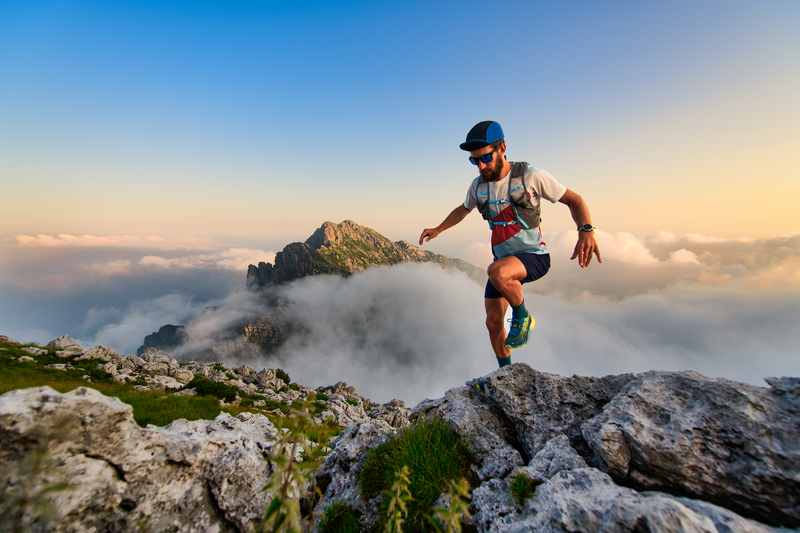
95% of researchers rate our articles as excellent or good
Learn more about the work of our research integrity team to safeguard the quality of each article we publish.
Find out more
REVIEW article
Front. Plant Sci. , 25 April 2022
Sec. Plant Nutrition
Volume 13 - 2022 | https://doi.org/10.3389/fpls.2022.802274
Magnesium (Mg) is an essential nutrient for a wide array of fundamental physiological and biochemical processes in plants. It largely involves chlorophyll synthesis, production, transportation, and utilization of photoassimilates, enzyme activation, and protein synthesis. As a multifaceted result of the introduction of high-yielding fertilizer-responsive cultivars, intensive cropping without replenishment of Mg, soil acidification, and exchangeable Mg (Ex-Mg) leaching, Mg has become a limiting nutrient for optimum crop production. However, little literature is available to better understand distinct responses of plants to Mg deficiency, the geographical distribution of soil Ex-Mg, and the degree of Mg deficiency. Here, we summarize the current state of knowledge of key plant responses to Mg availability and, as far as possible, highlight spatial Mg distribution and the magnitude of Mg deficiency in different cultivated regions of the world with a special focus on China. In particular, ~55% of arable lands in China are revealed Mg-deficient (< 120 mg kg−1 soil Ex-Mg), and Mg deficiency literally becomes increasingly severe from northern (227–488 mg kg−1) to southern (32–89 mg kg−1) China. Mg deficiency primarily traced back to higher depletion of soil Ex-Mg by fruits, vegetables, sugarcane, tubers, tea, and tobacco cultivated in tropical and subtropical climate zones. Further, each unit decline in soil pH from neutral reduced ~2-fold soil Ex-Mg. This article underscores the physiological importance of Mg, potential risks associated with Mg deficiency, and accordingly, to optimize fertilization strategies for higher crop productivity and better quality.
Since groundbreaking efforts in plant nutrition within the late nineteenth and twentieth centuries, complicated nutritional disorders and biological functions of most mineral nutrients are being systematically investigated. Research efforts have remained largely focused on crop yield- and quality-related, most frequently occurring limiting macro-elements such as nitrogen (N), phosphorus (P), and potassium (K). Nevertheless, the essentiality of other mineral nutrients, i.e., magnesium (Mg) has long been disregarded in terms of soil and plant tests and fertilization programs, and its deficiency is not regarded as a substantial concern in agriculture productivity. Consequently, Mg is called “a forgotten element in crop production” (Cakmak and Yazici, 2010). Indeed, Mg is an indispensable component in a large number of crucial physiological and biochemical processes throughout plant growth and development.
Mg is the fundamental component of chlorophyll (Chl) pigments in the light-capturing complex of chloroplasts and, hence, is involved in photosynthetic CO2 assimilation (Cakmak and Kirkby, 2008; Cakmak and Yazici, 2010; Gerendás and Führs, 2013). Nearly 15–35% of absorbed Mg by plants is fixed in Chl pigments, with the remaining (~65–85%) portion deposited in the vacuoles or utilized for protein synthesis and other related biological processes (Karley and White, 2009; Marschner, 2012). Mg also acts as a cofactor of numerous enzymes (more than 300) involved in Chl biosynthesis and photosynthetic CO2 fixation (Peng et al., 2015; Billard et al., 2016; Ma et al., 2016; Chen Z. C. et al., 2018). Various other enzymes such as ribulose-1,5-bisphosphate carboxylase/oxygenase (Rubisco), protein kinases, RNA polymerase, glutathione synthase, adenosine triphosphatases (ATPases), phosphatases, and carboxylases require Mg for activation (Maguire and Cowan, 2002; Shaul, 2002; Dann Iii et al., 2007; Marschner, 2012). Moreover, Mg participates in sucrose transport, energy metabolism, N utilization, pollen development and male fertility, stress tolerance, plant-microbe interactions, and other numerous biological processes (Li et al., 2008; Chen et al., 2009; Xu et al., 2015; Chen Z. C. et al., 2018; Li D. et al., 2020; Ishfaq et al., 2021; Tian et al., 2021).
Apart from plants, Mg is a key element for animal and human health. The suppression and rehabilitation of several human diseases, for instance, strokes, cardiovascular diseases, and diabetes involve the utilization of Mg (Al Alawi et al., 2018). Approximately two-thirds of adults in western countries do not consume satisfactory amounts of Mg, resulting in the nutritional disorder called “hypomagnesemia” that subsequently escalates to chronic diseases from its hidden deficiency (Hermans et al., 2013). The Mg-related nutritional disorders in humans are linked to declining Mg concentrations in the soil and food crops (Rosanoff, 2013; Rosanoff and Kumssa, 2020). Therefore, optimizing Mg nutrition is an imperative concern for food security and human health (Broadley and White, 2010).
The significance of Mg nutrition in crop production has long been overlooked and, consequently, its application seldom matches removal. Intensive Mg removal by high-yielding fertilizer-responsive cultivars and accelerated Mg leaching along with soil acidification continuously deplete indigenous exchangeable Mg reserves (Wang et al., 2020, 2022). Incidences of Mg deficiency (MGD) or approaching deficient levels is expanding in most production systems. As MGD is beginning to catch attention, still very limited literature is available to understand the biological functions and spatial distribution of Mg in distinct agroecosystems. Therefore, a better understanding of the physiological functions of Mg and the magnitude of MGD is vital to optimize future crop yield and quality.
Here, we compile research advances in Mg transport, physiology, and functions, and illustrate spatial patterning of MGD in diverse agricultural systems. In the first part of this study, plant morphological and related physiological responses to Mg availability are critically summarized. We also discussed the absorption and transport mechanism of Mg in view of the model plants Arabidopsis and rice. In the second part, spatial Mg distribution and magnitude of MGD in China's agricultural system are critically evaluated. There are also a few examples from other cultivated regions of the world, however, still scarce data is available to quantify them comprehensively. To our best knowledge, this is the first attempt of such kind that highlights geographical distribution and degree of MGD in distinct regions and even the provincial level in China. It can be beneficial to understand the overall biological functions of Mg, its nutritional status, and therefore, to optimize fertilization strategies as it highlights large spatial distribution variations. Thus, this review provides a unique reference for a wide range of scientific communities in plant sciences, and more critically draws attention toward potential threats associated with MGD for optimizing nutrient management to sustain agricultural productivity and food security.
Mg concentrations in the soil and plant are mediated by a wide range of intrinsic and extrinsic factors such as edaphic factors (soil type, pH, competing cations, moisture, aeration, etc.), environmental conditions (temperature, light, etc.), and anthropogenic influences (fertilization and other management practices). They also widely differ across plant species because of genotypic variations, heterogeneous growing conditions, growth stage, and age of analyzed tissues; hence, determining critical Mg levels is challenging (Gransee and Führs, 2013). For instance, different concentrations of Mg in leaves vary with temporal variations in Mg-deficient mulberry (Tewari et al., 2006), bean (Neuhaus et al., 2014), and barley (Tränkner et al., 2016) because Mg is a quite mobile element within the plant.
Concentrations of soil exchangeable Mg (Ex-Mg) fluctuate largely, and in most production systems more than 120 mg Ex-Mg kg−1 soil is generally considered sufficient for optimum crop yield (Metson and Gibson, 1977; Wang et al., 2020). In soil solution, values between 0.12 and 8.5 mM are categorized as satisfactory to support plant growth (Hariadi and Shabala, 2004; Karley and White, 2009; Marschner, 2012). Below this range, plant growth tends to be reduced, while overhead 8.5 mM can experience adverse influences (Hariadi and Shabala, 2004; Guo et al., 2016). In many plants, the optimum Mg concentration is 0.15–0.50% of leaf dry weight (Grzebisz, 2011; Marschner, 2012; Römheld, 2012); <0.1% is normally allied with chlorosis (Hermans and Verbruggen, 2005; Ding et al., 2006).
The leaf Mg concentration is found to be extremely species-dependent (Hauer-Jákli and Tränkner, 2019). The optimum Mg concentration in leaf dry weight falls in the range of 0.1 and 0.2% in rice, wheat, maize, barley, sorghum, and potato. While there is a usually higher demand of Mg for the dry matter formation in cotton, soybean, or peanut with the range between 0.2 and 0.3%, and even much higher 0.35% in the sunflower, alfalfa, and tomato. Based on dry matter formation, the optimum Mg concentration ranges 0.09–0.40% for woody plants, 0.07–0.21% for monocots, and 0.10–0.70% for dicots. Further, for optimum photosynthetic activity, woody species, monocots, and dicots require Mg concentrations between 0.10–0.5, 0.15–0.41, and 0.10–0.72%, respectively (Römheld, 2012; Hauer-Jákli and Tränkner, 2019).
The most obvious MGD symptoms generally appear on fully expanded mature (older) leaves and are critical tissues for MGD threshold setup. Nevertheless, interveinal chlorosis does not occur until a certain period of MGD latency and at that stage, it is literally difficult to attain full capacities of plant performance with Mg supplementation. Indeed, retarded root growth may serve as a reliable quicker indicator for MGD than chlorosis. Another much earlier and sensitive MGD indicator is elevated sugar concentrations in mature leaves which reduces photo-assimilate efflux and cumulatively reduces root growth (Hermans and Verbruggen, 2005; Koch et al., 2020). To better understand how plants respond to MGD, distinct plant responses regulated by Mg supply are summarized below.
Mg mediates various central physiological and biochemical processes, and in particular functions in the production, transportation, and utilization of photosynthates in plants. This section hierarchically summarizes comparative plant responses to Mg sufficiency and limitation (scheme depicted in Figure 1).
Figure 1. Scheme depicting distinct responses of plants to Mg availability. Plant response to MGD can be summarized as (1) sub-optimal photosynthetic CO2 assimilation; (2) impaired carbohydrate transportation from the source (fully expanded older leaves) to sinks (root, young growing points, or yield-forming organs); (3) photoassimilate (starch and sucrose) accumulation in source leaves; (4) reformed rounded and larger chloroplasts; (5) imbalanced light capture and utilization; (6) leakage of electrons from photosynthetic apparatus; (7) generation of reactive oxygen species (ROS); (8) oxidative stress; (9) degradation of chlorophyll (a & b) and reduced activities of Mg-depended enzymes, i.e., Rubisco; and (10) ultimately the appearance of interveinal chlorosis and/or necrotic spots on fully expanded (mature) leaves. Impaired phloem loading results in suppression of root growth, uptake of nutrients and water along with yield and quality traits of agricultural commodities.
