- 1MOA Key Laboratory of Crop Ecophysiology and Farming System in the Middle Reaches of the Yangtze River, College of Plant Science and Technology, Huazhong Agricultural University, Wuhan, China
- 2Henan Collaborative Innovation Centre of Modern Biological Breeding, Henan Institute of Science and Technology, Xinxiang, China
- 3Shenzhen Institute of Nutrition and Health, Huazhong Agricultural University, Shenzhen, China
- 4Shenzhen Branch, Guangdong Laboratory for Lingnan Modern Agriculture, Genome Analysis Laboratory of the Ministry of Agriculture, Agricultural Genomics Institute at Shenzhen, Chinese Academy of Agricultural Sciences, Shenzhen, China
The area of salinized land is gradually expanding cross the globe. Salt stress seriously reduces the yield and quality of crops and endangers food supply to meet the demand of the increased population. The mechanisms underlying nano-enabled plant tolerance were discussed, including (1) maintaining ROS homeostasis, (2) improving plant’s ability to exclude Na+ and to retain K+, (3) improving the production of nitric oxide, (4) increasing α-amylase activities to increase soluble sugar content, and (5) decreasing lipoxygenase activities to reduce membrane oxidative damage. The possible commonly employed mechanisms such as alleviating oxidative stress damage and maintaining ion homeostasis were highlighted. Further, the possible role of phytohormones and the molecular mechanisms in nano-enabled plant salt tolerance were discussed. Overall, this review paper aims to help the researchers from different field such as plant science and nanoscience to better understand possible new approaches to address salinity issues in agriculture.
Introduction
Salinity is a main stress-limiting agricultural production. Feeding over 9.3 billion populations in 2050 is a big challenge. It is estimated that in 2050, agricultural production needs to be increased over 60% at the 2005–2007 level (Fita et al., 2015). However, efficient agricultural production is always threatened by stress conditions such as salinity. In recent years, climate change, seawater backflow, groundwater infiltration, and human-being activities such as irrigation and fertilizer application increased salt concentration in soil, resulting in soil salinization (Zhang et al., 2016; Qian et al., 2021). Soil salinization inhibits plant growth, yield, and product quality (Yang and Guo, 2018a). While more than 950 million hectares of land are affected by salinity stress, the trend of soil salinization is increasing (Yang and Guo, 2018b).
The main components of salt stress in plants are osmotic stress, ionic stress, and secondary stress, i.e., ROS over-accumulation (Parihar et al., 2015; Morton et al., 2018). Firstly, upon the onset of salt stress, high salinity reduces the water potential around the plant roots, limiting root absorption of water (Negrão et al., 2016). Secondly, over-accumulation of sodium and chloride in plants causes ion toxicity. It not only disrupts ion homeostasis such as Na+ and K+ homeostasis (Zhu, 2002), but also hinders the efficient uptake of nutrient elements such as Ca2+, resulting in the lack of essential nutrients in plants (Zhang et al., 2017; Wu, 2018a; Li et al., 2021). Osmotic and ionic stresses lead to over-accumulation of reactive oxygen species (ROS) in plants, resulting in oxidative stress (Zhu, 2016; Wu et al., 2018b). For example, excessive superoxide anion (O2−) and hydrogen peroxide (H2O2) are accumulated in chloroplasts and mitochondria, affecting photosynthesis and respiration of plants under salt stress (Balal et al., 2011). Moreover, the structure of macromolecules such as DNA and protein can be damaged by excessive ROS (Hu et al., 2021; Liu et al., 2021a). Nowadays, besides genetic engineering and exogenous application of antioxidants, nanomaterials showed good potential in improving plant salt tolerance although the underlying mechanisms are less addressed. Nano-enabled plant salt tolerance could be an alternative approach to help to enable efficient agricultural production.
In this review, we summarized the molecular mechanisms underlying plant salt tolerance and emphasized the importance of nanotechnology in improving plant salt tolerance. We hope this review will set up an idea to help the researchers in plant science and nanoscience to better understand possible new approaches to address issues such as salinity in agriculture.
Use of Nanomaterials: an Emerging Approach to Improve Plant Salt Tolerance
In recent years, plant nano-biotechnology approach showed good potential to improve plant stress tolerance. Nano-enabled agriculture is a hot research topic. Nanotechnology refers to the technology of manipulating materials with a basic structure of 1–100 nm in at least one dimension (Farokhzad and Langer, 2009). The history of adoption of nanotechnology in agriculture is relatively short, but it showed great potential in agriculture, such as the development of nano-fertilizer, nano-pesticides, and smart plant construction (Giraldo et al., 2019; Kah et al., 2019). As an emerging strategy to promote agricultural production, plant nanotechnology shows good potential in agriculture, such as seed treatment and germination, plant growth and development, pathogen diagnosis, genetic engineering, plant stress tolerance, crop nutrition, and detection of toxic agrochemicals (Farooq et al., 2012; Bui, 2013; Kah et al., 2019). In recent years, use of nanomaterials (NMs) to enhance plant stress tolerance showed the potential to become an economical, effective, and sustainable strategy for efficient agricultural production. NMs enhance plant tolerance to salt by protecting plant photosynthesis, enabling ROS detoxification, and alleviating osmotic and ionic stress (Gao et al., 2007; Rico et al., 2015; Khan et al., 2016a). Nano-enabled plant salt tolerance has been reported in many species, including Arabidopsis, wheat, cotton, and so on (Mushtaq et al., 2017; Michael et al., 2018; Liu et al., 2021b). To date, the used nanomaterials which improved plant salt tolerance include silica nanoparticles, cerium oxide nanoparticles, put-carbon quantum dots (put-CQD) nanoparticles, titanium dioxide nanoparticles, carbon nanotubes, and nano-zinc (Figure 1). For example, multi-walled carbon nanotubes (MWCNTs), cerium oxide nanoparticles, and zinc oxide nanoparticles (SeNPs and ZnONPs) can significantly alleviate the inhibition of salt stress on the growth of rapeseed seedlings. Seed priming using cerium oxide nanoparticles, SeNPs and ZnONPs, also significantly improves the germination rate of rapeseed seeds under salt stress (Rossi et al., 2017; Zhao et al., 2019; El-Badri et al., 2021; Khan et al., 2021; Li et al., 2022). For more details, please refer to Table 1.
As mentioned in Table 1, many nanomaterials are used to improve plant salt tolerance. Among them, cerium oxide nanoparticles (nanoceria) are one of the widely used nanomaterials. Thus, here, we used nanoceria as example to discuss how nanomaterials can help to improve plant salt tolerance. Nanoceria are known as nanozyme and potent catalytic ROS (reactive oxygen species) scavenger, having a large number of surface oxygen vacancies which can convert ROS, i.e., H2O2, O2−, and •OH to its non-radical counterparts. To date, the mechanisms behind nanoceria improved plant salt tolerance (Figure 2) are: (1) maintaining ROS homeostasis via direct scavenging of ROS or modulating antioxidant system (Rossi et al., 2017; Wu et al., 2018c), (2) improving mesophyll cells’ ability to retain K+ (Wu et al., 2018d), (3) improving shoot Na+ exclusion ability to avoid over-accumulation of Na+ in leaf (Liu et al., 2021a), (4) improving the production of gas signaling molecules, i.e., NO (nitric oxide; Zhou et al., 2021), (5) increasing α-amylase activities to improving seed germination (Khan et al., 2021), (6) decreasing lipoxygenase activities to reduce membrane oxidative damage (Li et al., 2022 ES Nano), and (7) allowing Na+ being transported to shoot via shortening root apoplastic barriers (Rossi et al., 2017). Some of these mechanisms might be shared between different nanomaterials in terms of improving plant salt tolerance. For example, Mn3O4 nanoparticles scavenged over-accumulated ROS to improve salt tolerance in cucumber (Lu et al., 2020). Zinc oxide nanoparticles modulated the activities of antioxidant enzymes to help to maintain ROS homeostasis in tomato plants (Faizan et al., 2021). These results suggested that in different nanomaterials can execute similar role to improve salt tolerance in varied plant species. Further, MWCNTs increased rapeseed plants’ ability to tolerate salinity by maintaining Na+/K+ ratio (Zhao et al., 2019). This is similar to the findings that nanoceria can help to maintain Na+/K+ ratio to improve cotton salt tolerance (Liu et al., 2021a), further confirming that some common mechanisms might be employed in nano-enabled plant salt tolerance. More efforts are needed to investigate what are the commonly employed mechanisms in nano-enabled plant salt tolerance and what are the mechanisms specially associated with one or few types of nanomaterials.
