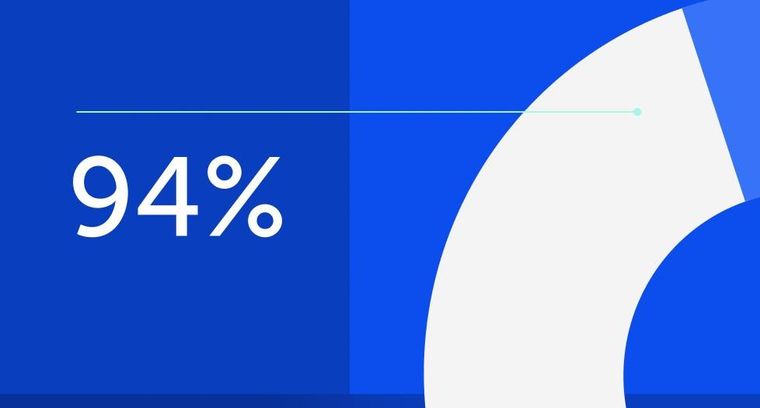
94% of researchers rate our articles as excellent or good
Learn more about the work of our research integrity team to safeguard the quality of each article we publish.
Find out more
ORIGINAL RESEARCH article
Front. Plant Sci., 12 July 2022
Sec. Plant Nutrition
Volume 13 - 2022 | https://doi.org/10.3389/fpls.2022.722710
This article is part of the Research TopicFactors affecting the efficacy of foliar fertilizers and the uptake of atmospheric aerosols, Volume IIView all 9 articles
Materials on plant leaf surfaces that attract water impact penetration of foliar-applied agrochemicals, foliar water uptake, gas exchange, and stomatal density. Few studies are available on the nature of these substances, and we quantify the hygroscopicity of these materials. Water vapor sorption experiments on twelve leaf washes of sample leaves were conducted and analyzed with inductively coupled plasma-optical emission spectroscopy (ICP-OES) and X-ray diffraction. All leaf surface materials studied were hygroscopic. Oils were found on the surface of the Eucalyptus studied. For mangroves that excrete salt to the leaf surfaces, significant sorption occurred at high humidity of a total of 316 mg (~0.3 ml) over 6–10 leaves and fitted a Guggenheim, Anderson, and de Böer sorption isotherm. Materials on the plant leaf surface can deliquesce and form an aqueous solution in a variety of environments where plants grow, including glasshouses and by the ocean, which is an important factor when considering plant-atmosphere relations.
The deposition of aerosols onto plant leaves is a common occurrence in the environment, yet its ecophysiological impacts are poorly understood. The aerosols are highly variable in composition and size (1 nm–100 μm; Lindberg et al., 1986). The impact of aerosols deposited onto plant leaves requires further attention and few studies are available on the implications of deposited aerosols on plants in regard to plant-atmosphere relations, plant physiology, and micrometeorology. We aim to test if hygroscopic particles on plant leaf surfaces are present in a range of environments and attempt to characterize them. Hygroscopic particles are contained in aerosols and may be deposited onto plant leaf surfaces, impacting many factors, both in experimental settings and field work. The deposition of the aerosol of calcium has increased in the western USA, due to mineral aerosols from dust storms, increased human activity upwind, increased aridity, and wind transport (Brahney et al., 2013). Leaf surface wetness can increase trace gas deposition and provide a trap for easily soluble compounds (Simon et al., 1994; Katata and Held, 2021). Ionic substances are often present on plant leaves (especially in saline environments, such as mangroves Coopman et al., 2021), in atmospheric particles, and sprinkler irrigation (Isla and Aragüés, 2009; Fernández et al., 2017). Hygroscopic materials can reduce the surface tension of droplets (Dutcher et al., 2010) and may allow stomatal penetration (Eichert and Goldbach, 2008; Burkhardt and Hunsche, 2013; Burkhardt et al., 2018). Gas exchange experiments, stomatal apertures and distribution (Burkhardt et al., 2018; Grantz et al., 2018), foliar water uptake (Coopman et al., 2021), and foliar-applied agrochemical penetration (Tredenick et al., 2018) may be impacted by hygroscopic materials. In the context of foliar-applied agrochemical spray penetration, the effect of additional hygroscopic materials on the surface is significant, including changing the point of deliquescence of the applied salt (Chen and Lee, 2001; Fernández et al., 2017), the total droplet evaporation time, droplet contact angle and area, and total amount of chemical penetrated (Tredenick et al., 2017, 2018, 2021; Tredenick and Farquhar, 2021).
The average thickness of a liquid layer present on a leaf surface due to hygroscopic particles is estimated to be approximately 1 μm (Burkhardt and Hunsche, 2013). Particulate matter present on leaf surfaces can reach a similar mass to that of leaf waxes; wax mass is around 50 μg cm−2 (Sæbø et al., 2012; Burkhardt and Hunsche, 2013). The electrical conductance, related to surface wetness, for a leaf and artificial leaf in a field, is similar at night (leaf stomata closed), while during the day (leaf stomata open) the leaf is more conductive. Stomata opening during the day play an important role in controlling leaf moisture (Burkhardt and Gerchau, 1994; Burkhardt and Hunsche, 2013).
Foliar water uptake may be important for the plant during drought. A large proportion of plant species studied have been found to have a capacity for foliar water uptake, totaling 124 species (Dawson and Goldsmith, 2018). Water may be present on the leaf surface from a variety of sources including rain, dew, and high humidity. Fog suppresses water loss from leaves, e.g., ameliorating daily water stress in a coastal redwood. A diurnal rhythm is present and older, well watered leaves take up the most water (Burgess and Dawson, 2004). Without fog, species with high foliar water uptake are more likely to lose turgor during seasonal droughts (Eller et al., 2016).
