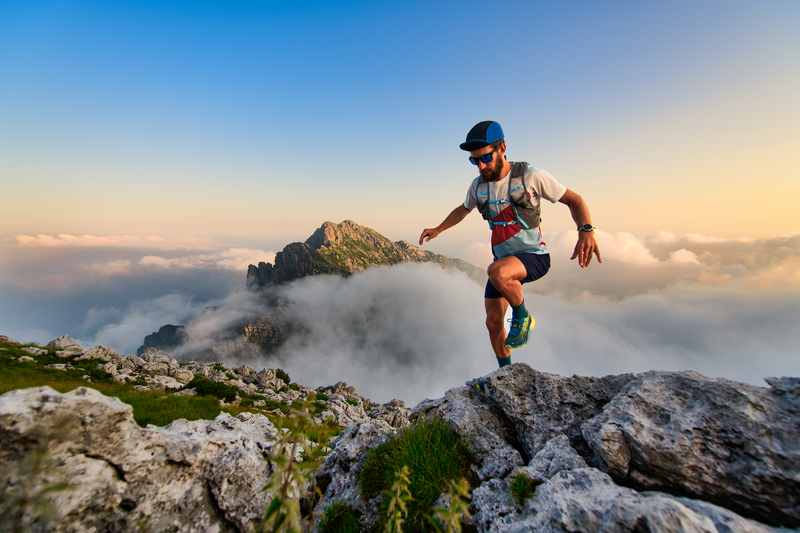
95% of researchers rate our articles as excellent or good
Learn more about the work of our research integrity team to safeguard the quality of each article we publish.
Find out more
ORIGINAL RESEARCH article
Front. Plant Sci. , 07 April 2022
Sec. Plant Breeding
Volume 13 - 2022 | https://doi.org/10.3389/fpls.2022.837613
This article is part of the Research Topic Insights in Plant Breeding: 2021 View all 6 articles
Rusts are among the most important foliar biotrophic fungal diseases in legumes. Lathyrus cicera crop can be severely damaged by Uromyces pisi, to which partial resistance has been identified. Nevertheless, the underlying genetic basis and molecular mechanisms of this resistance are poorly understood in L. cicera. To prioritise the causative variants controlling partial resistance to rust in L. cicera, a recombinant inbred line (RIL) population, segregating for response to this pathogen, was used to combine the detection of related phenotypic- and expression-quantitative trait loci (pQTLs and eQTLs, respectively). RILs’ U. pisi disease severity (DS) was recorded in three independent screenings at seedling (growth chamber) and in one season of exploratory screening at adult plant stage (semi-controlled field conditions). A continuous DS range was observed in both conditions and used for pQTL mapping. Different pQTLs were identified under the growth chamber and semi-controlled field conditions, indicating a distinct genetic basis depending on the plant developmental stage and/or the environment. Additionally, the expression of nine genes related to U. pisi resistance in L. cicera was quantified for each RIL individual and used for eQTL mapping. One cis-eQTL and one trans-eQTL were identified controlling the expression variation of one gene related to rust resistance – a member of glycosyl hydrolase family 17. Integrating phenotyping, gene expression and linkage mapping allowed prioritising four candidate genes relevant for disease-resistance precision breeding involved in adaptation to biotic stress, cellular, and organelle homeostasis, and proteins directly involved in plant defence.
Lathyrus cicera L. (chickling pea) is a dual-purpose cool season legume and a source of protein for animals (Hanbury et al., 2000) and humans (Peña-Chocarro and Peña, 1999) nutrition. This species-genus belongs to the tribe Fabeae (syn. Vicieae) along with Vicia, Lens, Pisum, and Vavilovia (reviewed in Smýkal et al., 2011). Lathyrus sativus (grass pea) is the most closely related relative to L. cicera and these two Lathyrus species have a close phylogenetic relationship with pea (Pisum sativum), so close that there are suggestions that the genus Pisum should be included in the genus Lathyrus (Schaefer et al., 2012).
Lathyrus cicera owns important agronomic traits as resistance to biotic and abiotic stresses (Vaz Patto and Rubiales, 2014; Hammer et al., 2019; Lambein et al., 2019). It is therefore an attractive choice for sustainable feed and food production, mainly in more marginal environments, and because of pathogen sharing, it could act as a promising alternative source of resistance to related species, such as grass pea and pea (Vaz Patto et al., 2006; Vaz Patto and Rubiales, 2014; Hammer et al., 2019).
Fungal diseases are major constraints for yield stability in legumes (Rubiales et al., 2015; Martins et al., 2020). Rusts are among the most important diseases recorded in grain and forage legumes (Sillero et al., 2006; Rubiales et al., 2011). Several rust species can infect legumes, most of them belonging to the Uromyces genus (Rubiales et al., 2011). A good example is the wide host range biotrophic Uromyces pisi that infects species of Lathyrus, Pisum, Lens, and Vicia genera (Farr, D.F., and Rossman, A.Y. Fungal Databases) (Barilli et al., 2012). Chemical control of rust is possible (Emeran et al., 2011), but the use of host plant resistance is the most economical and ecological desired means of control (Rubiales et al., 2011). Due to the reduced selective pressure imposed on the pathogen, the use of plant partial resistance is a potentially more durable approach than complete resistance (McDonald and Linde, 2002; Niks and Rubiales, 2002). Indeed, rusts are among the pathogens with the highest risk of breaking down the effectiveness of major resistance genes (R-genes) due to their effective air dispersal and the coexistence of sexual and asexual reproduction cycles (McDonald and Linde, 2002).
Partial resistance not associated with host cell necrosis (hypersensitive reaction) is common in major grain legumes against rusts (Sillero et al., 2006) and was already identified in Lathyrus spp. (Vaz Patto and Rubiales, 2009; Vaz Patto et al., 2009) or in Pisum spp. (Barilli et al., 2009a,b,c, 2012) against U. pisi and U. vicia-fabae. In the particular case of Pisum fulvum, a wild relative of pea, one to three quantitative trait loci (QTLs) controlling partial resistance to U. pisi have been identified (Barilli et al., 2010, 2018). In contrast, little is known about the genetic control of L. cicera resistance to U. pisi.
In an Iberian collection of L. cicera accessions, microscopic and macroscopic variable levels of resistance were identified against U. pisi. Resistant accessions partially restricted the formation of haustoria, resulting in a high percentage of early aborted fungal colonies, a decreased number of haustoria per colony, and a reduced intercellular growth of infection hyphae compared to susceptible accessions (Vaz Patto et al., 2009). A segregating recombinant inbred line (RIL) population was developed from the cross of the most contrasting L. cicera accessions of this Iberian collection. The RILs were later on used for the development and refinement of the first L. cicera linkage map with transcriptome based SNPs and e-SSR markers (retrieved from the RIL parental lines leaf RNAseq response to U. pisi infection) as well as genotype-by-sequencing based markers (Santos et al., 2018, 2020). The mentioned L. cicera RIL parental lines transcriptomic study also highlighted upregulated genes in response to rust infection involved in hormone metabolism, cell wall degradation, secondary metabolism, ROS production, signalling and regulation of transcription of defence (Santos et al., 2018). In spite of these recent efforts, the causative variants controlling partial resistance to U. pisi remain elusive, leading to a poor understanding of the partial genetic resistance and molecular mechanisms of Lathyrus spp. against rust disease.
Classical approaches to unveil quantitative resistance genetic basis in non-model species include QTL mapping in segregating populations and syntenic analyses using model/related species to search for orthologous genes within the QTL detected regions. Whereas much progress has been made in plant QTL mapping controlling phenotypic natural variation, this approach has been hampered by the complex interrelation of genetic variants and expression regulators (Hansen et al., 2008; Wallace et al., 2013; Albert and Kruglyak, 2015). In species with no sequenced or no fully assembled genome, as the Lathyrus spp. (Emmrich et al., 2020), alternative approaches may add value over classical QTL mapping. Genetical-genomics is considered a very powerful tool to improve our knowledge on the genetic architecture of complex traits, including disease response (Lima et al., 2018; Fauteux et al., 2019). In this approach, transcript expression levels are treated as quantitative phenotypes in a segregating population and the genomic variants that influence expression levels of each transcript are identified by conventional QTL analysis (Li and Burmeister, 2005). The found genomic regions controlling gene expression are referred to as expression-QTLs (eQTLs). Previous studies have reported that distant or trans-eQTLs may explain a higher proportion of expression variance than local (at the same locus as the structural gene) or cis-eQTLs (Liu et al., 2017; Carrasco-Valenzuela et al., 2019; Fauteux et al., 2019; Miculan et al., 2021). Hotspots of trans-eQTL may act as key regulators of phenotypes, whereas cis-eQTLs display local gene expression regulation, with co-regulated gene clusters (Wang et al., 2018; Miculan et al., 2021). Several studies have been using a hybrid approach (pQTL and eQTL analyses) to better understand the gene networks underlying traits of interest in plants (Lima et al., 2018; Carrasco-Valenzuela et al., 2019; Fauteux et al., 2019; Miculan et al., 2021).
