- 1Co-Innovation Center for Sustainable Forestry in Southern China, College of Forestry, Nanjing Forestry University, Nanjing, China
- 2Jiangsu Key Laboratory for Prevention and Management of Invasive Species, Nanjing Forestry University, Nanjing, China
Soil salinity is one of the main factors limiting agricultural development worldwide and has an adverse effect on plant growth and yield. To date, plant growth-promoting rhizobacteria (PGPR) are considered to be one of the most promising eco-friendly strategies for improving saline soils. The bacterium Bacillus megaterium ZS-3 is an excellent PGPR strain that induces growth promotion as well as biotic stress resistance and tolerance to abiotic stress in a broad range of host plants. In this study, the potential mechanisms of protection against salinity stress by B. megaterium ZS-3 in Arabidopsis thaliana were explored. Regulation by ZS-3 improved growth in A. thaliana under severe saline conditions. The results showed that ZS-3 treatment significantly increased the biomass, chlorophyll content and carotenoid content of A. thaliana. Compared to the control, the leaf area and total fresh weight of plants inoculated with ZS-3 increased by 245% and 271%, respectively; the chlorophyll a, chlorophyll b, and carotenoid contents increased by 335%, 146%, and 372%, respectively, under salt stress. Physiological and biochemical tests showed that ZS-3 regulated the content of osmotic substances in plants under salt stress. Compared to the control, the soluble sugar content of the ZS-3-treated group was significantly increased by 288%, while the proline content was significantly reduced by 41.43%. Quantification of Na+ and K+ contents showed that ZS-3 treatment significantly reduced Na+ accumulation and increased the K+/Na+ ratio in plants. ZS-3 also isolated Na+ in vesicles by upregulating NHX1 and AVP1 expression while limiting Na+ uptake by downregulating HKT1, which protected against Na+ toxicity. Higher levels of peroxidase and catalase activity and reduced glutathione were detected in plants inoculated with ZS-3 compared to those in uninoculated plants. In addition, it was revealed that ZS-3 activates salicylic acid (NPR1 and PR1) and jasmonic acid/ethylene (AOS, LOX2, PDF1.2, and ERF1) signaling pathways to induce systemic tolerance, thereby inducing salt tolerance in plants. In conclusion, the results of this study indicate that ZS-3 has the potential to act as an environmentally friendly salt tolerance inducer that can promote plant growth in salt-stressed environments.
Introduction
Soil salinity not only threatens plant growth and yield but also leads to a dramatic reduction in soil quality and availability (Abbas et al., 2019). It is a phenomenon of soil degradation caused by the enrichment of salt ions in the soil due to a combination of natural factors and human activities (Abbas et al., 2019). Low rainfall, high evaporation, native rocks, saline irrigation water, and poor water management are increasingly causing salinization problems in agricultural areas (Singh, 2021; Kumawat et al., 2022). In saline areas, the natural growth and development as well as the physiological metabolism of plants are severely disturbed by saline stress (Liu et al., 2020). The early response of salt stress is osmotic or drought stress, which can be sensed by cells within 5 min (Zelm et al., 2020). Ionic stress is induced at a later stage, when Na+ accumulation in the soil not only rapidly reduces water availability but also slowly accumulates in the shoots (Zelm et al., 2020). In addition, plants produce excess reactive oxygen species (ROS), which interfere with the electron transport chain in chloroplasts and mitochondria (Gill and Tuteja, 2010). Therefore, a range of regulatory mechanisms have evolved in response to adversity stress. For example, low molecular weight organic compounds such as amino acids, sugars, inorganic ions, and organic acids accumulate in the cytoplasm to reduce the osmotic potential (Abbas et al., 2019). To protect themselves from Na+ toxicity, plants enhance Na+ tolerance by regulating Na+ transport, translocation and intracellular compartmentalization, which are mediated by ion transport-associated proteins such as high-affinity K+ transporter 1 (HKT1) and Na+/H+ reverse transporter proteins (NHXs; Qin et al., 2016). However, defense mechanisms are not widespread in all plants. Sustainable methods to improve salt tolerance in saline soil crops are urgently needed.
Various strategies, such as improved irrigation techniques, physical flushing, leaching and scraping of saline soils, traditional genetic breeding, and genetic engineering of plants, have been used to mitigate this problem (Abbas et al., 2019; Kumar Arora et al., 2020). However, there are limitations to these methods, and some approaches may even have additional negative impacts on ecosystems. For example, irrigation techniques are usually very expensive and unsustainable (Singh, 2021). Soil leaching requires relatively low soil moisture and a low water table, making this a difficult approach for agro-ecosystems, and important nutrients in the soil are lost during the leaching process (Kumar Arora et al., 2020). Traditional genetic breeding and plant genetic engineering to produce salt-tolerant crops are widely considered to be effective strategies, but these methods usually require a lengthy selection and validation process (Kumawat et al., 2022). Moreover, it is still uncertain whether transgenic crops will be universally accepted (Qin et al., 2016). To date, plant growth-promoting rhizobacteria (PGPR) are considered to be one of the most promising eco-friendly strategies for improving saline soils (Kumar Arora et al., 2020).
PGPR, as biological inoculants, usually have various direct mechanisms, such as nitrogen fixation, IAA production and phosphorus solubilization, to promote plant growth. PGPR also have indirect mechanisms of plant growth promotion such as siderophore production, volatile organic compounds (VOC), and ACC deaminase (Etesami and Alikhani, 2016; Gong et al., 2020; Kumar Arora et al., 2020). When applied in the field, PGPR resulted in an improvement of the texture, EC, pH, and organic matter of saline lands (Kumar Arora et al., 2020). There is clear evidence that PGPR are essential for promoting plant adaptation to salinity (Qin et al., 2016; Kumar Arora et al., 2020). PGPR contribute to the tolerance of many plants to abiotic stresses by synthesizing osmotic substances, balancing ionic homeostasis in plants and inducing antioxidant mechanisms (Lucke et al., 2020a). Bacillus amyloliquefaciens FZB42 VOCs alleviate Na+ toxicity by significantly reducing Na+ contents in whole plants and upregulating the expression of ion transport genes (Liu et al., 2020). Increased accumulation of osmoregulatory substances, such as proline and soluble sugars, has been reported to be an important mechanism by which PGPR promote plant growth under saline conditions. Kocuria rhizophila Y1 significantly increased maize biomass, and an elevated proline content, soluble sugar content and K+/Na+ ratio were also observed under NaCl treatment (Li et al., 2020a). Shahid et al. (2021) also indicated that PGPR, such as the salinity-tolerant Kosakonia sacchari MSK1, increased the chlorophyll content and carotenoid content of Vigna radiata under different levels of salinity, mitigating the negative effects of salt stress on plant photosynthesis.
Molecular studies of gene expression, protein synthesis, and metabolite production in plants also confirm that the PGPR signaling network confers tolerance to plant stress (Ilangumaran and Smith, 2017). Jasmonic acid (JA), ethylene (ET), and salicylic acid (SA) are the major signaling molecules in plant stress responses, and their signaling cascades have been extensively studied (Khan et al., 2020). JA is a positive regulator of salt tolerance, which together with ethylene (ET) induces defense responses to abiotic stresses in plants (Takeuchi et al., 2011; Liu et al., 2017). SA plays an important role in systemic acquired resistance (SAR) to attack by biotic pathogens (Niu et al., 2016; Timmermann et al., 2017). These two processes show an antagonistic relationship when responding to biotic stresses (Timmermann et al., 2017). Hormone metabolism in signaling networks mediated by PGPR provides cross-protective properties and attenuates abiotic stress (Khan et al., 2020). Bacillus amyloliquefaciens FZB42 confers salt tolerance by upregulating the plant JA/ET pathway via PGPR-plant interactions (Liu et al., 2017). However, to our knowledge, only a few studies have revealed that PGPR induce salt tolerance in plants via SA (Liu et al., 2017). Furthermore, there is a general consensus that crosstalk between JA and SA is widely present in biotic stresses but is rarely reported under salt stress.
Previous studies have shown that Bacillus megaterium ZS-3 (ZS-3) is an excellent PGPR with the ability to solubilize phosphorus and produce organic acids and IAA (Lu et al., 2018; Qian and Ye, 2020). It can induce plant growth and coping responses to biotic stresses. We hypothesize that ZS-3 effectively mitigates the negative effects of salt stress on plants and reveal the physiological and molecular mechanisms of ZS-3-induced salt stress tolerance in plants so that this strain can be more scientifically applied as a biological inoculant to improve the productivity of crops in saline soils. In this work, the effects of ZS-3 on plant morphological and biochemical properties, such as plant growth, photosynthetic capacity, Osmotic substance accumulation (proline and soluble sugars), ROS production, antioxidant enzyme activity, and ion uptake (K + and Na +) were evaluated in the presence and absence of salt stress. In addition, the transcript levels of genes related to plant growth and stress tolerance, such as Na+ extrusion, SA, JA and ET-mediated defense response genes, were also dynamically observed.
Materials and Methods
Plant Materials and Treatments
Arabidopsis thaliana ecotype Columbia-0 (Col-0) seeds were surface sterilized with NaOCl (10%, v/v) for 5 min, followed by three washes with 75% ethanol and further rinsing at least three times with sterilized water. They were then placed on 10-cm Petri dishes containing one-half-strength Murashige and Skoog ( MS) agar medium supplemented with 1% sucrose and 0.8% agar (pH 5.8). All plates were covered and sealed with Parafilm and were stored at 4°C in the dark for 48 h to ensure stratification. Seeds were grown for 6 days under long-day conditions (16 h light/8 h dark) in a light incubator maintained at 22 ± 2°C.
Bacterial Cultures and Inoculation
The B. megaterium strain ZS-3 (GenBank: OM085668) was isolated from the branch tissue of Cinnamomum camphora. ZS-3 was grown on Luria-Bertani (LB) medium at 28°C for 16 h and then collected, washed, and diluted with phosphate buffered saline (PBS) solution to an OD600 of 0.1. Five microliters of bacterial dilution was inoculated 2 cm from the roots of 6-day-old Col-0 seedlings and placed vertically on MS plates.
