- 1Department of Genetics and Plant Breeding, Banda University of Agriculture and Technology, Banda, India
- 2ICAR-Indian Institute of Pulses Research, Kanpur, India
- 3World Vegetable Center South Asia, Hyderabad, India
Late embryogenesis abundant (LEA) proteins are identified in many crops for their response and role in adaptation to various abiotic stresses, such as drought, salinity, and temperature. The LEA genes have been studied systematically in several crops but not in Vigna crops. In this study, we reported the first comprehensive analysis of the LEA gene family in three legume species, namely, mung bean (Vigna radiata), adzuki bean (Vigna angularis), and cowpea (Vigna unguiculata), and the cross-species expression of VrLEA genes in a wild tetraploid species, Vigna glabrescens. A total of 201 LEA genes from three Vigna crops were identified harboring the LEA conserved motif. Among these 55, 64, and 82 LEA genes were identified in mung bean, adzuki bean, and cowpea genomes, respectively. These LEA genes were grouped into eight different classes. Our analysis revealed that the cowpea genome comprised all eight classes of LEA genes, whereas the LEA-6 class was absent in the mung bean genome. Similarly, LEA-5 and LEA-6 were absent in the adzuki bean genome. The analysis of LEA genes provides an insight into their structural and functional diversity in the Vigna genome. The genes, such as VrLEA-2, VrLEA-40, VrLEA-47, and VrLEA-55, were significantly upregulated in the heat-tolerant genotype under stress conditions indicating the basis of heat tolerance. The successful amplification and expression of VrLEA genes in V. glabrescens indicated the utility of the developed markers in mung bean improvement. The results of this study increase our understanding of LEA genes and provide robust candidate genes for future functional investigations and a basis for improving heat stress tolerance in Vigna crops.
Introduction
Mung bean (Vigna radiata) is an important short-duration pulse crop occupying a large area, predominantly in the Indian subcontinent, besides many other places in Australia, Africa, and United States. It is primarily consumed as food and animal feed and also as a green manuring crop (Pratap et al., 2021). It has a high nutrition value (Pratap et al., 2020) with a prominence of quality protein besides having soil ameliorative properties (Allito et al., 2015; Sehrawat et al., 2015; Singh et al., 2017). Although several high-yielding and superior varieties have been developed over the last few decades in the mung bean due to their climate sensitivity, improving their productivity in extreme environments is still a major challenge (Singh et al., 2014, 2019a; Manivannan and Mahalingam, 2021). In legume species, such as Vigna crops, seed yield is highly affected by various abiotic stresses (HanumanthaRao et al., 2016; Douglas et al., 2020). Drought and high temperature are the most limiting factors (Douglas et al., 2020; Kumar et al., 2020; Singh et al., 2021) among the abiotic stresses, affecting the crops at different growth stages, leading to severe yield penalties. Varieties respond differentially to abiotic stresses as per stress duration, crop growth stage, and genetic potential of a variety, which leads to moderate-to-severe yield loss (Bangar et al., 2019). Hence, there is an urgent need to develop abiotic stress-tolerant varieties to mitigate the effects of climate change.
The heat stress negatively affects the seedling vigor, biomass accumulation, and reproductive development in mung bean (Tzudir et al., 2014; HanumanthaRao et al., 2016). It also affects all reproductive traits, such as flower initiation, pollen viability, stigma receptivity, ovule size and viability, fertilization, pod set, grain filling, and seed quality (Barnabas et al., 2008; Wassmann et al., 2009; Douglas et al., 2020). Flower shedding is very common in mung bean under heat stress (Kumari and Varma, 1983) while it severely affects flower bud initiation, and this sensitivity may prevail for 10–15 days (Bita and Gerats, 2013). Terminal heat stress is a major problem in spring- and summer-grown crops, whereas heat stress during early vegetative growth is frequent in Kharif (rainy) season.
A few potential donors have been identified and characterized among the cultivated germplasm and wild relatives in earlier studies against various abiotic stresses (Pratap et al., 2014; Sharma et al., 2016; Singh et al., 2021), which can be included in breeding programs for mung bean improvement. Pratap et al. (2014) characterized two wild accessions belonging to Vigna umbellata and Vigna glabrescens for photo- and thermoperiod insensitivity. In this study, we investigated the gene expression of VrLEA genes in heat-sensitive (HS) mung bean and heat-tolerant (HT) creole bean (V. glabrescens) genotypes for understanding the response of selected candidate genes under high-temperature stress. Wild species can be especially useful toward integrating crossable donors in breeding programs and help in improving stress tolerance in domesticated cultivars and broadening the genetic base of the crop.
Abiotic stresses decline crop productivity and seed quality by serious disruptions in plant growth and development (Xiong and Zhu, 2002; Ahuja et al., 2010). Therefore, to defend these adverse climatic conditions, plants have evolved complex regulatory mechanisms at molecular, physiological, and biochemical levels to minimize the effects of abiotic stresses (Zhu, 2016). Proteins play a major role in regulating the various signaling pathways that activate under abiotic stresses and indirectly improve stress tolerance. The late embryogenesis abundant (LEA) protein gene family is considered as an important group of functional proteins to reduce the scope of abiotic stresses in plants (Hirayama and Shinozaki, 2010; Debnath et al., 2011). These proteins can eliminate the cellular content of reactive oxygen species (ROS) to protect the macromolecular substances and alleviate damages caused by the various abiotic stresses (Yu et al., 2019).
The LEA genes were first identified in mature cotton seeds (Dure et al., 1981), which are found to be accumulated during dehydration and maturation of seeds, and protect them from damage. They have also been detected in various plant parts, such as seedlings, leaves, stems, roots, and other organs, under abiotic stress (Hincha and Thalhammer, 2012). Later, LEA proteins have also been identified in other plants, such as Arabidopsis (Finkelstein, 1993; Hundertmark and Hincha, 2008), Brassica napus (Liang et al., 2016), maize (Li and Cao, 2016), rice (Wang et al., 2007), soybean (Liu et al., 2018), wheat (Liu et al., 2019), grape wine (İbrahime et al., 2019), poplar (Cheng et al., 2021), and many more species. LEA proteins are highly hydrophilic and intrinsically unstructured, whereas they partially fold into mainly α-helical structures under dehydration state (Hand et al., 2011). This allows them to contribute the function as chaperones (Hanin et al., 2011) and also in the stabilization of membranes, calcium-binding, and metal homeostasis and the protection of functional proteins against aggregation (Krueger et al., 2002; Tolleter et al., 2010; Rahman et al., 2011; Rosales et al., 2014). LEA proteins are divided into eight different clusters, including LEA-1, LEA-2, LEA-3, LEA-4, LEA-5, LEA-6, dehydrin (DHN), and seed maturation protein (SMP) (Hundertmark and Hincha, 2008; Finn et al., 2010; Hunault and Jaspard, 2010).
While the LEA gene family also plays an important role in growth and development under multiple stress conditions, the study of this gene family in Vigna is still lacking. Many Vigna species are now sequenced, such as mung bean (Kang et al., 2014), adzuki bean (Kang et al., 2015), cowpea (Lonardi et al., 2019), beach pea (Singh et al., 2019b), moth bean (Takahashi et al., 2019), and black gram (Souframanien et al., 2020), with their sequence data available in the public database and can be accessed for analysis. Keeping this in view, this experiment was conducted to survey LEA genes and study their comparative structural diversity analysis in mung bean, adzuki bean, and cowpea. The expression of selected VrLEA genes through quantitative real-time PCR (qRT-PCR) was performed on HS mung bean and HT creole bean genotypes. It provides new insights for understanding the mechanism of heat tolerance in Vigna species.
Materials and Methods
Plant Materials and Stress Treatment
The plant materials comprised a high-yielding and HS-advanced breeding line of mung bean, IPM 312-19, and one HT-wild creole bean (V. glabrescens), accession IC251372. The plants of both the genotypes were grown in the net house during Kharif (rainy season) 2021 at Banda University of Agriculture and Technology, Banda, located at 24°53′ latitude and 25°55′ latitude. Both the genotypes were subjected to heat-shock treatment through the detached leaf method following the study by Kumar et al. (2009) with modification as well as in planta.
Experiment 1
At the early vegetative stage (20 days after germination), the expanded second trifoliate leaves from the top of the plants were subjected to heat stress treatment through the detached leaf method. This was performed in a growth chamber. The leaves from both the test genotypes (IPM 312-19 and IC 251372) were plucked and kept in Petri plates in distilled water, and heat shock was induced at two levels, namely, HSI 1: 45°C for 3 h with 60% relative humidity (RH); and HSI 2: 45°C for 6 h with 60% RH. For the control treatment (non-stressed), the detached trifoliate leaves were kept in Petri plates in distilled water and put into the growth chamber for 6 h at 25°C temperature with 60% RH to recover them from the injury induced by plucking. Immediately after the heat shock, the stressed and control (non-stressed) samples were taken out, blot-dried by slightly pressing between two layers of filter paper, frozen in liquid nitrogen, and stored at −80°C for further study.
Experiment 2
Another experiment was performed in planta under laboratory conditions in a plant growth chamber. The plants of both the genotypes were raised in a potting mixture in plastic pots with ideal conditions, such as 30°C temperature with 60% RH. The 7-day-old seedlings were subjected to heat stress. The leaf samples without induction of heat stress were kept as control (non-stress) while those taken 24 h after heat stress (45°C, 60% RH) were considered as stressed plants. The leaf samples from the stressed plants were immediately frozen in liquid nitrogen after plucking at different time points and stored at −80°C for further study.
