- 1State Key Laboratory of Soil and Sustainable Agriculture, Institute of Soil Science, Chinese Academy of Sciences, Nanjing, China
- 2University of Chinese Academy of Sciences, Beijing, China
- 3School of BioSciences, The University of Melbourne, Parkville, VIC, Australia
- 4Faculty of Land and Food Systems, University of British Columbia, Vancouver, BC, Canada
- 5Amway Botanical R&D Center, Wuxi, China
Syringic acid (SA) is a novel biological nitrification inhibitor (BNIs) discovered in rice root exudates with significant inhibition of Nitrosomonas strains. However, the inhibitory effect of SA on nitrification and nitrous oxide (N2O) emissions in different soils and the environmental factors controlling the degree of inhibition have not been studied. Using 14-day microcosm incubation, we investigated the effects of different concentrations of SA on nitrification activity, abundance of ammonia-oxidizing microorganisms, and N2O emissions in three typical agricultural soils. The nitrification inhibitory efficacy of SA was strongest in acidic red soil, followed by weakly acidic paddy soil, with no significant effect in an alkaline calcareous soil. Potential nitrification activity (PNA) were also greatly reduced by SA additions in paddy and red soil. Pearson correlation analysis showed that the inhibitory efficacy of SA might be negatively correlated with soil pH and positively correlated with clay percentage. SA treatments significantly reduced N2O emissions by 69.1-79.3% from paddy soil and by 40.8%-46.4% from red soil, respectively, but no effect was recorded in the calcareous soil. SA addition possessed dual inhibition of both ammonia-oxidizing archaea (AOA) and ammonia-oxidizing bacteria (AOB) abundance in paddy and red soil. Structural equation modelling revealed that soil ammonium (NH4+) and dissolved organic carbon content (DOC) were the key variables explaining AOA and AOB abundance and subsequent N2O emissions. Our results support the potential for the use of the BNI SA in mitigating N2O emissions and enhancing N utilization in red and paddy soils.
Introduction
Ammonium (NH4+) is the main form of nitrogen (N) absorbed by plants (Kronzucker et al., 1997; Glass et al., 2002). NH4+ can also be readily oxidized by soil microbes, producing nitrite (NO2-) and nitrate (NO3-) through the process of nitrification, which leads to significant losses of N fertilizer, atmospheric N pollution caused by emissions of nitrous oxide (N2O), and NO3- pollution of waterways (Coskun et al., 2017a; Coskun et al., 2017b). Inhibiting nitrification of NH4+ into NO3- can reduce such N losses (Coskun et al., 2017a; Coskun et al., 2017b), an approach also recently proposed as a more generalized “ammonium solution” to reduce N pollution from agricultural fields and to enhance crop yield (Subbarao and Searchinger, 2021). Practices such as deep N-fertilizer placement and controlled-release fertilizers have also been proposed to stabilize reduced N in soils and minimize N conversion and losses (Zheng et al., 2016; Min et al., 2021a). While the application of several synthetic nitrification inhibitors (SNIs) has increased N utilization in fields (Zaman et al., 2009; Min et al., 2021b), limitations such as high cost, inconsistency in field performance, inability to function in acidic environments, and food safety risks have prevented their widespread adoption in modern agriculture (Subbarao et al., 2012).
The use of plant-derived biological nitrification inhibitors (BNIs) is an environmentally friendly strategy to reduce N pollution and boost crop yields (Subbarao et al., 2012; Coskun et al., 2017b). BNIs have the potential to overcome the limitations of SNIs through breeding crop varieties with higher BNI capacity (Subbarao and Searchinger, 2021). While BNI capacity has been well evaluated in tropical pasture plants, field crops, and trees (O'Sullivan et al., 2016; Laffite et al., 2020), relatively less is known about BNIs in the cereal crops, rice, wheat, and maize (Coskun et al., 2017b). In previous studies, we reported the first BNI 1,9-decanediol (a hydrophobic fatty alcohol) from root exudates of rice (Sun et al., 2016; Zhang et al., 2019). Subsequently, a second BNI exuded from roots of cultivated rices, syringic acid (SA, a hydrophilic phenolic acid), was discovered, which displayed synergism with 1,9-decanediol in inhibiting nitrification carried out by soil microorganisms (Lu et al., 2022).
