- 1Department of Biotechnology, School of Life Sciences, Central University of Kashmir, Ganderbal, India
- 2Centre of Research for Development, University of Kashmir, Srinagar, India
- 3Department of Biotechnology, School of Biosciences and Biotechnology, Baba Ghulam Shah Badshah University, Rajouri, India
- 4Key Laboratory of Ministry of Education for Genetics, Breeding and Multiple Utilization of Crops, Oil Crops Research Institute, Center of Legume Crop Genetics and Systems Biology/College of Agriculture, Fujian Agriculture and Forestry University (FAFU), Fuzhou, China
- 5Department of Chemistry, University of Kashmir, Srinagar, India
- 6State Key Laboratory of Rice Biology, China National Rice Research Institute, Chinese Academy of Agricultural Science, Hangzhou, China
- 7Department of Biology, Faculty of Applied Science, Umm Al-Qura University, Makkah, Saudi Arabia
- 8Division of Genetics and Plant Breeding, Sher-e-Kashmir University of Agricultural Sciences and Technology of Kashmir, Srinagar, India
- 9Proteomics Laboratory, Division of Plant Biotechnology, Sher-e-Kashmir University of Agricultural Sciences and Technology of Kashmir (SKUAST-K), Srinagar, India
Sustainable agricultural production is critically antagonistic by fluctuating unfavorable environmental conditions. The introduction of mineral elements emerged as the most exciting and magical aspect, apart from the novel intervention of traditional and applied strategies to defend the abiotic stress conditions. The silicon (Si) has ameliorating impacts by regulating diverse functionalities on enhancing the growth and development of crop plants. Si is categorized as a non-essential element since crop plants accumulate less during normal environmental conditions. Studies on the application of Si in plants highlight the beneficial role of Si during extreme stressful conditions through modulation of several metabolites during abiotic stress conditions. Phytohormones are primary plant metabolites positively regulated by Si during abiotic stress conditions. Phytohormones play a pivotal role in crop plants’ broad-spectrum biochemical and physiological aspects during normal and extreme environmental conditions. Frontline phytohormones include auxin, cytokinin, ethylene, gibberellin, salicylic acid, abscisic acid, brassinosteroids, and jasmonic acid. These phytohormones are internally correlated with Si in regulating abiotic stress tolerance mechanisms. This review explores insights into the role of Si in enhancing the phytohormone metabolism and its role in maintaining the physiological and biochemical well-being of crop plants during diverse abiotic stresses. Moreover, in-depth information about Si’s pivotal role in inducing abiotic stress tolerance in crop plants through metabolic and molecular modulations is elaborated. Furthermore, the potential of various high throughput technologies has also been discussed in improving Si-induced multiple stress tolerance. In addition, a special emphasis is engrossed in the role of Si in achieving sustainable agricultural growth and global food security.
Introduction
The reduction in crop productivity by 51–82% on a global scale is primarily influenced by abiotic stress factors, such as drought, salinity, heat, cold, toxic heavy metals, hypoxia and anoxia, waterlogging, and nutrient imbalance (Cooke and Leishman, 2016; Zahra et al., 2021; Raza et al., 2022). Contemporary climate change notably imparts drought and heat stress, two major abiotic stress factors resulting in crop loss and productivity (Zandalinas et al., 2018). For instance, studies report that about a 40% loss in yield in maize (Zea mays L.) and wheat (Triticum aestivum L.) is primarily due to drought stress (Daryanto et al., 2016). Therefore, it is evident that abiotic stress factors are the most severe constraints to global food production, leading to nutritional and food insecurity in the climate-changing era (Del Buono, 2021; Kamenya et al., 2021; Raza, 2022). The last few decades witnessed an increase in the prevalence of abiotic stressors primarily due to unpredictable weather changes induced by global climatic change (Del Buono, 2021; Kamenya et al., 2021; Raza, 2022). Abiotic stressors alter the homeostasis of the physiological, molecular, and biochemical setup of crop plants, such as water relations, nutrient uptake and assimilation, osmotic deregulation, loss of membrane integrity, impairment in enzyme activity, and the most notable decline in photosynthetic efficiency (Moradtalab et al., 2018; Ahanger et al., 2019; Raza, 2021; Raza et al., 2021e). Most of these impairments after exposure to the stress factors are associated with phytohormone production and their transport in underground and aerial parts of plants (Gangwar et al., 2014; Verma et al., 2016; Arif et al., 2021). Moreover, plants primarily respond to these abiotic stressors by producing reactive oxygen species (ROS), which, in turn, induce severe damage to the cellular structures, such as biomembranes and organelles (da Silva and Yücel, 2008; Hasanuzzaman et al., 2020).
To circumvent these harmful effects, plants respond through physiological and molecular mechanistic adjustments to mitigate the adverse impacts of abiotic stressors (Raza, 2021, 2022). Additionally, the defense response to these abiotic stressors includes synthesis of critical proteins linked to metabolism, activation of signal transduction, and regulatory pathways controlled by the expression of a large number of stress-tolerant genes, which, in turn, are regulated by diverse organic and inorganic molecules (da Silva and Yücel, 2008; Hasanuzzaman et al., 2020; Raza et al., 2021e). Among inorganic molecules, Si, formally categorized as the non-essential element, has been recently found to defend against the harmful effects of abiotic stressors in crop plants. The uptake of Si results in the activation of a diverse range of critical genes to mitigate stress conditions to regulate plant growth and development. Si is the second most abundant element found in the earth’s crust (Luyckx et al., 2017; Atta et al., 2019; Zargar et al., 2019). Si is crystalline in structure and forms at least two stable oxide forms, viz. Si monoxide (SiO) and silica (SiO2). Average soils contain about 28% Si by weight in silicates, alumina-silicates, and Si dioxide, most of which are unavailable to crop plants. Traces of monosilicic acid found in soil are the only form of Si available to plants (Pereira et al., 2013). A later form of Si is soluble in water and is weakly adsorbed by soil. The transport of Si into aerial parts of crop plants occurs through several specific and non-specific transporters. In several crop plants, such as maize, cucumber, rice, and barley; genes, such as Lsi2, Lsi1, and Lsi6, are responsible for uptake of Si in roots and aerial organs (Wang et al., 2015; Ouellette et al., 2017; Ratcliffe et al., 2017). Among these, Lsi1 and Lsi6 transporters belonging to aquaporins are abundantly distributed in shoot and root tissues, whereas Lsi2, an anion transporter, is found in membranes of root endodermis (Mitani et al., 2011).
Crop plants may grow well in the absence of Si, although in a few plants, such as rice and horsetail, the absence of Si may make them vulnerable to fungal infections (Law and Exley, 2011). Si has been reported to play numerous roles in mitigating abiotic stress conditions (Coskun et al., 2016; Luyckx et al., 2017; Rasoolizadeh et al., 2018; Zhu et al., 2019a; Alamri et al., 2020; Salim et al., 2021). For instance, Si mediates diverse strategies to sequester the metal ions by modulating soil pH, metal speciation, co-precipitation, and compartmentalization (Debona et al., 2017). Recent trends have proved the evolution of Si-based fertilizers to impart growth and developmental effects of crop plants, such as enhancing photosynthesis and regulating electrolytic leakage under stress conditions (Chen et al., 2011). For example, Si enhances photosynthesis in mango trees and increases water and nutrient uptake under abiotic stress conditions (Santos et al., 2014). Si is found in almost all the plants in variable amounts, imparting varying physiological effects (Cooke and Leishman, 2011). Apart from its critical role in stress tolerance imposed by excess salt and drought, uptake of Si results in enhanced mechanical support to shoots and leaf blades (Chen et al., 2011; Zhu et al., 2019a). Numerous reports reveal the application of Si in mitigating abiotic and biotic stresses in various plant species (Zargar et al., 2019; Gou et al., 2020). Moreover, Si plays a vital role in ameliorating metal toxicity in several crop plants (Chen et al., 2011; Singh et al., 2019; Wu et al., 2019). Moreover, Si helps to mitigate metal ion stresses like that of aluminum (Al) and manganese (Mn) stress in crop plants (Tripathi et al., 2016). Water uptake through aquaporins and root hydraulic conductance is upregulated by administering exogenous Si (Liu P. et al., 2015). For instance, supplementation of Si in Talh trees (Acacia gerrardii Benth) induces salinity tolerance, possibly through overproduction of glycine betaine and proline to conserve water in tissues, positively regulating metabolic activity (Al-Huqail et al., 2019). Inclusively, Si is critical to plant growth and metabolism under a diverse range of abiotic stress conditions (Figure 1; Song et al., 2014; Yin et al., 2016).
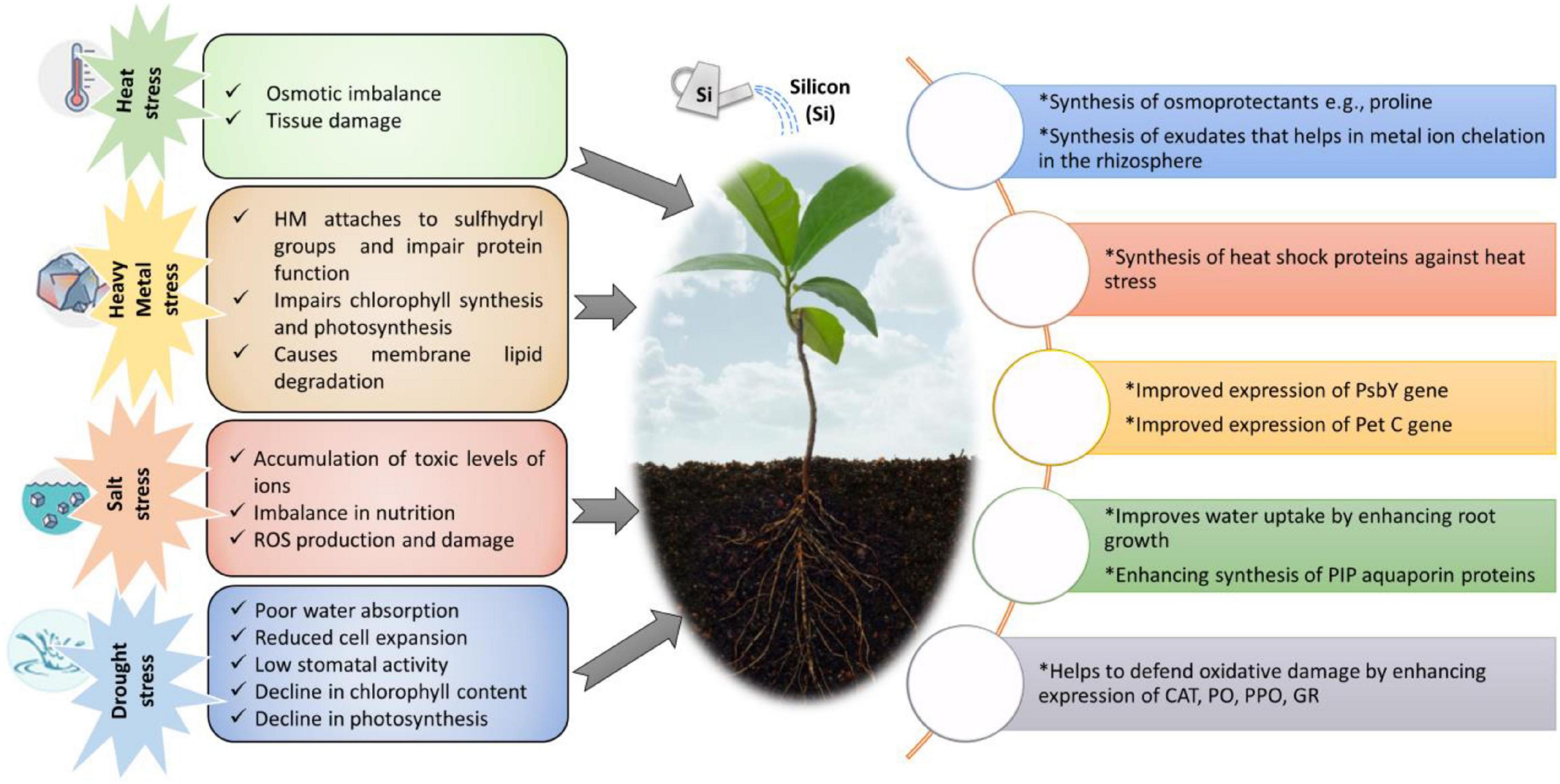
Figure 1. Impact of abiotic stress on various aspects of plant growth and defense mechanisms evoked by application of Si.
Phytohormones play a pivotal role in stress tolerance by modulating a wide range of physiological, cellular, and molecular responses by activating various signal transduction pathways critical to defense mechanisms (Fahad et al., 2015a; Fei et al., 2019; Mubarik et al., 2021; Sabagh et al., 2021). These critical phytohormone-controlled physiological processes during defense include osmotic adjustment, plant-water relations, source/sink transition, the adjustment in photosynthesis, nutrient allocation, and activation of antioxidant defense systems (Miura and Tada, 2014; Khan et al., 2020a). The main phytohormones proven to mitigate the stress conditions through physiological, biochemical, and molecular adjustments include auxin, CK, ETH, GAs, SA, ABA, BRs, and JA (Khan et al., 2020a,b). The levels of phytohormones are reported to be enhanced upon exogenous Si supplementation under abiotic stress conditions (Kim et al., 2016). Considering the beneficial role of Si in alleviating the abiotic stress in crop plants, we believe it is the need of the hour to discuss its clinical properties at the physiological and molecular levels. Against this background, the current review is aimed to provide an in-depth account of the role of Si as a critical element in sustaining plant growth and productivity under abiotic stress conditions. We have also discussed the intervention of Si in triggering the phytohormone synthesis, regulating expression of genes in alleviating the abiotic stress conditions, and detailed the updated account on its role in enhancing growth and metabolism. We believe the current review will pave the way to further open the window of exploring this golden element and its frontline role in attaining global food security.
Silicon – A Golden Key Element to Attain Sustainable Agriculture Growth
Population increase has put immense pressure on the agricultural system to overcome food insecurity. Even though diverse technological interventions increased the crop yield, at the same time, industrialization has triggered an increase in severity of environmental stresses, affecting crop production and, in turn, failure to achieve sustainable agriculture growth. Plants adjust to these factors by modulating their metabolic networks and inducing physiological changes (Raza et al., 2020a,b). Several reports suggest that Si plays an essential role in the production and yield of crops under extreme environmental conditions (Majeed Zargar et al., 2010; Liang et al., 2015b; Zargar et al., 2019; Salim et al., 2021). The growth and the metabolism of several monocots and dicot crops are enhanced by the active accumulation of Si in their organs in larger quantities (Liang et al., 2015c). High salinity results in reproductive disorders because higher Na+ competes with the transport of K+ and Ca2+ in the plasma membrane of cells (Zhu, 2016). Recent reports have suggested that Si alleviates Na+ accumulation, oxidative damage, and osmotic stress (Zhu et al., 2015; Flam-Shepherd et al., 2018; Yin et al., 2019). Several defense pathways related to phytohormone synthesis and compatible solutes are triggered by Si against abiotic stressors (Majeed Zargar et al., 2012; Mir et al., 2020). Elevated proline accumulation is observed in salt-sensitive genotypes of okra (Abelmoschus esculentus) upon exogenous application of Si to withstand salinity stress (Abbas et al., 2015). Si induced overproduction of total free amino acids, including proline and glycine betaine, in two Capsicum annuum cultivars, enhancing tolerance to salt stress (Pereira et al., 2013). Further accumulation of Si in wheat and soybean results in a declined trend of proline content, hence proving the efficacy of Si to induce its own mechanism to mitigate the salt stress (Lee et al., 2010; Bybordi, 2015).
Si administration positively affects the growth, yield, and fruit quality of different fruit crops (Neumann and Zur Nieden, 2001). Si induces water and energy-saving capability, improvement in fruit quality, and an increase in yield. Due to the extent of differences in the Si accumulation, plants differ in their Si-based positive responses to abiotic stressors (Deren, 2001; Mitani and Ma, 2005; Keeping and Reynolds, 2009). Si is accumulated through specific transporters by major crop plants, including dicots like cotton, soybean, cucurbits, tomato, and monocots, such as rice, maize, and wheat, to alleviate different stress factors (Zargar and Agnihotri, 2013; Liang et al., 2015a). Application of Si leads to the overexpression of defense-related genes and their activity, apart from its role in accumulating defensive compounds, such as phytoalexins, momi-lactones, and phenolics (Liang et al., 2003; Rémus-Borel et al., 2005; Rahman et al., 2015). For instance, in rice plants, the ROS scavenging activities are decreased upon supplementation of Si during exposure to stressful conditions (Kim et al., 2014c). A clue to adapt Si as a remedial measure for sustainable agriculture is evident from the role of Si in the upregulation of genes involved in several adaptations, such as phytohormone metabolism and cell wall synthesis under stressful conditions in canola (Brassica napus) (Haddad et al., 2019). A wide range of genes has been modulated against the abiotic stress factors upon Si supplementation (Figure 2). Exogenous supply of Si alleviates sulfur and osmotic stress by promoting root growth via increased root sucrose levels in barley (Maillard et al., 2018). Emamverdian et al. (2018) reported that Si is capable of heavy metal remediation by initiating different pathways through scavenging ROS. It is reported that Si enrichment improved the growth and development of plants through enhancements in nutrient use efficiency (NUE) in winter wheat (Neu et al., 2017). For instance, Si is reported to decrease metal ion toxicity by reducing the solubility of metals in the cell wall or by detoxifying the metal ions (Wu et al., 2019; Vaculík et al., 2020). Several reports strongly report the role of Si to enhance tolerance to UV-B radiation by activation of defense mechanisms, fortification of the cell wall, etc., in crop plants (Yao et al., 2011; Guntzer et al., 2012). These reports suggest that Si plays a significant role in maintaining plants’ physiological and metabolic functions under drought stress conditions. In the preceding section, we have discussed the ameliorating effects of silicon on phytohormone-based resilience under extreme abiotic conditions.
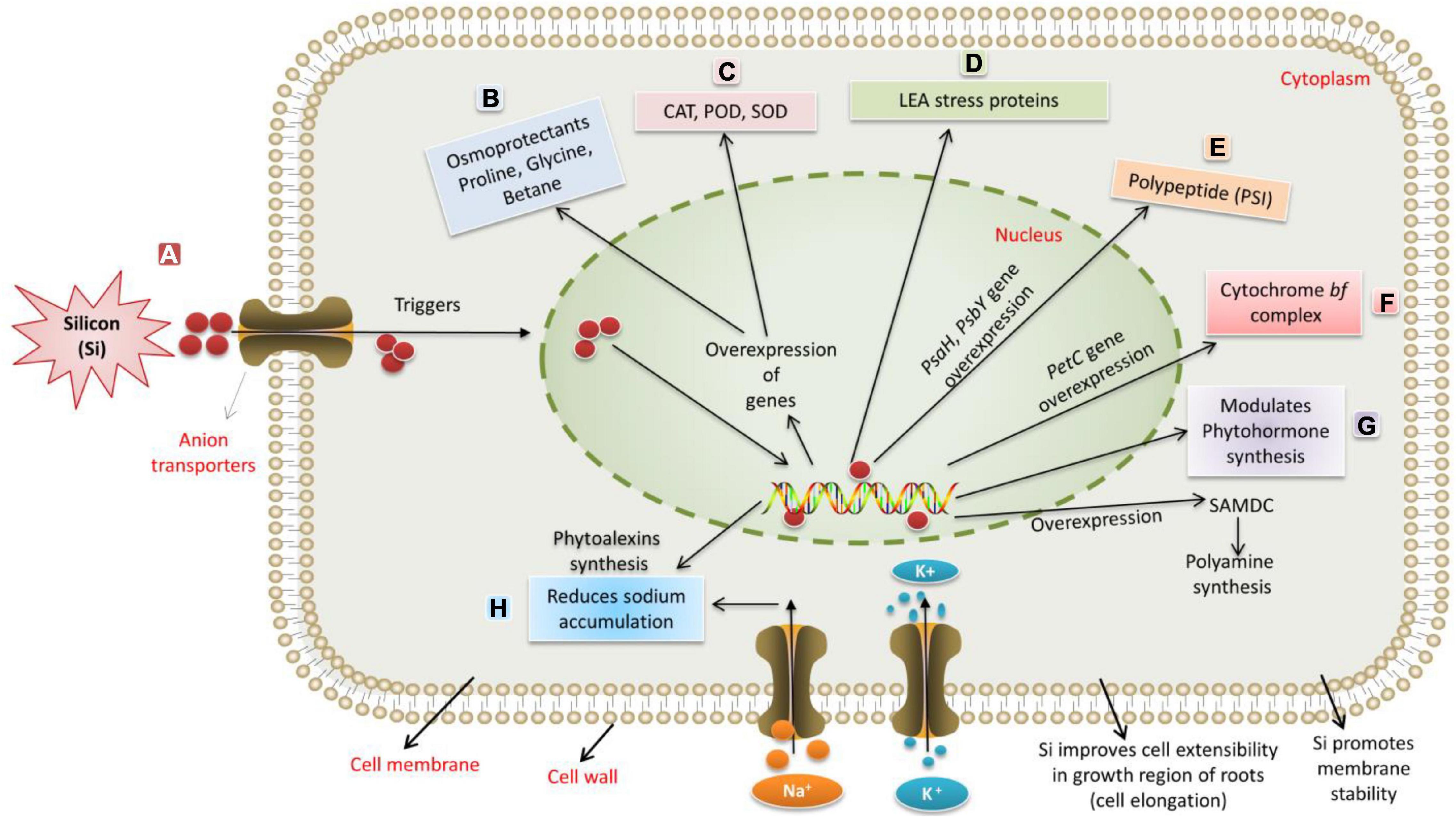
Figure 2. The silicon-induced mechanism for regulating abiotic stresses. (A) Silicon is taken up through anion transporters. (B) Drought and salinity stress induce osmotic stress and osmotic imbalance. Si enhances the synthesis of osmoprotectants like proline, glycine, and betaine. (C) Stress induces the formation of ROS. Si enhances the expression of CAT, SOD, and POD for protection against oxidative damage. (D–F) Si supplementation results in transcriptional regulation of genes related to photosynthesis, such as overexpression of PsaH, which encodes essential polypeptide subunits of photosystem-I (PSI) dimer, the PsbY (Os08g02530) gene encoding polyprotein component of Photosystem II and the PetC gene, encoding Rieske Fe-S center-binding polypeptide of cytochrome bf complex. (G) Si modulates the synthesis of plant growth regulators to alleviate stress. Si induces the S-adenosyl-L-methionine decarboxylase (SAMDC) gene, encoding essential enzymes responsible for synthesizing polyamines. (H) Salinity stress negatively affects plant growth by increasing the accumulation of ions up to toxic levels. Due to a higher influx of NaCl, plants experience more severe oxidative and ionic stress. Sodium-ion accumulation up to toxic levels triggers ROS production, which, in turn, severely damages the cellular components and accelerates senescence in mature leaves, leading to reduced growth and metabolism. Si administration improves uptake of K+, which, in turn, stimulates H+-ATPase enzymes in the plasma membrane that helps to overcome salt stress.
