- 1Department of Plant Science, North Dakota State University, Fargo, ND, United States
- 2USDA-Agricultural Research Service, Sugarcane Field Station, Canal Point, FL, United States
- 3Department of Plant Pathology, North Dakota State University, Fargo, ND, United States
- 4Department of Plant Pathology, Kansas State University, Manhattan, KS, United States
- 5Department of Global Development, Cornell University, Ithaca, NY, United States
- 6Department of Crop and Soil Sciences, Washington State University, Pullman, WA, United States
- 7USDA-Agricultural Research Service, Cereal Crops Research Unit, Edward T. Schafer Agricultural Research Center, Fargo, ND, United States
The necrotrophic fungal pathogen Pyrenophora tritici-repentis (Ptr) causes the foliar disease tan spot in both bread wheat and durum wheat. Wheat lines carrying the tan spot susceptibility gene Tsc1 are sensitive to the Ptr-produced necrotrophic effector (NE) Ptr ToxC. A compatible interaction results in leaf chlorosis, reducing yield by decreasing the photosynthetic area of leaves. Developing genetically resistant cultivars will effectively reduce disease incidence. Toward that goal, the production of chlorosis in response to inoculation with Ptr ToxC-producing isolates was mapped in two low-resolution biparental populations derived from LMPG-6 × PI 626573 (LP) and Louise × Penawawa (LouPen). In total, 58 genetic markers were developed and mapped, delineating the Tsc1 candidate gene region to a 1.4 centiMorgan (cM) genetic interval spanning 184 kb on the short arm of chromosome 1A. A total of nine candidate genes were identified in the Chinese Spring reference genome, seven with protein domains characteristic of resistance genes. Mapping of the chlorotic phenotype, development of genetic markers, both for genetic mapping and marker-assisted selection (MAS), and the identification of Tsc1 candidate genes provide a foundation for map-based cloning of Tsc1.
Introduction
Pyrenophora tritici-repentis (Died.) Drechs. (Ptr) is a necrotrophic homothallic ascomycete that causes the foliar disease tan spot in cultivated wheat, including common wheat (Triticum aestivum L., 2n = 6x = 42, AABBDD genomes), durum wheat [Triticum turgidum ssp. durum (Desf.) Husnot., 2n = 4x = 28, AABB genomes], and wild relatives (reviewed by Faris et al., 2013). Tan spot or yellow leaf spot, was first described as a minor pathogen in 1823 (Hosford Jr., 1982). Tan spot epidemics began in the 1970s, coinciding with the adoption of minimum tillage practices. Minimum tillage practices are believed to have caused an increase in disease incidence because Ptr overwinters on wheat residue, infecting crops the following season. Crop rotations and fungicide applications can reduce disease incidence and severity, but the most effective method for reducing disease incidence is through the development of genetically resistant varieties.
Pyrenophora tritici-repentis produces and secretes multiple necrotrophic effectors (NEs). The recognition of NEs by corresponding host sensitivity genes leads to a compatible interaction resulting in the development of necrotic and chlorotic lesions. The NEs and host sensitivity genes interact in an inverse gene-for-gene manner where the pathogen hijacks host defense pathways leading to necrotrophic effector triggered susceptibility (NETS; Liu et al., 2009; Friesen and Faris, 2010). These necrotic and chlorotic lesions reduce the photosynthetic area of the plant resulting in reduced kernel weight and grain number (Shabeer and Bockus, 1988).
Three host sensitivity gene-Ptr NE interactions have been characterized so far: Tsn1-Ptr ToxA, Tsc2-Ptr ToxB, and Tsc1-Ptr ToxC (reviewed by Faris et al., 2013). One host sensitivity gene, Tsn1 (Faris et al., 2010), and two NE genes, PtrToxA (Ballance et al., 1996; Ciuffetti et al., 1997) and PtrToxB (Martinez et al., 2001), have been cloned. The Tsn1-Ptr ToxA interaction produces necrosis, whereas the Tsc2-Ptr ToxB and Tsc1-Ptr ToxC interactions produce chlorosis. Ptr isolates are classified into races depending on their virulence patterns on a set of host differentials (reviewed by Faris et al., 2013).
In addition to the inverse gene-for-gene interactions, five tan spot resistance genes have also been identified (reviewed by Faris et al., 2013) including a major dominant gene, Tsr7, that confers race-nonspecific resistance in both tetraploid and hexaploid wheat (Faris et al., 2020). The other tan spot resistance genes, tsr2 (Singh et al., 2006), tsr3 (Tadesse et al., 2006a), tsr4 (Tadesse et al., 2006b), and tsr5 (Singh et al., 2008), confer recessive resistance. It is therefore possible that they are recessive alleles of host sensitivity genes that interact with yet unidentified NEs (reviewed in Faris et al., 2013).
A quantitative trait locus (QTL, QTsc.ndsu-1A) associated with resistance to chlorosis induced by Ptr ToxC-producing isolates was first identified in the International Triticeae Mapping Initiative W-7984 × Opata 85 recombinant inbred line (RIL) population (Faris et al., 1997; Effertz et al., 2002). The same QTL was shown to coincide with Ptr ToxC sensitivity (Effertz et al., 2002), and the gene underlying sensitivity was designated Tsc1. Ptr ToxC was predicted to be a small non-ionic, polar, molecule that induces chlorosis on wheat varieties possessing Tsc1.
A QTL designated QTs.zhl-1A was mapped to chromosome arm 1AS in two RIL populations (Kariyawasam et al., 2016; Liu et al., 2017) corresponding to the position of QTsc.ndsu-1A. In a RIL population derived from the cross Louise × Penawawa (LouPen; Carter et al., 2020), QTs.zhl-1A was associated with diseased caused by race 1, race 3, and AR CrossB10 Ptr isolates and explained up to 22% of the phenotypic variation (Kariyawasam et al., 2016). F1 plants from the same cross exhibited chlorosis after inoculation with the race 3 isolate 331-9, indicating that the chlorosis was conferred by a dominant susceptibility gene as opposed to the lack of a dominant resistance gene. In the LMPG-6 × PI 626573 (LP) RIL population, QTs.zhl-1A was associated with susceptibility explaining up to 27% of the variation in disease.
Additional QTL corresponding to the Tsc1 region have been identified in many hexaploid populations including, but not limited to, the biparental populations TA161-L1 × TAM105 (Kalia et al., 2018), IGW2547 × Annuello (Shankar et al., 2017), and Ernie × Betavia (Li et al., 2011), and a MAGIC population derived from Event, BAYP4535, Ambition, Firl3565, Format, Potenzial, Bussard, and Julius (Stadlmeier et al., 2019). A meta-QTL analysis identified two meta-QTLs in the Tsc1 region. However, they likely both correspond to Tsc1 (Liu et al., 2020). QTL in the Tsc1 region have also been identified in durum wheat. In a worldwide collection of durum wheat, a recent evaluation using a Ptr ToxC-producing isolate revealed a QTL, likely corresponding to Tsc1, on the short arm of chromosome 1A (Galagedara et al., 2020).
Wheat lines containing the Tsc1 gene exhibit a large amount of chlorosis resulting in severe tan spot susceptibility when infected with Ptr ToxC-containing isolates (Figure 1). Our long-term goal is to clone the Tsc1 gene using a map-based approach to gain a better understanding of the Tsc1-Ptr ToxC interaction at the molecular level. Toward this goal, the objectives of the current research were to: (1) develop molecular markers and saturated genetic linkage maps of the genomic region containing the Tsc1 gene, (2) define and characterize the genetic and physical interval containing the Tsc1 locus, and (3) identify candidate genes for Tsc1 in the wheat reference genome sequence. Achievement of these objectives provides a strong foundation for launching the next phase of objectives toward map-based cloning of Tsc1.