In vitro Salt Stress
Six-day-old Col-0 seedlings were placed on MS plates with an additional 0 m mol/L (mM) NaCl or 100 mM NaCl (high salt stress), for two treatments with ZS-3 and without ZS-3 inoculation. There were 20 replicate plates for each treatment and eight seedlings in each plate. Seedlings were harvested 14 days after inoculation for photography and analysis.
Growth and Biomass Yield
After 14 days of cultivation, plants were detached from the MS plates for biomass analysis. The plant fresh weight was measured by an analytical scale. The lengths of the main and lateral roots of the plants were measured with a ruler. The total leaf surface area was quantified by a root system scanner (Expression 11000XL, Japan).
Determination of Chlorophyll and Carotenoid Contents
The chlorophyll content of the leaves was measured as previously described Azarmi et al. (2015). Fresh leaves (0.1 g) were soaked and ground in 80% acetone. Absorbance was recorded at 663.6, 646.6, and 470 nm on a Multiskan spectrum (Thermo, United States) to quantify the chlorophyll a, chlorophyll b and carotenoids. Chlorophyll and carotenoid concentrations were calculated as follows:
Determination of Osmoregulatory Substances
Proline and total soluble sugars were extracted from 0.1 g of fresh leaves. The proline content was determined using the colorimetric assay of Bates et al. (1973). The absorbance at 520 nm was determined with a spectrophotometer (Lambda 365, United States). A standard curve was made with L-proline, and distilled water was used as a control.
The procedure was used to assess the soluble sugar content (μg mg−1 FW). Serial dilutions of D-glucose were used to make the standard curve. The optical density of the mixture was measured at 620 nm with a spectrophotometer (Lambda 365, United States).
Antioxidant Enzyme Activities and GSH, H2O2, and MDA Contents
CAT, POD, GSH, H2O2, and MDA were extracted from 0.1 g of fresh leaves. All absorbance readings were taken with a Multiskan spectrum (Thermo, United States).
The catalase (CAT) activity (nmol min−1 g−1 FW) of whole plants was determined by a CAT kit (Comin, Suzhou, China). The absorbance was recorded at 240 nm (Rady et al., 2019).
The peroxidase (POD) activity (U g−1 FW) of whole plants was assayed by a POD kit (Comin, Suzhou, China). The absorbance was recorded at 470 nm.
The content (μmol g−1 FW) of reduced glutathione (GSH) was determined by a reduced GSH kit (Comin, Suzhou, China). The absorbance was recorded at 412 nm.
To determine the hydrogen peroxide (H2O2) content, whole plants were homogenized in an ice bath with acetone (1 ml). The resulting homogenate was centrifuged at 8,000 rpm for 10 min at 4°C. Determination was carried out according to the procedure of the H2O2 kit (Comin, Suzhou, China), and the absorbance was read at 415 nm.
The MDA content in leaf samples was determined by the thiobarbiturate reaction according to a previously described method (Kumar et al., 2013).
Determination of K+, Na+, and K+/Na+ Contents
Fresh leaves and roots were harvested and dried at 85°C for 2 days to obtain a constant weight. The dried samples were ground with a mortar and filtered through a sieve with a pore size of 0.44 mm. The resulting powder (50 mg) was treated with a mixture of acids (5 ml HNO3 in 1 ml HClO4) at room temperature overnight. The sample was then digested at 200°C until the volume of the sample was reduced to 0.5 ml. Ion concentrations (K+ and Na+) were determined by flame spectrophotometry (AOE, China) based on the method described in Wolf (1982).
Real-Time Quantitative PCR
Quantitative real-time PCR (qRT–PCR) was performed on uninoculated and ZS-3-inoculated Col-0 seedlings to quantify the expression levels of ion transporter genes (NHX1; Na+/H+ antiporter, AVP1; encodes vacuolar H+-pyrophosphatase and HKT1; high-affinity K+ transporter 1) as well as plant immunity-related genes, including markers of the SA signaling pathway (PR1; pathogenesis-associated 1, NPR1 nonexpressor of pathogenesis-related 1) and the JA/ET-mediated defense response pathway (PDF1.2; plant defensin 1.2, LOX2; lipoxygenase 2, AOS; allene oxide synthase, ERF1; ethylene-responsive factor 1). Actin in Col-0 was used as an internal reference. The primers used in this study are listed in Supplementary Table S1.
RNA was extracted from the plants after 3, 5 and 8 days of salt treatment using the Fast Pure Plant Total RNA Isolation Kit (Vazyme, Nanjing) following the manufacturer’s instructions. Purified RNA was quantified using a UV scanning NanoDrop™ 2000 spectrophotometer (Thermo Scientific, Germany). Absorbance ratios at 260, 280 and 230 nm were recorded to assess the purity of the RNA. RNA was electrophoresed on a 1.5% (w/v) formaldehyde agarose gel and visualized using a UV transilluminator. The remaining genomic DNA was removed, and the first-strand cDNA was synthesized by HiScript II Q RT SuperMix for qPCR (Vazyme, Nanjing). qRT–PCR analysis was performed using SYBR qPCR Master Mix (Vazyme, Nanjing) with an ABI Prism 7,500 (Applied Biosystems, Foster City, CA, United States). PCR systems were set up as described in Li et al. (2020b). PCR amplification conditions were set up as described in Showmy and Yusuf (2020). For each treatment, three biological replicates were included. Gene expression levels were determined according to the 2-ΔΔCt method (Fu et al., 2020).
Data Analyses
The completely randomized design was the layout in this study. Statistical analysis of the data was performed using SPSS (version 22.0; SPSS, Inc.). One-way analysis of variance (ANOVA), Student’s t-test (t-test) and Duncan’s multiple range test were performed. Data represented in graphs are means ± standard deviations (SD) of at least three replicate samples (n = 3).
Results
ZS-3 Promotes Plant Growth and Increases Plant Salt Tolerance
To evaluate the effect of ZS-3 on the growth and salt tolerance of plants, in vitro salt stress experiments were carried out. After 14 days of coculture, plant biomass, including fresh weight, leaf area, and primary and lateral roots, was analyzed. Arabidopsis thaliana subjected to salt stress due to high concentrations of NaCl had a significantly smaller leaf area than that under the unstressed treatment, but ZS-3 treatment significantly increased the biomass of the plant and darkened the leaves (Figure 1A). In the absence of salt stress, ZS-3 treatment increased the leaf area of plants by 106% compared to that of uninoculated plants, while under salt stress, the leaf area of plants under the ZS-3 treatment increased by 245% compared to the control (Figure 1B). In addition, ZS-3 treatment increased the total fresh weight (FW) of plants by 231% and 271% in the absence and presence of salt stress, respectively (Figure 1C). The data showed that ZS-3 significantly increased the number of lateral roots of plants, while there was no significant difference in primary root length between inoculated and uninoculated plants (Figures 1D,E). These results indicated that ZS-3 promoted plant growth and enhanced plant tolerance to salt stress.
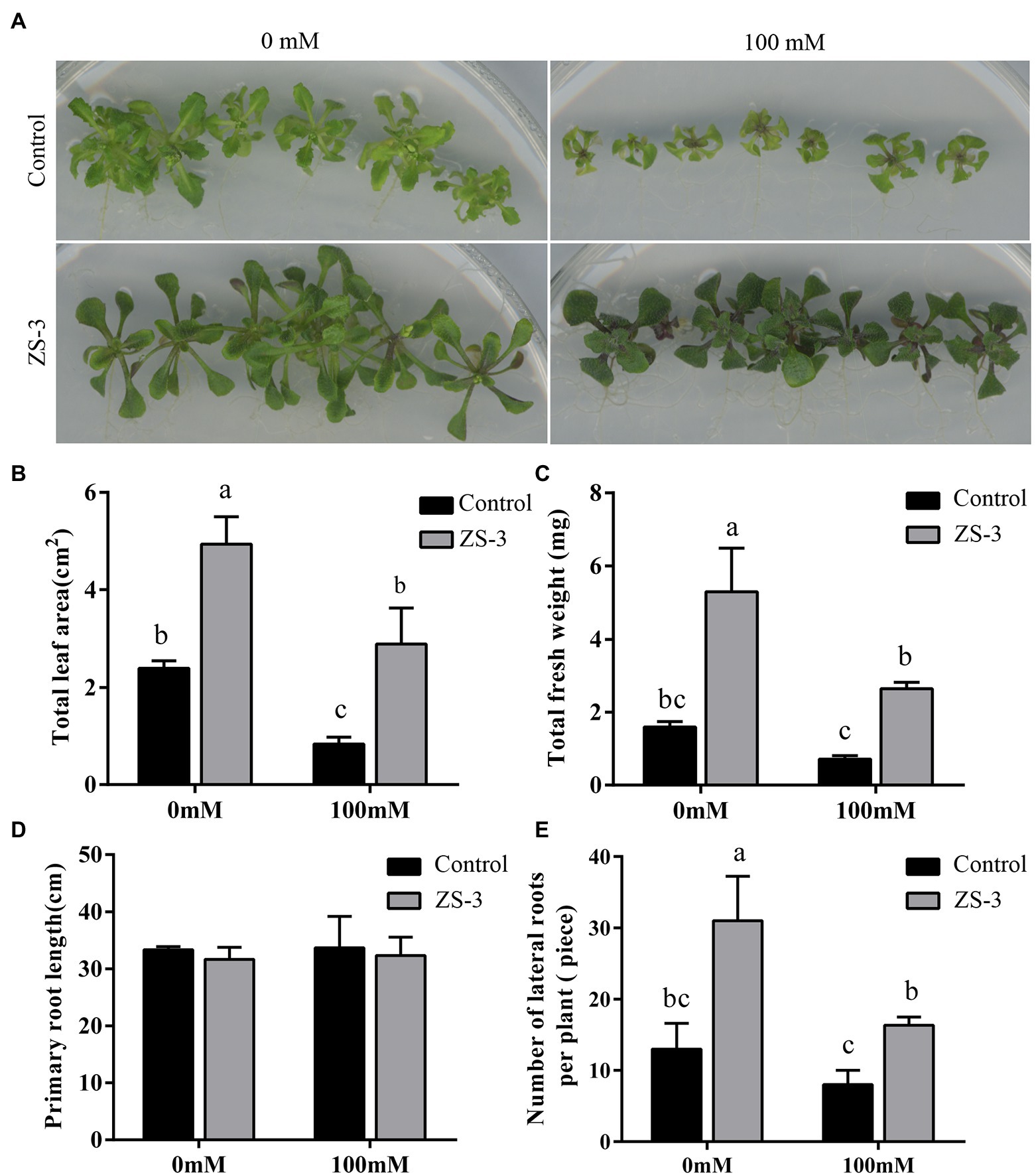
Figure 1. Inoculation with ZS-3 increases biomass in Arabidopsis thaliana. Plants were treated with salt stress for 14 days, pictures (A) were collected, and the plants were analyzed for total leaf area (B), total fresh weight (C), primary root length (D), and lateral root number (E). The results are the mean ± standard deviation from three independent experiments. Different lowercase letters above the bars represent significant differences based on one-way ANOVA (Duncan’s multiple range, p < 0.05).