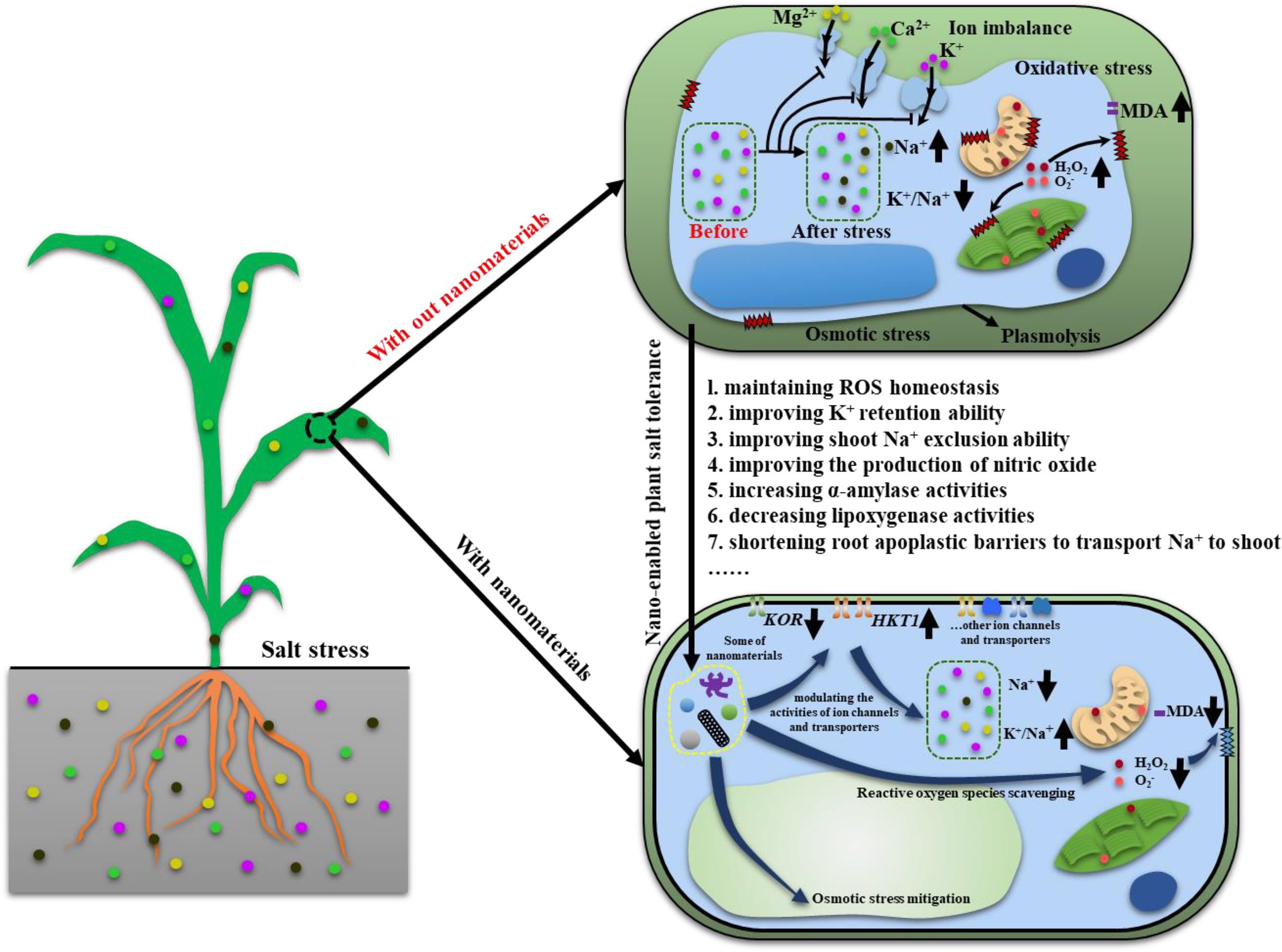
Figure 2. A model showing how nanomaterials can help with maintaining osmotic balance, ion homeostasis and ROS homeostasis.↑: increase, ↓: decrease, ┤: inhibition.
Plant’s Ability to Alleviate Oxidative Stress is Important for Salt Tolerance
Reactive oxygen species (ROS) mainly include superoxide anion (O2−), hydrogen peroxide (H2O2), hydroxyl radical (•OH), and singlet oxygen (1O2). Under normal conditions, there is a dynamic balance between the production and scavenging of ROS in plant cells. When plants are subjected to salt stress, apoplast and organelles such as chloroplasts and mitochondria accumulate excessive ROS, which in turn results in lipid peroxidation and damage of the structure of DNA and protein, causing oxidative stress (Mignolet-Spruyt et al., 2016; Wang et al., 2017; Jia et al., 2020). ROS scavenging in plants mainly involves the antioxidant enzyme system and the non-enzymatic system. The former one mainly includes superoxide dismutase (SOD), peroxidase (POD), catalase (CAT), ascorbate peroxidase (APX), and glutathione peroxidase (GPX), and the latter one mainly includes ascorbic acid, glutathione, vitamin E, carotenoid, and mannitol (Deinlein et al., 2014; Liang et al., 2017; Irshad et al., 2021; Li et al., 2021). SOD mainly catalyzes O2− to produce H2O2 and O2. This process was often regarded as the first defense for plants to scavenge excessive ROS (Zhu, 2002, 2016). POD, CAT, and APX mainly scavenge H2O2 accumulated in plants (Petridis et al., 2012; Hou et al., 2016; Liu et al., 2020). After being subjected to salt stress, the activity of antioxidant enzymes in salt tolerant plants is generally higher than the sensitive ones (Zhu, 2002, 2016; Wu et al., 2020). Overexpression of GhSOD1 and GhCAT significantly improves salt tolerance of cotton (Luo et al., 2000). Similarly, applying nanozymes with ROS scavenging ability in plants helped to improve plant salt tolerance via maintaining ROS homeostasis (Wu, 2018a; Wu et al., 2018c; Zhao et al., 2020). For example, nanozyme poly(acrylic) acid-coated cerium oxide nanoparticles (PNC) can improve salinity stress tolerance of cotton mainly by alleviating ROS accumulation in seedling roots (An et al., 2020). Similar results were also found in wheat (Mushtaq et al., 2017), tomato (Faizan et al., 2021), soybean (Farhangi-Abriz and Torabian, 2018), and rapeseed (Khan et al., 2021). Carbon quantum dots can significantly improve the activity of antioxidant enzyme system to reduce the content of ROS and to alleviate oxidative damage on cell membrane, thus enhancing grape salt tolerance (Gohari et al., 2021). Cucumber plants with foliar-delivered Mn3O4 nanoparticles showed less ROS accumulation and better salt tolerance than the control without nanoparticles (Lu et al., 2020). Spraying zinc oxide NMs on leaves can also significantly improve the protein content and antioxidant enzyme activity of POX, SOD, and CAT in tomato plants under salt stress (Faizan et al., 2021). It was found that compared with salt control, zinc oxide NMs upregulated the expression levels of SOD and GPX genes in tomato under salt stress (Alharby et al., 2016). Thus, improving the ability to maintain ROS homeostasis could be one of the important mechanisms under nano-enabled plant salt tolerance.
Nanomaterials might also improve plant salt tolerance via modulating the production of antioxidants. Glutathione, proline, and ascorbic acid are important soluble antioxidants in plant cells, playing an important role in maintaining ROS homeostasis in cells (Zhu, 2002). (1) Reduced glutathione (GSH) is a widely distributed antioxidant in cells. The dynamic balance between GSH and oxidized glutathione is also an important indicator of the antioxidant capacity of plant cells (Hameed et al., 2014). Indeed, exogenously applied GSH can improve salt tolerance in many plant species such as onion (Hamed and Al-Mutawa, 2009), tomato (Zhou et al., 2017), and mung bean (Nahar et al., 2015). To our surprise, to date, the role of GSH and how it works in nano-enabled plant salt tolerance is rarely investigated. CuNPs improved tomato salt tolerance via increasing the content of glutathione (Pérez-Labrada et al., 2019). Future studies are encouraged to investigate the role of GSH and its biosynthesis in nano-enabled plant salt tolerance. (2) Proline is known as one of the most important and effective organic osmotic regulatory substances, which plays an vital role in maintaining osmotic balance and cell membrane integrity (Siddiqui et al., 2015; Hu et al., 2021). Besides being effective osmotic regulatory substance, proline is also known as antioxidants to scavenge ROS (Siddiqui et al., 2015; Brahimova et al., 2021; Hu et al., 2021). Proline is often used as an important physiological indexes in plant salt tolerance (Siddiqui et al., 2015; Hu et al., 2021). There are some reports about the role of proline in nano-enabled salt tolerance. For example, nano-SiO2 can improve plant salt tolerance mainly by increasing proline content, photosynthetic rate, and water use efficiency of plant leaves (Siddiqui et al., 2015). Titanium dioxide NMs-treated plants not only induced the increase of antioxidant enzyme activity but also increased the content of proline and soluble sugar to improve osmotic balance in cells (Abdel-Latef et al., 2017). (3) Ascorbic acid plays an important role in plant growth and development (Zhu, 2002). After plants are subjected to abiotic stress, ascorbic acid can alleviate oxidative damage by maintaining ROS homeostasis and participating into the ASA-GSH cycle (Zhang et al., 2012). For example, exogenous nano-silicon can alleviate the damage of salt stress to soybean seedlings by increasing ascorbic acid content and enhancing antioxidant enzyme activity (Farhangi-Abriz and Torabian, 2018). Other studies showed that Cu NPs (Pérez-Labrada et al., 2019) and SiO2 nanoparticles (Pinedo-Guerrero et al., 2020) improved tomato salt tolerance via increasing ascorbic acid contents. Furthermore, it is well known that phenols and anthocyanins are also key members of non-enzymatic antioxidant system (Pinedo-Guerrero et al., 2020). ZnO NPs can increase the content of total phenols and anthocyanins in potatoes (Raigond et al., 2017). Adding copper nanoparticles (CuNPs) to chitosan–polyvinyl alcohol hydrogel (Cs-PVA) can also significantly increase the content of phenols, β-carotene, ascorbic acid, and lycopene in tomato, finally improving tomato salt tolerance (Hernández-Hernández et al., 2018a,b).
Besides modulating antioxidant enzymes and no enzymatic pathways, nanomaterials can also be used as delivery tool to deliver antioxidants to regulate plant salt tolerance. Chloroplast guiding peptide modified β-cyclodextrin conjugated quantum dots are able to do targeted delivery of methyl viologen and ascorbic acid to chloroplasts to regulate its redox status (Santana et al., 2020). Calcium-induced cross-linked pea protein nanoparticles can stably deliver the antioxidant resveratrol (Yf et al., 2020). The combination of resveratrol and α-tocopherol significantly improved the salt adaptability of citrus seedlings (Kostopoulou et al., 2014). Overall, use of nanomaterials to maintain ROS homeostasis through either direct ROS scavenging, or modulating antioxidant system, or delivery of antioxidants, could be an alternative way to improve plant salt tolerance.