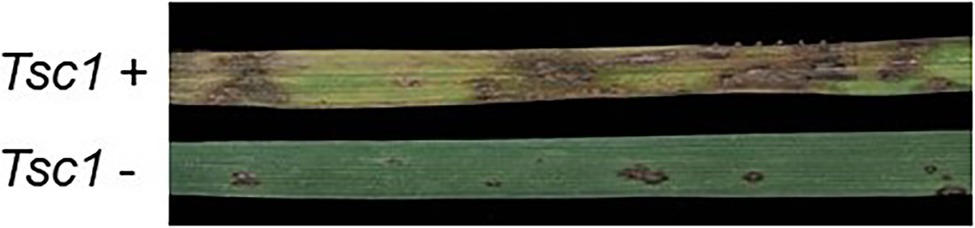
Figure 1. Leaves of wheat genotypes with Tsc1 (top) and without Tsc1 (bottom) inoculated with a Pyrenophora tritici-repentis (Ptr) ToxC-producing isolate.
Materials and Methods
Plant Materials
The LouPen and LP biparental populations were used to map newly developed markers within the Tsc1 region. Louise and LMPG-6 exhibit extensive chlorosis when inoculated with Ptr ToxC-producing isolates because they carry the dominant Tsc1 allele, whereas Penawawa and PI 626573 are free of chlorosis when inoculated with the same isolates because they harbor the recessive tsc1 allele (Kariyawasam et al., 2016; Liu et al., 2017). The LouPen population consists of 188 RILs and was originally developed to map stripe rust resistance derived from Louise (Carter et al., 2009). The LP population consists of 240 RILs and was originally developed to map stem rust Ug99 resistance in PI 626573 (Zurn et al., 2014). Sixteen hexaploid varieties were genotyped with markers closely linked to Tsc1 to test the usefulness of markers for marker-assisted selection (MAS; Table 1).
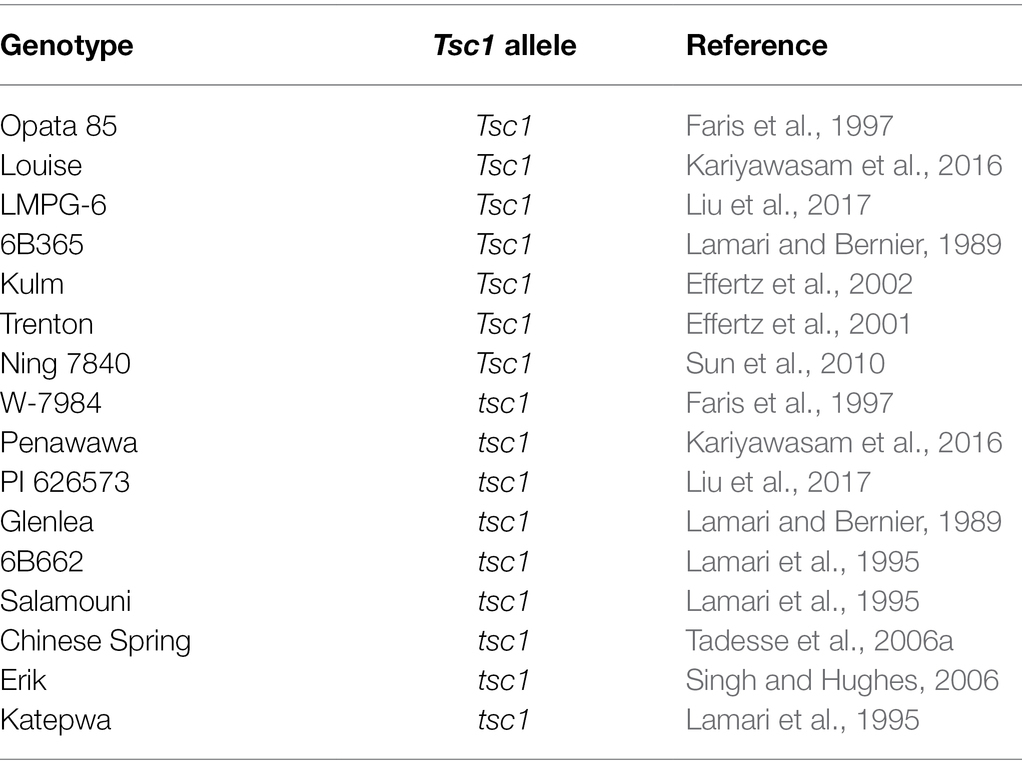
Table 1. Allelic state and corresponding references of hexaploid genotypes evaluated with markers developed in this research and linked to Tsc1.
Inoculations and Disease Evaluation
The LouPen and LP populations were inoculated with the Ptr ToxC-producing race 3 isolate 331-9 in Kariyawasam et al. (2016) and Liu et al. (2017), respectively. Although previously unreported, data on the presence and absence of chlorosis induced by isolate 331-9 were collected, and that data were used here to map chlorosis induction as a qualitative trait representing the Tsc1 locus in both populations.
Marker Development and Tsc1 Mapping
The LouPen and LP populations were previously genotyped with the wheat 9 K iSelect Assay BeadChip (Cavanagh et al., 2013), and whole genome maps were assembled (Zurn et al., 2014; Kariyawasam et al., 2016). Several methods were used to develop and/or identify additional markers within the Tsc1 genomic region of chromosome 1A. First, simple sequence repeat (SSR) markers previously mapped and known to detect loci on chromosome arm 1AS were identified from the Graingenes database.1
Second, contextual sequences of SNP markers derived from the 9 and 90 K arrays known to map to the short arm of chromosome 1A were used as queries in BLASTn searches of either Chinese Spring survey sequences (International Wheat Genome Sequencing Consortium (IWGSC), 2014), the Chinese Spring reference genome v1.0 (International Wheat Genome Sequencing Consortium (IWGSC) et al., 2018), or the wild emmer wheat genome sequence of Zavitan (Avni et al., 2017). The corresponding survey sequences and approximately 10 kb segments of the Chinese Spring and Zavitan genome sequences encompassing the SNP BLAST hits were then subjected to searches for SSRs using SSRIT2 and gene-like or low-copy DNA features by using the survey sequence or extracted genome segment sequence as a query in BLASTx searches against the NCBI non-redundant database.3 SSRs and gene-like features were used to develop SSR and sequence-tagged site (STS) markers, respectively, and primers were designed using Primer 3 (Rozen and Skaletsky, 1999).
Third, a genome-wide association study of tan spot resistance in durum wheat (Galagedara et al., 2020) revealed a genotype-by-sequencing (GBS) marker on chromosome arm 1AS associated with reaction to the Ptr ToxC-producing isolate Pti2 and was therefore likely associated with Tsc1. We used the sequence of this GBS marker to develop a semi-thermal asymmetric reverse PCR (STARP) marker (Long et al., 2017) to map the locus in the LouPen and LP populations.
All markers were amplified via PCR and electrophoresed on 6% nondenaturing polyacrylamide gels. Gels were stained with Gelred™ nucleic acid stain (Biotium Corporate, Hayward, CA, United States), and scanned with a Typhoon 9410 or FLA 9500 variable mode imager (GE healthcare Biosciences, Waukesha, WI, United States). Genetic linkage maps were constructed in MapDisto v2.1.7 (Heffelfinger et al., 2017) as described in Faris et al. (2014). Maps were visualized in MapChart 2.32 (Voorrips, 2002). All PCR primers used for the identification of markers in this research are listed in Supplementary Table 1.
Identification of Candidate Genes
The closest flanking markers to Tsc1 (fcp730 and fcp734) in the LouPen genetic map were used to identify candidate regions in the Chinese Spring v2.1 assembly (Zhu et al., 2021). High- and low-confidence annotated genes in the Chinese Spring v2.1 reference assembly were considered for analysis of protein domains (accessed December 7, 2021). Conserved protein domains of the annotated genes were identified by searching the Pfam database.4 Genes less than 500 bp long or those with no Pfam hits more significant than 1 × 10−5 were considered pseudogenes or gene fragments and were excluded from further analysis.
