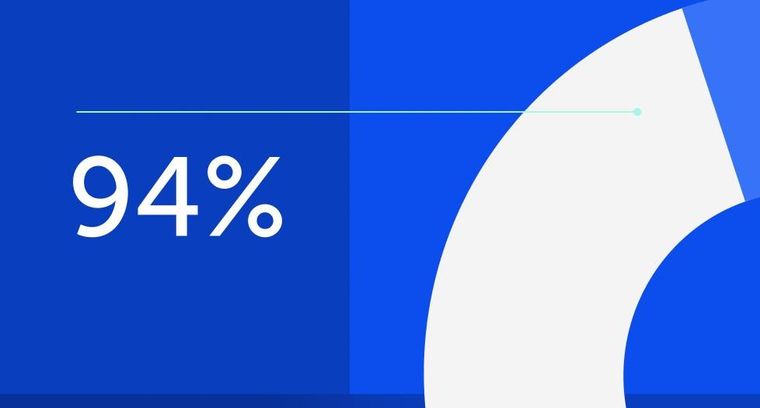
94% of researchers rate our articles as excellent or good
Learn more about the work of our research integrity team to safeguard the quality of each article we publish.
Find out more
ORIGINAL RESEARCH article
Front. Plant Sci., 27 April 2022
Sec. Crop and Product Physiology
Volume 13 - 2022 | https://doi.org/10.3389/fpls.2022.842317
This article is part of the Research TopicMetabolic and Molecular Responses of Fruit and Vegetables to Hypoxic Atmospheric ConditionsView all 5 articles
High CO2 (> 20 kPa) conditions are beneficial for suppressing spoilage caused by Botrytis cinerea in strawberry fruit; however, these conditions are often accompanied by discoloration, off-flavors, and faster softening. Stepwise increments of CO2 concentrations have been proposed to alleviate injuries in fruits caused by high CO2. In this study, we investigated whether stepwise increments of CO2, up to 30 kPa and under a reduced O2 concentration, are beneficial for reducing fungal spoilage without inducing CO2 injury symptoms in strawberry fruit. Based on recommended settings (5–10 kPa O2 with 15–20 kPa CO2), we first selected optimal O2 and CO2 concentrations that best-reduced spoilage caused by B. cinerea in red ripe “Sonsation” strawberry fruit. We found that higher O2 (10 kPa) and CO2 (20 kPa) concentrations were most beneficial for prolonging strawberry fruit shelf life. Subsequently, we studied the performance of red ripe “Arabella” strawberry fruit stored at 5°C under different controlled atmosphere (CA) conditions (10 kPa O2 with either 0, 20, or 30 kPa CO2). The CO2 concentrations were achieved either within 8 h or in a stepwise manner within the first 4 days of storage. As a control, 21 kPa O2 and 0 kPa CO2 were used. Following storage for up to 11 days, the spoilage incidence was assessed at 12°C for 5 days. The application of high CO2 (20 and 30 kPa) combined with 10 kPa O2 greatly suppressed fruit spoilage during storage and subsequent shelf life. High CO2 suppressed respiration as well as maintained a higher pH and firmness in treated fruit. The level of total sugars did not change, but during storage, a substantial part of sucrose was converted into glucose and fructose, especially under high CO2 conditions. High CO2 did not affect ascorbic acid and anthocyanin levels. The stepwise increments of CO2 did not result in beneficial effects compared to the static application of high CO2. Our results show that “Arabella” strawberry fruit are highly tolerant to elevated CO2 and can be stored under 30 kPa CO2 to prolong the shelf life.
Strawberry fruit are highly appreciated due to their desired quality characteristics, such as “heart shape,” shiny skin, full red color, sweetness, distinct aroma, and abundant nutritional compounds (Madrid, 2020). However, high perishability limits the shelf life of strawberry fruit. Among pathogens, the ubiquitous fungi Botrytis cinerea caused the most losses in strawberry production (Petrasch et al., 2019), particularly in ripe fruit during postharvest storage. The strategy of combining low temperature (LT) and controlled atmosphere (CA, i.e., high CO2 and low O2 concentrations) has been widely used in the supply chain to retain product quality for market demand. For strawberry fruit, a postharvest condition of 5–10 kPa O2 and 15–20 kPa CO2 at 0–5°C is recommended to extend the storage period (Kader, 2003). The suggested concentrations of O2 (5–10 kPa) decreased respiration in strawberry fruit without inducing fermentation, thereby extending the storage life (Kanellis et al., 2009). Similarly, high CO2 concentrations between 15 and 20 kPa further suppressed respiration, delayed ripening, and senescence (Li et al., 2019) and may affect the acidity of strawberry fruit (Rama and Narasimham, 2003). In addition, high CO2 concentrations are fungistatic to B. cinerea since the fungus did not grow above 30 kPa CO2 (Garcia-Gimeno et al., 2002). However, high CO2 concentrations, above 20 kPa, may also accelerate strawberry softening and impair coloration, taste, and antioxidant levels (Wszelaki and Mitcham, 2000; Nakata and Izumi, 2020), thereby reducing fruit’s commercial value.
Antioxidants such as ascorbic acid and anthocyanins may play a role in protecting fruit from fungal infection. Ascorbic acid showed inhibitory effects on B. cinerea growth in apple fruit (Davey et al., 2007; Bui et al., 2019) and tomato fruit (Bassolino et al., 2013; Zhang et al., 2013). High CO2 concentrations may cause the oxidation of ascorbic acid or inhibit the reduction of dehydroascorbic acid. For instance, 10–30 kPa CO2 decreased ascorbic acid content in berry species, especially strawberry fruit (Agar et al., 1997; Shin et al., 2008). Similarly, the application of 20–40 kPa CO2 to “Selva” strawberry fruit reduced fungal spoilage but induced discoloration and anthocyanin reduction, which was presumably caused by an increase in pH or a decrease in co-pigmentation with flavonols and other phenolics (Gil et al., 1997; Nakata and Izumi, 2020).
Soluble sugars and non-voltile organic acids contribute to the sweetness and acidity of fruit (Schwieterman et al., 2014), and these compounds are also substrates for respiratory metabolism (Saltveit, 2019). Soluble sugar content and acidity of “Camarosa” strawberry fruit declined after a 3-day 20 kPa CO2 treatment when compared to fruit under ambient atmosphere (Bodelón et al., 2010). High CO2 concentrations may also lead to the production of fermentative volatile compounds. The application of ambient oxygen combined with 20 kPa CO2 to “Camarosa” strawberry fruit resulted in a shift in the synthesis of methyl-to-ethyl esters, contributing to off-flavors (Pelayo-Zaldívar et al., 2007).