Enhanced Biosynthesis of Photosynthetic Pigments
ZS-3 inoculation significantly induced the biosynthesis of pigments under either the presence or absence of salt stress (Figure 2). In the absence of salt stress, ZS-3 treatment increased chlorophyll a, chlorophyll b and carotenoids by 202%, 112%, and 260%, respectively, compared to levels in uninoculated plants. Under 100 mM salt stress, the ability of plants to produce chlorophyll and carotenoids was inhibited compared to the absence of salt stress. However, inoculation with ZS-3 increased the content of chlorophyll a, chlorophyll b and carotenoids by 335%, 146% and 372%, respectively. This suggests that ZS-3 increases the photosynthetic capacity of plants in the presence and absence of salt stress.
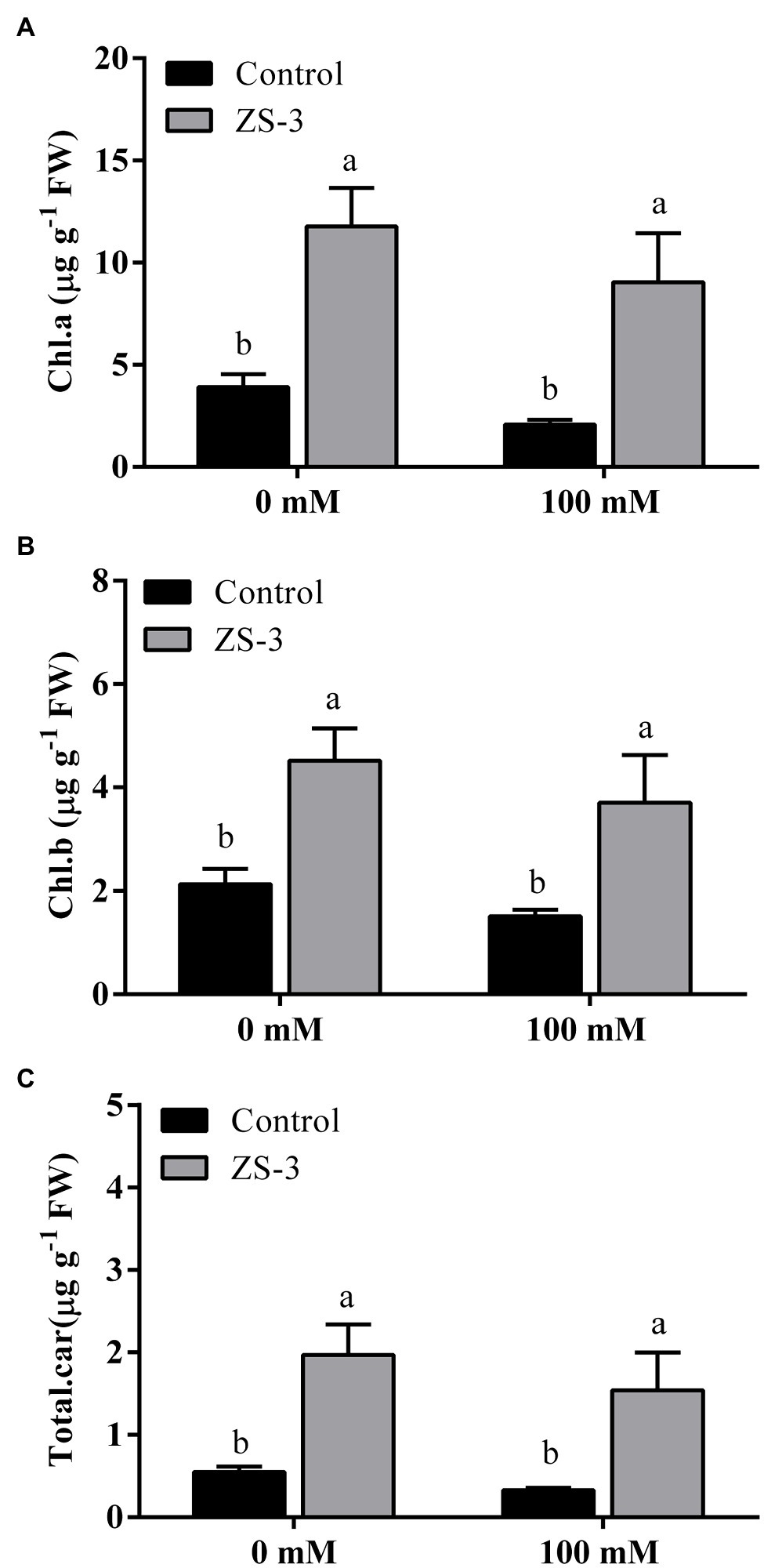
Figure 2. Effect of inoculation with ZS-3 on chlorophyll a (A), chlorophyll b (B) and carotenoid (C) contents in Arabidopsis thaliana. The results are the mean ± standard deviation from three independent experiments. Different lowercase letters above the bars represent significant differences based on one-way ANOVA (Duncan’s multiple range, p < 0.05).
Regulation of Osmoregulatory Substances by ZS-3
The accumulation of compatible substances protects cells from damage caused by salt stress. Therefore, the contents of proline and soluble sugars in plant leaves were measured. In the absence of salt stress, there was no significant difference in plant proline (Figure 3A) and soluble sugar contents (Figure 3B) between the control and ZS-3-treated groups. The soluble sugar content (Figure 3B) was significantly increased by 288% and the proline content (Figure 3A) was reduced by 41.43% in plants inoculated with ZS-3 compared to the control levels under 100 mM salt stress conditions.
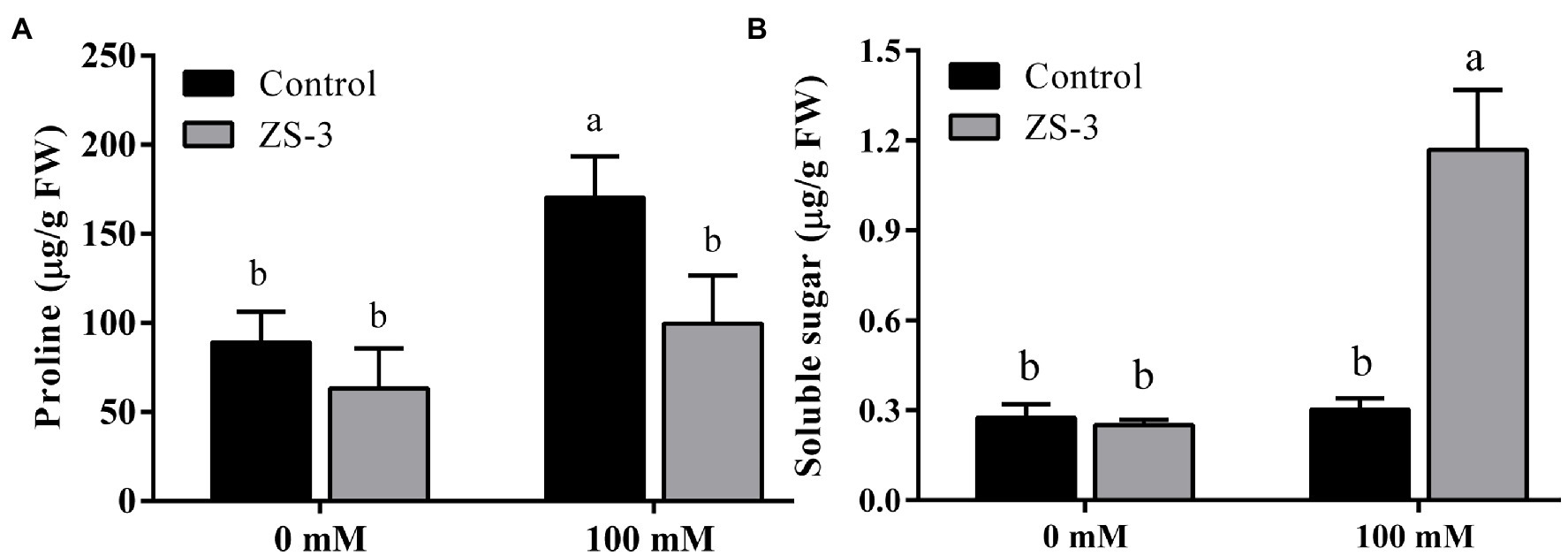
Figure 3. Effect of inoculation with ZS-3 on the proline content (A) and soluble sugar content (B) content of Arabidopsis thaliana. The results are the mean ± standard deviation from three independent experiments. Different lowercase letters above the bars represent significant differences based on one-way ANOVA (Duncan’s multiple range, p < 0.05).
ZS-3 Mediates Ionic Homeostasis in Plants
To reveal the effect of inoculation with strain ZS-3 on the major ions of Col-0 seedlings under NaCl stress, K+ and Na+ levels were measured. The salt stress treatment induced significantly higher Na+ content in the leaves, roots and whole plants than the treatment without salt stress (Figure 4A). Na+ accumulation in whole plants and leaves was reduced by 51.64% and 44.17%, respectively, in the ZS-3-treated group compared to the control, while the Na+ content in plant roots was not significantly affected. In the presence or absence of salt stress, ZS-3 significantly reduced the K + content of plant leaves compared to the uninoculated treatment (Figure 4B). In addition, the K+/Na+ ratio is considered an indicator of the balance between K+ and Na+ uptake by plants. In the absence of salt stress, ZS-3 significantly increased the K+/Na+ ratio in the shoots and roots of the plants. In the presence or absence of salt stress, inoculation with ZS-3 increased the K+/Na+ ratio of whole plants by 66.59% and 40.42%, respectively, compared to the control (Figure 4C).