Compared with research on SNIs, research on BNIs is still in its infancy. A small number of recent studies have focused on the nitrification-inhibitory effect of some BNIs in field soils when applied as pure compounds. Methyl 3,4-hydroxyphenyl propionate (MHPP) from sorghum roots was shown to suppress nitrification in a neutral soil, whereas the hydrophilic sakuranetin had no inhibitory effect (Subbarao et al., 2013). A recent study by Ma et al. (2021) demonstrated that two long-chain unsaturated fatty acids, linoleic acid (LA) and linolenic acid (LN), from the shoot tissue of pasture grass can cause nitrification inhibition in an acidic sandy loam. However, most of these BNI function tests have been verified in one type of soil at a time. Thus, it is necessary to assess the inhibitory profiles of BNIs on different soil types colonised by different ammonia-oxidizing microorganisms, as it will help identify precise targets and the range of possible applications of BNIs in agricultural N management.
The efficacies of SNIs have been extensively studied and can be highly variable across soils. These differences in efficacy have been ascribed to differences in soil pH, water content, temperature, organic matter content, clay percentage, and applied NI dose (Barth et al., 2008; Shi et al., 2016; Guardia et al., 2018; Cui et al., 2021). Only two soil-based incubation studies have thus far evaluated the efficacy of a BNI compound in different soil types. Lu et al. (2019) showed that 1,9-decanediol, exuded by rice roots, can act as a more potent BNI in acidic soil than in alkaline soil, underscoring that the inhibition profile of BNIs varies with soil pH and free BNI concentration. The nitrification inhibition of MHPP was higher in the acidic soil than in the calcareous soil (Lan et al., 2022). For the recently identified phenolic BNI SA from rice root exudates, the inhibition profile on different soils, and the key factors responsible for differences in inhibition profile, have not been examined.
BNIs are considered a “green” and cost-effective strategy to mitigating agricultural greenhouse gas emissions (Subbarao et al., 2017). In addition to planting tropical forage grasses or sorghum with high BNI capacity (Subbarao et al., 2009; Zhang et al., 2015; Byrnes et al., 2017; Villegas et al., 2020), several recent studies have evaluated the potential role of direct application of specific BNI compounds in reducing soil N2O emission. The fatty alcohol 1,9-decanediol obtained from rice root exudates was shown to significantly reduce N2O emissions by an average of 48% in three agricultural soils (Lu et al., 2019). N2O emissions could be reduced by >60% when the phenylpropanoid MHPP was combined with other N-management measures such as root-zone fertilization, the application of urease inhibitors, or that of biochar (Yao et al., 2020; Lan et al., 2021). However, Ma et al. (2021) have pointed out the risk of promoting N2O emissions by the addition of high concentrations of two fatty acids, LN and LA. Thus, not all BNIs are actually effective in mitigating N2O emissions, and the efficacy of BNIs to reduce N2O emissions may depend on BNI type. It remains unknown whether the newly-discovered phenolic acid SA can inhibit N2O emission in different soils.
As drivers of the first and rate-limiting step of nitrification, ammonia-oxidizing archaea (AOA) and ammonia-oxidizing bacteria (AOB) are considered the principal microbial contributors to N2O emissions (Santoro et al., 2011; Wang et al., 2015). Due to different metabolic pathways, AOB and AOA are likely to occupy different niches across soils, driven by soil pH, temperature, dissolved organic carbon and soil N level (Prosser et al., 2020; Tao et al., 2021a; Tao et al., 2021b). BNIs have the potential to regulate both the AOA and AOB community (Nardi et al., 2013; Lu et al., 2019; Sarr et al., 2020; Lan et al., 2022). However, changes in ammonia oxidizer communities are only contributory to nitrification, and the relationship to subsequent N2O emissions and the relevant abiotic control factors have not been characterised.