Results
Saturation Mapping of the Tsc1 Locus
In the first LouPen genetic map, Tsc1 mapped distal to the 9 K SNP markers IWA4643, IWA414, IWA3680, and IWA1388, thus placing Tsc1 within the first 15.2 Mb of the Chinese Spring v2.1 chromosome 1A short arm. Testing of SSR markers previously mapped to chromosome 1AS in other wheat mapping populations identified six markers polymorphic between Louise and Penawawa (Supplementary Table 1). Amplicon sequence analysis revealed the SSR markers gpw7072 and psp2999 targeted the same locus (data not shown). Once these six SSR markers were added to the genetic linkage map, the Tsc1 region was narrowed to approximately the first 5 Mb of the physical map. At this point, all markers mapped proximal to Tsc1, and more markers needed to be developed, particularly distal to Tsc1, to delineate the Tsc1 region.
Prior to the availability of the whole genome reference sequence of the hexaploid wheat cultivar Chinese Spring, SNPs from the 9 and 90 K SNP arrays known to map to chromosome 1AS were used to identify Chinese Spring survey sequences. Twelve STS markers and two SSR markers designed from the survey sequences were polymorphic and mapped in the LouPen population (Supplementary Table 1). An additional three SSRs and one STS were designed from the Zavitan genome assembly as well as 10 SSRs from the Chinese Spring reference v1.0. Some of the newly designed markers mapped distal to Tsc1 and further delineated the Tsc1 region. Tsc1 cosegregated with two markers, and the candidate gene region based on the genetic map constructed in the LouPen population was 184 kb. In total, the LouPen genetic map spanned 31.8 centiMorgans (cM) with 42 loci and had a marker density of 1.32 markers/cM (Figure 2).
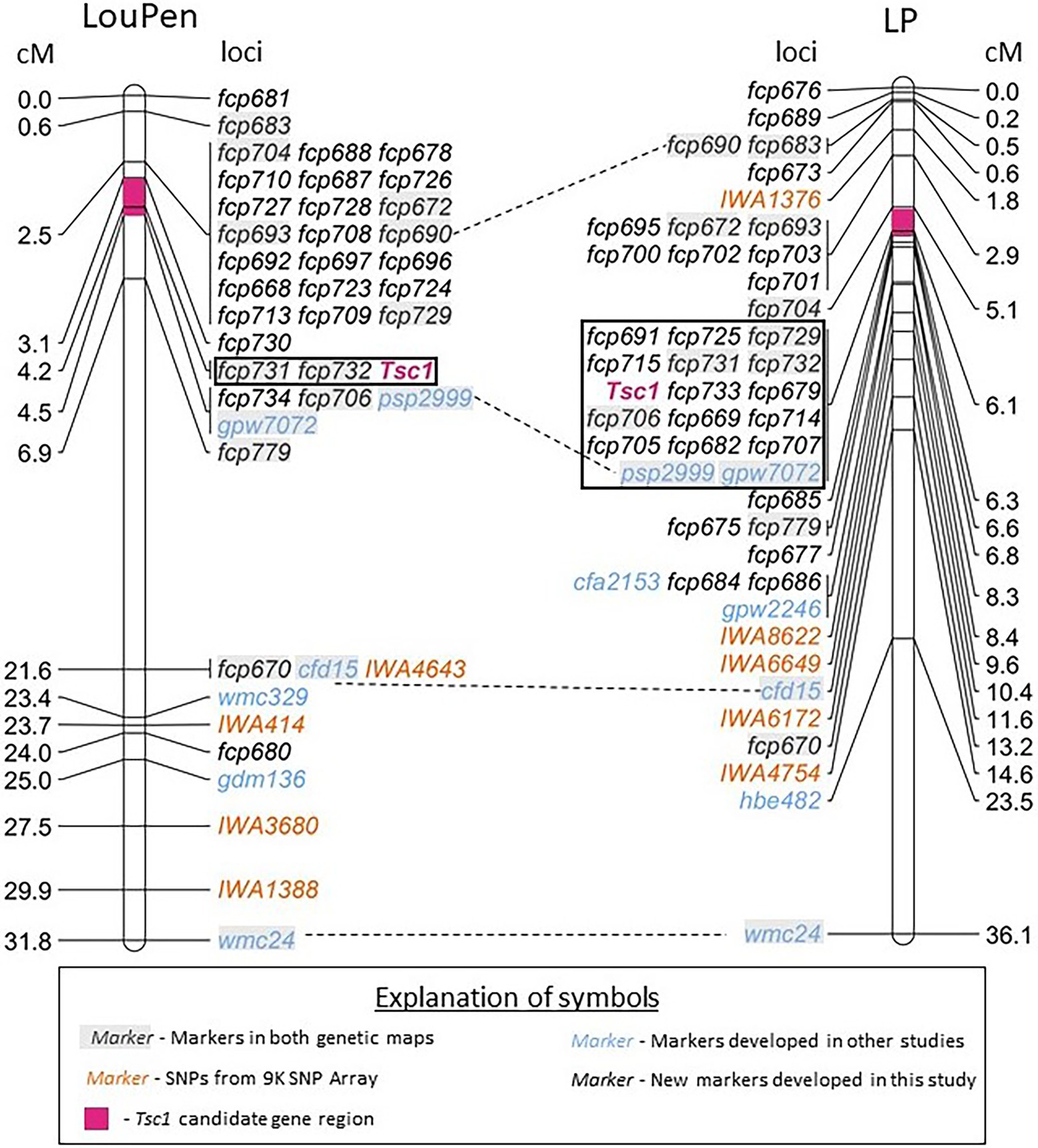
Figure 2. Saturation maps of the Tsc1 region developed in Louise × Penawawa (LouPen) and LMPG-6 × PI 626573 (LP) populations. The LouPen genetic map is on the left and the LP genetic map is on the right. Loci mapped are listed on the right of the LouPen genetic map and the left of the LP genetic map. Opposite the loci, the genetic distances are displayed in centiMorgans (cM). Markers in orange are SNP markers from the wheat 9 K iSelect Assay BeadChip. Markers in black are simple sequence repeat (SSR) markers designed in this study. Blue markers were designed in other studies. Dashed lines connect markers mapped in both populations. The black outlined rectangle indicates the loci cosegregating with Tsc1. The pink shaded portion of the chromosome represents the candidate gene region in each population.
The initial genetic map of the LP population placed Tsc1 within a ~7.2 Mb region of the short arm of chromosome 1A between the 9 K SNP markers IWA1376 and IWA8622. Seven previously mapped SSR markers were polymorphic between LMPG-6 and PI 626573, including four that were included in the LouPen genetic map. The inclusion of these seven markers on the LP genetic linkage map delineated the Tsc1 region to 3.9 Mb on the physical map.
To reduce the candidate gene region further, additional markers were designed in the same manner as they were for mapping in the LouPen population. Fourteen STS and five SSR markers designed from the Chinese Spring survey sequences and eight SSR and two STS markers derived from the Zavitan genome assembly were mapped in the LP population (Supplementary Table 1). An additional five SSR markers designed from the Chinese Spring reference v1.0 were added to the LP genetic map. These additional STS and SSR markers reduced the candidate gene region to approximately 1 Mb, an order of magnitude larger than the candidate gene region defined by mapping in the LouPen population. The LP map consisted of 47 loci spanning 36.1 cM, which gives a marker density of 1.30 markers/cM (Figure 2).
