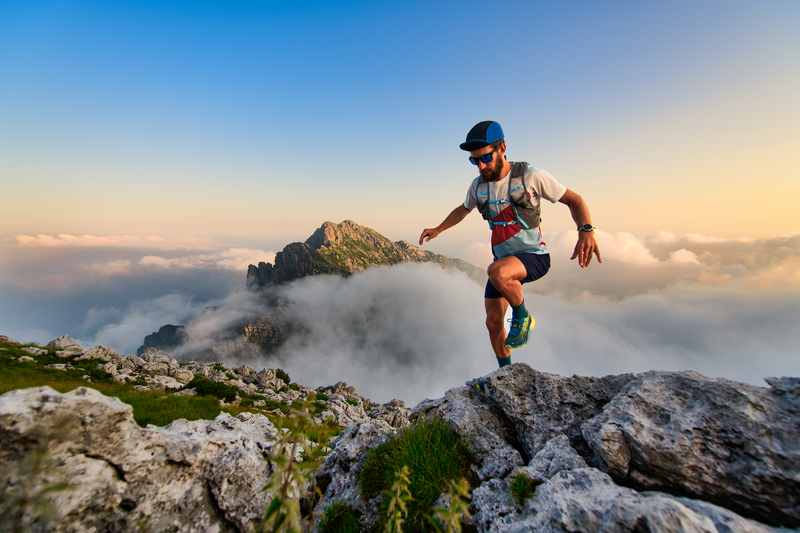
95% of researchers rate our articles as excellent or good
Learn more about the work of our research integrity team to safeguard the quality of each article we publish.
Find out more
REVIEW article
Front. Plant Sci. , 23 May 2022
Sec. Plant Abiotic Stress
Volume 13 - 2022 | https://doi.org/10.3389/fpls.2022.794782
This article is part of the Research Topic Bio-based Solutions for Sustainable Development of Agriculture View all 17 articles
Temperature is a significant parameter in agriculture since it controls seed germination and plant growth. Global warming has resulted in an irregular rise in temperature posing a serious threat to the agricultural production around the world. A slight increase in temperature acts as stress and exert an overall negative impact on different developmental stages including plant phenology, development, cellular activities, gene expression, anatomical features, the functional and structural orientation of leaves, twigs, roots, and shoots. These impacts ultimately decrease the biomass, affect reproductive process, decrease flowering and fruiting and significant yield losses. Plants have inherent mechanisms to cope with different stressors including heat which may vary depending upon the type of plant species, duration and degree of the heat stress. Plants initially adapt avoidance and then tolerance strategies to combat heat stress. The tolerance pathway involves ion transporter, osmoprotectants, antioxidants, heat shock protein which help the plants to survive under heat stress. To develop heat-tolerant plants using above-mentioned strategies requires a lot of time, expertise, and resources. On contrary, plant growth-promoting rhizobacteria (PGPRs) is a cost-effective, time-saving, and user-friendly approach to support and enhance agricultural production under a range of environmental conditions including stresses. PGPR produce and regulate various phytohormones, enzymes, and metabolites that help plant to maintain growth under heat stress. They form biofilm, decrease abscisic acid, stimulate root development, enhance heat shock proteins, deamination of ACC enzyme, and nutrient availability especially nitrogen and phosphorous. Despite extensive work done on plant heat stress tolerance in general, very few comprehensive reviews are available on the subject especially the role of microbes for plant heat tolerance. This article reviews the current studies on the retaliation, adaptation, and tolerance to heat stress at the cellular, organellar, and whole plant levels, explains different approaches, and sheds light on how microbes can help to induce heat stress tolerance in plants.
Crop growth is the function of temperature, soil fertility, and water status; all at optimum satisfaction. Temperature is the key determining factor of the vegetation of a particular region (Argosubekti, 2020) and a balance between optimal temperature range and time of incidence is important to regulate plant growth. A slight change/ deviation in the atmospheric temperature, can disrupt normal biological, structural, and molecular processes in plants, which ultimately results in stunted growth and reduced yield (Argosubekti, 2020). Heat stress is an increase in environmental temperature (above the upper limit of threshold) for a certain period that can cause irreversible plant damage. The level of crop adaptation and productivity affected by heat stress, however, depends upon the temperatures and the phase of plant development.
The onset of industrial revolution and massive mechanization has shown a gradual increase in CO2 emissions (30–150%) and greenhouse effect over the past 250 years (Friedlingstein et al., 2010) leading to a persistent rise in temperature during the last two centuries. The global land and ocean surface temperature increased by around 0.85°C from 1880 till 2012 and an annual rise of at least 0.2°C per decade is further anticipated (Change, 2014) while the greenhouse gases will further add 1.1–1.5°C to it. The National Aeronautics and Space Administration (NASA) released a comparative data of average global temperature from with a baseline of 1951–1980 (Figure 1) showing that average monthly temperature has increased >1°C during the past decade (2000–2020) and >1.5°C during the past 5 years (2015–2020). With 1.5–2.0°C change in the global mean temperature, the possibility of suffering from record-breaking, high-impact extreme climate disasters increase significantly, which ultimately cause a significant yield reduction and food supply chain around the globe (Chen et al., 2018; Kong et al., 2018). The IPCC (Intergovernmental Panel on Climate Change) reports that the world's major staple crops and food production have a great impact of climate change (Easterling et al., 2007; Porter et al., 2014) where an increase of one Celsius atmospheric temperature costs a decrease of 6% in crop yield (Asseng et al., 2015). In China, heat stress in the last decade resulted in rice yield losses of 5.18 million tons (Tian et al., 2009) while in South-East Asia upto 14%. In wheat, about 7 million ha in developing countries and 36 million ha in the temperate region had been affected by heat stress in 2001 (Reynolds et al., 2001) causing a yield reduction of 19 million tons (Lobell and Field, 2007) while USDA (United States Department of Agriculture) reported ≈5.5% yield reduction in wheat (Lobell et al., 2011).
Figure 1. Average global temperatures from 1850 to 2025 compared to a baseline average from 1850 to 1900 (A) source: Berkeley Earth (2019); and monthly global mean temperature 1851–2020 compared to 1850–1900 averages (B) source: Visual Capitalists (2019).
Agricultural and global food production is one of the most fragile sectors of society prone to climate uncertainty and changes (Meinke et al., 2006). Detailed multi-locational field analyses have shown that heat and drought stress are more closely linked. Increased heat imposes the drought stress hence, both stresses should be considered together rather than dealing individually. Zia et al. (2021) highlighted the impact of drought along with possible eco-friendly strategies to improve plant growth under drought stress, but the eco-friendly heat-stress management strategies is urgently needed. The plants are substantially affected by high day, low night or high air and surface temperatures. Each cultivar has a temperature optima (threshold) for a significant vegetative and reproductive growth (Zinn et al., 2010; Hatfield and Prueger, 2015) while growth ceases above or below the threshold (Kaushal et al., 2016). The lower threshold temperatures may vary from plant to plant, but is usually lower for most of the tropical, temperate, and cold season crops (Miller et al., 2001). Exposure to high temperature, e.g., 35°C significantly decreases pollen viability (Dupuis and Dumas, 1990), replication of amyloplast and cell division and growth rate ultimately decreasing the size and the total number of the grains (Commuri and Jones, 2001; Rangan et al., 2014). Table 1 describes in detail the growth destructive temperatures of different crops. The higher degree of heat stress (45°C or above) disrupts cellular homeostasis, induces extreme growth retardation, and severely affects the biological activity of proteins due to aggregation or misfolding rendering cells unable to defend themselves (Sarkar et al., 2013; Reddy et al., 2016). The deposition of improper-folded proteins may be permanent that alter the functioning of the cells.
The heat stress causes direct and indirect damage to multiple plant functions resulting in morphophysiological changes, hampering different growth phases and metabolic processes (Wu et al., 2018) and ultimately yield reduction (Mcclung and Davis, 2010; Grant et al., 2011). The detail the impact of heat stress on various plant functions at multiple levels has been described in Figure 2. The direct effect includes; protein denaturation and misfolding, increased membrane fluidity, the inactivation of chloroplast and mitochondrial enzymes, inhibition of protein synthesis and degradation, and loss of membrane integrity (Howarth, 2005). Indirect impact includes the changes in the pathogen behavior and disease pattern. Each pathogen has an optimum temperature for its replication and virulence (Velásquez et al., 2018), e.g., Globodera pallida nematode infect potato at 15°C (Jones et al., 2017), papaya ringspot virus (PRSV) infect papaya at 26–31°C (Mangrauthia et al., 2009). It has been predicted that many diseases will migrate into new geographical areas as temperatures rise, where they will encounter new hosts (Etterson and Shaw, 2001), cause severe and frequent epidemics (Ma et al., 2015), improve their survival under heat or desiccation, or become dormant for many years (Turkensteen et al., 2000; Ritchie et al., 2013). Change in the temperature also changes the pathogen behavior (Roberts et al., 2018), e.g., new pathogen strains adapted to high temperatures are being reported which are more active, more virulent, and widely transmitted worldwide (Hovmøller et al., 2008; Milus et al., 2009), e.g., Phytophthora infestans (cause late blight in potato and tomato) (Mariette et al., 2016) and Puccinia striiformis (rust fungus in wheat). Even pathogens can cause an outbreak by transient variations in temperature, e.g., soybean rust can be developed even after 1-h of exposure at 37°C, although, the optimum temperature for disease development is 12–25°C (Bonde et al., 2012).
Plant endophytic bacteria have a symbiotic relationship with their host plant, dwelling within it for the bulk of their life cycle and having the capacity to colonize the plant's interior tissues via penetrating the seed and root. These endophytic microbiomes fixed the atmospheric nitrogen, produced phytohormones, solubilized the inorganic phosphorus, zinc, potassium and calcium, produced exopolysaccharides and iron chelating siderophore compounds under heat stress condition which ultimately enhance the plant growth (Hakim et al., 2021). On the other hand, there are also some beneficial fungi, i.e., arbuscular mycorrhiza fungi (AMF) that is also help plants under heat stress condition. These AMF form symbiotic relationship with plant roots and improve photosynthetic process, increase nutrients uptake, secondary metabolites accumulation, regulate the oxidative defense system, and maintain the osmotic balance in plants (Begum et al., 2019). Plant genetic engineering and maker assisted selection breeding and multiple approaches have been published multiple reviews on heat stress and their management which are costly and time consuming. On the other hand, PGPR is cost-effective, eco-friendly, and ecologically safe solution to induce heat stress tolerance. So, current review will give a quick rundown of the current knowledge about the methods and pathways that PGPR use to promote tolerance in plants under heat stress. It will also discuss the heat stress response factors and genes and how PGPR regulate the expression of such genes to modify plant response under heat stress.
The response of plants toward heat stress varies with the degree and duration and the plant's developmental stage (Ruelland and Zachowski, 2010). These heat stress disorders may be recurrent or persistent in nature or both, therefore, plants have evolved different mechanisms to cope with them (Bäurle, 2016). The heat stress response (HSR) is an evolutionarily conserved mechanism that describes the plant's adaptation and induction of thermotolerance by either by activating the defense mechanisms to stop the disruption at the transcriptomic level in the cell or by the activation of heat shock proteins (HSPs), epigenetic pathways, and DNA methylation (Lämke and Bäurle, 2017). Table 2 describes few major effects of heat stress on plants.
Of all the growth phases, seed germination is the most adversely affected phase by heat stress, but, the response varies in different crops or even within varieties (Sita et al., 2017). The germination is significantly reduced above 45°C because of denaturation and embryonic cells (Cheng et al., 2009), along with severe impact on seedlings potency, the development of radicle and plumule, and seedling growth (Toh et al., 2008; Sita et al., 2017). At later stage, the effect varies with the time, length, and severity of stress (Fahad et al., 2016) but mainly heat stress decreases the cell water contents, the cell size, the plant size, growth and biomass, net assimilation rate (NAR) and relative growth rate (RGR) (Ashraf and Hafeez, 2004; Wahid and Close, 2007). Other visual symptoms include leaves and twigs scorching, growth inhibition, damaged leaves, early leaf senescence, discoloration of fruits/plants (Vollenweider and Günthardt-Goerg, 2005), reduced number of floret and spikes (Prasad et al., 2006; Fahad et al., 2016), decrease in the inter-nodal length (Siddiqui et al., 2015; Gray and Brady, 2016), plant height, total biomass, and the number of panicles (Modarresi et al., 2010). At the reproductive stage, one degree rise in temperature is detrimental because it degenerates mitochondria and proteins, loss of seed mass, quality, viability and vigor (Nahar, 2013; Balla et al., 2019).