To better understand different soil types where SA acts as an inhibitor of nitrification and N2O emission, 14-day microcosm experiments were conducted in three agricultural soils with varing properties. Different amounts of SA were applied to monitor the nitrogen dynamics, the abundance of ammonia oxidizers, and N2O emissions. The objectives were: (1) to explore the nitrification inhibitory impact of SA in different types of soil and relevant control factors, (2) to evaluate the potential of SA to reduce N2O emissions from soils, (3) to assess the effect of SA on the population of ammonia oxidizers, and (4) to establish the linkages between soil physicochemical properties, abundance of ammonia oxidizers, and N2O emissions by structural equation modeling (SEM).
Materials and methods
Soil sampling
Soil samples were collected from three sites, which represent the calcareous soil, paddy soil, and red soil. The calcareous soil (sandy loam) was collected from Dezhou (36°83′ N, 116°58′ E), a paddy soil (silt loam) was collected from Yinxin (31°39′ N, 119°28′ E), and the red soil (loamy clay) was sampled from Yintan (26°45′ N, 111°52′ E), which are located in typical agricultural areas of China. Soil samples (0–20 cm depth) were collected, air-dried, and sieved through 2-mm mesh before use.
Soil physicochemical analysis
Soil pH was measured by fresh soil (1:2.5 (w/v) soil to water solution) using pH electrodes (Mettler Toledo, Switzerland). Soil exchangeable NH4+-N and NO3−-N were colorimetrically quantified in 2 mol L-1 KCl extracts by continuous flow analysis (Skalar, Breda, Netherlands). Total C and total N were detected using a Vario Max CN analyzer (Elementar, Hanau, Germany). Soil organic matter (SOM) was determined following the K2Cr2O7 wet oxidation method. Soil texture (sand, silt, and clay fractions) was assessed with a laser diffraction particle size analyzer (LS13320, Beckman Coulter Co.). The dissolved organic carbon (DOC) concentration was determined by a TOC analyzer (Multi N/C 3100, Analytik Jena AG). Details of three soil physicochemical characteristics are shown in Table 1.
Soil microcosm experiments
The laboratory soil incubation was set up in 125-ml serum vials containing 20 g of soils (oven dry-weight equivalent). SA (C9H10O5; MW:198) and dicyandiamide (DCD) were purchased from Sigma-Aldrich (Shanghai, China). The five treatments were performed in triplicate: 1) (NH4)2SO4 control (N 200 mg kg−1 soil, CK); 2) (NH4)2SO4 plus SA at 500 mg kg−1 soil (SA-high dose, SA-500); 3) (NH4)2SO4 plus SA at 200 mg kg−1 soil (SA-medium dose, SA-200); 4) (NH4)2SO4 plus SA at 100 mg kg−1 soil (SA-low dose, SA-100); 5) (NH4)2SO4 plus DCD at 20 mg kg−1 soil (10% of applied NH4+-N according to the typically recommended rate, DCD) (McGeough et al., 2016). Inhibition by the biological nitrification inhibitor SA was compared with that by the synthetic nitrification inhibitor DCD, to gain a better understanding of how various soil types respond to different inhibitor types. The dosages of SA were chosen according to our previous study where the nitrification inhibitory efficacy was highest at 500 mg kg-1 and smallest at 100 mg kg-1 (Lu et al., 2022). These dosages also fall into the range of BNI application rates in other soil incubation experiments (Subbarao et al., 2008; Nardi et al., 2013; Subbarao et al., 2013; Ma et al., 2021). The SA powder was dissolved in (NH4)2SO4 solution by ultrasound exposure, the solution was then applied uniformly to soils according to Lu et al. (2019). The vials were incubated at 60% waterfilled pore space (WFPS) in the dark in a temperature-controlled incubator at 25°C. Every three days, the bottles were opened for aeration and weighed, and then the appropriate amount of deionised water was added for maintaining soil moisture. Soil samples were destructively collected on 0, 7, 14 days of incubation. Potential nitrification activity (PNA) was determined via the shaken slurry method described by Hart et al. (1994) and the details were given in Supplementary materials. Nitrification inhibitory efficacy (NIE, %) was calculated using the following formula, according to Lu et al. (2019): Nitrification inhibitory efficacy (NIE, %) = ((NO3−-N produced in the (NH4)2SO4 control) – (NO3−-N produced in the SA and DCD treatments))/(NO3−-N produced in the (NH4)2SO4 control) × 100.