A variety of mangrove species have glands in their leaves that excrete salts to the leaf surface, as shown for a mangrove grown in a growth cabinet (Figure 1). These salt crystals are large and visible to the naked eye. Mangroves are known to rely on non-saline water to maintain productivity and foliar water uptake may be key. Three species of mangroves growing in arid and humid environments have been shown to have a contribution from foliar water uptake of 32% in Avicennia germinans, 26% in Laguncularia racemosa, and 16% in Rhizophora mangle, and of these, Avicennia germinans excretes salts onto the leaf surface (Hayes et al., 2020). For these plants to achieve full leaf hydration, they require the input of water from additional sources other than root water, such as atmospheric water (Nguyen et al., 2017). Within the same species, uptake was comparable across the field and controlled environments, suggesting that uptake is not a plastic arid-zone adaptation but may be used as a supplemental water balance strategy in humid and arid neotropical mangroves (Hayes et al., 2020). Leaf water potential and reverse sap flow rate increase above the point of deliquescence (POD) (75%RH for sodium chloride, NaCl) (Coopman et al., 2021), indicating that surface salts are an important consideration in foliar water uptake for mangroves. Plants living in halophilic environments, such as saltbush (Atriplex halimus) (Simon et al., 1994) excrete salt onto the leaf surface. Species with hygroscopic salts on the leaf surface have leaves that will stay wet longer and trap aerosols. Species with more NaCl on their leaves have a very limited diversity of bacteria and fungi on the leaf surface, e.g., on saltbush (Atriplex halimus) and mangroves (Avicennia germinans and Laguncularia racemosa) (Simon et al., 1994; Gilbert et al., 2002), indicating that salt on plant leaves may be a defense mechanism. Thus, the presence of salts on the leaves may yield multiple benefits, including improved water relations and bacterial control.
Figure 1. Naturally occurring salts on the freshly cut abaxial leaf surface of “Cabinet mangrove” (gray/white mangrove Avicennia marina). The salts are present on the leaf due to glands that excrete salt and the leaf is visibly dry.
Ionic substances can sorb water from the air, due to their strong attractive forces for highly polar water molecules. The ability to attract (adsorb or absorb) water molecules from the air is termed hygroscopicity. The point, in terms of relative humidity, at which a hygroscopic material can sorb enough water to dissolve and form an aqueous solution is called the point of deliquescence (POD) or deliquescent relative humidity (DRH). Most deliquescent materials are ionic salts and their POD can vary significantly at room temperature, from 32%RH for CaCl 2 (Kolthoff et al., 1969), 75%RH for NaCl, to 97%RH for K2SO4 (Tang et al., 1997). If the relative humidity is above the POD, solid crystals will sorb moisture from the air until the salt dissolves and remains in the solution (Dow Chemical Company, 2003; OxyChem, 2014). The solution will continue to sorb water from the air until an equilibrium is reached between the vapor pressure of the solution and the air. For example, if the relative humidity of the air is 50%RH and is above the POD of CaCl 2 (32%RH), salt crystals or salt solution will attract water. If the relative humidity is below the POD, say 10%RH, the solution will continue to evaporate and eventually form crystals. Additional to relative humidity, the rate of sorption by hygroscopic material depends on temperature, the surface area of the salt exposed to the air, and the wind or air circulating over it (Dow Chemical Company, 2003). NaCl crystals are seen under fluctuating relative humidities around the POD of NaCl on astomatous isolated tomato fruit cuticles, and pools of water form (Burkhardt et al., 2012). The particular point of deliquescence of many ionic salts is well defined, though there is less known about the sorption of salt mixtures, and salt and oil mixtures. Plant matter sorption is significantly less than hygroscopic salts, where sorption at high humidity is 30% in cellulose, 49% in polar polysaccharides isolated from cuticles, and 21% in clays (smectite), while CaCl 2 can sorb 1,400% (Dominguez and Heredia, 1999; Likos and Lu, 2002; Dow Chemical Company, 2003; Belbekhouche et al., 2011).
Heterogeneous stomatal pore area or patchy stomatal conductance may have substantial implications for photosynthetic efficiency. Plants grown in filtered or unfiltered air were compared and aerosols deposited onto the leaf from unfiltered air suppressed the heterogeneity of stomatal pore opening and response to vapor pressure deficit (Grantz et al., 2020) while increasing the minimum epidermal conductance (Burkhardt et al., 2018); a key factor of drought tolerance in plants (Burkhardt and Pariyar, 2014). Hygroscopic aerosols may contribute to the formation of a thin aqueous film across the leaf surface that can connect stomata to each other and the leaf interior (Grantz et al., 2018, 2020). Salts artificially sprayed onto leaves increased the minimum epidermal conductance (Burkhardt and Pariyar, 2014), and the electrical leaf conductance related to leaf surface wetness (Burkhardt et al., 1999).
We aim to determine whether hygroscopic materials exist on plant leaves, across a wide range of locations where plants grow. We focus on any substance present on the plant leaf surface (in situ), which is easily washed off and dissolved in solution and remains in solution after centrifugation. These substances may originate from deposited aerosols, sea spray, impurities in the rain, soils, and salts, or inside the leaf due to leaching or excreted salts. We aim to determine the materials' compositions; how hygroscopic they are over a large range of relative humidities, and whether they visibly deliquesce and form an aqueous solution. Vapor sorption methods are used to analyze the leaf material sorption, while the leaf wash material composition is analyzed using powder X-ray diffraction and inductively coupled plasma-optical emission spectroscopy (ICP-OES). We compare the leaf wash sorption to control sorption experiments conducted alongside.
Plant samples were taken at a range of distances from the ocean, with locations and species described in Table 1, and total dry weights in Table 4. These locations included the ocean, river, farm, lake, indoor, town and inside glasshouses, and growth cabinets. The leaves were collected from the same individual plant at each location, and the same unshaded part of the plant. All samples were collected after at least 2 weeks without rain and were from mature plants unless otherwise stated. No artificial spray applications or pre-treatments were used during the experiment.
Leaves of around the same area (6–10 leaves washed in total, with a total leaf area including the 2 washed leaf surfaces of approximately 430 cm2), enough to fill a glass container (125 ml) with a screw cap lid, were collected intact on their branch (so a small area of petiole and stem was also washed) and removed carefully from the plant. The leaves were placed in the bottle with 60 ml of de-ionized water, immediately after collection from the plant and shaken lightly for 20 s (Burkhardt et al., 2018). Then the leaves were quickly removed from the wash solution and discarded. The glass bottle was thoroughly cleaned beforehand, with detergent, then rinsed with de-ionized water and ethanol. The wash was centrifuged at 2,000 g for 15 min (Orbital 420, Clements, Australia). Centrifuging was repeated once if particles remained suspended. We note that the wash was not filtered as we wanted to investigate small particles. For each plant species and location, a total of two sample sets were collected from one individual plant, 120 ml in total; one for the vapor experiment and one for the ICP-OES experiment. The ICP-OES sample had the leaf matter removed and discarded, and was then centrifuged and dried from 60 to 5 ml.