The Importance of Maintaining Na+/K+ Homeostasis for Plant Salt Tolerance
Maintaining Na+/K+ homeostasis is a hallmark for plant salt tolerance (Alsaeedi et al., 2018; Zhang et al., 2020; Liu et al., 2021a). Under salinity stress, plants evolved fine mechanisms to avoid over-accumulation of Na+ and massive loss of K+ to maintain Na+/K+ homeostasis. Shoot Na+ exclusion, root Na+ extrusion, and vacuolar Na+ sequestration are the main strategies for plants to avoid over-accumulation of Na+ in cytosol and thus the resulted Na+ toxicity (Yue et al., 2012; Flowers and Colmer, 2015; Wu, 2018a; Wu et al., 2018b, 2019, 2021; Zelm et al., 2020; Shah et al., 2021). For example, Wu et al. reported that vacuolar Na+ sequestration in the mature root zone might be responsible for the stronger salt tolerance of bread wheat than durum wheat (Wu et al., 2018b). Interestingly, previous studies showed that in root, the vacuolar Na+ sequestration was more important than Na+ exclusion for salinity tolerance in barley (Flowers and Colmer, 2015). These results suggest that the employed mechanisms for salt tolerance might be differed at tissue level in plant or between different plant species. No doubt, avoiding Na+ over-accumulation is also an important mechanism for nano-enabled plant salt tolerance. It has been reported that nanomaterials such as PNC (poly acrylic acid-coated cerium oxide nanoparticles) can enhance shoot Na+ exclusion to improve salt tolerance of cotton (Liu et al., 2021b). Also, cerium oxide nanoparticles shorten the apoplast barrier of Brassica roots to transport more Na+ to shoot, thus reducing the accumulation of Na+ in plant roots to improve its salt tolerance (Rossi et al., 2017). Other studies showed that silica nanoparticles can improve germination and growth of cucumber by decreasing Na+ content and maintaining K+/Na+ ratio under salinity stress (Alsaeedi et al., 2018).
Potassium plays important role in plant cell activities, i.e., adjusting of cell osmotic potential and charge balance, acting as a cofactor of many enzymes such as malate dehydrogenase and pyruvate kinase, promoting sugar transport and water retention of cells, and controlling of stomatal movement (Osakabe et al., 2013). Plants’ ability to maintain root and mesophyll K+ is known as important mechanisms for plant salt tolerance (Chen et al., 2005; Wu et al., 2013, 2014, 2015, 2018d). Not surprisingly, improving the ability to maintain K+ in plants is also a mechanism involved in nano-enabled plant salt tolerance. For example, through scavenging of ROS, cerium oxide nanoparticles modulate ROS-activated NSCC channels (non-selective cation channels) to reduce K+ loss to improve salt tolerance in Arabidopsis (Wu et al., 2018c). Similar results were also found in cotton (Liu et al., 2021b) and rapeseed (Khan et al., 2021). The foliar-applied poly(acrylic) acid-coated cerium oxide nanoparticles can promote shoot K+ retention and Na+ exclusion but not vacuolar Na+ sequestration to maintain Na+/K+ ratio to improve cotton salt tolerance (Liu et al., 2021b). It showed that nanoceria modulated the relative expression level of HKT1 (upregulation) and KOR (downregulation) genes and showed no effects on the relative expression level of NHX1 gene. This is in accordance with the findings of subcellular distribution of Na+ and K+ dye signals between control plants and nanoceria-treated cotton plants under salinity (Liu et al., 2021b). Nanosilica (SiNPs) treatment can increase K+ content of cucumber seedlings under high salt stress, thus improving cucumber salt tolerance mainly by maintaining K+/Na+ ratio (Alsaeedi et al., 2019). In addition, MWCNTs were also found to increase the transcriptional abundance of Na+ and K+ transporters through NO (nitric oxide) participation to maintain K+/Na+ ratio to increase rapeseed salt tolerance (Zhao et al., 2019). Taken together, maintaining Na+/K+ homeostasis might be a commonly employed mechanism for nano-enabled plant salt tolerance.
The Role of Phytohormone in Plant Salt Tolerance
Plant hormones and plant growth regulators are essential for plant growth and development, especially in regulating plant response to stress (Hou et al., 2016; Zhu, 2016; Yu et al., 2020; Zhao et al., 2020). Abscisic acid (ABA), gibberellin (GA), brassinosteroids (BR), jasmonic acid (JA), and salicylic acid (SA) are common hormones that play a vital role in crop salt stress response (Zhu, 2002; Yu et al., 2020). However, its role in nano-enabled plant salt tolerance are not well explored. How phytohormones was involved in nano-enable plant salt tolerance and the possible effect of nanomaterials on plant growth-related processes under salinity stress are still largely unknown. Regarding the role of these hormones in plant resistance to abiotic stress such as salinity stress and plant growth-related processes, some good review papers are available (Huang et al., 2017; Wang et al., 2020; Yu et al., 2020).
Abscisic Acid
Abscisic acid (ABA) is known as a stress responsive hormone. Its content was rapidly increased when plants faced to abiotic stress such as saline-alkali stress, water stress, and temperature stress (Liang et al., 2017; Yu et al., 2020). Besides modulating water absorption and proline accumulation, ABA improves plant salinity resistance by inducing the expression of salt tolerance genes (Zhao et al., 2020; Wu et al., 2021). The increase of ABA content under salt stress also maintains the stability of DELLA protein (a class of protein with the N-terminal having highly conserved DELLA domain), which attenuates cellular activity by regulating gibberellin and finally alleviates the damage of salt stress to plants (Lei et al., 2020). Generally, the increase of ABA content in plants under salt stress is positively correlated with its stress tolerance (Danquah et al., 2014; Zhu, 2016). Exogenous application of ABA always can alleviate plant salt stress symptom (Wei et al., 2015; Lei et al., 2020). Previous study showed that Ag nanoparticle can increase ABA content to improve plant abiotic stress tolerance (Khan and Bano, 2016b). Another study showed that nanopriming can improve germination percentage and germination rate of two rapeseed cultivars under salt stress by increasing the antioxidant enzyme activity and abscisic acid content (El-Badri et al., 2021). Furthermore, researchers used mesoporous silica nanoparticles to deliver ABA to improve drought tolerance in Arabidopsis (Sun et al., 2018).
Gibberellins and Brassinosteroids
Gibberellins (GA) not only play an important role in promoting plant growth, inducing flowering and breaking dormancy, but also participate in plant response to abiotic stress (Daviere and Achard, 2013). Carbon nanotubes (e.g., SWCNTs) can promote the growth of seedlings by increasing the GA content in rice (Zhang et al., 2017). CeO2 NPs can enhance rice tolerance to N-deficiency by regulating antioxidant enzyme system and the levels of phytohormones including IAA, GA and ABA (Wang et al., 2020). Brassinoids (BR) is known as the sixth hormone in plants, having the role of promoting cell elongation and division and improving tolerance to salinity, drought and heat stresses (Zhu, 2002; Flowers and Colmer, 2015; Hou et al., 2019). BR can alleviate the inhibition of salt stress on rice seed germination and seedling growth (Anuradha and Rao, 2003). However, to date, the role of BR in nano-enabled plant salt tolerance is still obscure. How nanomaterials modulate BR biosynthesis and its signaling pathways to improve plant salt tolerance are worthy to be investigated in future studies.
Jasmonic Acid and Salicylic Acid
Jasmonic acid (JA) and salicylic acid (SA) are main plant growth regulators in response to stress (Bernstein, 2019). Jasmonic acid is known to improve plant stress tolerance (Parvaiz et al., 2016). For example, under salinity stress, the level of JA content is positively correlated with salt tolerance in wheat (Zhao et al., 2014). Overexpression of JA related gene TaAOC1 promoted the accumulation of JA in Arabidopsis leaf and improved plant salt tolerance (Zhao et al., 2014). TiO2 NPs have been shown to activate the JA pathway in wheat (Jiang et al., 2017). Silica nanoparticles can improve salt tolerance of rice by regulating jasmonic acid signal (Abdel-Haliem et al., 2017). In addition, some studies have shown that chitosan activates the octadecanoic acid pathway of JA and protects plants from salt stress by regulating cell ion concentration (Pichyangkura and Chadchawan, 2015). Adding Cu NPs to chitosan hydrogel can reduce the activation of JA gene expression under salt stress (Hernández-Hernández et al., 2018a,b).
Similar to JA, SA is a well-recognized hormone which can improve plant stress tolerance. Exogenous application of SA can significantly improve salt stress tolerance in crops such as rice (Jini and Joseph, 2017) and potato (Faried et al., 2017). SA induced salt stress tolerance is mainly associated with enhancing the activities of SOD and CAT and other antioxidant enzymes to alleviate over-accumulation of ROS, or promoting lateral root growth, or executing synergistic action with other hormones (Blumwald, 2000; Shaki et al., 2018; Abdoli et al., 2020; Yu et al., 2020). Addition of nano Fe2O3 during exogenous spraying of SA can significantly improve K+ content, Fe content, endogenous level of SA, and antioxidant enzyme activity in Trachyspermum AMMI L. and thus can improve its salt tolerance (Abdoli et al., 2020). More studies are encouraged to investigate the role of JA and SA in nano-enabled plant salt tolerance.
Together, it suggests that nano-enabled plant salt tolerance is associated with modulating phytohormones, although the employed mechanisms might be varied with nanomaterials or in plant species. Also, exploring nanomaterials as tool to do efficient and targeted delivery of phytohormones to modulate plant salt tolerance could be a direction for future studies. More efforts are encouraged to study the role of phytohormones in nano-enabled plant salt tolerance and to better use phytohormones as plant growth regulators via nano-biotechnology.
Molecular Mechanisms Underlying Plant Salt Tolerance
Abscisic acid (ABA) signal transduction pathway, protein kinase pathway, and salt overly sensitive (SOS) signal transduction pathway are the common and well-studied signal pathways of plants in response to salt stress (Zhu, 2002; Shaki et al., 2018). Here, the discussion will be focused more on these pathways.
ABA Signal Transduction Pathway
The ABA pathway can be classified as ABA-dependent and ABA-independent pathways. The expression level of ABA-dependent genes is associated with the content of ABA in vivo. The expression of ABA-independent genes is affected by external environmental factors such as saline-alkali, drought, and temperature (Xiong et al., 2001). ABA receptor proteins PYR/PYL/RCARs (pyracbactin resistance/pyracbactin resistance-like/regulatory component of ABA receptor), PP2C (protein phosphatases type 2Cs), and SnPK2s (Sucrose non-fermenting 1-related protein kinases subfamily 2) act as core proteins for ABA signaling pathway (Ben-Ari, 2012). Under salt stress, ABA receptor protein PYR/PYL/RCARs sense ABA signals and bind ABA to inhibit protein phosphatase PP2C, which in turn enhance protein kinase SnRK2s activity, thus conveying ABA signals to downstream targets (Raghavendra et al., 2010). Reversible phosphorylation of proteins is also an important step in ABA signaling pathway. Protein kinases such as CDPKs (Calcium-Dependent Protein Kinases) and SnPKs (Sucrose non-fermenting-1-related protein kinases) positively regulate the expression of downstream genes in the ABA signaling pathway. However, they are negatively regulated by protein phosphorylases ABI1 and ABI2 (Lim et al., 2012). The ABA signaling pathway also coordinated with various calcium signaling systems to respond to salt stress in plants. To date, less attention was paid to unveil the involvement of ABA signaling pathway in nano-enabled plant salt tolerance.