Gas sampling and N2O flux measurement
After 24 h of closure, gas samples (5 mL) from the headspace using syringe (20 mL) were collected at 1, 2, 3, 4, 5, 7, 10 and 14 days, and were transferred to pre-evacuated 20-mL headspace gas containers. After sampling, all bottles were ventilated for 30 min and then resealed. Gas samples were determined for N2O concentrations by gas chromatograph (HP7820A, Agilent Technologies, CA, USA) equipped with an electron capture detector (ECD).
The N2O fluxes were calculated according to Tao et al. (2021b), using the equation described below:
where F: N2O emission flux, μg kg−1 soil d−1 (N2O-N); dc: gas concentration; dt: sampling interval; M: molar mass, 28 g mol−1 (N2O-N); Vm: molar volume of gas, 22.4 L mol−1; V: headspace of the bottle, L; T: incubation temperature,°C; m: soil dry weight, kg.
Cumulative N2O emissions (E, mg N2O-N kg-1 soil) were calculated according to Tao et al. (2021b), using the following equation:
where F represents the N2O flux (μg N2O-N kg−1 soil d−1), n is the nth sampling, and (Dn+1-Dn) represents the number of days between two adjacent samplings.
Soil DNA extraction and quantitive PCR analysis
DNA was extracted from 0.25 g of freeze-dried soil using MoBio PowerSoil DNA-isolation kits (MoBio Laboratories, Carlsbad, CA, USA). The purity and quantity of the extracted DNA were determined by a NanoDrop ND1000 spectrophotometer (NanoDrop Technologies, Wilmington, USA) and the samples were stored at −20°C until use.
Real-time quantitative PCR (qPCR) was performed to quantify the abundance of AOA and AOB ammonia monooxygenase genes (amoA). The PCR assays were conducted on a LightCycler 480 (Roche Diagnostics, Mannheim, Germany), using the primer pairs Arch-amoAF/Arch-amoA (Francis et al., 2005) and amoA-1F/amoA-2R (Rotthauwe et al., 1997), respectively. The 10-μL reaction mixture contained 5 μL of SYBR Premix Ex Taq (TaKaRa, Tokyo, Japan), 0.4 μL of each of the forward and reverse primers (10 μM), and 0.5 μL of dilluted DNA as a template. Standard curves dilution series from 1×101 to 1×107 copies were created. qPCR was conducted in triplicate, and amplification efficiencies ranged from 86.2–94.6%, with R2 values > 0.99.
Statistical analysis
One-way ANOVA was applied in SPSS Statistics 18.0 to determine the effect of SA on soil NH4+-N and NO3−-N, PNA, cumulative N2O emissions, AOA and AOB abundance. In addition, soil pH, SOM, clay percentage, PNA, and percent nitrification inhibitory efficacy among the three soils were analyzed by using the Pearson correlation test with Origin 2022. A linear regression analysis was used to study the relationship between soil NO3−-N and the abundance of ammonia oxidizers using Origin 2022. SEM was conducted to explore the causal linkages among N2O emissions AOA and AOB abundance, and soil properties, using the software AMOS 22.0. Several indicators were used to evaluate the overall fit of the model, ie., the P value, Chi-square value (χ2), comparative fit index (CFI), and root mean square error of approximation (RMSEA).
