- 1Kunming Institute of Botany, Chinese Academy of Sciences, Kunming, China
- 2University of Chinese Academy of Sciences, Beijing, China
- 3Institute of Environment and Sustainable Development in Agriculture, Chinese Academy of Agriculture Sciences, Beijing, China
The response of photosynthetic CO2 assimilation to changes of illumination affects plant growth and crop productivity under natural fluctuating light conditions. However, the effects of nitrogen (N) supply on photosynthetic physiology after transition from low to high light are seldom studied. To elucidate this, we measured gas exchange and chlorophyll fluorescence under fluctuating light in tomato (Solanum lycopersicum) seedlings grown with different N conditions. After transition from low to high light, the induction speeds of net CO2 assimilation (AN), stomatal conductance (gs), and mesophyll conductance (gm) delayed with the decline in leaf N content. The time to reach 90% of maximum AN, gs and gm was negatively correlated with leaf N content. This delayed photosynthetic induction in plants grown under low N concentration was mainly caused by the slow induction response of gm rather than that of gs. Furthermore, the photosynthetic induction upon transfer from low to high light was hardly limited by photosynthetic electron flow. These results indicate that decreased leaf N content declines carbon gain under fluctuating light in tomato. Increasing the induction kinetics of gm has the potential to enhance the carbon gain of field crops grown in infertile soil.
Introduction
Plants capture light energy to produce chemical energy ATP and NADPH, which are used to drive nitrogen assimilation and the conversion of CO2 to sugar. Enhancing net CO2 assimilation rate (AN) is thought to be one of the most important targets for improving plant growth and crop productivity (Kromdijk et al., 2016; Yamori et al., 2016a; South et al., 2019; Ferroni et al., 2020). Many previous studies indicated that increasing AN under constant high light can boost plant biomass (Kebeish et al., 2007; Timm et al., 2012, 2015). Recently, some studies reported that the response of AN to the increases of illumination significantly affects the carbon gain and thus influences plant growth (Slattery et al., 2018; Adachi et al., 2019; Kimura et al., 2020; Yamori et al., 2020; Zhang et al., 2020). Therefore, altering the photosynthetic performance under dynamic illumination is a promising way to improve photosynthesis under natural fluctuating light (FL) conditions.
Plants grown under high nitrogen (N) concentration usually have higher biomass than plants grown under low N concertation (Makino, 2011). An important explanation for this is that leaf photosynthetic capacity is related to the leaf N content in many higher plants (Yamori et al., 2011; Fan et al., 2020; Li et al., 2020), since stromal enzymes and thylakoid proteins account for the majority of leaf N (Makino and Osmond, 1991; Sudo et al., 2003; Takashima et al., 2004). Furthermore, stomatal conductance (gs) and mesophyll conductance (gm) under constant high light are also increased in plants grown under high N concentration, which speeds up CO2 diffusion from atmosphere to chloroplast carboxylation sites and thus favors the operation of AN under constant high light (Yamori et al., 2011). However, few is known about the effects of leaf N content on non-steady-state photosynthetic performances under FL.
Under natural field conditions, light intensity exposed on leaf surface dynamically changes on timescales from milliseconds to hours (Pearcy, 1990; Slattery et al., 2018). Furthermore, FL and N deficiency usually occurs concomitantly, but how FL and N deficiency interacts to influence photosynthetic physiology in crop plants is poorly understood. After a sudden transitioning from low to high light, the gradual increase of AN is termed “photosynthetic induction.” Recent studies indicated that the induction response of AN was significantly affected by the induction speed of gs (De Souza et al., 2020; Kimura et al., 2020). Gene expression plays a crucial role in the induction response of gs under FL. For example, the slow anion channel-associated 1 (slac1), open stomata 1 (ost1) and abscisic acid-deficient flacca mutants, and the proton ATPase translocation control 1 (PATROL1) overexpression line had faster stomatal opening responses than WT types in Arabidopsis thaliana, rice and tomato (De Souza et al., 2020; Kaiser et al., 2020; Kimura et al., 2020; Yamori et al., 2020). Furthermore, the stomatal opening during photosynthetic induction can be affected by environment conditions such as drought, target light intensity, magnitude of change, gs at low light, the time of day, and vapor pressure deficit (Zivcak et al., 2013; Kaiser et al., 2020; Sakoda et al., 2020; Eyland et al., 2021). However, there have been few studies that examined the effect of leaf N content on the induction response of gs after transition from low to high light (Li et al., 2020).
In addition to gs, gm is a major factor that affects CO2 concentration in chloroplast, because gm determines the CO2 diffusion from intercellular space into the chloroplast (Flexas et al., 2013; Carriquí et al., 2015). In general, gm can be determined by structure across leaf profiles, genetic types, biochemical components, and environmental conditions (Yamori et al., 2011; Xiong et al., 2015; Théroux-Rancourt and Gilbert, 2017; Ferroni et al., 2021). Previous studies have highlighted that gm is the most important limiting factor for AN in many angiosperms (Peguero-Pina et al., 2017; Xiong et al., 2018; Yang Z.-H. et al., 2018, Yang et al., 2021; Gago et al., 2020). Short-term response of gm to light intensity has been determined and found that it varies between plant species (Tazoe et al., 2009; Yamori et al., 2010a; Xiong et al., 2018; Yang et al., 2020). However, the induction response of gm after transition from low to high light is less known. The gm level under constant light is also significantly affected by leaf N content (Yamori et al., 2011). Furthermore, the rapid responses of gm to CO2 concentration and temperature were also affected by leaf N content (Xiong et al., 2015). However, no studies have elucidated the effect of leaf N content on induction response of gm upon transfer from low to high light.
In this study, we aimed to characterize the effects of leaf N content on induction kinetics of AN, gs, and gm after a sudden transition from low to high light. Gas exchange and chlorophyll fluorescence were measured in tomato plants grown under contrasting N concentrations. The dynamic limitations of gs, gm, and biochemical factors imposed on AN were analyzed based on the biochemical model for C3 photosynthesis (Farquhar et al., 1980). The effects of leaf N content on photosynthetic performances during photosynthetic induction were revealed.
Materials and Methods
Plant Materials and Growth Conditions
Tomato (Solanum lycopersicum cv. Hupishizi) plants were grown in a greenhouse with the light condition of 40% full sunlight. The day or night air temperatures were approximately 30 or 20°C, the relative air humidity was approximately 60–70%, and the maximum light intensity exposed to leaves was approximately 800 μmol photons m–2 s–1. Plants were grown in 19-cm plastic pots with humus soil, and the initial soil N content was 2.1 mg/g. Plants were fertilized with Peters professional water solution (N:P:K = 15:4.8:24.1, quality ratio) or water as follows: high nitrogen (HN, 0.15 g N/plant every 2 days), middle nitrogen (MN, 0.05 g N/plant once a week), and low nitrogen (LN, 0 mM N/plant). The fertilizer was dissolved in 0.3% water solution and subsequently was used for fertilization, and the nitrogen sources were 24% (NH4)3PO4, 65% KNO3, and 9.5% CH4N2O. To prevent any water stress, these plants were watered every day. After cultivation for 1 month, youngest fully developed leaves were used for measurements. For each N treatment, five leaves form five independent plants were used for gas exchange and chlorophyll fluorescence measurements.
Gas Exchange and Chlorophyll Fluorescence Measurements
An open gas exchange system (LI-6400XT; Li-Cor Biosciences, Lincoln, NE, United States) was used to simultaneously measure gas exchange and chlorophyll fluorescence. Measurements were taken at a leaf temperature of approximately 25°C, leaf-to-air vapor pressure deficit of 1.2–1.4 kpa, and flow rate of air through the system of 300 mmol min–1. To measure photosynthetic induction after a short-term shadefleck, leaves were first adapted to a light intensity of 1,500 μmol photons m–2 s–1 and air CO2 concentration of 400 μmol mol–1 for > 20 min until AN and gs reached steady state. Then, leaves were subjected to 5 min of low light (50 μmol photons m–2 s–1) followed by 30 min of high light (1,500 μmol photons m–2 s–1), and gas exchange and chlorophyll fluorescence were logged every minute. iWUE was calculated as iWUE = AN/gs. The relative AN, gs, and gm curves were obtained from the standardization against the maximum values after 30 min photosynthetic induction at high light. The time required to reach 90% of the maximum AN, gs, and gm was estimated by the first time at which the relative values were higher than 90%. After photosynthetic induction measurement, the response of CO2 assimilation rate to incident intercellular CO2 concentration (A/Ci) curves was measured by decreasing the CO2 concentration to a lower limit of 50 μmol mol–1 and then increasing stepwise to an upper limit of 1,500 μmol mol–1. For each CO2 concentration, photosynthetic measurement was completed in 3 min. Using the A/Ci curves, the maximum rates of RuBP regeneration (Jmax) and carboxylation (Vcmax) were calculated (Long and Bernacchi, 2003).