Protein Kinase Pathways
The protein kinase pathways mainly include mitogen-activated protein kinase (MAPK) cascade pathway and calcium-dependent protein kinases (CDPK) cascade pathway (Chen et al., 2021). Both of the protein kinases are serine/threonine protein kinases which are widely distributed in plants (Chen et al., 2021). The MAPK cascade signals are mainly transmitted to downstream proteins through phosphorylation and dephosphorization and finally activate the expression of relevant stress-resistant genes to respond to plant abiotic stress (Nakagami et al., 2005). MAPK pathway executes signal transduction through sequential phosphorylation of three serine/threonine phosphoprotein kinases: MAPKKKs, MAPKKs, and MAPKs (Danquah et al., 2014). Overexpression of OsMAPK5 and OsMAPK44 genes in rice significantly alleviate the negative effect of saline stress to plants (Jeong et al., 2006; Xie et al., 2012). Overexpression of ZmMKK1 and ZmMKK4 genes in maize can improve salinity tolerance of transgenic Arabidopsis thaliana, and overexpression of GhMPK2 and GhMAP3K40 genes improve cotton salt tolerance (Kong et al., 2011; Liang et al., 2011; Chen et al., 2015). Similarly, less attention was paid to the possible modulation of protein kinase activities in nano-enabled plant salt tolerance.
As a second messenger, calcium plays a central role in plant cell signal transduction. Ca2+ signal sensing proteins in plants mainly include calmodulin (CaM), calmodulin-like protein (CMLs), calmodulin B-like protein (CBL), and calcium-dependent protein kinase (CDPKs; Zhu, 2016; Cao et al., 2020). For example, CaM is the most widely distributed and important calcium-dependent protein kinase, and CDPKs are unique serine/threonine protein kinases in plants (Kim et al., 2013). These Ca2+ signal sensing proteins work together to form a large signal transduction regulatory network and transmit Ca2+ signals to downstream response elements (Batisti and Kudla, 2012). Ca2+ signaling pathways also play a vital role in nanoceria-induced response to salt stress (Brahimova et al., 2021).
SOS Signal Transduction Pathway
SOS signal transduction pathway is an important way for plants to maintain Na+ homeostasis under salt stress and mainly executed by three kinds of proteins: SOS1, SOS2, and SOS3 (Yue et al., 2012; Zhu, 2016). The general process of SOS signal transduction is: under salt stress, intracellular Ca2+ concentration increases rapidly, the SOS3 (the upstream Ca2+ binding protein) and SCaBP8/CBL10 (SOS3-LKE calcium-binding protein8/calcineurin B-like Protein10) sense Ca2+ signals and interact with SOS2 to form SOS3-SOS2 protein kinase complex to regulate SOS1 activity to exclude Na+. Studies have shown that SOS2 interacts with CAT2 and CAT3, and the SOS signal transduction pathway may also coordinate with ROS signal to participate in plant salt stress response (Verslues et al., 2007). Singh et al. reported that iron oxide nanoparticles can enhance salt tolerance of trees by increasing the expression levels of genes such as HKT1, SOS1, and NHX and the activity of antioxidant enzymes (Singh et al., 2021). Similarly, Liu et al. found that nanoceria treatment upregulated the relative expression level of HKT1 (shoot Na+ exclusion) but not SOS1 to improve cotton salt tolerance (Liu et al., 2021b). The mechanisms of nanomaterials on maintaining Na+ homeostasis in salt-stressed plants are different in nanomaterials and also in plant species.
Transcription Factors and Stress-Responsive Related Genes
Transcription factors such as WRKY (a class of protein with the N-terminal having highly conserved WRKYGQK domain), NAC [NAM (no apical meristem, Petunia), ATAF1-2 (Arabidopsis thaliana activating factor), and CUC2 (cup-shaped cotyledon, Arabidopsis)], bZIP (basic leucine zipper), and AP2/ERF (APETALA2/Ethylene Responsive Factor) play an important role in plant response to salt stress. WRKY is a plant-specific transcription factor and also responds to biotic and abiotic stresses. Overexpression of WRKY25 or WRKY33 genes can improve salt tolerance of Arabidopsis thaliana, and the double mutant plants of wrky25 and wrky33 are more sensitive to salt stress (Ding et al., 2014). Plant-specific NAC transcription factors are also play a role in abiotic stress response (Ding et al., 2014). Overexpression of OsNAC2, OsNAC6, and OsNAC045 genes in rice significantly improved salt and drought tolerance (Ohnishi et al., 2005; Hu et al., 2010; Xiang et al., 2020). However, AtNAC2 overexpressed plants are more sensitive to salt stress, indicating the complexity of NAC transcription factors in response to plant abiotic stress (Balazadeh et al., 2010). Basic leucine zipper (bZIP) protein was widely involved in plant stress response. bZIP transcription factors participate in ABA signal transduction pathway and regulate the expression of related abiotic stress responsive genes. Overexpression of AtbZIP1 improved salt tolerance in Arabidopsis (Sun et al., 2012). Furthermore, AtERF98 gene can improve the tolerance of Arabidopsis to salt stress by promoting the synthesis of ascorbic acid (Zhang et al., 2012). Plants with GmERF3 gene overexpression showed better salt and drought tolerance, and significantly higher contents of proline and soluble sugar than control plants (Zhang et al., 2009).
Moreover, the expression of genes involved in osmotic regulation, ion balance, antioxidant, and hormone regulation will also change after plants are subjected to abiotic stress such as saline-alkali stress (Cao et al., 2020; Zhang et al., 2020). For example, the expression level of HKT1 (high-affinity K+ transporter for Na+ exclusion) gene was significantly increased in PNC treated cotton seedlings compared with the non-PNC treated seedlings under salt stress (Liu et al., 2021b). NHX1 (Na+/H+ exchanger 1), a Na+/H+ antiporter gene, plays an important role in Na+ compartmentalization. Overexpression of AtNHX1 gene showed improved salt tolerance in wheat (Xue et al., 2004), cotton (He et al., 2005), and soybean (Li et al., 2010). DELLA protein is a vital negative regulator of the gibberellin signaling pathway and also plays an important role in other hormone signaling and environmental signaling systems (Zhu, 2002). The higher content of DELLA protein in Arabidopsis, the stronger its salt tolerance (Zhu, 2002). Halophytes can promote the accumulation of DELLA protein by inhibiting GA signal transduction to prolong the static growth period of plants to improve their salt tolerance (Murase et al., 2008). DELLA protein can also improve the activity of SOD and CAT in Arabidopsis and wheat under salt stress and enhance the ability of scavenging ROS in plants, suggesting that the overexpression of the DELLA gene can significantly improve plant salt tolerance (Murase et al., 2008; Dobrikova et al., 2017).
Conclusion and Perspectives
Nowadays, nanomaterials showed potential in improving plant salt tolerance. However, the relevant mechanisms need to be further explored. Also, to date, nano-enabled plant salt tolerance is still largely demonstrated at the laboratory research stage. To facilitate the adoption of nano-enabled plant salt tolerance in agricultural production, discussions and setup of widely accepted policies and regulations are urgently called on task. Also, more studies should be conducted to explore the possible effect of nanomaterials on plants under salt stress from the viewpoint of source-sink regulation. For example, if nanomaterials are foliar-sprayed to plants, its effects on sink capacity should be studied. Studies have shown that Zn chitosan nanomaterials significantly increased the accumulation of starch biosynthetic enzymes in wheat grains and thus the yield (by 21%) compared with the control group treated with ZnSO4, which further verified that the wheat treated with nanomaterials on the leaf had better sink strength (Kumar et al., 2021). Overall, we believe that nanotechnology can play an important role in sustainable development of agriculture.
Author Contributions
HW and ZZ conceived this review paper. ZL and LZ summarized and analyzed literatures regarding mechanisms underlying plant salinity stress tolerance and nano-enabled plant salt tolerance. FZ and JL contributed to make the tables and figures. XZ and XK contributed to the discussion of manuscript. HW, ZZ, ZL, and LZ wrote the manuscript. All authors contributed to the article and approved the submitted version.
Funding
This work was supported by National Natural Science Foundation of China (32071971, 31901464), joint project SZYJY2021008 from Huazhong Agricultural University and Agricultural Genomics Institute at Shenzhen, Chinese Academy of Agricultural Sciences, and project 2662020ZKPY001 supported by the Fundamental Research Funds for the Central Universities to HW and the Key Science and Technology Special Project of Xinxiang City of China (ZD2020004), Leading Talent Project in Science and Technology Innovation of Central Plain of China (214200510021), and the Program for Innovative Research Team (in Science and Technology) in the University of Henan Province (21IRTSTHN023) to ZZ.
Conflict of Interest
The authors declare that the research was conducted in the absence of any commercial or financial relationships that could be construed as a potential conflict of interest.
Publisher’s Note
All claims expressed in this article are solely those of the authors and do not necessarily represent those of their affiliated organizations, or those of the publisher, the editors and the reviewers. Any product that may be evaluated in this article, or claim that may be made by its manufacturer, is not guaranteed or endorsed by the publisher.