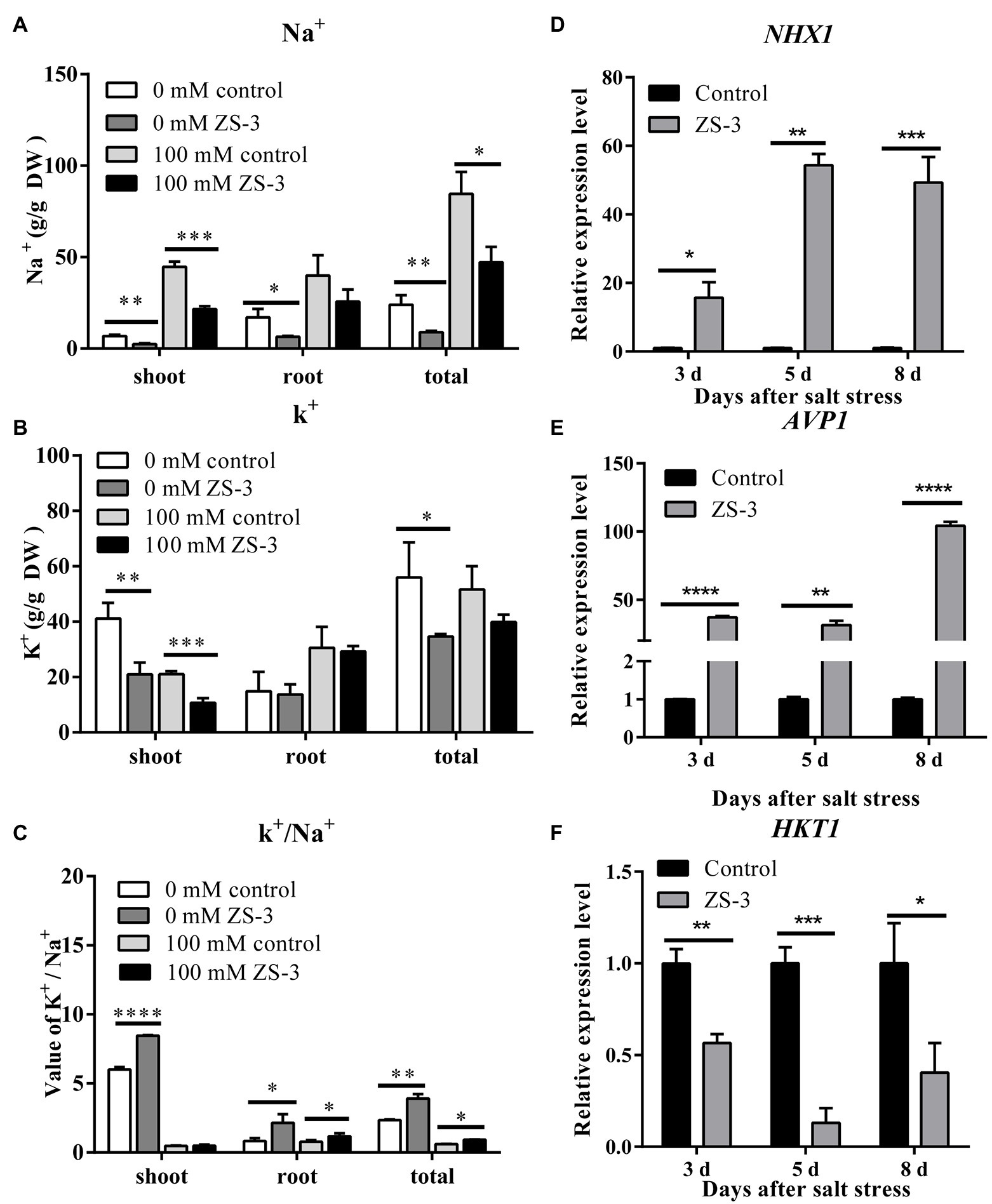
Figure 4. Effect of inoculation with ZS-3 on ion uptake in Arabidopsis thaliana. Na+ accumulation (A), K+ production (B), and the K+/Na+ ratio (C) in Arabidopsis thaliana roots and leaves were measured at 14 days after inoculation with strain ZS-3 in the absence or presence of 100 mM NaCl. The expression levels of the Arabidopsis thaliana defense-related genes NHX1 (D), AVP1 (E) and HKT1 (F) were quantified by qRT–PCR at days 3, 5 and 8 after inoculation with strain ZS-3. Arabidopsis thaliana inoculated with inactivated bacterial dilutions was used as a control. The results are the means ± standard deviations from three independent experiments. Asterisks indicate significant differences between treatments according to Student’s t-test (*p < 0.05; **p < 0.01; ***p < 0.001; ****p < 0.0001).
Ion transporters are known as terminal determinants of ionic homeostasis under salt-stressed conditions. ZS-3 inoculation markedly enhanced the expression levels of the NHX1 and AVP1 genes after day three under salt stress conditions compared to those in the noninoculated groups (Figures 4D,E). However, in contrast to the other two genes, HKT1 was negatively regulated in the ZS-3 treatment group on the 3rd day (Figure 4F).
ZS-3 Increases the Antioxidant Content of Plants
Antioxidant enzyme activity and nonenzymatic antioxidant levels in leaves were quantified at 10, 12 and 14 days to investigate the potential role of ZS-3 in the presence or absence of salt stress. Under the absence of salt stress, inoculation with ZS-3 did not cause higher POD and CAT activity than that in uninoculated plants, but inoculation with ZS-3 significantly increased GSH levels at 14 days (Figure 5C). Under salt stress conditions, CAT, POD and GSH contents were significantly increased in ZS-3-inoculated plants. Compared to the uninoculated treatment, the CAT activity of the bacteria-treated plants showed a continuous upward trend, being significantly increased by 82.68%, 44.83%, and 77.19% at 10, 12, and 14 days, respectively (Figure 5A). POD activity increased significantly by 31.53% compared to those of the control at 14 days (Figure 5B). GSH contents increased by 111% and 133% at 12 and 14 days, respectively, compared to the control levels (Figure 5C).
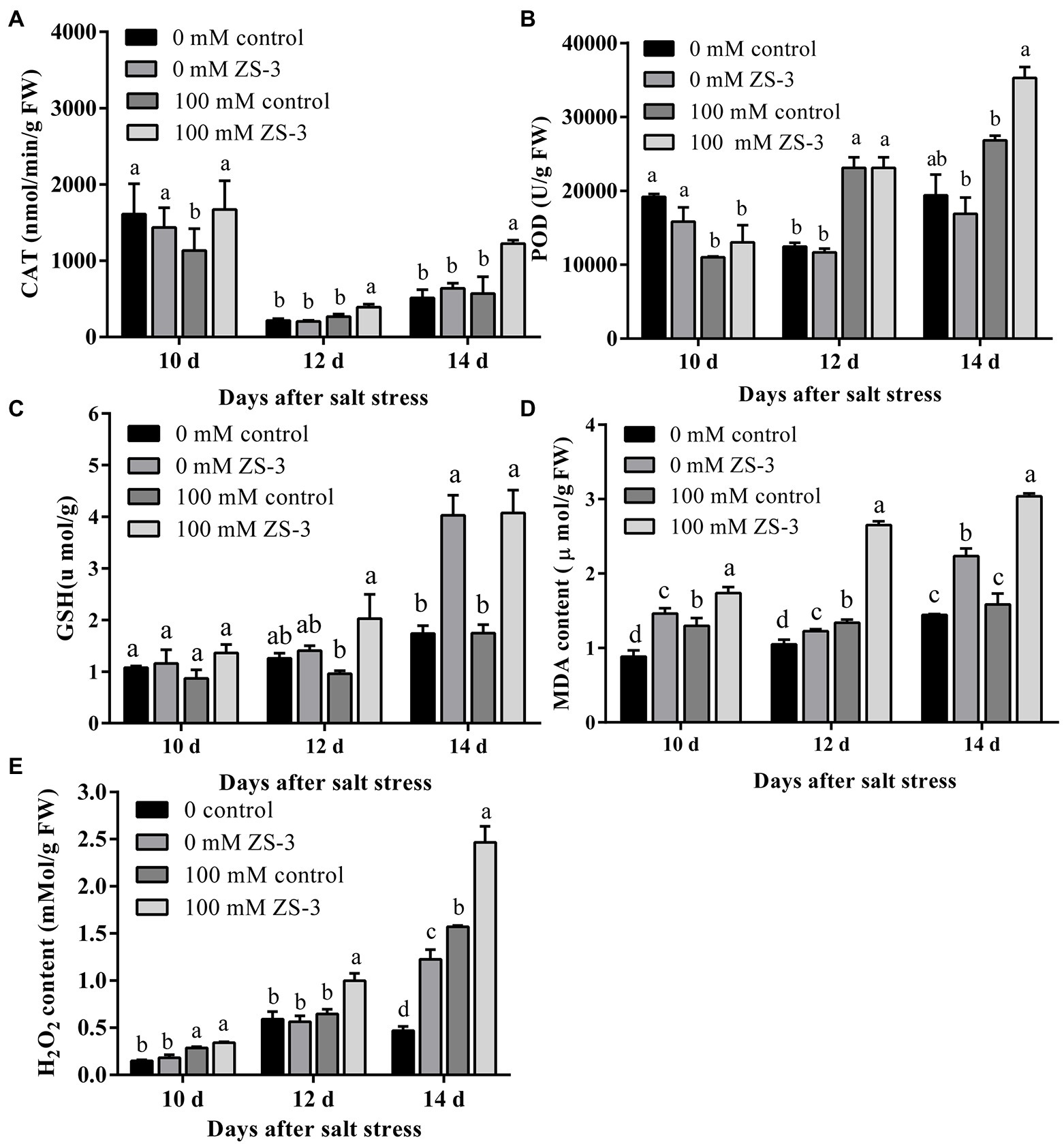
Figure 5. Effect of ZS-3 inoculation on antioxidant substances and ROS in Arabidopsis thaliana. CAT (A), POD (B), GSH (C), MDA (D), and H2O2 (E) were measured in Arabidopsis thaliana at 10, 12, and 14 days after inoculation with strain ZS-3 in the absence or presence of 100 mM NaCl. The results are the mean ± standard deviation of three independent experiments. Different lowercase letters on the bars represent significant differences between treatments based on one-way ANOVA (Duncan’s multiple range, p < 0.05).
