- 1HortiCell, Department of Plants and Crops, Faculty of Bioscience Engineering, Ghent University, Ghent, Belgium
- 2Laboratoire de Chimie Agro-Industrielle, Université de Toulouse, Institut National de Recherche pour l’Agriculture, l’Alimentation et l’Environnement (INRAE), École Nationale Supérieure des Ingénieurs en Arts Chimiques et Technologiques (ENSIACET), Toulouse, France
- 3Ovalie Innovation, Auch, France
- 4Biotechnology Research and Development Institute (BiRDI), Can Tho University, Can Tho, Vietnam
- 5Flanders Research Institute for Agriculture, Fisheries and Food (ILVO), Melle, Belgium
- 6SynBioC, Department of Green Chemistry and Technology, Faculty of Bioscience Engineering, Ghent University, Ghent, Belgium
A survey of plant-based wastes identified sunflower (Helianthus annuus) bark extract (SBE), produced via twin-screw extrusion, as a potential biostimulant. The addition of SBE to Arabidopsis (Arabidopsis thaliana) seedlings cultured in vitro showed a dose-dependent response, with high concentrations causing severe growth inhibition. However, when priming seeds with SBE, a small but significant increase in leaf area was observed at a dose of 0.5 g of lyophilized powder per liter. This optimal concentration of SBE in the culturing medium alleviated the growth inhibition caused by 100 mM NaCl. The recovery in shoot growth was accompanied by a pronounced increase in photosynthetic pigment levels and a stabilization of osmotic homeostasis. SBE-primed leaf discs also showed a similar protective effect. SBE mitigated salt stress by reducing the production of reactive oxygen species (ROS) (e.g., hydrogen peroxide) by about 30% and developing more expanded true leaves. This reduction in ROS levels was due to the presence of antioxidative agents in SBE and by activating ROS-eliminating enzymes. Polyphenols, carbohydrates, proteins, and other bioactive compounds detected in SBE may have contributed to the cellular redox homeostasis in salt-stressed plants, thus promoting early leaf development by relieving shoot apical meristem arrest. Sunflower stalks from which SBE is prepared can therefore potentially be valorized as a source to produce biostimulants for improving salt stress tolerance in crops.
Introduction
Substantial losses in biomass accompany crop production and downstream processing because of inadequate harvesting methods and a lack of valorization of by-products (Parfitt et al., 2010). To reduce the ecological footprint of agricultural practices, the Food and Agriculture Organization of the United Nations (FAO) identified two primary targets: “agricultural sustainability” and “global food losses” (FAO, 2021). In view of these targets, we urgently need to transform agricultural waste into value-added products. Crop waste is a natural resource for refining and recovering bioactive ingredients (Van Tang, 2017). Indeed, various molecules are abundant in unused biomass, some of which can be developed as plant biostimulants (PBs) (Xu and Geelen, 2018; Huang et al., 2021). The development and commercialization of PBs is a rapidly growing business, estimated at USD 3.2 billion in 2021 with a projected compound annual growth rate (CAGR) of 12.1% (Markets and Markets, 2021). Strikingly, plant extract-based PBs exhibited the highest effectiveness in yield enhancement of field crops (Li et al., 2022). Compared with synthetic chemical additives for crop improvement, PBs derived from natural resources like plant byproducts are poised to encompass a lower environmental risk and impact (Kumar et al., 2019). PBs are more likely to pass the regulatory restriction of fertilizers from natural origins imposed by legislation (Regulation [EU], 2019).
The main methods of PBs application are foliar spraying, seed priming, and soil drenching (Vertified Market Research, 2021). Hence, most primary screening assays are designed to screen putative biostimulant activity starting from seed germination and the growth responses of seedlings (García-García et al., 2020). The monitoring of seedling growth allows for in vitro assays under controlled conditions, short evaluation periods, and assessment of a broad spectrum of responses (Colegate and Molyneux, 2007). Subsequent bioassays are dedicated to monitoring specific plant responses. For example, seed priming tests report the effect of chemical reagents on seed germination and early seedling development (Lutts et al., 2016). Arabidopsis (Arabidopsis thaliana) seedlings grown in vitro are widely used to study the effects of exogenous chemicals on root and shoot growth (Trinh et al., 2018). An alternative to growth assays is measuring the longevity of mature leaf discs punched from mature leaves that normally senescence in a matter of days (Chiu et al., 2021). In vitro bioassays also allow for the quantitative impact of stress responses, and the combining of the results from multiple assays provides a reasonable indication for possible biostimulant activity under field conditions.
Plant-based raw materials are typically rich in diverse metabolites (Zulfiqar et al., 2020). Various plant extracts have also improved stress tolerance, often attributed to antioxidants (De Diego and Spíchal, 2020). Polyphenols, abundant in many plant extracts, are a class of bioactive antioxidants that scavenge in vitro and in vivo reactive oxygen species (ROS) (Stagos, 2020). For instance, many polyphenols are found in various bark by-products from woody species such as oak and willow (Dróżdż and Pyrzynska, 2017; Dou et al., 2018; Tanase et al., 2019). Sunflower (Helianthus annuus) seeds and florets are also rich in polyphenols with antioxidant activity (Karamać et al., 2012; Ye et al., 2015). The trichomes isolated from the surface of sunflower stems contain many flavonoids, which are typically showing antioxidant activity (Brentan Silva et al., 2017).
Sunflower is an annual crop produced for its seed and is the fourth most important oilseed crop responsible for 10% of the world’s edible plant-derived oil (Dantas et al., 2017). The leaves and stems are usually not harvested and left on the field as organic compost. The worldwide production of sunflower foliage and stems is an estimated 15.2 megatons per year (United Nations Industrial Development, 2007). Because of this substantial amount of biomass, stem material is considered a source of fiber used in biocomposite panels and other fiber-rich materials. The stalks are separated into the bark (external “woody” part, 90% w/w), which is rich in lignocellulose, and the pith (internal part, 10% w/w) (Evon et al., 2018; Verdier et al., 2020). In addition to fiber, the stalks can potentially be refined through the advanced twin-screw extrusion technology and used for various added-value applications in the agrochemical industry.
This study shows that (1) sunflower bark extract (SBE) can be produced as a side stream during twin-screw extrusion of fiber from stems; (2) SBE is a complex mixture of water-soluble molecules, several of which have bioactivity on plant growth; (3) exogenous application of SBE mitigates salt stress-induced growth inhibition of in vitro grown Arabidopsis.
Materials and Methods
Sunflower Stalk Collection and Bark Extraction
Sunflower stalks were collected with a forage harvester with the assistance of Ovalie Innovation in Autumn 2018 (Samaran, Gers department, southwest France). The stalks were stored in a ventilated box and dried with ventilated air at 40°C for 24 h. The bark was mechanically separated from the pith using a three-step procedure: (1) grinding of stalks using a hammer mill (Electra Goulu N, France) fitted with a 32 mm sieve; (2) de-dusting of the ground material using a vibrating sieve shaker (Ritec 600, France) equipped with a 1 mm screen; (3) aspiration of pith particles. Pith and bark particles were separated based on their differential density (i.e., 30 and 140 kg/m3, respectively).
Here, the twin-screw extrusion technology was used as an innovative technique for the thermo-mechanical and organic solvent-free extraction of biomolecules (Evon et al., 2018; Vandenbossche et al., 2019). The bark was then fractionated into a pulp and a liquid extract made of water-soluble compounds using a co-rotating and co-penetrating twin-screw extruder (Clextral Evolum HT 53, France). The extruder barrel (1.9 m in length) consisted of eight modules, each 4D in length (D is the screw diameter, i.e., 53 mm), except for module 1, which had an 8D length. A filter section consisting of six hemispherical dishes with 1 mm diameter perforations outfitted on module 7 enabled filtrate collection. During the liquid/solid fractionation process, bark with 10.0 ± 0.1% moisture content was fed at the level of module 1 using a gravimetric feeder. Water was injected at the end of module 2 using a piston pump at a liquid/solid ratio of 2.9 (i.e., 10.2 and 29.6 kg/h for the inlet flow rates of bark and water, respectively). For optimal operation, a specific screw configuration was applied. Bilobe paddles (BL22) were positioned in module 5 to favor strong mixing between the liquid and the solid. In addition, reversed pitch screws (CF2C) were positioned in module 8, immediately downstream from the filtering sieves to separate the liquid (i.e., the filtrate) and solid (i.e., the pulp) phases continuously by compression action. The temperature was set at 80°C in module 2, at 100°C along the extracting zone (modules 3–6), and 110°C in the pressing part (module 8). The rotation speed of the screws was set at 250 rpm.
The filtrate collected at the bottom of the filtration module (module 7) was centrifuged to remove the small solid particles and driven through the filter. Then, the clarified filtrate was concentrated by partial water evaporation and freeze-dried, producing SBE as a powder product stored in the dark at 4°C until use.
Chemical Characterization of Starting Sunflower Bark and Sunflower Bark Extract
The moisture and dry matter contents of solids in the starting sunflower bark were determined according to ISO 665:2000 (ISO, 2000). The mineral content of starting bark was quantified according to ISO 749:1977 (ISO, 1977), and lipids were assessed according to ISO 659:2009 (ISO, 2009). Cell wall polymers, including cellulose, hemicellulose, and lignin, were quantified using the ADF–NDF method (ADF for acid detergent fiber, and NDF for neutral detergent fiber) of Van Soest and Wine (1967, 1968). After 1 h of boiling in water, water-soluble compounds were calculated from biomass losses.
Inside the SBE, the soluble protein and digestible carbohydrate contents were analyzed by colorimetric methods (Deans et al., 2018). Total phenolics content (TPC) was estimated by the Folin–Ciocalteu method (Sánchez-Rangel et al., 2013). The total flavonoid content (TFC) was measured following two aluminum complexation methods using quercetin and rutin as reference flavonoids (Pękal and Pyrzynska, 2014). The total in vitro antioxidant capacity (TAC) of SBE was determined using the 2,2-diphenyl-1-picrylhydrazyl (DPPH) and the 2,2′-azino-bis (3-ethylbenzothiazoline-6-sulfonic acid) (ABTS) assay (Xiao et al., 2020). Trolox (TE) was used as a standard antioxidant to calculate the equivalent antioxidant capacity of samples. The DPPH assay was slightly modified (Xiao et al., 2020). A total of 20 μl sample was added with 100 μl 200 mM DPPH and 80 μl 50 mM Tris–HCl buffer (pH 7.4).
Chemical Profiling of Sunflower Bark Extract Using UHPLC-PDA-High-Resolution Mass Spectrometer Analysis
Sunflower bark extract was dissolved in water at 1 mg/mL and pushed through a 0.22 μm filter (Millix-GV, Millipore). Samples were subjected to ultra-high-performance liquid chromatography (Acquity UPLC) coupled to a PDA detector (UPLC eLambda 800 nm) and a SYNAPT G2-S High-Resolution Mass Spectrometer (HRMS) (Waters, Milford, MA, United States). Prepared samples were chromatographically separated on an ACQUITY UPLC BEH C18 column (1.7 μm, 2.1 mm × 150 mm) protected by an ACQUITY UPLC BEH C18 VanGuard Precolumn (1.7 μm, 2.1 mm × 5 mm) (Waters). The mobile phase A was 0.1% formic acid in water (solvent A) and the mobile phase B was 0.1% formic acid in acetonitrile (solvent B) at a flow rate of 0.35 mL/min with following gradient: 95% A–5% B (0–18 min), 100% B (18–25 min), 95% A–5% B (25.1–30 min). PDA data was recorded between 220 and 550 nm. Ions were detected in the positive electrospray ionization (ESI+) and negative electrospray ionization (ESI−) modes. The ESI conditions were set as follows: capillary voltage of 3.0 kV (ESI+)/2.0 kV (ESI−), source temperature of 120°C, cone voltage of 30 V (ESI+)/40 V (ESI−), and desolvation temperature of 500°C with a desolvation gas flow of 800 L/h. The collision-induced dissociation (CID) was set at 4 eV for precursor ion, and MS/MS fragment ion information was obtained with a collision energy ramp from 8 to 40 eV. The HRMS was calibrated between 50 and 1200 Da with a sodium formate solution prior to analysis. The injection volume was set at 5 μl, and each sample was analyzed in duplicate. All data were recorded at resolution mode (20,000 FWHM) in centroid full scan MSe mode (data-independent acquisition, DIA). A 200 pg/μl leucine enkephalin solution was continuously infused during analysis to perform lockmass correction (m/z 556.2771 in positive ion mode and m/z 554.2615 in negative ion mode) during analysis. Blanks containing only the mobile phase without any sample were injected between each batch of samples.
To determine the chemical composition of SBE, the raw MS/MS data were processed for feature detection and alignment with MS-DIAL software version 4.48 (Blaženović et al., 2017). The detailed settings are listed in Supplementary Table 1, and are adapted from Lee et al. (2019). To gain a high confidence in the peak identification, only the features present in both runs from the same sample were considered for alignment correction. Next, aligned features meeting the criteria were selected with a total weighted similarity score of over 60 (overall library-matching score based on retention time, accurate mass, isotope ratio, and MSE spectra) (Tsugawa et al., 2015). Then, we searched these chosen features for further compound prediction in MS-FINDER software version 3.50 (Tsugawa et al., 2016) among online metabolites databases, including PlantCyc (plant), KNApSAcK (natural product), FoodDB (Food), ChEBI (Biomolecules), and PubChem (Biomolecules), with the agreement of identification confidence levels (level 3) (Schymanski et al., 2014). In each feature, the predicted formula and structure with the top total score were reported as the final candidate compound (Tsugawa et al., 2019). Finally, the identified compounds were assigned taxonomy based on their chemical characterization represented as InChIKey (International Chemical Identifier) in ChemOnt ontology via ClassyFire (Djoumbou Feunang et al., 2016).