Results
NO3–-N, NH4+-N concentrations
In the (NH4)2SO4 control of the calcareous soil, the NO3–-N concentrations showed an increasing trend from 19.7 mg kg-1 soil to 189.6 mg kg-1 at day 14 (Figure 1A). The NH4+-N contents decreased rapidly from 195 mg kg-1 soil to 72.5 mg kg-1 soil during the first 7 days, and showed further reduction to 2.25 mg kg-1 soil at day 14 (Figure 1B). There was no evidence of significant inhibition by SA at all three dose treatments, and the concentrations of soil NH4+-N and NO3–-N remained unchanged both at day 7 and day 14 compared to the control. By contrast, DCD addition significantly (P < 0.05) decreased the formation of NO3–-N (Figure 1A) and slowed the NH4+-N oxidation down at two sampling points, with about 87.7 mg NH4+-N kg-1 soil remaining in the end (Figure 1B).
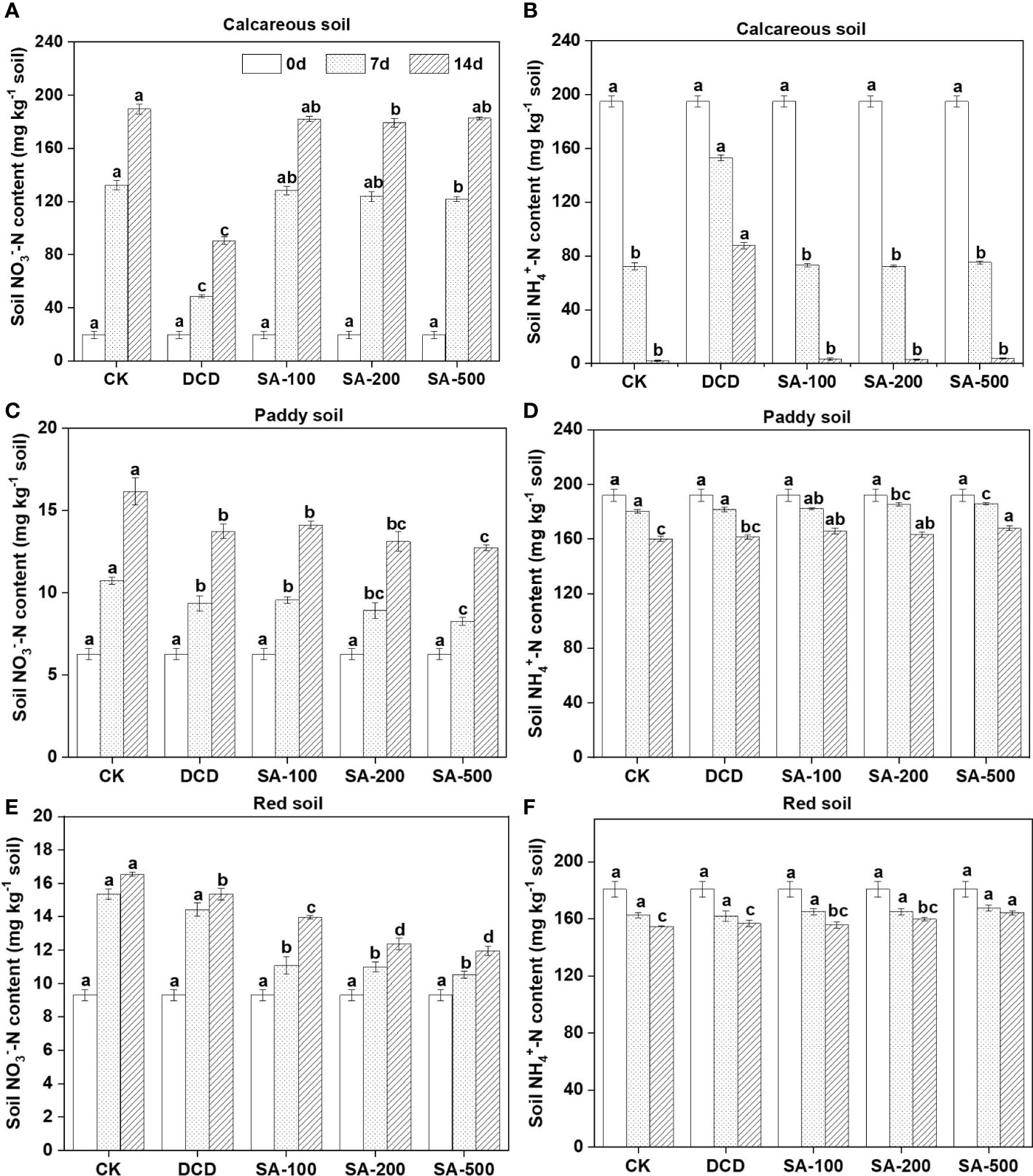
Figure 1 Effect of different concentrations of syringic acid (SA) and dicyandiamide (DCD) on soil NO3- and NH4+ concentrations in the calcareous (A, B), paddy (C, D), and red soil (E, F) during a 14-d incubation. Values are means ± SE (n=3). Different letters indicate significant differences at P < 0.05 (LSD test) among treatments at each sampling time.
