- Department of Agricultural, Food and Nutritional Science, University of Alberta, Edmonton, AB, Canada
Clubroot, caused by the obligate parasite Plasmodiophora brassicae, is one of the most devastating diseases of canola (Brassica napus) in Canada. The identification of novel genes that contribute to clubroot resistance is important for the sustainable management of clubroot, as these genes may be used in the development of resistant canola cultivars. Phospholipase As (PLAs) play important roles in plant defense signaling and stress tolerance, and thus are attractive targets for crop breeding. However, since canola is an allopolyploid and has multiple copies of each PLA gene, it is time-consuming to test the functions of PLAs directly in this crop. In contrast, the model plant Arabidopsis thaliana has a simpler genetic background and only one copy of each PLA. Therefore, it would be reasonable and faster to validate the potential utility of PLA genes in Arabidopsis first. In this study, we identified seven homozygous atpla knockout/knockdown mutants of Arabidopsis, and tested their performance following inoculation with P. brassicae. Four mutants (pla1-iiα, pla1-iγ3, pla1-iii, ppla-iiiβ, ppla-iiiδ) developed more severe clubroot than the wild-type, suggesting increased susceptibility to P. brassicae. The homologs of these Arabidopsis PLAs (AtPLAs) in B. napus (BnPLAs) were identified through Blast searches and phylogenic analysis. Expression of the BnPLAs was subsequently examined in transcriptomic datasets generated from canola infected by P. brassicae, and promising candidates for further characterization identified.
Introduction
Clubroot, caused by the obligate parasite Plasmodiophora brassicae, is one of the most devastating diseases of canola (oilseed rape; Brassica napus) in Canada and other regions. The infected host plants develop characteristic root galls, which interfere with water and nutrient uptake, leading to aboveground symptoms including yellowing and wilting of the leaves, stunting, premature ripening, and losses in seed yield and quality (Pageau et al., 2006; Hwang et al., 2012). The deployment of clubroot resistant (CR) cultivars has been the main strategy to manage clubroot in western Canada (Hwang et al., 2014). Unfortunately, the effectiveness of resistance can be quickly lost as a result of the selection pressure imposed on pathogen populations by short rotations with CR cultivars carrying similar sources of resistance. In Canada, “new” pathotypes of P. brassicae able to overcome the resistance in most CR canola cultivars were identified in 2013, only 4 years after the introduction of the resistance trait (Strelkov et al., 2016). Since then, resistance-breaking pathotypes, many of which exhibit novel virulence patterns, have documented in many fields (Strelkov et al., 2018; Hollman et al., 2021). Given these rapid shifts in the virulence of pathogen populations, it is necessary to identify additional sources of resistance, including novel gene targets in canola (Zhou et al., 2020a), to aid in sustainable clubroot management. These could be used in rotations with existing CR canola cultivars, helping to reduce selection pressure on pathogen populations and contributing to resistance stewardship (Hwang et al., 2019).
Phospholipase As (PLAs), which catalyze the hydrolysis of membrane phospholipids into free fatty acids and lysophospholipids, are attractive targets for crop breeding due to their important roles in plant defense signaling and stress tolerance (Yang et al., 2007; Canonne et al., 2011; Chen et al., 2013). PLAs are involved in the plant response to biotic stress by inducing jasmonic acid (JA), oxylipin and phytoalexin biosynthesis (Canonne et al., 2011; Ruelland et al., 2015). In addition, PLA activity was suggested to be important in elicitor-induced oxidative burst (e.g., Verticillium dahliae extract) (Chandra et al., 1996). Recent transcriptomic studies indicate the involvement of phospholipases in the host response to P. brassicae. For example, the expression of many genes encoding phospholipases was increased in the roots of P. brassicae-infected Arabidopsis at 24 days after inoculation (dai) (Irani et al., 2018). Expression profiling of B. napus transcriptome datasets enabled the identification of differentially expressed genes, including PLAs, following P. brassicae inoculation (Galindo-González et al., 2020; Zhou et al., 2020b).
Since PLAs appear to contribute to the response of host plants to pathogens, functional validation of their role against P. brassicae will provide valuable information regarding their potential utility in the development of CR canola cultivars. However, since the model plant Arabidopsis thaliana (Arabidopsis thereafter) possesses over 20 PLAs and canola has several homologs of each of these genes (Iqbal et al., 2020), it is time-consuming to test the functions of BnPLAs directly in canola. In contrast, Arabidopsis has a simpler genetic background (Cheng et al., 2014) and only one copy of each PLA gene (Chen et al., 2013), and as a crucifer, also serves as a host of P. brassicae. Therefore, it would be more feasible and faster to validate the roles of PLA genes in clubroot resistance in Arabidopsis, to select promising candidates for further characterization in canola.
In this study, we identified and selected seven T-DNA insertion pla knockout/knockdown mutants of Arabidopsis from the Arabidopsis Biological Resource Center, and compared their performance with the wild-type plants following inoculation with P. brassicae. We believe that, this study would be first step in characterizing the role of PLAs in clubroot resistance, and will serve as the foundation of additional work in this area. The results showed that four mutants (pla1-iiα, pla1-iγ3, pla1-iii, ppla-iiiδ) developed more severe clubroot than the wild-type, suggesting increased susceptibility to P. brassicae. After identifying the homologs of the seven AtPLAs in canola (BnPLAs), and examining their expression profiles in published transcriptomic datasets generated from B. napus inoculated with P. brassicae, several BnPLAs were identified as promising candidates for further characterization in canola.
Materials and Methods
Plant Materials and Growth Conditions
The Arabidopsis mutants pla1-iγ1 (CS855673), pla1-iiα (SALK_086894C), pla1-iγ3 (SALK_012432C), pla1-iii (SALK_033291), spla2-α (SALK_099415C), ppla-iiiβ (SALK_057212C) and ppla-iiiδ (SALK_029470), derived from the wild-type ecotype Columbia, were obtained from the Arabidopsis Biological Resource Center (abrc.osu.edu; Table 1). The seeds were multiplied by growing into the next generation, and the homozygosity of T-DNA insertion of all the pla mutants was confirmed by PCR using two gene-specific primers (LP and RP) and a T-DNA border primer (Table 2). The seed was multiplied in a growth chamber set at 22/18°C with a photoperiod of 16 h day/8 h night. For inoculation with P. brassicae, all of the pla mutants and Columbia were grown in Sunshine LA4 potting mix (SunGro Horticulture, Vancouver, BC, Canada) in a greenhouse under 16 h light (natural light supplemented by artificial lighting) at 22°C. Three to five cold stratified (at 4°C for 3 days) seeds were placed in each pot (6 cm × 6 cm × 6 cm) and were thinned to one plant per pot after 1 week.