Pesticide Residue Detection in Sunflower Bark Extract
Lyophilized powder of SBE was analyzed for the presence of over 500 pesticide residues (Regulation [EC], 2005, 2009) (Primoris, Belgium). Briefly, the pesticide(s) were extracted from the crude sunflower bark material with acidified acetonitrile (QuEChERS extraction) or acetonitrile with 0.5% acetic acid. Pesticides were quantitatively determined using LC–MS/MS and GC–MS/MS, respectively. No chemicals above the maximum residue levels were reported (data not shown).
Plant-Based Bioassays Using Arabidopsis as the Model Plant
Root Development
Arabidopsis (Col-0) seeds were chlorine gas-sterilized for 3 h (Lindsey et al., 2017), sown on full strength Murashige and Skoog medium, and vernalized for 3 days at 4°C (Trinh et al., 2018). Next, the plates were exposed 8 h to light (room temperature 21.4°C, 40–60% humidity, light intensity 140 μmol m–2 s–1, 14/10 h day/night photoperiod) and transferred to the dark for another 3 days to induce etiolation. Then, uniform-size of 3 DAG (days after germination) seedlings were transplanted onto the growth medium and incubated vertically for 10 days. For the dose-response experiment, the medium was supplemented with 0.1, 0.5, 1, 2, 3, 3.67, 4, and 5 g/L SBE. For the time-course analysis of primary root development, the medium was supplemented with 1 g/L or 3 g/L SBE and incubated for 6, 12, and 24 h after etiolation. Seedlings were scored for the numbers of adventitious roots (ARs), junction roots (JRs), and lateral roots (LRs) under a binocular microscope (Olympus, SZX9, Tokyo, Japan). The primary root length (PRL) was measured by image analysis using ImageJ software version 1.53n (Schindelin et al., 2012) coupled with the “NeuronJ” plugin (Meijering et al., 2004). Each treatment consisted of three plates, and each plate contained 10 seedlings.
Shoot Growth
Arabidopsis shoot growth in response to SBE application was evaluated using a shoot assay adapted from De Diego et al. (2017). Briefly, seedlings were grown as described in the root assay and, at 3 DAG, transferred to 24-well tissue culture plates (VWR, CA, United States) containing Murashige and Skoog medium (4 mL per well) supplemented with 0.5 g/L SBE and 100 mM NaCl. Since at 100 mM NaCl, Arabidopsis rosettes are more compact (Claeys et al., 2014), we assessed the shoot growth at 13 DAG by measuring the green surface area using ImageJ. Each data point corresponds to 16 seedlings per treatment. The electrolyte leakage (also called conductivity) from seedlings was measured with a conductivity meter (inoLab Cond level 1) (Jiang et al., 2017).
Floating Leaf Disc
The senescence leaf disc assay was modified from Ghosh et al. (2015) and Chiu et al. (2021). Arabidopsis was cultivated in Jiffy-7® peat pellets (Jiffy Products International AS, Norway) in a growth room (room temperature 18°C, less than 70% relative humidity, light intensity 100 μmol m–2 s–1, 16/8 h day/night photoperiod). The chlorophyll content in fully expanded rosette leaves of the same developmental stage was determined by the SPAD-502 chlorophyll meter (Konica Minolta, Tokyo, Japan). Leaves with SPAD values from 25 to 35 were harvested from healthy plants at 30 DAG. Around 20 leaf discs were punched with a 7 mm cork borer and floated on a 5 mL solution in each petri dish (55 mm diameter). Each treatment consisted of six individual plates. Distilled water (dH2O) was considered the blank, while 200 mM NaCl was considered the salt treatment. For exogenous SBE treatment, leaf discs were pretreated with 5 mL of dH2O or 0.5 g/L SBE for 1 day before incubating in the blank or salt solution for another 2 days. The plates were sealed with 3M Micropore tape (3M, St. Paul, MN, United States) and placed in the growth room (room temperature 25°C, 40–60% humidity, light intensity 200 μmol m–2 s–1, 24 h light photoperiod). After incubation, the leaf disc samples were first wrapped in dust-free tissue paper, then homogenized into powder with liquid nitrogen, and stored at −80°C for further analysis. Photosynthetic pigments of chlorophyll a (Chl a), chlorophyll b (Chl b), and carotenoids (Car) were assessed from frozen samples using a microplate reader (Tecan Infinite M200), according to Chiu et al. (2021). Malondialdehyde (MDA), as a biomarker of lipid peroxidation, was analyzed via the thiobarbituric acid (TBA)-reactive substances assay following (Hodges et al., 1999).
True Leaf Development
The true leaf assay was adapted from Rosa and Scheid (2014). Arabidopsis seeds were sown directly on the treated medium and vernalized as mentioned before (Trinh et al., 2018). Since the addition of NaCl (100 mM) in the medium induced moderate salt stress (Claeys et al., 2014), medium supplemented with 0.5 g/L SBE was prepared with or without 100 mM NaCl for the exogenous SBE application. The plates were positioned horizontally for germination in the light without etiolation and early leaf development monitoring for 10 days. The germination rate and the early development phenotypes were recorded daily at the same time each day. At 2 DAG, seed germination was evaluated by checking if the radicle was visible after testa rupture. Later, at 10 DAG, the successful emergence and expansion of the first pair of true leaves were determined by a binocular microscope at 20× zoom. If the side-view width of the true leaf had expanded larger than the hypocotyl diameter, it was then scored as a plant with expanded true leaves (Supplementary Figure 1). The percentage (%) of seedlings with true leaves was calculated following Equation 1:
This assay was performed as nine replicates for each treatment, and each plate contained 25 seeds. The harvested samples at 10 DAG were pooled into four biological replications and stored at −80°C for further analysis. The fresh weight of whole seedlings was measured and subsequently dried at 65°C for 48 h in an oven for dry biomass determination. In addition, the conductivity and MDA content in seedlings were measured as mentioned above to evaluate plasma membrane damage.
Hydrogen peroxide (H2O2), as one of the main ROS products, was quantified based on potassium iodide (KI) oxidation (Junglee et al., 2014). To detect in situ H2O2, histological staining with 3,3′-diaminobenzidine (DAB) on whole seedlings was performed as previously described (Daudi and O’Brien, 2012). The seedlings were imaged via an Olympus BX51 microscope (Olympus, Tokyo, Japan) equipped with differential interference contrast (DIC) optics at 10× zoom. The relative DAB staining intensity was calibrated in pseudo color and quantified in four tissues, inclusive of cotyledons, hypocotyls, shoot apical meristems (SAMs), and root, using ImageJ coped with the “Colour Deconvolution 2” plugin (Ruifrok and Johnston, 2001; Landini et al., 2021).
The antioxidant enzyme activity was then determined following a semi high-throughput protocol (Fimognari et al., 2020) with the adapted extraction method (Noctor et al., 2016). About 300 mg grounded sample was extracted with 2 mL extraction buffer (0.1 M phosphate buffer plus 1 M EDTA; pH 7.5) and 50 mg polyvinylpyrrolidone (PVP). The mixture was centrifuged for 10 min at 4°C. Next, the total protein content was quantified in the desalted supernatant by Wizard® SV minicolumns using a spectrophotometer (DeNovix Inc., United States). Finally, the enzyme kinetic assays were performed for the activity measurement of ascorbate peroxidase (APX, EC:1.11.1.11), catalase (CAT, EC:1.11.1.6), glutathione reductase (GR, EC:1.8.1.7), glutathione S-transferase (GST, EC:2.5.1.18), monodehydroascorbate reductase (MR, EC:1.6.5.4), (cytoplasmic) peroxidase (POX, EC:1.11.1.5), and superoxide dismutase (SOD, EC:1.15.1.1). The antioxidative enzyme capacity, indicating the rate of catalyzed reaction by the enzyme, was calculated as a unit per mg of protein (Vanhoudt, 2014).
Statistical Analysis
The treemap was generated indicating the chemical classification of compounds identified in SBE by R software version 4.1.1 (R Core Team, 2021) coupled with “treemap” package version 2.4-3 (Tennekes and Ellis, 2021). Non-parametric Kruskal–Wallis test was used with post hoc Dunn’s analysis (α = 0.05) for variances in root numbers of different types. PRL was normalized and fitted in a 5-parameter logistic model of dose-response analysis using “nplr” package version 0.1-7 (Commo and Bot, 2016). The half-maximal-effect concentration (EC50) was calculated on the PRL inhibition effect by getEstimates function (Venturelli et al., 2016). Dynamic growth models of plant leaf area were fitted in exponential growth curves illustrating the early development patterns. One-way ANOVA analysis was applied with post hoc Tukey HSD test (α = 0.05) to compare the treatment difference for other plant traits. Besides, two-way ANOVA analysis was used for two independent variables: the addition of growth media and timepoints. The statistical analysis of the remaining parameters and data visualization were performed with GraphPad Prism software version 8.0.2 (GraphPad, San Diego, CA, United States).
Results
Preparation of Sunflower Bark Extract and Chemical Analysis
Aqueous extraction of sunflower bark was obtained using a twin-screw extruder as described in the materials and methods. Supplementary Table 2 shows the chemical composition of the starting bark materials, with the insoluble fraction constituting 88% of the bark containing 50% cellulose and 15% lignin. The high content in lignocellulose promoted the separation into fiber (pulp) and a liquid filtrate, which contained the water-soluble compounds. The chemical composition and antioxidant activity of the freeze-dried liquid filtrate (SBE) contained 1.5% (w/w) soluble protein and 0.7% (w/w) carbohydrate (Table 1). The polyphenol content (TPC and TFC) per gram dry biomass of SBE was 6.46 mg AsA equivalents and 29.68 mg CHA equivalents with phenolic acids, and 9.70 μg QE equivalents and 15.93 μg rutin equivalents with flavonoids. TAC of SBE was represented as IC50 from in vitro antioxidant assays, which were 20.66 and 117.34 TE equivalents in the DPPH and ABTS assay, respectively.
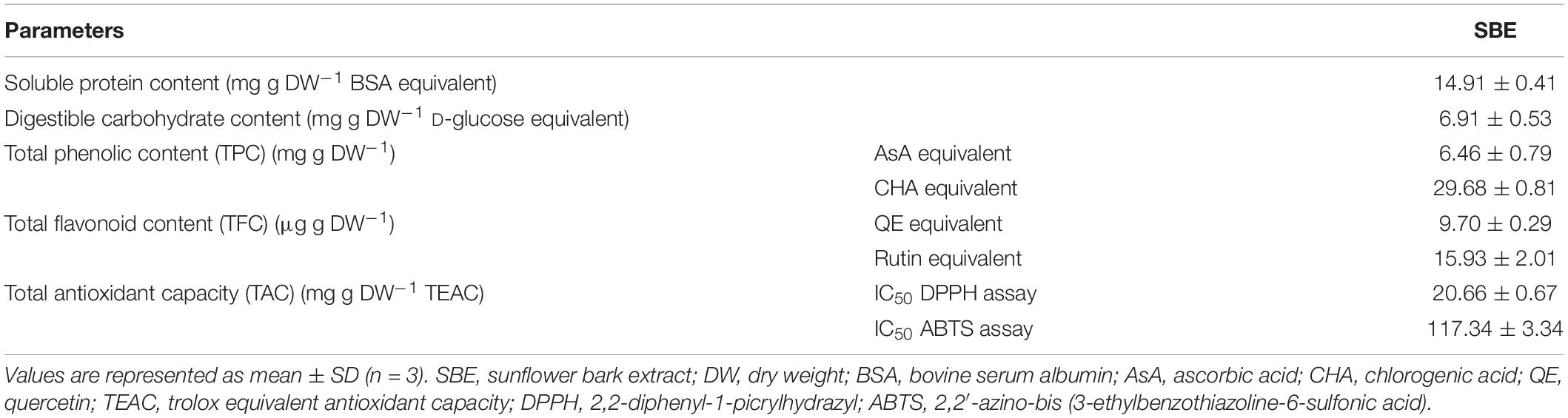
Table 1. Contents of soluble protein, digestible carbohydrate, polyphenols, and in vitro antioxidant capacity in SBE.
The low molecular weight primary and secondary metabolites were characterized by untargeted metabolic profiling using UHPLC-PDA-HRMS. It resulted in 2369 LC–MS features in the ESI+ mode and 814 under the ESI− mode (the chromatograms are shown in Supplementary Figure 2, all the identified compounds are listed in Supplementary Data 1). A total of 26.03% of the ESI+ and 57.97% of the ESI− detected peaks were tentatively identified. These compounds were classified according to 35 distinct categories of plant metabolites (Figure 1). The lipids and lipid-like molecules, organic acids, phenylpropanoids and polyketides, benzenoids, and organoheterocyclic compounds formed the five most extensive groups representing half of the classified metabolites. SBE was particularly rich in compounds across chemical superclasses of phenylpropanoids and polyketides, organic acids and derivatives, and benzenoids, of which polyphenols are well-known antioxidants with cytoprotective activity (Kiokias et al., 2020; Šamec et al., 2021). Thereby, we further focused on the diverse proportions of non-flavonoid and flavonoid compounds of interest under the phenylpropanoids and polyketides superclass in SBE. Eleven polyphenol classes involved 20 subclasses, and 45 tentatively identified compounds were illustrated in Table 2.