The quantum yield of PSII photochemistry was calculated as ΦPSII = (Fm′-Fs)/Fm′ (Genty et al., 1989), where Fm′ and Fs represent the maximum and steady-state fluorescence after light adaptation, respectively (Baker, 2004). The total electron transport rate (ETR) through PSII (JPSII) was calculated as follows (Krall and Edwards, 1992):
where PPFD is the photosynthetic photon flux density, and leaf absorbance (Labs) is assumed to be 0.84. We applied the constant of 0.5 based on the assumption that photons were equally distributed between PSI and PSII.
Estimation of Mesophyll Conductance and Chloroplast CO2 Concentration
Mesophyll conductance was calculated according to the following equation (Harley et al., 1992):
where AN represents the net rate of CO2 assimilation; Ci is the intercellular CO2 concentration; Γ* is the CO2 compensation point in the absence of daytime respiration (Yamori et al., 2010b; von Caemmerer and Evans, 2015). We used a typical value of 40 μmol mol–1 in our current study (Xiong et al., 2018). Respiration rate in the dark (Rd) was considered to be half of the dark-adapted mitochondrial respiration rate as measured after 10 min of dark adaptation (Carriquí et al., 2015).
Based on the estimated gm, the chloroplast CO2 concentration (Cc) was calculated according to the following equation (Long and Bernacchi, 2003; Warren and Dreyer, 2006):
Quantitative Limitation Analysis of AN
Relative photosynthetic limitations were assessed as follows (Grassi and Magnani, 2005):
where Ls, Lmc, and Lb represent the relative limitations of stomatal conductance, mesophyll conductance, and biochemical capacity, respectively, in setting the observed value of AN. gtot is the total conductance of CO2 between the leaf surface and sites of RuBP carboxylation (calculated as 1/gtot = 1/gs + 1/gm).
SPAD Index and Nitrogen Content Measurements
A handy chlorophyll meter (SPAD-502 Plus; Minolta, Tokyo, Japan) was used to nondestructively measure the SPAD index (relative content of chlorophyll per unit leaf area) of leaves used for photosynthetic measurements. Thereafter, leaf area was measured using a LI-3000A portable leaf area meter (Li-Cor, Lincoln, NE, United States). After leaf material was dried at 80°C for 48 h, dry weight was measured and leaf N content was determined with a Vario MICRO Cube Elemental Analyzer (Elementar Analysensysteme GmbH, Langenselbold, Germany) (Sakowska et al., 2018).
Statistical Analysis
For each N treatment, five leaves form five independent plants were used for gas exchange and chlorophyll fluorescence measurements. One-way ANOVA and t-tests were used to determine whether significant differences existed between different treatments (α = 0.05). The software SigmaPlot 10.0 was used for graphing and fitting.
Results
Effect of Leaf N Content on Steady-State Physiological Characteristics Under High Light
The leaf N content in LN-, MN-, and HN-plants was 0.42 ± 0.03, 0.71 ± 0.3, and 1.2 ± 0.07 g m–2, respectively (Table 1). The HN-plants displayed the highest relative chlorophyll content, measured by SPAD value, followed by MN- and LN-plants. After 30 min light adaptation at 1,500 μmol photons m–2 s–1 and 400 μmol mol–1 CO2 concentration, HN-plants had the highest net CO2 assimilation rate (AN), stomatal conductance (gs), mesophyll conductance (gm), and ETR. Therefore, the steady-state photosynthetic capacities were significantly affected by leaf N content. Furthermore, HN-, MN-, and LN-plants showed slight difference in gs but significant difference in gm, which indicates that gm is more responsive to leaf N content than gs in tomato.
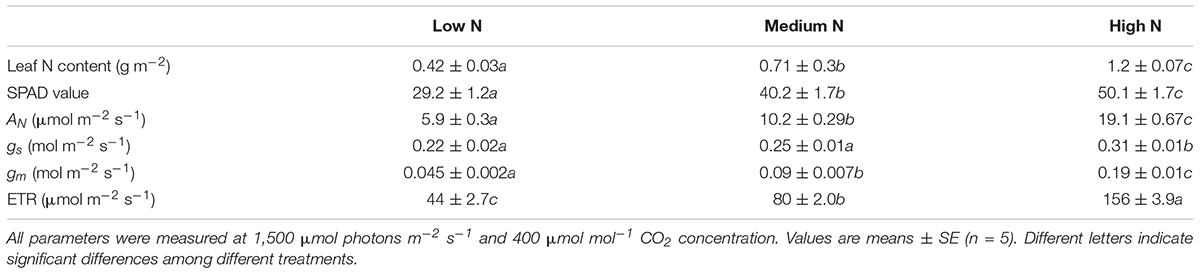
Table 1. Physiological characteristics of leaves from plants grown under three different nutrient concentrations (low, medium and high nitrogen).
Effects of Leaf N Content on Photosynthetic Induction Upon Transfer From Low to High Light
During this photosynthetic induction after 5 min of shadefleck, HN-plants showed the highest induction speeds of AN, gs, and gm, followed by MN- and LN-plants (Figure 1). The time required to reach 90% of the maximum AN (t90AN) significantly increased with the decrease in leaf N content (Figure 1G). The time required to reach 90% of the maximum gs and gm (t90gs and t90gm, respectively) was significantly shorter in HN-plants than MN- and LN-plants, whereas t90gs and t90gm did not differ significantly between MN- and LN-plants (Figure 1G). Interestingly, t90gm was lower than t90gs in all plants. The higher t90gs and t90AN in MN- and LN-plants were
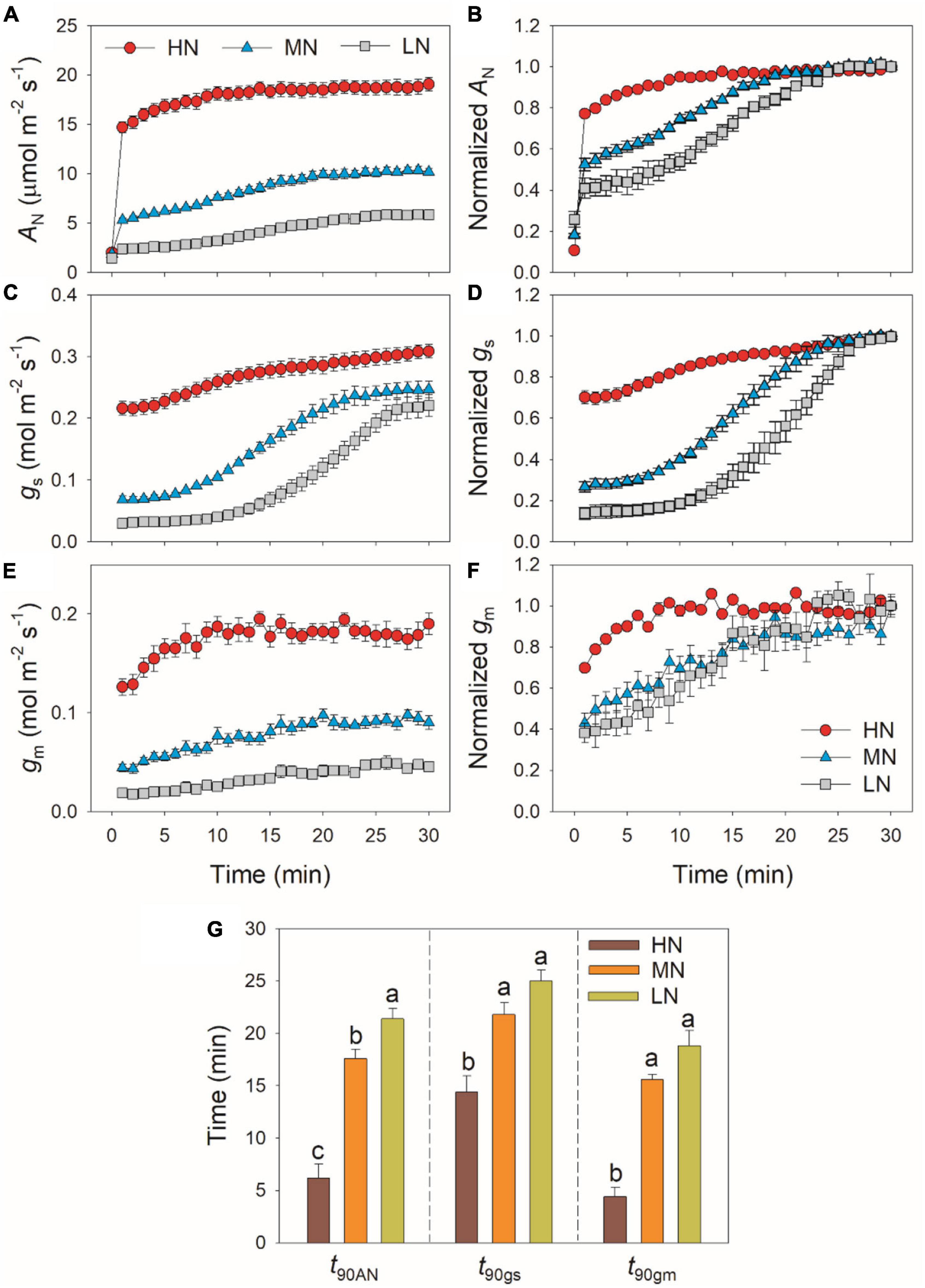
Figure 1. Induction response of net CO2 assimilation rate (AN) (A,B), stomatal conductance (gs) (C,D) and mesophyll conductance (gm) (E,F), and the time required to reach 90% of the maximum values of AN, gs and gm (t90AN, t90gs, t90gm) (G) after transition from 50 to 1,500 μmol photons m–2 s–1. AN, gs, and gm were measured every 1 min. Values are means ± SE (n = 5). Different letters indicate significant differences among different treatments. The relative AN, gs, and gm curves were obtained from the standardization against the maximum values after 30 min photosynthetic induction at high light. HN, MN, and LN represent tomato plants grown under high, medium, and low N concentrations, respectively.