A novel CA strategy has been proposed recently to extend blueberry shelf life: a stepwise increment of CO2 concentration at the start of CA storage (Falagán et al., 2020). A stepwise increment of CO2, reaching 10 kPa CO2 in 3 or 7 days (under 5 kPa O2), was applied. This resulted in a lower CO2 production peak, a reduced spoilage incidence, higher firmness, and higher ascorbic acid levels compared to static CA (5 kPa O2 and 10 kPa CO2) or ambient atmosphere conditions.
In this study, we aimed to investigate if stepwise increments of high CO2 extend strawberry shelf life without damaging important quality characteristics such as firmness, color, and nutrient content. We first determined the spoilage incidence by B. cinerea in strawberry fruit as a function of O2 and CO2 concentrations, which were based on recommended settings (Kader, 2003). High concentrations of both O2 (10 kPa) and CO2 (20 kPa) resulted in lower spoilage incidences and similar levels of firmness and sweetness compared to the other tested O2 and CO2 concentrations. In a second experiment, we investigated the effects of both static and stepwise increments of CO2 concentrations, up to 20 and 30 kPa, on the spoilage incidence, nutritional compounds, and antioxidant content during shelf life. The highest CO2 level, 30 kPa, considerably lowered the spoilage incidence and respiration rate without causing any nutrient or firmness loss in strawberry fruit. However, stepwise increments of CO2 concentrations did not provide any additional benefits compared to static CA storage at 20 or 30 kPa CO2.
Strawberry fruit (Fragaria × ananassa cv. Sonsation) were harvested in November 2019 from a greenhouse located in Dongen, Netherlands. Fruit were stored at 4°C overnight and were transported to the lab at Wageningen University and Research the next day (day 0). Red ripe fruit (with calyxes attached) without mechanical damage or visible spoilage were collected into round plastic cups (ø 10 cm × H 5 cm) and covered with a transparent lid. Each lid had nine small holes to allow sufficient gas diffusion. A total of 150 cups were packed, and the initial weight of all the cups with fruit was individually recorded. Six cups were used for chemical analyses on day 0. The remaining 144 cups were divided into cups of 24 and distributed over six CA treatments, resulting in three blocks per treatment. Six 70-L stainless steel CA containers were used. The CA containers were connected to a flow-through system flushing humidified gas mixture at a flow rate of 250 ml min–1 for the duration of the experiment. Strawberry fruit were subjected to the following CA treatments (balanced with N2 in all treatments):
(i) Static 10 O2 kPa + 10 CO2 kPa;
(ii) Static 5 O2 kPa + 15 CO2 kPa;
(iii) Static 7.5 O2 kPa + 15 CO2 kPa;
(iv) Static 10 O2 kPa + 15 CO2 kPa;
(v) Static 10 O2 kPa + 20 CO2 kPa;
(vi) Control: Static 20 O2 kPa + 0 CO2 kPa.
The set points for CA treatments were reached within 5–8 h. Fruit were treated for 0, 3, 6, 9, and 12 days under different CA conditions at 5°C and ∼100% relative humidity. Following CA storage, the fruit were held for 3 days under an ambient atmosphere at 12°C and ∼95% relative humidity. The CA containers were opened and closed within 2 min when strawberry fruit had to be measured for minimal disturbance of the atmosphere. Six cups per CA container per time point were sampled for measurements. Spoilage incidence, weight, firmness, and soluble solids content (Brix) were measured immediately after the CA treatments (3 cups per treatment) and after 3 days of shelf life at ambient temperature (3 cups per treatment).
Strawberry fruit (Fragaria × ananassa cv. Arabella) were grown in an open field tabletop system in Genderen, Netherlands, and harvested in August 2020. Fruit were cooled down for several hours before being transported to the lab at Wageningen University and Research (day 0). Red ripe fruit (with calyx still attached) without mechanical damage or visible spoilage were selected and collected into paper punnets with approximately 25 fruit per punnet. A total of 98 punnets were packed and individually weighed. Two punnets were used for chemical analyses on day 0. The remaining 96 punnets were divided into punnets of eight and distributed over six CA treatments, resulting in two blocks per treatment. Twelve 70-L stainless steel CA containers were used, and two for each treatment. The CA containers were connected to a flow-through system flushing humidified gas mixture at a flow rate of 250 ml min–1 for the duration of the experiment. Strawberry fruit were subjected to the following CA treatments (balanced with N2 in all treatments):
(i) GCA30: 10 kPa O2 with a stepwise increment to 30 kPa CO2 (7.5 kPa per day in 4 days);
(ii) GCA20: 10 kPa O2 with a stepwise increment to 20 kPa CO2 (5 kPa per day in 4 days);
(iii) CA30: Static10 kPa O2 + 30 kPa CO2;
(iv) CA20: Static10 kPa O2 + 20 kPa CO2;
(v) CA0: Static 10 kPa O2 + 0 kPa CO2;
(vi) Control: Static 20 O2 kPa + 0 CO2 kPa.
The set points for all CA treatments were reached within 5–8 h. Fruit were stored for 11 days under different CA conditions at 5°C and ∼100% relative humidity; thereafter, they were held for 5 days under an ambient atmosphere of 12°C and ∼95% relative humidity (shelf life). The CA containers were opened and closed within 2 min when strawberry fruit were removed for measurements. One individual CA unit (regarded as one replication) contained 8 punnets as described above. During the CA treatment, fruit were sampled on days 4 and 11; during the subsequent shelf life, fruit were sampled on day 3 (total period 14 days) and day 5 (total period 16 days). At each sampling point, one punnet (approximately 25 fruit) per treatment was randomly taken for quality analyses, including fresh weight, firmness, and biochemical compounds. Another 4 punnets (approximately 100 fruit) were used for non-destructive spoilage incidence evaluation on day 11 of CA storage and days 3 and 5 of shelf life.
Both “Sonsation” and “Arabella” strawberry fruit are commercially used with good firmness, medium red color, good tolerance to B. cinerea, and prolonged shelf life during storage. “Sonsation” is a short-day cultivar, whereas “Arabella” has the potential to crop over an extended season. Since “Sonsation” fruit were no longer available in July 2020, we chose a very similar cultivar for Experiment II.