The heat usually correlates with water because it cause dehydration in plant tissues especially in tropical and subtropical ecosystems (Giri, 2013; Giri et al., 2017). High temperature is lethal under both insufficient (Giri et al., 2017) or sufficient soil water because it affect the water conductivity and root permeability (Giri, 2013) which ultimately restrict the water and nutrients supply to the plant causing starvation and reduced growth (Wahid et al., 2007; Huang et al., 2016). The exact basis of the crop nutrient relationships under heat is not clearly known but overall, it lowers the photosynthesis and water viscosity and the activity of enzymes, e.g., nitrate reductase that is involved in the breakdown of nutrients, recycling, absorption, and accessibility to the plants (Kumarathunge et al., 2019).
Photosynthesis is among the most thermosensitive pathways in plants which is adversely affected due to the heat-induced reduced water contents, numbers of leaves, premature senescence and cell death (Hu et al., 2020). The major impact is the heat-induced injury to the photosynthetic machinery, i.e., stroma and thylakoid lamellae (Kmiecik et al., 2016; Sun and Guo, 2016). This disruption inhibits the thylakoid activity and the functional efficiency of photosystem II (Morales et al., 2003). Other plant processes that hamper photosynthesis under thermal stress include the reduction of photosynthetic pigments (Marchand et al., 2005), leaf moisture, transpiration rate, low CO2 concentration and supply to photosystem due to stomatal closure (Ashraf and Hafeez, 2004), reduction of amino acids, rubisco binding proteins (Li et al., 2018), carbohydrate depletion and plant malnutrition due to reduced activity of ADP-glucose pyro-phosphorylase, sucrose phosphate synthase, and invertase (Djanaguiraman et al., 2009). The reduction in chlorophyll pigment is linked with the activity of thylakoid membranes, lipid peroxidation of chloroplast, and reduced photochemistry (Fv/Fm ratio) of photosystem II, which ultimately reduce the overall photosynthesis in crop plants (Mohammed and Tarpley, 2010). Reduction of chlorophyll may be due to the reduced (about 60–90%) biosynthesis under high or low temperature, or increased chlorophyll-pigments degradation, or an accumulative effect of the two (Hemantaranjan et al., 2014).
Heat exposure stimulates oxidative stress due to the generation of activated, extremely reactive, and toxic oxygen species. The reaction of reactive oxygen species (ROS) includes supper oxide radical (O) singlet oxygen (O2), hydroxide ions (hydroxyl) radical (OH−), and hydrogen peroxide (H2O2) (Marutani et al., 2012; Suzuki et al., 2012). ROS causes lipid membrane to lose the ability to regulate substance exchange across the cell membrane (Suzuki et al., 2012). Oxidative stress enhance peroxidation and further damage the proteins, lipids, carbohydrates, and DNA contributing to premature aging (Savicka and Škute, 2010). Continuous thermal stress increases the ROS deposition in the plasma membranes with cell membrane depolarization, which leads to the activation of the RBOHD enzyme (respiratory burst oxidase homolog protein-D) that produces ROS and initiates programmed cell death signal (Mittler et al., 2011). The elevated temperature raises the O2 content in the root by 68%, and leaf malondialdehyde (MDA) content by 27% at the early and 58% at later stages (Medina et al., 2021). Though, the plant has a special mechanism to avoid unnecessary reactive oxygen species (ROS) by the production of specific antioxidants.
Heat stress affects mitochondrial functions by influencing respiration. The respiration often rises with increasing temperature, but at a certain duration of photoperiod, the respiration process declines due to damage to the respiratory system (Prasad et al., 2008; Rasmusson et al., 2020). In the heat-prone varieties under heat stress (35/25°C day/night) the respiration rate in the flag leaf of wheat was considerably higher compared to that of the control (23/18°C day/night) (Akter and Islam, 2017). The solubility of O2 and CO2 as well as Rubisco's kinetics, loss of respiratory carbon due to heat enhances the ROS and reduces ATP production and respiration in plants (Cossani and Reynolds, 2012).
HS induces significant yield reduction mainly due to decrease in the number, size and quality of grain, starch synthesis and accumulation, protein concentration, pod, fiber content, and breakdown of the Ca content (van Es, 2020). HS usually accelerates the rate of grain-filling but shortens the grain-filling time leading to significant decrease in grain length, width, and weight, grain quality (Högy et al., 2013; Lamaoui et al., 2018) reduced accumulation of storage compounds (Hurkman et al., 2013) and increased male sterility (Suwa et al., 2010). HS also increase proteinogenic amino acid and maltose content and decrease the concentrations of starch, sugars, raffinose, sucrose and lipids (Vasseur et al., 2011). A slight increase in temperature (1–1.5°C) reduces the harvest index and yield up to 10% in different crops (Table 3) (Tubiello et al., 2007; Ahamed et al., 2010; Hatfield et al., 2011). Heat-susceptible cultivars show more yield losses compared with thermo-tolerant cultivars (Ahamed et al., 2010; Hussain et al., 2019).
Plant HS adaptation involves a variety of strategies and various processes including basal heat tolerance (BHT), acquired heat tolerance (AHT), and avoidance (Fitter and Hay, 2012; Bäurle, 2016). BHT is the natural capacity of plants to tolerate heat while AHT (also called priming or acclimation), is acquired tolerance via short pre-exposure to heat (Yeh et al., 2012). A sudden heat exposure results in short-term reaction, i.e., leaves orientation, osmotic modification, evaporation, and adjustments in cell membrane structure (Bäurle, 2016; Zhongming and Wei, 2021) whereas, under long-term exposure, multiple adaptation mechanisms work synergistically to minimize the impact of heat stress. Heat tolerance involves the activation of some important immunity pathways, including ions transporters, proteins late embryogenesis abundant (LEA), phytohormones, antioxidant-resistance, and factors implicated in transduction signaling and transcriptional control (Li, 2020; Li et al., 2021). AHT is distinguished between short-term acquired tolerance (SAT) and long-term acquired tolerance (LAT) and establish a molecular stress memory state that protect themselves from acute heat damage and death (Sani et al., 2013). The stress-sensitive feature is characterized by an early signal that can be in the form of anionic and osmotic effects or alterations in membrane fluidity which restore homeostasis and sustain defective proteins and membranes (Kumarathunge et al., 2019). Plants HS avoidance includes long-lasting developmental morphological and physiological adaptations or short-lived accommodation strategies (Figure 2), e.g., intensive transpiration from leaves prevents damage by lowering the temperature of the leaves by 6°C or even 10–15°C than the normal temperature (Fitter and Hay, 2012; Li, 2020). Other plant varieties have special features that enable them to escape from warm conditions, e.g., having heat-sensitive buds, leaf abscission, annual desert buds, and by completing the entire regenerative period during the winter (Fitter and Hay, 2012). These adaptations are correlated to each other and increases the net process of photosynthesis at HS (especially for C4 and CAM plants) (Sarieva et al., 2010; Li, 2020).
Plants have evolved a series of detoxification systems to fight against oxidative damage. These systems include peroxidase (POX), ascorbate peroxidase (APX), glutathione reductase (GR), superoxide dismutase (SOD), and catalase (CAT) which show mitigating effects against different kinds of stress including HS (Suzuki et al., 2012; Caverzan et al., 2016). These antioxidants prevent the excessive free radicals and repair the damage effects that function as a catalyst to change the dismutation reaction from SOD anion to H2O2 and O2 molecules. They also affect other physiological phenomena including biosynthesis of lignin, enzyme catabolism, defense against injury and insect/pathogen attack, and physiological damage caused by temperature stress (Wani et al., 2016; Devireddy et al., 2021).
The production of a wide range of metabolites of low molecular mass including soluble carbohydrates, amino acids, variety of sugars, and sugar alcohols and phenolics have been linked to stabilization of cellular membranes, protection of protein structures, maintenance of the cell water balance, and buffering of the cellular redox potential under abiotic stress in plants (Kumarathunge et al., 2019). Accumulation of different osmoprotectants (e.g., proline, glycine betaine, etc.) and carotenoids (e.g., xanthophlls, terpenoids, etc.) under extreme temperature participate directly in the osmotic adjustment and show positive correlation with more negative leaf osmotic potential and production of protective pigments (Wani et al., 2016; Li et al., 2021). Glycine betaine maintains the activity of Rubisco by preventing its thermal inactivation (Devireddy et al., 2021). Sucrose and other carbohydrates act as antioxidant (Lang-Mladek et al., 2010; Devireddy et al., 2021) as well as regulate carbon allocation and sugar signaling consequently protecting the pollen viability. Plant growth regulators are also involved in enhancing plants' ability to tolerate stress, e.g., ABA, SA, IAA or CK (Ding et al., 2010; Hsu et al., 2010; Devireddy et al., 2021).
Many stress-inducible transcriptions factors (known as heat shock factors; HSF, e.g., Hsf6A, DREB1A, OsMYB55), stress-related genes, and proteins (HSP) are synthesized and overexpressed to induce thermotolerance (Lamaoui et al., 2018). The HS response is preceded by HSF that acts further on the transcription of HSP mRNA. HSFs are components of a complex signaling system that control responses not only to high temperatures but many other abiotic stresses. They are transcriptional activators of HS genes and bind specifically to heat shock sequence elements throughout the genome. Figure 3 describes in detail the mechanism of HSP/HSF activation under heat stress in the plant cell. A rapid increase in temperature up to 5–10°C, causes the plant to trigger the “heat-responsive genes” which translate into a special protein called heat shock proteins (HSPs). HSPs protect the plants by activating the chaperons and proper folding of proteins, inhibit the denaturation and aggregation of intercellular proteins and maintain their function by proper folding of proteins (Baniwal et al., 2005). HSPs are miscellaneous, highly diverse and normally present in living cells but under heat stress, different small and large HSPs are produced which help in (i) folding of newly synthesized proteins (Hsp60 and Hsp70), (ii) translocation the proteins between the cellular membrane and from organelle to organelle (Hsp70), (iii) prevent from deterioration, self-aggregation, improper folding, and the production of the polymeric compound of proteins (sHsp, Hsp70, Hsp90, Hsp100), (iv) the breakdown of defective proteins by proteolytic (Hsp70, Hsp100), (v) activation of signaling molecules, transduction, transcriptional factors and transcription (Hsp70, Hsp90), (vi) production of oligomeric complexes of high molecular weight that act as a cellular matrix for unfolded protein stabilization (Hsp20 or small Hsp (sHSP) (Hasanuzzaman et al., 2013).
CRISPR/Cas9 is a genome editing tool, widely use to edit the eukaryotic genome specially in plants The transient expression of this system is very useful to activate or limit the desired targeted gene in plants (LeBlanc et al., 2018). Recently, the scientist explained the heat-shock inducible CRISPR/Cas9 system for genome editing and demonstrate its effectiveness in rice. They combined the promoter of heat shock protein of rice U3 and soybean to induce the HS-CRISPR/Cas9 system via Cas9 and single guider RNA (Nandy et al., 2019). In another study, the Enhanced response to ABA1 (ERA1) gene present in Arabidopsis thaliana was targeted, that encode the farnesyltransferase and upregulate the abscisic acid (ABA) signaling under heat and drought stress. By using CRISPR/Cas9 system, they knock in the ERA1 gene into rice genome and enhanced the ABA in rice under heat and water deficit condition (Ogata et al., 2020).
By proper agronomic management, such as water, the methods and quantity for fertilization, the sowing time and method, the addition of protectants, plants can be grown effectively under heat stress (Ortiz et al., 2008). The usual practices are as follows:
• Mulching is done to preserve optimum moisture in soil, because it reduces soil evaporation (Chen et al., 2015), improves nitrogen and water efficiency (Singh et al., 2011), prevents yield loss (Chakraborty et al., 2008; Głab and Kulig, 2008) in temperate and tropical regions under thermal stress (Akter and Islam, 2017).
• Proper sowing time is very important for crops as early or late plantation shortens the heading and maturity period, improves pathogen infestation, eventually affecting economic yield and grain quality (Khichar and Niwas, 2007; Al-Karaki, 2012; Hakim et al., 2012; Hossain and Da Silva, 2012).
• Increasing crop nutrition (e.g., N, Ca, K, Mg, B, Se, and Mn) alter the stomatal function, activate physiological and molecular processes, improve tissue water potential, decrease ROS toxicity by increasing the antioxidant concentration stimulate heat stress tolerance (Waraich et al., 2012; Mengutay et al., 2013). The post-anthesis foliar application also improve grain proteins, slows down the ABA synthesis, improves cytokinin production, stimulates the photosynthesis and accumulation of assimilates (Dias and Lidon, 2010; Singh et al., 2011).
• The exogenous application of growth promoters [e.g., phytohormones, osmoregulatory, receptor molecules, polyamines along with spermidine, putrescine, spermine, putrescine, alpha-tocopherol (vitamin E), arginine etc.] regulate the ROS and enhance the efficiency of the antioxidant thus protect plants under HS (Farooq et al., 2011; Sharma and Chahal, 2012; Hemantaranjan et al., 2014; Uprety and Reddy, 2016).