The well-recognized morphological disorders associated with MGD can be categorized as (1) shrinkage of the root system, (2) appearance of interveinal chlorosis, and/or necrosis typically on fully expanded (mature) leaves and (3) reduction in the yield and quality of agricultural commodities.
One of the distinct and earliest responses of plants to MGD is the noticeable shrinkage of the root system and the smaller root-to-shoot dry weight (DW) ratio, as a result of restricted photosynthate transportation from source leaves to sink organs (Cakmak et al., 1994a; Cakmak and Kirkby, 2008; Farhat et al., 2016; Ishfaq et al., 2021; Jia et al., 2021). MGD from the initial growth stage remarkably suppresses dry matter production by 34% in barley (Tränkner et al., 2016) and 43% in tomato seedlings (Ishfaq et al., 2021), which when sufficiently supplied, Mg improves root biomass by 77% compared to a smaller increase in shoot biomass (59%) (Hauer-Jákli and Tränkner, 2019). Disrupted root and shoot growth, and resulting decreases in the root/shoot ratio under MGD has been widely investigated (Cakmak et al., 1994a; Ding et al., 2006; Tewari et al., 2006; Mengutay et al., 2013; da Silva et al., 2017; Chen C. T. et al., 2018; Ishfaq et al., 2021; Xu et al., 2021). MGD hampers energy-requiring steps (i.e., Mg-ATP) for carbohydrate translocation toward the root (Section Impaired Carbohydrate Loading and Outflow for detailed description), leading to over-accumulation of carbohydrates in Mg-deficient leaves and substantial reduction of sucrose transport toward roots (Hermans et al., 2005; Koch et al., 2020). Further, down-tuning of auxin accumulation and signaling in Mg-deficient root preconditions a smaller root system (Ishfaq et al., 2021). A smaller root system under MGD diminishes the root surface area for resource forage in the soil, which further reduces uptake of other nutrients and increases the risk of multiple environmental stresses (Cakmak and Kirkby, 2008). However, Mg is a quite mobile element within the plant and older leaves (mostly in vacuoles) serve as a pool for actively growing points with higher requirements for Mg (Xu et al., 2021). Plants cultivated with sufficient Mg during the initial growth stages absorb and store sufficient Mg to endure upcoming MGD up to a certain extent, with minor changes in root/shoot ratios even if Mg supply is abruptly reduced (Hermans and Verbruggen, 2005; Niu et al., 2014; Tränkner et al., 2016; Liu et al., 2018). In addition, source-sink distance influences the translocation efficiency of carbohydrates and dry matter distribution, thus the ultimate root/shoot ratio may vary across plant species reliant upon the proximity among the source (leaves) and sink organs (Hermans et al., 2005).
Mg acts as the fundamental atom of the Chl molecule; MGD leads to degradation of Chl pigments and subsequent interveinal chlorosis (Cakmak and Yazici, 2010; Hermans et al., 2013; Ye et al., 2019). Plants re-allocate Mg from older leaves toward actively growing younger leaves under MGD (White and Broadley, 2009; Taiz and Zeiger, 2010). Therefore, interveinal chlorosis usually first appears on older fully expanded (mature) leaves (Cakmak and Kirkby, 2008; White and Broadley, 2009; Gransee and Führs, 2013), and gradually encompasses young leaves as MGD becomes more severe (Billard et al., 2016; Li et al., 2017; Cai et al., 2019) (as shown in Figure 2).
Figure 2. The visual Mg deficiency symptoms on tomato seedling. Under low Mg supply, interveinal chlorosis usually first appears on older, fully expanded (mature) leaves, and gradually encompasses younger leaves as Mg deficiency becomes more severe. Where adequate and low Mg were applied as 1.0 mM and 0.02 mM Mg, respectively, in the nutrient solution. Other details on plant growth have been described elsewhere (Ishfaq et al., 2021).
Over-accumulation of photosynthates (starch and sucrose) in Mg-deficient leaves down-regulates the photosynthetic efficiency and utilization of fixed light energy (Sections Weakened Photosynthetic Activity and Impaired Carbohydrate Loading and Outflow for detailed description). The latter scenario reduces CO2 fixation and amplifies electron transmission to O2, resulting in over-yielding of reactive oxygen species (ROS) that injures the photosynthetic apparatus and accelerates leaf chlorosis or necrosis (Cakmak and Kirkby, 2008). Thus, Mg-deficient plants are fairly sensitive to the extent of light intensity. Their leaves swiftly display prominent MGD symptoms when exposed to high light-intensity (Mengutay et al., 2013). Partial shading efficiently suppresses such light-intensity-dependent response, even shading itself does not change Mg concentrations in different leaf parts. Induction of leaf chlorosis by the photooxidative stress under MGD signifies that plants have a higher physiological prerequisite for Mg under high-light intensity conditions (Cakmak and Kirkby, 2008). In addition, lower efficiencies of Mg-chelatase (e.g., low ATP accessibility under MGD) also trigger leaf chlorosis with protoporphyrin IX accumulation in leaves (Cakmak and Kirkby, 2008). MGD may further alter abscisic acid and ethylene signaling, redox status, and metal homeostasis, giving rise to leaf chlorosis and necrosis (Cakmak and Kirkby, 2008).
Mg serves as a crucial mineral nutrient for the generation and partitioning of carbohydrates and related biomass accumulation in most crop species (Cakmak and Kirkby, 2008; Chen Z. C. et al., 2018; Koch et al., 2019; Zhang et al., 2020). Sucrose also functions to mediate a wide array of developmental and growth processes as a signaling molecule to fine-tune organ differentiation and genesis for higher crop yield (Hackel et al., 2006; Chen Z. C. et al., 2018). Mg-regulated photosynthesis generates various carbohydrates providing carbon skeletons for the synthesis of a majority of quality components (Gerendás and Führs, 2013). MGD at least disrupts plant photosynthesis and metabolism, and thus affects a range of yield and quality traits of field crops (Gerendás and Führs, 2013; D'Egidio et al., 2019), for instance, cereals (Graeff et al., 2001; Grzebisz, 2013), horticultural crops (Morton et al., 2008; Yang et al., 2012), and forestry (Vacek et al., 2006). Hence, Mg fertilization improves the yield and quality of agricultural produce, and ultimately reduces the risk of rejection when the product is offered for sale (Gerendás and Führs, 2013; Wang et al., 2020; Zhang et al., 2020).
Crops that store a considerable amount of carbohydrates or oil in grain or tuber require adequate Mg supply to optimize their yield and quality traits (Grzebisz, 2013). For instance, sufficient Mg supply is required to ensure optimal yield and quality of wheat (Grzebisz, 2013; Ceylan et al., 2016), rapeseed (Bogdevich and Mishuk, 2006), and apple (von Bennewitz et al., 2011). In particular, Mg application improves dry matter and starch concentrations of potato (Koch et al., 2019), sugar concentrations of sugar beet (Poglodzinski et al., 2021), vitamin C, and protein concentrations in Chinese cabbage (Lu et al., 2021), grain yield and crude protein concentrations in lentil (Azizi et al., 2011), protein and oil contents in soybean (Vrataric et al., 2006), and yield and next-generation seed germination in a wax gourd (Zhang et al., 2020). Mg supply also improves polyphenol, catechin, and amino acid concentrations in black tea (Jayaganesh et al., 2011; Ruan et al., 2012). For grain crops, Mg contents relates to processing traits such as milling performance (Greffeuille et al., 2006; Gerendás and Führs, 2013). However, further field experiments are necessary to corroborate the broad beneficial effects of Mg on crop quality and to deepen our understanding of underlying mechanisms.
Notably, crop plants are exposed to interactive environmental stresses under field-grown conditions, and MGD exaggerates plant vulnerability under these multifactorial stresses and consequently reduces biomass formation and quality traits. Adequate Mg supply can alleviate harmful effects of abiotic stresses through antagonistic competition with cations (i.e., aluminum), enhancing anti-oxidant systems, and modulating protein activities and gene expression (Chen et al., 2012, 2017; Mengutay et al., 2013; Siddiqui et al., 2016; Boaretto et al., 2020; Kibria et al., 2020; Tian et al., 2021). According to a recent meta-analysis, Mg application improves crop yield and agronomic efficiency (AE) by ~8.5% and 34.4 kg kg−1, respectively, under diverse growing conditions (Wang et al., 2020). Thus, Mg nutrition definitely calls for more attention to ensure high yield and quality of agricultural commodities toward efficient resource utilization, sustainable agricultural development, and food security although, under certain extreme circumstances, Mg oversupply adversely affects crop development and quality (Kwon et al., 2019).
The aforementioned MGD-associated phenotypes are largely attributed to: (i) weakened photosynthetic activities, (ii) impaired source-sink carbohydrate allocation, (iii) reactive oxygen species (ROS) over-yielding and chlorophyll degradation, and (iv) disrupted energy metabolism and protein synthesis.
Mg is preferentially required for Chl structuring and functioning to capture solar energy (Verbruggen and Hermans, 2013), with 15–35% of total available Mg in chloroplasts (Cakmak and Yazici, 2010; Guo et al., 2016). Mg insufficiency negatively affects the expression of genes regulating Chl synthesis and functioning such as ChlG encoding Chl synthase, ChlH, and ChlI, respectively encoding Mg-chelatase subunits H and I, Cab2 encoding Chl a and b binding proteins, and PPMT encoding Mg-protoporphyrin IX methyltransferase, which ultimately diminishes photosynthetic efficiencies (Hermans and Verbruggen, 2005; Zhou et al., 2011; Neuhaus et al., 2013). In the chloroplast, Mg ion (Mg2+) drapes negative charges on the thylakoid membrane to initiate grana formation (Puthiyaveetil et al., 2017). Rubisco, the most abundant globular protein controlling plant photosynthesis, harvests CO2 in the initial step of the Calvin cycle, which requires Mg to bind to the carbamate group and residues Glu194 and Asp193 on the side chain of Rubisco for its activation (Portis, 2003; Hazra et al., 2015). Mg inadequacy reduces net CO2 assimilation rates by weakening Rubisco activities (Li J. et al., 2020; Tian et al., 2021); exogenous supplementation of MgSO4 can increase Rubisco abundance to optimal levels and improve photosynthesis efficiencies in Mg-deficient plants (Wolf et al., 2019). In C4 and CAM plants, MGD hinders substrate-binding of phosphoenolpyruvate carboxylase and reduces CO2 assimilation (Zhao et al., 2012). Other crucial enzymes for starch synthesis, i.e., ADP-glucose pyrophosphorylase also need Mg2+ as a cofactor (Stitt and Zeeman, 2012).
In summary, Mg-deficient leaves show sub-optimal photosynthetic CO2 assimilation mainly due to less abundant Chl, impaired critical enzymes, and over-accumulation of photosynthates in leaves (Laing et al., 2000; Hariadi and Shabala, 2004; Yang et al., 2019), which is well-exemplified in various plant species such as maize (Jezek et al., 2015), barley (Tränkner et al., 2016; Jaghdani et al., 2020), common bean (Canizella et al., 2015), sugar beet (Hermans et al., 2004), sunflower (Lasa et al., 2000; Tränkner and Jaghdani, 2019), radish (Samborska et al., 2018), spinach (Ze et al., 2009; Jaghdani et al., 2021), fruit trees (Tang et al., 2012; Yang et al., 2012; Peng et al., 2015; Boaretto et al., 2020), and pine seedlings (Laing et al., 2000; Sun et al., 2001) under diverse growth conditions with Mg limitation.