RNA Extraction and cDNA Synthesis
A total of 200 mg of frozen leaf samples were homogenized in liquid nitrogen, and RNA was extracted using a Plant RNA Extraction Kit (RNeasy Mini Kit, QIAGEN) following the manufacturer’s instructions. Subsequently, RNA was reverse-transcribed from 1 μg of RNA by the RevertAid First-Strand cDNA Synthesis Kit (Thermo Fisher Scientific). The cDNA was normalized by 50 ng/μl for qRT-PCR analysis.
Identification of Late Embryogenesis Abundant Protein Sequences
The genome-wide data of mung bean (V. radiata), adzuki bean (Vigna angularis), and cowpea (Vigna unguiculata) were obtained from Legume Information System (LIS) online website1. The typical LEA protein genes were retrieved through Pfam analysis with PF03760, PF03168, PF03242, PF02987, PF0477, PF10714, PF0492, and PF002572. The redundancy of sequences was eliminated by ExPASy3 to generate a unique set of LEA proteins. The LEA proteins were scanned against the mung bean, adzuki bean, and cowpea genomes by using the HMMER 3.0 program4 followed by manual verification with the Pfam database5. The protein sequences that did not comprise LEA domains were removed and not included in the analysis.
Chromosomal Distribution and Phylogenetic Analysis of Late Embryogenesis Abundant Genes
The physical positions (in bps) of the VrLEA, VaLEA, and VuLEA genes were identified in this study, and the length of chromosomes was obtained from the LIS database. These genes from short arm to long arm were mapped onto their corresponding chromosomes in ascending order using MapChart 2.3 (Voorrips, 2002). The LEA genes identified in the scaffold were not assigned on the chromosome. The putative VrLEA, VaLEA, and VuLEA genes were classified into eight different groups. The phylogenetic trees were constructed with all the putative LEA genes identified in this study. Multiple alignments of the deduced amino acid sequences were performed using ClustalW (Thompson et al., 2002). The phylogenetic tree was constructed through the maximum likelihood (ML) method (Guindon and Gascuel, 2003) provided in the MEGA 7.0 tool (Tamura et al., 2013) based on the Jones-Taylor-Thornton (JTT) matrix-based model. The bootstrap analysis was set with 1,000 replications for the accuracy of the constructed tree.
Gene Structure Prediction and Protein Analysis of Late Embryogenesis Abundant Genes
The CDS and the genomic DNA sequences of the corresponding LEA genes were retrieved by blast analysis on the NCBI database. The gene structures of LEA were determined by comparing the genomic DNA sequences and their corresponding coding sequences using the Gene Structure Display Server version 2.0 (Hu et al., 2015)6. The molecular weight (kDa) and isoelectric point (pI) of LEA sequences were predicted with EndMemo7 and pI calculator8, respectively (Gasteiger et al., 2003). The motifs were analyzed through the MEME program9 (Bailey et al., 2009). In motif analysis, the maximum number of motifs was preset to 10 while the optimum width of motifs was set to 6–50 amino acid residues, and other settings were kept as default.
Gene-Based Primer Designing and Quantitative Real-Time PCR Analysis
All the VrLEA candidate genes were blasted against the V. glabrescens genome, and the qRT-PCR primers were designed using the Primer-BLAST tool10. Six gene-specific qRT-PCR primers from each clade based on their functions were selected for expression analysis. For PCRs, 10 μl 2X SYBR Green qPCR Master Mix, 1 μl of 10 pmol each forward and reverse primers, and 6 μl nuclease-free water were used. The three-step program was run with 10 min: initial denaturation at 96°C, 40 cycles of 45 s; denaturation at 96°C, 45 s; annealing at 58°C, 45 s; and extension at 72°C, followed by melting at the default setting. The Actin gene was used as an internal control. The qRT-PCR analysis was carried out using a Rotor-Gene Q-6000 Real-Time PCR machine (QIAGEN). Three biological replicates were taken for expression profiling, and two technical replicates were used for analysis. The relative expression levels of the genes were calculated using the 2–ΔΔCT method (Livak and Schmittgen, 2001).
Results
Genome-Wide Identification of VrLEA, VaLEA, and VuLEA Genes
A total of 201 LEA genes were retrieved from three Vigna species, namely, mung bean, adzuki bean, and cowpea. A total of 55 non-redundant LEA-sequences from mung bean, 64 from adzuki bean, and 82 from cowpea genomes were identified and considered as putative LEA genes (Table 1). However, 96 MtLEA-genes were retrieved from the analysis of the Medicago truncatula genome. These sequences were classified into eight different groups, namely, LEA-1, LEA-2, LEA-3, LEA-4, LEA-5, LEA-6, SMP, and DHN. LEA genes accounted for 0.20% of all genes present in mung bean (VrLEA), 0.24% of genes present in adzuki bean (VaLEA), 0.29% of genes present in cowpea (VuLEA), and 0.30% of genes present in model legume Medicago. Among the eight classes of the LEA gene subfamily, VrLEA-6 was absent in mung bean, whereas VaLEA-5 and VaLEA-6 were found absent in adzuki bean. The maximum proportion of LEA-2 was noticed in all the three Vigna species as 38 VrLEA-2 genes were observed in mung bean, 45 VaLEA-2 in adzuki bean, 55 VuLEA-2 in cowpea, and 68 VrLEA-2 genes in Medicago. The average LEA genes per Mb were found as 0.12 genes per Mb in mung bean, 0.15 in adzuki bean, 0.13 in cowpea, and 0.23 genes per Mb in M. truncatula.
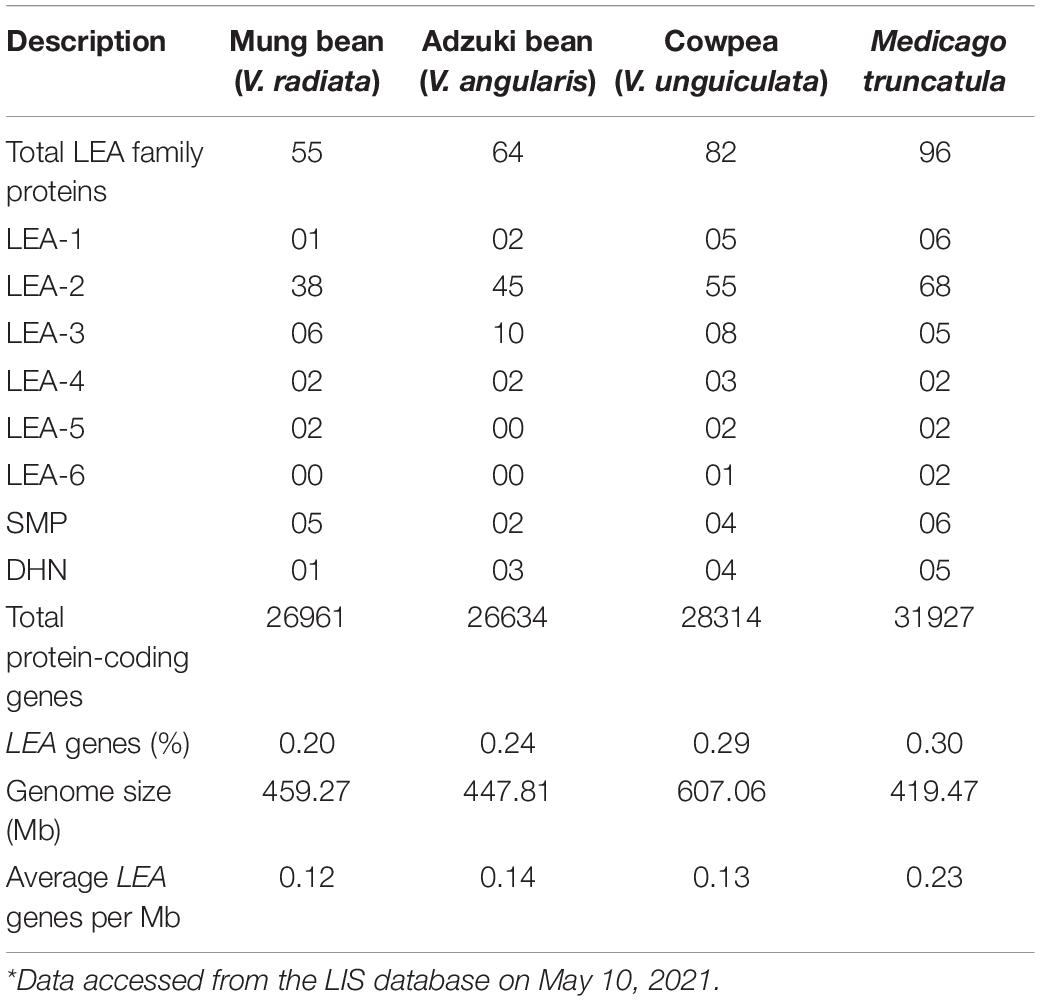
Table 1. The proportions of late embryogenesis abundant (LEA) genes in three Vigna and Medicago truncatula genomes*.