• Exogenous calcium application activates MDA that enhances the activity of guaiacol peroxidase, CAT, SOD, which ultimately induce heat resistance in plants. It can also protect chlorophyll from solar radiation destruction and sustain stomatal functioning (Dias and Lidon, 2009; Waraich et al., 2012). Activation of different enzymatic, e.g., CAT, SOD, ascorbate peroxidase (APX), and non-enzymatic antioxidants, e.g., tocopherol, and ascorbic acid have a significant effect on oxidative management under multiple stresses (Balla et al., 2007).
• Plant treatment with reactive short-chain leaf volatiles (RSLVs also known as oxylipins; a group of C4–C9 straight chain carbonyls categorized by an alpha, β-unsaturated carbonyl bond) show high thermotolerance (Yamauchi, 2018).
• Co-polymers of Poly (N-isopropyl acrylamide) (Dimitrov et al., 2007) or poly acrylic acid (PAA) have been used as thermo-tolerant nanoparticle to transport specific chemicals within the plant to develop resistant against HS (Xu et al., 2015).
• The use of natural bio-stimulants, e.g., amino acids, microorganisms, fruit extracts, seaweeds, inorganic materials, and chitin or engineered, e.g., plant hormones, phenols, salts, chemical substances, and other elements with plant regulating properties (Calvo et al., 2014; Van Oosten et al., 2017).
• Transfer of heat shock regulatory proteins (HSFs, HSPs) or development of heat shock transcriptional elements (HSEs) enhance gene expression within minute and induced heat tolerance in plants.
Plants are home to large collection of microbes collectively known as plant microbiome (Liu and Tan, 2017; Álvarez-Pérez et al., 2019) which is highly dynamic in nature and show significant shift in the composition in response to external stimuli and environmental stresses, e.g., heat (Santos-Medellín et al., 2017; Timm et al., 2018). This shift is not a passive plant reaction, but a deliberate response because both plants and microbes have been coevolved since millions of years (Durán et al., 2018; Kwak et al., 2018). Plants use precise combinations of chemical stimuli under abiotic, or pathogen-induced stress which trigger specific microbes to overexpress particular proteins or enzymes for inducing stress tolerance (Bakker et al., 2018; Kumar and Verma, 2018; Liu and Brettell, 2019; Hakim et al., 2021). Overall, the plant microbiome controls nutrient availability, root growth, plant yield and modulates resistance to stresses (Chialva et al., 2018; Lu et al., 2018).
The microbial application (inoculation) is cheap, eco-friendly, low-input, and time-saving strategy as compared to the development of stress-tolerant crop variety or germplasm screening (Shrivastava and Kumar, 2015). Plant growth-promoting microbes (PGPM) require optimum condition for maximum efficiency (e.g., production of phytohormones, nitrogen fixation, and solubilization of nutrients P, Zn, Ca, Fe, etc.). Even though stress factors (salinity, drought, heat, and heavy metals) decrease their efficacy, but some PGPR modify themselves for maximum efficiency under stress. Species of Enterobacter, Acetobacter, and Pseudomonas solubilize the phosphorus 74%, 75%, and 80% respectively at normal (30–32 ± 2°C) and high temperature (38–40 ± 2°C) (Kachhap et al., 2015). Similarly, the Acetobacter spp. produced 100%, Enterobacter spp. produced 82% and Pseudomonas spp. produced 50% IAA both at high and low temperatures. While high temperature reduced the efficacy of nitrogen fixation in rhizobia. High temperature reduced the symbiotic relationship between plant roots and microbes which ultimately decreased the rate of nitrogen fixation. Heat stress inhibits microbial adherence on root hair and root nodules (Alexandre and Oliveira, 2013) and also disturb the molecular signals between microbes and root hair which leads to forming a weak symbiotic relationship between partners (Sadowsky, 2005). However, some rhizobial species from Acacia have thermotolerance up to 44°C which enable them to fix nitrogen at high temperature and give benefit to plant (Zahran et al., 1994).
PGPM are stable under chilling and heat stress (Barka et al., 2006; Ali et al., 2011) but the molecular and physiological changes connected to this stress management are poorly understood. Inoculation studies reports that systemic effects are involved during heat/chilling stress that change metabolic and regulatory function of plant supporting both growth and stress management (Abd El-Daim et al., 2019). Table 4 describes the role of PGPR in plant under stress condition. Decrease in the ROS production by seed treatment with Bacillus amyloliquefaciens and Azospirillum brasilense has also been reported under HS (Abd El-Daim et al., 2014). A recent review published the meta-analysis of microbe-mediated thermotolerance in plants and their mechanisms from 39 published research articles (Dastogeer et al., 2022). They have reported a significant decrease in accumulation of MDA and H2O2 in colonized plants showing lower oxidation activity but a corresponding increase in the activities of catalase, peroxidase, glutathione reductase under heat stress. However, the activities of SOD, ascorbate oxidase, ascorbate peroxidase and proline were variable. The overall impact of microbial colonization was more pronounced under heat stress.
A variety of PGPM have been known to induce heat stress using various mechanisms (Dodd and Pérez-Alfocea, 2012; Ramadoss et al., 2013). For instance, Pseudomonas putida produces heat shock protein that plays a vital role in increasing plant thermal tolerance (Ali et al., 2011) (Figure 4). Many of PGPM activate structural changes in plants that impart tolerance to heat stress, a phenomenon known as induced systemic tolerance (Yang et al., 2009). Apart from inducing direct stress tolerance, several plant-beneficial traits exhibited by these bacteria support plant growth in a synergistic manner under stress (Etesami and Beattie, 2017; Imran et al., 2021). They benefit plants either directly, through the phytohormones production, nutrient mobilization, and nitrogen fixation or indirectly by triggering the signaling cascades in the host plant. Burkholderia phytofirmans strain PsJN is a well-reported PGPR that enhances heat tolerance in tomatoes, cold tolerance in grapevine, water stress tolerance in wheat, salt, and freezing tolerance in Arabidopsis. The same bacterium also has the antifungal property that protects the plant from biotic stress (Issa et al., 2018) revealing that a single bacterium can induce multiple benefits in same or different hosts (Imran et al., 2021).
Phytohormones are the key regulators of plant development, and the plants have a natural ability to synthesize, perceive and respond to these hormones. This response is modulated upon exposure to external/internal stimuli (Khan et al., 2014; Hakim et al., 2021), e.g., decreased levels of auxin, cytokinin, and gibberellin and increased ABA level under HS (Wu et al., 2016). One of the mechanisms of PGPM-mediated plant growth improvement is the phytohormone production trait (Etesami et al., 2015) which affect the metabolism of endogenous (Hashem et al., 2016; Sorty et al., 2016) and ultimately play a key role in modulating the plant's response under stress, uptake etc. (Spaepen et al., 2008; Khan et al., 2011). These facts validate that the phytohormones-producing PGPM reverse the adverse effects of heat stress.
Auxin (IAA) is the most important hormone, imperative for cell division, differentiation that controls seed germination, roots development, and apical dominance (Maheshwari et al., 2015). The majority of the rhizospheric microbes (>80%) synthesize and release IAA in the rhizosphere which elongates plant roots, increases the number of root hairs for enhanced uptake of water and nutrients under normal (Oleńska et al., 2020) as well as stress (Imran et al., 2015; Nawaz et al., 2020; Zia et al., 2021). IAA producing Ochrobactrum sp. and Pseudomonas sp. (volcanos isolates) have shown improved root and shoot length, fresh weight, and biomass of maize under high temperature (40°C), drought (up to 60% Poly Ethylene Glycol 6000), and salt (500 mM NaCl) (Mishra et al., 2017).
Gibberellins (GAs) regulate plant developmental processes such as embryogenesis, leaf expansion, stem elongation, flowering, and fruit ripening (Binenbaum et al., 2018) while abscisic acid (ABA) regulates cell division and elongation, seed dormancy and germination, embryo maturation, floral induction, and responses to stresses (Finkelstein, 2013). PGPM capable to synthesize gibberellins stimulate plant growth and stress tolerance by modulating the endogenous levels of GAs and ABA. For instance, inoculation with GAs producing Serratia nematodiphila and B. tequilensis increase the endogenous synthesis of GA4 and ABA while reduced the salicylic and jasmonic acids and improved plant biomass under HS (Kang et al., 2015). Phytohormones-producing endophytic fungi (Phoma and Penicillium sp.) and Bacillus spp. also modulate the level of endogenous abscisic acid, salicylic acid, and jasmonic acid under multiple stresses and improve thermotolerance (Waqas et al., 2012). Inoculation with a multiple hormone (IAA, CK, JA, SA, GAs, and ABA) producing B. aryabhattai strain significantly improved nodule number, overall plant growth, and increased stress tolerance of soybean to drought and high temperatures (38°C) (Park et al., 2017).
Cytokinins are involved in processes such as seed germination, apical dominance, roots development, nodule organogenesis, development of vascular tissues, flower and fruit, and plant-pathogen interactions (Osugi and Sakakibara, 2015). Different microbes (such as Bacillus, Escherichia, Agrobacterium, Methylobacterium, Proteus, Pseudomonas, and Klebsiella) inhabiting plant rhizosphere are capable to synthesize and release CK in the rhizosphere which stimulate plant growth under stress including heat (Liu et al., 2013). Exogenous application of INCYDE-F (an inhibitor of CK-oxidase/dehydrogenase) in Arabidopsis increased the contents of CK trans-zeatin and cis-zeatin in roots and IAA in all tissues after HS. It further reduced the level of ABA in leaves and ethylene in apices of roots which shows that inhibition of CK-degradation helped the Arabidopsis to cope with HS (Prerostova et al., 2020).
Ethylene is a gaseous hormone involved in abscission, senescence, reproductive development, and a(biotic) stress response (Liu et al., 2021). Pollen development is the most thermosensitive stage during reproduction, therefore, regulation of ethylene signaling in reproductive tissues is critical to gain reproductive success (Jegadeesan et al., 2018). Various abiotic and biotic stressors enhanced the levels of ethylene in plant tissues which is detrimental for plants. Enterobacter sp. SA187-induced thermo-tolerance to wheat in field has been reported (Shekhawat et al., 2021) which is mediated by the ethylene signaling via the TF EN13 and constitutive H3K4me3 modification of HS memory genes, generating robust thermotolerance in plants.
Biosynthesis of ethylene is undertaken by two transcripts (i) PsACS [encode enzymes that convert S-adenosyl-L-methionine to 1-aminocyclopropane-1-carboxylic acid (ACC)] and (ii) PsACO (encode enzymes that convert ACC to ethylene (Savada et al., 2017). Various microbes, i.e., Methylobacterium, Bacillus, Alcaligenes, Enterobacter, Pseudomonas, Azospirillum, Rhizobium, and Bradyrhizobium have an enzyme “1-aminocyclopropane-1-carboxylate (ACC) deaminase,” which metabolize ACC (an immediate precursor of ethylene) into α-ketobutyrate and ammonia ultimately lowering down the ethylene levels and detrimental impact on plants under stress including HS (Saleem et al., 2007). Burkholderia phytofirmans, Pseudomonas frederiksbergensis, P. vancouverensis, P. putida, and B. cereus are few examples of heat resilient microbes that utilize the ACC-deaminase enzyme to reduce the endogenous ethylene levels in plants produced under a range of different stresses including salinity (Liu et al., 2021), drought (Danish et al., 2020), and heat (Mukhtar S. et al., 2020).
Exopolysaccharides (EPSs) are bacterial extracellular polymers that form 3D structure of complex compound (sugars, enzymes, polysaccharides, lipids, nucleic acids, extracellular DNA structural proteins) which is used in energy exchange mechanism in response to environmental signals (Mishra, 2013) and have a direct role in heat stress tolerance (Nishihata et al., 2018; Ogden et al., 2019). These EPSs are involved in cell aggregation, adhesion, water retention, building a protective barrier and supplying nutrients. They contain high-molecular-weight polymers that enable bacteria to cling with the soil particles via hydrogen bonding, van der wall forces, anionic and cationic bonding, and keep them alive. The inoculation with heat-resilient, ESPs-producing B. cereus increased root and shoot length, chlorophyll and proline contents, water intake, number of flowers and fruits in tomato under high temperature (Mukhtar T. et al., 2020). HS induces changes in EPSs production as well as other cellular proteins, i.e., HSPs which prevents protein aggregation, misfolding, and target abnormally folded proteins for degradation (Parsell and Lindquist, 1993). Nguyen et al. (2014) reported that Pseudomonas sp. strain PsJN produced ESPs at high temperature and enhanced growth of the potato. Application of Bacillus, Pseudomonas and Azospirillum spp. (Ali et al., 2011; Abd El-Daim et al., 2019; Da Jeong Shin et al., 2019) have been shown to induce metabolic and regulatory proteins modulation to develop heat stress tolerance along with the cold and drought stresses. Apart from this, EPS act as chemoattractant and help to develop microbial biofilm matrix on the root surface that protect root surfaces from any damage under any kind of stress including HS.