Phloem loading of carbohydrates involves H+-ATPase-mediated establishment of electrochemical gradients and following co-transport of H+-sucrose into the phloem. MGD impairs phloem loading by reducing H+-ATPase and Mg-ATP activities and the proton gradient, restricting the functioning of sucrose/H+ symporters (i.e., SUT1) that demand Mg-ATPase for H+-motive force, or affecting other yet to be fully understood key processes (Cakmak and Kirkby, 2008; Ayre, 2011). There is a nearly eight-fold reduction in the translocation of 14C-labeled sucrose in sugar beet after 11 days of the MGD treatment (Hermans et al., 2005); resupplying Mg for 12–24 h transiently restores the sucrose transport rate in Mg-deficient plants to the Mg-sufficient level (Cakmak et al., 1994b; Cakmak and Kirkby, 2008). Mg deficient plants over-accumulate sucrose as well as starch in source leaves (Cakmak et al., 1994b; Cakmak and Kirkby, 2008; Gransee and Führs, 2013; Tanoi and Kobayashi, 2015; Koch et al., 2020; Zhang et al., 2020), compared to over-accumulation of starch in N or P deficient leaves and that of sucrose under K deficiency (Cakmak and Kirkby, 2008; Verbruggen and Hermans, 2013; Wakeel and Ishfaq, 2022a). Elevated accumulation of photosynthates in source leaves is observed in plants such as rice (Ding et al., 2006; Cai et al., 2012), Arabidopsis (Hermans and Verbruggen, 2005; Hermans et al., 2006), and citrus (Yang et al., 2012) under MGD. This typical observation appears within days, prior to visible leaf chlorosis, for more timely diagnosis in the field (Hermans et al., 2005; Cakmak and Kirkby, 2008; Yang et al., 2019).
Mg deficiency hampers photosynthetic electron transport and over-accumulates unutilized energy, and carbohydrate stacking partially decouples light capture and utilization, which together adversely boosts ROS production (Cakmak and Kirkby, 2008; Tanoi and Kobayashi, 2015). ROS scavenging over-consumes ascorbic acid and SH-containing compounds, with involvement of glutathione reductase, superoxide dismutase, ascorbate peroxidase, and catalase, disrupting cellular redox homeostasis, and endangering various physiological processes (Cakmak and Kirkby, 2008; Chou et al., 2011; Yang et al., 2012; Peng et al., 2015). With Mg application, ROS accumulation in Mg-deficient plants declines by 31% compared to control plants (Hauer-Jákli and Tränkner, 2019).
Over-produced ROS in Mg-deficient leaves stimulates expression of STAY-GREEN (OsSGR) to trigger Chl degradation (Peng et al., 2019), and sequentially reduces the concentration of Chl b and a (Mittler, 2002; Hermans et al., 2004; Mengutay et al., 2013; Tränkner et al., 2016). Alternatively, over-accumulation of starch in thylakoids of Mg-deficient plants makes chloroplasts rounder and larger (Farhat et al., 2014); more sugar deposition down-regulates the expression of Cab2 which is involved in encoding Chl pigments (Hermans et al., 2004). ROS also damages the D1 protein in the PSII reaction center and reduces Rubisco abundance in the leaves (Nakano et al., 2006; Zhou et al., 2006; Takahashi and Badger, 2011; Järvi et al., 2013).
ROS generated in the chloroplast thylakoid need to be instantly detoxified before they diffuse to adjacent compartments (Asada, 2006). MGD thus increase antioxidant activities by related enzymes and metabolites in wheat (Mengutay et al., 2013), maize (Tewari et al., 2004), Mulberry (Tewari et al., 2006), and citrus (Tang et al., 2012) in response to ROS oxidation, particularly when exposed to intensive light (Cakmak and Kirkby, 2008). The concentration of antioxidative can be considered as a biochemical indicator to estimate Mg levels in the leaves (Riga et al., 2005).
Mg plays a crucial role in energy metabolism. Briefly, light stimulates proton flux into thylakoid space within chloroplasts, which is compensated by Mg2+ transfer from thylakoid lumen into the stroma (Shaul, 2002). This bi-directional ion movement is required for the establishment of the cross-membrane electrochemical gradient for adenosine triphosphate (ATP) synthesis (photophosphorylation). In ATP, Mg attaches to two negatively charged phosphoryl groups along with two N bases of adenine, thus enabling binding of Mg-ATP to the hydrophobic cleft of the reactive site of ATP demanding enzymes (Marschner, 2012). Nearly 50% of total cellular Mg2+ is bound to ATP (Maguire and Cowan, 2002), therefore, Mg2+ supply directly affects ATP synthesis and provision (Shabala and Hariadi, 2005).
Mg is also required to assemble ribosomal subunits and maintain protein abundance (Yamamoto et al., 2010; Hazra et al., 2015; Chen Z. C. et al., 2018). A single functional ribosomal unit recruits more than 100 Mg2+ (Petrov et al., 2012). Mg2+ usually attaches with multiple rRNA phosphate groups, equilibrates electrostatic repulsion, and fixates the tertiary structure of 23S rRNA to promote aggregation of ribosomal subunits (Klein et al., 2004). Maize root hairs significantly down-regulate the abundance of most proteins under MGD (Li et al., 2013), indicating that MGD negatively regulates protein synthesis, as confirmed in maize, wheat (Mengutay et al., 2013), and sugar beet (Faust and Schubert, 2016). In leaves, Rubisco accounts for 30% of the total protein (Jensen, 2000) and nearly 40% of stromal proteins (Hazra et al., 2015). Rubisco concentrations decrease under MGD in maize (Jezek et al., 2015), barley (Tränkner et al., 2016), sunflower (Lasa et al., 2000), spinach (Yuguan et al., 2009), citrus (Peng et al., 2015; Li et al., 2017), and Pinus radiata (Laing et al., 2000; Sun et al., 2001). Further, proteomic analysis shows considerably reduced activities of Rubisco and its activase (Rca) under MGD in Citrus sinensis (Peng et al., 2015).
In well-aerated, virtually neutral pH soils, Mg uptake by plant roots largely depends on passive transport along mass flow driven by the transpiration pull and active influx against the electrochemical gradient across the membrane established by pumping protons out of the root cytoplasm (Mayland and Wilkinson, 1989; Marschner, 2012). Hence, the total amount of Mg2+ available to plants is primarily determined by Mg2+ concentrations in the soil solution, soil pH, the cation exchange capacity of the soil, and the soil water content (Kobayashi et al., 2013; Jezek et al., 2015; Wang et al., 2020). Mg2+ has a smaller ionic radius (0.072 nm) and larger hydrated radius (0.428 nm) (Bose et al., 2011), more easily subject to leaching compared to other cations. The uptake rate of Mg2+ in a pH 4.5 solution reduces to half compared to in the pH 6.5 solution (Kobayashi et al., 2013). The Mg transporters (MGT/MRS2) per se are generic and permit other cations to pass. Competing cations, for instance, K+, Ca2+, , and/or Na+ generally antagonize Mg uptake (Gransee and Führs, 2013; Xie et al., 2021; Garcia et al., 2022; Wakeel and Ishfaq, 2022b).
A second-level modulator of Mg availability to plants is Mg transporters. CorA, identified during the screening for cobalt (Co) resistant bacterium strains, is the initial Mg transporter mediating the influx of Mg, Co, and nickel into S. typhimurium (Hmiel et al., 1986). CorA functionally substitutes for mitochondrial RNA splicing 2 (MRS2) in regulating Mg transport and homeostasis in yeast (Bui et al., 1999; Gregan et al., 2001). Each CorA monomer has two transmembrane helices that only permit divalent cations to move through (Lunin et al., 2006). Homologous to CorA, MRS2, and MGT encode a 10-member Mg transporter family (referred as the MGT/MRS2 family hereinafter) in Arabidopsis (Schock et al., 2000; Li et al., 2001), after pioneering work in functional characterization of the Mg2+/H+ exchanger (MHX) in Mg transport in higher plant Arabidopsis (Shaul et al., 1999). Phylogenetic analysis suggested that MGT/MRS2 family constitutes a three-clade tree in planta with two rounds of asymmetric duplications (Ishfaq et al., 2021). The MGT/MRS2 family members function in plants as Mg translocators for root absorption, root-shoot distribution, organelle allocation, and homeostasis (Li et al., 2001, 2016; Marschner, 2012; Saito et al., 2013; Ishfaq et al., 2021). Mg transporters/exchangers in model plants Arabidopsis and rice are depicted in Figure 3.
Figure 3. Mg uptake, transport, and homeostasis in model plants Arabidopsis and rice. Mg2+ absorption from the soil by plants is affected by a range of intrinsic and extrinsic factors. In soil, Mg2+ is susceptible to leaching due to its larger hydrated and smaller ionic radius. Once the Mg2+ reached the root surface, plants acquire it via CorA/MRS2/MGT, unspecific K-transporters, and MHX; using such transporters/exchanger, Mg2+ enters into root epidermis, cortex, and then to the xylem after passing through the Casparian strip. The symplastic route depicts Mg movement from cell to cell, whereas, apoplastic refers to movement through extracellular spaces. Followed by, it transfers to shoot and leaves through xylem and stores in vacuole via specific transporters/exchanger. MGT/MRS2, Magnesium Transporters; MHX, Mg2+/H+ Exchanger; MRS, Mitochondrial RNA Splicing; HATS, High-Affinity Transport System; LATS, Low-Affinity Transport System; Dual-ATS, Dual-Affinity Transport System.
Six MGT/MRS2 members (MGT1/MRS2-10, MGT2/MRS2-1, MGT3/MRS2-5, MGT4/MRS2-3, MGT7/MRS2-7, and MGT9/MRS2-2) may be involved in root uptake and within-plant translocation of Mg2+ according to their varying expression patterns in Arabidopsis (Gebert et al., 2009). MGT1/MRS2-10 overexpression enhances Nicotiana benthamiana tolerance to MGD (Deng et al., 2006), and knockout of MGT1/MRS2-10 and MGT2/MRS2-1 makes Arabidopsis plants more sensitive to Mg limitation (Lenz et al., 2013). Tonoplast-localized MGT2/MRS2-1 and MGT3/MRS2-5 control Mg2+ transport into mesophyll vacuoles in Arabidopsis (Conn et al., 2011) for osmotic potential adjustment (up to 80 mM Mg2+ stored in vacuoles) (Shaul, 2002; Conn et al., 2011). MGT3/MRS2-5 also partially modulates diel Mg2+ fluctuations for optimal Rubisco and photosynthesis activities in rice (Li J. et al., 2020). AtMGT6/AtMRS2-4, a dual-affinity transporter, regulates Mg uptake by roots under Mg limitation and plays an essential role in maintaining cellular homeostasis of Mg2+ (Mao et al., 2014; Oda et al., 2016; Yan et al., 2018). Mutation of MGT7/MRS2-7 severely perturbs Arabidopsis growth under low Mg (Gebert et al., 2009). Functions of another chloroplast Mg transporter MGT9/MRS2-2 remain to be identified (Saito et al., 2013). In addition, AtCNGC10 participates in long-distance transportation of Mg2+ (Guo K. M. et al., 2010). Further efforts are required to better understand the diverse functions of MGT/MRS2 transporters and underlying regulatory mechanisms for Mg absorption and allocation in plants.
As noted in the preceding part, Mg limitation disrupts many fundamental physiological and biochemical processes in plants. Efficient MGD remedy roadmaps for optimum crop production depend on a clear understanding of the distribution pattern and magnitude of MGD in different cultivated regions of the world. For instance, South China has large areas of acidic soils with greater MGD risks due to low pH and intense leaching. Yield-oriented agricultural production maintains a high level of Mg removal from the soil without proper Mg replenishment. With further crop yield increase (projected to ~50% in 2030) (Zhang et al., 2011; Cui et al., 2014) to feed a 1.4 billion population, MGD is becoming a serious limiting factor in crop production in China, which calls for a better understanding of Ex-Mg concentrations in the soil and Mg fertilization strategies across diverse soil, crop, and ecological zones. Here, we provide a focused overview of MGD in China according to the available data to guide balanced fertilization and close yield gaps among different cultivated regions of the country.
