- 1Co-Innovation Center for Sustainable Forestry in Southern China, Nanjing Forestry University, Nanjing, China
- 2The Key Laboratory of Biotechnology for Medicinal Plants of Jiangsu Province, School of Life Science, Jiangsu Normal University, Xuzhou, China
- 3School of Agricultural Sciences, Zhengzhou University, Zhengzhou, China
The ascomycete Colletotrichum gloeosporioides is a causal agent of anthracnose on crops and trees and causes enormous economic losses in the world. Protein kinases have been implicated in the regulation of growth and development, and responses to extracellular stimuli. However, the mechanism of the protein kinases regulating phytopathogenic fungal-specific processes is largely unclear. In the study, a serine/threonine CgSat4 was identified in C. gloeosporioides. The CgSat4 was localized in the cytoplasm. Targeted gene deletion showed that CgSat4 was essential for vegetative growth, sporulation, and full virulence. CgSat4 is involved in K+ uptake by regulating the localization and expression of the potassium transporter CgTrk1. CgSat4 is required for the cation stress resistance by altering the phosphorylation of CgHog1. Our study provides insights into potassium acquisition and the pathogenesis of C. gloeosporioides.
Introduction
Protein phosphorylation catalyzed by protein kinases is essential for the regulation of growth, development, and responses to extracellular stimuli in eukaryotic cells (Wang et al., 2011; Chen et al., 2017). Based on the catalytic domains, protein kinases have been divided into several families, including the STE (homologs of yeast Sterile 7, Sterile 11, Sterile 20 kinases), CK1 (casein kinase 1), CAMK (Ca2+/calmodulin-dependent protein kinase), CMGC (cyclin-dependent, mitogen-activated, glycogen synthase, and cyclin-dependent protein kinase-like kinases), AGC (protein kinase A, G, and C families), HisK (histidine kinase), RGC (receptor guanylate cyclase kinases), TK (tyrosine kinases), TKL (tyrosine like kinases), atypical families, and others (Miranda-Saavedra and Barton, 2007; De Souza et al., 2013). Several transcription factors, enzymes, membrane proteins including transporters and ion channels, and other kinases have been identified as the substrates of protein kinases and have been found to be regulated by phosphorylation (Smith et al., 2010; Turrà et al., 2014; Martin et al., 2015). In phytopathogenic fungi, expansion of kinases was considered to be beneficial for the pathogen to adapt to stresses encountered both external and internal of its host (Deiulio et al., 2018).
Potassium is essential for maintaining the cell shape, membrane potential, intracellular pH, and enzyme activity (Pérez-Valle et al., 2007; Kahm et al., 2012). Potassium acquisition against a concentration gradient into the cell is derived by conserved families of proteins TRK (derived from the Transporter of K+), HAK (derived from High-Affinity K), ACU ATPases (derived from Alkali Cation Uptake transporters), and PAT (derived from P-type ATPase) in fungi (Benito et al., 2004, 2011; Corratgé et al., 2007; Haro and Benito, 2019). In the yeast Saccharomyces cerevisiae, the protein kinases Hal4, Hal5, and Sky1 have been determined as regulators to involve in potassium uptake (Rodríguez-Navarro, 2000; Wang et al., 2005; Pérez-Valle et al., 2007). The serine/threonine kinase Sat4 (Hal4) positively regulates potassium influx by stabilizing potassium transporters Trk1 and Trk2 (Mulet et al., 1999; Hirasaki et al., 2011). The serine/threonine kinase Sat4 is one of the CAMK kinases. The deletion of SAT4 led to significantly reduced K+ in the yeast cells and increased sensitivity to NaCl, LiCl, and CaCl2 (Mulet et al., 1999). The orthologs of Sat4 have been identified in the filamentous fungi (Wang et al., 2011; De Souza et al., 2013; Yang et al., 2018). Fusarium graminearum FgSat4 was required for vegetative growth, sporulation, conidial morphology, and pathogenicity (Wang et al., 2011). In Colletotrichum higginsiaum, ChSat4 was determined to be involved in cell wall integrity, hyperoxide stress response, and pathogenicity (Yang et al., 2018). These studies have reported the biological phenotype of Δsat4 in fungi, but the specific molecular mechanism of Sat4 regulating the pathogenicity as a protein kinase in plant pathogenic fungi remains to be studied.
The ascomycete Colletotrichum gloeosporioides employs a hemibiotrophic strategy to infect the plant hosts and causes enormous economic losses to crop production and forest industry worldwide (Dean et al., 2012; Wang et al., 2020). Anthracnose caused by C. gloeosporioides is one of the most serious diseases on Cunninghamia lanceolata (Huang et al., 2019). The C. gloeosporioides genome has been sequenced and 16,287 protein-coding genes were identified (Huang et al., 2019). Several genes encoding laccases, peroxidases, plant cell wall-degrading enzymes, Cytochrome P450, and secretory protein have been predicted, which were considered potential pathogenicity contributors in other phytopathogenic fungi (Dean et al., 2005; Yin et al., 2015). In addition, a large number of protein kinases have been predicted. Several protein kinases, such as CgRhoB, CgSte50, CgSte11, CgSte7, CgMk1, CgMck1, have been proved to play important roles in the growth, development, reproduction, and pathogenicity in C. gloeosporioides (Xu et al., 2016; He et al., 2017; Fang et al., 2018; Wang et al., 2021). However, despite these advances, the majority of protein kinases await functionally characterization.
In this study, a yeast serine/threonine kinase Sat4 homolog CgSat4 was identified and characterized in C. gloeosporioides. Our data showed that CgSat4 is involved in K+ uptaking by regulating the accurate localization and expression of the potassium transporter CgTrk1. CgSat4 is required for the osmotic resistance by altering the phosphorylation level of high osmolarity glycerol response kinase CgHog1 that plays important role in the normal response to hyperosmotic stress and cation stress. Deletion of the CgSAT4 also resulted in defects of vegetative growth, sporulation, and pathogenicity in C. gloeosporioide.
Materials and Methods
Fungal Strain and Culture Conditions
Colletotrichum gloeosporioides strain SMCG1#C (Huang et al., 2019) was used for the wild type (WT). The WT, gene deletion mutants, and the complemented strains were maintained on the potato dextrose agar (PDA) plates at 25°C. Complete medium (CM) was employed to culture fungal mycelia for DNA extraction and protoplast preparation as aforedescribed (Wang et al., 2020).
Mutagenesis of CgSAT4 and Complementation of the Mutant
The CgSAT4 was identified in the C. gloeosporioides genome database1 using BLASTP with amino acid sequences of Sat4/Hal4 (NM_001178721.1) from S. cerevisiae. Protein sequences were aligned with ClustalX 2.1, and a phylogenetic tree of the CgSat4 and its orthologs from different fungi was generated using MEGA 6.0 (Tamura et al., 2013).
The knockout deletion mutant of CgSAT4 was obtained using the method described by Wang et al. (2020). Firstly, the upstream and downstream flanking regions (ca. 1.2 kb) flanking sequences of CgSAT4 were amplified using the primer sets of 1F/2R and 3F/4R (Supplementary Table S1), respectively. The PCR products were ligated to either side of the hygromycin B phosphotransferase (HPH) cassette to generate the gene replacement fragment using overlap PCR with the primer set OuterF/OuterR (Supplementary Table S1). Secondly, the gene replacement fragments were purified and transformed into the protoplasts of the WT according to the aforedescribed procedure (Yang et al., 2018). Thirdly, the candidate transformants were screened on the TB3 plate and confirmed by Southern blotting as described previously (Wang et al., 2020), with the probe generated with the primer sets CgSAT4_SN_F/CgSAT4_SN_R and F1111/F1112 (Supplementary Table S1), respectively.
For complementation, a 3.0-kb fragment containing the CgSAT4 ORF region and its native promoter (~1,500 bp) was amplified with the primers CgSAT4 -GFP-1F and CgSAT4-GFP-2R (Supplementary Table S1). The PCR products were inserted into the vector pYF11 that was linearized with the restriction endonuclease Xho I using the yeast gap repair approach (Zhou et al., 2011). The resulting fusion construct CgSAT4-GFP was verified by sequencing and transformed into the CgSAT4 gene deletion mutant. The positive complemented strains were screened by GFP signals and Western blotting described by Bruno et al. (2004).
Localization Pattern Analyses of CgSat4
To observe the localization of CgSat4, mycelial plugs of the complemented strain ΔCgsat4/SAT4 expressing fusion protein CgSat4-GFP were cultured in liquid CM medium at 25°C for 24 h. Then, fresh mycelia of the corresponding strains expressing fusion protein CgTrk1-GFP were prepared for fluorescence microscopy observation as described above. Photographs were taken under a confocal laser scanning microscope (Zeiss, Oberkochen, Germany). To evaluate the effect of deletion of CgSAT4 on the localization of CgTrk1, the construct of CgTRK1-GFP was introduced into the WT and the ΔCgsat4 mutant, respectively, as described above. The localization of CgTrk1 in these strains were observed under a confocal laser scanning microscope. The experiment was performed twice.