Plant growth-promoting rhizobacteria not only produce phytohormones but also help plants by modulation of different genes under heat stress. Heat stress upregulates the genes involved in autophagy (e.g., SlWRKY33b and SlATG5 genes) that are harmful to plants. But the application of Bacillus cereus sp. isolate (SA1) and humic acid (HA) down-regulate the expression of these genes which not only give relief but provide thermotolerance to the tomato plants (Khan et al., 2020) and increase the uptake of potassium (K) phosphorus (P), and iron (Fe). B. cereus SA1 enhanced the activity of antioxidants (CAT, APX, SOD) as well as the efficacy of chlorophyll pigments. The inoculation also resulted in a reduced level of abscisic acid (ABA) and enhanced the level of salicylic acid (SA). The treated plant showed a reduced level of glutathione (GSH). It has been shown that thermotolerant bacteria (Bacillus spp. actinobacterium Kocuria sp.) and cyanobacteria (Calothrix elenkinii and Anabaena laxa) show plant growth-promoting activities (30–50% root and shoot length, biomass, and dry weight) and stimulate peroxidase (5–10%) and endoglucanase activities in a range of different plants (Kumar et al., 2013).
Thermotolerant P. putida strain AKMP7 inoculated to wheat plants increased the plant biomass and dry weight, enhanced root and shoot length, increase the number of tillers and spikelet, and enhance grain formation (Ali et al., 2011). The inoculated bacteria prevent the plant from cellular injury, enhance the antioxidant enzymatic activities (SOD, APX, CAT), and improve cellular metabolism, e.g., the level of protein, proline contents, cellular sugar, amino acid, and starch, and the efficiency of chlorophyll under heat stress condition. AKMP7 formed biofilm on plant roots which help plants to combat adverse heat stress conditions.
Global warming has become a critical challenge to food security, causing severe yield losses of major crops worldwide. Given the expanding needs for global food supply as well as the extreme pressure of population growth and climate change trajectories, strategies should primarily be focused on the right investigation on different abiotic stresses especially on heat stress that becomes a key problem in the last decade. The plants are more vulnerable to high temperatures because of their sessile nature. They exhibit physio-morphological, biochemical, and molecular adaptations against heat, but further investigations are required to understand the thermotolerance mechanism active in different plant species. The plants themselves produce antioxidants, reduce the stomatal conductance, activate heat-responsive genes, and produce heat shock proteins, but on the other hand, exogenous or foliar application of chemicals like calcium chloride (CaCl2), salicylic acid, bio-stimulants, nanoparticles, and osmoprotectants are useful in sustainable agriculture. Furthermore, heat-sensitive genes can be targeted through CRISPR-Cas9 to develop heat-insensitive crops in the future. The heat resilient microbes produces phytohormones, ethylene, ACC deaminase, antioxidant enzymes, and HSP under heat stress enabling plants to maintain their growth under stress. A recent review publishes the meta-analysis of 39 published studies in support of PGPR-mediated thermotolerance in plants. This supports that microbe-mediated solutions are more sustainable than developing heat-tolerant varieties as microbes are usually present in the soil and rhizosphere and can form associations with homologous as well as non-homologous hosts. So, a single heat resilient microbe-inoculum may be effective in more than one crop or plants. Furthermore, the microbes are multi-purpose and exhibit many other traits of plant interest apart from giving tolerance against heat stress making the microbe-therapy more effective than chemical or genetic engineering or breeding approaches. However, the molecular mechanisms involved in this tolerance may be studied in detail in different crops prone to heat stress. As plants can't live in isolation in any environment, they have a direct impact of environment and also interact with the microbes present in soil. Whereas microbes also interact with other microbes and to the environment. So, this tri-partite association is very important for the stable functioning of the plants and microbes and over all agricultural sustainability in any given environment.
MA collected data, made illustrations and figures, and wrote the initial draft. MI, FM, and MS helped in the analysis and writing. AI conceptualized and finalized the review. All authors contributed to the article and approved the submitted version.
The authors declare that the research was conducted in the absence of any commercial or financial relationships that could be construed as a potential conflict of interest.
All claims expressed in this article are solely those of the authors and do not necessarily represent those of their affiliated organizations, or those of the publisher, the editors and the reviewers. Any product that may be evaluated in this article, or claim that may be made by its manufacturer, is not guaranteed or endorsed by the publisher.
Abd El-Daim, I., Bejai, S., Fridborg, I., and Meijer, J. (2018). Identifying potential molecular factors involved in Bacillus amyloliquefaciens 5113 mediated abiotic stress tolerance in wheat. Plant Biol. 20, 271–279. doi: 10.1111/plb.12680
Abd El-Daim, I. A., Bejai, S., and Meijer, J. (2014). Improved heat stress tolerance of wheat seedlings by bacterial seed treatment. Plant Soil 379, 337–350. doi: 10.1007/s11104-014-2063-3
Abd El-Daim, I. A., Bejai, S., and Meijer, J. (2019). Bacillus velezensis 5113 induced metabolic and molecular reprogramming during abiotic stress tolerance in wheat. Sci. Rep. 9, 1–18. doi: 10.1038/s41598-019-52567-x
Ahamed, K.-U., Nahar, K., Fujita, M., and Hasanuzzaman, M. (2010). Variation in plant growth, tiller dynamics and yield components of wheat (Triticum aestivum L.) due to high temperature stress. Adv. Agric. Botanics 2, 213–224.
Akter, N., and Islam, M. R. (2017). Heat stress effects and management in wheat. A review. Agron. Sustain. Dev. 37, 37. doi: 10.1007/s13593-017-0443-9
Alexandre, A., and Oliveira, S. (2013). Response to temperature stress in rhizobia. Critic. Rev. Microbiol. 39, 219–228. doi: 10.3109/1040841X.2012.702097
Ali, S. Z., Sandhya, V., Grover, M., Linga, V. R., and Bandi, V. (2011). Effect of inoculation with a thermotolerant plant growth promoting Pseudomonas putida strain AKMP7 on growth of wheat (Triticum spp.) under heat stress. J. Plant Interact. 6, 239–246. doi: 10.1080/17429145.2010.545147
Al-Karaki, G. N. (2012). Phenological development-yield relationships in durum wheat cultivars under late-season high-temperature stress in a semiarid environment. ISRN Agron. 2012, 456856. doi: 10.5402/2012/456856
Álvarez-Pérez, S., Lievens, B., and Fukami, T. (2019). Yeast–bacterium interactions: the next frontier in nectar research. Trends Plant Sci. 24, 393–401. doi: 10.1016/j.tplants.2019.01.012
Argosubekti, N. (2020). “A review of heat stress signaling in plants,” in IOP Conference Series: Earth and Environmental Science. Bristol: IOP Publishing, 012041.
Ashraf, M., and Hafeez, M. (2004). Thermotolerance of pearl millet and maize at early growth stages: growth and nutrient relations. Biol. Plant. 48, 81–86. doi: 10.1023/B:BIOP.0000024279.44013.61
Asseng, S., Ewert, F., Martre, P., Rötter, R. P., Lobell, D. B., Cammarano, D., et al. (2015). Rising temperatures reduce global wheat production. Nat. Clim. Change 5, 143–147. doi: 10.1038/nclimate2470
Bakker, P. A., Pieterse, C. M., de Jonge, R., and Berendsen, R. L. (2018). The soil-borne legacy. Cell. 172, 1178–1180. doi: 10.1016/j.cell.2018.02.024
Balla, K., Bed,o, Z., and Veisz, O. (2007). Heat stress induced changes in the activity of antioxidant enzymes in wheat. Cereal Res. Commun. 35, 197–200. doi: 10.1556/CRC.35.2007.2.8
Balla, K., Karsai, I., Bónis, P., Kiss, T., Berki, Z., Horváth, Á., et al. (2019). Heat stress responses in a large set of winter wheat cultivars (Triticum aestivum L.) depend on the timing and duration of stress. PLoS One 14, e0222639. doi: 10.1371/journal.pone.0222639
Balla, K., Rakszegi, M., Li, Z., Bekes, F., Bencze, S., and Veisz, O. (2011). Quality of winter wheat in relation to heat and drought shock after anthesis. Czech J. Food Sci. 29, 117–128. doi: 10.17221/227/2010-CJFS
Baniwal, S., Bharti, K., Chan, K., Fauth, M., Ganguli, A., Kotak, S., et al. (2005). Heat stress response in plants: A complex game with chaperones and more than twenty heat stress transcription factors. J. Biosci. 29, 471–487. doi: 10.1007/BF02712120
Barka, E. A., Nowak, J., and Clément, C. (2006). Enhancement of chilling resistance of inoculated grapevine plantlets with a plant growth-promoting rhizobacterium, Burkholderia phytofirmans strain PsJN. Appl. Environ. Microbiol. 72, 7246–7252. doi: 10.1128/AEM.01047-06
Bäurle, I. (2016). Plant heat adaptation: priming in response to heat stress. F1000Research 5, F1000 Faculty Rev-694. doi: 10.12688/f1000research.7526.1
Begum, N., Qin, C., Ahanger, M. A., Raza, S., Khan, M. I., Ashraf, M., et al. (2019). Role of arbuscular mycorrhizal fungi in plant growth regulation: implications in abiotic stress tolerance. Front. Plant Sci. 10, 1068. doi: 10.3389/fpls.2019.01068
Bensalim, S., Nowak, J., and Asiedu, S. K. (1998). A plant growth promoting rhizobacterium and temperature effects on performance of 18 clones of potato. Am. J. Potato Res. 75, 145–152.
Binenbaum, J., Weinstain, R., and Shani, E. (2018). Gibberellin localization and transport in plants. Trends Plant Sci. 23, 410–421. doi: 10.1016/j.tplants.2018.02.005
Bonde, M., Nester, S., and Berner, D. (2012). Effects of daily temperature highs on development of Phakopsora pachyrhizi on soybean. Phytopathology 102, 761–768. doi: 10.1094/PHYTO-01-12-0011-R
Calvo, P., Nelson, L., and Kloepper, J. W. (2014). Agricultural uses of plant biostimulants. Plant Soil 383, 3–41. doi: 10.1007/s11104-014-2131-8
Caverzan, A., Casassola, A., and Brammer, S. P. (2016). Antioxidant responses of wheat plants under stress. Genet. Mol. Biol. 39, 1–6. doi: 10.1590/1678-4685-GMB-2015-0109
Chakraborty, D., Nagarajan, S., Aggarwal, P., Gupta, V., Tomar, R., Garg, R., et al. (2008). Effect of mulching on soil and plant water status, and the growth and yield of wheat (Triticum aestivum L.) in a semi-arid environment. Agric. Water Manag. 95, 1323–1334. doi: 10.1016/j.agwat.2008.06.001
Change, I. C. (2014). Mitigation of Climate Change. Contribution of Working Group III to the Fifth Assessment Report of the Intergovernmental Panel on Climate Change. Geneva: IPCC, 1454.