Si-Induced Differential Phytohormone Synthesis Under Abiotic Stress Conditions: An Update
Phytohormones play a critical role in growth and development and are essential to circumventing a diverse range of environmental stressors (Sato et al., 2010; Raza et al., 2019; Hafeez et al., 2021b; Mubarik et al., 2021). Phytohormones, such as auxin, CK, ABA, GAs, and BR, are vital to growth and metabolism during normal and stressful conditions (Sato et al., 2010; Raza et al., 2019; Hafeez et al., 2021b; Mubarik et al., 2021). These hormones modulate a wide range of physiological networks at low concentrations, once transported directly to the corresponding cells or transported to distant tissues (Colebrook et al., 2014), thus resilience to environmental stressors. The combinatorial effects of phytohormonal regulation, such as JA, ABA, and SA, play a vital role in mediating heat and drought tolerance in crop plants (Ilyas et al., 2021; Mostofa et al., 2021). Upregulation of GAs can ameliorate the abiotic stress conditions to maintain the growth and development of plants. In addition, upon exogenous application of SA, plants exhibit tolerance to various abiotic stresses like drought, heat, chilling, and tolerance to other stressors like viral, fungal, and bacterial attacks (Prakash et al., 2021; Saleem et al., 2021). Phytohormones like ABA are upregulated during osmotic stress to enhance tolerance by expressing several genes (Al Murad et al., 2020). Even though ABA levels were increased under salt stress, reverse trends were observed once Si was applied in soybean plants (Lee et al., 2010). ABA induces stomatal closure to prevent water loss under extreme saline conditions (de Ollas et al., 2013). In addition, ABA biosynthetic genes ZEP and NCED3 are reported to be upregulated under abiotic stresses (Ma et al., 2018; Müller, 2021). On the other hand, ETH also senses external stimuli to adjust plant metabolism as per environmental conditions. It helps ameliorate abiotic stress in corroboration with other phytohormones, such as SA and JA (Rai et al., 2020). ETH regulates numerous physiological responses, such as triple response in the etiolated seedlings of a pea, induction of flowering, and seed germination (Kim et al., 2011). Higher levels of ETH are attributed to stress tolerance in plants, particularly tolerance to heat stress (Huang et al., 2021; Poór et al., 2021; Chen et al., 2022). Moreover, significant genes are upregulated by ETH to mediate diverse beneficial functions to plants (Kazan, 2015; Poór et al., 2021).
On the other hand, Si interacts with several phytohormones and other signaling molecules, such as polyamines, hydrogen sulfide, and nitric oxide, in improving multiple abiotic stresses (Figure 3; Raza et al., 2021g; Sabagh et al., 2021; Tripathi et al., 2021). As a result, the interaction network improves the antioxidant defense system, reduces oxidative damage, and increases resistance to multiple abiotic stresses (Figure 3). In recent years, Si has been reported to enhance phytohormone synthesis to upregulate the physiological process of crop plants under extreme environmental conditions. Under abiotic stress conditions, Si enhanced the metabolism and synthesis of phytohormones in plants (Zhu and Gong, 2014; Kim et al., 2016). The major phytohormones upregulated by Si under abiotic stress conditions include ABA, JA, GA, ET, SA, BR, and IAA (Arif et al., 2021). Si-induced phytohormones synergistically regulate the growth, metabolism, and stress tolerance in pepper, as shown by in silico data analysis (Gómez-Merino et al., 2020). In tobacco and rice, Si regulates gene expression of plant hormones like ABA, JA, SA, and ETH to adjust metabolism against abiotic stress factors (Table 1). Lee et al. (2010), Kim et al. (2014c), Liang et al. (2015b) reported that Si-treated rice plants exposed to heavy metals increase ABA concentration, followed by a decrease after 14th day of treatment. In addition, reduction of SA’s and JA’s endogenous concentration has also been observed in these Si-treated rice plants. Various cellular and developmental processes, such as embryo development and dormancy, stomatal opening, and seed development, are regulated by ABA in plants (Sreenivasulu et al., 2012). Stress conditions trigger the upregulation of genes-encoding enzymes for the biosynthesis of ABA. In Arabidopsis thaliana, the signals from roots to regulate the decreased leaf water potential and conductance are accomplished by ABA triggered by the closure of stomata under drought stress (Hasan et al., 2021; Liu et al., 2021). ABA levels in Si-treated plants are decreased under salinity stress and increased when plants are exposed to heavy metals like cadmium (Cd) and copper (Cu) (Khan et al., 2021b; Mostofa et al., 2021; Tripathi et al., 2021).
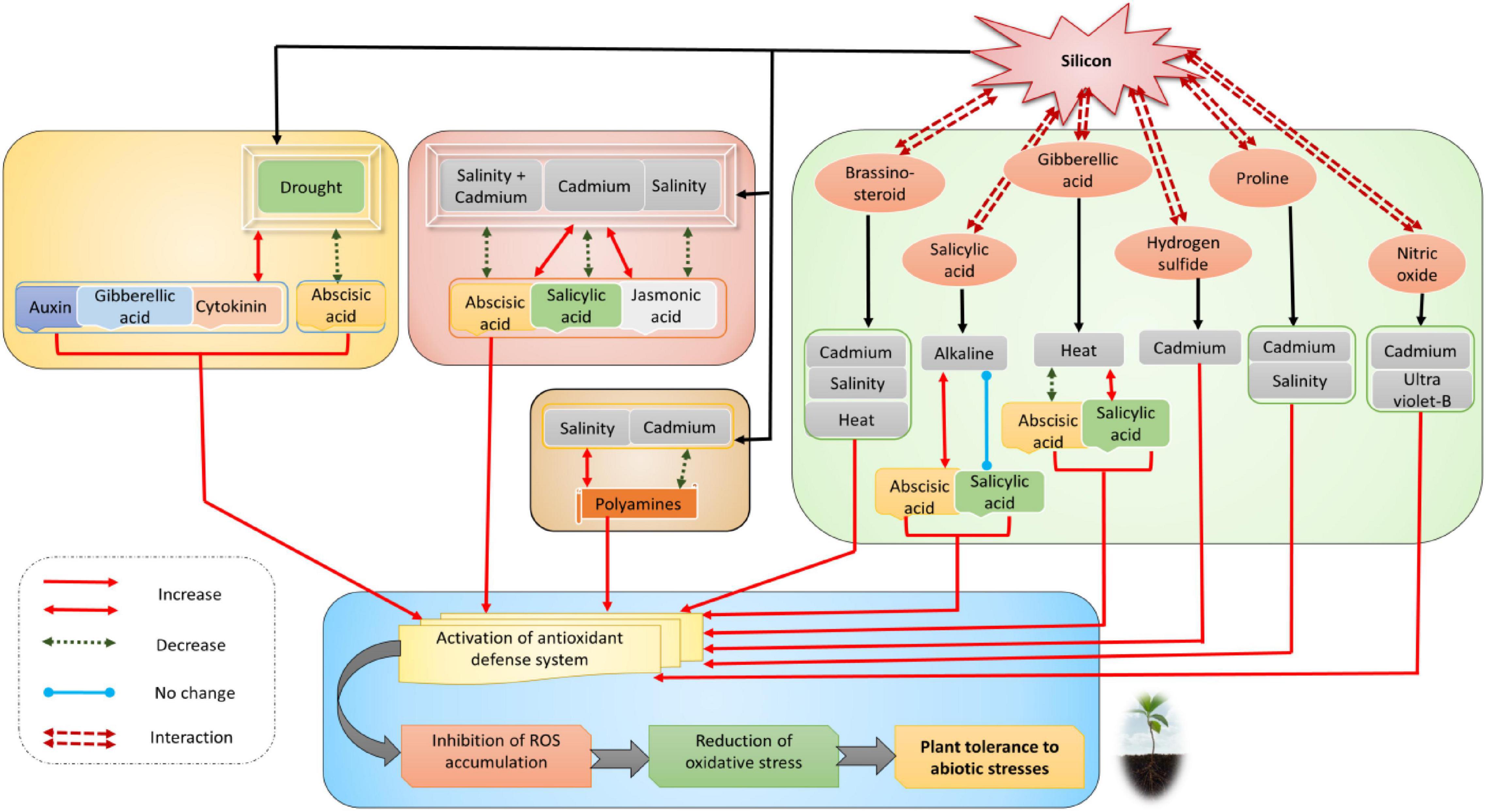
Figure 3. Interaction and crosstalk network of silicon with phytohormones and other signaling molecules in response to various abiotic and oxidative stresses. Read the text for further information.
Moreover, Si-treated plants ameliorate metal toxicity, leading to low production of ABA. Time-dependent effects of Si on ABA and JA synthesis were also reported under abiotic stressors (Kim et al., 2014c). Overexpression of Si transporter genes, such as TaLsi1 (Si-Influxer) and TaLsi2 (Si-effluxer), under salinity-induced ABA was reported upon supplementation of exogenous Si in wheat (Zia et al., 2021). Si-induced alteration of ABA-related genes glyceraldehydes-3-phosphate dehydrogenase (GAPDH), cyclophilin (CYC), ADP-ribosylation factor (ADP-RF1), and sulfur transporter (HsST1) alleviates sulfur deficiency and osmotic stress in crop plants (Maillard et al., 2018). Moreover, ABA levels are declined due to downregulation of genes by exogenous supplementation of Si to enhance the stress tolerance in barley (Maillard et al., 2018). It is reported that, in rice plants exposed to salt stress, Si upregulated expression of ABA biosynthesis genes, such as ZEP-encoding zeaxanthin epoxidase, NCED1-encoding 9-cis-epoxicarotenoid oxygenase 1, and NCED4-encoding 9-cis-epoxicarotenoid oxygenase 2 (Kim et al., 2014b). A versatile transcription factor, NAC encoded by RD25 genes, was found to be induced by stress factors, such as salinity and drought, in addition to its induction upon treatment of crop plants by JA and ABA (Singh et al., 2021).
JA is another hormone critical to generate a response against a wide range of stress factors through activation of defense signaling pathways (Pieterse et al., 2012; Caarls et al., 2015). JA production is lowered upon exogenous treatment of Si; later, decline in JA can be correlated by low-stress levels exerted by Si treatment (Wang et al., 2020; Raza et al., 2021b). The reduction in the levels of JA induced by Si involves the modulation of several signaling pathways (Liu and Timko, 2021; Tripathi et al., 2021). Si application drastically improves the signaling of JA to initiate a defense system during wounding stress in rice plants (Hamayun et al., 2010). The expression of genes related to JA synthesis, like LOX and other genes, is upregulated when Si is administered to wound-stressed rice plants (Kim et al., 2011, 2014b). Under wound stress via feedforward activation, the LOX gene further amplifies the expression of JA in A. thaliana (Sato et al., 2010). Genes related to JA like OsAOS1, OsAOS2, OsOPR3, and OsLOX were downregulated upon exogenous application of Si during wound stress, highlighting the role of Si in triggering metabolic networks to heal the mechanical injuries in rice plants (Kim et al., 2014b). In addition, overexpression genes like AOC, AOS, OPR3, and LOX during early stages occurred during wound stress; downregulation of JA biosynthesis was also demonstrated in tomatoes. GAs are an important class of phytohormones critical to plants, such as seed germination and fruiting (Colebrook et al., 2014). The endogenous levels of GA are upregulated under salinity stress in Glycine max upon application of Si at 2.5-mM concentration (Arif et al., 2021; Dhiman et al., 2021). The Si-triggered upregulation of GA1 and GA4 content in cucumber plants helps to withstand drought and salinity stress (Hamayun et al., 2010). The combinatorial effect of Si and nitrogen on the growth and metabolism of two rice cultivars led to upregulation of GA20 and GA1, initiating vegetative and anthesis stages (Lee et al., 2010). Moreover, the decreased levels of GA under salt stress were restored by applying Si in rice plants (Lee et al., 2010). In another study, Si-mediated increase in the synthesis of GA, such as GA1 and GA4 levels, profoundly enhanced salt tolerance in Glycyrrhiza uralensis Fisch (Zhang et al., 2018). Likewise, Si-treated plants enhanced GA concentration in saline conditions and reduced JA concentration under wound response (Lee et al., 2010; Kim et al., 2011).
Auxin is another phytohormone critical to a wide range of development and growth of plants (Du and Scheres, 2018). It has been reported in several studies that IAA plays an essential role in the tolerance of crop plants to salinity stress (Fahad et al., 2015b; Hussain et al., 2019). Activation and upregulation of numerous genes triggered by auxins have been reported in rice, soybean, and Arabidopsis, which are important for the development and growth of plants (Wani et al., 2016). It is reported that, upon administration of Si, IAA levels decreased in crop plants, resulting in a delay of leaf senescence in canola (Brassica napus) (Haddad et al., 2018). The synthesis of IAA and ABA was transcriptionally downregulated by Si supplementation, resulting in osmotic stress tolerance in barley (Maillard et al., 2018).
Likewise, Si applied to the rice plants having wound stress resulting in decreased ETH production compared to rice plants not treated with Si (Kim et al., 2011). The delaying of senescence was observed in crop plants upon Si supplementation through increased synthesis of CK biosynthesis in Arabidopsis and sorghum (Markovich et al., 2017). Si enhanced the growth and development of rice plants by its effect on endogenous ETH and JA levels, even though JA and JA show inverse correlation under wound stress (Kim et al., 2011). SA, another potential phytohormone, has important physiological implications on crop plants, such as transport and uptake of ions, photosynthetic metabolism, and membrane permeability during stress (Noreen et al., 2009). More importantly, SA is reported to outwit abiotic stressors like the generation of ROS, attacks by pathogens, salinity, and drought stress (Hara et al., 2012). Tolerance to stress conditions, such as salinity, is initiated by SA-based interplay of phytohormones (El-Mashad and Mohamed, 2012). Combinatorial supplementation of SA and Si reduces damage caused by boron toxicity through increased carotenoid, chlorophyll content, biomass production, and reduction in oxidative stress (Eraslan et al., 2008). A possible mechanism of stress tolerance induced by SA occurs through expression of several genes, such as those coding for pathogenesis-related proteins (PR-proteins) (Kidokoro et al., 2009). Exogenous application of SA helps in the resistance and adaptation of crop plants in various abiotic stress factors, such as drought, salinity, and iron deficiency (Mostofa et al., 2021; Tariq et al., 2022). In pea plants, abiotic stress, such as oxidative stress, was reported to be remedied by exogenous supplementation of Si and SA (Rahman et al., 2017). Moreover, soybean plants withstand salt stress through modulation of SA and JA upon treatment with Si (Kim et al., 2014a). In conclusion, the reports suggest that interplay of Si and phytohormones is critical to the growth and metabolism of plants under drought stress conditions.
Physiological Benefits of Silicon Under Abiotic Stress
The growth and the metabolism of plants are improved through their mechanisms to develop tolerance against abiotic stressors. Later attributes were primarily alleviated by supplementation of Si to withstand negative environmental stress factors (Arif et al., 2021; Souri et al., 2021). In sugarcane, Si has been attributed to enhancing leaves’ rigidity and erectness, resulting in improved photosynthesis (Verma et al., 2019). Pfannschmidt and Yang (2012) reported overexpression of PsaH, which encodes essential polypeptide subunits of photosystem-I (PSI) dimer upon supplementation of Si. A comparative study showed that Si-administered cucumber exhibited increased perception of light, the enhanced concentration of chlorophyll pigments, and rough petioles (Adatia and Besford, 1986; Lozano-González et al., 2021; Meng et al., 2021). Several reports suggest that Si positively modulates the plant growth and yield in rice plants (Dorairaj et al., 2017; Garg and Singh, 2018). It has been demonstrated that Si positively modulates the growth, development, and metabolism in sugarcane (Saccharum officinarum) (Verma et al., 2021), beans (Phaseolus vulgaris) (Zuccarini, 2008; Nosrati et al., 2014; El-Saadony et al., 2021), cotton, (Gossypium hirsutum) (Wang et al., 2001; dos Santos et al., 2020), rice (Hwang et al., 2007; Feng et al., 2011), and soybean (Glycine max) (Hamayun et al., 2010).
Moreover, Si has been shown to positively modulate the metabolism of several crop plants, such as spinach (Spinacia oleracea) (Eraslan et al., 2008), wheat (Gong et al., 2005; Sattar et al., 2019; Bukhari et al., 2021a), barley (Savant et al., 1999; Benslima et al., 2021; Sierra et al., 2021), and maize (Liang et al., 2005; El-Mageed et al., 2021). Shi et al. (2013) reported that tomato seedlings completely recover their growth and photosynthesis once exposed to Si under water stress conditions. Si can ameliorate the metal ion toxicity and, in turn, able to enhance the quality and quantity of biomass in Phaseolus vulgaris (Zuccarini, 2008; Artyszak, 2018). The application of Si detoxifies Na+ through modification of the antioxidant defense system. Later effect positively modulates important biochemical and physiological metabolic networks in sunflower and sorghum under abiotic stresses, particularly salt stress, to increase shoot dry matter production (Hurtado et al., 2020). Si-treated rice plants significantly improved the shoot, and root morphology restored photosynthetic efficiency amid Cd toxicity (Bari et al., 2020). In addition, Si was reported to mediate a wide range of physiological benefits like enhanced growth, nutrient uptake, provision of mechanical strength, and resistance to biotic stressors like fungal diseases (Akhtar and Hussain, 2008; Bakhat et al., 2018; Souri et al., 2021). Moreover, Si helps to reduce lipid peroxidation in crop plants exposed to wound stress in rice plants (Kim et al., 2014b). The germination characteristics like the germination rate and percentage and shoot length are highly enhanced by applying Si under normal and drought and salinity stress conditions in tomatoes (Shi et al., 2014). In crop plants, such as maize, rice, wheat, tomato, and cucumber, Si enhanced shoot and root growth under salt stress (Wu et al., 2015). Water balance in cucumber is improved by exogenous supplementation of Si through its effect on increasing the root-shoot ratio under salt stress (Wang et al., 2015). For instance, the supplementation of Si leads to the overexpression of stress-responsive genes, such as Oryza sativa RING domain-containing protein (OsRDCP1), Oryza sativa choline monooxygenase (OsCMO), DREB2A, dehydrin OsRAB16b, and NAC5, under abiotic stress conditions (Khattab et al., 2014). Production of ROS is disastrous to crop plants as it induces lipid peroxidation (Gill and Tuteja, 2010). The foliar application of Si in okra leads to overexpression of ROS-scavenging genes coding for CAT, POD, and SOD during salinity stress. Later, enzymes help decrease the lipid peroxidation in okra plants (Abbas et al., 2015). Differentially enhanced activity of ROS-scavenging enzymes, such as APX, CAT, and POD in roots, shoots, and leaves, was reported in alfalfa under salinity stress by application of Si (Wang et al., 2010).
Moreover, Si reduces oxidative stress in tomato plants under salinity stress, indicating the effect of Si on the activity of antioxidant enzymes (Li et al., 2015). Similar results were also observed in rice plants grown under salinity stress when Si was applied (Kim et al., 2014c). Numerous studies back up the role of Si in alleviating abiotic stress in crop plants (Mir et al., 2019). The combined ameliorative effect of Si ad Bacillus subtilis fbl-10 was found to enhance tolerance to Pb (Lead) toxicity in brinjal (Solanum melongena) (Shah et al., 2021). Moreover, the effect of Si on delaying leaf senescence in mustard (Brassica juncea) under drought and salinity stress was accomplished by modulation of homeostasis of ions and antioxidative defensive systems (Alamri et al., 2020). It is reported that, after deposition, Si helps to thicken the xylem cell wall and the Casparian strips of endodermis to impede the metal ion transport in crop plants (da Cunha and do Nascimento, 2009; Mandlik et al., 2020; Souri et al., 2021). In combination with SA, in mung bean seeds, Si enhanced physiological parameters through the decreased accumulation of Na+ and increased proliferation of K+ (Lotfi and Ghassemi-Golezani, 2015). Several physiological parameters, such as the transpiration rate, stomatal conductance, chlorophyll levels, and membrane permeability, are maintained by Si during abiotic stress, particularly during salinity stress (Coskun et al., 2016). Besides, the adverse effects of heat stress in tomato plants are mitigated by exogenous application of Si, further enhancing biomass production and shoot length (Khan et al., 2020b). Plants overexpress several genes pertaining to transpiration, photosynthesis, synthesis of polyamines, and secondary metabolism in response to abiotic stressors (Abdel-Haliem et al., 2017). For instance, in sorghum, it is reported that, upon application of Si, the root growth was stimulated by root elongation due to cell wall extensibility in the growth region (Hattori et al., 2003; de Oliveira et al., 2019). Several reports suggest that Si induces modulation of phytohormones to delay senescence in rice and sorghum (Lee et al., 2010; Kim et al., 2014c; Yin et al., 2014). In chickpea cultivars, drought tolerance was regulated by supplementation of Si through improvement of various growth parameters. High salinity in rice and Mn, Al, Cu, and Cd toxicity in sorghum and wheat is alleviated by supplementation of Si (Kang et al., 2015; Keller et al., 2015; Rizwan et al., 2015).
A large number of stress-tolerant genes are upregulated by exposure of crop plants to Si. For instance, studies have reported Si results in transcriptional regulation of genes related to photosynthesis during zinc stress in rice plants (Song et al., 2014). In rice, wide ranges of stressful conditions are acclimatized by the expression of LEA genes-encoding stress tolerance LEA proteins (Lenka et al., 2011). Several studies report overexpression of the OsNAC5 gene is attributed to alleviating the stress tolerance through the abundant expression of LEA3 in rice plants (Takasaki et al., 2010). Likewise, in rice, upregulation of OsRDCP1 and OsCMO gene levels alleviated the dehydration stress upon supplementation of exogenous Si (Khattab et al., 2014). The overexpression of the S-adenosyl-L-methionine decarboxylase (SAMDC) gene, encoding vital enzymes responsible for the synthesis of polyamines, is augmented by supplementation of Si (Pottosin and Shabala, 2014). These polyamines are critical to withstand the onslaught by environmental stressors (Liu P. et al., 2015). The wonderful effects of Si under drought stress conditions have considerably shifted scientists’ focus on its emerging role in maintaining the physiological hemostasis of crop plants. In the preceding sections, we have discussed the role of Si in alleviating the stress tolerance of plants against salinity, drought, heavy metal, temperature, and other abiotic stressors.
Salinity Tolerance Mediated by Silicon
Agricultural crop production is grossly limited by salinity stress leading to a drastic decline in food production at the commercial and industrial levels (Munns and Gilliham, 2015). Plants are negatively affected by salinity through an increase in ionic and osmotic stress. On a global scale, almost 6% of cultivated land is graded as saline and is expected to increase by 2050 due to global climatic change, further worsening the scenario of crop production. Moreover, salinity stress induces a reduction in osmosis, imbalance in nutrient supply, and enhanced accumulation of ions up to toxic levels (Hasegawa et al., 2000; Hafeez et al., 2021a; Saddiq et al., 2021). Further, it is evident that salt stress is a major abiotic factor that inhibits crop plants’ growth and metabolism, resulting in reduced crop yield (Han et al., 2021). The elevation of salt stress is further compounded by anthropogenic activities, such as saline water irrigation, pollution through industries, and higher use of chemical fertilizers (Zhu et al., 2019a). Sodium-ion accumulation up to toxic levels triggers the production of ROS, which, in turn, severely damages the cellular organelles, such as chloroplast, cell membrane, mitochondria, and peroxisomes, to impair the metabolic networks in crop plants (Munns and Gilliham, 2015).