Recombination rates were compared between the LP and LouPen populations within the mapped regions to determine which population delineated the Tsc1 locus to the smallest genomic region, or if a composite of the two maps could be used to define the Tsc1 locus to a smaller region. The most distal and proximal markers in common between the two maps were fcp683 and wmc24, respectively. The region defined by these markers encompassed 26.3 Mb on the Chinese Spring v2.1 reference genome, and it spanned 31.2 and 35.5 cM of genetic distance in the LouPen and LP populations, respectively. Therefore, the recombination rate across this region was higher in the LP population (1.35 cM/Mb) compared to the LouPen population (1.19 cM/Mb).
Comparison of recombination rates in the vicinity of the Tsc1 locus revealed a different scenario. The markers fcp704 and fcp779, which were the two markers in common to both maps that detect recombination events most closely flanking Tsc1 on the distal and proximal sides, respectively, were separated by 4.4 cM on the LouPen map and 1.5 cM on the LP map (Figure 2). Unfortunately, the amplicon sequence for fcp704 was not present in the Chinese Spring v2.1 genome making it impossible to determine the physical distance between these common flanking markers. The next closest marker on the distal side of Tsc1 common to both maps and present in Chinese Spring was fcp693. The genetic distances between fcp779 and fcp693 in the LouPen and LP populations were 4.4 and 3.7 cM, respectively. The physical distance between these two markers in the Chinese Spring reference genome was 5.7 Mb, which translates to 0.77 cM/Mb in the LouPen population and 0.64 cM/Mb in the LP population. Therefore, the recombination frequency near the Tsc1 locus was higher in the LouPen population compared to the LP population.
The genetic order of the markers in LouPen was compared to the physical order in the Chinese Spring v2.1 reference genome due to the higher genetic resolution near Tsc1 (Figure 3). There were two instances of non-collinearity. Firstly, marker fcp683 mapped more distal in LouPen than its physical position, which would place it within the markers cosegregating at 2.5 cM. On the proximal side of Tsc1, the markers IWA414 and fcp680 were inverted relative to their physical position. These minor inconsistencies between genetic and physical order of the markers are indicative of rearrangements in the Chinese Spring genome relative to Louise and Penawawa. However, the rearrangements do not encompass or alter the candidate gene region.
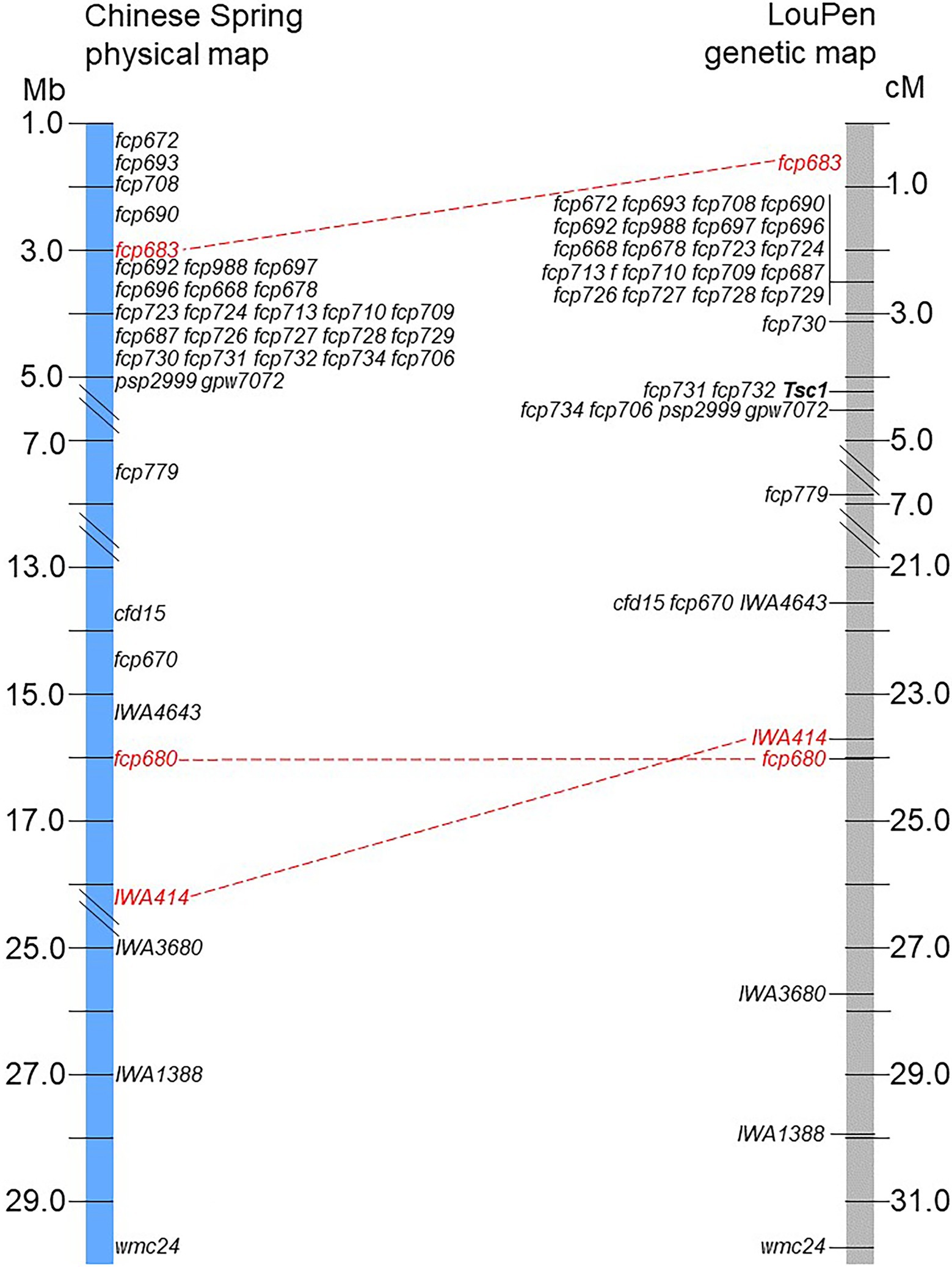
Figure 3. Comparison of the physical and genetic order of markers. The LouPen genetic map is on the right and the Chinese Spring v2.1 physical map is on the left. Markers in red font connected by red dashed lines are not colinear. All other markers are colinear.
Delineation of the Candidate Gene Region and Identification of Candidate Genes
In the LouPen population, the Tsc1 candidate gene region was delineated by fcp730 and fcp734, which were 1.4 cM apart (Figure 2). This region corresponded to approximately 184 kb in the Chinese Spring reference v2.1 genome (Figure 4). Two markers, fcp732 and fcp731 cosegregated with Tsc1, and they spanned just 17 kb.