Chen, J., Zhou, X., Wang, W., Liu, B., Lv, Y., Yang, W., et al. (2018). A review on fundamental of high entropy alloys with promising high–temperature properties. J. Alloys Compd. 760, 15–30. doi: 10.1016/j.jallcom.2018.05.067
Chen, Y., Liu, T., Tian, X., Wang, X., Li, M., Wang, S., et al. (2015). Effects of plastic film combined with straw mulch on grain yield and water use efficiency of winter wheat in Loess Plateau. Field Crops Res. 172, 53–58. doi: 10.1016/j.fcr.2014.11.016
Cheng, L., Zou, Y., Ding, S., Zhang, J., Yu, X., Cao, J., et al. (2009). Polyamine accumulation in transgenic tomato enhances the tolerance to high temperature stress. J. Integr. Plant Biol. 51, 489–499. doi: 10.1111/j.1744-7909.2009.00816.x
Chialva, M., Salvioli Di Fossalunga, A., Daghino, S., Ghignone, S., Bagnaresi, P., Chiapello, M., et al. (2018). Native soils with their microbiotas elicit a state of alert in tomato plants. New Phytol. 220, 1296–1308. doi: 10.1111/nph.15014
Commuri, P., and Jones, R. (2001). High temperatures during endosperm cell division in maize: a genotypic comparison under in vitro and field conditions. Crop Sci. 41, 1122–1130. doi: 10.2135/cropsci2001.4141122x
Cossani, C. M., and Reynolds, M. P. (2012). Physiological traits for improving heat tolerance in wheat. Plant Physiol. 160, 1710–1718. doi: 10.1104/pp.112.207753
Da Costa, M. V. J., Ramegowda, Y., Ramegowda, V., Karaba, N. N., Sreeman, S. M., and Udayakumar, M. (2021). Combined drought and heat stress in rice: responses, phenotyping and strategies to improve tolerance. Rice Sci. 28, 233–242. doi: 10.1016/j.rsci.2021.04.003
Da Jeong Shin, S.-J. Y., Hong, J. K., Weon, H.-Y., Song, J., and Sang, M. K. (2019). Effect of Bacillus aryabhattai H26-2 and B. siamensis H30-3 on growth promotion and alleviation of heat and drought stresses in Chinese cabbage. Plant Pathol. J. 35, 178. doi: 10.5423/PPJ.NT.08.2018.0159
Damayanti, T., and Putra, L. (2010). Hot water treatment of cutting-cane infected with Sugarcane streak mosaic virus (SCSMV). J. ISSAAS 16, 17–25. doi: 10.5423/20113256683
Danish, S., Zafar-Ul-Hye, M., Mohsin, F., and Hussain, M. (2020). ACC-deaminase producing plant growth promoting rhizobacteria and biochar mitigate adverse effects of drought stress on maize growth. PLoS ONE 15, e0230615. doi: 10.1371/journal.pone.0230615
Dastogeer, K. M., Zahan, M. I., Rhaman, M. S., Sarker, M. S., and Chakraborty, A. (2022). Microbe-mediated thermotolerance in plants and pertinent mechanisms-a meta-analysis and review. Front. Microbiol. 13, 833566. doi: 10.3389/fmicb.2022.833566
Devireddy, A. R., Tschaplinski, T. J., Tuskan, G. A., Muchero, W., and Chen, J.-G. (2021). Role of reactive oxygen species and hormones in plant responses to temperature changes. Int. J. Mol. Sci. 22, 8843. doi: 10.3390/ijms22168843
Dias, A., and Lidon, F. (2009). Evaluation of grain filling rate and duration in bread and durum wheat, under heat stress after anthesis. J. Agron. Crop Sci. 195, 137–147. doi: 10.1111/j.1439-037X.2008.00347.x
Dias, A. S., and Lidon, F. C. (2010). Bread and durum wheat tolerance under heat stress: a synoptical overview. Emir. J. Food Agric. 2010, 412–436. doi: 10.9755/ejfa.v22i6.4660
Dimitrov, I., Trzebicka, B., Müller, A. H., Dworak, A., and Tsvetanov, C. B. (2007). Thermosensitive water-soluble copolymers with doubly responsive reversibly interacting entities. Prog. Polym. Sci. 32, 1275–1343. doi: 10.1016/j.progpolymsci.2007.07.001
Ding, W., Song, L., Wang, X., and Bi, Y. (2010). Effect of abscisic acid on heat stress tolerance in the calli from two ecotypes of Phragmites communis. Biol. Plant. 54, 607–613. doi: 10.1007/s10535-010-0110-3
Djanaguiraman, M., Annie Sheeba, J., Durga Devi, D., and Bangarusamy, U. (2009). Cotton leaf senescence can be delayed by nitrophenolate spray through enhanced antioxidant defence system. J. Agron. Crop Sci. 195, 213–224. doi: 10.1111/j.1439-037X.2009.00360.x
Djanaguiraman, M., Narayanan, S., Erdayani, E., and Prasad, P. V. (2020). Effects of high temperature stress during anthesis and grain filling periods on photosynthesis, lipids and grain yield in wheat. BMC Plant Biol. 20, 1–12. doi: 10.1186/s12870-020-02479-0
Djanaguiraman, M., Prasad, P., and Al-Khatib, K. (2011). Ethylene perception inhibitor 1-MCP decreases oxidative damage of leaves through enhanced antioxidant defense mechanisms in soybean plants grown under high temperature stress. Environ. Exp. Bot. 71, 215–223. doi: 10.1016/j.envexpbot.2010.12.006
Djanaguiraman, M., Prasad, P. V., and Seppanen, M. (2010). Selenium protects sorghum leaves from oxidative damage under high temperature stress by enhancing antioxidant defense system. Plant Physiol. Biochem. 48, 999–1007. doi: 10.1016/j.plaphy.2010.09.009
Dodd, I. C., and Pérez-Alfocea, F. (2012). Microbial amelioration of crop salinity stress. J. Exp. Bot. 63, 3415–3428. doi: 10.1093/jxb/ers033
Dubey, R., Pathak, H., Chakrabarti, B., Singh, S., Gupta, D. K., and Harit, R. (2020). Impact of terminal heat stress on wheat yield in India and options for adaptation. Agric. Syst. 181, 102826. doi: 10.1016/j.agsy.2020.102826
Dupuis, I., and Dumas, C. (1990). Influence of temperature stress on in vitro fertilization and heat shock protein synthesis in maize (Zea mays L.) reproductive tissues. Plant Physiol. 94, 665–670. doi: 10.3389/fpls.2015.00999
Durán, P., Thiergart, T., Garrido-Oter, R., Agler, M., Kemen, E., Schulze-Lefert, P., et al. (2018). Microbial interkingdom interactions in roots promote Arabidopsis survival. Cell 175, 973–983.e914. doi: 10.1016/j.cell.2018.10.020
Easterling, W. E., Aggarwal, P. K., Batima, P., Brander, K. M., Erda, L., Howden, S. M., et al. (2007). Food, fibre and forest products. Clim. Change 2007, 273–313.
Etesami, H., Alikhani, H. A., and Hosseini, H. M. (2015). Indole-3-acetic acid (IAA) production trait, a useful screening to select endophytic and rhizosphere competent bacteria for rice growth promoting agents. MethodsX. 2, 72–78. doi: 10.1016/j.mex.2015.02.008
Etesami, H., and Beattie, G. A. (2017). “Plant-microbe interactions in adaptation of agricultural crops to abiotic stress conditions,” in Probiotics and Plant Health (Singapore: Springer), 163–200. doi: 10.1007/978-981-10-3473-2_7
Etterson, J. R., and Shaw, R. G. (2001). Constraint to adaptive evolution in response to global warming. Science 294, 151–154. doi: 10.1126/science.1063656
Fahad, S., Hussain, S., Saud, S., Hassan, S., Ihsan, Z., Shah, A. N., et al. (2016). Exogenously applied plant growth regulators enhance the morpho-physiological growth and yield of rice under high temperature. Front. Plant Sci. 7, 1250. doi: 10.3389/fpls.2016.01250
Farooq, M., Bramley, H., Palta, J. A., and Siddique, K. H. (2011). Heat stress in wheat during reproductive and grain-filling phases. Crit. Rev. Plant Sci. 30, 491–507. doi: 10.1080/07352689.2011.615687
Finkelstein, R. (2013). Abscisic acid synthesis and response. Am. Soci. Plant Biol. 11, 166. doi: 10.1199/tab.0166
Friedlingstein, P., Houghton, R., Marland, G., Hackler, J., Boden, T. A., Conway, T., et al. (2010). Update on CO 2 emissions. Nat. Geosci. 3, 811–812. doi: 10.1038/ngeo1022
Giri, A. (2013). Effect of Acute Heat Stress on Nutrient Uptake by Plant Roots. Toledo: The University of Toledo.
Giri, A., Heckathorn, S., Mishra, S., and Krause, C. (2017). Heat stress decreases levels of nutrient-uptake and-assimilation proteins in tomato roots. Plants 6, 6.
Głab, T., and Kulig, B. (2008). Effect of mulch and tillage system on soil porosity under wheat (Triticum aestivum). Soil Tillage Res. 99, 169–178. doi: 10.1016/j.still.2008.02.004
Grant, R., Kimball, B., Conley, M., White, J., Wall, G., and Ottman, M. J. (2011). Controlled warming effects on wheat growth and yield: field measurements and modeling. Agron. J. 103, 1742–1754. doi: 10.2134/agronj2011.0158
Gray, S. B., and Brady, S. M. (2016). Plant developmental responses to climate change. Dev. Biol. 419, 64–77. doi: 10.1016/j.ydbio.2016.07.023
Hakim, M., Hossain, A., Da Silva, J. a.T, Zvolinsky, V., and Khan, M. (2012). Protein and starch content of 20 wheat (Triticum aestivum L.) genotypes exposed to high temperature under late sowing conditions. J. Sci. Res. 4, 477–477. doi: 10.3329/jsr.v4i2.8679
Hakim, S., Naqqash, T., Nawaz, M. S., Laraib, I., Siddique, M. J., Zia, R., et al. (2021). Rhizosphere engineering with plant growth-promoting microorganisms for agriculture and ecological sustainability. Front. Sustain. Food Syst. 5, 16. doi: 10.3389/fsufs.2021.617157
Hasanuzzaman, M., Nahar, K., Alam, M., Roychowdhury, R., and Fujita, M. (2013). Physiological, biochemical, and molecular mechanisms of heat stress tolerance in plants. Int. J. Mol. Sci. 14, 9643–9684. doi: 10.3390/ijms14059643
Hashem, A., Abd Allah, E. F., Alqarawi, A. A., Al-Huqail, A. A., Wirth, S., and Egamberdieva, D. (2016). The interaction between arbuscular mycorrhizal fungi and endophytic bacteria enhances plant growth of Acacia gerrardii under salt stress. Front. Microbiol. 7, 1089. doi: 10.3389/fmicb.2016.01089
Hatfield, J. L., Boote, K. J., Kimball, B., Ziska, L., Izaurralde, R. C., Ort, D., et al. (2011). Climate impacts on agriculture: implications for crop production. Agron. J. 103, 351–370. doi: 10.2134/agronj2010.0303
Hatfield, J. L., and Prueger, J. H. (2015). Temperature extremes: effect on plant growth and development. Weather Clim. Extrem. 10, 4–10. doi: 10.1016/j.wace.2015.08.001
Hemantaranjan, A., Bhanu, A. N., Singh, M., Yadav, D., Patel, P., Singh, R., et al. (2014). Heat stress responses and thermotolerance. Adv. Plants Agric. Res 1, 1–10. doi: 10.15406/apar.2014.01.00012
Högy, P., Poll, C., Marhan, S., Kandeler, E., and Fangmeier, A. (2013). Impacts of temperature increase and change in precipitation pattern on crop yield and yield quality of barley. Food Chem. 136, 1470–1477. doi: 10.1016/j.foodchem.2012.09.056
Hossain, A., and Da Silva, J. T. (2012). Phenology, growth and yield of three wheat (Triticum aestivum L.) varieties as affected by high temperature stress. Not. Sci. Biol. 4, 97–109. doi: 10.15835/nsb437879
Hovmøller, M. S., Yahyaoui, A. H., Milus, E. A., and Justesen, A. F. (2008). Rapid global spread of two aggressive strains of a wheat rust fungus. Mol. Ecol. 17, 3818–3826. doi: 10.1111/j.1365-294X.2008.03886.x
Howarth, C. (2005). “Genetic improvements of tolerance to high temperature,” in Abiotic Stresses–Plant Resistance Through Breeding and Molecular Approaches, eds M. Ashraf, P. J. C. Harris (New York: The Haworth Press), 277–300.
Hsu, S.-F., Lai, H.-C., and Jinn, T.-L. (2010). Cytosol-localized heat shock factor-binding protein, AtHSBP, functions as a negative regulator of heat shock response by translocation to the nucleus and is required for seed development in Arabidopsis. Plant Physiol. 153, 773–784. doi: 10.1104/pp.109.151225
Hu, S., Ding, Y., and Zhu, C. (2020). Sensitivity and responses of chloroplasts to heat stress in plants. Front. Plant Sci. 11, 375. doi: 10.3389/fpls.2020.00375
Huang, Y.-C., Niu, C.-Y., Yang, C.-R., and Jinn, T.-L. (2016). The heat stress factor HSFA6b connects ABA signaling and ABA-mediated heat responses. Plant Physiol. 172, 1182–1199. doi: 10.1104/pp.16.00860
Hurkman, W. J., Tanaka, C. K., Vensel, W. H., Thilmony, R., and Altenbach, S. B. (2013). Comparative proteomic analysis of the effect of temperature and fertilizer on gliadin and glutenin accumulation in the developing endosperm and flour from Triticum aestivum L. cv. Butte 86. Proteome Sci. 11, 1–15. doi: 10.1186/1477-5956-11-8
Hurkman, W. J., Vensel, W. H., Tanaka, C. K., Whitehand, L., and Altenbach, S. B. (2009). Effect of high temperature on albumin and globulin accumulation in the endosperm proteome of the developing wheat grain. J. Cereal Sci. 49, 12–23. doi: 10.1016/j.jcs.2008.06.014
Hussain, H. A., Men, S., Hussain, S., Chen, Y., Ali, S., Zhang, S., et al. (2019). Interactive effects of drought and heat stresses on morpho-physiological attributes, yield, nutrient uptake and oxidative status in maize hybrids. Sci. Rep. 9, 1–12. doi: 10.1038/s41598-019-40362-7
Hussain, S., Khaliq, A., Mehmood, U., Qadir, T., Saqib, M., Iqbal, M. A., et al. (2018). Sugarcane Production Under Changing Climate: Effects of Environmental Vulnerabilities on Sugarcane Diseases, Insects and Weeds. Vienna: Intech Open.