The main aim of this work was to elucidate the genetic basis and putative molecular strategies of chickling pea partial resistance against rust disease. Using L. cicera RIL population phenotypic response to U. pisi infection (mainly at the seedling stage, under controlled growth chamber, and complemented by an exploratory assay at the adult plant stage, under semi-controlled field conditions), in the expression analysis of genes related to rust resistance and genomic data, we performed a combined pQTL/eQTL linkage mapping analysis. This will allow prioritising candidate genes for validation and future use in precision breeding, and advancing our understanding on the molecular mechanisms underlying partial resistance to U. pisi in L. cicera.
A segregating population of 103 F6 RILs, derived by single seed descendent from a cross between L. cicera genotypes BGE023542 and BGE008277, was repetitively phenotyped in response to rust (U. pisi) infection under controlled growth chamber conditions at the seedling stage and under semi-controlled field conditions at the adult plant stage.
The two parental genotypes showed contrasting phenotypes to U. pisi infection in an exploratory growth chamber condition (seedling stage) screening of Iberian germplasm (Vaz Patto et al., 2009). BGE023542 was partial resistant [Disease Severity (DS) = 36%, scored as the percentage of leaf area coverage by rust pustules; and Infection Type (IT) = 4 representing a compatible interaction with well-formed pustules with no associated chlorosis or necrosis (Stakman et al., 1662)], and BGE008277 susceptible (DS = 80%, IT = 4) (Vaz Patto et al., 2009).
Phenotypic response of L. cicera RIL individuals and parental lines to rust disease were studied at the seedling stage under a controlled growth chamber and the adult plant stage in one season exploratory experiment, under semi-controlled field conditions. L. cicera RIL individuals were inoculated with U. pisi monosporic isolate Up-CO-01, derived from a rust population earlier collected on pea fields at Córdoba and stored at Institute for Sustainable Agriculture-CSIC at –80°C. Prior to use for the screenings, rust spores were retrieved from the store and multiplied on the susceptible pea cv. “Messire.”
The L. cicera RIL individuals and their parental genotypes were sown in Córdoba during the 2018/2019 growing season, under a tunnel covered with insect-proof net and drop irrigation. Five seeds per genotype were sown on 19 November 2018 in a one-row plot, with three plot repetitions (15 plants in total), using an alfa-lattice design. On the day of field inoculation, the conserved spores were heat-shocked at 40°C for 5 min and then diluted in a Tween-20 aqueous solution (0.03%, v:v), used as a wetting agent. Three-month-old seedlings were spray-inoculated at the sunset to benefit from darkness and higher relative humidity of the night promoting spore adhesion and germination. At plant maturity (6 months old and 3 months after inoculation), DS and IT were assessed. DS was visually estimated as the percentage of canopy covered by rust pustules. IT was assessed using the 0–4 scale of Stakman et al. (1962), where IT 0 = no symptoms, IT 1 = necrotic halo surrounding minute pustules barely sporulating; IT 2 = necrotic halo surrounding small pustules, IT 3 = chlorotic halo, and IT 4 = well-formed pustules with no associated chlorosis or necrosis.
The response of the L. cicera RIL population was also evaluated at the seedling stage under controlled growth chamber conditions, in three independent inoculation assays (assay repetitions). For each inoculation assay, five seedlings per RIL were grown in pots (one plant per pot), containing 250 cm3 of 1:1 sand-peat mixture, in a growth chamber at 20°C with a 12 h light/12 h dark photoperiod. Twenty-day-old seedlings were dust-inoculated with U. pisi spores diluted in pure talk (1:10) with the help of a small manual-dusting device. After inoculation, seedlings were incubated for 24 h at 20°C in complete darkness and 100% relative humidity and then transferred to a growth chamber at 20°C with a 12 h light/12 h dark photoperiod. The response to rust inoculation was assessed 11 days after inoculation by measuring DS and IT.
For gene expression quantification, leaves of the parental lines and each F5 RIL individual were inoculated with U. pisi Up-CO-01 under growth chamber conditions. One to three biological replicates of 15-days-old L. cicera seedlings were inoculated using the same procedure described previously. Inoculated leaves were collected at 37 h after inoculation (hai) to be consistent with the time-point used in the already mentioned L. cicera RIL parental lines rust response transcriptomic studies (Santos et al., 2018). This time-point corresponds to the infection stage between fungus growth prior to stoma penetration and the early stages of infection, till colony development and if applicable, the presence of host cell necrosis observed at a microscopic level (Vaz Patto and Rubiales, 2009). Collected leaves were immediately frozen in liquid nitrogen and stored at –80°C until RNA extraction. Total RNA was extracted from about 100 mg of inoculated leaves using the GeneJET Plant RNA Purification Mini Kit (Thermo Scientific, Vilnius, Lithuania), according to the manufacturer’s instructions. The extracted RNA was treated with Turbo DNase I kit (Ambion, Austin, TX, United States), according to the manufacturer’s instructions. RNA concentrations were measured by a Qubit 2.0 Fluorometer (Invitrogen, Life Technologies, Carlsbad, CA, United States) using a Qubit dsRNA BR Assay kit. The RNA purity was checked by measuring the ratios of absorbance at 260/280 nm and 230/280 nm using a NanoDrop device (Thermo Scientific, Passau, Germany).
The cDNA was synthesised from 1.5 μg of total RNA from each sample following the manufacturer’s instructions from the iScript cDNA Synthesis Kit (Biorad, Hercules, CA, United States).
Differentially expressed genes (DEGs) related to rust resistance were selected from the L. cicera RIL parental lines RNAseq leaf-transcriptome in response to U. pisi infection previously obtained by Santos et al. (2018). The gene selection criteria were: (1) non-redundant DEGs with the log2 of the ratio between BGE023542 (partially resistant) and BGE008277 (susceptible) inoculated reads higher than 2 and lower than –2; (2) DEGs not directly involved in specific resistance mechanisms, such as oxidative, metabolic, and transporter activities; (3) DEGs involved in defence response and categorised in pathogen recognition, antifungal proteins, cell wall modification proteins or involved in the regulation of other defence related processes and (4) nucleotide sequences of DEGs suitable for primer design, as described in the next section “Primer design” and resulting in a single amplification (primer specificity).
Differentially expressed gene annotation was revised and updated from the previous study (Santos et al., 2018) by BLASTn search against the pea reference genome v1a1 (Kreplak et al., 2019) and against genomic sequences of other legume species deposited at NCBI databases. The molecular function and biological process from each DEG related to rust resistance were also investigated using InterPro (Hunter et al., 2009) and UniProt2 databases.
Primers were designed for the selected DEGs using as a template the gene sequence obtained by the JBrowse tool at https://urgi.versailles.inra.fr/Species/Pisum. The Primer3Plus tool3 (Boston, MA, United States) was used for primer design, with the default setting for Reverse Transcribed quantitative PCR (RT-qPCR) optimal conditions. Primer specificity was predicted using the Primer-BLAST NCBI tool (National Center for Biotechnology Information, United States), using the legume genomes deposited at NCBI. Specific primers were preferably designed in the 3′ intra-exonic regions and were synthesised by STABVida (Caparica, Portugal) (Supplementary Table 1).
The relative gene expression of selected DEGs was analysed by RT-qPCR on a Light Cycler® 480 System, using the LightCycler® 480 SYBR Green I Master protocol. PCR amplification efficiencies were tested for all primers for target and reference genes using cDNA two-fold dilution series. As reference genes, β-tubulin, photosystem I P700 apoprotein A2, γ-tubulin, chromodomain helicase DNA-binding protein, and histone H2A.2, previously described by Almeida et al. (2015) and Santos et al. (2018), were tested. Using the geNorm and NormFinder software packages from the GenEx v.5 software (MultiD, Goteborg, Sweden), two reference genes were selected for the gene relative expression analysis. Thermo cycling reactions were carried out following the described conditions: denaturation step at 90°C for 5 min; 45 cycles of amplification at 95°C for 10 s; 10 s at 60°C and 10 s at 72°C. For each reaction, a melting curve (dissociation stage) was performed to detect non-specific PCR products and/or contaminants. A non-template control (NTC), without cDNA, was also included for each primer mix to detect possible contaminations.
Relative expression levels (Fold change, FC) were calculated using the Pfaffl method (Pfaffl, 2001) compared with expression levels of the reference genes (β-tubulin and γ-tubulin) and using the susceptible parental line BGE008277 as a calibrator. Finally, FC data were transformed into a logarithmic scale (base 2) to meet the data normality assumptions for statistical analysis and graphical representation.