Moreover, it is reported that the accumulation of Na+ to toxic levels in older leaves accelerates senescence in mature leaves, leading to reduced growth and metabolism (Munns and Gilliham, 2015; Saddiq et al., 2021). Global production of agricultural food crops is reduced up to 20% by salinity stress among all the abiotic stresses (Kumar et al., 2017; Hussain et al., 2018). High salinity reduces the potential of plants to absorb the water, resulting in a reduction of cell expansion and low stomatal activity due to less inter- and intracellular water levels. Due to a higher influx of NaCl, plants experience more severe oxidative and ionic stress (Ferchichi et al., 2018). The salt stress is attenuated more frequently by Si administration (Etesami and Jeong, 2018; Liu et al., 2019). In maize, salinity stress is alleviated by exposure to Si through improvements in growth and maintaining ion homeostasis via decline in Na+ uptake (Ali et al., 2021). Si improves uptake of K+, which, in turn, stimulates H+-ATPase enzymes in the plasma membrane to overcome salt stress in maize and other plants (Liang et al., 2005; Liu et al., 2019; Dhiman et al., 2021). Under salinity stress, the application of Si in rice was reported to decrease the accumulation of sodium and chloride ions through apoplastic transport (Shi et al., 2013). Hyper-accumulators of Si were found to form double cuticle layers, enhancing photosynthesis by preventing evapotranspiration. Moreover, Si influences starch metabolism, glycolytic pathways, and the Krebs cycle under osmotic stress in barley (Hosseini et al., 2017). In addition, cucumber seedlings could ameliorate the extreme oxidative stress upon the influx of Si (Gou et al., 2020). Declined chlorophyll content due to salinity stress was reported to be significantly ameliorated by the supplementation of exogenous Si to root zones or foliar in tomato (Al-aghabary et al., 2005), pea (Ahmad and Jhon, 2005), and cucumber (Hamayun et al., 2010) crop plants. In plants, Si accumulates and deposits in discrete silica bodies in the form of phytoliths, usually beneath the cell walls on different parts, such as roots, stems, and leaves. These phytoliths then bind with Na+ to reduce their uptake and increase K+ uptake to different regions of plants. Concomitantly, it is reported that Si induces greater H + -ATPase activity to lower the electrolyte leakage resulting in higher secretion of Na+ from cells in Puccinellia distans (Soleimannejad et al., 2019). Furthermore, to avoid multiple abiotic stressors, Si deposition in leaf blades during salinity results in converting complexions to non-toxic stable ions to regulate ROS production (Mandlik et al., 2020; Ahire et al., 2021; Dhiman et al., 2021). Due to the deposition of Si in the cell walls of endodermis and rhizodermis, the transport of Na+ is blocked through a reduction in apoplastic movement of Si across the root system, leading to a drastic decline in the transport of Si from roots to shoots, hence salinity tolerance (Khan et al., 2019). Moreover, negative impacts of higher salt levels are greatly tolerated by Si application through the production of glycine betaine to maintain metabolic activity to regulate water levels in plants (Al-Huqail et al., 2019). The compatible osmolytes, such as glycine betaine, are critical to the stability of membrane, functional proteins, protein complexes, and enzymes under saline conditions (Rajasheker et al., 2019). In addition, Si enhances proline content to induce salt tolerance in cucumber (Gou et al., 2020). Plants synthesize proline as major osmoprotectants that protect the integrity of membrane and protein stability under salinity stress (Ahanger et al., 2020a). Moreover, the variable concentration of Si resulted in enhanced activities of enzymes, such as glutamine synthetase (GS), nitrate reductase (NR), sucrose synthetase (SS), and sucrose phosphate synthetase (SPS), to enhance nitrogen and carbon metabolism under salinity stress in Glycyrrhiza uralensis Fisch (Cui et al., 2021). In tomato, several genes, such as leDREB-1, leDREB-2, leDREB-3, leLsi-1, leLsi-2, leLsi-3, leAPX, leSOD, and leCAT, are upregulated upon supplementation of Si upon exposure to salt stress (Ngwepe et al., 2019), whereas the exposure of Arabidopsis to salinity and dehydration resulted in transient expression of DREB2A and DREB2B (Liu et al., 1998; Sakuma et al., 2006; Manna et al., 2021; Singh and Chandra, 2021). The increase in contents of minerals, such as Ca and Mg in tomato leaves and Mg, K, Ca, and P in Trifolium alexandrinum, was reported upon supplementation of Si to regulate growth under salt stress (Abdalla, 2011; Li et al., 2015). Overall, Si plays a critical role in circumventing salt stress through the induction of diverse biochemical and physiological mechanisms (Al Murad et al., 2020). See Table 2 for some recent examples of Si-induced salinity tolerance.
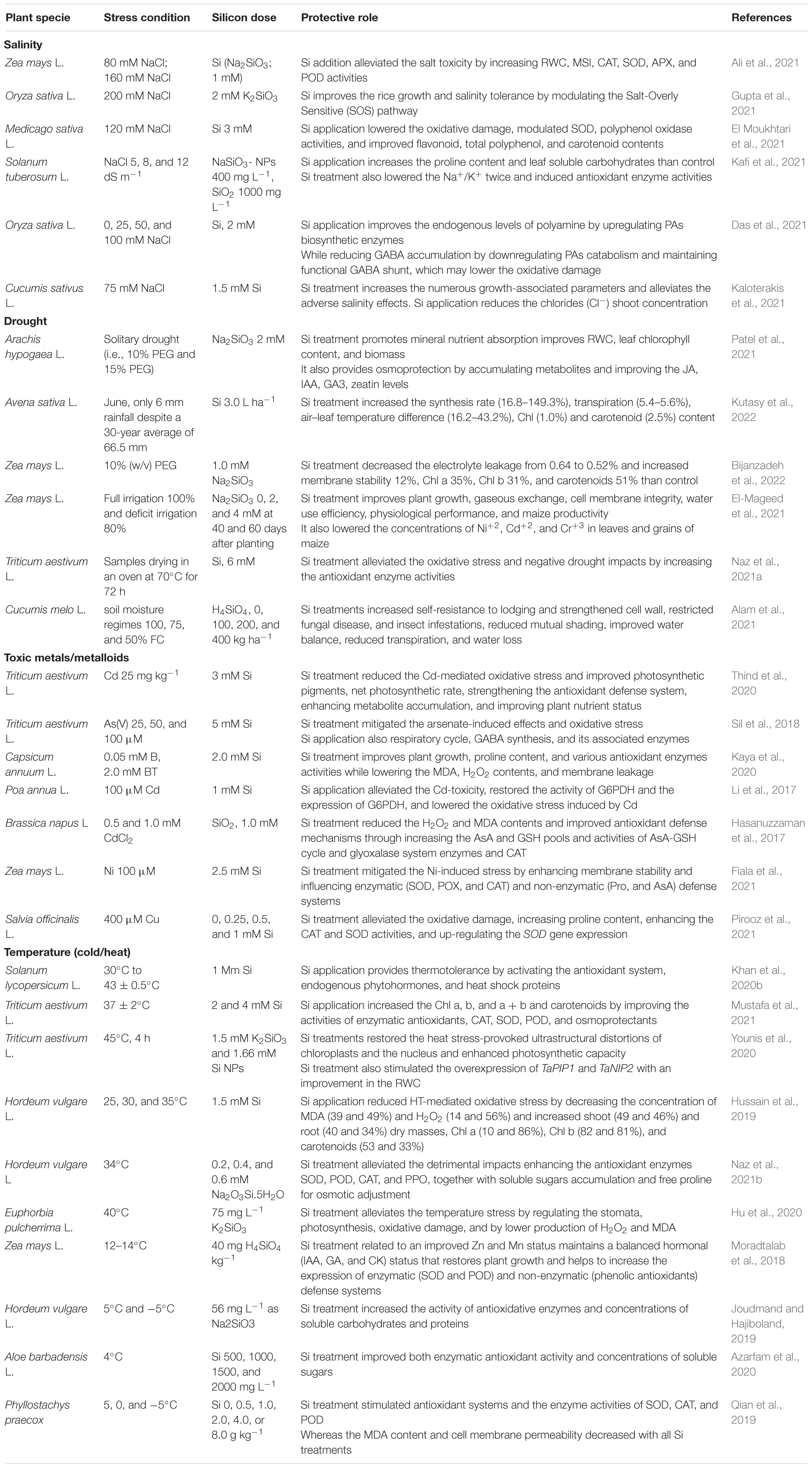
Table 2. A summary of some recent reports on silicon-induced abiotic stress tolerance in crop plants.
Drought Tolerance Mediated by Silicon
The normal growth and the metabolism of plants are severely affected by drought stress, and, additionally, it creates a negative impact on ecosystem balance and agricultural systems (Kutasy et al., 2022). Drought markedly inhibits photosynthesis rather than respiration in crop plants (Vanlerberghe et al., 2016). Several studies approved the role of Si to mitigate the adverse effects of drought stress to crop plants and, hence, to regulate normal metabolism, such as photosynthesis and alleviation of photoinhibition (Habibi and Hajiboland, 2013; Zhu and Gong, 2014; Alamri et al., 2020; Ahmad et al., 2021; Malik et al., 2021). Some recent examples of Si-induced drought tolerance are presented in Table 2. The crop plants respond to temperature and drought stress by activating regulons, such as dehydration-responsive element-binding protein (DREB2) (Nakashima et al., 2012). For instance, exogenous supplementation of Si in tomatoes helps cope with drought to regulate osmotic adjustment and accumulation of drought-induced ROS (Cao et al., 2020a). Moreover, it is reported that drought-mediated accumulation of ROS can be circumvented through supplementation of Si to promote energy dissipation in tomato crop plants (Cao et al., 2020a). In a recent study, Kaya et al. (2006) have reported that Si highly enhanced the growth of shoots in maize under water-scarce conditions. In addition, drought stress can be mitigated by accumulating mineral nutrients like K+ ions induced by Si in wheat seedlings (Keller et al., 2015). Si results in enhanced uptake of macronutrient Ca, Mg, P, and K and micronutrients Cu, Mn, and Fe during drought stress in sunflower (Gunes et al., 2007). Water uptake was enhanced by Si-induced overexpression of SbPIP1;6, SbPIP2;6, and SbPIP2;2, encoding plasma membrane intrinsic protein (PIP) aquaporins in roots of Sorghum bicolor (Liu P. et al., 2015). Similar results were also displayed in rice where genes, such as OsRDCP1-encoding choline monooxygenase and dehydrin OsRAB16b, were upregulated under drought stress (Khattab et al., 2014). Several studies reported that Si supplementation enhanced root growth in crop plants under drought stress (Ahmed et al., 2011; Hameed et al., 2013).
Moreover, drought tolerance is incrementally enhanced by Si supplementation to alleviate the deficiency of minerals in plants (Habibi and Hajiboland, 2013; Bityutskii et al., 2017; Hosseini et al., 2017). Moreover, Verma et al. (2021) investigated that Si played a defensive role against drought stress in sugarcane. Another mechanism responsible for enhancing tolerance by Si depends on its capability to boost the binding of nutrients to plant tissues and also stimulates the translocation of nutrients from the root apoplast to the shoots (Greger et al., 2018). The versatility of Si to defend drought stress largely depends on its capability to induce the expression of genes to mitigate the stress. For instance, Kapoor et al. (2020) detailed the Si-regulated expression of genes coding for MYB, no apical meristem (NAC), C-repeat/dehydration-responsive element-binding factors (CBF/DREB), zinc-finger proteins (ZFPs), and cup-shaped cotyledon (CUC) to activate brought a tolerant-signaling cascade in plants. Additionally, Si also induces the expression of genes linked with flavonoid biosynthesis and elevation of genes pertaining to antioxidant enzymes to enhance drought tolerance in plants (Ma et al., 2016; Bhardwaj and Kapoor, 2021). Recently, under water-deficit conditions, the foliar application of Si has enhanced the antioxidants activities, osmolytes contents, growth, flowering, and yield of squash plants (Salim et al., 2021). These studies clearly indicate that Si is a central player in mitigating drought stress and further opens the wide scope to explore the molecular and genetic mechanisms to have in-depth knowledge for its resilience mechanism against drought.
Heavy Metals Stress Tolerance Mediated by Silicon
The frequently found heavy metals, such as Al, Cd, arsenic (As), lead (Pb), mercury (Hg), copper (Cu), and zinc (Zn), in cultivated crop plants available through irrigation greatly influence the dynamics of soils (Shimo et al., 2011; Nazli et al., 2020; Asaad Bashir et al., 2021; Mehmood et al., 2021; Haseeb et al., 2022; Raza et al., 2022). These heavy metals induce devastating impacts on crop production and are potentially toxic to human health. The accumulation of heavy metals, such as Cd and Cu, binds to sulfhydryl groups of proteins, resulting in inhibition of major metabolic networks, such as lipid peroxidation, photosynthesis, and electron transport chain (ETC) (Sudo et al., 2008; Raza et al., 2021a,c). Crop production may be primarily affected by heavy metal ion-mediated adverse effects on tissues, such as induction of necrosis, leaf chlorosis, impairment in the synthesis of chlorophyll, and membrane lipid breakage (Sharma and Dietz, 2009; Takahashi et al., 2011; Raza et al., 2021d,2022). In the rhizosphere of crop plants, Si leads to exudates rich in chelated metal ions, hence reducing metal ion uptake (Khan et al., 2021a).
Additionally, due to the deposition of Si in the endodermis, a physical barrier is created to retard the uptake of metal ions through the xylem to reduce metal ion toxicity in wheat seedlings (Keller et al., 2015). The majority of crop plants like rice, barley, broad bean, summer squash, cucumber, and cowpea ameliorate Mn-based toxicity upon accumulation of Si (Liang et al., 2007). Si accumulators show considerably lower Mn toxicity, as they help reduce more than 90% of Mn in cell walls (Rogalla et al., 2002; Ahire et al., 2021; Mundada et al., 2021). The tolerance to Mn induced by Si is due to stronger affinity of Mn to cells walls of cucumber and cowpea (Iwasaki et al., 2002; Rogalla et al., 2002; Pavlovic et al., 2021). Moreover, Si is reported to enhance the tolerance of crop plants to salinity and heavy metals like Mn and Al (Liang et al., 2007). In addition, Si ameliorates the Cd toxicity in crop plants like wheat, cotton, rice, Bok choy, and peanut (Singh et al., 2019).
Additionally, Si mediates two critical functions in crop plants: reduced Cd uptake from shoots to grains and reduced uptake of Cd in plants (Fahad et al., 2015b). Moreover, it is documented that, in wheat plants, Si plays a vital role in Cd tolerance by augmenting the uptake of metals and inducing the translocation of metal-binding proteins associated with Cd (Greger et al., 2016). The concentration of Si ranging from 1.0–1.5 mM is reported to mitigate Cd toxicity in wheat, alfalfa, and rice. Liang et al. (2005) and Farooq et al. (2016) reported that tolerance to Cd in maize was obtained by administration of exogenous Si. Exogenous Si treatment in canola declined the adverse impacts of Cd on photosynthesis and other plant growth parameters (Baryla et al., 2001; Bukhari et al., 2021b). See Table 2 for some recent examples of Si-induced metal tolerance.
The possible mechanism of counteracting the toxic effects of Cd and Cu through the application of Si was correlated with overexpression of metal ion transporter genes, such as OsLsi and OsHMA3 (Kim et al., 2014b). Moreover, Si resulted in declined Al and Cr (Chromium) uptake through roots, stems, and leaves of peanut and rice seedlings, ameliorating toxic effects of these heavy metals (Ali et al., 2013; Shen et al., 2014; Tripathi et al., 2015). Si in the form of silica gel helps to shuttle the influx transporter (Lsi1) to transport Si rather than arsenic, reducing its toxicity in rice (Rizwan et al., 2015). Comparative studies reported that Si supplementation enhanced the transcription levels of the PsbY (Os08g02530) gene-encoding polyprotein component of Photosystem II, whereas Zn administration downregulates its transcription in rice plants (Song et al., 2014). Under Zn stress, it is reported that Si mediates overexpression of the PetC gene, encoding Rieske Fe-S center-binding polypeptide of cytochrome bf complex, an essential component of cytochromes in plant electron chain system (Song et al., 2014). Under metal toxicity, it was reported that Si supplementation mediated positive effects on plants through enhancing chlorophyll biosynthesis and, in turn, photosynthetic machinery (Adrees et al., 2015; Imtiaz et al., 2016). The stomatal conductance, net photosynthetic quotient, transpiration ratio increase by enhanced synthesis of carotenoids, chlorophyll a, and b upon administration of Si under heavy metal stress in cotton and wheat plants (Bharwana et al., 2013; Hussain et al., 2015; Tripathi et al., 2015). Si supplementation downregulated the expression of zinc (Zn) transporters under zinc toxicity in maize roots (Bokor et al., 2015).
Wound Stress Tolerance Mediated by Silicon
Plant wound stress occurs due to physical injuries usually induced by wind or herbivory. The damaged cells/tissue injuries are easily excess to bacterial or fungal origin pathogens. To be very specific, most of the time, injuries to C3 and C4 plants are primarily caused due to lodging (Savatin et al., 2014). Apart from injuries, unsaturated fatty acids of the plasma membrane are also damaged by wound-induced oxidative stress and lipid peroxidation (Farmer, 2005). Later response, i.e., lipid peroxidation, initiates a defensive signaling pathway mediated by the triggering of JA biosynthesis. Therefore, it is evident that wounding stress markedly results in increased lipid peroxidation and JA synthesis as reported in A. thaliana (Gfeller et al., 2011). Several reports have demonstrated the role of Si in providing strength to plant membranes and protection against injury during extreme stress conditions in crop plants, such as in Paeonia lactiflora Pall (Liang et al., 2007; Zhao et al., 2013). In response to oxidative stress, Si-supplied rice plants overexpress genes and activated oxidative enzymes, such as peroxidase (PO), catalase (CAT), and polyphenol oxidase (PPO), to alleviate the wound-induced oxidative damage (Kim et al., 2014b). Comparable trends were also observed in other crop plants, such as maize, wheat, and barley (Gong et al., 2005). In Si-administered crop plants, the silicified cells obstruct the pathogen entry at wound sites to impede the dissemination of infection (Ma et al., 2008). They may cause mandibular wear in grasshoppers due to abrasiveness in leaf tissue of rescue grass (Mir et al., 2019). The growth and the development of rice plants during the wound were maintained by exogenous supplementation of Si through enhancement of endogenous levels of ETH and JA.
Temperature Stress Tolerance Mediated by Silicon
Heat Stress
Crop production is deeply hindered by heat stress, resulting in the loss of economy and global food insecurity (Zhou et al., 2017; Raza, 2020). Furthermore, heat stress negatively affects tomato plant growth and production (Kumar and Kaushik, 2021; Ul Hassan et al., 2021). The rate of osmotic potential is impeached by heat stress, leading to an imbalance in water potential, resulting in the negative impact of biochemical pathways and tissue damage. To overcome the fluctuating temperatures, plants have evolved a diverse array of tolerance mechanisms, which include the production of heat shock proteins (HSPs), mechanisms to scavenge the ROS, and induction of specific phytohormones (Khan et al., 2020b; Haider et al., 2021a,b; Raza et al., 2021f). Khan et al. (2020b) reported that Si helps to induce thermotolerance by stimulating heat shock proteins, phytohormone production, and antioxidant system in tomatoes. Moreover, it is reported that small HSPs improve heat stress resistance in peanuts (Fragkostefanakis et al., 2016). Exogenous administration of Si was shown to enhance the tolerance to heat stress, resulting in improving shoot length and biomass production in sword fern, cucumber, and rice (Liu et al., 2009; Sivanesan et al., 2014). The heat tolerance in crop plants was inferred to Si-enhanced synthesis in photosynthetic pigments, such as chlorophyll a, chlorophyll b, and carotenoids in plants like grapevine and submerged macrophytes (Wang et al., 2010; Chalanika De Silva and Asaeda, 2017). The expression of SlLsi1 and SlLsi2 genes-encoding Si transporters was upregulated in Si-treated plants upon exposure to heat stress to improve heat tolerance in tomato plants (Khan et al., 2020b). See Table 2 for some recent examples of Si-induced heat stress tolerance.
Studies reveal that Si significantly triggered overexpression of heat tolerance genes, such as HSFA3, HSFA5, and HSF30 in date palm and DREB2, MAPK1, HSFA1a, HSFA1b, HSFA2, HSFA3, and HSFA7 in tomatoes (Khan et al., 2020b; Saha et al., 2021). For instance, Janni et al. (2020) reviewed the detailed mechanistic response of plants to overcome heat stress through activation of conserved pathways, viz. overexpression of ABA-responsive genes, Ca2+-sensing proteins, HSP-induced protein folding, and ROS-scavenging genes. Furthermore, it is reported that these signaling cascades lead to activation of HSP genes, such as HSP18, HSP70, and HSP90, through activation of HSFA2c to maintain folding and prevent non-specific protein formation under heat stress in tall fescue (Wang and Huang, 2017). Most remarkably, the activation of HSFs and HSPs interact with diverse signaling cascades triggered by Ca2+, phospholipids, SA, ET, ABA, H2O2, and NO to circumvent the negative impacts of heat stress on crop plants (Liu J. et al., 2015; Sharma et al., 2020). Hence, these reports strongly suggest that Si-induced HSPs are at the forefront of establishing proper folding, preventing denaturation and aggregation of cellular proteins, and inducing stay green traits through phytohormones under heat-stress conditions (Abdelrahman et al., 2017).
Cold Stress
The cold stress, including the temperatures < 15°C referred to as chilling and below 0°C for freezing, drastically influences the development and growth of plants (Habibi, 2015). Cold stress damages lipids, proteins, carbohydrates, and nucleic acids through the production of ROS (Suzuki et al., 2012). To avoid this, plants have adopted several strategies to combat the cold stress for maintaining growth and development (Dey et al., 2022). Habibi (2015) reported that foliar-applied Si effectively alleviates the cold stress by maintaining the integrity of bio-membranes and decreasing photoinhibition in grapevine plants. Reports suggest that, upon Si treatment, plants modulate levels of phytohormones, such as JA, SA, and ABA, which, in turn, activate several cold tolerance-signaling pathways (Miura and Furumoto, 2013; Eremina et al., 2016; Rastogi et al., 2021). Moreover, Si helps to enhance the tolerance to cold stress by expressing a wide array of genes, most notably transcription factors. For instance, three cold-tolerant genes, viz. DREB1A, DREB1B, and DREB1C, transiently expressed during the cold stress in Arabidopsis to regulate growth (Liu et al., 1998; Lee et al., 2005; Gujjar et al., 2014). Subsequently, the overexpression of OsDREB1 was reported to enhance tolerance to chilling and drought stress (Ito et al., 2006). Qian et al. (2019) reported that Si induced cold tolerance in Phyllostachys praecox by stimulating the activities of CAT, SOD, and POD during cold stress.
Similarly, Si enhances cold tolerance by affecting the hormonal balance and micronutrient homeostasis during early growth in maize plants (Bradáčová et al., 2016; Moradtalab et al., 2018). Earlier studies also reported that the Si-induced homeostasis of micronutrients is critical to protection under cold stress in maize (Imran et al., 2013; Bradáčová et al., 2016). Si alleviates the cold stress by modulating the concentration of metabolites and activity of apoplastic enzymes in leaf apoplasm in barley plants (Joudmand and Hajiboland, 2019). Recent reports have suggested that Si ameliorates the negative impacts of cold stress by regulating cellular redox homeostasis (Basu and Kumar, 2021; Mostofa et al., 2021). For instance, Hu et al. (2022) have recently reported that Si, in combination with selenium (Se), improves the yield, growth, and other physiological attributes of cucumber plants under field trails to circumvent extreme conditions, such as cold stress. See Table 2 for some recent examples of Si-induced cold stress tolerance. All these reports strongly suggest that Si plays a pivotal role in evading the negative impacts of cold stress on plants, even though scientists are still underway to explore the clear-cut molecular mechanism behind the resilience mediated by Si to alleviate cold stress.