Ikeda, H., Kinoshita, T., Yamamoto, T., and Yamasaki, A. (2019). Sowing time and temperature influence bulb development in spring-sown onion (Allium cepa L.). Sci. Hortic. 244, 242–248. doi: 10.1016/j.scienta.2018.09.050
Imran, A., Hakim, S., Tariq, M, Nawaz, M. S., Laraib, I., Gulzar, U., et al. (2021). Diazotrophs for lowering nitrogen pollution crises; looking deep into the roots. Front. Microbiol. 12, 637815. doi: 10.3389/fmicb.2021.637815
Imran, A., Mirza, M. S., Shah, T. M., Malik, K. A., and Hafeez, F. Y. (2015). Differential response of kabuli and desi chickpea genotypes toward inoculation with PGPR in different soils. Front. Microbiol. 6, 859. doi: 10.3389/fmicb.2015.00859
Issa, A., Esmaeel, Q., Sanchez, L., Courteaux, B., Guise, J.-F., Gibon, Y., et al. (2018). Impacts of Paraburkholderia phytofirmans strain PsJN on tomato (Lycopersicon esculentum L.) under high temperature. Front. Plant Sci. 9, 1397. doi: 10.3389/fpls.2018.01397
Jegadeesan, S., Chaturvedi, P., Ghatak, A., Pressman, E., Meir, S., Faigenboim, A., et al. (2018). Proteomics of heat-stress and ethylene-mediated thermotolerance mechanisms in tomato pollen grains. Front. Plant Sci. 9, 1558. doi: 10.3389/fpls.2018.01558
Jones, L. M., Koehler, A. K., Trnka, M., Balek, J., Challinor, A. J., Atkinson, H. J., et al. (2017). Climate change is predicted to alter the current pest status of Globodera pallida and G. rostochiensis in the United Kingdom. Glob. Change Biol. 23, 4497–4507. doi: 10.1111/gcb.13676
Kachhap, S., Chaudhary, A., and Singh, S. (2015). Response of plant growth promoting rhizobacteria (pgpr) in relation to elevated temperature conditions in groundnut (Arachis hypogaea L.). Ecoscan 9, 771–778.
Kandil, A., Attia, A., Sharief, A., and Leilh, A. (2011). Response of onion (Allium cepa L.) yield to water stress and mineral biofertilization. Acta. Agron. Hung. 59, 361–370. doi: 10.1556/AAgr.59.2011.4.7
Kang, S.-M., Khan, A. L., Waqas, M., You, Y.-H., Hamayun, M., Joo, G.-J., et al. (2015). Gibberellin-producing Serratia nematodiphila PEJ1011 ameliorates low temperature stress in Capsicum annuum L. Eur. J. Soil Biol. 68, 85–93. doi: 10.1016/j.ejsobi.2015.02.005
Kaushal, N., Bhandari, K., Siddique, K. H., and Nayyar, H. (2016). Food crops face rising temperatures: an overview of responses, adaptive mechanisms, and approaches to improve heat tolerance. Cogent Food Agric. 2, 1134380. doi: 10.1080/23311932.2015.1134380
Khan, A. L., Hamayun, M., Kim, Y. H., Kang, S. M., Lee, J. H., and Lee, I. J. (2011). Gibberellins producing endophytic Aspergillus fumigatus sp. LH02 influenced endogenous phytohormonal levels, isoflavonoids production and plant growth in salinity stress. Process Biochem. 6, 440–447. doi: 10.1016/j.procbio.2010.09.013
Khan, M. A., Asaf, S., Khan, A. L., Jan, R., Kang, S.-M., Kim, K.-M., et al. (2020). Extending thermotolerance to tomato seedlings by inoculation with SA1 isolate of Bacillus cereus and comparison with exogenous humic acid application. PLoS ONE 15, e0232228. doi: 10.1371/journal.pone.0232228
Khan, M. I. R., Asgher, M., and Khan, N. A. (2014). Alleviation of salt-induced photosynthesis and growth inhibition by salicylic acid involves glycinebetaine and ethylene in mungbean (Vigna radiata L.). Plant Physiol. Biochem. 80, 67–74. doi: 10.1016/j.plaphy.2014.03.026
Khichar, M., and Niwas, R. (2007). Thermal effect on growth and yield of wheat under different sowing environments and planting systems. Indian J. Agric. Sci. 41, 92–96.
Kmiecik, P., Leonardelli, M., and Teige, M. (2016). Novel connections in plant organellar signalling link different stress responses and signalling pathways. J. Exp. Bot. 67, 3793–3807. doi: 10.1093/jxb/erw136
Kong, B., Li, Z., Wang, E., Lu, W., Chen, L., and Qi, G. (2018). An experimental study for characterization the process of coal oxidation and spontaneous combustion by electromagnetic radiation technique. Process Saf. Environ. 119, 285–294. doi: 10.1016/j.psep.2018.08.002
Kumar, A., and Verma, J. P. (2018). Does plant-microbe interaction confer stress tolerance in plants: a review?. Microbiol. Res. 207, 41–52. doi: 10.1016/j.micres.2017.11.004
Kumar, M., Prasanna, R., Bidyarani, N., Babu, S., Mishra, B. K., Kumar, A., et al. (2013). Evaluating the plant growth promoting ability of thermotolerant bacteria and cyanobacteria and their interactions with seed spice crops. Sci. Hortic. 164, 94–101. doi: 10.1016/j.scienta.2013.09.014
Kumarathunge, D. P., Medlyn, B. E., Drake, J. E., Tjoelker, M. G., Aspinwall, M. J., Battaglia, M., et al. (2019). Acclimation and adaptation components of the temperature dependence of plant photosynthesis at the global scale. New phytol. 222, 768–784. doi: 10.1111/nph.15668
Kwak, M.-J., Kong, H. G., Choi, K., Kwon, S.-K., Song, J. Y., Lee, J., et al. (2018). Rhizosphere microbiome structure alters to enable wilt resistance in tomato. Nat. Biotechnol. 36, 1100–1109. doi: 10.1038/nbt.4232
Lamaoui, M., Jemo, M., Datla, R., and Bekkaoui, F. (2018). Heat and drought stresses in crops and approaches for their mitigation. Front. Chem. 6, 26. doi: 10.3389/fchem.2018.00026/full
Lämke, J., and Bäurle, I. (2017). Epigenetic and chromatin-based mechanisms in environmental stress adaptation and stress memory in plants. Genome Biol. 18, 1–11. doi: 10.1186/s13059-017-1263-6
Lang-Mladek, C., Popova, O., Kiok, K., Berlinger, M., Rakic, B., Aufsatz, W., et al. (2010). Transgenerational inheritance and resetting of stress-induced loss of epigenetic gene silencing in Arabidopsis. Mol. Plant. 3, 594–602. doi: 10.1093/mp/ssq014
LeBlanc, C., Zhang, F., Mendez, J., Lozano, Y., Chatpar, K., Irish, V. F., et al. (2018). Increased efficiency of targeted mutagenesis by CRISPR/Cas9 in plants using heat stress. Plant J. 93, 377–386.
Li, N., Euring, D., Cha, J. Y., Lin, Z., Lu, M., Huang, L.-J., et al. (2021). Plant hormone-mediated regulation of heat tolerance in response to global climate change. Front. Plant Sci. 11, 2318. doi: 10.3389/fpls.2020.627969/full
Li, X., Cai, C., Wang, Z., Fan, B., Zhu, C., and Chen, Z. (2018). Plastid translation elongation factor Tu is prone to heat-induced aggregation despite its critical role in plant heat tolerance. Plant Physiol. 176, 3027–3045. doi: 10.1104/pp.17.01672
Li, Z., Peng, T., Xie, Q., Han, S., and Tian, J. (2010). Mapping of QTL for tiller number at different stages of growth in wheat using double haploid and immortalized F 2 populations. Genetic. 89, 409. doi: 10.1007/s12041-010-0059-1
Li, Z.-G. (2020). “Mechanisms of plant adaptation and tolerance to heat stress,” in Plant Ecophysiology and Adaptation Under Climate Change: Mechanisms and Perspectives II (Berlin: Springer), 39–59.
Liu, C.-H., Siew, W., Hung, Y.-T., Jiang, Y.-T., and Huang, C.-H. (2021). 1-Aminocyclopropane-1-carboxylate (ACC) deaminase gene in pseudomonas azotoformans is associated with the amelioration of salinity stress in tomato. J. Agric. Food Chem. 69, 913–921. doi: 10.1021/acs.jafc.0c05628
Liu, F., Xing, S., Ma, H., Du, Z., and Ma, B. (2013). Cytokinin-producing, plant growth-promoting rhizobacteria that confer resistance to drought stress in Platycladus orientalis container seedlings. Appl. Microbiol. Biotechnol. 97, 9155–9164. doi: 10.1007/s00253-013-5193-2
Liu, H., and Brettell, L. E. (2019). Plant defense by VOC-induced microbial priming. Trends Plant Sci. 24, 187–189. doi: 10.1016/j.tplants.2019.01.008
Liu, Z., and Tan, V. Y. F. (2017). “Relative error bounds for nonnegative matrix factorization under a geometric assumption,” in 2017 IEEE International Conference on Acoustics, Speech and Signal Processing (ICASSP), 2552–2556.
Lobell, D. B., and Field, C. B. (2007). Global scale climate–crop yield relationships and the impacts of recent warming. Environ. Res. Lett. 2, 014002. doi: 10.1088/1748-9326/2/1/014002/meta
Lobell, D. B., Schlenker, W., and Costa-Roberts, J. (2011). Climate trends and global crop production since 1980. Science 333, 616–620. doi: 10.1126/science.1204531
Lu, T., Ke, M., Lavoie, M., Jin, Y., Fan, X., Zhang, Z., et al. (2018). Rhizosphere microorganisms can influence the timing of plant flowering. Microbiome 6, 1–12. doi: 10.1186/s40168-018-0615-0
Ma, L., Qiao, J., Kong, X., Zou, Y., Xu, X., Chen, X., et al. (2015). Effect of low temperature and wheat winter-hardiness on survival of Puccinia striiformis f. sp. tritici under controlled conditions. PLoS ONE 10, e0130691. doi: 10.1371/journal.pone.0130691
Maheshwari, D. K., Dheeman, S., and Agarwal, M. (2015). “VPhytohormone-producing PGPR for sustainable agriculture,” in Bacterial Metabolites in Sustainable Agroecosystem (Berlin: Springer), 159–182.
Majeed, S., Rana, I. A., Mubarik, M. S., Atif, R. M., Yang, S.-H., Chung, G., et al. (2021). Heat stress in cotton: a review on predicted and unpredicted growth-yield anomalies and mitigating breeding strategies. Agronomy 11, 1825. doi: 10.3390/agronomy11091825
Mangrauthia, S. K., Shakya, V. P. S., Jain, R., and Praveen, S. (2009). Ambient temperature perception in papaya for papaya ringspot virus interaction. Virus Genes 38, 429–434. doi: 10.1007/s11262-009-0336-3
Marchand, F. L., Mertens, S., Kockelbergh, F., Beyens, L., and Nijs, I. (2005). Performance of High Arctic tundra plants improved during but deteriorated after exposure to a simulated extreme temperature event. Glob. Chang Biol. 11, 2078–2089. doi: 10.1111/j.1365-2486.2005.01046.x
Mariette, N., Androdias, A., Mabon, R., Corbiere, R., Marquer, B., Montarry, J., et al. (2016). Local adaptation to temperature in populations and clonal lineages of the Irish potato famine pathogen Phytophthora infestans. Ecol. Evol. 6, 6320–6331. doi: 10.1002/ece3.2282
Marutani, Y., Yamauchi, Y., Kimura, Y., Mizutani, M., and Sugimoto, Y. (2012). Damage to photosystem II due to heat stress without light-driven electron flow: involvement of enhanced introduction of reducing power into thylakoid membranes. Planta 236, 753–761. doi: 10.1007/s00425-012-1647-5
Mcclung, C. R., and Davis, S. J. (2010). Ambient thermometers in plants: from physiological outputs towards mechanisms of thermal sensing. Curr. Biol. 20, R1086–R1092. doi: 10.1016/j.cub.2010.10.035
Medina, E., Kim, S.-H., Yun, M., and Choi, W.-G. (2021). Recapitulation of the function and role of ROS generated in response to heat stress in plants. Plants 10, 371. doi: 10.3390/plants10020371
Meinke, H., Nelson, R., Kokic, P., Stone, R., Selvaraju, R., and Baethgen, W. (2006). Actionable climate knowledge: from analysis to synthesis. Clim. Res. 33, 101–110. doi: 10.3354/cr033101
Mengutay, M., Ceylan, Y., Kutman, U. B., and Cakmak, I. (2013). Adequate magnesium nutrition mitigates adverse effects of heat stress on maize and wheat. Plant soil 368, 57–72. doi: 10.1007/s11104-013-1761-6
Miller, P., Lanier, W., and Brandt, S. (2001). Using Growing Degree Days to Predict Plant Stages. Bozeman: Montana State University-Bozeman, 994–2721.