For the vapor experiment to find the initial dry weights of the vessel, 3 empty 1.5 ml polypropylene Eppendorf tubes were prepared by drying them in the oven and then weighed to determine the vessel dry weights, dwv. For the leaf washes, each 60 ml sample was divided equally into 3. Samples were dried in an oven for 3 days at 50°C. The wash was dried down to a smaller volume, then placed in the dry Eppendorf tubes (the same 3 from the dry weight measurement) and dried further to a solid. The total dry weight of the sample and vessel was recorded, dwv+s. Sample mass was determined for all dry weight and sorption experiments using a Mettler AT21 Comparator, Mettler Toledo, Italy, d = 1 μg, max = 22 g.
The static vapor gravimetric sorption technique (Ajibola, 1986) was used to determine the sorption of water by the samples. Wash samples start out oven dry, then are successively placed in environments of increasing relative humidity to test their adsorption properties. To create a specific relative humidity, a range of salt solutions were used, being MgCl 2, Ca(NO 3) 2, NaCl, KCl, KNO3, and K2SO4, with the equilibrium RH being 33.4, 55.5, 75, 84, 93, and 97%RH, respectively. To create these saturated salt solutions, 50 ml of de-ionized water was placed in a large petri dish, next to the leaf sample with the cap open, inside an air-tight larger glass vessel. The larger vessel was then stored in a temperature controlled environment for 3 days (21.9 ± 0.4°C). At the end of 3 days, samples were quickly taken out of the large vessel, re-capped, and weighed in their Eppendorf tubes to provide the wet weight of the sample, wwv+s, at that particular relative humidity. It was noted if the liquid was clearly visible over the material in the Eppendorf tube without the need for special equipment. Triplicates were compared and averaged. Error bars were produced for the standard error of the mean. The polypropylene Eppendorf tubes can also sorb moisture, so the wet weight of the tubes was approximated from 6 empty tubes exposed to each humidity.
We compare the sorption of the leaf washes for similar weights to controls based on salts and oils including mixtures. Salts and oils were weighed as shown in Supplementary Table S1 and added to 1 mL of de-ionized water. The samples were then mixed and dissolved where possible, and dried in the oven at 50°C for 3 days. The same procedure as Section 2.2 was conducted for measuring water sorption. The mangrove nutrient (that the “Cabinet mangrove” grew in a solution of), Eucalyptus oil (100% pure), and tea tree oil (100% pure, Melaleuca alternifolia, Australia) were purchased. The mangrove nutrient comprises mainly NaCl, along with 1,295 ppm Mg, 430 ppm Ca, and 390 ppm K.
Powder X-ray diffraction (XRD) analysis was carried out with a Malvern Panalytical Empyrean Series 3 diffractometer, with Bragg-BrentanoHD divergent beam optic, and a PIXcel3D detector (1D scanning mode, 3.347° active length), using CoKα radiation. Two samples were analyzed with the leaf wash method (Table 2). Samples were analyzed with a broad beam (long-fine focus) over a range of 4–85° 2θ, with a step of 0.0131303° and scan speeds ranging from 298 to 2,598 ss per step depending on sample requirements. Samples were rotated horizontally to increase the sampling size. Two other methods were utilized with 6 plant species; by directly studying the leaf, and its scrapings. Samples were obtained by scraping materials off the leaves (Supplementary Figures S9, S10) with a scalpel and washing any residual sample into an agate mortar with ethanol, then grinding the material with an agate pestle by hand as finely as possible. The sample was then deposited with a Pasteur pipette onto a low-background sample holder (made of Si or quartz), dried, and presented to the X-ray beam without the leaf substrate. The only possible contaminant of samples prepared this way is small amounts of epicuticular wax from the plant (paraffin). Leaf wash samples (refer to Section 2.1, Table 2, and Supplementary Figures S7, S8) were also prepared on such low background holders. The wash samples proved somewhat sub-optimal for XRD analysis because salts readily dissolve and precipitated as potentially different compounds. The suitability of each sample for yielding instructive powder XRD data varied, depending on the sample preparation (leaf wash, leaf scraping, or direct analysis of the leaf surface), the amount of material on the leaf surface, the crystal sizes (1–10 μm ideal), and leaf shape (flat better than curled for direct leaf analysis), requiring individual evaluation of analytical conditions and methods for each sample. Phase identification was carried out with the software DiffracPlus Eva 10 (Bruker AXS GmbH, 2004) and ICDD PDF-2 database (ICDD, 2004), and quantification with Siroquant V4 (Taylor, 1991).
Table 2. X-ray diffraction (XRD) results for the two leaf wash samples that were expected to have the most and least amounts of materials present on the surface; “Brackish mangrove” and “Town euc rain.”
The quantification of 70 elements was carried out using an Agilent 5110 ICP-OES (Agilent Technologies, Australia), operating in Synchronous Vertical Dual View (SVDV) mode, allowing for the simultaneous detection of axial and radial emission signals. Only concentrations for Al, B, Ca, Cu, Fe, K, Mg, Na, P, Si, Sr, and Zn were detected above the method detection limit of 0.1 μg/g. A double pass cyclonic spray chamber, a SeaSpray nebulizer, and a 2.4 mm quartz injector were used as the introduction system. Operating parameters for the ICP-OES analysis are tabulated in Supplementary Table S3. All dilutions and sample preparation of samples for ICP-OES measurement were performed using ultrapure water (MilliQ, Merck), as well as sub-boiling distilled HNO3. A custom multi-element calibration solution for the elements of interest was prepared from single element standard solutions (Inorganic Ventures) and diluted to concentrations ranging from 0.1 to 20 μg/ml. All samples were diluted and acidified to fall within the calibration curve and repeat analyses were carried out with multiple dilution steps for samples that initially exceeded the calibration range. The blank contribution was monitored by acidifying and analyzing the de-ionized water used for the wash. The presence of chloride (Cl–) and sulfate (SO2–4) was tested, as described in Supplementary Section S1.