Assays of Vegetative Growth and Fruiting Bodies Development
For vegetative growth assays, the mycelial blocks (6 mm in diameter.) of the WT, the ΔCgsat4 mutant, and the complemented strain were inoculated onto PDA plates, respectively. The plates were kept in an incubator at 25°C. Colony growth kinetics was measured at 5 days post-inoculation. Fruiting bodies were induced on V8 juice agar (V8) plates for 10 days after inoculation at 25°C according to the method of Fang et al. (2018). The experiment was carried out three times, and each treatment had three replicates.
Stress Resistance Assays and Determination of Potassium in Fungal Mycelia
To test the role of CgSat4 on stress resistance, the WT, the ΔCgsat4 mutant, and the complemented strains were inoculated on CM plates supplemented with NaCl (0.7 M), KCl (0.7 M), or LiCl (0.3 M). These plates were kept at 25°C for 4 days. The experiment was carried out three times, and each treatment had three replicates.
To evaluate the effect of CgSat4 on potassium uptake, the WT and ΔCgsat4 mutant were cultured in liquid CM medium containing 7 mM potassium for 2 days as described previously (Yang et al., 2018). The fungal mycelia were harvested and dried in a freeze-dryer. Then, the dried mycelia were digested with H2SO4, and mycelial potassium was examined using a flame spectrophotometer (Yang et al., 2018). The experiment was conducted three times, and each treatment had three replicates.
Sporulation, Appressorium Formation, and Invasive Hypha Development
For sporulation, the mycelial blocks (6 mm in dia.) of the WT, the ΔCgsat4 mutant, and the complemented strains were inoculated in the carboxymethyl cellulose (CMC) medium to induce sporulation, and the conidia were collected and counted as described by Fang et al. (2018). The experiment was carried out three times, and each treatment had three replicates.
To induce conidial germination and appressorium formation, the conidial suspensions of the WT, the ΔCgsat4 mutant, and the complemented strains were adjusted to 105/ml, respectively. Ten microliters of conidial suspension of each strain was placed on the glass coverslip (Fisher Scientific, St. Louis, MO, United States) and kept at 25°C. The conidial germination rate of each strain was calculated at 2, 4, and 8 h postinoculation, respectively. The percentage of appressorium formation of each strain was tested at 4, 8, and 12 h postinoculation, respectively. Appressorium turgor pressure was analyzed by the incipient cytorrhysis assay using 1–4 M of glycerol solution, as described by Wang et al. (2020). The experiment was conducted three times with at least 100 structures per replicate.
Onion penetration assays were conducted as described by Wang et al. (2020). Ten microliters of conidial suspension of the WT, the ΔCgsat4 mutant, and the complemented strains was inoculated on the adaxial surface of onion epidermal strips to induce invasive hyphae (IH), respectively. At 24 h post-inoculation, IH was observed under a Zeiss Axio Imager A2M microscope (Carl Zeiss, Jena, Germany). IH was divided into four types (type I, no hyphae penetration; type II, IH with one branch; type III, IH with at least two branches, but having limited expansion; type IV, IH with numerous branching and extensive hyphal growth). The experiment was performed three times, and at least 30 invasive structures were observed in each treatment.
Pathogenicity Tests
Pathogenicity of the WT, the ΔCgsat4 mutant, and the complemented strain was tested as described by Wang et al. (2020). Conidial suspensions of the WT, the ΔCgsat4 mutant, and the complemented strains were adjusted to 1 × 105 spores/ml, respectively. Five microliters of conidial suspension of each strain was inoculated on healthy leaves of C. lanceolata, Populus × euramericana cv. “Nanlin895” and Liriodendron chinense × tulipifera, respectively. The inoculated leaves were kept in a moist chamber at 25°C, and lesion size was measured at 5 days post-inoculation. DNA was isolated from the L. chinense × tulipifera leaves inoculated by the WT and the ΔCgsat4 mutant, and the fungal biomass in planta was examined using qPCR as described by Yang et al. (2018). The experiment was performed three times, and each treatment had three replicates.
Protein Expression, Protein Extraction, and Western Blot
Mycelial plugs of the WT and the ΔCgsat4 mutant were inoculated into 100 ml of CM medium, and shaken at 150 rpm for 2 days at 25°C, respectively. The mycelia of each strain were collected using a layer of Miracloth. Total protein was extracted from the mycelia following the method described by Bruno et al. (2004). Twenty microliters of total proteins were isolated on the SDS-PAGE gel and transferred to a polyvinylidene fluoride (PVDF) membrane using a Bio-Rad imprinting device (Bio-Rad Laboratories, Inc., CA, United States). A primary anti-GFP antibody (GFP labeled mouse monoclonal antibody, Shanghai Antibody Market, China) and a secondary antibody (goat anti-mouse IgG horseradish peroxidase, Shanghai Antibody Market, China) were used to detect GFP. Signal strength corresponding to phosphorylated Hog1 was detected by binding of anti-phosphorylated p38 MAPK (Thr180/Tyr182, rabbit monoclonal antibody) (Cell Signaling Technology, Boston, MA, United States). P38 MAPK antibody (ABMART) was used as the control. The experiment was carried out twice.
To evaluate the effect of the CgSat4 on the potassium transporter CgTrk1 localization and expression, the CgTRK1 coding region, and its native promoter sequence (~1,500 bp) were amplified with the primers CgTRK1-GFP-1F and CgTRK1-GFP-2R. The PCR products were purified and inserted into the vector pYF11 as aforedescribed (Zhou et al., 2011). The fusion construct CgTRK1-GFP was transformed into the WT and the ΔCgsat4 mutant, respectively. The positive candidate strains expressing fusion protein CgTrk1-GFP were screened by GFP signals observation. The expression levels of CgTrk1-GFP in the WT and the ΔCgsat4 mutant were detected using Western blotting with anti-GFP antibody as aforedescribed. The experiment was carried out twice.
Phos-tag Analysis
The CgTRK1-GFP fusion construct was transferred, respectively, into the wild-type strain and ∆Cgsat4 mutant. The positive transformants were cultured in liquid CM for 48 h. For protein isolation, about 150–200 mg of mycelia were ground into powder in liquid nitrogen and resuspended in 1 ml of extraction buffer [10 mM Tris–HCl (pH 7.5), 150 mM NaCl, 0.5 mM EDTA, 0.5% NP40, 1 mM PMSF, 10 μl of protease inhibitor cocktail (Sigma, United States), and 10 μl of phosphatase inhibitor cocktail 3 (Sigma, United States)]. For the preparation of the phosphatase-treated Cell lysates, the phosphatase inhibitor cocktail was omitted for 2.5 U/ml alkaline phosphatase (final concentration; P6774; Sigma) and the sample was incubated for 1 h with the addition of 1 mM MgCl2 (37°C). The samples were then resolved on a 8% SDS-polyacrylamide gel prepared with 50 μM acrylamide-pendant Phos-tag ligand (Wako, Japan) and 100 μM MnCl2, according to the instruction provided by the Phos-tag.
Quantitative Real-Time PCR, and Statistical Analyses
To evaluate the effect of deletion of CgSAT4 on the transcription level of CgTrk1, the mycelia of the WT and the ΔCgsat4 mutant were, respectively, cultured in liquid CM for 3 days at 25°C. The mycelia were collected and total RNA was extracted using the TRIzol LS reagent (Invitrogen, Carlsbad, CA, United States). The first-strand cDNA was synthesized and used as the templates of quantitative RT-PCR as described by Yang et al. (2018). The experiment was conducted three times, and each treatment had three replicates.
Data are presented as mean ± SD. Statistical analyses were carried out with the data processing system (DPS) version 9.50 using a one-way ANOVA (p < 0.01).
Results
Identification of CgSat4 and Gene Deletion Mutant of CgSAT4
An orthologue of Sat4 was identified by a BLAST_P search using the Sat4/Hal5 (NM_001178721.1) of S. cerevisiae as the reference to the genome database of C. gloeosporioides.2 The orthologue shared a 65% amino acid sequence identity with S. cerevisiae Sat4, which was named CgSat4. Phylogenetic analysis showed that Sat4 anthologies in filamentous fungi have significantly diverged from that of S. cerevisiae. The CgSat4 was most similar to its orthologues from phytopathogenic fungi of Colletotrichum species (Supplementary Figure S1A). CgSat4 contained a low complexity region and a Pfam Pkinase motif at the C-terminus (Supplementary Figure S1B).
A gene deletion mutant ΔCgsat4 was obtained by replacing the open reading frame with the hygromycin phosphotransferase resistance (HPH) gene (Supplementary Figure S1C). Southern blotting analysis confirmed that the CgSAT4 was encoded by a single-copy gene in the WT, which was replaced by the HPH gene in the ΔCgsat4 mutant (Supplementary Figure S1D). A complemented strain ΔCgsat4/CgSAT4 was generated by reintroducing the CgSAT4 encoding region with its native promoter into the ΔCgsat4 mutant.
To investigate the localization pattern of CgSat4 in C. gloeosporioides, the CgSat4-GFP fusion construct was introduced into the ΔCgsat4 mutant to generate the complemented strain ΔCgsat4/CgSAT4. The complemented strain recovered the defects of the CgSAT4 deletion mutant as described above, which indicated the CgSat4 has been properly expressed. Thus, the GFP signals of the complemented strain were employed to determine the localization of CgSat4. The fluorescence microscopy showed that strong GFP signals were distributed in the cytoplasm of the conidia and hyphae (Supplementary Figure S1E). Furthermore, an 80-kDa of the predicted CgSat4-GFP fusion protein was detected using an anti-GFP antibody (Supplementary Figure S1F). The data indicated that CgSat4 was expressed in the cytoplasm of C. gloeosporioides.