Milus, E. A., Kristensen, K., and Hovmøller, M. S. (2009). Evidence for increased aggressiveness in a recent widespread strain of Puccinia striiformis f. sp. tritici causing stripe rust of wheat. Phytopathology 99, 89–94. doi: 10.1094/PHYTO-99-1-0089
Mishra, J. (2013). “Microbial exopolysaccharides,” in The Prokaryotes–Applied Bacteriology and Biotechnolgy, ED E. Rosenberg. Berlin: Springer-Verlag.
Mishra, S. K., Khan, M. H., Misra, S., Dixit, V. K., Khare, P., Srivastava, S., et al. (2017). Characterisation of Pseudomonas spp. and Ochrobactrum sp. isolated from volcanic soil. Antonie Van Leeuwenhoek 110, 253–270. doi: 10.1007/s10482-016-0796-0
Mittler, R., Vanderauwera, S., Suzuki, N., Miller, G., Tognetti, V. B., Vandepoele, K., et al. (2011). ROS signaling: the new wave? Trends Plant Sci. 16, 300–309. doi: 10.1016/j.tplants.2011.03.007
Modarresi, M., Mohammadi, V., Zali, A., and Mardi, M. (2010). Response of wheat yield and yield related traits to high temperature. Cereal Res. Commun. 38, 23–31. doi: 10.1556/CRC.38.2010.1.3
Mohammed, A. R., and Tarpley, L. (2010). Effects of high night temperature and spikelet position on yield-related parameters of rice (Oryza sativa L.) plants. Eur. J. Agron. 33, 117–123. doi: 10.1016/j.eja.2009.11.006
Momčilović, I. (2019). Effects of heat stress on potato productivity and nutritive quality. Hrana i ishrana 60, 43–48. doi: 10.5937/hraIsh1902043M
Morales, D., Rodríguez, P., Dell'amico, J., Nicolas, E., Torrecillas, A., and Sánchez-Blanco, M. J. (2003). High-temperature preconditioning and thermal shock imposition affects water relations, gas exchange and root hydraulic conductivity in tomato. Biol. Plant. 47, 203. doi: 10.1023/B:BIOP.0000022252.70836.fc
Mukhtar, S., Zareen, M., Khaliq, Z., Mehnaz, S., and Malik, K. (2020). Phylogenetic analysis of halophyte-associated rhizobacteria and effect of halotolerant and halophilic phosphate-solubilizing biofertilizers on maize growth under salinity stress conditions. J. Appl. Microbiol. 128, 556–573. doi: 10.1111/jam.14497
Mukhtar, T., Smith, D., Sultan, T., Seleiman, M. F., Alsadon, A. A., Ali, S., et al. (2020). Mitigation of heat stress in Solanum lycopersicum L. by ACC-deaminase and exopolysaccharide producing Bacillus cereus: effects on biochemical profiling. Sustainability 12, 2159. doi: 10.3390/su12062159
Nahar, K. (2013). Castor bean (Ricinus communis L.)-a biofuel plant: morphological and physiological parameters propagated from seeds in Bangladesh. Asian Bus. Rev. 2, 64–66.
Nandy, S., Pathak, B., Zhao, S., and Srivastava, V. (2019). Heat-shock-inducible CRISPR/Cas9 system generates heritable mutations in rice. Plant Dir. 3, e00145. doi: 10.1002/pld3.145
Nawaz, M. S., Arshad, A., Rajput, L., Fatima, K., Ullah, S., Ahmad, M., et al. (2020). Growth-stimulatory effect of quorum sensing signal molecule n-acyl-homoserine lactone-producing multi-trait Aeromonas spp. on wheat genotypes under salt stress. Front. Microbiol. 11, 553621. doi: 10.3389/fmicb.2020.553621
Nguyen, H. T., Razafindralambo, H., Blecker, C., N'yapo, C., Thonart, P., and Delvigne, F. (2014). Stochastic exposure to sub-lethal high temperature enhances exopolysaccharides (EPS) excretion and improves Bifidobacterium bifidum cell survival to freeze–drying. Biochem. Eng. J. 88, 85–94. doi: 10.1016/j.bej.2014.04.005
Nishihata, S., Kondo, T., Tanaka, K., Ishikawa, S., Takenaka, S., Kang, C.-M., et al. (2018). Bradyrhizobium diazoefficiens USDA110 PhaR functions for pleiotropic regulation of cellular processes besides PHB accumulation. BMC Microbiol. 18, 1–17. doi: 10.1186/s12866-018-1317-2
Ogata, T., Ishizaki, T., Fujita, M., and Fujita, Y. (2020). CRISPR/Cas9-targeted mutagenesis of OsERA1 confers enhanced responses to abscisic acid and drought stress and increased primary root growth under nonstressed conditions in rice. PLoS ONE 15, e0243376. doi: 10.1371/journal.pone.0243376
Ogden, A. J., Mcaleer, J. M., and Kahn, M. L. (2019). Characterization of the Sinorhizobium meliloti HslUV and ClpXP protease systems in free-living and symbiotic states. J. Bacteriol. 201, e00498–e00418. doi: 10.1128/JB.00498-18
Oleńska, E., Małek, W., Wójcik, M., Swiecicka, I., Thijs, S., and Vangronsveld, J. (2020). Beneficial features of plant growth-promoting rhizobacteria for improving plant growth and health in challenging conditions: A methodical review. Sci. Total Environ. 2020, 140682. doi: 10.1016/j.scitotenv.2020.140682
Ortiz, R., Sayre, K. D., Govaerts, B., Gupta, R., Subbarao, G., Ban, T., et al. (2008). Climate change: can wheat beat the heat? Agric. Ecosyst. Environ. 126, 46–58. doi: 10.1016/j.agee.2008.01.019
Osugi, A., and Sakakibara, H. (2015). QandA: How do plants respond to cytokinins and what is their importance? BMC Biol. 13, 1–10. doi: 10.1186/s12915-015-0214-5
Park, H., Weier, S., Razvi, F., Peña, P. A., Sims, N. A., Lowell, J., et al. (2017). Towards the development of a sustainable soya bean-based feedstock for aquaculture. Plant Biotechnol. J. 15, 227–236. doi: 10.1111/pbi.12608
Parsell, D., and Lindquist, S. (1993). The function of heat-shock proteins in stress tolerance: degradation and reactivation of damaged proteins. Annu. Rev. Genet. 27, 437–496.
Porter, J. R., Xie, L., Challinor, A. J., Cochrane, K., Howden, S. M., Iqbal, M. M., et al. (2014). Food Security and Food Production Systems. IPPC. Available online at: https://www.ipcc.ch/site/assets/uploads/2018/02/WGIIAR5-Chap7_FINAL.pdf (accessed May 9, 2022).
Prasad, P., Boote, K., Allen Jr, L., Sheehy, J., and Thomas, J. (2006). Species, ecotype and cultivar differences in spikelet fertility and harvest index of rice in response to high temperature stress. Field Crops Res. 95, 398–411. doi: 10.1016/j.fcr.2005.04.008Get
Prasad, P., Staggenborg, S., and Ristic, Z. (2008). Impacts of Drought and/or Heat Stress on Physiological, Developmental, Growth, and Yield Processes of Crop Plants. Response of Crops to Limited Water: Understanding and Modeling Water Stress Effects on Plant Growth Processes 1, 301–355.
Prerostova, S., Dobrev, P. I., Kramna, B., Gaudinova, A., Knirsch, V., Spichal, L., et al. (2020). Heat acclimation and inhibition of cytokinin degradation positively affect heat stress tolerance of Arabidopsis. Front. Plant Sci 11, 87. doi: 10.3389/fpls.2020.00087
Rahman, M. A., Chikushi, J., Yoshida, S., and Karim, A. (2009). Growth and yield components of wheat genotypes exposed to high temperature stress under control environment. Bangl. J. Agr. Res. 34, 360–372. doi: 10.3329/bjar.v34i3.3961
Ramadoss, D., Lakkineni, V. K., Bose, P., Ali, S., and Annapurna, K. (2013). Mitigation of salt stress in wheat seedlings by halotolerant bacteria isolated from saline habitats. SpringerPlus 2, 6. doi: 10.1186/2193-1801-2-6
Rangan, P., Subramani, R., Kumar, R., Singh, A. K., and Singh, R. (2014). Recent advances in polyamine metabolism and abiotic stress tolerance. Biomed. Res. Int. 2014, 239621. doi: 10.1155/2014/239621
Rasmusson, L. M., Buapet, P., George, R., Gullström, M., Gunnarsson, P. C., and Björk, M. (2020). Effects of temperature and hypoxia on respiration, photorespiration, and photosynthesis of seagrass leaves from contrasting temperature regimes. ICES J. Marine Sci. 77, 2056–2065. doi: 10.1093/icesjms/fsaa093
Ratnarajah, V., and Gnanachelvam, N. (2021). Effect of abiotic stress on onion yield: a review. Adv. Technol. 2021, 147–160.
Reddy, P. S., Chakradhar, T., Reddy, R. A., Nitnavare, R. B., Mahanty, S., and Reddy, M. K. (2016). “Role of heat shock proteins in improving heat stress tolerance in crop plants,” in Heat Shock Proteins and Plants. (Berlin: Springer), 283–307.
Reynolds, M., Calderini, D., Condon, A., and Rajaram, S. (2001). “Physiological basis of yield gains in wheat associated with the LR19 translocation from Agropyron elongatum,” in Wheat in a Global Environment (Berlin: Springer), 345–351.
Ritchie, F., Bain, R., and Mcquilken, M. (2013). Survival of sclerotia of R hizoctonia solani AG 3 PT and effect of soil-borne inoculum density on disease development on potato. J. Phytopathol. 161, 180–189. doi: 10.1111/jph.12052
Roberts, K. E., Hadfield, J. D., Sharma, M. D., and Longdon, B. (2018). Changes in temperature alter the potential outcomes of virus host shifts. PLoS Pathog. 14, e1007185.
Ruelland, E., and Zachowski, A. (2010). How plants sense temperature. Environ. Exp. Bot. 69, 225–232. doi: 10.1016/j.envexpbot.2010.05.011
Rykaczewska, K. (2015). The effect of high temperature occurring in subsequent stages of plant development on potato yield and tuber physiological defects. Am. J. Potato Res. 92, 339–349. doi: 10.1007/s12230-015-9436-x
Sadowsky, M. J. (2005). “Soil stress factors influencing symbiotic nitrogen fixation,” in Nitrogen Fixation in Agriculture, Forestry, Ecology, and the Environment (Berlin: Springer), 89–112.
Saleem, M., Arshad, M., Hussain, S., and Bhatti, A. S. (2007). Perspective of plant growth promoting rhizobacteria (PGPR) containing ACC deaminase in stress agriculture. J. Ind. Microbiol. Biot. 34, 635–648. doi: 10.1104/pp.126.3.1281
Sani, E., Herzyk, P., Perrella, G., Colot, V., and Amtmann, A. (2013). Hyperosmotic priming of Arabidopsis seedlings establishes a long-term somatic memory accompanied by specific changes of the epigenome. Genome Biol. 14, 1–24. doi: 10.1186/gb-2013-14-6-r59
Santos-Medellín, C., Edwards, J., Liechty, Z., Nguyen, B., and Sundaresan, V. (2017). Drought stress results in a compartment-specific restructuring of the rice root-associated microbiomes. MBio 8, e764. doi: 10.1128/mBio.00764-17
Sarieva, G., Kenzhebaeva, S., and Lichtenthaler, H. (2010). Adaptation potential of photosynthesis in wheat cultivars with a capability of leaf rolling under high temperature conditions. Russ. J. Plant Physl. 57, 28–36. doi: 10.1134/S1021443710010048
Sarkar, N. K., Kundnani, P., and Grover, A. (2013). Functional analysis of Hsp70 superfamily proteins of rice (Oryza sativa). Cell Stress Chaperon 18, 427–437. doi: 10.1007/s12192-012-0395-6
Sarsu, F. (2018). “Screening protocols for heat tolerance in rice at the seedling and reproductive stages,” in Pre-Field Screening Protocols for Heat-Tolerant Mutants in Rice (Berlin: Springer), 9–24.
Savada, R. P., Ozga, J. A., Jayasinghege, C. P., Waduthanthri, K. D., and Reinecke, D. M. (2017). Heat stress differentially modifies ethylene biosynthesis and signaling in pea floral and fruit tissues. Plant Mol. Biol. 95, 313–331. doi: 10.1007/s11103-017-0653-1
Savicka, M., and Škute, N. (2010). Effects of high temperature on malondialdehyde content, superoxide production and growth changes in wheat seedlings (Triticum aestivum L.). Ekologija 56, 26–33. doi: 10.2478/v10055-010-0004-x
Sharma, N., and Chahal, K. (2012). Protectant potential of Marigold oil (Tagetes erecta L.) and its fractions against Tribolium castaneum (Herbst). Ann. Plant. Protect Sci. 20, 294–297.