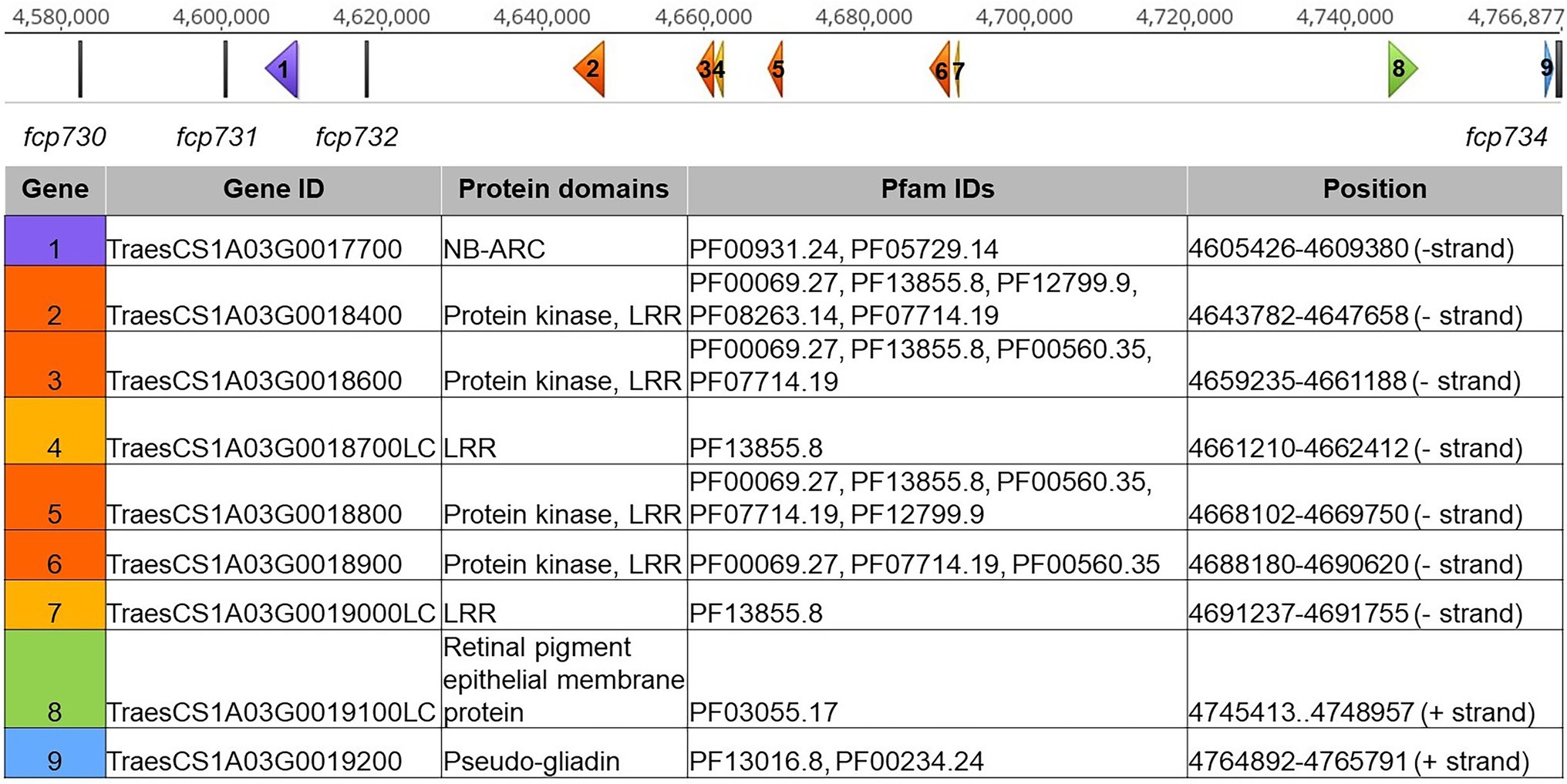
Figure 4. Tsc1 candidate gene region in Chinese Spring reference genome v2.1. The scale on the top represents the physical position in base pairs. Genetic markers are displayed as vertical gray bars. Genes are displayed as arrows, labeled 1–5, corresponding to the genes in the table below. Genes with nucleotide binding and ARC (NB-ARC), protein kinase (PK) and leucine rich repeat (LRR), LRR, retinal pigment epithelial membrane, and gliadin domains are shown in purple, orange, yellow, green, and blue, respectively. Gene IDs, protein domains, Pfam IDs, and physical positions of each gene are included in the table.
The candidate gene region, delineated by fcp704 and fcp685, was larger in the LP population. As fcp704 is not in the Chinese Spring reference genome, the next closest marker, fcp701, was selected to delineate the candidate gene region to 3.9 Mb in the LP population. The 16 markers that cosegregated with Tsc1 spanned a total of 967 kb in the Chinese Spring v2.1 reference genome.
Given this finding, the delineated region on the genetic map developed in the LouPen population was used to define the Tsc1 candidate region and to identify candidate genes based on the Chinese Spring reference sequence (Figure 4). No genes were identified between the distal flanking marker fcp730 and the markers fcp731 and fcp732, which cosegregated with Tsc1. A gene containing nucleotide binding and ARC (NB-ARC) domains was identified between fcp731 and fcp732. Between fcp732 and the proximal flanking marker, fcp734, there were four protein kinase and leucine rich repeat (PK-LRR) domain-containing genes and two genes with only an LRR domain. Two additional genes within this segment included a gene with a retinal pigment epithelial membrane protein domain and a pseudo-gliadin gene. The former was considered a gene fragment as it did not contain a start codon. A large family of gliadins is known to exist on chromosome 1A in wheat, so it is not surprising that a pseudo-gliadin was identified. However, gliadins have not been shown to be involved in disease resistance or susceptibility, and therefore the pseudo-gliadin gene was not considered a candidate. In total, nine genes were identified in Chinese Spring and seven are considered candidates including one NB-ARC, four PK-LRRs, and two LRR domain-containing genes (Figure 4).
Evaluation of Markers Closely Linked to Tsc1
To identify markers that could be potentially used for MAS of Ptr ToxC-insensitive lines, i.e., elimination of the dominant Tsc1 allele, markers closely linked to the Tsc1 locus were evaluated on a panel of hexaploid wheat lines on which phenotypic evaluations with Ptr ToxC-producing isolates has been conducted, and therefore the allelic status at the Tsc1 locus is known (Table 1). The markers fcp731 and fcp732, which cosegregated with Tsc1 in the LouPen population, were selected for evaluation as well as fcp729 and flanking markers fcp730, fcp734, and psp2999. Among the hexaploid lines evaluated, seven were resistant to chlorosis induced by Ptr ToxC-producing isolates of Ptr, and nine were susceptible and developed extensive chlorosis (Table 1).
Analysis of amplified fragments for these six markers revealed that no marker allele was associated with the allelic state of Tsc1 (Figure 5). The best association was with fcp732 where five out of nine resistant lines had null marker alleles.