Oxidative Stress Tolerance Mediated by Silicon
The major consequence of environmental stress elevated by oxidative stress by augmenting ROS generation, which damages cellular proteins and biomembranes, hinders the host antioxidant system and impairs major metabolic activities (Bhardwaj and Kapoor, 2021). Against these reactive species, plants have evolved enzymatic as well as non-enzymatic antioxidant systems to protect against damage induced by oxidative stress (Hussain et al., 2016). For instance, Si-supplied plants withstand the harmful effects of ROS-induced oxidation of membrane lipids and other metabolic imbalances exposed to abiotic stresses, particularly heat stress (Chalanika De Silva and Asaeda, 2017; Li et al., 2018; Hussain et al., 2019; Merewitz and Liu, 2019). In a recent study, Abdelaal et al. (2020) have reported that sweet pepper Si alleviated the oxidative stress by accretion of protective proteins and decreasing the ion toxicity, hence enhancing the activity of oxidative enzymes and eliminating O2– and H2O2 from cells. Si helps to enhance the activity of antioxidant enzymes, which, in turn, enhance the scavenging of ROS, declining lipid peroxidation (Liu P. et al., 2015; Coskun et al., 2019). Hasanuzzaman et al. (2020) reported that, even under optimal conditions, many metabolism processes produce ROS. Sorghum and sunflower were found to mitigate salt stress when Si was added to the growth medium (Liu P. et al., 2015; Yin et al., 2016; Hurtado et al., 2021). Foliar application of Si (150 mg L–1) in okra was found to enhance salt tolerance through its positive effect on activities of an antioxidant enzyme, leading to the reduction of lipid peroxidation (Abbas et al., 2015, 2017). Moreover, Si-alleviated salt stress in several crop plants like sorghum, sunflower, rice, wheat, maize, and mungbean, when supplemented in a growth medium (Liu P. et al., 2015; Abdel-Haliem et al., 2017; Alzahrani et al., 2018; Bosnic et al., 2018; Flam-Shepherd et al., 2018; Ahmad et al., 2019; Khan et al., 2020b; Hurtado et al., 2021). The Si supplemented in salt stress conditions resulted in increased activity of the antioxidant enzyme, leading to a decrease in the generation of ROS and reduced lipid peroxidation (Coskun et al., 2019). The lipid peroxidation and the production of H2O2 were alleviated by overexpression of the OsNAC5 gene upon supplementation of Si (Hu et al., 2008). The ROS-scavenging enzymes, such as SOD, CAT, and GR, are upregulated upon exogenous administration of Si in barley to stand oxidative stress (Liang et al., 2003). In sorghum, wheat, rice, tomato, grapes, and okra, water uptake through aquaporins is improved by foliar application of Si due to reduced production of H2O2 (Soylemezoglu et al., 2009; Liu P. et al., 2015; Abbas et al., 2017; Abdel-Haliem et al., 2017). Overall, these reports validate the pivotal role played by Si to mitigate the oxidative stress by accretion of defense proteins, enhancing activity of antioxidant enzymes, reduction in membrane damage, and overexpression of ROS-scavenging genes.
High-Throughput Approaches to Investigate Si-Induced Tolerance to Abiotic Stressors
Even though conventional breeding programs for combating abiotic stressors are achievable, the process is slow and tedious. The transfer of traits between species is incompatible and involves multiple backcrossing to screen desired traits out of thousands of genes (Ahmad and Mukhtar, 2017; Parmar et al., 2017). The traditional breeding methods are surmounted by genetic engineering techniques to precisely modify the gene of interest and its transfer to desired organisms (Singh and Singh, 2014). The genetic engineering approaches are aided by the advent of omics approaches to understand and identify genes/traits linked with the metabolic pathways critical to stress response, hence facilitating the transfer of identified traits to develop stress-tolerant crop plants (Mohanta et al., 2017).
High-throughput techniques, such as transcriptomics, proteomics, microarray analysis, and ionomics, have been widely used to identify differentially expressed genes or proteins in crop plants under control and stress conditions (Zargar et al., 2016; Khan et al., 2018; Yang and Guo, 2018; Afzal et al., 2022). The role of Si in alleviating stress is now obvious and has been reported to have a beneficial effect on plant growth and development under salt stress. However, the mechanism involved is not fully understood.
Transcriptomic studies are grossly employed to investigate the role of Si through the identification of a wide array of genes whose expression is altered in response to Si treatment under stress conditions. These genes are reportedly involved in solute transport, hormone biosynthesis and signaling, and biotic and abiotic stress response. A good percentage of differentially expressed genes in response to Si treatment are transcription factors (Zhu et al., 2019b; Arif et al., 2021). Liu P. et al. (2015) and Manivannan and Ahn (2017) reported that Si-upregulated SbPIP gene coding plasma membrane intrinsic protein (PIP) is vital for maintaining uptake of water by positively regulating the aquaporin activity and additionally enhanced salt tolerance in sorghum. Similarly, upregulation of stress-tolerant genes, such as Os03g57120, Os09g26810, and enhanced activity of S-adenosyl-L-methionine decarboxylase (SAMDC), to increase the synthesis of polyamines by Si is essential in mitigating the abiotic stressors (Manivannan and Ahn, 2017). The Si-upregulated expression of the OsRDCP1 gene led to a decrease in dehydration of plant cells, hence mitigating drought stress (Khattab et al., 2014).
Transcriptome studies revealed that Si mitigates stress by upregulating the expression of genes PsbY, PsaH, PsII, and LHC involved in modulating PSII, oxygen-evolving manganese subunit PSI, and light harvesting complex activities during the photosynthesis process (Song et al., 2014; Lesharadevi et al., 2021). In wheat, Si improves heavy metal stress tolerance by increasing gene expression of metallothionein synthase 1 (TaMT1) and phytochelatin synthase (TaPCS1), and, in pea, Si application improves heavy metal stress tolerance by upregulating the expression of MTA (metallothionein) and GSH1 (a precursor of phytochelatin) genes in roots (Kaushik and Saini, 2019; Arif et al., 2021). Si upregulates the expression of transcription factors, such as dehydration-responsive element-binding protein (DREB2) and NAC, involved in mitigating osmotic stress via the ABA independent pathway (Manivannan and Ahn, 2017; Almutairi, 2019). Comparative transcriptome studies by Zhu et al. (2019b) suggested that Si acts as an elicitor to induce salt stress in cucumber. They reported that, among the genes whose expression was altered (708 upregulated and 774 downregulated) under salt stress, the majority of them (609 and 595, respectively) reverted to the normal expression levels upon Si treatment, shifting the transcriptome of salt-stressed cucumber back to normal.
The transcriptome analysis of different tissues, such as flag leaves (FLs), spikelets (SPs), and node Is (NIs) of Cd-exposed rice after Si application, revealed that the gene expression profiles associated with the Si-mediated alleviation of Cd stress were tissue-specific. Si may alter the expression pattern of genes associated with transport, biosynthesis and metabolism, and oxidation-reduction. In Cd-exposed rice, the expression of ATP-binding cassette (ABC)-transporters, essential nutrient transporters, carbohydrate, secondary metabolite biosynthesis, and cytochrome oxidase activity were mostly upregulated in FL and SP with Si treatment, while the expression of bivalent cation transporters was mostly downregulated, possibly to reduce Cd accumulation (Sun et al., 2022). In another study, Hao et al. (2021) used RNA-seq technology to identify DEGs in wheat seedlings treated with Si. They reported that 3,057 genes related to transcription factors, chaperons, phenylpropanoid biosynthesis, and protein processing were differentially expressed upon Si treatment. Approximately, 28 differentially expressed transcription factors from the MYB TF family were downregulated under Si treatment.
The proteomics offers a new approach to discovering a myriad of stress-inducible proteins that respond to abiotic stresses at transcriptional and translational levels to identify proteins and understand the pathways associated with stress tolerance (Kosová et al., 2011, 2018). Understanding the key metabolic proteins and pathways of stress tolerance can help in biotechnological interventions for the development of varieties with improved stress tolerance. Abiotic stresses interfere with protein synthesis and cause loss of protein. Si has been reported to efficiently lower stress by upregulating the synthesis of a protein associated with signal transduction processes and antioxidant defense machinery. Proteomics studies have identified that, upon exposure of plants to Si, 17% stress-tolerant proteins, 11% proteins related to cellular and hormone biosynthesis, RNA synthesis, and synthesis of secondary metabolites are upregulated to alleviate abiotic stressors (Al Murad et al., 2020). Likewise, proteomic studies have aided in tracing the upregulation of proteins, such as RNA polymerase mediator, homologs of transcription elongation (SPT4), MADS-box TF, TFs for elongation, tRNA-lysidine synthase, ribosomal protein L16, ribosome-recycling factor, and reverse transcriptase, by Si to confer tolerance against abiotic stress in plants (Muneer and Jeong, 2015; Al Murad et al., 2020).
Furthermore, proteomic studies have confirmed that Si mitigates environmental stressors, including salinity and thermal stresses by inducing synthesis of β-carotene synthesis protein carotene desaturase, chaperone protein, such as ClpC3, CF1α subunit of ATP-synthase, cupin family protein phosphoglycerate kinase, glutelin, and β-keto acyl reductase, plasma membrane intrinsic protein (PIP1), and nodulin 26-like intrinsic protein (NIP2) (Choi, 2009; Cao et al., 2020b; Younis et al., 2020). Like other grasses, rice accumulates Si and stores most of it in leaves. Study of change in leaf proteome in Si-induced Cd tolerance in rice has revealed that Si significantly regulated synthesis of proteins involved in photosynthesis, redox homeostasis, protein synthesis regulation, chaperone activity, and pathogen response, class III peroxidase, disulfide isomerase, an NADH-ubiquinone oxidoreductase alone, or in combination with Cd. It suggests a strong role of Si in induced tolerance (Nwugo and Huerta, 2011). A similar study on Si-induced salinity stress tolerance in Capsicum annuum, Manivannan et al. (2016) carried out proteomic analysis by two-dimensional gel electrophoresis (2DE), followed by matrix-assisted laser desorption/ionization time-of-flight mass spectrometry (MALDI-TOF-MS), revealed that Si treatment upregulated the accumulation of proteins with nucleotide-binding and transferase activity involved in several metabolic processes and also modulated the expression of vital proteins involved in the ubiquitin-mediated nucleosome pathway and carbohydrate metabolism (Manivannan et al., 2016).
Metabolomic studies helped to identify the accumulation of metabolites critical to the growth, sustenance, and development of plants under the influence of Si to mitigate adverse abiotic stress conditions (Jana et al., 2019; Raza, 2020). The Si upregulated the metabolites that include ascorbate, glutathione, phenol, tocopherol, and flavonoids to attenuate the harmful effects of abiotic stressors such that normal growth and metabolism will be sustained (Metwally et al., 2018). Recent ionomic studies have revealed that Si application induced the accumulation of critical cofactors of enzymes, such as Cu, Fe, and Zn, to mitigate stressors (Banerjee et al., 2021). Si application increases the accumulation of several nutrients, such as N, P, K, Mn, Fe, Na, Ca, and Mg, which reduce stress-induced ionic damage, increase drought tolerance, and enhance plant growth (Ahanger et al., 2020b; Pavlovic et al., 2021).
These omics reports serve as the backbone for applying genetic engineering approaches to mediate resilience in transgenic crop plants. For instance, the development of transgenic cultivars of rice plants through a transfer of the Lsi1 gene modulated the physiological and molecular activities to mitigate arsenic stress upon exposure to Si (Boorboori et al., 2021). Likewise, overexpression of the VyDOF8 transcription factor gene derived from Chinese wild grapevine was reported to mitigate drought stress in transgenic tobacco plants (Li et al., 2021). Similarly, OsMADS57 TF was upregulated by Si, overexpressed in rice, and A. thaliana mitigated salinity stress. All these reports strongly suggest that genetic engineering technology speeds up the development of stress-resilient crop plants for food and nutritional security. The last few decades have witnessed shifting research on genome-editing technologies, such as ZFN, TALEN, and CRISPR/Cas systems, for higher accuracy and precision to develop desired crop plants with tolerance to different abiotic stressors (Mushtaq et al., 2021; Pandita, 2021). For instance, Li et al. (2019) employed CRISPR/Cas9 to mediate mutagenesis of SlNPR1 to enhance drought tolerance in tomatoes. Almost no other report is available on the editing of the Si-encoding genes; thus, in the future, the CRISPR/Cas system should be applied to Si-encoding genes to increase the tolerance against various abiotic stresses in major crop plants. We believe the application of high-throughput technologies will aid in speeding up the quest to develop stress-tolerant crop varieties for food and nutritional security (Varshney et al., 2018, 2020, 2021a,b; Pazhamala et al., 2021).
Conclusion and Future Perspectives
Even though the metabolic demand of Si is deficient, its presence in plant systems is critical to alleviating abiotic stress. Keeping in view the importance of Si to crop plants, the last decade witnessed many publications pertaining to its role in alleviating abiotic stress. Si is proven to play a vital role in combating abiotic stress conditions by positively modulating the physiological attributes of crop plants. Central to the role of Si is its upregulation of phytohormones and their signaling cascades operated to withstand abiotic stresses. Remarkably, Si is reported to impart more excellent resistance to abiotic stress factors through overexpression of genes regulating the synthesis of phytohormones. The stress tolerance mediated by Si-induced phytohormones is largely regulated through signal transduction pathways. Although the effect of Si to alleviate the abiotic stress in crop plants is variable, the multidimensional role of Si in plant development, such as the alleviation of sulfur deficiency, osmotic stress tolerance, alteration of genes related to glyceraldehydes-3-phosphate dehydrogenase, cyclophilin (CYC), ADP-Ribosylation Factor (ADP-RF1), and positively modulating metabolic pathways under abiotic stress, is well accustomed. The effect may vary concerning stress duration and intensity, in vitro methods of application, and cultivation. We conclude that Si can be integrated as a requisite part of fertilizers to benefit the crop plants in adverse ecological conditions. The focus on Si-based fertilizers may help to attain global sustainable agricultural growth.
The clear insights into the pivotal role of Si in circumventing the stress tolerance are still scanty, primarily due to a poor understanding of the molecular mechanism imparted by this multitalented element. Furthermore, extensive molecular investigation into the integrated effects of Si and phytohormones on alleviating the drought tolerance in plants is still at its early stage. In addition, the genes pertaining to the physiological improvements need to be further unveiled to authenticate the role of Si as the leading element in fertilizers for fortification and sustainable agricultural growth. Advanced omics technologies, such as transcriptomic, proteomic, and metabolomic studies, can further aid in-depth exploration of crosstalk of Si with phytohormones to unravel novel signaling molecules responsible for alleviating the stress tolerance in crop plants. In addition, special attention should be put on the role of Si in regulating signal transduction pathways under a wide range of abiotic stress conditions. Furthermore, shifting focus upon deciphering the role of Si in cop plants in felid trails rather than in controlled laboratory conditions is of dire need. In the future, the CRISPR/Cas system should be applied to Si-encoding genes to increase the tolerance against various abiotic stresses in major crop plants.
Author Contributions
RM and SZ conceived the idea. RM, BB, HY, SI, MR, MA, PS, SC, and AR collected the literature and participated in writing. RM, BB, AR, SI, and SZ proofread and edited the final version. All authors have read and approved the final version of the manuscript.
Funding
SZ acknowledges the support of the National Mission on Himalayan Studies (NMHS) implemented by the Ministry of Environment, Forest and Climate Change (MoEFCC) vide project sanction No.: GBPNI/NMHS-2017-18/SG24/622. SZ and PS also acknowledge the Hon’ble Vice-Chancellor SKUAST-K (Prof. Nazir A. Ganai) for his support.
Conflict of Interest
The authors declare that the research was conducted in the absence of any commercial or financial relationships that could be construed as a potential conflict of interest.
Publisher’s Note
All claims expressed in this article are solely those of the authors and do not necessarily represent those of their affiliated organizations, or those of the publisher, the editors and the reviewers. Any product that may be evaluated in this article, or claim that may be made by its manufacturer, is not guaranteed or endorsed by the publisher.
References
Abbas, T., Balal, R. M., Shahid, M. A., Pervez, M. A., Ayyub, C. M., Aqueel, M. A., et al. (2015). Silicon-induced alleviation of NaCl toxicity in okra (Abelmoschus esculentus) is associated with enhanced photosynthesis, osmoprotectants and antioxidant metabolism. Acta Physiol. Plant. 37:6.
Abbas, T., Sattar, A., Ijaz, M., Aatif, M., Khalid, S., and Sher, A. (2017). Exogenous silicon application alleviates salt stress in okra. Horticult. Environ. Biotechnol. 58, 342–349. doi: 10.1007/s13580-017-0247-5
Abdalla, M. M. (2011). Impact of diatomite nutrition on two Trifolium alexandrinum cultivars differing in salinity tolerance. Int. J. Plant Physiol. Biochem. 3, 233–246.
Abdelaal, K. A. A., Mazrou, Y. S. A., and Hafez, Y. M. (2020). Silicon foliar application mitigates salt stress in sweet pepper plants by enhancing water status, photosynthesis, antioxidant enzyme activity and fruit yield. Plants 9:733. doi: 10.3390/plants9060733
Abdel-Haliem, M. E. F., Hegazy, H. S., Hassan, N. S., and Naguib, D. M. (2017). Effect of silica ions and nano silica on rice plants under salinity stress. Ecol. Engine. 99, 282–289.
Abdelrahman, M., El-Sayed, M., Jogaiah, S., Burritt, D. J., and Tran, L.-S. P. (2017). The “STAY-GREEN” trait and phytohormone signaling networks in plants under heat stress. Plant Cell Rep. 36, 1009–1025. doi: 10.1007/s00299-017-2119-y
Adatia, M. H., and Besford, R. T. (1986). The effects of silicon on cucumber plants grown in recirculating nutrient solution. Ann. Bot. 58, 343–351. doi: 10.1093/oxfordjournals.aob.a087212
Adrees, M., Ali, S., Rizwan, M., Zia-ur-Rehman, M., Ibrahim, M., Abbas, F., et al. (2015). Mechanisms of silicon-mediated alleviation of heavy metal toxicity in plants: a review. Ecotoxicol. Environ. Safety 119, 186–197. doi: 10.1016/j.ecoenv.2015.05.011
Afzal, S., Chaudhary, N., Sharma, D., and Singh, N. K. (2022). Towards understanding abiotic stress physiological studies in plants: Conjunction of genomic and proteomic approaches. Plant Perspect. Glob. Clim. Changes 2022, 25–49. doi: 10.1016/b978-0-323-85665-2.00013-3
Agrawal, G. K., Jwa, N.-S., Agrawal, S. K., Tamogami, S., Iwahashi, H., and Rakwal, R. (2003). Cloning of novel rice allene oxide cyclase (OsAOC): mRNA expression and comparative analysis with allene oxide synthase (OsAOS) gene provides insight into the transcriptional regulation of octadecanoid pathway biosynthetic genes in rice. Plant Sci. 164, 979–992. doi: 10.1016/s0168-9452(03)00082-7
Ahanger, M. A., Aziz, U., Alsahli, A. A., Alyemeni, M. N., and Ahmad, P. (2020a). Influence of exogenous salicylic acid and nitric oxide on growth, photosynthesis, and ascorbate-glutathione cycle in salt stressed Vigna angularis. Biomolecules 10:42. doi: 10.3390/biom10010042
Ahanger, M. A., Bhat, J. A., Siddiqui, M. H., Rinklebe, J., and Ahmad, P. (2020b). Integration of silicon and secondary metabolites in plants: a significant association in stress tolerance. J. Exp. Bot. 71, 6758–6774. doi: 10.1093/jxb/eraa291
Ahanger, M. A., Qin, C., Maodong, Q., Dong, X. X., Ahmad, P., Abd_Allah, E. F., et al. (2019). Spermine application alleviates salinity induced growth and photosynthetic inhibition in Solanum lycopersicum by modulating osmolyte and secondary metabolite accumulation and differentially regulating antioxidant metabolism. Plant Physiol. Biochem. 144, 1–13. doi: 10.1016/j.plaphy.2019.09.021
Ahire, M. L., Mundada, P. S., Nikam, T. D., Bapat, V. A., and Penna, S. (2021). Multifaceted roles of silicon in mitigating environmental stresses in plants. Plant Physiol. Biochem. 169, 291–310. doi: 10.1016/j.plaphy.2021.11.010
Ahmad, A., Aslam, Z., Naz, M., Hussain, S., Javed, T., Aslam, S., et al. (2021). Exogenous salicylic acid-induced drought stress tolerance in wheat (Triticum aestivum L.) grown under hydroponic culture. PLoS One 16:e0260556. doi: 10.1371/journal.pone.0260556
Ahmad, N., and Mukhtar, Z. (2017). Genetic manipulations in crops: challenges and opportunities. Genomics 109, 494–505. doi: 10.1016/j.ygeno.2017.07.007
Ahmad, P., Ahanger, M. A., Alam, P., Alyemeni, M. N., Wijaya, L., Ali, S., et al. (2019). Silicon (Si) supplementation alleviates NaCl toxicity in mung bean [Vigna radiata (L.) Wilczek] through the modifications of physio-biochemical attributes and key antioxidant enzymes. J. Plant Growth Regul. 38, 70–82. doi: 10.1007/s00344-018-9810-2
Ahmad, P., and Jhon, R. (2005). Effect of salt stress on growth and biochemical parameters of Pisum sativum L. (Einfluss von Salzstress auf Wachstum und biochemische Parameter von Pisum sativum L.). Arch. Agron. Soil Sci. 51, 665–672.
Ahmed, M., Qadeer, U., and Aslam, M. A. (2011). Silicon application and drought tolerance mechanism of sorghum. Afr. J. Agricult. Res. 6, 594–607.
Akhtar, P., and Hussain, F. (2008). Salinity tolerance of three range grasses at germination and early growth stages. Pak. J. Bot. 40, 2437–2441.
