- 1School of Science and the Environment, Memorial University of Newfoundland, Corner Brook, NL, Canada
- 2Department of Agronomy, Shahrekord University, Shahrekord, Iran
- 3Environmental Science Program, Memorial University of Newfoundland, St. John's, NL, Canada
- 4Department of Agriculture Extension, Government of Punjab, Khanewal, Pakistan
- 5INRAE, UMR 1391 ISPA, Bordeaux Science Agro, Villenave d'Ornon, France
- 6Department of Plant Science, McGill University, Ste-Anne-de-Bellevue, QC, Canada
Being a macronutrient, phosphorus (P) is the backbone to complete the growth cycle of plants. However, because of low mobility and high fixation, P becomes the least available nutrient in podzolic soils; hence, enhancing phosphorus use efficiency (PUE) can play an important role in different cropping systems/crop production practices to meet ever-increasing demands in food, fiber, and fuel. Additionally, the rapidly decreasing mineral phosphate rocks/stocks forced to explore alternative resources and methods to enhance PUE either through improved seed P reserves and their remobilization, P acquisition efficiency (PAE), or plant's internal P utilization efficiency (IPUE) or both for sustainable P management strategies. The objective of this review article is to explore and document important domains to enhance PUE in crop plants grown on Podzol in a boreal agroecosystem. We have discussed P availabilities in podzolic soils, root architecture and morphology, root exudates, phosphate transporters and their role in P uptake, different contributors to enhance PAE and IPUE, and strategies to improve plant PUE in crops grown on podzolic soils deficient in P and acidic in nature.
Introduction
Phosphorus (P) is a critical and important mineral required by growing plants to sustain their growth and productivity (Malhotra et al., 2018). It is a fundamental player in various biochemical processes occurring in growing plants (White et al., 2008; Malhotra et al., 2018) and is, therefore, considered as the backbone of seed germination, seedling establishment, and plant growth, development, and reproduction. Thus, it is necessary to complete the life cycle of plants. Heterotrophic seed P is the primary source to support seedling establishment during the onset of germination and the first few weeks of early growth (Nadeem et al., 2011). For onward plant growth and development, P is taken by root systems from soils. However, because of low P mobility and availability in soils, substantial amounts of autotrophic P (soil P) must be supplied from organic or inorganic fertilizer sources (Grant and Flaten, 2019). A major source of inorganic P is fertilizers; therefore, farmlands receive significant quantities of P fertilizers each year because of low soil P status worldwide (Lu and Tian, 2017; Ros et al., 2020). However, the applied P becomes unavailable because of higher affinities toward cations and soil organic matter (SOM), rendering it the least mobile and phytoavailable nutrient (Hinsinger, 2001), particularly, in podzolic soils that are rich in aluminum (Al) and iron (Fe) minerals that fix available P pools (Kedir et al., 2021).
Generally, podzols have a coarse sandy texture, shallow depth, acidic pH, and low fertility, which restricts crop growth and productivity (DeBruyn et al., 2011; Ali et al., 2019b). Higher applications of P fertilizers are, therefore, critical to enhancing crop production, but there are alarming concerns on long-term environmentally sustainable use of nonrenewable P resource (Ros et al., 2020). Despite high P applications, PUE is only 15–20% in agricultural croplands, indicating that most of the applied P is not accessible for plant use (Malhotra et al., 2018). It is, therefore, important to review knowledge gaps to enhance PUE on podzolic soils in managed boreal agroecosystems. Therefore, improving soil P availabilities, reducing P fixation, and enhancing P uptake and internal plant P utilization would improve our understating to enhance overall PUE on podzolic soils. Hence, exploring different plant adaptive strategies to enhance PUE is crucial to sustaining agricultural crop productivity and nonrenewable P reserves management to meet growing food and feed requirements (Ramaekers et al., 2010). This review covers three objectives: first, we give an overview of P availabilities in podzolic soils and different factors governing the dynamics of P. Second, we discuss plants' adaptive approaches in P acquisition in podzolic soils. Furthermore, our focus is to explore the contributions of P acquisition and P utilization in enhancing PUE in growing plants. Finally, we suggest potential strategies and interventions to improve crop P uptake and PUE on podzols in boreal agroecosystems for agricultural crop production and maintenance of high farm outputs.
Phosphorus in Podzolic Soils
Phosphorus Stocks and Field Applications
Globally, phosphate rock is the main source of phosphatic fertilizer (Cordell et al., 2009). Approximately 90% of all mined phosphate rock is used for agricultural crop production (Nesme et al., 2018). The current global supply of phosphatic fertilizer is 50 MT and is projected to increase to 90 MT by 2050 (FAO, 2019; Nedelciu et al., 2020). Expansion of fertilizer usage in agriculture will boost food production; however, high consumption of nonrenewable P resources will put more pressure on already depleting global P reserves (Ros et al., 2020). Although precision agriculture practices promote the optimum utilization of P resources, only 30% of applied P is used by crops to complete their growth cycle, and 70% of applied P is fixed or released to the environment. Such P losses have significant detrimental effects on ecosystem function highlighted in the last few decades such as reduced water quality and ecological disturbances (Weihrauch and Opp, 2018).
Phosphorus (P) is a complex nutrient existing in organic or inorganic forms in soils (Requejo and Eichler-Löbermann, 2014). Based on soil types and management practices, P is the least phytoavailable nutrient in soils because of its higher fixation capacities by reactive minerals like aluminum (Al3+), iron (Fe2+), magnesium (Mg2+), calcium (Ca2+), and organic compounds, which slow its diffusion rate in plant root rhizospheres (Vance et al., 2003). Phytoavailable P species in a soil solution either exist in the form of primary orthophosphate () or secondary orthophosphate () anions depending on soil pH. Primary orthophosphate is the dominant form of podzols common in boreal agroecosystems. The phytoavailability of organic P forms in soil is less studied and depends on microbial activities in the rhizosphere and on environmental factors. P concentrations in a soil solution are typically lower than 10 μM because of P's high fixation and low solubility in acidic and calcareous soils (Bieleski, 1973), leaving significant global croplands (70%) P-deficient (von Uexküll and Mutert, 1995). The podzol rich in Al3+ and Fe2+ minerals has high P retention capacity and, consequently, lower P availability (Peltovuori, 2007). Mineral P fertilizers are the principal input to enhance P availability in modern agricultural production systems; however, the applied P is rapidly immobilized by SOM and minerals in acidic soils (Hinsinger, 2001). Hence, the supplemented P through fertilizers or inherent soil P becomes unavailable for plant utilization unless hydrolyzed to release P in plant rhizosphere with various root exudates or active soil microbial communities (Alori et al., 2017; Ali et al., 2019b). Therefore, efficient utilization of P resources is a recent pressing question regarding sustainable crop production, better economic return, human wellbeing, and environmental sustainability. Therefore, it is crucial to explore advanced strategies and agronomic management to enhance plant P uptake and internal P translocation and remobilization to develop P-efficient genotypes in boreal agroecosystems to effectively utilize P resources and improve crop production.
Based on the dose-response, high fertilizer input has been thought to obtain a high crop yield. However, the growing concern on depletion of P reserves and environmental pollution created opportunities to explore the efficient utilization of P in the agriculture industry (Cordell and White, 2013). A meta-analysis study documented that overuse of P would not improve crop yield (Ros et al., 2020); instead, it causes aquatic pollution. For example, a field experiment on podzolic soil revealed that 22 kg P ha−1 resulted in a higher carrot yield than 110 kg P ha−1 (Sanderson and Sanderson, 2006). Thus, it is essential to understand P availability in podzolic soils, and utilization efficiency by plants might help reduce environmental P buildup and losses for economic and environmental benefits.
Factors Affecting P Availabilities in Podzolic Soils
Generally, the availability of P in mineral soils like Podzol depends on its buffering capacity, mineral and organic matter content, management history, and environmental factors (Quintero et al., 2003; Pierzynski et al., 2005; Kang et al., 2009). Unique characteristics of podzolic soils, such as increased acidity, higher exchangeable Al3+ and Fe2+ content, lower cation exchange, and water retention capacity result in higher P retention capacity and low soil P concentration (<0.01 mg P L−1), typical in boreal agroecosystems (Pierzynski et al., 2005; Paul et al., 2011). Under natural conditions, the podzolization process facilitates leaching of cations such as Al3+, Fe2+, and Ca2+, dissolved organic matter, and chelates from organic layers or eluviated profiles that accumulated in illuviated profiles (Paul et al., 2011; Grand and Lavkulich, 2013; Krettek and Rennert, 2021). However, most agricultural podzolic soils have lost the organic and eluviated profile or a small proportion is mixed with illuviated B horizon rich in Al and Fe minerals during initial or successive soil preparation (Paul et al., 2011). Mainly in converted podzol, different forms of Al species (exchangeable, charged organometallic and poorly crystalline surfaces, aluminosilicates, and oxyhydroxides) and charged soil organic matters may be responsible for fixing or retaining plant-available P in soil solutions (Grand and Lavkulich, 2013). Thus, based on soil pH, mineral soils act as P sink until buffering capacity is satisfied. For example, long-term managed orthic humo-ferric podzol developed on a glacial till, with 10% organic matter (loss-on-ignition), Melich-3 Al3+ to Fe2+ ratio of 8:3, and P of 57 mg L−1 had higher P retention capacity (Kedir et al., 2021). Also, there might be counter effects of long-term liming application to suppress acidity and Al3+ toxicity, which could lead to calcium phosphate precipitation in long-term managed soils with near neutral pH, supported with high (2,155 mg L−1) Ca2+ content compared to 41 mg L−1 in recently converted podzolic soils in a boreal agroecosystem (Kedir et al., 2021). Grand and Lavkulich (2013) reported that in most acidic soils, the highest proportion of P is firmly fixed by Al3+-based amorphous minerals or organic Al3+ materials. Hence, the intensity of Al3+ in acidic soils might reflect P dynamics based on pH that can be used as an indicator to improve P availability for plant uptake.
Additionally, soil management methods such as tillage, liming, manure applications, and the shallow nature of soils could affect P availability in podzolic soils (Pierzynski et al., 2005; Shen et al., 2011). Plant root morphology and physiology also play a crucial role in plant growth by recycling P in the rhizosphere (Fageria et al., 2014). The rooting depth of different plant species varies and can be affected by soil compaction, adaptability, nutrient availability, and soil characteristics such as moisture, temperature, pH, and mineral horizon (Eh) for redox potential (Fageria et al., 2014). Furthermore, P uptake by plant roots may be influenced by genotypes, root architecture, length, hair, density and structure, and the rhizobium (Shen et al., 2011; Fageria et al., 2013). Root exudates, mainly organic acids (citric acid, malic acid, and oxalic acids), might solubilize and mobilize fixed P forms (Tawaraya et al., 2013), but this requires further investigation in podzolic soils to improve P acquisition efficiency. Therefore, it is important to understand all factors affecting P availability to enhance PUE in growing plants on podzolic soils in boreal agroecosystems.
Importance of Soil P Tests to Enhance PUE
The first step in enhancing PUE is to assess accurately the P status of soil by proper soil P testing. About a dozen P-tests was developed to quantify plant-available P in the soils depending on the soil types and properties, specifically soil pH (Table 1). It is important to choose and calibrate proper soil P test to assess plant-available P in podzolic soils. Different agri-environmental P tests targeted different P pools (Table 1); water and resin extracted readily available dissolved P and represented instantaneous P supply to roots via mass flow effect (Nafiu, 2006; Wang et al., 2015). Generally, diluted acid or base solutions are used to extract different P pools in soils. For example, acidic solutions of the Bray method (Bray and Kurtz, 1945), Mehlich (Mehlich, 1984), and citric acid (Thompson, 1995) can extract easily soluble and plant-available P forms as an indicator of P fertility status (Jones, 2001). These methods are designed for podzolic soils rich in Al3+ and Fe2+ minerals and slightly neutral soils (Ketterings and Flock, 2005) that are affected by soil management and environmental factors (Messiga et al., 2015). Most P tests only target to measure P pools, while potentially soluble and available organic P is not correctly accounted for in P recommendation (Ziadi et al., 2013). For example, the Mehlich-3 test performed in most North American laboratories only targets to measure P and multiple cations relevant to plant nutrition (Ziadi et al., 2009). On the other hand, specific P tests developed for particular soil types or regions might over- or underestimate P when applied on different soil types (Nafiu, 2006). Kedir et al. (2021) evaluated P extractability in podzolic soils by nine P-tests following the order water ≤ Morgan ≤ ammonium bicarbonate-diethylenetriaminepentaacetic acid (AB-DTPA) < Mehlich-1 ≤ Bray-1 ≤ Mehlich-3 ≤ Olsen < Bray-2 < citric acid. Similarly, Zehetner et al. (2018) reported weaker extraction methods to correlate better while grouping based on pH. Thus, proper selection and calibration of P tests against P uptake or crop yield under local environmental and field management conditions help to improve plant P use efficiency in podzolic soils. Additionally, more than one P test might be beneficial to measure plant-relevant P pools in podzolic soils.
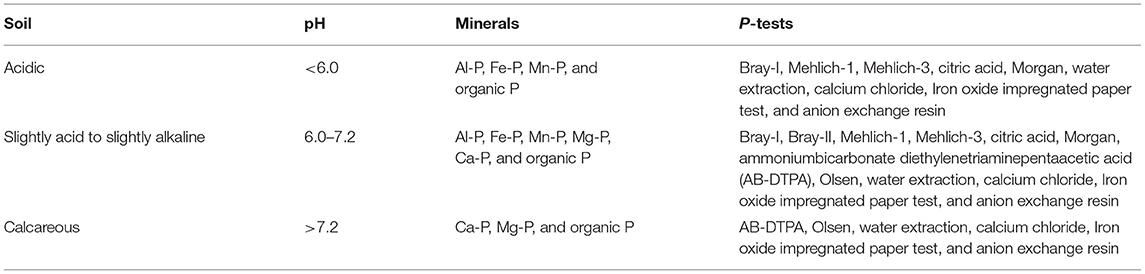
Table 1. Selection of proper soil phosphorus test based on soil properties and targeted phosphorus species (Elrashidi, 2010; Zehetner et al., 2018; Kedir et al., 2021).
Phosphorus Use Efficiency
Mineral nutrition efficiency concepts on crop plants have been defined based on how plants acquire essential mineral nutrients with the help of an underground extensive root system, mineral transport within a plant body, their translocation, storage, and remobilization, and re-translocation within plant organs to produce a desirable harvest (Ciarelli et al., 1998). Nutrient efficiency starts with the onset of ontogeny, which is marked with successful hydrolysis and remobilization of stored seed reserves (Figure 1), an important step for early seedling establishment and crucial for early plant development (White and Veneklaas, 2012). Therefore, the concept of nutrient use efficiency might be a combination of successful remobilization of heterotrophic stored seed nutrient reserves, autotrophic nutrient acquisition by roots or accumulation through shoots, and their internal translocation or re-translocation within actively growing plant organs (Figures 2, 3).
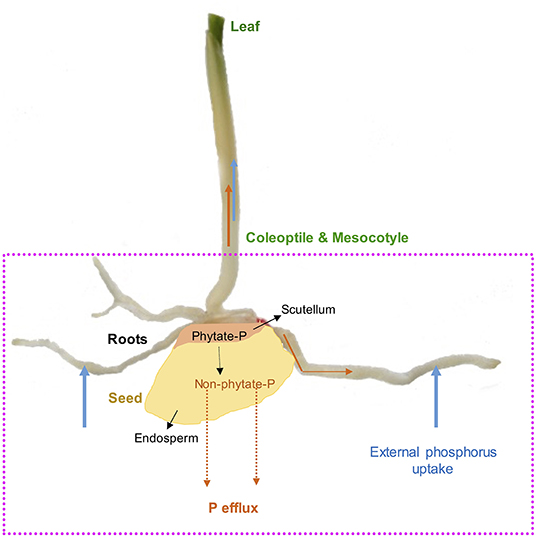
Figure 1. Maize (Zea mays L.) as a model seed showing endosperm and scutellum. Seed phytate-P hydrolysis during germination, translocations to developing roots, coleoptile, mesocotyle, and leaves as well as external P uptake and P efflux during germination and early growth stages.
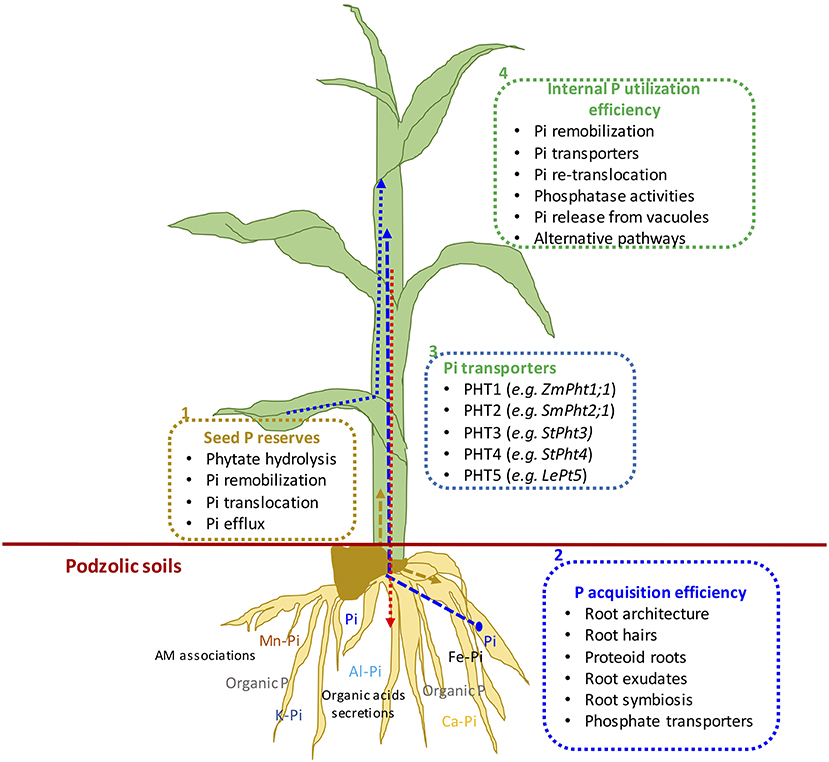
Figure 2. (1) Schematic representation of mechanisms of seed phosphorus remobilization, (2) P acquisition efficiency, (3) P transporters, and (4) internal P utilization efficiency from germination to final plant harvest in maize plant. Dotted arrows show different P fluxes in growing seedlings: Blue represents external P uptake, gold represents remobilized seed P, and pink arrow shows P translocation from leaves to roots.
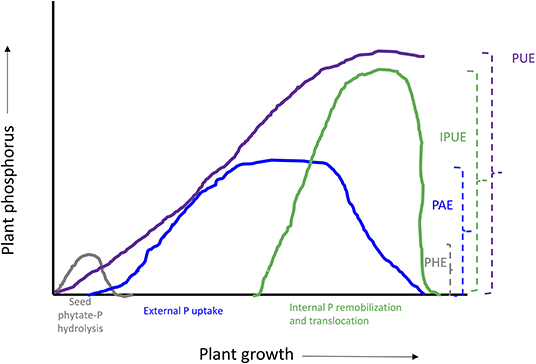
Figure 3. Schematic representation of plant phosphorus use efficiency and different components. PUE, phosphorus use efficiency; IPUE, internal phosphorus utilization efficiency; PAE, phosphorus acquisition efficiency; PHE, seed phytate-P hydrolysis efficiency.
Many studies have been conducted to define different methods and means regarding plant nutrient use efficiencies; the most common is the ability of plants to convert nutrient inputs into biomass or minimize nutrient wastage in an agroecosystem (Batten, 1992; Lynch, 1998). The PUE of plants could be divided into PAE and IPUE; early seed P remobilization and seedling P nutrition are also an integral part of plant harvestable P (White and Veneklaas, 2012), particularly in soils with low inherent P status and seed P effluxes during germination (Nadeem et al., 2012). Therefore, higher PUE of plants could be achieved through improved seed with P reserves for better seedling establishment in P-deficient soils, P acquisition by root systems, and internal P utilization during vegetative and reproductive growth, or their combination (Shenoy and Kalagudi, 2005; Parentoni and Souza Júnior, 2008; Nadeem et al., 2011). The contribution from seed P from hydrolysis of phytate-P, PAE, or IPUE to PUE varies with crop species, phytate-P content in seeds, soil, and environmental factors. Modern agricultural practices include selecting crop genotypes with higher-quality seed with better nutrient stock (biomass) to support the early seedling establishment and adapt to scavenge soil P by improved root architecture and morphology that facilitate PAE (Vance et al., 2003). Some genotypes express higher P uptake capacity (Ciarelli et al., 1998; Corrales et al., 2007; Parentoni and Souza Júnior, 2008); however, low remobilization and re-translocation of P by plants is a challenge to harvest-acquired P (Figure 2). Therefore, it becomes important to explore better strategies that could improve seed P remobilization and translocation to newly growing seedlings while minimizing P effluxes (Nadeem et al., 2011), better PAE through the root system, and higher IPUE during the growing season. Here, we discuss different strategies of three important plant PUE contributors and possible improvements in podzolic soils in boreal climate.