References
Abdel-Haliem, M. E. F., Hegazy, H. S., Hassan, N. S., and Naguib, D. M. (2017). Effect of silica ions and nano silica on rice plants under salinity stress. Ecol. Eng. 99, 282–289. doi: 10.1016/j.ecoleng.2016.11.060
Abdel-Latef, A. A. H., Srivastava, A. K., El-Sadek, M. S. A., Kordrostami, M., and Tran, L. S. P. (2017). Titanium dioxide nanoparticles improve growth and enhance tolerance of broad bean plants under saline soil conditions. Land Degrad. Dev. 29, 1065–1073. doi: 10.1002/ldr.2780
Abdoli, S., Ghassemi-Golezani, K., and Alizadeh-Salteh, S. (2020). Responses of ajowan (Trachyspermum ammi L.) to exogenous salicylic acid and iron oxide nanoparticles under salt stress. Environ. Sci. Pollut. Res. 27, 36939–36953. doi: 10.1007/s11356-020-09453-1
Alharby, H. F., Metwali, E. M. R., Fuller, M. P., and Aldhbiani, A. Y. (2016). The alteration of mRNA expression of SOD and GPX genes, and proteins in tomato (Lycopersicon esculentum mill) under stress of NaCl and/or ZnO nanoparticles. Saudi J. Biol. Sci. 23, 773–781. doi: 10.1016/j.sjbs.2016.04.012
Alsaeedi, A., El-Ramady, H., Alshaal, T., El-Garawani, M., Elhawat, N., and Al-Otaibi, A. (2018). Exogenous nanosilica improves germination and growth of cucumber by maintaining K+/Na+ ratio under elevated Na+ stress. Plant Physiol. Biochem. 125, 164–171. doi: 10.1016/j.plaphy.2018.02.006
Alsaeedi, A., El-Ramady, H., Alshaal, T., El-Garawany, M., Elhawat, N., and Al-Otaibi, A. (2019). Silica nanoparticles boost growth and productivity of cucumber under water deficit and salinity stresses by balancing nutrients uptake. Plant Physiol. Biochem. 139, 1–10. doi: 10.1016/j.plaphy.2019.03.008
An, J., Hu, P., Li, F., Wu, H., Shen, Y., White, J. C., et al. (2020). Emerging investigator series: molecular mechanisms of plant salinity stress tolerance improvement by seed priming with cerium oxide nanoparticles. Environ. Sci. Nano 7, 2214–2228. doi: 10.1039/D0EN00387E
Anuradha, S., and Rao, S. (2003). Application of brassinosteroids to rice seeds (Oryza sativa L.) reduced the impact of salt stress on growth, prevented photosynthetic pigment loss and increased nitrate reductase activity. Plant Growth Regul. 40, 29–32. doi: 10.1023/A:1023080720374
Avestan, S., Ghasemnezhad, M., Esfahani, M., and Byrt, C. S. (2019). Application of nano-silicon dioxide improves salt stress tolerance in strawberry plants. Agronomy 9:246. doi: 10.3390/agronomy9050246
Balal, R. M., Ashraf, M. Y., Khan, M. M., Jaskani, M. J., and Ashfaq, M. (2011). Influence of salt stress on growth and biochememical parameters of citrus rootstocks. Pak. J. Bot. 43, 2135–2141. doi: 10.1186/1746-4811-7-25
Balazadeh, S., Siddiqui, H., Ad, A., Lp, M. R., Caldana, C., Mehrnia, M., et al. (2010). A gene regulatory network controlled by the NAC transcription factor ANAC092/AtNAC2/ORE1 during salt-promoted senescence. Plant J. 62, 250–264. doi: 10.1111/j.1365-313X.2010.04151.x
Batisti, O., and Kudla, J. (2012). Analysis of calcium signaling pathways in plants. Biochim. Biophys. Acta 1820, 1283–1293. doi: 10.1016/j.bbagen.2011.10.012
Ben-Ari, G. (2012). The ABA signal transduction mechanism in commercial crops: learning from Arabidopsis. Plant Cell Rep. 31, 1357–1369. doi: 10.1007/s00299-012-1292-2
Bernstein, N. (2019). Plants and salt: plant response and adaptations to salinity. Model Eco. Extreme Environ. 101–112. doi: 10.1016/B978-0-12-812742-1.00005-2
Blumwald, E. (2000). Sodium transport and salt tolerance in plants. Curr. Opin. Cell Biol. 12, 431–434. doi: 10.1016/S0955-0674(00)00112-5
Brahimova, U., Kumari, P., Yadav, S., Rastogi, A., and Brestic, M. (2021). Progress in understanding salt stress response in plants using biotechnological tools. J. Biotechnol. 329, 180–191. doi: 10.1016/j.jbiotec.2021.02.007
Bui, E. N. (2013). Soil salinity: A neglected factor in plant ecology and biogeography. J. Arid Environ. 92, 14–25. doi: 10.1016/j.jaridenv.2012.12.014
Cao, Y., Zhang, M., Liang, X., Li, F., and Jiang, C. (2020). Natural variation of an EF-hand Ca2+-binding-protein coding gene confers saline-alkaline tolerance in maize. Nat. Commun. 11:186. doi: 10.1038/s41467-019-14027-y
Chen, Z., Newman, I., Zhou, M., Mendham, N., Zhang, G., and Shabala, S. (2005). Screening plants for salt tolerance by measuring K+ flux: a case study for barley. Plant Cell Environ. 28, 1230–1246. doi: 10.1111/j.1365-3040.2005.01364.x
Chen, M., Ni, L., Chen, J., Sun, M., and Jiang, M. (2021). Rice calcium/calmodulin-dependent protein kinase directly phosphorylates a mitogen-activated protein kinase kinase to regulate abscisic acid responses. Plant Cell 33, 1790–1812. doi: 10.1093/plcell/koab071
Chen, X., Wang, J., Zhu, M., Jia, H., Liu, D., Hao, L., et al. (2015). A cotton Raf-like MAP3K gene, GhMAP3K40, mediates reduced tolerance to biotic and abiotic stress in Nicotiana benthamiana by negatively regulating growth and development. Plant Sci. 240, 10–24. doi: 10.1016/j.plantsci.2015.08.012
Danquah, A., Zelicourt, A. D., Colcombet, J., and Hirt, H. (2014). The role of ABA and MAPK signaling pathways in plant abiotic stress responses. Biotechnol. Adv. 32, 40–52. doi: 10.1016/j.biotechadv.2013.09.006
Daviere, J. M., and Achard, P. (2013). Gibberellin signaling in plants. Development 140, 1147–1151. doi: 10.1242/dev.087650
Deinlein, U., Stephan, A. B., Horie, T., Luo, W., and Schroeder, J. I. (2014). Plant salt-tolerance mechanisms. Trends Plant Sci. 19, 371–379. doi: 10.1016/j.tplants.2014.02.001
Ding, Z. J., Yan, J. Y., Xu, X. Y., Yu, D. Q., Li, G. X., Zhang, S. Q., et al. (2014). Transcription factor WRKY46 regulates osmotic stress responses and stomatal movement independently in Arabidopsis. Plant J. 79, 13–27. doi: 10.1111/tpj.12538
Dobrikova, A. G., Yotsova, E. K., Börner, A., Landjeva, S. P., and Apostolova, E. L. (2017). The wheat mutant DELLA-encoding gene (Rht-B1c) affects plant photosynthetic responses to cadmium stress. Plant Physiol. Biochem. 114, 10–18. doi: 10.1016/j.plaphy.2017.02.015
El-Badri, A. M., Batool, M., Wang, C., Hashem, A. M., Tabl, K. M., Nishawy, E., et al. (2021). Selenium and zinc oxide nanoparticles modulate the molecular and morpho-physiological processes during seed germination of Brassica napus under salt stress. Ecotoxicol. Environ. Saf. 225:112695. doi: 10.1016/j.ecoenv.2021.112695
Faizan, M., Bhat, J. A., Chen, C., Alyemeni, M. N., and Yu, F. (2021). Zinc oxide nanoparticles (ZnO-NPs) induce salt tolerance by improving the antioxidant system and photosynthetic machinery in tomato. Plant Physiol. Biochem. 161, 122–130. doi: 10.1016/j.plaphy.2021.02.002
Farhangi-Abriz, S., and Torabian, S. (2018). Nano-silicon alters antioxidant activities of soybean seedlings under salt toxicity. Protoplasma 255, 953–962. doi: 10.1007/s00709-017-1202-0
Faried, H. N., Ayyub, C. M., Amjad, M., Ahmed, R., Wattoo, F. M., Butt, M., et al. (2017). Salicylic acid confers salt tolerance in potato plants by improving water relations, gaseous exchange, antioxidant activities and osmoregulation. J. Sci. Food Agric. 97, 1868–1875. doi: 10.1002/jsfa.7989
Farokhzad, O. C., and Langer, R. (2009). Impact of nanotechnology on drug delivery. ACS Nano 3, 16–20. doi: 10.1021/nn900002m
Farooq, M., Hussain, M., Wahid, A., and Siddique, K. H. (2012). “Drought stress in plants: an overview,” in Plant Responses to Drought Stress. ed. R. Aroca (Berlin, Heidelberg: Springer), 1–33.