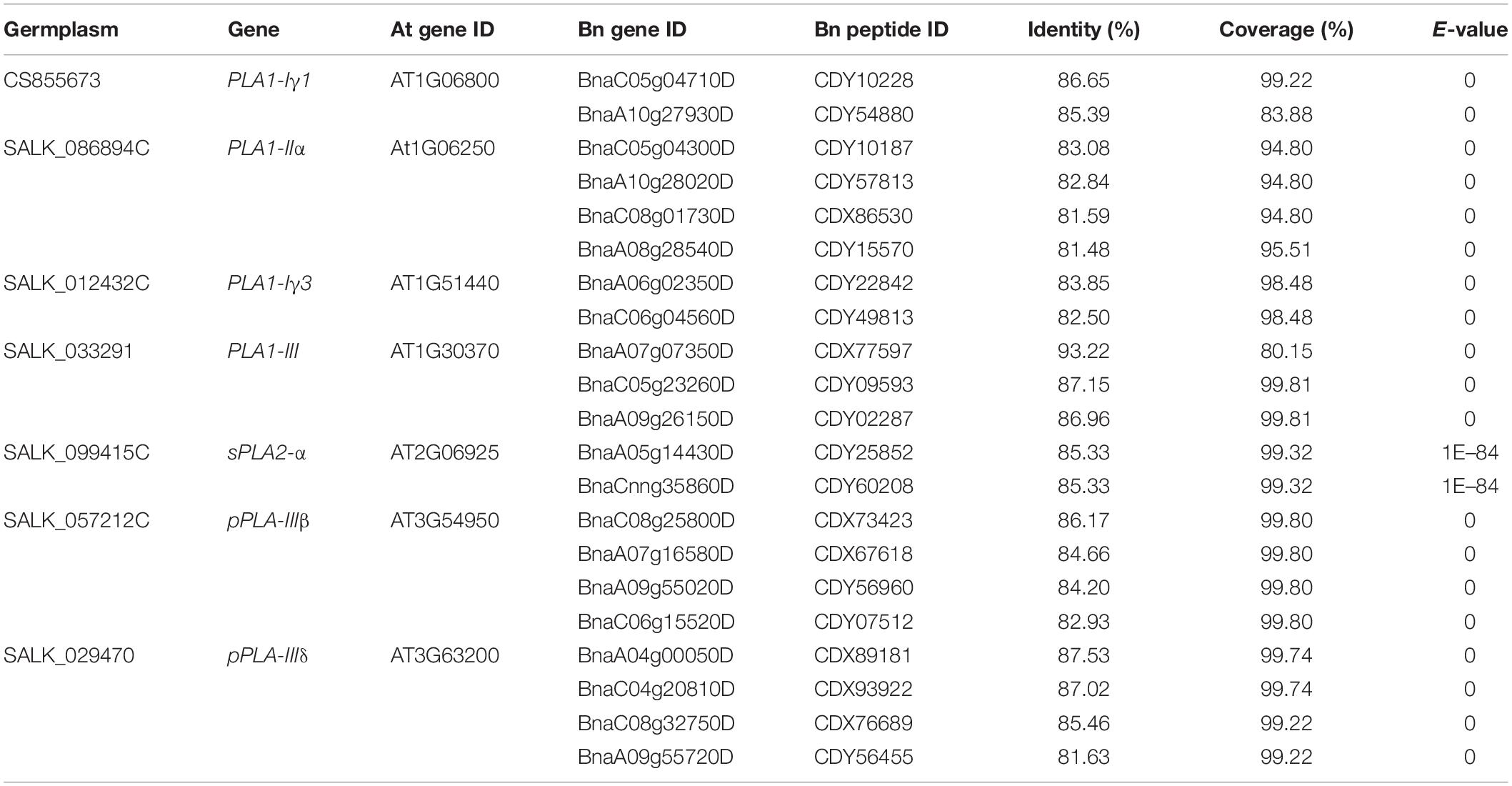
Table 1. Arabidopsis thaliana (At) PLAs included in this study and their homologs in Brassica napus (Bn).
Pathogen Material and Inoculation
Inoculations were conducted with a single-spore isolate of P. brassicae pathotype 3H, as classified on the Canadian Clubroot Differential set (Strelkov et al., 2018), which had been stored at −20°C in infected root galls of the B. napus cultivar “Brutor.” The resting spore inoculum suspension was prepared following Strelkov et al. (2006). Briefly, 5 g of the frozen galls were homogenized in 100 mL water and filtered through eight layers of cheesecloth to remove plant debris. The resting spore concentration was measured with a hemocytometer and adjusted to 1.0 × 105 resting spores/mL with sterile distilled water. Two-week old seedlings were inoculated by modifying the method of Ludwig-Müller et al. (2017). Briefly, 2 mL of the inoculum suspension was added to the soil near the base of each plant with pipette. The non-inoculated control plants were treated with same amount of water using the same method.
The severity of clubroot was evaluated at 3 to 4 weeks after inoculation when the aboveground parts of inoculated plants were yellowing and purpling. Treatments were replicated six times with 24 plants per replicate. Clubroot severity was evaluated on a 0–3 scale (Figure 2A), where: 0 = no galls, 1 = galls mainly on the lateral roots, 2 = obvious galls on both the primary and lateral roots with a moderately reduced root system, and 3 = large galls on the primary roots with a significantly reduced root system. The individual severity ratings were used to calculate a disease index (DI) for each replicate using the formula described by Strelkov et al. (2006): DI (%) = [(n1 × 1 + n2 × 2 + n3 × 3)/(N × 3)] × 100, where n1, n2, and n3 are the number of plants in each severity class and N are the total number of plants tested. An one-way Anova followed by the Dunnett’s test in R (r-project.org) was used to compare the mean DIs of each Arabidopsis mutant to the wild-type, with differences regarded as significant at p < 0.05.
Identification of AtPLA Homologs in Brassica napus
Homologs of the seven AtPLAs from Arabidopsis were identified in B. napus through BLAST and phylogenic analysis. Specifically, peptide sequences of the seven AtPLA obtained from the Arabidopsis (TAIR 11) database1 were blasted against the B. napus genome (Chalhoub et al., 2014) using BLASTP (E-value ≤1e–10, coverage >60%, identity >60%, and the top 20 hits).2 Homologs of the AtPLAs also were identified in B. rapa and B. oleracea using the same parameters, to improve the accuracy of the subsequent phylogenic analysis. The sequences of selected peptides of the three Brassica species were aligned with the seven AtPLA peptide sequences using Muscle in MEGA7 (Kumar et al., 2016). A rooted phylogenic tree of the selected PLA peptides in the four species, using WRKY2 (AT5G56270) in Arabidopsis as the root, was generated with fasttress (Price et al., 2009) and visualized in MEGA7 to identify the AtPLA homologs in B. napus. In addition we have identified pylogenic relationship between all the available Arabidopsis PLAs and Brassica species. The expression profiles of the BnPLAs were examined in two published transcriptomic datasets generated from B. napus infected by P. brassicae (Galindo-González et al., 2020; Zhou et al., 2020b; Supplementary Figure 2).
Results
We identified homozygous T-DNA insertion knockout/knockdown mutants representing each PLA subtype, pla1-iγ1 (WiscDsLox434H9), pla1-iiα (SALK_086894C), pla1-iγ3 (SALK_012432C), spla2-α (SALK_099415C), ppla-iiiβ (SALK_057212C), pla1-iii (SALK_033291), and ppla-iiiδ (SALK_029470), using two gene-specific primers and the T-DNA left border primers (Alonso et al., 2003; Figure 1A). Insertion sites of pla1-iγ, pla1-iiα, pla1-iγ3, ppla-iiiβ, and pla1-iii were located in the exon regions of each PLA genes, while ppla-iiiδ contained its mutant sites within the promoter region (Figure 1B). Furthermore, while the spla2-α mutant lines were reported to have multiple insertion sites within the promoter and exon regions of the gene, the exact map location was not indicated on the TAIR web site (Seo et al., 2008; TAIR-ABRC). Furthermore, this T-DNA mutant line (SALK_099415C) previously been confirmed as a complete gene knockout line as no detectable mRNA was observed (Seo et al., 2008).
