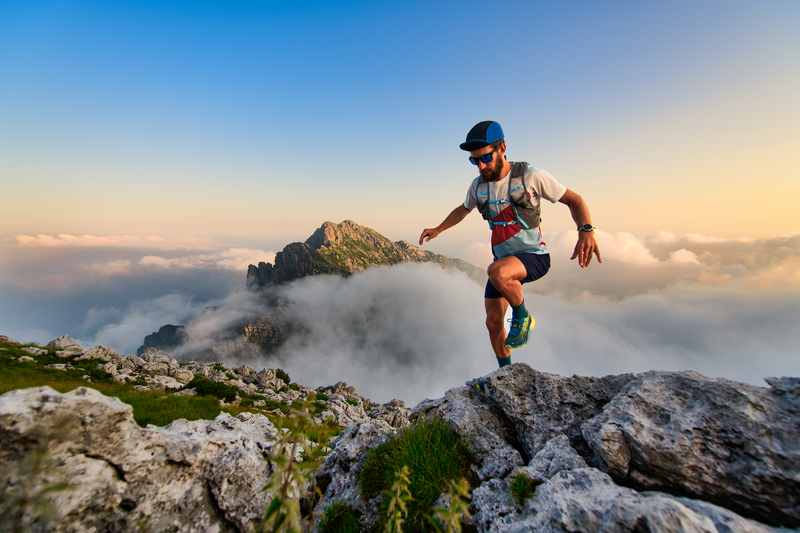
95% of researchers rate our articles as excellent or good
Learn more about the work of our research integrity team to safeguard the quality of each article we publish.
Find out more
ORIGINAL RESEARCH article
Front. Plant Sci. , 10 February 2022
Sec. Plant Cell Biology
Volume 13 - 2022 | https://doi.org/10.3389/fpls.2022.836519
This article is part of the Research Topic Molecular Basis for Photomorphogenic Development View all 7 articles
Light-triggered transcriptome reprogramming is critical for promoting photomorphogenesis in Arabidopsis seedlings. Nonetheless, recent studies have shed light on the importance of alternative pre-mRNA splicing (AS) in photomorphogenesis. The splicing factors splicing factor for phytochrome signaling (SFPS) and reduced red-light responses in cry1cry2 background1 (RRC1) are involved in the phytochrome B (phyB) signaling pathway and promote photomorphogenesis by controlling pre-mRNA splicing of light- and clock-related genes. However, splicing factors that serve as repressors in phyB signaling pathway remain unreported. Here, we report that the splicing factor SWELLMAP 2 (SMP2) suppresses photomorphogenesis in the light. SMP2 physically interacts with phyB and colocalizes with phyB in photobodies after light exposure. Genetic analyses show that SMP2 antagonizes phyB signaling to promote hypocotyl elongation in the light. The homologs of SMP2 in yeast and human belong to second-step splicing factors required for proper selection of the 3' splice site (3'SS) of an intron. Notably, SMP2 reduces the abundance of the functional REVEILLE 8 a (RVE8a) form, probably by determining the 3'SS, and thereby inhibits RVE8-mediated transcriptional activation of clock genes containing evening elements (EE). Finally, SMP2-mediated reduction of functional RVE8 isoform promotes phytochrome interacting factor 4 (PIF4) expression to fine-tune hypocotyl elongation in the light. Taken together, our data unveil a phyB-interacting splicing factor that negatively regulates photomorphogenesis, providing additional information for further mechanistic investigations regarding phyB-controlled AS of light- and clock-related genes.
As one of the most essential environmental factors for plants, light modulates various developmental processes of plants throughout their life cycles. Seedlings undergo skotomorphogenesis when grown in darkness, which is characterized by elongated hypocotyls, closed cotyledons and curved apical hooks. While grown in the light, seedlings possess short hypocotyls, expanded cotyledons and developed chloroplasts. This seedling photomorphogenesis process is vital for the survival and autotrophic growth of plants. Plants have evolved multiple photoreceptors to perceive different wavelength of sunlight (Galvão and Fankhauser, 2015; Paik and Huq, 2019). Among these, phytochromes (phyA-phyE in Arabidopsis) are responsible for the perception of red (R) and far-red (FR) light. Phytochromes harbor two functional modules: the N-terminal photosensory module (PSM) which perceives light through the chromophore, and the C-terminal output module (OPM) responsible for dimerization, nuclear localization as well as signal transduction (Cheng et al., 2021). Phytochromes exist as two photo-convertible forms, the active Pfr form absorbing FR light and the inactive Pr form absorbing R light (Burgie and Vierstra, 2014; Burgie et al., 2014). After exposure to R light or high R/FR ratio light conditions, the phytochromes (Pr form) will rapidly convert to Pfr form and translocate from cytosol into the nucleus, after which nuclear speckles called photobodies will appear (Klose et al., 2015; Cheng et al., 2021). The size and number of photobodies tightly correlate with the activity of phytochromes (Huq et al., 2003; Chen and Chory, 2011; Klose et al., 2015). In the past decades, numerous case studies have been reported for signaling transduction from phytochromes to transcriptional factors. Photo-activated phytochromes globally regulate transcriptome reprogramming by modulating stability or activity of many key transcription factors, such as PHYTOCHROME-INTERACTING FACTORS (PIFs) and ELONGATED HYPOCOTYL 5 / HY5-HOMOLOG (HY5/HYH) (Al-Sady et al., 2006; Shen et al., 2008; Legris et al., 2019; Cheng et al., 2021). Interestingly, recent evidences have shown that phytochromes also regulate alternative pre-mRNA splicing in response to red light (Shikata et al., 2014; Xin et al., 2017, 2019; Dong et al., 2020).