Al Murad, M., Khan, A. L., and Muneer, S. (2020). Silicon in horticultural crops: cross-talk, signaling, and tolerance mechanism under salinity stress. Plants 9:460. doi: 10.3390/plants9040460
Al-aghabary, K., Zhu, Z., and Shi, Q. (2005). Influence of silicon supply on chlorophyll content, chlorophyll fluorescence, and antioxidative enzyme activities in tomato plants under salt stress. J. Plant Nutrit. 27, 2101–2115. doi: 10.1081/pln-200034641
Alam, A., Hariyanto, B., Ullah, H., Salin, K. R., and Datta, A. (2021). Effects of silicon on growth, yield and fruit quality of cantaloupe under drought stress. Silicon 13, 3153–3162. doi: 10.1007/s12633-020-00673-1
Alamri, S., Hu, Y., Mukherjee, S., Aftab, T., Fahad, S., Raza, A., et al. (2020). Silicon-induced postponement of leaf senescence is accompanied by modulation of antioxidative defense and ion homeostasis in mustard (Brassica juncea) seedlings exposed to salinity and drought stress. Plant Physiol. Biochem. 157, 47–59. doi: 10.1016/j.plaphy.2020.09.038
Al-Huqail, A. A., Alqarawi, A. A., Hashem, A., Malik, J. A., and Abd_Allah, E. F. (2019). Silicon supplementation modulates antioxidant system and osmolyte accumulation to balance salt stress in Acacia gerrardii Benth. Saudi J. Biol. Sci. 26, 1856–1864. doi: 10.1016/j.sjbs.2017.11.049
Ali, M., Afzal, S., Parveen, A., Kamran, M., Javed, M. R., Abbasi, G. H., et al. (2021). Silicon mediated improvement in the growth and ion homeostasis by decreasing Na+ uptake in maize (Zea mays L.) cultivars exposed to salinity stress. Plant Physiol. Biochem. 158, 208–218. doi: 10.1016/j.plaphy.2020.10.040
Ali, S., Farooq, M. A., Yasmeen, T., Hussain, S., Arif, M. S., Abbas, F., et al. (2013). The influence of silicon on barley growth, photosynthesis and ultra-structure under chromium stress. Ecotoxicol. Environ. Safety 89, 66–72. doi: 10.1016/j.ecoenv.2012.11.015
Almutairi, Z. M. T. (2019). Plant molecular defense mechanisms promoted by nanoparticles against environmental stress. Int. J. Agric. Biol. 21, 259–270. doi: 10.3390/ijms23041947
Alzahrani, Y., Kuşvuran, A., Alharby, H. F., Kuşvuran, S., and Rady, M. M. (2018). The defensive role of silicon in wheat against stress conditions induced by drought, salinity or cadmium. Ecotoxicol. Environ. Safety 154, 187–196. doi: 10.1016/j.ecoenv.2018.02.057
Arif, Y., Singh, P., Bajguz, A., Alam, P., and Hayat, S. (2021). Silicon mediated abiotic stress tolerance in plants using physio-biochemical, omic approach and cross-talk with phytohormones. Plant Physiol. Biochem. 166, 278–289. doi: 10.1016/j.plaphy.2021.06.002
Artyszak, A. (2018). Effect of silicon fertilization on crop yield quantity and quality—A literature review in Europe. Plants 7:54. doi: 10.3390/plants7030054
Asaad Bashir, M., Wang, X., Naveed, M., Mustafa, A., Ashraf, S., Samreen, T., et al. (2021). Biochar Mediated-Alleviation of Chromium Stress and Growth Improvement of Different Maize Cultivars in Tannery Polluted Soils. Int. J. Environ. Res. Public Health 18:4461. doi: 10.3390/ijerph18094461
Atta, B., Rizwan, M., Sabir, A. M., Gogi, M. D., and Ayub, M. A. (2019). Silicon mediated induced resistance in plants for the management of agricultural insect pests: A review. World J. Biol. Biotechnol. 4, 19–27. doi: 10.1016/j.pestbp.2020.104641
Azarfam, S., Nadian, H., Moezzi, A., and Gholami, A. (2020). Effect of silicon on phytochemical and medicinal properties of aloe vera under cold stress. Appl. Ecol. Environ. Res. 18, 561–575. doi: 10.15666/aeer/1801_561575
Bakhat, H. F., Bibi, N., Zia, Z., Abbas, S., Hammad, H. M., Fahad, S., et al. (2018). Silicon mitigates biotic stresses in crop plants: a review. Crop Protect. 104, 21–34.
Banerjee, A., Singh, A., Sudarshan, M., and Roychoudhury, A. (2021). Silicon nanoparticle-pulsing mitigates fluoride stress in rice by fine-tuning the ionomic and metabolomic balance and refining agronomic traits. Chemosphere 262:127826. doi: 10.1016/j.chemosphere.2020.127826
Bari, M. A., Prity, S. A., Das, U., Akther, M. S., Sajib, S. A., Reza, M. A., et al. (2020). Silicon induces phytochelatin and ROS scavengers facilitating cadmium detoxification in rice. Plant Biol. 22, 472–479. doi: 10.1111/plb.13090
Baryla, A., Carrier, P., Franck, F., Coulomb, C., Sahut, C., and Havaux, M. (2001). Leaf chlorosis in oilseed rape plants (Brassica napus) grown on cadmium-polluted soil: causes and consequences for photosynthesis and growth. Planta 212, 696–709. doi: 10.1007/s004250000439
Basu, S., and Kumar, G. (2021). Exploring the significant contribution of silicon in regulation of cellular redox homeostasis for conferring stress tolerance in plants. Plant Physiol. Biochem. 166, 393–404. doi: 10.1016/j.plaphy.2021.06.005
Benslima, W., Zorrig, W., Bagues, M., Abdelly, C., and Hafsi, C. (2021). Silicon mitigates potassium deficiency in Hordeum vulgare by improving growth and photosynthetic activity but not through polyphenol accumulation and the related antioxidant potential. Plant Soil 2021, 1–18.
Bhardwaj, S., and Kapoor, D. (2021). Fascinating regulatory mechanism of silicon for alleviating drought stress in plants. Plant Physiol. Biochem. 166, 1044–1053. doi: 10.1016/j.plaphy.2021.07.005
Bharwana, S. A., Ali, S., Farooq, M. A., Iqbal, N., Abbas, F., and Ahmad, M. S. A. (2013). Alleviation of lead toxicity by silicon is related to elevated photosynthesis, antioxidant enzymes suppressed lead uptake and oxidative stress in cotton. J. Bioremed. Biodeg. 4, 10–4172. doi: 10.1016/j.ecoenv.2013.07.006
Bijanzadeh, E., Barati, V., and Egan, T. P. (2022). Foliar application of sodium silicate mitigates drought stressed leaf structure in corn (Zea mays L.). South Afr. J. Bot. 147, 8–17. doi: 10.1016/j.sajb.2021.12.032
Bityutskii, N. P., Yakkonen, K. L., Petrova, A. I., and Shavarda, A. L. (2017). Interactions between aluminium, iron and silicon in Cucumber sativus L. grown under acidic conditions. J. Plant Physiol. 218, 100–108. doi: 10.1016/j.jplph.2017.08.003
Bokor, B., Bokorová, S., Ondoš, S., Švubová, R., Lukačová, Z., Hýblová, M., et al. (2015). Ionome and expression level of Si transporter genes (Lsi1, Lsi2, and Lsi6) affected by Zn and Si interaction in maize. Environ. Sci. Poll. Res. 22, 6800–6811. doi: 10.1007/s11356-014-3876-6
Boorboori, M. R., Lin, W., Jiao, Y., and Fang, C. (2021). Silicon Modulates Molecular and Physiological Activities in Lsi1 Transgenic and Wild Rice Seedlings under Arsenic Stress. Agronomy 11:1532. doi: 10.3390/agronomy11081532
Bosnic, P., Bosnic, D., Jasnic, J., and Nikolic, M. (2018). Silicon mediates sodium transport and partitioning in maize under moderate salt stress. Environ. Exp. Bot. 155, 681–687. doi: 10.1016/j.envexpbot.2018.08.018
Bradáčová, K., Weber, N. F., Morad-Talab, N., Asim, M., Imran, M., Weinmann, M., et al. (2016). Micronutrients (Zn/Mn), seaweed extracts, and plant growth-promoting bacteria as cold-stress protectants in maize. Chem. Biol. Technol. Agricult. 3, 1–10. doi: 10.1142/9781848160521_0001
Bukhari, M. A., Ahmad, Z., Ashraf, M. Y., Afzal, M., Nawaz, F., Nafees, M., et al. (2021a). Silicon mitigates drought stress in wheat (Triticum aestivum L.) through improving photosynthetic pigments, biochemical and yield characters. Silicon 13, 4757–4772. doi: 10.1007/s12633-020-00797-4
Bukhari, M. A., Sharif, M. S., Ahmad, Z., Barutçular, C., Afzal, M., Hossain, A., et al. (2021b). Silicon mitigates the adverse effect of drought in canola (Brassica napus L.) through promoting the physiological and antioxidants activity. Silicon 13, 3817–3826. doi: 10.1007/s12633-020-00685-x
Bybordi, A. (2015). Influence of exogenous application of silicon and potassium on physiological responses, yield, and yield components of salt-stressed wheat. Commun. Soil Sci. Plant Anal. 46, 109–122.
Caarls, L., Pieterse, C. M. J., and Van Wees, S. (2015). How salicylic acid takes transcriptional control over jasmonic acid signaling. Front. Plant Sci. 6:170. doi: 10.3389/fpls.2015.00170
Cao, B. L., Ma, Q., and Xu, K. (2020a). Silicon restrains drought-induced ROS accumulation by promoting energy dissipation in leaves of tomato. Protoplasma 257, 537–547.
Cao, F., Dai, H., Hao, P.-F., and Wu, F. (2020b). Silicon regulates the expression of vacuolar H+-pyrophosphatase 1 and decreases cadmium accumulation in rice (Oryza sativa L.). Chemosphere 240:124907.
Carneiro, J. M. T., Chacón-Madrid, K., Galazzi, R. M., Campos, B. K., Arruda, S. C. C., Azevedo, R. A., et al. (2017). Evaluation of silicon influence on the mitigation of cadmium-stress in the development of Arabidopsis thaliana through total metal content, proteomic and enzymatic approaches. J. Trace Elements Med. Biol. 44, 50–58.
Chalanika De Silva, H. C., and Asaeda, T. (2017). Effects of heat stress on growth, photosynthetic pigments, oxidative damage and competitive capacity of three submerged macrophytes. J. Plant Interact. 12, 228–236.
Chen, H., Bullock, D. A., Alonso, J. M., and Stepanova, A. N. (2022). To Fight or to Grow: The Balancing Role of Ethylene in Plant Abiotic Stress Responses. Plants 11:33.
Chen, W., Yao, X., Cai, K., and Chen, J. (2011). Silicon alleviates drought stress of rice plants by improving plant water status, photosynthesis and mineral nutrient absorption. Biol. Trace Element Res. 142, 67–76.
Choi, W.-G. (2009). Nodulin 26-like Intrinsic Protein NIP2; 1 and NIP7; 1: Characterization of Transport Functions and Roles in Developmental and Stress Responses in Arabidopsis. Knoxville, TN: University of Tennessee.
Colebrook, E. H., Thomas, S. G., Phillips, A. L., and Hedden, P. (2014). The role of gibberellin signalling in plant responses to abiotic stress. J. Exp. Biol. 217, 67–75.
Cooke, J., and Leishman, M. R. (2011). Is plant ecology more siliceous than we realise? Trends Plant Sci. 16, 61–68.
Cooke, J., and Leishman, M. R. (2016). Consistent alleviation of abiotic stress with silicon addition: a meta-analysis. Funct. Ecol. 30, 1340–1357.
Coskun, D., Britto, D. T., Huynh, W. Q., and Kronzucker, H. J. (2016). The role of silicon in higher plants under salinity and drought stress. Front. Plant Sci. 7:1072. doi: 10.3389/fpls.2016.01072
Coskun, D., Deshmukh, R., Sonah, H., Menzies, J. G., Reynolds, O., Ma, J. F., et al. (2019). The controversies of silicon’s role in plant biology. New Phytol. 221, 67–85.
Cui, J., Zhang, E., Zhang, X., and Wang, Q. (2021). Silicon alleviates salinity stress in licorice (Glycyrrhiza uralensis) by regulating carbon and nitrogen metabolism. Sci. Rep. 11, 1–12.
Daryanto, S., Wang, L., and Jacinthe, P. A. (2016). Global synthesis of drought effects on maize and wheat production. PloS one 11:e0156362.
da Cunha, K. P. V., and do Nascimento, C. W. A. (2009). Silicon effects on metal tolerance and structural changes in maize (Zea mays L.) grown on a cadmium and zinc enriched soil. Water Air Soil Poll. 197, 323–330.
da Silva, J. A. T., and Yücel, M. (2008). Revealing response of plants to biotic and abiotic stresses with microarray technology. Genes Genomes Genomics 2, 14–48.
Das, P., Manna, I., Sil, P., Bandyopadhyay, M., and Biswas, A. K. (2021). Silicon augments salt tolerance through modulation of polyamine and GABA metabolism in two indica rice (Oryza sativa L.) cultivars. Plant Physiol. Biochem. 166, 41–52.
de Oliveira, R. L. L., de Mello Prado, R., Felisberto, G., and Cruz, F. J. R. (2019). Different sources of silicon by foliar spraying on the growth and gas exchange in sorghum. J. Soil Sci. Plant Nutrit. 19, 948–953.
de Ollas, C., Hernando, B., Arbona, V., and Gómez-Cadenas, A. (2013). Jasmonic acid transient accumulation is needed for abscisic acid increase in citrus roots under drought stress conditions. Physiol. Plant. 147, 296–306.
Debona, D., Rodrigues, F. A., and Datnoff, L. E. (2017). Silicon’s role in abiotic and biotic plant stresses. Annu. Rev. Phytopathol. 55, 85–107.
Del Buono, D. (2021). Can biostimulants be used to mitigate the effect of anthropogenic climate change on agriculture? It is time to respond. Sci. Total Environ. 751:141763.
Deren, C. W. (2001). Plant genotype, silicon concentration, and silicon-related responses. Stud. Plant Sci. 8, 149–158.
Dey, P., Datta, D., Pattnaik, D., Dash, D., Saha, D., Panda, D., et al. (2022). Physiological, biochemical, and molecular adaptation mechanisms of photosynthesis and respiration under challenging environments. Plant Perspect. Glob. Clim. Changes 2022, 79–100.
Dhiman, P., Rajora, N., Bhardwaj, S., Sudhakaran, S. S., Kumar, A., Raturi, G., et al. (2021). Fascinating role of silicon to combat salinity stress in plants: An updated overview. Plant Physiol. Biochem. 162, 110–123.
Dorairaj, D., Ismail, M. R., Sinniah, U. R., and Kar Ban, T. (2017). Influence of silicon on growth, yield, and lodging resistance of MR219, a lowland rice of Malaysia. J. Plant Nutrit. 40, 1111–1124.
dos Santos, A. F. B., Teixeira, G. C. M., Campos, C. N. S., Baio, F. H. R., de Mello Prado, R., Teodoro, L. P. R., et al. (2020). Silicon increases chlorophyll and photosynthesis and improves height and NDVI of cotton (Gossypium hirsutum L. r. latifolium Hutch). Res. Soc. Dev. 9, e548973826–e548973826.
Du, Y., and Scheres, B. (2018). Lateral root formation and the multiple roles of auxin. J. Exp. Bot. 69, 155–167.
El Moukhtari, A., Carol, P., Mouradi, M., Savoure, A., and Farissi, M. (2021). Silicon improves physiological, biochemical, and morphological adaptations of alfalfa (Medicago sativa L.) during salinity stress. Symbiosis 2021, 1–20.
El-Mageed, A., Taia, A., Shaaban, A., El-Mageed, A., Shimaa, A., Semida, W. M., et al. (2021). Silicon defensive role in maize (Zea mays L.) against drought stress and metals-contaminated irrigation water. Silicon 13, 2165–2176.
El-Mashad, A. A. A., and Mohamed, H. I. (2012). Brassinolide alleviates salt stress and increases antioxidant activity of cowpea plants (Vigna sinensis). Protoplasma 249, 625–635.
El-Saadony, M. T., Desoky, E.-S. M., Saad, A. M., Eid, R. S. M., Selem, E., and Elrys, A. S. (2021). Biological silicon nanoparticles improve Phaseolus vulgaris L. yield and minimize its contaminant contents on a heavy metals-contaminated saline soil. J. Environ. Sci. 106, 1–14.
Emamverdian, A., Ding, Y., Xie, Y., and Sangari, S. (2018). Silicon mechanisms to ameliorate heavy metal stress in plants. BioMed Res. Int. 2018:8492898. doi: 10.1155/2018/8492898
Eraslan, F., Inal, A., Pilbeam, D. J., and Gunes, A. (2008). Interactive effects of salicylic acid and silicon on oxidative damage and antioxidant activity in spinach (Spinacia oleracea L. cv. Matador) grown under boron toxicity and salinity. Plant Growth Regul. 55, 207–219.
Eremina, M., Rozhon, W., and Poppenberger, B. (2016). Hormonal control of cold stress responses in plants. Cell. Mol. Life Sci. 73, 797–810.
Etesami, H., and Jeong, B. R. (2018). Silicon (Si): Review and future prospects on the action mechanisms in alleviating biotic and abiotic stresses in plants. Ecotoxicol. Environ. Safety 147, 881–896.
Fahad, S., Hussain, S., Bano, A., Saud, S., Hassan, S., Shan, D., et al. (2015a). Potential role of phytohormones and plant growth-promoting rhizobacteria in abiotic stresses: consequences for changing environment. Environ. Sci. Poll. Res. 22, 4907–4921.
Fahad, S., Hussain, S., Matloob, A., Khan, F. A., Khaliq, A., Saud, S., et al. (2015b). Phytohormones and plant responses to salinity stress: a review. Plant Growth Regulat. 75, 391–404.
Farmer, V. C. (2005). Forest vegetation does recycle substantial amounts of silicon from and back to the soil solution with phytoliths as an intermediate phase, contrary to recent reports. Eur. J. Soil Sci. 56, 271–272.
Farooq, M. A., Detterbeck, A., Clemens, S., and Dietz, K. J. (2016). Silicon-induced reversibility of cadmium toxicity in rice. J. Exp. Bot. 67, 3573–3585.
Fei, Q., Liang, L., Li, F., Zhang, L., Li, Y., Guo, Y., et al. (2019). Transcriptome profiling and phytohormone responses of Arabidopsis roots to different ambient temperatures. J. Plant Interact. 14, 314–323.
Feng, J., Yamaji, N., and Mitani-Ueno, N. (2011). Transport of silicon from roots to panicles in plants. Proc. Jap. Acad. Ser. B 87, 377–385.
Ferchichi, S., Hessini, K., Dell’Aversana, E., D’Amelia, L., Woodrow, P., Ciarmiello, L. F., et al. (2018). Hordeum vulgare and Hordeum maritimum respond to extended salinity stress displaying different temporal accumulation pattern of metabolites. Funct. Plant Biol. 45, 1096–1109.
Fiala, R., Fialová, I., Vaculík, M., and Luxová, M. (2021). Effect of silicon on the young maize plants exposed to nickel stress. Plant Physiol. Biochem. 166, 645–656.
Flam-Shepherd, R., Huynh, W. Q., Coskun, D., Hamam, A. M., Britto, D. T., and Kronzucker, H. J. (2018). Membrane fluxes, bypass flows, and sodium stress in rice: the influence of silicon. J. Exp. Bot. 69, 1679–1692.
Fragkostefanakis, S., Mesihovic, A., Simm, S., Paupière, M. J., Hu, Y., Paul, P., et al. (2016). HsfA2 controls the activity of developmentally and stress-regulated heat stress protection mechanisms in tomato male reproductive tissues. Plant Physiol. 170, 2461–2477.
Gangwar, S., Singh, V. P., Tripathi, D. K., Chauhan, D. K., Prasad, S. M., and Maurya, J. N. (2014). Plant responses to metal stress: the emerging role of plant growth hormones in toxicity alleviation. Emerg. Technol. Manage. Crop Stress Toler. 2014, 215–248.
Garg, N., and Singh, S. (2018). Arbuscular mycorrhiza Rhizophagus irregularis and silicon modulate growth, proline biosynthesis and yield in Cajanus cajan L. Millsp.(pigeonpea) genotypes under cadmium and zinc stress. J. Plant Growth Regul. 37, 46–63.
Gfeller, A., Baerenfaller, K., Loscos, J., Chételat, A., Baginsky, S., and Farmer, E. E. (2011). Jasmonate controls polypeptide patterning in undamaged tissue in wounded Arabidopsis leaves. Plant Physiol. 156, 1797–1807.
Gill, S. S., and Tuteja, N. (2010). Reactive oxygen species and antioxidant machinery in abiotic stress tolerance in crop plants. Plant Physiol. Biochem. 48, 909–930.
Gómez-Merino, F. C., Trejo-Téllez, L. I., García-Jiménez, A., Escobar-Sepúlveda, H. F., and Ramírez-Olvera, S. M. (2020). Silicon flow from root to shoot in pepper: a comprehensive in silico analysis reveals a potential linkage between gene expression and hormone signaling that stimulates plant growth and metabolism. PeerJ 8:e10053.
Gong, H., Zhu, X., Chen, K., Wang, S., and Zhang, C. (2005). Silicon alleviates oxidative damage of wheat plants in pots under drought. Plant Sci. 169, 313–321.
Gou, T., Chen, X., Han, R., Liu, J., Zhu, Y., and Gong, H. (2020). Silicon can improve seed germination and ameliorate oxidative damage of bud seedlings in cucumber under salt stress. Acta Physiol. Plant. 42, 1–11.
Greger, M., Kabir, A. H., Landberg, T., Maity, P. J., and Lindberg, S. (2016). Silicate reduces cadmium uptake into cells of wheat. Environ. Poll. 211, 90–97.
Greger, M., Landberg, T., and Vaculík, M. (2018). Silicon influences soil availability and accumulation of mineral nutrients in various plant species. Plants 7:41.
Gujjar, R. S., Akhtar, M., and Singh, M. (2014). Transcription factors in abiotic stress tolerance. Ind. J. Plant Physiol. 19, 306–316.
Gunes, A., Pilbeam, D. J., Inal, A., Bagci, E. G., and Coban, S. (2007). Influence of silicon on antioxidant mechanisms and lipid peroxidation in chickpea (Cicer arietinum L.) cultivars under drought stress. J. Plant Interact. 2, 105–113.
Guntzer, F., Keller, C., and Meunier, J.-D. (2012). Benefits of plant silicon for crops: a review. Agron. Sustain. Dev. 32, 201–213.
Gupta, B. K., Sahoo, K. K., Anwar, K., Nongpiur, R. C., Deshmukh, R., Pareek, A., et al. (2021). Silicon nutrition stimulates Salt-Overly Sensitive (SOS) pathway to enhance salinity stress tolerance and yield in rice. Plant Physiol. Biochem. 166, 593–604.
Habibi, G. (2015). Effects of soil-and foliar-applied silicon on the resistance of grapevine plants to freezing stress. Acta Biol. Szegediensis 59, 109–117.
Habibi, G., and Hajiboland, R. J. F. H. (2013). Alleviation of drought stress by silicon supplementation in pistachio (Pistacia vera L.) Plants. Folia Horticult. 25, 21–29.
Haddad, C., Arkoun, M., Jamois, F., Schwarzenberg, A., Yvin, J.-C., Etienne, P., et al. (2018). Silicon promotes growth of Brassica napus L. and delays leaf senescence induced by nitrogen starvation. Front. Plant Sci. 9:516. doi: 10.3389/fpls.2018.00516
Haddad, C., Trouverie, J., Arkoun, M., Yvin, J.-C., Caïus, J., Brunaud, V., et al. (2019). Silicon supply affects the root transcriptome of Brassica napus L. Planta 249, 1645–1651.
Hafeez, M. B., Zahra, N., Zahra, K., Raza, A., Khan, A., Shaukat, K., et al. (2021b). Brassinosteroids: molecular and physiological responses in plant growth and abiotic stresses. Plant Stress 2:100029.
Hafeez, M. B., Raza, A., Zahra, N., Shaukat, K., Akram, M. Z., Iqbal, S., et al. (2021a). Gene regulation in halophytes in conferring salt tolerance. Handb. Bioremed. 2021, 341–370.
Haider, S., Iqbal, J., Naseer, S., Yaseen, T., Shaukat, M., Bibi, H., et al. (2021a). Molecular mechanisms of plant tolerance to heat stress: current landscape and future perspectives. Plant Cell Rep. 2021, 1–25.