Since the number of biological replicates varied from 1 to 3, the absence of significant differences between biological replicates was confirmed by ANOVA using the Genstat software (Genstat® for Windows 19th edition), considering the genotypes represented by three biological replicates. Therefore, the average of relative expression levels per RIL was used as a metric for eQTL detection.
The descriptive statistical analyses of phenotypic DS data collected at the seedling stage under growth chamber and adult plant stage under semi-controlled field conditions, as well as of gene expression normalised data, were performed using the Genstat software (Genstat® for Windows 19th edition). Graphical inspection of residuals was used to assess normality (Q-Q plot), homogeneity of variance (residuals versus fitted values), and to identify outliers. Observations exceeding 1.5 times the interquartile range were removed from the analysis. ANOVA was independently conducted for DS scored under growth chamber, semi-controlled field conditions, and gene expression normalised data, using the Genstat procedure. A t-test (P < 0.05) was used for means comparisons between relative expression of parental lines for each DEG under study. Broad-sense heritabilities, representing the percentage of the genetic variance in the total phenotypic variance, were calculated for phenotypic data using the VHERITABILITY Genstat procedure.
Phenotypic QTL (pQTL) and expression QTL (eQTL) linkage mapping analyses for rust resistance were performed using the MapQTL software version 5.0 (Van Ooijen, 2009). For pQTL analysis, the DS averages obtained across repetitions for each RIL under growth chamber or semi-controlled field conditions were used. The mean of the relative expression value of a gene in each RIL was treated as phenotypic data for the eQTL analysis. Interval mapping (Lander and Botstein, 1989) and multiple QTL mapping approaches (MQM) (Jansen and Stam, 1994) were applied. The significant LOD thresholds corresponding to a confidence level of P < 0.05 were estimated for each trait (DS scored under growth chamber and semi-controlled field conditions and gene expression data) using a permutation test with 1,000 permutations available in MapQTL software. Phenotypic QTLs and eQTLs were declared significant when LOD scores (MQM) exceeded the minimum significance LOD threshold. The coefficient of determination (R2) for the marker located at the pQTL/eQTL peak was used to estimate the percentage of the phenotypic/transcript abundance variance explained by the pQTL/eQTL. The 1-LOD support interval was also determined for each QTL LOD peak. The additive effect for each detected pQTL/eQTL was estimated using the MQM procedure.
Each significant pQTL/eQTL was characterised by the peak marker, the coefficient of determination (R2), LOD score, the QTL interval (including 1-LOD confidence support), and the additive effect. pQTL/eQTL representations were drawn using the MapChart 2.3 software (Voorrips, 2002). pQTL nomenclature was set as follows: UpDSLG_chamber and UpDSLG_field, where the “LG” was replaced by the number of the L. cicera map Linkage Group in which the pQTLs were detected for U. pisi under growth chamber and semi-controlled field conditions, respectively. eQTL nomenclature was set as the acronym of the gene followed by the LG, where the eQTLs for relative expression were detected. For pQTL/eQTLs mapped in the same LGs, those QTLs were distinguished with “a,” “b,” or “c” after the LG number, where “a” corresponds to the pQTL/eQTL with the highest LOD value and “c” to the pQTL/eQTL with the lowest LOD value. As an example, GIucIVa and GlucIVb referred to both eQTLs identified for relative expression of the Gluc gene detected on LGIV.
The syntenic locations of L. cicera DEGs and eQTL intervals here studied were identified in the legume genomes publicly available to classify eQTLs into cis or trans. Using the DEGs and eQTL flanking markers’ nucleotide sequences, BLASTn tools were applied against P. sativum genome and the legume genomes deposited at NCBI (e-value < 1–5). The best BLAST hit obtained was used to define eQTLs as cis or trans-eQTLs. Cis-eQTLs were defined as being overlapping the transcribed region of the respective DEG (structural gene), while trans-eQTLs were defined as being located distant from the respective DEG (more than 1 Mbp) in the same chromosome or in a different chromosome. L. cicera DEGs and eQTL syntenic positions in the pea genome were represented and drawn using MapChart 2.3 software (Voorrips, 2002).
Candidate genes underlying pQTLs and eQTLs were predicted by the flanking markers’ sequence positional alignment to the legume sequence NCBI database and to the P. sativum reference genome v1a (Kreplak et al., 2019), using the BLASTn tool (e-value < 1–5). Flanking markers were defined as the closest markers to the boundaries of the QTL 1-LOD confidence interval (but still within the interval) with the possibility that only one marker (peak marker) was found within this interval. Additional candidate genes located within QTL regions were searched by comparative mapping of those pQTL/eQTL intervals in the pea genome, using the P. sativum Jbrowse platform4. The inferred position of the QTL intervals flanking markers in the pea genome was used to delimit the pea genome regions where to search for potential additional candidate genes.
All L. cicera RIL individuals showed a compatible interaction (IT = 4) against U. pisi at the seedling stage under the growth chamber and at the adult plant stage under semi-controlled field conditions. This means that well-formed pustules with no associated macroscopically visible chlorosis or necrosis were observable on the leaf surface (Supplementary Figure 1). However, for both conditions, DS population frequency showed a continuous variation, ranging from 21.7 to 44.3% and from 10 to 50%, at the seedling stage under controlled and at adult plant stage under semi-controlled field conditions, respectively (Figure 1). The partially resistant parental accession BGE023542 showed a DS = 25.8 and 11.7% (IT = 4), at the seedling and adult plant stage, respectively (Figure 1). On the other hand, the susceptible parental accession BGE008277 showed a DS = 43.1% at the seedling and DS = 30% at the adult plant stage (Figure 1). Transgressive segregation was detected for DS against rust infection mainly under the adult plant stage, with a fraction of the individual RILs showing higher susceptibility than the susceptible parental genotype (BGE008277) (Figure 1). Little transgressive segregation was also observed at the seedling stage, with individual RILs more resistant than the partial resistant BGE023542 (Figure 1).
Figure 1. Frequency distributions of the Lathyrus cicera recombinant inbred line (RIL) population (BGE023542 × BGE008277) disease severity (DS) after inoculation with Uromyces pisi: (A) under growth chamber (controlled conditions) and (B) under semi-controlled field conditions. The average values of U. pisi DS of the two parental lines are indicated with arrows.
Since the residual’s variance followed a normal distribution for rust DS under growth chamber and semi-controlled field conditions, no data transformation was applied. Analysis of variance of rust DS revealed significant differences among the RIL individuals (P < 0.001) under both conditions (Supplementary Table 2). The calculated broad-sense heritability for rust DS across repetitions was similar at the seedling and adult plant stage, being 66.3 and 65.1%, respectively. Rust DS between seedling under growth chamber and adult plant stage under semi-controlled field conditions were positively, but weekly correlated (0.22, P < 0.05) (Table 1).
Table 1. Pearson’s correlation coefficients determined among rust disease severity (DS) and expression of genes related to rust resistance in the Lathyrus cicera RIL population (BGE023542 × BGE008277).
For the gene relative expression analysis, the β-tubulin and γ-tubulin were selected as reference genes, since both genes showed the most stable average expression among the reference genes tested.
Using the gene selection criteria previously defined, nine DEGs related to rust resistance were selected from the work of Santos et al. (2018; Table 2). For these genes, annotation was revised and updated from the original annotation (Santos et al., 2018; Table 2). The RIL individuals’ relative gene expression levels were measured for the nine selected DEGs and normalised to the mean of the susceptible parental line BGE008277. The relative gene expressions (evaluated as log2Fold Change) obtained among the L. cicera RIL population are depicted in comparative dot-histograms (Figure 2). Significant gene expression variation among the RIL individuals was observed for all genes under study (P < 0.001), being greater for the Gluc and Extensin genes (Figure 2). Nevertheless, when comparing the two RIL parental lines (partially resistant BGE023542 and the susceptible BGE008277), only two of the nine genes under study (Pi49 and Gluc) showed significant differences (P < 0.05) (Figure 2). Moreover, significant correlations between gene expression and rust DS were only observed between DS under semi-controlled field conditions and Gluc relative expression (–0.33, P < 0.01) (Table 1).
Table 2. Genes related to rust resistance, selected from previously identified in a leaf-transcriptomic RNAseq study between BGE023542 (partially resistant) and BGE008277 (susceptible) Lathyrus cicera RIL parental lines in response to Uromyces pisi infection (Santos et al., 2018).