partially related to the relatively lower initial gs prior to light change (Supplementary Figure 1). Within the first 15 min after transition from low to high light, all plants showed similar intrinsic water use efficiency (iWUE) (Supplementary Figure 2). However, during prolonged photosynthetic induction, HN-plants displayed much higher iWUE than MN- and LN-plants (Supplementary Figure 2). Further analysis found that leaf N content was negatively correlated with t90AN, t90gs, and t90gm (Figure 2). Therefore, leaf N content plays a crucial role in affecting the induction responses of AN, gs, and gm after transition from low to high light. The comparative extent of the reductions of t90AN was more correlated to t90gm than t90gs (Figure 3A). Furthermore, the change in AN during photosynthetic induction was more related to gm than gs (Figures 3B,C). These results suggest that, upon transfer from low to high light, gm plays a more important role in determining the induction response of AN than gs.
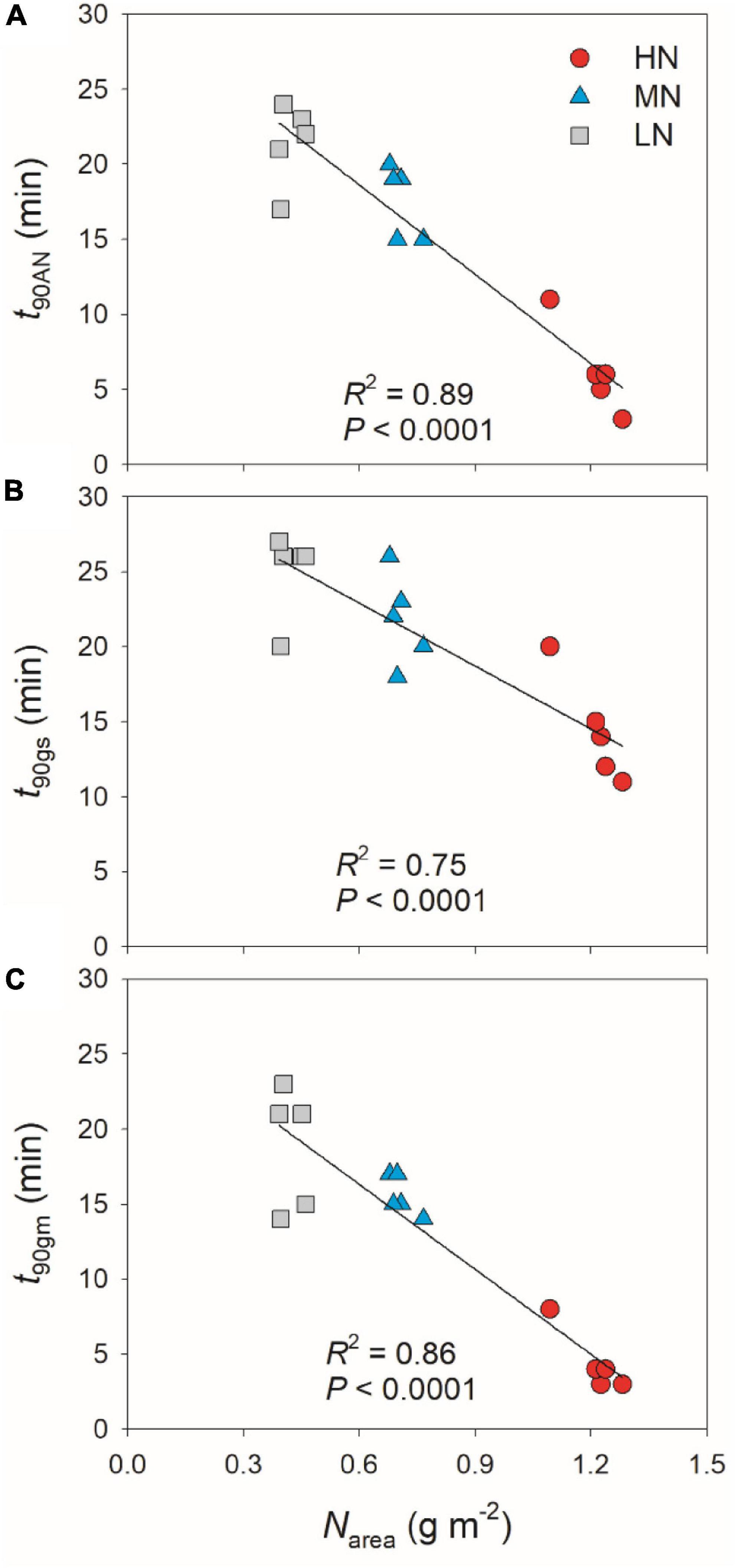
Figure 2. Effects of leaf N content on the time required to reach 90% of the maximum values of AN (A), gs (B), and gm (C) (t90AN, t90gs, t90gm) after transition from 50 to 1,500 μmol photons m–2 s–1. HN, MN, and LN represent tomato plants grown under high, medium, and low N concentrations, respectively.
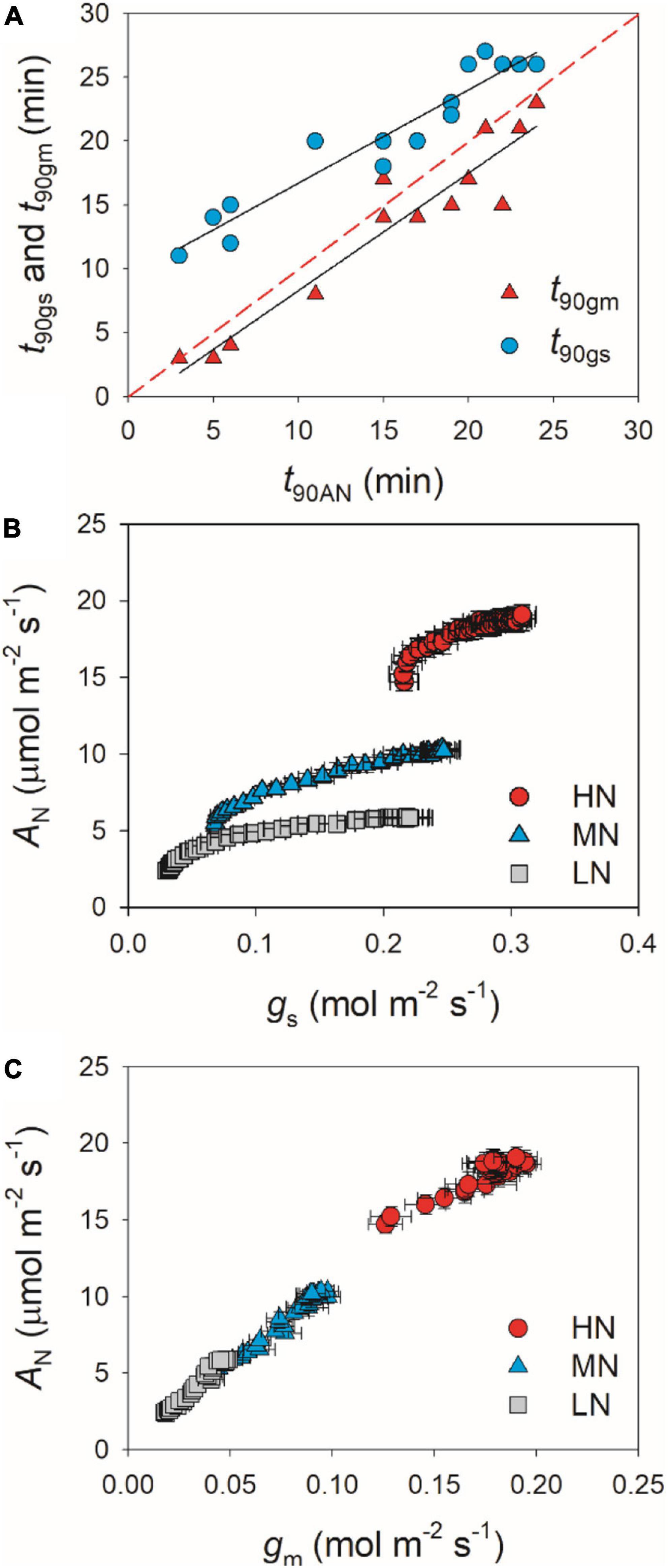
Figure 3. (A) Relationships between t90AN, t90gs, and t90gm after transition from 50 to 1,500 μmol photons m–2 s–1. (B,C) Relationships between gs, gm, and AN after transition from 50 to 1,500 μmol photons m–2 s–1. Values are means ± SE (n = 5). HN, MN, and LN represent tomato plants grown under high, medium, and low N concentrations, respectively.