However, in the presence or absence of salt stress, the contents of H2O2 and MDA were significantly increased in ZS-3-inoculated plants compared to those in noninoculated plants (Figures 5D,E). This would suggest that although ZS-3 enhanced plant antioxidant enzyme activity and nonenzymatic antioxidant levels, inoculation with the ZS-3 strain did not result in ROS scavenging and even exposed the plants to oxidative stress.
ZS-3 Activates the Systemic Defense Response in Plants
The expression of genes related to the SA and JA/ET pathways was examined after ZS-3 inoculation in A. thaliana, and the results showed that all three pathway-related genes examined were activated to varying degrees by the ZS-3 strain. In the JA pathway, AOS, a key gene for JA synthesis, showed an upward trend from days 3 to 8 (Figure 6A), while LOX2 was always downregulated (Figure 6B). ERF1, a transcription factor downstream of the ET signaling pathway, was upregulated (2.0-fold) at day 5, with the highest (18.2-fold) expression at day 8 (Figure 6C). PDF1.2, a critical gene in the JA/ET pathway, was significantly upregulated (54.4-fold) from day 5 onward (Figure 6D). In the SA pathway, the defense protein PR1 and the most critical receptor protein NPR1, which can be induced by SA, reached their highest expression simultaneously at day 8 (27.7-fold and 6.0-fold, respectively) (Figures 6E,F). Evidently, the SA and JA/ET pathways were all activated by strain ZS-3.
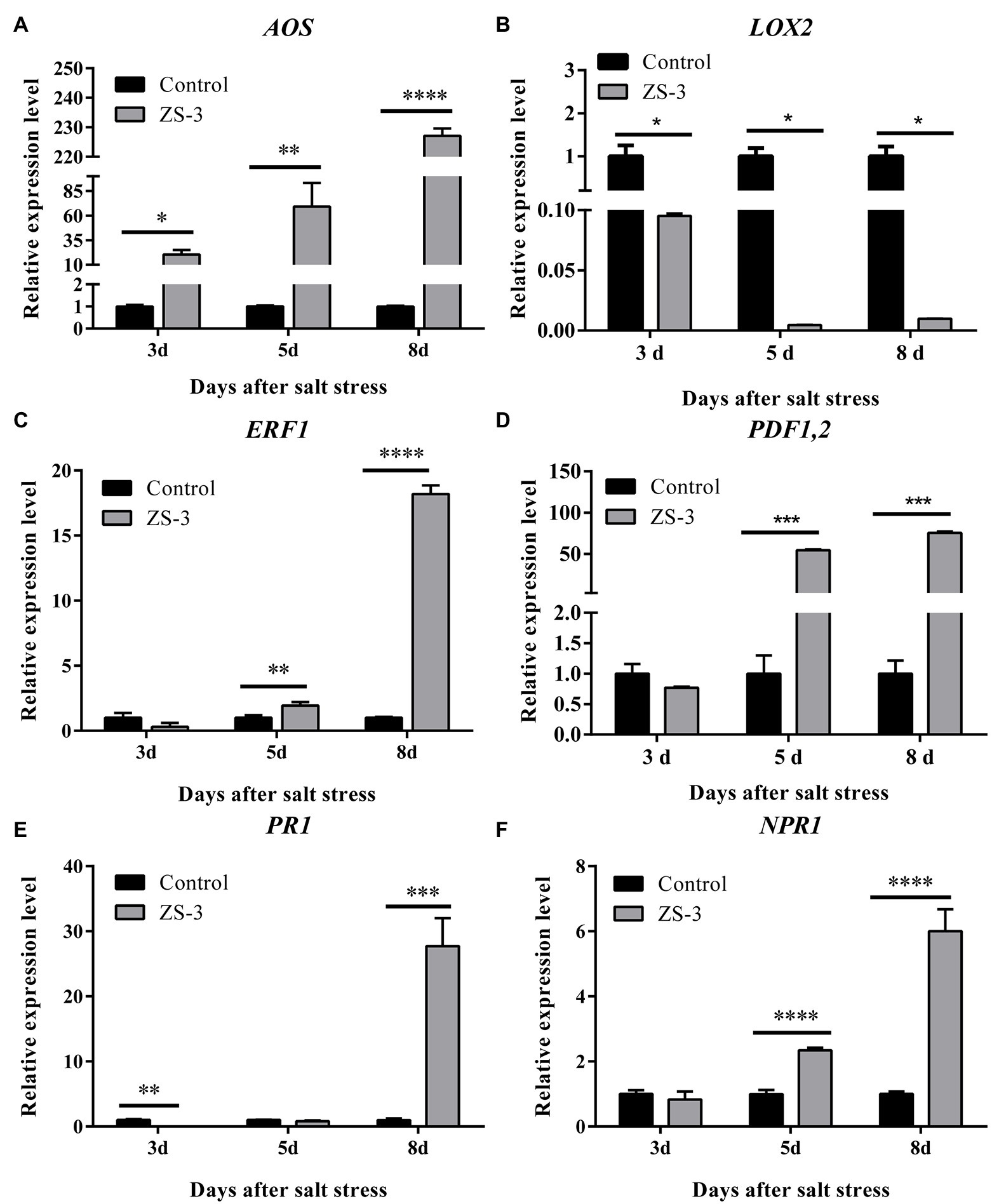
Figure 6. Real-time polymerase chain reaction (PCR) analysis of gene expression under salt stress. The expression levels of the Arabidopsis thaliana defense-related genes AOS (A), LOX2 (B), ERF1 (C), PDF1.2 (D), PR1 (E), and NPR1 (F) were quantified by qRT–PCR at days 3, 5 and 8 after inoculation with strain ZS-3. Arabidopsis thaliana inoculated with inactivated bacterial dilutions was used as a control. The results are the mean ± standard deviation of three independent experiments. Asterisks on the bars indicate significant differences between treatments based on t-test analysis (*p < 0.05; **p < 0.01; ***p < 0.001; ****p < 0.0001).
Discussion
High salinity causes osmotic stress, ion toxicity, oxidative damage, and nutrient imbalance in plants, which reduces photosynthetic rates, inhibits plant growth, and reduces plant yield and quality. There is growing evidence that PGPR release a variety of metabolites to promote plant growth and reduce the negative effects of salt stress (Abbas et al., 2019; Lucke et al., 2020a). This study evaluated the effect of inoculation with ZS-3 on the growth and development of plants under 100 mM NaCl stress. The growth of A. thaliana was significantly inhibited by salt stress, but inoculation with ZS-3 reduced the negative effects and significantly improved growth.
The dynamic modulation of root structure (RSA) can be considered one of the most important defense mechanisms for roots to resist stress (Postma and Lynch, 2011). A deep and robust root system facilitates plant uptake of nutrients from the soil (Zhou et al., 2016). In this study, plants inoculated with ZS-3 showed no significant promotion of primary root growth but had more lateral root formation than control plants. This is typically associated with growth hormone accumulation in plant roots (Wakeel et al., 2018; Wang et al., 2021). Bacillus megaterium WW1211 has been shown to inhibit the growth of primary roots by modification of the auxin cycle and auxin redistribution in the root meristem (Wang et al., 2021). However, the mechanism of growth promotion is different among different isolates of B. megaterium (Wang et al., 2021). The possibility that polyamines improve the RSA of plants cannot be ruled out. Polyamines control cell division and differentiation in plants (Wu et al., 2012), playing an important role in primary root, lateral root and adventitious root development (Wu et al., 2012). Plants inoculated with Spd-producing BOFC15 had longer primary roots and more lateral roots (Zhou et al., 2016). In contrast to BOFC15, ZS-3 not only produces spermidine but also produces putrescine (unpublished). The altered root architecture may be attributed to the comediation of multiple metabolites by ZS-3.
Chlorophyll content is a key predictor of both plant health and photosynthetic tolerance to NaCl stress (Li et al., 2010; Heidari, 2011). The chlorophyll content in the leaves of A. thaliana was reduced under salt stress. The color of plant leaves was darker under the treatment with strain ZS-3, together with increased photosynthesis in the plants. This is directly related to the increase in chlorophyll content. Chlorophyll, which is usually responsible for green color in plants, is an important pigment for converting light into chemical energy (Kang et al., 2019). In transgenic lines with dark green leaves, the chlorophyll content was significantly higher than that in the wild type (Yang et al., 2011). In addition, other possible reasons for the darkening of leaf color were induction by the VOCs of ZS-3. Compared with the control, the ZS-3-treated leaves all showed a darker green color in the presence and absence of salt stress (Supplementary Figure S1A).
The accumulation of osmotic regulators maintains intracellular osmotic pressure homeostasis, which is one of the important mechanisms of plant tolerance to salt stress (Abbas et al., 2019). Soluble sugars are one of the key substances in plant osmoregulation (Zang et al., 2019). The results showed that ZS-3 promoted the accumulation of soluble sugars in plants under salt stress. Many studies have confirmed that PGPR promote the accumulation of soluble sugars and proline in plants and maintain the water status of cells to resist salinity-induced osmotic stress. For example, inoculation with Kocuria rhizophila Y1 increased the soluble sugar and proline contents of maize under NaCl stress (Li et al., 2020a). However, inoculation with ZS-3 significantly reduced the plant proline content. This is consistent with the results of studies such as Hmaeid et al. (2019) and Liu et al. (2020), where bacterial inoculation significantly reduced the proline content of plants under salt stress conditions. Plants inoculated with PGPR were not exposed to high salt stress and therefore accumulated less proline in the presence of PGPR (Shukla et al., 2012; Liu et al., 2020).