Figure 2. Dot plots histogram showing the distribution of relative expression (Log2 Fold change) of the selected genes related to rust partial resistance in the Lathyrus cicera recombinant inbred line (RIL) population (BGE023542 × BGE008277). The log2Fold Change values are represented in relation to the calibrator susceptible BGE008277 parental line, displayed by the horizontal line crossing Y-axis origin (log2 Fold Change = 0). Each black and green dot represents an RIL individual and the BGE023542 (partial resistant) parental line, respectively. P-values obtained from t-test calculated between relative expression of parental lines for each gene are indicated by asterisks as follows: **P < 0.01 (Gluc) and ***P < 0.001 (Pi49).
No significant differences were found between plot repetitions for DS under the semi-controlled field conditions (Supplementary Table 2). Although significant differences were found between inoculation assay repetitions for DS evaluated under growth chamber conditions (P < 0.001), the effect for genotype × inoculation assay interaction (F = 4.63) was much smaller than the genotype effect (F = 14.2). This supported the use of DS means across the three inoculation assays on the QTL analysis, increasing the power of QTL detection (Supplementary Table 2). Therefore, a univariate pQTL analysis was carried out using the means for DS obtained across repetitions under the semi-controlled field, as well as under the growth chamber conditions.
Several and different pQTLs were identified for U. pisi response in the L. cicera RIL population at the seedling and adult plant stage. Five genomic regions associated with response to U. pisi DS at the adult plant stage under semi-controlled field conditions were mapped on LGII (UpDSIIa_field, UpDSIIb_field, and UpDSIIc_field) and on LGIV (UpDSIVa_field and UpDSIVb_field). On the other hand, only one pQTL was identified in response to U. pisi at the seedling stage under growth chamber conditions on LGIV–UpDSIV_chamber (Figure 3). pQTLs identified for U. pisi DS at the adult plant stage explained from 7.1 to 19% of the phenotypic variance observed (Table 3). The only detected UpDSIV_chamber QTL for U. pisi DS measured in seedlings under growth chamber conditions explained 10.7% of the phenotypic variance observed (Table 3). Resistant pQTL alleles (the ones contributing to a reduction in DS values) were derived from the partial resistant parental line (BGE023542) in all the pQTLs, except for the UpDSIIa_field QTL, where the resistant allele was derived from the most susceptible parental line BGE008277 (Table 3). This was also the strongest pQTL (based on LOD score, here 6.47), with the SSR LCI336 as peak marker (Table 3 and Figure 3). For each detected pQTL, besides the two flanking markers or peak marker, no other marker was found within the defined 1-LOD pQTL confidence intervals.
Figure 3. Phenotypic quantitative trait loci (pQTLs, in black) and expression QTLs (eQTLs, in pink) for rust (Uromyces pisi) inoculation response mapped on linkage groups (LG) of the high-density Lathyrus cicera genetic linkage map based on a recombinant inbred line population (BGE023542 × BGE008277) (Santos et al., 2020). Genetic distances given in centimorgans (Kosambi mapping function) are indicated by the ruler on the left. Horizontal black lines indicate marker positions along LGs. Boxes, extended by lines depicting the 1-LOD confidence interval, represent pQTL/eQTL intervals: in black pQTLs identified for U. pisi DS (disease severity [%]), under semi-controlled field conditions and growth chamber conditions; eQTLs are represented in pink (GlucIVa and GlucIVb). In brown are represented pQTL intervals for powdery mildew disease response (Erysiphe pisi and E. trifolii), previously identified in the same L. cicera RIL population (Santos et al., 2020).
Table 3. Phenotypic quantitative trait loci (pQTLs) and expression QTLs (eQTLs) identified for response against Uromyces pisi in the Lathyrus cicera RIL population (BGE023542 × BGE008277).
Glycosyl hydrolases family 17 (Gluc) was the only gene showing a significant (negative) correlation with DS and differential expression between the two RIL parental lines in the present study. Although Pi49 expression also showed to be differential between parental lines, no significant correlation was observed with DS. Therefore, eQTL mapping was conducted only for the relative gene expression of Gluc, which led to the detection of two eQTLs, located on LGIV of the L. cicera linkage map (Figure 3 and Table 3). The eQTLs identified explained individually 7.6 (GlucIVb) and 32.2% (GlucIVa) of the gene expression variance observed (Table 3). The two eQTLs showed negative and positive additive effects (Table 3), indicating that alleles for increased gene expression came from both partial resistant and susceptible L. cicera RIL parental lines. The strongest eQTL (based on LOD score, here 9.29) was the GlucIVa, with the Silico DArT 100000355 as peak marker (Table 3). For each detected eQTL, besides the flanking markers or peak marker, no other marker was found within the defined 1-LOD eQTL confidence intervals.
By considering the predicted localisation of L. cicera eQTLs and differentially expressed gene sequences in the pea genome obtained by synteny analysis, we could define the detected eQTLs as one cis-eQTL and one trans-eQTLs. In particular, GlucIVa eQTL was located in the same locus of the Gluc structural gene (cis-eQTL) (Supplementary Figure 2). On the contrary, the GlucIVb eQTL was mapped in different chromosomes (considering the best BLAST hit against – against M. truncatula genome, Table 4) and distant to the structural gene (considering P. sativum genome) – trans-eQTL (Supplementary Figure 2).
Table 4. Phenotypic QTLs and expression QTLs’ flanking/peak markers and candidate genes identified for response to Uromyces pisi inoculation in the Lathyrus cicera RIL population (BGE023542 × BGE008277).
Potential candidate genes underlying the detected pQTLs and eQTLs associated with rust response in L. cicera RIL were inferred using BLASTn of nucleotide sequences from pQTL/eQTL intervals flanking or peak markers. Overall, identified candidate genes were predicted to be involved in cell cycle and division, adaptation to biotic stress, cellular and organelle homeostasis, mitochondrial redox, and proteins directly involved in plant defence (Table 4). In particular, when considering the U. pisi DS pQTLs, five candidate genes were identified: a pentatricopeptide repeat (PPR)-containing protein and a Cyclin + N-terminal domain at the seedling stage under growth chamber conditions; and a Diacylglycerol kinase, a Mitochondrial carrier, and a Utp21 specific WD40 associated putative domain, at the adult plant stage under semi-controlled field conditions (Table 4). The other three candidate genes were identified as underlying flanking markers of eQTLs for Gluc expression. Beyond the structural gene of GlucIVa cis-eQTL (Glycosyl hydrolases family 17), two more candidate genes were identified underlying the flanking markers of GlucIVb trans-eQTL: a rust resistance kinase Lr10 protein and a SCO1 homologue 2 (Table 4).
Additional candidate genes were searched within the pQTL/eQTL regions by comparative mapping of these intervals with the pea genome, using the syntenic flanking markers as delimitation of the genome windows where to search for potential candidate genes. Based on this approach, two syntenic regions were identified in pea for two of the detected pQTLs (UpDSIV_chamber and UpDSIIc_field). The candidate genes identified based on the comparative mapping are listed in Supplementary Table 3. Among all candidate genes found in homologous pea genome regions, some genes or gene families are known to be involved in host-pathogen interactions (Supplementary Table 3).
Most of the rust resistance reactions described so far in cool season legumes are incomplete and so potentially durable, but in most cases, the genetic basis of these resistances is still largely unknown, hampering their use in precision breeding (Rubiales et al., 2015). In this study, L. cicera RIL population segregating for rust resistance was used to elucidate the genetic basis and putative molecular strategies of chickling pea partial resistance against rust disease. By integrating the phenotypic response to U. pisi infection (at two different developmental plant stages and growing conditions), with the expression analysis of genes related to rust resistance and genomic data, we performed a combined pQTL/eQTL analysis. This allowed us to prioritise candidate genes that after validation, may be relevant for resistance precision breeding and advance our understanding of the molecular mechanisms underlying partial resistance to U. pisi in L. cicera.
All analysed L. cicera RIL individuals showed a compatible reaction with U. pisi, at the seedling stage, under a controlled growth chamber, as well as at the adult plant stage under semi-controlled field conditions in one-season exploratory analysis, characterised by well-formed pustules with no associated chlorosis or necrosis (IT = 4). As previously described in L. cicera (Vaz Patto et al., 2009), the most resistant genotypes presently identified, showed a low DS despite this compatible infection type, confirming their partial resistant nature (low DS, high IT) (McDonald and Linde, 2002; Niks and Rubiales, 2002). In the well-studied wheat-rust pathosystem, partial resistance commonly has a polygenic nature (controlled by adult plant resistance or APR genes), being expressed only in adult plants (except under very specific conditions) by a reduced and slow pathogen growth, without hypersensitive response. In contrast, pathogen race- or strain-specific major resistance genes (R-genes), generally conferring complete resistance, mostly function from seedling to adult growth stages (Dakouri et al., 2013; Ellis et al., 2014; Zegeye et al., 2014). In this study, DS evaluated in seedlings and adult plants were weakly correlated, indicating that also in L. cicera as in cereals, a different genetic basis, with multiple “minor effect” genes (explaining 7.1–19% observed variance) depending on the plant developmental stage may be involved in resistance. Indeed, it is widely acknowledged that partial resistance to rust is better identified in polycyclic infections, and even further, clearer on the adult plant stage than on seedlings (Sillero et al., 2006; Barilli et al., 2009a). This also seems to be the case in L. cicera. By considering even just one-season exploratory experiment at the adult plant stage, under semi-controlled field conditions, we allowed polycyclic infection vs. the monocyclic infection to occurr in the well-replicated growth chamber experiments at the seedling stage. Therefore, also in L. cicera there might be valuable small “adult plant” factors, not seen in the accurate monocyclic infections’ evaluations in seedlings. These findings are of utmost importance as currently, breeders put a higher emphasis on the discovery, characterisation, and complementary use of genes for partial, more durable resistance than on using only major effect R-genes, due to their potential lack of durability (Ellis et al., 2014).