Effects of Leaf N Content on Intercellular and Chloroplast CO2 Concentrations Upon Transfer From Low to High Light
We calculated the response kinetics of intercellular (Ci) and chloroplast CO2 concentration (Cc) using AN, gs, and gm. After transitioning from low to high light, Ci and Cc gradually increased in all plants (Figure 4). HN-plants had the lowest values of Ci and Cc after photosynthetic sufficient photosynthetic induction. The change in AN during photosynthetic induction was tightly and positively correlated with Cc in all plants, which suggests the importance of Cc in determining AN. Because Cc can be affected by gs and gm, we analyzed the relationships between Cc, gs, and gm. Compared with gs, a smaller change in gm could result in a larger change in Cc (Figure 5), which suggests that the change of Cc upon transfer from low to high light was more determined by gm than gs.
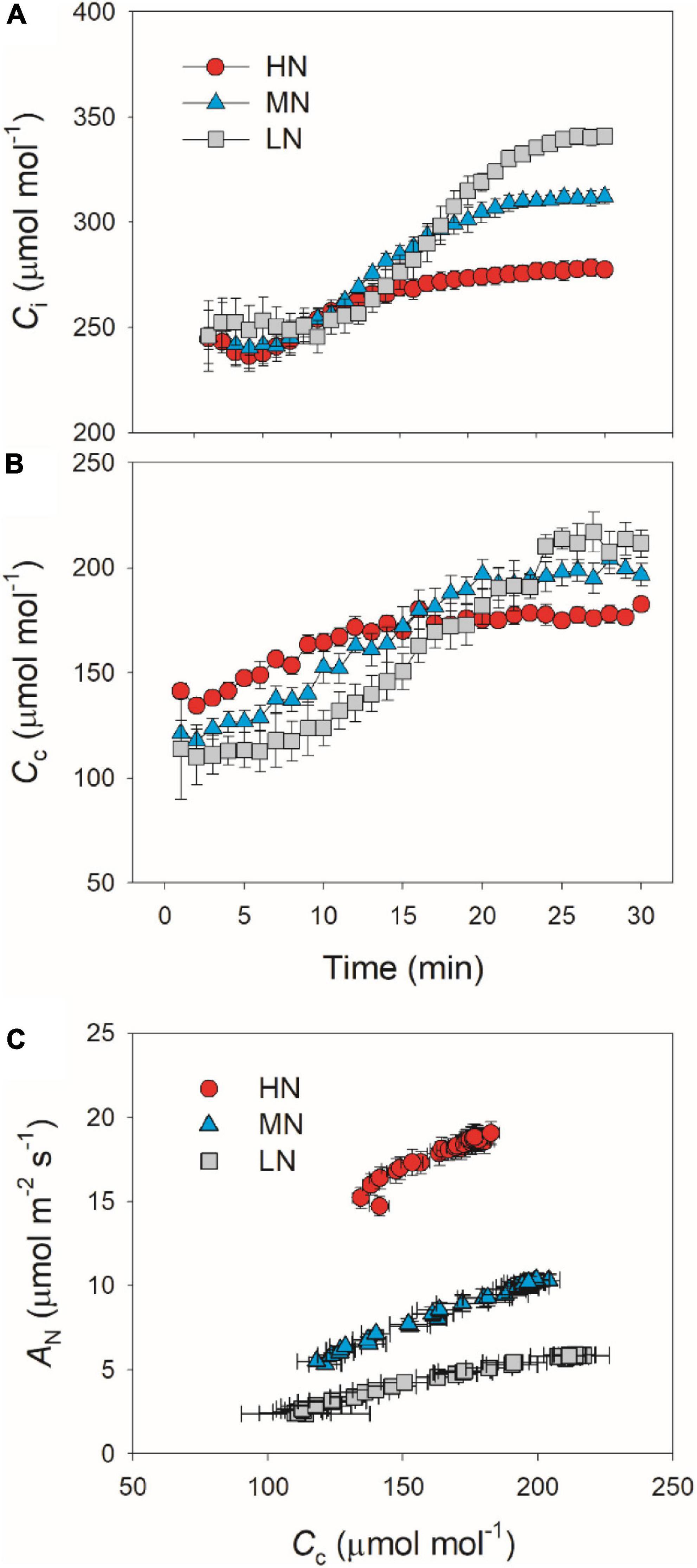
Figure 4. (A,B) Response of intercellular CO2 concentration (Ci) and chloroplast CO2 concentration (Cc) after transition from 50 to 1,500 μmol photons m–2 s–1. (C) Relationship between Cc and AN after transition from 50 to 1,500 μmol photons m–2 s–1. Values are means ± SE (n = 5). HN, MN, and LN represent tomato plants grown under high, medium, and low N concentrations, respectively.
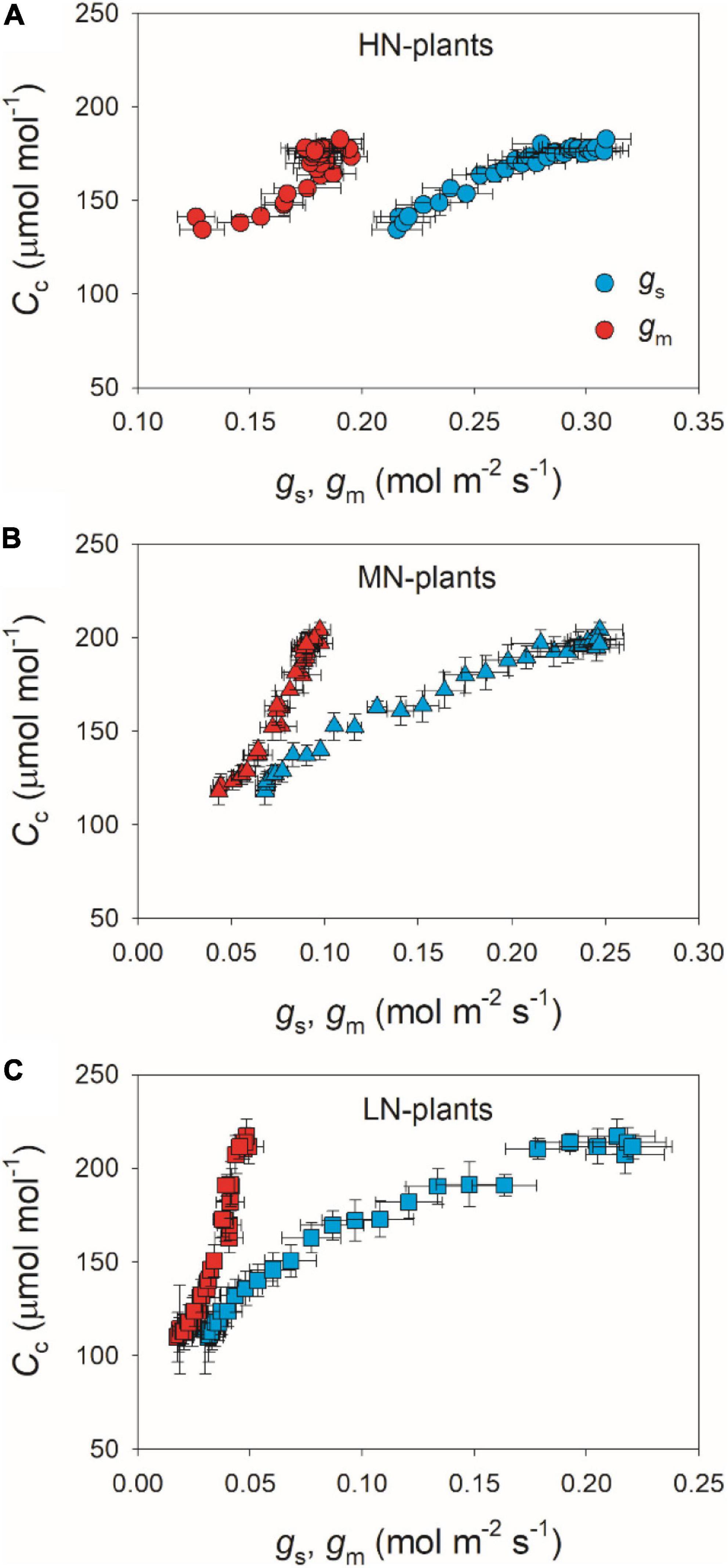
Figure 5. Relationships between gs, gm and Cc after transition from 50 to 1,500 μmol photons m–2 s–1 in HN-plants (A), MN-plants (B), and LN-plants (C). Values are means ± SE (n = 5). HN, MN, and LN represent tomato plants grown under high, medium, and low N concentrations, respectively.