The control of Na + homeostasis is essential for maintaining normal plant growth under salt stress (Jiang et al., 2019). ZS-3 increases the K+/Na+ ratio by directly limiting the accumulation of Na+, rather than by increasing the K+ content, in the presence or absence of salt stress. This is closely related to the regulation of ion transport proteins by ZS-3. PGPR downregulate HKT1 expression and limit Na+ uptake by roots, thereby improving plant salt tolerance (Pinedo et al., 2015; Liu et al., 2020). In addition, segregation of Na+ from the cytoplasm to vesicles is one of the key strategies for mitigating Na+ toxicity in plant cells. AVP1 encodes a vacuolar H+-pyrophosphatase that pumps H+ into vesicles, which acidify to generate an H+ gradient across the membrane. NHX1 pumps Na+ into the vesicle compartment to exploit the potential difference in H+, thus effectively reducing the toxic effect of Na+ (Rivandi et al., 2011). ZS-3 regulates HKT1, NHX1, and AVP1, so we speculate that ZS-3 restricts Na+ uptake and sequesters Na+ in plant vesicles, which is one of the effective strategies by which ZS-3 alleviates salt stress in plants. Another possible strategy is that ZS-3 phagocytoses Na+ from the environment. A general consensus is that high external Na+ concentrations interfere with the ability of plants to access K+ due to competition between Na+ and K+ for the major binding sites of K+ channels or transport proteins (Wang et al., 2020). This is one of the pathways by which PGPR alleviate ionic stress in plants by reducing plant Na+ accumulation or increasing K+ uptake, thereby increasing the K+/Na+ ratio (Gong et al., 2020). However, plants inoculated with ZS-3 showed less Na+ than uninoculated plants in the absence of salt stress. This indicates that ZS-3 has an additional mechanism to limit plant Na+ accumulation. The concentrations of intracellular and extracellular Na+ were lower in Rahnella aquatilis JZ-GX1 inoculated with LB liquid medium (CK) under salt stress, indicating that the bacteria can consume Na+ in the medium (Li et al., 2021). ZS-3 is a salt-tolerant strain that tolerates 8% salt in LB, corresponding to moderate salt stress (Lu et al., 2018). The salt tolerance mechanism of the ZS-3 strain may also play an important role in the process of interaction with plants.
Antioxidant enzyme systems and non-enzymatic antioxidants are important defense systems that effectively mitigate the oxidative stress of plants under environmental stress (Gill and Tuteja, 2010). ZS-3 increased the contents of POD, CAT, and GSH under salt stress. POD and CAT reduce H2O2 to H2O and O2 (Nie et al., 2019). GSH is a potential quencher of ROS in organisms (Zheng et al., 2020). Interestingly, ZS-3 did not reduce the MDA and H2O2 contents of plants but instead promoted the accumulation of MDA and H2O2. Although the accumulation of ROS affects many cellular functions, it is noteworthy that whether ROS exert destructive, protective or signaling effects depends on the delicate balance of ROS production and scavenging at the right site and time (Gill and Tuteja, 2010). Since the plants showed excellent resistance to salt stress by interacting with ZS-3, we hypothesized that the accumulation of ROS might have been due to the excessive accumulation of bacteria and their metabolites in the medium at the late growth stages. Consistent with our speculation, there was no excessive accumulation of ROS in plants treated with VOC of ZS-3 (Supplementary Figure S1B). The high concentration of nutrients caused an additional burden on the plants, which could be one of the reasons for the excessive accumulation of ROS. The regulation of salt tolerance in plants by ZS-3 may not depend on the mechanism of action of antioxidant enzymes to scavenge ROS.
Three plant hormones, SA, JA, and ET, regulate plant responses to a range of biotic and abiotic stresses, as well as plant growth and development (Dar et al., 2015; Niu et al., 2016). Transcriptomics has shown that PGPR mediate plant tolerance to salt stress through activation of the JA/ET signaling pathway (Liu et al., 2017). Moreover, the transcriptome data show that all 20 genes encoding PR proteins were upregulated under 100 mM NaCl stress (Liu et al., 2017). Consistent with our results, the three defense signaling pathways of JA/ET and SA in Arabidopsis were activated by ZS-3. The results indicate that IST of Arabidopsis may be activated by ZS-3 in response to salt stress.
In addition, several studies have shown that PGPR alter the epigenetic characteristics of host plants to provide beneficial selectable variation and regulate crosstalk between phytohormones (Tiwari et al., 2021). Evidence supports the coordinated role of the Arabidopsis transcription factor ERF1 in integrating JA and ET signaling (Dimopoulou et al., 2019). We found that the JA signaling pathway was first regulated by ZS-3 (3d). ZS-3 then integrated the JA/ET signaling pathway by upregulating ERF1 expression (5 days; Lucke et al., 2020b). At the same time, the transcript levels of PDF1.2 were upregulated (5 days). JA/ET signaling induces PDF1.2, and SA signaling upregulates PR1; these two processes show an antagonistic relationship when responding to biotic stresses (Sooklal et al., 2020). However, it has been reported that NPR1 has a role in fine-tuning the antagonistic regulation of SA and JA/ET signaling to control gene expression (Zhang et al., 2019). Our results indicate that PR1 gene (8 days) expression is upregulated following activation of the NPR1 gene (5d) by ZS-3. In other words, the SA signaling pathway in A. thaliana is well-prepared to cope with salt stress. However, PR1 was downregulated in the early stage (3 days). This implies that SA may be antagonized by JA/ET in early signaling crosstalk but undergoes dynamic changes at a later stage. What activates their coordinated action needs to be further investigated. Crosstalk between plant hormones can reprogram genetic mechanisms to influence defense responses and minimize stress effects, thereby improving stress tolerance in plants (Per et al., 2018). Although the physiological and molecular mechanisms of PGPR-plant interactions have been reported in many studies, little is known about the interaction of proteins and small molecules from beneficial microorganisms with different branches of the plant immune system (Lucke et al., 2020b). Further insights are needed in the future to reveal which metabolites trigger plant defense responses. A large number of metabolites associated with different plant hormones play a role in plant defense and plant-environment interactions (Lucke et al., 2020b). In addition, further studies are needed to evaluate the effectiveness of ZS-3 in mitigating salt stress in crops under field conditions.
Conclusion
In this study, ZS-3 was found to promote plant growth under salt stress. The improvement in the growth of A. thaliana induced by ZS-3 can be attributed to the integration of multiple physiological processes, including regulation of photosynthetic pigment synthesis (chlorophyll and carotenoids), accumulation of osmotic regulators (soluble sugars), increases in antioxidant substances (CAT, POD, GSH), or activation of ion transporter gene expression (NHX1, AVP1, and HKT1), which led to an increased ratio of K+/Na+ (Figure 7). In addition, the transcript levels of defense response genes related to the SA and JA/ET synthesis pathways (NPR1, PR1, ERF1, PDF1.2, LOX2, and AOS) were differentially activated, which helped to improve the salt tolerance of A. thaliana. In summary, this report shows that ZS-3 mitigates the negative effects of salt stress on plants. We demonstrate that inoculation with ZS-3 is a valuable and eco-friendly biotechnological approach to improve agricultural practices and production under saline conditions. The results of this study provide new insights into the induction of salinity tolerance by PGPR, and in the next step of our research, we will determine which substances of ZS-3 induce systemic salinity tolerance and activate systemic tolerance in plants.
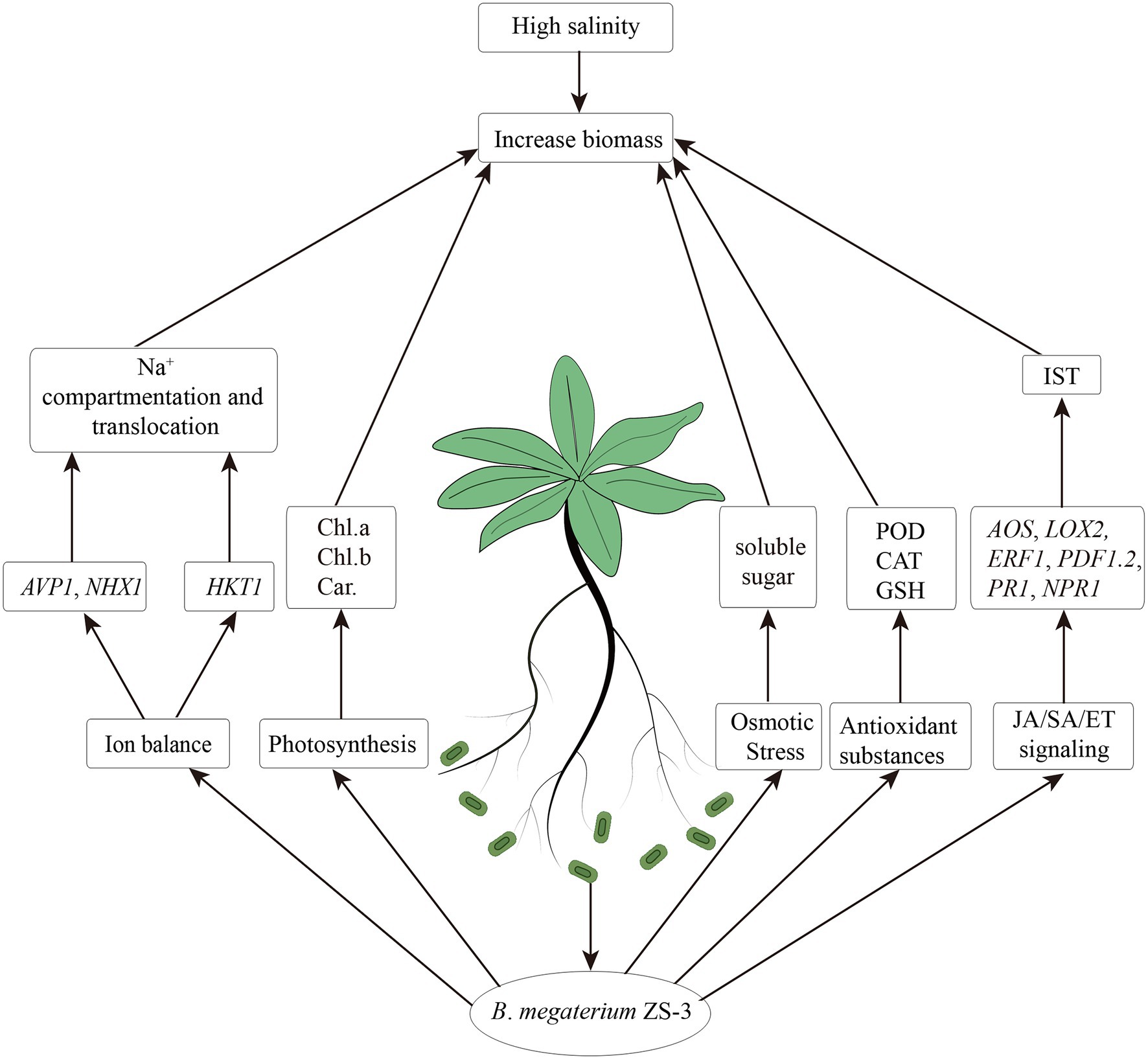
Figure 7. Possible mechanisms by which Bacillus megaterium strain ZS-3 mediates salt stress tolerance in Arabidopsis thaliana under salt stress.