Seed Phytate-P Hydrolysis, Remobilization, and Use Efficiency
Phosphorus is highly mobile in plants and exists as free inorganic orthophosphate or organic phosphate ester; however, in seeds, P is loaded and stored in the form of phytate-P during crop maturity (Raboy, 1997). Simultaneously, P and cellular P are other forms of P in seeds and are generally referred to as available P (Raboy, 1997). As the primary storage form of P in seeds (Figure 1), phytate-P represents 50–90% of total P in mature seeds and accounts for 0.5–5% of seed dry weight (Greiner and Egli, 2003; Nadeem et al., 2011). In maize grains, phytate-P is mainly located in the scutellum compared to the endosperm, which is rich in carbon (C) and nitrogen (N) (Nadeem et al., 2011). It has been reported that mature seeds or grains contain enough phytate-P to provide P to sustain root and shoot growth for several weeks in developing maize seedlings (Nadeem et al., 2011), and this could be a potential point to enhance seed P content for prolonged seed P contribution in developing seedlings on P-deficient podzol soils for improving PUE (Figure 1). Phytate-P hydrolysis is also considered to provide other bound mineral nutrients to developing seedlings during the seedling establishment stage (Lott et al., 1995). We demonstrated earlier that phytate-P hydrolysis in seeds is not a limiting factor for P availability in germinating seeds for seedling P nutrition (Nadeem et al., 2012), and seedlings start to uptake significant external P soon after the emergence of radicle contributing to PUE (Nadeem et al., 2011). Boreal agroecosystems are characterized by a cool, short growing season particularly during early growth and crop maturity. Cool growing conditions during germination and early growth conditions affect seedling establishment because of low crop heat unit accumulation (Kwabiah et al., 2003), affecting biomass allocation to the shoot, which might further enhance P effluxes during early growth stages in podzolic soils. Such P effluxes depend on seed P reserves, variations among growing seedlings (Nadeem et al., 2012), and seedling growth-limiting conditions (Ali et al., 2019a), which could further reduce PUE in early crop growth stages in podzolic soils in boreal agroecosystems. Thus, initial heterotrophic P nutrition supported by seed P reserves is essential for early seedling establishment when external P uptake is restricted because of unavailability in P-deficient soils (White and Veneklaas, 2012), particularly in podzolic soils (Kedir et al., 2021). Therefore, enhancing phytate-P concentrations in seeds could have beneficial effects on early seedling success in podzolic soils; however, such an approach might increase P effluxes (Nadeem et al., 2012) in germinating seeds and growing seedlings because of low C assimilation under cool climatic conditions.
Phosphorus Acquisition Efficiency
Following successful seed germination, growing seedlings depend on autotrophic P in soil acquired by developing root systems (Hinsinger, 2001). Seedlings absorb only small quantities of external P during the first few weeks of early growth (Nadeem et al., 2011); however, this early P nutrition is extremely important to enhance PUE and final crop harvest. Therefore, after exhaustion of heterotrophic seed P reserves, PUE could be explained by considering the role of various root physiological traits and biochemical processes in the rhizosphere zone and P availabilities in podzol soils (Figure 3). Plants have evolved several mechanisms to scavenge unevenly distributed P in the soil profile and uptake against a concentration gradient (Bieleski, 1973; Hinsinger, 2001; Ho et al., 2005). The main mechanisms associated with higher PAE include changes in root morphology, architecture, and functions of mycorrhizae hyphae that help plant roots to explore the rhizosphere zone, particularly in shallow natured podzol soils (Figure 3). The genetic ability of plants to associate with soil rhizosphere microorganisms, such as mycorrhizae, bacteria, and fungi (root symbiosis), and plants' inherent capacity to sense and secrete different organic compounds (root exudates) in the rhizosphere, such as phosphatases and organic acids, to release P from organic or inorganic soil sources (Raghothama, 1999). Here, we discuss the inherent ability of plants to scavenge P by plant root systems and different potential strategies to enhance PAE.
Root Morphology
Roots are the primary source to acquire the nutrients essential for growth and development in both monocot and dicot plants (Gregory, 2006). Efficient three-dimensional configuration of the root system is vital for improved nutrient uptake (Mollier et al., 2008; Li et al., 2016). Root growth and its developmental response are highly plastic and influenced by many factors such as soil P status, water availability, oxygen concentration, soil density, and pH (Péret et al., 2011, 2014). Growing plants respond to P deprivation in the root rhizosphere in several ways including allocating more C stocks to roots rather than shoots, thereby increasing root to shoot ratio, changes in lateral root formation (Mollier and Pellerin, 1999), longer roots, increased root hair density and length (Lynch and Brown, 2001), enhanced exudation of organic acids (Carvalhais et al., 2011), active microbial communities, higher acid phosphatase activities (Ali et al., 2019b), P transporters in roots (Shin et al., 2004; Kanno et al., 2016), higher association with mycorrhizae (Smith et al., 2011), formation of aerenchyma (Pujol and Wissuwa, 2018), as well as the growth angle of basal roots (Miguel et al., 2013). Such characteristics could enable roots to explore the rhizosphere in shallow podzolic soils to acquire the required plant P for optimum growth and development. However, not all crops have the ability to equally explore the rhizosphere P (Lynch and Brown, 2001; Williamson et al., 2001). Therefore, improved root morphological traits can play an important role in enhancing P uptake in shallow podzolic soils rich in Al3+ and Fe2+ minerals in boreal agroecosystems. Here, we briefly discuss root architecture and morphological traits that contribute to higher PAE in podzolic soils in boreal agroecosystems.
Root Architecture
The spatial configuration of the root system in the rhizosphere is often referred to as root architecture. It relates root distribution patterns and root topology in response to soil physiochemical properties including any nutrient deficiency (Lynch, 1995; Lynch and Brown, 2001). Therefore, root architecture determines the extent of root exploration capacities throughout the soil (Péret et al., 2014). Such plant strategies in the root architecture could occur at low C cost resulting in enhanced PAE (Wang et al., 2004a; Yan et al., 2004). Phosphorus is a major nutrient that is influenced by root architecture and development (Williamson et al., 2001). Modifying root architecture is an important strategy to improve PUE under P-deficient podzol soil conditions, allowing for improved plant performance (Li et al., 2016). The shallowness of podzolic soils might affect P uptake since upper soil layers are often rich in P; therefore, plant adaptation in root architecture and density might (van de Wiel et al., 2016) help to enhance PAE in podzolic soils. Additionally, root architecture adaptations including horizontal growth of basal-root, shallower growth angles of axil roots, increased adventitious roots, enhanced lateral root formation, and increased root hair density result in enhanced axial root lengths (Lynch and Brown, 2001; Vance et al., 2003; Lynch, 2007; Richardson et al., 2011; van de Wiel et al., 2016). Campos et al. (2018) demonstrated that these adaptations improve PAE in the topsoil under low-mobility P conditions that could be employed in podzolic soils. Lynch (1995) demonstrated that PAE is positively associated with basal root branching angles and the number of lateral branching roots per unit root area in common bean, especially under low P conditions. The shallow growth angle of axial roots increases PAE by increasing topsoil foraging in most soils (Lynch, 2011) and possibly in podzolic soils with shallow nature. Scavenging capacity in the upper soil layer increases with changes in growth angle and lateral root density (Ao et al., 2010).
Zhao et al. (2004) also reported a critical role of root shallowness in scavenging P in growing soybean for higher PAE, and, therefore, considered that podzolic soils might provide higher adventitious roots and lateral root growth to enhance PAE. However, in the study of Mori et al. (2016), shallow root angle was not beneficial, a genotype of rice with a steep root angle (37°) had a similar ratio of total root biomass (78%) compared with a shallow-rooted genotype (17°) (82%) in that soil top layer. This is probably due to the shallowness being related with root mass in 5 cm topsoil, but not with root biomass above 20–25 cm. Another important aspect is localized P supplies that can further enhance grass root proliferation to maximize PAE. Selecting crop genotypes with better root architectural traits would not only increase exploration of greater rhizosphere area but could also provide opportunities to uptake unevenly distributed P in podzol soils. Therefore, minor changes in root architecture could result in greater differences among different crop genotypes for PAE (Wissuwa, 2003), which could provide a better solution to scavenge P in podzolic soils. Mori et al. (2016) confirmed that a large root system is highly correlated with reduced P availability in soils. They also reported that root size and root efficiency represent two distinct tolerance mechanisms that increase plant performance and P uptake under P deficiency conditions.
Root Hair
Root hair formation from trichoblast, a tubular-shaped root cell (Gilroy and Jones, 2000; Singh et al., 2008), is a root architectural characteristic and plays a critical role in acquiring essential plant nutrients (Gilroy and Jones, 2000; Wang et al., 2016). Root hair is a low-cost way to explore a greater rhizosphere area and is a major contributors to nutrient uptake in plants (Wang et al., 2016). Parker et al. (2000) reported that about 77% of root surface areas are composed of root hair in Arabidopsis. Alteration in root tip growth and root length extension provides more surface areas to uptake nutrients from soils. Root hair formation and growth are associated with less mobile nutrients such as P, and an earlier study demonstrated that increased lateral root development and root hair intensity are related to higher PAE (Gilroy and Jones, 2000; Jungk, 2001; Wang et al., 2016). Ma et al. (2001) reported that P-deprived growth conditions improved trichoblast number, which likely increased root hair formation. Richardson et al. (2011) suggested allocation of carbon for root hair formation as a relatively minor plant metabolic cost to enhance P efficiency. Researchers demonstrated that the number of root hair and length are correlated with P concentration. For instance, the density of root hair in P-deficient soils is five-fold higher than in P-enriched soils (Bates and Lynch, 2000; Ma et al., 2001). Higher root hair density and total root length in soybean in basal roots than in taproots indicated highly positive correlations with root hair density and P concentration in soybean (Wang et al., 2004a). Similarly, common beans also expressed higher root hair density and longer root hair length in P-efficient genotypes (Yan et al., 2004). Moreover, Miguel et al. (2015) demonstrated phene synergism between root hair length and basal root growth angle for P uptake.
Sugars, auxin, and ethylene have been well-documented for their roles in modulating root hair formation during P deficiency (Niu et al., 2013; Song and Liu, 2015). Response to P deficiency in shoots causes signals in roots to enhance root hair elongation and abundance (Jungk, 2001). Parry (2018) reported that auxin homeostasis is crucial for root hair elongation under low external P availability conditions. Auxin in the lateral root cap and epidermal cells transported by AUX1 is satisfied for low-P induced root hair elongation. Giri et al. (2018) demonstrated that OsAUX1, the ortholog of Arabidopsis AUX1 sequence, functions in rice to mobilize auxin from root apex to differentiation zone to promote root hair elongation and improve PAE under low-P conditions. Bhosale et al. (2018) showed that the root hair elongation process was disrupted in auxin synthesis (TAA1) and transport (AUX1) mutants under P starvation conditions. In addition, auxin response transcriptional factors such as ARF19, RSL12, and RSL14 are also induced in response to low P (Bhosale et al., 2018). Similarly, about 40 genes have been identified in Arabidopsis that affect the initiation and development of root hair based on P availability in a growing medium (Grierson et al., 2001). Plant genotypes exhibiting higher changes in auxin for better root hair formation to explore more root rhizosphere can enhance PUE in acidic soils. Therefore, selecting crop genotypes with a better ability to enhance root hair formation could provide a better way to enhance P uptake from podzolic soils in boreal agroecosystems.
Proteoid Roots
Proteoid or cluster root formation is considered a major adaptation in plant species to enhance P uptake (Shane et al., 2003; Abdolzadeh et al., 2010; Cheng et al., 2011; Niu et al., 2013), particularly when P is present in sorbed or insoluble forms in soils (Lambers et al., 2006). Marschner et al. (1989) explained for the first time that extractability of nutrients is increased by several folds in the rhizosphere of proteoid roots. On the contrary, plants in P-rich soils do not invest energy for cluster root formation (Shane et al., 2003, 2006; Abdolzadeh et al., 2010). P deficiency in soils causes signal transduction from shoots to roots for cluster root formation and a variety of genes (Suc synthase, triose-P isomerase, NAD-dependent glyceraldehyde 3-P dehydrogenase, enolase, and phosphoenolpyruvate carboxylase) are involved in such process. This process causes changes in primary and secondary metabolism, and P scavenging and remobilization in Lupinus albus L. (Uhde-Stone et al., 2003). Cluster root formation is important in Leguminosae and Proteaceae families, especially in P-deficient soils, and common in plant species that lack mycorrhizal association. The morphological and physiological characteristics of cluster roots enhance P uptake by releasing protons and carboxylates in the rhizosphere (Cheng et al., 2011; Lambers et al., 2012a). As such, cluster roots geared to enhance root surface area, exude significant amounts of carboxylates as exudative burst, generate localized concentrations, forage insoluble forms of soil P, and enhance P uptake (Lambers et al., 2006). Exudation of organic acids, such as malate and citrate, by cluster roots (Marschner et al., 1986; Massonneau et al., 2001) in podzolic soils could help in P release from bounded to plant-available form. Therefore, considering crop species with higher abilities and investment in cluster root formation could result in higher PAE and PUE in P-deficient soils compared to species lacking cluster root formation abilities. Such plant root characteristics would be very useful in agricultural production systems on acidic soil to enhance crop growth and biomass production with improved PUE.
Root Exudates
Plant roots exude a variety of organic compounds in the rhizosphere under normal growth conditions, which leads to a wide array of processes such as plant-microbe signaling, nutrient availability, and plant nutrient uptake (Harrison, 1997; Ali et al., 2019b). Root exudates include organic acids, phenolics, amino acids, sugars, proteins, and polysaccharides (Ryan et al., 2001; Marschner, 2011; Nadeem et al., 2016); however, these exudates vary in terms of quantity and quality under abiotic or biotic stress (Ryan et al., 2001; Neumann and Martinoia, 2002; Marschner, 2011). For instance, in P-deficient environments, growing plant roots show enhanced synthesis and exudation of several organic acids such as citric acid, malate, malic acid, cis-aconitic acid, and oxalic acid (Dinkelaker et al., 1989; Delhaize et al., 1993; Delhaize and Ryan, 1995; Larsen et al., 1998; Neumann et al., 2000), which induce changes in rhizosphere chemistry, soil microbial populations, and plant growth (Ali et al., 2019b). Ryan et al. (2001) explained that root exudates enhance phosphatase enzymes and P acquisition in growing plants. The release of organic acids in the rhizosphere displaces or mobilizes P from complex metal cations of soil colloids that compete for adsorbed P, thereby releasing P into the soil solution for plant uptake (Hinsinger, 2001; Ryan et al., 2001). Similarly, organic acids in root exudates destabilize organic matters and promote the cycling of organic P and P into soil solutions (Menezes-Blackburn et al., 2016). Vance et al. (2003) reported 20-to 40-fold higher citrate and malate exudates by cluster root system in P-deficient soils. Malate, citrate, and oxalate exudates are enhanced by lupine roots under P-deficient growth conditions. Citrate is considered most effective in releasing P through chelation of Al3+ in acidic soils (Menezes-Blackburn et al., 2016). Ström et al. (2002) reported enhanced P uptake by exogenous application of oxalate and citrate into maize plant rhizosphere, and such exogenous application upregulated P diffusion coefficient by three times in a soil solution. Enhanced citrate exudation by transgenic tobacco plants increased P acquisition, leading to enhanced PAE (López-Bucio et al., 2000). Higher acid phosphatase activities and P uptake were also observed in manure-applied corn silage under field conditions on podzolic soils in a boreal agroecosystem (Ali et al., 2019b). Such enhanced uptake due to root exudates contributes to increased PUE in growing plants. Crop genotypes exhibiting higher abilities of root exudation to cope with lower soil P availability could provide a sustainable solution to forage P and enhance PAE on podzolic soils in boreal agroecosystems.
Root Symbiosis
Arbuscular mycorrhizal fungi (AMF) and plant root symbiotic association is ubiquitous and plays an important role to enhance PAE in growing plants on P-deficient soils (Haran et al., 2000; George and Richardson, 2008; Adesemoye and Kloepper, 2009; Smith and Read, 2010). AMF colonize plant roots to obtain energy and, in exchange, release phosphate from arbuscular hyphae for plant uptake. Under P deficient conditions in the rhizosphere, AMF colonization promotes root growth by increasing the length and number of lateral roots and root biomass (Qiang-Sheng et al., 2011; Wu et al., 2011a; Chun-Yan et al., 2014). Fungi enter the inner cortex of roots and form highly branched hyphae enveloped by the root cell plasma membrane, thus forming an efficient signaling and nutrient exchange interface. Drew et al. (2003) reported that fungi also develop an extensive network of hyphae outside roots, which can easily penetrate into soil micropores. Li et al. (1991) reported that these effects are mainly due to the ability of AMF hyphae to scavenge inorganic P well beyond the limits of the rhizosphere depletion zone depending on plant genotype, P nutrition, and growth stage. Ryan and Graham (2002), however, documented that such roles of AMF are not always beneficial to growing plants because of greater carbon cost where they act as a parasite. Researchers demonstrated that 70–90% of plant species including ferns, bryophytes, flowering plants, and most agricultural crop plants can develop interdependent connections with AMF (Zhu et al., 2010; Ahanger et al., 2014; Begum et al., 2019; Diagne et al., 2020). Hidden mechanisms are not fully explored; however, AMF contributions to growing plants in terms of plant P nutrition and plant health and fitness are well-documented (Smith and Read, 2010; Plassard et al., 2019). Battini et al. (2016) demonstrated that a culture-dependent approach allows for the isolation in a pure culture of 374 bacterial strains that are associated with plant growth-promoting activities such as phytate-P mineralization and solubilization of P. These positive effects of AMF association with plants are essential for upcoming challenges in terms of enhanced PAE and PUE in podzolic soils for sustainable crop production.
Beneficial microorganisms also play a vital role in the biogeochemical cycling of organic and inorganic P in the rhizosphere (Harvey et al., 2009; Khan et al., 2010; Richardson and Simpson, 2011). Researchers demonstrated that plant root inoculation with plant growth-promoting bacteria (PGPB) enhanced soil organic P, e.g., inositol phosphates (Richardson et al., 2011; Pereira et al., 2020). These bacteria with extracellular phytase activity and their interactions in the rhizosphere increase plant access to P from inositol phosphates (Unno et al., 2005; Richardson and Simpson, 2011) and improve plant PUE. Microbial inoculants in the rhizosphere have been known as systems of integrated nutrient management, with a specific interest in their ability to enhance the availability of P for crops (Richardson et al., 2011). Bacteria (most commonly Azospirillum, Bacillus, Pseudomonas, and Actinomycetes) have been able to release orthophosphate from organic and inorganic substrates by liberating protons, organic anions (e.g., citrate, oxalate, and gluconate), phosphatases, and cation-chelating compounds (El-Tarabily et al., 2008; Taurian et al., 2010). However, performing microbial inoculation on P-solubilizing fungi, especially species of Penicillium (Penicillium radicum and Penicillium bilaiae), has been the main effort to increase P availability (Wakelin et al., 2007; Richardson and Simpson, 2011). Generally, the major mechanism of PGPB may be related to the production of phytohormones such as auxins, salicylic acid, cytokinins, and gibberellins, and to P solubilization that enhances density and length of roots and increases a plant's ability to capture available nutrients (Pereira et al., 2020).