Chromosomal Distribution of VrLEA, VaLEA, and VuLEA Genes
The study observed that 48 out of 55 VrLEA, 38 out of 62 VaLEA, and 80 out of 84 VuLEA genes were distributed across 11 chromosomes of mung bean, adzuki bean, and cowpea, respectively (Figure 1), whereas the rest of the VrLEA, VaLEA, and VuLEA genes were present in the scaffold (Supplementary Tables 1–3). Chromosome 10 (ch. 10) had a minimum number of two VrLEA genes, whereas ch. 8 had the maximum number of nine VrLEA genes in the mung bean genome. Four chromosomes, namely, 1, 2, 10, and 11 had only VrLEA-2 genes, whereas the rest of the VrLEA genes were distributed over seven chromosomes. Similarly, in adzuki bean, ch. 5 comprised only one VaLEA gene, whereas ch. 4 had a maximum number of seven VaLEA genes. The ch. 1, 7, and 10 are comprised only VaLEA genes. In the cowpea genome, ch. 8 had a minimum of four VuLEA genes, whereas ch. 5 had a maximum of 11 VuLEA genes. Out of 11 chromosomes of cowpea, three chromosomes, namely, ch. 2, 8, and 11 consisted of only VuLEA genes while the rest of the 8 chromosomes comprised a mixture of different classes of VuLEA genes.
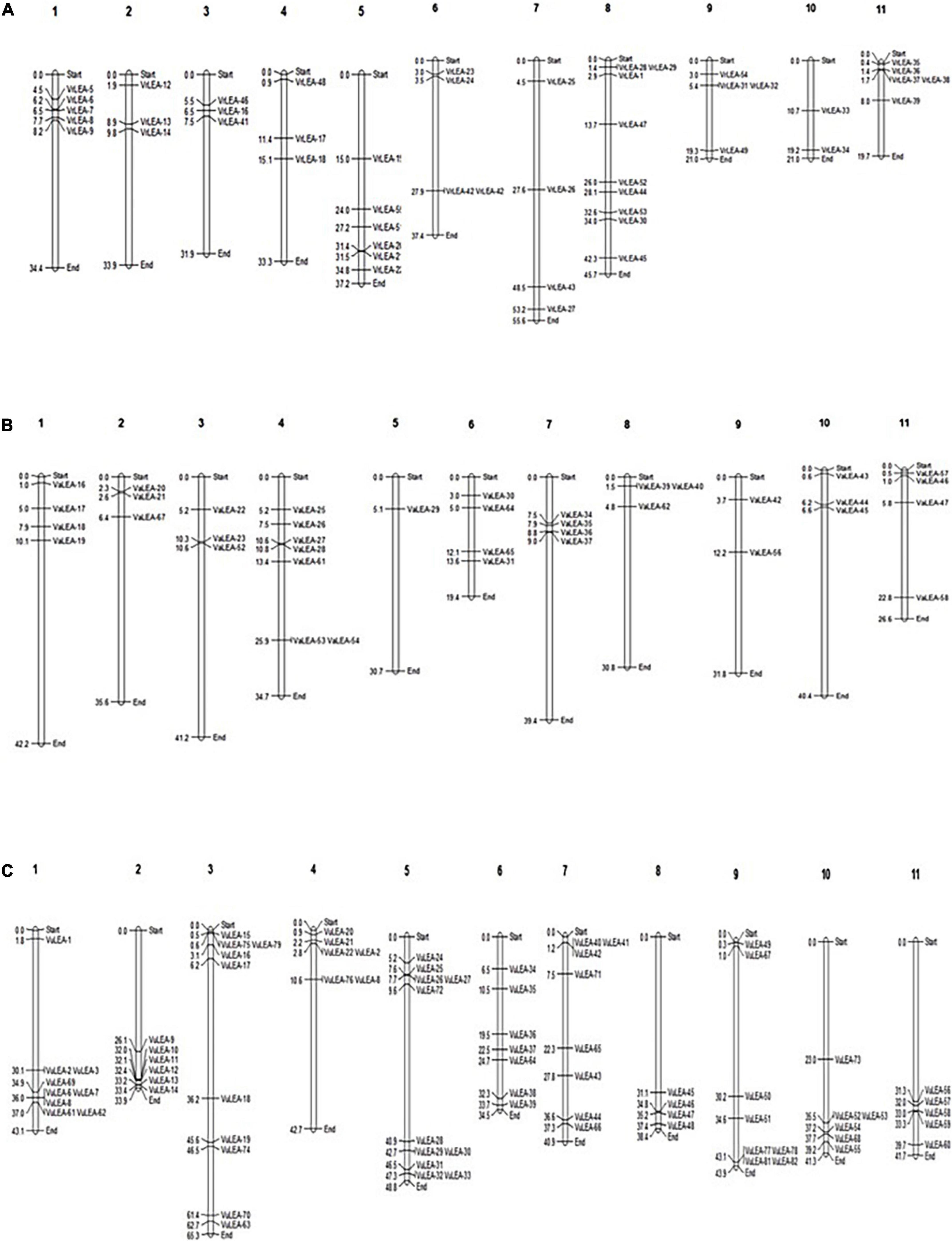
Figure 1. (A) Chromosomal distribution of late embryogenesis abundant (LEA) genes on mung bean (Vigna radiata) genome. (B) Chromosomal distribution of LEA genes on adzuki bean (Vigna angularis) genome. (C) Chromosomal distribution of LEA genes on cowpea (Vigna unguiculata) genome.
Phylogenetic Relationship of VrLEA, VaLEA, and VuLEA Genes
For the domain-based classification of LEA proteins, all the 201 LEA genes from three Vigna species identified in this study were considered for the construction of the phylogenetic tree (Figure 2). The LEA genes verified through Pfam for late embryogenesis-abundant domains were considered as putative LEA genes. All of the 201 LEA genes were clearly grouped into 15 major classes indicating their diversity. In mung bean, all the 55 LEA genes were grouped into eight clusters (Figure 3). Clade I comprised 27 VrLEA-2 genes, forming the largest cluster. Clade II consisted of 10 VrLEA genes, including 3 VrLEA-2 genes, 6 VrLEA-3 genes, and 1 VrLEA-4 gene. Clades III and IV were the single-cluster clades comprising 1 VrLEA-2 gene each. Clade V had three types of LEA genes, including 1 VrLEA-1 and VrLEA-4 gene each, and 4 VrLEA-2 genes. Clade VI had 4 VrSMP genes, and Clade VII had 2 VrLEA-2 genes. Similarly, Clade VIII had four VrLEA genes, including 2 VrLEA-5 and VrLEA-6 genes each. The LEA genes in adzuki bean clearly grouped into three major clades (Figure 4). Clade I comprised 44 VaLEA-2 genes and 1 VaLEA-3 gene. Clade II consisted of only one gene of VrLEA-1. Clade III had 2 VaLEA-2, 3 VaDHN, 9 VaLEA-3, 2 VaLEA-4, and SMP genes each. Similarly, all the 82 LEA genes from the cowpea genome were grouped into four clades (Figure 5). Clade I comprised 50 VuLEA-2 genes, and 1 VuLEA-3 and VuSMP gene each whereas Clade II consisted of five different types of VuLEA genes. Clade III had 4 VuLEA-2 genes and 1 VuLEA-3 gene. Six VuLEA-3 genes and 1 VuLEA-4 gene were grouped together in Clade IV. This phylogenetic analysis also provided information about diversification and evolutionary relationships among the LEA genes.
Characterization of VrLEA, VaLEA, and VuLEA Genes
The analyses about the length of LEA protein, genomic and CDS sequence, number of exons, molecular weight, and pI are presented in Supplementary Tables 1–3. The genomic length of LEA genes ranged from 282 bp (VrLEA-40) to 22,112 bp (VrLEA-34) in mung bean, 249 (VaLEA-56) to 30,173 bp (VaLEA-21) in adzuki bean, and 410 (VuLEA-61) to 4711 bp (VuLEA-70) in cowpea. Simultaneously, the length of coding sequences varied from 282 bp (VrLEA-40) to 7,113 bp (VrLEA-34) in mung bean, 249 (VaLEA-56) to 3,993 bp (VaLEA-21) in adzuki bean, and 270 (VuLEA-76) to 2,386 bp (VuLEA-24) in cowpea. The number of exons ranged from 1 to 7 in mung bean, whereas it varied from 1 to 4 in adzuki bean and cowpea, respectively. The LEA protein length varied from 94 (VrLEA-34) to 2,354 (VrLEA-34) in mung bean, 83 (VrLEA-55) to 1,050 (VrLEA-64) in adzuki bean, and 83 to 1,050 in cowpea. The predicted molecular weight of LEA proteins from mung bean, adzuki bean, and cowpea ranged from 10.22 kDa (VrLEA-48) to 263.50 kDa (VrLEA-34), 9.36 kDa (VaLEA-55) to 1063.50 kDa (VaLEA-59), and 21.11 kDa (VuLEA-76) to 199.46 kDa (VuLEA-44), respectively. Similarly, pI ranged from 4.47 (VrLEA-50) to 9.78 (VrLEA-31), 4.40 (VaLEA-60) to 11.02 (VaLEA-54), and 3.85 (VuLEA-60) to 4.37 (VuLEA-76) in the three Vigna species under study, indicating the structural diversity of LEA genes.
Gene Structure and Motif Analysis of VrLEA, VaLEA, and VuLEA Genes
The exon-intron organization of the 201 LEA genes from mung bean, adzuki bean, and cowpea was constructed using the coding and genomic sequences (Figures 3–5). The gene structures of LEA genes revealed that all the LEA genes exhibited variable exon-intron organization. It ranged from 1 to 7 exons in mung bean and 1 to 4 exons in both adzuki bean and cowpea. The maximum number of introns was found in mung bean as compared to adzuki bean and cowpea, indicating the diversification of the mung bean genome. A total of 10 distinct motifs were identified for LEA genes from mung bean, adzuki bean, and cowpea (Figure 6).