The data used in this part of the review regarding soil Ex-Mg (0–20 cm depth, NH4OAc-extractable Mg) in distinct croplands of China were extracted from available published articles (field experiments) in English as well as Chinese journals. Soil Ex-Mg in different provinces was also obtained from the website of “National Earth System Science Data Center, National Science and Technology Infrastructure of China” (http://www.geodata.cn), and the recent annual report of “International Magnesium Institute (IMI)” China (http://www.magnesiuminstitute.org/) (details in Supplementary Files 1, 2). The soil database (n = 2,544) was compiled from five distinct regions covering 24 provinces (provincial-level administrative divisions) in China. The information about soil pH and climate of different provinces was adapted from previous reports (Han et al., 2011; Li et al., 2015; Chen S. et al., 2018). The country-wise database concerning soil Ex-Mg was assembled from the Food and Agricultural Organization (FAO) of the United Nations (http://www.fao.org/3/a-at167e.pdf).
Global Ex-Mg distribution in croplands varies dramatically depending on soil and climate types and land utilization practices. With regard to the overall abundance of soil Ex-Mg, China ranked 16th among 25 data-available countries. Many countries such as Mexico, Ethiopia, Italy, India, New Zealand, Pakistan, and Thailand had higher Ex-Mg concentrations in the soil in spite of some other less abundant countries, i.e., Brazil, Malawi, Finland, and Sri Lanka as compared to China (Figures 4A,B).
Figure 4. Geographical distribution of soil Ex-Mg in agricultural systems. (A) Global soil Ex-Mg status for data available countries, (B) Global ranking of soil Ex-Mg for data available countries (value for China is highlighted by the dark green bar), (C) Frequency per cent of soil Ex-Mg in China's farming systems. Gray color in map indicated data not found.
To better interpret the collected data, soil Ex-Mg is categorized into four groups as severely deficient (<30 mg kg−1), deficient (31–60 mg kg−1), moderate (61–120 mg kg−1), and relatively sufficient (>120 mg kg−1). Our categorization is well in agreement with previous rating standards (Metson and Brooks, 1975; Metson and Gibson, 1977). Surprisingly, ~55% of croplands in China were Mg-deficient including 7% severely deficient, ~27% deficient, and 21% moderate, and the remaining was Mg sufficient for plant growth (Figure 4C). On the broader perspective, concentrations of soil Ex-Mg generally decreased from northern China to southern China (Figures 5A,B). The mean soil Ex-Mg in China is ~133 mg kg−1 with a range of 227–488 mg kg−1 (331 mg kg−1 on average) in Northeast China, 251–357 mg kg−1 (275 mg kg−1 on average) in the north-central region, 54–418 mg kg−1 (214 mg kg−1 on average) in the middle and lower reaches of the Yangtze River, 49–233 mg kg−1 (133 mg kg−1 on average) southwest, and 32–89 mg kg−1 soil (65 mg kg−1 on average) in South China (Figure 5A, Supplementary File 1).
Figure 5. Spatial distribution and magnitude of Mg deficiency in China's farming system. (A) Spatial distribution of soil Ex-Mg in distinct regions of China, (B) Provincial-level soil Ex-Mg distribution in agricultural soils of China. In China, ~55% of arable lands are Mg-deficient (Ex-Mg < 120 mg kg−1), with an obvious spatially declining trend from northern (331 mg kg−1 on average) toward southern (65 mg kg−1 on average) China. Gray color in maps indicated data not found.
At the provincial-level, Hainan, Guangxi, Guangdong, Fujian, and Zhejiang in South China, Hunan and Jiangxi in the middle and lower reaches of the Yangtze River, and Guizhou in the southwest were found deficient in soil Ex-Mg. In particular, Hainan and Guangxi showed the lowest values 32 and 35 mg kg−1, respectively. Chongqing (~127 mg kg−1) and Sichuan (~133 mg kg−1) approached to the sufficient level, while Shanghai, Jiangsu, Anhui, Shandong, Henan, Shanxi, Beijing, Liaoning, Jilin, and Heilongjiang were categorized sufficient in soil Ex-Mg. The highest provincial concentration was reported as 488 mg kg−1 in Heilongjiang. Soil Ex-Mg data were not well-reported in northwestern areas such as Inner Mongolia, Shaanxi, Gansu, Qinghai, and Xinjiang (Figure 5B, Supplementary File 1).
MGD primarily traced back to Ex-Mg depletion in the soil. Long-term soil mining as a result of intensive cropping accelerates Mg removal. For instance, intensive cultivation of fruits, vegetables, sugarcane, tubers, tea, and tobacco largely contributed to the depletion of soil Ex-Mg (Figure 6A). The amount of Ex-Mg released from parental minerals likely becomes insufficient to support optimum crop production, especially in sandy soils. Chinese farmers usually do not apply Mg fertilizers to replenish the soil, mostly relying on N, P, and K fertilizers (Liu et al., 2003; Zhang and Zhang, 2008; Guo J. H. et al., 2010; Wang et al., 2022). The data presented in Figure 6B shows that MGD becomes increasingly severe in acidic soils and each unit decrease in soil pH from neutral reduced ~two-fold soil Ex-Mg. In South China, acidic soils promoted Mg2+ solubilization, and dissolved Mg2+ is frequently subject to strong leaching associated with precipitation and irrigation (Guo J. H. et al., 2010; Zhu et al., 2018; Zhang et al., 2021). N over-application further lowers soil pH, exaggerating the MGD situation in certain areas. Climatic conditions also affect Mg dynamics in the soil–plant system. The availability of soil Ex-Mg in different climatic zones of China is found in the following order: temperate > plateau and mountain > subtropical > tropical (Figure 6C). Finally, abundant competing cations such as high concentrations of Ca2+ in calcareous soils, , H+, and Al3+ in acidic soils, or Na+ in saline soils, counteracted Mg2+ to reduce its availability to crops (Mengel et al., 2001; Gransee and Führs, 2013).
Figure 6. Soil Ex-Mg variations with plant type, soil pH, and climate in China's agricultural system. (A) Fruits, vegetables, sugarcane, tubers, tea, and tobacco contribute higher in the depletion of soil Ex-Mg. Mg deficiency becomes increasingly severe (B) in acidic soils, and (C) in tropical and subtropical climate zones of China.
With continuous Mg removal by high-yielding fertilizer-responsive crop varieties without Mg supplementation, MGD is becoming a rising concern for optimum crop production in different cultivated regions of the world (Guo et al., 2016; Senbayram et al., 2016; Wang et al., 2020). Beyond the soil data indicating apparent MGD in China, increasingly more evidence corroborates the responsiveness of different crop plants to Mg supplementation under field conditions. In South China, with frequently occurring severe Mg deficiency, the combined application of Mg and lime in Pinghe, Fujian province, a major pomelo production county, increases yield and total soluble solids by 30.5 and 4.2%, respectively (Zhang et al., 2021). In the same region, Mg application also improves the number of amino acids and total sugars in tea plants in Zhejiang (Ruan et al., 2012) and the yield of wax gourd in Guangzhou under field conditions (Zhang et al., 2020). Field studies in the lower and middle reaches of the Yangtze River, an Mg-deficient region, confirm that Mg fertilization improves the yield, oil content, unsaturated fatty acids of the important regional crop rapeseed (Geng et al., 2021). Mg fertilization also promotes tobacco yield and quality in South Anhui (Zhang et al., 2015). Even in Mg-sufficient soils (northeast and north-central China), field-based studies show 8.5 and 9.3% yield increases in Mg supplemented rice and cabbage plots, respectively (Ding et al., 2012; Liu et al., 2021). Indeed, most crop plants have highly dynamic Mg demand over the growing season and may show MGD symptoms at certain critical growth stages even in Mg sufficient fields, therefore, Mg application improves crop yield or quality in a species-dependent manner (Wang et al., 2020; Liu et al., 2021). How dramatic spatial variations in the abundance of soil Ex-Mg affect crop yield and quality improvement, and what are crop-, soil-, and region-oriented strategic solutions for fertilizer providers have emerged as two fundamental challenges to be tackled seriously.
Our soil database suggests that agroecosystems in South China, the southwest, and the middle and lower reaches of the Yangtze River are more responsive to Mg fertilization, compared to other cultivated regions in China. These regions are particularly important for the production of cash crops such as vegetables, fruits, tea, and tobacco. Mg fertilization not only prevents crops from potential MGD at the seedling stage but also promotes photosynthesis for higher yield and better quality. Meanwhile, it improves nutrient utilization efficiency by balanced elemental provision and synergistic effects and reduces nutrient loss to the environment (Tian et al., 2021; Wang et al., 2022). Notably, MGD can lead to hypomagnesemia grass tetany (hypomagnesemia), reported in ~30–50% of dairy herds in New Zealand, a foremost reason for the reduction of milk production in grazing animals (Loganathan et al., 2005). Mg supplementation in the grasslands of southwest and northwest China ensures pasture and grazing animal productivity. To efficiently combat rising MGD in agricultural systems, significantly more efforts are required to match soil providing capacities with crop demand by precise plant and soil testing, proper fertilizer formulation, and optimal fertilization rates and timing to minimize net soil mining (Figure 7).
Figure 7. A proposed model to combat rising Mg deficiency in different agroecosystems. Mg availability to plants highly depends on the abundance of indigenous Mg and other soil factors. To close yield gaps in different croplands, Mg recommendations should be optimized primarily according to its net removal and indigenous soil reserves.
This review narrates the biological functions of Mg and draws attention to the rising risks of MGD in croplands. Numerous classic literature and the latest explorations agglomerated in this article demonstrate that Mg mediates a wide array of central physiological and biochemical processes in plants, while MGD undoubtedly weakens the performance and productivity of different agricultural systems. In China, ~55% of arable lands are found Mg-deficient (Ex-Mg < 120 mg kg−1 soil), with an obvious spatially declining trend of soil Ex-Mg from northern (227–488 mg kg−1) toward southern (32–89 mg kg−1) China. Mg deficiency can be primarily traced back to higher depletion of soil Ex-Mg by fruits, vegetables, sugarcane, tubers, tea, and tobacco in tropical and subtropical climate zones. Further, each unit decline in soil pH from neutral can reduce ~2-fold Ex-Mg. This focused documentation provides a useful quantitative framework for a wide range of plant scientists and growers to understand Mg dynamics in the soil, and accordingly, to combat the rising MGD for higher crop yield and better quality. It also signifies the call for a global effort to better evaluate the spatial distribution of MGD and related risks.
Although significant advances have been made in understanding the physiological functions of Mg, a large knowledge gap still exists to be closely compared to N, P, and K. Much more efforts are required to investigate molecular and genetic mechanisms of physiological functions of Mg, MGD signaling, and adaptation to local heterogeneous Mg conditions, which lays the foundation for breeding for Mg efficient crop germplasm and cultivars. Genome editing and omics techniques may provide valuable resources for this purpose.
Given spatial variations of soil Ex-Mg, a considerable amount of research work can be projected to study responses of different crops to Mg supplementation and develop site-specific Mg benchmarks in distinct agroecosystems. To counteract competing cations, it is important to rationalize fertilizer formulation and application strategies to improve Mg availability. Finally, creating awareness and eliminating misconceptions among local growers, together with subsidizing Mg fertilizers, especially in Mg-deficient regions, can be beneficial to close yield and quality gaps among different cultivated regions.
MI and XL are involved in conceptualization, data curation, analysis, software, writing—original draft, writing—review, and editing. YW, MY, and ZW are involved in data collection. LW and CL are involved in writing—review and editing. All authors revised and approved the submitted version of the manuscript.
This work was supported by the National Natural Science Foundation of China (32172663).
The authors declare that the research was conducted in the absence of any commercial or financial relationships that could be construed as a potential conflict of interest.
All claims expressed in this article are solely those of the authors and do not necessarily represent those of their affiliated organizations, or those of the publisher, the editors and the reviewers. Any product that may be evaluated in this article, or claim that may be made by its manufacturer, is not guaranteed or endorsed by the publisher.
The authors gratefully acknowledge the National Earth System Science Data Center, National Science and Technology Infrastructure of China, and International Magnesium Institute (IMI)_China for making the soil database accessible.