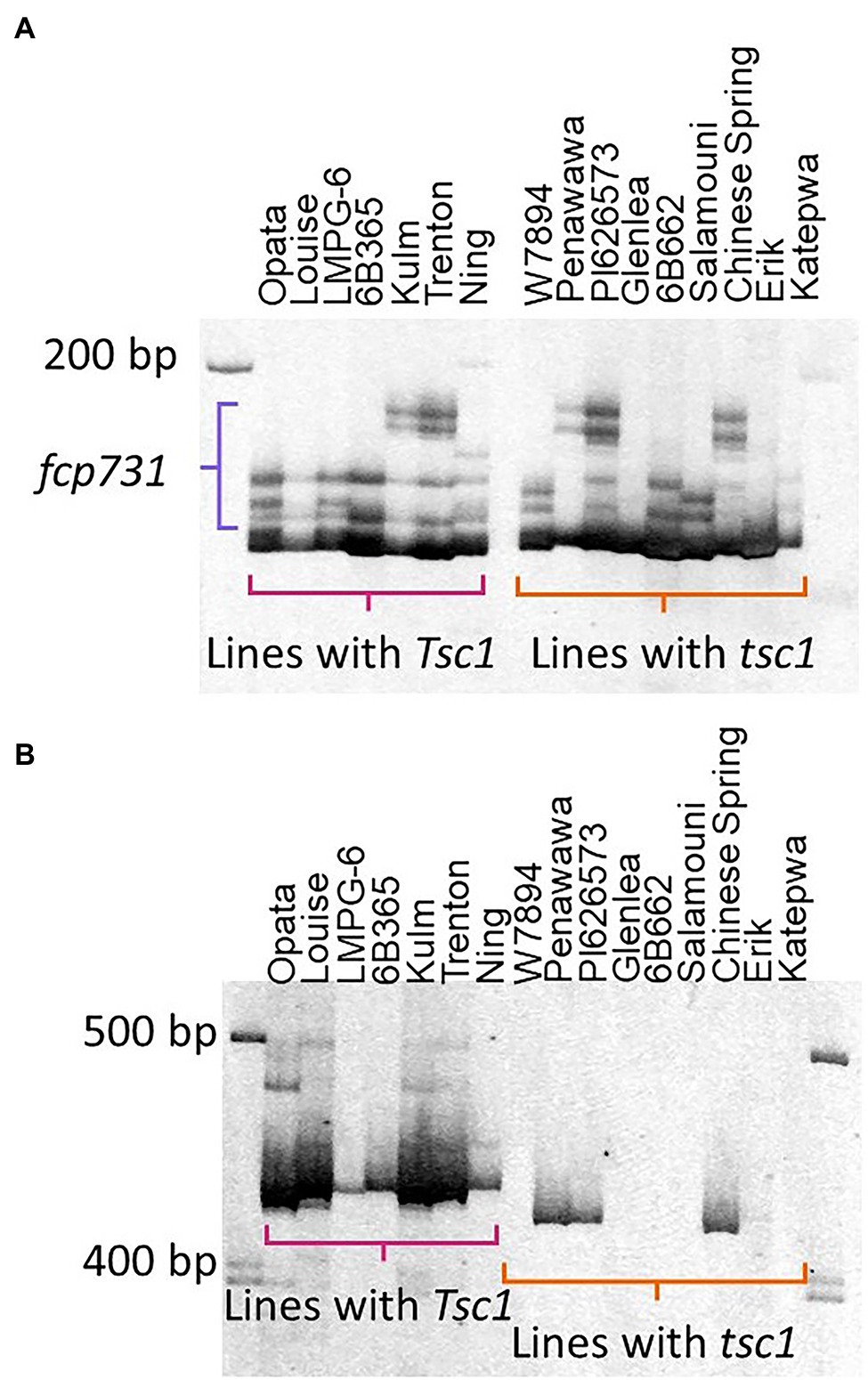
Figure 5. Evaluation of markers cosegregating with Tsc1. The polyacrylamide gel images of markers fcp731 (A) and fcp732 (B) run on lines with known sensitivity statuses (Table 1) are shown. Horizontal brackets in pink and orange denote amplicons in lines with Tsc1 and tsc1, respectively. The primary amplicon was scored for marker fcp732 (B). The amplicons denoted by the purple bracket were scored for marker fcp731 (A).
Discussion
The Tsc1-Ptr ToxC interaction plays a significant role in tan spot development in both hexaploid and tetraploid backgrounds (Galagedara et al., 2020; Liu et al., 2020). Identification of Tsc1 would allow further characterization of the Tsc1-ToxC interaction, including the molecular mechanisms underlying the interaction. While there are extensive tan spot QTL analyses, Tsn1 is the only host sensitivity gene cloned to date (Faris et al., 2010).
The markers in this study delineate Tsc1 to 1.2 cM in the LP population and 1.4 cM in the LouPen population. Interestingly, Tsc1 cosegregated with 16 markers in the LP population that spanned 967 kb, demonstrating reduced recombination in the LP population relative to the LouPen population. Although relative recombination rates indicate that future mapping would likely be more successful in the LouPen population than the LP population, candidate gene analysis indicates that future high-resolution mapping may be unnecessary.
Candidate gene analysis revealed seven genes with domains common among resistance genes, which are often hijacked by necrotrophic pathogens to induce disease (Faris and Friesen, 2020). The first candidate gene on the distal side of the candidate gene region is an NB-ARC domain-containing gene. NB-ARC proteins are a subclass within the protein super family “signal transduction ATPases with numerous domains” (STAND; Danot et al., 2009). Often, NB-ARC domain-containing proteins have an additional domain, like an LRR domain that acts as a sensor. Although the NB-ARC candidate gene in this region does not contain an LRR domain, it is still possible that it is involved in the recognition of Ptr ToxC or the signal transduction that leads to programmed cell death.
Between the markers that cosegregated with Tsc1 and the proximal flanking marker, there were four PK-LRR domain-containing genes and two genes containing only an LRR domain predicted in the Chinese Spring v2.1 reference genome. We consider the four PK-LRR genes to be the stronger candidates. The receptor-like protein kinase family can recognize signal peptides, either directly or indirectly, dimerize, and initiate signaling pathways through phosphorylation cascades (Butenko et al., 2009). All four PK-LRR genes have transmembrane domains and therefore, are likely cell surface proteins. Genetic mutation analysis of these candidate genes is underway to determine which of the seven candidates, if any, is Tsc1.
It is promising that many of the genes identified in the candidate gene region of Chinese Spring contain domains identified in previously characterized necrotrophic susceptibility genes (reviewed by Faris and Friesen, 2020). In the wheat-Parastagonospora nodorum pathosystem, three necrotrophic susceptibility genes have been cloned. The first, Tsn1, is also the tan spot susceptibility gene that confers susceptibility to isolates producing Ptr ToxA. Tsn1 has NB, PK, and LRR domains (Faris et al., 2010). The second cloned P. nodorum susceptibility gene, Snn1, is a wall-associated kinase. The third cloned P. nodorum susceptibility gene, Snn3-D1, is a protein kinase-major sperm domain-containing gene (Zhang et al., 2021). So far, all cloned necrotrophic susceptibility genes in wheat contain a PK domain. As such, we believe that the PK-LRR genes are stronger candidates for Tsc1.
Although the Tsc1 candidates in the Chinese Spring genome are logical susceptibility genes, it is possible that an allele of Tsc1 is not present in the Chinese Spring genome. Chinese Spring is not susceptible to Ptr ToxC-induced chlorosis, and therefore does not harbor a functional Tsc1 allele. We are characterizing the 10+ wheat genomes to determine if any of the sequenced wheat varieties are susceptible to Ptr ToxC chlorosis. Combining genomic analysis of the gene content, gene alleles, and the phenotypes across the 10+ wheat genomes may allow us to further reduce the number of Tsc1 candidate genes.
Analysis of markers closely linked to Tsc1 on a set of genotypes with known sensitivity statuses revealed multiple alleles for each marker as well as multiple haplotypes, suggesting that Tsc1 lies within a region of high recombination in natural populations. For example, marker fcp731, which cosegregated with Tsc1, had four alleles within the susceptible lines and five alleles within the resistant lines. This diversity in marker alleles was likely helpful in finding polymorphic markers to use in genetic mapping, but it are less useful in MAS. As such, it is not recommended that these markers be used to select resistant genotypes in a natural population. The markers may be suitable for selection within a breeding population where the susceptibility status of the parents is known and can be associated with a particular marker allele. Rearrangements on the proximal and distal sides of Tsc1 relative to the Chinese Spring v2.1 reference genome is further evidence that the Tsc1 region is a high recombination region, resulting in highly polymorphic markers, and increasing the difficulty in finding a marker that cosegregates with Tsc1 in a natural population. These findings emphasize the need for cloning the Tsc1 gene, which will allow the development of SNP markers based on causal polymorphisms within the gene itself and can be used to select genotypes that lack Tsc1 using high-throughput genotyping platforms.
Data Availability Statement
The datasets presented in this study can be found in the Supplementary Material.
Author Contributions
KR, AM, and JF initiated and planned the study and developed markers. MA and AC developed the mapping populations. GK and JZ performed initial genotyping analyses with SNP arrays. GK and ZL performed tan spot inoculation experiments and analyses. KR performed linkage and genomic analyses. KR and JF interpreted the data and wrote the manuscript. All authors reviewed and edited the manuscript.
Funding
This work was supported by the U.S. Department of Agriculture-Agricultural Research Service. USDA is an equal opportunity provider and employer.
Conflict of Interest
The authors declare that the research was conducted in the absence of any commercial or financial relationships that could be construed as a potential conflict of interest.
Publisher’s Note
All claims expressed in this article are solely those of the authors and do not necessarily represent those of their affiliated organizations, or those of the publisher, the editors and the reviewers. Any product that may be evaluated in this article, or claim that may be made by its manufacturer, is not guaranteed or endorsed by the publisher.