Phosphate Transporters
The movement of P in soils is mainly dependent on diffusion, so plants themselves contribute to the spatial heterogeneity of P by depleting it from the rhizosphere (Miguel et al., 2013). Poirier et al. (1991) documented the concentration of P in the xylem membrane to be as high as 10 mM, so an active P transport mechanism is required to uptake P by growing roots and within the plant body. In recent years, two different groups of P transporters have been cloned for growing plants with either low-affinity or high-affinity P transporters. Low-affinity P transporters operate in the chloroplast membrane (Versaw and Harrison, 2002), whereas the high-affinity ones (Km ranging from 50 to 300 μM) are expressed in root epidermal cells during growth under low P conditions (Harrison et al., 2002; Raghothama and Karthikeyan, 2005). This active transport system comprised of a high-affinity and energy-rich transport mechanism is pivotal (Smith et al., 2003) for P uptake from a soil system (Figure 2). Mimura (1995) demonstrated the existence of an active transport system as well as channel activity, which enables the translocation of P across the tonoplast membrane during uptake in the vacuole. So far, P-H+ co-transporters located in the plasma membrane belong to the PHT1 gene family, and most of them are expressed in root cells (Table 2). Raghothama and Karthikeyan (2005), and Smith et al. (2003) explained that under P-deprived conditions, PHT1 genes are significantly induced to enhance the ability to grow roots to uptake P from soil as well as to re-translocate it within growing plant compartments (Table 2). In contrast, repression of high-affinity PHT1 genes could alleviate P toxicity under high P-concentration soil conditions (Dissanayaka et al., 2020). The family of PHT1 transporters has been identified in several plant species (Table 2), and most of its members exhibit strong expression in roots and possess a range of affinities for P uptake (Nussaume et al., 2011). For instance, there are 9 PHT1 genes identified in Arabidopsis where AtPHT1;1 and AtPHT1;4 is highly expressed at the root-soil interface, including root hair, root cap, and epidermis cells under P deprivation conditions (Mudge et al., 2002). All these major genes are responsible for P uptake by roots from P-enriched or deficient rhizospheres (Misson et al., 2004; Shin et al., 2004; Catarecha et al., 2007). Kanno et al. (2016) employed a real-time 33P microimaging technique and showed that root cap accounts for 20% of total Arabidopsis seedling P uptake. Moreover, compared to wild-type and complemented lines, the expression of P-induced genes is higher in impaired P, and they function together in the endoplasmic reticulum of root tips that mediate root meristems during P deprivation (Ticconi et al., 2009). Recently, Balzergue et al. (2017) identified a new root growth inhibition signaling pathway, STOP1-ALMT1, under low-P stress in Arabidopsis. The authors proposed that P limitations enhance STOP1 abundance to activate ALMT1 expression that further promotes malate exudation by root apex internal cell layers that rapidly inhibits root cell elongation (Balzergue et al., 2017).
Transcription factor STOP1 (sensitive to proton toxicity 1) induces ALMT1 (aluminum-activated malate transporter-1) expression followed by cell elongation rapid inhibition by peroxide-dependent transportation lines (phf1-2) in a P-rich medium (Kanno et al., 2016). A previous study has identified a QTL that encodes ferroxidase, LPR1 (low phosphate root 1), which controls root growth under P starvation conditions in Arabidopsis thaliana (Reymond et al., 2006). β-glucuronidase (GUS) staining and qRT-PCR analysis showed that LPR1 is mainly expressed in root tips, and that loss of function of LPR1 and its close paralog LPR2 lines did not arrest primary root growth in a low-P medium (Svistoonoff et al., 2007). LPR1 could counteract PDR2 phosphate deficiency and cell wall stiffening in the stem cell niche and root apical meristem, suggesting that Fe accumulation mediated by malate is a crucial checkpoint for root development in response to P deficiency (Balzergue et al., 2017; Mora-Macías et al., 2017). Defects in P uptake in a growth medium under low P conditions have been noticed in AtPHT1;1 and AtPHT1;4 mutants (Misson et al., 2004; Shin et al., 2004). In rice, 10 out of 13 PHT1 genes were expressed in roots (Table 2), whereas OsPHT1;11 expression was exclusively induced by mycorrhiza symbiosis (Paszkowski et al., 2002). Ai et al. (2009) reported a significant increase in OsPHT1;2 and OsPHT1;6 in roots of rice under P deprived growth conditions. Similarly, 11 PHT1 genes have been reported in barley roots under P deprivation conditions, as shown in Table 2 (Preuss et al., 2011; Sisaphaithong et al., 2012). In addition to OsPHT1 genes, PHOSPHORUS-STARVATION TOLERANCE1 (OsPSTOL1) has been found to be up-regulated in root tissues and shown to increase P uptake under P-deficient conditions because of enhancement of early root growth and development (Gamuyao et al., 2012). The homologs of OsPSTOL1 in sorghum (SbPSTOL1) also alter root morphology and root system architecture, and significantly improve grain yield under low P conditions (Hufnagel et al., 2014). PSTOL1 proteins possess structural features of wall-associated kinase (WAK) proteins such as Ser/Thr kinase, transmembrane, and extracellular domains important for their functionality in the cell wall (He et al., 1999). Analysis of single-copy Ds transposon mutant repository of barley (Singh et al., 2006, 2012; Bregitzer et al., 2019) revealed that WAK proteins are implicated in the root growth of barley (Kaur et al., 2013; Tripathi et al., 2021), and that they are potentially new gene candidates for P starvation tolerance.
Post-translational regulations, such as ubiquitination and phosphorylation, are also involved in controlling PHT1s (Table 2). In Arabidopsis, the E2 ubiquitin conjugate UBC24/PHOSPHATE 2 (PHO2), regulated by miRNA399, could interact with PHT1s in the endoplasmic reticulum and mediate their ubiquitination, thereby regulating PHT1s activities negatively toward the plasma membrane under P-sufficient conditions (Bari et al., 2006; Chiou et al., 2006; Huang et al., 2013). Nitrogen limitation adaption (NLA) is a ring-type ubiquitin E3 ligase, and transcripts of NLA are targeted by miRNA827 and required for the degradation of PHT1;1 and PHT1;4 via ubiquitination (Peng et al., 2007; Pant et al., 2009). Loss of function of the NLA mutant increases the protein level of PHT1s in the plasma membrane (Lin et al., 2013). Previous studies have shown that PHT1 proteins require a phosphate transporter traffic facilitator (PHF1) to exit the endoplasmic reticulum (González et al., 2005; Bayle et al., 2011), but phosphorylation of PHT1s will inhibit their trafficking from the endoplasmic reticulum to the plasma membrane (Dissanayaka et al., 2020). Chen et al. (2015) indicated that Casein kinase-2 (CK2) could phosphorylate both low-affinity transporter OsPT2 and high-affinity PHT1 member OsPT8 during P-sufficient conditions in rice, thereby inhibiting their interaction with OsPHF1. In contrast, the OsCK2β3 subunit is degraded during P-deficient conditions, which increases P uptake (Chen et al., 2015). Moreover, OsCk2 could also phosphorylate OsPHF1 and OsPHO2 to modulate P homeostasis in rice (Wang et al., 2020; Yang et al., 2020). Plant species with an active transport system with higher expressions of transporter are required to enhance PAE and PUE in P-deficient soil and represent promising targets for engineering high PUE crop genotypes.
Internal Phosphorus Utilization
Internal phosphorus utilization efficiency (IPUE) is defined as the biomass produced per unit of P that accumulated in tissues. Higher IPUE has been attributed to optimum P translocation to harvestable plant parts by P re-translocation through phosphatase activities, P transporters (Table 2), and related mechanisms to transfer P from shoots to grains in different crop species (Parentoni and Souza Júnior, 2008; Rose and Wissuwa, 2012; Li et al., 2017; Adem et al., 2020). Plant P status largely determines IPUE through allocation and remobilization processes from different plant compartments. During the vegetative growth period, P uptake by the root system is continuous (Figure 3); however, remobilization and re-translocation of P from older/senescence tissues can become a very significant internal P source for new developing organs such as young leaves and grains (Figure 3). Therefore, during reproductive growth, remobilization and re-translocation from senescence vegetative tissues are typically the primary source of P for sink tissues as uptake by the root system starts declining and available soil P is depleted (Glassop et al., 2005; Rose and Wissuwa, 2012; El-Mazlouzi et al., 2020). Therefore, the major contributing traits in IPUE include P remobilization, P transporters, phosphatase activities, P release from vacuoles, and alternative biochemical pathways in different plant organs (Figures 2, 3).
Phosphorus Remobilization
Efficient P remobilization and re-translocation within plant parts are attributed to higher IPUE in growing plants. Remobilization of P from senescent vegetative tissues is critically important to plant growth and development (Veneklaas et al., 2012), particularly to soils with low P availability. Phosphorus re-translocation is assisted by specific transporters, namely, the PHO1 family (Table 2). Teng et al. (2017) reported that the Pht1 family of P transporters play an important role in the transport process across plant membranes (Table 2). Plants can remobilize more than 50% of P from senescent leaves (Aerts, 1996). However, similar to seed P contributions during early growth stages, potential P benefits from senescent vegetative parts are significantly smaller during the active growth period (Veneklaas et al., 2012). During this active growth period, external P uptake is the prime source for higher PUE. However, with the decline in growth rate during the later growing season, remobilization of P from older vegetative parts could sustain new growth and development and leaf function (Robinson et al., 2012). Remobilization of P reserves from older vegetative parts to newly growing parts is critically important in soils with low inherent P or low P inputs from organic or inorganic fertilizers. For instance, El-Mazlouzi et al. (2020) demonstrated that wheat plants grown under low P supply conditions translocate 72% of post-anthesis P uptake to grains compared to 56% share in plants grown with adequate P supply. Aerts (1996) demonstrated that growing plants tend to remobilize at least 50% of P from senescent vegetative parts. Akhtar et al. (2007) and Smith (2001) showed that re-translocation of P from metabolically inactive sites to actively growing plant compartments under low P conditions enhances PUE in Brassica napus. Similarly, the decreased growth rate under P deficiency conditions is always higher than the effects of senescence, making remobilization and re-translocation contributions significantly higher than uptake. Besides senescence, other plant species expressed the re-translocation of P in young and non-mature plant tissues showing remobilization and re-translocation recycling patterns (Akhtar et al., 2008; Nadeem et al., 2012). Moreover, a higher pre-translocation rate from shoots to roots impels tolerant Brassica napus cultivars to establish a better root system, thereby aiding plant growth under low P conditions and increasing PUE (Akhtar et al., 2008). Hence, to enhance PUE in growing crops on podzol soils in boreal ecosystems, it is important to understand and improve remobilization and re-translocation efficiency.
Phosphate Transporters in Growing Plants
Phosphate-1 (PHO1) protein plays a critical role in the transfer of P from roots to shoots once P is assimilated by the roots (Wang et al., 2021). In Arabidopsis, the PHO1 mutant has low P content in shoots and leaves due to a deficiency in loading P into xylem (Poirier et al., 1991). The Arabidopsis genome contains 11 members of the PHO1 gene family, and they all have the same topology and harbor an SYG1/PHO81/XPR1 (SPX) domain in the N-terminal hydrophilic portion and an ERD1/XPR1/SYG1 (EXS) domain in the C-terminal hydrophilic portion, which shows different functions in plant P homeostasis (Wang et al., 2004b; Wege et al., 2016; Wild et al., 2016). There are three homologs in the rice genome, OsPHO1;1, OsPHO1;2, and OsPHO1;3, as shown in Table 2 (Secco et al., 2010). Two node-localized transporter members (OsPHO1;1 and OsPHO1;2) of the PHO1 family are involved in P allocation to seeds in rice (Che et al., 2020). TOS17 insertion mutants of these two transporters showed lower grain yield and longer germination days because of impairment of P distribution to seeds (Che et al., 2020). Recently, Ma et al. (2021) identified the predominant role of OsPHO1;2 as a plasma membrane transporter in P reallocation during rice grain filling; OsPHO1;2 could export excess P from developing endosperm and unload P from pericarp into endosperm cells to maintain P homeostasis. The Ospho1;2 mutant showed a poor grain-filling phenotype, and the activity of a starch synthesis enzyme, ADP-glucose pyrophosphorylase (AGPase), was also inhibited. Overexpression of OsPHO1;2 enhances grain yield and PUE under P-deficient conditions in the field, which suggests that OsPHO1;2 is a target gene for enhancing grain yield for sustainable agriculture (Ma et al., 2021). In addition, PHO1 is a direct degradation target of PHO2 upon P resupply to P-starved plants (Bari et al., 2006; Liu et al., 2012). A recent research study indicated that an upstream open reading frame (uORF) of PHO1 strongly inhibits translation of the main open reading frame (ORF; Reis et al., 2020). AUG-to-UUG point mutation of PHO1 uORF enhances shoot P content and improves P deprivation response (Reis et al., 2020). Therefore, regulation of PHO1 expression via uORF editing shows a great potential for enhancing IPUE in different crops under P-deficient conditions (Table 2).
Intercellular re-distribution of P is regulated by four other phosphate transporter family members, PHT2, PHT3, PHT4, and PHT5 (Table 2), which are localized to plastid membranes, mitochondrial membranes, Golgi compartments, and tonoplasts, respectively (Rausch and Bucher, 2002; López-Arredondo et al., 2014). The primary function of these transporters is the transportation of P to each organelle, and they are also coordinating cytosolic P homeostasis and various metabolic processes by controlling P exchange among different compartments. Low-affinity P transporters from the PHT2 family are involved in the loading and unloading of P in vascular tissues, thereby affecting IPUE and overall PUE (Smith, 2001; Smith et al., 2003). In Arabidopsis, chloroplast envelope-localized PHT2;1 was identified as the low-affinity chloroplast P transporter (Table 2). Mutant analysis showed that PHT2;1 could influence related gene expression and P allocation in plant leaves during P-starvation (Daram et al., 1999; Versaw and Harrison, 2002). PHT3 proteins are responsible for translocating P into the mitochondrial matrix, where it is used for adenosine triphosphate (ATP) synthesis (Młodzińska and Zboińska, 2016). Zhu et al. (2012) showed that overexpression of three PHT3 genes (AtPHT3;1, AtPHT3;2, and AtPHT3;3) increased salt stress sensitivity by affecting ATP and gibberellin homeostasis in Arabidopsis. The PHT4 family in the Arabidopsis genome has six members. Their locations vary and they are considered to have additional distinct functions (Guo et al., 2008; Miyaji et al., 2015). Karlsson et al. (2015) identified PHT4;1 as a thylakoid transporter that functions in chloroplast phosphate compartmentation and ATP synthesis in the chloroplast stroma. PHT4;6 is located in the Golgi apparatus, which plays a critical role in preventing plant dark-induced senescence caused by cytosolic P-starvation. The PHT4;6 mutant showed low cytosolic P content and blockage of Golgi-related biosynthesis processes in Arabidopsis (Hassler et al., 2016), suggesting that PHT4 proteins are involved in P transportation among cytosol, chloroplasts, non-photosynthesis plastids, and the Golgi apparatus (Guo et al., 2008). Therefore, crop species with an active transport system could perform better in the redistribution of P within plant compartments after uptake when plants are grown on podzolic soils in boreal agroecosystems.
Phosphorus Re-translocation in Plant Parts
Plant leaves reserve P in various chemical forms, including nucleic acids, phospholipids, free P, and phosphorylated metabolites (Robinson et al., 2012). Intercellular and secreted ribonucleases (RNases) and acid phosphatases (APases) play an essential role in remobilizing P to growing plant parts from the degradation of organic P in senescent leaves (Dissanayaka et al., 2018). APases contribute to increased PUE in beans through P remobilization from old leaves (Akhtar et al., 2008). Also, APases produced by plants and microbes are involved in organic P degradation, which catalyzes the hydrolysis of easter-phosphate bonds and anhydrides to release P with an optimum acid pH at the 4.7–7 level (Raghothama and Karthikeyan, 2005; López-Arredondo et al., 2014). Purple acid phosphatases (PAPs) represent a specific group of APases, which hydrolyze P-ester to facilitate P use efficiency in plants (Ghahremani et al., 2019). Synthesis and secretion of PAPs have been identified in many crop species as a response to low P availability (Bozzo et al., 2006; Zhang et al., 2011; González-Muñoz et al., 2015; Li et al., 2017; Dissanayaka et al., 2018). The function of intercellular (vacuolar) PAPs is to recycle P from intercellular organic P pools. This process is accompanied by a decrease in cytoplasmic phosphate metabolites such as glycerol-3-phosphate, glucose-6-phosphate, and ATP under P-depleted conditions (Hurley et al., 2010; Tian and Liao, 2018). In the Arabidopsis genome, the AtPAP family is encoded by 29 genes and divided into three polygenetic groups according to amino acid sequences (Raghothama and Karthikeyan, 2005; Tran et al., 2010; Wang et al., 2014). Physiological functions of some AtPAPs genes were identified in transgenic plants. Wang et al. (2014) used all AtPAP mutants from Arabidopsis and showed that AtPAP10 secreted APases, and that AtPAP12 and AtPAP26 isozymes were both intracellular and secreted APases. AtPAP26 was reported to be a dual-targeted gene that played a vital role in maintaining P homeostasis in vacuolar P recycling and rhizosphere organic P utilization during P-deficient conditions (Veljanovski et al., 2006; Hurley et al., 2010). Loss of function of the AtPAP26 mutant interferes in P remobilization metabolism during Arabidopsis leaf senescence status (Robinson et al., 2012). Also, OsPAP26 played dual functions (intercellular and extracellular) in rice; overexpressed OsPAP26 plants increased P remobilization from senescent leaves to non- senescent leaves compared to OsPAP26-RNAi lines (Gao et al., 2017).
Nucleic acid pools make a great contribution for P scavenging and recycling in P-starved plants (Stigter and Plaxton, 2015; Dissanayaka et al., 2020), and ribosomal RNA (rRNA) is the largest organic P pool in mature leaves. During early leaf senescence and P deficiency, degrading RNA by some ribonucleases (Rnases) is considered a rescue system so P could be remobilized to young organs in higher plants (Tran et al., 2010; Robinson et al., 2012). Two of the S-like Rnase genes, RNS1 and RNS2, belong to the T2/S superfamily and significantly induce mediating the response of low-P treatments and senescence. Higher content of anthocyanin was observed in antisense RNS1 or RNS2 transgenic Arabidopsis lines, which was the main symptom of P-deficiency in plants (Bariola et al., 1999). RNS1 was predicted as an extracellular protein, whereas RNS2 localized to the vacuole and endoplasmic reticulum (Poirier and Bucher, 2002; Hillwig et al., 2011). RNS2 can degrade RNA to 3'NMP; then, 3'-NMP is subsequently dephosphorylated by PAPs such as AtPAP26 or phosphorylases (Bassham and MacIntosh, 2017; Dissanayaka et al., 2018). rRNA degradation and turnover also depend on ribophagy (an autophagy-like process) that has been documented in yeast and plants (Kraft et al., 2008; Floyd et al., 2015; Huang et al., 2015). Higher levels of rRNA in vacuoles accumulated in rns2 atg9 double mutant plants, which revealed that both S-like Rnase and autophagy were involved in an RNA turnover mechanism (Floyd et al., 2015). These findings suggest the importance of RNA as a P source for remobilization during P-starvation and late plant growth stages. However, the role of Rnase in RNA turnover and cellular P homeostasis in other crops needs to be further investigated.
Organelle DNAs from mitochondria and chloroplasts are known to be highly abundant and act as an internal reservoir of phosphate in plant cells (Oldenburg and Bendich, 2015; Takami et al., 2018). Degradation of organelle DNA by DPD1, a conserved and Mg2+-dependent exonuclease, was demonstrated to have a positive correlation with PUE during P-starvation and leaf senescence in Arabidopsis (Matsushima et al., 2011; Takami et al., 2018). T-DNA insertion DPD1 mutants showed higher chloroplast and mitochondria DNA copy numbers than the wild-type plant and attenuated P derivation response (Takami et al., 2018). Therefore, the DPD1 system showed a great engineering breeding potential for enhancing PUE in crop production.