Fita, A., Rodriguez-Burruezo, A., Boscaiu, M., Prohens, J., and Vicente, O. (2015). Breeding and domesticating crops adapted to drought and salllinity: a new paradigm for increasing food production. Front. Plant Sci. 6:978. doi: 10.3389/fpls.2015.00978
Flowers, T. J., and Colmer, T. D. (2015). Plant salt tolerance: adaptations in halophytes. Ann. Bot. 115, 327–331. doi: 10.1093/aob/mcu267
Ganjavi, A. S., Oraei, M., Gohari, G., Akbari, A., and Faramarzi, A. (2021). Glycine betaine functionalized graphene oxide as a new engineering nanoparticle lessens salt stress impacts in sweet basil (Ocimum basilicum L.). Plant Physiol. Biochem. 162, 14–26. doi: 10.1016/j.plaphy.2021.02.028
Gao, L., Zhuang, J., Nie, L., Zhang, J., Zhang, Y., Gu, N., et al. (2007). Intrinsic peroxidase-like activity of ferromagnetic nanoparticles. Nat. Nanotechnol. 2, 577–583. doi: 10.1038/nnano.2007.260
Giraldo, J. P., Wu, H., Newkirk, G. M., and Kruss, S. (2019). Nanobiotechnology approaches for engineering smart plant sensors. Nat. Nanotechnol. 14, 541–553. doi: 10.1038/s41565-019-0470-6
Gohari, G., Panahirad, S., Sadeghi, M., Akbari, A., Zareei, E., Zahedi, S. M., et al. (2021). Putrescine-functionalized carbon quantum dot (put-CQD) nanoparticles effectively prime grapevine (Vitis vinifera cv. 'Sultana') against salt stress. BMC Plant Biol. 21:120. doi: 10.1186/s12870-021-02901-1
Hamed, K., and Al-Mutawa, A. M. M. (2009). Glutathione-triggered mitigation in salt-induced alterations in plasmalemma of onion epidermal cells. Int. J. Agric. Biol. 11, 639–642. doi: 10.1039/C9TB01400D
Hameed, A., Sharma, I., Kumar, A., Azooz, M. M., Lone, H. A., and Ahmad, P. (2014). Glutathione metabolism in plants under environmental stress. Oxi. Damage Plants 183–200. doi: 10.1016/B978-0-12-799963-0.00006-X
He, C., Yan, J., Shen, G., Fu, L., Holaday, A. S., Auld, D., et al. (2005). Expression of an Arabidopsis vacuolar sodium/proton antiporter gene in cotton improves photosynthetic performance under salt conditions and increases fiber yield in the field. Plant Cell Physiol. 46, 1848–1854. doi: 10.1093/pcp/pci201
Hernández-Hernández, H., González-Morales, S., Benavides-Mendoza, A., Ortega-Ortiz, H., Cadenas-Pliego, G., and Juárez-Maldonado, A. (2018a). Effects of chitosan–PVA and cu nanoparticles on the growth and antioxidant capacity of tomato under saline stress. Molecules 23:178. doi: 10.3390/molecules23010178
Hernández-Hernández, H., Juárez-Maldonado, A., Benavides-Mendoza, A., Ortega-Ortiz, H., Cadenas-Pliego, G., and Sánchez-Aspeytia, D. (2018b). Chitosan-PVA and copper nanoparticles improve growth and overexpress the SOD and JA genes in tomato plants under salt stress. Agronomy 8:175. doi: 10.3390/agronomy8090175
Hou, Q., Ufer, G., and Bartels, D. (2016). Lipid signalling in plant responses to abiotic stress. Plant Cell Environ. 39, 1029–1048. doi: 10.1111/pce.12666
Hou, L., Zhang, A., Wang, R., Zhao, P., Zhang, D., Jiang, Y., et al. (2019). Brassinosteroid regulates root development with highly redundant genes in hexaploid wheat. Plant Cell Physiol. 60, 1761–1777. doi: 10.1093/pcp/pcz088
Hu, D., Lv, G., Qie, Y., Wang, H., Yang, F., and Jiang, L. (2021). Response of morphological characters and photosynthetic characteristics of Haloxylon ammodendron to water and salt stress. Sustainability 13:388. doi: 10.3390/su13010388
Hu, H., You, J., Fang, Y., Zhu, X., Qi, Z., and Xiong, L. (2010). Characterization of transcription factor gene SNAC2 conferring cold and salt tolerance in rice. Plant Mol. Biol. 67, 169–181. doi: 10.1007/s11103-008-9309-5
Huang, H., Liu, B., Liu, L., and Song, S. (2017). Jasmonate action in plant growth and development. J. Exp. Bot. 68, 1349–1359. doi: 10.1093/jxb/erw495
Hussein, M. M., and Abou-Baker, N. H. (2018). The contribution of nano-zinc to alleviate salinity stress on cotton plants. R. Soc. Open Sci. 5:171809. doi: 10.1098/rsos.171809
Irshad, A., Rehman, R. N. U., Abrar, M. M., Saeed, Q., Sharif, R., and Hu, T. (2021). Contribution of rhizobium–legume symbiosis in salt stress tolerance in Medicago truncatula evaluated through photosynthesis, antioxidant enzymes, and compatible solutes accumulation. Sustainability 13:3369. doi: 10.3390/su13063369
Jeong, M. J., Lee, S. K., Kim, B. G., Kwon, T. R., Cho, W. S., Park, Y. T., et al. (2006). A rice (Oryza sativa L.) MAP kinase gene, OsMAPK44, is involved in response to abiotic stresses. Plant Cell Tissue Organ Cult. 85, 151–160. doi: 10.1007/s11240-005-9064-0
Jia, J., Bai, J., Wang, W., Yin, S., Zhang, G., Zhao, Q., et al. (2020). Salt stress alters the short-term responses of nitrous oxide emissions to the nitrogen addition in salt-affected coastal soils. Sci. Total Environ. 742:140124. doi: 10.1016/j.scitotenv.2020.140124
Jiang, F., Shen, Y., Ma, C., Zhang, X., and Rui, Y. (2017). Effects of TiO2 nanoparticles on wheat (Triticum aestivum L.) seedlings cultivated under super-elevated and normal CO2 conditions. PLoS One 12:e0178088. doi: 10.1371/journal.pone.0178088
Jini, D., and Joseph, B. (2017). Physiological mechanism of salicylic acid for alleviation of salt stress in rice. Rice Sci. 24, 97–108. doi: 10.1016/j.rsci.2016.07.007
Kah, M., Tufenkji, N., and White, J. C. (2019). Nano-enabled strategies to enhance crop nutrition and protection. Nat. Nanotechnol. 14, 532–540. doi: 10.1038/s41565-019-0439-5
Khan, N., and Bano, A. (2016b). Role of plant growth promoting rhizobacteria and Ag-nano particle in the bioremediation of heavy metals and maize growth under municipal wastewater irrigation. Int. J. Phytoremediation 18, 211–221. doi: 10.1080/15226514.2015.1064352
Khan, M. N., Li, Y., Khan, Z., Chen, L., Liu, J., Hu, J., et al. (2021). Nanoceria seed priming enhanced salt tolerance in rapeseed through modulating ROS homeostasis and α-amylase activities. J. Nanobiotechnology 19:276. doi: 10.1186/s12951-021-01026-9
Khan, M. N., Mobin, M., Abbas, Z. K., Almutairi, K. A., and Siddiqui, Z. H. (2016a). Role of nanomaterials in plants under challenging environments. Plant Physiol. Biochem. 110, 194–209. doi: 10.1016/j.plaphy.2016.05.038
Kim, W. Y., Ali, Z., Park, H. J., Park, S. J., Cha, J. Y., Perez-Hormaeche, J., et al. (2013). Release of SOS2 kinase from sequestration with GIGANTEA determines salt tolerance in Arabidopsis. Nat. Commun. 4:1352. doi: 10.1038/ncomms2357
Kong, X., Pan, J., Zhang, M., Xin, X., Yan, Z., Yang, L., et al. (2011). ZmMKK4, a novel group C MAPK kinase in maize (Zea mays), confers salt and cold tolerance in transgenic Arabidopsis. Plant Cell Environ. 34, 1291–1303. doi: 10.1111/j.1365-3040.2011.02329.x
Kostopoulou, Z., Therios, I., and Molassiotis, A. (2014). Resveratrol and its combination with α-tocopherol mediate salt adaptation in citrus seedlings. Plant Physiol. Biochem. 78, 1–9. doi: 10.1016/j.plaphy.2014.02.011
Kumar, A., Prajapati, D., Devi, K. A., Pal, A., Choudhary, U., Dashora, A., et al. (2021). Slow-release Zn application through Zn-chitosan nanoparticles in wheat to intensify source activity and sink strength. Plant Physiol. Biochem. 168, 272–281. doi: 10.1016/j.plaphy.2021.10.013
Lei, L., Zheng, H., Bi, Y., Yang, L., and Zou, D. (2020). Identification of a major QTL and candidate gene analysis of salt tolerance at the bud burst stage in rice (Oryza sativa L.) using QTL-Seq and RNA-Seq. Rice 13:55. doi: 10.1186/s12284-020-00416-1
Li, Z., Hu, Y., Chang, M., Kashif, M. H., Tang, M., Luo, D., et al. (2021). 5-azacytidine pre-treatment alters DNA methylation levels and induces genes responsive to salt stress in kenaf (Hibiscus cannabinus L.). Chemosphere 271:129562. doi: 10.1016/j.chemosphere.2021.129562
Li, Y., Liu, J., Fu, C., Khan, M. N., Hu, J., Zhao, F., et al. (2022). CeO2 Nanoparticles Modulate Cu-Zn Superoxide dismutase and lipoxygenase-IV isozyme activities to alleviate membrane oxidative damage to improve rapeseed salt tolerance. Environ. Sci. Nano. doi: 10.1039/d1en00845e
Li, T. X., Zhang, Y., Liu, H., Wu, Y. T., Li, W. B., and Zhang, H. X. (2010). Stable expression of Arabidopsis vacuolar Na+/H+ antiporter gene AtNHX1, and salt tolerance in transgenic soybean for over six generations. Chin. Sci. Bull. 55, 1127–1134. doi: 10.1007/s11434-010-0092-8
Liang, W., Ma, X., Wan, P., and Liu, L. (2017). Plant salt-tolerance mechanism: A review. Biochem. Biophys. Res. Commun. 495, 286–291. doi: 10.1016/j.bbrc.2017.11.043
Liang, Z., Xi, D., Li, S., Gao, Z., Zhao, S., Jing, S., et al. (2011). A cotton group C MAP kinase gene, GhMPK2, positively regulates salt and drought tolerance in tobacco. Plant Mol. Biol. 77, 17–31. doi: 10.1007/s11103-011-9788-7
Lim, C. W., Baek, W., Lim, S., and Lee, S. C. (2012). ABA signal transduction from ABA receptors to ion channels. Genes Genom. 34, 345–353. doi: 10.1007/s13258-012-0081-1
Liu, J., Fu, C., Li, G., Khan, M. N., and Wu, H. (2021a). ROS homeostasis and plant salt tolerance: plant nanobiotechnology updates. Sustainability 13:3552. doi: 10.3390/su13063552
Liu, J., Li, G., Chen, L., Gu, J., and Li, Z. (2021b). Cerium oxide nanoparticles improve cotton salt tolerance by enabling better ability to maintain cytosolic K+/Na+ ratio. J. Nanobiotechnology 19:153. doi: 10.1186/s12951-021-00892-7
Liu, J., Shen, F., Xiao, Y., Fang, H., and Han, Z. (2020). Genomics-assisted prediction of salt and alkali tolerances and functional marker development in apple rootstocks. BMC Genomics 21:550. doi: 10.21203/rs.3.rs-17008/v3
Lu, L., Huang, M., Huang, Y., Corvini, P. F. X., Ji, R., and Zhao, L. (2020). Mn3O4 nanozymes boost endogenous antioxidant metabolites in cucumber (Cucumis sativus) plant and enhance resistance to salinity stress. Environ. Sci. Nano 7, 1692–1703. doi: 10.1039/D0EN00214C
Luo, M., Bilodeau, P., Dennis, E. S., Peacock, W. J., and Chaudhury, A. (2000). Expression and parent-of-origin effects for FIS2, MEA, and FIE in the endosperm and embryo of developing Arabidopsis seeds. Proc. Natl. Acad. Sci. U. S. A. 97, 10637–10642. doi: 10.1073/pnas.170292997
Michael, N. G., Wu, H., Israel, S., and Pablo, G. J. (2018). Catalytic scavenging of plant reactive oxygen species in vivo by anionic cerium oxide nanoparticles. J. Vis. Exp. 138:58373. doi: 10.3791/58373
Mignolet-Spruyt, L., Xu, E., Idnheimo, N., Hoeberichts, F. A., Mühlenbock, P., Brosché, M., et al. (2016). Spreading the news: subcellular and organellar reactive oxygen species production and signalling. J. Exp. Bot. 67, 3831–3844. doi: 10.1093/jxb/erw080
Morton, M. J. L., Awlia, M., Al-Tamimi, N., Saade, S., Pailles, Y., Negrão, S., et al. (2018). Salt stress under the scalpel – dissecting the genetics of salt tolerance. Plant J. 97, 148–163. doi: 10.1111/tpj.14189
Murase, K., Hlirano, Y., Sun, T. P., and Hakoshima, T. (2008). Gibberellin-induced DELLA recognition by the gibberellin receptor GID1. Nature 456, 459–463. doi: 10.1038/nature07519
Mushtaq, A., Jamil, N., Riaz, M., Hornyak, G. L., Ahmed, N., Ahmed, S. S., et al. (2017). Synthesis of silica nanoparticles and their effect on priming of wheat (Triticum aestivum L.) under salinity stress. Int. J. Biol. Macromol. 9, 150–157.