Effects of Leaf N Content on Relative Limitations of Photosynthesis Upon Transfer From Low to High Light
After transition from low to high light, the limitations of photosynthesis by gs (Lgs), gm (Lgm), and biochemical factors (Lb) changed slightly in HN-plants (Figure 6). In MN- and LN-plants, Lgs gradually decreased over time. Within the first 15 min, Lgs was lower in HN-plants than MN- and LN-plants. However, the LN-plants had the lowest Lgs after sufficient photosynthetic induction. Lgm was also maintained stable during whole photosynthetic induction in MN- and LN-plants, but Lb gradually increased from 0.3 to 0.5 in them. Therefore, leaf N content could affect the kinetics of relative limitations of photosynthesis during photosynthetic induction after transfer from low to high light. To explore whether the induction of AN is limited by photosynthetic electron transport, we estimated the dynamic change in ETR. Upon a sudden increase in illumination, ETR rapidly increased and the ETR/(AN + Rd) ratio first increased and then gradually decreased in all plants (Figure 7). These results indicated that the activation speed of ETR was much faster than that of AN. Therefore, during photosynthetic induction, the limitation of ETR imposed to AN was negligible in all samples.
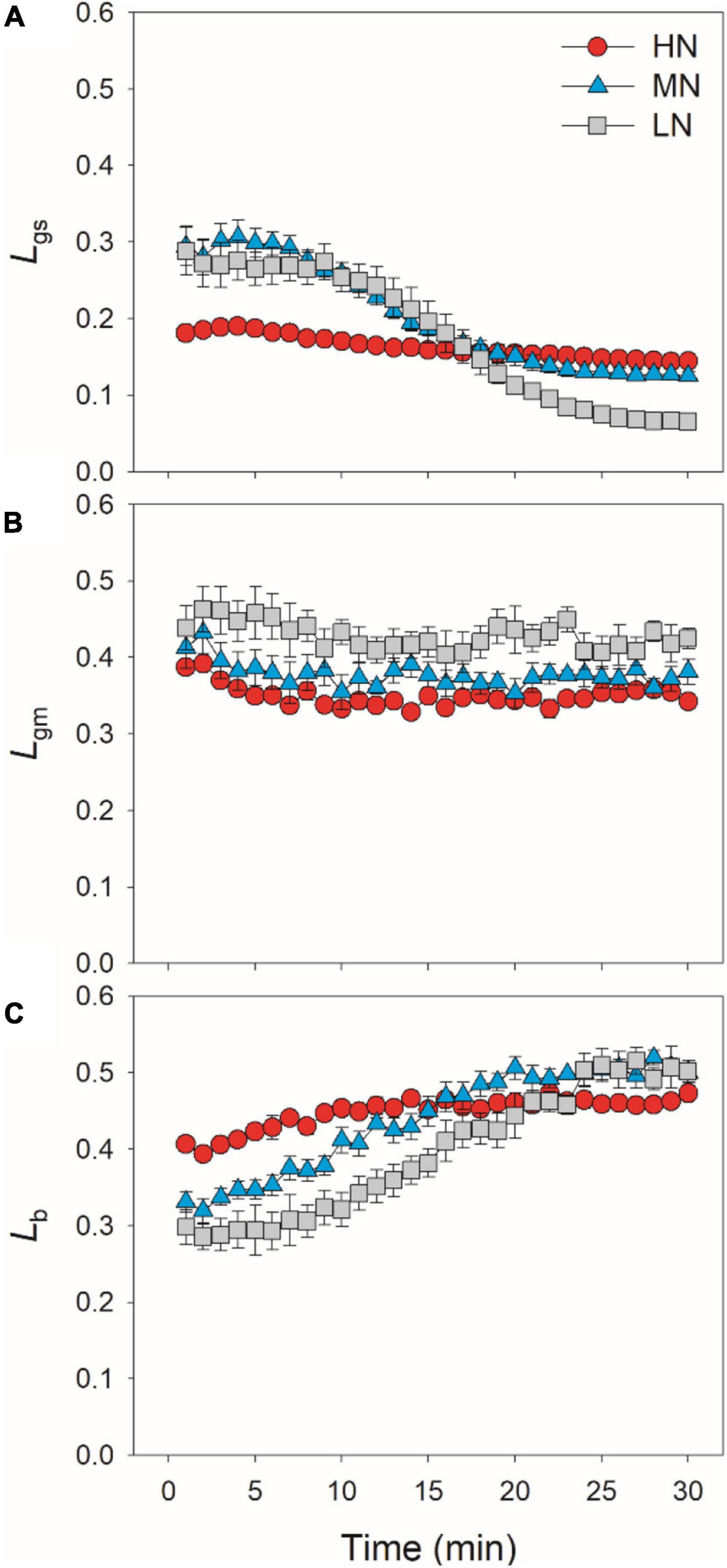
Figure 6. Quantitative analysis of the relative limitations of gs (A), gm (B) and biochemical factors (C) imposed to photosynthesis after transition from 50 to 1,500 μmol photons m–2 s–1. Values are means ± SE (n = 5). HN, MN, and LN represent tomato plants grown under high, medium, and low N concentrations, respectively.
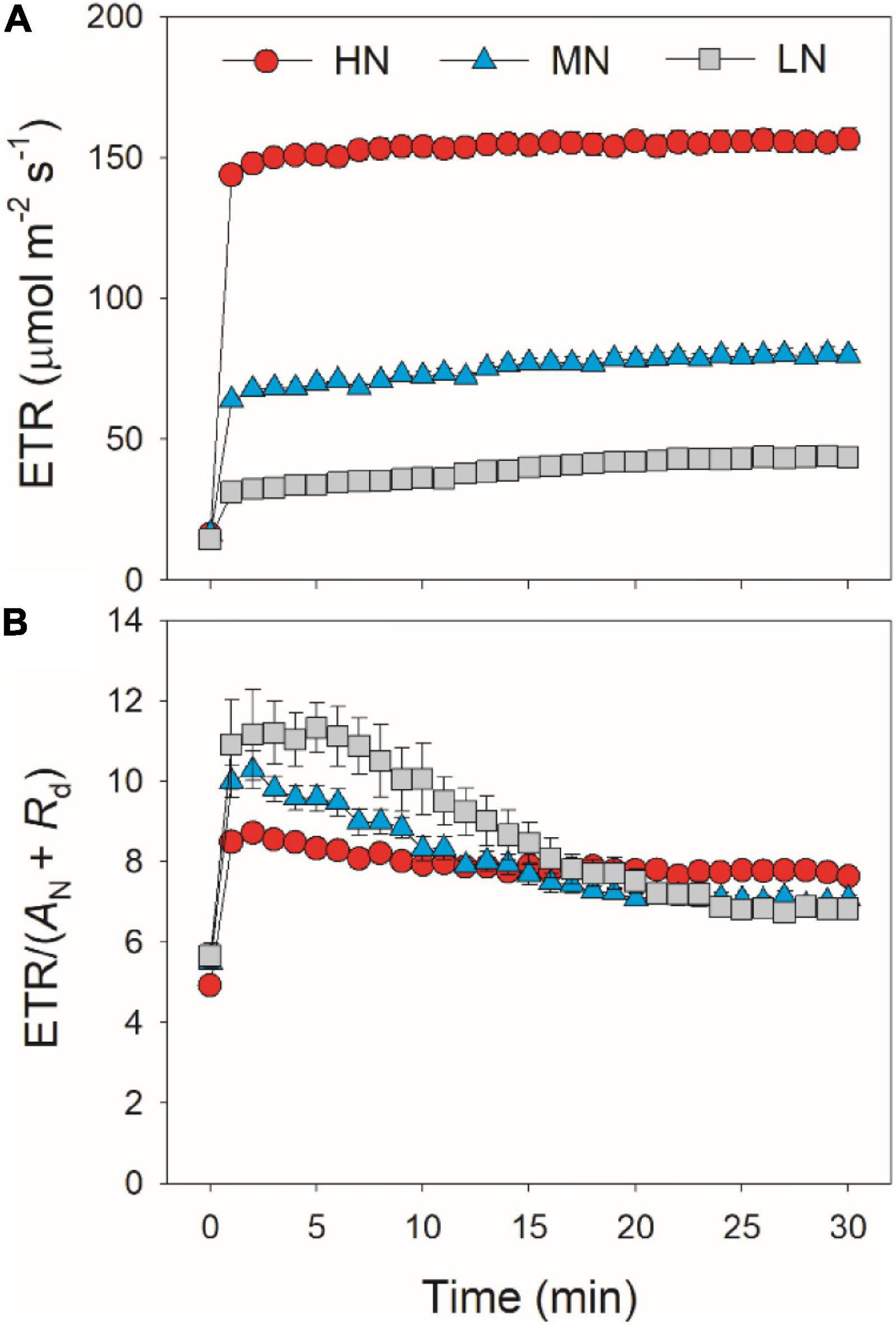
Figure 7. Response of ETR (A) and the ratio of ETR to (AN + Rd) (B) after transition from 50 to 1,500 μmol photons m–2 s–1. Values are means ± SE (n = 5). HN, MN, and LN represent tomato plants grown under high, medium, and low N concentrations, respectively.