Expression Pattern of VrLEA Genes in Response to Heat Stress
All the 55 VrLEA candidate genes were used for primer designing, and subsequently, 55 primer pairs were developed. These primers were BLAST-searched against the V. glabrescens genome, and their annotation was performed (Supplementary Table 4). Seven representative candidates from each subclade were selected for the expression analysis (Figure 7). The expression of these seven genes (VrLEA-1, VrLEA-2, VrLEA-40, VrLEA-47, VrLEA-48, VrLEA-54, and VrLEA-55) in the HS (IPM 312-19) and HT (IC251372) genotypes was analyzed. The gene VrLEA-1 exhibited a significant increase (about 15-fold) in HS genotypes on heat shock, whereas a decrease was noticed in the HT genotype. The VrLEA-2 transcript significantly increased in HS genotype and decreased in HT genotype (IC 251372) at both the levels of heat stress (HSI-1 and HSI-2; 45°C for 3 and 6 h, respectively). The expression of VrLEA-40 decreased in both the genotypes at HSI-1, whereas significantly increased in both the genotypes at HSI-2. The VrLEA-47 exhibited a significant decrease in HS genotype, whereas it increased significantly to about 2.5-fold at HSI-2. The pattern of expression of VrLEA-48 was similar to that of VrLEA-47, although the level of expression was higher than VrLEA-47. The VrLEA-54 transcript was downregulated in the HS genotype after the heat sock, whereas it was upregulated significantly to about 2-fold in the HT genotype after the heat-shock induction. Similarly, about a 5-fold increase was noticed in HT genotypes at HSI-2 as compared to control.
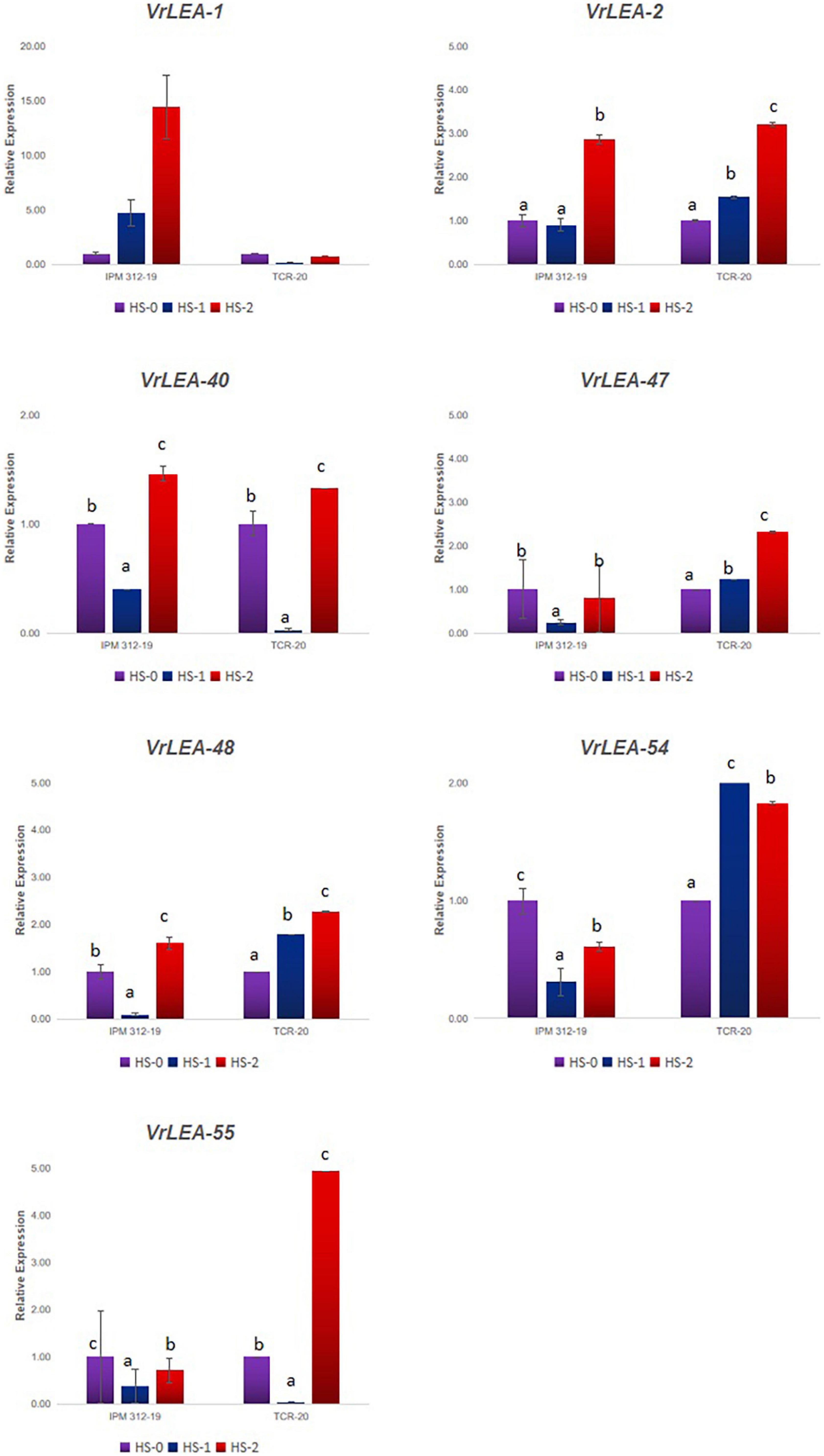
Figure 7. qRT-PCR analysis of selected VrLEA candidate genes on HS-mungbean (IPM 312-19) and HR-V. glabrescens (IC251372) under heat shock stress through detached leaf method. The statistical test was performed separately in both genotypes. The same letters show non-significant differences, whereas different letters show significant differences.
In the second experiment, the 7-day-old seedlings of HS and HT genotypes were subjected to 24-h heat-shock induction and they exhibited differential expression of the genes (Figure 8). The gene VrLEA-1 exhibited a similar pattern of expression as in the case of the detached leaf method although it was comparatively lower. The expression of three genes, namely, VrLEA-2, VrLEA-40, and VrLEA-47, was downregulated in the HS genotypes after 24 h of heat stress, whereas it was significantly upregulated in the HT genotype. In contrast, VrLEA-48 and VrLEA-54 exhibited upregulation in both HS and HT genotypes, although the level was higher in the HS genotype. Similarly, VrLEA-55 exhibited upregulation in both HS and HT genotypes with a higher expression in the HT genotype as compared to the HS genotype.
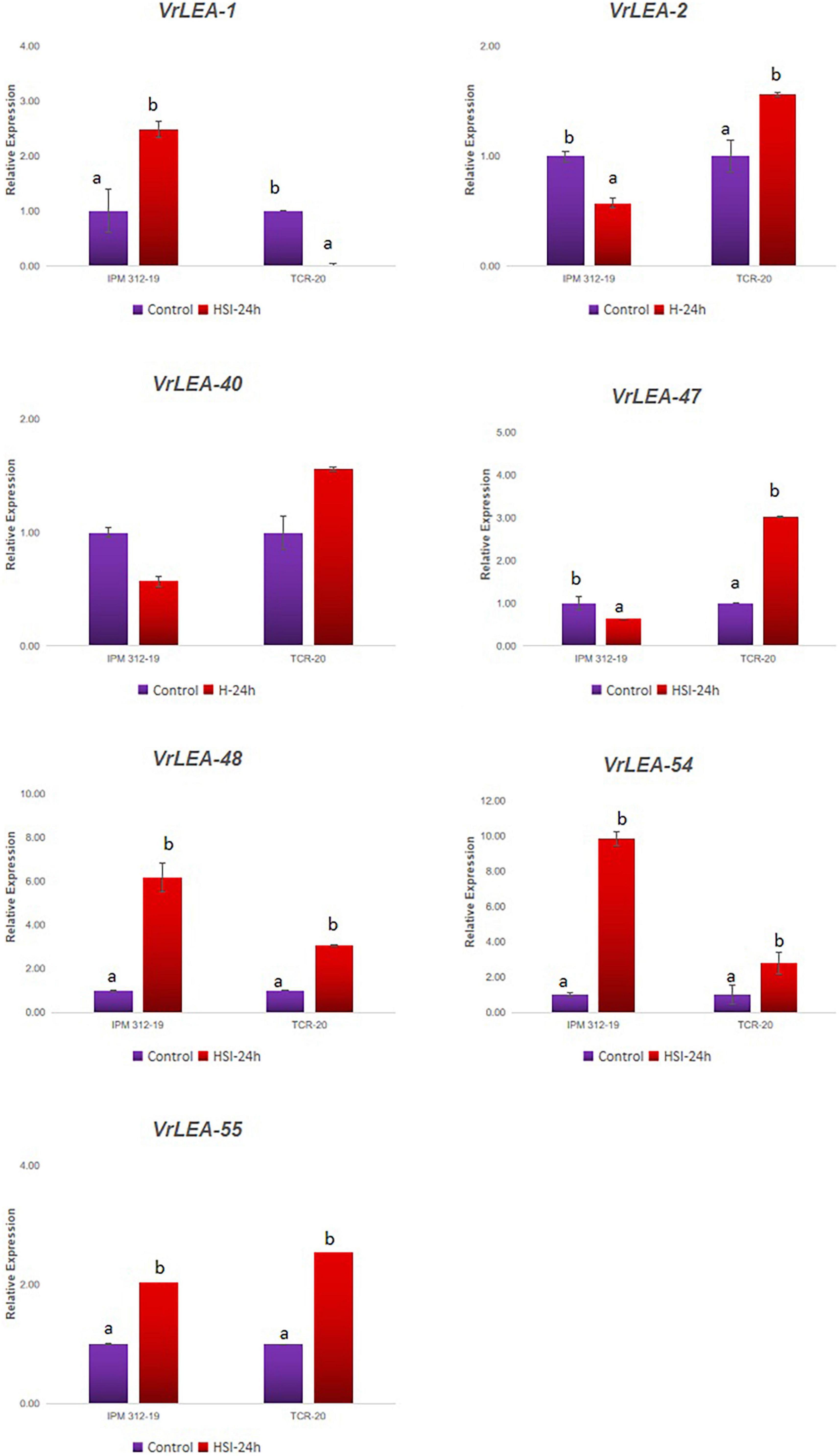
Figure 8. qRT-PCR analysis of selected VrLEA candidate genes on HS-mungbean (IPM 312-19) and HR-V. glabrescens (IC251372) under heat shock stress at seedling stage. The statistical test was performed separately in both genotypes. The same letters show non-significant differences, whereas different letters show significant differences.