Nahar, K., Hasanuzzaman, M., Alam, M. M., and Fujita, M. (2015). Roles of exogenous glutathione in antioxidant defense system and methylglyoxal detoxification during salt stress in mung bean. Biol. Plant. 59, 745–756. doi: 10.1007/s10535-015-0542-x
Nakagami, H., Pitzschke, A., and Hirt, H. (2005). Emerging MAP kinase pathways in plant stress signalling. Trends Plant Sci. 10, 339–346. doi: 10.1016/j.tplants.2005.05.009
Negrão, S., Schmöckel, S. M., and Tester, M. (2016). Evaluating physiological responses of plants to salinity stress. Ann. Bot. 119, 1–11. doi: 10.1093/aob/mcw191
Ohnishi, T., Sugahara, S., Yamada, T., Kikuchi, K., Yoshiba, Y., Hirano, H. Y., et al. (2005). OsNAC6, a member of the NAC gene family, is induced by various stresses in rice. Genes Genetic Syst. 80, 135–139. doi: 10.1266/ggs.80.135
Osakabe, Y., Arinaga, N., Umezawa, T., Katsura, S., Nagamachi, K., Tanaka, H., et al. (2013). Osmotic stress responses and plant growth controlled by potassium transporters in Arabidopsis. Plant Cell 25, 609–624. doi: 10.1105/tpc.112.105700
Parihar, P., Singh, S., Singh, R., Singh, V. P., and Prasad, S. M. (2015). Effect of salinity stress on plants and its tolerance strategies: a review. Environ. Sci. Pollut. Res. Int. 22, 4056–4075. doi: 10.1007/s11356-014-3739-1
Parvaiz, A., Saiema, R., Alvina, G., Sheikh, S. A., Akram, N. A., Muhammad, A., et al. (2016). Jasmonates: multifunctional roles in stress tolerance. Front. Plant Sci. 7:813. doi: 10.3389/fpls.2016.00813
Pérez-Labrada, F., López-Vargas, E. R., Ortega-Ortiz, H., Cadenas-Pliego, G., and Juárez-Maldonado, A. (2019). Responses of tomato plants under saline stress to foliar application of copper nanoparticles. Plan. Theory 8:151. doi: 10.3390/plants8060151
Petridis, A., Therios, I., Samouris, G., and Tananaki, C. (2012). Salinity-induced changes in phenolic compounds in leaves and roots of four olive cultivars (Olea europaea L.) and their relationship to antioxidant activity. Environ. Exp. Bot. 79, 37–43. doi: 10.1016/j.envexpbot.2012.01.007
Pichyangkura, R., and Chadchawan, S. (2015). Biostimulant activity of chitosan in horticulture. Sci. Hortic. 196, 49–65. doi: 10.1016/j.scienta.2015.09.031
Pinedo-Guerrero, Z. H., Cadenas-Pliego, G., Ortega-Ortiz, H., Gonzalez-Morales, S., Benavideds-Mendoza, A., Valdes-Reyna, J., et al. (2020). Form of silica improves yield, fruit quality and antioxidant defense system of tomato plants under salt stress. Agriculture 10, 1–21. doi: 10.3390/agriculture10090367
Qian, D., Xiong, S., Li, M., Tian, L., and Qu, L. Q. (2021). OsFes1C, a potential nucleotide exchange factor for OsBiP1, is involved in the ER and salt stress responses. Plant Physiol. 187, 396–408. doi: 10.1093/plphys/kiab263
Raghavendra, A. S., Gonugunta, V. K., Christmann, A., and Grill, E. (2010). ABA perception and signalling. Trends Plant Sci. 15, 395–401. doi: 10.1016/j.tplants.2010.04.006
Raigond, P., Raigond, B., Kaundal, B., Singh, B., Joshi, A., and Dutt, S. (2017). Effect of zinc nanoparticles on antioxidative system of potato plants. J. Environ. Sci. 38, 435–439. doi: 10.22438/jeb/38/3/MS-209
Rico, C. M., Peralta-Videa, J. R., and Gardea-Torresdey, J. L. (2015). “Chemistry, biochememistry of nanoparticles, and their role in antioxidant defense system in plants,” in Nanotechnology and Plant Sciences. eds. M. Siddiqui, M. Al-Whaibi, and F. Mohammad (Cham: Springer), 1–17.
Rossi, L., Zhang, W., and Ma, X. (2017). Cerium oxide nanoparticles alter the salt stress tolerance of Brassica napus L. by modifying the formation of root apoplastic barriers. Environ. Pollut. 229, 132–138. doi: 10.1016/j.envpol.2017.05.083
Santana, I., Wu, H., Hu, P., and Giraldo, J. P. (2020). Targeted delivery of nanomaterials with chemical cargoes in plants enabled by a biorecognition motif. Nat. Commun. 11:2045. doi: 10.1038/s41467-020-15731-w
Shah, A. N., Tanveer, M., Abbas, A., Fahad, S., and Song, Y. (2021). Targeting salt stress coping mechanisms for stress tolerance in brassica: A research perspective. Plant Physiol. Biochem. 158, 53–64. doi: 10.1016/j.plaphy.2020.11.044
Shaki, F., Mabou, D. H. E., and Niknam, V. (2018). Growth enhancement and salt tolerance of safflower (Carthamus tinctorius L.) by salicylic acid. Curr. Opin. Plant Biol. 13, 16–22. doi: 10.1016/j.cpb.2018.04.001
Siddiqui, M. H., Al-Whaibi, M. H., Faisal, M., and Sahli, A. (2015). Nano-silicon dioxide mitigates the adverse effects of salt stress on Cucurbita pepo L. Environ. Toxicol. Chem. 33, 2429–2437. doi: 10.1002/etc.2697
Singh, D., Sillu, D., Kumar, A., and Agnihotri, S. (2021). Dual nanozyme characteristics of iron oxide nanoparticles alleviate salinity stress and promote the growth of an agroforestry tree. Eucalyptus tereticornis Sm. Environ. Sci. Nano 8, 1308–1325. doi: 10.1039/d1en00040c
Sun, D., Hussain, H. I., Yi, Z., Rookes, J. E., Kong, L., and Cahill, D. M. (2018). Delivery of abscisic acid to plants using glutathione responsive mesoporous silica nanoparticles. J. Nanosci. Nanotechnol. 18, 1615–1625. doi: 10.1166/jnn.2018.14262
Sun, X., Yong, L., Hua, C., Xi, B., Wei, J., Ding, X., et al. (2012). The Arabidopsis AtbZIP1 transcription factor is a positive regulator of plant tolerance to salt, osmotic and drought stresses. J. Plant Res. 125, 429–438. doi: 10.1007/s10265-011-0448-4
Tantawy, A. S., Salama, Y. A. M., El-Nemr, M. A., and Abdel-Mawgoud, A. M. R. (2015). Nano silicon application improves salinity tolerance of sweet pepper plants. Int. J. ChemTech Res. 8, 11–17.