As the second organic P source in plants, phospholipids account for 25% of the total organic P pool of a mature leaf (Veneklaas et al., 2012; Dissanayaka et al., 2018). Therefore, phospholipases also play an important role in P utilization by altering membrane lipid compositions (Shen et al., 2011). During P starvation, decreasing phospholipids and increasing non-phosphorus lipids such as galactolipid and sulfolipid, mostly digalactosyldiacylglycerol (DGDG), monogalactosyldiacylglycerol (MGDG), and sulfosulfoquinovosyldiacylglycerol (SQDG), could be the strategy for optimization of P utilization in plants (Härtel et al., 1998, 2000; Li et al., 2008). For instance, compared to Arabidopsis, six Proteaceae species from southwestern Australia in severely P-impoverished soil showed a higher percentage of galactolipids and sulfolipids during the vegetative stage (Lambers et al., 2012b). Expression of galactolipids and sulfolipid biosynthesis-related genes in younger leaves is generally up-regulated during P starvation in high-PUE rice genotypes (Adem et al., 2020). Diacylglycerol is considered an important intermediate in phospholipid degradation, which is the precursor for galactolipid biosynthesis. Phospholipases such as phospholipase C (PLC) and phospholipase D (PLD) have been suggested to be involved in the process of degradation. Low-P treatment and leaf senescence resulted in up-regulation of PLC, PLD, and phosphatide phosphatase (PAH) genes in plants (Hong and Lu, 2014; Jeong et al., 2017). Phosphatidic acid (PA) is generated by PLD and subsequently hydrolyzed by PAH to produce DAG and release P (Wang et al., 2006; Dissanayaka et al., 2018). Lipidomic analysis of PLDζ2 mutant Arabidopsis plants showed reduced capacity for galactolipid accumulation under P-limited stress (Cruz-Ramírez et al., 2006; Lin et al., 2020). Moreover, Cruz-Ramírez et al. (2006) found that both PLDζ1 and PLDζ2 were essential for lipid turnover by hydrolyzing phosphatidylcholine (PC) in Arabidopsis rosettes and roots for P deficiency acclimation. PLC can directly hydrolyze phospholipids to produce DAG. NPC4, a nonspecific PLC in Arabidopsis, was significantly upregulated during P deprivation. However, there was no apparent effect on phospholipid hydrolysis and DGDG biosynthesis in the npc4 T-DNA insertion mutant (Nakamura et al., 2005). NPC5 was reported to be responsible for around 50% of DGDG biosynthesis in leaves by hydrolyzing plasma membrane phospholipids in response to P limitation (Gaude et al., 2008). Recently, Okazaki et al. (2013) found a novel class of plant lipid, glucuronosyldiacylglycerol (GlcADG), which accumulated under P-depleted conditions in Arabidopsis and rice. Moreover, in the biosynthesis of GlcADG that shared the same pathway of SQDG in chloroplast, the SQDG synthase mutant sqd2 showed that neither SQDG nor GlcADG accumulated under P-starved conditions (Okazaki et al., 2013). Taken together, the research findings mentioned above revealed that these intercellular P pools may play a prominent role in internal P utilization when plants are facing P starvation.
P Release From Vacuoles
Plants distribute P in different cell compartments selectively, such as for metabolic activities P is located in cytoplasts, whereas a vacuole is used for stored P pools (Theodorou and Plaxton, 1993). Therefore, vacuoles are known as major storage compartments under sufficient P supply conditions. Bieleski (1973) demonstrated that more than 70% of intercellular P was stored in vegetative plant vacuoles. The vacuole P in buffering cytoplasmic P maintains cellular homeostasis under short-term P starvation conditions (Yang et al., 2017). Therefore, translocation of P to and from a vacuole under P deficient conditions mainly maintains P homeostasis in growing plants. Luan and Lan (2019) demonstrated that vacuolar phosphate transporters (VPTs) play an essential role in buffering the level of cytoplasmic P to maintain cellular P homeostasis in response to changes in external P supply and metabolic demand. In yeast, Saccharomyces cerevisiae, ScVTC1, ScVTC2, ScVTC3, and ScVTC4 are four vacuolar transmembrane proteins responsible for polyphosphate accumulation (Secco et al., 2012). In contrast, ScPHO91 acts as an efflux transporter during P-starvation (Hürlimann et al., 2007). However, the transportation system from the tonoplast in plants is still unclear; tonoplast P transporters are not fully understood yet (Panigrahy et al., 2009). In a recent research study, the VPTs/phosphate transporter 5 (PHT5) family with an SYG1/PHO81/XPR1 (SPX) domain, as well as a major facilitator superfamily (MFS) domain, has been identified functioning as a vacuolar influx P transporter, which has three members in the Arabidopsis SPX-MFS family (Liu et al., 2015, 2016b). Loss of function of the PHT5;1 mutant impairs P accumulation, and a decrease of 40% in P levels of the mutant line was observed compared to the wild type (Liu et al., 2016b). Similarly, the OsSPX-MFS1 and OsSPX-MFS3 homologs were also identified as a major tonoplast-localized influx P transporter in rice (Luan and Lan, 2019). Under P-deficient conditions, the influx of P from apoplast to the PHT1 family and the efflux of P from vacuoles by vacuolar P efflux transporters (VPEs) facilitate cytosolic P homeostasis. In rice, Xu et al. (2019) identified two tonoplast-localized VPE proteins, which evolved from ancestral plasma membrane glycerol-3-phosphate transporter protein (GlpT), and named them OsVPE1 and OsVPE2. Overexpression of either of these two genes showed decreased vacuolar P content. Moreover, the vacuolar P content of the OsVPE1 OsVPE2 double mutant was higher than that of the wild types. Therefore, it is important to understand P homeostasis to enhance PUE in plant species grown in podzolic soils. Vacuole P could serve an important source to enhance IPUE particularly during P remobilization under P starvation conditions.
Alternative Biochemical Pathways
Metabolic redundancy could help plants to respond quickly to an unfavorable environment (Plaxton and Tran, 2011). Induction of P-independent or inorganic pyrophosphate (PPi)-dependent glycolytic metabolic reactions might enhance PUE by P recycling and reduction of cellular ATP pool consumption by utilizing alternative P pools under P starvation conditions (Wang et al., 2010). During long-term P stress, a marked decline in nucleoside pools such as ATP and ADP was observed because of cytoplasmic P exhaustion (Plaxton and Podestá, 2006). P-dependent cytosolic glycolysis and the phosphorylating pathway of the mitochondrial electron transport chain could be inhibited. Therefore, alternative reactions of cytosolic glycolysis contribute to maintaining carbon flux to generate energy (Plaxton and Tran, 2011). Ppi is a by-product of various biosynthesis reactions, such as polymerization reactions during nucleic acid and protein, polysaccharide, and macromolecule synthesis, with a concentration of up to 0.5 mM in the cytosol. Unlike animals, plant cells lack soluble Ppiase. The level of Ppi appears to be unaffected compared to adenine nucleotide and uridine nucleotide energy donor systems and shows the importance of Ppi as an initiative energy donor in P-deficient cytosol cells (Theodorou and Plaxton, 1993).
Some energy-poor anaerobic microorganisms use Ppi-phosphofructokinase (Ppi-PFK) instead of ATP-phosphofructokinase (ATP-PFK) to convert Ppi and Fru-6-P into Fru-1,6-P2, such as Priopionibacterium shermanii and Entamoeba histolytica (Mertens, 1991). Additionally, pyruvate P dikinase (PPDK) could also use Ppi to convert adenosine monophosphate (AMP) and phosphoenolpyruvate (PEP) to pyruvate, ATP, and P for P-starved plants (Tran et al., 2010). Significant upregulation of glycolytic enzyme activities of sucrose synthase, UDP-glcpyrophosphorylase, Ppi-PFK, PPDK, and tonoplast Ppi-dependent proton pump (H+-Ppiase) has been confirmed during P-starvation in plants (Theodorou and Plaxton, 1994; Palma et al., 2000; Nasr Esfahani et al., 2016). Ppi-dependent phosphorylation of Fru-6-P is catalyzed by Ppi-PFK, which appears to be an adaptive enzyme for coping with P deficiency (Theodorou et al., 1992). Overexpression of H+-Ppiase in transgenic Arabidopsis, tomato, and rice showed better performance in limited P conditions (Yang et al., 2007). The use of Ppi rather than nucleoside Ps such as ATP and ADP could confer a significant bioenergetic advantage to plants subjected to environmental stresses like in podzolic soils.
PHE, PAE, And IPUE: Potential Strategies to Enhance Pue in Podzolic Soils
From the above review, it is evident that seed phytate-P hydrolysis efficiency (PHE), PAE, and IPUE could be fundamental targets to enhance the overall plant PUE during plant growth and harvest. However, it is important to understand which one is more critical considering all available options right from seed phytate hydrolysis, external P uptake, to efficient internal P utilization to attain desirable harvest or produce (Wang et al., 2010; Veneklaas et al., 2012; van de Wiel et al., 2016). For instance, in podzolic soils, the initial seed P stock could play an important role in sustaining early seedling growth and successful establishment for an extended growth period (Figure 3). Boreal climate conditions during early growth stages may restrict root architecture and root morphological traits, seedling establishment, and, finally, biomass accumulation in young seedlings, which could enhance P efflux from seeds, resulting in P losses to the environment in podzolic soils. However, agronomic interventions such as plastic mulching and changing seeding time or depth could provide more crop heat units during early growth stages (Kwabiah et al., 2003; Kwabiah, 2005) and enhance PHE and translocation to developing seedling sinks. After seedling establishment, P uptake is more critical compared to internal P utilization to support plant growth. Organic P sources from soil amendments could provide a better solution to enhance PAE through improved soil microbial communities and enhanced acid phosphatase activities and availabilities of P in podzolic soils (Ali et al., 2019b). Biochar, a recalcitrant carbon, could be an important soil amendment to improve soil physicochemical properties, reduce carbon footprints, and enhance crop productivity in podzolic soils (Ahmed et al., 2016; Altdorff et al., 2018; Ashiq et al., 2020). Literature review and meta-analysis studies reported a wide range of biochar effects, including positive, negative, and neutral, on physicochemical and biological soil fertility and plant responses involving multiple complex mechanisms (Spokas et al., 2012; Ding et al., 2016; Joseph et al., 2021). Effects of biochar application on boreal podzolic soils are still in the initial stages (Abedin and Unc, 2020; Ashiq et al., 2020; Abedin et al., 2021). Only a few studies have documented the superior performance of biochar in improving the fertility of acidic and course soils via different mechanisms. The meta-analysis of Glaser and Lehr (2019) calculated an average of 4.6-fold increase in P availability for all soil groups amended with biochar and fertilizers; especially, acidic soils (with pH <6.5) had a 5.1-fold increase in P availability compared to neutral and alkaline soils. The increased P availability in soil groups that received 5–20 Mg biochar ha−1 might partly explain the reported crop yield increase by 12–40% (Joseph et al., 2021). P availability and use efficiency could be related to several soil properties improved by biochar addition; one of them is increased (75%) mycorrhizal colonization of crop root compared to 20% for fertilizer alone (Blackwell et al., 2015). Chemical reactions such as ion exchange, redox, adsorption, and desorption activities on the surface of biochar and its liming effect contribute to P availability and use efficiency (Blackwell et al., 2015; Glaser and Lehr, 2019).
Manske et al. (2001) reported that in spring wheat, PUE was determined by PAE with restricted P availability in the growth environment in both acidic and alkaline soils. Low P availability in podzolic soils and shallow nature might reduce overall PUE in growing plants under field conditions particularly from early seedling growth because of low crop heat units. Similarly, the importance of PAE has been highlighted in common bean (Beebe et al., 2006), soybean (Zhao et al., 2004), and wheat (El Mazlouzi et al., 2020). Fujita et al. (2004) and Zhang et al. (2009) suggested that enhancing PAE could be one of the main strategies to enhance PUE in P-deficient soils. This could be achieved through the selection of crop species with better root architecture, more root hair formation, proteoid roots, root exudates, root symbiosis, and phosphate transporters to acquire unevenly distributed P in podzolic soils of boreal agroecosystems. However, in P-adequate production systems, higher IPUE could provide more benefits to improve PUE than PAE. Balemi and Schenk (2009a,b) reported variations in PAE in different potato cultivars, whereas no difference in PUE was observed. Therefore, such reports support the idea that PAE and PUE are mainly controlled by the genetic potential of plants under given growth conditions (Clark and Duncan, 1991; Shenoy and Kalagudi, 2005). For instance, maize plants showed higher PUE associated with PAE rather than IPUE under low P conditions (Corrales et al., 2007). El Mazlouzi et al. (2020) reported higher P translocation of external P to wheat grains under P deficient conditions. Therefore, selecting crop genotypes with better genetic makeup, uptake, and internal utilization efficiency (George and Richardson, 2008; Zhang et al., 2009) could provide a better solution to enhance PUE in crops and at cropping systems level in podzolic soils. Modern tools such as gene- editing could be valuable for precise alteration in genes to improve the genetics of crop plants for PUE.
Summary
Phosphorus-efficient plants may play a major role in increasing crop yields in podzolic soils because of low soil pH and non-availability of P, rapidly decreasing P resources, and growing environmental concerns. Also, podzolic soils have high P fixation capacities due to Al3+ and Fe2+, which is a big challenge in enhancing PAE. Literature-reported improvements in PUE are often attributed more to PAE under limited P supply and to IPUE under high P conditions. Therefore, improvement of both PAE and IPUE in a given species in podzolic soils seems to be the appropriate breeding approach along with enhanced seed P reserves that can support good seedling stand. However, conventional breeding strategies are mainly employed to achieve higher biomass or yield in a high-input environment, such as super-hybrid varieties, which require more P fertilizer than other strains to achieve an economically optimum yield. This could enhance financial and environmental burdens. Future further research needs to improve the understanding of podzolic soil amendments, effects of amendments on soil physicochemical properties, soil microbial communities, enzyme activities, and P availability under acidic soil conditions. Accumulation of P in acidic soils due to heavy manure/fertilizer application may cause P toxicity to crop plants in different cropping systems, and this needs to be investigated to assess the effects of excessive P loading to leaves and grains, and the possible deleterious effects on plant growth and development. Furthermore, assessing the organic acid profile of different crop species grown on podzolic soils would enhance the understanding of PUE. Additionally, it is important to investigate the role of P transporters and internal P redistribution and/or any specific plant part that contributes significant P loading to grains and that could be a potentially powerful strategy for increasing P efficiency in modern crops grown in intensive cropping systems. New genetic strategies could also be used to sequester the unavailable P from soil through active and efficient P transporters, and reutilized in P-deficient situations.
Author Contributions
MN and MC conceived the concept. MN, JW, HG, AK, and SS wrote the first version. MC, AM, and JS provided critical comments. All authors contributed in the final article and approved the submission.
Conflict of Interest
The authors declare that the research was conducted in the absence of any commercial or financial relationships that could be construed as a potential conflict of interest.
Publisher's Note
All claims expressed in this article are solely those of the authors and do not necessarily represent those of their affiliated organizations, or those of the publisher, the editors and the reviewers. Any product that may be evaluated in this article, or claim that may be made by its manufacturer, is not guaranteed or endorsed by the publisher.
References
Abdolzadeh, A., Wang, X., Veneklaas, E. J., and Lambers, H. (2010). Effects of phosphorus supply on growth, phosphate concentration and cluster-root formation in three Lupinus species. Ann. Bot. 105, 365–374. doi: 10.1093/aob/mcp297
Abedin, J., and Unc, A. (2020). Addition of biochar to acidic boreal podzolic soils enhances micronutrient availability and crop productivity. Open Agricult. 5, 188–201. doi: 10.1515/opag-2020-0021
Abedin, J., Unc, A., and Anne Naeth, M. (2021). The utility of biochar for increasing the fertility of new agricultural lands converted from boreal forests. Can. J. Soil Sci. 2, 1–12. doi: 10.1139/cjss-2021-0002
Adem, G. D., Ueda, Y., Hayes, P. E., and Wissuwa, M. (2020). Genetic and physiological traits for internal phosphorus utilization efficiency in rice. PLoS ONE 15:e0241842. doi: 10.1371/journal.pone.0241842
Adesemoye, A. O., and Kloepper, J. W. (2009). Plant–microbes interactions in enhanced fertilizer-use efficiency. Appl. Microbiol. Biotechnol. 85, 1–12. doi: 10.1007/s00253-009-2196-0
Aerts, R (1996). Nutrient resorption from senescing leaves of perennials: are there general patterns? J. Ecol. 84, 597–608. doi: 10.2307/2261481
Ahanger, M. A., Hashem, A., Abd-Allah, E. F., and Ahmad, P. (2014). “Arbuscular mycorrhiza in crop improvement under environmental stress,” in Emerging Technologies and Management of Crop Stress Tolerance, eds P. Ahmad and S. Rasool (San Diego, CA: Academic Press), 69–95.