In Experiment II, the respiration rate of the fruit under different CA conditions was monitored. For this, the outlet of each CA container was connected to a 2 L glass cuvette filled with approximately 600–750 g of fruit. In this way, the cuvettes received the same gaseous conditions as the other stored fruit. At the start of the respiration measurement, the glass cuvettes were disconnected from the flow-through system and all valves were closed. Concentrations of O2 and CO2 were measured immediately after closure and again after about 6 h of incubation; from this, the respiration rate was calculated. The calculation considered the O2 consumption rate, cuvette volume, and fruit mass; the results were expressed in nmol O2 kg–1 s–1. CO2 and O2 were measured using a headspace gas analyzer (Checkmate 3, PBI Dansensor, Ringstead, Denmark).
Fruit showed typical B. cinerea infection symptoms (e.g., brown spots or visible gray mycelium). Only fruit showing Botrytis rot symptoms (Feliziani and Romanazzi, 2016; Petrasch et al., 2019) were considered for spoilage calculation. Botrytis rot was the pivotal fungal pathogen throughout the experiment. Occasionally, some other fungal symptoms were observed, such as Rhizopus fruit rot, and these were not considered in the calculation. The spoilage incidence was expressed as the percentage of fruit per block affected by a fungal infection. The weight of an individual cup or punnet with fruit was recorded using an MS6002TS balance (Mettler-Toledo GmbH, Giessen, Germany) at harvest. Weight loss was expressed as the percentage of weight loss compared to the initial fruit weight, corrected for the weight of cups or punnets.
In Experiment I, firmness (limited compression) was measured using FirmTech FT7 (UP GmbH, Ibbenbüren, Germany). Firmness was expressed as the force-displacement (in g mm–1) after about 1 mm of compression of the fruit shoulder without a calyx. Each replicate (cup) had 5 fruit. The same fruit were used for Brix measurement using a refractometer (PAL-1, Atago, Japan).
In Experiment II, fruit firmness (penetration) was measured using a universal testing machine (Zwick Z2.5/TS1S materials testing machine; Ulm, Germany). Fruit were cut in half lengthwise and compressed at the equator with a probe (ø 2.5 mm) at a constant plunger speed (2.5 mm s–1) to a fixed penetration depth (5 mm). The maximum force (N) was recorded during the penetration of the fruit piece. Each replicate (punnet) had 15 strawberry fruit.
Fruit from one punnet (approximately 25 fruit) were cut into small pieces, immediately frozen in liquid N2, and ground into powder. Part of the frozen powder was freeze-dried for sugar and organic acid extraction and also for expressing all nutrients as per gram dry weight. Frozen powder (0.3 g) was dissolved in 1.5 ml of water, thoroughly shaken, and centrifuged at 21,000 × g at 20°C for 15 min. The supernatant was used for pH measurement.
Frozen powder (0.2 g) was thawed on ice in darkness with 1 ml of ice-cold 3.3% meta-phosphoric acid. Samples were sonicated for 10 min, then centrifuged at 21,000 × g at 4°C for 10 min. The supernatant was filtered through a 0.45 μm cellulose filter and injected into an HPLC system consisting of a GS50 pump (Dionex), a 340S UV-VIS detector (Dionex), and a MIDAS autosampler (Spark Holland) equipped with a ProntoSIL 120-3 C18 AQ, 250 mm × 3 mm column (Knauer). The column was eluted with 400 μl L–1 H3PO4 + 2.5 ml L–1 MeOH + 0.1 mM EDTA in distilled water, followed by a wash step with 30% acetonitrile in distilled water at a flow rate of 0.35 ml min–1 at 35°C. Ascorbic acid was detected at 243 nm. The system was calibrated using an authentic ascorbic acid standard (Acros Organics) prepared at 3.3% MPA.
The frozen sample (0.3 g) was mixed with 1.5 ml of 50% methanol containing 1% formic acid extraction solvent. The supernatant was filtered through a 0.45 μm cellulose filter prior to injection into an HPLC system (Ultimate 3000, Dionex, Sunnyvale, CA, United States). Solvents used were (A) 0.1% trifluoroacetic acid in distilled water, and (B) 0.1% trifluoroacetic acid in HPLC-grade acetonitrile, establishing the following gradient: 5–28% of B for 0–35 min, 28–75% of B for 35–37 min, isocratic 75% of B for 37–40 min, 75–5% of B for 40–42 min, and isocratic 5% of B for 42–50 min, using a flow rate of 0.8 ml min–1. Separation was achieved using a C18 column (150 mm × 3 mm, 3 μm; HyPURITY C18, Thermo Scientific, New York, NY, United States), and peaks were identified using a UV/vis detector at 520 nm using pelargonidin 3-glucoside as an authentic standard (Extrasynthese).
A total of 15 mg of freeze-dried tissue was mixed with 5 ml of 75% ethanol, followed by shaking and incubating in a water bath at 80°C for 20 min before centrifuging at 8,500 × g for 5 min at 4°C. A vacuum centrifuge (Savant SpeedVac SPD2010, Thermo Fisher Scientific, Germany) was used to dry 1 ml of the supernatant at 55°C for 2.5 h. The pellet was resuspended in 1 ml of distilled water and sonicated for 10 min, followed by centrifugation for 10 min at 4°C at 14,800 × g. Before analysis, the supernatant was diluted with distilled water 50 times for sugar and 5 times for organic acids.
Soluble sugar measurements were carried out using a High-Performance Anion Exchange Chromatography with Pulsed Amperometric Detection (HPAEC-PAD; Dionex ICS5000, Thermo Fisher Scientific, Germany) equipped with a CarboPac1 column (250 mm × 2 mm) eluted with 100 mM NaOH at a flow rate of 0.25 ml min–1 at 25°C. Quantification was performed using glucose, fructose, and sucrose standards from Sigma-Aldrich.
Citric and malic acids in the extracts were analyzed using an IC system equipped with a Triathlon autosampler (Spark Holland), GS50 pump (Dionex), ED50A detector (Dionex) operating in the conductivity mode, and an ASRS ultra II 2 mm suppressor (Dionex). Anions were separated at 30°C on an IonPac AS11HC (250 mm × 2 mm) column (Dionex), using the following multistep gradients: 1 mM NaOH, 0 min; 1 mM NaOH, 1 min; 14 mM NaOH, 23 min; 30 mM NaOH, 31 min; 60 mM NaOH, 41 min at a flow rate of 0.38 ml min–1. Quantification was performed using authentic DL-malic acid and citric acid standards from Sigma-Aldrich.