To find the total weight and percentage increase in moisture gained for each relative humidity step for the sample, we consider the dry weight and the blank vessel water sorption. We first consider
We formulate Δw (%), the weight increase of moisture adsorbed above the dry weight of the sample (sample being the salt taken from the leaf surface) scaled with the blank, as follows:
where wwv+s (mg) is the total wet weight of the vessel and sample together at a given RH, dwv (mg) is the empty vessel/ Eppendorf tube dry weight, and b (%) is the blank percentage moisture weight gain at a given RH and dwv+s (mg) is the total vessel and sample dry weight. Simplifying, the final equation for Δw becomes:
and Total mg, the total weight of the wet sample including the sample dry weight is:
The parameters are direct weight measurements, except Δw, Total mg, and b, which are calculated. Dry weights were determined following oven drying. As Eppendorf tubes vary in weight, each tube is weighed dry and with the sample. To correct for water sorption by the polypropylene, 6 blank Eppendorf tubes were weighed with successive humidity steps, and averaged, to find b using Equation (1), producing sorption between 0.033 and 0.23%, at 33%RH to 97%RH.
To investigate what materials are on the surface of plant leaves, we use a range of techniques, with the plant species and locations described in Table 1. We use the word “materials” due to their highly mixed nature. The total dry weights of the triplicates after centrifugation and drying are shown in Table 4 and indicate that both mangroves have a large amount of materials present, 40 and 41 mg (when appropriately scaled), compared to the other samples (3–8 mg). The total weight of dry material is relevant in this study, as increasing the hygroscopic material also increases the total sorption weight, and our main interest is the total amount of water that could accumulate on a leaf surface. To investigate the types of materials present on the leaf, our initial analysis was performed using leaf washes with the XRD, as shown in Table 2 and Supplementary Figures S7, S8. “Brackish mangrove” contained significant amounts of ionic hygroscopic compounds such as NaCl and KCl, along with other minerals, while “Town euc rain” contained a range of materials but only trace amounts of hygroscopic KCl. These samples were dissolved in de-ionized water and represent the compounds on the leaf but may not represent the original in situ configuration of the elements, e.g., NaCl and K 2SO 4 might become Na2SO4 and KCl, having different PODs. Tests were carried out to determine what the original compounds were on the leaf and the results were similar to those in Table 2, as shown in Supplementary Figures S9, S10. Scrapings from the leaves gave better results than viewing the leaf directly and significant portions of wax were also present on the leaves but not quantified. XRD is unable to detect and analyze materials without a repetitive or crystalline structure that occurs in very small amounts (lower detection limits for XRD range from about 0.1 to 2 wt.% depending on the compound). Therefore, ICP-OES analyses were carried out to examine the elemental composition of the samples, including trace elements.
The ICP-OES results are shown in Table 3 in μg/g [ppm (parts per million)] and gray shading indicates relatively higher concentrations. The results indicate that the samples having significant amounts of hygroscopic salts are the “Brackish mangrove,” “Cabinet mangrove,” “Brackish euc,” “Glasshouse chili,” and perhaps the “Euc farm” and “Ocean common reed,” based on the determined concentration of Ca, Mg, and Na. The “Brackish mangrove” and “Cabinet mangrove” have similarly large amounts of most elements. The presence of Cl– was tested separately, indicating “Brackish mangrove,” “Cabinet mangrove,” and “Brackish euc” contained significant amounts of Cl–, consistent with the calculated Na values. The silver nitrate test changed all 5 Eucalyptus samples, containing Eucalyptus oil, brown as seen in Supplementary Figure S1. “Town euc no rain” shows the darkest color, indicating the largest amount of oil. Testing for SO2– 4 was also carried out on these samples, with no positive indications.
Table 3. Inductively coupled plasma-optical emission spectroscopy (ICP-OES) results in μg/g (ppm) of similar leaf area for all leaf wash samples studied.
The water vapor sorption experiment results with the leaf wash samples are shown with standard error bars in Figure 2. The percentage weight increase of moisture sorbed above the dry weight, Δw, is plotted with relative humidity, RH, as calculated in Equation (3). Significant sorption occurs at high humidities at and above 75%RH with the “Brackish mangrove” and “Cabinet mangrove.” Less so but still significant sorption occurs with the “Glasshouse chili” and the “Brackish euc.” All leaf washes appear to be somewhat hygroscopic. As the leaf sample washes are mixtures of different salts, minerals, oils, waxes, and other materials, sorption behaves less predictably, especially above 84%RH and this is discussed further in Figure 5. The two mangrove samples on the other hand behave more predictably due to their high NaCl content and minimal oils. As our focus is total water on the leaves, we consider the same data, plotted as the total of the triplicates, as total weight (mg) with relative humidity in Supplementary Figure S2, calculated with Equation (4). We compare the strongly deliquescent leaf wash samples that produced a clearly visible aqueous solution, in Figure 3. The “Brackish mangrove” as expected, was the most hygroscopic and becomes visibly liquid at 75%RH (POD of NaCl), aided by its high initial dry weight. The “Cabinet mangrove” also sorbed large amounts of water and became visibly wet at 75%RH, compatible with the dominance of NaCl in the coating. The “Brackish euc” became visibly wet at 84%RH, and the “Glasshouse chili” and “Ocean common reed” at 97%RH. In Table 4, we compare these results to the ICP-OES data in Table 3 for the combined weight of Ca, Mg, and Na in μg (that are postulated to be the most hygroscopic). We see the five samples that visibly deliquesce in Figure 3 correspond well to the five highest combined masses of these cations. The highest to lowest of the sum of Ca, Mg, and Na also correspond reasonably well to the ranking of the maximum percentage weight increase over the dry weight, Δw- %, in Figure 2, which suggests that hygroscopicity of the leaf wash material may be predicted from the combined mass of Ca, Mg, and Na from the ICP-OES data. For the “Brackish mangrove,” the materials present significantly deliquesced, over the total sample (total of three repeats), at 97%RH, produced 316 mg, as shown in Figure 3. This equates to 0.03–0.05 ml on one leaf (assuming uniformity over 6–10 leaves), with a thickness of liquid on one leaf of 7.3 μm (or 14.6 μm considering salt glands are mainly on one abaxial surface), which is similar but higher than estimates of 1 μm using other methods with plants that do not excrete salt (Burkhardt and Hunsche, 2013). We note other samples were hygroscopic and could also be deliquescent but were not included in Figure 3 as they did not visibly deliquesce. Table 5 compares the dry weight and maximum sorption to the leaf area, ordered in the same way as Table 4. The two mangrove samples produce similar dry weight and maximum sorption.
Figure 2. The percentage weight increase of moisture adsorbed of the leaf wash samples, Δw- %, vs. relative humidity, RH - %, plotted with standard error bars. Each leaf wash sample is shown in (A–L). Note the x-axis range is always the same but the y-axis range changes for each subfigure.