Acknowledgments
The authors thank Megan Overlander for technical support.
Supplementary Material
The Supplementary Material for this article can be found online at: https://www.frontiersin.org/articles/10.3389/fpls.2022.793925/full#supplementary-material
Supplementary Table 1 | Molecular markers developed for the saturation mapping of the Tsc1 locus.
Nomenclature
LP - LMPG-6 × PI626573; LouPen - Louise × Penawawa; NE - necrotrophic effector; Ptr - Pyrenophora tritici-repentis; SNP - single nucleotide polymorphism; SSR - simple sequence repeat; DH - doubled haploids; RIL - recombinant inbred line; QTL - quantitative trait locus.
Footnotes
1. ^https://wheat.pw.usda.gov/GG3/
2. ^https://archive.gramene.org/db/markers/ssrtool
References
Avni, R., Nave, M., Barad, O., Baruch, K., Twardziok, S. O., Gundlach, H., et al. (2017). Wild emmer genome architecture and diversity elucidate wheat evolution and domestication. Science 357, 93–97. doi: 10.1126/science.aan0032
Ballance, G. M., Lamari, L., Kowatsch, R., and Bernier, C. C. (1996). Cloning, expression and occurrence of the gene encoding the Ptr necrosis toxin from Pyrenophora tritici-repentis. Mol. Plant Pathol. doi: 10.1007/978-94-011-5218-1_21
Butenko, M. A., Vie, A. K., Brembu, T., Aalen, R. B., and Bones, A. M. (2009). Plant peptides in signalling: looking for new partners. Trends Plant Sci. 14, 255–263. doi: 10.1016/j.tplants.2009.02.002
Carter, A. H., Chen, X. M., Garland-Campbell, K., and Kidwell, K. K. (2009). Identifying QTL for high-temperature adult-plant resistance to stripe rust (Puccinia striiformis f. sp. tritici) in the spring wheat (Triticum aestivum L.) cultivar ‘Louise’. Theor. Appl. Genet. 119, 1119–1128. doi: 10.1007/s00122-009-1114-2
Carter, A. H., Kidwell, K. K., DeMacon, V. L., Shelton, G. B., Burke, A. B., Balow, K. A., et al. (2020). Registration of the Louise-Penawawa spring wheat recombinant inbred line mapping population. J. Plant Regist. 14, 474–480. doi: 10.1002/plr2.20077
Cavanagh, C. R., Chao, S., Wang, S., Huang, B. E., Stephen, S., Kiani, S., et al. (2013). Genome-wide comparative diversity uncovers multiple targets of selection for improvement in hexaploid wheat landraces and cultivars. Proc. Natl. Acad. Sci. U. S. A. 110, 8057–8062. doi: 10.1073/pnas.1217133110
Ciuffetti, L. M., Tuori, R. P., and Gaventa, J. M. (1997). A single gene encodes a selective toxin causal to the development of tan spot of wheat. Plant Cell 9, 135–144. doi: 10.1105/tpc.9.2.135
Danot, O., Marquenet, E., Vidal-Ingigliardi, D., and Richet, E. (2009). Wheel of life, wheel of death: a mechanistic insight into signaling by STAND proteins. Structure 17, 172–182. doi: 10.1016/j.str.2009.01.001
Effertz, R. J., Anderson, J. A., and Francl, L. J. (2001). Restriction fragment length polymorphism mapping of resistance to two races of Pyrenophora tritici-repentis in adult and seedling wheat. Phytopathology 91, 572–578. doi: 10.1094/PHYTO.2001.91.6.572
Effertz, R. J., Meinhardt, S. W., Anderson, J. A., Jordahl, J. G., and Francl, L. J. (2002). Identification of a chlorosis-inducing toxin from Pyrenophora tritici-repentis and the chromosomal location of an insensitivity locus in wheat. Phytopathology 92, 527–533. doi: 10.1094/PHYTO.2002.92.5.527
Faris, J. D., Anderson, J. A., Francl, L. J., and Jordahl, J. G. (1997). RFLP mapping of resistance to chlorosis induction by Pyrenophora tritici-repentis in wheat. Theor. Appl. Genet. 94, 98–103. doi: 10.1007/s001220050387
Faris, J. D., and Friesen, T. L. (2020). Plant genes hijacked by necrotrophic fungal pathogens. Curr. Opin. Plant Biol. 56, 74–80. doi: 10.1016/j.pbi.2020.04.003
Faris, J. D., Liu, Z., and Xu, S. S. (2013). Genetics of tan spot resistance in wheat. Theor. Appl. Genet. 126, 2197–2217. doi: 10.1007/s00122-013-2157-y
Faris, J. D., Overlander, M. E., Kariyawasam, G. K., Carter, A., Xu, S. S., and Liu, Z. (2020). Identification of a major dominant gene for race-nonspecific tan spot resistance in wild emmer wheat. Theor. Appl. Genet. 133, 829–841. doi: 10.1007/s00122-019-03509-8
Faris, J. D., Zhang, Q., Chao, S., Zhang, Z., and Xu, S. S. (2014). Analysis of agronomic and domestication traits in a durum × cultivated emmer wheat population using a high-density single nucleotide polymorphism-based linkage map. Theor. Appl. Genet. 127, 2333–2348. doi: 10.1007/s00122-014-2380-1
Faris, J. D., Zhang, Z., Lu, H., Lu, S., Reddy, L., Cloutier, S., et al. (2010). A unique wheat disease resistance-like gene governs effector-triggered susceptibility to necrotrophic pathogens. Proc. Natl. Acad. Sci. U. S. A. 107, 13544–13549. doi: 10.1073/pnas.1004090107
Friesen, T. L., and Faris, J. D. (2010). Characterization of the wheat- Stagonospora nodorum disease system: what is the molecular basis of this quantitative necrotrophic disease interaction? Can. J. Plant Pathol. 32, 20–28. doi: 10.1080/07060661003620896
Galagedara, N., Liu, Y., Fiedler, J., Shi, G., Chiao, S., Xu, S. S., et al. (2020). Genome-wide association mapping of tan spot resistance in a worldwide collection of durum wheat. Theor. Appl. Genet. 133, 2227–2237. doi: 10.1007/s00122-020-03593-1
Heffelfinger, C., Fragoso, C. A., and Lorieux, M. (2017). Constructing linkage maps in the genomics era with MapDisto 2.0. Bioinformatics 33, 2224–2225. doi: 10.1093/bioinformatics/btx177
Hosford, R. M. Jr. (1982). “Tan spot—developing knowledge 1902–1981, virulent races and differentials, methodology, rating systems, other leaf diseases, literature” in Tan Spot of Wheat and Related Diseases Workshop. ed. R. M. Hosford Jr. (Fargo: North Dakota Agric Exp Station), 1–24.