Treatment effects on all measured variables were tested using a one-way analysis of variance (ANOVA) at each time point during the experimental period. Experiment I was carried out with 3 blocks, and Experiment II was carried out with 2 blocks. In each block, replicated fruit were measured as an individual or a pooled sample, depending on variables. An average value of each block was used for statistical analysis. Homogeneity and normality of residuals in the ANOVA were tested using Bartlett’s test and Shapiro–Wilk test, respectively. Fisher’s protected least significant difference (LSD) test was used as a post hoc test. All statistical analyses were performed in Genstat (19th edition, VSN International Ltd., Hemel Hempstead, United Kingdom). All tests were conducted at α = 0.05.
During CA storage, the spoilage incidence of CA-treated fruit was lower compared to control-treated fruit (Figure 1A). During shelf life, the spoilage incidence increased dramatically in fruit from the ambient atmosphere and 10 kPa CO2 + 10 kPa O2 (Figure 1B). Fruit stored at 15 kPa CO2 showed reduced spoilage incidence with increasing O2 concentrations from 5 to 10 kPa. Under conditions of 10 kPa O2, fruit treated with 15 and 20 kPa CO2 showed the lowest spoilage incidence among all treatments. Overall Brix values of fruit showed a decreasing trend during CA storage but did not show a further change during the 3-day subsequent shelf life; fruit firmness did not change during CA storage but showed a slight decrease during the 3-day subsequent shelf-life period. The different treatments had no significant effects on the changes of Brix values and the firmness of fruit (Supplementary Figure 1).
Figure 1. Spoilage incidence of “Sonsation” strawberry fruit stored under static controlled atmosphere (CA) conditions and shelf life. (A) Spoilage incidence at different time points during CA storage at 5°C and ∼100% relative humidity. (B) Spoilage incidence at different time points of CA storage followed by 3-day shelf life at 12°C and ∼95% relative humidity in ambient atmosphere. The first number is the number of days in CA storage, and the second number is the number of days in shelf life. As a control condition, 21 kPa O2 and 0 kPa CO2 were used. Data represent means of 3 blocks (n = 3) with five replicated fruit per block. The error bars represent the standard error of means. Different letters denote significant differences according to Fisher’s protected LSD test (α = 0.05).
The fruit respiration rate was higher in CA0 and the control conditions compared to the elevated CO2 treatments (20 and 30 kPa), indicating that increased CO2 concentrations suppressed respiration (Figure 2A). The respiration rate fluctuated during the first 5 days of storage, regardless of treatments. After day 9, the respiration rate started to increase. This increase was smaller and not significant for fruit stored under 30 kPa CO2 conditions. There were no apparent differences in respiration related to the immediate or stepwise application of high CO2 (20 and 30 kPa).
Figure 2. Respiration rate and spoilage incidence of “Arabella” strawberry fruit during CA storage and shelf life. (A) Respiration rate during CA storage at 5°C and ∼100% relative humidity. (B) Spoilage incidence during shelf life at 12°C and ∼95% relative humidity in ambient atmosphere. As a control condition, 21 kPa O2 and 0 kPa CO2 were used. Data represent means of 2 blocks (n = 2) with approximately 35 replicated fruit for respiration and approximately 25 replicated fruit for spoilage measurements per block. The error bars represent the standard error of means. Different letters denote significant differences according to Fisher’s protected LSD test (α = 0.05).
Fruit stored under CA0 and control conditions showed high spoilage incidence at the start of shelf life (Figure 2B). The spoilage incidence of CA0 overlapped with that of the control treatment, reaching about 58% spoilage at the end of the CA storage. In contrast, fruit stored under elevated CO2 with 10 kPa O2 treatments did not show any spoilage during the CA storage; during the subsequent shelf life at 12°C and ambient atmosphere, fruit spoilage did occur in these treatments. After the 3-day shelf life, fruit stored under CA30 had the lowest spoilage incidence (32%), followed by GCA30 (53%) and CA20 and GCA20 (over 60%). After 5 days of shelf life, the spoilage incidence was above 80% in all treatments.
During the CA storage and shelf life, the glucose content did not show a clear trend (Figure 3A), whereas the fructose content increased (Figure 3B), and the sucrose content decreased (Figure 3C). The total sugar content (glucose + fructose + sucrose) showed a downward trend for all treatments (Figure 3D). No apparent differences in the rate of change in individual sugars among treatments were observed. However, the sucrose content in fruit from both the static and stepwise 30 kPa CO2 treatments showed a more rapid decrease in the CA storage than the sucrose level in fruit from other treatments (Figure 3E). This indicates that under high CO2 conditions, there was a more pronounced conversion of sucrose into glucose and fructose.
Figure 3. Soluble sugars of “Arabella” strawberry fruit during CA storage and shelf life. (A) Glucose, (B) fructose, (C) sucrose, and (D) total sugar (sum of glucose, fructose, and sucrose) during CA storage at 5°C and ∼100% relative humidity and subsequent shelf life at 12°C and ∼95% relative humidity in ambient atmosphere. Data represent means of 2 blocks (n = 2; each block being a pooled sample of 20 replicated fruit). Different letters denote significant differences according to Fisher’s protected LSD test (α = 0.05). The error bars represent the standard error of means. (E) Correlation between the increased content of glucose and fructose and the decreased content of sucrose between day 0 and day 11 during CA storage. Data represent individual blocks. As a control condition, 21 kPa O2 and 0 kPa CO2 were used.
Fruit pH increased from about 3.2 to 3.7 with minimal differences between treatments (Figure 4A). Fruit from the CA30 condition generally showed a slightly higher pH than the control and CA0 treatments. Citric acid did not show any changes throughout the CA storage and shelf life, and no treatment effects were observed (Figure 4B). The malic acid content in fruit was 2.2-fold lower than citric acid at harvest and showed a slight decrease after fruit were transferred to the shelf life condition in all treatments. No differences with respect to the CA treatments were observed (Figure 4C). The increase in pH during the experimental period can be ascribed to the decrease in the two major acids; fruit pH during the CA storage was lower than during the subsequent shelf life, regardless of atmospheric composition. In addition, high CO2 treatments led to a higher pH compared to control and CA0 treatments after fruit were transferred to the ambient atmosphere (Figure 4D).
Figure 4. pH and main organic acids of “Arabella” strawberry fruit during CA storage and shelf life. (A) pH, (B) citric acid, and (C) malic acid during CA storage at 5°C and ∼100% relative humidity and subsequent shelf life at 12°C and ∼95% relative humidity in ambient atmosphere. Data represent means of 2 blocks (n = 2; each block being a pooled sample of 20 replicated fruit). The error bars represent the standard error of means. Different letters denote significant differences according to Fisher’s protected LSD test (α = 0.05). (D) Correlation between pH and sum of citric and malic acids of CA treatments during CA storage and subsequent shelf life. The closed black squares represent data of day 0. Data represent individual blocks. As a control condition, 21 kPa O2 and 0 kPa CO2 were used.