Ahmed, A., Kurian, J., and Raghavan, V. (2016). Biochar influences on agricultural soils, crop production, and the environment: a review. Environ. Rev. 24, 495–502. doi: 10.1139/er-2016-0008
Ai, P., Sun, S., Zhao, J., Fan, X., Xin, W., Guo, Q., et al. (2009). Two rice phosphate transporters, OsPht1;2 and OsPht1;6, have different functions and kinetic properties in uptake and translocation. Plant J. 57, 798–809. doi: 10.1111/j.1365-313X.2008.03726.x
Akhtar, M. S., Oki, Y., and Adachi, T. (2008). Intraspecific variations of phosphorus absorption and remobilization, P forms, and their internal buffering in brassica cultivars exposed to a P-stressed environment. J. Integr. Plant Biol. 50, 703–716. doi: 10.1111/j.1744-7909.2008.00675.x
Akhtar, M. S., Oki, Y., Adachi, T., Murata, Y., and Khan, M. H. R. (2007). Relative phosphorus utilization efficiency, growth response, and phosphorus uptake kinetics of brassica cultivars under a phosphorus stress environment. Commun. Soil Sci. Plant Anal. 38, 1061–1085. doi: 10.1080/00103620701280266
Ali, W., Nadeem, M., Ashiq, W., Zaeem, M., Gilani, S. S. M., Rajabi-Khamseh, S., et al. (2019b). The effects of organic and inorganic phosphorus amendments on the biochemical attributes and active microbial population of agriculture podzols following silage corn cultivation in boreal climate. Sci. Rep. 9:17297. doi: 10.1038/s41598-019-53906-8
Ali, W., Nadeem, M., Ashiq, W., Zaeem, M., Thomas, R., Kavanagh, V., et al. (2019a). Forage yield and quality indices of silage-corn following organic and inorganic phosphorus amendments in podzol soil under boreal climate. Agronomy 9:489. doi: 10.3390/agronomy9090489
Alori, E. T., Glick, B. R., and Babalola, O. O. (2017). Microbial phosphorus solubilization and its potential for use in sustainable agriculture. Front. Microbiol. 8:971. doi: 10.3389/fmicb.2017.00971
Altdorff, D., Galagedara, L., Nadeem, M., Cheema, M., and Unc, A. (2018). Effect of agronomic treatments on the accuracy of soil moisture mapping by electromagnetic induction. CATENA 164, 96–106. doi: 10.1016/j.catena.2017.12.036
Ao, J., Fu, J., Tian, J., Yan, X., and Liao, H. (2010). Genetic variability for root morph-architecture traits and root growth dynamics as related to phosphorus efficiency in soybean. Funct. Plant Biol. 37, 304–312. doi: 10.1071/FP09215
Ashiq, W., Nadeem, M., Ali, W., Zaeem, M., Wu, J., Galagedara, L., et al. (2020). Biochar amendment mitigates greenhouse gases emission and global warming potential in dairy manure based silage corn in boreal climate. Environ. Poll. 265:114869. doi: 10.1016/j.envpol.2020.114869
Aziz, T., Finnegan, P. M., Lambers, H., and Jost, R. (2014). Organ-specific phosphorus-allocation patterns and transcript profiles linked to phosphorus efficiency in two contrasting wheat genotypes. Plant Cell Environ. 37, 943–960. doi: 10.1111/pce.12210
Balemi, T., and Schenk, M. K. (2009a). Genotypic variation of potato for phosphorus efficiency and quantification of phosphorus uptake with respect to root characteristics. J. Plant Nutr. Soil Sci. 172, 669–677. doi: 10.1002/jpln.200800246
Balemi, T., and Schenk, M. K. (2009b). Genotypic difference of potato in carbon budgeting as a mechanism of phosphorus utilization efficiency. Plant Soil 322, 91–99. doi: 10.1007/s11104-009-9897-0
Balzergue, C., Dartevelle, T., Godon, C., Laugier, E., Meisrimler, C., Teulon, J.-M., et al. (2017). Low phosphate activates STOP1-ALMT1 to rapidly inhibit root cell elongation. Nat. Commun. 8:15300. doi: 10.1038/ncomms15300
Bari, R., Datt Pant, B., Stitt, M., and Scheible, W.-R. (2006). PHO2, MicroRNA399, and PHR1 define a phosphate-signaling pathway in plants. Plant Physiol. 141, 988–999. doi: 10.1104/pp.106.079707
Bariola, P. A., MacIntosh, G. C., and Green, P. J. (1999). Regulation of S-like ribonuclease levels in Arabidopsis. Antisense inhibition of RNS1 or RNS2 elevates anthocyanin accumulation. Plant Physiol. 119, 331–342. doi: 10.1104/pp.119.1.331
Bassham, D. C., and MacIntosh, G. C. (2017). Degradation of cytosolic ribosomes by autophagy-related pathways. Plant Sci. 262, 169–174. doi: 10.1016/j.plantsci.2017.05.008
Bates, T. R., and Lynch, J. P. (2000). The efficiency of Arabidopsis thaliana (Brassicaceae) root hairs in phosphorus acquisition. Am. J. Bot. 87, 964–970. doi: 10.2307/2656995
Batten, G. D (1992). A review of phosphorus efficiency in wheat. Plant Soil 146, 163–168. doi: 10.1007/BF00012009
Battini, F., Cristani, C., Giovannetti, M., and Agnolucci, M. (2016). Multifunctionality and diversity of culturable bacterial communities strictly associated with spores of the plant beneficial symbiont Rhizophagus intraradices. Microbiol. Res. 183, 68–79. doi: 10.1016/j.micres.2015.11.012
Bayle, V., Arrighi, J.-F., Creff, A., Nespoulous, C., Vialaret, J., Rossignol, M., et al. (2011). Arabidopsis thaliana high-affinity phosphate transporters exhibit multiple levels of posttranslational regulation. Plant Cell 23, 1523–1535. doi: 10.1105/tpc.110.081067
Beebe, S. E., Rojas-Pierce, M., Yan, X., Blair, M. W., Pedraza, F., Muñoz, F., et al. (2006). Quantitative trait loci for root architecture traits correlated with phosphorus acquisition in common bean. Crop Sci. 46, 413–423. doi: 10.2135/cropsci2005.0226
Begum, N., Qin, C., Ahanger, M. A., Raza, S., Khan, M. I., Ashraf, M., et al. (2019). Role of arbuscular mycorrhizal fungi in plant growth regulation: implications in abiotic stress tolerance. Front. Plant Sci. 10, 1068. doi: 10.3389/fpls.2019.01068
Bhosale, R., Giri, J., Pandey, B. K., Giehl, R. F. H., Hartmann, A., Traini, R., et al. (2018). A mechanistic framework for auxin dependent Arabidopsis root hair elongation to low external phosphate. Nat. Commun. 9:1409. doi: 10.1038/s41467-018-03851-3
Bieleski, R. L (1973). Phosphate pools, phosphate transport, and phosphate availability. Ann. Rev. Plant Physiol. 24, 225–252. doi: 10.1146/annurev.pp.24.060173.001301
Blackwell, P., Joseph, S., Munroe, P., Anawar, H. M., Storer, P., Gilkes, R. J., et al. (2015). Influences of biochar and biochar-mineral complex on mycorrhizal colonisation and nutrition of wheat and sorghum. Pedosphere 25, 686–695. doi: 10.1016/S1002-0160(15)30049-7
Bozzo, G. G., Dunn, E. L., and Plaxton, W. C. (2006). Differential synthesis of phosphate-starvation inducible purple acid phosphatase isozymes in tomato (Lycopersicon esculentum) suspension cells and seedlings. Plant Cell Environ. 29, 303–313. doi: 10.1111/j.1365-3040.2005.01422.x
Bray, R. H., and Kurtz, L. T. (1945). Determination of total, organic, and available forms of phosphorus in soils. Soil Sci. 59, 39–46. doi: 10.1097/00010694-194501000-00006
Bregitzer, P., Brown, R. H., Dahleen, L. S., Singh, J., Cooper, L. D., Hayes, P. M., et al. (2019). Registration of the barley transposon-tagged population II: sixty-one lines each characterized for Ds insertion sites. J. Plant Registr. 13, 109–112. doi: 10.3198/jpr2017.10.0070crgs
Campos, P., Borie, F., Cornejo, P., López-Ráez, J. A., López-García, Á., and Seguel, A. (2018). Phosphorus acquisition efficiency related to root traits: is mycorrhizal symbiosis a key factor to wheat and barley cropping? Front. Plant Sci. 9:752. doi: 10.3389/fpls.2018.00752
Carvalhais, L. C., Dennis, P. G., Fedoseyenko, D., Hajirezaei, M.-R., Borriss, R., and von Wirén, N. (2011). Root exudation of sugars, amino acids, and organic acids by maize as affected by nitrogen, phosphorus, potassium, and iron deficiency. J. Plant Nutr. Soil Sci. 174, 3–11. doi: 10.1002/jpln.201000085
Catarecha, P., Segura, M. D., Franco-Zorrilla, J. M., García-Ponce, B., Lanza, M., Solano, R., et al. (2007). A mutant of the Arabidopsis phosphate transporter PHT1;1 displays enhanced arsenic accumulation. Plant Cell 19, 1123–1133. doi: 10.1105/tpc.106.041871
Ceasar, S. A., Hodge, A., Baker, A., and Baldwin, S. A. (2014). Phosphate concentration and arbuscular mycorrhizal colonisation influence the growth, yield and expression of twelve pht1 family phosphate transporters in foxtail millet (Setaria italica). PLoS ONE 9, e108459. doi: 10.1371/journal.pone.0108459
Che, J., Yamaji, N., Miyaji, T., Mitani-Ueno, N., Kato, Y., Shen, R. F., et al. (2020). Node-localized transporters of phosphorus essential for seed development in rice. Plant Cell Physiol. 61, 1387–1398. doi: 10.1093/pcp/pcaa074
Chen, A., Hu, J., Sun, S., and Xu, G. (2007). Conservation and divergence of both phosphate- and mycorrhiza-regulated physiological responses and expression patterns of phosphate transporters in solanaceous species. New Phytologist 173, 817–831. doi: 10.1111/j.1469-8137.2006.01962.x
Chen, J., Wang, Y., Wang, F., Yang, J., Gao, M., Li, C., et al. (2015). The rice CK2 kinase regulates trafficking of phosphate transporters in response to phosphate levels. Plant Cell 27, 711–723. doi: 10.1105/tpc.114.135335
Cheng, L., Bucciarelli, B., Liu, J., Zinn, K., Miller, S., Patton-Vogt, J., et al. (2011). White lupin cluster root acclimation to phosphorus deficiency and root hair development involve unique glycerophosphodiester phosphodiesterases. Plant Physiol. 156, 1131–1148. doi: 10.1104/pp.111.173724
Chiou, T.-J., Aung, K., Lin, S.-I., Wu, C.-C., Chiang, S.-F., and Su, C.-L. (2006). Regulation of phosphate homeostasis by MicroRNA in arabidopsis. Plant Cell 18, 412–421. doi: 10.1105/tpc.105.038943
Chiou, T.-J., Liu, H., and Harrison, M. J. (2001). The spatial expression patterns of a phosphate transporter (MtPT1) from Medicago truncatula indicate a role in phosphate transport at the root/soil interface. Plant J. 25, 281–293. doi: 10.1046/j.1365-313x.2001.00963.x
Chun-Yan, L., Huang, Y.-M., Ying-Ning, Z., and Qiang-Sheng, W. (2014). Regulation of root length and lateral root number in trifoliate orange applied by peroxide hydrogen and arbuscular mycorrhizal fungi. Notulae Botanicae Horti Agrobotanici Cluj-Napoca 42, 94–98. doi: 10.15835/nbha4219442
Ciarelli, D. M., Furlani, A. M. C., Dechen, A. R., and Lima, M. (1998). Genetic variation among maize genotypes for phosphorus-uptake and phosphorus-use efficiency in nutrient solution. J. Plant Nutr. 21, 2219–2229. doi: 10.1080/01904169809365556
Clark, R. B., and Duncan, R. R. (1991). Improvement of plant mineral nutrition through breeding. Field Crops Res. 27, 219–240. doi: 10.1016/0378-4290(91)90063-2
Cordell, D., Drangert, J.-O., and White, S. (2009). The story of phosphorus: global food security and food for thought. Glob. Environ. Change 19, 292–305. doi: 10.1016/j.gloenvcha.2008.10.009
Cordell, D., and White, S. (2013). Sustainable phosphorus measures: strategies and technologies for achieving phosphorus security. Agronomy 3, 86–116. doi: 10.3390/agronomy3010086
Corrales, I., Amenós, M., Poschenrieder, C., and Barceló, J. (2007). Phosphorus efficiency and root exudates in two contrasting tropical maize varieties. J. Plant Nutr. 30, 887–900. doi: 10.1080/15226510701375085
Cruz-Ramírez, A., Oropeza-Aburto, A., Razo-Hernández, F., Ramírez-Chávez, E., and Herrera-Estrella, L. (2006). Phospholipase DZ2 plays an important role in extraplastidic galactolipid biosynthesis and phosphate recycling in Arabidopsis roots. Proc. Natl. Acad. Sci. U. S. A. 103, 6765–6770. doi: 10.1073/pnas.0600863103
Daram, P., Brunner, S., Persson, B. L., Amrhein, N., and Bucher, M. (1998). Functional analysis and cell-specific expression of a phosphate transporter from tomato. Planta 206, 225–233. doi: 10.1007/s004250050394
Daram, P., Brunner, S., Rausch, C., Steiner, C., Amrhein, N., and Bucher, M. (1999). Pht2;1 encodes a low-affinity phosphate transporter from arabidopsis. Plant Cell 11, 2153–2166. doi: 10.1105/tpc.11.11.2153
Davies, T. G. E., Ying, J., Xu, Q., Li, Z. S., Li, J., and Gordon-Weeks, R. (2002). Expression analysis of putative high-affinity phosphate transporters in Chinese winter wheats. Plant Cell Environ. 25, 1325–1339. doi: 10.1046/j.1365-3040.2002.00913.x
DeBruyn, J. M., Nixon, L. T., Fawaz, M. N., Johnson, A. M., and Radosevich, M. (2011). Global biogeography and quantitative seasonal dynamics of gemmatimonadetes in soil. Appl. Environ. Microbiol. 77, 6295–6300. doi: 10.1128/AEM.05005-11
Delhaize, E., and Ryan, P. R. (1995). Aluminum toxicity and tolerance in plants. Plant Physiol. 107, 315–321. doi: 10.1104/pp.107.2.315
Delhaize, E., Ryan, P. R., and Randall, P. J. (1993). Aluminum tolerance in wheat (Triticum aestivum L.) II. Aluminum-stimulated excretion of malic acid from root apices. Plant Physiol. 103, 695–702. doi: 10.1104/pp.103.3.695
Diagne, N., Ngom, M., Djighaly, P. I., Fall, D., Hocher, V., and Svistoonoff, S. (2020). Roles of arbuscular mycorrhizal fungi on plant growth and performance: importance in biotic and abiotic stressed regulation. Diversity 12:370. doi: 10.3390/d12100370
Ding, Y., Liu, Y., Liu, S., Li, Z., Tan, X., Huang, X., et al. (2016). Biochar to improve soil fertility. A review. Agron. Sustain. Dev. 36:36. doi: 10.1007/s13593-016-0372-z
Dinkelaker, B., Römheld, V., and Marschner, H. (1989). Citric acid excretion and precipitation of calcium citrate in the rhizosphere of white lupin (Lupinus albus L.). Plant Cell Environ. 12, 285–292. doi: 10.1111/j.1365-3040.1989.tb01942.x
Dissanayaka, D. M. S. B., Ghahremani, M., Siebers, M., Wasaki, J., and Plaxton, W. C. (2020). Recent insights into the metabolic adaptations of phosphorus-deprived plants. J. Exp. Bot. 2020:eraa482. doi: 10.1093/jxb/eraa482
Dissanayaka, D. M. S. B., Plaxton, W. C., Lambers, H., Siebers, M., Marambe, B., and Wasaki, J. (2018). Molecular mechanisms underpinning phosphorus-use efficiency in rice. Plant Cell Environ. 41, 1483–1496. doi: 10.1111/pce.13191
Drew, E. A., Murray, R. S., Smith, S. E., and Jakobsen, I. (2003). Beyond the rhizosphere: growth and function of arbuscular mycorrhizal external hyphae in sands of varying pore sizes. Plant Soil 251, 105–114. doi: 10.1023/A:1022932414788
El Mazlouzi, M., Morel, C., Chesseron, C., Robert, T., and Mollier, A. (2020). Contribution of external and internal phosphorus sources to grain P loading in durum wheat (Triticum durum L.) grown under contrasting P levels. Front. Plant Sci. 11:870. doi: 10.3389/fpls.2020.00870
El-Tarabily, K. A., Nassar, A. H., and Sivasithamparam, K. (2008). Promotion of growth of bean (Phaseolus vulgaris L.) in a calcareous soil by a phosphate-solubilizing, rhizosphere-competent isolate of Micromonospora endolithica. Appl. Soil Ecol. 39, 161–171. doi: 10.1016/j.apsoil.2007.12.005
Fageria, N. K., Carvalho, M. C. S., and dos Santos, F. C. (2014). Root growth of upland rice genotypes as influenced by nitrogen fertilization. J. Plant Nutr. 37, 95–106. doi: 10.1080/01904167.2013.849731
Fageria, N. K., Moraes, O. P., and Vasconcelos, M. J. (2013). Upland rice genotypes evaluaiton for phoshphorus efficiency. J. Plant Nutr. 36, 1868–1880. doi: 10.1080/01904167.2013.818153
Fan, C., Wang, X., Hu, R., Wang, Y., Xiao, C., Jiang, Y., et al. (2013). The pattern of phosphate transporter 1 genes evolutionary divergence in Glycine max L. BMC Plant Biol. 13:48. doi: 10.1186/1471-2229-13-48
Fan, X., Che, X., Lai, W., Wang, S., Hu, W., Chen, H., et al. (2020). The auxin-inducible phosphate transporter AsPT5 mediates phosphate transport and is indispensable for arbuscule formation in Chinese milk vetch at moderately high phosphate supply. Environ. Microbiol. 22, 2053–2079. doi: 10.1111/1462-2920.14952
Floyd, B. E., Morriss, S. C., MacIntosh, G. C., and Bassham, D. C. (2015). Evidence for autophagy-dependent pathways of rRNA turnover in Arabidopsis. Autophagy 11, 2199–2212. doi: 10.1080/15548627.2015.1106664
Fujita, K., Kai, Y., Takayanagi, M., El-Shemy, H., Adu-Gyamfi, J. J., and Mohapatra, P. K. (2004). Genotypic variability of pigeonpea in distribution of photosynthetic carbon at low phosphorus level. Plant Sci. 166, 641–649. doi: 10.1016/j.plantsci.2003.10.032
Gamuyao, R., Chin, J. H., Pariasca-Tanaka, J., Pesaresi, P., Catausan, S., Dalid, C., et al. (2012). The protein kinase Pstol1 from traditional rice confers tolerance of phosphorus deficiency. Nature 488, 535–539. doi: 10.1038/nature11346
Gao, W., Lu, L., Qiu, W., Wang, C., and Shou, H. (2017). OsPAP26 encodes a major purple acid phosphatase and regulates phosphate remobilization in rice. Plant Cell Physiol. 58, 885–892. doi: 10.1093/pcp/pcx041
Gaude, N., Nakamura, Y., Scheible, W.-R., Ohta, H., and Dörmann, P. (2008). Phospholipase C5 (NPC5) is involved in galactolipid accumulation during phosphate limitation in leaves of Arabidopsis. Plant J. 56, 28–39. doi: 10.1111/j.1365-313X.2008.03582.x
George, T. S., and Richardson, A. E. (2008). “Potential and limitations to improving crops for enhanced phosphorus utilization,” in The Ecophysiology of Plant-Phosphorus Interactions, eds P. J. White and J. P. Hammond (Dordrecht: Springer Netherlands), 247–270. doi: 10.1007/978-1-4020-8435-5_11
Ghahremani, M., Park, J., Anderson, E. M., Marty-Howard, N. J., Mullen, R. T., and Plaxton, W. C. (2019). Lectin AtGAL1 interacts with high-mannose glycoform of the purple acid phosphatase AtPAP26 secreted by phosphate-starved Arabidopsis. Plant Cell Environ. 42, 1158–1166. doi: 10.1111/pce.13463
Gilroy, S., and Jones, D. L. (2000). Through form to function: root hair development and nutrient uptake. Trends Plant Sci. 5, 56–60. doi: 10.1016/S1360-1385(99)01551-4
Giri, J., Bhosale, R., Huang, G., Pandey, B. K., Parker, H., Zappala, S., et al. (2018). Rice auxin influx carrier OsAUX1 facilitates root hair elongation in response to low external phosphate. Nat. Commun. 9:1408. doi: 10.1038/s41467-018-03850-4
Glaser, B., and Lehr, V.-I. (2019). Biochar effects on phosphorus availability in agricultural soils: a meta-analysis. Sci. Rep. 9:9338. doi: 10.1038/s41598-019-45693-z
Glassop, D., Smith, S. E., and Smith, F. W. (2005). Cereal phosphate transporters associated with the mycorrhizal pathway of phosphate uptake into roots. Planta 222, 688–698. doi: 10.1007/s00425-005-0015-0
Gómez-Ariza, J., Balestrini, R., Novero, M., and Bonfante, P. (2009). Cell-specific gene expression of phosphate transporters in mycorrhizal tomato roots. Biol. Fertil. Soils 45, 845–853. doi: 10.1007/s00374-009-0399-2
González, E., Solano, R., Rubio, V., Leyva, A., and Paz-Ares, J. (2005). Pphosphate transporter traffic facilitator1 is a plant-specific SEC12-related protein that enables the endoplasmic reticulum exit of a high-affinity phosphate transporter in Arabidopsis. Plant Cell 17, 3500–3512. doi: 10.1105/tpc.105.036640
González-Muñoz, E., Avendaño-Vázquez, A.-O., Montes, R. A. C., de Folter, S., Andrés-Hernández, L., Abreu-Goodger, C., et al. (2015). The maize (Zea mays ssp. mays var. B73) genome encodes 33 members of the purple acid phosphatase family. Front. Plant Sci. 6:341. doi: 10.3389/fpls.2015.00341
Grand, S., and Lavkulich, L. M. (2013). Potential influence of poorly crystalline minerals on soil chemistry in Podzols of southwestern Canada. Eur. J. Soil Sci. 64, 651–660. doi: 10.1111/ejss.12062
Grant, C. A., and Flaten, D. N. (2019). 4R management of phosphorus fertilizer in the Northern Great Plains. J. Environ. Qual. 48, 1356–1369. doi: 10.2134/jeq2019.02.0061
Gregory, P (2006). Roots: Growth, Activity and Interactions With Soils. Wiley-Blackwell Press: Hoboken, NJ, USA
Greiner, R., and Egli, I. (2003). Determination of the activity of acidic phytate-degrading enzymes in cereal seeds. J. Agricult. Food Chem. 51, 847–850. doi: 10.1021/jf0204405
Grierson, C. S., Parker, J. S., and Kemp, A. C. (2001). Arabidopsis genes with roles in root hair development. J. Plant Nutr. Soil Sci. 164, 131–140. doi: 10.1002/1522-2624(200104)164:2<131::AID-JPLN131>3.0.CO;2-2
Guo, B., Jin, Y., Wussler, C., Blancaflor, E. B., Motes, C. M., and Versaw, W. K. (2008). Functional analysis of the Arabidopsis PHT4 family of intracellular phosphate transporters. New Phytologist 177, 889–898. doi: 10.1111/j.1469-8137.2007.02331.x
Haran, S., Logendra, S., Seskar, M., Bratanova, M., and Raskin, I. (2000). Characterization of arabidopsis acid phosphatase promoter and regulation of acid phosphatase expression. Plant Physiol. 124, 615–626. doi: 10.1104/pp.124.2.615