Discussion
Abiotic stresses encompass extreme environmental factors which alter a plant’s biochemical and physiological processes constraining its productivity (Douglas et al., 2020). Abiotic stresses, either individually or in combination, hinder the crops from reaching their actual genetic yield potential. Among the abiotic stresses, heat stress is one of the most important and frequent constraints that limit the productivity of crop plants, especially during the current scenario of climate change, and is, therefore, a concern for crop cultivation worldwide. The crop yield of mung bean is highly affected by the fluctuating temperatures as the progression and the phenological development of the plant are mainly driven by temperature (Carberry, 2007; Chauhan et al., 2010). While the optimum temperatures for mung bean are 28–30°C (Kaur et al., 2015), the key temperatures are 7.5°C (baseline), 30°C (optimum), and 40°C (maximum). Most of the Vigna crops are very frequently subjected to extreme environmental conditions that are many times at the upper extremes as far as temperature is concerned. However, a very limited study has been conducted till present on the aspect of high-temperature tolerance in mung bean (Kumar et al., 2016, 2017). The linkage of high-throughput phenotyping platforms for the screening of target traits with omics interventions can give a clue for its mitigation (Pratap et al., 2019). Besides, the genome-wide analysis of gene families and transcriptional factors helps in designing crops for improved heat tolerance in mung bean.
The LEA gene family is large and widely diversified across the plant kingdom (Gao and Lan, 2016). It is an important gene family that responds to plant developmental activities, biotic and abiotic stresses (Hincha and Thalhammer, 2012), and hormonal regulations (Liu et al., 2019). This gene family has been characterized in many crops, such as Arabidopsis (Hundertmark and Hincha, 2008), wheat (Liu et al., 2019), tea (Jin et al., 2019), potato (Chen et al., 2019), and poplar (Cheng et al., 2021). Besides, this gene family was also identified in fungi and bacteria (Garay-Arroyo et al., 2000; Gal et al., 2004; Gao et al., 2016). In contrast, the genome-wide identification and characterization of the LEA gene family in mung bean, adzuki bean, and cowpea have not yet been performed systematically. In this study, a total of 201 LEA genes were identified, in which 55, 64, and 82 LEA genes were found in mung bean, adzuki bean, and cowpea genomes, respectively. The analysis revealed that these genes belong to six to eight classes of the LEA gene family. Based on the conserved domain and phylogenetic tree analyses, 55 VrLEA genes were categorized in seven distinct groups, 64 VaLEA genes in six groups, and 82 VuLEA genes in eight groups. The cowpea genome had all the eight types of LEA subfamilies whereas the adzuki bean genome lacked two subfamilies of VaLEA genes (i.e., VaLEA-5 and VaLEA-6), and the mung bean genome lacked the VrLEA-6 subfamily. It was indicated that LEA-1 to LEA-4, SMP, and DHN were highly conserved. The LEA-6 group has also been found to be absent in a few higher plants, such as Dendrobium officinale (Ling et al., 2016) and Solanum lycopersicum (Cao and Li, 2015). This finding indicated that the variation exists in the LEA protein gene family groups in some of the plant species. In the three Vigna species studied, the maximum proportion of the LEA gene family was accounted for LEA-2 as compared to other LEA groups. A similar type of contribution of LEA-2 has been observed earlier by Jin et al. (2019) in tea. In contrast, a similar proportion of the LEA-2 group was not observed earlier in many plant species, such as Arabidopsis thaliana (Hundertmark and Hincha, 2008), Cleistogenes songorica (Muvunyi et al., 2018), Manihot esculenta (Wu et al., 2018), Pinus tabuliformis (Gao et al., 2016), and Populus trichocarpa (Lan et al., 2013). This difference may be attributed to the improvement in the annotation of genomes of plants species. The differences were observed in all the groupings of VrLEA, VaLEA, and VuLEA genes in the phylogenetic analysis, indicating their diversification and differential genetic pattern. This finding also indicated the variation and complexity of the LEA gene family in plants. The gene structure prediction of LEA genes revealed that most of the LEA genes contained only a few or no introns, and all encoded the conserved LEA domain.
Based on the physiochemical properties of LEA genes, it was noticed that most of the LEA genes (58.18%) encode relatively small proteins (<25 kDa) in the mung bean genome (Figure 9A), whereas only 3.60% of them are large proteins (>50 kDa) (Figure 9B). Similarly, about 43.75% of genes encode small proteins in the adzuki bean genome and 7.81% of them encode larger proteins. On the contrary, only 2.44% of genes are <25 kDa in the cowpea genome, whereas most of the genes (about 52.44%) had a high molecular weight (50.1–100 kDa). This result was in conformity with the findings of Chen et al. (2019) in Solanum tuberosum (94.6%) and those of Liang et al. (2016) in B. napus (90.7%). Therefore, while this report is in conformity with the earlier reports in the case of mung bean and adzuki bean, it is contrasting in the case of the cowpea genome. The analysis also revealed that each LEA group contained conserved motifs that have been previously reported in other plant species (Liang et al., 2016; Magwanga et al., 2018). This was indicated that LEA genes may encode functional LEA proteins that have specific functions within the group, and the members of the same protein within the group may have originated from gene expansion. The members of the different groups may be due to the evolution from different ancestors.
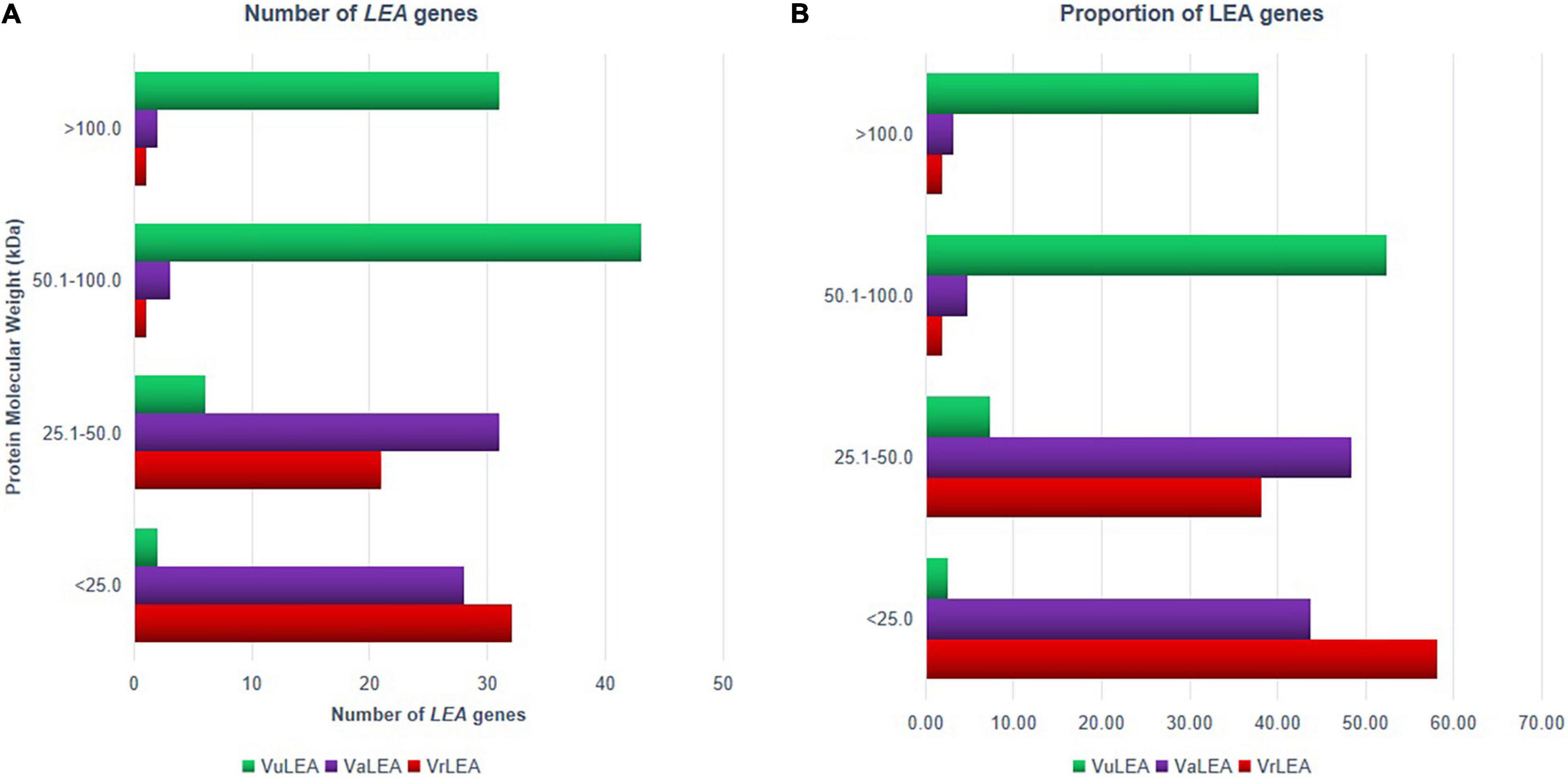
Figure 9. (A) Number and (B) proportion of LEA protein in VrLEA, VaLEA, and VuLEA gene families based on their molecular weight.