Softening was observed in fruit from control and CA0 treatments during the subsequent shelf life (Figure 5A). Fruit from high CO2 treatments (except for CA30) retained their firmness during the shelf life and no promising effect of the stepwise elevation of CO2 was observed. Fruit weight loss during the CA storage was minimal; in fact, fruit weight loss increased up to a maximum of 3% in the subsequent shelf life, and no differences were apparent between treatments (Figure 5B).
Figure 5. Firmness and weight loss of “Arabella” strawberry fruit during CA storage and shelf life. (A) Firmness and (B) weight loss during CA storage at 5°C and ∼100% relative humidity and subsequent shelf life at 12°C and ∼95% relative humidity in ambient atmosphere. Data represent means of 2 blocks (n = 2; each block being averaged of 20 replicated fruit). The error bars represent the standard error of means. Different letters denote significant differences according to Fisher’s protected LSD test (α = 0.05). As a control condition, 21 kPa O2 and 0 kPa CO2 were used.
Pelargonidin-3-glucoside and pelargonidin-3-malonyl glucoside were the major anthocyanins in “Arabella” strawberry fruit, and the content of the former was lower (∼75%) than the content of the latter at harvest (Figures 6A,B). Overall, pelargonidin-3-glucoside content in fruit increased during the CA storage with decreasing trends during shelf life. There were no consistent differences between treatments. Similar trends were observed in the pelargonidin-3-malonyl glucoside content of fruit. Ascorbic acid levels of “Arabella” fruit showed slightly increasing trends in fruit from control and CA0 treatments throughout CA storage and shelf life. In contrast, the ascorbic acid level did not change in fruit treated with elevated CO2 concentrations, where it was maintained around 10 mg g–1 DW (Figure 6C). Collectively, until the end of shelf life, the antioxidants of fruit showed similar levels compared to fruit at harvest.
Figure 6. Anthocyanin and ascorbic acid content of “Arabella” strawberry fruit during CA storage and shelf life. (A) Pelargonidin-3-malonyl glucoside, (B) pelargonidin-3-glucoside, and (C) ascorbic acid during CA storage at 5°C and ∼100% relative humidity and subsequent shelf life at 12°C and ∼95% relative humidity in ambient atmosphere. As a control condition, 21 kPa O2 and 0 kPa CO2 were used. Data represent means of 2 blocks (n = 2; each block being a pooled sample of 20 replicated fruit). The error bars represent the standard error of means. Different letters denote significant differences according to Fisher’s protected LSD test (α = 0.05).
Controlled atmosphere storage retains product quality via reducing O2 and increasing CO2 concentrations relative to the ambient atmosphere. Generally, the lower the O2 and the higher the CO2 concentrations, the more the storage life can be extended by delaying senescence and ripening of commodities (Rama and Narasimham, 2003). However, based on our observations, this concept may not fully apply to strawberry fruit. In Experiment I, moderately decreased O2 concentrations (5–10 kPa) combined with 15 kPa CO2 greatly decreased the spoilage incidence of “Sonsation” strawberry fruit, which was observed only after fruit were transferred to post-storage shelf life (Figure 1B). Notably, 10 kPa O2 clearly performed better than 5 or 7.5 kPa O2. Generally, when high CO2 is applied to strawberry fruit, the risk of initiating fermentation increases, which leads to the accumulation of acetaldehyde and ethanol, resulting in off-flavors (Rama and Narasimham, 2003; Kanellis et al., 2009). This could be prevented and/or attenuated by introducing more O2 into the environment. In contrast, Ke et al. (1991) found that decreasing concentrations of O2 (down to 0.25% O2 balanced with N2 only) led to reduced spoilage incidence in “Selva” strawberry fruit at both 0 and 5°C and did not affect the soluble solids content (brix), pH, or titratable acidity after 10-day CA treatments compared to air-treated fruit. However, this study also reported a higher ethanol content of fruit under this treatment compared to fruit from other treatments. It is unclear if these beneficial effects of ultralow O2 persisted during the shelf life, as authors only did quality measurements at the end of the storage period.
We observed that increased CO2 concentrations (10–20 kPa) combined with 10 kPa O2 drastically lowered the spoilage incidence of fruit. Fruit firmness and Brix did not exhibit differences during CA storage and shelf life among treatments (Supplementary Figure 1). Application of 20 kPa CO2 in air did not induce the fermentation of “Selva” strawberry fruit and ultimately resulted in a lower spoilage incidence (Ke et al., 1991). This shows that in order to benefit from CA storage, optimal O2 concentrations might not be lower than 10 kPa and CO2 should not be lower than 15 kPa.
Therefore, in Experiment II, we referred to the optimal 10 kPa O2 CA conditions for “Sonsation” strawberry fruit, which showed a lower spoilage incidence without affecting fruit firmness and Brix, and applied that in combination with different concentrations of CO2 to “Arabella” strawberry fruit. Both conditions with 10 kPa O2 and high (20 and 30 kPa) CO2 significantly suppressed spoilage of “Arabella” strawberry fruit. This confirms the results with “Sonsation” fruit, indicating the two cultivars may respond to high CO2 conditions similarly.
Notably, in Experiment II, the condition of 10 kPa O2 alone (Figure 2B, CA0) did not suppress the spoilage development compared to the control condition at the end of the CA storage. Combing the results of two experiments suggests that high CO2 combined with reduced O2 could be effective for reducing spoilage. When high CO2 is applied to strawberry fruit, the concentration of O2 should not be too low; otherwise, the additive effects of high CO2 and low O2 may induce fermentation.
CO2 concentrations higher than 30 kPa can cause faster softening, berry discoloration, and the production of off-flavors, leading to quality loss. Elevating CO2 concentrations in a stepwise manner may improve the adaption of products to high CO2 compared to static CO2 treatments such that the storage life, fruit firmness, color, nutritional compounds, and antioxidants are retained (Falagán et al., 2020). Raising gas concentrations over a 3- or 7-day period to reach final concentrations of 10 kPa CO2 and 5 kPa O2 resulted in a lower CO2 production peak and reduced spoilage incidence in blueberries when compared to static CA or ambient atmospheric conditions (Falagán et al., 2020).