Figure 3. Samples that deliquesce visibly to the naked eye. The total weight (of three repeats with the dry weight, scaled with the blank) vs. humidity (%). The orange symbols and dashed lines indicate water is not yet visible (but may have hygroscopic growth), and the blue symbols and solid lines indicate where an aqueous solution was clearly visible. Note the significant weight of the brackish mangrove sample at 97%RH of 316 mg.
Control experiments for salt and oil sorption were conducted, for comparison to the leaf washes, to better understand whether the results were similar when salt mixtures and oils were present, as shown in Supplementary Figures S3–S6. All the controls deliquesced and formed an aqueous solution that was visible to the naked eye, except the two pure oils—Eucalyptus and tea tree. The salts and mixtures with CaCl 2 generally formed liquid at 32%RH (POD of CaCl2). Similarly, mixtures with NaCl deliquesce at 75% (POD of NaCl). The mangrove nutrient (that the “Cabinet mangrove” grows in a solution of) is dominantly made up of NaCl but also contains other salts with a lower POD. When comparing the mixture of NaCl plus Eucalyptus oil sample to the large NaCl sample, the POD is similar, however, the change in weight is less when oil is included, indicating the oil was able to prevent some level of sorption but did not prevent deliquescence. When applying this outcome to the leaf wash results, it could be relevant for the “Brackish euc,” which was able to form an aqueous solution, despite the presence of some oils but to a lesser extent than the mangroves (Figure 3). We note that the small NaCl sample has higher than expected sorption at 33%RH and this might be corrected with additional repeats.
The experimental data in Figure 4, for the “Brackish” and “Cabinet mangrove,” compared to the mangrove nutrient and NaCl control, is modeled with the Guggenheim, Anderson, and de Böer (GAB) isotherm (Anderson, 1946; de Böer, 1953; Guggenheim, 1966) (Equation S1). Figure 4 shows that the percentage moisture gain is similar for both mangroves, the GAB isotherm has a similar trend, and the percentage sorption is very similar at nearly all humidities. The sorption of the two mangrove samples compares well to the NaCl control and mangrove nutrient (mostly NaCl), and therefore, the sorption is likely driven largely by NaCl. The “Brackish” and “Cabinet mangrove” sorption at humidities between 75–100%RH, is somewhat higher than the mangrove nutrient and NaCl, which indicates that additional hygroscopic compounds are present, e.g., CaCl 2. When comparing the percentage moisture gain to the total weight in Figure 3, the “Brackish mangrove” has a much greater total weight at 97%RH but also started out with a heavier dry weight. When scaled with the dry weight, the percentage moisture gains of both mangrove samples are similar (Figure 4). The GAB constants have physical meaning and the values of k for the four isotherms are similar, so the interaction energy between the multiple layers of water is similar. The values for ws are higher for the two controls, indicating that they have a larger monolayer saturation value but possibly fewer multilayers than the two leaf washes. In summary, the two mangroves studied from a cabinet and near brackish water, both excrete salt through the leaves, and have sorption properties similar to, but greater than, NaCl and the mangrove nutrient, suggesting a dominance of NaCl but also with other hygroscopic materials.
Figure 4. A selection of adsorption isotherms including cabinet and brackish mangroves, along with the controls of the mangrove nutrient and NaCl with large sample size. The plot is the percentage moisture gain over the dry weight vs. relative humidity. The data are fitted with the GAB isotherm, as described in Equation (S1). The controls were formulated with a similar dry weight to the leaf wash samples. The parameters are described in Supplementary Table S2 and R2 values are greater than 98.6%.
We found a significant presence of lipophilic compounds, possibly oils or waxes. The presence of Eucalyptus oils in some leaf wash samples are indicated by the silver nitrate study, shown in Supplementary Figure S1, and waxes were found with the XRD (e.g., Supplementary Figure S10). In Figure 5, as leaf washes are mixtures of salts and lipophilic compounds, the sorption of the samples is less predictable. In Figure 5, by comparing the Eucalyptus leaf wash samples to the Eucalyptus and tea tree oil controls, the presence of oils in the leaf wash samples results in increased sorption followed by a decrease at high humidity, as the oils dominate the signature, similar to the pure oils. The “Brackish euc” is able to overcome the effect of the oil at high humidity and increased sorption is possible, to the point of visibly deliquescing. “Brackish euc” has a large presence of salts comparing Eucalyptus samples (μg of Ca, Mg, and Na in Table 4). The sorption overcomes that of the oil after a critical mass of water is reached, the salts dissociate, and high sorption occurs. The “NaCl with euc oil” control sample in Supplementary Figure S3J, indicates a similar behavior.
Figure 5. Percentage weight gain of moisture vs. relative humidity of all Eucalyptus leaf wash samples, along with two oil controls for comparison. The oils contributed to the increasing sorption at low humidity and then decrease in sorption between 33 and 84%RH, while the other hygroscopic ionic compounds present in the sample contributed to weight gain at high humidities such as shown by “Brackish euc.” The oil controls follow a similar trend to the leaf washes.
Materials including atmospheric aerosols on leaf surface substantially impact the interaction between the leaf surface and atmospheric moisture, even for plants grown in comparatively clean environments (e.g., growth cabinets and glasshouses). Our results, in line with other recent studies (Grantz et al., 2018, 2020), show that not only microscopic amounts of water but relatively large volumes of water visible to the naked eye can form on plant leaf surfaces. This water can influence stomatal function and anatomy (Grantz et al., 2018, 2020). Our results suggest that some experimental designs may require the incorporation of tests, to determine if leaf washing and air filtration are necessary and if a range of relative humidities is required instead of a single humidity, depending on the research question. These considerations may be particularly important when comparing crops/field work to glasshouse/cabinet studies, agrochemical penetration experiments with foliar sprays, foliar water uptake studies, and certain gas exchange experiments. We note the surprising case of the sorption of “Glasshouse chili” that was able to form water visible at 97%RH. This plant was 2 years old and did not experience any leaf washing. It is demonstrably possible that plants grown in a range of locations from glasshouses to growth cabinets may experience this phenomenon if washing of their leaves does not occur over prolonged periods. The “Town euc rain” sample contains impurities present in rain with larger amounts of Al, Cu, Fe, Si, and Zn present in the ICP-OES data in Table 3. “Brackish euc” has a larger than expected amount of Ca, unexpected as it is higher than the adjacent “Brackish mangrove” plant. The majority of the dry weight of both mangroves originates from the interior of the plant excreted through salt glands, though some aerosols and saltwater spray exist on the “Brackish mangrove” sample. The “Euc farm” sample has greater levels of K, Al, Mn, and Zn detected in the ICP-OES data, likely from agrochemical spray drift or particles from the nearby highway. Some samples in Table 3 including “Euc farm” had large amounts of K present but were usually associated with Eucalyptus and, hence, had reduced sorption caused by the presence of oils.