In eukaryotes, intron-containing pre-mRNA need to undergo a splicing process which removes the introns and joins the flanking exons together to make a mature mRNA. A large dynamic ribonucleoprotein complex called spliceosome accomplish the splicing process through recognizing four loosely conserved nucleotide sequences (Lorković et al., 2000; Wahl et al., 2009; Kornblihtt et al., 2013; Shi, 2017). They are the 5' splice site (5'SS) with a conserved GU nucleotides, the 3'SS with a conserved AG, a branch point (BP) with a conserved adenosine residue and a polypyrimidine tract upstream of the 3'SS (Lorković et al., 2000; Kornblihtt et al., 2013). The major core spliceosome complex is composed of five uridine-rich (U-rich) small nuclear ribonucleoproteins (snRNPs), including U1, U2, U4, U5, and U6 (Wahl et al., 2009; Lee and Rio, 2015; Shi, 2017). Mandatory inclusion of exons and exclusion of introns in mRNA is termed constitutive splicing (CS). By contrast, alternative splicing (AS) events involve the selective inclusion of introns or exons from pre-mRNA into mature mRNA, and different mRNA isoforms from a single gene will be produced. There are four major types of AS events in plants, including exon skipping (ES), intron retention (IR), alternative 5' splice site (A5'SS) and alternative 3' splice site (A3'SS) (Marquez et al., 2012; Reddy et al., 2013). The subtle regulation of AS is rather complicated. Splicing factors (SFs), such as heterogeneous nuclear ribonucleoproteins (hnRNPs) and serine–arginine repeat proteins (SRs), are essential for regulating AS through binding to cis-regulatory elements (silencers or enhancers) (Kornblihtt et al., 2013; Lee and Rio, 2015). The interaction between SFs and snRNPs can also change the splice sites determination in pre-mRNA (Kornblihtt et al., 2013; Lee and Rio, 2015). In addition, the chromatin-based effects, such as recruitment of SFs by “adaptors” of histone modifications and transcriptional elongation rate, also play roles in changing the splicing patterns (Kornblihtt et al., 2013; Lee and Rio, 2015).
Genome-wide analyses have revealed that a large scale of AS profiles changed in response to light in Arabidopsis thaliana (Shikata et al., 2014; Hartmann et al., 2016). Gene ontology (GO) analyses show that numerous genes regulated by light-controlled AS are involved in “response to light stimulus,” “circadian clock” and “photosynthesis” biological processes (Shikata et al., 2014; Xin et al., 2017, 2019). To better understand the molecular mechanism by which light regulates these AS patterns, numerous studies have focused on this in the past few years. It is reported that light-triggered nuclear AS is regulated by a chloroplast retrograde signal (Petrillo et al., 2014). Moreover, light-increased transcriptional elongation rate modulates AS decisions (Godoy Herz et al., 2019). Recently, it is shown that photosynthesized sugars, a shoot-to-root mobile signal, coordinate AS responses to light throughout the whole plant in a TOR kinase-dependent manner (Riegler et al., 2021). Phytochromes are the best studied photoreceptors involved in the regulation of red light-mediated AS till date (Shikata et al., 2012, 2014; Wu et al., 2014; Shih et al., 2019; Lin et al., 2020; Kathare and Huq, 2021). Photo-activated phyB induces a specific intron retention in 5' UTR of PIF3 mRNA, thereby inhibits PIF3 protein synthesis to promote photomorphogenesis (Dong et al., 2020). REDUCED RED-LIGHT RESPONSES IN CRY1CRY2 BACKGROUND1 (RRC1) and SPLICING FACTOR FOR PHYTOCHROME SIGNALING (SFPS) are identified as the two splicing factors which can directly interact with phyB in Arabidopsis (Xin et al., 2017, 2019). These two SFs can form a complex and coordinate pre-mRNA splicing of a subset of light- and clock-associated genes to promote photomorphogenesis (Xin et al., 2017, 2019). For instance, RRC1/SFPS can directly associate with clock regulator EARLY FLOWERING 3 (ELF3) pre-mRNA to regulate its proper splicing. Moreover, PIFs are reported to act downstream of RRC1/SFPS in the regulation of photomorphogenesis (Xin et al., 2017, 2019).
Here we identify another splicing factor SWELLMAP 2 (SMP2) that can physically interact with phyB. SMP2 genetically acts downstream of phyB, and promotes A3'SS of key clock regulator RVE8 to negatively regulate seedling photomorphogenesis in Arabidopsis.
The ecotype of Arabidopsis thaliana used in this study was Columbia-0 (Col-0). T-DNA insertion mutant smp2-1 (Salk_022202) was from Arabidopsis Biological Resource Center (ABRC), smp2-3 mutant was generated by CRISPR/Cas9 (Wang et al., 2015). phyB-9 mutant, phyB-CFP and PIF4-ox transgenic plants were reported previously (Reed et al., 1993; Chen et al., 2005; Lee et al., 2020). Seeds were sterilized with 20% (v/v) bleach containing 0.1% Triton X-100 for 10 min, washed at least five times with sterile water, and sown on 1 × Murashige and Skoog (MS) medium supplemented with 1% sucrose and 0.8% agar. After 3 days of stratification at 4°C in darkness, seeds were transferred into the plant growth chamber (PERCIVAL) maintained at 22°C.
To measure the hypocotyl length of seedlings, seeds were sown on plates and stratified in darkness at 4°C for 3 d, followed by incubation in continuous white light for 12 h to induce synchronous germination. The plates were then transferred to continuous dark (D), white (W), red (R), far-red (FR), and blue (B) light conditions and incubated at 22°C for 5 days and the hypocotyl length of seedlings were measured by ImageJ software.
To generate pLexA-phyB constructs for yeast two-hybrid assay, N-terminal (1-651 aa) and C-terminal (652-1172 aa) fragments of phyB CDS were amplified by Super-Fidelity DNA Polymerase (Vazyme) and inserted into the EcoR I/Xho I sites of pLexA vector (Clontech). For pB42AD-SMP2 plasmid, full-length SMP2 CDS was inserted into EcoR I/Xho I sites of pB42AD (Clontech).
For firefly luciferase complementation imaging (LCI) assays, the full-length phyB CDS were inserted into the Kpn I/Sal I sites of pCambia1300-nLUC, and SMP2 CDS were inserted into the BamH I/Sal I sites of pCambia1300-cLUC.
To generate overexpression of YFP-SMP2 construct, the Gateway cloning technology was used. Full-length SMP2 open reading frame was cloned into the pDONR-223 vector using Gateway BP Clonase Enzyme mix (Invitrogen), and introduced into the plant binary vector pEarley Gateway 104 under the control of the 35S promoter using Gateway LR Clonase Enzyme mix (Invitrogen).
The pEarley Gateway-YFP-SMP2 construct was transformed into Agrobacterium tumefaciens GV3101 by the freeze-thaw method and introduced into Col-0 via the floral dip method (Clough and Bent, 1998). Transgenic plants were selected on MS medium containing 20 mg/L Basta.