From all the highlighted pQTL candidate genes, only the PPR (candidate for the UpDSIV_chamber pQTL), DGK, and mitochondria carrier genes (UpDSIIa_field and UpDSIIc_field pQTLs, respectively) were also identified differentially expressed between the RIL parental lines in the previous RNAseq L. cicera-U. pisi transcriptomic study (Fold Change of 0.94, 1.48, and 2.56, respectively) (Santos et al., 2018). For the remaining pQTL candidate genes, it was not possible to identify any DEG in the mentioned transcriptomic study, possibly due to the short nucleotide sequence available from DartSeq markers (65 bp), hampering a precise alignment between marker and transcriptomic sequences. Thus, the discussion of putative function in the variation of DS L. cicera response against U. pisi will focus on PPR, DGK, and mitochondria carrier genes, as the most promising candidate resistance genes considering the available information.
Pentatricopeptide proteins have been identified as playing important roles in organellar RNA metabolism, organ development, and in abiotic and biotic stresses (Lurin et al., 2004; Koussevitzky et al., 2007; Laluk et al., 2011; Xing et al., 2018). The function of PPR proteins has been reported in plant response to necrotrophic fungi and pathogenic bacteria (Laluk et al., 2011; Park et al., 2014). More recently, genes from the PPR gene family were identified underlying QTLs for partial resistance to the biotrophic Erysiphe pisi and E. trifolii powdery mildew pathogens in L. cicera (Santos et al., 2020) and in L. sativus (Martins et al., 20225). All these studies reporting PPR as a gene involved in Lathyrus spp. response against biotrophic pathogens, instigate further analysis to the function of genes encoding for members of the PPR gene family on chickling pea response to rust fungi.
Concerning DGK genes, some studies have revealed their involvement in the modulation of plant growth and adaptation to both biotic and abiotic stresses. DGKs are the main moderators of lipid signalling in plants, and this enzymatic activity is increased upon pathogen infection or elicitor treatment in different species (Foka et al., 2020). In pea, for instance, the inhibition of DGK activity promoted an elicitor-mediated accumulation of the phytoalexin pisatin, inducing phenylalanine ammonia-lyase expression (Toyoda et al., 2000). In the present study, UpDSIIa_field pQTL (for which the DGK gene was proposed) showed a positive additive effect indicating that alleles for increased DS came from susceptible L. cicera parental line, and so, resistance may be caused by inhibition of DGK activity, as suggested for pea. However, in the previous L. cicera transcriptomic study (Santos et al., 2018), the partial resistant parental line showed higher expression of this DGK gene than the susceptible line (Fold Change of 1.48). Therefore, the involvement of DGK genes in the L. cicera response against U. pisi remains unclear.
The mitochondrial carrier protein family is over-represented among the stress-responsive genes, suggesting that stress induces altered needs for metabolite transport across the mitochondrial inner membrane (Van Aken et al., 2009). Mitochondrial carriers are highly expressed in stress conditions, such as application of cadmium or auxin, exposure to cold, and induction of cell death. Abscisic acid application, on the other hand, decreased the expression of some mitochondrial carriers (Millar and Heazlewood, 2003). The potential role of L. cicera mitochondrial carriers against U. pisi infection here indicated is in accordance with the previous transcriptomic study (Santos et al., 2018).
To better understand the complex interrelation of genetic variants and expression regulators, the identification of eQTLs for some genes related to L. cicera resistance against U. pisi was integrated in this study. From the initial nine genes selected from the transcriptomic data obtained for L. cicera RIL parental lines (BGE023542 and BGE008277) inoculated with U. pisi (Santos et al., 2018), Gluc (Glycosyl hydrolase family 17) was the only analysed gene which RILs’ expression levels correlated with RILs’ DS scorings in the present study. Glycosyl hydrolases family 17 serves diverse roles in plant defence and development, since it comprises degrading enzymes of 1,3-β-glucan polysaccharides found in the cell wall matrix of plants and fungi (Thomas et al., 2000; Gaudioso-Pedraza and Benitez-Alfonso, 2014). The significant negative correlation (P < 0.01) observed between DS at adult plant stage under semi-controlled field conditions and Gluc relative expression support that this gene expression may increase L. cicera resistance against U. pisi. Indeed, the partial resistant BGE023542 parental line showed higher transcript abundance than BGE008277 for this gene, suggesting that its expression may increase resistance to the pathogen. Positive and negative additive effects were found in the eQTLs for Gluc, indicating that both parental lines may harbour alleles for L. cicera resistance to rust.
Two eQTLs were detected for Gluc RIL expression variation. Strong eQTLs are typically cis-regulated (Võsa et al., 2021), and this was also observed in the present study as GlucIVa eQTL (the only detected cis-eQTL) showed the highest LOD score (9.29) and the highest percentage of explained expression variation (32.2%). Nevertheless, the influence of distal regulations is not trivial and generally numerous plant genes are controlled by distant-factors (Swanson-Wagner et al., 2009; Wang et al., 2010; Hammond et al., 2011; Cubillos et al., 2012). Indeed, a distant-eQTL (trans-eQTL) was detected in the present study associated with the expression variation of Gluc but explaining a smaller percentage of variation.
The candidate genes for the GlucIVb trans-eQTL were proposed to be a SCO1 protein and a rust resistance kinase Lr10. SCO1 protein has been described as playing a role in cellular copper homeostasis and mitochondrial redox signalling (Attallah et al., 2011). Regarding the Lr10 leaf rust resistance gene, in wheat encodes a coiled coil–nucleotide-binding site–leucine-rich repeat (CC–NBS–LRR) (Loutre et al., 2009). The majority of disease resistance genes (R-genes) isolated from plants, conferring resistance to pathogens, encode proteins containing an NBS–LRR domain, used for pathogen perception triggering host response (Jones and Dangl, 2006). To the best of our knowledge, no direct relation between glycosyl hydrolases and SCO1 and Lr10 genes were described so far. Therefore, further studies are crucial to unveil if and how the rust resistance kinase Lr10 and SCO1 may regulate Glycosyl hydrolases family 17 members in response to rust infection in L. cicera.
Noteworthy, the function and position of genes underlying the identified pQTLs and eQTLs should be confirmed when the scaffolding of the assembly of the L. sativus genome (Emmrich et al., 2020) to the pseudochromosome level and gene annotation becomes completed.
Disease resistance genes are commonly clustered in genetic regions, conferring resistance to different pathogens and/or to different races of the same pathogen (Michelmore and Meyers, 1998; Loridon et al., 2005). This has been previously reported in Pisum spp. for fungal and oomycete pathogens (Barilli et al., 2018; Jha et al., 2021; Wu et al., 2021). In L. cicera, a similar situation seems to occur to a certain extent. One of the recently identified QTLs for partial resistance to E. pisi (EpDSIV in Santos et al., 2020) locates within a close distance to pQTLs and eQTLs here identified for partial resistance to U. pisi: about 13.8 cM to the UpDSIV_chamber, 22 cM to the UpDSIVa_field and GlucIVa, and 17.5 cM to the GlucIVb eQTL. Therefore, a QTL hotspot for partial disease resistance to different biotrophic fungi (U. pisi and E. pisi) may be suggested in L. cicera LGIV (considering UpDSIV_chamber, UpDSIVa_field, GlucIVa, and GlucIVb and EpDSIV; Santos et al., 2020).