Hygroscopic materials on the plant leaf surface will be affected by the adjacent/local humidity. Due to the boundary layer (Burkhardt and Hunsche, 2013), temperature, and the action of stomata releasing water vapor from the interior of the leaf, the humidity on the leaf surface may be higher than the ambient relative humidity in the air. Comparing air to leaf surface humidity, an increase of 35%RH above the daytime ambient humidity has been found (Boulard et al., 2002). Applying this 35%RH increase, e.g., if the ambient humidity is 40% and the leaf surface humidity is at 75%, then NaCl (POD 75%RH) on the leaf surface will be able to deliquesce and form liquid water. When suspended or on a passive surface, such as rock, the humidity would be below the POD of NaCl and closer to the ambient humidity (of 40%), thus the NaCl cannot deliquesce. When applying this boundary layer effect to the sorption of salts in situ on the plant leaf, salts will sorb moisture from the local environment even though the ambient relative humidity is less than the salts' POD. Due to the boundary layer, salts on plant leaves may form an aqueous solution at a much larger range of ambient humidities, further increasing the significance of this study. The boundary layer is relevant to plant leaves in their natural environment but may have less importance in artificial experimental settings, e.g., in the cuvette of a photosynthesis system (e.g., Li-6400 or Li-6800) where a high fan speed is used to minimize the boundary layer thickness and in an environmental scanning electron microscope (ESEM) the vacuum conditions would minimize the boundary layer, therefore, results of salts on leaves viewed with ESEM may not be influenced by this boundary layer effect.
From observations during the salt control experiments, 1 g of solid CaCl 2 can sorb significant proportions of water and visibly deliquesce very quickly, in a matter of 5–10 mins in relatively low humidities, while it may take days to reach adsorption equilibrium, or completely dry out again in the oven (desorption). We note that in the context of hygroscopic materials on plant leaves in the environment, these adsorption and desorption timescales are very relevant as temperature and humidity change throughout the day. For example, if the air humidity increases, water may be quickly adsorbed by these materials, or if the air temperature increases in the morning leading to a decrease in air humidity, hygroscopic particles on the leaf surface will extend evaporation times of residual water, and keep the leaf wet longer. These adsorption and desorption timescales for hygroscopic particles in situ on the plant leaf require further research. The experimental data of the current study on the sorption of materials on leaves, Δw, can be utilized in a mechanistic model (Tredenick et al., 2018) for droplet evaporation. If considering evaporation where the RH is changing significantly with time, over the evaporation timescale, it may be necessary to consider additional mechanisms (Gregson et al., 2019) including desorption hysteresis (involving crystallization or the point of efflorescence, e.g., NaCl Tang et al., 1997) of the material on the plant leaf.
Future study could include desorption, sorption data measured with time increments over a time span of longer than 3 days, conducting a similar study but looking more specifically at one location including many plant species, and individuals/biological replicates within that species. Water vapor sorption experiments could be performed on the leaf wash pellet left behind after centrifugation, which may contain insoluble waxes and mineral grains, organic debris, insect material, and material sloughed from the leaf itself. This is relevant for the brackish mangrove sample, where the leaves had a larger amount of dried mineral dust present as they were periodically submerged at certain tides. The pellets were tested via XRD for the same species as in Supplementary Figures S7, S8 but no NaCl or other salts were found, and were not investigated further.
Six-week-old spongy tobacco leaves [native Australian tobacco (Nicotiana benthamiana)] were deemed unsuitable for experimentation as when shaken the leaves stained the water green, likely with chlorophyll leaching from ruptured cells. We note that it is uncommon for sorption isotherms of oils to be studied. When studied, stabilizers are often included and sorption experiments on oils can take 1–2 weeks to reach equilibrium (Charoen et al., 2015). Further research is required, especially for prolific Eucalyptus. Hygroscopic salts on leaves assist in maintaining leaf surface wetness when stomata are open during the day even at low humidity, maintain stomatal function and development, assist with foliar water uptake and extend the droplet evaporation time of dewfall. In terms of foliar applied agrochemicals, hygroscopic salts on the leaf either in situ or in the applied droplet formulation can impact agrochemical penetration by altering the formulation's effectiveness at a given relative humidity, rate of droplet evaporation, penetration effectiveness of active ingredients, and surface tension. Salts can also impact the experimental setup and design, and if leaves are regularly washed with water or not, especially when comparing field and glasshouse grown plants. As the surface properties of leaves vary a great deal, as do environmental properties, no single study can be specifically applicable to other plants and areas. Despite this, the general cases explored here have wide-reaching implications in ecological, physiological, and agricultural studies.
This study has demonstrated our aim of showing that the materials on plant leaves are hygroscopic and that an aqueous solution can form over small amounts of particles on the plant leaf surface grown in a range of environments. Five leaf washes attracted to water to the point of visibly deliquescing, even in a glasshouse plant. Mangroves that excrete salt are covered with a layer that can form up to a total of 0.3 ml of liquid (for 6–10 leaves or 30 μl on one leaf) at high humidities. This salt is mostly NaCl but also contains other hygroscopic particles. An unexpected outcome of our study was the higher than expected levels of oils on the surface for all Eucalyptus samples studied.
The original contributions presented in the study are included in the article/Supplementary Materials, further inquiries can be directed to the corresponding author/s.
ET is responsible for project motivation, experimental design, conducting vapor sorption experiments, analysis, model fitting, results and article writing, editing, and revision. HS-W is responsible for experimental and biological consultation, article editing, and revision. TE is responsible for conducting ICP-OES experiments and methodology, article editing, and revision. All authors contributed to the article and approved the submitted version.
The authors acknowledge funding provided by the Australian Research Council Centre of Excellence for Translational Photosynthesis (CE1401000015).
The authors declare that the research was conducted in the absence of any commercial or financial relationships that could be construed as a potential conflict of interest.
All claims expressed in this article are solely those of the authors and do not necessarily represent those of their affiliated organizations, or those of the publisher, the editors and the reviewers. Any product that may be evaluated in this article, or claim that may be made by its manufacturer, is not guaranteed or endorsed by the publisher.