In this study, the respiration rate (Experiment II) of fruit under high CO2 treatments was reduced throughout the storage period compared to fruit from low CO2 treatments (Figure 2A). However, the pattern was not affected by applying high CO2 in a stepwise manner. Similarly, the spoilage incidence was greatly suppressed by high CO2 but was not affected by the stepwise application. The suppressed respiration caused by high CO2 is due to the inhibition of succinate dehydrogenase that catalyzes the conversion of succinate to fumarate at the tricarboxylic acid (Kanellis et al., 2009). Malic acid, as the upstream metabolite of succinate and fumarate, was reduced by high CO2 (20 kPa CO2 in air) (Fernández-Trujillo et al., 1999; Ponce-Valadez et al., 2005). The observed decreasing trend of malic acid, especially in fruit treated with elevated CO2 (Figure 4C), is in line with previous findings.
In strawberry fruit, sucrose is degraded via the invertase pathway to form fructose and glucose (Kanellis et al., 2009; Durán-Soria et al., 2020). We found that fructose and glucose in strawberry fruit slightly increased during the experimental period in all CA treatments, whereas sucrose decreased. High CO2 treatments seemed to induce a more significant conversion from sucrose to glucose and, in particular, fructose (Figure 3E). Bang et al. (2019) also observed increases in glucose and fructose but a decrease in sucrose content in strawberry fruit after 1 day of treatment with 30 kPa CO2 at 25°C compared to the ambient atmosphere condition. The transcriptome analysis indicated that the invertase inhibitor was downregulated under 30 kPa CO2, triggering invertase activity; therefore, more glucose and fructose are synthesized in fruit.
A decreasing trend in firmness in control and CA0-treated fruit was observed, especially after the fruit were returned to the ambient atmosphere (Figure 5A). The firmness of fruit from high CO2 treatments was maintained at approximately the same value at the end of shelf life compared to the firmness at harvest. The different firmness behaviors between 0 kPa CO2 and high CO2 corroborate that CO2 treatments inhibit firmness loss of strawberry fruit in a non-reversible manner (Harker et al., 2000). Fruit treated with static 30 kPa CO2 tended to be softer compared to fruit from other high CO2 treatments. This could be due to the adverse effect of long-term storage under static 30 kPa CO2 as it was not observed under stepwise high CO2 treatments.
Increased firmness in strawberry fruit under elevated CO2 is likely due to reinforcement of cell-to-cell bonding, which is associated with the increase in pH of the apoplast (Harker et al., 2000). According to our finding, the pH of “Arabella” strawberry fruit increased throughout the whole experimental period due to a reduction in organic acids, especially malic acid (Supplementary Figure 2), which is in line with previous findings (Ke et al., 1991; Holcroft and Kader, 1999; Blanch et al., 2015). Both H+ and HCO3– are produced by the solubilization of CO2, which could affect pH (Lucas, 1979; Bown, 1985). The presence of H+ decreased pH, whereas the uptake of HCO3– into cells increased apoplastic pH due to the presence of an OH– efflux instead of an H+ influx transport system (Laing and Browse, 1985). This increase in pH of the apoplast possibly enables Ca2+, rather than H+, as the ion species that binds to negatively charged carboxyl groups of the cell wall (Harker et al., 2000) to promote linking of neighboring pectin polymers through the egg-box model (Demarty et al., 1984). This idea is in line with our observations. We observed that fruit firmness increased during high CO2 treatment, whereas it slightly decreased after fruit were transferred to the ambient atmosphere (Figure 5A). In contrast, firmness continuously declined in fruit in the control and CA0 treatments.
The differences in the anthocyanin content of fruit from different treatments during CA storage and shelf life were not consistent, presumably due to biological variation in samples. The ascorbic acid content in fruit did not change over the experiment, and no differences between the control and different CA treatments were apparent. From these aspects, our findings in “Arabella” strawberry fruit are different from most studies which found firmness loss, discoloration, and antioxidant losses in strawberry fruit treated with elevated CO2 (Gil et al., 1997; Lee and Kader, 2000; Shin et al., 2008; Li et al., 2019).
Application of high CO2 might induce fermentation. The extent of fermentation with increased CO2 concentrations is cultivar-dependent in strawberry fruit, and some cultivars do not produce fermentation metabolites at all (Fernández-Trujillo et al., 1999; Watkins et al., 1999; Pelayo-Zaldívar et al., 2007). As the ratio of CO2 production and O2 consumption in fruit approached 1, both under 20 and 30 kPa CO2 (data not shown), we assume that fermentative metabolism was not activated during CA storage. Therefore, “Arabella” strawberry fruit seem relatively tolerant to elevated CO2. This could explain that although high CO2 lowered the spoilage incidence, other quality properties, such as firmness, anthocyanins, and ascorbic acid, were hardly affected. The lower spoilage incidence not only resulted from the physiological effects of high CO2, as we discussed above but may also be due to the direct suppression of high CO2 on B. cinerea growth. In vitro inhibition tests showed that the B. cinerea colony diameter decreased with elevated CO2 from 0 to 20 kPa, and it could not grow when CO2 concentrations were higher than 30 kPa (Garcia-Gimeno et al., 2002). After fruit were transferred to the ambient atmosphere condition, the inhibitory effect was relieved, but the residual effect of elevated CO2 still existed, which was observed in both experiments and the two cultivars studied in our research.
The application of high CO2 (20 and 30 kPa) combined with 10 kPa O2 effectively reduced strawberry fruit spoilage by B. cinerea, probably resulting from lower respiration rates and higher pH. High CO2 did not affect total soluble sugar content but caused a more pronounced conversion of sucrose to glucose and fructose. High CO2 did not induce fermentation or affect antioxidant levels of fruit. Stepwise increments of CO2 did not show beneficial effects on overall fruit quality compared to the static application of high CO2. “Arabella” strawberry fruit can be stored under 30 kPa CO2 for prolonged periods without loss of overall quality.
The original contributions presented in the study are included in the article/Supplementary Material, further inquiries can be directed to the corresponding author.
HL, CZ, and EW conceived and designed the experiments. HL and CZ conducted Experiment I. HL and YY conducted Experiment II and analyzed the data. HL wrote the manuscript with the help of EW. RS and FA provided critical comments on the overall structure of the manuscript. All authors reviewed and approved the final manuscript.
HL was supported by the China Scholarship Council (CSC; Grant No. 201706300037) and Fresh Forward B. V. CZ was involved in the Innovative Talents Training Program of the Chinese Academy of Sciences funded by the Ministry of Science and Technology (2019) (P193041059).
The authors declare that the research was conducted in the absence of any commercial or financial relationships that could be construed as a potential conflict of interest.