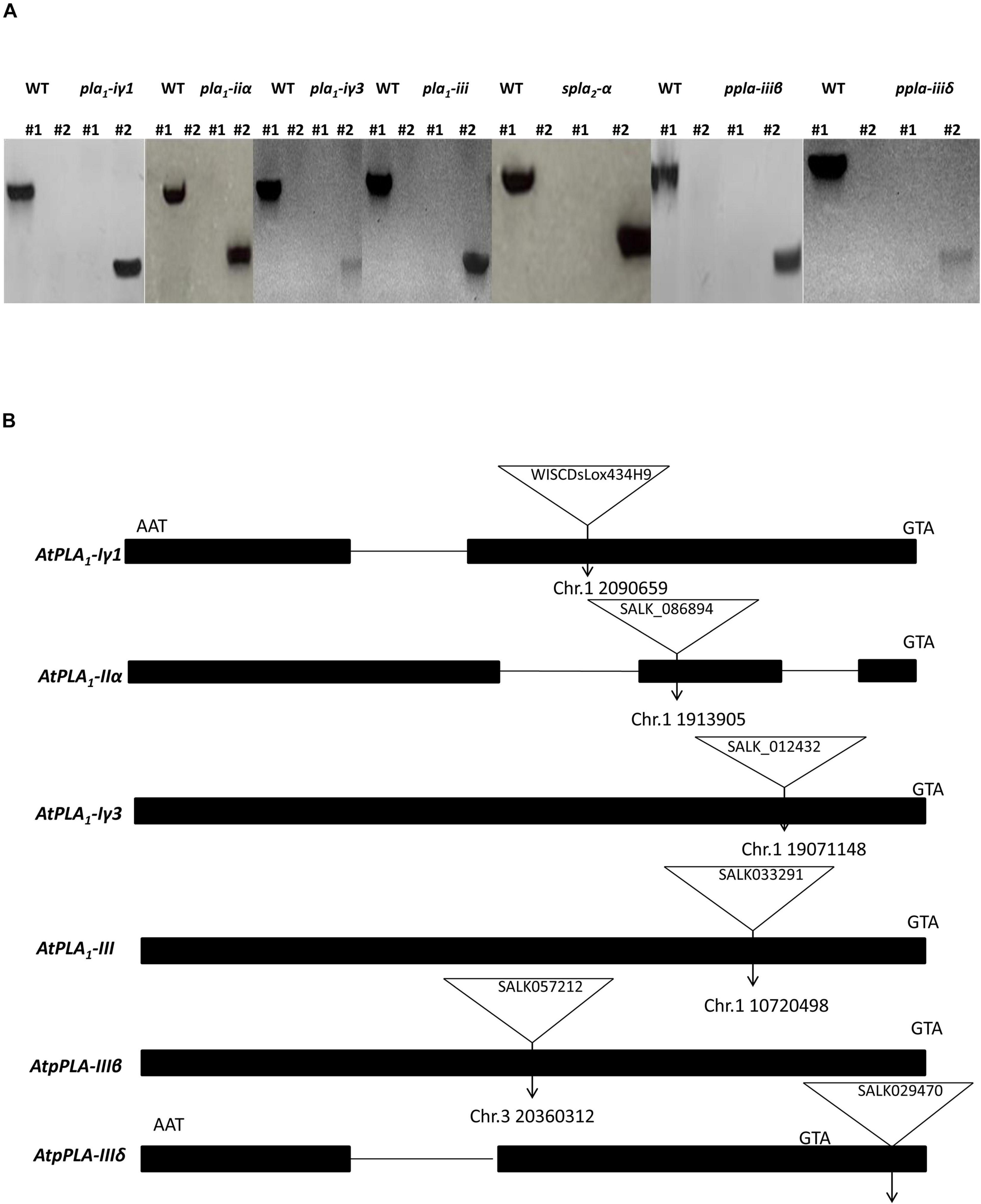
Figure 1. Identification and characterization of Arabidopsis T-DNA insertion lines. (A) Identification of T-DNA insertion lines by PCR. LP and RP primers are located at the left and right sides of T-DNA insertion, respectively. LBb1.3 and P745 are used as flanking primers for SALK and WiSC lines, respectively. In each gel picture #1 is the PCR sample with primers LP + RP and #2 is the PCR sample with primers LB1.3/P745 + RP. (B) Schematic diagrams of the positions of T-DNA insertions in PLA single mutant alleles. Start and stop codons are indicated.
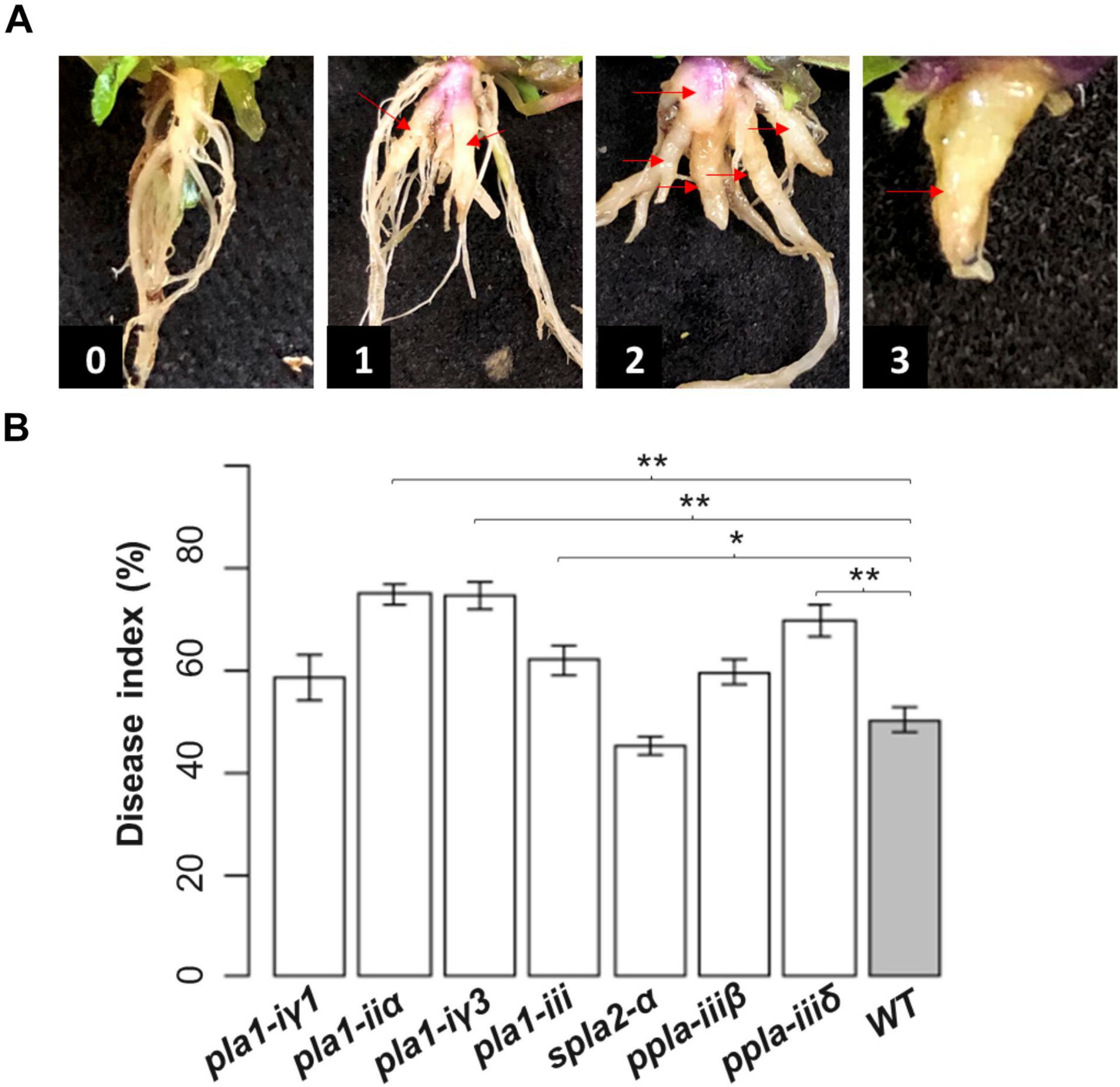
Figure 2. Evaluation of reaction of seven pla mutants and wild-type (Columbia) Arabidopsis to inoculation with Plasmodiophora brassicae pathotype 3H. (A) The rating scale used to evaluate clubroot disease severity, where: 0 = no galls, 1 = galls mainly on the lateral roots, 2 = obvious galls on both the primary and lateral roots with a moderately reduced root system, and 3 = large galls on the primary roots with a significantly reduced root system. The individual severity ratings were used to calculate a disease index. (B) The disease index on the seven pla mutants and the wild-type Columbia 26 days after inoculation with P. brassicae. Values are means ± SE of six independent replicates with 24 plants per replicate. The asterisks indicate significant differences at p < 0.05 (*) and p < 0.01 (**) compared with the wild-type, based on the Dunnett’s test in R.