Most of the LEA proteins are predicted to have no stable secondary structure in solution, but they may acquire α-helical structures on dehydration (Bremer et al., 2017), which allows them to conformational change according to the changes in their microenvironment, resulting in multiple functions in abiotic stresses (Hanin et al., 2011). Previous studies have shown that LEA genes play an important role in low-temperature, drought, and high-salinity stresses (Jin et al., 2019). The expression of LEA proteins is often induced by abiotic stresses, such as drought, temperature, salt, and exogenous application of hormones, at different development stages and tissues of plants (Chen et al., 2019), and an increased expression of the LEA genes can improve stress tolerance. The overexpression of the wheat DHN-5 was reported to enhance osmotic stress tolerance in Arabidopsis (Brini et al., 2011). Ding et al. (2021) reported the maximum expression of LEA genes under polyethylene glycol (PEG) treatment, low temperature, and light intensity deviations in the case of rye. Therefore, the above-mentioned studies demonstrate that the LEA genes could potentially be used to improve the abiotic stress tolerance of crops. The expression analysis of HS and HT genotypes in this study indicated that the genes VrLEA-1, VrLEA-47, VrLEA-48, VrLEA-54, and VrLEA-55 play an important role toward heat stress tolerance in the HT-wild Vigna, accession IC 251372. Similarly, LEA genes, such as VrLEA-2, VrLEA-40, VrLEA-47, and VrLEA-55, were significantly overexpressed in the HT genotype, indicating the basis of heat tolerance in IC251372. The VrLEA-55 (DHN protein) gene showed a 5-fold increase in the expression in HT genotype in Experiment-1 (detached leaf method), whereas about two-fold increase was noticed at the seedling stage under 24 h of heat induction in the intact seedling (in planta heat stress treatment). The differential expression of the VrLEA-55 gene in both treatments could be attributed to mechanical injury in addition to heat stress in the detached leaf method as compared to the in planta method. Ding et al. (2021) also noticed the expression of this gene in different tissues, including seeds of rye, in drought stress although the expression was non-significant. Our study reports the expression of this candidate gene in the leaves of the HT-wild Vigna genotype and provides a new insight to heat tolerance. Therefore, the results of this study laid the foundation for further investigating the functional characterization of LEA proteins and their potential use in the genetic improvement of mung bean for heat tolerance.
Conclusion
A total of 201 LEA genes were identified in three Vigna species which were further grouped into seven distinct clades based on their phylogenetic relationships. The LEA-6 group was found absent in the mung bean genome, whereas all the seven groups were present in the cowpea genome. The gene structure exhibited diversification of candidates in the Vigna genome. Additionally, the expression profiling of seven VrLEA genes in leaves under four heat stress treatments revealed that these genes had an important role in response to heat stress. These results, therefore, provide valuable information for the future functional studies of LEA genes toward the genetic improvement of mung bean for heat stress tolerance.
Data Availability Statement
The datasets presented in this study can be found in online repositories. The names of the repository/repositories and accession number(s) can be found in the article/Supplementary Material.
Author Contributions
CMS, MK, and AP conceived the idea and planned the work. SS, AT, AM, and HK retrieved the data from the database. CMS and AP analyzed the data. CMS drafted the manuscript. AP, RN, and NS edited the manuscript. All authors read the manuscript and approved it for publication.
Funding
This study was financially supported by the Ministry of Agriculture, Agriculture Education and Research, Government of Uttar Pradesh, under the project “Center of Excellence in Dryland Agriculture (CEDA/2018-23)” and “ACIAR Funded International Mungbean Improvement Network (Grant No. CIM/2014/079).”
Conflict of Interest
The authors declare that the research was conducted in the absence of any commercial or financial relationships that could be construed as a potential conflict of interest.
Publisher’s Note
All claims expressed in this article are solely those of the authors and do not necessarily represent those of their affiliated organizations, or those of the publisher, the editors and the reviewers. Any product that may be evaluated in this article, or claim that may be made by its manufacturer, is not guaranteed or endorsed by the publisher.
Supplementary Material
The Supplementary Material for this article can be found online at: https://www.frontiersin.org/articles/10.3389/fpls.2022.843107/full#supplementary-material
Footnotes
- ^ https://legumeinfo.org/
- ^ https://legumeinfo.org/search/gene
- ^ https://web.expasy.org/decrease_redundancy/
- ^ https://www.ebi.ac.uk/Tools/hmmer/
- ^ http://pfam.xfam.org/
- ^ http://gsds.gao-lab.org/
- ^ http://www.endmemo.com/bio/promw.php
- ^ http://isoelectric.org/
- ^ https://meme-suite.org/meme/tools/meme
- ^ https://www.ncbi.nlm.nih.gov/tools/primer-blast/
References
Ahuja, I., de-Vos, R. C. H., Bones, A. M., and Hall, R. D. (2010). Plant molecular stress responses face climate change. Trends Plant Sci. 15, 664–674. doi: 10.1016/j.tplants.2010.08.002
Allito, B. B., Nana, E. M., and Alemneh, A. A. (2015). Rhizobia strain and legume genome interaction effects on nitrogen fixation and yield of grain legume: a review. Mol. Soil Biol. 2, 1–6. doi: 10.5376/msb.2015.06.0002
Bailey, T. L., Boden, M., Buske, F. A., Frith, M., Grant, C. E., Clementi, L., et al. (2009). MEME Suite: tools for motif discovery and searching. Nucleic Acids Res. 37, W202–W208. doi: 10.1093/nar/gkp335
Bangar, P., Chaudhury, A., Tiwari, B., Kumar, S., Kumari, R., and Bhat, K. V. (2019). Morphophysiological and biochemical response of mungbean [Vigna radiata (L.) Wilczek] varieties at different developmental stages under drought stress. Turk. J Biol. 43, 58–69. doi: 10.3906/biy-1801-64
Barnabas, B., Jäger, K., and Fehér, A. (2008). The effect of drought and heat stress on reproductive processes in cereals. Plant Cell Environ. 31, 11–38. doi: 10.1111/j.1365-3040.2007.01727.x
Bita, C. E., and Gerats, T. (2013). Plant tolerance to high temperature in a changing environment: scientific fundamentals and production of heat stress tolerant crops. Front. Plant Sci. 4:273. doi: 10.3389/fpls.2013.00273
Bremer, A., Wolff, M., Thalhammer, A., and Hincha, D. K. (2017). Folding of intrinsically disordered plant LEA proteins is driven by glycerol-induced crowding and the presence of membranes. FEBS J. 284, 919–936. doi: 10.1111/febs.14023
Brini, F., Yamamoto, A., Jlaiel, L., Takeda, S., Hobo, T., Dinh, H. Q., et al. (2011). Pleiotropic effects of the wheat Dehydrin DHN-5 on stress responses in Arabidopsis. Plant Cell Physiol. 52, 676–688. doi: 10.1093/pcp/pcr030
Cao, J., and Li, X. (2015). Identification and phylogenetic analysis of late embryogenesis abundant proteins family in tomato (Solanum lycopersicum). Planta 241, 757–772. doi: 10.1007/s00425-014-2215-y
Carberry, P. (2007). “Crop development models,” in Encyclopedia of Science Encyclopedia of Water Science, 2nd Edn. (Boca Raton, FL: CRC Press), 122–124.
Chauhan, Y. S., Douglas, C. A., Rachaputi, R. C. N., Agius, P., Martin, W. D., King, K., et al. (2010). “Physiology of mungbean and development of the mungbean crop model,” in Proceedings of the 1st Australian Summer Grains Conference, Gold Coast, QLD, 21–24.