All claims expressed in this article are solely those of the authors and do not necessarily represent those of their affiliated organizations, or those of the publisher, the editors and the reviewers. Any product that may be evaluated in this article, or claim that may be made by its manufacturer, is not guaranteed or endorsed by the publisher.
We thank Arjen van de Peppel for technical support of chemical measurement and also thank Gerard Leentfaar and Marcel G. Staal for the technical support of the controlled atmosphere system. We thank Sarah Berman for English improvement.
The Supplementary Material for this article can be found online at: https://www.frontiersin.org/articles/10.3389/fpls.2022.842317/full#supplementary-material
Supplementary Figure 1 | Brix and firmness of “Sonsation” strawberry fruit stored under controlled atmosphere (CA) conditions and shelf life. (A) Brix and (C) firmness at different time points during CA storage at 5°C and 100% relative humidity. (B) Brix and (D) firmness at different time points of CA storage followed by 3-day shelf life at 12°C and 100% relative humidity in ambient atmosphere. As a control condition, 21 kPa O2 and 0 kPa CO2 were used. Data represent means of 3 blocks (n = 3) with five replicate fruit per block. The error bars represent the standard error of means. Different letters denote significant differences according to Fisher’s protected LSD test (α = 0.05).
Supplementary Figure 2 | Correlation between pH and firmness of fruit from different CA treatments during storage and subsequent shelf life. The closed black squares represent data of day 0. Data represent individual blocks.
Agar, I. T., Streif, J., and Bangerth, F. (1997). Effect of high CO2 and controlled atmosphere (CA) on the ascorbic and dehydroascorbic acid content of some berry fruits. Postharvest Biol. Technol. 11, 47–55. doi: 10.1016/S0925-5214(97)01414-2
Bang, J., Lim, S., Yi, G., Lee, J. G., and Lee, E. J. (2019). Integrated transcriptomic-metabolomic analysis reveals cellular responses of harvested strawberry fruit subjected to short-term exposure to high levels of carbon dioxide. Postharvest Biol. Technol. 148, 120–131. doi: 10.1016/j.postharvbio.2018.11.003
Bassolino, L., Zhang, Y., Schoonbeek, H.-J., Kiferle, C., Perata, P., and Martin, C. (2013). Accumulation of anthocyanins in tomato skin extends shelf life. New Phytol. 200, 650–655. doi: 10.1111/nph.12524
Blanch, M., Rosales, R., Mateos, R., Perez-Gago, M. B., Sanchez-Ballesta, M. T., Escribano, M. I., et al. (2015). Effects of high CO2 levels on fermentation, peroxidation, and cellular water stress in Fragaria vesca stored at low temperature in conditions of unlimited O2. J. Agric. Food Chem. 63, 761–768. doi: 10.1021/jf505715s
Bodelón, O. G., Blanch, M., Sanchez-Ballesta, M. T., Escribano, M. I., and Merodio, C. (2010). The effects of high CO2 levels on anthocyanin composition, antioxidant activity and soluble sugar content of strawberries stored at low non-freezing temperature. Food Chem. 122, 673–678. doi: 10.1016/j.foodchem.2010.03.029
Bown, A. W. (1985). CO2 and intracellular pH. Plant Cell Environ. 8, 459–465. doi: 10.1111/j.1365-3040.1985.tb01681.x
Bui, T. T., Wright, S. A., Falk, A. B., Vanwalleghem, T., Van Hemelrijck, W., Hertog, M. L., et al. (2019). Botrytis cinerea differentially induces postharvest antioxidant responses in “Braeburn” and “Golden Delicious” apple fruit. J. Sci. Food Agric. 99, 5662–5670. doi: 10.1002/jsfa.9827
Davey, M. W., Auwerkerken, A., and Keulemans, J. (2007). Relationship of apple vitamin C and antioxidant contents to harvest date and postharvest pathogen infection. J. Sci. Food Agric. 87, 802–813. doi: 10.1002/jsfa.2777
Demarty, M., Morvan, C., and Thellier, M. (1984). Calcium and the cell wall. Plant Cell Environ. 7, 441–448. doi: 10.1111/j.1365-3040.1984.tb01434.x
Durán-Soria, S., Pott, D. M., Osorio, S., and Vallarino, J. G. (2020). Sugar signaling during fruit ripening. Front. Plant Sci. 11:564917. doi: 10.3389/fpls.2020.564917
Falagán, N., Miclo, T., and Terry, L. A. (2020). Graduated controlled atmosphere: a novel approach to increase “Duke” blueberry storage life. Front. Plant Sci. 11:221. doi: 10.3389/fpls.2020.00221
Feliziani, E., and Romanazzi, G. (2016). Postharvest decay of strawberry fruit: etiology, epidemiology, and disease management. J. Berry Res. 6, 47–63. doi: 10.3233/JBR-150113
Fernández-Trujillo, J. P., Nock, J. F., and Watkins, C. B. (1999). Fermentative metabolism and organic acid concentrations in fruit of selected strawberry cultivars with different tolerances to carbon dioxide. J. Am. Soc. Hortic. Sci. 124, 696–701. doi: 10.21273/JASHS.124.6.696
Garcia-Gimeno, R. M., Sanz-Martinez, C., Garcia-Martos, J. M., and Zurera-Cosano, G. (2002). Modeling Botrytis cinerea spores growth in carbon dioxide enriched atmospheres. J. Food Sci. 67, 1904–1907. doi: 10.1111/j.1365-2621.2002.tb08744.x
Gil, M. I., Holcroft, D. M., and Kader, A. A. (1997). Changes in strawberry anthocyanins and other polyphenols in response to carbon dioxide treatments. J. Agric. Food Chem. 45, 1662–1667. doi: 10.1021/jf960675e
Harker, F. R., Elgar, H. J., Watkins, C. B., Jackson, P. J., and Hallett, I. C. (2000). Physical and mechanical changes in strawberry fruit after high carbon dioxide treatments. Postharvest Biol. Technol. 19, 139–146. doi: 10.1016/S0925-5214(00)00090-9
Holcroft, D. M., and Kader, A. A. (1999). Controlled atmosphere-induced changes in pH and organic acid metabolism may affect color of stored strawberry fruit. Postharvest Biol. Technol. 17, 19–32. doi: 10.1016/S0925-5214(99)00023-X
Kader, A. A. (2003). A summary of CA requirements and recommendations for fruits other than apples and pears. Acta Hortic. 600, 737–740. doi: 10.17660/ActaHortic.2003.600.112