To validate the roles of the selected PLAs in the host response to clubroot, we first evaluated the disease severity of the seven homozygous Arabidopsis pla mutants and the wild-type Arabidopsis, following inoculation with P. brassicae pathotype 3H. Four of the mutants (pla1-iiα, pla1-iγ3, pla1-iii, and ppla-iiiδ) had significantly greater DIs than the wild-type (Figure 2B), indicating increased susceptibility to P. brassicae pathotype 3H. Among these, the pla1-iiα and pla1-iγ3 appeared the most susceptible, with DIs of 75.0 and 74.7%, respectively, followed by ppla-iiiδ (69.8%) and pla1-iii (62.1%). The reactions for the remaining mutants were similar to the wild-type, which developed an DI of 50.4%.
We identified homologs of the seven PLAs in B. napus by BLAST and phylogenic analysis. We first aligned the peptide sequences of seven AtPLA to the three Brassica species (B. rapa, B. oleracea, and B. napus) using BLASTP (see text footnote 2) to select potential PLA homologs with high identity (%) and coverage (%) and low E-values. After that, we performed phylogenetic analysis using Mega7 (Kumar et al., 2016) and fasttree (Price et al., 2009), and filtered Brassica PLAs that were not properly clustered with the targeted AtPLAs. In total, 21 homologs of BnPLAs were identified in B. napus, which were evolved from either B. rapa or B. oleracea (Figure 3), indicating duplication and loss of PLAs during the divergence of Arabidopsis and Brassica, but a conserved evolution of PLAs in Brassica. All the BnPLAs had >80% identity and coverage, and E-values near 0, to each of corresponding AtPLA (Table 1).
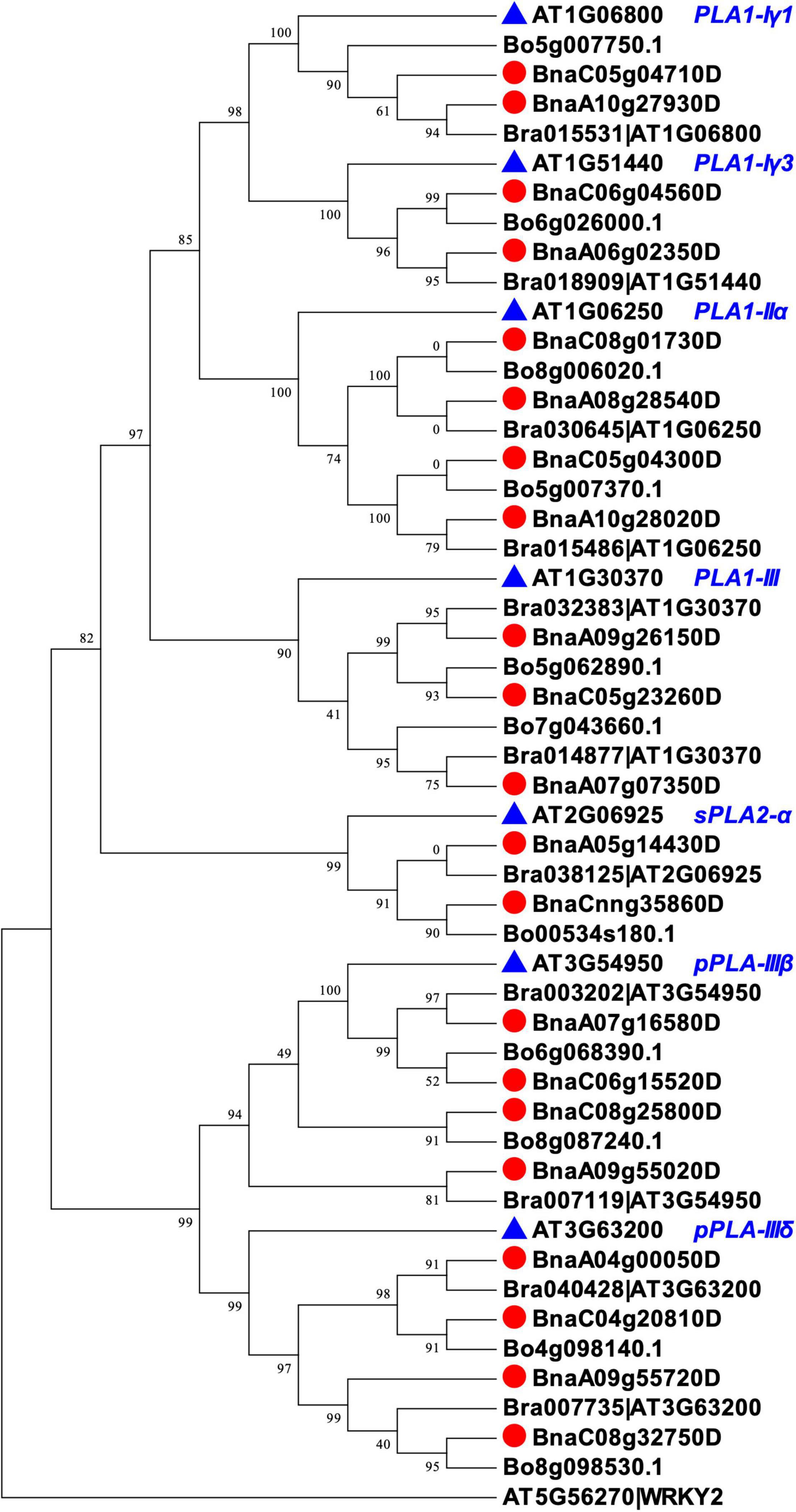
Figure 3. Phylogenic relationships of PLAs in Arabidopsis and Brassica species (B. rapa, B. oleracea, and B. napus). The phylogenic tree was constructed with fasttree using PLA peptide sequences aligned by MUSCEL in Mega7. Arabidopsis WKRY2 (AT5G56270) was used as the root. Each PLA encoding gene in B. rapa was followed with an Arabidopsis gene ID in the genome annotation, which was kept in the phylogenic tree to confirm the clustering of the PLAs.