The Supplementary Material for this article can be found online at: https://www.frontiersin.org/articles/10.3389/fpls.2022.802274/full#supplementary-material
Al Alawi, A. M., Majoni, S. W., and Falhammar, H. (2018). Magnesium and human health: perspectives and research directions. Int. J. Endocrinol. 2018, 9041694. doi: 10.1155/2018/9041694
Asada, K. (2006). Production and scavenging of reactive oxygen species in chloroplasts and their functions. Plant Physiol. 141, 391–396. doi: 10.1104/pp.106.082040
Ayre, B. G. (2011). Membrane-transport systems for sucrose in relation to whole-plant carbon partitioning. Mol. Plant 4, 377–394. doi: 10.1093/mp/ssr014
Azizi, K., Yaghobi, M., Hidary, S., Chaeichi, M. R., and Roham, R. (2011). Effects of different methods of magnesium sulphate application on qualitative and quantitative yield of lentil (Lens culinaris Medik.) cultivars under Khorramabad climatic conditions of Iran. Res. Crops 12, 103–111.
Billard, V., Maillard, A., Coquet, L., Jouenne, T., Cruz, F., Garcia-Mina, J. M., et al. (2016). Mg deficiency affects leaf Mg remobilization and the proteome in Brassica napus. Plant Physiol. Biochem. 107, 337–343. doi: 10.1016/j.plaphy.2016.06.025
Boaretto, R. M., Hippler, F. W. R., Ferreira, G. A., Azevedo, R. A., Quaggio, J. A., and Mattos, D. (2020). The possible role of extra magnesium and nitrogen supply to alleviate stress caused by high irradiation and temperature in lemon trees. Plant Soil 457, 57–70. doi: 10.1007/s11104-020-04597-y
Bogdevich, I. M., and Mishuk, O. L. (2006). Efficiency of magnesium and sulfur-containing fertilizers under spring rape seed on the podzoluvisol loam soil with different magnesium content. Proc. Nat. Acad. Sci. U.S.A. 2, 68–72.
Bose, J., Babourina, O., and Rengel, Z. (2011). Role of magnesium in alleviation of aluminium toxicity in plants. J. Exp. Bot. 62, 2251–2264. doi: 10.1093/jxb/erq456
Broadley, M. R., and White, P. J. (2010). Eats roots and leaves. Can edible horticultural crops address dietary calcium, magnesium and potassium deficiencies? Proc. Nutr. Soc. 69, 601–612. doi: 10.1017/S0029665110001588
Bui, D. M., Gregan, J., Jarosch, E., Ragnini, A., and Schweyen, R. J. (1999). The bacterial magnesium transporter CorA can functionally substitute for its putative homologue MRS2p in the yeast inner mitochondrial membrane. J. Biol. Chem. 274, 20438–20443. doi: 10.1074/jbc.274.29.20438
Cai, J., Chen, L., Qu, H., Lian, J., Liu, W., Hu, Y., et al. (2012). Alteration of nutrient allocation and transporter genes expression in rice under N, P, K, and Mg deficiencies. Acta Physiol. Plant. 34, 939–946. doi: 10.1007/s11738-011-0890-x
Cai, Y. T., Zhang, H., Qi, Y. P., Ye, X., Huang, Z. R., Guo, J. X., et al. (2019). Responses of reactive oxygen species and methylglyoxal metabolisms to magnesium-deficiency differ greatly among the roots, upper and lower leaves of Citrus sinensis. BMC Plant Biol. 19, 76. doi: 10.1186/s12870-019-1683-4
Cakmak, I., Hengeler, C., and Marschner, H. (1994a). Partitioning of shoot and root dry-matter and carbohydrates in bean-plants suffering from phosphorus, potassium and magnesium-deficiency. J. Exp. Bot. 45, 1245–1250. doi: 10.1093/jxb/45.9.1245
Cakmak, I., Hengeler, C., and Marschner, H. (1994b). Changes in phloem export of sucrose in leaves in response to phosphorus, potassium and magnesium-deficiency in bean-plants. J. Exp. Bot. 45, 1251–1257. doi: 10.1093/jxb/45.9.1251
Cakmak, I., and Kirkby, E. A. (2008). Role of magnesium in carbon partitioning and alleviating photooxidative damage. Physiol. Plant. 133, 692–704. doi: 10.1111/j.1399-3054.2007.01042.x
Cakmak, I., and Yazici, A. M. (2010). Magnesium: a forgotten element in crop production. Better Crops 94, 23–25. Available online at: https://www.ks-minerals-and-agriculture.com/en/pdf-articles/article-201006-better-crops-magnesium.pdf
Canizella, B. T., Moreira, A., Moraes, L. A. C., and Fageria, N. K. (2015). Efficiency of magnesium use by common bean varieties regarding yield, physiological components, and nutritional status of plants. Commun. Soil Sci. Plant Anal. 46, 1376–1390. doi: 10.1080/00103624.2015.1043452
Ceylan, Y., Kutman, U. B., Mengutay, M., and Cakmak, I. (2016). Magnesium applications to growth medium and foliage affect the starch distribution, increase the grain size and improve the seed germination in wheat. Plant Soil 406, 145–156. doi: 10.1007/s11104-016-2871-8
Chen, C. T., Lee, C. L., and Yeh, D. M. (2018). Effects of nitrogen, phosphorus, potassium, calcium, or magnesium deficiency on growth and photosynthesis of Eustoma. Hort. Sci. 53, 795–798. doi: 10.21273/HORTSCI12947-18
Chen, J., Li, L. G., Liu, Z. H., Yuan, Y. J., Guo, L. L., Mao, D. D., et al. (2009). Magnesium transporter AtMGT9 is essential for pollen development in Arabidopsis. Cell Res. 19, 887–898. doi: 10.1038/cr.2009.58
Chen, S., Liang, Z., Webster, R., Zhang, G., Zhou, Y., Teng, H., et al. (2018). A high-resolution map of soil pH in China made by hybrid modelling of sparse soil data and environmental covariates and its implications for pollution. Sci. Total Environ. 655, 273–283. doi: 10.1016/j.scitotenv.2018.11.230
Chen, Z. C., Peng, W. T., Li, J., and Liao, H. (2018). Functional dissection and transport mechanism of magnesium in plants. Semin. Cell Dev. Biol. 74, 142–152. doi: 10.1016/j.semcdb.2017.08.005
Chen, Z. C., Yamaji, N., Horie, T., Che, J., Li, J., An, G., et al. (2017). A magnesium transporter OsMGT1 plays a critical role in salt tolerance in rice. Plant Physiol. 174, 1837–1849. doi: 10.1104/pp.17.00532
Chen, Z. C., Yamaji, N., Motoyama, R., Nagamura, Y., and Ma, J. F. (2012). Up-regulation of a magnesium transporter gene OsMGT1 is required for conferring aluminum tolerance in rice. Plant Physiol. 159, 1624–1633. doi: 10.1104/pp.112.199778
Chou, T. S., Chao, Y. Y., Huang, W. D., Hong, C. Y., and Kao, C. H. (2011). Effect of magnesium deficiency on antioxidant status and cadmium toxicity in rice seedlings. J. Plant Physiol. 168, 1021–1030. doi: 10.1016/j.jplph.2010.12.004
Conn, S. J., Conn, V., Tyerman, S. D., Kaiser, B. N., Leigh, R. A., and Gilliham, M. (2011). Magnesium transporters, MGT2/MRS2-1 and MGT3/MRS2-5, are important for magnesium partitioning within Arabidopsis thaliana mesophyll vacuoles. New Phytol. 190, 583–594. doi: 10.1111/j.1469-8137.2010.03619.x
Cui, Z., Dou, Z., Chen, X., Ju, X., and Zhang, F. (2014). Managing agricultural nutrients for food security in China: past, present, and future. Agron. J. 106, 191–198. doi: 10.2134/agronj2013.0381
da Silva, D. M., de Souza, K. R. D., Boas, L. V. V., Alves, Y. S., and Alves, J. D. (2017). The effect of magnesium nutrition on the antioxidant response of coffee seedlings under heat stress. Sci. Hortic. 224, 115–125. doi: 10.1016/j.scienta.2017.04.029
Dann Iii, C. E., Wakeman, C. A., Sieling, C. L., Baker, S. C., Irnov, I., and Winkler, W. C. (2007). Structure and mechanism of a metal-sensing regulatory RNA. Cell 130, 878–892. doi: 10.1016/j.cell.2007.06.051
D'Egidio, S., Galieni, A., Stagnari, F., Pagnani, G., and Pisante, M. (2019). Yield, quality and physiological traits of red beet under different magnesium nutrition and light intensity levels. Agron 9, 379. doi: 10.3390/agronomy9070379
Deng, W., Luo, K., Li, D., Zheng, X., Wei, X., Smith, W., et al. (2006). Overexpression of an Arabidopsis magnesium transport gene, AtMGT1, in Nicotiana benthamiana confers Al tolerance. J. Exp. Bot. 57, 4235–4243. doi: 10.1093/jxb/erl201
Ding, Y., Jiao, X., Nie, D., Li, L., and Huang, M. (2012). Effects of combined application of different nitrogen sources and magnesium fertilizers on cabbage yield, quality and nutrient uptake. Zhongguo Shengtai Nongye Xuebao 20, 996–1002. doi: 10.3724/SP.J.1011.2012.00996
Ding, Y., Luo, W., and Xu, G. (2006). Characterisation of magnesium nutrition and interaction of magnesium and potassium in rice. Ann. Appl. Biol. 149, 111–123. doi: 10.1111/j.1744-7348.2006.00080.x
Farhat, N., Elkhouni, A., Zorrig, W., Smaoui, A., Abdelly, C., and Rabhi, M. (2016). Effects of magnesium deficiency on photosynthesis and carbohydrate partitioning. Acta Physiol. Plant. 38, 145. doi: 10.1007/s11738-016-2165-z
Farhat, N., Rabhi, M., Krol, M., Barhoumi, Z., Ivanov, A. G., McCarthy, A., et al. (2014). Starch and sugar accumulation in Sulla carnosa leaves upon Mg2+ starvation. Acta Physiol. Plant. 36, 2157–2165. doi: 10.1007/s11738-014-1592-y
Faust, F., and Schubert, S. (2016). Protein synthesis is the most sensitive process when potassium is substituted by sodium in the nutrition of sugar beet (Beta vulgaris). Plant Physiol. Biochem. 107, 237–247. doi: 10.1016/j.plaphy.2016.06.009
Garcia, A., Crusciol, C. A. C., Rosolem, C. A., Bossolani, J. W., Nascimento, C. A. C., McCray, J. M., et al. (2022). Potassium-magnesium imbalance causes detrimental effects on growth, starch allocation and rubisco activity in sugarcane plants. Plant Soil. 472, 225–238 doi: 10.21203/rs.3.rs-244222/v1
Gebert, M., Meschenmoser, K., Svidov,á, S., Weghuber, J., Schweyen, R., Eifler, K., et al. (2009). A root-expressed magnesium transporter of the MRS2/MGT gene family in Arabidopsis thaliana allows for growth in low-Mg2+ environments. Plant Cell 21, 4018–4030. doi: 10.1105/tpc.109.070557
Geng, G., Cakmak, I., Ren, T., Lu, Z., and Lu, J. (2021). Effect of magnesium fertilization on seed yield, seed quality, carbon assimilation and nutrient uptake of rapeseed plants. Field Crops Res. 264, 108082. doi: 10.1016/j.fcr.2021.108082
Gerendás, J., and Führs, H. (2013). The significance of magnesium for crop quality. Plant Soil 368, 101–128. doi: 10.1007/s11104-012-1555-2
Graeff, S., Steffens, D., and Schubert, S. (2001). Use of reflectance measurements for the early detection of N, P, Mg, and Fe deficiencies in Zea mays L. J. Plant Nutr. Soil Sci. 164, 445–450.3. doi: 10.1002/1522-2624(200108)164:4<445::AID-JPLN445>3.0.CO;2-1
Gransee, A., and Führs, H. (2013). Magnesium mobility in soils as a challenge for soil and plant analysis, magnesium fertilization and root uptake under adverse growth conditions. Plant Soil 368, 5–21. doi: 10.1007/s11104-012-1567-y
Greffeuille, V., Abecassis, J., Lapierre, C., and Lullien-Pellerin, V. (2006). Bran size distribution at milling and mechanical and biochemical characterization of common wheat grain outer layers: a relationship assessment. Cereal Chem. 83, 641–646. doi: 10.1094/CC-83-0641
Gregan, J., Bui, D. M., Pillich, R., Fink, M., Zsurka, G., and Schweyen, R. J. (2001). The mitochondrial inner membrane protein LPE10p, a homologue of MRS2p, is essential for magnesium homeostasis and group II intron splicing in yeast. Mol. Gen. Genet. 264, 773–781. doi: 10.1007/s004380000366
Grzebisz, W. (2011). Magnesium-food and human health. J. Elem. 16, 299–323. doi: 10.5601/jelem.2011.16.2.13
Grzebisz, W. (2013). Crop response to magnesium fertilization as affected by nitrogen supply. Plant Soil 368, 23–39. doi: 10.1007/s11104-012-1574-z
Guo, J. H., Liu, X. J., Zhang, Y., Shen, J. L., Han, W. X., Zhang, W. F., et al. (2010). Significant acidification in major Chinese croplands. Science 327, 1008–1010. doi: 10.1126/science.1182570