International Wheat Genome Sequencing Consortium (IWGSC) (2014). A chromosome-based draft sequence of the hexaploid bread wheat (Triticum aestivum) genome. Science 345:1251788. doi: 10.1126/science.1251788
International Wheat Genome Sequencing Consortium (IWGSC),IWGSC RefSeq principal 409 investigators, Appels, R., Eversole, K., Feuillet, C., Keller, B., et al. (2018). Shifting the limits in wheat research and breeding using a fully annotated reference genome. Science 361:eaar7191. doi: 10.1126/science.aar7191
Kalia, B., Bockus, W. W., Singh, S., Tiwari, V. K., and Gill, B. S. (2018). Mapping of quantitative trait loci for resistance to race 1 of Pyrenophora tritici-repentis in synthetic hexaploid wheat. Plant Breed. 137, 313–319. doi: 10.1111/pbr.12586
Kariyawasam, G. K., Carter, A. H., Rasmussen, J. B., Faris, J., Xu, S. S., Mergoum, M., et al. (2016). Genetic relationships between race-nonspecific and race-specific interactions in the wheat–Pyrenophora tritici-repentis pathosystem. Theor. Appl. Genet. 129, 897–908. doi: 10.1007/s00122-016-2670-x
Lamari, L., and Bernier, C. C. (1989). Virulence of isolates of Pyrenophora tritici-repentis on 11 wheat cultivars and cytology of the differential host reactions. Can. J. Plant Pathol. 11, 284–290. doi: 10.1080/07060668909501114
Lamari, L., Sayoud, R., Boulif, M., and Bernier, C. C. (1995). Identification of a new race in Pyrenophora tritici-repentis: implications for the current pathotype classification system. Can. J. Plant Pathol. 17, 312–318. doi: 10.1080/07060669509500668
Li, H. B., Yan, W., Liu, G. R., Wen, S. M., and Liu, C. J. (2011). Identification and validation of quantitative trait loci conferring tan spot resistance in the bread wheat variety Ernie. Theor. Appl. Genet. 122, 395–403. doi: 10.1007/s00122-010-1455-x
Liu, Z., Faris, J. D., Oliver, R. P., Tan, K.-C., Solomon, P. S., McDonald, M. C., et al. (2009). SnTox3 acts in effector triggered susceptibility to induce disease on wheat carrying the Snn3 gene. PLoS Pathog. 5:e1000581. doi: 10.1371/journal.ppat.1000581
Liu, Y., Salsman, E., Wang, R., Galagedara, N., Zhang, Q., Fiedler, J. D., et al. (2020). Meta-QTL analysis of tan spot resistance in wheat. Theor. Appl. Genet. 133, 2363–2375. doi: 10.1007/s00122-020-03604-1
Liu, Z., Zurn, J. D., Kariyawasam, G., Faris, J. D., Shi, G., Hansen, J., et al. (2017). Inverse gene-for-gene interactions contribute additively to tan spot susceptibility in wheat. Theor. Appl. Genet. 130, 1267–1276. doi: 10.1007/s00122-017-2886-4
Long, Y. M., Chao, W. S., Ma, G. J., Xu, S. S., and Qi, L. L. (2017). An innovative SNP genotyping method adapting to multiple platforms and throughputs. Theor. Appl. Genet. 130, 597–607. doi: 10.1007/s00122-016-2838-4
Martinez, J. P., Ottum, S. A., Ali, S., Francl, L. J., and Ciuffetti, L. M. (2001). Characterization of the ToxB gene from Pyrenophora tritici-repentis. Mol. Plant-Microbe Interact. 14, 675–677. doi: 10.1094/MPMI.2001.14.5.675
Rozen, S., and Skaletsky, H. (1999). “Primer3 on the WWW for general users and for biologist programmers,” in Bioinformatics Methods and Protocols. eds. Misener S. and Krawetz S. A. (New Jersey: Humana Press), 365–386.
Shabeer, A., and Bockus, W. W. (1988). Tan spot effects on yield and yield components relative to growth stage in winter wheat. Plant Dis. 72:599. doi: 10.1094/PD-72-0599
Shankar, M., Jorgensen, D., Taylor, J., Chalmers, K. J., Fox, R., Hollaway, G. J., et al. (2017). Loci on chromosomes 1A and 2A affect resistance to tan (yellow) spot in wheat populations not segregating for tsn1. Theor. Appl. Genet. 130, 2637–2654. doi: 10.1007/s00122-017-2981-6
Singh, P. K., Gonzalez-Hernandez, J. L., Mergoum, M., Ali, S., Adhikari, T. B., Kianian, S. F., et al. (2006). Identification and molecular mapping of a gene conferring resistance to Pyrenophora tritici-repentis race 3 in tetraploid wheat. Phytopathology 96, 885–889. doi: 10.1094/PHYTO-96-0885
Singh, P. K., and Hughes, G. R. (2006). Inheritance of resistance to the chlorosis component of tan spot of wheat caused by Pyrenophora tritici-repentis, races 1 and 3. Euphytica 152, 413–420. doi: 10.1007/s10681-006-9229-x
Singh, P. K., Mergoum, M., Gonzalez-Hernandez, J. L., Ali, S., Adhikari, T. B., Kianian, S. F., et al. (2008). Genetics and molecular mapping of resistance to necrosis inducing race 5 of Pyrenophora tritici-repentis in tetraploid wheat. Mol. Breed. 21, 293–304. doi: 10.1007/s11032-007-9129-3
Stadlmeier, M., Jørgensen, L. N., Corsi, B., Cockram, J., Hartl, L., and Mohler, V. (2019). Genetic dissection of resistance to the three fungal plant pathogens Blumeria graminis, Zymoseptoria tritici, and Pyrenophora tritici-repentis using a multiparental winter wheat population. G3 9, 1745–1757. doi: 10.1534/g3.119.400068
Sun, X.-C., Bockus, W., and Bai, G. (2010). Quantitative trait loci for resistance to Pyrenophora tritici-repentis race 1 in a Chinese wheat. Phytopathology 100, 468–473. doi: 10.1094/PHYTO-100-5-0468
Tadesse, W., Hsam, S. L. K., Wenzel, G., and Zeller, F. J. (2006a). Identification and monosomic analysis of tan spot resistance genes in synthetic wheat lines (Triticum turgidum L. × Aegilops tauschii Coss.). Crop Sci. 46, 1212–1217. doi: 10.2135/cropsci2005.10-0396
Tadesse, W., Hsam, S. L. K., and Zeller, F. J. (2006b). Evaluation of common wheat cultivars for tan spot resistance and chromosomal location of a resistance gene in the cultivar “Salamouni.” Plant Breed. 125, 318–322. doi: 10.1111/j.1439-0523.2006.01243.x
Voorrips, R. E. (2002). MapChart: software for the graphical presentation of linkage maps and QTLs. J. Hered. 93, 77–78. doi: 10.1093/jhered/93.1.77
Zhang, Z., Running, K. L. D., Seneviratne, S., Peters Haugrud, A. R., Szabo-Hever, A., Shi, G., et al. (2021). A protein kinase–major sperm protein gene hijacked by a necrotrophic fungal pathogen triggers disease susceptibility in wheat. Plant J. 106, 720–732. doi: 10.1111/tpj.15194
Zhu, T., Wang, L., Rimbert, H., Rodriguez, J. C., Deal, K. R., De Oliveira, R., et al. (2021). Optical maps refine the bread wheat Triticum aestivum cv Chinese spring genome assembly. Plant J. 107, 303–314. doi: 10.1111/tpj.15289
Keywords: tan spot, wheat, Triticum, Pyrenophora tritici-repentis, Ptr ToxC, Tsc1, disease resistance
Citation: Running KLD, Momotaz A, Kariyawasam GK, Zurn JD, Acevedo M, Carter AH, Liu Z and Faris JD (2022) Genomic Analysis and Delineation of the Tan Spot Susceptibility Locus Tsc1 in Wheat. Front. Plant Sci. 13:793925. doi: 10.3389/fpls.2022.793925
Edited by:
Marco Maccaferri, University of Bologna, ItalyReviewed by:
Nikolai Maria Adamski, John Innes Center, United KingdomAwais Rasheed, Quaid-i-Azam University, Pakistan
Francis Chuks Ogbonnaya, Grains Research and Development Corporation, Australia
Copyright © 2022 Running, Momotaz, Kariyawasam, Zurn, Acevedo, Carter, Liu and Faris. This is an open-access article distributed under the terms of the Creative Commons Attribution License (CC BY). The use, distribution or reproduction in other forums is permitted, provided the original author(s) and the copyright owner(s) are credited and that the original publication in this journal is cited, in accordance with accepted academic practice. No use, distribution or reproduction is permitted which does not comply with these terms.
*Correspondence: Justin D. Faris, anVzdGluLmZhcmlzQHVzZGEuZ292