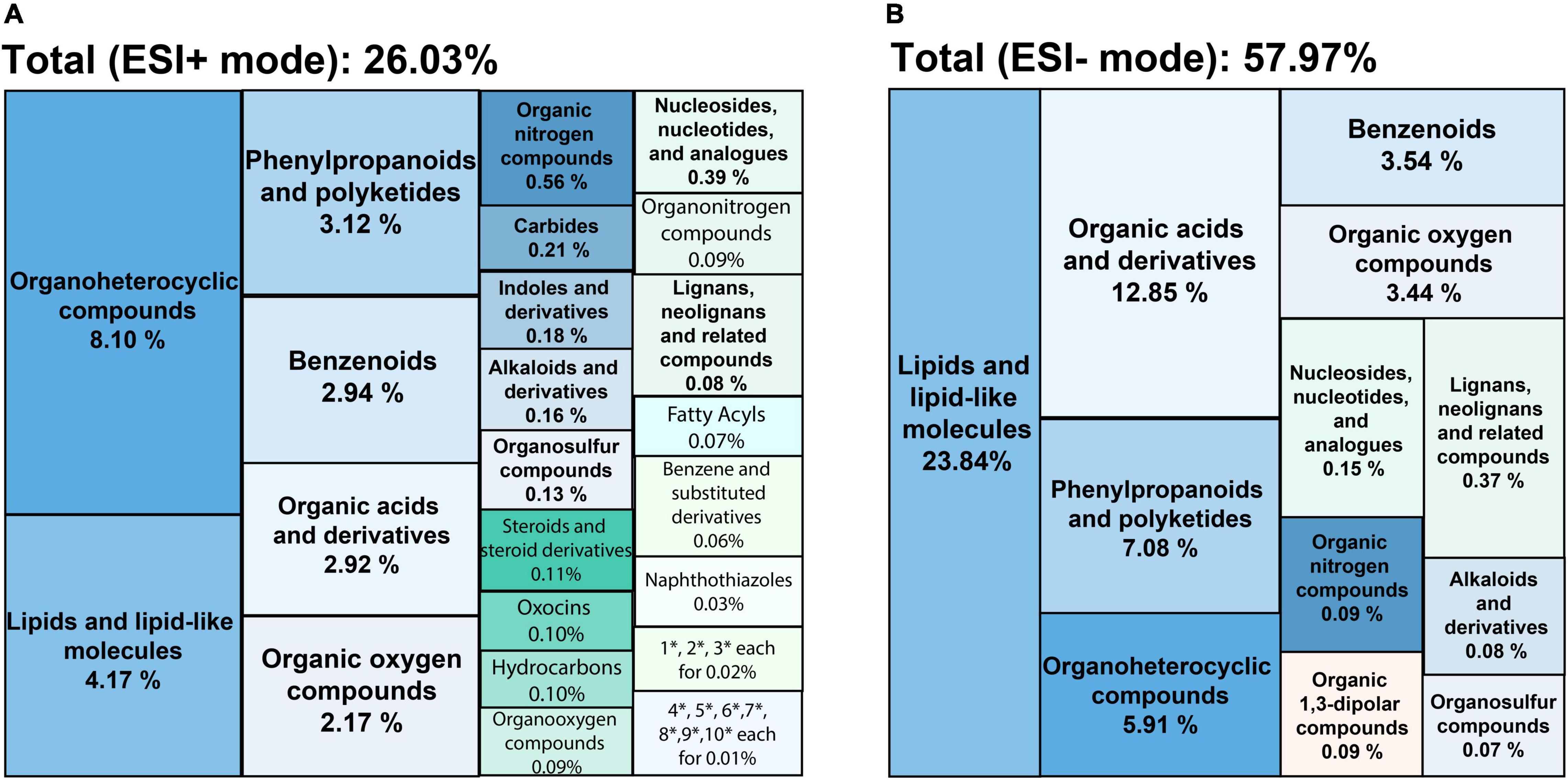
Figure 1. Treemaps of chemical classifications of SBE compounds detected by UHPLC-PDA-HRMS in (A) ESI+ and (B) ESI– modes. Each color shows the chemical taxonomy at the superclass level from ChemOnt ontology. Each value indicates the relative distribution of each superclass by total peak areas. SBE, sunflower bark extract; ESI+ (–), positive (negative) electrospray ionization. Where * is short for the superclasses following, 1: cinnamic acids and derivatives; 2: quinolines and derivatives; 3: imidazopyrimidines; 4: organic phosphoric acids and derivatives; 5: pyrroloazepines; 6: coumarins and derivatives; 7: pumiliotoxins, homopumiliotoxins, and allopumiliotoxins; 8: benzothiazoles; 9: azoles; 10: lupin alkaloids.
Sunflower Bark Extract Inhibited the Primary Root Growth of Non-stressed Arabidopsis Seedlings in a Dose-Response Manner
The root tip is a sensory organ that evaluates the presence of mineral nutrients and physical obstructions, adapting its growth in response to the conditions by altering the growth rate and orientation of cell division and expansion (Svolacchia et al., 2020). Hence, the primary root is very susceptible to environmental conditions, and its plasticity facilitates the detection of slight changes in the composition of the growing medium (Malamy, 2005). To test the impact of SBE application on root growth, root length (PRL) and branching (AR, LR, and JR) of in vitro grown Arabidopsis seedlings were determined (Supplementary Figure 3). Significantly less AR was formed when treated with SBE at higher doses. A strong reduction in LR number was observed at SBE levels from 1 g/L, while the effect on JR formation was more complex with a promotion up to 1 g/L SBE but a reduction from a higher concentration above 1 g/L. Furthermore, the EC50 of SBE inhibition of PRL was 0.63 g/L (Figure 2). Since root growth often shows an adaptive behavior to exogenous stimuli, the inhibition of PRL was examined at different time intervals after transfer to the medium containing SBE. Growth inhibition was observed 24 h after treatment with 1 g/L SBE, while at 3 g/L SBE inhibition occurred 6 h after treatment (Supplementary Figure 4). To avoid secondary effects following primary root growth inhibition, SBE was applied at 0.5 g/L in the subsequent assays (Supplementary Figure 3).
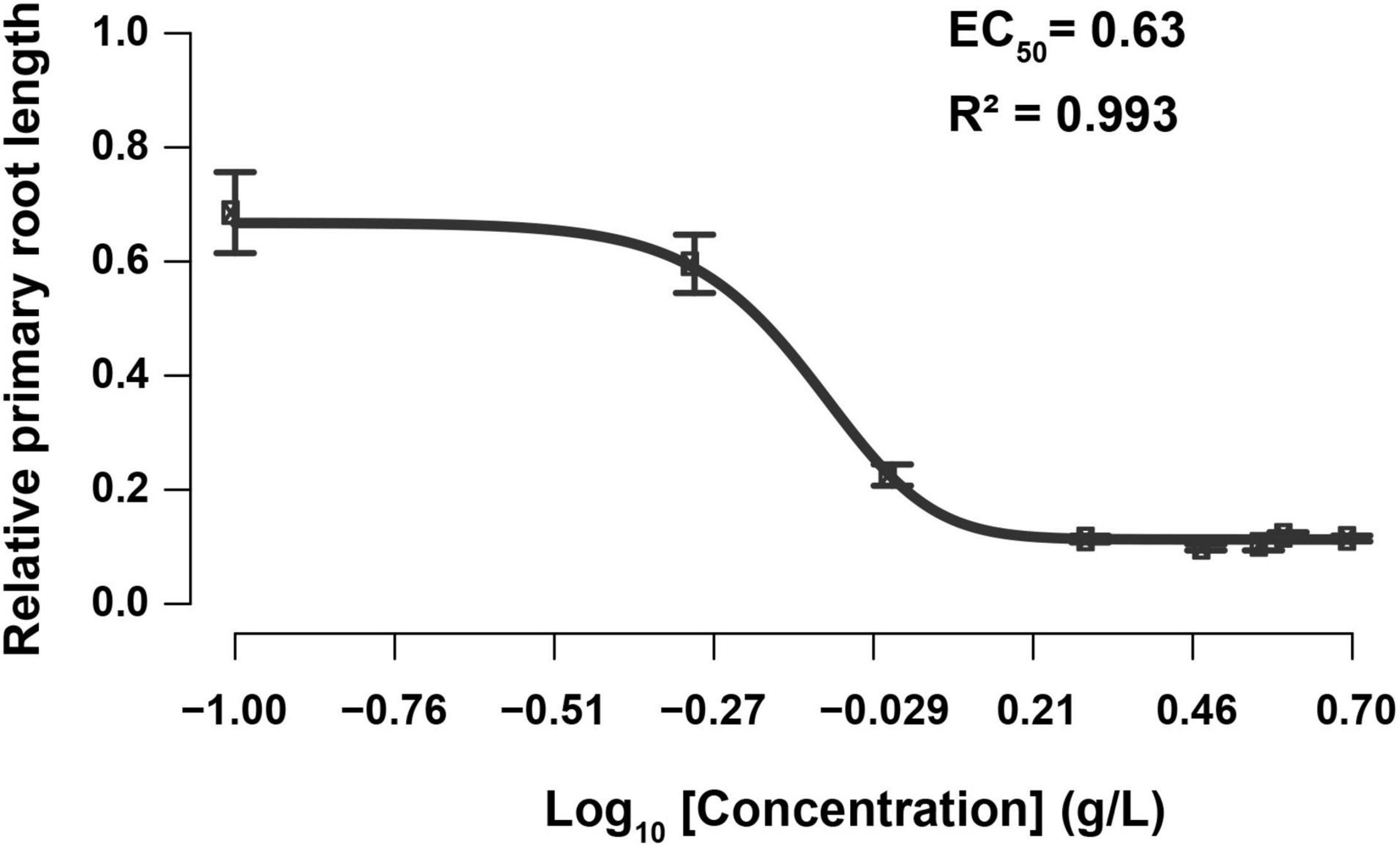
Figure 2. Dose-response curve of relative primary root length to the increase of SBE concentration in the Arabidopsis root assay. Each data point indicates the mean ± SD (n > 15). The black line represents the 5-parameter logistic regression. SBE, sunflower bark extract; EC50, half-maximal-effect concentration.
Priming With Sunflower Bark Extract Did Not Alter Germination Rate but Stimulated Shoot Growth
We did not observe a notable change in shoot growth after transferring 3 DAG Arabidopsis seedlings to SBE containing medium (Figure 3B). In addition to the seed priming experiment, there was no impairment of seed germination rate after 2 days in any of the treatments (Supplementary Figure 5). After 4 days, however, we observed a slight, statistically significant increase in the leaf area of seedlings grown on SBE containing medium (Figure 4A).
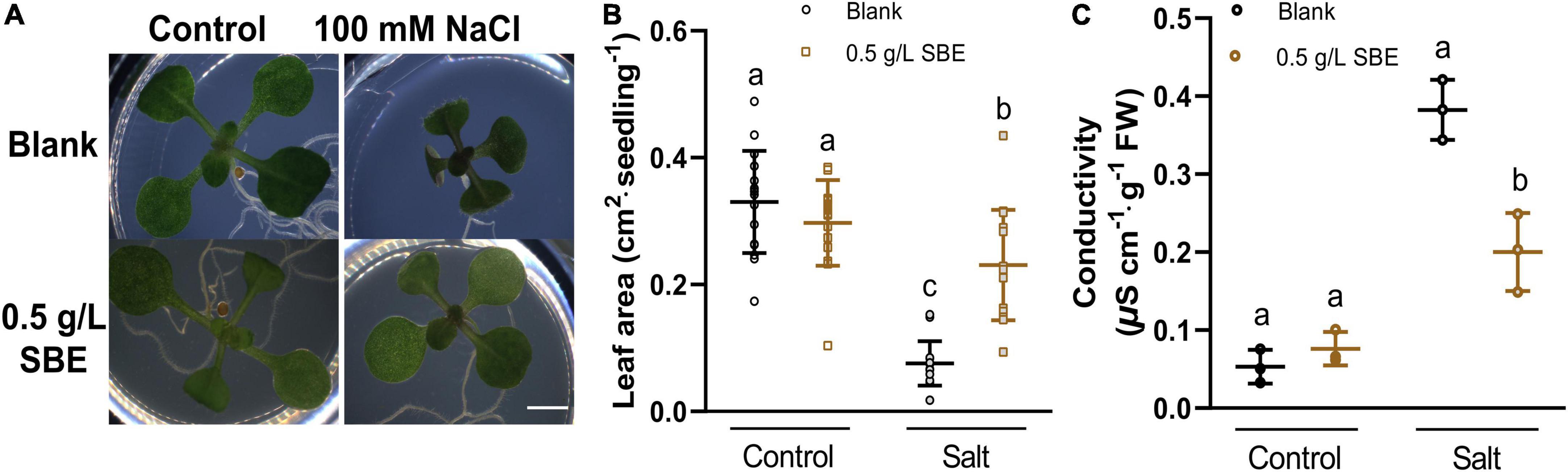
Figure 3. Salt stress alleviation of SBE treatment in the Arabidopsis shoot assay. (A) Phenotypes of representative seedlings at 13 DAG grown on multiwell plates. Bar = 2 mm. The changes of (B) leaf area (n = 16) and (C) conductivity (n = 4) of 13 DAG seedlings. Error bars indicate SDs of the means. Different letters represent significant differences between treatments using Tukey’s HSD test (p < 0.05). SBE, sunflower bark extract; DAG, days after germination.