Harrison, M. J (1997). The arbuscular mycorrhizal symbiosis: an underground association. Trends Plant Sci. 2, 54–60. doi: 10.1016/S1360-1385(97)82563-0
Harrison, M. J., Dewbre, G. R., and Liu, J. (2002). A phosphate transporter from medicago truncatula involved in the acquisition of phosphate released by arbuscular mycorrhizal fungi. Plant Cell 14, 2413–2429. doi: 10.1105/tpc.004861
Härtel, H., Dörmann, P., and Benning, C. (2000). DGD1-independent biosynthesis of extraplastidic galactolipids after phosphate deprivation in Arabidopsis. Proc. Natl. Acad. Sci. U. S. A. 97, 10649–10654. doi: 10.1073/pnas.180320497
Härtel, H., Essigmann, B., Lokstein, H., Hoffmann-Benning, S., Peters-Kottig, M., and Benning, C. (1998). The phospholipid-deficient pho1 mutant of Arabidopsis thaliana is affected in the organization, but not in the light acclimation, of the thylakoid membrane. Biochimica et Biophysica Acta 1415, 205–218. doi: 10.1016/S0005-2736(98)00197-7
Harvey, P. R., Warren, R. A., and Wakelin, S. (2009). Potential to improve root access to phosphorus: the role of non-symbiotic microbial inoculants in the rhizosphere. Crop Pasture Sci. 60, 144–151. doi: 10.1071/CP08084
Hassler, S., Jung, B., Lemke, L., Novák, O., Strnad, M., Martinoia, E., et al. (2016). Function of the Golgi-located phosphate transporter PHT4;6 is critical for senescence-associated processes in Arabidopsis. J. Exp. Bot. 67, 4671–4684. doi: 10.1093/jxb/erw249
He, Z. H., Cheeseman, I., He, D., and Kohorn, B. D. (1999). A cluster of five cell wall-associated receptor kinase genes, Wak1-5, are expressed in specific organs of Arabidopsis. Plant Mol. Biol. 39, 1189–1196. doi: 10.1023/A:1006197318246
Hillwig, M. S., Contento, A. L., Meyer, A., Ebany, D., Bassham, D. C., and MacIntosh, G. C. (2011). RNS2, a conserved member of the RNase T2 family, is necessary for ribosomal RNA decay in plants. Proc. Natl. Acad. Sci. U. S. A. 108, 1093–1098. doi: 10.1073/pnas.1009809108
Hinsinger, P (2001). Bioavailability of soil inorganic P in the rhizosphere as affected by root-induced chemical changes: a review. Plant Soil 237, 173–195. doi: 10.1023/A:1013351617532
Ho, M. D., Rosas, J. C., Brown, K. M., and Lynch, J. P. (2005). Root architectural tradeoffs for water and phosphorus acquisition. Funct. Plant Biol. 32, 737–748. doi: 10.1071/FP05043
Hong, J. J., Park, Y.-S., Bravo, A., Bhattarai, K. K., Daniels, D. A., and Harrison, M. J. (2012). Diversity of morphology and function in arbuscular mycorrhizal symbioses in Brachypodium distachyon. Planta 236, 851–865. doi: 10.1007/s00425-012-1677-z
Hong, Y., and Lu, S. (2014). “Phospholipases in plant response to nitrogen and phosphorus availability,” in Phospholipases in Plant Signaling, ed X. Wang (Berlin; Heidelberg: Springer Berlin Heidelberg), 159–180. doi: 10.1007/978-3-642-42011-5_9
Huang, H., Kawamata, T., Horie, T., Tsugawa, H., Nakayama, Y., Ohsumi, Y., et al. (2015). Bulk RNA degradation by nitrogen starvation-induced autophagy in yeast. EMBO J. 34, 154–168. doi: 10.15252/embj.201489083
Huang, T.-K., Han, C.-L., Lin, S.-I., Chen, Y.-J., Tsai, Y.-C., Chen, Y.-R., et al. (2013). Identification of downstream components of ubiquitin-conjugating enzyme PHOSPHATE2 by quantitative membrane proteomics in Arabidopsis roots. Plant Cell 25, 4044–4060. doi: 10.1105/tpc.113.115998
Hufnagel, B., de Sousa, S. M., Assis, L., Guimaraes, C. T., Leiser, W., Azevedo, G. C., et al. (2014). Duplicate and conquer: multiple homologs of phosphorus-starvation tolerance1 enhance phosphorus acquisition and sorghum performance on low-phosphorus soils. Plant Physiol. 166, 659–677. doi: 10.1104/pp.114.243949
Hurley, B. A., Tran, H. T., Marty, N. J., Park, J., Snedden, W. A., Mullen, R. T., et al. (2010). The dual-targeted purple acid phosphatase isozyme AtPAP26 is essential for efficient acclimation of Arabidopsis to nutritional phosphate deprivation. Plant Physiol. 153, 1112–1122. doi: 10.1104/pp.110.153270
Hürlimann, H. C., Stadler-Waibel, M., Werner, T. P., and Freimoser, F. M. (2007). Pho91 is a vacuolar phosphate transporter that regulates phosphate and polyphosphate metabolism in Saccharomyces cerevisiae. Mol. Biol. Cell 18, 4438–4445. doi: 10.1091/mbc.e07-05-0457
Jeong, K., Baten, A., Waters, D. L. E., Pantoja, O., Julia, C. C., Wissuwa, M., et al. (2017). Phosphorus remobilization from rice flag leaves during grain filling: an RNA-seq study. Plant Biotechnol. J. 15, 15–26. doi: 10.1111/pbi.12586
Jia, H., Ren, H., Gu, M., Zhao, J., Sun, S., Zhang, X., et al. (2011). The phosphate transporter gene OsPht1;8 is involved in phosphate homeostasis in rice. Plant Physiol. 156, 1164–1175. doi: 10.1104/pp.111.175240
Jones, J. B (2001). Laboratory Guide for Conducting Soil Tests and Plant Analysis. Boca Raton, FL: CRC Press. doi: 10.1201/9781420025293
Joseph, S., Cowie, A. L., Van Zwieten, L., Bolan, N., Budai, A., Buss, W., et al. (2021). How biochar works, and when it doesn't: a review of mechanisms controlling soil and plant responses to biochar. GCB Bioenergy 13, 1731–1764. doi: 10.1111/gcbb.12885
Jungk, A (2001). Root hairs and the acquisition of plant nutrients from soil. J. Plant Nutr. Soil Sci. 164, 121–129. doi: 10.1002/1522-2624(200104)164:2<121::AID-JPLN121>3.0.CO;2-6
Kai, M., Takazumi, K., Adachi, H., Wasaki, J., Shinano, T., and Osaki, M. (2002). Cloning and characterization of four phosphate transporter cDNAs in tobacco. Plant Sci. 163, 837–846. doi: 10.1016/S0168-9452(02)00233-9
Kang, J., Hesterberg, D., and Osmond, D. L. (2009). Soil organic matter effects on phosphorus sorption: a path analysis. Soil Sci. Soc. Am. J. 73, 360–366. doi: 10.2136/sssaj2008.0113
Kanno, S., Arrighi, J.-F., Chiarenza, S., Bayle, V., Berthomé, R., Péret, B., et al. (2016). A novel role for the root cap in phosphate uptake and homeostasis. eLife 5:e14577. doi: 10.7554/eLife.14577
Karlsson, P. M., Herdean, A., Adolfsson, L., Beebo, A., Nziengui, H., Irigoyen, S., et al. (2015). The Arabidopsis thylakoid transporter PHT4;1 influences phosphate availability for ATP synthesis and plant growth. Plant J. 84, 99–110. doi: 10.1111/tpj.12962
Kaur, R., Singh, K., and Singh, J. (2013). A root-specific wall-associated kinase gene, HvWAK1, regulates root growth and is highly divergent in barley and other cereals. Funct. Integr. Genom. 13, 167–177. doi: 10.1007/s10142-013-0310-y
Kedir, A. J., Nyiraneza, J., Galagedara, L., Cheema, M., Hawboldt, K. A., McKenzie, D. B., et al. (2021). Phosphorus adsorption characteristics in forested and managed podzolic soils. Soil Sci. Soc. Ame. J. 85, 249–262. doi: 10.1002/saj2.20180
Ketterings, Q. M., and Flock, M. (2005). Comparison of Bray-1 and Mehlich-3 tests in high phosphorus soils. Soil Sci. 170, 212–219. doi: 10.1097/00010694-200503000-00007
Khan, M. S., Zaidi, A., Ahemad, M., Oves, M., and Wani, P. A. (2010). Plant growth promotion by phosphate solubilizing fungi – current perspective. Archiv. Agron. Soil Sci. 56, 73–98. doi: 10.1080/03650340902806469
Kraft, C., Deplazes, A., Sohrmann, M., and Peter, M. (2008). Mature ribosomes are selectively degraded upon starvation by an autophagy pathway requiring the Ubp3p/Bre5p ubiquitin protease. Nat. Cell Biol. 10, 602–610. doi: 10.1038/ncb1723
Krettek, A., and Rennert, T. (2021). Mobilisation of Al, Fe, and DOM from topsoil during simulated early Podzol development and subsequent DOM adsorption on model minerals. Sci. Rep. 11:19741. doi: 10.1038/s41598-021-99365-y
Kwabiah, A. B (2005). Growth, maturity, and yield responses of silage maize (Zea mays L.) to hybrid, planting date and plastic mulch. J. New Seeds 7, 37–59. doi: 10.1300/J153v07n02_03
Kwabiah, A. B., MacPherson, M., and McKenzie, D. B. (2003). Corn heat unit variability and potential of corn (Zea mays L.) production in a cool climate ecosystem. Can. J. Plant Sci. 83, 689–698. doi: 10.4141/P02-127
Lambers, H., Bishop, J. G., Hopper, S. D., Laliberté, E., and Zúñiga-Feest, A. (2012a). Phosphorus-mobilization ecosystem engineering: the roles of cluster roots and carboxylate exudation in young P-limited ecosystems. Ann. Bot. 110, 329–348. doi: 10.1093/aob/mcs130
Lambers, H., Cawthray, G. R., Giavalisco, P., Kuo, J., Laliberté, E, Pearse, S. J., et al. (2012b). Proteaceae from severely phosphorus-impoverished soils extensively replace phospholipids with galactolipids and sulfolipids during leaf development to achieve a high photosynthetic phosphorus-use-efficiency. New Phytologist 196, 1098–1108. doi: 10.1111/j.1469-8137.2012.04285.x
Lambers, H., Shane, M. W., Cramer, M. D., Pearse, S. J., and Veneklaas, E. J. (2006). Root structure and functioning for efficient acquisition of phosphorus: matching morphological and physiological traits. Ann. Bot. 98, 693–713. doi: 10.1093/aob/mcl114
Lapis-Gaza, H. R., Jost, R., and Finnegan, P. M. (2014). Arabidopsis Phosphate transporter1 genes PHT1;8 and PHT1;9 are involved in root-to-shoot translocation of orthophosphate. BMC Plant Biol. 14:334. doi: 10.1186/s12870-014-0334-z
Larsen, P. B., Degenhardt, J., Tai, C.-Y., Stenzler, L. M., Howell, S. H., and Kochian, L. V. (1998). Aluminum-resistant Arabidopsis mutants that exhibit altered patterns of aluminum accumulation and organic acid release from roots1. Plant Physiol. 117, 9–17. doi: 10.1104/pp.117.1.9
Leggewie, G., Willmitzer, L., and Riesmeier, J. W. (1997). Two cDNAs from potato are able to complement a phosphate uptake-deficient yeast mutant: identification of phosphate transporters from higher plants. Plant Cell 9, 381–392. doi: 10.1105/tpc.9.3.381
Li, C., Li, C., Zhang, H., Liao, H., and Wang, X. (2017). The purple acid phosphatase GmPAP21 enhances internal phosphorus utilization and possibly plays a role in symbiosis with rhizobia in soybean. Physiol. Plant. 159, 215–227. doi: 10.1111/ppl.12524
Li, W.-Y. F., Shao, G., and Lam, H.-M. (2008). Ectopic expression of GmPAP3 alleviates oxidative damage caused by salinity and osmotic stresses. New Phytologist 178, 80–91. doi: 10.1111/j.1469-8137.2007.02356.x
Li, X., Zeng, R., and Liao, H. (2016). Improving crop nutrient efficiency through root architecture modifications. J. Integr. Plant Biol. 58, 193–202. doi: 10.1111/jipb.12434
Li, X.-L., Marschner, H., and George, E. (1991). Acquisition of phosphorus and copper by VA-mycorrhizal hyphae and root-to-shoot transport in white clover. Plant Soil 136, 49–57. doi: 10.1007/BF02465219
Lin, D.-L., Yao, H.-Y., Jia, L.-H., Tan, J.-F., Xu, Z.-H., Zheng, W.-M., et al. (2020). Phospholipase D-derived phosphatidic acid promotes root hair development under phosphorus deficiency by suppressing vacuolar degradation of PIN-FORMED2. New Phytologist 226, 142–155. doi: 10.1111/nph.16330
Lin, W.-Y., Huang, T.-K., and Chiou, T.-J. (2013). Nitorgen limitation adaptation, a target of MicroRNA827, mediates degradation of plasma membrane–localized phosphate transporters to maintain phosphate homeostasis in Arabidopsis. Plant Cell 25, 4061–4074. doi: 10.1105/tpc.113.116012
Liu, C., Muchhal, U. S., Uthappa, M., Kononowicz, A. K., and Raghothama, K. G. (1998). Tomato phosphate transporter genes are differentially regulated in plant tissues by phosphorus1. Plant Physiol. 116, 91–99. doi: 10.1104/pp.116.1.91
Liu, F., Xu, Y., Jiang, H., Jiang, C., Du, Y., Gong, C., et al. (2016a). Systematic identification, evolution and expression analysis of the Zea mays PHT1 gene family reveals several new members involved in root colonization by arbuscular mycorrhizal fungi. Int. J. Mol. Sci. 17:930. doi: 10.3390/ijms17060930
Liu, J., Versaw, W. K., Pumplin, N., Gomez, S. K., Blaylock, L. A., and Harrison, M. J. (2008). Closely related members of the medicago truncatula pht1 phosphate transporter gene family encode phosphate transporters with distinct biochemical activities. J. Biol. Chem. 283, 24673–24681. doi: 10.1074/jbc.M802695200
Liu, J., Yang, L., Luan, M., Wang, Y., Zhang, C., Zhang, B., et al. (2015). A vacuolar phosphate transporter essential for phosphate homeostasis in Arabidopsis. Proc. Natl. Acad. Sci. U. S. A. 112, E6571–E6578. doi: 10.1073/pnas.1514598112
Liu, T.-Y., Huang, T.-K., Tseng, C.-Y., Lai, Y.-S., Lin, S.-I., Lin, W.-Y., et al. (2012). PHO2-dependent degradation of PHO1 modulates phosphate homeostasis in arabidopsis. Plant Cell 24, 2168–2183. doi: 10.1105/tpc.112.096636
Liu, T.-Y., Huang, T.-K., Yang, S.-Y., Hong, Y.-T., Huang, S.-M., Wang, F.-N., et al. (2016b). Identification of plant vacuolar transporters mediating phosphate storage. Nat. Commun. 7:11095. doi: 10.1038/ncomms11095
López-Arredondo, D. L., Leyva-González, M. A., González-Morales, S. I., López-Bucio, J., and Herrera-Estrella, L. (2014). Phosphate nutrition: improving low-phosphate tolerance in crops. Ann. Rev. Plant Biol. 65, 95–123. doi: 10.1146/annurev-arplant-050213-035949
López-Bucio, J., de la Vega, O. M., Guevara-García, A., and Herrera-Estrella, L. (2000). Enhanced phosphorus uptake in transgenic tobacco plants that overproduce citrate. Nat. Biotechnol. 18, 450–453. doi: 10.1038/74531
Lott, J. N., Greenwood, J. S., and Batten, G. D. (1995). “Mechanisms and regulation of mineral nutrient storage during seed development,” in Seed Development and Germination, eds J. Kigel and G. Gad (London: Routledge), 215–235. doi: 10.1201/9780203740071-9
Lu, C., and Tian, H. (2017). Global nitrogen and phosphorus fertilizer use for agriculture production in the past half century: shifted hot spots and nutrient imbalance. Earth Syst. Sci. Data 9, 181–192. doi: 10.5194/essd-9-181-2017
Luan, M., and Lan, W. (2019). Escape routes for vacuolar phosphate. Nat. Plants 5, 9–10. doi: 10.1038/s41477-018-0343-2
Lynch, J (1995). Root architecture and plant productivity. Plant Physiol. 109, 7–13. doi: 10.1104/pp.109.1.7
Lynch, J (1998). The role of nutrient-efficient crops in modern agriculture. J. Crop Prod. 1, 241–264. doi: 10.1300/J144v01n02_10
Lynch, J. P (2007). Roots of the second green revolution. Austral. J. Bot. 55, 493–512. doi: 10.1071/BT06118
Lynch, J. P (2011). Root phenes for enhanced soil exploration and phosphorus acquisition: tools for future crops. Plant Physiol. 156, 1041–1049. doi: 10.1104/pp.111.175414
Lynch, J. P., and Brown, K. M. (2001). Topsoil foraging – an architectural adaptation of plants to low phosphorus availability. Plant Soil 237, 225–237. doi: 10.1023/A:1013324727040
Ma, B., Zhang, L., Gao, Q., Wang, J., Li, X., Wang, H., et al. (2021). A plasma membrane transporter coordinates phosphate reallocation and grain filling in cereals. Nat. Genet. 53, 906–915. doi: 10.1038/s41588-021-00855-6
Ma, Z., Bielenberg, D. G., Brown, K. M., and Lynch, J. P. (2001). Regulation of root hair density by phosphorus availability in Arabidopsis thaliana. Plant Cell Environ. 24, 459–467. doi: 10.1046/j.1365-3040.2001.00695.x
Malhotra, H., Vandana, S. S., and Pandey, R. (2018). “Phosphorus nutrition: plant growth in response to deficiency and excess,” in Plant Nutrients and Abiotic Stress Tolerance, eds M. Hasanuzzaman, M. Fujita, H. Oku, K. Nahar, and B. Hawrylak-Nowak (Singapore: Springer Singapore), 171–190. doi: 10.1007/978-981-10-9044-8_7
Manske, G. G. B., Ortiz-Monasterio, J. I., van Ginkel, M., González, R. M., Fischer, R. A., Rajaram, S., et al. (2001). Importance of P uptake efficiency versus P utilization for wheat yield in acid and calcareous soils in Mexico. Eur. J. Agron. 14, 261–274. doi: 10.1016/S1161-0301(00)00099-X
Marschner, H., Römheld, V., Horst, W. J., and Martin, P. (1986). Root-induced changes in the rhizosphere: importance for the mineral nutrition of plants. Zeitschrift für Pflanzenernährung und Bodenkunde 149, 441–456. doi: 10.1002/jpln.19861490408
Marschner, H., Treeby, M., and Römheld, V. (1989). Role of root-induced changes in the rhizosphere for iron acquisition in higher plants. Zeitschrift für Pflanzenernährung und Bodenkunde 152, 197–204. doi: 10.1002/jpln.19891520210
Massonneau, A., Langlade, N., Léon, S., Smutny, J., Vogt, E., Neumann, G., et al. (2001). Metabolic changes associated with cluster root development in white lupin (Lupinus albus L.): relationship between organic acid excretion, sucrose metabolism and energy status. Planta 213, 534–542. doi: 10.1007/s004250100529
Matsushima, R., Tang, L. Y., Zhang, L., Yamada, H., Twell, D., and Sakamoto, W. (2011). A Conserved, Mg2+-dependent exonuclease degrades organelle DNA during Arabidopsis pollen development. Plant Cell 23, 1608–1624. doi: 10.1105/tpc.111.084012
Mehlich, A (1984). Mehlich 3 soil test extractant: a modification of Mehlich 2 extractant. Commun. Soil Sci. Plant Anal. 15, 1409–1416. doi: 10.1080/00103628409367568
Menezes-Blackburn, D., Paredes, C., Zhang, H., Giles, C. D., Darch, T., Stutter, M., et al. (2016). Organic acids regulation of chemical–microbial phosphorus transformations in soils. Environ. Sci. Technol. 50, 11521–11531. doi: 10.1021/acs.est.6b03017
Mertens, E (1991). Pyrophosphate-dependent phosphofructokinase, an anaerobic glycolytic enzyme? FEBS Lett. 285, 1–5. doi: 10.1016/0014-5793(91)80711-B
Messiga, A. J., Ziadi, N., Jouany, C., Virkajärvi, P., Suomela, R., Sinaj, S., et al. (2015). Soil test phosphorus and cumulative phosphorus budgets in fertilized grassland. AMBIO 44, 252–262. doi: 10.1007/s13280-015-0628-x
Miguel, M. A., Postma, J. A., and Lynch, J. P. (2015). Phene synergism between root hair length and basal root growth angle for phosphorus acquisition. Plant Physiol. 167, 1430–1439. doi: 10.1104/pp.15.00145
Miguel, M. A., Widrig, A., Vieira, R. F., Brown, K. M., and Lynch, J. P. (2013). Basal root whorl number: a modulator of phosphorus acquisition in common bean (Phaseolus vulgaris). Ann. Bot. 112, 973–982. doi: 10.1093/aob/mct164
Mimura, T (1995). Physiological characteristics and regulation mechanisms of the H+ pumps in the plasma membrane and tonoplast of characean cells. J. Plant Res. 108, 249–256. doi: 10.1007/BF02344350
Misson, J., Thibaud, M.-C., Bechtold, N., Raghothama, K., and Nussaume, L. (2004). Transcriptional regulation and functional properties of Arabidopsis Pht1;4, a high affinity transporter contributing greatly to phosphate uptake in phosphate deprived plants. Plant Mol. Biol. 55, 727–741. doi: 10.1007/s11103-004-1965-5
Miyaji, T., Kuromori, T., Takeuchi, Y., Yamaji, N., Yokosho, K., Shimazawa, A., et al. (2015). AtPHT4;4 is a chloroplast-localized ascorbate transporter in Arabidopsis. Nat. Commun. 6:5928. doi: 10.1038/ncomms6928
Młodzińska, E., and Zboińska, M. (2016). Phosphate uptake and allocation – a closer look at Arabidopsis thaliana L. and Oryza sativa L. Front. Plant Sci. 7:1198. doi: 10.3389/fpls.2016.01198
Mollier, A., De Willigen, P., Heinen, M., Morel, C., Schneider, A., and Pellerin, S. (2008). A two-dimensional simulation model of phosphorus uptake including crop growth and P-response. Ecol. Model. 210, 453–464. doi: 10.1016/j.ecolmodel.2007.08.008
Mollier, A., and Pellerin, S. (1999). Maize root system growth and development as influenced by phosphorus deficiency. J. Exp. Bot. 50, 487–497. doi: 10.1093/jxb/50.333.487
Mora-Macías, J., Ojeda-Rivera, J. O., Gutiérrez-Alanís, D., Yong-Villalobos, L., Oropeza-Aburto, A., Raya-González, J., et al. (2017). Malate-dependent Fe accumulation is a critical checkpoint in the root developmental response to low phosphate. Proc. Natl. Acad. Sci. U. S. A. 114, E3563–E3572. doi: 10.1073/pnas.1701952114
Mori, A., Fukuda, T., Vejchasarn, P., Nestler, J., Pariasca-Tanaka, J., and Wissuwa, M. (2016). The role of root size versus root efficiency in phosphorus acquisition in rice. J. Exp. Bot. 67, 1179–1189. doi: 10.1093/jxb/erv557
Muchhal, U. S., Pardo, J. M., and Raghothama, K. G. (1996). Phosphate transporters from the higher plant Arabidopsis thaliana. Proc. Natl. Acad. Sci. U. S. A. 93, 10519–10523. doi: 10.1073/pnas.93.19.10519
Mudge, S. R., Rae, A. L., Diatloff, E., and Smith, F. W. (2002). Expression analysis suggests novel roles for members of the Pht1 family of phosphate transporters in Arabidopsis. Plant J. 31, 341–353. doi: 10.1046/j.1365-313X.2002.01356.x
Nadeem, M., Cheema, M., Thomas, R., Ali, W., Ashiq, W., and Kavanagh, V. (2016). “Root exudate profile in five silage corn genotypes grown in western Newfoundland,” in Our Food Our Future Symposium. St. Johns, NL.