The authors thank Ulrike Troitzsch (ANU) for conducting the XRD experiments, Brett Knowles (ANU) for assisting with the ICP-OES experiments, and Graham Farquhar (ANU) for his advice and thoughtful conversations.
The Supplementary Material for this article can be found online at: https://www.frontiersin.org/articles/10.3389/fpls.2022.722710/full#supplementary-material
Ajibola, O (1986). Desorption isotherms for plantain at several temperatures. J. Food Sci. 51, 169–171. doi: 10.1111/j.1365-2621.1986.tb10862.x
Anderson, R. B (1946). Modifications of the brunauer, emmett and teller equation. J. Am. Chem. Soc. 68, 686–691. doi: 10.1021/ja01208a049
Belbekhouche, S., Bras, J., Siqueira, G., Chappey, C., Lebrun, L., Khelifi, B., et al. (2011). Water sorption behavior and gas barrier properties of cellulose whiskers and microfibrils films. Carbohydr. Polym. 83, 1740–1748. doi: 10.1016/j.carbpol.2010.10.036
Boulard, T., Mermier, M., Fargues, J., Smits, N., Rougier, M., and Roy, J. C. (2002). Tomato leaf boundary layer climate: implications for microbiological whitefly control in greenhouses. Agric. Forest Meteorol. 110, 159–176. doi: 10.1016/S0168-1923(01)00292-1
Brahney, J., Ballantyne, A. P., Sievers, C., and Neff, J. C. (2013). Increasing Ca deposition in the western us: the role of mineral aerosols. Aeolian Res. 10, 77–87. doi: 10.1016/j.aeolia.2013.04.003
Burgess, S., and Dawson, T. (2004). The contribution of fog to the water relations of Sequoia sempervirens (D. Don): foliar uptake and prevention of dehydration. Plant, Cell Environ. 27, 1023–1034. doi: 10.1111/j.1365-3040.2004.01207.x
Burkhardt, J., Basi, S., Pariyar, S., and Hunsche, M. (2012). Stomatal penetration by aqueous solutions-an update involving leaf surface particles. New Phytol. 196, 774–787. doi: 10.1111/j.1469-8137.2012.04307.x
Burkhardt, J., and Gerchau, J. (1994). A new device for the study of water vapour condensation and gaseous deposition to plant surfaces and particle samples. Atmos Environ. 28, 2012–2017. doi: 10.1016/1352-2310(94)90470-7
Burkhardt, J., and Hunsche, M. (2013). Breath figures on leaf surfaces–formation and effects of microscopic leaf wetness. Front. Plant Sci. 4, 422. doi: 10.3389/fpls.2013.00422
Burkhardt, J., Kaiser, H., Goldbach, H., and Kappen, L. (1999). Measurements of electrical leaf surface conductance reveal recondensation of transpired water vapour on leaf surfaces. Plant Cell Environ. 22, 189–196. doi: 10.1046/j.1365-3040.1999.00387.x
Burkhardt, J., and Pariyar, S. (2014). Particulate pollutants are capable to ‘degrade' epicuticular waxes and to decrease the drought tolerance of Scots pine (Pinus sylvestris L.). Environ. Pollut. 184, 659–667. doi: 10.1016/j.envpol.2013.04.041
Burkhardt, J., Zinsmeister, D., Grantz, D. A., Vidic, S., Sutton, M. A., Hunsche, M., et al. (2018). Camouflaged as degraded wax: hygroscopic aerosols contribute to leaf desiccation, tree mortality, and forest decline. Environ. Res. Lett. 13, 085001. doi: 10.1088/1748-9326/aad346
Charoen, R., Jangchud, A., Jangchud, K., Harnsilawat, T., and McClements, D. J. (2015). The physical characterization and sorption isotherm of rice bran oil powders stabilized by food-grade biopolymers. Drying Technol. 33, 479–492. doi: 10.1080/07373937.2014.962142
Chen, Y., and Lee, W. G. (2001). The effect of surfactants on the deliquescence of sodium chloride. J. Environ. Sci. Health A 36, 229–242. doi: 10.1081/ESE-100102620
Coopman, R. E., Nguyen, H. T., Mencuccini, M., Oliveira, R. S., Sack, L., Lovelock, C. E., et al. (2021). Harvesting water from unsaturated atmospheres: deliquescence of salt secreted onto leaf surfaces drives reverse sap flow in a dominant arid climate mangrove, Avicennia marina. New Phytol. 231, 1401–1414. doi: 10.1111/nph.17461
Dawson, T. E., and Goldsmith, G. R. (2018). The value of wet leaves. New Phytol. 219, 1156–1169. doi: 10.1111/nph.15307
Dominguez, E., and Heredia, A. (1999). Water hydration in cutinized cell walls: a physico-chemical analysis. Biochim. Biophys. Acta 1426, 168–176. doi: 10.1016/S0304-4165(98)00152-4
Dow Chemical Company (2003). Calcium Chloride Handbook. A Guide to Properties, Forms, Storage and Handling. Midland, MI: Dow Chemical Company.
Dutcher, C. S., Wexler, A. S., and Clegg, S. L. (2010). Surface tensions of inorganic multicomponent aqueous electrolyte solutions and melts. J. Phys. Chem. A 114, 12216–12230. doi: 10.1021/jp105191z
Eichert, T., and Goldbach, H. E. (2008). Equivalent pore radii of hydrophilic foliar uptake routes in stomatous and astomatous leaf surfaces-further evidence for a stomatal pathway. Physiol. Plant 132.4, 491–502. doi: 10.1111/j.1399-3054.2007.01023.x
Eller, C. B., Lima, A. L., and Oliveira, R. S. (2016). Cloud forest trees with higher foliar water uptake capacity and anisohydric behavior are more vulnerable to drought and climate change. New Phytol. 211, 489–501. doi: 10.1111/nph.13952
Fernández, V., Bahamonde, H. A., Javier Peguero-Pina, J., Gil-Pelegrín, E., Sancho-Knapik, D., Gil, L., et al. (2017). Physico-chemical properties of plant cuticles and their functional and ecological significance. J. Exp. Bot. 68, 5293–5306. doi: 10.1093/jxb/erx302
Gilbert, G. S., Mejía-Chang, M., and Rojas, E. (2002). Fungal diversity and plant disease in mangrove forests: salt excretion as a possible defense mechanism. Oecologia 132, 278–285. doi: 10.1007/s00442-002-0966-9
Grantz, D. A., Karr, M., and Burkhardt, J. (2020). Heterogeneity of stomatal pore area is suppressed by ambient aerosol in the homobaric species, vicia faba. Front. Plant Sci. 11, 897. doi: 10.3389/fpls.2020.00897
Grantz, D. A., Zinsmeister, D., and Burkhardt, J. (2018). Ambient aerosol increases minimum leaf conductance and alters the aperture-flux relationship as stomata respond to vapor pressure deficit (vpd). New Phytol. 219, 275–286. doi: 10.1111/nph.15102
Gregson, F., Robinson, J., Miles, R., Royall, C., and Reid, J. (2019). Drying kinetics of salt solution droplets: water evaporation rates and crystallization. J. Phys. Chem. B 123, 266–276. doi: 10.1021/acs.jpcb.8b09584
Hayes, M. A., Chapman, S., Jesse, A., O'Brien, E., Langley, J. A., Bardou, R., et al. (2020). Foliar water uptake by coastal wetland plants: a novel water acquisition mechanism in arid and humid subtropical mangroves. J. Ecol. 108, 2625–2637. doi: 10.1111/1365-2745.13398
ICDD (2004). PDF-2. Phase Identification + Value, International Centre for Diffraction Data. Pennsylvania, United States.