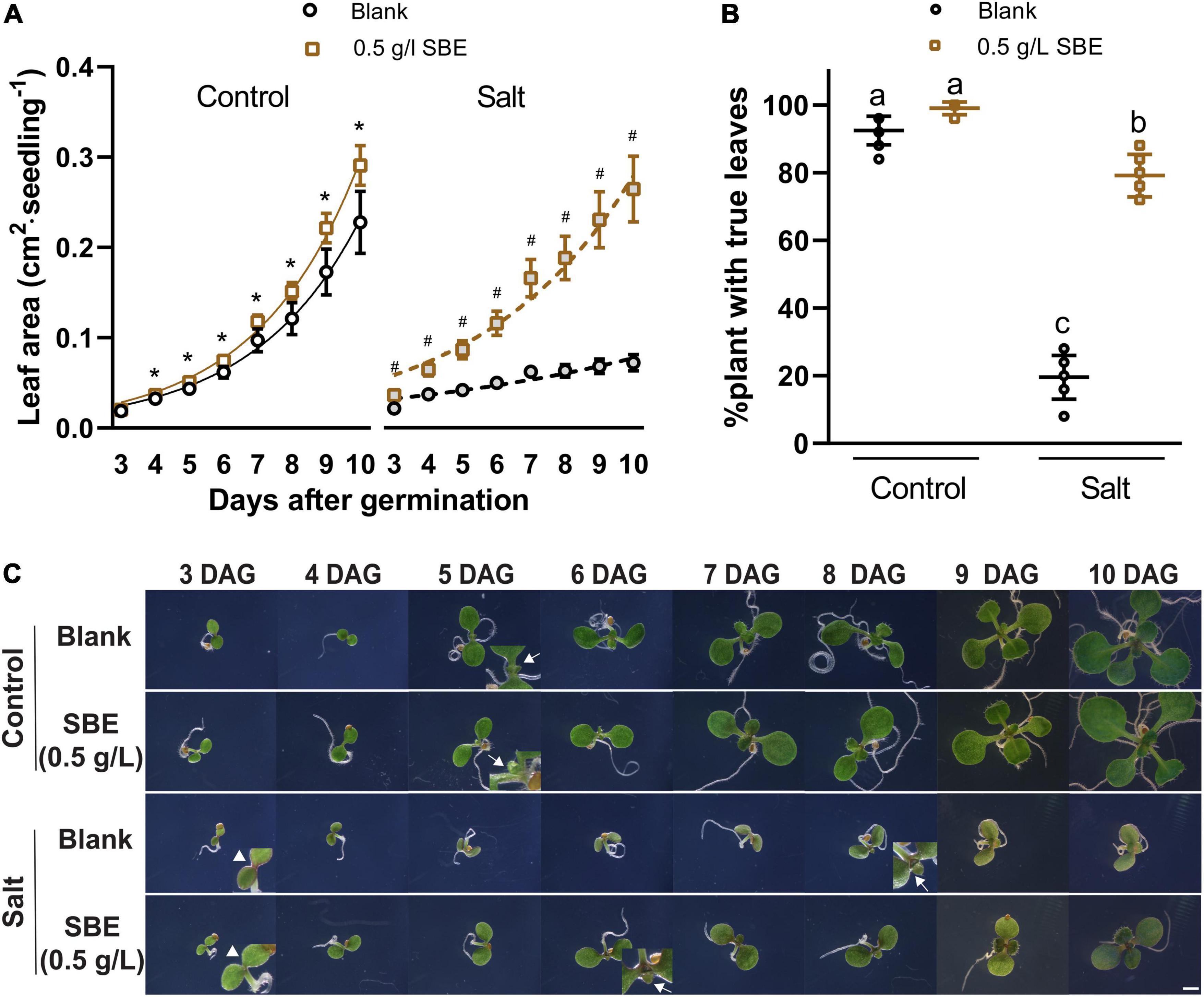
Figure 4. Salt stress alleviation of SBE treatment in the Arabidopsis true leaf assay. (A) The dynamic growth of leaf area from 3 DAG to 10 DAG. Error bars indicate SDs of the means (n = 9). Different letters represent significant differences between treatments using Tukey’s HSD test (p < 0.05). Fitted lines were exponential curves of dynamic leaf area growth. The symbols * and # represent significant differences between treatment with or without SBE addition under control or salt stress conditions at the same time point, respectively (p < 0.05). Both salt treatments were significantly different from the two non-stress treatments at every time point (label not shown). (B) The percentages of plants successfully developed with true leaves at 10 DAG. (C) Phenotype changes of representative seedlings from 3 DAG to 10 DAG focusing on the center of two cotyledons under binocular 6.3× zoom. Bar = 2 mm. Arrows without tails indicate salt stress alleviation by SBE addition on anthocyanin accumulation at the cotyledon edges of 3 DAG seedlings. Arrows with tails showed the earliest starting time point of visible true leaves. SBE, sunflower bark extract; DAG, days after germination.
Sunflower Bark Extract Alleviated Shoot Growth Inhibition Under Salt Stress
Biostimulants typically show a more pronounced effect in plants grown under stress (Rouphael and Colla, 2020). The impact of SBE was therefore assessed under conditions of salt stress. As the suppression of shoot growth by NaCl was very notable (Claeys et al., 2014), the projected leaf area was used as a proxy for determining the effect of SBE. In the shoot assay, the shoot growth was severely inhibited in the presence of 100 mM NaCl, showing a reduced petiole length, smaller cotyledons, and delayed or even arrested emergence of the first true leaves (Figure 3A). In the presence of SBE, the NaCl-stressed seedlings generated a significantly larger green surface area than in control salt-stressed plants (Figure 3B). NaCl causes osmotic stress and ionic imbalances, affecting the integrity of the plasma membrane (Dubois and Inzé, 2020). Therefore, the seedlings were collected after 13 DAG to determine the electrolyte leakage (Figure 3C). Salt stressed plants grown on SBE containing medium showed much lower conductivity than the control plants, suggesting that SBE treatment protected the plants from cell membrane damage.
We then put more attention to early true leaf development in seed priming treatment. SBE did not influence the germination rate of Arabidopsis under salt stress conditions (Supplementary Figure 5). Since we noticed that shoot growth was enhanced in SBE containing medium, a time-course analysis was performed to determine this response in more detail (Figure 4). On 100 mM NaCl-containing medium, shoot development was significantly reduced, while this growth inhibition was strongly alleviated when SBE was included in the medium (Figure 4A). Under normal conditions, all plants expanded their first true leaves at 10 DAG, while in the presence of NaCl, only 20% of the plants produced expanded true leaves. However, the number of salt-stressed plants with expanded true leaves increased to around 80% when treated with SBE (Figure 4B). The addition of SBE advanced true leaf development by about 2 days, and the effects were already noticeable from 3 DAG when NaCl-induced anthocyanin accumulation as a red discoloring of the cotyledons and at the upper hypocotyl margin was observed. While less intense red coloring was shown in the presence of SBE (Figure 4C).
Sunflower Bark Extract Preserved Photosynthesis Pigments and Stabilized the Cell Membrane Under Salt Stress
The Arabidopsis response to salt stress includes a reduction in growth, reflected in lower fresh weight and dry weight (Figures 5A,B), and a bleaching effect that entails a decline of pigments, the photosynthetic chlorophyll, and carotenoids (Leschevin et al., 2021). These pigments were quantified, and chla+b was reduced twofold, whereas carotenoids were down by about threefold in 10 DAG salt-stressed seedlings (Figures 5C,D). This protective effect of SBE was accompanied by diminished salt stress-induced electrolyte leakage (Figure 5E) and MDA overaccumulation (Figure 5F).
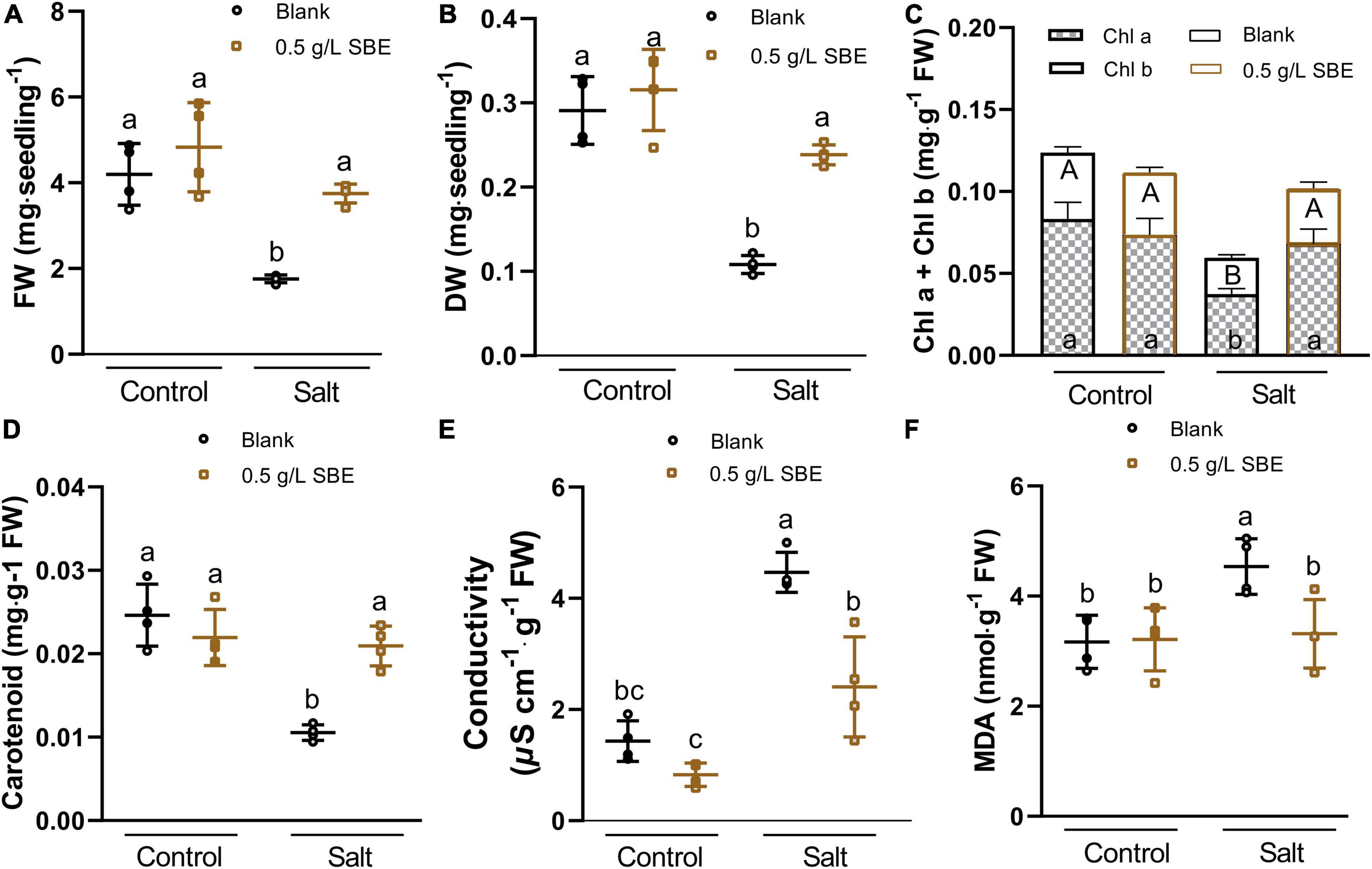
Figure 5. Salt stress alleviation of SBE treatment on the physiological traits of 10 DAG Arabidopsis in the true leaf assay. (A) Fresh weight, and (B) dry weight of the whole seedlings at 10 DAG. The changes of photosynthetic pigments containing (C) chlorophyll a and b, (D) carotenoid content, (E) conductivity, and (F) MDA content. Error bars indicate SDs of the means (n = 4). Different letters represent significant differences between treatments using Tukey’s HSD test (p < 0.05). SBE, sunflower bark extract; DAG, days after germination; FW, fresh weight; DW, dry weight; Chl a, chlorophyll a; Chl b, chlorophyll b; MDA, malondialdehyde.
To investigate whether SBE exerts a priming protective effect on mature leaves, punched leaf discs from fully developed leaves were pretreated for 1 day with SBE or water as a control (Figure 6A). Next, the leaves were floated for 2 days on a solution with or without 200 mM NaCl. The SBE pretreated leaves maintained a higher level of chla+b and carotenoid content under salt stress conditions (Figures 6B,C). Similar to the salt-stressed seedlings, SBE pretreatment dampened the accumulation of MDA production induced by salt (Figure 6D).