Chen, Y., Li, C., Zhang, B., Yi, J., Yang, Y., Kong, C., et al. (2019). The role of the late embryogenesis-abundant (LEA) protein family in development and the abiotic stress response: a comprehensive expression analysis of potato (Solanum tuberosum). Genes 10:148. doi: 10.3390/genes10020148
Cheng, Z., Zhang, X., Yao, W., Zhao, K., Liu, L., Fan, G., et al. (2021). Genome-wide search and structural and functional analyses for late embryogenesis-abundant (LEA) gene family in poplar. BMC Plant Biol. 21:110. doi: 10.1186/s12870-021-02872-3
Debnath, M., Pandey, M., and Bisen, P. S. (2011). An omics approach to understand the plant abiotic stress. OMICS 15, 739–762. doi: 10.1089/omi.2010.0146
Ding, M., Wang, L., Zhan, W., Sun, G., Jia, X., Chen, S., et al. (2021). Genome-wide identification and expression analysis of late embryogenesis abundant protein-encoding genes in rye (Secale cereale L.). PLoS One 16:e0249757. doi: 10.1371/journal.pone.0249757
Douglas, C., Pratap, A., Hanumantharao, B., Manu, B., Dubey, S., Singh, P., et al. (2020). “Breeding progress and future challenges: abiotic stresses,” in The Mungbean Genome, Compendium of Plant Genomes, eds R. M. Nair, R. Schafleitner, and S. H. Lee (Cham: Springer). doi: 10.1007/978-3-030-20008-4_6
Dure, L. R., Greenway, S. C., and Galau, G. A. (1981). Developmental biochemistry of cottonseed embryogenesis and germination: changing messenger ribonucleic acid populations as shown by in vitro and in vivo protein synthesis. Biochemistry-US 20, 4162–4168. doi: 10.1021/bi00517a033
Finkelstein, R. R. (1993). Abscisic acid-insensitive mutations provide evidence for stage-specific signal pathways regulating expression of an Arabidopsis late embryogenesis-abundant (lea) gene. Mol. Gen. Genet. 238, 401–408. doi: 10.1007/BF00291999
Finn, R. D., Mistry, J., Tate, J., Coggill, P., Heger, A., Pollington, J. E., et al. (2010). The Pfam protein families database. Nucleic Acids Res. 38, 11–22. doi: 10.1093/nar/gkp985
Gal, T. Z., Glazer, I., and Koltai, H. (2004). An LEA group 3 family member is involved in survival of C. elegans during exposure to stress. FEBS Lett. 577, 21–26. doi: 10.1016/j.febslet.2004.09.049
Gao, J., and Lan, T. (2016). Functional characterization of the late embryogenesis abundant (LEA) protein gene family from Pinus tabuliformis (Pinaceae) in Escherichia Coli. Nat. Publ. Gr. Nat. Publ. Group. 6, 1–10. doi: 10.1038/srep19467
Gao, W., Huang, X. L., and Tao, Y. (2016). A critical review of NanoSIMS in analysis of microbial metabolic activities at single cell level. Crit. Rev. Biotechnol. 36, 884–890. doi: 10.3109/07388551.2015.1057550
Garay-Arroyo, A., Colmenero-Flores, J. M., Garciarrubio, A., and Covarrubias, A. A. (2000). Highly hydrophilic proteins in prokaryotes and eukaryotes are common during conditions of water deficit. J. Biol. Chem. 275, 5668–5674. doi: 10.1074/jbc.275.8.5668
Gasteiger, E., Gattiker, A., Hoogland, C., Ivanyi, I., Appel, R. D., and Bairoch, A. (2003). ExPASy: the proteomics server for in-depth protein knowledge and analysis. Nucleic Acids Res. 31, 3784–3788. doi: 10.1093/nar/gkg563
Guindon, S., and Gascuel, O. (2003). A simple, fast, and accurate algorithm to estimate large phylogenies by maximum likelihood. Syst. Biol. 52, 696–704.
Hand, S. C., Menze, M. A., Toner, M., Boswell, L., and Moore, D. (2011). LEA proteins during water stress: not just for plants anymore. Annu. Rev. Physiol. 73, 115–134. doi: 10.1146/annurev-physiol-012110-142203
Hanin, M., Brini, F., Ebel, C., Toda, Y., Takeda, S., and Masmoudi, K. (2011). Plant dehydrins and stress tolerance: versatile proteins for complex mechanisms. Plant Signal. Behav. 6, 1503–1509. doi: 10.4161/psb.6.10.17088
HanumanthaRao, B., Nair, R. M., and Nayyar, H. (2016). Salinity and high temperature tolerance in mungbean from a physiological perspective. Front. Plant Sci. 7:957. doi: 10.3389/fpls.2016.00957
Hincha, D. K., and Thalhammer, A. (2012). LEA proteins: IDPs with versatile functions in cellular dehydration tolerance. Biochem. Soc. 40, 1000–1003. doi: 10.1042/BST20120109
Hirayama, T., and Shinozaki, K. (2010). Research on plant abiotic stress responses in the post-genome era: past, present and future. Plant J. 61, 1041–1052. doi: 10.1111/j.1365-313X.2010.04124.x
Hu, B., Jin, J., Guo, A. Y., Zhang, H., Luo, J., and Gao, G. (2015). GSDS 2.0: an upgraded gene feature visualization server. Bioinformatics 31, 1296–1297. doi: 10.1093/bioinformatics/btu817
Hunault, G., and Jaspard, E. (2010). LEAPdb: a database for the late embryogenesis abundant proteins. BMC Genomics 11:221. doi: 10.1186/1471-2164-11-221
Hundertmark, M., and Hincha, D. K. (2008). LEA. (Late embryogenesis abundant) proteins and their encoding genes in Arabidopsis thaliana. BMC Genomics 9:118. doi: 10.1186/1471-2164-9-118
İbrahime, M., Kibar, U., Kazan, K., Ozmen, C. Y., Mutaf, F., Asci, S. D., et al. (2019). Genome-wide identification of the LEA protein gene family in grapevine (Vitis vinifera L.). Tree Genet. Genomes 15:55. doi: 10.1007/s11295-019-1364-3
Jin, X., Cao, D., Wang, Z., Ma, L., Tian, K., Liu, Y., et al. (2019). Genome-wide identification and expression analyses of the LEA protein gene family in tea plant reveal their involvement in seed development and abiotic stress responses. Sci. Rep. 9:14123. doi: 10.1038/s41598-019-50645-8
Kang, J. Y., Kim, S. K., Kim, M. Y., Lestari, P., Kim, K. H., Ha, B.-K., et al. (2014). Genome sequence of mungbean and insights into evolution within Vigna species. Nat. Commun. 5:5443. doi: 10.1038/ncomms6443
Kang, Y. J., Satyawan, D., Shim, S., Lee, T., Lee, J., Hwang, W. J., et al. (2015). Draft genome sequence of adzuki bean, Vigna angularis. Sci. Rep. 5:8069. doi: 10.1038/srep08069
Kaur, R., Bains, T. S., Bindumadhava, H., and Nair, H. (2015). Responses of mungbean genotypes to heat stress: effects on reproductive biology, leaf function and yield traits. Sci. Hortic. 197, 527–541. doi: 10.1016/j.scienta.2015.10.015
Krueger, C., Berkowitz, O., Stephan, U. W., and Hell, R. (2002). A metal-binding member of the late embryogenesis abundant protein family transports iron in the phloem of Ricinus communis L. J. Biol. Chem. 277, 25062–25069. doi: 10.1074/jbc.M201896200
Kumar, C., Mishra, S. B., and Nilanjaya Singh, C. M. (2017). Investigating morpho-physiological traits and agrometeorological indices in green gram for terminal heat tolerance. Legume Res. 40, 271–276.
Kumar, M., Busch, W., Birke, H., Kemmerling, B., Nurnberger, T., and Schoff, F. (2009). Heat shock factors HsfB1 and HsfB2are involved in the regulation of Pdf1.2 expression and pathogen resistance in Arabodopsis. Mol. Plant 2, 152–165. doi: 10.1093/mp/ssn095
Kumar, C., Mishra, S. B., and Nilanjay Singh, C. M. (2016). Evaluation of selection indices for improving terminal heat tolerance in greengram (Vignaradiata L. Wilczek). J. Agrometeorol. 18, 216–221.
Kumar, R., Singh, C. M., Arya, M., Kumar, R., Mishra, S. B., Singh, U. K., et al. (2020). Investigating stress indices to discriminate the physiologically efficient heat tolerant genotypes of mungbean [Vigna radiata (L.) Wilczek]. Legume Res. 43, 43–49.
Kumari, P., and Varma, S. K. (1983). Genotypic differences in flower production/shedding and yield in mungbean (Vigna radiata). Indian J. Plant Physiol. 26, 402–405.
Lan, T., Gao, J., and Zeng, Q. Y. (2013). Genome-wide analysis of the LEA (late embryogenesis abundant) protein gene family in Populus trichocarpa. Tree Genet. Genomes 9, 253–264.
Li, X., and Cao, J. (2016). Late embryogenesis abundant (LEA) gene family in maize: identification, evolution, and expression profiles. Plant Mol. Biol. Rep. 34, 15–28. doi: 10.1007/s11105-015-0901-y
Liang, Y., Xiong, Z., Zheng, J., Xu, D., Zhu, Z., Xiang, J., et al. (2016). Genome-wide identification, structural analysis and new insights into late embryogenesis abundant (LEA) gene family formation pattern in Brassica napus. Sci. Rep. 6:24265. doi: 10.1038/srep24265
Ling, H., Zeng, X., and Guo, S. X. (2016). Functional insights into the late embryogenesis abundant (LEA) protein family from Dendrobium ofcinale (Orchidaceae) using an Escherichia coli system. Sci. Rep. 6:39693. doi: 10.1038/srep39693
Liu, H., Xing, M., Yang, W., Mu, X., Wang, X., Lu, F., et al. (2019). Genome-wide identification of and functional insights into the late embryogenesis abundant (LEA) gene family in bread wheat (Triticum aestivum). Sci. Rep. 9:13375. doi: 10.1038/s41598-019-49759-w
Liu, P. L., Huang, Y., Shi, P. H., Yu, M., Xie, J. B., and Xie, L. (2018). Duplication and diversification of lectin receptor-like kinases (LecRLK) genes in soybean. Sci. Rep. 8:5861. doi: 10.1038/s41598-018-24266-6
Livak, K. J., and Schmittgen, T. D. (2001). Analysis of relative gene expression data using real-time quantitative PCR and the 2−ΔΔCT method. Methods 25, 402–408.
Lonardi, S., Munoz-Amatria, M., Liang, Q., Shu, S., Wanamaker, S. I., Lo, S., et al. (2019). The genome of cowpea (Vigna unguiculata (L.) Walp.). Plant J. 98, 767–782.