Yeast two hybrid assays in the LexA system were performed according to the Yeast Protocols Handbook (Clontech). Yeast strain EGY48 containing p8op-LacZ plasmid is used in the study. Transformants were first selected and grown on minimal synthetic defined (SD) base supplemented with -His-Trp-Ura dropout at 30°C, and then transferred to SD/-His-Trp-Ura dropout plates containing 80 mg/L X-gal for blue color development.
LCI assays were performed as described previously (Chen et al., 2008). The nLUC- and cLUC-fused plasmids were transformed into Agrobacterium strain GV3101, and the indicated transformants were mixed and infiltrated into Nicotiana benthamiana leaves. The plants were grown in darkness for 2 d followed by 24h red light exposure. After that, the luciferase signals were measured using NightShade LB985 (Berthold Technologies). The experiments were performed with three biological replicates.
For Co-IP assay, YFP-SMP2, Col-0 and phyB-9 seedlings were used. The total proteins were extracted by Lysis buffer [50 mM Tris-HCl pH7.5, 150 mM NaCl, 10% glycerol, 0.05% Tween 20, 1 mM PMSF, 1 × protease inhibitor cocktail (Roche)]. Four hundred μg total proteins were incubated with 10 μL GFP-Trap agarose (Chromotek) and rotated at 4°C for 4 h. After incubation, the agarose was washed five times with wash buffer [50 mM Tris-HCl pH7.5, 300 mM NaCl, 10% glycerol, 1 mM PMSF, 1 × protease inhibitor cocktail (Roche)]. The precipitates were boiled in 1 × SDS loading buffer for 10 min, and then the supernatants were analyzed by western blot using anti-GFP and anti-phyB antibodies, respectively.
Five-day-old Arabidopsis seedlings were homogenized in a protein denatured extraction buffer (100 mM NaH2PO4 pH8.0, 10 mM Tris-HCl pH8.0, 200 mM NaCl, 8 M urea, 1 mM PMSF, 1 × protease inhibitor cocktail). Antibodies used in this study were anti-phyB (PhytoAB), anti-GFP (Abmart) and anti-Actin (Sigma-Aldrich).
Subcellular localization observation of YFP-SMP2 and PHYB-CFP were performed using a confocal laser scanning microscope Zeiss LSM880 (Carl Zeiss). For YFP fluorescence detection, the excitation wavelength was 514 nm and the emission spectra were collected from 519 to 620 nm. For CFP fluorescence detection, the excitation wavelength was 405 nm and the emission spectra were collected from 410 to 513 nm.
Total RNA was extracted from Arabidopsis whole seedlings with indicated treatments using the Plant RNA kit (Omega). First-strand cDNAs were synthesized from 2 μg of total RNA using 5 × All-In-One RT Master Mix (Applied Biological Materials) according to the manufacturer's instructions. Real-time qPCR was performed using QuanStudioTM 6 Flex Real-Time PCR detection system (Applied Biosystems) and Hieff qPCR SYBR Green Master Mix (YEASEN). The expression levels were normalized to that of PP2A gene. The primers used in this study were listed in Supplementary Table 1.
Using the C-terminal output module of phyB as bait, we performed a yeast two-hybrid screen to look for additional splicing regulators involved in light signaling. We found that a protein called SWELLMAP 2 (SMP2), whose homolog Slu7/hSlu7 is a second-step splicing factor required for proper 3' splice site selection (Frank and Guthrie, 1992; Chua and Reed, 1999; Clay and Nelson, 2005), interacted with C-terminal of phyB in yeast (Figures 1A,B). To further verify the interaction between phyB and SMP2 in vivo, we first carried out split-luciferase complementation assay. As shown in Figure 1C, an appreciable bioluminescence signal was detected only when phyB-nLUC and cLUC-SMP2 were co-expressed in tobacco leaf. We further performed co-IP assay using transgenic plant overexpressing YFP-SMP2 (Supplementary Figure 1), and the data show that endogenous phyB protein could be co-immunoprecipitated by YFP-SMP2 in red-light condition (Figure 1D), suggesting that phyB was associated with SMP2 in vivo. What's more, to investigate whether SMP2 and phyB colocalized with each other in vivo, we crossed YFP-SMP2 with phyB-CFP transgenic plants. As shown in Figure 1E, YFP-SMP2 distributed mainly in the nucleus when transgenic plants were grown under continuous red light, and some YFP-SMP2 could colocalize with phyB-CFP in photobodies. Taken together, these results demonstrate that phyB physically interacts with SMP2 in vitro and in vivo.
Figure 1. phyB physically interacts with SMP2 in vitro and in vivo. (A) Schematic diagram of phyB fragments. Numbers indicate the amino acid positions in phyB protein. (B) The interaction of SMP2 and phyB in yeast. AD, activation domain; BD, DNA-binding domain. (C) Firefly luciferase complementation imaging (LCI) assay showing interaction between phyB and SMP2 in tobacco leaf. nLUC, the N-terminal fragment of firefly luciferase (LUC); cLUC, the C-terminal fragment of LUC. Full-length phyB and SMP2 were fused to the nLUC and cLUC, respectively. (D) Co-IP assay showing the association between SMP2 and phyB. Seedlings grown in the dark were transferred to red light (145 μmol/m2·s) for 1 h. YFP-SMP2 proteins were pulled down with GFP-trap beads. α-GFP, anti-GFP antibody; α-phyB, anti-phyB antibody. (E) Colocalization analysis of SMP2 and phyB in vivo. Transgenic plants co-expressing YFP-SMP2 and phyB-CFP were grown in continuous red light (145 μmol/m2·s) for 5 days. The images of nucleus came from a hypocotyl cell. YFP-SMP2 fusion proteins were excited by laser at 514 nm, and the emitted fluorescence signaling was collected from 519 nm to 620 nm; phyB-CFP were excited by laser at 405 nm, and the emitted fluorescence was collected from 410 nm to 513 nm. Scale bar, 5 μm. White arrowheads indicate SMP2 and phyB colocalized in photobodies; Red arrowheads indicate the SMP2-specific nuclear speckles.