Discussion
Leaf N content plays an important role in determining photosynthesis, plant growth, and crop productivity (Makino, 2011). Under natural field conditions, FL and N deficiency usually occurs concomitantly. However, it is unknown how FL and N deficiency interacts to influence photosynthetic physiology in crop plants. In this study, we here for the first time examined the effects of leaf N content on photosynthetic induction after transition from low to high light in tomato. We found that leaf N content significantly affected the induction responses of gs and gm and thus affected induction kinetics of AN. However, the activation speed of photosynthetic electron flow was not influenced by leaf N content. Therefore, the effect of leaf N content on photosynthetic induction was more attributed to the induction kinetics of diffusional conductance rather than the activation speed of electron transport.
In addition to steady-state photosynthetic capacity under high light, the photosynthetic responses to the changes in illumination significantly affect the carbon gain and plant biomass (Adachi et al., 2019; Kimura et al., 2020; Zhang et al., 2020). Many previous studies have documented that leaf N content influences the steady-state photosynthetic performances under high light (Evans and Terashima, 1988; Makino and Osmond, 1991), but few is known about the influence of leaf N content on photosynthetic induction under FL conditions. Similar to previous studies, the maximum steady-state AN under high light significantly declined with the decrease in leaf N content (Table 1). Moreover, we here found that, after transition from low to high light, the HN-plants showed much faster induction response of AN than MN- and LN-plants (Figure 1). The time required to reach 90% of the steady state of photosynthesis (t90AN) was negatively correlated to leaf N content (Figure 2). Therefore, leaf N content significantly affects the photosynthetic induction after transition from low to high light in tomato. This finding is similar to the photosynthetic induction of dark-adapted leaves among canola genotypes (Brassica napus L.) (Liu et al., 2021), but was inconsistent with the phenomenon in soybean (Li et al., 2020) and Panax notoginseng (Chen et al., 2014). In soybean, the induction rate of AN under high light after shading for 5 min was very fast (Pearcy et al., 1996; Li et al., 2020). Furthermore, this fast photosynthetic induction in soybean was not affected by leaf N content (Li et al., 2020). In the shade-establishing plant Panax notoginseng, the higher leaf N content in shade leaves was accompanied with slower photosynthetic induction rate than sun leaves (Chen et al., 2014). Therefore, the effect of leaf N content on fast photosynthetic induction following shade fleck depends on the species and on growth conditions. In MN- and LN-plants of tomato, the delayed induction of AN caused a larger loss of carbon gain under FL. This finding provides insight into why plants grown under low N concentrations display reduction in plant biomass under natural field FL conditions.
After transition from low to high light, the time to reach the maximum Cc was less in HN-plants than MN- and LN-plants (Figure 4). Furthermore, tight and positive relationships were found between Cc and AN in all plants (Figure 4). These results suggested that the induction response of AN was largely determined by the change of CO2 concentration in the site of RuBP carboxylation. The value of Cc in a given leaf is largely affected by CO2 diffusional conductance, which includes gs and gm (Sagardoy et al., 2010; Carriquí et al., 2015; Yang Z.-H. et al., 2018). However, it is unclear whether the photosynthetic induction of AN upon transfer from low to high light is more determined by the induction response of gs or gm. We found that the induction responses of gs and gm were largely delayed in MN- and LN-plants than HN-plants (Figure 1), and the induction rates of gs and gm were negatively correlated with leaf N content (Figure 2). Furthermore, the change of Cc during photosynthetic induction was more related to gm rather than gs (Figure 5), which pointing out the important role of gm response in determining Cc upon transfer from low to high light. Therefore, the delayed photosynthetic induction of AN in plants grown under low N concentrations was more attributed to the slower induction response of gm than gs.
In HN-plants of tomato, photosynthetic limitations by gs, gm, and biochemical factors changed slightly upon transfer from low to high light. Meanwhile, gs imposed to the smallest limitation to AN, owing to the high levels of gs (Figure 6). Therefore, improving the induction response of gs might have a minor factor for improving photosynthesis under FL in HN-plants of tomato under optimal conditions (Kaiser et al., 2020). By comparison, increased gs has a significant effect on photosynthetic CO2 assimilation under FL in Arabidopsis thaliana and rice (Kimura et al., 2020; Yamori et al., 2020). These results indicate that the effects of altered gs kinetics on photosynthesis under FL are species-dependent. In MN- and LN-plants, the relatively slower kinetics of gs led to a higher Lgs of AN during the initial 15 min after transition from low to high light (Figure 6). Therefore, altered gs kinetics would have more significant effects on photosynthetic carbon gain in crop plants grown under low N concentrations.
Many previous studies have indicated that gm act as a major limitation for steady-state AN under high light in many angiosperms (Peguero-Pina et al., 2017; Théroux-Rancourt and Gilbert, 2017; Yang Y.-J. et al., 2018; Huang et al., 2019). Increasing gm has been thought to be a potential target for improving crop productivity and water use efficiency under constant high light (Flexas et al., 2013; Gago et al., 2016). However, the limitation of gm imposed to AN under FL is poorly understood. Upon transition from dark to light, the induction response of gm was much faster than that of gs, which leads to the smallest limitation of gm imposed to AN in Arabidopsis thaliana and tobacco (Sakoda et al., 2021). Consequently, one concluded that altering gm kinetics would have less impact on AN under FL. However, we found that, after transfer from low to high light, Lgm was higher than Lgs in tomato plants (Figure 6). Furthermore, the time to reach 90% of AN was closer to that of gm rather than that of gs (Figure 3). Therefore, altering gm kinetics would significantly influence AN upon transfer from low to high light, at least in tomato. These results suggested that the photosynthetic limitation upon transfer from low to high light was largely different from the photosynthetic induction during illumination of dark-adapted leaves. Improving the induction rate of gm has a potential to enhance carbon gain and plant biomass under natural FL conditions.
A recent study reported that, if RuBP regeneration limitation was assumed, electron transport imposed the greatest limitation to AN during illumination of dark-adapted leaves (Sakoda et al., 2021). Based on this result, it is hypothesized that increased activation of electron transport has the potential to enhance carbon gain under naturally FL environments. Controversially, this study indicated that electron transport was rapidly activated upon transfer from low to high light. After transition from low to high light, the ETR/(AN + Rd) value rapidly increased to the peak within 1–2 min and then gradually decreased over time (Figure 7). These results indicated that, upon transfer from low to high light, the induction response of electron transport was much faster than that of AN, which was consistent with the photosynthetic performance in rice (Yamori et al., 2016b). Therefore, induction response of AN after transition from low to high light was hardly limited by electron transport in tomato. The effect of electron transport on AN upon transition from low to high light is largely different from that upon transition from dark to light. Therefore, to improve photosynthesis under FL in tomato, more attention should be focused on the induction kinetics of CO2 diffusional conductance rather than the activation of electron transport.
Conclusion
We studied the effects of leaf N content on photosynthetic induction after transfer from low to high light in tomato. The induction speeds of AN, gs, and gm significantly decreased with the decrease in leaf N content. Such delayed photosynthetic induction in plants grown under low N concentration caused a larger loss of carbon gain under FL conditions, which further explained why N deficiency reduced plant biomass under natural FL environments. After transition from low to high light, increasing the induction responses of gs and gm has the potential to improve AN in tomato, especially when plants are grown under low N concentration, whereas photosynthetic induction of AN was hardly limited by electron transport. Therefore, altering induction kinetics of CO2 diffusional conductance is likely the most effective target for improving photosynthesis under FL conditions in tomato.
Data Availability Statement
The raw data supporting the conclusions of this article will be made available by the authors, without undue reservation.
Author Contributions
WH and S-BZ designed the study. HS performed the photosynthetic measurements. HS, Y-QZ, and WH performed the data analysis. WH wrote the first draft of the manuscript, which was extensively edited by all authors.
Funding
This work was supported by the National Natural Science Foundation of China (grant nos. 31971412 and 32171505) and the Project for Innovation Team of Yunnan Province (202105AE160012).
Conflict of Interest
The authors declare that the research was conducted in the absence of any commercial or financial relationships that could be construed as a potential conflict of interest.
Publisher’s Note
All claims expressed in this article are solely those of the authors and do not necessarily represent those of their affiliated organizations, or those of the publisher, the editors and the reviewers. Any product that may be evaluated in this article, or claim that may be made by its manufacturer, is not guaranteed or endorsed by the publisher.
Supplementary Material
The Supplementary Material for this article can be found online at: https://www.frontiersin.org/articles/10.3389/fpls.2022.835571/full#supplementary-material
Supplementary Figure 1 | Relationships between t90AN (A), t90gs (B), and the initial gs prior to light change. HN, MN, and LN represent tomato plants grown under high, medium, and low N concentrations, respectively.
Supplementary Figure 2 | Response of intrinsic water use efficiency (iWUE) after transition from 50 to 1,500 μmol photons m−2 s−1. Values are means ± SE (n = 5). HN, MN, and LN represent tomato plants grown under high, medium, and low N concentrations, respectively.