CgSat4 Required for Vegetative Growth, Fruiting Body Development, and Sporulation
To assess the role of CgSat4, the WT, the ΔCgsat4 mutant, and the complemented strain ΔCgsat4/CgSAT4 were, respectively, inoculated on the PDA. The growth kinetics was observed. On the 4th and 5th day, the colony diameter of the ΔCgsat4 mutant significantly reduced compared to the WT and complemented strains (Figures 1A,B).
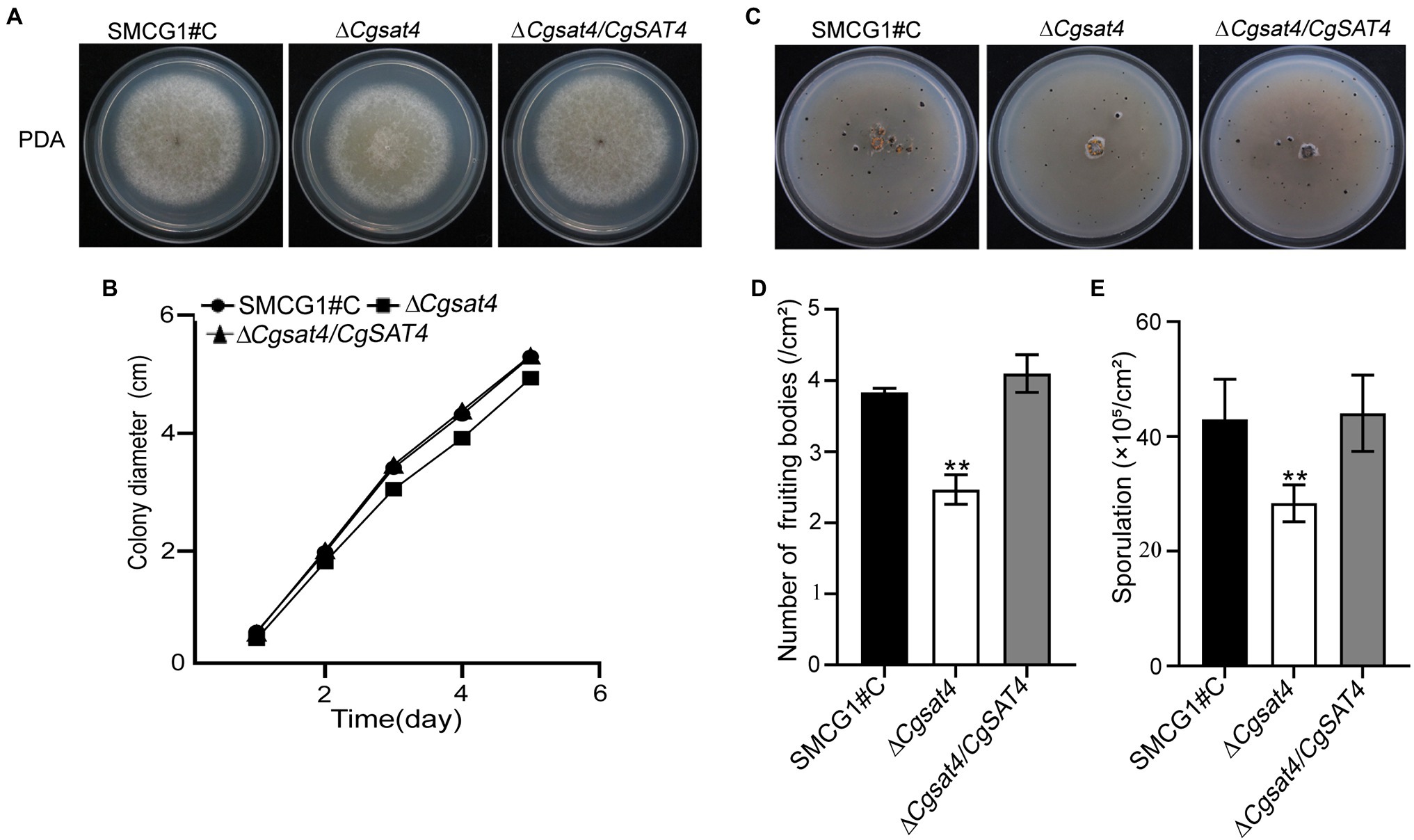
Figure 1. Involvement of CgSat4 in vegetative growth, fruiting body development and sporulation. (A) Colonies of the WT (SMCG1#C), ∆Cgsat4 mutant and the complemented strain ∆Cgsat4/CgSAT4 were cultured on potato dextrose agar (PDA) at 25°C for 5 days. n = 3. (B) Colony growth kinetics in (A). n = 3. (C) Fruiting bodies of the WT, ∆Cgsat4 mutant and the complemented strain formed on the V8 medium for 10 days with 16 h light/8 h dark cycle. n = 3. (D) Quantitative statistics of fruiting bodies in (C). n = 3. (E) Sporulation of the WT, ∆Cgsat4 mutant and the complemented strain. n = 3. Error bars represent the SD, and asterisks indicate significant difference at p < 0.01.
Fruiting body quantification showed that fruiting bodies produced by the ΔCgsat4 mutant were significantly less than those of the WT and complemented strains (Figures 1C,D). There are no morphological differences in the conidia among the WT, ΔCgsat4 mutant, and complemented strain ΔCgsat4/CgSAT4. However, conidial enumeration showed that conidia produced by the ΔCgsat4 mutant were considerably less than those of the WT and complemented strain (Figure 1E). These results indicated that CgSat4 is required for vegetative growth, fruiting body development, and sporulation in C. gloeosporioides.
CgSat4 Required for Potassium Uptake
In the yeast S. cerevisiae, the Sat4 is involved in potassium influx which is mediated by the Trk1-Trk2 transport system (Mulet et al., 1999; Hirasaki et al., 2011). To evaluate whether the orthologue C. gloeosporioides CgSat4 is required for potassium uptake, the mycelial potassium content of the WT and the ΔCgsat4 mutant was examined. Data showed that the potassium was significantly reduced in the ΔCgsat4 mutant than in the WT (Figure 2A).
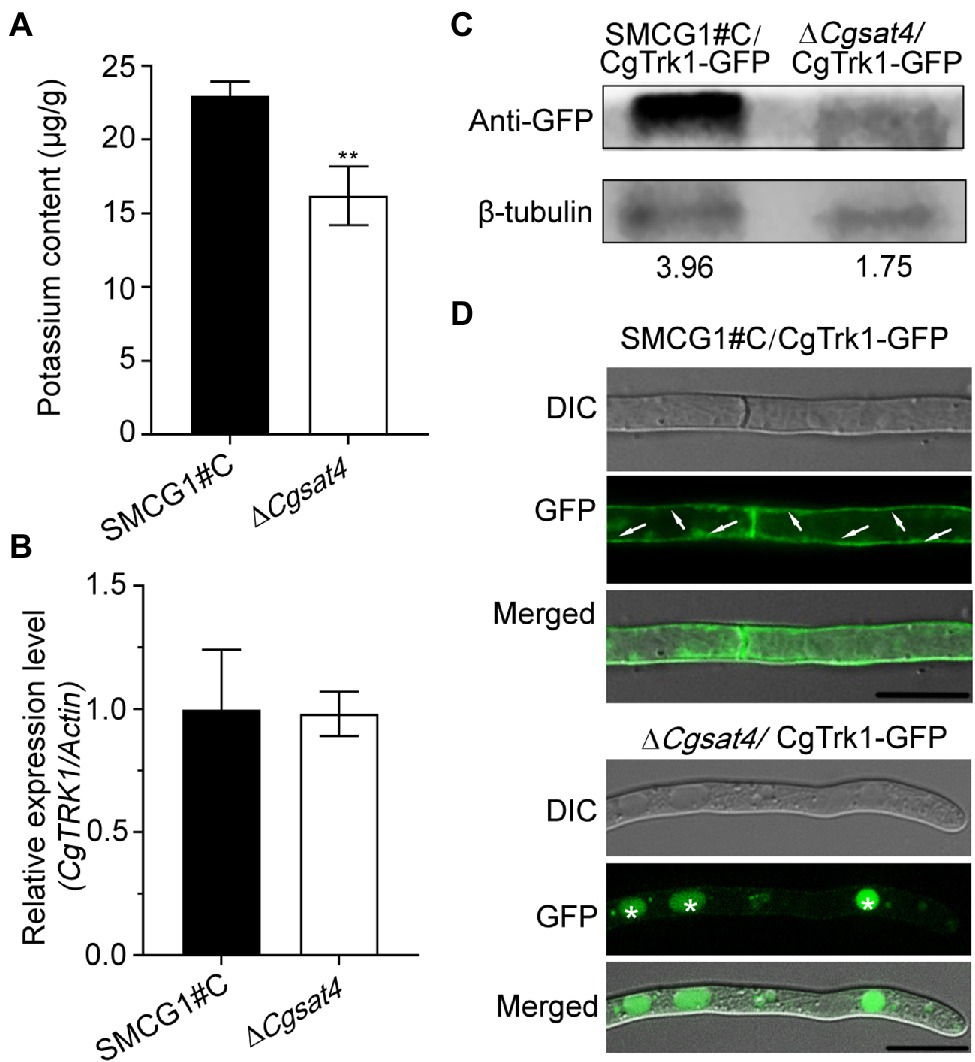
Figure 2. CgSat4 required for potassium uptake and the localization of potassium transporter CgTrk1. (A) Mycelia K+ concentration of the WT and ∆Cgsat4 mutant cultured in CM medium. n = 3. (B) Transcription level analysis of CgTRK1 in the WT and ∆Cgsat4 mutant using qRT-PCR. (C) Western blotting analysis of CgTrk1 in the WT and ∆Cgsat4 mutant using an anti-GFP antibody. (D) Localization pattern of the fusion protein CgTrk1-GFP in the WT and ∆Cgsat4 mutant. The arrows and asterisks represent the plasma membrane and vacuole, respectively. Error bars represent the SD, and asterisks indicate significant difference at p < 0.01.