To investigate whether SMP2 is involved in light-controlled morphogenesis, a smp2 null mutant, smp2-3, was generated by the Clustered Regulatory Interspaced Short Palindromic Repeats (CRISPR)/Cas9 technique. Smp2-3 contained a 519-bp deletion within the SMP2 genomic DNA and could produce a truncated 62-amino-acid protein resulting from a premature stop codon (Supplementary Figure 2). Additionally, the T-DNA insertion mutant smp2-1 (SALK_022202) (Clay and Nelson, 2005; Liu et al., 2016) was also used in this study. Both smp2 mutants showed a similar etiolated phenotype as Col-0 (wild-type) when grown in the dark (Figure 2; Supplementary Figure 3). However, both smp2-1 and smp2-3 displayed significantly shorter hypocotyls compared to Col-0 when grown in continuous white, red, far-red and blue light conditions (Figure 2; Supplementary Figure 3). These data indicate that SMP2 promotes hypocotyl elongation of seedlings in the light.
Figure 2. smp2 mutants are hypersensitive to light. (A) Visual phenotypes of 5-day-old wild-type (Col-0) and smp2 seedlings grown in the dark or different light conditions. White light, 7.5 μmol·m−2·s−1; Red light, 47 μmol·m−2·s−1; Far-red light, 4.5 μmol·m−2·s−1; Blue light, 3.0 μmol·m−2·s−1. Scale bars, 5 mm. (B) Quantification of hypocotyl lengths of Col-0 and smp2 seedlings grown under conditions as indicated in (A). Error bars represent standard deviation (SD), n ≥ 25; ***P < 0.001 (t-test); NS, not significant (t-test). Experiments were performed three times with similar results.
As shown in Figure 1, the biochemical evidences show that SMP2 physically interacts with phyB. To further explore the genetic relationship between phyB and SMP2, we respectively generated the double mutant smp2-1 phyB-9 and smp2-3 phyB-9 through genetic crossing (Supplementary Figure 4). Given that phyB plays prominent role in red light signaling, these mutants were grown in red light and hypocotyl lengths were measured. phyB-9 exhibited extremely long hypocotyl in red light as previously reported, while both smp2-1 phyB-9 and smp2-3 phyB-9 mutants showed significantly shorter hypocotyl compared with phyB-9 in red light (Figure 3; Supplementary Figure 5). This genetic analysis indicates that SMP2 genetically acts downstream of phyB to promote hypocotyl elongation in red light.
Figure 3. SMP2 genetically acts downstream of phyB. (A) Visual phenotypes of wild-type (Col-0), smp2-1, smp2-3, phyB-9, smp2-1 phyB-9, and smp2-3 phyB-9 seedlings grown for 5 days in continuous red-light condition (145 μmol·m−2·s−1). Scale bars, 5 mm. (B) Quantification of hypocotyl length of different seedlings as shown in (A). Error bars represent SD, n ≥ 25. Letters above the bars indicate significant differences (P < 0.05), as determined by one-way analysis of variance (ANOVA) with Duncan's post-hoc analysis. Experiments were performed three times with similar results.
Previous transcriptome analyses have revealed that several circadian clock regulators are subject to light-induced alternative splicing (Shikata et al., 2014; Mancini et al., 2016). In addition, phyB-interacting splicing factors, SFPS and RRC1, could also modulate photomorphogenesis by controlling pre-mRNA splicing of light signaling and clock genes such as CBK1, ELF3, and RVE8 (Xin et al., 2017, 2019). To further investigate whether SMP2 was involved in regulating AS of circadian clock regulators, we harvested seedlings released to constant white light (LL) after 6-day diurnal entrainment (Figure 4A) and monitored the AS patterns of various circadian clock genes in Col-0 and smp2-3 by RT-PCR and qPCR. Interestingly, we found that the AS patterns of RVE8 (James et al., 2012; Mancini et al., 2016) were altered in smp2-3 (Supplementary Figure 6). RVE8a was generated by the complete exclusion of intron 7, and two extra AS events happened within RVE8 intron 7 were also validated by sequencing. RVE8b was generated by an alternative 3' splice site selection that inserted 22nt into RVE8 mRNA, whereas RVE8c was generated by the complete retention of the intron 7 (Figure 4B). To quantify the abundance of these three isoforms, we designed three primer pairs to amplify specific transcripts (Figure 4B). The results showed that the expression of all three isoforms exhibited rhythmic changes in both Col-0 and smp2-3 (Figures 4C–E). However, the peak abundance of the functional isoform RVE8a was higher in smp2-3 than in Col-0 (Figure 4C), whereas the isoform RVE8b was significantly lower in smp2-3 than in Col-0 (Figure 4D). In contrast, the abundance of the isoform RVE8c was similar in smp2-3 and Col-0 (Figure 4E). RVE8, which encodes a MYB-like transcription factor, is a homolog of the key clock regulators CCA1 and LHY (Farinas and Mas, 2011; Rawat et al., 2011). The three transcription factors all bind specifically to the Evening Element (EE) promoter motif (Hsu et al., 2013; Shalit-Kaneh et al., 2018). The difference is that RVE8 activates the expression of EE-containing clock genes, while CCA1/LHY represses the expression of these genes (Hsu et al., 2013; Shalit-Kaneh et al., 2018). To test whether increasing RVE8a expression led to increased function of RVE8 in smp2-3, we quantified the expression of RVE8-activated genes in smp2-3. The results showed that consistent with the higher abundance of the functional RVE8a isoform in smp2-3, these evening-phased genes, including PRR5, TOC1, ELF4 and GI, exhibited increased rhythmic amplitudes in smp2-3 than in Col-0 (Figures 4F–I). Taken together, these data suggest that SMP2 is involved in promoting A3'SS in RVE8 intron 7 and disturbing the expression of RVE8-activated genes.
Figure 4. SMP2 regulates A3'SS in RVE8 and inhibits the expression of RVE8-activated genes. (A) Simplified diagram of the diurnal entrainment of seedlings. Plants were entrained in the diurnal cycles (12 h light/12 h dark) for 6 days and then transferred to continuous white light (145 μmol·m−2·s−1). Seedlings were harvested at the indicated time points. (B) The schematic graph of splice variants of RVE8 in intron 7. Red arrows indicated primers used to amplify specific RVE8 transcript isoforms. Exons were depicted as black rectangles; introns were depicted as horizontal lines; alternative splicing regions were depicted as gray rectangles. (C–E) The relative abundance of three RVE8 isoforms in Col-0 and smp2-3. Total RNA was extracted from Col-0 and smp2-3 seedlings treated as described in (A). PP2A was used as the internal control. (F–I) The relative expressions of PRR5, TOC1, ELF4, and GI in Col-0 and smp2-3. Error bars represent SD, n = 3. All of these experiments were performed three times with similar results.