Discussion
Plant phospholipids are major structural components of biological membranes (Adigun et al., 2021). Accumulating evidence indicates that phospholipid-based signal transduction and phospholipid-derived products mediated by PLAs are important for plant growth, development, and responses to abiotic and biotic stress (Ryu, 2004; Grienenberger et al., 2010; Canonne et al., 2011; Chen et al., 2013; Adigun et al., 2021). Upon perception of invading pathogens, PLAs can be activated to hydrolyze phospholipids and galactolipids at their sn-1 or sn-2 positions to generate free fatty acids and lysophospholipids. These function as precursors of second messengers to mediate downstream defense reactions, including the production of oxylipins and JA, and stimulation or inhibition of key signaling enzymes (e.g., MAKP, protein kinase and H+-ATPase) (Ryu, 2004; Canonne et al., 2011). PLAs are also involved in plant growth and development by auxin-related cell elongation in plants (Ryu, 2004), which might be associated with root galling in P. brassicae-infected plants. In addition, a recent expression analysis of drought-related lipid genes in soybean (Glycine max) suggested the involvement of PLAs (PLA1-II and -III, sPLA, pPLA-I, -II, and -III) in response to water deficit (Ferreira, 2017). Since infection by P. brassicae interferes with root water uptake and leads to drought stress symptoms in the aboveground canopy (Ludwig-Müller, 2009), PLAs might be involved in a clubroot-related drought response in plants. While little is known regarding the role of PLAs in the host response to clubroot, recently published transcriptomic profiles from P. brassicae-infected B. napus allowed us to identify PLAs showing differential expression following inoculation (Fu et al., 2019; Galindo-González et al., 2020; Zhou et al., 2020b). Therefore, study of the role(s) of PLAs in the host response to clubroot could provide insights into the utility of PLAs in clubroot resistance breeding.
In plants, PLAs are classified into three main subtypes based on their catalysis site: phospholipase A1 (PLA1), secretory phospholipase A2 (sPLA2), and patatin-like PLA (pPLA). Arabidopsis has about 30 PLAs, including 12 PLA1s, four sPLA2s, and 13 pPLAs (Eastmond, 2006; Chen et al., 2013; Li and Wang, 2014). Furthermore, in canola, each PLA has several homologs (Supplementary Figure 1) and it is difficult to study their roles in clubroot resistance directly given the complexity of the canola genome. To improve understanding of the roles of PLAs in clubroot resistance and to help to identify candidate PLA genes in canola contributing to resistance, we selected seven pla knockout/knockdown Arabidopsis mutants and compared their susceptibilities with the wild-type plants, following inoculation with P. brassicae pathotype 3H. This is a common pathotype found across much of the canola producing regions of western Canada, and is highly virulent on canola that does not carry any clubroot resistance (Strelkov et al., 2018; Hollman et al., 2021).
Phospholipase A1s specifically hydrolyze the sn-1 position in phospholipids. They further divided into groups I, II, and III PLA1s and phosphatidic acid-specific PLA1 (PA-PLA1) (Kim and Ryu, 2014). Class I, II, and III PLA1s are predicted to localize to the chloroplast, cytosol and mitochondria, respectively, based on the presence of N-terminal stretches (Ryu, 2004; Seo et al., 2009; Chen et al., 2011). While there is no clear evidence of PLA1s in plant immunity, expression of several genes (e.g., PLA1-Iγ1, PLA1-Iγ2, and PLA1-III) encoding PLA1 proteins was induced in Arabidopsis response to pathogen attack (e.g., B. cinerea and P. syringae) (Grienenberger et al., 2010). In addition, increased expression of several PLA1s (VviPLA1-Iβ1, VviPLA1-Iγ1, and VviPLA1-IIδ) in grapevine (Vitis vinifera L.) was found within 24 h following inoculation with the biotrophic oomycete Plasmopara viticola (Laureano, 2018). Three of the four pla1 mutants (pla1-iiα, pla1-iγ3, and pla1-iii) in the current were more susceptible to P. brassicae than the wild-type Arabidopsis, indicating the importance of PLA1 in host defense to this pathogen.
Class I PLA1s are involved in JA production (Wasternack and Song, 2017), including the two genes PLA1-Iγ1 and PLA1-Iγ3 in this study (Ellinger et al., 2010). However, the genes may mediate different pathways for JA production, with PLA1-Iγ1 contributing to wound-induced JA production and PLA1-Iγ3 contributing to JA production under non-wounded conditions (Ellinger et al., 2010). Transcription of PLA1-Iγ1 in Arabidopsis was induced upon B. cinerea and P. syringae inoculation (Grienenberger et al., 2010), and expression of PLA1-Iγ1 in grapevine was upregulated within 24 h of inoculation with P. viticola (Laureano, 2018). A homolog of AtPLA1-Iγ1 in B. napus was downregulated (BnaC05g04710D) during the late infection stage in a compatible (susceptible) interaction with P. brassicae pathotype 3A (Zhou et al., 2020b), while the same gene was upregulated during early infection of a resistant B. napus genotype by pathotype 5X (Galindo-González et al., 2020; Supplementary Figure 2). Galindo-González et al. (2020) also identified another BnPLA1-Iγ1 (BnaA10g27920D), which was upregulated in both susceptible and resistant B. napus, but with greater upregulation in the resistant reaction. In this study, pla1-iγ1 and pla1-iγ3 mutants showed greater susceptibility than the wild-type, but only pla1-iγ3 showed a significant difference, suggesting that PLA1-Iγ3 but not PLA1-Iγ1 plays an important role in the response of Arabidopsis to P. brassicae. Thus, homologs of PLA1-Iγ3 in canola may contribute to enhanced clubroot resistance. Given the fact that BnPLA1-Iγ1 (BnaC05g04710D) was downregulated in a clubroot susceptible reaction and upregulated in a resistant reaction, this gene might also be associated with canola defense to clubroot.
There are four class II PLA1s in Arabidopsis (PLA1-IIα, -IIβ, -IIγ, and -IIδ) (Chen et al., 2013) but little is known regarding the role of class II PLA1s in response to pathogens. Nonetheless, the expression of PLA1-IIδ increased in grapevine following challenge by P. viticola (Laureano, 2018), suggesting the involvement of class II PLA1s in the defense reaction. Our results showed that the pla1-iiα Arabidopsis mutant was more susceptible to clubroot than the wild-type, suggesting a role for PLA1-IIα in the host response to P. brassicae. In addition, a homolog of this gene in B. napus (BnaA10g28020D) was downregulated during the late stage of susceptible reaction to clubroot (Zhou et al., 2020b), which is consistent with the present results. Since a PLA1-II (GmPLA1-IIγa) in soybean was upregulated under drought conditions (Ferreira, 2017), PLA1-IIα-mediated clubroot defense might include mitigating water stress. Collectively, these results suggest the importance of PLA1-IIα in clubroot resistance, and the gene BnaA10g28020D might be a good candidate for further characterization of its role.
Only one class III PLA1 is found in Arabidopsis (PLA1-III) (Chen et al., 2013). PLA1-III is important for plant development and stress tolerance. The expression of PLA1-III was modulated in Arabidopsis upon B. cinerea (Grienenberger et al., 2010) challenge, respectively. Expression of an AtPLA1-III homolog in CR Chinese cabbage (B. rapa) was induced (BraA07g010560.3C) during the early infection stage (4 dai) following inoculation with a virulent pathotype of P. brassicae (Fu et al., 2019). A homolog of AtPLA1-III was downregulated in B. napus (BnaC05g23260D) during the late stage of the clubroot-susceptible response (Galindo-González et al., 2020; Zhou et al., 2020b; Supplementary Figure 2), while two other BnPLA1-III homologs (BnaA07g07350D and BnaA09g26150D) were downregulated in both resistant and susceptible reactions, with greater downregulation in the latter (Galindo-González et al., 2020). These findings are consistent with our results, since the pla1-iii mutant was more susceptible to clubroot than the wild-type, indicating the importance of PLA1-III in plant defense to clubroot. Similarly, PLA1-III in soybean was regulated under drought conditions, with a downregulation in moderate and severe drought but an upregulation in extreme drought (Ferreira, 2017), further suggestion a role in mitigating drought stress.