The transcription level of CgTrk1 was detected in the WT and ΔCgsat4 mutant by qRT-PCR. The result showed that there was no significant transcription difference of CgTRK1 between the WT and ΔCgsat4 mutant (Figure 2B). However, western blotting analysis showed the expression of CgTrk1 in WT was more than twice as much as that in the ΔCgsat4 mutant (Figure 2C).
The localization pattern analysis also showed that the potassium transporter CgTrk1 was distributed in the plasma membrane in the WT. However, the CgTrk1 was mainly distributed in the vacuoles in the ΔCgsat4 mutant (Figure 2D). We therefore examined the interaction between CgSat4 and CgTrk1 by co-immunoprecipitation (co-IP) and yeast two-hybrid (Y2H) assays. However, the results show that CgSat4 does not interact directly with CgTrk1 (Supplementary Figures S2A,B). We also examined whether CgSat4 regulates the level of CgTrk1 phosphorylation through Phos-tag SDS-PAGE gel electrophoresis. The shifts in the mobility of CgTrk1-GFP from the wild-type strain and ∆Cgsat4 mutant cells had no obvious difference (Supplementary Figure S2C), suggesting that CgSat4 does not regulate the level of CgTrk1 phosphorylation. Combining the aforementioned results, we concluded that CgSat4 may be involved in potassium uptake by regulating the localization of the high-affinity potassium transporter CgTrk1.
CgSat4 Required for Extracellular Ion Stress Resistance
In eukaryotic cells, the Hog1 mitogen-activated protein kinase (MAPK) pathway is essential to osmotic stress response (Brewster et al., 1993; Román et al., 2020). As an intracellular osmotic stress regulator, potassium participates in various physiological processes (Pérez-Valle et al., 2007; Kahm et al., 2012). Since the deletion of CgSAT4 resulted in the decrease of mycelial potassium, we hypothesized that decreased potassium concentration may alter the intracellular osmotic pressure and resistance against osmotic stress. To verify this hypothesis, the WT, ΔCgsat4 mutant, complemented strain ΔCgsat4/CgSAT4 were, respectively, inoculated onto the CM plates subjected to osmatic stressors KCl, NaCl, and LiCl, respectively. Compared with the WT and complemented strains, the ΔCgsat4 mutant was more sensitive to the osmatic stressors and displayed a higher growth inhibition rate (Figures 3A,B). The effect of CgSAT4 deletion on the phosphorylation of CgHog1 was further evaluated. The result showed that deletion of CgSAT4 significantly increased the phosphorylation level of CgHog1 compared to the WT (Figure 3C). However, an interaction between CgSat4 and CgHog1 cannot be reproduced (Supplementary Figure S2D).
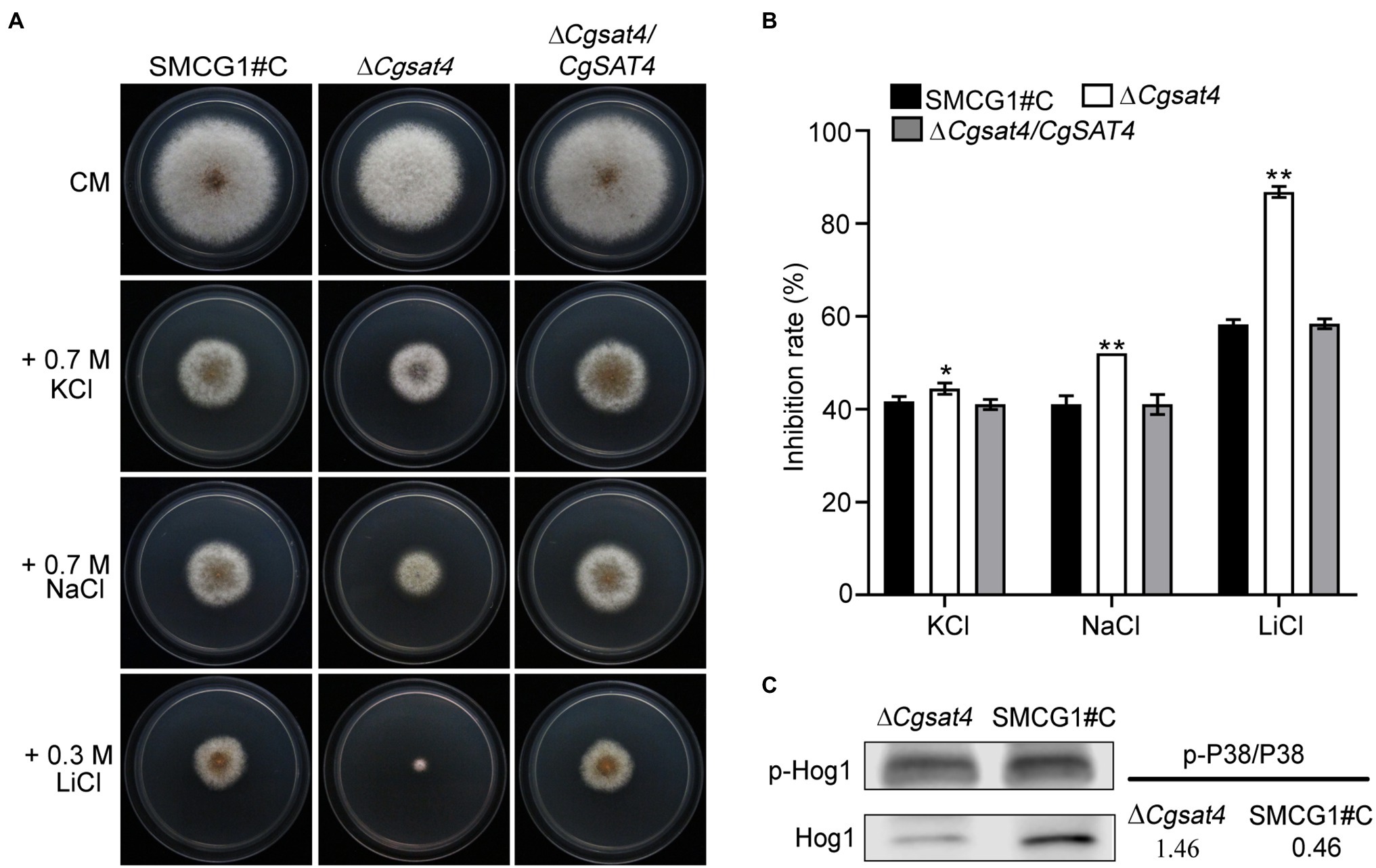
Figure 3. CgSat4 required for extracellular cation stress resistance. (A) Colonies of the WT, ∆Cgsat4 mutant, and the complemented strain ∆Cgsat4/CgSAT4 cultured on CM medium supplemented with different cations for 4 days. n = 3. (B) Colony diameter inhibition rate in (A). Error bars represent the SD. * and ** indicate significant difference at p < 0.05 and 0.01, respectively. n = 3. (C) Phosphorylation level of CgHog1 in the WT and ∆Cgsat4 mutant was detected using an antiphospho-p38 antibody.
CgSat4 Is Required for Functional Appressorium and Invasive Hyphal Development
We found that deletion of CgSAT4 did not affect the conidial morphology. Compared with the WT and complemented strains, the conidial germination rate of the ΔCgsat4 mutant significantly decreased at 2 and 4 h. However, there was no significant difference in the conidial germination among the WT, ΔCgsat4 mutant, complemented strains at 8 h (Figures 4A,B). These data indicate that deletion of the CgSAT4 significantly delayed conidial germination in the early stage.