To investigate whether SMP2 indeed affects the output of circadian clock, we also performed qPCR to examine the expression level of PIF4, whose expression exhibited diurnal rhythm with a peak in the subjective afternoon (Figure 5A; Nusinow et al., 2011). However, the peak amplitude of PIF4 expression was depressed in the smp2-3 mutant (Figure 5A), which possibly resulted from the higher abundance of RVE8a transcript in smp2-3 (Gray et al., 2017).
Figure 5. PIF4 contributes to SMP2-mediated hypocotyl elongation. (A) The relative expression of PIF4 in Col-0 and smp2-3. Seedlings were entrained in the diurnal cycles (12 h light/12 h dark) for 6 days and then transferred to continuous white light (145 μmol·m−2·s−1). Seedlings were harvested at the indicated times. The relative expression levels of PIF4 were normalized to PP2A. Error bars represent SD, n = 3. (B) Visual phenotypes of 5-day-old Col-0, smp2-3, PIF4-ox and PIF4-ox smp2-3 seedlings grown in continuous red light condition (145 μmol·m−2·s−1). Scale bars, 5 mm. (C) Quantification of hypocotyl length of Col-0, smp2-3, PIF4-ox and PIF4-ox smp2-3 seedlings grown in continuous red light. Error bars represent SD, n ≥ 20. Letters above the bars indicate significant differences (P < 0.05), as determined by one-way analysis of variance (ANOVA) with Duncan's post-hoc analysis.
To further confirm whether PIF4 was involved in SMP2-mediated hypocotyl elongation, we crossed an over-expression line of PIF4 (PIF4-ox) with smp2-3 and analyzed their phenotypes. The hypocotyls of PIF4-ox seedlings were dramatically longer than those of Col-0 in red light as previously described, indicating the amplification of functional PIF4 proteins in PIF4-ox lines. Furthermore, the hypocotyls of seedlings overexpressing PIF4 in smp2-3 were significantly longer than those of smp2-3, but slightly shorter than those of PIF4-ox in red light (Figures 5B,C; Supplementary Figure 7). Together, these results demonstrate that SMP2-mediated hypocotyl elongation in red light partially depends on functional PIF4.
SMP2 and its paralog SMP1 are conserved in evolution, for example, Slu7 and hSlu7 are the homolog of SMP2 in yeast and human, respectively (Frank and Guthrie, 1992; Chua and Reed, 1999). Slu7/hSlu7 is a second-step splicing factor required for proper selection of the 3' splice site (Frank and Guthrie, 1992; Chua and Reed, 1999). The smp1smp2 double mutant in Arabidopsis is not viable, indicating their indispensable roles in plant development (Clay and Nelson, 2005; Liu et al., 2016). Moreover, the interaction between SMP1/2 and SKIP, a component of the spliceosome in Arabidopsis, is reported to be conserved in yeast and human (Liu et al., 2016). Similar to the splicing defect in skip mutant, knockout of SMP1/2 in Arabidopsis protoplasts leads to significant accumulation of aberrant splicing products of certain genes (Liu et al., 2016), suggesting that SMP1/2 are also involved in pre-mRNA splicing in plants. In this study, we demonstrated that phyB physically and functionally interacted with SMP2 in response to light. SMP2 decreased the abundance of functional RVE8a, likely by regulating the 3'SS determination, resulting in a decrease in evening-phased genes expression and a subsequent promotion of PIF4 expression to fine-tune seedling photomorphogenesis. In addition, smp2 mutants also showed short hypocotyls under far-red and blue light (Figure 2), suggesting that SMP2 might also be involved in the phyA and cryptochromes signaling pathways to regulate photomorphogenesis.
The key clock regulator RVE8 gene produces various mRNA isoforms, and our work revealed that SMP2 might be involved in the 3'SS determination of RVE8 intron 7 (Figure 4). Lack of SMP2 resulted in a decrease in the abundance of RVE8b isoform with a concomitant increase in the abundance of the functional RVE8a isoform (Figures 4C,D). RT-qPCR confirmed that expression levels of RVE8 target genes were increased in smp2-3 mutant, suggesting that the higher expression of RVE8a was responsible for the increased function of RVE8 in smp2-3. However, the mechanism of how the different isoforms of RVE8 affect RVE8 function remains unclear. In general, AS events can lead to the production of premature termination codons (PTCs) in mRNA, which can subsequently be degraded by nonsense-mediated mRNA decay (NMD) or produce truncated proteins with distinct functions (Seo et al., 2012; Reddy et al., 2013; Chaudhary et al., 2019). In addition, some AS transcripts can be sequestered in the nucleus and spliced as needed to respond to varying environmental conditions (Reddy et al., 2013; Petrillo et al., 2014; Filichkin et al., 2015). Therefore, further investigation of the fates of the different RVE8 AS isoforms is required to unravel the biological significance of SMP2-mediated AS.
RVE8 can directly activate the expression of genes containing EE-motif in their promoters (Hsu et al., 2013), which leads to mis-regulation of PIF4 and PIF5 expression and modulation of seedling photomorphogenesis (Gray et al., 2017). In this study, we verified the PIF4 expression is lower in smp2-3 than in Col-0, and overexpression of PIF4 in smp2-3 can restore the short hypocotyl of smp2-3 in the light (Figure 5). These demonstrate that PIF4 contributes to SMP2-mediated hypocotyl elongation in the light. In addition, we also detected the rhythmic PIF5 expression in smp2-3 (Supplementary Figure 8). Similar to the PIF4 expression pattern, PIF5 also showed reduced peak expression in smp2-3, this suggests that PIF5 may also play a role in SMP2-mediated regulation of photomorphogenesis. In this way, the slightly shorter hypocotyl of PIF4-ox smp2-3 compared to PIF4-ox seedlings can be partially explained (Figure 5).