sPLA2s, which specifically hydrolyze the sn-2 position of phospholipids, are the only PLA2s identified in plants (Kim and Ryu, 2014). Only four AtsPLA isoforms are found in Arabidopsis (sPLA2-α, -β, -γ, and -δ), with sPLA2-α suggested to play a negative role in defense to pathogen attack by subcellular localizing to the cell nucleus and physically binding to MYB30 to repress defense in Arabidopsis (Froidurea et al., 2010; Canonne et al., 2011; Kim and Ryu, 2014). sPLA2 have been suggested to be involved in the auxin signaling pathway, which promotes cell elongation (Ryu, 2004). For instance, overexpression of AtsPLA2-β in Arabidopsis resulted in elongation of the leaf petioles and inflorescence stems, while silencing of the gene resulted in the opposite phenotype (Lee et al., 2003). Expression of BnsPLA2-α was increased in susceptible hosts and decreased in resistant hosts, following inoculation with P. brassicae (Galindo-González et al., 2020; Zhou et al., 2020b; Supplementary Figure 2), suggesting their negative roles in clubroot resistance. Considering that auxin is a crucial hormone for gall development in roots of P. brassicae-infected plants (Jahn et al., 2013), sPLA2-α might be involved in auxin-related pathways to promote gall development. Nonetheless, in this study, clubroot severity on the atspla2-α mutant was not significantly different from that of the wild-type.
Patatin-like PLAs, which hydrolyze phospholipids and galactolipids at both the sn-1 and sn-2 positions (Chen et al., 2011), are involved in defense signaling in plants upon infection by many pathogens. A member of pPLAs in pepper (Capsicum annuum), CaPLP1, plays a positive role in plant innate immunity (Kim et al., 2014). Silencing of CaPLP1 increased plant susceptibility to the bacterium Xanthomonas campestris pv. vesicatoria and was associated with compromised defense responses, including reactive oxygen species production, hypersensitive cell death and expression of a SA-dependent gene CaPR1. Overexpression of this gene in Arabidopsis increased plant resistance to P. syringae and Hyaloperonospora arabidopsidis, which was associated with an enhanced oxidative burst, expression of SA- and JA-dependent genes, and cell death (Kim et al., 2014). Another pPLA, pPLA-IIα/PLP2, which contributes JA- and oxylipin-mediated cell death, positively regulated Arabidopsis resistance to the obligate parasite cucumber mosaic virus and the fungus V. dahliae by inducing oxylipins and JA biosynthesis, respectively. This gene, however, negatively regulated resistance to B. cinerea and P. syringae in Arabidopsis (la Camera et al., 2005, 2009; Zhu et al., 2021). In addition, the expression of several pPLAs (VvipPLA-I, VvipPLA-IIβ, VvipPLA-IIδ2, and VvipPLA-IIIβ) was induced in grapevine after inoculation with P. viticola (Laureano, 2018). Transcriptomic studies of B. napus inoculated with P. brassicae pathotype 3A indicated that expression of two copies of BnPLP2 increased earlier during the infection process, but not at later stages, in both the resistant and susceptible reactions (Zhou et al., 2020b). When B. napus was inoculated with P. brassicae pathotype 5X, expression patterns of the two genes were generally similar in both the resistant and susceptible reactions, except that the genes were also upregulated in the susceptible host during the late infection stage (Galindo-González et al., 2020; Supplementary Figure 2), suggesting a negative role of this genes in clubroot resistance. In addition, pPLAs are involved in the regulation of auxin-related responses. A study investing the role of pPLAs in the regulation of auxin responses found delayed upregulation of auxin-responsive gene expression in all nine ppla mutants studied (Labusch et al., 2013). The authors also demonstrated that knocking out/down pPLA-IIIδ in Arabidopsis affected auxin-related phenotypes, including shortened main roots and more lateral roots, and knocking out of pPLA-IIIβ resulted in slightly longer roots and hypocotyls (Labusch et al., 2013).
Other pPLA enzymes, including PLAIVA/PLP1, are also positively associated with plant auxin signaling to modulate root development (Rietz et al., 2010). In addition, pPLAs are involved in response to water deficit in plants (Ferreira, 2017). Therefore, pPLAs could regulate the host response to clubroot via their involvement in defense pathways and drought- and auxin-related pathways. In this study, both ppla-iiiβ and ppla-iiiδ appeared to develop more severe symptoms than the wild-type Arabidopsis, but DI was significantly more severe only for ppla-iiiδ (Figure 2B), indicating a positive effect of pPLA-IIIδ in clubroot resistance. In constrast, B. napus inoculated with pathotype 3A or 5X of P. brassicae showed reduced expression of BnpPLA-IIIδ in both the resistant and susceptible reactions during early infection, and was downregulated in only the resistant hosts during late infection (Galindo-González et al., 2020; Zhou et al., 2020b; Supplementary Figure 2). These contrasting findings may reflect host-pathotype specific interactions, and suggest that functional validation of AtpPLA-IIIδ in response to additional P. brassicae pathotypes may improve understanding of its role in host defense.
Conclusion
To the best of our knowledge, this is the first study investigating the host response of PLAs to clubroot. We selected seven Arabidopsis atpla knockout/knockdown mutants, and identified four mutants (pla1-iiα, pla1-iγ3, pla1-iii, ppla-iiiδ) that were more susceptible to P. brassicae than the wild-type plants. These results indicate that PLAs may play positive roles in host defense to clubroot. We identified homologs of the seven PLAs in B. napus and its parental species (B. rapa and B. oleracea) and explored their expression patterns following P. brassicae inoculation using available transcriptomic datasets; this information was used to select candidate genes that can be further characterized in canola. The pathways mediated by these PLAs are unknown, but could be related to JA biosynthesis, and auxin- and drought-responses. However, additional studies will be needed with more PLA mutants in Arabidopsis to confirm their role in clubroot resistance and elucidate the precise mechanism(s) of each PLA gene.
Data Availability Statement
The original contributions presented in the study are included in the article/Supplementary Material, further inquiries can be directed to the corresponding author.
Author Contributions
SS, S-FH, and GC conceived, designed and supervised the experiment, and edited the manuscript. QZ and KJ performed the experiments and wrote the first draft of the manuscript. All authors contributed to the preparation of the final article.
Funding
Financial support from Results Driven Agriculture Research (2019F135R) is gratefully acknowledged.
Conflict of Interest
The authors declare that the research was conducted in the absence of any commercial or financial relationships that could be construed as a potential conflict of interest.
Publisher’s Note
All claims expressed in this article are solely those of the authors and do not necessarily represent those of their affiliated organizations, or those of the publisher, the editors and the reviewers. Any product that may be evaluated in this article, or claim that may be made by its manufacturer, is not guaranteed or endorsed by the publisher.
Acknowledgments
We thank Victor Manolii and Ileana Strelkov for their assistance with this work.
Supplementary Material
The Supplementary Material for this article can be found online at: https://www.frontiersin.org/articles/10.3389/fpls.2022.799142/full#supplementary-material
Supplementary Figure 1 | Phylogenic relationships of the 29 Arabidopsis PLAs and their homologs in Brassica species (B. rapa, B. oleracea, and B. napus). The homologs were identified by blasting Arabidopsis peptide sequences against Brassica peptide sequences using the BLASTP tool (E-value ≤1e–10, coverage >60%, identity >60%, and the top 20 hits). The phylogenic tree was constructed with fasttree using PLA peptide sequences aligned by MUSCEL in Mega7. Arabidopsis WKRY2 (AT5G56270) was used as the root. Each PLA encoding gene in B. rapa was followed with an Arabidopsis gene ID in the genome annotation, which was kept in the phylogenic tree to confirm the clustering of the PLAs.