Guo, K. M., Babourina, O., Christopher, D. A., Borsic, T., and Rengel, Z. (2010). The cyclic nucleotide-gated channel AtCNGC10 transports Ca2+ and Mg2+ in Arabidopsis. Physiol. Plant. 139, 303–312. doi: 10.1111/j.1399-3054.2010.01366.x
Guo, W., Nazim, H., Liang, Z., and Yang, D. (2016). Magnesium deficiency in plants: an urgent problem. Crop J. 4, 83–91. doi: 10.1016/j.cj.2015.11.003
Hackel, A., Schauer, N., Carrari, F., Fernie, A. R., Grimm, B., and Kühn, C. (2006). Sucrose transporter LeSUT1 and LeSUT2 inhibition affects tomato fruit development in different ways. Plant J. 45, 180–192. doi: 10.1111/j.1365-313X.2005.02572.x
Han, W., Chen, Y., Zhao, F. J., Tang, L., Jiang, R., and Zhang, F. (2011). Floral, climatic and soil pH controls on leaf ash content in China's terrestrial plants. Glob. Ecol. Biogeogr. 21, 376–382. doi: 10.1111/j.1466-8238.2011.00677.x
Hariadi, Y., and Shabala, S. (2004). Screening broad beans (Vicia faba) for magnesium deficiency. II. Photosynthetic performance and leaf bioelectrical responses. Funct. Plant Biol. 31, 539–549. doi: 10.1071/FP03202
Hauer-Jákli, M., and Tränkner, M. (2019). Critical leaf magnesium thresholds and the impact of magnesium on plant growth and photo-oxidative defense: a systematic review and meta-analysis on 70 years of research. Front. Plant Sci. 10, 766. doi: 10.3389/fpls.2019.00766
Hazra, S., Henderson, J. N., Liles, K., Hilton, M. T., and Wachter, R. M. (2015). Regulation of ribulose-1, 5-bisphosphate carboxylase/oxygenase (Rubisco) activase product inhibition, cooperativity, and magnesium activation. J. Biol. Chem. 290, 24222–24236. doi: 10.1074/jbc.M115.651745
Hermans, C., Bourgis, F., Faucher, M., Strasser, R. J., Delrot, S., and Verbruggen, N. (2005). Magnesium deficiency in sugar beets alters sugar partitioning and phloem loading in young mature leaves. Planta 220, 541–549. doi: 10.1007/s00425-004-1376-5
Hermans, C., Conn, S. J., Chen, J., Xiao, Q., and Verbruggen, N. (2013). An update on magnesium homeostasis mechanisms in plants. Metallomics 5, 1170–1183. doi: 10.1039/c3mt20223b
Hermans, C., Hammond, J. P., White, P. J., and Verbruggen, N. (2006). How do deficiencies of essential mineral elements alter biomass allocation. Trends Plant Sci. 11, 610–617. doi: 10.1016/j.tplants.2006.10.007
Hermans, C., Johnson, G. N., Strasser, R. J., and Verbruggen, N. (2004). Physiological characterisation of magnesium deficiency in sugar beet: acclimation to low magnesium differentially affects photosystems I and II. Planta 220, 344–355. doi: 10.1007/s00425-004-1340-4
Hermans, C., and Verbruggen, N. (2005). Physiological characterization of Mg deficiency in Arabidopsis thaliana. J Exp. Bot. 56, 2153–2161. doi: 10.1093/jxb/eri215
Hmiel, S. P., Snavely, M. D., Miller, C. G., and Maguire, M. E. (1986). Magnesium transport in salmonella-typhimurium - characterization of magnesium influx and cloning of a transport gene. J. Bacteriol.168, 1444–1450. doi: 10.1128/jb.168.3.1444-1450.1986
Ishfaq, M., Zhong, Y., Wang, Y., and Li, X. (2021). Magnesium limitation leads to transcriptional down-tuning of auxin synthesis, transport, and signaling in the tomato root. Front. Plant Sci. 12, 802399. doi: 10.3389/fpls.2021.802399
Jaghdani, S. J., Jahns, P., and Tränkner, M. (2020). Mg deficiency induces photo-oxidative stress primarily by limiting CO2 assimilation and not by limiting photosynthetic light utilization. Plant Sci. 302, 110751. doi: 10.1016/j.plantsci.2020.110751
Jaghdani, S. J., Jahns, P., and Tränkner, M. (2021). The impact of magnesium deficiency on photosynthesis and photoprotection in Spinacia oleracea. Plant Stress 2, 100040. doi: 10.1016/j.stress.2021.100040
Järvi, S., Gollan, P. J., and Aro, E. M. (2013). Understanding the roles of the thylakoid lumen in photosynthesis regulation. Front. Plant Sci. 4, 434. doi: 10.3389/fpls.2013.00434
Jayaganesh, S., Venkatesan, S., and Senthurpandian, V. K. (2011). Impact of different sources and doses of magnesium fertilizer on biochemical constituents and quality parameters of black tea. Asian J. Biochem. 6, 273–281. doi: 10.3923/ajb.2011.273.281
Jensen, R. G. (2000). Activation of Rubisco regulates photosynthesis at high temperature and CO2. PNAS 97, 12937–12938. doi: 10.1073/pnas.97.24.12937
Jezek, M., Geilfus, C. M., Bayer, A., and Mühling, K. H. (2015). Photosynthetic capacity, nutrient status, and growth of maize (Zea mays L.) upon MgSO4 leaf-application. Front. Plant Sci. 5, 781. doi: 10.3389/fpls.2014.00781
Jia, Y., Xu, H., Wang, Y., Xin, Y., Ningwei, L., Zengrong, H., et al. (2021). Differences in morphological and physiological features of citrus seedlings are related to Mg transport from the parent to branch organs. BMC Plant Biol. 21, 239. doi: 10.1186/s12870-021-03028-z
Karley, A. J., and White, P. J. (2009). Moving cationic minerals to edible tissues: potassium, magnesium, calcium. Curr. Opin. Plant Biol. 12, 291–298. doi: 10.1016/j.pbi.2009.04.013
Kibria, M. G., Barton, L., and Rengel, Z. (2020). Foliar application of magnesium mitigates soil acidity stress in wheat. J. Agron. Crop Sci. 207, 378–389. doi: 10.1111/jac.12468
Klein, D. J., Moore, P. B., and Steitz, T. A. (2004). The contribution of metal ions to the structural stability of the large ribosomal subunit. RNA 10, 1366–1379. doi: 10.1261/rna.7390804
Kobayashi, N. I., Iwata, N., Saito, T., Suzuki, H., Iwata, R., Tanoi, K., et al. (2013). Application of 28 Mg for characterization of Mg uptake in rice seedling under different pH conditions. J. Radioanal. Nucl. Chem. 296, 531–534. doi: 10.1007/s10967-012-2010-9
Koch, M., Busse, M., Naumann, M., Jákli, B., Smit, I., Cakmak, I., et al. (2019). Differential effects of varied potassium and magnesium nutrition on production and partitioning of photoassimilates in potato plants. Physiol. Plant. 166, 921–935. doi: 10.1111/ppl.12846
Koch, M., Winkelmann, M. K., Hasler, M., Pawelzik, E., and Naumann, M. (2020). Root growth in light of changing magnesium distribution and transport between source and sink tissues in potato (Solanum tuberosum L.). Sci. Rep. 10, 8796. doi: 10.1038/s41598-020-65896-z
Kwon, M. C., Kim, Y. X., Lee, S., Jung, E. S., Singh, D., Sung, J., et al. (2019). Comparative metabolomics unravel the effect of magnesium oversupply on tomato fruit quality and associated plant metabolism. Metabolites 9, 231. doi: 10.3390/metabo9100231
Laing, W., Greer, D., Sun, O., Beets, P., Lowe, A., and Payn, T. (2000). Physiological impacts of Mg deficiency in Pinus radiata: growth and photosynthesis. New Phytol. 146, 47–57. doi: 10.1046/j.1469-8137.2000.00616.x
Lasa, B., Frechilla, S., Aleu, M., González-Moro, B., Lamsfus, C., and Aparicio-Tejo, P. M. (2000). Effects of low and high levels of magnesium on the response of sunflower plants grown with ammonium and nitrate. Plant Soil 225, 167–174. doi: 10.1023/A:1026568329860
Lenz, H. N., Dombinov, V., Dreistein, J., Reinhard, M. R., Gebert, M., and Knoop, V. (2013). Magnesium deficiency phenotypes upon multiple knockout of Arabidopsis thaliana MRS2 clade B genes can be ameliorated by concomitantly reduced calcium supply. Plant Cell Physiol. 54, 1118–1131. doi: 10.1093/pcp/pct062
Li, C. P., Qi, Y. P., Zhang, J., Yang, L. T., Wang, D. H., Ye, X., et al. (2017). Magnesium-deficiency-induced alterations of gas exchange, major metabolites and key enzymes differ among roots, and lower and upper leaves of Citrus sinensis seedlings. Tree Physiol. 37, 1564–1581. doi: 10.1093/treephys/tpx067
Li, D., Ma, W., Wei, J., Mao, Y., Peng, Z., Zhang, J., et al. (2020). Magnesium promotes root growth and increases aluminum tolerance via modulation of nitric oxide production in Arabidopsis. Plant Soil 457, 83–95. doi: 10.1007/s11104-019-04274-9
Li, H., Du, H., Huang, K., Chen, X., Liu, T., Gao, S., et al. (2016). Identification, and functional and expression analyses of the CorA/MRS2/MGT-type magnesium transporter family in maize. Plant Cell Physiol. 57, 1153–1168. doi: 10.1093/pcp/pcw064
Li, J., Yokosho, K., Liu, S., Cao, H. R., Yamaji, N., Zhu, X. G., et al. (2020). Diel magnesium fluctuations in chloroplasts contribute to photosynthesis in rice. Nat. Plants 6, 848–859. doi: 10.1038/s41477-020-0686-3
Li, L., Tutone, A. F., Drummond, R. S. M., Gardner, R. C., and Luan, S. (2001). A novel family of magnesium transport genes in Arabidopsis. Plant Cell 13, 2761–2775. doi: 10.1105/tpc.010352
Li, L. G., Sokolov, L. N., Yang, Y. H., Li, D. P., Ting, J., Pandy, G. K., et al. (2008). A mitochondrial magnesium transporter functions in Arabidopsis pollen development. Mol. Plant 1, 675–685. doi: 10.1093/mp/ssn031
Li, X. X., Wang, L. X., Zhang, H., Du, X., Jiang, S. W., Shen, T., et al. (2013). Seasonal variations in notification of active tuberculosis cases in China, 2005-2012. PLoS ONE. 8, e68102. doi: 10.1371/journal.pone.0068102
Li, Z., Phillip, D., Neuhäuser, B., Schulze, W. X., and Ludewig, U. (2015). Protein dynamics in young maize root hairs in response to macro-and micronutrient deprivation. J. Proteome Res. 14, 3362–3371. doi: 10.1021/acs.jproteome.5b00399
Liu, M., Zhang, H., Fang, X., Zhang, Y., and Jin, C. (2018). Auxin acts downstream of ethylene and nitric oxide to regulate magnesium deficiency-induced root hair development in Arabidopsis thaliana. Plant Cell Physiol. 59, 1452–1465. doi: 10.1093/pcp/pcy078
Liu, X., Ju, X., Zhang, F., Pan, J., and Christie, P. (2003). Nitrogen dynamics and budgets in a winter wheat-maize cropping system in the North China Plain. Field Crops Res. 83, 111–124. doi: 10.1016/S0378-4290(03)00068-6
Liu, Z., Huang, Q., Liu, X., Li, P., Naseer, M. R., Che, Y., et al. (2021). Magnesium fertilization affected rice yields in magnesium sufficient soil in Heilongjiang province, northeast China. Front. Plant Sci. 12, 645806. doi: 10.3389/fpls.2021.645806
Loganathan, P., Hanly, J. A., and Currie, L. D. (2005). Effect of serpentine rock and its acidulated products as magnesium fertilisers for pasture, compared with magnesium oxide and epsom salts, on a pumice soil. 2. Dissolution and estimated leaching loss of fertiliser magnesium. NZJAR 48, 461–471. doi: 10.1080/00288233.2005.9513680
Lu, M., Liang, Y., Lakshmanan, P., Guan, X., Liu, D., and Chen, X. (2021). Magnesium application reduced heavy metal-associated health risks and improved nutritional quality of field-grown Chinese cabbage. Environ. Pollut. 289, 117881. doi: 10.1016/j.envpol.2021.117881
Lunin, V. V., Dobrovetsky, E., Khutoreskaya, G., Zhang, R., Joachimiak, A., Doyle, D. A., et al. (2006). Crystal structure of the CorA Mg2+ transporter. Nature 440, 833–837. doi: 10.1038/nature04642
Ma, C. L., Qi, Y. P., Liang, W. W., Yang, L. T., Lu, Y. B., Guo, P., et al. (2016). MicroRNA regulatory mechanisms on Citrus sinensis leaves to magnesium-deficiency. Front. Plant Sci. 7, 201. doi: 10.3389/fpls.2016.00201
Maguire, M. E., and Cowan, J. A. (2002). Magnesium chemistry and biochemistry. Biometals 15, 203–210. doi: 10.1023/A:1016058229972
Mao, D., Chen, J., Tian, L., Liu, Z., Yang, L., Tang, R., et al. (2014). Arabidopsis transporter MGT6 mediates magnesium uptake and is required for growth under magnesium limitation. Plant Cell 26, 2234–2248. doi: 10.1105/tpc.114.124628
Marschner, H. (2012). Marschner's Mineral Nutrition of Higher Plants, Vol. 89. San Diego, CA: Academic Press.