Kanellis, A., Tonutti, P., and Perata, P. (2009). “Biochemical and molecular aspects of modified and controlled atmospheres,” in Modified and Controlled Atmospheres for the Storage, Transportation, and Packaging of Horticultural Commodities, ed. E. M. Yahia (Boca Raton, FL: CRC Press). doi: 10.1201/9781420069587.ch22
Ke, D., Goldstein, L., O’mahony, M., and Kader, A. A. (1991). Effects of short-term exposure to low O2 and high CO2 atmospheres on quality attributes of strawberries. J. Food Sci. 56, 50–54. doi: 10.1111/j.1365-2621.1991.tb07973.x
Laing, W. A., and Browse, J. (1985). A dynamic model for photosynthesis by an aquatic plant, Egeria densa. Plant Cell Environ. 8, 639–649. doi: 10.1111/1365-3040.ep11611677
Lee, S. K., and Kader, A. A. (2000). Preharvest and postharvest factors influencing vitamin C content of horticultural crops. Postharvest Biol. Technol. 20, 207–220. doi: 10.1016/S0925-5214(00)00133-2
Li, D., Zhang, X., Li, L., Aghdam, M. S., Wei, X., Liu, J., et al. (2019). Elevated CO2 delayed the chlorophyll degradation and anthocyanin accumulation in postharvest strawberry fruit. Food Chem. 285, 163–170. doi: 10.1016/j.foodchem.2019.01.150
Lucas, W. J. (1979). Alkaline band formation in Chara corallina: due to OH efflux or H influx? Plant Physiol. 63, 248–254. doi: 10.1104/pp.63.2.248
Madrid, M. (2020). “Small fruits: strawberries,” in Controlled and Modified Atmospheres for Fresh and Fresh-Cut Produce (Amsterdam: Elsevier), 329–334. doi: 10.1016/B978-0-12-804599-2.00019-3
Nakata, Y., and Izumi, H. (2020). Microbiological and quality responses of strawberry fruit to high CO2 controlled atmosphere and modified atmosphere storage. HortScience 55, 386–391. doi: 10.21273/HORTSCI14771-19
Pelayo-Zaldívar, C., Abda, J. B., Ebeler, S. E., and Kader, A. A. (2007). Quality and chemical changes associated with flavor of ‘Camarosa’ strawberries in response to a CO2-enriched atmosphere. HortScience 42, 299–303. doi: 10.21273/HORTSCI.42.2.299
Petrasch, S., Knapp, S. J., van Kan, J. A. L., and Blanco-Ulate, B. (2019). Grey mould of strawberry, a devastating disease caused by the ubiquitous necrotrophic fungal pathogen Botrytis cinerea. Mol. Plant Pathol. 20, 877–892. doi: 10.1111/mpp.12794
Ponce-Valadez, M., Watkins, C. B., Moore, S., and Giovannoni, J. J. (2005). Differential gene expression analysis of strawberry cultivar responses to elevated O2 concentrations during storage using a tomato cDNA microarray. Acta Hortic. 682, 255–262. doi: 10.17660/ActaHortic.2005.682.27
Rama, M. V., and Narasimham, P. (2003). “Controlled-atmosphere storage | effects on fruit and vegetables,” in Encyclopedia of Food Sciences and Nutrition, 2nd Edn. ed. B. Caballero (Oxford: Academic Press), 1607–1615. doi: 10.1016/B0-12-227055-X/00292-3
Saltveit, M. E. (2019). “Chapter 4 – Respiratory metabolism,” in Postharvest Physiology and Biochemistry of Fruits and Vegetables, ed. E. M. Yahia (Duxford: Woodhead Publishing), 73–91. doi: 10.1016/B978-0-12-813278-4.00004-X
Schwieterman, M. L., Colquhoun, T. A., Jaworski, E. A., Bartoshuk, L. M., Gilbert, J. L., Tieman, D. M., et al. (2014). Strawberry flavor: diverse chemical compositions, a seasonal influence, and effects on sensory perception. PLoS One 9:e88446. doi: 10.1371/journal.pone.0088446
Shin, Y., Ryu, J. A., Liu, R. H., Nock, J. F., Polar-Cabrera, K., and Watkins, C. B. (2008). Fruit quality, antioxidant contents and activity, and antiproliferative activity of strawberry fruit stored in elevated CO2 atmospheres. J. Food Sci. 73, S339–S344. doi: 10.1111/j.1750-3841.2008.00857.x
Watkins, C. B., Manzano-Mendez, J. E., Nock, J. F., Zhang, J., and Maloney, K. E. (1999). Cultivar variation in response of strawberry fruit to high carbon dioxide treatments. J. Sci. Food Agric. 79, 886–890. doi: 10.1002/(SICI)1097-0010(19990501)79:6<886::AID-JSFA303<3.0.CO;2-0
Wszelaki, A. L., and Mitcham, E. J. (2000). Effects of superatmospheric oxygen on strawberry fruit quality and decay. Postharvest Biol. Technol. 20, 125–133. doi: 10.1016/S0925-5214(00)00135-6
Keywords: Fragaria × ananassa, CA storage, shelf life, stepwise atmosphere, grey mold disease, pH, sugar and acid metabolism
Citation: Li H, Yin Y, Affandi FY, Zhong C, Schouten RE and Woltering EJ (2022) High CO2 Reduces Spoilage Caused by Botrytis cinerea in Strawberry Without Impairing Fruit Quality. Front. Plant Sci. 13:842317. doi: 10.3389/fpls.2022.842317
Received: 23 December 2021; Accepted: 10 March 2022;
Published: 27 April 2022.
Edited by:
Brian Farneti, Fondazione Edmund Mach, ItalyReviewed by:
Qingguo Wang, Shandong Agricultural University, ChinaCopyright © 2022 Li, Yin, Affandi, Zhong, Schouten and Woltering. This is an open-access article distributed under the terms of the Creative Commons Attribution License (CC BY). The use, distribution or reproduction in other forums is permitted, provided the original author(s) and the copyright owner(s) are credited and that the original publication in this journal is cited, in accordance with accepted academic practice. No use, distribution or reproduction is permitted which does not comply with these terms.
*Correspondence: Ernst J. Woltering, ZXJuc3Qud29sdGVyaW5nQHd1ci5ubA==
Disclaimer: All claims expressed in this article are solely those of the authors and do not necessarily represent those of their affiliated organizations, or those of the publisher, the editors and the reviewers. Any product that may be evaluated in this article or claim that may be made by its manufacturer is not guaranteed or endorsed by the publisher.
Research integrity at Frontiers
Learn more about the work of our research integrity team to safeguard the quality of each article we publish.