Haider, S., Rehman, S., Ahmad, Y., Raza, A., Tabassum, J., Javed, T., et al. (2021b). In Silico Characterization and Expression Profiles of Heat Shock Transcription Factors (HSFs) in Maize (Zea mays L.). Agronomy 11:2335.
Hamayun, M., Sohn, E. Y., Khan, S. A., Shinwari, Z. K., Khan, A. L., and Lee, I. J. (2010). Silicon alleviates the adverse effects of salinity and drought stress on growth and endogenous plant growth hormones of soybean (Glycine max L.). Pak. J. Bot. 42, 1713–1722.
Hameed, A., Sheikh, M. A., Jamil, A., and Basra, S. M. A. (2013). Seed priming with sodium silicate enhances seed germination and seedling growth in wheat (Triticum aestivum L.) under water deficit stress induced by polyethylene glycol. Pak. J. Life Soc. Sci. 11, 19–24.
Han, W., Jia, J., Hu, Y., Liu, J., Guo, J., Shi, Y., et al. (2021). Maintenance of root water uptake contributes to salt-tolerance of a wild tomato species under salt stress. Arch. Agron. Soil Sci. 67, 205–217.
Hao, L., Shi, S., Guo, H., Zhang, J., Li, P., and Feng, Y. (2021). Transcriptome analysis reveals differentially expressed MYB transcription factors associated with silicon response in wheat. Sci. Rep. 11, 1–9.
Hara, M., Furukawa, J., Sato, A., Mizoguchi, T., and Miura, K. (2012). Abiotic stress and role of salicylic acid in plants. Abiotic Stress Responses Plants 2021, 235–251.
Hasan, M. M., Gong, L., Nie, Z.-F., Li, F.-P., Ahammed, G. J., and Fang, X.-W. (2021). ABA-induced stomatal movements in vascular plants during dehydration and rehydration. Environ. Exp. Bot. 2021:104436.
Hasanuzzaman, M., Bhuyan, M. H. M., Zulfiqar, F., Raza, A., Mohsin, S. M., Mahmud, J. A., et al. (2020). Reactive oxygen species and antioxidant defense in plants under abiotic stress: revisiting the crucial role of a universal defense regulator. Antioxidants 9:681.
Hasanuzzaman, M., Nahar, K., Anee, T. I., and Fujita, M. (2017). Exogenous silicon attenuates cadmium-induced oxidative stress in Brassica napus L. by modulating AsA-GSH pathway and glyoxalase system. Front. Plant Sci. 8:1061. doi: 10.3389/fpls.2017.01061
Haseeb, M., Iqbal, S., Hafeez, M. B., Saddiq, M. S., Zahra, N., Raza, A., et al. (2022). Phytoremediation of nickel by quinoa: Morphological and physiological response. PLoS One 17:e0262309. doi: 10.1371/journal.pone.0262309
Hasegawa, P. M., Bressan, R. A., Zhu, J. K., and Bohnert, H. J. (2000). Plant cellular and molecular responses to high salinity. Annu. Rev. Plant Biol. 51, 463–499.
Hattori, T., Inanaga, S., Tanimoto, E., Lux, A., Luxová, M., and Sugimoto, Y. (2003). Silicon-induced changes in viscoelastic properties of sorghum root cell walls. Plant Cell Physiol. 44, 743–749.
Hosseini, S. A., Maillard, A., Hajirezaei, M. R., Ali, N., Schwarzenberg, A., Jamois, F., et al. (2017). Induction of Barley Silicon Transporter HvLsi1 and HvLsi2, increased silicon concentration in the shoot and regulated Starch and ABA Homeostasis under Osmotic stress and Concomitant Potassium Deficiency. Front. Plant Sci. 8:1359. doi: 10.3389/fpls.2017.01359
Hu, H., You, J., Fang, Y., Zhu, X., Qi, Z., and Xiong, L. (2008). Characterization of transcription factor gene SNAC2 conferring cold and salt tolerance in rice. Plant Mol. Biol. 67, 169–181.
Hu, J., Li, Y., and Jeong, B. R. (2020). Silicon alleviates temperature stresses in poinsettia by regulating stomata, photosynthesis, and oxidative damages. Agronomy 10:1419.
Hu, W., Su, Y., Zhou, J., Zhu, H., Guo, J., Huo, H., et al. (2022). Foliar application of silicon and selenium improves the growth, yield and quality characteristics of cucumber in field conditions. Sci. Horticult. 294:110776.
Huang, J., Zhao, X., Bürger, M., Wang, Y., and Chory, J. (2021). Two interacting ethylene response factors regulate heat stress response. Plant Cell 33, 338–357.
Hurtado, A. C., Chiconato, D. A., de Mello Prado, R., da Silveira Sousa Junior, G., Viciedo, D. O., Díaz, Y. P., et al. (2021). Silicon alleviates sodium toxicity in Sorghum and sunflower plants by enhancing ionic homeostasis in roots and shoots and increasing dry matter accumulation. Silicon 13, 475–486.
Hurtado, A. C., Chiconato, D. A., de Mello Prado, R., Junior, G. D. S. S., Gratão, P. L., Felisberto, G., et al. (2020). Different methods of silicon application attenuate salt stress in sorghum and sunflower by modifying the antioxidative defense mechanism. Ecotoxicol. Environ. Safety 203:110964.
Hussain, I., Ashraf, M. A., Rasheed, R., Asghar, A., Sajid, M. A., and Iqbal, M. (2015). Exogenous application of silicon at the boot stage decreases accumulation of cadmium in wheat (Triticum aestivum L.) grains. Brazil. J. Bot. 38, 223–234.
Hussain, I., Parveen, A., Rasheed, R., Ashraf, M. A., Ibrahim, M., Riaz, S., et al. (2019). Exogenous Silicon Modulates Growth, Physio-Chemicals and Antioxidants in Barley (Hordeum vulgare L.) Exposed to Different Temperature Regimes. Silicon 11, 2753–2762.
Hussain, M., Ahmad, S., Hussain, S., Lal, R., Ul-Allah, S., and Nawaz, A. (2018). Rice in saline soils: physiology, biochemistry, genetics, and management. Adv. Agronomy 148, 231–287.
Hussain, S., Khan, F., Hussain, H. A., and Nie, L. (2016). Physiological and biochemical mechanisms of seed priming-induced chilling tolerance in rice cultivars. Front. Plant Sci. 7:116. doi: 10.3389/fpls.2016.00116
Hwang, S.-J., Hamayun, M., Kim, H.-Y., Na, C.-I., Kim, K.-U., Shin, D.-H., et al. (2007). Effect of nitrogen and silicon nutrition on bioactive gibberellin and growth of rice under field conditions. J. Crop Sci. Biotechnol. 10, 281–286.
Ilyas, M., Nisar, M., Khan, N., Hazrat, A., Khan, A. H., Hayat, K., et al. (2021). Drought tolerance strategies in plants: A mechanistic approach. J. Plant Growth Regul. 40, 926–944.
Imran, M., Mahmood, A., Römheld, V., and Neumann, G. (2013). Nutrient seed priming improves seedling development of maize exposed to low root zone temperatures during early growth. Eur. J. Agronomy 49, 141–148.
Imtiaz, M., Rizwan, M. S., Mushtaq, M. A., Ashraf, M., Shahzad, S. M., Yousaf, B., et al. (2016). Silicon occurrence, uptake, transport and mechanisms of heavy metals, minerals and salinity enhanced tolerance in plants with future prospects: a review. J. Environ. Manage. 183, 521–529.
Islam, M. S., and Wang, M.-H. (2009). Expression of dehydration responsive element-binding protein-3 (DREB3) under different abiotic stresses in tomato. BMB Rep. 42, 611–616.
Ito, Y., Katsura, K., Maruyama, K., Taji, T., Kobayashi, M., Seki, M., et al. (2006). Functional analysis of rice DREB1/CBF-type transcription factors involved in cold-responsive gene expression in transgenic rice. Plant Cell Physiol. 47, 141–153.
Iwasaki, K., Maier, P., Fecht, M., and Horst, W. J. (2002). Leaf apoplastic silicon enhances manganese tolerance of cowpea (Vigna unguiculata). J. Plant Physiol. 159, 167–173.
Jana, G. A., Al Kharusi, L., Sunkar, R., Al-Yahyai, R., and Yaish, M. W. (2019). Metabolomic analysis of date palm seedlings exposed to salinity and silicon treatments. Plant Signal. Behav. 14:1663112.
Janni, M., Gullì, M., Maestri, E., Marmiroli, M., Valliyodan, B., Nguyen, H. T., et al. (2020). Molecular and genetic bases of heat stress responses in crop plants and breeding for increased resilience and productivity. J. Exp. Bot. 71, 3780–3802.
Joudmand, A., and Hajiboland, R. (2019). Silicon mitigates cold stress in barley plants via modifying the activity of apoplasmic enzymes and concentration of metabolites. Acta Physiol. Plant. 41:29.
Kafi, M., Nabati, J., Ahmadi-Lahijani, M. J., and Oskoueian, A. (2021). Silicon compounds and potassium sulfate improve salinity tolerance of potato plants through instigating the defense mechanisms, cell membrane stability, and accumulation of osmolytes. Commun. Soil Sci. Plant Anal. 52, 843–858. doi: 10.1080/00103624.2020.1869768
Kaloterakis, N., van Delden, S. H., Hartley, S., and De Deyn, G. B. (2021). Silicon application and plant growth promoting rhizobacteria consisting of six pure Bacillus species alleviate salinity stress in cucumber (Cucumis sativus L). Sci. Horticult. 288:110383. doi: 10.1016/j.scienta.2021.110383
Kamenya, S. N., Mikwa, E. O., Song, B., and Odeny, D. A. (2021). Genetics and breeding for climate change in Orphan crops. Theoret. Appl. Genet. 2021, 1–29.
Kang, J., Zhao, W., Zhao, M., Zheng, Y., and Yang, F. (2015). NaCl and Na 2 SiO 3 coexistence strengthens growth of the succulent xerophyte Nitraria tangutorum under drought. Plant Growth Regulat. 77, 223–232. doi: 10.1007/s10725-015-0055-9
Kapoor, D., Bhardwaj, S., Landi, M., Sharma, A., Ramakrishnan, M., and Sharma, A. (2020). The impact of drought in plant metabolism: how to exploit tolerance mechanisms to increase crop production. Appl. Sci. 10:5692. doi: 10.1111/pce.12417
Kaushik, P., and Saini, D. K. (2019). Silicon as a vegetable crops modulator—A review. Plants 8:148. doi: 10.3390/plants8060148
Kaya, C., Ashraf, M., Al-Huqail, A. A., Alqahtani, M. A., and Ahmad, P. (2020). Silicon is dependent on hydrogen sulphide to improve boron toxicity tolerance in pepper plants by regulating the AsA-GSH cycle and glyoxalase system. Chemosphere 257:127241. doi: 10.1016/j.chemosphere.2020.127241
Kaya, C., Tuna, L., and Higgs, D. (2006). Effect of silicon on plant growth and mineral nutrition of maize grown under water-stress conditions. J. Plant Nutrit. 29, 1469–1480.
Kazan, K. (2015). Diverse roles of jasmonates and ethylene in abiotic stress tolerance. Trends Plant Sci. 20, 219–229. doi: 10.1016/j.tplants.2015.02.001
Keeping, M. G., and Reynolds, O. L. (2009). Silicon in agriculture: new insights, new significance and growing application. Ann. Appl. Biol. 155:153. doi: 10.1111/j.1744-7348.2009.00358.x
Keller, C., Rizwan, M., Davidian, J. C., Pokrovsky, O. S., Bovet, N., Chaurand, P., et al. (2015). Effect of silicon on wheat seedlings (Triticum turgidum L.) grown in hydroponics and exposed to 0 to 30 μM Cu. Planta 241, 847–860. doi: 10.1007/s00425-014-2220-1
Khan, A., Bilal, S., Khan, A. L., Imran, M., Shahzad, R., Al-Harrasi, A., et al. (2020a). Silicon and Gibberellins: Synergistic Function in Harnessing ABA Signaling and Heat Stress Tolerance in Date Palm (Phoenix dactylifera L.). Plants 9:620. doi: 10.3390/plants9050620
Khan, A., Khan, A. L., Imran, M., Asaf, S., Kim, Y.-H., Bilal, S., et al. (2020b). Silicon-induced thermotolerance in Solanum lycopersicum L. via activation of antioxidant system, heat shock proteins, and endogenous phytohormones. BMC Plant Biol. 20:7. doi: 10.1186/s12870-020-02456-7
Khan, A., Khan, A. L., Muneer, S., Kim, Y.-H., Al-Rawahi, A., and Al-Harrasi, A. (2019). Silicon and salinity: crosstalk in crop-mediated stress tolerance mechanisms. Front. Plant Sci. 10:1429. doi: 10.3389/fpls.2019.01429
Khan, M. I. R., Ashfaque, F., Chhillar, H., Irfan, M., and Khan, N. A. (2021b). The intricacy of silicon, plant growth regulators and other signaling molecules for abiotic stress tolerance: An entrancing crosstalk between stress alleviators. Plant Physiol. Biochem. 162, 36–47. doi: 10.1016/j.plaphy.2021.02.024
Khan, I., Awan, S. A., Rizwan, M., Ali, S., Hassan, M. J., Brestic, M., et al. (2021a). Effects of silicon on heavy metal uptake at the soil-plant interphase: A review. Ecotoxicol. Environ. Safety 222:112510. doi: 10.1016/j.ecoenv.2021.112510
Khan, S.-A., Li, M.-Z., Wang, S.-M., and Yin, H.-J. (2018). Revisiting the role of plant transcription factors in the battle against abiotic stress. Int. J. Mol. Sci. 19:1634. doi: 10.3390/ijms19061634
Khattab, H. I., Emam, M. A., Emam, M. M., Helal, N. M., and Mohamed, M. R. (2014). Effect of selenium and silicon on transcription factors NAC5 and DREB2A involved in drought-responsive gene expression in rice. Biol. Plant. 58, 265–273.
Kidokoro, S., Maruyama, K., Nakashima, K., Imura, Y., Narusaka, Y., Shinwari, Z. K., et al. (2009). The phytochrome-interacting factor PIF7 negatively regulates DREB1 expression under circadian control in Arabidopsis. Plant Physiol. 151, 2046–2057. doi: 10.1104/pp.109.147033
Kim, Y. H., Khan, A. L., Waqas, M., Shim, J. K., Kim, D. H., Lee, K. Y., et al. (2014c). Silicon application to rice root zone influenced the phytohormonal and antioxidant responses under salinity stress. J. Plant Growth Regul. 33, 137–149. doi: 10.1007/s00344-013-9356-2
Kim, Y.-H., Khan, A. L., Waqas, M., Jeong, H.-J., Kim, D.-H., Shin, J. S., et al. (2014b). Regulation of jasmonic acid biosynthesis by silicon application during physical injury to Oryza sativa L. J. Plant Res. 127, 525–532. doi: 10.1007/s10265-014-0641-3
Kim, Y.-H., Khan, A. L., Kim, D.-H., Lee, S.-Y., Kim, K.-M., Waqas, M., et al. (2014a). Silicon mitigates heavy metal stress by regulating P-type heavy metal ATPases, Oryza sativa low silicon genes, and endogenous phytohormones. BMC Plant Biol. 14, 1–13. doi: 10.1186/1471-2229-14-13
Kim, Y.-H., Khan, A. L., and Lee, I.-J. (2016). Silicon: a duo synergy for regulating crop growth and hormonal signaling under abiotic stress conditions. Crit. Rev. Biotechnol. 36, 1099–1109. doi: 10.3109/07388551.2015.1084265
Kim, Y.-H., Khan, A. L., Hamayun, M., Kang, S. M., Beom, Y. J., and Lee, I.-J. (2011). Influence of short-term silicon application on endogenous physiohormonal levels of Oryza sativa L. under wounding stress. Biol. Trace Element Res. 144, 1175–1185. doi: 10.1007/s12011-011-9047-4
Kosová, K., Vítámvás, P., Prášil, I. T., and Renaut, J. (2011). Plant proteome changes under abiotic stress—contribution of proteomics studies to understanding plant stress response. J. Proteom. 74, 1301–1322. doi: 10.1016/j.jprot.2011.02.006
Kosová, K., Vítámvás, P., Urban, M. O., Prášil, I. T., and Renaut, J. (2018). Plant abiotic stress proteomics: the major factors determining alterations in cellular proteome. Front. Plant Sci. 9:122. doi: 10.3389/fpls.2018.00122
Kramell, R., Atzorn, R., Schneider, G., Miersch, O., Brückner, C., Schmidt, J., et al. (1995). Occurrence and identification of jasmonic acid and its amino acid conjugates induced by osmotic stress in barley leaf tissue. J. Plant Growth Regul. 14, 29–36. doi: 10.1007/bf00212643
Kumar, A., and Kaushik, P. (2021). Heat Stress and its Impact on Plant Function: An Update. [Preprint].
Kumar, J., Singh, S., Singh, M., Srivastava, P. K., Mishra, R. K., Singh, V. P., et al. (2017). Transcriptional regulation of salinity stress in plants: A short review. Plant Gene 11, 160–169.
Kutasy, E., Buday-Bódi, E., Virág, I. C., Forgács, F., Melash, A. A., Zsombik, L., et al. (2022). Mitigating the Negative Effect of Drought Stress in Oat (Avena sativa L.) with Silicon and Sulphur Foliar Fertilization. Plants 11:30. doi: 10.3390/plants11010030
Law, C., and Exley, C. (2011). New insight into silica deposition in horsetail (Equisetum arvense). BMC Plant Biol. 11:112. doi: 10.1186/1471-2229-11-112
Lee, B.-H., Henderson, D. A., and Zhu, J.-K. (2005). The Arabidopsis cold-responsive transcriptome and its regulation by ICE1. Plant Cell 17, 3155–3175. doi: 10.1105/tpc.105.035568
Lee, S. K., Sohn, E. Y., Hamayun, M., Yoon, J. Y., and Lee, I. J. (2010). Effect of silicon on growth and salinity stress of soybean plant grown under hydroponic system. Agroforestry Syst. 80, 333–340. doi: 10.1007/s10457-010-9299-6
Lenka, S. K., Katiyar, A., Chinnusamy, V., and Bansal, K. C. (2011). Comparative analysis of drought-responsive transcriptome in Indica rice genotypes with contrasting drought tolerance. Plant Biotechnol. J. 9, 315–327. doi: 10.1111/j.1467-7652.2010.00560.x
Lesharadevi, K., Parthasarathi, T., and Muneer, S. (2021). Silicon biology in crops under abiotic stress: A paradigm shift and cross-talk between genomics and proteomics. J. Biotechnol. 333, 21–38. doi: 10.1016/j.jbiotec.2021.04.008
Li, G., Xu, W., Jing, P., Hou, X., and Fan, X. (2021). Overexpression of VyDOF8, a Chinese wild grapevine transcription factor gene, enhances drought tolerance in transgenic tobacco. Environ. Exp. Bot. 190:104592. doi: 10.1016/j.envexpbot.2021.104592
Li, H., Zhu, Y., Hu, Y., Han, W., and Gong, H. (2015). Beneficial effects of silicon in alleviating salinity stress of tomato seedlings grown under sand culture. Acta Physiol. Plant. 37:71.