In the present study, only the Gluc and Pi49 genes showed significant differences in gene expression by RT-qPCR between the two L. cicera parental lines. A motive that might be influencing our results is that different biological material was used on the RNAseq (a pool of equally mixed RNA individually extracted from 24 biological replicates per genotype) vs. the RT-qPCR (3 biological replicates individual RNA samples per genotype). Some residual heterozygosity potentially present in the RIL parental lines might have been exposed during different processing and contribute to the different fold changes (and consequently significant differences) obtained between gene expression of the RIL parental lines using RNA-seq and RT-qPCR techniques. The limited number of DEG from the RIL parental lines RNAseq data screened in the RIL individuals hampered the scope of the integration approach for prioritising candidate genes for chickling pea rust resistance. Nevertheless, the information obtained from the eQTL linkage mapping allowed a better understanding of the complex interrelation of genetic variants and expression regulators of the DEG Gluc.
Different pQTLs for U. pisi partial resistance were identified in L. cicera RIL, suggesting that a different genetic control may be involved in different stages of plant development. The integration of these linkage mapping results and the previously obtained RIL parental lines transcriptomic data (Santos et al., 2018), helped to prioritise QTLs candidate genes (if DEG between the parental lines) as a cross-validation approach. This integration has materialised also by the selection of à priori genes related to resistance to rust from the RIL parental lines transcriptomic study (Santos et al., 2018) to “validate” at the RIL level by RT-qPCR. Taking all the above into consideration, the L. cicera response to U. pisi candidate gene prioritised list for future validation and use in precision breeding is constituted by PPR, DGK, Mitochondrial carrier, and Gluc genes. Furthermore, the presence of a putative hotspot of resistance-related genes in the L. cicera LGIV is suggested. Candidate genes underlying the identified pQTLs/eQTLs hotspot will be useful for a better understanding of the complex interrelation of genetic variants and regulators of expression related to the partial resistance of L. cicera against rust.
The original contributions presented in the study are included in the article/Supplementary Material, further inquiries can be directed to the corresponding author/s.
CS, DCM, and MJG-B contributed to phenotyping and methodology. CS contributed to gene expression analysis, QTL and eQTL analysis, software, and data curation. DR contributed to RIL population development. MCVP and CS contributed to conceptualisation, formal analysis, and writing—original draft preparation. CS, DCM, DR, and MCVP contributed to writing, reviewing, and editing. MCVP and DR contributed to funding acquisition, supervision, and resources. MCVP contributed to project administration. All authors have read and agreed to the published version of the manuscript.
This work was supported by the EU project LEGATO (FP7 grant agreement no. 613551), the Spanish Agencia Estatal Investigación project PID2020-11468RB-100, and the Portuguese Fundação para a Ciência e Tecnologia project PTDC/AGR-TEC/0992/2014 and Research Unit UID/04551/2020. CS and DCM were also supported by Fundação para a Ciência e Tecnologia: CEECIND/00198/2017, Research Contract by Stimulus of Scientific Employment, Individual Support 2017 program; and PD/BD/128498/2017, Ph.D. grant, respectively.
The authors declare that the research was conducted in the absence of any commercial or financial relationships that could be construed as a potential conflict of interest.
All claims expressed in this article are solely those of the authors and do not necessarily represent those of their affiliated organizations, or those of the publisher, the editors and the reviewers. Any product that may be evaluated in this article, or claim that may be made by its manufacturer, is not guaranteed or endorsed by the publisher.
We thank the CRF-INIA, Madrid, Spain for supplying the parental genotypes. We also acknowledge Nuno Almeida for inoculating and collecting plant material used for eQTL analysis. The authors are grateful to Eleonora Barilli for assisting with the maintenance of U. pisi isolate.
The Supplementary Material for this article can be found online at: https://www.frontiersin.org/articles/10.3389/fpls.2022.837613/full#supplementary-material
Supplementary Figure 1 | Leaves of Lathyrus cicera inoculated with Uromyces pisi, showing a compatible reaction between plant and pathogen (IT = 4), (A) under controlled conditions (growth chamber) 11 days after inoculation with U. pisi and (B) under semi-controlled field conditions 3 months after inoculation with U. pisi.
Supplementary Figure 2 | Expression QTLs syntenic regions of Lathyrus cicera recombinant inbred lines population (BGE023542 × BGE008277) for rust (Uromyces pisi) response mapped on the physical map of Pisum sativum. Physical distances given in Mbp are indicated for each P. sativum chromosome. Horizontal pink line indicates the syntenic position of L. cicera differentially expressed gene in P. sativum chromosomes. Black horizontal lines represent the syntenic regions of L. cicera eQTL intervals mapped on P. sativum genome.
Albert, F. W., and Kruglyak, L. (2015). The role of regulatory variation in complex traits and disease. Nat. Rev. Genet. 16, 197–212. doi: 10.1038/NRG3891
Almeida, N. F., Krezdorn, N., Rotter, B., Winter, P., Rubiales, D., and Vaz Patto, M. C. (2015). Lathyrus sativus transcriptome resistance response to Ascochyta lathyri investigated by deepSuperSAGE analysis. Front. Plant Sci. 6:178. doi: 10.3389/fpls.2015.00178
Attallah, C. V., Welchen, E., Martin, A. P., Spinelli, S. V., Bonnard, G., Palatnik, J. F., et al. (2011). Plants contain two SCO proteins that are differentially involved in cytochrome c oxidase function and copper and redox homeostasis. J. Exp. Bot. 62, 4281–4294. doi: 10.1093/JXB/ERR138
Barilli, E., Cobos, M. J., Carrillo, E., Kilian, A., Carling, J., and Rubiales, D. (2018). A high-density integrated DArTseq SNP-based genetic map of Pisum fulvum and identification of QTLs controlling rust resistance. Front. Plant Sci. 9:167. doi: 10.3389/fpls.2018.00167
Barilli, E., Moral, A., Sillero, J. C., and Rubiales, D. (2012). Clarification on rust species potentially infecting pea (Pisum sativum L.) crop and host range of Uromyces pisi (Pers.) Wint. Crop Prot. 37, 65–70. doi: 10.1016/j.cropro.2012.01.019
Barilli, E., Satovic, Z., Rubiales, D., and Torres, A. M. (2010). Mapping of quantitative trait loci controlling partial resistance against rust incited by Uromyces pisi (Pers.) Wint. in a Pisum fulvum L. intraspecific cross. Euphytica 175, 151–159. doi: 10.1007/s10681-010-0141-z
Barilli, E., Sillero, J. C., Fernández-Aparicio, M., and Rubiales, D. (2009a). Identification of resistance to Uromyces pisi (Pers.) Wint. in Pisum spp. germplasm. Field Crops Res. 114, 198–203. doi: 10.1016/j.fcr.2009.07.017
Barilli, E., Sillero, J. C., Moral, A., and Rubiales, D. (2009b). Characterization of resistance response of pea (Pisum spp.) against rust (Uromyces pisi). Plant Breed. 128, 665–670. doi: 10.1111/j.1439-0523.2008.01622.x
Barilli, E., Sillero, J. C., Serrano, A., and Rubiales, D. (2009c). Differential response of pea (Pisum sativum) to rusts incited by Uromyces viciae-fabae and U. pisi. Crop Prot. 28, 980–986. doi: 10.1016/j.cropro.2009.06.010
Carrasco-Valenzuela, T., Muñoz-Espinoza, C., Riveros, A., Pedreschi, R., Arús, P., Campos-Vargas, R., et al. (2019). Expression QTL (eQTLs) analyses reveal candidate genes associated with fruit flesh softening rate in peach [Prunus persica (L.) Batsch]. Front. Plant Sci. 10:1581. doi: 10.3389/fpls.2019.01581
Cubillos, F. A., Coustham, V., and Loudet, O. (2012). Lessons from eQTL mapping studies: Non-coding regions and their role behind natural phenotypic variation in plants. Curr. Opin. Plant Biol. 15, 192–198. doi: 10.1016/j.pbi.2012.01.005
Dakouri, A., McCallum, B. D., Radovanovic, N., and Cloutier, S. (2013). Molecular and phenotypic characterization of seedling and adult plant leaf rust resistance in a world wheat collection. Mol. Breed. 32, 663–677. doi: 10.1007/S11032-013-9899-8
Ellis, J. G., Lagudah, E. S., Spielmeyer, W., and Dodds, P. N. (2014). The past, present and future of breeding rust resistant wheat. Front. Plant Sci. 5:641. doi: 10.3389/FPLS.2014.00641
Emeran, A. A., Sillero, J. C., Fernández-Aparicio, M., and Rubiales, D. (2011). Chemical control of faba bean rust (Uromyces viciae-fabae). Crop Prot. 30, 907–912. doi: 10.1016/j.cropro.2011.02.004
Emmrich, P. M. F., Sarkar, A., Njaci, I., Kaithakottil, G. G., Ellis, N., Moore, C., et al. (2020). A draft genome of grass pea (Lathyrus sativus), a resilient diploid legume. Biorxiv doi: 10.1101/2020.04.24.058164