Nadeem, M., Mollier, A., Morel, C., Vives, A., Prud'homme, L., and Pellerin, S. (2011). Relative contribution of seed phosphorus reserves and exogenous phosphorus uptake to maize (Zea mays L.) nutrition during early growth stages. Plant Soil 346, 231–244. doi: 10.1007/s11104-011-0814-y
Nadeem, M., Mollier, A., Morel, C., Vives, A., Prud'homme, L., and Pellerin, S. (2012). Maize (Zea mays L.) endogenous seed phosphorus remobilization is not influenced by exogenous phosphorus availability during germination and early growth stages. Plant Soil 357, 13–24. doi: 10.1007/s11104-011-1111-5
Nafiu, A (2006). Soil-phosphorus extraction methodologies: a review. Afri. J. Agri. Res. 1, 159–161. doi: 10.5897/AJAR.9000645
Nagy, R., Karandashov, V., Chague, V., Kalinkevich, K., Tamasloukht, M. B., Xu, G., et al. (2005). The characterization of novel mycorrhiza-specific phosphate transporters from Lycopersicon esculentum and Solanum tuberosum uncovers functional redundancy in symbiotic phosphate transport in solanaceous species. Plant J. 42, 236–250. doi: 10.1111/j.1365-313X.2005.02364.x
Nagy, R., Vasconcelos, M. J. V., Zhao, S., McElver, J., Bruce, W., Amrhein, N., et al. (2006). Differential regulation of five Pht1 phosphate transporters from maize (Zea mays L.). Plant Biol. 8, 186–197. doi: 10.1055/s-2005-873052
Nakamura, Y., Awai, K., Masuda, T., Yoshioka, Y., Takamiya, K.-I., and Ohta, H. (2005). A novel phosphatidylcholine-hydrolyzing phospholipase C induced by phosphate starvation in Arabidopsis. J. Biol. Chem. 280, 7469–7476. doi: 10.1074/jbc.M408799200
Nasr Esfahani, M., Kusano, M., Nguyen, K. H., Watanabe, Y., Ha, C. V., Saito, K., et al. (2016). Adaptation of the symbiotic Mesorhizobium–chickpea relationship to phosphate deficiency relies on reprogramming of whole-plant metabolism. Proc. Natl. Acad. Sci. U. S. A. 113, E4610–E4619. doi: 10.1073/pnas.1609440113
Nedelciu, C. E., Ragnarsdottir, K. V., Schlyter, P., and Stjernquist, I. (2020). Global phosphorus supply chain dynamics: assessing regional impact to 2050. Glob. Food Secur. 26:100426. doi: 10.1016/j.gfs.2020.100426
Nesme, T., Metson, G. S., and Bennett, E. M. (2018). Global phosphorus flows through agricultural trade. Glob. Environ. Change 50, 133–141. doi: 10.1016/j.gloenvcha.2018.04.004
Neumann, G., and Martinoia, E. (2002). Cluster roots – an underground adaptation for survival in extreme environments. Trends Plant Sci. 7, 162–167. doi: 10.1016/S1360-1385(02)02241-0
Neumann, G., Massonneau, A., Langlade, N., Dinkelaker, B., Hengeler, C., Römheld, V., et al. (2000). Physiological aspects of cluster root function and development in phosphorus-deficient white lupin (Lupinus albus L.). Ann. Bot. 85, 909–919. doi: 10.1006/anbo.2000.1135
Niu, Y. F., Chai, R. S., Jin, G. L., Wang, H., Tang, C. X., and Zhang, Y. S. (2013). Responses of root architecture development to low phosphorus availability: a review. Ann. Bot. 112, 391–408. doi: 10.1093/aob/mcs285
Nussaume, L., Kanno, S., Javot, H., Marin, E., Nakanishi, T. M., and Thibaud, M.-C. (2011). Phosphate import in plants: focus on the PHT1 transporters. Front. Plant Sci. 2:83. doi: 10.3389/fpls.2011.00083
Okazaki, Y., Otsuki, H., Narisawa, T., Kobayashi, M., Sawai, S., Kamide, Y., et al. (2013). A new class of plant lipid is essential for protection against phosphorus depletion. Nat. Commun. 4:1510. doi: 10.1038/ncomms2512
Oldenburg, D. J., and Bendich, A. J. (2015). DNA maintenance in plastids and mitochondria of plants. Front. Plant Sci. 6:883. doi: 10.3389/fpls.2015.00883
Palma, D. A., Blumwald, E., and Plaxton, W. C. (2000). Upregulation of vacuolar H+-translocating pyrophosphatase by phosphate starvation of Brassica napus (rapeseed) suspension cell cultures. FEBS Lett. 486, 155–158. doi: 10.1016/S0014-5793(00)02266-3
Panigrahy, M., Rao, D. N., and Sarla, N. (2009). Molecular mechanisms in response to phosphate starvation in rice. Biotechnol. Adv. 27, 389–397. doi: 10.1016/j.biotechadv.2009.02.006
Pant, B. D., Musialak-Lange, M., Nuc, P., May, P., Buhtz, A., Kehr, J., et al. (2009). Identification of nutrient-responsive arabidopsis and rapeseed MicroRNAs by comprehensive real-time polymerase chain reaction profiling and small RNA sequencing. Plant Physiol. 150, 1541–1555. doi: 10.1104/pp.109.139139
Parentoni, S. N., and Souza Júnior, C. L. d. (2008). Phosphorus acquisition and internal utilization efficiency in tropical maize genotypes. Pesquisa Agropecuária Brasileira 43, 893–901. doi: 10.1590/S0100-204X2008000700014
Parker, J. S., Cavell, A. C., Dolan, L., Roberts, K., and Grierson, C. S. (2000). Genetic interactions during root hair morphogenesis in arabidopsis. Plant Cell 12, 1961–1974. doi: 10.1105/tpc.12.10.1961
Parry, G (2018). Low phosphate puts auxin in the root hairs. Trends Plant Sci. 23, 845–847. doi: 10.1016/j.tplants.2018.07.009
Paszkowski, U., Kroken, S., Roux, C., and Briggs, S. P. (2002). Rice phosphate transporters include an evolutionarily divergent gene specifically activated in arbuscular mycorrhizal symbiosis. Proc. Natl. Acad. Sci. U. S. A. 99, 13324–13329. doi: 10.1073/pnas.202474599
Paul, S., Luc, L., and William, H. (2011). Podzolic soils of Canada: genesis, distribution, and classification. Can. J. Soil Sci. 91, 843–880. doi: 10.4141/cjss10024
Peltovuori, T (2007). Sorption of phosphorus in field-moist and air-dried samples from four weakly developed cultivated soil profiles. Eur. J. Soil Sci. 58, 8–17. doi: 10.1111/j.1365-2389.2006.00789.x
Peng, M., Hannam, C., Gu, H., Bi, Y.-M., and Rothstein, S. J. (2007). A mutation in NLA, which encodes a RING-type ubiquitin ligase, disrupts the adaptability of Arabidopsis to nitrogen limitation. Plant J. 50, 320–337. doi: 10.1111/j.1365-313X.2007.03050.x
Pereira, N. C. M., Galindo, F. S., Gazola, R. P. D., Dupas, E., Rosa, P. A. L., Mortinho, E. S., et al. (2020). Corn yield and phosphorus use efficiency response to phosphorus rates associated with plant growth promoting bacteria. Front. Environ. Sci. 8:40. doi: 10.3389/fenvs.2020.00040
Péret, B., Clément, M., Nussaume, L., and Desnos, T. (2011). Root developmental adaptation to phosphate starvation: better safe than sorry. Trends Plant Sci. 16, 442–450. doi: 10.1016/j.tplants.2011.05.006
Péret, B., Desnos, T., Jost, R., Kanno, S., Berkowitz, O., and Nussaume, L. (2014). Root architecture responses: in search of phosphate. Plant Physiol. 166, 1713–1723. doi: 10.1104/pp.114.244541
Pierzynski, G. M., McDowell, R. W., and Thomas Sims, J. (2005). Chemistry, cycling, and potential movement of inorganic phosphorus in soils. Phosphorus 46, 51–86. doi: 10.2134/agronmonogr46.c3
Plassard, C., Becquer, A., and Garcia, K. (2019). Phosphorus transport in mycorrhiza: how far are we? Trends Plant Sci. 24, 794–801. doi: 10.1016/j.tplants.2019.06.004
Plaxton, W. C., and Podestá, F. E. (2006). The functional organization and control of plant respiration. Crit. Rev. Plant Sci. 25, 159–198. doi: 10.1080/07352680600563876
Plaxton, W. C., and Tran, H. T. (2011). Metabolic adaptations of phosphate-starved plants. Plant Physiol. 156, 1006–1015. doi: 10.1104/pp.111.175281
Poirier, Y., and Bucher, M. (2002). Phosphate transport and homeostasis in Arabidopsis. Arabidopsis 1:e0024. doi: 10.1199/tab.0024
Poirier, Y., Thoma, S., Somerville, C., and Schiefelbein, J. (1991). Mutant of Arabidopsis deficient in xylem loading of phosphate 1. Plant Physiol. 97, 1087–1093. doi: 10.1104/pp.97.3.1087
Preuss, C. P., Huang, C. Y., Gilliham, M., and Tyerman, S. D. (2010). Channel-like characteristics of the low-affinity barley phosphate transporter PHT1;6 when expressed in Xenopus oocytes. Plant Physiol. 152, 1431–1441. doi: 10.1104/pp.109.152009
Preuss, C. P., Huang, C. Y., and Tyreman, S. D. (2011). Proton-coupled high-affinity phosphate transport revealed from heterologous characterization in Xenopus of barley-root plasma membrane transporter, HvPHT1;1. Plant Cell Environ. 34, 681–689. doi: 10.1111/j.1365-3040.2010.02272.x
Pujol, V., and Wissuwa, M. (2018). Contrasting development of lysigenous aerenchyma in two rice genotypes under phosphorus deficiency. BMC Res. Not. 11:60. doi: 10.1186/s13104-018-3179-y
Qiang-Sheng, W., Guo-Huai, L., and Ying-Ning, Z. (2011). Improvement of root system architecture in peach (Prunus persica) seedlings by arbuscular mycorrhizal fungi, related to allocation of glucose/sucrose to root. Notulae Botanicae Horti Agrobotanici Cluj-Napoca 39, 232–236. doi: 10.15835/nbha3926232
Quintero, C. E., Boschetti, N. G., and Benavidez, R. A. (2003). Effect of soil buffer capacity on soil test phosphorus interpretation and fertilizer requirement. Commun. Soil Sci. Plant Anal. 34, 1435–1450. doi: 10.1081/CSS-120020455
Raboy, V (1997). “Accumulation and storage of phosphate and minerals,” in Cellular and Molecular Biology of Plant Seed Development, eds B. A. Larkins, I. K. Vasil (Dordrecht: Springer Netherlands), 441–477. doi: 10.1007/978-94-015-8909-3_12
Rae, A. L., Cybinski, D. H., Jarmey, J. M., and Smith, F. W. (2003). Characterization of two phosphate transporters from barley; evidence for diverse function and kinetic properties among members of the Pht1 family. Plant Mol. Biol. 53, 27–36. doi: 10.1023/B:PLAN.0000009259.75314.15
Raghothama, K (1999). Phosphate acquisition. Ann. Rev. Plant Biol. 50, 665–693. doi: 10.1146/annurev.arplant.50.1.665
Raghothama, K. G., and Karthikeyan, A. S. (2005). Phosphate acquisition. Plant Soil 274:37. doi: 10.1007/s11104-004-2005-6
Ramaekers, L., Remans, R., Rao, I. M., Blair, M. W., and Vanderleyden, J. (2010). Strategies for improving phosphorus acquisition efficiency of crop plants. Field Crops Res. 117, 169–176. doi: 10.1016/j.fcr.2010.03.001
Rausch, C., and Bucher, M. (2002). Molecular mechanisms of phosphate transport in plants. Planta 216, 23–37. doi: 10.1007/s00425-002-0921-3
Rausch, C., Daram, P., Brunner, S., Jansa, J., Laloi, M., Leggewie, G., et al. (2001). A phosphate transporter expressed in arbuscule-containing cells in potato. Nature 414, 462–465. doi: 10.1038/35106601
Reis, R. S., Deforges, J., Sokoloff, T., and Poirier, Y. (2020). Modulation of shoot phosphate level and growth by PHOSPHATE1 upstream open reading frame1. Plant Physiol. 183, 1145–1156. doi: 10.1104/pp.19.01549
Requejo, M. I., and Eichler-Löbermann, B. (2014). Organic and inorganic phosphorus forms in soil as affected by long-term application of organic amendments. Nutr. Cycl. Agroecosyst. 100, 245–255. doi: 10.1007/s10705-014-9642-9
Reymond, M., Svistoonoff, S., Loudet, O., Nussaume, L., and Desnos, T. (2006). Identification of QTL controlling root growth response to phosphate starvation in Arabidopsis thaliana. Plant Cell Environ. 29, 115–125. doi: 10.1111/j.1365-3040.2005.01405.x
Richardson, A. E., Lynch, J. P., Ryan, P. R., Delhaize, E., Smith, F. A., Smith, S. E., et al. (2011). Plant and microbial strategies to improve the phosphorus efficiency of agriculture. Plant Soil 349, 121–156. doi: 10.1007/s11104-011-0950-4
Richardson, A. E., and Simpson, R. J. (2011). Soil microorganisms mediating phosphorus availability update on microbial phosphorus. Plant Physiol. 156, 989–996. doi: 10.1104/pp.111.175448
Robinson, W. D., Carson, I., Ying, S., Ellis, K., and Plaxton, W. C. (2012). Eliminating the purple acid phosphatase AtPAP26 in Arabidopsis thaliana delays leaf senescence and impairs phosphorus remobilization. New Phytologist 196, 1024–1029. doi: 10.1111/nph.12006
Ros, M. B. H., Koopmans, G. F., van Groenigen, K. J., Abalos, D., Oenema, O., Vos, H. M. J., et al. (2020). Towards optimal use of phosphorus fertiliser. Sci. Rep. 10:17804. doi: 10.1038/s41598-020-74736-z
Rose, T. J., and Wissuwa, M. (2012). “Chapter five - rethinking internal phosphorus utilization efficiency: a new approach is needed to improve PUE in grain crops,” in Advances in Agronomy, ed D. L. Sparks (Cambridge, MA: Academic Press), 185–217. doi: 10.1016/B978-0-12-394277-7.00005-1
Ryan, M. H., and Graham, J. H. (2002). Is there a role for arbuscular mycorrhizal fungi in production agriculture? Plant Soil 244, 263–271. doi: 10.1007/978-94-017-1284-2_26
Ryan, P. R., Delhaize, E., and Jones, D. L. (2001). Function and mechanims of organic anion exudation from plant roots. Ann. Rev. Plant Physiol. Plant Mol. Biol. 52, 527–560. doi: 10.1146/annurev.arplant.52.1.527
Sanderson, K. R., and Sanderson, J. B. (2006). Prince Edward Island growers can reduce soil phosphorus buildup while maintaining carrot crop yield. Can. J. Plant Sci. 86, 1401–1403. doi: 10.4141/P06-109
Schünmann, P. H. D., Richardson, A. E., Smith, F. W., and Delhaize, E. (2004). Characterization of promoter expression patterns derived from the Pht1 phosphate transporter genes of barley (Hordeum vulgare L.). J. Exp. Bot. 55, 855–865. doi: 10.1093/jxb/erh103
Secco, D., Baumann, A., and Poirier, Y. (2010). Characterization of the rice PHO1 gene family reveals a key role for OsPHO1;2 in phosphate homeostasis and the evolution of a distinct clade in dicotyledons. Plant Physiol. 152, 1693–1704. doi: 10.1104/pp.109.149872
Secco, D., Wang, C., Shou, H., and Whelan, J. (2012). Phosphate homeostasis in the yeast Saccharomyces cerevisiae, the key role of the SPX domain-containing proteins. FEBS Lett. 586, 289–295. doi: 10.1016/j.febslet.2012.01.036
Seo, H.-M., Jung, Y., Song, S., Kim, Y., Kwon, T., Kim, D.-H., et al. (2008). Increased expression of OsPT1, a high-affinity phosphate transporter, enhances phosphate acquisition in rice. Biotechnol. Lett. 30, 1833–1838. doi: 10.1007/s10529-008-9757-7
Shane, M. W., Cawthray, G. R., Cramer, M. D., Kuo, J., and Lambers, H. (2006). Specialized 'dauciform' roots of Cyperaceae are structurally distinct, but functionally analogous with 'cluster' roots. Plant Cell Environ. 29, 1989–1999. doi: 10.1111/j.1365-3040.2006.01574.x
Shane, M. W., De Vos, M., De Roock, S., and Lambers, H. (2003). Shoot P status regulates cluster-root growth and citrate exudation in Lupinus albus grown with a divided root system. Plant Cell Environ. 26, 265–273. doi: 10.1046/j.1365-3040.2003.00957.x
Shen, J., Yuan, L., Zhang, J., Li, H., Bai, Z., Chen, X., et al. (2011). Phosphorus dynamics: from soil to plant. Plant Physiol. 156, 997–1005. doi: 10.1104/pp.111.175232
Shenoy, V. V., and Kalagudi, G. M. (2005). Enhancing plant phosphorus use efficiency for sustainable cropping. Biotechnol. Adv. 23, 501–513. doi: 10.1016/j.biotechadv.2005.01.004
Shin, H., Shin, H.-S., Dewbre, G. R., and Harrison, M. J. (2004). Phosphate transport in Arabidopsis: Pht1;1 and Pht1;4 play a major role in phosphate acquisition from both low- and high-phosphate environments. Plant J. 39, 629–642. doi: 10.1111/j.1365-313X.2004.02161.x
Singh, J., Zhang, S., Chen, C., Cooper, L., Bregitzer, P., Sturbaum, A., et al. (2006). High-frequency Ds remobilization over multiple generations in barley facilitates gene tagging in large genome cereals. Plant Mol. Biol. 62, 937–950. doi: 10.1007/s11103-006-9067-1
Singh, S., Tan, H. Q., and Singh, J. (2012). Mutagenesis of barley malting quality QTLs with Ds transposons. Funct. Integr. Genom. 12, 131–141. doi: 10.1007/s10142-011-0258-8
Singh, S. K., Fischer, U., Singh, M., Grebe, M., and Marchant, A. (2008). Insight into the early steps of root hair formation revealed by the procuste1 cellulose synthase mutant of Arabidopsis thaliana. BMC Plant Biol. 8:57. doi: 10.1186/1471-2229-8-57
Sisaphaithong, T., Kondo, D., Matsunaga, H., Kobae, Y., and Hata, S. (2012). Expression of plant genes for arbuscular mycorrhiza-inducible phosphate transporters and fungal vesicle formation in sorghum, barley, and wheat roots. Biosci. Biotechnol. Biochem. 76, 2364–2367. doi: 10.1271/bbb.120782
Smith, F. W (2001). Sulphur and phosphorus transport systems in plants. Plant Soil 232, 109–118. doi: 10.1023/A:1010390120820
Smith, F. W., Mudge, S. R., Rae, A. L., and Glassop, D. (2003). Phosphate transport in plants. Plant Soil 248, 71–83. doi: 10.1007/978-94-010-0243-1_6