Supplementary Figure 2 | Expression changes of BnPLAs in resistant and susceptible Brassica napus following Plasmodiophora brassicae inoculation. (A) The expression changes of plants in response to P. brassicae pathotype 3A (Zhou et al., 2020b). (B) The expression changes of plants in response to P. brassicae pathotype 5X (Galindo-González et al., 2020).
Footnotes
References
Adigun, O. A., Nadeem, M., Pham, T. H., Jewell, L. E., Cheema, M., and Thomas, R. (2021). Recent advances in biochemical, molecular and physiological aspects of membrane lipid derivatives in plant pathology. Plant Cell Environ. 44, 1–16. doi: 10.1111/pce.13904
Alonso, J. M., Stepanova, A. N., Leisse, T. J., Kim, C. J., Chen, H., Shinn, P., et al. (2003). Genome-wide insertional mutagenesis of Arabidopsis thaliana. Science 301, 653–657. doi: 10.1126/science.1086391
Canonne, J., Froidure-Nicolas, S., and Rivas, S. (2011). Phospholipases in action during plant defense signaling. Plant Signal. Behav. 6, 13–18. doi: 10.4161/psb.6.1.14037
Chalhoub, B., Denoeud, F., Liu, S., Parkin, I. A. P., Tang, H., Wang, X., et al. (2014). Early allopolyploid evolution in the post-neolithic Brassica napus oilseed genome. Science 345, 950–953. doi: 10.1126/science.1253435
Chandra, S., Heinstein, P. F., and Low, P. S. (1996). Activation of phospholipase A by plant defense elicitors. Plant Physiol. 11, 979–986. doi: 10.1104/pp.110.3.979
Chen, G., Greer, M. S., and Weselake, R. J. (2013). Plant phospholipase A: advances in molecular biology, biochemistry, and cellular function. Biomol. Concepts 4, 527–532. doi: 10.1515/bmc-2013-0011
Chen, G., Snyder, C. L., Greer, M. S., and Weselake, R. J. (2011). Biology and biochemistry of plant phospholipases. Crit. Rev. Plant Sci. 30, 239–258. doi: 10.1080/07352689.2011.572033
Cheng, F., Wu, J., and Wang, X. (2014). Genome triplication drove the diversification of Brassica plants. Hortic. Res. 1:14024. doi: 10.1038/hortres.2014.24
Eastmond, P. J. (2006). Sugar-dependent1 encodes a patatin domain triacylglycerol lipase that initiates storage oil breakdown in germinating Arabidopsis seeds. Plant Cell 18, 665–675. doi: 10.1105/tpc.105.040543
Ellinger, D., Stingl, N., Kubigsteltig, I. I., Bals, T., Juenger, M., Pollmann, S., et al. (2010). DONGLE and DEFECTIVE IN ANTHER DEHISCENCE1 lipases are not essential for wound- and pathogen-induced jasmonate biosynthesis: redundant lipases contribute to jasmonate formation. Plant Physiol. 153, 114–127. doi: 10.1104/pp.110.155093
Ferreira, D. (2017). Identification and Real Time PCR Expression Analysis of Drought-Related Lipid Metabolism Genes in Soybean. Ph.D thesis, Portugal: Universidade de Lisboa.
Froidurea, S., Canonnea, J., Daniela, X., Jauneaub, A., Brièrec, C., Robya, D., et al. (2010). AtsPLA2-α nuclear relocalization by the Arabidopsis transcription factor AtMYB30 leads to repression of the plant defense response. Proc. Natl. Acad. Sci. U.S.A. 107, 15281–15286. doi: 10.1073/pnas.1009056107
Fu, P., Piao, Y., Zhan, Z., Zhao, Y., Pang, W., Li, X., et al. (2019). Transcriptome profile of Brassica rapa L. reveals the involvement of jasmonic acid, ethylene, and brassinosteroid signaling pathways in clubroot resistance. Agronomy 9:589. doi: 10.3390/agronomy9100589
Galindo-González, L., Manolii, V., Hwang, S.-F., and Strelkov, S. E. (2020). Response of Brassica napus to Plasmodiophora brassicae involves salicylic acid-mediated immunity: an RNA-seq-based study. Front. Plant Sci. 11:1025. doi: 10.3389/fpls.2020.01025
Grienenberger, E., Geoffroy, P., Mutterer, J., Legrand, M., and Heitz, T. (2010). The interplay of lipid acyl hydrolases in inducible plant defense. Plant. Signal. Behav. 5, 1181–1186. doi: 10.4161/psb.5.10.12800
Hollman, K. B., Hwang, S. F., Manolii, V. P., and Strelkov, S. E. (2021). Pathotypes of Plasmodiophora brassicae collected from clubroot resistant canola (Brassica napus L.) cultivars in western Canada in 2017-2018. Can. J. Plant Pathol. 43, 622–630. doi: 10.1080/07060661.2020.1851893
Hwang, S. F., Ahmed, H. U., Zhou, Q., Fu, H., Turnbull, G. D., Fredua-Agyeman, R., et al. (2019). Influence of resistant cultivars and crop intervals on clubroot of canola. Can. J. Plant Pathol. 99, 862–872. doi: 10.1139/cjps-2019-0018
Hwang, S. F., Howard, R. J., Strelkov, S. E., Gossen, B. D., and Peng, G. (2014). Management of clubroot (Plasmodiophora brassicae) on canola (Brassica napus) in western Canada. Can. J. Plant Pathol. 36, 49–65. doi: 10.1080/07060661.2013.863806
Hwang, S. F., Strelkov, S. E., Feng, J., Gossen, B. D., and Howard, R. J. (2012). Plasmodiophora brassicae: a review of an emerging pathogen of the Canadian canola (Brassica napus) crop. Mol. Plant Pathol. 13, 105–113. doi: 10.1111/j.1364-3703.2011.00729.x
Iqbal, S., Ali, U., Fadlalla, T., Li, Q., Liu, H., Lu, S., et al. (2020). Genome wide characterization of phospholipase A & C families and pattern of lysolipids and diacylglycerol changes under abiotic stresses in Brassica napus L. Plant. Physiol. Biochem. 147, 101–112. doi: 10.1016/j.plaphy.2019.12.017
Irani, S., Trost, B., Waldner, M., Nayidu, N., Tu, J., Kusalik, A. J., et al. (2018). Transcriptome analysis of response to Plasmodiophora brassicae infection in the Arabidopsis shoot and root. BMC Genomics. 19:23. doi: 10.1186/s12864-017-4426-7
Jahn, L., Mucha, S., Bergmann, S., Horn, C., Staswick, P., Steffens, B., et al. (2013). The clubroot pathogen (Plasmodiophora brassicae) influences auxin signaling to regulate auxin homeostasis in Arabidopsis. Plants 2, 726–749. doi: 10.3390/plants2040726
Kim, D. S., Jeun, Y., and Hwang, B. K. (2014). The pepper patatin-like phospholipase CaPLP1 functions in plant cell death and defense signaling. Plant Mol. Biol. 84, 329–344. doi: 10.1007/s11103-013-0137-x
Kim, H. J., and Ryu, S. B. (2014). “sPLA2 and PLA1: secretory phospholipase A2 and phospholipase A1 in plants,” in Phospholipases, ed. X. Wang (Berlin: Springer), 109–118. doi: 10.1007/978-3-642-42011-5_6
Kumar, S., Stecher, G., and Tamura, K. (2016). MEGA7: molecular evolutionary genetics analysis version 7.0 for bigger datasets. Mol. Biol. Evol. 33, 1870–1874. doi: 10.1093/molbev/msw054
la Camera, S., Balagué, C., Göbel, C., Geoffroy, P., Legrand, M., Feussner, I., et al. (2009). The Arabidopsis patatin-like protein 2 (PLP2) plays an essential role in cell death execution and differentially affects biosynthesis of oxylipins and resistance to pathogens. Mol. Plant Microbe Interact. 22, 469–481. doi: 10.1094/MPMI-22-4-0469
la Camera, S., Geoffroy, P., Samaha, H., Ndiaye, A., Rahim, G., Legrand, M., et al. (2005). A pathogen-inducible patatin-like lipid acyl hydrolase facilitates fungal and bacterial host colonization in Arabidopsis. Plant J. 44, 810–825. doi: 10.1111/j.1365-313X.2005.02578.x
Labusch, C., Shishova, M., Effendi, Y., Li, M., Wang, X., and Scherer, G. F. E. (2013). Patterns and timing in expression of early auxin-induced genes imply involvement of phospholipases a (pPLAS) in the regulation of auxin responses. Mol. Plant 6, 1473–1486. doi: 10.1093/mp/sst053
Laureano, G. M. M. P. C. (2018). Fatty Acids and Lipid Signaling in Grapevine Resistance to Plasmopara Viticola. Available online at: https://repositorio.ul.pt/handle/10451/32692 (accessed on May 24, 2021).