Data Availability Statement
The original contributions presented in the study are included in the article/Supplementary Material, and further inquiries can be directed to the corresponding author.
Author Contributions
L-NS and J-RY conceived and designed the experiments. L-XL provided valuable advice on data analysis and the manuscript. H-MS assisted with the statistical analysis. L-NS analyzed the data and drafted the manuscript. J-RY revised the manuscript. All authors contributed to the article and approved the submitted version.
Funding
This work was financially supported by the Science and Technology Project of Shanghai Greening Bureau (no. G191208), the Chinese State Forestry Administration Special Research Program for Forestry Sectors Beneficial to Public (no. 201304404), and the Priority Academic Program Development of Jiangsu Higher Education Institutions (PAPD).
Conflict of Interest
The authors declare that the research was conducted in the absence of any commercial or financial relationships that could be construed as a potential conflict of interest.
Publisher’s Note
All claims expressed in this article are solely those of the authors and do not necessarily represent those of their affiliated organizations, or those of the publisher, the editors and the reviewers. Any product that may be evaluated in this article, or claim that may be made by its manufacturer, is not guaranteed or endorsed by the publisher.
Supplementary Material
The Supplementary Material for this article can be found online at: https://www.frontiersin.org/articles/10.3389/fpls.2022.820837/full#supplementary-material
Supplementary Figure S1 | Effect of volatiles gases (VOC) from ZS-3 on phenotypic (A) and MDA content (B) in A. thaliana. Six-day-old seedlings were transplanted and cultured in dichotomous dishes for 14 days. VOC from ZS-3 exhibited a significant pro-growth effect on plants and induced dark green leaves in the presence or absence of salt stress. The MDA content of A. thaliana was measured at 14 d. Control: PBS inoculation only, ZS-3: bacterial inoculation, Voc-ZS-3: VOCs inoculation. The results are the mean ± standard deviation of three independent experiments. Different lowercase letters on the bars represent significant differences between treatments, based on one-way ANOVA (Duncan’s multiple range, p < 0.05).
References
Abbas, R., Rasul, S., Aslam, K., Baber, M., Shahid, M., Mubeen, F., et al. (2019). Halotolerant PGPR: A hope for cultivation of saline soils. J. King Saud. Univ. Sci. 31, 1195–1201. doi: 10.1016/j.jksus.2019.02.019
Azarmi, F., Mozafari, V., Abbaszadeh Dahaji, P., and Hamidpour, M. (2015). Biochemical, physiological and antioxidant enzymatic activity responses of pistachio seedlings treated with plant growth promoting rhizobacteria and Zn to salinity stress. Acta Physiol. Plant. 38:21. doi: 10.1007/s11738-015-2032-3
Bates, L. S., Waldren, R. P., and Teare, I. D. (1973). Rapid determination of free proline for water-stress studies. Plant Soil 39, 205–207. doi: 10.1007/BF00018060
Dar, T. A., Uddin, M., Khan, M. M. A., Hakeem, K. R., and Jaleel, H. (2015). Jasmonates counter plant stress: a review. Environ. Exp. Bot. 115, 49–57. doi: 10.1016/j.envexpbot.2015.02.010
Dimopoulou, A., Theologidis, I., Liebmann, B., Kalantidis, K., Vassilakos, N., and Skandalis, N. (2019). Bacillus amyloliquefaciens MBI600 differentially induces tomato defense signaling pathways depending on plant part and dose of application. Sci. Rep. 9, 19120–19120. doi: 10.1038/s41598-019-55645-2
Etesami, H., and Alikhani, H. A. (2016). Rhizosphere and endorhiza of oilseed rape (Brassica napus L.) plant harbor bacteria with multifaceted beneficial effects. Biol. Control 94, 11–24. doi: 10.1016/j.biocontrol.2015.12.003
Fu, H., Chen, F., Liu, W., Kong, W., Wang, C., Fang, X., et al. (2020). Adding nutrients to the biocontrol strain JK-SH007 promotes biofilm formation and improves resistance to stress. AMB Express 10, 32. doi: 10.1186/s13568-019-0929-8
Gill, S. S., and Tuteja, N. (2010). Reactive oxygen species and antioxidant machinery in abiotic stress tolerance in crop plants. Plant Physiol. Biochem. 48, 909–930. doi: 10.1016/j.plaphy.2010.08.016
Gong, Y., Chen, L.-J., Pan, S.-Y., Li, X.-W., Xu, M.-J., Zhang, C.-M., et al. (2020). Antifungal potential evaluation and alleviation of salt stress in tomato seedlings by a halotolerant plant growth-promoting actinomycete Streptomyces sp. KLBMP5084. Rhizosphere 16:100262. doi: 10.1016/j.rhisph.2020.100262
Heidari, M. (2011). Effects of salinity stress on growth, chlorophyll content and osmotic components of two basil (Ocimum basilicum L.) genotypes. Afr. J. Biotechnol. 11, 379–384. doi: 10.5897/AJB11.2572
Hmaeid, N., Wali, M., Metoui-Ben Mahmoud, O., Pueyo, J. J., Ghnaya, T., and Abdelly, C. (2019). Efficient rhizobacteria promote growth and alleviate NaCl-induced stress in the plant species Sulla carnosa. Appl. Soil Ecol. 133, 104–113. doi: 10.1016/j.apsoil.2018.09.011
Ilangumaran, G., and Smith, D. L. (2017). Plant growth promoting Rhizobacteria in amelioration of salinity stress: A systems biology perspective. Front. Plant Sci. 8:1768. doi: 10.3389/fpls.2017.01768
Jiang, J.-L., Tian, Y., Li, L., Yu, M., Hou, R.-P., and Ren, X.-M. (2019). H(2)S alleviates salinity stress in cucumber by maintaining the Na(+)/K(+) balance and regulating H(2)S metabolism and oxidative stress response. Front. Plant Sci. 10:678. doi: 10.3389/fpls.2019.00678
Kang, S.-M., Shahzad, R., Bilal, S., Khan, A. L., Park, Y.-G., Lee, K.-E., et al. (2019). Indole-3-acetic-acid and ACC deaminase producing Leclercia adecarboxylata MO1 improves Solanum lycopersicum L. growth and salinity stress tolerance by endogenous secondary metabolites regulation. BMC Microbiol. 19:80. doi: 10.1186/s12866-019-1450-6
Khan, N., Bano, A., Ali, S., and Babar, M. A. (2020). Crosstalk amongst phytohormones from planta and PGPR under biotic and abiotic stresses. Plant Growth Regul. 90, 189–203. doi: 10.1007/s10725-020-00571-x
Kumar Arora, N., Fatima, T., Mishra, J., Mishra, I., Verma, S., Verma, R., et al. (2020). Halo-tolerant plant growth promoting rhizobacteria for improving productivity and remediation of saline soils. J. Adv. Res. 26, 69–82. doi: 10.1016/j.jare.2020.07.003
Kumar, D., Yusuf, M. A., Singh, P., Sardar, M., and Sarin, N. B. (2013). Modulation of antioxidant machinery in α-tocopherol-enriched transgenic Brassica juncea plants tolerant to abiotic stress conditions. Protoplasma 250, 1079–1089. doi: 10.1007/s00709-013-0484-0
Kumawat, K. C., Nagpal, S., and Sharma, P. (2022). Potential of plant growth-promoting rhizobacteria-plant interactions in mitigating salt stress for sustainable agriculture: a review. Pedosphere 32, 223–245. doi: 10.1016/S1002-0160(21)60070-X
Li, G., Wan, S., Zhou, J., Yang, Z., and Qin, P. (2010). Leaf chlorophyll fluorescence, hyperspectral reflectance, pigments content, malondialdehyde and proline accumulation responses of castor bean (Ricinus communis L.) seedlings to salt stress levels. Ind. Crop. Prod. 31, 13–19. doi: 10.1016/j.indcrop.2009.07.015
Li, P.-S., Kong, W.-L., and Wu, X.-Q. (2021). Salt tolerance mechanism of the Rhizosphere bacterium JZ-GX1 and its effects on tomato seed germination and seedling growth. Front. Microbiol. 12:657238. doi: 10.3389/fmicb.2021.657238
Li, X., Sun, P., Zhang, Y., Jin, C., and Guan, C. (2020a). A novel PGPR strain Kocuria rhizophila Y1 enhances salt stress tolerance in maize by regulating phytohormone levels, nutrient acquisition, redox potential, ion homeostasis, photosynthetic capacity and stress-responsive genes expression. Environ. Exp. Bot. 174:104023. doi: 10.1016/j.envexpbot.2020.104023
Li, Y., Hu, L.-J., Wu, X.-Q., and Ye, J.-R. (2020b). A Bursaphelenchus xylophilus effector, Bx-FAR-1, suppresses plant defense and affects nematode infection of pine trees. Eur. J. Plant Pathol. 157, 637–650. doi: 10.1007/s10658-020-02031-8
Liu, S., Hao, H., Lu, X., Zhao, X., Wang, Y., Zhang, Y., et al. (2017). Transcriptome profiling of genes involved in induced systemic salt tolerance conferred by Bacillus amyloliquefaciens FZB42 in Arabidopsis thaliana. Sci. Rep. 7, 10795. doi: 10.1038/s41598-017-11308-8
Liu, S., Tian, Y., Jia, M., Lu, X., Yue, L., Zhao, X., et al. (2020). Induction of salt tolerance in Arabidopsis thaliana by volatiles from Bacillus amyloliquefaciens FZB42 via the jasmonic acid signaling pathway. Front. Microbiol. 11:562934. doi: 10.3389/fmicb.2020.562934
Lu, L., Jiang, M., Wang, Y., Zhang, Y., Zhang, H., and Ye, J. (2018). Isolation, screening and identification of endophytic bacteria from Cihhamomum camphoYa that promote growth and antagonistic pathogen. J. Nanjing Univ. Nat. Sci. 42, 128–136. doi: 10.3969/j.issn.1000-2006.201802022