Magwanga, R. O., Lu, P., Kirungu, J. N., Lu, H., Wang, X., Cai, X., et al. (2018). Characterization of the late embryogenesis abundant (LEA) proteins family and their role in drought stress tolerance in upland cotton. BMC Genet. 19:6. doi: 10.1186/s12863-017-0596-1
Manivannan, N., and Mahalingam, A. (2021). “Cowpea,” in The Beans and the Peas: From Orphan to Mainstream Crops, eds A. Pratap and S. Gupta (Dufford: Wood Head Publishing Elsevier), 241–254.
Muvunyi, B. P., Yan, Q., Wu, F., Min, X., Yan, Z. Z., Kanzana, G., et al. (2018). Mining late embryogenesis abundant (LEA) family genes in Cleistogenes songorica, a xerophyte perennial desert plant. Int. J. Mol. Sci. 19:3430. doi: 10.3390/ijms19113430
Pratap, A., Basu, P. S., Gupta, S., Malviya, N., Rajan, N., Tomar, R., et al. (2014). Identification and characterization of sources for photo- and thermo-insensitivity in Vigna species. Plant Breed. 133, 756–764. doi: 10.1111/pbr.12215
Pratap, A., Douglas, C., Prajapati, U., Kumari, G., War, A. R., Tomar, R., et al. (2020). “Breeding progress and future challenges: biotic stresses,” in The Mungbean Genome, Compendium of Plant Genomes, eds R. Nair, R. Schafleitner, and S. H. Lee (Cham: Springer), 55–80. doi: 10.1007/978-3-030-20008-4_5
Pratap, A., Gupta, S., Rathore, M., Basavraj, T. S., Singh, C. M., Prajapati, U., et al. (2021). “Mungbean,” in The Beans and the Peas: From Orphan to Mainstream Crops, eds A. Pratap and S. Gupta (Dufford: Wood Head Publishing Elsevier), 1–32.
Pratap, A., Gupta, S., Basu, P. S., Tomar, R., Dubey, S., Rathore, M., et al. (2019). “Towards development of climate smart mungbean: challenges and opportunities,” in Genomic Designing of Climate Smart Pulse Crops, ed. C. Kole (Cham: Springer), 235–264.
Rahman, L. N., Smith, G. S. T., Bamm, V. V., Voyer-Grant, J. A. M., Moffatt, B. A., Dutcher, J. R., et al. (2011). Phosphorylation of Thellungiella salsuginea dehydrins TsDHN-1 and TsDHN-2 facilitates cation-induced conformational changes and actin assembly. Biochemistry-US 50, 9587–9604. doi: 10.1021/bi201205m
Rosales, R., Romero, I., Escribano, M. I., Merodio, C., and Sanchez-Ballesta, M. T. (2014). The crucial role of Phi- and K-segments in the in vitro functionality of Vitis vinifera dehydrin DHN1a. Phytochemistry 108, 17–25.
Sehrawat, N., Yadav, M., Bhat, K. V., Sairam, R. K., and Jaiwal, P. K. (2015). Effect of salinity stress on mungbean [Vigna radiata (L.) Wilczek] during consecutive summer and spring seasons. J. Agric. Sci. 60, 23–32.
Sharma, L., Priya, M., Bindumadhava, H., Nair, R. M., and Nayyar, H. (2016). Influence of high temperature stress on growth, phenology and yield performance of mungbean (Vigna radiata (L.) Wilczek) under managed growth conditions. Sci. Hortic. 213, 379–391.
Singh, A. K., Velmurugan, A., Gupta, D. S., Kumar, J., Kesari, R., Konda, A., et al. (2019b). Draft genome sequence of a less-known wild Vigna: beach pea (V. marina cv. ANBp-14-03). Crop J. 7, 660–666.
Singh, C. M., Mishra, S. B., Pandey, A., and Arya, M. (2014). Eberhart-Russell’ and AMMI approaches of genotype by environment interaction (GEI) for yield and yield component traits in Vigna radiata (L.) Wilczek. Int. J. Agric. Environ. Biotech. 7, 272–292.
Singh, C. M., Singh, P., Pratap, A., Pandey, R., Purwar, S., and Vibha, et al. (2019a). Breeding for enhancing Legumovirus resistance in mungbean: current understanding and future directions. Agronomy 9:622. doi: 10.3390/agronomy9100622
Singh, C. M., Singh, P., Tiwari, C., Purwar, S., Kumar, M., Pratap, A., et al. (2021). Improving drought tolerance in Mungbean (Vigna radiata L. Wilczek): morpho-physiological, biochemical and molecular Perspectives. Agronomy 11:1534. doi: 10.3390/agronomy11081534
Singh, D. P., Singh, B. B., and Pratap, A. (2017). Genetic improvement of Mungbean and Urdbean and their role in enhancing pulse production in India. Indian J. Genet. 76, 550–567.
Souframanien, J., Raizada, A., Dhanasekar, P., and Suprasanna, P. (2020). Draft genome sequence of the pulse crop blackgram [Vigna mungo (L.) Hepper] reveals potential R-genes. bioRxiv [preprint]. doi: 10.1101/2020.06.21.163923
Takahashi, Y., Sakai, H., Yoshitsu, Y., Muto, C., Anai, T., Pandiyan, M., et al. (2019). Domesticating Vigna stipulacea: a potential legume crop with broad resistance to biotic stresses. Front. Plant Sci. 10:1607. doi: 10.3389/fpls.2019.01607
Tamura, K., Stecher, G., Peterson, D., Filipski, A., and Kumar, S. (2013). MEGA6: molecular evolutionary genetics analysis, version 6.0. Mol. Biol. Evol. 30, 2725–2729.
Thompson, J. D., Gibson, T., and Higgins, D. G. (2002). Multiple sequence alignment using ClustalW and ClustalX. Curr. Protoc. Bioinformatics Chapter 2:Unit2.3. doi: 10.1002/0471250953.bi0203s00
Tolleter, D., Hincha, D. K., and Macherel, D. (2010). A mitochondrial late embryogenesis abundant protein stabilizes model membranes in the dry state. Biochim. Biophys. Acta Biomembranes 10:1926. doi: 10.1016/j.bbamem.2010.06.029
Tzudir, L., Bera, P. S., and Chakraborty, P. K. (2014). Impact of temperature on the reproductive development in mungbean (Vigna radiata) varieties under different dates of sowing. Int. J. Bioresour. Stress Manage. 5, 194–199. doi: 10.5958/0976-4038.2014.00555.7
Voorrips, R. E. (2002). Mapchart: sofware for the graphical presentation of linkage maps and QTLs. J. Hered. 93, 77–78. doi: 10.1093/jhered/93.1.77
Wang, X.-S., Zhu, H.-B., Jin, G.-L., Liu, H.-L., Wu, W.-R., and Zhu, J. (2007). Genome-scale identification and analysis of LEA genes in rice (Oryza sativa L.). Plant Sci. 172, 414–420. doi: 10.1016/j.plantsci.2006.10.004
Wassmann, R., Jagadish, S., Sumfleth, K., Pathak, H., Howell, G., and Ismail, A. (2009). Regional vulnerability of climate change impacts on Asian rice production and scope for adaptation. Adv. Agron. 102, 91–133. doi: 10.1016/S0065-2113(09)01003-7
Wu, C., Hu, W., Yan, Y., Tie, W., Ding, Z., Guo, J., et al. (2018). The late embryogenesis abundant protein family in cassava (Manihot esculenta Crantz): genome-wide characterization and expression during abiotic stress. Molecules 23:1196. doi: 10.3390/molecules23051196
Xiong, L., and Zhu, J. K. (2002). Molecular and genetic aspects of plant responses to osmotic stress. Plant Cell Environ. 25, 131–139. doi: 10.1046/j.1365-3040.2002.00782.x
Yu, X., Yue, W., Yang, Q., Zhang, Y., Han, X., Yang, F., et al. (2019). Identification of the LEA family members from Caragana korshinskii (Fabaceae) and functional characterization of CkLEA2–3 in response to abiotic stress in Arabidopsis. Braz. J. Bot. 42, 227–238.
Keywords: abiotic stress, candidate genes, expression analysis, heat stress, mung bean, wild Vigna, LEA genes
Citation: Singh CM, Kumar M, Pratap A, Tripathi A, Singh S, Mishra A, Kumar H, Nair RM and Singh NP (2022) Genome-Wide Analysis of Late Embryogenesis Abundant Protein Gene Family in Vigna Species and Expression of VrLEA Encoding Genes in Vigna glabrescens Reveal Its Role in Heat Tolerance. Front. Plant Sci. 13:843107. doi: 10.3389/fpls.2022.843107
Received: 24 December 2021; Accepted: 02 February 2022;
Published: 22 March 2022.
Edited by:
Rajeev K. Varshney, International Crops Research Institute for the Semi-Arid Tropics (ICRISAT), IndiaReviewed by:
Harsh Nayyar, Panjab University, IndiaMonika Dalal, ICAR-NIPB, India
Charu Lata, National Institute of Science Communication and Information Resources (CSIR), India
Copyright © 2022 Singh, Kumar, Pratap, Tripathi, Singh, Mishra, Kumar, Nair and Singh. This is an open-access article distributed under the terms of the Creative Commons Attribution License (CC BY). The use, distribution or reproduction in other forums is permitted, provided the original author(s) and the copyright owner(s) are credited and that the original publication in this journal is cited, in accordance with accepted academic practice. No use, distribution or reproduction is permitted which does not comply with these terms.
*Correspondence: Chandra Mohan Singh, Y21zaW5naC5ncGJAZ21haWwuY29t; orcid.org/0000-0002-3857-7361; Aditya Pratap, QWRpdHlhLlByYXRhcEBpY2FyLmdvdi5pbg==; orcid.org/0000-0001-7280-0953