Verslues, P. E., Batelli, G., Grillo, S., Agius, F., Kim, Y. S., Zhu, J., et al. (2007). Interaction of SOS2 with nucleoside diphosphate kinase 2 and catalases reveals a point of connection between salt stress and H2O2 signaling in Arabidopsis thaliana. Mol. Cell. Biol. 27, 7771–7780. doi: 10.1128/MCB.00429-07
Wang, Q., Yu, F., and Xie, Q. (2020). Balancing growth and adaptation to stress: crosstalk between brassinosteroid and abscisic acid signaling. Plant Cell Environ. 43, 2325–2335. doi: 10.1111/pce.13846
Wang, W., Zhang, X., Deng, F., Yuan, R., and Shen, R. (2017). Genome-wide characterization and expression analyses of superoxide dismutase (SOD) genes in Gossypium hirsutum. BMC Genomics 18:376. doi: 10.1007/s13205-018-1502-x
Wei, L. X., Lv, B. S., Wang, M. M., Ma, H. Y., Yang, H. Y., Liu, X. L., et al. (2015). Priming effect of abscisic acid on alkaline stress tolerance in rice (Oryza sativa L.) seedlings. Plant Physiol. Biochem. 90, 50–57. doi: 10.1016/j.plaphy.2015.03.002
Wu, H. (2018a). Plant salt tolerance and Na+ sensing and transport. Crop J. 6, 215–225. doi: 10.1016/j.cj.2018.01.003
Wu, H., Hill, C. B., Stefano, G., and Bose, J. (2021). Editorial: new insights into salinity sensing, signaling and adaptation in plants. Front. Plant Sci. 11:604139. doi: 10.3389/fpls.2020.604139
Wu, X., Jia, Q., Ji, S., Gong, B., and Gao, H. (2020). Gamma-aminobutyric acid (GABA) alleviates salt damage in tomato by modulating Na+ uptake, the GAD gene, amino acid synthesis and reactive oxygen species metabolism. BMC Plant Biol. 20:465. doi: 10.1186/s12870-020-02669-w
Wu, H., Lana, S., Elisa, A., Huang, Y., Camilla, P., Su, N., et al. (2018b). Na+ extrusion from the cytosol and tissue-specific Na+ sequestration in roots confer differential salt stress tolerance between durum and bread wheat. J. Exp. Bot. 69, 3987–4001. doi: 10.1093/jxb/ery194
Wu, H., Shabala, L., Barry, K., Zhou, M., and Shabala, S. (2013). Ability of leaf mesophyll to retain potassium correlates with salinity tolerance in wheat and barley. Physiol. Plant. 149, 515–527. doi: 10.1111/ppl.12056
Wu, H., Shabala, L., Shabala, S., and Giraldo, J. P. (2018c). Hydroxyl radical scavenging by cerium oxide nanoparticles improves Arabidopsis salinity tolerance by enhancing leaf mesophyll potassium retention. Environ. Sci. Nano 5, 1567–1583. doi: 10.1039/C8EN00323H
Wu, H., Shabala, L., Zhou, M., and Shabala, S. (2014). Durum and bread wheat differ in their ability to retain potassium in leaf mesophyll: implications for salinity stress tolerance. Plant Cell Physiol. 55, 1749–1762. doi: 10.1093/pcp/pcu105
Wu, H., Shabala, L., Zhou, M., Su, N., and Shabala, S. (2019). Root vacuolar Na+ sequestration but not exclusion from uptake correlates with barley salt tolerance. Plant J. 100, 55–67. doi: 10.1111/tpj.14424
Wu, H., Zhang, X., Pablo, G. J., and Sergey, S. (2018d). It is not all about sodium: revealing tissue specificity and signalling roles of potassium in plant responses to salt stress. Plant Soil 431, 1–17. doi: 10.1007/s11104-018-3770-y
Wu, H., Zhu, M., Shabala, L., Zhou, M., Shabala, S., Landfood, S. O., et al. (2015). K+ retention in leaf mesophyll, an overlooked component of salinity tolerance mechanism: A case study for barley. J. Integr. Plant Biol. 57, 171–185. doi: 10.1111/jipb.12238
Xiang, Z., Yan, L., Jingjing, H., and Jixing, X. (2020). OsNAC45 is involved in ABA response and salt tolerance in rice. Rice 13:79. doi: 10.1186/s12284-020-00440-1
Xie, G., Kato, H., and Imai, R. (2012). Biochememical identification of the OsMKK6-OsMPK3 signalling pathway for chilling stress tolerance in rice. Biochem. J. 443, 95–102. doi: 10.1016/j.devcel.2017.11.016
Xiong, L., Ishitani, M., Lee, H., and Zhu, J. K. (2001). The Arabidopsis LOS5/ABA3 locus encodes a molybdenum cofactor sulfurase and modulates cold stress and osmotic stress-responsive gene expression. Plant Cell 13, 2063–2083. doi: 10.1105/tpc.010101
Xue, Z. Y., Zhi, D. Y., Xue, G. P., Zhang, H., Zhao, Y., and X., and Xia, G., M., (2004). Enhanced salt tolerance of transgenic wheat (Tritivum aestivum L.) expressing a vacuolar Na+/H+ antiporter gene with improved grain yields in saline soils in the field and a reduced level of leaf Na+. Plant Sci. 167, 849–859. doi: 10.1016/j.plantsci.2004.05.034
Yang, Y., and Guo, Y. (2018a). Elucidating the molecular mechanisms mediating plant salt stress responses. New Phytol. 217, 523–539. doi: 10.1111/nph.14920
Yang, Y., and Guo, Y. (2018b). Unraveling salt stress signaling in plants. J. Integr. Plant Biol. 60, 796–804. doi: 10.1111/jipb.12689
Yf, A., Xz, B., Jiang, Y. B., and Yz, C. (2020). Fabrication of pea protein nanoparticles with calcium-induced cross-linking for the stabilization and delivery of antioxidative resveratrol. Int. J. Biol. Macromol. 152, 189–198. doi: 10.1016/j.ijbiomac.2020.02.248
Yu, Z., Duan, X., Luo, L., Dai, S., and Xia, G. (2020). How plant hormones mediate salt stress responses. Trends Plant Sci. 25, 1117–1130. doi: 10.1016/j.tplants.2020.06.008
Yue, Y., Zhang, M., Zhang, J., Duan, L., and Li, Z. (2012). SOS1 gene overexpression increased salt tolerance in transgenic tobacco by maintaining a higher K+/Na+ ratio. J. Plant Physiol. 169, 255–261. doi: 10.1016/j.jplph.2011.10.007
Zelm, E. V., Zhang, Y., and Testerink, C. (2020). Salt tolerance mechanisms of plants. Annu. Rev. Plant Biol. 71, 403–433. doi: 10.1146/annurev-arplant-050718-100005
Zhang, M., Cao, Y., Wang, Z., Wang, Z. Q., Shi, J., Liang, X., et al. (2017). A retrotransposon in an HKT1 family sodium transporter causes variation of leaf Na+ exclusion and salt tolerance in maize. New Phytol. 217, 1161–1176. doi: 10.1111/nph.14882
Zhang, H., Feng, H., Zhang, J., Ge, R., Zhang, L., Wang, Y., et al. (2020). Emerging crosstalk between two signaling pathways coordinates K+ and Na+ homeostasis in the halophyte Hordeum brevisubulatum. J. Exp. Bot. 71, 4345–4358. doi: 10.1093/jxb/eraa191
Zhang, G., Ming, C., Li, L., Xu, Z., Chen, X., Guo, J., et al. (2009). Overexpression of the soybean GmERF3 gene, an AP2/ERF type transcription factor for increased tolerances to salt, drought, and diseases in transgenic tobacco. J. Exp. Bot. 60, 3781–3796. doi: 10.1093/jxb/erp214
Zhang, X., Shi, Z., Tian, Y., Zhou, Q., Cai, J., Dai, T., et al. (2016). Salt stress increases content and size of glutenin macropolymers in wheat grain. Food Chem. 197, 516–521. doi: 10.1016/j.foodchem.2015.11.008
Zhang, Z., Wang, J., Zhang, R., and Huang, R. (2012). The ethylene response factor AtERF98 enhances tolerance to salt through the transcriptional activation of ascorbic acid synthesis in Arabidopsis. Plant Mol. Biol. 71, 273–287. doi: 10.1111/j.1365-313X.2012.04996.x
Zhao, L., Lu, L., Wang, A., Zhang, H., Huang, M., Wu, H., et al. (2020). Nano-biotechnology in agriculture: use of nanomaterials to promote plant growth and stress tolerance. J. Agric. Food Chem. 68, 1935–1947. doi: 10.1021/acs.jafc.9b06615
Zhao, Y., Wei, D., Zhang, N., Ai, X., Wang, M., Huang, Z., et al. (2014). A wheat allene oxide cyclase gene enhances salinity tolerance via jasmonate signaling. Plant Physiol. 164, 1068–1076. doi: 10.1104/pp.113.227595
Zhao, G., Zhao, Y., Lou, W., Su, J., Wei, S., Yang, X., et al. (2019). Nitrate reductase-dependent nitric oxide is crucial for multi-walled carbon nanotube-induced plant tolerance against salinity. Nanoscale 11, 10511–10523. doi: 10.1039/c8nr10514f
Zhou, Y., Wen, Z. L., Zhang, J. W., Chen, X. J., Cui, J. X., Xu, W., et al. (2017). Exogenous glutathione alleviates salt-induced oxidative stress in tomato seedlings by regulating glutathione metabolism, redox status, and the antioxidant system. Sci. Hortic. 220, 90–101. doi: 10.1016/j.scienta.2017.02.021
Zhou, H., Wu, H., Zhang, F., Su, Y., Guan, W., Xie, Y., et al. (2021). Molecular basis of cerium oxide nanoparticle enhancement of rice salt tolerance and yield. Environ. Sci. Nano 8, 3294–3311. doi: 10.1039/d1en00390a
Zhu, J. K. (2002). Salt and drought stress signal transduction in plants. Annu. Rev. Plant Biol. 53, 247–273. doi: 10.1146/annurev.arplant.53.091401.143329
Keywords: mechanisms, nanomaterials, phytohormones, reactive oxygen species, salt tolerance, Na+/K+ homeostasis
Citation: Li Z, Zhu L, Zhao F, Li J, Zhang X, Kong X, Wu H and Zhang Z (2022) Plant Salinity Stress Response and Nano-Enabled Plant Salt Tolerance. Front. Plant Sci. 13:843994. doi: 10.3389/fpls.2022.843994
Edited by:
Meixue Zhou, University of Tasmania, AustraliaReviewed by:
Lijuan Zhao, Nanjing University, ChinaSuprasanna Penna, Bhabha Atomic Research Centre (BARC), India
Copyright © 2022 Li, Zhu, Zhao, Li, Zhang, Kong, Wu and Zhang. This is an open-access article distributed under the terms of the Creative Commons Attribution License (CC BY). The use, distribution or reproduction in other forums is permitted, provided the original author(s) and the copyright owner(s) are credited and that the original publication in this journal is cited, in accordance with accepted academic practice. No use, distribution or reproduction is permitted which does not comply with these terms.
*Correspondence: Honghong Wu, aG9uZ2hvbmcud3VAbWFpbC5oemF1LmVkdS5jbg==; Zhiyong Zhang, el96eTEyM0AxMjYuY29t
†These authors have contributed equally to this work