Mayland, H. F., and Wilkinson, S. R. (1989). Soil factors affecting magnesium availability in plant-animal systems: a review. J. Anim. Sci. 67, 3437–3444. doi: 10.2527/jas1989.67123437x
Mengel, K., Kirkby, E. A., Kosegarten, H., and Appel, T. (2001). “Nitrogen,” in Principles of Plant Nutrition, eds K. Mengel, E. A. Kirkby, H. Kosegarten, and T. Appel (Dordrecht: Springer) 397–434. doi: 10.1007/978-94-010-1009-2_7
Mengutay, M., Ceylan, Y., Kutman, U. B., and Cakmak, I. (2013). Adequate magnesium nutrition mitigates adverse effects of heat stress on maize and wheat. Plant Soil 368, 57–72. doi: 10.1007/s11104-013-1761-6
Metson, A. J., and Brooks, J. M. (1975). Magnesium in New Zealand soils. NZJAR 18, 317–335. doi: 10.1080/00288233.1975.10421055
Metson, A. J., and Gibson, E. J. (1977). Magnesium in New Zealand soils. NZJAR 20, 163–184. doi: 10.1080/00288233.1977.10427321
Mittler, R. (2002). Oxidative stress, antioxidants and stress tolerance. Trends Plant Sci. 7, 405–410. doi: 10.1016/S1360-1385(02)02312-9
Morton, A. R., Trolove, S. N., and Kerckhoffs, L. H. J. (2008). Magnesium deficiency in citrus grown in the Gisborne district of New Zealand. N. Z. J. Crop Hortic. Sci. 36, 199–213. doi: 10.1080/01140670809510236
Nakano, R., Ishida, H., Makino, A., and Mae, T. (2006). In vivo fragmentation of the large subunit of ribulose-1,5-bisphosphate carboxylase by reactive oxygen species in an intact leaf of cucumber under chilling-light conditions. Plant Cell Physiol. 47, 270–276. doi: 10.1093/pcp/pci245
Neuhaus, C., Geilfus, C. M., and Mühling, K. H. (2014). Increasing root and leaf growth and yield in Mg-deficient faba beans (Vicia faba) by MgSO4 foliar fertilization. J. Plant Nutr. Soil Sci. 177, 741–747. doi: 10.1002/jpln.201300127
Neuhaus, C., Geilfus, C. M., Zörb, C., and Mühling, K. H. (2013). Transcript expression of Mg-chelatase and H+-ATPase isogenes in Vicia faba leaves as influenced by root and foliar magnesium supply. Plant Soil 368, 41–50. doi: 10.1007/s11104-013-1711-3
Niu, Y., Chai, R., Liu, L., Jin, G., Liu, M., Tang, C., et al. (2014). Magnesium availability regulates the development of root hairs in Arabidopsis thaliana (L.) Heynh. Plant Cell Environ. 37, 2795–2813. doi: 10.1111/pce.12362
Oda, K., Kamiya, T., Shikanai, Y., Shigenobu, S., Yamaguchi, K., and Fujiwara, T. (2016). The Arabidopsis Mg transporter, MRS2-4, is essential for Mg homeostasis under both low and high Mg conditions. Plant Cell Physiol. 57, 754–763. doi: 10.1093/pcp/pcv196
Peng, H. Y., Qi, Y. P., Lee, J., Yang, L. T., Guo, P., Jiang, H. X., et al. (2015). Proteomic analysis of Citrus sinensis roots and leaves in response to long-term magnesium-deficiency. BMC Genom. 16, 253. doi: 10.1186/s12864-015-1462-z
Peng, Y. Y., Liao, L. L., Liu, S., Nie, M. M., Li, J., Zhang, L. D., et al. (2019). Magnesium deficiency triggers SGR-mediated chlorophyll degradation for magnesium remobilization. Plant Physiol. 181, 262–275. doi: 10.1104/pp.19.00610
Petrov, A. S., Bernier, C. R., Hsiao, C., Okafor, C. D., Tannenbaum, E., Stern, J., et al. (2012). RNA-magnesium-protein interactions in large ribosomal subunit. J. Physical Chem. B 116, 8113–8120. doi: 10.1021/jp304723w
Poglodzinski, R., Barlog, P., and Grzbisz, W. (2021). Effect of nitrogen and magnesium sulfate application on sugar beet yield and quality. Plant Soil Environ. 67, 507–513. doi: 10.17221/336/2021-PSE
Portis, A. R. (2003). Rubisco activase-Rubisco's catalytic chaperone. Photosyn. Res. 75, 11–27. doi: 10.1023/A:1022458108678
Puthiyaveetil, S., van Oort, B., and Kirchhoff, H. (2017). Surface charge dynamics in photosynthetic membranes and the structural consequences. Nat. Plants 3, 1–9. doi: 10.1038/nplants.2017.20
Riga, P., Anza, M., and Garbisu, C. (2005). Suitability of the antioxidative system as marker of magnesium deficiencyin Capsicum annuum L. plants under controlled conditions. Plant Growth Regul. 46, 51–59. doi: 10.1007/s10725-005-5466-6
Römheld, V. (2012). “Diagnosis of deficiency and toxicity of nutrients,” in Marschner's Mineral Nutrition of Higher Plants, ed P. Marschner (San Diego, CA: Elsevier), 299–312. doi: 10.1016/B978-0-12-384905-2.00011-X
Rosanoff, A. (2013). Changing crop magnesium concentrations: impact on human health. Plant Soil 368, 139–153. doi: 10.1007/s11104-012-1471-5
Rosanoff, A., and Kumssa, D. B. (2020). Impact of rising body weight and cereal grain food processing on human magnesium nutrition. Plant Soil 457, 5–23. doi: 10.1007/s11104-020-04483-7
Ruan, J. Y., Ma, L. F., and Yang, Y. J. (2012). Magnesium nutrition on accumulation and transport of amino acids in tea plants. J. Sci. Food Agric. 92, 1375–1383. doi: 10.1002/jsfa.4709
Saito, T., Kobayashi, N. I., Tanoi, K., Iwata, N., Suzuki, H., Iwata, R., et al. (2013). Expression and functional analysis of the CorA-MRS2-ALR-type magnesium transporter family in rice. Plant Cell Physiol. 54, 1673–1683. doi: 10.1093/pcp/pct112
Samborska, I. A., Kalaji, H. M., Sieczko, L., Goltsev, V., Borucki, W., and Jajoo, A. (2018). Structural and functional disorder in the photosynthetic apparatus of radish plants under magnesium deficiency. Funct. Plant Biol. 45, 668–679. doi: 10.1071/FP17241
Schock, I., Gregan, J., Steinhauser, S., Schweyen, R., Brennicke, A., and Knoop, V. (2000). A member of a novel Arabidopsis thaliana gene family of candidate Mg2+ ion transporters complements a yeast mitochondrial group II intron-splicing mutant. Plant J. 24, 489–501. doi: 10.1046/j.1365-313x.2000.00895.x
Senbayram, M., Gransee, A., Wahle, V., and Thiel, H. (2016). Role of magnesium fertilisers in agriculture: plant-soil continuum. Crop Pasture Sci. 66, 1219–1229. doi: 10.1071/CP15104
Shabala, S., and Hariadi, Y. (2005). Effects of magnesium availability on the activity of plasma membrane ion transporters and light-induced responses from broad bean leaf mesophyll. Planta 221, 56–65. doi: 10.1007/s00425-004-1425-0
Shaul, O. (2002). Magnesium transport and function in plants: the tip of the iceberg. Biometals 15, 307–321. doi: 10.1023/A:1016091118585
Shaul, O., Hilgemann, D. W., de-Almeida-Engler, J., Van Montagu, M., Inze, D., and Galili, G. (1999). Cloning and characterization of a novel Mg2+/H+ exchanger. EMBO J. 18, 3973–3980. doi: 10.1093/emboj/18.14.3973
Siddiqui, M. H., Alamri, S. A., Al-Khaishany, M. Y. Y., Al-Qutami, M. A., Ali, H. M., Al-Whaibi, M. H., et al. (2016). Mitigation of adverse effects of heat stress on Vicia faba by exogenous application of magnesium. Saudi J. Biol. Sci. 25, 1393–1401. doi: 10.1016/j.sjbs.2016.09.022
Stitt, M., and Zeeman, S. C. (2012). Starch turnover: pathways, regulation and role in growth. Curr. Opin. Plant Biol. 15, 282–292. doi: 10.1016/j.pbi.2012.03.016
Sun, O. J., Gielen, G. J., Sands, R., Smith, T. C., and Thorn, A. J. (2001). Growth, Mg nutrition and photosynthetic activity in Pinus radiata: evidence that NaCl addition counteracts the impact of low Mg supply. Trees 15, 335–340. doi: 10.1007/s004680100111
Taiz, L., and Zeiger, E. (2010). “Responses and adaptations to abiotic stress,” in Plant Physiol, 5th Edn., eds L. Taiz and E. Zeiger (Sunderland, MA: Sinauer Associates, Inc),755–778.