Li, J., Leisner, S. M., and Frantz, J. (2008). Alleviation of copper toxicity in Arabidopsis thaliana by silicon addition to hydroponic solutions. J. Am. Soc. Horticult. Sci. 133, 670–677. doi: 10.21273/jashs.133.5.670
Li, P., Zhao, C., Zhand, Y., Wang, X., Wang, J., Wang, F., et al. (2017). Silicon enhances the tolerance of Poa annua to cadmium by inhibiting its absorption and oxidative stress. Biol. Plant. 61, 741–750. doi: 10.1007/s10535-017-0731-x
Li, R., Liu, C., Zhao, R., Wang, L., Chen, L., Yu, W., et al. (2019). CRISPR/Cas9-Mediated SlNPR1 mutagenesis reduces tomato plant drought tolerance. BMC Plant Biol. 19, 1–13. doi: 10.1186/s12870-018-1627-4
Li, X., Wei, J.-P., Scott, E. R., Liu, J.-W., Guo, S., Li, Y., et al. (2018). Exogenous melatonin alleviates cold stress by promoting antioxidant defense and redox homeostasis in Camellia sinensis L. Molecules 23:165. doi: 10.3390/molecules23010165
Liang, Y., Nikolic, M., Bélanger, R., Gong, H., and Song, A. (2015b). Effect of silicon on crop growth, yield and quality. Silicon Agricult. 2015, 209–223. doi: 10.1007/978-94-017-9978-2_11
Liang, Y., Nikolic, M., Bélanger, R., Gong, H., and Song, A. (2015c). Silicon in agriculture, Vol. 10. Dordrecht: Springer, 978–994. doi: 10.1007/978-94-017-9978-2
Liang, X., Wang, H., Hu, Y., Mao, L., Sun, L., Dong, T., et al. (2015a). Silicon does not mitigate cell death in cultured tobacco BY-2 cells subjected to salinity without ethylene emission. Plant Cell Rep. 34, 331–343. doi: 10.1007/s00299-014-1712-6
Liang, Y., Chen, Q. I. N., Liu, Q., Zhang, W., and Ding, R. (2003). Exogenous silicon (Si) increases antioxidant enzyme activity and reduces lipid peroxidation in roots of salt-stressed barley (Hordeum vulgareL.). J. Plant Physiol. 160, 1157–1164. doi: 10.1078/0176-1617-01065
Liang, Y., Li, X., Yang, R., Gao, B., Yao, J., Oliver, M. J., et al. (2021). BaDBL1, a unique DREB gene from desiccation tolerant moss Bryum argenteum, confers osmotic and salt stress tolerances in transgenic Arabidopsis. Plant Sci. 313:111047. doi: 10.1016/j.plantsci.2021.111047
Liang, Y., Sun, W., Zhu, Y.-G., and Christie, P. J. E. (2007). Mechanisms of silicon-mediated alleviation of abiotic stresses in higher plants: a review. Environ. Pollut. 147, 422–428. doi: 10.1016/j.envpol.2006.06.008
Liang, Y., Wong, J., and Wei, L. J. C. (2005). Silicon-mediated enhancement of cadmium tolerance in maize (Zea mays L.) grown in cadmium contaminated soil. Chemosphere 58, 475–483. doi: 10.1016/j.chemosphere.2004.09.034
Liu, B., Soundararajan, P., and Manivannan, A. (2019). Mechanisms of silicon-mediated amelioration of salt stress in plants. Plants 8:307. doi: 10.3390/plants8090307
Liu, H., and Timko, M. P. (2021). Jasmonic Acid Signaling and Molecular Crosstalk with Other Phytohormones. Int. J. Mol. Sci. 22:2914. doi: 10.3390/ijms22062914
Liu, H., Shen, J., Yuan, C., Lu, D., Acharya, B. R., Wang, M., et al. (2021). The Cyclophilin ROC3 Regulates ABA-Induced Stomatal Closure and the Drought Stress Response of Arabidopsis thaliana. Front. Plant Sci. 12:668792. doi: 10.3389/fpls.2021.668792
Liu, P., Yin, L., Wang, S., Zhang, M., Deng, X., Zhang, S., et al. (2015). Enhanced root hydraulic conductance by aquaporin regulation accounts for silicon alleviated salt-induced osmotic stress in Sorghum bicolor L. Environ. Exp. Bot. 111, 42–51. doi: 10.1016/j.envexpbot.2014.10.006
Liu, J., Feng, L., Li, J., and He, Z. (2015). Genetic and epigenetic control of plant heat responses. Front. Plant Sci. 6:267. doi: 10.3389/fpls.2015.00267
Liu, J.-J., Lin, S.-H., Xu, P.-L., Wang, X.-J., and Bai, J.-G. (2009). Effects of exogenous silicon on the activities of antioxidant enzymes and lipid peroxidation in chilling-stressed cucumber leaves. Agricult. Sci. China 8, 1075–1086. doi: 10.1016/s1671-2927(08)60315-6
Liu, Q., Kasuga, M., Sakuma, Y., Abe, H., Miura, S., Yamaguchi-Shinozaki, K., et al. (1998). Two transcription factors, DREB1 and DREB2, with an EREBP/AP2 DNA binding domain separate two cellular signal transduction pathways in drought-and low-temperature-responsive gene expression, respectively, in Arabidopsis. Plant Cell 10, 1391–1406. doi: 10.1105/tpc.10.8.1391
Lotfi, R., and Ghassemi-Golezani, K. (2015). Influence of salicylic acid and silicon on seed development and quality of mung bean under salt stress. Seed Sci. Technol. 43, 52–61. doi: 10.15258/sst.2015.43.1.06
Lozano-González, J. M., Valverde, C., Hernández, C. D., Martin-Esquinas, A., and Hernández-Apaolaza, L. (2021). Beneficial Effect of Root or Foliar Silicon Applied to Cucumber Plants under Different Zinc Nutritional Statuses. Plants 10:2602. doi: 10.3390/plants10122602
Luyckx, M., Hausman, J.-F., Lutts, S., and Guerriero, G. (2017). Silicon and plants: current knowledge and technological perspectives. Front. Plant Sci. 8:411. doi: 10.3389/fpls.2017.00411
Ma, D., Sun, D., Wang, C., Qin, H., Ding, H., Li, Y., et al. (2016). Silicon application alleviates drought stress in wheat through transcriptional regulation of multiple antioxidant defense pathways. J. Plant Growth Regul. 35, 1–10. doi: 10.1007/s00344-015-9500-2
Ma, J., Yamaji, N. J. C., and Sciences, M. L. (2008). Functions and transport of silicon in plants. Cell Mol. Life Sci. 65, 3049–3057. doi: 10.1007/s00018-008-7580-x
Ma, Y., Cao, J., He, J., Chen, Q., Li, X., and Yang, Y. (2018). Molecular mechanism for the regulation of ABA homeostasis during plant development and stress responses. Int. J. Mol. Sci. 19:3643. doi: 10.3390/ijms19113643
Maillard, A., Ali, N., Schwarzenberg, A., Jamois, F., Yvin, J.-C., and Hosseini, S. A. (2018). Silicon transcriptionally regulates sulfur and ABA metabolism and delays leaf senescence in barley under combined sulfur deficiency and osmotic stress. Environ. Exp. Bot. 155, 394–410. doi: 10.1016/j.envexpbot.2018.07.026
Majeed Zargar, S., Ahmad Macha, M., Nazir, M., Kumar Agrawal, G., and Rakwal, R. (2012). Silicon: A Multitalented Micronutrient in OMICS Perspective–An Update. Curr. Proteom. 9, 245–254. doi: 10.2174/157016412805219152
Majeed Zargar, S., Nazir, M., Kumar Agrawal, G., Kim, D.-W., and Rakwal, R. (2010). Silicon in plant tolerance against environmental stressors: towards crop improvement using omics approaches. Curr. Proteom. 7, 135–143. doi: 10.2174/157016410791330507
Malik, M. A., Wani, A. H., Mir, S. H., Rehman, I. U., Tahir, I., Ahmad, P., et al. (2021). Elucidating the role of silicon in drought stress tolerance in plants. Plant Physiol. Biochem. 165, 187–195. doi: 10.1016/j.plaphy.2021.04.021
Mandlik, R., Thakral, V., Raturi, G., Shinde, S., Nikoliæ, M., Tripathi, D. K., et al. (2020). Significance of silicon uptake, transport, and deposition in plants. J. Exp. Bot. 71, 6703–6718. doi: 10.1093/jxb/eraa301
Manivannan, A., and Ahn, Y.-K. (2017). Silicon regulates potential genes involved in major physiological processes in plants to combat stress. Front. Plant Sci. 8:1346. doi: 10.3389/fpls.2017.01346
Manivannan, A., Soundararajan, P., Muneer, S., Ko, C. H., and Jeong, B. R. (2016). Silicon mitigates salinity stress by regulating the physiology, antioxidant enzyme activities, and protein expression in Capsicum annuum ‘Bugwang’. BioMed Res. Int. 2016:076357. doi: 10.1155/2016/3076357
Manna, M., Thakur, T., Chirom, O., Mandlik, R., Deshmukh, R., and Salvi, P. (2021). Transcription factors as key molecular target to strengthen the drought stress tolerance in plants. Physiol. Plant. 172, 847–868. doi: 10.1111/ppl.13268
Markovich, O., Steiner, E., Kouøil, Š, Tarkowski, P., Aharoni, A., and Elbaum, R. (2017). Silicon promotes cytokinin biosynthesis and delays senescence in Arabidopsis and Sorghum. Plant Cell Environ. 40, 1189–1196. doi: 10.1111/pce.12913
Mehmood, S., Wang, X., Ahmed, W., Imtiaz, M., Ditta, A., Rizwan, M., et al. (2021). Removal mechanisms of slag against potentially toxic elements in soil and plants for sustainable agriculture development: a critical review. Sustainability 13:5255. doi: 10.3390/su13095255
Meng, X., Luo, S., Dawuda, M. M., Gao, X., Wang, S., Xie, J., et al. (2021). Exogenous silicon enhances the systemic defense of cucumber leaves and roots against CA-induced autotoxicity stress by regulating the ascorbate-glutathione cycle and photosystem II. Ecotoxicol. Environ. Safety 227:112879. doi: 10.1016/j.ecoenv.2021.112879
Merewitz, E. B., and Liu, S. (2019). Improvement in heat tolerance of creeping Bentgrass with melatonin, Rutin, and silicon. J. Am. Soc. Horticult. Sci. 144, 141–148. doi: 10.21273/jashs04643-19
Metwally, A. M., Radi, A. A., El-Shazoly, R. M., and Hamada, A. M. (2018). The role of calcium, silicon and salicylic acid treatment in protection of canola plants against boron toxicity stress. J. Plant Res. 131, 1015–1028. doi: 10.1007/s10265-018-1008-y
Mir, R. A., Bhat, K. A., Shah, A. A., and Zargar, S. M. (2020). Role of Silicon in Abiotic Stress Tolerance of Plants. Improving Abiotic Stress Toler. Plants 2020, 271–290. doi: 10.1201/9780429027505-15
Mir, S. H., Rashid, I., Hussain, B., Reshi, Z. A., Assad, R., and Sofi, I. A. (2019). Silicon supplementation of rescuegrass reduces herbivory by a grasshopper. Front. Plant Sci. 10:671. doi: 10.3389/fpls.2019.00671
Mitani, N., and Ma, J. F. (2005). Uptake system of silicon in different plant species. J. Exp. Bot. 56, 1255–1261. doi: 10.1093/jxb/eri121
Mitani, N., Yamaji, N., Ago, Y., Iwasaki, K., and Ma, J. F. (2011). Isolation and functional characterization of an influx silicon transporter in two pumpkin cultivars contrasting in silicon accumulation. Plant J. 66, 231–240. doi: 10.1111/j.1365-313X.2011.04483.x
Miura, K., and Furumoto, T. (2013). Cold signaling and cold response in plants. Int. J. Mol. Sci. 14, 5312–5337. doi: 10.3390/ijms14035312
Miura, K., and Tada, Y. (2014). Regulation of water, salinity, and cold stress responses by salicylic acid. Front. Plant Sci. 5:4. doi: 10.3389/fpls.2014.00004
Mohanta, T. K., Bashir, T., Hashem, A., and AbduAllah, E. F. (2017). Systems biology approach in plant abiotic stresses. Plant Physiol. Biochem. 121, 58–73. doi: 10.1016/j.plaphy.2017.10.019
Moradtalab, N., Weinmann, M., Walker, F., Höglinger, B., Ludewig, U., and Neumann, G. (2018). Silicon improves chilling tolerance during early growth of maize by effects on micronutrient homeostasis and hormonal balances. Front. Plant Sci. 9:420. doi: 10.3389/fpls.2018.00420
Mostofa, M. G., Rahman, M. M., Ansary, M. M. U., Keya, S. S., Abdelrahman, M., Miah, M. G., et al. (2021). Silicon in mitigation of abiotic stress-induced oxidative damage in plants. Crit. Rev. Biotechnol. 41, 918–934. doi: 10.1080/07388551.2021.1892582
Mubarik, M. S., Khan, S. H., Sajjad, M., Raza, A., Hafeez, M. B., Yasmeen, T., et al. (2021). A manipulative interplay between positive and negative regulators of phytohormones: A way forward for improving drought tolerance in plants. Physiol. Plant. 172, 1269–1290. doi: 10.1111/ppl.13325
Müller, M. (2021). Foes or friends: ABA and ethylene interaction under abiotic stress. Plants 10:448. doi: 10.3390/plants10030448
Mundada, P. S., Jadhav, S. V., Salunkhe, S. S., Gurme, S. T., Umdale, S. D., Barmukh, R. B., et al. (2021). Silicon and Plant Responses Under Adverse Environmental Conditions. Plant Perform. Under Environ. Stress 2021, 357–385. doi: 10.1007/978-3-030-78521-5_14
Muneer, S., and Jeong, B. R. (2015). Proteomic analysis of salt-stress responsive proteins in roots of tomato (Lycopersicon esculentum L.) plants towards silicon efficiency. Plant Growth Regulat. 77, 133–146. doi: 10.1007/s10725-015-0045-y
Munns, R., and Gilliham, M. J. (2015). Salinity tolerance of crops–what is the cost? New Phytol. 208, 668–673. doi: 10.1111/nph.13519
Mushtaq, M., Dar, A. A., Basu, U., Bhat, B. A., Mir, R. A., Vats, S., et al. (2021). Integrating CRISPR-Cas and Next Generation Sequencing in Plant Virology. Front. Genet. 12:735489. doi: 10.3389/fgene.2021.735489
Mustafa, T., Sattar, A., Sher, A., Ul-Allah, S., Ijaz, M., Irfan, M., et al. (2021). Exogenous application of silicon improves the performance of wheat under terminal heat stress by triggering physio-biochemical mechanisms. Sci. Rep. 11, 1–12. doi: 10.1038/s41598-021-02594-4
Nakashima, K., Takasaki, H., Mizoi, J., Shinozaki, K., and Yamaguchi-Shinozaki, K. J. B. (2012). NAC transcription factors in plant abiotic stress responses. Biochim. Biophys. Acta 1819, 97–103.
Naz, R., Batool, S., Shahid, M., Keyani, R., Yasmin, H., Nosheen, A., et al. (2021a). Exogenous silicon and hydrogen sulfide alleviates the simultaneously occurring drought stress and leaf rust infection in wheat. Plant Physiol. Biochem. 166, 558–571. doi: 10.1016/j.plaphy.2021.06.034
Naz, R., Gul, F., Zahoor, S., Nosheen, A., Yasmin, H., Keyani, R., et al. (2021b). Interactive effects of hydrogen sulphide and silicon enhance drought and heat tolerance by modulating hormones, antioxidant defence enzymes and redox status in barley (Hordeum vulgare L.). Plant Biol. 10, 1435–8603. doi: 10.1111/plb.13374
Nazli, F., Mustafa, A., Ahmad, M., Hussain, A., Jamil, M., Wang, X., et al. (2020). A Review on Practical Application and Potentials of Phytohormone-Producing Plant Growth-Promoting Rhizobacteria for Inducing Heavy Metal Tolerance in Crops. Sustainability 12:9056. doi: 10.3390/su12219056
Neu, S., Schaller, J., and Dudel, E. G. (2017). Silicon availability modifies nutrient use efficiency and content. C: N: P stoichiometry, and productivity of winter wheat. Sci. Rep. 7, 1–8. doi: 10.1038/srep40829
Neumann, D., and Zur Nieden, U. (2001). Silicon and heavy metal tolerance of higher plants. Phytochemistry 56, 685–692. doi: 10.1016/s0031-9422(00)00472-6
Ngwepe, R. M., Mashilo, J., Shimelis, H. J. G. R., and Evolution, C. (2019). Progress in genetic improvement of citron watermelon (Citrullus lanatus var. citroides): a review. Genet. Resour. Crop Evol. 66, 735–758. doi: 10.1007/s10722-018-0724-4
Noreen, S., Ashraf, M., Hussain, M., and Jamil, A. (2009). Exogenous application of salicylic acid enhances antioxidative capacity in salt stressed sunflower (Helianthus annuus L.) plants. Pak. J. Bot. 41, 473–479.
Nosrati, H., Roshandel, P., and Mohamadkhani, A. R. (2014). The role of silicon to increase salt tolerance in red and white varieties of bean (Phaseolus vulgaris L.). J. Plant Sci. Res. 30:81.
Nwugo, C. C., and Huerta, A. J. (2011). The effect of silicon on the leaf proteome of rice (Oryza sativa L.) plants under cadmium-stress. J. Proteome Res. 10, 518–528. doi: 10.1021/pr100716h
Ouellette, S., Goyette, M.-H., Labbé, C., Laur, J., Gaudreau, L., Gosselin, A., et al. (2017). Silicon transporters and effects of silicon amendments in strawberry under high tunnel and field conditions. Front. Plant Sci. 8, 1664–1462. doi: 10.3389/fpls.2017.00949
Pandita, D. (2021). “CRISPR/Cas-mediated genome editing for improved stress tolerance in plants,” in Frontiers in Plant-Soil Interaction. Elsevier 2021, 259–291. doi: 10.1016/b978-0-323-90943-3.00001-8
Parmar, N., Singh, K. H., Sharma, D., Singh, L., Kumar, P., Nanjundan, J., et al. (2017). Genetic engineering strategies for biotic and abiotic stress tolerance and quality enhancement in horticultural crops: a comprehensive review. 3 Biotech 7, 1–35. doi: 10.1007/s13205-017-0870-y
Patel, M., Fatnani, D., and Parida, A. K. (2021). Silicon-induced mitigation of drought stress in peanut genotypes (Arachis hypogaea L.) through ion homeostasis, modulations of antioxidative defense system, and metabolic regulations. Plant Physiol. Biochem. 166, 290–313. doi: 10.1016/j.plaphy.2021.06.003
Pavlovic, J., Kostic, L., Bosnic, P., Kirkby, E. A., and Nikolic, M. (2021). Interactions of silicon with essential and beneficial elements in plants. Front. Plant Sci. 12:1224. doi: 10.3389/fpls.2021.697592
Pazhamala, L. T., Kudapa, H., Weckwerth, W., Millar, A. H., and Varshney, R. K. (2021). Systems biology for crop improvement. Plant Genome 10, 1–23. doi: 10.1002/tpg2.20098
Pereira, T. S., da Silva Lobato, A. K., Tan, D. K. Y., da Costa, D. V., Uchoa, E. B., and do Nascimento, et al. (2013). Positive interference of silicon on water relations, nitrogen metabolism, and osmotic adjustment in two pepper (‘capsicum annuum’) cultivars under water deficit. Austral. J. Crop Sci. 7, 1064–1071.
Pfannschmidt, T., and Yang, C. (2012). The hidden function of photosynthesis: a sensing system for environmental conditions that regulates plant acclimation responses. Protoplasma 249, 125–136. doi: 10.1007/s00709-012-0398-2
Pieterse, C. M. J., Van der Does, D., Zamioudis, C., Leon-Reyes, A., and Van Wees, S. C. M. (2012). Hormonal modulation of plant immunity. Annu. Rev. Cell Dev. Biol. 28, 489–521. doi: 10.1146/annurev-cellbio-092910-154055
Pirooz, P., Amooaghaie, R., Ahadi, A., and Sharififar, F. (2021). Silicon-induced nitric oxide burst modulates systemic defensive responses of Salvia officinalis under copper toxicity. Plant Physiol. Biochem. 162, 752–761. doi: 10.1016/j.plaphy.2021.02.048
Poór, P., Nawaz, K., Gupta, R., Ashfaque, F., and Khan, M. I. R. (2021). Ethylene involvement in the regulation of heat stress tolerance in plants. Plant Cell Rep. 13, 1–24. doi: 10.1007/s00299-021-02675-8
Pottosin, I., and Shabala, S. J. F. (2014). Polyamines control of cation transport across plant membranes: implications for ion homeostasis and abiotic stress signaling. Front. Plant Sci. 5:154. doi: 10.3389/fpls.2014.00154
Prakash, V., Singh, V. P., Tripathi, D. K., Sharma, S., and Corpas, F. J. (2021). Nitric oxide (NO) and salicylic acid (SA): A framework for their relationship in plant development under abiotic stress. Plant Biol. 23, 39–49. doi: 10.1111/plb.13246
Qian, Z. Z., Zhuang, S. Y., Li, Q., and Gui, R. Y. (2019). Soil Silicon Amendment Increases Phyllostachys praecox Cold Tolerance in a Pot Experiment. Forests 10:405. doi: 10.3390/f10050405
Rahman, A., Wallis, C. M., and Uddin, W. (2015). Silicon-induced systemic defense responses in perennial ryegrass against infection by Magnaporthe oryzae. Phytopathology 105, 748–757. doi: 10.1094/PHYTO-12-14-0378-R
Rahman, M. F., Ghosal, A., Alam, M. F., and Kabir, A. H. (2017). Remediation of cadmium toxicity in field peas (Pisum sativum L.) through exogenous silicon. Ecotoxicol. Environ. Safety 135, 165–172. doi: 10.1016/j.ecoenv.2016.09.019
Rai, K. K., Pandey, N., and Rai, S. P. (2020). Salicylic acid and nitric oxide signaling in plant heat stress. Physiol. Plant. 168, 241–255. doi: 10.1111/ppl.12958
Rajasheker, G., Jawahar, G., Jalaja, N., Kumar, S. A., Kumari, P. H., Punita, D. L., et al. (2019). Role and regulation of osmolytes and ABA interaction in salt and drought stress tolerance. Plant Signal. Mol. 2019, 417–436. doi: 10.1016/b978-0-12-816451-8.00026-5
Rasoolizadeh, A., Labbé, C., Sonah, H., Deshmukh, R. K., Belzile, F., Menzies, J. G., et al. (2018). Silicon protects soybean plants against Phytophthora sojae by interfering with effector-receptor expression. BMC Plant Biol. 18:7. doi: 10.1186/s12870-018-1312-7
Rastogi, A., Yadav, S., Hussain, S., Kataria, S., Hajihashemi, S., Kumari, P., et al. (2021). Does silicon really matter for the photosynthetic machinery in plants? Plant Physiol. Biochem. 169, 40–48. doi: 10.1016/j.plaphy.2021.11.004
Ratcliffe, S., Jugdaohsingh, R., Vivancos, J., Marron, A., Deshmukh, R., Ma, J. F., et al. (2017). Identification of a mammalian silicon transporter. Am. J. Physiol. Cell Physiol. 312, C550–C561. doi: 10.1152/ajpcell.00219.2015
Raza, A. (2020). Metabolomics: a systems biology approach for enhancing heat stress tolerance in plants. Plant Cell Rep. 2020, 1–23. doi: 10.1007/s00299-020-02635-8
Raza, A. (2021). Eco-physiological and Biochemical Responses of Rapeseed (Brassica napus L.) to Abiotic Stresses: Consequences and Mitigation Strategies. J. Plant Growth Regul. 40, 1368–1388. doi: 10.1007/s00344-020-10231-z
Raza, A. (2022). Plant biotechnological tools: Solutions for raising climate-resilient crop plants. Modern Phytomorphol. 15, 132–133.
Raza, A., Ashraf, F., Zou, X., Zhang, X., and Tosif, H. (2020a). Plant Adaptation and Tolerance to Environmental Stresses: Mechanisms and Perspectives. Plant Ecophysiol. Adaptat. Under Clim. Change Mechanisms Perspect. 1, 117–145. doi: 10.1007/978-981-15-2156-0_5
Raza, A., Charagh, S., Sadaqat, N., and Jin, W. (2020b). Arabidopsis thaliana: Model Plant for the Study of Abiotic Stress Responses. Plant Fam. Brassicaceae 2020, 129–180. doi: 10.1007/978-981-15-6345-4_3
Raza, A., Su, W., Hussain, M. A., Mehmood, S. S., Zhang, X., Cheng, Y., et al. (2021e). Integrated analysis of metabolome and transcriptome reveals insights for cold tolerance in rapeseed (Brassica napus L.). Front. Plant Sci. 12:721681. doi: 10.3389/fpls.2021.721681
Raza, A., Tabassum, J., Mubarik, M., Anwar, S., Zahra, N., Sharif, Y., et al. (2021g). Hydrogen sulfide: an emerging component against abiotic stress in plants. Plant Biol. 2021, 1435–8603. doi: 10.1111/plb.13368
Raza, A., Charagh, S., Zahid, Z., Mubarik, M. S., Javed, R., Siddiqui, M. H., et al. (2021b). Jasmonic acid: a key frontier in conferring abiotic stress tolerance in plants. Plant Cell Rep. 40, 1513–1541. doi: 10.1007/s00299-020-02614-z
Raza, A., Charagh, S., Najafi-Kakavand, S., and Siddiqui, M. H. (2021a). The Crucial Role of Jasmonates in Enhancing Heavy Metals Tolerance in Plants. Jasmonates Salicylates Signal. Plants 2021, 159–183. doi: 10.1007/s00299-017-2168-2
Raza, A., Habib, M., Charagh, S., and Kakavand, S. N. (2021c). Genetic engineering of plants to tolerate toxic metals and metalloids. Handb. Bioremed. 2021, 411–436. doi: 10.1016/b978-0-12-819382-2.00026-0
Raza, A., Hussain, S., Javed, R., Hafeez, M. B., and Hasanuzzaman, M. (2021d). Antioxidant Defense Systems and Remediation of Metal Toxicity in Plants. Approaches Remediat. Inorganic Poll. 2021, 91–124. doi: 10.1007/978-981-15-6221-1_6
Raza, A., Tabassum, J., Kudapa, H., and Varshney, R. K. (2021f). Can omics deliver temperature resilient ready-to-grow crops? Crit. Rev. Biotechnol. 41, 1209–1232. doi: 10.1080/07388551.2021.1898332
Raza, A., Mehmood, S. S., Tabassum, J., and Batool, R. (2019). Targeting plant hormones to develop abiotic stress resistance in wheat. Wheat Product. Changing Environ. 2019, 557–577. doi: 10.1007/978-981-13-6883-7_22
Raza, A., Tabassum, J., Zahid, Z., Charagh, S., Bashir, S., Barmukh, R., et al. (2022). Advances in “Omics” Approaches for Improving Toxic Metals/Metalloids Tolerance in Plants. Front. Plant Sci. 12:794373. doi: 10.3389/fpls.2021.794373
Rehman, M. U., Ilahi, H., Adnan, M., Wahid, F., Rehman, F. U., Ullah, A., et al. (2021). Application of Silicon: A Useful Way to Mitigate Drought Stress: An Overview. Curr. Res. Agric. Farm. 2, 9–17. doi: 10.18782/2582-7146.134
Rémus-Borel, W., Menzies, J. G., and Bélanger, R. R. (2005). Silicon induces antifungal compounds in powdery mildew-infected wheat. Physiol. Mol. Plant Pathol. 66, 108–115. doi: 10.1016/j.pmpp.2005.05.006
Rizwan, M., Ali, S., Ibrahim, M., Farid, M., Adrees, M., Bharwana, S. A., et al. (2015). Mechanisms of silicon-mediated alleviation of drought and salt stress in plants: a review. Environ. Sci. Poll. Res. 22, 15416–15431. doi: 10.1007/s11356-015-5305-x
Rogalla, H., Römheld, V. J. P., and Cell, and Environment. (2002). Role of leaf apoplast in silicon-mediated manganese tolerance of Cucumis sativus L. Plant Cell Environ. 25, 549–555. doi: 10.1046/j.1365-3040.2002.00835.x
Sabagh, A. E., Mbarki, S., Hossain, A., Iqbal, M., Islam, M., Raza, A., et al. (2021). Potential Role of Plant Growth Regulators in Administering Crucial Processes Against Abiotic Stresses. Front. Agronomy 3:648694. doi: 10.3389/fagro.2021.648694
Saddiq, M. S., Afzal, I., Iqbal, S., Hafeez, M. B., and Raza, A. (2021). Low leaf sodium content improves the grain yield and physiological performance of wheat genotypes in saline-sodic soil. Pesquisa Agropecuária Tropical 51, e67663–e67663.