References
Adachi, S., Tanaka, Y., Miyagi, A., Kashima, M., Tezuka, A., Toya, Y., et al. (2019). High-yielding rice Takanari has superior photosynthetic response to a commercial rice Koshihikari under fluctuating light. J. Exp. Bot. 70, 5287–5297. doi: 10.1093/jxb/erz304
Baker, N. R. (2004). Applications of chlorophyll fluorescence can improve crop production strategies: an examination of future possibilities. J. Exp. Bot. 55, 1607–1621. doi: 10.1093/jxb/erh196
Carriquí, M., Cabrera, H. M., Conesa, M., Coopman, R. E., Douthe, C., Gago, J., et al. (2015). Diffusional limitations explain the lower photosynthetic capacity of ferns as compared with angiosperms in a common garden study. Plant Cell Environ. 38, 448–460. doi: 10.1111/pce.12402
Chen, J.-W., Kuang, S.-B., Long, G.-Q., Meng, Z.-G., Li, L.-G., Chen, Z.-J., et al. (2014). Steady-state and dynamic photosynthetic performance and nitrogen partitioning in the shade-demanding plant Panax notoginseng under different levels of growth irradiance. Acta Physiol. Plant. 36, 2409–2420. doi: 10.1007/s11738-014-1614-9
De Souza, A. P., Wang, Y., Orr, D. J., Carmo−Silva, E., and Long, S. P. (2020). Photosynthesis across African cassava germplasm is limited by Rubisco and mesophyll conductance at steady state, but by stomatal conductance in fluctuating light. New Phytol. 225, 2498–2512. doi: 10.1111/nph.16142
Evans, J. R., and Terashima, I. (1988). Photosynthetic characteristics of spinach leaves grown with different nitrogen treatments. Plant Cell Physiol. 29, 157–165. doi: 10.1093/oxfordjournals.pcp.a077462
Eyland, D., van Wesemael, J., Lawson, T., and Carpentier, S. (2021). The impact of slow stomatal kinetics on photosynthesis and water use efficiency under fluctuating light. Plant Physiol. 186, 998–1012. doi: 10.1093/PLPHYS/KIAB114
Fan, X., Cao, X., Zhou, H., Hao, L., Dong, W., He, C., et al. (2020). Carbon dioxide fertilization effect on plant growth under soil water stress associates with changes in stomatal traits, leaf photosynthesis, and foliar nitrogen of bell pepper (Capsicum annuum L.). Environ. Exp. Bot. 179:104203. doi: 10.1016/j.envexpbot.2020.104203
Farquhar, G. D., von Caemmerer, S., and Berry, J. A. (1980). A biochemical model of photosynthetic CO2 assimilation in leaves of C3 species. Planta 149, 78–90. doi: 10.1007/BF00386231
Ferroni, L., Brestiè, M., Živèak, M., Cantelli, R., and Pancaldi, S. (2021). Increased photosynthesis from a deep-shade to high-light regime occurs by enhanced CO2 diffusion into the leaf of Selaginella martensii. Plant Physiol. Biochem. 160, 143–154. doi: 10.1016/j.plaphy.2021.01.012
Ferroni, L., Živèak, M., Sytar, O., Kovár, M., Watanabe, N., Pancaldi, S., et al. (2020). Chlorophyll-depleted wheat mutants are disturbed in photosynthetic electron flow regulation but can retain an acclimation ability to a fluctuating light regime. Environ. Exp. Bot. 178:104156. doi: 10.1016/j.envexpbot.2020.104156
Flexas, J., Niinemets, Ü, Gallé, A., Barbour, M. M., Centritto, M., Diaz-Espejo, A., et al. (2013). Diffusional conductances to CO2 as a target for increasing photosynthesis and photosynthetic water-use efficiency. Photosynth. Res. 117, 45–59. doi: 10.1007/s11120-013-9844-z
Gago, J., Daloso, D. M., Carriquí, M., Nadal, M., Morales, M., Araújo, W. L., et al. (2020). The photosynthesis game is in the “inter-play”: mechanisms underlying CO2 diffusion in leaves. Environ. Exp. Bot. 178:104174. doi: 10.1016/j.envexpbot.2020.104174
Gago, J., Daloso, D., de, M., Figueroa, C. M., Flexas, J., Fernie, A. R., et al. (2016). Relationships of Leaf Net photosynthesis, stomatal conductance, and mesophyll conductance to primary metabolism: a multispecies meta-analysis approach. Plant Physiol. 171, 265–279. doi: 10.1104/pp.15.01660
Genty, B., Briantais, J.-M., and Baker, N. R. (1989). The relationship between the quantum yield of photosynthetic electron transport and quenching of chlorophyll fluorescence. Biochim. Biophys. Acta Gen. Subj. 990, 87–92. doi: 10.1016/S0304-4165(89)80016-9
Grassi, G., and Magnani, F. (2005). Stomatal, mesophyll conductance and biochemical limitations to photosynthesis as affected by drought and leaf ontogeny in ash and oak trees. Plant Cell Environ. 28, 834–849. doi: 10.1111/j.1365-3040.2005.01333.x
Harley, P. C., Loreto, F., Di Marco, G., and Sharkey, T. D. (1992). Theoretical considerations when estimating the mesophyll conductance to CO2 flux by analysis of the response of photosynthesis to CO2. Plant Physiol. 98, 1429–1436. doi: 10.1104/pp.98.4.1429
Huang, W., Yang, Y.-J., Wang, J.-H., and Hu, H. (2019). Photorespiration is the major alternative electron sink under high light in alpine evergreen sclerophyllous Rhododendron species. Plant Sci. 289:110275. doi: 10.1016/j.plantsci.2019.110275
Kaiser, E., Morales, A., Harbinson, J., Heuvelink, E., and Marcelis, L. F. M. (2020). High stomatal conductance in the tomato flacca mutant allows for faster photosynthetic induction. Front. Plant Sci. 11:1317. doi: 10.3389/fpls.2020.01317
Kebeish, R., Niessen, M., Thiruveedhi, K., Bari, R., Hirsch, H.-J., Rosenkranz, R., et al. (2007). Chloroplastic photorespiratory bypass increases photosynthesis and biomass production in Arabidopsis thaliana. Nat. Biotechnol. 25, 593–599. doi: 10.1038/nbt1299
Kimura, H., Hashimoto-Sugimoto, M., Iba, K., Terashima, I., and Yamori, W. (2020). Improved stomatal opening enhances photosynthetic rate and biomass production in fluctuating light. J. Exp. Bot. 71, 2339–2350. doi: 10.1093/jxb/eraa090
Krall, J. P., and Edwards, G. E. (1992). Relationship between photosystem II activity and CO2 fixation in leaves. Physiol. Plant. 86, 180–187. doi: 10.1111/j.1399-3054.1992.tb01328.x
Kromdijk, J., Głowacka, K., Leonelli, L., Gabilly, S. T., Iwai, M., Niyogi, K. K., et al. (2016). Improving photosynthesis and crop productivity by accelerating recovery from photoprotection. Science 354, 857–861. doi: 10.1126/science.aai8878
Li, Y.-T., Li, Y., Li, Y.-N., Liang, Y., Sun, Q., Li, G., et al. (2020). Dynamic light caused less photosynthetic suppression, rather than more, under nitrogen deficit conditions than under sufficient nitrogen supply conditions in soybean. BMC Plant Biol. 20:339. doi: 10.1186/s12870-020-02516-y
Liu, J., Zhang, J., Estavillo, G. M., Luo, T., and Hu, L. (2021). Leaf N content regulates the speed of photosynthetic induction under fluctuating light among canola genotypes (Brassica napus L.). Physiol. Plant. 172, 1844–1852. doi: 10.1111/ppl.13390
Long, S. P., and Bernacchi, C. J. (2003). Gas exchange measurements, what can they tell us about the underlying limitations to photosynthesis? Procedures and sources of error. J. Exp. Bot. 54, 2393–2401. doi: 10.1093/jxb/erg262
Makino, A. (2011). Photosynthesis, grain yield, and nitrogen utilization in rice and wheat. Plant Physiol. 155, 125–129. doi: 10.1104/pp.110.165076
Makino, A., and Osmond, B. (1991). Effects of nitrogen nutrition on nitrogen partitioning between chloroplasts and mitochondria in pea and wheat. Plant Physiol. 96, 355–362. doi: 10.1104/pp.96.2.355
Pearcy, R. W. (1990). Sunflecks and photosynthesis in plant canopies. Annu. Rev. Plant Physiol. Plant Mol. Biol. 41, 421–453. doi: 10.1146/annurev.pp.41.060190.002225
Pearcy, R. W., Krall, J. P., and Sassenrath-Cole, G. F. (1996). “Photosynthesis in fluctuating light environments,” in Photosynthesis and the Environment, ed. N. R. Baker (Dordrecht: Kluwer Academic Publishers), 321–346. doi: 10.1007/0-306-48135-9_13
Peguero-Pina, J. J., Sisó, S., Flexas, J., Galmés, J., García-Nogales, A., Niinemets, Ü, et al. (2017). Cell-level anatomical characteristics explain high mesophyll conductance and photosynthetic capacity in sclerophyllous Mediterranean oaks. New Phytol. 214, 585–596. doi: 10.1111/nph.14406