Takahashi, S., and Badger, M. R. (2011). Photoprotection in plants: a new light on photosystem II damage. Trends Plant Sci. 16, 53–60. doi: 10.1016/j.tplants.2010.10.001
Tang, N., Li, Y., and Chen, L. S. (2012). Magnesium deficiency-induced impairment of photosynthesis in leaves of fruiting Citrus reticulata trees accompanied by up-regulation of antioxidant metabolism to avoid photo-oxidative damage. J. Plant Nutr. Soil Sci. 175, 784–793. doi: 10.1002/jpln.201100329
Tanoi, K., and Kobayashi, N. I. (2015). Leaf senescence by magnesium deficiency. Plants 4, 756–772. doi: 10.3390/plants4040756
Tewari, R. K., Kumar, P., and Sharma, P. N. (2006). Magnesium deficiency induced oxidative stress and antioxidant responses in mulberry plants. Sci. Hortic. 108, 7–14. doi: 10.1016/j.scienta.2005.12.006
Tewari, R. K., Kumar, P., Tewari, N., Srivastava, S., and Sharma, P. N. (2004). Macronutrient deficiencies and differential antioxidant responses-influence on the activity and expression of superoxide dismutase in maize. Plant Sci. 166, 687–694. doi: 10.1016/j.plantsci.2003.11.004
Tian, X. Y., He, D. D., Bai, S., Zeng, W. Z., Wang, Z., Wang, M., et al. (2021). Physiological and molecular advances in magnesium nutrition of plants. Plant Soil 468, 1–17. doi: 10.1007/s11104-021-05139-w
Tränkner, M., and Jaghdani, S. J. (2019). Minimum magnesium concentrations for photosynthetic efficiency in wheat and sunflower seedlings. Plant Physiol. Biochem. 144, 234–243. doi: 10.1016/j.plaphy.2019.09.040
Tränkner, M., Jákli, B., Tavakol, E., Geilfus, C. M., Cakmak, I., Dittert, K., et al. (2016). Magnesium deficiency decreases biomass water-use efficiency and increases leaf water-use efficiency and oxidative stress in barley plants. Plant Soil 406, 409–423. doi: 10.1007/s11104-016-2886-1
Vacek, S., Podrázsk,ý, V., Hejcman, M., and Reme,š, J. (2006). Effect of Mg fertilization on yellowing disease of Norway spruce at higher elevations of the Šumava Mts., Czech Republic. J. Forest Sci. 52, 474–481. doi: 10.17221/4528-JFS
Verbruggen, N., and Hermans, C. (2013). Physiological and molecular responses to magnesium nutritional imbalance in plants. Plant Soil 368, 87–99. doi: 10.1007/s11104-013-1589-0
von Bennewitz, E., Cooper, T., Benavides, C., Losak, T., and Hlusek, J. (2011). Response of “Jonagold” apple trees to Ca, K and Mg fertilization in an andisol in southern Chile. J. Soil Sci. Plant Nutr. 11, 71–81. doi: 10.4067/S0718-95162011000300006
Vrataric, M., Sudaric, A., Kovacevic, V., Duvnjak, T., Krizmanic, M., and Mijic, A. (2006). Response of soybean to foliar fertilization with magnesium sulfate (Epsom salt). Cereal Res. Commun. 34, 709–712. doi: 10.1556/CRC.34.2006.1.177
Wakeel, A., and Ishfaq, M. (2022a). “Potash for quality of agricultural commodities,” in Potash Use and Dynamics in Agriculture, eds A. Wakeel and M. Ishfaq (Singapore: Springer).
Wakeel, A., and Ishfaq, M. (2022b). “Phytoavailability of potassium,” in Potash Use and Dynamics in Agriculture (Singapore: Springer).
Wang, Y., Long, Q., Li, Y., Kang, F., Fan, Z., Xiong, H., et al. (2022). Mitigating magnesium deficiency for sustainable citrus production: a case study in Southwest China. Sci. Hortic. 295, 110832. doi: 10.1016/j.scienta.2021.110832
Wang, Z., Hassan, M. U., Nadeem, F., Wu, L., Zhang, F., and Li, X. (2020). Magnesium fertilization improves crop yield in most production systems: a meta-analysis. Front. Plant Sci. 10, 1727. doi: 10.3389/fpls.2019.01727
White, P. J., and Broadley, M. R. (2009). Biofortification of crops with seven mineral elements often lacking in human diets-iron, zinc, copper, calcium, magnesium, selenium and iodine. New Phytol. 182, 49–84. doi: 10.1111/j.1469-8137.2008.02738.x
Wolf, J., Straten, S. T., Pitann, B., and Mühling, K. H. (2019). Foliar magnesium supply increases the abundance of RuBisCO of Mg-deficient maize plants. J. App. Bot. Food Qual. 92, 274–280. doi: 10.5073/JABFQ.2019.092.038
Xie, K. L., Cakmak, I., Wang, S. Y., Zhang, F. S., and Guo, S. W. (2021). Synergistic and antagonistic interactions between potassium and magnesium in higher plants. Crop J. 9, 249–256. doi: 10.1016/j.cj.2020.10.005
Xu, H., Luo, Z., Hu, W., Jia, Y., Wang, Y., Ye, X., et al. (2021). Magnesium absorption, translocation, subcellular distribution and chemical forms in citrus seedlings. Tree Physiol. 148, 1–15. doi: 10.1093/treephys/tpab148
Xu, X. F., Wang, B., Lou, Y., Han, W. J., Lu, J. Y., Li, D. D., et al. (2015). Magnesium Transporter 5 plays an important role in Mg transport for male gametophyte development in Arabidopsis. Plant J. 84, 925–936. doi: 10.1111/tpj.13054
Yamamoto, T., Shimizu, Y., Ueda, T., and Shiro, Y. (2010). Mg2+ dependence of 70 S ribosomal protein flexibility revealed by hydrogen/deuterium exchange and mass spectrometry. J. Biol. Chem. 285, 5646–5652. doi: 10.1074/jbc.M109.081836
Yan, Y. W., Mao, D. D., Yang, L., Qi, J. L., Zhang, X. X., Tang, Q. L., et al. (2018). Magnesium transporter MGT6 plays an essential role in maintaining magnesium homeostasis and regulating high magnesium tolerance in Arabidopsis. Front. Plant Sci. 9, 274. doi: 10.3389/fpls.2018.00274
Yang, G. H., Yang, L. T., Jiang, H. X., Li, Y., Wang, P., and Chen, L. S. (2012). Physiological impacts of magnesium-deficiency in citrus seedlings: photosynthesis, antioxidant system and carbohydrates. Trees 26, 1237–1250. doi: 10.1007/s00468-012-0699-2
Yang, L. T., Zhou, Y. F., Wang, Y. Y., Wu, Y. M., Ye, X., Guo, J. X., et al. (2019). Magnesium deficiency induced global transcriptome change in Citrus sinensis leaves revealed by RNA-Seq. Int. J. Mol. Sci. 20, 3129. doi: 10.3390/ijms20133129
Ye, X., Chen, X. F., Deng, C. L., Yang, L. T., Lai, N. W., Guo, J. X., et al. (2019). Magnesium-deficiency effects on pigments, photosynthesis and photosynthetic electron transport of leaves, and nutrients of leaf blades and veins in Citrus sinensis seedlings. Plants 8, 389. doi: 10.3390/plants8100389
Yuguan, Z., Min, Z., Luyang, L., Zhe, J., Chao, L., Sitao, Y., et al. (2009). Effects of cerium on key enzymes of carbon assimilation of spinach under magnesium deficiency. Biol. Trace Elem. Res. 131, 154–164. doi: 10.1007/s12011-009-8354-5
Ze, Y., Yin, S., Ji, Z., Luo, L., Liu, C., and Hong, F. (2009). Influences of magnesium deficiency and cerium on antioxidant system of spinach chloroplasts. Biometals 22, 941. doi: 10.1007/s10534-009-9246-z
Zhang, B., Cakmak, I., Feng, J., Yu, C., Chen, X., Xie, D., et al. (2020). Magnesium deficiency reduced the yield and seed germination in wax gourd by affecting the carbohydrate translocation. Front. Plant Sci. 11, 797. doi: 10.3389/fpls.2020.00797
Zhang, F., Cui, Z., Fan, M., Zhang, W., Chen, X., and Jiang, R. (2011). Integrated soil-crop system management: reducing environmental risk while increasing crop productivity and improving nutrient use efficiency in China. J. Environ. Qual. 40, 1051–1057. doi: 10.2134/jeq2010.0292
Zhang, G., Zhu, Q., Xiang, Z., Zhu, Y., Xia, D., and Ji, X. (2015). The influence of magnesium fertilizer on flue-cured tobacco growth yield and quality in South Anhui. Soils 47, 177–182.
Zhang, S., Yang, W., Muneer, M. A., Ji, Z., Tong, L., Zhang, X., et al. (2021). Integrated use of lime with Mg fertilizer significantly improves the pomelo yield, quality, economic returns and soil physicochemical properties under acidic soil of southern China. Sci. Hortic. 290, 110502. doi: 10.1016/j.scienta.2021.110502
Zhang, Z. F., and Zhang, W. F. (2008). The situation and trend of fertilizer application in China. Phosphate Comp. Ferti. 6, 23: 99612 (in Chinese).
Zhao, H., Zhou, Q., Zhou, M., Li, C., Gong, X., Liu, C., et al. (2012). Magnesium deficiency results in damage of nitrogen and carbon cross-talk of maize and improvement by cerium addition. Biol. Trace Elem. Res. 148, 102–109. doi: 10.1007/s12011-012-9340-x
Zhou, M., Gong, X., Ying, W., Chao, L., Hong, M., Wang, L., et al. (2011). Cerium relieves the inhibition of chlorophyll biosynthesis of maize caused by magnesium deficiency. Biol. Trace Elem. Res. 143, 468–477. doi: 10.1007/s12011-010-8830-y
Zhou, Y. H., Yu, J. Q., Mao, W. H., Huang, L. F., Song, X. S., and Nogues, S. (2006). Genotypic variation of Rubisco expression, photosynthetic electron flow and antioxidant metabolism in the chloroplasts of chill-exposed cucumber plants. Plant Cell Physiol. 47, 192–199. doi: 10.1093/pcp/pci234
Keywords: magnesium deficiency, physiological functions, photosynthetic activity, crop yield and quality, Mg transporter family (MRS2/MGT), China's agricultural system
Citation: Ishfaq M, Wang Y, Yan M, Wang Z, Wu L, Li C and Li X (2022) Physiological Essence of Magnesium in Plants and Its Widespread Deficiency in the Farming System of China. Front. Plant Sci. 13:802274. doi: 10.3389/fpls.2022.802274
Received: 26 October 2021; Accepted: 14 March 2022;
Published: 25 April 2022.
Edited by:
Dirceu Mattos Jr, Instituto Agronômico de Campinas (IAC), BrazilReviewed by:
Franz Hippler, Yara International, BrazilCopyright © 2022 Ishfaq, Wang, Yan, Wang, Wu, Li and Li. This is an open-access article distributed under the terms of the Creative Commons Attribution License (CC BY). The use, distribution or reproduction in other forums is permitted, provided the original author(s) and the copyright owner(s) are credited and that the original publication in this journal is cited, in accordance with accepted academic practice. No use, distribution or reproduction is permitted which does not comply with these terms.
*Correspondence: Xuexian Li, c3RldmVAY2F1LmVkdS5jbg==
Disclaimer: All claims expressed in this article are solely those of the authors and do not necessarily represent those of their affiliated organizations, or those of the publisher, the editors and the reviewers. Any product that may be evaluated in this article or claim that may be made by its manufacturer is not guaranteed or endorsed by the publisher.
Research integrity at Frontiers
Learn more about the work of our research integrity team to safeguard the quality of each article we publish.