To date, the question of how splicing factors are regulated by light signal remains rather elusive. Previous studies have shown that the transcription level and protein stability of SFPS/RRC1 are not regulated by phyB and light signal (Xin et al., 2017, 2019). However, in moss the splicing regulator PphnRNP-F1 is reported to be stabilized by red light, which depends on PpPHY4 (Lin et al., 2020). In this study, the expression level of SMP2 was also not regulated by red light (Supplementary Figure 9). Whether the protein stability of SMP2 is regulated by light requires further investigation. The genetic interaction of SMP2 and phyB suggests that functional phyB contributes to the short hypocotyl of smp2 mutants (Figure 3). The shorter hypocotyls of smp2-1 phyB-9 and smp2-3 phyB-9 compared with the phyB-9 single mutant suggest that the other phytochromes (Cheng et al., 2021) may also be involved in SMP2-mediated regulation of photomorphogenesis in red light. Given that active phytochromes can induce phosphorylation and degradation of PIFs (Legris et al., 2019; Cheng et al., 2021), it is likely that phyB induces the phosphorylation of SMP2 to fine-tune its activity in response to red light. In addition, these phyB-interacting splicing factors, previously reported SFPS/RRC1 and SMP2 in this study, partially co-localized with phyB in nuclear photobodies in prolonged red light condition (Figure 1E) (Xin et al., 2017, 2019). The size and number of photobodies correlate closely with phyB activity (Klose et al., 2015; Legris et al., 2019; Cheng et al., 2021). And the relative concentration of splicing regulators is crucial for splice site selection during spliceosome assembly (Shomron et al., 2005; Saltzman et al., 2011; Kornblihtt et al., 2013). These facts provide another hypothesis that activated phyB may regulate the subnuclear localization of SMP2 and modulate its functions in alternative pre-mRNA splicing of certain light- and clock-associated genes.
The original contributions presented in the study are included in the article/Supplementary Material, further inquiries can be directed to the corresponding authors.
TY, JL, and XD designed the research and wrote the paper. TY and WW performed the experiments. TY, YH, JL, and XD analyzed the data. All authors contributed to the article and approved the submitted version.
This work was funded by Southern University of Science and Technology (Y01226026), Key Laboratory of Molecular Design for Plant Cell Factory of Guangdong Higher Education Institute (2019KSYS006), the National Key R&D Program of China (grant 2017YFA0503800), and the National Natural Science Foundation of China (31621001).
The authors declare that the research was conducted in the absence of any commercial or financial relationships that could be construed as a potential conflict of interest.
All claims expressed in this article are solely those of the authors and do not necessarily represent those of their affiliated organizations, or those of the publisher, the editors and the reviewers. Any product that may be evaluated in this article, or claim that may be made by its manufacturer, is not guaranteed or endorsed by the publisher.
We thank Joanne Chory for providing phyB-9 and phyB-CFP seeds, and Enamul Huq for providing PIF4-ox seeds. We thank Jie Dong (Zhejiang University) for his valuable suggestions on the manuscript.
The Supplementary Material for this article can be found online at: https://www.frontiersin.org/articles/10.3389/fpls.2022.836519/full#supplementary-material
Al-Sady, B., Ni, W., Kircher, S., Schäfer, E., and Quail, P. H. (2006). Photoactivated phytochrome induces rapid PIF3 phosphorylation prior to proteasome-mediated degradation. Mol. Cell 23, 439–446. doi: 10.1016/j.molcel.2006.06.011
Burgie, E. S., Bussell, A. N., Walker, J. M., Dubiel, K., and Vierstra, R. D. (2014). Crystal structure of the photosensing module from a red/far-red light-absorbing plant phytochrome. Proc. Natl. Acad. Sci. USA 111, 10179–10184. doi: 10.1073/pnas.1403096111
Burgie, E. S., and Vierstra, R. D. (2014). Phytochromes: an atomic perspective on photoactivation and signaling. Plant Cell 26, 4568–4583. doi: 10.1105/tpc.114.131623
Chaudhary, S., Jabre, I., Reddy, A. S. N., Staiger, D., and Syed, N. H. (2019). Perspective on alternative splicing and proteome complexity in plants. Trends Plant Sci. 24, 496–506. doi: 10.1016/j.tplants.2019.02.006
Chen, H., Zou, Y., Shang, Y., Lin, H., Wang, Y., Cai, R., et al. (2008). Firefly luciferase complementation imaging assay for protein-protein interactions in plants. Plant Physiol. 146, 368–376. doi: 10.1104/pp.107.111740
Chen, M., and Chory, J. (2011). Phytochrome signaling mechanisms and the control of plant development. Trends Cell Biol. 21, 664–671. doi: 10.1016/j.tcb.2011.07.002
Chen, M., Tao, Y., Lim, J., Shaw, A., and Chory, J. (2005). Regulation of phytochrome B nuclear localization through light-dependent unmasking of nuclear-localization signals. Curr. Biol. 15, 637–642. doi: 10.1016/j.cub.2005.02.028
Cheng, M.-C., Kathare, P. K., Paik, I., and Huq, E. (2021). Phytochrome signaling networks. Annu. Rev. Plant Biol. 72, 217–244. doi: 10.1146/annurev-arplant-080620-024221
Chua, K., and Reed, R. (1999). The RNA splicing factor hSlu7 is required for correct 3′ splice-site choice. Nature 402, 207–210. doi: 10.1038/46086
Clay, N. K., and Nelson, T. (2005). The recessive epigenetic swellmap mutation affects the expression of two step II splicing factors required for the transcription of the cell proliferation gene STRUWWELPETER and for the timing of cell cycle arrest in the Arabidopsis Leaf. Plant Cell 17, 1994–2008. doi: 10.1105/tpc.105.032771