Sagardoy, R., Vázquez, S., Florez-Sarasa, I. D., Albacete, A., Ribas-Carbó, M., Flexas, J., et al. (2010). Stomatal and mesophyll conductances to CO2 are the main limitations to photosynthesis in sugar beet (Beta vulgaris) plants grown with excess zinc. New Phytol. 187, 145–158. doi: 10.1111/j.1469-8137.2010.03241.x
Sakoda, K., Yamori, W., Groszmann, M., and Evans, J. R. (2021). Stomatal, mesophyll conductance, and biochemical limitations to photosynthesis during induction. Plant Physiol. 185, 146–160. doi: 10.1093/plphys/kiaa011
Sakoda, K., Yamori, W., Shimada, T., Sugano, S. S., Hara-Nishimura, I., and Tanaka, Y. (2020). Higher stomatal density improves photosynthetic induction and biomass production in Arabidopsis under fluctuating light. Front. Plant Sci. 11:1308. doi: 10.3389/fpls.2020.589603
Sakowska, K., Alberti, G., Genesio, L., Peressotti, A., Delle Vedove, G., Gianelle, D., et al. (2018). Leaf and canopy photosynthesis of a chlorophyll deficient soybean mutant. Plant. Cell Environ. 41, 1427–1437. doi: 10.1111/pce.13180
Slattery, R. A., Walker, B. J., Weber, A. P. M., and Ort, D. R. (2018). The impacts of fluctuating light on crop performance. Plant Physiol. 176, 990–1003. doi: 10.1104/pp.17.01234
South, P. F., Cavanagh, A. P., Liu, H. W., and Ort, D. R. (2019). Synthetic glycolate metabolism pathways stimulate crop growth and productivity in the field. Science 363:eaat9077. doi: 10.1126/science.aat9077
Sudo, E., Makino, A., and Mae, T. (2003). Differences between rice and wheat in ribulose-1,5-bisphosphate regeneration capacity per unit of leaf-N content. Plant Cell Environ. 26, 255–263. doi: 10.1046/j.1365-3040.2003.00955.x
Takashima, T., Hikosaka, K., and Hirose, T. (2004). Photosynthesis or persistence: nitrogen allocation in leaves of evergreen and deciduous Quercus species. Plant Cell Environ. 27, 1047–1054. doi: 10.1111/j.1365-3040.2004.01209.x
Tazoe, Y., Von Caemmerer, S., Badger, M. R., and Evans, J. R. (2009). Light and CO2 do not affect the mesophyll conductance to CO2 diffusion in wheat leaves. J. Exp. Bot. 60, 2291–2301. doi: 10.1093/jxb/erp035
Théroux-Rancourt, G., and Gilbert, M. E. (2017). The light response of mesophyll conductance is controlled by structure across leaf profiles. Plant Cell Environ. 40, 726–740. doi: 10.1111/pce.12890
Timm, S., Florian, A., Arrivault, S., Stitt, M., Fernie, A. R., and Bauwe, H. (2012). Glycine decarboxylase controls photosynthesis and plant growth. FEBS Lett. 586, 3692–3697. doi: 10.1016/j.febslet.2012.08.027
Timm, S., Wittmiß, M., Gamlien, S., Ewald, R., Florian, A., Frank, M., et al. (2015). Mitochondrial dihydrolipoyl dehydrogenase activity shapes photosynthesis and photorespiration of Arabidopsis thaliana. Plant Cell 27, 1968–1984. doi: 10.1105/tpc.15.00105
von Caemmerer, S., and Evans, J. R. (2015). Temperature responses of mesophyll conductance differ greatly between species. Plant Cell Environ. 38, 629–637. doi: 10.1111/pce.12449
Warren, C. R., and Dreyer, E. (2006). Temperature response of photosynthesis and internal conductance to CO2: results from two independent approaches. J. Exp. Bot. 57, 3057–3067. doi: 10.1093/jxb/erl067
Xiong, D., Douthe, C., and Flexas, J. (2018). Differential coordination of stomatal conductance, mesophyll conductance, and leaf hydraulic conductance in response to changing light across species. Plant. Cell Environ. 41, 436–450. doi: 10.1111/pce.13111
Xiong, D., Liu, X., Liu, L., Douthe, C., Li, Y., Peng, S., et al. (2015). Rapid responses of mesophyll conductance to changes of CO2 concentration, temperature and irradiance are affected by N supplements in rice. Plant Cell Environ. 38, 2541–2550. doi: 10.1111/pce.12558
Yamori, W., Evans, J. R., and Von Caemmerer, S. (2010a). Effects of growth and measurement light intensities on temperature dependence of CO2 assimilation rate in tobacco leaves. Plant Cell Environ. 33, 332–343. doi: 10.1111/j.1365-3040.2009.02067.x
Yamori, W., Noguchi, K., Hikosaka, K., and Terashima, I. (2010b). Phenotypic plasticity in photosynthetic temperature acclimation among crop species with different cold tolerances. Plant Physiol. 152, 388–399. doi: 10.1104/pp.109.145862
Yamori, W., Kondo, E., Sugiura, D., Terashima, I., Suzuki, Y., and Makino, A. (2016a). Enhanced leaf photosynthesis as a target to increase grain yield: insights from transgenic rice lines with variable Rieske FeS protein content in the cytochrome b6/f complex. Plant Cell Environ. 39, 80–87. doi: 10.1111/pce.12594
Yamori, W., Makino, A., and Shikanai, T. (2016b). A physiological role of cyclic electron transport around photosystem I in sustaining photosynthesis under fluctuating light in rice. Sci. Rep. 6:20147. doi: 10.1038/srep20147
Yamori, W., Kusumi, K., Iba, K., and Terashima, I. (2020). Increased stomatal conductance induces rapid changes to photosynthetic rate in response to naturally fluctuating light conditions in rice. Plant Cell Environ. 43, 1230–1240. doi: 10.1111/pce.13725
Yamori, W., Nagai, T., and Makino, A. (2011). The rate-limiting step for CO2 assimilation at different temperatures is influenced by the leaf nitrogen content in several C3 crop species. Plant Cell Environ. 34, 764–777. doi: 10.1111/j.1365-3040.2011.02280.x
Yang, K., Yang, J., Lv, C., Cao, P., Deng, X., Wang, Y., et al. (2021). Reduced mesophyll conductance induces photosynthetic acclimation of japonica rice under elevated CO2. Environ. Exp. Bot. 190:104590. doi: 10.1016/j.envexpbot.2021.104590
Yang, Y.-J., Hu, H., and Huang, W. (2020). The light dependence of mesophyll conductance and relative limitations on photosynthesis in evergreen Sclerophyllous Rhododendron species. Plants 9:1536. doi: 10.3390/plants9111536
Yang, Y.-J., Tong, Y.-G., Yu, G.-Y., Zhang, S.-B., and Huang, W. (2018). Photosynthetic characteristics explain the high growth rate for Eucalyptus camaldulensis: implications for breeding strategy. Ind. Crops Prod. 124, 186–191. doi: 10.1016/j.indcrop.2018.07.071
Yang, Z.-H., Huang, W., Yang, Q.-Y., Chang, W., and Zhang, S.-B. (2018). Anatomical and diffusional determinants inside leaves explain the difference in photosynthetic capacity between Cypripedium and Paphiopedilum, Orchidaceae. Photosynth. Res. 136, 315–328. doi: 10.1007/s11120-017-0466-8
Zhang, Y., Kaiser, E., Marcelis, L. F. M., Yang, Q., and Li, T. (2020). Salt stress and fluctuating light have separate effects on photosynthetic acclimation, but interactively affect biomass. Plant Cell Environ. 43, 2192–2206. doi: 10.1111/pce.13810
Keywords: fluctuating light, nitrogen, photosynthesis, mesophyll conductance, photosynthetic limitation
Citation: Sun H, Zhang Y-Q, Zhang S-B and Huang W (2022) Photosynthetic Induction Under Fluctuating Light Is Affected by Leaf Nitrogen Content in Tomato. Front. Plant Sci. 13:835571. doi: 10.3389/fpls.2022.835571
Received: 14 December 2021; Accepted: 17 January 2022;
Published: 17 February 2022.
Edited by:
Marian Brestic, Slovak University of Agriculture, SlovakiaReviewed by:
Xinghong Yang, Shandong Agricultural University, ChinaAlfred Holzwarth, Max Planck Institute for Chemical Energy Conversion, Germany
Copyright © 2022 Sun, Zhang, Zhang and Huang. This is an open-access article distributed under the terms of the Creative Commons Attribution License (CC BY). The use, distribution or reproduction in other forums is permitted, provided the original author(s) and the copyright owner(s) are credited and that the original publication in this journal is cited, in accordance with accepted academic practice. No use, distribution or reproduction is permitted which does not comply with these terms.
*Correspondence: Wei Huang, aHVhbmd3ZWlAbWFpbC5raWIuYWMuY24=