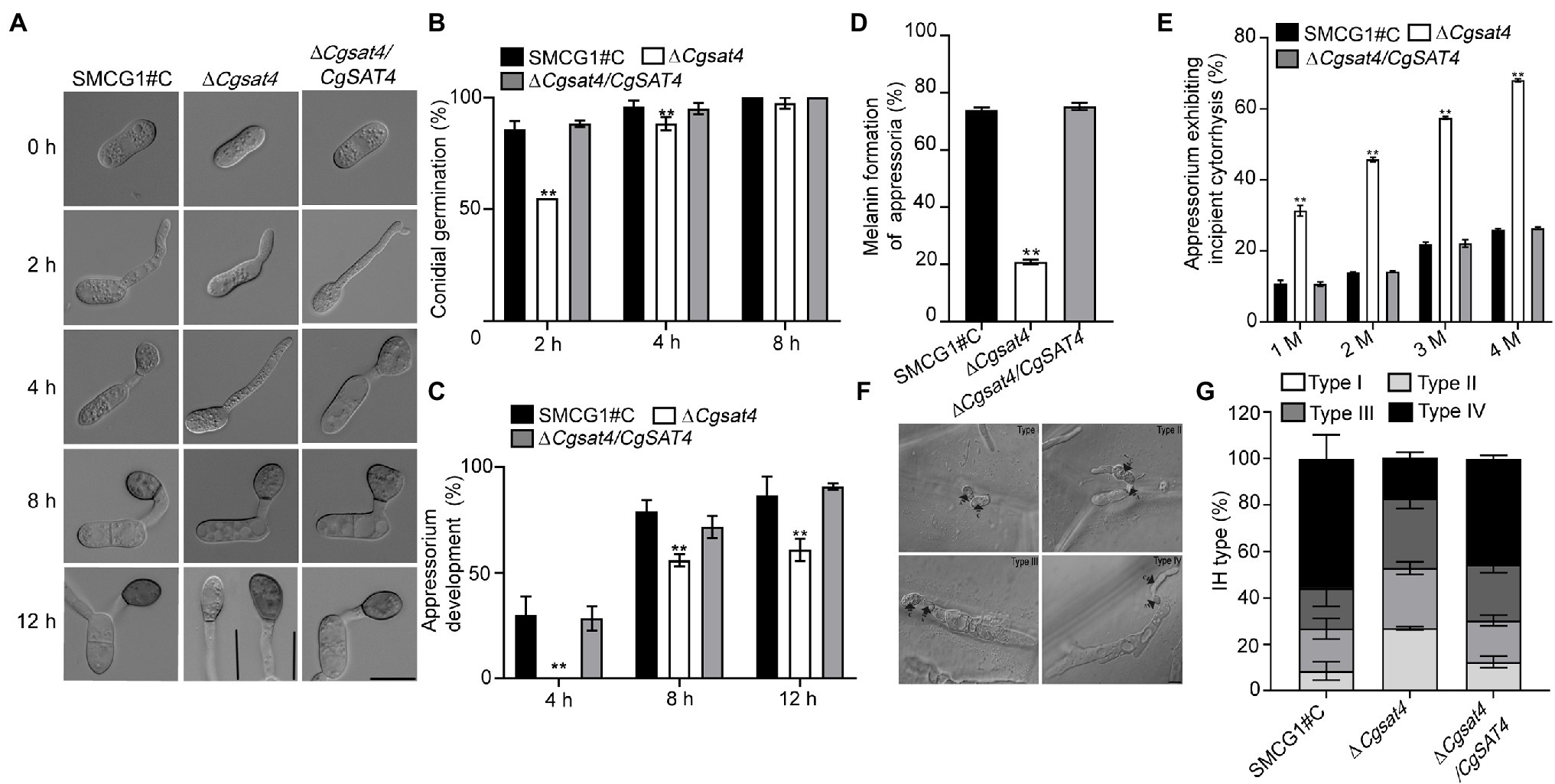
Figure 4. CgSat4 is required for functional appressorium and invasive hyphal development. (A) Conidia and appressoria generated by the WT, ∆Cgsat4 mutant and the complemented strain on hydrophobic slides at 0, 2, 4, 8, and 12 h and photographed. n = 100. Bar = 10 μm. (B) Conidial germination rate of the WT, ∆Cgsat4 mutant and the complemented strain at 2, 4, and 8 h, respectively. n = 100. (C) Appressorium formation rate of the WT, ∆Cgsat4 mutant and the complemented strain at 4, 8, and 12 h, respectively. Error bars represent the SD. Asterisks indicates significant difference at p < 0.01. n = 100. (D) Melanized appressoria rate of the WT, ∆Cgsat4 mutant, and complemented strain. n = 100. (E) Collapsed appressoria rate of the WT, ∆Cgsat4 mutant, and complemented strain. n = 100. Error bars represent the SD. Asterisks indicates significant difference at p < 0.01. (F) Morphology of different types of invasive hypha developed on onion epidermal cells for 24 h. n = 100. (G) Proportion of different type of invasive hypha (type I, no hyphae penetration; type II, IH with one branch; type III, IH with at least two branches, but having limited expansion; type IV, IH with numerous branching and extensive hyphal growth) in the WT, ∆Cgsat4 mutant and complemented strain. Error bars represent the SD. n = 100. Bar = 10 μm.
The data showed that, compared with the WT and complemented strains, appressorium formation rate of the ΔCgsat4 mutant was significantly decreased (Figure 4C). In the ΔCgsat4 mutant, 80% of appressoria failed to melanize, while the WT and the complemented strains formed normal melanized appressoria (Figure 4D). At different concentrations of glycerol, the appressorium collapse rate of the ΔCgsat4 mutant was significantly higher than those of the WT and the complemented strains (Figure 4E). These results indicated that deletion of the CgSAT4 caused the defects in appressorium formation and appressorial turgor pressure.
The onion epidermis penetration assays also showed that the percentages of Types I, II, III, and IV infectious hyphal growth of the ΔCgsat4 mutant were, respectively, 27%, 25.9%, 29.9%, and 21%, compared with 8.6%, 18.2%, 17.5%, and 55.6% in the WT (Figures 4F,G).
CgSat4 Required for Full Virulence
To explore the role of CgSat4 in host infection, conidial suspensions of the WT, ΔCgsat4 mutant, and the complemented strains were, respectively, inoculated on the healthy leaves of Populus × euramericana cv. Nanlin895. Five days post-inoculation (dpi), the ΔCgsat4 mutant produced smaller lesions than those of the WT and complemented strains (Figures 5A,B,F). Similarly, compared with the WT and complemented strains, the ΔCgsat4 mutant showed a decreased virulence on Liriopendron chinense × tulipifera, and Cunninghamia lanceolata (Figures 5A,C,D,G,H). Fungal biomass assays using the qPCR method also showed that the biomass of the ΔCgsat4 mutant in planta was significantly reduced than that of the WT (Figure 5E). These data indicated that CgSat4 played a key role in infectious growth and full virulence in C. gloeosporioides.
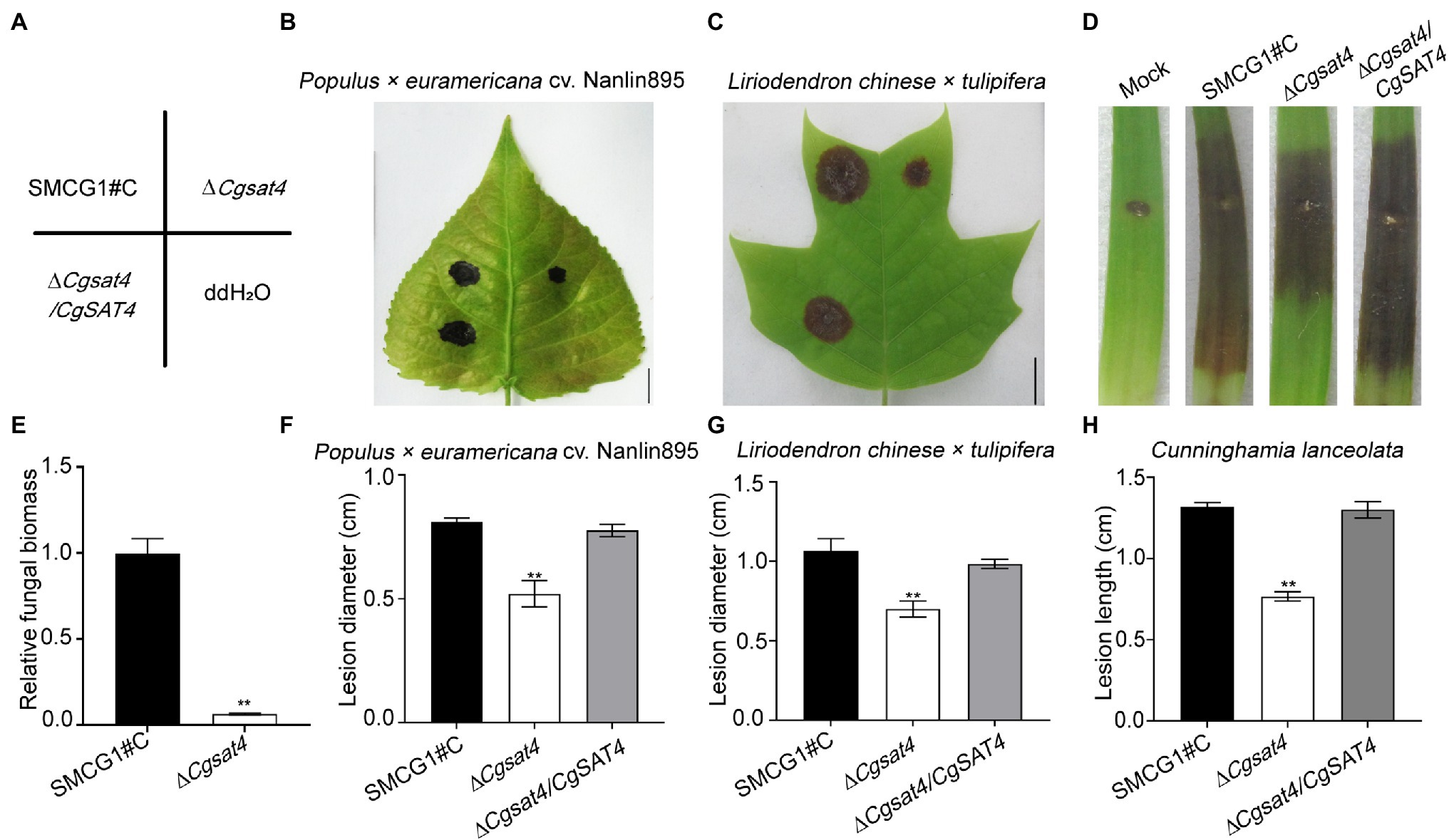
Figure 5. CgSat4 required for full virulence in C. gloeosporioides. (A) Diagram of inoculation sites of the WT, ∆Cgsat4 mutant and complemented strain on the unwounded leaves of Populus × euramericana cv. Nanlin895 and Lirioden dronchinensis × tulipifera. n = 3. (B,C) Pathogenicity assays on leaves of Populus × euramericana cv. Nanlin895 and L. dronchinensis × tulipifera for 5 days indicated in (A), respectively. n = 3. (D) Pathogenicity assays on wounded leaves of C. lanceolata for 5 days. n = 3. (E) Relative fungal biomass of the WT and ∆Cgsat4 mutant monitored from infected tissues of L. dronchinensis × tulipifera at 3 dpi, respectively. n = 3. (F–H) Lesion diameter of lesions on the inoculated leaves showed in (B–D), respectively. n = 3. Error bars represent the SD. Asterisks indicates significant difference at p < 0.01.