Clough, S. J., and Bent, A. F. (1998). Floral dip: a simplified method for Agrobacterium-mediated transformation of Arabidopsis thaliana. Plant J. 16, 735–743. doi: 10.1046/j.1365-313x.1998.00343.x
Dong, J., Chen, H., Deng, X. W., Irish, V. F., and Wei, N. (2020). Phytochrome B induces intron retention and translational inhibition of PHYTOCHROME-INTERACTING FACTOR3. Plant Physiol. 182, 159–166. doi: 10.1104/pp.19.00835
Farinas, B., and Mas, P. (2011). Functional implication of the MYB transcription factor RVE8/LCL5 in the circadian control of histone acetylation. Plant J. 66, 318–329. doi: 10.1111/j.1365-313X.2011.04484.x
Filichkin, S. A., Cumbie, J. S., Dharmawardhana, P., Jaiswal, P., Chang, J. H., Palusa, S. G., et al. (2015). Environmental stresses modulate abundance and timing of alternatively spliced circadian transcripts in Arabidopsis. Mol. Plant 8, 207–227. doi: 10.1016/j.molp.2014.10.011
Frank, D., and Guthrie, C. (1992). An essential splicing factor, SLU7, mediates 3' splice site choice in yeast. Genes Dev. 6, 2112–2124. doi: 10.1101/gad.6.11.2112
Galvão, V. C., and Fankhauser, C. (2015). Sensing the light environment in plants: photoreceptors and early signaling steps. Curr. Opin. Neurobiol. 34, 46–53. doi: 10.1016/j.conb.2015.01.013
Godoy Herz, M. A., Kubaczka, M. G., Brzyzek, G., Servi, L., Krzyszton, M., Simpson, C., et al. (2019). Light regulates plant alternative splicing through the control of transcriptional elongation. Mol. Cell 73, 1066–1074.e3. doi: 10.1016/j.molcel.2018.12.005
Gray, J. A., Shalit-Kaneh, A., Chu, D. N., Hsu, P. Y., and Harmer, S. L. (2017). The REVEILLE clock genes inhibit growth of juvenile and adult plants by control of cell size. Plant Physiol. 173, 2308–2322. doi: 10.1104/pp.17.00109
Hartmann, L., Drewe-Bo,ß, P., Wießner, T., Wagner, G., Geue, S., Lee, H.-C., et al. (2016). Alternative splicing substantially diversifies the transcriptome during early photomorphogenesis and correlates with the energy availability in Arabidopsis. Plant Cell 28, 2715–2734. doi: 10.1105/tpc.16.00508
Hsu, P. Y., Devisetty, U. K., and Harmer, S. L. (2013). Accurate timekeeping is controlled by a cycling activator in Arabidopsis. eLife 2, e00473. doi: 10.7554/eLife.00473.018
Huq, E., Al-Sady, B., and Quail, P. H. (2003). Nuclear translocation of the photoreceptor phytochrome B is necessary for its biological function in seedling photomorphogenesis. Plant J. 35, 660–664. doi: 10.1046/j.1365-313X.2003.01836.x
James, A. B., Syed, N. H., Brown, J. W. S., and Nimmo, H. G. (2012). Thermoplasticity in the plant circadian clock: How plants tell the time-perature. Plant Signal. Behav. 7, 1219–1223. doi: 10.4161/psb.21491
Kathare, P. K., and Huq, E. (2021). Light-regulated pre-mRNA splicing in plants. Curr. Opin. Plant Biol. 63, 102037. doi: 10.1016/j.pbi.2021.102037
Klose, C., Viczián, A., Kircher, S., Schäfer, E., and Nagy, F. (2015). Molecular mechanisms for mediating light-dependent nucleo/cytoplasmic partitioning of phytochrome photoreceptors. New Phytol. 206, 965–971. doi: 10.1111/nph.13207
Kornblihtt, A. R., Schor, I. E., Alló, M., Dujardin, G., Petrillo, E., and Muñoz, M. J. (2013). Alternative splicing: a pivotal step between eukaryotic transcription and translation. Nat. Rev. Mol. Cell Biol. 14, 153–165. doi: 10.1038/nrm3525
Lee, S., Paik, I., and Huq, E. (2020). SPAs promote thermomorphogenesis by regulating the phyB-PIF4 module in Arabidopsis. Development 147, dev189233. doi: 10.1242/dev.189233
Lee, Y., and Rio, D. C. (2015). Mechanisms and regulation of alternative pre-mRNA splicing. Annu. Rev. Biochem. 84, 291–323. doi: 10.1146/annurev-biochem-060614-034316
Legris, M., Ince, Y. Ç., and Fankhauser, C. (2019). Molecular mechanisms underlying phytochrome-controlled morphogenesis in plants. Nat. Commun. 10, 5219. doi: 10.1038/s41467-019-13045-0
Lin, B.-Y., Shih, C.-J., Hsieh, H.-Y., Chen, H.-C., and Tu, S.-L. (2020). Phytochrome coordinates with a hnRNP to regulate alternative splicing via an exonic splicing silencer. Plant Physiol. 182, 243–254. doi: 10.1104/pp.19.00289
Liu, L., Wu, F., and Zhang, W. (2016). A conserved interaction between SKIP and SMP1/2 aids in recruiting the second-step splicing factors to the spliceosome in Arabidopsis. Mol. Plant 9, 1660–1663. doi: 10.1016/j.molp.2016.09.007
Lorković, Z. J., Kirk, D. A. W., Lambermon, M. H. L., and Filipowicz, W. (2000). Pre-mRNA splicing in higher plants. Trends Plant Sci. 5, 160–167. doi: 10.1016/S1360-1385(00)01595-8
Mancini, E., Sanchez, S. E., Romanowski, A., Schlaen, R. G., Sanchez-Lamas, M., Cerdán, P. D., et al. (2016). Acute effects of light on alternative splicing in light-grown plants. Photochem. Photobiol. 92, 126–133. doi: 10.1111/php.12550
Marquez, Y., Brown, J. W. S., Simpson, C., Barta, A., and Kalyna, M. (2012). Transcriptome survey reveals increased complexity of the alternative splicing landscape in Arabidopsis. Genome Res. 22, 1184–1195. doi: 10.1101/gr.134106.111
Nusinow, D. A., Helfer, A., Hamilton, E. E., King, J. J., Imaizumi, T., Schultz, T. F., et al. (2011). The ELF4–ELF3–LUX complex links the circadian clock to diurnal control of hypocotyl growth. Nature 475, 398–402. doi: 10.1038/nature10182
Paik, I., and Huq, E. (2019). Plant photoreceptors: Multi-functional sensory proteins and their signaling networks. Semin. Cell Dev. Biol. 92, 114–121. doi: 10.1016/j.semcdb.2019.03.007
Petrillo, E., Herz, M. A. G., Fuchs, A., Reifer, D., Fuller, J., Yanovsky, M. J., et al. (2014). A chloroplast retrograde signal regulates nuclear alternative splicing. Science 344, 427–430. doi: 10.1126/science.1250322