Isla, R., and Aragüés, R. (2009). Response of alfalfa (Medicago sativa L.) to diurnal and nocturnal saline sprinkler irrigations. I: Total dry matter and hay quality. Irrigat. Sci. 27, 497–505. doi: 10.1007/s00271-009-0167-y
Katata, G., and Held, A. (2021). Combined measurements of microscopic leaf wetness and dry-deposited inorganic compounds in a spruce forest. Atmos Pollut. Res. 12, 217–224. doi: 10.1016/j.apr.2020.11.004
Kolthoff, I. M., Sandell, E. B., Meehan, E., and Bruckenstein, S. (1969). Quantitative Chemical Analysis, Vol. 826. London: Macmillan London.
Likos, W. J., and Lu, N. (2002). Water vapor sorption behaviour of smectite-kaolinite mixtures. Clays Clay Miner. 50, 553–561. doi: 10.1346/000986002320679297
Lindberg, S. E., Lovett, G. M., Richter, D., and Johnson, D. W. (1986). Atmospheric deposition and canopy interactions of major ions in a forest. Science 231, 141–145. doi: 10.1126/science.231.4734.141
Nguyen, H. T., Meir, P., Sack, L., Evans, J. R., Oliveira, R. S., and Ball, M. C. (2017). Leaf water storage increases with salinity and aridity in the mangrove Avicennia marina: integration of leaf structure, osmotic adjustment and access to multiple water sources. Plant Cell Environ. 40, 1576–1591. doi: 10.1111/pce.12962
OxyChem (2014). Calcium Chloride Properties. Dallas, TX: Occidental Chemical Corporation (OxyChem). Available online at: http://www.oxy.com/OurBusinesses/Chemicals/Products/Documents/CalciumChloride/173-01791.pdf (accessed March 05, 2014).
Sæbø, A., Popek, R., Nawrot, B., Hanslin, H. M., Gawronska, H., and Gawronski, S. (2012). Plant species differences in particulate matter accumulation on leaf surfaces. Sci. Total Environ. 427, 347–354. doi: 10.1016/j.scitotenv.2012.03.084
Simon, R. D., Abeliovich, A., and Belkin, S. (1994). A novel terrestrial halophilic environment: the phylloplane of Atriplex halimus, a salt-excreting plant. FEMS Microbiol. Ecol. 14, 99–109. doi: 10.1111/j.1574-6941.1994.tb00097.x
Tang, I. N., Tridico, A. C., and Fung, K. H. (1997). Thermodynamic and optical properties of sea salt aerosols. J. Geophys. Res. 102, 23269. doi: 10.1029/97JD01806
Taylor, J (1991). Computer programs for standardless quantitative analysis of minerals using the full powder diffraction profile. Powder diffract. 6, 2–9. doi: 10.1017/S0885715600016778
Tredenick, E. C., and Farquhar, G. D. (2021). Dynamics of moisture diffusion and adsorption in plant cuticles including the role of cellulose. Nat. Commun. 12, 5042. doi: 10.1038/s41467-021-25225-y
Tredenick, E. C., Farrell, T. W., and Forster, W. A. (2018). Mathematical modeling of diffusion of a hydrophilic ionic fertilizer in plant cuticles: surfactant and hygroscopic effects. Front. Plant Sci. 9, 1888. doi: 10.3389/fpls.2018.01888
Tredenick, E. C., Farrell, T. W., Forster, W. A., and Psaltis, S. T. P. (2017). Nonlinear porous diffusion modeling of hydrophilic ionic agrochemicals in astomatous plant cuticle aqueous pores: a mechanistic approach. Front. Plant Sci. 8, 746. doi: 10.3389/fpls.2017.00746
Keywords: foliar, water use, hygroscopic, point of deliquescence, sorption, plant leaf, aerosol, adsorption isotherm
Citation: Tredenick EC, Stuart-Williams H and Enge TG (2022) Materials on Plant Leaf Surfaces Are Deliquescent in a Variety of Environments. Front. Plant Sci. 13:722710. doi: 10.3389/fpls.2022.722710
Received: 09 June 2021; Accepted: 13 June 2022;
Published: 12 July 2022.
Edited by:
Nacer Bellaloui, Agricultural Research Service (USDA), United StatesReviewed by:
Jürgen Burkhardt, University of Bonn, GermanyCopyright © 2022 Tredenick, Stuart-Williams and Enge. This is an open-access article distributed under the terms of the Creative Commons Attribution License (CC BY). The use, distribution or reproduction in other forums is permitted, provided the original author(s) and the copyright owner(s) are credited and that the original publication in this journal is cited, in accordance with accepted academic practice. No use, distribution or reproduction is permitted which does not comply with these terms.
*Correspondence: E. C. Tredenick, ZWxvaXNlLnRyZWRlbmlja0BhbnUuZWR1LmF1
Disclaimer: All claims expressed in this article are solely those of the authors and do not necessarily represent those of their affiliated organizations, or those of the publisher, the editors and the reviewers. Any product that may be evaluated in this article or claim that may be made by its manufacturer is not guaranteed or endorsed by the publisher.
Research integrity at Frontiers
Learn more about the work of our research integrity team to safeguard the quality of each article we publish.