Saha, G., Mostofa, M. G., Rahman, M. M., and Tran, L.-S. P. (2021). Silicon-mediated heat tolerance in higher plants: a molecular outlook. Plant Physiol. Biochem. 166, 341–347. doi: 10.1016/j.plaphy.2021.05.051
Sakuma, Y., Maruyama, K., Osakabe, Y., Qin, F., Seki, M., Shinozaki, K., et al. (2006). Functional analysis of an Arabidopsis transcription factor, DREB2A, involved in drought-responsive gene expression. Plant Cell 18, 1292–1309. doi: 10.1105/tpc.105.035881
Saleem, M., Fariduddin, Q., and Janda, T. (2021). Multifaceted role of salicylic acid in combating cold stress in plants: a review. J. Plant Growth Regul. 40, 464–485. doi: 10.1007/s00344-020-10152-x
Salim, B., Abou El-Yazied, A., Salama, Y., Raza, A., and Osman, H. S. (2021). Impact of silicon foliar application in enhancing antioxidants, growth, flowering and yield of squash plants under deficit irrigation condition. Ann. Agricult. Sci. 66, 176–183.
Santos, M. R. D., Martinez, M. A., Donato, S. L. R., and Coelho, E. F. (2014). ‘Tommy Atkins’ mango yield and photosynthesis under water deficit in semiarid region of Bahia. Rev. Brasil. Engenharia Agrícola Ambiental 18, 899–907.
Sato, M., Tsuda, K., Wang, L., Coller, J., Watanabe, Y., Glazebrook, J., et al. (2010). Network modeling reveals prevalent negative regulatory relationships between signaling sectors in Arabidopsis immune signaling. PLoS Pathog. 6:e1001011. doi: 10.1371/journal.ppat.1001011
Sattar, A., Cheema, M. A., Sher, A., Ijaz, M., Ul-Allah, S., Nawaz, A., et al. (2019). Physiological and biochemical attributes of bread wheat (Triticum aestivum L.) seedlings are influenced by foliar application of silicon and selenium under water deficit. Acta Physiol. Plant. 41, 1–11.
Savant, N. K., Korndörfer, G. H., Datnoff, L. E., and Snyder, G. H. (1999). Silicon nutrition and sugarcane production: a review. J. Plant Nutrit. 22, 1853–1903.
Savatin, D. V., Gramegna, G., Modesti, V., and Cervone, F. (2014). Wounding in the plant tissue: the defense of a dangerous passage. Front. Plant Sci. 5:470. doi: 10.3389/fpls.2014.00470
Shah, A. A., Yasin, N. A., Akram, K., Ahmad, A., Khan, W. U., Akram, W., et al. (2021). Ameliorative role of Bacillus subtilis FBL-10 and silicon against lead induced stress in Solanum melongena. Plant Physiol. Biochem. 158, 486–496. doi: 10.1016/j.plaphy.2020.11.037
Sharma, L., Priya, M., Kaushal, N., Bhandhari, K., Chaudhary, S., Dhankher, O. P., et al. (2020). Plant growth-regulating molecules as thermoprotectants: functional relevance and prospects for improving heat tolerance in food crops. J. Exp. Bot. 71, 569–594. doi: 10.1093/jxb/erz333
Sharma, S. S., and Dietz, K.-J. J. T. (2009). The relationship between metal toxicity and cellular redox imbalance. Trends Plant Sci. 14, 43–50. doi: 10.1016/j.tplants.2008.10.007
Shen, X., Xiao, X., Dong, Z., and Chen, Y. J. A. P. P. (2014). Silicon effects on antioxidative enzymes and lipid peroxidation in leaves and roots of peanut under aluminum stress. Acta Physiol. Plant. 36, 3063–3069.
Shi, Y., Wang, Y., Flowers, T. J., and Gong, H. (2013). Silicon decreases chloride transport in rice (Oryza sativa L.) in saline conditions. J. Plant Physiol. 170, 847–853. doi: 10.1016/j.jplph.2013.01.018
Shi, Y., Zhang, Y., Yao, H., Wu, J., Sun, H., and Gong, H. (2014). Silicon improves seed germination and alleviates oxidative stress of bud seedlings in tomato under water deficit stress. Plant Physiol. Biochem. 78, 27–36. doi: 10.1016/j.plaphy.2014.02.009
Shimo, H., Ishimaru, Y., An, G., Yamakawa, T., Nakanishi, H., and Nishizawa, N. K. J. J. (2011). Low cadmium (LCD), a novel gene related to cadmium tolerance and accumulation in rice. J. Exp. Bot. 62, 5727–5734. doi: 10.1093/jxb/err300
Sierra, M. J., Schmid, T., Guirado, M., Escolano, O., and Millán, R. (2021). How management practices affect silicon uptake by Hordeum vulgare grown in a highly calcareous soil. Soil Use Manage. 2021:12723.
Sil, P., Das, P., and Biswas, A. K. (2018). Silicon induced mitigation of TCA cycle and GABA synthesis in arsenic stressed wheat (Triticum aestivum L.) seedlings. South Afr. J. Bot. 119, 340–352.
Singh, H. P., and Singh, B. P. (2014). Genetic engineering of field, industrial and pharmaceutical crops. Am. J. Plant Sci. 5:3974.
Singh, K., and Chandra, A. (2021). DREBs-potential transcription factors involve in combating abiotic stress tolerance in plants. Biologia 76, 3043–3055.
Singh, S., Koyama, H., Bhati, K. K., and Alok, A. (2021). The biotechnological importance of the plant-specific NAC transcription factor family in crop improvement. J. Plant Res. 2021, 1–21.
Singh, S., Singh, V. P., Prasad, S. M., Sharma, S., Ramawat, N., Dubey, N. K., et al. (2019). Interactive effect of silicon (Si) and salicylic acid (SA) in maize seedlings and their mechanisms of cadmium (Cd) toxicity alleviation. J. Plant Growth Regul. 38, 1587–1597.
Sivanesan, I., Son, M. S., Soundararajan, P., and Jeong, B. R. (2014). Effect of Silicon on Growth and Temperature Stress Tolerance of Nephrolepis exaltata’Corditas’. Horticult. Sci. Technol. 32, 142–148.
Soleimannejad, Z., Abdolzadeh, A., and Sadeghipour, H. R. (2019). Beneficial effects of silicon application in alleviating salinity stress in halophytic puccinellia distans plants. Silicon 11, 1001–1010.
Song, A., Li, P., Fan, F., Li, Z., and Liang, Y. (2014). The effect of silicon on photosynthesis and expression of its relevant genes in rice (Oryza sativa L.) under high-zinc stress. PLoS One 9:e113782. doi: 10.1371/journal.pone.0113782
Souri, Z., Khanna, K., Karimi, N., and Ahmad, P. (2021). Silicon and plants: current knowledge and future prospects. J. Plant Growth Regul. 40, 906–925.
Soylemezoglu, G., Demir, K., Inal, A., and Gunes, A. (2009). Effect of silicon on antioxidant and stomatal response of two grapevine (Vitis vinifera L.) rootstocks grown in boron toxic, saline and boron toxic-saline soil. Sci. Horticult. 123, 240–246.
Sreenivasulu, N., Harshavardhan, V. T., Govind, G., Seiler, C., and Kohli, A. (2012). Contrapuntal role of ABA: does it mediate stress tolerance or plant growth retardation under long-term drought stress? Gene 506, 265–273. doi: 10.1016/j.gene.2012.06.076
Sudo, E., Itouga, M., Yoshida-Hatanaka, K., Ono, Y., and Sakakibara, H. J. J. (2008). Gene expression and sensitivity in response to copper stress in rice leaves. J. Exp. Bot. 59, 3465–3474. doi: 10.1093/jxb/ern196
Sun, L., Wang, R., Tang, W., Chen, Y., Zhou, J., Ma, H., et al. (2022). Robust identification of low-Cd rice varieties by boosting the genotypic effect of grain Cd accumulation in combination with marker-assisted selection. J. Hazard. Mater. 424:127703. doi: 10.1016/j.jhazmat.2021.127703
Suzuki, N., Koussevitzky, S., Mittler, R. O. N., and Miller, G. A. D. (2012). ROS and redox signalling in the response of plants to abiotic stress. Plant Cell Environ. 35, 259–270. doi: 10.1111/j.1365-3040.2011.02336.x
Takahashi, R., Ishimaru, Y., Senoura, T., Shimo, H., Ishikawa, S., Arao, T., et al. (2011). The OsNRAMP1 iron transporter is involved in Cd accumulation in rice. J. Exp. Bot. 62, 4843–4850. doi: 10.1093/jxb/err136
Takasaki, H., Maruyama, K., Kidokoro, S., Ito, Y., Fujita, Y., Shinozaki, K., et al. (2010). The abiotic stress-responsive NAC-type transcription factor OsNAC5 regulates stress-inducible genes and stress tolerance in rice. Mol. Genet. Genomics 284, 173–183. doi: 10.1007/s00438-010-0557-0
Tariq, R. M. S., Ahmad, T., Ayub, M. A., Dogar, W. A., Ahmad, Z., and Aslam, M. M. (2022). Management of Abiotic Stress Conditions by Salicylic Acid. Plant Abiotic Stress Physiol. 2:157.
Thind, S., Hussain, I., Ali, S., Hussain, S., Rasheed, R., Ali, B., et al. (2020). Physiological and biochemical bases of foliar silicon-induced alleviation of cadmium toxicity in wheat. J. Soil Sci. Plant Nutrit. 20, 2714–2730.
Tripathi, D. K., Singh, V. P., Ahmad, P., Chauhan, D. K., and Prasad, S. M. (2016). Silicon in plants: advances and future prospects. Florida, FL: CRC Press.
Tripathi, D. K., Singh, V. P., Prasad, S. M., Chauhan, D. K., Dubey, N. K., Rai, A. K. J. E., et al. (2015). Silicon-mediated alleviation of Cr (VI) toxicity in wheat seedlings as evidenced by chlorophyll florescence, laser induced breakdown spectroscopy and anatomical changes. Ecotoxicol. Environ. Safety 113, 133–144. doi: 10.1016/j.ecoenv.2014.09.029
Tripathi, D. K., Vishwakarma, K., Singh, V. P., Prakash, V., Sharma, S., Muneer, S., et al. (2021). Silicon crosstalk with reactive oxygen species, phytohormones and other signaling molecules. J. Hazard. Mater. 408:124820. doi: 10.1016/j.jhazmat.2020.124820
Ul Hassan, M., Rasool, T., Iqbal, C., Arshad, A., Abrar, M., Abrar, M. M., et al. (2021). Linking Plants Functioning to Adaptive Responses Under Heat Stress Conditions: A Mechanistic Review. J. Plant Growth Regul. 2021, 1–18.
Vaculík, M., Lukačová, Z., Bokor, B., Martinka, M., Tripathi, D. K., and Lux, A. (2020). Alleviation mechanisms of metal (loid) stress in plants by silicon: a review. J. Exp. Bot. 71, 6744–6757. doi: 10.1093/jxb/eraa288
Vanlerberghe, G. C., Martyn, G. D., and Dahal, K. (2016). Alternative oxidase: a respiratory electron transport chain pathway essential for maintaining photosynthetic performance during drought stress. Physiol. Plant. 157, 322–337. doi: 10.1111/ppl.12451
Varshney, R. K., Bohra, A., Roorkiwal, M., Barmukh, R., Cowling, W. A., Chitikineni, A., et al. (2021a). Fast-forward breeding for a food-secure world. Trends Genet. 37, 1124–1136. doi: 10.1016/j.tig.2021.08.002
Varshney, R. K., Bohra, A., Yu, J., Graner, A., Zhang, Q., and Sorrells, M. E. (2021b). Designing future crops: genomics-assisted breeding comes of age. Trends Plant Sci. 26, 631–649. doi: 10.1016/j.tplants.2021.03.010
Varshney, R. K., Singh, V. K., Kumar, A., Powell, W., and Sorrells, M. E. (2018). Can genomics deliver climate-change ready crops? Curr. Opin. Plant Biol. 45, 205–211. doi: 10.1016/j.pbi.2018.03.007
Varshney, R. K., Sinha, P., Singh, V. K., Kumar, A., Zhang, Q., and Bennetzen, J. L. (2020). 5Gs for crop genetic improvement. Curr. Opin. Plant Biol. 56, 190–196. doi: 10.1016/j.pbi.2019.12.004
Verma, K. K., Liu, X.-H., Wu, K.-C., Singh, R. K., Song, Q.-Q., Malviya, M. K., et al. (2019). The impact of silicon on photosynthetic and biochemical responses of sugarcane under different soil moisture levels. Silicon 2019, 1–13.
Verma, K. K., Song, X.-P., Tian, D.-D., Singh, M., Verma, C. L., Rajput, V. D., et al. (2021). Investigation of defensive role of silicon during drought stress induced by irrigation capacity in sugarcane: Physiological and biochemical characteristics. ACS Omega 6, 19811–19821. doi: 10.1021/acsomega.1c02519
Verma, V., Ravindran, P., and Kumar, P. P. (2016). Plant hormone-mediated regulation of stress responses. BMC Plant Biol. 16, 1–10. doi: 10.1186/s12870-016-0771-y
Wang, H., Li, C., and Liang, Y. (2001). Agricultural utilization of silicon in China. Stud. Plant Sci. 2001, 343–358.
Wang, J., Song, L., Gong, X., Xu, J., and Li, M. (2020). Functions of jasmonic acid in plant regulation and response to abiotic stress. Int. J. Mol. Sci. 21:1446. doi: 10.3390/ijms21041446
Wang, L.-J., Fan, L., Loescher, W., Duan, W., Liu, G.-J., Cheng, J.-S., et al. (2010). Salicylic acid alleviates decreases in photosynthesis under heat stress and accelerates recovery in grapevine leaves. BMC Plant Biol. 10, 1–10. doi: 10.1186/1471-2229-10-34
Wang, M., Wang, R., Mur, L. A. J., Ruan, J., Shen, Q., and Guo, S. (2021). Functions of silicon in plant drought stress responses. Horticult. Res. 8, 1–13. doi: 10.1038/s41438-021-00681-1
Wang, S., Liu, P., Chen, D., Yin, L., Li, H., and Deng, X. (2015). Silicon enhanced salt tolerance by improving the root water uptake and decreasing the ion toxicity in cucumber. Front. Plant Sci. 6:759. doi: 10.3389/fpls.2015.00759
Wang, X., and Huang, B. (2017). Lipid-and calcium-signaling regulation of HsfA2c-mediated heat tolerance in tall fescue. Environ. Exp. Bot. 136, 59–67.
Wani, S. H., Kumar, V., Shriram, V., and Sah, S. K. (2016). Phytohormones and their metabolic engineering for abiotic stress tolerance in crop plants. Crop J. 4, 162–176.
Wu, J., Guo, J., Hu, Y., and Gong, H. (2015). Distinct physiological responses of tomato and cucumber plants in silicon-mediated alleviation of cadmium stress. Front. Plant Sci. 6:453. doi: 10.3389/fpls.2015.00453
Wu, J., Mock, H.-P., Giehl, R. F. H., Pitann, B., and Mühling, K. H. (2019). Silicon decreases cadmium concentrations by modulating root endodermal suberin development in wheat plants. J. Hazard. Mater. 364, 581–590. doi: 10.1016/j.jhazmat.2018.10.052
Yang, Y., and Guo, Y. (2018). Elucidating the molecular mechanisms mediating plant salt-stress responses. N. Phytol. 217, 523–539. doi: 10.1111/nph.14920
Yao, X., Chu, J., Cai, K., Liu, L., Shi, J., and Geng, W. (2011). Silicon improves the tolerance of wheat seedlings to ultraviolet-B stress. Biol. Trace Element Res. 143, 507–517. doi: 10.1007/s12011-010-8859-y
Yin, J., Jia, J., Lian, Z., Hu, Y., Guo, J., Huo, H., et al. (2019). Silicon enhances the salt tolerance of cucumber through increasing polyamine accumulation and decreasing oxidative damage. Ecotoxicol. Environ. Safety 169, 8–17. doi: 10.1016/j.ecoenv.2018.10.105
Yin, L., Wang, S., Liu, P., Wang, W., Cao, D., Deng, X., et al. (2014). Silicon-mediated changes in polyamine and 1-aminocyclopropane-1-carboxylic acid are involved in silicon-induced drought resistance in Sorghum bicolor L. Plant Physiol. Biochem. 80, 268–277. doi: 10.1016/j.plaphy.2014.04.014
Yin, L., Wang, S., Tanaka, K., Fujihara, S., Itai, A., Den, X., et al. (2016). Silicon-mediated changes in polyamines participate in silicon-induced salt tolerance in S orghum bicolor L. Plant Cell Environ. 39, 245–258. doi: 10.1111/pce.12521
Younis, A., Khattab, H., and Emam, M. (2020). Impacts of silicon and silicon nanoparticles on leaf ultrastructure and TaPIP1 and TaNIP2 gene expressions in heat stressed wheat seedlings. Biol. Plant. 64, 343–352.
Zahra, N., Hafeez, M. B., Shaukat, K., Wahid, A., Hussain, S., Naseer, R., et al. (2021). Hypoxia and Anoxia Stress: Plant responses and tolerance mechanisms. J. Agronomy Crop Sci. 207, 249–284.
Zandalinas, S. I, Mittler, R., Balfagón, D., Arbona, V., and Gómez-Cadenas, A. (2018). Plant adaptations to the combination of drought and high temperatures. Physiol. Plant. 162, 2–12. doi: 10.1111/ppl.12540
Zargar, S. M., and Agnihotri, A. (2013). Impact of silicon on various agro-morphological and physiological parameters in maize and revealing its role in enhancing water stress tolerance. Emirates J. Food Agricult. 2013, 138–141.
Zargar, S. M., Gupta, N., Mir, R. A., and Rai, V. (2016). Shift from gel based to gel free proteomics to unlock unknown regulatory network in plants: a comprehensive review. J. Adv. Res. Biotechnol. 19:15226.
Zargar, S. M., Mahajan, R., Bhat, J. A., Nazir, M., and Deshmukh, R. (2019). Role of silicon in plant stress tolerance: opportunities to achieve a sustainable cropping system. 3 Biotech 9:73. doi: 10.1007/s13205-019-1613-z
Zhang, X., Zhang, W., Lang, D., Cui, J., and Li, Y. (2018). Silicon improves salt tolerance of Glycyrrhiza uralensis Fisch. by ameliorating osmotic and oxidative stresses and improving phytohormonal balance. Environ. Sci. Poll. Res. 25, 25916–25932. doi: 10.1007/s11356-018-2595-9
Zhao, D., Hao, Z., Tao, J., and Han, C. (2013). Silicon application enhances the mechanical strength of inflorescence stem in herbaceous peony (Paeonia lactiflora Pall.). Sci. Horticult. 151, 165–172.
Zhou, R., Yu, X., Ottosen, C.-O., Rosenqvist, E., Zhao, L., Wang, Y., et al. (2017). Drought stress had a predominant effect over heat stress on three tomato cultivars subjected to combined stress. BMC Plant Biol. 17, 1–13. doi: 10.1186/s12870-017-0974-x
Zhu, Y. X., Gong, H. J., and Yin, J. L. (2019a). Role of silicon in mediating salt tolerance in plants: a review. Plants 8:147. doi: 10.3390/plants8060147
Zhu, Y., and Gong, H. (2014). Beneficial effects of silicon on salt and drought tolerance in plants. Agron. Sustain. Dev. 34, 455–472.
Zhu, Y., Yin, J., Liang, Y., Liu, J., Jia, J., Huo, H., et al. (2019b). Transcriptomic dynamics provide an insight into the mechanism for silicon-mediated alleviation of salt stress in cucumber plants. Ecotoxicol. Environ. Safety 174, 245–254. doi: 10.1016/j.ecoenv.2019.02.075
Zhu, Y.-X., Xu, X.-B., Hu, Y.-H., Han, W.-H., Yin, J.-L., Li, H.-L., et al. (2015). Silicon improves salt tolerance by increasing root water uptake in Cucumis sativus L. Plant Cell Rep. 34, 1629–1646. doi: 10.1007/s00299-015-1814-9
Zia, A., Hegazy, H. S., Hassan, N. S., Naguib, D. M., and Abdel-Haliem, M. E. (2021). Biochemical responses of wheat to silicon application under salinity. J. Plant Nutrit. Soil Sci. 184, 255–262. doi: 10.1016/j.plaphy.2021.02.023
Keywords: silicon, phytohormones, abiotic stress, sustainable agriculture, climate change, non-essential elements
Citation: Mir RA, Bhat BA, Yousuf H, Islam ST, Raza A, Rizvi MA, Charagh S, Albaqami M, Sofi PA and Zargar SM (2022) Multidimensional Role of Silicon to Activate Resilient Plant Growth and to Mitigate Abiotic Stress. Front. Plant Sci. 13:819658. doi: 10.3389/fpls.2022.819658
Received: 22 November 2021; Accepted: 31 January 2022;
Published: 23 March 2022.
Edited by:
Mohammad Anwar Hossain, Bangladesh Agricultural University, BangladeshReviewed by:
Norollah Kheyri, Islamic Azad University of Gorgan, IranAdnan Mustafa, Academy of Sciences of the Czech Republic (ASCR), Czechia
Athar Mahmood, University of Agriculture Faisalabad, Pakistan
Copyright © 2022 Mir, Bhat, Yousuf, Islam, Raza, Rizvi, Charagh, Albaqami, Sofi and Zargar. This is an open-access article distributed under the terms of the Creative Commons Attribution License (CC BY). The use, distribution or reproduction in other forums is permitted, provided the original author(s) and the copyright owner(s) are credited and that the original publication in this journal is cited, in accordance with accepted academic practice. No use, distribution or reproduction is permitted which does not comply with these terms.
*Correspondence: Rakeeb Ahmad Mir, cmFrZWViYWhtYWRAYmdzYnUuYWMuaW4=; Ali Raza, YWxpcmF6YW11Z2hhbDE0M0BnbWFpbC5jb20=; Sajad Majeed Zargar, c216YXJnYXJAc2t1YXN0a2FzaG1pci5hYy5pbg==