Rawat, R., Takahashi, N., Hsu, P. Y., Jones, M. A., Schwartz, J., Salemi, M. R., et al. (2011). REVEILLE8 and PSEUDO-REPONSE REGULATOR5 form a negative feedback loop within the Arabidopsis circadian clock. PLoS Genet. 7, e1001350. doi: 10.1371/journal.pgen.1001350
Reddy, A. S. N., Marquez, Y., Kalyna, M., and Barta, A. (2013). Complexity of the alternative splicing landscape in plants. Plant Cell 25, 3657–3683. doi: 10.1105/tpc.113.117523
Reed, J. W., Nagpal, P., Poole, D. S., Furuya, M., and Chory, J. (1993). Mutations in the gene for the red/far-red light receptor phytochrome B alter cell elongation and physiological responses throughout Arabidopsis development. Plant Cell 5, 147–157. doi: 10.1105/tpc.5.2.147
Riegler, S., Servi, L., Scarpin, M. R., Herz, M. A. G., Kubaczka, M. G., Venhuizen, P., et al. (2021). Light regulates alternative splicing outcomes via the TOR kinase pathway. Cell Rep. 3:109676. doi: 10.1016/j.celrep.2021.109676
Saltzman, A. L., Pan, Q., and Blencowe, B. J. (2011). Regulation of alternative splicing by the core spliceosomal machinery. Genes Dev. 25, 373–384. doi: 10.1101/gad.2004811
Seo, P. J., Park, M.-J., Lim, M.-H., Kim, S.-G., Lee, M., Baldwin, I. T., et al. (2012). A self-regulatory circuit of CIRCADIAN CLOCK-ASSOCIATED1 underlies the circadian clock regulation of temperature responses in Arabidopsis. Plant Cell 24, 2427–2442. doi: 10.1105/tpc.112.098723
Shalit-Kaneh, A., Kumimoto, R. W., Filkov, V., and Harmer, S. L. (2018). Multiple feedback loops of the Arabidopsis circadian clock provide rhythmic robustness across environmental conditions. Proc. Natl. Acad. Sci. USA 115, 7147–7152. doi: 10.1073/pnas.1805524115
Shen, H., Zhu, L., Castillon, A., Majee, M., Downie, B., and Huq, E. (2008). Light-induced phosphorylation and degradation of the negative regulator PHYTOCHROME-INTERACTING FACTOR1 from Arabidopsis depend upon its direct physical interactions with photoactivated phytochromes. Plant Cell 20, 1586–1602. doi: 10.1105/tpc.108.060020
Shi, Y.. (2017). Mechanistic insights into precursor messenger RNA splicing by the spliceosome. Nat. Rev. Mol. Cell Biol. 18, 655–670. doi: 10.1038/nrm.2017.86
Shih, C.-J., Chen, H.-W., Hsieh, H.-Y., Lai, Y.-H., Chiu, F.-Y., Chen, Y.-R., et al. (2019). Heterogeneous nuclear ribonucleoprotein H1 coordinates with phytochrome and the U1 snRNP complex to regulate alternative splicing in Physcomitrella patens. Plant Cell 31, 2510–2524. doi: 10.1105/tpc.19.00314
Shikata, H., Hanada, K., Ushijima, T., Nakashima, M., Suzuki, Y., and Matsushita, T. (2014). Phytochrome controls alternative splicing to mediate light responses in Arabidopsis. Proc. Natl. Acad. Sci. USA 111, 18781–18786. doi: 10.1073/pnas.1407147112
Shikata, H., Shibata, M., Ushijima, T., Nakashima, M., Kong, S.-G., Matsuoka, K., et al. (2012). The RS domain of Arabidopsis splicing factor RRC1 is required for phytochrome B signal transduction. Plant J. 70, 727–738. doi: 10.1111/j.1365-313X.2012.04937.x
Shomron, N., Alberstein, M., Reznik, M., and Ast, G. (2005). Stress alters the subcellular distribution of hSlu7 and thus modulates alternative splicing. J. Cell. Sci. 118, 1151–1159. doi: 10.1242/jcs.01720
Wahl, M. C., Will, C. L., and Lührmann, R. (2009). The spliceosome: Design principles of a dynamic RNP machine. Cell 136, 701–718. doi: 10.1016/j.cell.2009.02.009
Wang, Z.-P., Xing, H.-L., Dong, L., Zhang, H.-Y., Han, C.-Y., Wang, X.-C., et al. (2015). Egg cell-specific promoter-controlled CRISPR/Cas9 efficiently generates homozygous mutants for multiple target genes in Arabidopsis in a single generation. Genome Biol. 16, 144. doi: 10.1186/s13059-015-0715-0
Wu, H.-P., Su, Y., Chen, H.-C., Chen, Y.-R., Wu, C.-C., Lin, W.-D., et al. (2014). Genome-wide analysis of light-regulated alternative splicing mediated by photoreceptors in Physcomitrella patens. Genome Biol. 15, R10. doi: 10.1186/gb-2014-15-1-r10
Xin, R., Kathare, P. K., and Huq, E. (2019). Coordinated regulation of pre-mRNA splicing by the SFPS-RRC1 complex to promote photomorphogenesis. Plant Cell 31, 2052–2069. doi: 10.1105/tpc.18.00786
Keywords: light signaling, photomorphogenesis, phytochrome B, alternative pre-mRNA splicing, splicing factor
Citation: Yan T, Heng Y, Wang W, Li J and Deng XW (2022) SWELLMAP 2, a phyB-Interacting Splicing Factor, Negatively Regulates Seedling Photomorphogenesis in Arabidopsis. Front. Plant Sci. 13:836519. doi: 10.3389/fpls.2022.836519
Received: 15 December 2021; Accepted: 17 January 2022;
Published: 10 February 2022.
Edited by:
Enamul Huq, University of Texas at Austin, United StatesReviewed by:
Marcelo Javier Yanovsky, Consejo Nacional de Investigaciones Científicas y Técnicas (CONICET), ArgentinaCopyright © 2022 Yan, Heng, Wang, Li and Deng. This is an open-access article distributed under the terms of the Creative Commons Attribution License (CC BY). The use, distribution or reproduction in other forums is permitted, provided the original author(s) and the copyright owner(s) are credited and that the original publication in this journal is cited, in accordance with accepted academic practice. No use, distribution or reproduction is permitted which does not comply with these terms.
*Correspondence: Jian Li, bGlqOEBzdXN0ZWNoLmVkdS5jbg==; Xing Wang Deng, ZGVuZ0Bwa3UuZWR1LmNu
Disclaimer: All claims expressed in this article are solely those of the authors and do not necessarily represent those of their affiliated organizations, or those of the publisher, the editors and the reviewers. Any product that may be evaluated in this article or claim that may be made by its manufacturer is not guaranteed or endorsed by the publisher.
Research integrity at Frontiers
Learn more about the work of our research integrity team to safeguard the quality of each article we publish.