Fauteux, F., Wang, Y., Rocheleau, H., Liu, Z., Pan, Y., Fedak, G., et al. (2019). Characterization of QTL and eQTL controlling early Fusarium graminearum infection and deoxynivalenol levels in a Wuhan 1 x Nyubai doubled haploid wheat population. BMC Plant Biol. 19:536. doi: 10.1186/s12870-019-2149-4
Foka, I. C. K., Ketehouli, T., Zhou, Y., Li, X.-W., Wang, F.-W., and Li, H. (2020). The emerging roles of Diacylglycerol Kinase (DGK) in plant stress tolerance, growth, and development. Agronomy 10:1375. doi: 10.3390/AGRONOMY10091375
Gaudioso-Pedraza, R., and Benitez-Alfonso, Y. (2014). A phylogenetic approach to study the origin and evolution of plasmodesmata-localized glycosyl hydrolases family 17. Front. Plant Sci. 5:212. doi: 10.3389/FPLS.2014.00212
Hammer, K., Laghetti, G., Direnzo, P., Castelli, A., and Mikić, A. (2019). Resources and opportunities for re-establishing Lathyrus cicera L. as a multipurpose cultivated plant. Genet. Resour. Crop Evol. 66, 523–544. doi: 10.1007/s10722-018-0717-3
Hammond, J. P., Mayes, S., Bowen, H. C., Graham, N. S., Hayden, R. M., Love, C. G., et al. (2011). Regulatory hotspots are associated with plant gene expression under varying soil phosphorus supply in Brassica rapa. Plant Physiol. 156, 1230–1241. doi: 10.1104/pp.111.175612
Hanbury, C., White, C., Mullan, B., and Siddique, K. H. (2000). A review of the potential of Lathyrus sativus L. and L. cicera L. grain for use as animal feed. Anim. Feed Sci. Technol. 87, 1–27. doi: 10.1016/S0377-8401(00)00186-3
Hansen, B. G., Halkier, B. A., and Kliebenstein, D. J. (2008). Identifying the molecular basis of QTLs: eQTLs add a new dimension. Trends Plant Sci. 13, 72–77. doi: 10.1016/j.tplants.2007.11.008
Hunter, S., Apweiler, R., Attwood, T. K., Bairoch, A., Bateman, A., Binns, D., et al. (2009). InterPro: the integrative protein signature database. Nucleic Acids Res. 37, D211–D215. doi: 10.1093/nar/gkn785
Jansen, R. C., and Stam, P. (1994). High resolution of quantitative traits into multiple loci via interval mapping. Genetics 136, 1447–1455. doi: 10.1093/genetics/136.4.1447
Jha, A. B., Gali, K. K., Alam, Z., Lachagari, V. B. R., and Warkentin, T. D. (2021). Potential application of genomic technologies in breeding for fungal and oomycete disease resistance in pea. Agronomy 11:1260. doi: 10.3390/AGRONOMY11061260
Jones, J., and Dangl, J. (2006). The plant immune system. Nature 444, 323–329. doi: 10.1038/nature05286
Koussevitzky, S., Nott, A., Mockler, T. C., Hong, F., Sachetto-Martins, G., Surpin, M., et al. (2007). Signals from chloroplasts converge to regulate nuclear gene expression. Science 316, 715–719. doi: 10.1126/science.
Kreplak, J., Madoui, M.-A., Cápal, P., Novák, P., Labadie, K., Aubert, G., et al. (2019). A reference genome for pea provides insight into legume genome evolution. Nat. Genet. 51, 1411–1422. doi: 10.1038/s41588-019-0480-1
Laluk, K., AbuQamar, S., and Mengiste, T. (2011). The Arabidopsis mitochondria-localized pentatricopeptide repeat rotein PGN unctions in defense against necrotrophic fungi and abiotic stress tolerance. Plant Physiol. 156, 2053–2068. doi: 10.1104/pp.111.177501
Lambein, F., Travella, S., Kuo, Y.-H., Van Montagu, M., and Heijde, M. (2019). Grass pea (Lathyrus sativus L.): orphan crop, nutraceutical or just plain food? Planta 250, 821–838. doi: 10.1007/s00425-018-03084-0
Lander, E. S., and Botstein, D. (1989). Mapping mendelian factors underlying quantitative traits using RFLP linkage maps. Genetics 121, 185–199. doi: 10.1093/genetics/121.1.185
Li, J., and Burmeister, M. (2005). Genetical genomics: combining genetics with gene expression analysis. Hum. Mol. Genet. 14, R163–R169. doi: 10.1093/hmg/ddi267
Lima, R. P. M., Curtolo, M., Merfa, M. V., Cristofani-Yaly, M., and Machado, M. A. (2018). QTLs and eQTLs mapping related to citrandarins’ resistance to Citrus gummosis disease. BMC Genomics 19:516. doi: 10.1186/s12864-018-4888-2
Liu, H., Luo, X., Niu, L., Xiao, Y., Chen, L., Liu, J., et al. (2017). Distant eQTLs and non-coding sequences play critical roles in regulating gene expression and quantitative trait variation in maize. Mol. Plant 10, 414–426. doi: 10.1016/J.MOLP.2016.06.016
Loridon, K., McPhee, K., Morin, J., Dubreuil, P., Pilet-Nayel, M. L., Aubert, G., et al. (2005). Microsatellite marker polymorphism and mapping in pea (Pisum sativum L.). Theor. Appl. Genet. 111, 1022–1031. doi: 10.1007/s00122-005-0014-3
Loutre, C., Wicker, T., Travella, S., Galli, P., Scofield, S., Fahima, T., et al. (2009). Two different CC-NBS-LRR genes are required for Lr10-mediated leaf rust resistance in tetraploid and hexaploid wheat. Plant J. 60, 1043–1054. doi: 10.1111/J.1365-313X.2009.04024.X
Lurin, C., Andrés, C., Aubourg, S., Bellaoui, M., Bitton, F., Bruyère, C., et al. (2004). Genome-wide analysis of Arabidopsis pentatricopeptide repeat proteins reveals their essential role in organelle biogenesis. Plant Cell 16, 2089–2103. doi: 10.1105/tpc.104.022236
Martins, D., De Sousa Araújo, S., Rubiales, D., and Vaz Patto, M. C. (2020). Legume crops and biotrophic pathogen interactions: a continuous cross-talk of a multilayered array of defense mechanisms. Plants 9:1460. doi: 10.3390/plants9111460
McDonald, B. A., and Linde, C. (2002). Pathogen population genetics, evolutionary potential, and durable resistance. Annu. Rev. Phytopathol. 40, 349–379. doi: 10.1146/annurev.phyto.40.120501.101443
Michelmore, R. W., and Meyers, B. C. (1998). Clusters of resistance genes in plants evolve by divergent selection and a birth-and-death process. Genome Res. 8, 1113–1130. doi: 10.1101/gr.8.11.1113
Miculan, M., Nelissen, H., Hassen, M., Ben Marroni, F., Inzé, D., Pè, M. E., et al. (2021). A forward genetics approach integrating genome-wide association study and expression quantitative trait locus mapping to dissect leaf development in maize (Zea mays). Plant J. 107, 1056–1071. doi: 10.1111/TPJ.15364
Millar, A. H., and Heazlewood, J. L. (2003). Genomic and proteomic analysis of mitochondrial carrier proteins in Arabidopsis. Plant Physiol. 131, 443–453. doi: 10.1104/PP.009985
Niks, R. E., and Rubiales, D. (2002). Potentially durable resistance mechanisms in plants to specialised fungal pathogens. Euphytica 124, 201–216. doi: 10.1023/A:1015634617334
Park, Y. J., Lee, H. J., Kwak, K. J., Lee, K., Hong, S. W., and Kang, H. (2014). MicroRNA400-guided cleavage of pentatricopeptide repeat protein mrnas renders Arabidopsis thaliana more susceptible to pathogenic bacteria and fungi. Plant Cell Physiol. 55, 1660–1668. doi: 10.1093/pcp/pcu096
Peña-Chocarro, L., and Peña, L. Z. (1999). History and traditional cultivation of Lathyrus sativus L. and Lathyrus cicera L. in the Iberian peninsula. Veg. Hist. Archaeobot. 8, 49–52. doi: 10.2307/23417642
Pfaffl, M. W. (2001). A new mathematical model for relative quantification in real-time RT-PCR. Nucleic Acids Res. 29:e45. doi: 10.1093/nar/29.9.e45
Rubiales, D., Castillejo, M. A., Madrid, E., Barilli, E., and Rispail, N. (2011). Legume breeding for rust resistance: lessons to learn from the model Medicago truncatula. Euphytica 180, 89–98. doi: 10.1007/s10681-011-0367-4
Rubiales, D., Fondevilla, S., Chen, W., Gentzbittel, L., Higgins, T. J. V., Castillejo, M. A., et al. (2015). Achievements and challenges in legume breeding for pest and disease resistance. Crit. Rev. Plant Sci. 34, 195–236. doi: 10.1080/07352689.2014.898445
Santos, C., Almeida, N. F., Alves, M. L., Horres, R., Krezdorn, N., Leitão, S. T., et al. (2018). First genetic linkage map of Lathyrus cicera based on RNA sequencing-derived markers: Key tool for genetic mapping of disease resistance. Hortic. Res. 5:45. doi: 10.1038/s41438-018-0047-9
Santos, C., Martins, D., Rubiales, D., and Vaz Patto, M. C. (2020). Partial resistance against Erysiphe pisi and E. trifolii under different genetic control in Lathyrus cicera: outcomes from a linkage mapping approach. Plant Dis. 104, 2875–2884. doi: 10.1094/PDIS-03-20-0513-RE
Schaefer, H., Hechenleitner, P., Santos-Guerra, A., Menezes de Sequeira, M., Pennington, R. T., Kenicer, G., et al. (2012). Systematics, biogeography, and character evolution of the legume tribe Fabeae with special focus on the middle Atlantic island lineages. BMC Evol. Biol. 12:250. doi: 10.1186/1471-2148-12-250
Sillero, J. C., Fondevilla, S., Davidson, J., Vaz Patto, M. C., Warkentin, T. D., Thomas, J., et al. (2006). Screening techniques and sources of resistance to rusts and mildews in grain legumes. Euphytica 147, 255–272. doi: 10.1007/s10681-006-6544-1
Smýkal, P., Kenicer, G., Flavell, A., Corander, J., Kosterin, O., Redden, R., et al. (2011). Phylogeny, phylogeography and genetic diversity of the Pisum genus. Plant Genet. Res. 9, 4–18. doi: 10.1017/S147926211000033X
Stakman, E. C., Stewart, D. M., and Loegering, W. Q. (1662). Identification of Physiologic Races of Puccinia Graminis Var Tritici. United States: CABI.