Discussion
Colletotrichum gloeosporioides employs a hemibiotrophic lifestyle (Dean et al., 2012; Yan and Talbot, 2016). The infection of C. gloeosporioides is mediated by the appressoria, which drive the penetration peg through leaf cuticles and cell walls (Fang et al., 2018; Wang et al., 2020). In this study, a serine/threonine protein kinase CgSat4 was found to be required for vegetative growth, functional appressorium formation, invasive hyphal development, and full virulence. Further study showed that deletion of CgSAT4 resulted in defects of potassium uptake and higher sensitivity to salt ions than wild type. In the phytopathogenic fungus F. graminearum, the ΔFgsat4 mutant also displayed an increased sensitivity to salt ions (Wang et al., 2011). Deletion of ChSAT4 led to a similar phenotype defect in C. higginsianum (Yang et al., 2018). In this study, we found that CgSat4 regulated potassium accumulation, and played an essential role against cation toxicity of high concentration of Na+, K+, and Li+. Since potassium is important for cell growth (Hušeková et al., 2016), the inhibited vegetative and invasive growth of C. gloeosporioides in the ΔCgsat4 mutant are due to the reduction of potassium accumulation. The abnormal potassium transport caused imbalance of the mutant’s ability to regulate ion stress and osmotic stress. As a hemibiotrophic pathogenic fungus, C. gloeosporioides will also be stressed by ions and osmotic pressure from host cells and environment.
Potassium (K+) is the most abundant intracellular cation in living cells (Pérez-Valle et al., 2007; Kahm et al., 2012). In fungi, potassium uptake against electrical and concentration gradient is derived by conserved families of proteins Trk, Hak, Acu ATPases, and Pat (Corratgé et al., 2007; Benito et al., 2011; Haro and Benito, 2019). In S. cerevisiae, the kinase Sat4/Hal4 and its homolog Hal5 have been determined to involve in ion homeostasis. Overexpression of SAT4 increased tolerance to sodium and lithium. The mutant lacking SAT4 displays a higher sensitivity to toxic cations than the wild type. The Trk1 functions as a potassium transporter and facilitates cells to survive in a low potassium environment (Gaber et al., 1988). The potential regulating mechanism study shows that the Trk1-Trk2 potassium transporter increase the influx of potassium, and decrease the membrane potential, which results in reduced the uptake of toxic cations and improved salt tolerance (Mulet et al., 1999). Trk1 has been determined to be localized in the plasma membrane of S. cerevisiae, which ensures its normal physiological function (Kale et al., 2019). Our results showed that the localization and protein levels of CgTrk1 are regulated by the CgSat4. The localization of CgTrk1 was changed from the plasma membrane to the vacuole in the mutant ΔCgsat4. The abnormal localization and reduced protein levels of CgTrk1 may suppress the potassium uptake, and result in a significant decrease of potassium accumulation and resistance to ion stress in C. gloeosporioides. The difference of CgTrk1 localization in ∆Cgsat4 mutant may be due to the increased endocytosis of lipid membrane proteins after deletion of CgSAT4. In S. cerevisiae, the absence of SAT4 leads to increased internalization of many lipid membrane proteins and receptors of some signaling pathways (Tumolo et al., 2020). In addition, in phytopathogenic fungi, endocytosis also has been charactered as a determiner of virulence (Fuchs et al., 2006). Lipid membrane proteins that complete signal transmission often enter the vacuole and are degraded. The CgTrk1 was mainly distributed in the vacuoles in the ΔCgsat4 mutant. The lower protein levels for CgTrk1 in ΔCgsat4 mutant may be due to more degradation of CgTrk1 in ΔCgsat4 mutant.
The high osmolarity glycerol (HOG) pathway required for osmoregulation depends on the mitogen-activated protein kinase (MAPK) Hog1 cascade, which is highly conserved from single-cell yeast to filamentous fungi (Hohmann, 2009; de Assis et al., 2020; Román et al., 2020). Hog1 induces cellular response to high osmolarity stress in S. cerevisiae (Brewster et al., 1993; Román et al., 2020). In this study, deletion of CgSAT4 significantly altered the phosphorylation level of CgHog1 and sensitivity to osmotic stress. In the fission yeast Schizosaccharomyces pombe, the Hog1 ortholog, Spc1, is responsive to multiple environmental stresses (Shiozaki and Russell, 1995; Kato et al., 1996). Proper phosphorylation and activation of Spc1 is required for activation of transcription factor Atf1 that induce the expression of genes involved in resistance against various stress conditions (Shiozaki and Russell, 1995; Gaits et al., 1998). Spc1 regulates the expression of a plasma membrane Na+/H+ antiporter SOD2 to export Na+ and Li+ (Jia et al., 1992), which is required for cellular survival of cation toxicity. Deletion of SAT4 also shows hypersensitivity to cation stress in S. pombe (Wang et al., 2005). So the hypersensitivity of ∆Cgsat4 mutant to osmotic pressure may be due to the inappropriate phosphorylation of CgHog1. The increased phosphorylation level of CgHog1 in ∆Cgsat4 mutant may be caused by the increased internalization of membrane receptor protein upstream of Hog1 MAPK signaling pathway. In S. cerevisiae, the absence of SAT4 leads to increased internalization of many lipid membrane proteins and receptors of some signaling pathways, such as cell wall stress response protein Wsc1 that is a receptor of MAPK pathway (Tumolo et al., 2020). Combining these data, we propose that CgSat4 is necessary for cation stress resistance via balancing the phosphorylation levels of the CgHog1, which is a key component of the high-osmolarity pathway. In fact, in Magnaporthe oryzae, another plant hemibiotrophic fungi similar to C. gloeosporioides, several conserved signaling pathways are essential for appressorium formation and pathogenicity, including cAMP signaling, Hog1, Pmk1, and Mps1 MAP kinase pathways (Li et al., 2012). In C. gloeosporioides, the homologue MAPK pathway kinases CgPka, CgMek1/CgMkk1, CgSlt2/CgMps1, and CgMck1 also have been determined to be involved in the appressorium formation and pathogenicity (Kim et al., 2000; Priyatno et al., 2012; Yong et al., 2013). These results indicated that the invasive growth and pathogenicity of C. gloeosporioides were regulated by various complex gene networks.
In summary, we have identified and discussed the potential roles of S/T protein kinase CgSat4 in growth, developmental, environmental stress responses, and pathogenicity. The findings will help illuminate the underlying mechanisms of potassium uptake and its functions in plant infection of C. gloeosporioides.
Conclusion
CgSat4 is required for K+ uptake by regulating the localization of the potassium transporter CgTrk1 and cation stress resistance by altering the phosphorylation of CgHog1, and full virulence.
Data Availability Statement
The original contributions presented in the study are included in the article/Supplementary Material; further inquiries can be directed to the corresponding author.
Author Contributions
LH and J-YY conceived and designed the experiments. Y-TP, J-YY, Y-ZZ, BL, and PW performed the experiments. Y-TP, LL, J-YY, and BL analyzed the experiment data. LH contributed to reagents, materials, and analysis tools. Y-TP, LL, and LH wrote the paper. All authors contributed to the article and approved the submitted version.
Funding
This research was supported by the Nature Science Foundation of China (31870631 and 32101530), Youth Programme for Natural Science Foundation of Jiangsu Province (grant no BK20181005), and Priority Academic Program Development of Jiangsu Higher Education Institutions.