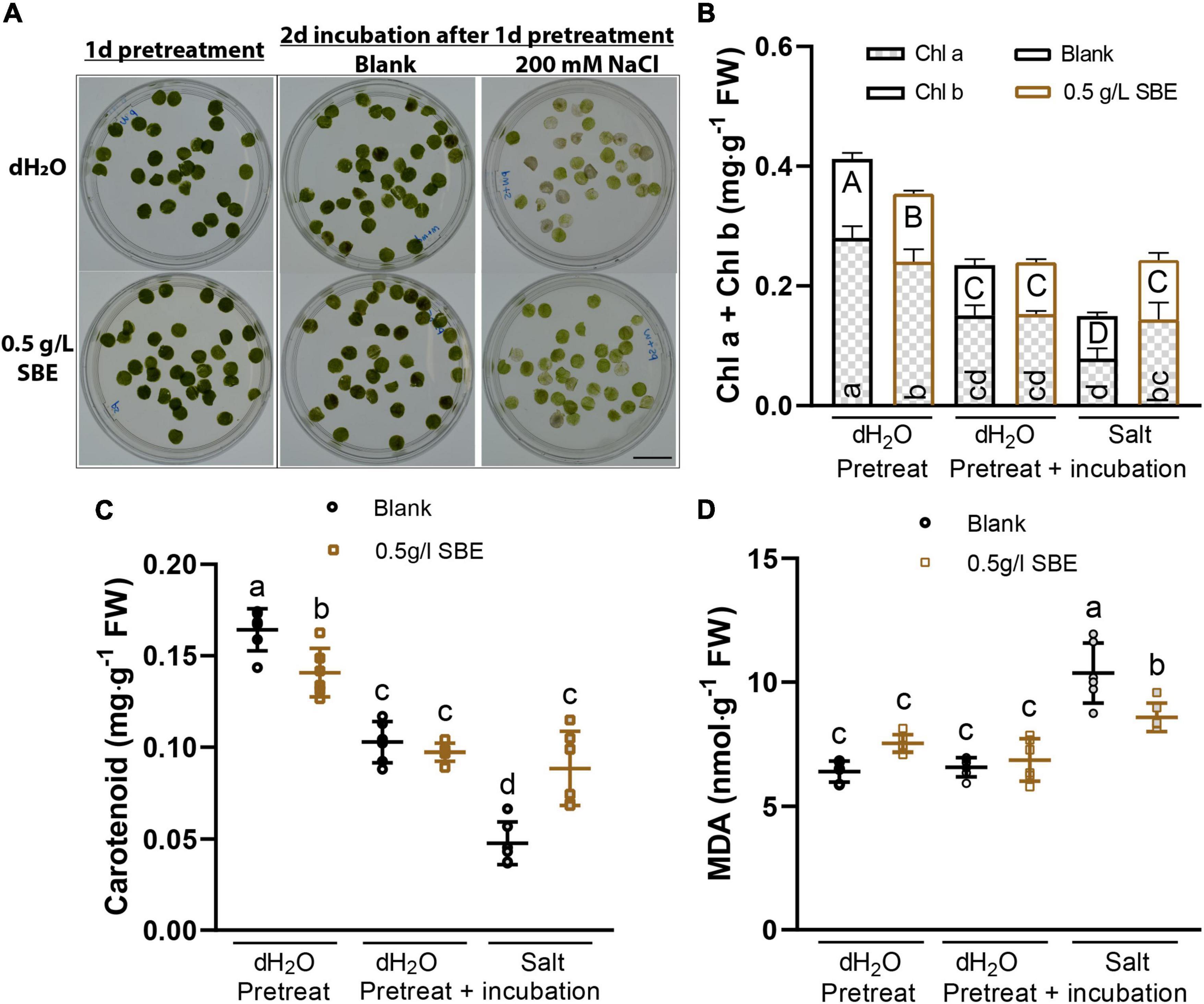
Figure 6. Salt stress alleviation of SBE pretreatment on expanded Arabidopsis leaves in the leaf disc assay. (A) The representative image of treated leaf discs was taken before sampling. Bar = 2 cm. Photosynthetic pigments contain (B) chlorophyll a and b, and (C) carotenoid content. (D) MDA content. Error bars indicate SDs of the means (n = 6). Different letters represent significant differences between treatments using Tukey’s HSD test (p < 0.05). SBE, sunflower bark extract; DAG, days after germination; FW, fresh weight; Chl a, chlorophyll a; Chl b, chlorophyll b; MDA, malondialdehyde.
Sunflower Bark Extract Mitigates NaCl Toxicity by Suppressing Hydrogen Peroxide Overaccumulation
Salinity-induced osmotic and ionic stress affects cellular redox homeostasis by H2O2 overproduction causing oxidative damage to proteins and lipids (Munns and Tester, 2008; Huang et al., 2019). We, therefore, asked if SBE scavenges ROS in salt-stressed plants. The H2O2 levels more than doubled in salt stress seedlings at 10 DAG (Figure 7A). The salt-induced increase in H2O2 was reduced to about 60% by SBE application (Figure 7A). ROS reduction was already apparent after 3 DAG upon ROS staining with DAB (Supplementary Figure 6 and Figure 7B), which coincides with the earliest time point when SBE started showing a significant improvement in shoot growth on NaCl containing medium (Figure 4C). The relative DAB staining intensity in plants grown in the presence of SBE was lower than in plants without SBE (Figure 7B). The quantification of DAB intensity in the cotyledons, hypocotyl, root, and SAM showed that the dampening effect of SBE occurred in all seedling organs (Figure 7C). H2O2 levels were relatively higher in the root and SAM than in cotyledons and hypocotyl. The SBE mediated reduction of ROS was most pronounced in the SAM in line with the protective effect of SBE on true leaf development under salt stress conditions.
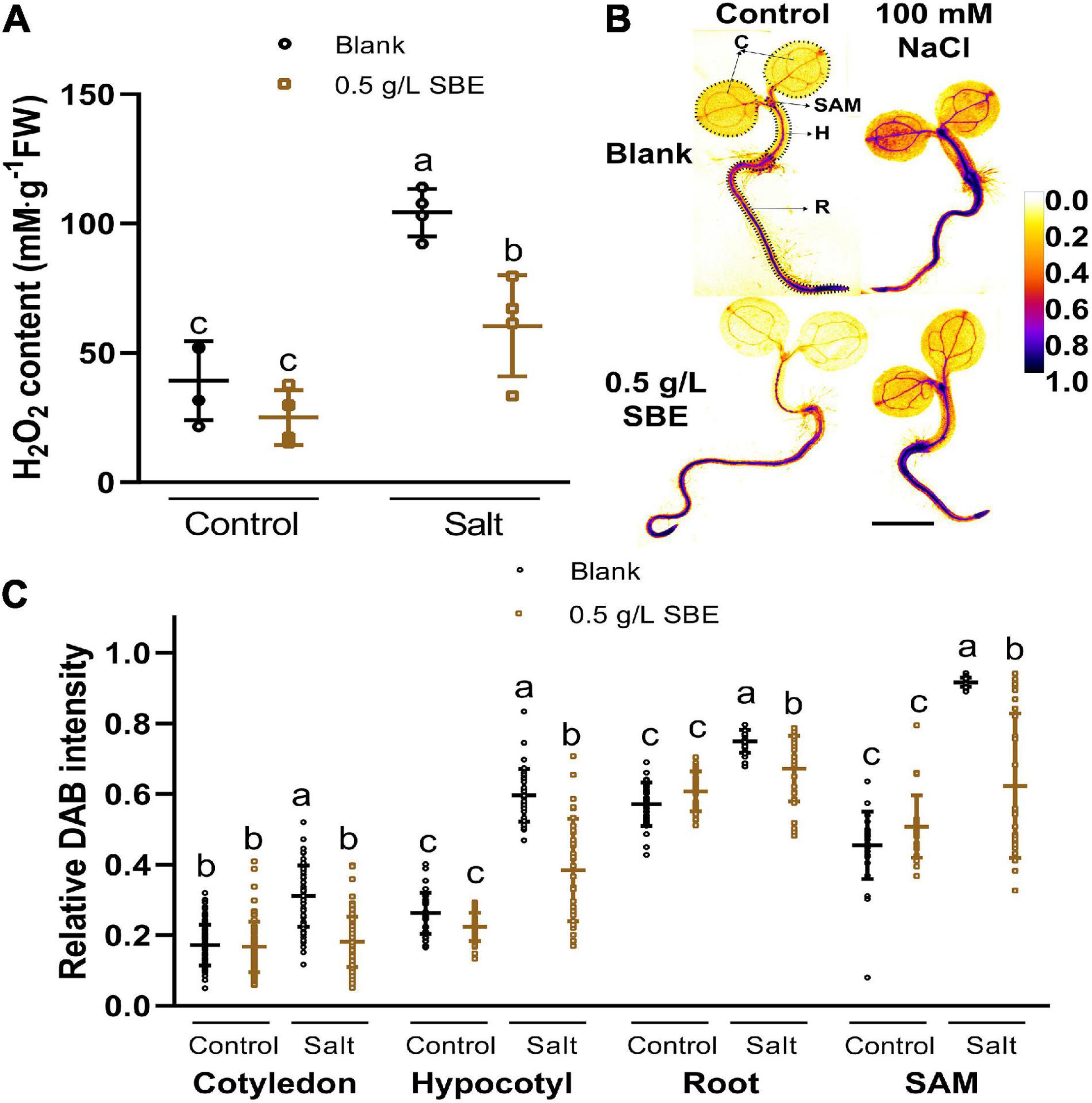
Figure 7. Hydrogen peroxide accumulation of 3 DAG and 10 DAG Arabidopsis seedlings under salt stress in the true leaf assay. (A) H2O2 content in 10 DAG seedlings. (B) Representative images of 3 DAG seedlings after histological staining of H2O2 by DAB are shown in pseudo-colors of relative DAB intensity as shown in the color bar. Regions of interested organs are shown in circles with black dotted lines. Bar = 1 mm. (C) Relative DAB staining intensity of four organs in 3 DAG seedlings. Error bars indicate SDs of the means [n = 4 (A) and >25 (B)]. Different letters represent significant differences between treatments (p < 0.05). SBE, sunflower bark extract; DAG, days after germination; H2O2, hydrogen peroxide; DAB, 3′,3-diaminobenzidine; C, cotyledon; H, hypocotyl; R, root; SAM, shoot apical meristem.
Next, we asked whether SBE neutralizes ROS formation via the overactivation of antioxidant enzymes that are part of the plant defense system (Apel and Hirt, 2004; Bobrovskikh et al., 2020). To this end, the activity of the antioxidant enzymes was measured in control and salt-stressed seedlings grown with or without SBE supplement (Figure 8). In control conditions, no difference in antioxidant enzymes activity was investigated. SBE treatment of salt-stressed plants significantly increased the activity of CAT, APX, and POX (Figures 8B–D), which directly participate in eliminating ROS pathway by catalyzing the conversion from H2O2 to H2O (Dumanović et al., 2021). In contrast, SOD, GR, GST, and MR were not significantly altered by SBE treatment (Figure 8A and Supplementary Figure 7).
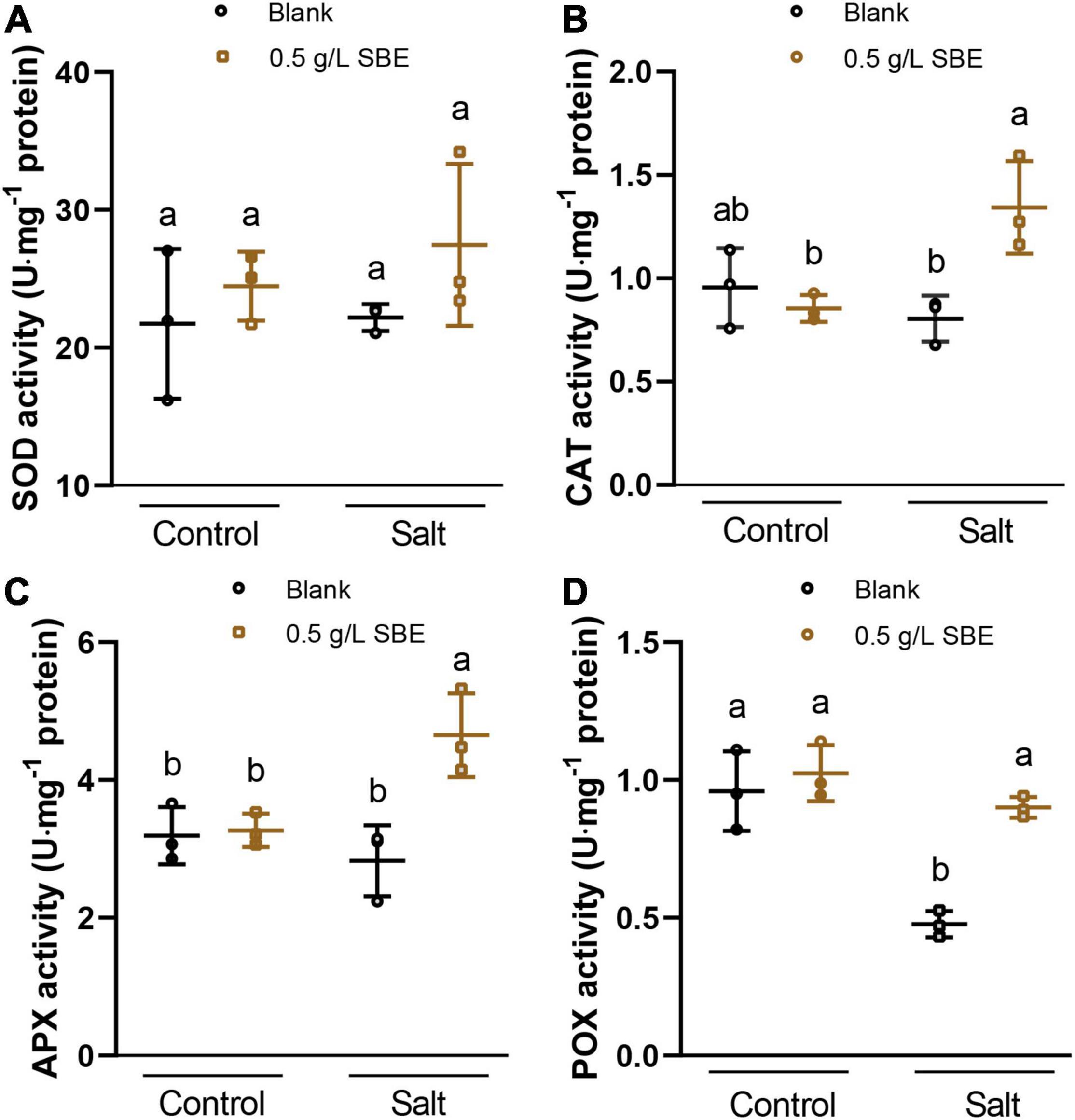
Figure 8. The antioxidant activities of Arabidopsis seedlings under salt stress at 10 DAG in the true leaf assay. (A) SOD activity, (B) CAT activity, (C) APX activity, (D) POX activity. Error bars indicate SDs of the means. Different letters represent significant differences between treatments using Tukey’s HSD test (p < 0.05). SBE, sunflower bark extract; DAG, days after germination; U, enzyme activity unit (μmol/min); SOD, superoxide dismutase; CAT, catalase; APX, ascorbate peroxidase; POX, peroxidases.