Stackman, E. C., Stewart, D. M., and Loegering, W. Q. (1962). Identification of physiologic races of Puccinia graminis var. tritici. Washington, DC: USDA, Agricultural Research Service, E617.
Swanson-Wagner, R. A., Decook, R., Jia, Y., Bancroft, T., Ji, T., Zhao, X., et al. (2009). Paternal dominance of trans-eQTL influences gene expression patterns in maize hybrids. Science 326, 1118–1120. doi: 10.1126/science.1178294
Thomas, B. R., Romero, G. O., Nevins, D. J., and Rodriguez, R. L. (2000). New perspectives on the endo-beta-glucanases of glycosyl hydrolase Family 17. Int. J. Biol. Macromol. 27, 139–144. doi: 10.1016/S0141-8130(00)00109-4
Toyoda, K., Kawahara, T., Ichinose, Y., Yamada, T., and Shiraishi, T. (2000). Potentiation of phytoalexin accumulation in elicitor-treated epicotyls of pea (Pisum sativum) by a diacylglycerol kinase inhibitor. J. Phytopathol. 148, 633–636. doi: 10.1111/J.1439-0434.2000.00568.X
Van Aken, O., Zhang, B., Carrie, C., Uggalla, V., Paynter, E., Giraud, E., et al. (2009). Defining the mitochondrial stress response in Arabidopsis thaliana. Mol. Plant 2, 1310–1324. doi: 10.1093/MP/SSP053
Van Ooijen, J. W. (2009). MapQTL 6, Software for the Mapping of Quantitative Trait Loci in Experimental Populations of Diploid Species. Wageningen: Kyazma B. V.
Vaz Patto, M. C., Fernández-Aparicio, M., Moral, A., and Rubiales, D. (2009). Pre and posthaustorial resistance to rusts in Lathyrus cicera L. Euphytica 165, 27–34. doi: 10.1007/s10681-008-9737-y
Vaz Patto, M. C., and Rubiales, D. (2009). Identification and characterization of partial resistance to rust in a germplasm collection of Lathyrus sativus L. Plant Breed. 128, 495–500. doi: 10.1111/j.1439-0523.2008.01601.x
Vaz Patto, M. C., and Rubiales, D. (2014). Lathyrus diversity: available resources with relevance to crop improvement – L. sativus and L. cicera as case studies. Ann. Bot. 113, 895–908. doi: 10.1093/aob/mcu024
Vaz Patto, M. C., Skiba, B., Pang, E. C. K., Ochatt, S. J., Lambein, F., and Rubiales, D. (2006). Lathyrus improvement for resistance against biotic and abiotic stresses: From classical breeding to marker assisted selection. Euphytica 147, 133–147. doi: 10.1007/s10681-006-3607-2
Voorrips, R. E. (2002). MapChart: software for the graphical presentation of linkage maps and QTLs. J. Hered. 93, 77–78. doi: 10.1093/JHERED/93.1.77
Võsa, U., Claringbould, A., Westra, H. J., Bonder, M. J., Deelen, P., Zeng, B., et al. (2021). Large-scale cis- and trans-eQTL analyses identify thousands of genetic loci and polygenic scores that regulate blood gene expression. Nat. Genet. 53, 1300–1310. doi: 10.1038/s41588-021-00913-z
Wallace, J. G., Larsson, S. J., and Buckler, E. S. (2013). Entering the second century of maize quantitative genetics. Heredity 112, 30–38. doi: 10.1038/hdy.2013.6
Wang, G., Kong, H., Sun, Y., Zhang, X., Zhang, W., Altman, N., et al. (2004). Genome-wide analysis of the cyclin family in Arabidopsis and comparative phylogenetic analysis of plant cyclin-like proteins. Plant Physiol. 135, 1084–1099. doi: 10.1104/PP.104.040436
Wang, J., Yu, H., Xie, W., Xing, Y., Yu, S., Xu, C., et al. (2010). A global analysis of QTLs for expression variations in rice shoots at the early seedling stage. Plant J. 63, 1063–1074. doi: 10.1111/j.1365-313X.2010.04303.x
Wang, X., Chen, Q., Wu, Y., Lemmon, Z. H., Xu, G., Huang, C., et al. (2018). Genome-wide analysis of transcriptional variability in a large maize-teosinte population. Mol. Plant 11, 443–459. doi: 10.1016/J.MOLP.2017.12.011
Wu, L., Fredua-Agyeman, R., Hwang, S.-F., Chang, K.-F., Conner, R. L., McLaren, D. L., et al. (2021). Mapping QTL associated with partial resistance to Aphanomyces root rot in pea (Pisum sativum L.) using a 13.2 K SNP array and SSR markers. Theor. Appl. Genet. 134, 2965–2990. doi: 10.1007/S00122-021-03871-6
Xing, H., Fu, X., Yang, C., Tang, X., Guo, L., Li, C., et al. (2018). Genome-wide investigation of pentatricopeptide repeat gene family in poplar and their expression analysis in response to biotic and abiotic stresses. Sci. Rep. 8:2817. doi: 10.1038/s41598-018-21269-1
Keywords: quantitative trait loci-QTL, expression QTL-eQTL, Lathyrus cicera, Uromyces pisi, partial resistance, QTL hotspots
Citation: Santos C, Martins DC, González-Bernal MJ, Rubiales D and Vaz Patto MC (2022) Integrating Phenotypic and Gene Expression Linkage Mapping to Dissect Rust Resistance in Chickling Pea. Front. Plant Sci. 13:837613. doi: 10.3389/fpls.2022.837613
Received: 16 December 2021; Accepted: 23 February 2022;
Published: 07 April 2022.
Edited by:
Marcelino Perez De La Vega, Universidad de León, SpainReviewed by:
Debjyoti Sen Gupta, Indian Institute of Pulses Research (ICAR), IndiaCopyright © 2022 Santos, Martins, González-Bernal, Rubiales and Vaz Patto. This is an open-access article distributed under the terms of the Creative Commons Attribution License (CC BY). The use, distribution or reproduction in other forums is permitted, provided the original author(s) and the copyright owner(s) are credited and that the original publication in this journal is cited, in accordance with accepted academic practice. No use, distribution or reproduction is permitted which does not comply with these terms.
*Correspondence: Carmen Santos, Y3NzQGl0cWIudW5sLnB0; Maria Carlota Vaz Patto, Y3BhdHRvQGl0cWIudW5sLnB0
Disclaimer: All claims expressed in this article are solely those of the authors and do not necessarily represent those of their affiliated organizations, or those of the publisher, the editors and the reviewers. Any product that may be evaluated in this article or claim that may be made by its manufacturer is not guaranteed or endorsed by the publisher.
Research integrity at Frontiers
Learn more about the work of our research integrity team to safeguard the quality of each article we publish.