Conflict of Interest
The authors declare that the research was conducted in the absence of any commercial or financial relationships that could be construed as a potential conflict of interest.
Publisher’s Note
All claims expressed in this article are solely those of the authors and do not necessarily represent those of their affiliated organizations, or those of the publisher, the editors and the reviewers. Any product that may be evaluated in this article, or claim that may be made by its manufacturer, is not guaranteed or endorsed by the publisher.
Supplementary Material
The Supplementary Material for this article can be found online at: https://www.frontiersin.org/articles/10.3389/fpls.2022.773898/full#supplementary-material
Supplementary Figure S1 | Phylogenetic analysis of CgSat4 and its homologs from different fungi and identification of gene deletion mutant of CgSat4. (A) Phylogenetic tree of CgSat4 and its homologs constructed based on alignment of the Sat proteins from different fungi. (B) The predicted domains of the CgSat4. The hexagon and box indicate the S_TKc (Serine/Threonine protein kinases, catalytic) domain and a low complexity region, respectively. (C) The strategy of gene deletion of the CgSAT4. (D) Southern blot analysis of the WT and ∆Cgsat4 mutant using the probes of CgSAT4 and HPH, respectively. (E) Cellular localization of CgSat4-GFP in the conidia and vegetative hypha of C. gloeosporioides. (F) Western blot analysis of total proteins isolated from the complemented strain expressing the CgSat4-GFP fusion protein.
Supplementary Figure S2 | CgSat4 does not directly interact with CgTrk1 and CgHog1. (A) Co-immunoprecipitation (Co-IP) assays or the interaction between CgSat4 and CgTrk1. The CgTRK1-GFP/CgSAT4-S were co-expressed in the wild-type strain. Total proteins isolated from mycelium of transformants and proteins eluted from the GFP-Trap-A beads were analyzed by Western blotting with anti-GFP and anti-S antibodies. (B) Yeast two hybrid assay for the interaction between CgSat4 and CgTrk1. The AD and BD plasmids were co-transformed into yeast AH109, and transformants were plated on SD-Leu-Trp for 3 d and on selective SD-Leu-Trp-His-Ade for 5 days. (C) Phos-tag assay analysis of CgTrk1 phosphorylation. The green fluorescence CgTrk1 (CgTrk1-GFP) fusion protein from the ∆Cgsat4 mutant cell extracts treated with phosphatase inhibitors were subjected to Phos-tag SDS-PAGE and normal SDS-PAGE followed by immunoblotting with the anti-GFP antibody. The CgTrk1-GFP from wild-type strain cell extracts treated with phosphatase inhibitors or alkaline phosphatase CIP were used as controls. (D) Yeast two hybrid assay for the interaction between CgSat4 and CgHog1.
Footnotes
1. ^http://genome.jgi.doe.gov/Gloci1/Gloci1.home.html
2. ^http://www.broad.mit.edu/annotation/genome/colletotrichum_gloeosporioides/home.html
References
Benito, B., Garciadeblás, B., Fraile-Escanciano, A., and Rodríguez-Navarro, A. (2011). Potassium and sodium uptake systems in fungi. The transporter diversity of Magnaporthe oryzae. Fungal Genet. Biol. 48, 812–822. doi: 10.1016/j.fgb.2011.03.002
Benito, B., Garciadeblás, B., Schreier, P., and Rodríguez-Navarro, A. (2004). Novel p-type ATPases mediate high-affinity potassium or sodium uptake in fungi. Eukaryot. Cell 3, 359–368. doi: 10.1128/ec.3.2.359-368.2004
Brewster, J. L., De Valoir, T., Dwyer, N. D., Winter, E., and Gustin, M. C. (1993). An osmosensing signal transduction pathway in yeast. Science 259, 1760–1763. doi: 10.1126/science.7681220
Bruno, K. S., Tenjo, F., Li, L., Hamer, J. E., and Xu, J.-R. (2004). Cellular localization and role of kinase activity of PMK1 in Magnaporthe grisea. Eukaryot. Cell 3, 1525–1532. doi: 10.1128/EC.3.6.1525-1532.2004
Chen, Y., Wang, Y., and Nielsen, J. (2017). Systematic inference of functional phosphorylation events in yeast metabolism. Bioinformatics 33, 1995–2001. doi: 10.1093/bioinformatics/btx110
Corratgé, C., Zimmermann, S., Lambilliotte, R., Plassard, C., Marmeisse, R., Thibaud, J. B., et al. (2007). Molecular and functional characterization of a Na+-K+ transporter from the Trk family in the ectomycorrhizal fungus Hebeloma cylindrosporum. J. Biol. Chem. 282, 26057–26066. doi: 10.1074/jbc.M611613200
de Assis, L. J., Silva, L. P., Liu, L., Schmitt, K., Valerius, O., Braus, G. H., et al. (2020). The high osmolarity glycerol mitogen-activated protein kinase regulates glucose catabolite repression in filamentous fungi. PLoS Genet. 16:e1008996. doi: 10.1371/journal.pgen.1008996
De Souza, C. P., Hashmi, S. B., Osmani, A. H., Andrews, P., Ringelberg, C. S., Dunlap, J. C., et al. (2013). Functional analysis of the Aspergillus nidulans kinome. PLoS One 8:e58008. doi: 10.1371/journal.pone.0058008
Dean, R. A., Talbot, N. J., Ebbole, D. J., Farman, M. L., Mitchell, T. K., Orbach, M. J., et al. (2005). The genome sequence of the rice blast fungus Magnaporthe grisea. Nature 434, 980–986. doi: 10.1038/nature03449
Dean, R., Van Kan, J. A., Pretorius, Z. A., Hammond-Kosack, K. E., Di Pietro, A., Spanu, P. D., et al. (2012). The top 10 fungal pathogens in molecular plant pathology. Mol. Plant Pathol. 13, 414–430. doi: 10.1111/j.1364-3703.2011.00783.x
Deiulio, G. A., Guo, L., Zhang, Y., Goldberg, J. M., Kistler, H. C., and Ma, L. J. (2018). Kinome expansion in the Fusarium oxysporum species complex driven by accessory chromosomes. mSphere 3, e00231–e00318. doi: 10.1128/mSphere.00231-18
Fang, Y. L., Xia, L. M., Wang, P., Zhu, L. H., Ye, J. R., and Huang, L. (2018). The MAPKKK CgMck1 is required for cell wall integrity, appressorium development, and pathogenicity in Colletotrichum gloeosporioides. Gene 9:543. doi: 10.3390/genes9110543
Fuchs, U., Hause, G., Schuchardt, I., and Steinberg, G. (2006). Endocytosis is essential for pathogenic development in the corn smut fungus Ustilago maydis. Plant Cell 18, 2066–2081. doi: 10.1105/tpc.105.039388
Gaber, R. F., Styles, C. A., and Fink, G. R. (1988). TRK1 encodes a plasma membrane protein required for high-affinity potassium transport in Saccharomyces cerevisiae. Mol. Cell. Biol. 8, 2848–2859. doi: 10.1128/mcb.8.7.2848-2859.1988
Gaits, F., Degols, G., Shiozaki, K., and Russell, P. (1998). Phosphorylation and association with the transcription factor Atf1 regulate localization of Spc1/Sty1 stress-activated kinase in fission yeast. Genes Dev. 12, 1464–1473. doi: 10.1101/gad.12.10.1464
Haro, R., and Benito, B. (2019). The role of soil fungi in K+ plant nutrition. Int. J. Mol. Sci. 20:3169. doi: 10.3390/ijms20133169
He, P., Wang, Y., Wang, X., Zhang, X., and Tian, C. (2017). The mitogen-activated protein kinase CgMK1 governs appressorium formation, melanin synthesis, and plant infection of Colletotrichum gloeosporioides. Front. Microbiol. 8:2216. doi: 10.3389/fmicb.2017.02216
Hirasaki, M., Horiguchi, M., Numamoto, M., Sugiyama, M., Kaneko, Y., Nogi, Y., et al. (2011). Saccharomyces cerevisiae protein phosphatase Ppz1 and protein kinases Sat4 and Hal5 are involved in the control of subcellular localization of Gln3 by likely regulating its phosphorylation state. J. Biosci. Bioeng. 111, 249–254. doi: 10.1016/j.jbiosc.2010.11.013
Hohmann, S. (2009). Control of high osmolarity signalling in the yeast Saccharomyces cerevisiae. FEBS Lett. 583, 4025–4029. doi: 10.1016/j.febslet.2009.10.069
Huang, L., Kim, K. T., Yang, J. Y., Song, H., Choi, G., Jeon, J., et al. (2019). A high-quality draft genome sequence of Colletotrichum gloeosporioides sensu stricto SMCG1#C, a causal agent of anthracnose on Cunninghamia lanceolata in China. Mol. Plant-Microbe Interact. 32, 139–141. doi: 10.1094/mpmi-05-18-0144-a