Smith, S. E., Jakobsen, I., Grønlund, M., and Smith, F. A. (2011). Roles of arbuscular mycorrhizas in plant phosphorus nutrition: interactions between pathways of phosphorus uptake in arbuscular mycorrhizal roots have important implications for understanding and manipulating plant phosphorus acquisition. Plant Physiol. 156, 1050–1057. doi: 10.1104/pp.111.174581
Song, L., and Liu, D. (2015). Ethylene and plant responses to phosphate deficiency. Front. Plant Sci. 6, 796–796. doi: 10.3389/fpls.2015.00796
Spokas, K. A., Cantrell, K. B., Novak, J. M., Archer, D. W., Ippolito, J. A., Collins, H. P., et al. (2012). Biochar: a synthesis of its agronomic impact beyond carbon sequestration. J. Environ. Qual. 41, 973–989. doi: 10.2134/jeq2011.0069
Stigter, K. A., and Plaxton, W. C. (2015). Molecular mechanisms of phosphorus metabolism and transport during leaf senescence. Plants 4, 773–798. doi: 10.3390/plants4040773
Ström, L., Owen, A. G., Godbold, D. L., and Jones, D. L. (2002). Organic acid mediated P mobilization in the rhizosphere and uptake by maize roots. Soil Biol. Biochem. 34, 703–710. doi: 10.1016/S0038-0717(01)00235-8
Sun, S., Gu, M., Cao, Y., Huang, X., Zhang, X., Ai, P., et al. (2012). A constitutive expressed phosphate transporter, OsPht1;1, modulates phosphate uptake and translocation in phosphate-replete rice. Plant Physiol. 159, 1571–1581. doi: 10.1104/pp.112.196345
Svistoonoff, S., Creff, A., Reymond, M., Sigoillot-Claude, C., Ricaud, L., Blanchet, A., et al. (2007). Root tip contact with low-phosphate media reprograms plant root architecture. Nat. Genet. 39, 792–796. doi: 10.1038/ng2041
Takami, T., Ohnishi, N., Kurita, Y., Iwamura, S., Ohnishi, M., Kusaba, M., et al. (2018). Organelle DNA degradation contributes to the efficient use of phosphate in seed plants. Nat. Plants 4, 1044–1055. doi: 10.1038/s41477-018-0291-x
Tan, Z., Hu, Y., and Lin, Z. (2012). Expression of NtPT5 is correlated with the degree of colonization in tobacco roots inoculated with Glomus etunicatum. Plant Mol. Biol. Report. 30, 885–893. doi: 10.1007/s11105-011-0402-6
Taurian, T., Anzuay, M. S., Angelini, J. G., Tonelli, M. L., Ludueña, L., Pena, D., et al. (2010). Phosphate-solubilizing peanut associated bacteria: screening for plant growth-promoting activities. Plant Soil 329, 421–431. doi: 10.1007/s11104-009-0168-x
Tawaraya, K., Horie, R., Saito, A., Shinano, T., Wagatsuma, T., Saito, K., et al. (2013). Metabolite profilin of shoor extracts, root extracts, and root exudates of rice plant under phosphorus deficiency. J. Plant Nutr. 36, 1138–1159. doi: 10.1080/01904167.2013.780613
Teng, W., Deng, Y., Chen, X.-P., Xu, X.-F., Chen, R.-Y., Lv, Y., et al. (2013). Characterization of root response to phosphorus supply from morphology to gene analysis in field-grown wheat. J. Exp. Bot. 64, 1403–1411. doi: 10.1093/jxb/ert023
Teng, W., Zhao, Y.-Y., Zhao, X.-Q., He, X., Ma, W.-Y., Deng, Y., et al. (2017). Genome-wide identification, characterization, and expression analysis of PHT1 phosphate transporters in wheat. Front. Plant Sci. 8:543. doi: 10.3389/fpls.2017.00543
Theodorou, M., and Plaxton, W. (1994). Induction of PPi-dependent phosphofructokinase by phosphate starvation in seedlings of Brassica nigra. Plant Cell Environ. 17, 287–294. doi: 10.1111/j.1365-3040.1994.tb00294.x
Theodorou, M. E., Cornel, F. A., Duff, S. M., and Plaxton, W. C. (1992). Phosphate starvation-inducible synthesis of the alpha-subunit of the pyrophosphate-dependent phosphofructokinase in black mustard suspension cells. J. Biol. Chem. 267, 21901–21905. doi: 10.1016/S0021-9258(19)36697-9
Theodorou, M. E., and Plaxton, W. C. (1993). Metabolic adaptations of plant respiration to nutritional phosphate deprivation. Plant Physiol. 101, 339–344. doi: 10.1104/pp.101.2.339
Thompson, G (1995). The direct determination of phosphorus in citric acid soil extracts by colorimetry and direct-current plasma emission spectroscopy. South Afri. J. Plant Soil 12, 152–157. doi: 10.1080/02571862.1995.10634356
Tian, J., and Liao, H. (2018). The role of intracellular and secreted purple acid phosphatases in plant phosphorus scavenging and recycling. Ann. Plant Rev. 2018, 265–287. doi: 10.1002/9781119312994.apr0525
Ticconi, C. A., Lucero, R. D., Sakhonwasee, S., Adamson, A. W., Creff, A., Nussaume, L., et al. (2009). ER-resident proteins PDR2 and LPR1 mediate the developmental response of root meristems to phosphate availability. Proc. Natl. Acad. Sci. U. S. A. 106, 14174–14179. doi: 10.1073/pnas.0901778106
Tran, H. T., Hurley, B. A., and Plaxton, W. C. (2010). Feeding hungry plants: the role of purple acid phosphatases in phosphate nutrition. Plant Sci. 179, 14–27. doi: 10.1016/j.plantsci.2010.04.005
Tripathi, R. K., Aguirre, J. A., and Singh, J. (2021). Genome-wide analysis of wall associated kinase (WAK) gene family in barley. Genomics 113, 523–530. doi: 10.1016/j.ygeno.2020.09.045
Uhde-Stone, C., Zinn, K. E., Ramirez-Yogez, M., Li, A., Vance, C. P., and Allan, D. L. (2003). Nylon filter arrays reveal differential gene expression in proteoid roots of white lupin in response to phosphorus deficiency. Plant Physiol. 131, 1064–1079. doi: 10.1104/pp.102.016881
Unno, Y., Okubo, K., Wasaki, J., Shinano, T., and Osaki, M. (2005). Plant growth promotion abilities and microscale bacterial dynamics in the rhizosphere of Lupin analysed by phytate utilization ability. Environ. Microbiol. 7, 396–404. doi: 10.1111/j.1462-2920.2004.00701.x
van de Wiel, C. C. M., van der Linden, C. G., and Scholten, O. E. (2016). Improving phosphorus use efficiency in agriculture: opportunities for breeding. Euphytica 207, 1–22. doi: 10.1007/s10681-015-1572-3
Vance, C. P., Uhde-Stone, C., and Allan, D. L. (2003). Phosphorus acquisition and use: critical adaptations by plants for securing a nonrenewable resource. New Phytologist 157, 423–447. doi: 10.1046/j.1469-8137.2003.00695.x
Veljanovski, V., Vanderbeld, B., Knowles, V. L., Snedden, W. A., and Plaxton, W. C. (2006). Biochemical and molecular characterization of AtPAP26, a vacuolar purple acid phosphatase up-regulated in phosphate-deprived arabidopsis suspension cells and seedlings. Plant Physiol. 142, 1282–1293. doi: 10.1104/pp.106.087171
Veneklaas, E. J., Lambers, H., Bragg, J., Finnegan, P. M., Lovelock, C. E., Plaxton, W. C., et al. (2012). Opportunities for improving phosphorus-use efficiency in crop plants. New Phytologist 195, 306–320. doi: 10.1111/j.1469-8137.2012.04190.x
Versaw, W. K., and Harrison, M. J. (2002). A chloroplast phosphate transporter, PHT2;1, influences allocation of phosphate within the plant and phosphate-starvation responses. Plant Cell 14, 1751–1766. doi: 10.1105/tpc.002220
von Uexküll, H. R., and Mutert, E. (1995). Global extent, development and economic impact of acid soils. Plant Soil 171, 1–15. doi: 10.1007/BF00009558
Wakelin, S. A., Gupta, V. V., Harvey, P. R., and Ryder, M. H. (2007). The effect of Penicillium fungi on plant growth and phosphorus mobilization in neutral to alkaline soils from southern Australia. Can. J. Microbiol. 53, 106–115. doi: 10.1139/w06-109
Wang, F., Deng, M., Chen, J., He, Q., Jia, X., Guo, H., et al. (2020). CASEIN KINASE2-dependent phosphorylation of PHOSPHATE2 fine-tunes phosphate homeostasis in rice. Plant Physiol. 183, 250–262. doi: 10.1104/pp.20.00078
Wang, L., Liao, H., Yan, X., Zhuang, B., and Dong, Y. (2004a). Genetic variability for root hair traits as related to phosphorus status in soybean. Plant Soil 261, 77–84. doi: 10.1023/B:PLSO.0000035552.94249.6a
Wang, L., Lu, S., Zhang, Y., Li, Z., Du, X., and Liu, D. (2014). Comparative genetic analysis of Arabidopsis purple acid phosphatases AtPAP10, AtPAP12, and AtPAP26 provides new insights into their roles in plant adaptation to phosphate deprivation. J. Integr. Plant Biol. 56, 299–314. doi: 10.1111/jipb.12184
Wang, X., Devaiah, S. P., Zhang, W., and Welti, R. (2006). Signaling functions of phosphatidic acid. Progr. Lip. Res. 45, 250–278. doi: 10.1016/j.plipres.2006.01.005
Wang, X., Shen, J., and Liao, H. (2010). Acquisition or utilization, which is more critical for enhancing phosphorus efficiency in modern crops? Plant Sci. 179, 302–306. doi: 10.1016/j.plantsci.2010.06.007
Wang, Y., Chen, Y. F., and Wu, W. H. (2021). Potassium and phosphorus transport and signaling in plants. J. Integr. Plant Biol. 63, 34–52. doi: 10.1111/jipb.13053
Wang, Y., Ribot, C., Rezzonico, E., and Poirier, Y. (2004b). Structure and expression profile of the Arabidopsis PHO1 gene family indicates a broad role in inorganic phosphate homeostasis. Plant Physiol. 135, 400–411. doi: 10.1104/pp.103.037945
Wang, Y., Thorup-Kristensen, K., Jensen, L. S., and Magid, J. (2016). Vigorous root growth is a better indicator of early nutrient uptake than root hair traits in spring wheat grown under low fertility. Front. Plant Sci. 7:865. doi: 10.3389/fpls.2016.00865
Wang, Y. T., O'Halloran, I. P., Zhang, T. Q., Hu, Q. C., and Tan, C. S. (2015). Phosphorus sorption parameters of soils and their relationships with soil test phosphorus. Soil Sci. Soc. Am. J. 79, 672–680. doi: 10.2136/sssaj2014.07.0307
Wege, S., Khan, G. A., Jung, J.-Y., Vogiatzaki, E., Pradervand, S., Aller, I., et al. (2016). The EXS domain of PHO1 participates in the response of shoots to phosphate deficiency via a root-to-shoot signal. Plant Physiol. 170, 385–400. doi: 10.1104/pp.15.00975
Weihrauch, C., and Opp, C. (2018). Ecologically relevant phosphorus pools in soils and their dynamics: the story so far. Geoderma 325, 183–194. doi: 10.1016/j.geoderma.2018.02.047
White, P. J., Hammond, J. P., White, P. J., and Hammond, J. P. (2008). “Phosphorus nutrition of terrestrial plants,” in The Ecophysiology of Plant-Phosphorus Interactions, eds P. J. White and J. P. Hammond (Dordrecht: Springer Netherlands), 51–81. doi: 10.1007/978-1-4020-8435-5_4
White, P. J., and Veneklaas, E. J. (2012). Nature and nurture: the importance of seed phosphorus content. Plant Soil 357, 1–8. doi: 10.1007/s11104-012-1128-4
Wild, R., Gerasimaite, R., Jung, J.-Y., Truffault, V., Pavlovic, I., Schmidt, A., et al. (2016). Control of eukaryotic phosphate homeostasis by inositol polyphosphate sensor domains. Science 352, 986–990. doi: 10.1126/science.aad9858
Williamson, L. C., Ribrioux, S. P. C. P., Fitter, A. H., and Leyser, H. M. O. (2001). Phosphate availability regulates root system architecture in arabidopsis. Plant Physiol. 126, 875–882. doi: 10.1104/pp.126.2.875
Wissuwa, M (2003). How do plants achieve tolerance to phosphorus deficiency? Small causes with big effects. Plant Physiol. 133, 1947–1958. doi: 10.1104/pp.103.029306
Wu, Q.-S., Zou, Y.-N., He, X.-H., and Luo, P. (2011a). Arbuscular mycorrhizal fungi can alter some root characters and physiological status in trifoliate orange (Poncirus trifoliata L. Raf.) seedlings. Plant Growth Regul. 65, 273–278. doi: 10.1007/s10725-011-9598-6
Wu, Z., Zhao, J., Gao, R., Hu, G., Gai, J., Xu, G., et al. (2011b). Molecular cloning, characterization and expression analysis of two members of the Pht1 family of phosphate transporters in Glycine max. PLoS ONE 6:e19752. doi: 10.1371/journal.pone.0019752
Xie, X., Huang, W., Liu, F., Tang, N., Liu, Y., Lin, H., and Zhao, B. (2013). Functional analysis of the novel mycorrhiza-specific phosphate transporter AsPT1 and PHT1 family from Astragalus sinicus during the arbuscular mycorrhizal symbiosis. New Phytol. 198, 836–852. doi: 10.1111/nph.12188
Xu, L., Zhao, H., Wan, R., Liu, Y., Xu, Z., Tian, W., et al. (2019). Identification of vacuolar phosphate efflux transporters in land plants. Nat. Plants 5, 84–94. doi: 10.1038/s41477-018-0334-3
Yan, X., Liao, H., Beebe, S. E., Blair, M. W., and Lynch, J. P. (2004). QTL mapping of root hair and acid exudation traits and their relationship to phosphorus uptake in common bean. Plant Soil 265, 17–29. doi: 10.1007/s11104-005-0693-1
Yang, H., Knapp, J., Koirala, P., Rajagopal, D., Peer, W. A., Silbart, L. K., et al. (2007). Enhanced phosphorus nutrition in monocots and dicots over-expressing a phosphorus-responsive type I H+-pyrophosphatase. Plant Biotechnol. J. 5, 735–745. doi: 10.1111/j.1467-7652.2007.00281.x
Yang, S.-Y., Huang, T.-K., Kuo, H.-F., and Chiou, T.-J. (2017). Role of vacuoles in phosphorus storage and remobilization. J. Exp. Bot. 68, 3045–3055. doi: 10.1093/jxb/erw481
Yang, Z., Yang, J., Wang, Y., Wang, F., Mao, W., He, Q., et al. (2020). Protein phosphatase95 regulates phosphate homeostasis by affecting phosphate transporter trafficking in rice. Plant Cell 32, 740–757. doi: 10.1105/tpc.19.00685
Ye, Y., Yuan, J., Chang, X., Yang, M., Zhang, L., Lu, K., et al. (2015). The phosphate transporter gene OsPht1;4 is involved in phosphate homeostasis in rice. PLoS ONE 10:e0126186. doi: 10.1371/journal.pone.0126186
Zehetner, F., Wuenscher, R., Peticzka, R., and Unterfrauner, H. (2018). Correlation of extractable soil phosphorus (P) with plant P uptake: 14 extraction methods applied to 50 agricultural soils from Central Europe. Plant Soil Environ. 64, 192–201. doi: 10.17221/70/2018-PSE
Zhang, H., Huang, Y., Ye, X., Shi, L., and Xu, F. (2009). Genotypic differences in phosphorus acquisition and the rhizosphere properties of Brassica napus in response to low phosphorus stress. Plant Soil 320, 91–102. doi: 10.1007/s11104-008-9873-0
Zhang, Q., Wang, C., Tian, J., Li, K., and Shou, H. (2011). Identification of rice purple acid phosphatases related to posphate starvation signalling. Plant Biol. 13, 7–15. doi: 10.1111/j.1438-8677.2010.00346.x
Zhao, J., Fu, J., Liao, H., He, Y., Nian, H., Hu, Y., et al. (2004). Characterization of root architecture in an applied core collection for phosphorus efficiency of soybean germplasm. Chinese Sci. Bullet. 49, 1611–1620. doi: 10.1007/BF03184131
Zhu, W., Miao, Q., Sun, D., Yang, G., Wu, C., Huang, J., et al. (2012). The mitochondrial phosphate transporters modulate plant responses to salt stress via affecting ATP and gibberellin metabolism in Arabidopsis thaliana. PLoS ONE 7:e43530. doi: 10.1371/journal.pone.0043530
Zhu, X. -C., Song, F. -B., and Xu, H. -W. (2010). Arbuscular mycorrhizae improves low temperature stress in maize via alterations in host water status and photosynthesis. Plant Soil 331, 129–137. doi: 10.1007/s11104-009-0239-z
Ziadi, N., Bélanger, G., Gagnon, B., and Mongrain, D. (2009). Mehlich 3 soil phosphorus as determined by colorimetry and inductively coupled plasma. Commun. Soil Sci. Plant Anal. 40, 132–140. doi: 10.1080/00103620802649377
Keywords: acidic soils, nutrient use efficiency, podzol, P transporters, root architecture, seed P reserves, short growth seasons, sustainable agroecosystem
Citation: Nadeem M, Wu J, Ghaffari H, Kedir AJ, Saleem S, Mollier A, Singh J and Cheema M (2022) Understanding the Adaptive Mechanisms of Plants to Enhance Phosphorus Use Efficiency on Podzolic Soils in Boreal Agroecosystems. Front. Plant Sci. 13:804058. doi: 10.3389/fpls.2022.804058
Received: 28 October 2021; Accepted: 28 January 2022;
Published: 15 March 2022.
Edited by:
Anoop Kumar Srivastava, Indian Council of Agricultural Research (ICAR), IndiaReviewed by:
Sanjay Kumar Ray, National Bureau of Soil Survey and Land Use Planning (ICAR), IndiaIbrahim Ortas, Çukurova University, Turkey
Copyright © 2022 Nadeem, Wu, Ghaffari, Kedir, Saleem, Mollier, Singh and Cheema. This is an open-access article distributed under the terms of the Creative Commons Attribution License (CC BY). The use, distribution or reproduction in other forums is permitted, provided the original author(s) and the copyright owner(s) are credited and that the original publication in this journal is cited, in accordance with accepted academic practice. No use, distribution or reproduction is permitted which does not comply with these terms.
*Correspondence: Muhammad Nadeem, bW5hZGVlbUBncmVuZmVsbC5tdW4uY2E=; Mumtaz Cheema, bWNoZWVtYUBncmVuZmVsbC5tdW4uY2E=