Shekhawat, K., Saad, M. M., Sheikh, A., Mariappan, K., Al-Mahmoudi, H., Abdulhakim, F., et al. (2021). Root endophyte induced plant thermotolerance by constitutive chromatin modification at heat stress memory gene loci. EMBO Rep. 22, e51049. doi: 10.15252/embr.202051049
Shrivastava, P., and Kumar, R. (2015). Soil salinity: a serious environmental issue and plant growth promoting bacteria as one of the tools for its alleviation. Saudi J. Biol. Sci. 22, 123–131. doi: 10.1016/j.sjbs.2014.12.001
Siddiqui, M. H., Al-Khaishany, M. Y., Al-Qutami, M. A., Al-Whaibi, M. H., Grover, A., Ali, H. M., et al. (2015). Morphological and physiological characterization of different genotypes of faba bean under heat stress. Saudi J. Biol. Sci. 22, 656–663. doi: 10.1016/j.sjbs.2015.06.002
Singh, B., Pathak, K., Verma, A., Verma, V., and Deka, B. (2011). Effects of vermicompost, fertilizer and mulch on plant growth, nodulation and pod yield of French bean (Phaseolus vulgaris L.). Veg. Crop. Res. Bull. 74, 153–165. doi: 10.2478/v10032-011-0013-7
Singh, R. P., Jha, P., and Jha, P. N. (2015). The plant-growth-promoting bacterium Klebsiella sp. SBP-8 confers induced systemic tolerance in wheat (Triticum aestivum) under salt stress. J. Plant Physiol. 184, 57–67. doi: 10.1016/j.jplph.2015.07.002
Sita, K., Sehgal, A., Hanumantharao, B., Nair, R. M., Vara Prasad, P. V., Kumar, S., et al. (2017). Food Legumes and Rising Temperatures: Effects, Adaptive Functional Mechanisms Specific to Reproductive Growth Stage and Strategies to Improve Heat Tolerance. Front. Plant Sci. 8, 1658. doi: 10.3389/fpls.2017.01658
Sorty, A. M., Meena, K. K., Choudhary, K., Bitla, U. M., Minhas, P. S., and Krishnani, K. K. (2016). Effect of plant growth promoting bacteria associated with halophytic weed (Psoralea corylifolia L) on germination and seedling growth of wheat under saline conditions. Appl. Biochem. Biotechnol. 180, 872–882. doi: 10.1007/s12010-016-2139-z
Spaepen, S., Dobbelaere, S., Croonenborghs, A., and Vanderleyden, J. (2008). Effects of Azospirillum brasilense indole-3-acetic acid production on inoculated wheat plants. Plant Soil. 312, 15–23. doi: 10.1007/s11104-008-9560-1
Sun, A.-Z., and Guo, F.-Q. (2016). Chloroplast retrograde regulation of heat stress responses in plants. Front. Plant Sci. 7, 398. doi|: doi: 10.3389/fpls.2016.00398/full
Sutherland, I. W. (2001). Microbial polysaccharides from Gram-negative bacteria. Int. Dairy J. 11, 663–674. doi: 10.1016/S0958-6946(01)00112-1
Suwa, R., Hakata, H., Hara, H., El-Shemy, H. A., Adu-Gyamfi, J. J., Nguyen, N. T., et al. (2010). High temperature effects on photosynthate partitioning and sugar metabolism during ear expansion in maize (Zea mays L.) genotypes. Plant Physiol. Bioch. 48, 124–130. doi: 10.1016/j.plaphy.2009.12.010
Suzuki, N., Koussevitzky, S., Mittler, R., and Miller, G. (2012). ROS and redox signalling in the response of plants to abiotic stress. Plant Cell Environ. 35, 259–270. doi: 10.1111/j.1365-3040.2011.02336.x
Tian, F., Li, B., Ji, B., Zhang, G., and Luo, Y. (2009). Identification and structure–activity relationship of gallotannins separated from Galla chinensis. LWT-Food Sci. Technol. 42, 1289–1295. doi: 10.1016/j.lwt.2009.03.004
Timm, C. M., Carter, K. R., Carrell, A. A., Jun, S.-R., Jawdy, S. S., Vélez, J. M., et al. (2018). Abiotic stresses shift belowground Populus-associated bacteria toward a core stress microbiome. MSystems 3. doi: 10.1128/mSystems.00070-17
Toh, S., Imamura, A., Watanabe, A., Nakabayashi, K., Okamoto, M., Jikumaru, Y., et al. (2008). High temperature-induced abscisic acid biosynthesis and its role in the inhibition of gibberellin action in Arabidopsis seeds. Plant Physiol. 146, 1368–1385. doi: 10.1104/pp.107.113738
Tubiello, F. N., Soussana, J.-F., and Howden, S. M. (2007). Crop and pasture response to climate change. Proc. Natl. Acad. Sci. 104, 19686–19690. doi: 10.1073/pnas.0701728104
Turkensteen, L., Flier, W., Wanningen, R., and Mulder, A. (2000). Production, survival and infectivity of oospores of Phytophthora infestans. Plant Physiol. 49, 688–696. doi: 10.1046/j.1365-3059.2000.00515.x
Uprety, D. C., and Reddy, V. (2016). “Mitigation technologies to control high-temperature stress in crop plants,” in Crop Responses to Global Warming. (Berlin: Springer), 117–125.
van Es, S. W. (2020). Too hot to handle, the adverse effect of heat stress on crop yield. Physiol. Plant. 169, 499–500. doi: 10.1111/ppl.13165
Van Oosten, M. J., Pepe, O., De Pascale, S., Silletti, S., and Maggio, A. (2017). The role of biostimulants and bioeffectors as alleviators of abiotic stress in crop plants. Chem. Biol. Technol. 4, 5. doi: 10.1186/s40538-017-0089-5
Vasseur, F., Pantin, F., and Vile, D. (2011). Changes in light intensity reveal a major role for carbon balance in Arabidopsis responses to high temperature. Plant Cell Environ. 34, 1563–1576. doi: 10.1111/j.1365-3040.2011.02353.x
Velásquez, A. C., Castroverde, C. D. M., and He, S. Y. (2018). Plant–pathogen warfare under changing climate conditions. Curr Biol. 28, R619–R634. doi: 10.1016/j.cub.2018.03.054
Vollenweider, P., and Günthardt-Goerg, M. S. (2005). Diagnosis of abiotic and biotic stress factors using the visible symptoms in foliage. Environ. Pollut. 137, 455–465. doi: 10.1016/j.envpol.2005.01.032
Wahid, A., and Close, T. (2007). Expression of dehydrins under heat stress and their relationship with water relations of sugarcane leaves. Biol. Plant. 51, 104–109. doi: 10.1007/s10535-007-0021-0
Wahid, A., Gelani, S., Ashraf, M., and Foolad, M. R. (2007). Heat tolerance in plants: an overview. Environ. Exp. Bot. 61, 199–223. doi: 10.1016/j.envexpbot.2007.05.011
Wani, S. H., Kumar, V., Shriram, V., and Sah, S. K. (2016). Phytohormones and their metabolic engineering for abiotic stress tolerance in crop plants. Crop J. 4, 162–176. doi: 10.1016/j.cj.2016.01.010
Waqas, M., Khan, A. L., Kamran, M., Hamayun, M., Kang, S.-M., Kim, Y.-H., et al. (2012). Endophytic fungi produce gibberellins and indoleacetic acid and promotes host-plant growth during stress. Molecules 17, 10754–10773. doi: 10.3390/molecules170910754
Waraich, E., Ahmad, R., Halim, A., and Aziz, T. (2012). Alleviation of temperature stress by nutrient management in crop plants: a review. J. Soil Sci. Plant Nut. 12, 221–244. doi: 10.4067/S0718-95162012000200003
Wu, C., Cui, K., Wang, W., Li, Q., Fahad, S., Hu, Q., et al. (2016). Heat-induced phytohormone changes are associated with disrupted early reproductive development and reduced yield in rice. Sci. Rep. 6, 1–14. doi: 10.1038/srep34978
Wu, H.-C., Bulgakov, V. P., and Jinn, T.-L. (2018). Pectin methylesterases: cell wall remodeling proteins are required for plant response to heat stress. Front. Plant Sci. 9, 1612. doi: 10.3389/fpls.2018.01612
Xu, Y., Chu, C., and Yao, S. (2021). The impact of high-temperature stress on rice: challenges and solutions. Crop J. 9, 963–976. doi: 10.1016/j.cj.2021.02.011
Xu, Z., Liu, S., Liu, H., Yang, C., Kang, Y., and Wang, M. (2015). Unimolecular micelles of amphiphilic cyclodextrin-core star-like block copolymers for anticancer drug delivery. Chem. Commun. 51, 15768–15771. doi: 10.1039/C5CC02743H
Yamauchi, Y. (2018). “Integrated chemical control of abiotic stress tolerance using biostimulants,” in Plant, Abiotic Stress and Responses to Climate Change, 133–143.
Yang, J., Kloepper, J. W., and Ryu, C.-M. (2009). Rhizosphere bacteria help plants tolerate abiotic stress. Trends Plant Sci. 14, 1–4. doi: 10.1016/j.tplants.2008.10.004
Yeh, C.-H., Kaplinsky, N. J., Hu, C., and Charng, Y.-Y. (2012). Some like it hot, some like it warm: phenotyping to explore thermotolerance diversity. Plant Sci. 195, 10–23. doi: 10.1111/nph.14212
Yin, Y., Li, S., Liao, W., Lu, Q., Wen, X., and Lu, C. (2010). Photosystem II photochemistry, photoinhibition, and the xanthophyll cycle in heat-stressed rice leaves. J. Plant Physiol. 167, 959–966. doi: 10.1016/j.jplph.2009.12.021
Zafar, S. A., Noor, M. A., Waqas, M. A., Wang, X., Shaheen, T., Raza, M., et al. (2018). “Temperature extremes in cotton production and mitigation strategies,” in Past, Present and Future Trends in Cotton Breeding. eds M. R. Rahman and Y. Zafar (New York: Intechopen), 65-91.
Zahran, H., Räsänen, L. A., Karsisto, M., and Lindström, K. (1994). Alteration of lipopolysaccharide and protein profiles in SDS-PAGE of rhizobia by osmotic and heat stress. World J. Microbiol. Biotechnol. 10, 100–105. doi: 10.1007/BF00357572
Zhang, X., Cai, J., Wollenweber, B., Liu, F., Dai, T., Cao, W., et al. (2013). Multiple heat and drought events affect grain yield and accumulations of high molecular weight glutenin subunits and glutenin macropolymers in wheat. J. Cereal Sci. 57, 134–140. doi: 10.1016/j.jcs.2012.10.010
Zhao, D., and Li, Y.-R. (2015). Climate change and sugarcane production: potential impact and mitigation strategies. Int. J. Agron. 2015, 547386. doi: 10.1155/2015/547386
Zhongming, Z., and Wei, L. (2021). How to Beat the Heat: Memory Mechanism Allows Plants to Adapt to Heat Stress. Science Daily.
Zia, R., Nawaz, M., Yousaf, S., Amin, I., Hakim, S., Mirza, M., et al. (2021). Seed inoculation of desert-PGPR induce biochemical alterations and develop resistance against water stress in wheat. Physiol. Plant. 172:990–1006. doi: 10.1111/ppl.13362
Keywords: climate change, heat stress, heat stress effect on plants, heat tolerant PGPRs, sustainable agriculture
Citation: Ahmad M, Imtiaz M, Shoib Nawaz M, Mubeen F and Imran A (2022) What Did We Learn From Current Progress in Heat Stress Tolerance in Plants? Can Microbes Be a Solution? Front. Plant Sci. 13:794782. doi: 10.3389/fpls.2022.794782
Received: 14 October 2021; Accepted: 21 April 2022;
Published: 23 May 2022.
Edited by:
Eduardo V. Soares, Instituto Superior de Engenharia do Porto (ISEP), PortugalReviewed by:
Habib-ur-Rehman Athar, Bahauddin Zakariya University, PakistanCopyright © 2022 Ahmad, Imtiaz, Shoib Nawaz, Mubeen and Imran. This is an open-access article distributed under the terms of the Creative Commons Attribution License (CC BY). The use, distribution or reproduction in other forums is permitted, provided the original author(s) and the copyright owner(s) are credited and that the original publication in this journal is cited, in accordance with accepted academic practice. No use, distribution or reproduction is permitted which does not comply with these terms.
*Correspondence: Muhammad Imtiaz, bS5pbXRpYXpwazkyQGhvdG1haWwuY29t; Asma Imran, YXNtYWFzbGFtMjAwMUB5YWhvby5jb20=
Disclaimer: All claims expressed in this article are solely those of the authors and do not necessarily represent those of their affiliated organizations, or those of the publisher, the editors and the reviewers. Any product that may be evaluated in this article or claim that may be made by its manufacturer is not guaranteed or endorsed by the publisher.
Research integrity at Frontiers
Learn more about the work of our research integrity team to safeguard the quality of each article we publish.