trends Plant Sci. 15, 227–235. doi: 10.1016/j.tplants.2010.01.004
Xu, C. Q., Liu, H., Zhou, S. S., Zhang, D. X., Zhao, W., Wang, S., et al. (2019). Genome sequence of Malania oleifera, a tree with great value for nervonic acid production. Gigascience 8:giy164. doi: 10.1093/gigascience/giy164
Xu, W., Fiume, E., Coen, O., Pechoux, C., Lepiniec, L., and Magnani, E. (2016). Endosperm and Nucellus Develop Antagonistically in Arabidopsis Seeds. Plant Cell 28, 1343–1360. doi: 10.1105/tpc.16.00041
Yang, T., Sahu, S. K., Yang, L., Liu, Y., Mu, W., Liu, X., et al. (2022). Comparative Analyses of 3,654 Plastid Genomes Unravel Insights Into Evolutionary Dynamics and Phylogenetic Discordance of Green Plants. Front. Plant Sci. 13:808156. doi: 10.3389/fpls.2022.808156
Yoo, S. K., Lee, J. S., and Ahn, J. H. (2006). Overexpression of AGAMOUS-LIKE 28 (AGL28) promotes flowering by upregulating expression of floral promoters within the autonomous pathway. Biochem. Biophys. Res. Commun. 348, 929–936. doi: 10.1016/j.bbrc.2006.07.121
Yoshida, S., Kim, S., Wafula, E. K., Tanskanen, J., Kim, Y.-M., Honaas, L., et al. (2019). Genome sequence of Striga asiatica provides insight into the evolution of plant parasitism. Curr. Biol. 29, 3041–3052.e4. doi: 10.1016/j.cub.2019.07.086
Keywords: parasitic plants, MADS-box gene family, gene loss, gene redundancy, flowering, holoparasitic plant, Balanophora, phylogeny
Citation: Duan K, Fu H, Fang D, Wang K, Zhang W, Liu H, Sahu SK and Chen X (2022) Genome-Wide Analysis of the MADS-Box Gene Family in Holoparasitic Plants (Balanophora subcupularis and Balanophora fungosa var. globosa). Front. Plant Sci. 13:846697. doi: 10.3389/fpls.2022.846697
Received: 31 December 2021; Accepted: 26 April 2022;
Published: 31 May 2022.
Edited by:
Natalia Pabón-Mora, University of Antioquia, ColombiaReviewed by:
Guiling Sun, Henan University, ChinaCopyright © 2022 Duan, Fu, Fang, Wang, Zhang, Liu, Sahu and Chen. This is an open-access article distributed under the terms of the Creative Commons Attribution License (CC BY). The use, distribution or reproduction in other forums is permitted, provided the original author(s) and the copyright owner(s) are credited and that the original publication in this journal is cited, in accordance with accepted academic practice. No use, distribution or reproduction is permitted which does not comply with these terms.
*Correspondence: Sunil Kumar Sahu, c3VuaWxrdW1hcnNhaHVAZ2Vub21pY3MuY24=; Xiaoli Chen, Y2hlbnhpYW9saUBnZW5vbWljcy5jbg==
Disclaimer: All claims expressed in this article are solely those of the authors and do not necessarily represent those of their affiliated organizations, or those of the publisher, the editors and the reviewers. Any product that may be evaluated in this article or claim that may be made by its manufacturer is not guaranteed or endorsed by the publisher.
Research integrity at Frontiers
Learn more about the work of our research integrity team to safeguard the quality of each article we publish.