Discussion
This study on the analysis of potential biostimulant activity is a major step in valorizing sunflower stalks. Here, we report that SBE, a side stream obtained during fiber isolation by twin-screw extrusion, contains polyphenols and other bioactive molecules that activate ROS scavenging enzymes, thereby suppressing the oxidative damage to Arabidopsis seedlings grown on the NaCl-containing medium. Together, the results suggest that SBE is a potential source of biostimulant.
The twin-screw extrusion method is an extraction technique for separating insoluble parts (e.g., fibers) from solvent-soluble molecules in a single step. The solvent, thermal and mechanical actions are customized to extract fiber-rich plant biomass from crop waste such as sunflower stems (Evon et al., 2018; Vandenbossche et al., 2019). The extrusion method is an ecofriendly biorefinery process that meets the criteria of “green chemistry” extraction of natural products (Chemat et al., 2012). Yet, the bark extract is not economically valorized from the sunflower fiber part. SBE is an aqueous extract adhering to the guidelines of the European directive for certification of natural substances without pesticide residue and, as such, can be used to produce a novel biostimulant.
The outer bark constitutes about 90% dry weight of the whole stalk, indicating that it is much denser than the pith (Xu et al., 2020). The stems contain a considerable amount of polyphenols (Kamal, 2013). The chemical composition of an aqueous extract from sunflower bark has not yet been reported. An estimation of phenolics content (TPC) in SBE (Table 1) was obtained by applying a modified Folin–Ciocalteu assay to quantify TPC values against CHA (Sánchez-Rangel et al., 2013). However, the TFC values for evaluating “total” flavonoid content are not adequate based on aluminum complex reaction as the two procedures we performed are specific for different flavonoid structures (Pękal and Pyrzynska, 2014). In this situation, it is not possible to compare the accurate polyphenol content across the studies using different protocols. Therefore, to improve the coverage of present plant metabolites in SBE besides polyphenols, UHPLC-PDA-HRMS was performed for more precise detection and identification (Lai et al., 2018). Thanks to the validated algorithm increasing the accuracy of compound identification (Tsugawa et al., 2016), MS-DIAL combined with MS-FINDER is recommended to match MSe spectra in silico for untargeted metabolomics (Blaženović et al., 2017; Vaniya et al., 2017). Our study thus provides the chemical composition of SBE, to some degree, revealing the plant metabolomics with both colorimetric and UHPLC-PDA-HRMS analysis.
Sunflower bark extract shows conspicuously in vitro activity, completely inhibiting primary root growth at doses above 1 g/L (Supplementary Figure 3). This strong growth inhibition contrasts with the growth-promoting effects observed in salt-stressed plants treated with more diluted SBE. Sunflowers produce a diverse set of allelochemicals that either positively or negatively affect the growth of other plant species (Macías et al., 2002). Some of these allelochemicals were already identified in sunflower stalks (Maheswari et al., 2019). Allelopathic activity in sunflower was closely linked with the presence of polyphenols and terpenes (Rawat et al., 2017). Sunflower aqueous shoot extract partially inhibited rapeseed and Cephalaria seedling growth (Hamad, 2017). Natural polyphenols were extensively reported to induce cytotoxicity in plant normal cells as well as cancer cell in dose-dependent manners (Rasouli et al., 2016; Perveen, 2017). These or other allelopathic compounds are likely also present in SBE and could be responsible for the primary root growth inhibition at high doses and may also prime to trigger a plant defense response, a property of many biostimulants (Kerchev et al., 2020).
However, at 0.5 g/L, SBE protected Arabidopsis from oxidative damage induced by a moderate concentration of NaCl in the medium (Figure 4). Also, allelopathic extracts from Levisticum officinale Koch were recently identified to have a positive performance on soybean yield (Szparaga et al., 2021). One of the reasons is that some polyphenols have antioxidant activity and play a role in controlling oxidative stress in plants (reviewed in Ferdinando et al., 2012; Šamec et al., 2021). For example, quercetin suppressed the ROS toxicity of paraquat in seedlings of Arabidopsis, tobacco, and duckweed (Kurepa et al., 2016) and heavy-metal stress in Arabidopsis (Zhang et al., 2017). Recent evidence shows that flavonoids are active as cytoprotective antioxidants preventing mitochondrial signaling that regulates autophagy and apoptosis (Kicinska and Jarmuszkiewicz, 2020). Phenolic acids are also stress-relieving molecules due to their high antioxidative properties (Šamec et al., 2021), namely, caffeic and sinapic acids enhance salt tolerance in wheat seedlings when exogenously applied (Kaur et al., 2017). The reduction in ROS levels mediated by SBE is likely due to the antioxidant activity of polyphenols and possibly other molecules within a non-toxic concentration range.
Next to polyphenols, SBE contained digestible carbohydrates and soluble protein. Sugars released from carbohydrates function as energy metabolites, osmoprotectants, and signaling molecules and mitigate stress responses in plants (Rook et al., 2006; Krasensky and Jonak, 2012). Likewise, several amino acids derived from proteins have been proved to be precursors of secondary metabolites and signaling molecules tightly related to plant responses under stress (Batista-Silva et al., 2019). These water-soluble primary compounds may support plant stress adaptation and complement the ROS suppressing activity of the above-mentioned bioactive agents.
The true leaf development assay in Arabidopsis is a sensitive method for evaluating DNA damaging agents (Rosa et al., 2013). High concentrations of NaCl induce ROS formation in germinating eggplant leading to DNA damage (Kiran et al., 2020). In particular stem cells in germinating seeds and in shoot meristems are highly susceptible to DNA damage causing an arrest in leaf development (Fulcher and Sablowski, 2009). We speculate that the accumulation of H2O2 in SAM causes oxidative damage, including DNA damage and that this prevents the development of the first true leaves in our experiments (Figure 7C). Polyphenols suppress ROS overaccumulation by neutralizing free radicals with donated electrons or hydrogen atoms with concomitant formation of stabilized phenolic radicals (Dumanović et al., 2021). In addition, polyphenols activate ROS scavenging enzymes (Kerchev et al., 2020). These enzymes function in plant defense and regulate cell growth and cell death (Mhamdi and Van Breusegem, 2018). Therefore, SBE will likely affect shoot growth under salt stress by activating ROS scavenging enzymes.
In future experiments, fractionation of SBE into less complex mixtures will be necessary to define the extent of synergism between the different bioactive molecules. Plant extract-based biostimulants are typically mixtures of bioactive compounds (García-García et al., 2020), which may explain why certain extracts are active despite the applied low dilutions. Given that the large biomass of sunflower stalks is currently underused, we anticipate that it is a suitable resource for biostimulant development and will contribute to valorization of the stems. Further studies will also focus on the consistency and reproducibility of bioactivity across separate harvested materials.
Conclusion
Taken together, we demonstrated that SBE can be refined from sunflower bark using water as an extraction solvent in a twin-screw extruder and that it contains bioactive molecules that act as protectants against salt stress by maintaining cellular redox homeostasis. The results highlight the potential of SBE as a source for biostimulant production that can be used for seed biopriming, soil, and foliar application. Future studies are underway to test the effectiveness of SBE biostimulant under field conditions on various crops. The characterization of the bioactive ingredients is a critical target to unravel the chemical structure and underlying mode of action.
Data Availability Statement
The raw data supporting the conclusions of this article will be made available by the authors, without undue reservation.
Author Contributions
JL, AR, and DG: conceptualization. JL: methodology and investigation and writing – original draft preparation. HKT: initial screening. SB and PE: resources. PE and JL: chemical characterization. CVP, PM, SM, and JL: chemical profiling. PE, SB, CVP, SM, TVG, and DG: writing – review and editing. DG: supervision. TVG, AR, LX, and DG: project administration. BVD, SB, PE, SM, and DG: funding acquisition. All authors contributed to the article and approved the submitted version.
Funding
This research was supported by Fonds Wetenschappelijk Onderzoek – Vlaanderen (FWO) under project Bio2Bio (no. S006017N), under project BioSUNmulant by European Union’s Horizon 2020 Research and Innovation Program under grant agreement of sustainable and resilient agriculture for food and non-food systems (FACCE SURPLUS, no. 652615) and with Flanders Innovation and Entrepreneurship (VLAIO) (no. HBC.2019.2244). JL was supported by the China Scholarship Council (CSC) grant no. 201706350259.
Conflict of Interest
The authors declare that the research was conducted in the absence of any commercial or financial relationships that could be construed as a potential conflict of interest.
Publisher’s Note
All claims expressed in this article are solely those of the authors and do not necessarily represent those of their affiliated organizations, or those of the publisher, the editors and the reviewers. Any product that may be evaluated in this article, or claim that may be made by its manufacturer, is not guaranteed or endorsed by the publisher.
Acknowledgments
We thank Dr. Maaike Perneel (CropFit, Ghent University), Ms. Patricia Delaere, Mr. Christophe Petit, and Ms. Ellen van Gysegem (HortiCell Lab, Ghent University) for their technical and administrative support and Ms. Halimat Ogunsanya and Ms. Brechtje de Haas (HortiCell Lab, Ghent University) for the critical comments and suggestions.
Supplementary Material
The Supplementary Material for this article can be found online at: https://www.frontiersin.org/articles/10.3389/fpls.2022.837441/full#supplementary-material
References
Apel, K., and Hirt, H. (2004). Reactive oxygen species: metabolism, oxidative stress, and signal transduction. Annu. Rev. Plant Biol. 55, 373–399. doi: 10.1146/ANNUREV.ARPLANT.55.031903.141701
Batista-Silva, W., Heinemann, B., Rugen, N., Nunes-Nesi, A., Araújo, W. L., Braun, H. P., et al. (2019). The role of amino acid metabolism during abiotic stress release. Plant Cell Environ. 42, 1630–1644. doi: 10.1111/PCE.13518
Blaženović, I., Kind, T., Torbašinović, H., Obrenović, S., Mehta, S. S., Tsugawa, H., et al. (2017). Comprehensive comparison of in silico MS/MS fragmentation tools of the CASMI contest: database boosting is needed to achieve 93% accuracy. J. Cheminform. 9:32. doi: 10.1186/s13321-017-0219-x
Bobrovskikh, A., Zubairova, U., Kolodkin, A., and Doroshkov, A. (2020). Subcellular compartmentalization of the plant antioxidant system: an integrated overview. PeerJ. 8:e9451. doi: 10.7717/PEERJ.9451
Brentan Silva, D., Aschenbrenner, A.-K., Lopes, N. P., and Spring, O. (2017). Direct Analyses of Secondary Metabolites by Mass Spectrometry Imaging (MSI) from Sunflower (Helianthus annuus L.) Trichomes. Molecules 22:774. doi: 10.3390/molecules22050774
Chemat, F., Vian, M. A., and Cravotto, G. (2012). Green Extraction of Natural Products: concept and Principles. Int. J. Mol. Sci. 13, 8615–8627. doi: 10.3390/IJMS13078615
Chiu, Y.-C., Chen, B.-J., Su, Y.-S., Huang, W.-D., and Chen, C.-C. (2021). A Leaf Disc Assay for Evaluating the Response of Tea (Camellia sinensis) to PEG-Induced Osmotic Stress and Protective Effects of Azoxystrobin against Drought. Plants 10:546. doi: 10.3390/plants10030546
Claeys, H., Landeghem, S., Van, Dubois, M., Maleux, K., Inzé, D., et al. (2014). What Is Stress? Dose-response effects in commonly used in vitro stress assays. Plant Physiol. 165, 519–527. doi: 10.1104/pp.113.234641
Colegate, S., and Molyneux, R. (2007). Bioactive Natural Products: Detection, Isolation, and Structural Determination. Florida: CRC Press.