Lee, H. Y., Bahn, S. C., Kang, Y.-M., Lee, K. H., Kim, H. J., Noh, E. K., et al. (2003). Secretory low molecular weight phospholipase A2 plays important roles in cell elongation and shoot gravitropism in Arabidopsis. Plant Cell. 15, 1990–2002. doi: 10.1105/tpc.014423
Li, M., and Wang, X. (2014). “pPLA: patatin-related phospholipase As with multiple biological functions,” in Phospholipases in Plant Signaling, ed. X. Wang (Berlin: Springer), 93–108.
Ludwig-Müller, J. (2009). Plant defence – what can we learn from clubroots? Australas. Plant Pathol. 38, 318–324. doi: 10.1071/AP09020
Ludwig-Müller, J., Auer, S., Jülke, S., and Marschollek, S. (2017). “Manipulation of auxin and cytokinin balance during the Plasmodiophora brassicae–Arabidopsis thaliana interaction,” in Auxins and Cytokinins in Plant Biology,.eds. T. Dandekar and M. Naseem (New York, NY: Humana Press), 41–60. doi: 10.1007/978-1-4939-6831-2_3
Pageau, D., Lajeunesse, J., and Lafond, J. (2006). Impact de l’hernie des crucifères Plasmodiophora brassicae sur la productivité et la qualité du canola. Can. J. Plant Pathol. 28, 137–143. doi: 10.1080/07060660609507280
Price, M. N., Dehal, P. S., and Arkin, A. P. (2009). Fasttree: computing large minimum evolution trees with profiles instead of a distance matrix. Mol. Biol. Evol. 26, 1641–1650. doi: 10.1093/molbev/msp077
Rietz, S., Dermendjiev, G., Oppermann, E., Tafesse, F. G., Effendi, Y., Holk, A., et al. (2010). Roles of Arabidopsis patatin-related phospholipases a in root development are related to auxin responses and phosphate deficiency. Mol.Plant. 3, 524–538. doi: 10.1093/mp/ssp109
Ruelland, E., Kravets, V., Derevyanchuk, M., Martinec, J., Zachowski, A., and Pokotylo, I. (2015). Role of phospholipid signalling in plant environmental responses. Environ. Exp. Bot. 114, 129–143. doi: 10.1016/j.envexpbot.2014.08.009
Ryu, S. B. (2004). Phospholipid-derived signaling mediated by phospholipase A in plants. Trends Plant Sci. 9, 229–235. doi: 10.1016/j.tplants.2004.03.004
Seo, J., Lee, H. Y., Choi, H., Choi, Y., Lee, Y., Kim, Y.-W., et al. (2008). Phospholipase A2β mediates light-induced stomatal opening in Arabidopsis. J. Exp. Bot. 59, 3587–3594. doi: 10.1093/JXB/ERN208
Seo, Y. S., Kim, E. Y., Kim, J. H., and Kim, W. T. (2009). Enzymatic characterization of class I DAD1-like acylhydrolase members targeted to chloroplast in Arabidopsis. FEBS Lett. 583, 2301–2307. doi: 10.1016/j.febslet.2009.06.021
Strelkov, S. E., Hwang, S.-F., Manolii, V. P., Cao, T., and Feindel, D. (2016). Emergence of new virulence phenotypes of Plasmodiophora brassicae on canola (Brassica napus) in Alberta. Can. Eur. J. Plant Pathol. 145, 517–529. doi: 10.1007/s10658-016-0888-8
Strelkov, S. E., Hwang, S.-F., Manolii, V. P., Cao, T., Fredua-Agyeman, R., Harding, M. W., et al. (2018). Virulence and pathotype classification of Plasmodiophora brassicae populations collected from clubroot resistant canola (Brassica napus) in Canada. Can. J. Plant Pathol. 40, 284–298. doi: 10.1080/07060661.2018.1459851
Strelkov, S. E., Tewari, J. P., and Smith-Degenhardt, E. (2006). Characterization of Plasmodiophora brassicae populations from Alberta. Can. J. Plant Pathol. 28, 467–474. doi: 10.1080/07060660609507321
Wasternack, C., and Song, S. (2017). Jasmonates: biosynthesis, metabolism, and signaling by proteins activating and repressing transcription. J. Exp. Bot. 68, 1303–1321. doi: 10.1093/JXB/ERW443
Yang, W., Devaiah, S. P., Pan, X., Isaac, G., Welti, R., and Wang, X. (2007). AtPLAI is an acyl hydrolase involved in basal jasmonic acid production and Arabidopsis resistance to Botrytis cinerea. J. Biol. Chem. 282, 18116–18128. doi: 10.1074/jbc.M700405200
Zhou, Q., Galindo-González, L., Hwang, S. F., and Strelkov, S. E. (2020a). Application of genomics and transcriptomics to accelerate development of clubroot resistant canola. Can. J. Plant Pathol. 43, 189–208. doi: 10.1080/07060661.2020.1794541
Zhou, Q., Galindo-Gonzalez, L., Manolii, V., Hwang, S. F., and Strelkov, S. E. (2020b). Comparative transcriptome analysis of rutabaga (Brassica napus) cultivars indicates activation of salicylic acid and ethylene-mediated defenses in response to Plasmodiophora brassicae. Int. J. Mol. Sci. 21:8381. doi: 10.3390/ijms21218381
Keywords: clubroot, Arabidopsis, canola, phospholipase A, PLA genes, resistance
Citation: Zhou Q, Jayawardhane KN, Strelkov SE, Hwang S-F and Chen G (2022) Identification of Arabidopsis Phospholipase A Mutants With Increased Susceptibility to Plasmodiophora brassicae. Front. Plant Sci. 13:799142. doi: 10.3389/fpls.2022.799142
Received: 21 October 2021; Accepted: 28 January 2022;
Published: 18 February 2022.
Edited by:
Chunyu Zhang, Huazhong Agricultural University, ChinaReviewed by:
Nannan Li, Southwest University, ChinaYueyun Hong, Huazhong Agricultural University, China
Copyright © 2022 Zhou, Jayawardhane, Strelkov, Hwang and Chen. This is an open-access article distributed under the terms of the Creative Commons Attribution License (CC BY). The use, distribution or reproduction in other forums is permitted, provided the original author(s) and the copyright owner(s) are credited and that the original publication in this journal is cited, in accordance with accepted academic practice. No use, distribution or reproduction is permitted which does not comply with these terms.
*Correspondence: Guanqun Chen, gc24@ualberta.ca
†These authors have contributed equally to this work