Hušeková, B., Elicharová, H., and Sychrová, H. (2016). Pathogenic Candida species differ in the ability to grow at limiting potassium concentrations. Can. J. Microbiol. 62, 394–401. doi: 10.1139/cjm-2015-0766
Jia, Z. P., Mccullough, N., Martel, R., Hemmingsen, S., and Young, P. G. (1992). Gene amplification at a locus encoding a putative Na+/H+ antiporter confers sodium and lithium tolerance in fission yeast. EMBO J. 11, 1631–1640. doi: 10.1002/j.1460-2075
Kahm, M., Navarrete, C., Llopis-Torregrosa, V., Herrera, R., Barreto, L., Yenush, L., et al. (2012). Potassium starvation in yeast: mechanisms of homeostasis revealed by mathematical modeling. PLoS Comput. Biol. 8:e1002548. doi: 10.1371/journal.pcbi.1002548
Kale, D., Spurny, P., Shamayeva, K., Spurna, K., Kahoun, D., Ganser, D., et al. (2019). The S. cerevisiae cation translocation protein Trk1 is functional without its "long hydrophilic loop" but LHL regulates cation translocation activity and selectivity. Biochim. Biophys. Acta Biomembr. 1861, 1476–1488. doi: 10.1016/j.bbamem.2019.06.010
Kato, T., Okazaki, K., Murakami, H., Stettler, S., Fantes, P. A., and Okayama, H. (1996). Stress signal, mediated by a Hog1-like MAP kinase, controls sexual development in fission yeast. FEBS Lett. 378, 207–212. doi: 10.1016/0014-5793(95)01442-x
Kim, Y. K., Kawano, T., Li, D., and Kolattukudy, P. E. (2000). A mitogen-activated protein kinase kinase required for induction of cytokinesis and appressorium formation by host signals in the conidia of Colletotrichum gloeosporioides. Plant Cell 12, 1331–1343. doi: 10.1105/tpc.12.8.1331
Li, G., Zhou, X., and Xu, J. R. (2012). Genetic control of infection-related development in Magnaporthe oryzae. Curr. Opin. Microbiol. 15, 678–684. doi: 10.1016/j.mib.2012.09.004
Martin, H., Shales, M., Fernandez-Piñar, P., Wei, P., Molina, M., Fiedler, D., et al. (2015). Differential genetic interactions of yeast stress response MAPK pathways. Mol. Syst. Biol. 11:800. doi: 10.15252/msb.20145606
Miranda-Saavedra, D., and Barton, G. J. (2007). Classification and functional annotation of eukaryotic protein kinases. Proteins 68, 893–914. doi: 10.1002/prot.21444
Mulet, J. M., Leube, M. P., Kron, S. J., Rios, G., Fink, G. R., and Serrano, R. (1999). A novel mechanism of ion homeostasis and salt tolerance in yeast: the Hal4 and Hal5 protein kinases modulate the Trk1-Trk2 potassium transporter. Mol. Cell. Biol. 19, 3328–3337. doi: 10.1128/mcb.19.5.3328
Pérez-Valle, J., Jenkins, H., Merchan, S., Montiel, V., Ramos, J., Sharma, S., et al. (2007). Key role for intracellular K+ and protein kinases Sat4/Hal4 and Hal5 in the plasma membrane stabilization of yeast nutrient transporters. Mol. Cell. Biol. 27, 5725–5736. doi: 10.1128/mcb.01375-06
Priyatno, T. P., Abu Bakar, F. D., Kamaruddin, N., Mahadi, N. M., and Abdul Murad, A. M. (2012). Inactivation of the catalytic subunit of cAMP-dependent protein kinase A causes delayed appressorium formation and reduced pathogenicity of Colletotrichum gloeosporioides. Scientific World Journal 2012:545784. doi: 10.1100/2012/545784
Rodríguez-Navarro, A. (2000). Potassium transport in fungi and plants. Biochim. Biophys. Acta 1469, 1–30. doi: 10.1016/s0304-4157(99)00013-1
Román, E., Correia, I., Prieto, D., Alonso, R., and Pla, J. (2020). The HOG MAPK pathway in Candida albicans: more than an osmosensing pathway. Int. Microbiol. 23, 23–29. doi: 10.1007/s10123-019-00069-1
Shiozaki, K., and Russell, P. (1995). Cell-cycle control linked to extracellular environment by MAP kinase pathway in fission yeast. Nature 378, 739–743. doi: 10.1038/378739a0
Smith, D. A., Morgan, B. A., and Quinn, J. (2010). Stress signalling to fungal stress-activated protein kinase pathways. FEMS Microbiol. Lett. 306, 1–8. doi: 10.1111/j.1574-6968.2010.01937.x
Tamura, K., Stecher, G., Peterson, D., Filipski, A., and Kumar, S. (2013). MEGA6: molecular evolutionary genetics analysis version 6.0. Mol. Biol. Evol. 30, 2725–2729. doi: 10.1093/molbev/mst197
Tumolo, J. M., Hepowit, N. L., Joshi, S. S., and Macgurn, J. A. (2020). A Snf1-related nutrient-responsive kinase antagonizes endocytosis in yeast. PLoS Genet. 16:e1008677. doi: 10.1371/journal.pgen.1008677
Turrà, D., Segorbe, D., and Di Pietro, A. (2014). Protein kinases in plant-pathogenic fungi: conserved regulators of infection. Annu. Rev. Phytopathol. 52, 267–288. doi: 10.1146/annurev-phyto-102313-050143
Wang, P., Li, B., Pan, Y. T., Zhang, Y. Z., Li, D. W., and Huang, L. (2020). Calcineurin-responsive transcription factor CgCrzA is required for cell wall integrity and infection-related morphogenesis in Colletotrichum gloeosporioides. Plant Pathol. J. 36, 385–397. doi: 10.5423/ppj.oa.04.2020.0071
Wang, X., Lu, D., and Tian, C. (2021). Mitogen-activated protein kinase cascade CgSte50-Ste11-Ste7-Mk1 regulates infection-related morphogenesis in the poplar anthracnose fungus Colletotrichum gloeosporioides. Microbiol. Res. 248:126748. doi: 10.1016/j.micres.2021.126748
Wang, L. Y., Shimada, K., Morishita, M., and Shiozaki, K. (2005). Response of fission yeast to toxic cations involves cooperative action of the stress-activated protein kinase Spc1/Sty1 and the Hal4 protein kinase. Mol. Cell. Biol. 25, 3945–3955. doi: 10.1128/mcb.25.10.3945-3955.2005
Wang, C., Zhang, S., Hou, R., Zhao, Z., Zheng, Q., Xu, Q., et al. (2011). Functional analysis of the kinome of the wheat scab fungus Fusarium graminearum. PLoS Pathog. 7:e1002460. doi: 10.1371/journal.ppat.1002460
Xu, X., Wang, Y., Tian, C., and Liang, Y. (2016). The Colletotrichum gloeosporioides RhoB regulates cAMP and stress response pathways and is required for pathogenesis. Fungal Genet. Biol. 96, 12–24. doi: 10.1016/j.fgb.2016.09.002
Yan, X., and Talbot, N. J. (2016). Investigating the cell biology of plant infection by the rice blast fungus Magnaporthe oryzae. Curr. Opin. Microbiol. 34, 147–153. doi: 10.1016/j.mib.2016.10.001
Yang, J. Y., Fang, Y. L., Wang, P., Ye, J. R., and Huang, L. (2018). Pleiotropic roles of ChSat4 in asexual development, cell wall integrity maintenance, and pathogenicity in Colletotrichum higginsianum. Front. Microbiol. 9:2311. doi: 10.3389/fmicb.2018.02311
Yin, Z., Liu, H., Li, Z., Ke, X., Dou, D., Gao, X., et al. (2015). Genome sequence of Valsa canker pathogens uncovers a potential adaptation of colonization of woody bark. New Phytol. 208, 1202–1216. doi: 10.1111/nph.13544
Yong, H. Y., Bakar, F. D., Illias, R. M., Mahadi, N. M., and Murad, A. M. (2013). Cgl-SLT2 is required for appressorium formation, sporulation and pathogenicity in Colletotrichum gloeosporioides. Braz. J. Microbiol. 44, 1241–1250. doi: 10.1590/s1517-83822013000400031
Keywords: anthracnose, pathogenicity, phosphorylation, potassium accumulation, protein kinase
Citation: Pan Y-T, Li L, Yang J-Y, Li B, Zhang Y-Z, Wang P and Huang L (2022) Involvement of Protein Kinase CgSat4 in Potassium Uptake, Cation Tolerance, and Full Virulence in Colletotrichum gloeosporioides. Front. Plant Sci. 13:773898. doi: 10.3389/fpls.2022.773898
Edited by:
Zuhua He, Center for Excellence in Molecular Plant Sciences (CAS), ChinaReviewed by:
Sabine Dagmar Zimmermann, Délégation Languedoc Roussillon (CNRS), FranceChengming Tian, Beijing Forestry University, China
Copyright © 2022 Pan, Li, Yang, Li, Zhang, Wang and Huang. This is an open-access article distributed under the terms of the Creative Commons Attribution License (CC BY). The use, distribution or reproduction in other forums is permitted, provided the original author(s) and the copyright owner(s) are credited and that the original publication in this journal is cited, in accordance with accepted academic practice. No use, distribution or reproduction is permitted which does not comply with these terms.
*Correspondence: Lin Huang, bGh1YW5nQG5qZnUuZWR1LmNu
†These authors have contributed equally to this work