Lucke, M., Correa, M. G., and Levy, A. (2020a). The role of secretion systems, effectors, and secondary metabolites of beneficial Rhizobacteria in interactions with plants and microbes. Front. Plant Sci. 11:589416. doi: 10.3389/fpls.2020.589416
Lucke, M., Correa, M. G., and Levy, A. (2020b). The role of secretion systems, effectors, and secondary metabolites of beneficial Rhizobacteria in interactions With plants and microbes. Front. Plant Sci. 11:589416. doi: 10.3389/fpls.2020.589416
Nie, Z., Wan, C., Chen, C., and Chen, J. (2019). Comprehensive evaluation of the postharvest antioxidant capacity of Majiayou Pomelo harvested at different maturities based on PCA. Antioxidants 8:136. doi: 10.3390/antiox8050136
Niu, D., Wang, X., Wang, Y., Song, X., Wang, J., Guo, J., et al. (2016). Bacillus cereus AR156 activates PAMP-triggered immunity and induces a systemic acquired resistance through a NPR1-and SA-dependent signaling pathway. Biochem. Biophys. Res. Commun. 469, 120–125. doi: 10.1016/j.bbrc.2015.11.081
Per, T. S., Khan, M. I. R., Anjum, N. A., Masood, A., Hussain, S. J., and Khan, N. A. (2018). Jasmonates in plants under abiotic stresses: crosstalk with other phytohormones matters. Environ. Exp. Bot. 145, 104–120. doi: 10.1016/j.envexpbot.2017.11.004
Pinedo, I., Ledger, T., Greve, M., and Poupin, M. J. (2015). Burkholderia phytofirmans PsJN induces long-term metabolic and transcriptional changes involved in Arabidopsis thaliana salt tolerance. Front. Plant Sci. 6:466. doi: 10.3389/fpls.2015.00466
Postma, J. A., and Lynch, J. P. (2011). Root cortical aerenchyma enhances the growth of maize on soils with suboptimal availability of nitrogen, phosphorus, and potassium. Plant Physiol. 156, 1190–1201. doi: 10.1104/pp.111.175489
Qian, T., and Ye, J. (2020). The mechanism of dissolving inorganic phosphorus by bacillus megaterium ZS-3 and its growth promotion of Ciuuamomum camphora. Biotechnol. Bull. 36, 45–52. doi: 10.13560/j.cnki.biotech.bull.1985.2020-0114
Qin, Y., Druzhinina, I. S., Pan, X., and Yuan, Z. (2016). Microbially mediated plant salt tolerance and microbiome-based solutions for saline agriculture. Biotechnol. Adv. 34, 1245–1259. doi: 10.1016/j.biotechadv.2016.08.005
Rady, M. M., Kuşvuran, A., Alharby, H. F., Alzahrani, Y., and Kuşvuran, S. (2019). Pretreatment with proline or an organic bio-stimulant induces salt tolerance in wheat plants by improving antioxidant redox state and enzymatic activities and reducing the oxidative stress. J. Plant Growth Regul. 38, 449–462. doi: 10.1007/s00344-018-9860-5
Rivandi, J., Miyazaki, J., Hrmova, M., Pallotta, M., Tester, M., and Collins, N. C. (2011). A SOS3 homologue maps to HvNax4, a barley locus controlling an environmentally sensitive Na+ exclusion trait. J. Exp. Bot. 62, 1201–1216. doi: 10.1093/jxb/erq346
Shahid, M., Ameen, F., Maheshwari, H. S., Ahmed, B., AlNadhari, S., and Khan, M. S. (2021). Colonization of Vigna radiata by a halotolerant bacterium Kosakonia sacchari improves the ionic balance, stressor metabolites, antioxidant status and yield under NaCl stress. Appl. Soil Ecol. 158:103809. doi: 10.1016/j.apsoil.2020.103809
Showmy, K. S., and Yusuf, A. (2020). Characterization of disease resistance in nine traditional rice (Oryza sativa L.) cultivars and expression of chennellu PR1 gene in response to Xanthomonas oryzae pv. Oryzae. Indian Phytopathol. 73, 281–291. doi: 10.1007/s42360-020-00220-3
Shukla, P. S., Agarwal, P. K., and Jha, B. (2012). Improved salinity tolerance of Arachishypogaea (L.) by the interaction of halotolerant plant-growth-promoting Rhizobacteria. J. Plant Growth Regul. 31, 195–206. doi: 10.1007/s00344-011-9231-y
Singh, A. (2021). Soil salinization management for sustainable development: a review. J. Environ. Manag. 277:111383. doi: 10.1016/j.jenvman.2020.111383
Sooklal, S. A., Mpangase, P. T., Tomescu, M.-S., Aron, S., Hazelhurst, S., Archer, R. H., et al. (2020). Functional characterisation of the transcriptome from leaf tissue of the fluoroacetate-producing plant, Dichapetalum cymosum, in response to mechanical wounding. Sci. Rep. 10:20539. doi: 10.1038/s41598-020-77598-7
Takeuchi, K., Gyohda, A., Tominaga, M., Kawakatsu, M., Hatakeyama, A., Ishii, N., et al. (2011). RSOsPR10 expression in response to environmental stresses is regulated antagonistically by jasmonate/ethylene and salicylic acid signaling pathways in rice roots. Plant Cell Physiol. 52, 1686–1696. doi: 10.1093/pcp/pcr105
Timmermann, T., Armijo, G., Donoso, R., Seguel, A., Holuigue, L., and González, B. (2017). Paraburkholderia phytofirmans PsJN protects Arabidopsis thaliana Against a virulent strain of Pseudomonas syringae through the activation of induced resistance. Mol. Plant-Microbe Interact. 30, 215–230. doi: 10.1094/mpmi-09-16-0192-r
Tiwari, S., Gupta, S. C., Chauhan, P. S., and Lata, C. (2021). An OsNAM gene plays important role in root rhizobacteria interaction in transgenic Arabidopsis through abiotic stress and phytohormone crosstalk. Plant Cell Rep. 40, 143–155. doi: 10.1007/s00299-020-02620-1
Wakeel, A., Ali, I., Upreti, S., Azizullah, A., Liu, B., Khan, A. R., et al. (2018). Ethylene mediates dichromate-induced inhibition of primary root growth by altering AUX1 expression and auxin accumulation in Arabidopsis thaliana. Plant Cell Environ. 41, 1453–1467. doi: 10.1111/pce.13174
Wang, P., Wang, C.-M., Gao, L., Cui, Y.-N., Yang, H.-L., de Silva, N. D. G., et al. (2020). Aliphatic suberin confers salt tolerance to Arabidopsis by limiting Na+ influx, K+ efflux and water backflow. Plant Soil 448, 603–620. doi: 10.1007/s11104-020-04464-w
Wang, S., Na, X., Yang, L., Liang, C., He, L., Jin, J., et al. (2021). Bacillus megaterium strain WW1211 promotes plant growth and lateral root initiation via regulation of auxin biosynthesis and redistribution. Plant Soil 466, 491–504. doi: 10.1007/s11104-021-05055-z
Wolf, B. (1982). A comprehensive system of leaf analyses and its use for diagnosing crop nutrient status. Commun. Soil Sci. Plant Anal. 13, 1035–1059. doi: 10.1080/00103628209367332
Wu, Q.-S., He, X.-H., Zou, Y.-N., Liu, C.-Y., Xiao, J., and Li, Y. (2012). Arbuscular mycorrhizas alter root system architecture of citrus tangerine through regulating metabolism of endogenous polyamines. Plant Growth Regul. 68, 27–35. doi: 10.1007/s10725-012-9690-6
Yang, M., Yang, Q., Fu, T., and Zhou, Y. (2011). Overexpression of the brassica napusBnLAS gene in Arabidopsis affects plant development and increases drought tolerance. Plant Cell Rep. 30, 373–388. doi: 10.1007/s00299-010-0940-7
Zang, D., Wang, J., Zhang, X., Liu, Z., and Wang, Y. (2019). Arabidopsis heat shock transcription factor HSFA7b positively mediates salt stress tolerance by binding to an E-box-like motif to regulate gene expression. J. Exp. Bot. 70, 5355–5374. doi: 10.1093/jxb/erz261
Zelm, E. V., Zhang, Y., and Testerink, C. (2020). Salt tolerance mechanisms of plants. Annu. Rev. Plant Biol. 71, 403–433. doi: 10.1146/annurev-arplant-050718-100005
Zhang, X., Xu, Z., Chen, L., and Ren, Z. (2019). Comprehensive analysis of multiprotein bridging factor 1 family genes and SlMBF1c negatively regulate the resistance to Botrytis cinerea in tomato. BMC Plant Biol. 19, 437–437. doi: 10.1186/s12870-019-2029-y
Zheng, Q., Chen, Y., Jia, X., Wang, Y., Wu, T., Xu, X., et al. (2020). MicroRNA156 (miR156) negatively impacts mg-Protoporphyrin IX (mg-proto IX) biosynthesis and its plastid-nucleus retrograde signaling in apple. Plants 9, 653. doi: 10.3390/plants9050653
Keywords: salt stress, Bacillus megaterium, salicylic acid, PGPR, ion regulation
Citation: Shi L-N, Lu L-X, Ye J-R and Shi H-M (2022) The Endophytic Strain ZS-3 Enhances Salt Tolerance in Arabidopsis thaliana by Regulating Photosynthesis, Osmotic Stress, and Ion Homeostasis and Inducing Systemic Tolerance. Front. Plant Sci. 13:820837. doi: 10.3389/fpls.2022.820837
Edited by:
Juan Manuel Ruiz-Lozano, Spanish National Research Council (CSIC), SpainReviewed by:
Shalini Tiwari, Jawaharlal Nehru University, IndiaSunita Varjani, Gujarat Pollution Control Board (GPCB), India
Copyright © 2022 Shi, Lu, Ye and Shi. This is an open-access article distributed under the terms of the Creative Commons Attribution License (CC BY). The use, distribution or reproduction in other forums is permitted, provided the original author(s) and the copyright owner(s) are credited and that the original publication in this journal is cited, in accordance with accepted academic practice. No use, distribution or reproduction is permitted which does not comply with these terms.
*Correspondence: Jian-Ren Ye, anJ5ZUBuamZ1LmVkdS5jbg==