Commo, F., and Bot, B. M. (2016). R Package nplr n-Parameter Logistic Regressions. V. 0.1–7. Seattle, Washington: Fred Hutchinson Cancer Research Center.
Dantas, M. S. M., Rolim, M. M., Duarte, A., de, S., and Lima, L. E. (2017). Production and morphological components of sunflower on soil fertilized with cassava wastewater. Rev. Ceres. 64, 77–82. doi: 10.1590/0034-737X201764010011
Daudi, A., and O’Brien, J. A. (2012). Detection of Hydrogen Peroxide by DAB Staining in Arabidopsis Leaves. Bio. Protoc. 2:e263. doi: 10.21769/bioprotoc.263
De Diego, N., Fürst, T., Humplík, J. F., Ugena, L., Podlešáková, K., and Spíchal, L. (2017). An Automated Method for High-Throughput Screening of Arabidopsis Rosette Growth in Multi-Well Plates and Its Validation in Stress Conditions. Front. Plant Sci. 8:1702. doi: 10.3389/FPLS.2017.01702
De Diego, N., and Spíchal, L. (2020). “Use of plant metabolites to mitigate stress effects in crops,” in The Chemical Biology of Plant Biostimulants, eds D. Geelen and L. Xu (Hoboken: Wiley Online Library), 261–300. doi: 10.1002/9781119357254.ch11
Deans, C. A., Sword, G. A., Lenhart, P. A., Burkness, E., Hutchison, W. D., and Behmer, S. T. (2018). Quantifying Plant Soluble Protein and Digestible Carbohydrate Content, Using Corn (Zea mays) As an Exemplar. J. Vis. Exp. 138:e58164. doi: 10.3791/58164
Djoumbou Feunang, Y., Eisner, R., Knox, C., Chepelev, L., Hastings, J., Owen, G., et al. (2016). ClassyFire: automated chemical classification with a comprehensive, computable taxonomy. J. Cheminform. 8:61. doi: 10.1186/s13321-016-0174-y
Dou, J., Xu, W., Koivisto, J. J., Mobley, J. K., Padmakshan, D., Kögler, M., et al. (2018). Characteristics of Hot Water Extracts from the Bark of Cultivated Willow (Salix sp.). ACS Sustain. Chem. Eng. 6, 5566–5573. doi: 10.1021/ACSSUSCHEMENG.8B00498
Dróżdż, P., and Pyrzynska, K. (2017). Assessment of polyphenol content and antioxidant activity of oak bark extracts. Eur. J. Wood Wood Prod. 76, 793–795. doi: 10.1007/S00107-017-1280-X
Dubois, M., and Inzé, D. (2020). Plant growth under suboptimal water conditions: early responses and methods to study them. J. Exp. Bot. 71, 1706–1722. doi: 10.1093/jxb/eraa037
Dumanović, J., Nepovimova, E., Natić, M., Kuča, K., and Jaćević, V. (2021). The significance of reactive oxygen species and antioxidant defense system in plants: a concise overview. Front. Plant Sci. 11:2106. doi: 10.3389/fpls.2020.552969
Evon, P., Vandenbossche, V., Candy, L., Pontalier, P.-Y., and Rouilly, A. (2018). “Twin-screw extrusion: A key technology for the biorefinery,”,” in Biomass Extrusion and Reaction Technologies: Principles to Practices and Future Potential, (ACS Publications), 25–44. doi: 10.1021/bk-2018-1304.ch002
FAO (2021). Sustainable Development Goals. Available online at: http://www.fao.org/sustainable-development-goals/indicators/en/ (accessed Jul 30, 2021)
Ferdinando, M., Di, Brunetti, C., Fini, A., Tattini, M., Di Ferdinando, M., et al. (2012). Flavonoids as Antioxidants in Plants Under Abiotic Stresses. In: P. Ahmad and M. Prasad (eds) Abiotic Stress Responses in Plants. Springer, New York, NY. doi: 10.1007/978-1-4614-0634-1_9
Fimognari, L., Dölker, R., Kaselyte, G., Jensen, C. N. G., Akhtar, S. S., Großkinsky, D. K., et al. (2020). Simple semi-high throughput determination of activity signatures of key antioxidant enzymes for physiological phenotyping. Plant Methods 16, 1–19. doi: 10.1186/S13007-020-00583-8
Fulcher, N., and Sablowski, R. (2009). Hypersensitivity to DNA damage in plant stem cell niches. Proc. Natl. Acad. Sci. 106, 20984–20988. doi: 10.1073/PNAS.0909218106
García-García, A. L., García-Machado, F. J., Borges, A. A., Morales-Sierra, S., Boto, A., and Jiménez-Arias, D. (2020). Pure Organic Active Compounds Against Abiotic Stress: a Biostimulant Overview. Front. Plant Sci. 0:1839. doi: 10.3389/FPLS.2020.575829
Ghosh, A., Pareek, A., and Singla-Pareek, S. (2015). Leaf Disc Stress Tolerance Assay for Tobacco. Bio-Protocol 5, e1440. doi: 10.21769/bioprotoc.1440
Hamad, S. W. (2017). Bioherbicidal Properties of Sunflower (Helianthus annuus L.) and its Activities in Weed Management. Available online at: http://theses.ncl.ac.uk/jspui/handle/10443/3755 (accessed Sep 9, 2021)
Hodges, D. M., DeLong, J. M., Forney, C. F., and Prange, R. K. (1999). Improving the thiobarbituric acid-reactive-substances assay for estimating lipid peroxidation in plant tissues containing anthocyanin and other interfering compounds. 207, 604–611. Planta 245:1067. doi: 10.1007/s00425-017-2699-3
Huang, H., Ullah, F., Zhou, D.-X., Yi, M., and Zhao, Y. (2019). Mechanisms of ROS regulation of plant development and stress responses. Front. Plant Sci. 10:800. doi: 10.3389/fpls.2019.00800
Huang, S., Zheng, X., Luo, L., Ni, Y., Yao, L., and Ni, W. (2021). Biostimulants in bioconversion compost of organic waste: a novel booster in sustainable agriculture. J. Clean. Prod. 319:128704.
ISO (2000). ISO 665:2000, Oilseeds—Determination of Moisture and Volatile Matter Content. Geneva: ISO.
Jiang, B., Shi, Y., Zhang, X., Xin, X., Qi, L., Guo, H., et al. (2017). PIF3 is a negative regulator of the CBF pathway and freezing tolerance in Arabidopsis. Proc. Natl. Acad. Sci. 114, E6695–E6702. doi: 10.1073/pnas.1706226114
Junglee, S., Urban, L., Sallanon, H., and Lopez-Lauri, F. (2014). Optimized Assay for Hydrogen Peroxide Determination in Plant Tissue Using Potassium Iodide. Am. J. Anal. Chem. 05, 730–736. doi: 10.4236/ajac.2014.511081
Kamal, J. (2013). Quantification of alkaloids, phenols and flavonoids in sunflower (Helianthus annuus L.). African J. Biotechnol. 10, 3149–3151. doi: 10.4314/ajb.v10i16
Karamać, M., Kosińska, A., Estrella, I., Hernández, T., and Duenas, M. (2012). Antioxidant activity of phenolic compounds identified in sunflower seeds. Eur. Food Res. Technol. 235, 221–230. doi: 10.1007/s00217-012-1751-6
Kaur, H., Bhardwaj, R. D., and Grewal, S. K. (2017). Mitigation of salinity-induced oxidative damage in wheat (Triticum aestivum L.) seedlings by exogenous application of phenolic acids. Acta Physiol. Plant. 39:221. doi: 10.1007/s11738-017-2521-7
Kerchev, P., van der Meer, T., Sujeeth, N., Verlee, A., Stevens, C. V., Van Breusegem, F., et al. (2020). Molecular priming as an approach to induce tolerance against abiotic and oxidative stresses in crop plants. Biotechnol. Adv. 40:107503. doi: 10.1016/j.biotechadv.2019.107503
Kicinska, A., and Jarmuszkiewicz, W. (2020). Flavonoids and Mitochondria: activation of Cytoprotective Pathways? Mol 25:3060. doi: 10.3390/molecules25133060
Kiokias, S., Proestos, C., and Oreopoulou, V. (2020). Phenolic acids of plant origin—A review on their antioxidant activity in vitro (o/w emulsion systems) along with their in vivo health biochemical properties. Foods 9:534. doi: 10.3390/foods9040534
Kiran, K. R., Deepika, V. B., Swathy, P. S., Prasad, K., Kabekkodu, S. P., Murali, T. S., et al. (2020). ROS-dependent DNA damage and repair during germination of NaCl primed seeds. J. Photochem. Photobiol. B Biol. 213:112050. doi: 10.1016/J.JPHOTOBIOL.2020.112050
Krasensky, J., and Jonak, C. (2012). Drought, salt, and temperature stress-induced metabolic rearrangements and regulatory networks. J. Exp. Bot. 63, 1593–1608. doi: 10.1093/jxb/err460
Kumar, R., Kumar, R., and Prakash, O. (2019). “Chapter-5 The Impact of Chemical Fertilizers on Our Environment and Ecosystem,” in Research Trends in Environmental Sciences, ed. P. Sharma (Delhi: Akinik Publications).
Kurepa, J., Shull, T. E., and Smalle, J. A. (2016). Quercetin feeding protects plants against oxidative stress. F1000Research 5:2430. doi: 10.12688/f1000research.9659.1
Lai, Z., Tsugawa, H., Wohlgemuth, G., Mehta, S., Mueller, M., Zheng, Y., et al. (2018). Identifying metabolites by integrating metabolome databases with mass spectrometry cheminformatics. Nat. Methods 15, 53–56. doi: 10.1038/nmeth.4512
Landini, G., Martinelli, G., and Piccinini, F. (2021). Colour deconvolution: stain unmixing in histological imaging. Bioinformatics 37, 1485–1487. doi: 10.1093/BIOINFORMATICS/BTAA847
Lee, J., da Silva, R. R., Jang, H. S., Kim, H. W., Kwon, Y. S., Kim, J.-H. H., et al. (2019). In silico annotation of discriminative markers of three Zanthoxylum species using molecular network derived annotation propagation. Food Chem. 295, 368–376. doi: 10.1016/j.foodchem.2019.05.099
Leschevin, M., Ismael, M., Quero, A., San Clemente, H., Roulard, R., Bassard, S., et al. (2021). Physiological and biochemical traits of two major Arabidopsis accessions, Col-0 and Ws, under salinity. Front. Plant Sci 12:639154. doi: 10.3389/fpls.2021.639154
Li, J., Van Gerrewey, T., and Geelen, D. (2022). A meta-analysis of biostimulant yield effectiveness in field trials. Front. Plant Sci 13:836702. doi: 10.3389/fpls.2022.836702
Lindsey, B. E., Rivero, L., Calhoun, C. S., Grotewold, E., and Brkljacic, J. (2017). Standardized method for high-throughput sterilization of Arabidopsis seeds. J. Vis. Exp. 2017:56587. doi: 10.3791/56587
Lutts, S., Benincasa, P., Wojtyla, L., Kubala, S S., Pace, R., Lechowska, K., et al. (2016). “Seed priming: new comprehensive approaches for an old empirical technique,” in New Challenges in Seed Biology, eds S. Araujo and A. Balestrazzi (London: IntechOpen), 1–46. doi: 10.5772/64420
Macías, F. A., Varela, R. M., Torres, A., Galindo, J. L. G., and Molinillo, J. M. G. (2002). Allelochemicals from sunflowers: chemistry, bioactivity and applications. In:A.U. Mallik ed Chemical Ecology of Plants: allelopathy in Aquatic and Terrestrial Ecosystems. Birkhäuser, Basel. doi: 10.1007/978-3-0348-8109-8_5
Maheswari, U. M., Murali Arthanari, P., and Uma Maheswari, C. M. (2019). Sunflower dried stalk extract: a natural Preemergence herbicide: effect on crops and weeds seed germination. J. Pharmacogn. Phytochem. 8, 135–137.
Malamy, J. E. (2005). Intrinsic and environmental response pathways that regulate root system architecture. Plant Cell Environ. 28, 67–77. doi: 10.1111/J.1365-3040.2005.01306.X
Markets and Markets (2021). Biostimulants research global trends in 2021. MarketsandMarkets. Available online at:https://www.biostimulant.com/blog-29-biostimulants-research-global-trends-in-2021/ (accessed on Mar 24, 2022)
Meijering, E., Jacob, M., Sarria, J. C. F., Steiner, P., Hirling, H., and Unser, M. (2004). Design and Validation of a Tool for Neurite Tracing and Analysis in Fluorescence Microscopy Images. Cytom. Part A 58, 167–176. doi: 10.1002/CYTO.A.20022
Mhamdi, A., and Van Breusegem, F. (2018). Reactive oxygen species in plant development. Development 145:dev164376. doi: 10.1242/dev.164376
Munns, R., and Tester, M. (2008). Mechanisms of salinity tolerance. Annu. Rev. Plant Biol. 59, 651–681. doi: 10.1146/annurev.arplant.59.032607.092911
Noctor, G., Mhamdi, A., and Foyer, C. H. (2016). Oxidative stress and antioxidative systems: recipes for successful data collection and interpretation. Plant Cell Environ. 39, 1140–1160. doi: 10.1111/PCE.12726
Parfitt, J., Barthel, M., and Macnaughton, S. (2010). Food waste within food supply chains: quantification and potential for change to 2050. Philos. Trans. R. Soc. B Biol. Sci. 365, 3065–3081. doi: 10.1098/RSTB.2010.0126
Pękal, A., and Pyrzynska, K. (2014). Evaluation of Aluminium Complexation Reaction for Flavonoid Content Assay. Food Anal. Methods 7, 1776–1782. doi: 10.1007/S12161-014-9814-X
Perveen, S. (2017). ““Phenolic Compounds from the Natural Sources and Their Cytotoxicity,”,” in Natural Sources, Importance and Applications, eds M. Soto-Hernandez, M. Palma-Tenango, and M. D. R. Garcia-Mateos (London: IntechOpen), doi: 10.5772/66898
R Core Team (2021). R: A Language and Environment for Statistical Computing. Vienna, Austria: R Version 4. 1. 1, R Foundation for Statistical Computing.
Rasouli, H., Farzaei, M. H., Mansouri, K., Mohammadzadeh, S., and Khodarahmi, R. (2016). Plant Cell Cancer: may Natural Phenolic Compounds Prevent Onset and Development of Plant Cell Malignancy? A Literature Review. Moecules 21, 1104. doi: 10.3390/molecules21091104
Rawat, L. S., Maikhuri, R. K., Bahuguna, Y. M., Jha, N. K., and Phondani, P. C. (2017). Sunflower allelopathy for weed control in agriculture systems. J. Crop Sci. Biotechnol. 20, 45–60. doi: 10.1007/S12892-016-0093-0
Regulation [EC] (2005). Regulation (EC) No 396/2005 of the European Parliament and of the Council of 23 February 2005 on maximum residue levels of pesticides in or on food and feed of plant and animal origin and amending Council Directive 91/414/EEC. Available online at:https://eur-lex.europa.eu/legal-content/EN/ALL/?uri=celex%3A32005R0396 (accessed on Nov 10, 2021].
Regulation [EC] (2009). Regulation (EC) No 1107/2009 of the European Parliament and of the Council of 21 October 2009 concerning the placing of plant protection products on the market and repealing Council Directives 79/117/EEC and 91/414/EEC. Available online at:https://eur-lex.europa.eu/legal-content/EN/TXT/?uri=celex%3A32009R1107 (Accessed on Nov 10, 2021).
Regulation [EU] (2019). Regulation (EU) 2019/1009 of the European Parliament and of the Council of 5 June 2019 laying down rules on the making available on the market of EU fertilising products and amending Regulations (EC) No 1069/2009 and (EC) No 1107/2009 and repealing Regula. Available online at:https://eur-lex.europa.eu/legal-content/EN/TXT/PDF/?uri=CELEX:32019R1009&from=EN (accessed on Jul 30, 2021).
Rook, F., Hadingham, S. A., Li, Y., and Bevan, M. W. (2006). Sugar and ABA response pathways and the control of gene expression. Plant Cell Environ. 29, 426–434. doi: 10.1111/j.1365-3040.2005.01477.x
Rosa, M., Harder, M., Von, Cigliano, R. A., Schlögelhofer, P., and Scheid, O. M. (2013). The Arabidopsis SWR1 Chromatin-Remodeling Complex Is Important for DNA Repair. Somatic Recombination, and Meiosis. Plant Cell 25, 1990–2001. doi: 10.1105/TPC.112.104067
Rosa, M., and Scheid, O. (2014). DNA Damage Sensitivity Assays with Arabidopsis Seedlings. Bio-Protocol 4:e1093. doi: 10.21769/bioprotoc.1093
Rouphael, Y., and Colla, G. (2020). Biostimulants in agriculture. Front. Plant Sci. 11:40. doi: 10.3389/FPLS.2020.00040
Ruifrok, A. C., and Johnston, D. A. (2001). Quantification of histochemical staining by color deconvolution. Anal. Quant. Cytol. Histol. 23, 291–299.
Šamec, D., Karalija, E., Šola, I., Vujčić Bok, V., Salopek-Sondi, B., Bok, V. V., et al. (2021). The Role of polyphenols in abiotic stress response: the influence of molecular structure. Plants 10:118. doi: 10.3390/PLANTS10010118
Sánchez-Rangel, J. C., Benavides, J., Heredia, J. B., Cisneros-Zevallos, L., and Jacobo-Velázquez, D. A. (2013). The Folin–Ciocalteu assay revisited: improvement of its specificity for total phenolic content determination. Anal. Methods 5, 5990–5999. doi: 10.1039/C3AY41125G
Schindelin, J., Arganda-Carreras, I., Frise, E., Kaynig, V., Longair, M., Pietzsch, T., et al. (2012). Fiji: an open-source platform for biological-image analysis. Nat. Methods 9, 676–682. doi: 10.1038/nmeth.2019
Schymanski, E. L., Jeon, J., Gulde, R., Fenner, K., Ruff, M., Singer, H. P., et al. (2014). Identifying small molecules via high resolution mass spectrometry: communicating confidence. Environ. Sci. Technol. 48, 2097–2098. doi: 10.1021/es5002105
Van Soest, P. J., and Wine, R. H. (1967). Use of Detergents in the Analysis of Fibrous Feeds. IV. Determination of Plant Cell-Wall Constituents. J. AOAC Int. 50, 50–55. doi: 10.1093/JAOAC/50.1.50
Stagos, D. (2020). Antioxidant Activity of Polyphenolic Plant Extracts. Antioxidants 9:19. doi: 10.3390/ANTIOX9010019
Svolacchia, N., Salvi, E., and Sabatini, S. (2020). Arabidopsis primary root growth: let it grow, can’t hold it back anymore! Curr. Opin. Plant Biol. 57, 133–141. doi: 10.1016/J.PBI.2020.08.005
Szparaga, A., Kocira, S., Findura, P., Kapusta, I., Zaguła, G., and Świeca, M. (2021). Uncovering the Multi-level Response of Glycine Max L. To the Application of Allelopathic Biostimulant From Levisticum Officinale Koch. Sci. Rep. 11:15360. doi: 10.1038/s41598-021-94774-5
Tanase, C., Coşarcă, S., and Muntean, D. L. (2019). A critical review of phenolic compounds extracted from the bark of woody vascular plants and their potential biological activity. Molecules 24:1182. doi: 10.3390/MOLECULES24061182
Tennekes, M., and Ellis, P. (2021). treemap: Treemap visualization. R Packag. version, 2–4. Available online at:https://cran.r-project.org/package=treemap. (accessed March 24, 2022).
Trinh, H. K., Verstraeten, I., and Geelen, D. (2018). In Vitro Assay for Induction of Adventitious Rooting on Intact Arabidopsis Hypocotyls,”. Methods Mol. Biol. 1761, 95–102. doi: 10.1007/978-1-4939-7747-5_7
Tsugawa, H., Cajka, T., Kind, T., Ma, Y., Higgins, B., Ikeda, K., et al. (2015). MS-DIAL: data-independent MS/MS deconvolution for comprehensive metabolome analysis. Nat. Methods 12, 523–526. doi: 10.1038/nmeth.3393
Tsugawa, H., Kind, T., Nakabayashi, R., Yukihira, D., Tanaka, W., Cajka, T., et al. (2016). Hydrogen Rearrangement Rules: computational MS/MS Fragmentation and Structure Elucidation Using MS-FINDER Software. Anal. Chem. 88, 7946–7958. doi: 10.1021/acs.analchem.6b00770
Tsugawa, H., Nakabayashi, R., Mori, T., Yamada, Y., Takahashi, M., Rai, A., et al. (2019). A cheminformatics approach to characterize metabolomes in stable-isotope-labeled organisms. Nat. Methods 16, 295–298. doi: 10.1038/s41592-019-0358-2
United Nations Industrial Development (2007). Industrial Biotechnology and Biomass Utilisation.Prospects and Challenges for the Developing World. Vienna: United Nations Industrail Development
Van Soest, P. J., and Wine, R. H. (1968). Determination of Lignin and Cellulose in Acid-Detergent Fiber with Permanganate. J. AOAC Int. 51, 780–785. doi: 10.1093/JAOAC/51.4.780
Van Tang, N. (2017). Recovering Bioactive Compounds from Agricultural Wastes. Hoboken: John Wiley & Sons, Ltd, doi: 10.1002/9781119168850
Vandenbossche, V., Candy, L., Evon, P., Rouilly, A., and Pontalier, P.-Y. (2019). “0 - Extrusion,” in 1, eds F. Chemat and E. Vorobiev (Cambridge: Academic Press), 289–314. doi: 10.1016/B978-0-12-815353-6.00010-0
Vanhoudt, N. (2014). Enzyme Analysis by Spectrophotometry - Plate Reader method. Available online at: https://radioecology-exchange.org/sites/default/files/Enzyme%20Analysis%20by%20Spectrophotometry%20-%20Plate%20Reader%20method.pdf (accessed March 24, 2022).
Vaniya, A., Samra, S. N., Palazoglu, M., Tsugawa, H., and Fiehn, O. (2017). Using MS-FINDER for identifying 19 natural products in the CASMI 2016 contest. Phytochem. Lett. 21, 306–312. doi: 10.1016/j.phytol.2016.12.008
Venturelli, S., Petersen, S., Langenecker, T., Weigel, D., Lauer, U. M., and Becker, C. (2016). Allelochemicals of the phenoxazinone class act at physiologically relevant concentrations. Plant Signal. Behav. 11:e1176818. doi: 10.1080/15592324.2016.1176818
Verdier, T., Balthazard, L., Montibus, M., Magniont, C., Evon, P., and Bertron, A. (2020). Using glycerol esters to prevent microbial growth on sunflower-based insulation panels. Proc. ICE - Constr. Mater. 174, 140–149. doi: 10.1680/JCOMA.20.00002
Vertified Market Research (2021). Global Biostimulants Market Size By Active Ingredient(Humic Substances, Microbial Amendments, Seaweed Extracts), By Application Method(Seed Treatment, Soil Treatment, Foliar Treatment), By Crop Type(Turfs and Ornamentals, Fruits and Vegetables, Row Crops). Available online at:https://www.verifiedmarketresearch.com/product/biostimulants-market/ (accessed on Jul 30, 2021)
Xiao, F., Xu, T., Lu, B., and Liu, R. (2020). Guidelines for antioxidant assays for food components. Food Front. 1, 60–69. doi: 10.1002/fft2.10
Xu, L., and Geelen, D. (2018). Developing biostimulants from agro-food and industrial by-products. Front. Plant Sci. 9:1567. doi: 10.3389/fpls.2018.01567
Xu, M., Qi, M., Goff, H. D., and Cui, S. W. (2020). Polysaccharides from sunflower stalk pith: chemical, structural and functional characterization. Food Hydrocoll. 100:105082. doi: 10.1016/J.FOODHYD.2019.04.053
Ye, F., Liang, Q., Li, H., and Zhao, G. (2015). Solvent effects on phenolic content, composition, and antioxidant activity of extracts from florets of sunflower (Helianthus annuus L.). Ind. Crops Prod. 76, 574–581. doi: 10.1016/J.INDCROP.2015.07.063
Zhang, X., Yang, H., and Cui, Z. (2017). Alleviating Effect and Mechanism of Flavonols in Arabidopsis Resistance under Pb-HBCD Stress. ACS Sustain. Chem. Eng. 5, 11034–11041. doi: 10.1021/acssuschemeng.7b02971
Keywords: Helianthus annuus, plant extract, biostimulant, in vitro assay, salt stress, antioxidant
Citation: Li J, Evon P, Ballas S, Trinh HK, Xu L, Van Poucke C, Van Droogenbroeck B, Motti P, Mangelinckx S, Ramirez A, Van Gerrewey T and Geelen D (2022) Sunflower Bark Extract as a Biostimulant Suppresses Reactive Oxygen Species in Salt-Stressed Arabidopsis. Front. Plant Sci. 13:837441. doi: 10.3389/fpls.2022.837441
Received: 16 December 2021; Accepted: 30 May 2022;
Published: 01 July 2022.
Edited by:
Youssef Rouphael, University of Naples Federico II, ItalyReviewed by:
Anket Sharma, University of Maryland, College Park, United StatesMiroslava Konstantinova Zhiponova, Sofia University, Bulgaria
Copyright © 2022 Li, Evon, Ballas, Trinh, Xu, Van Poucke, Van Droogenbroeck, Motti, Mangelinckx, Ramirez, Van Gerrewey and Geelen. This is an open-access article distributed under the terms of the Creative Commons Attribution License (CC BY). The use, distribution or reproduction in other forums is permitted, provided the original author(s) and the copyright owner(s) are credited and that the original publication in this journal is cited, in accordance with accepted academic practice. No use, distribution or reproduction is permitted which does not comply with these terms.
*Correspondence: Danny Geelen, RGFubnkuR2VlbGVuQFVHZW50LmJl
†Present address: Lin Xu, Department of Food Technology, Safety and Health, Faculty of Bioscience Engineering, Ghent University, Kortrijk, Belgium