Although the nitrification rate of the weakly acidic paddy soil was less than that of the alkaline calcareous soil, low-dose, medium-dose, and high-dose applications of SA all significantly inhibited nitrate production at day 7 by 10.9%, 16.7%, and 23.0%, respectively compared to the control (Figure 1C). This is in line with the higher NH4+-N level compared to the control (Figure 1D). Similarly, the addition of DCD slowed down the formation of NO3–-N by 12.8% compared to the control, but the NO3–-N amount was higher than that in the high-dose SA treatment at the end of incubation (Figure 1C), suggesting a weaker inhibition of DCD than of SA in the paddy soil.
In the acidic red soil, there was no apparent dose-response relationship between SA and nitrification inhibitory efficacy (Figures 1E, F). Compared to the N control, the low-dose, medium-dose, and high-dose applications of SA significantly inhibited nitrate production by 27.8%, 28.4%, and 31.4% at day 7, respectively (Table 2), whereas DCD showed no significant inhibition. Moreover, the inhibition by SA in the red soil was persistent and superior to that in the other two soils during the 14-day incubation.
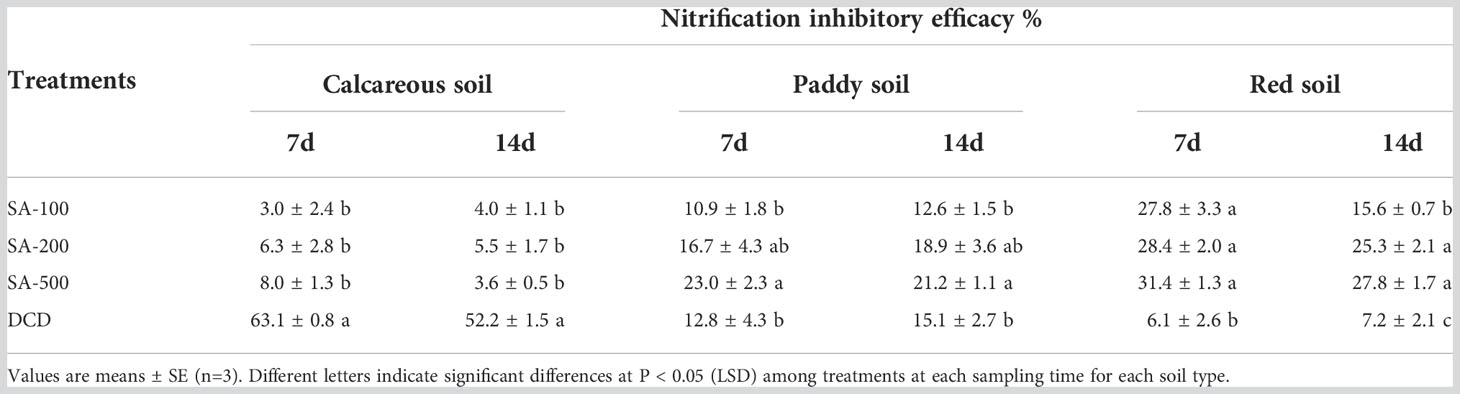
Table 2 Nitrification inhibitory efficacy (%) by SA and DCD treatments among three agricultural soils at day 7 and day 14.
Potential nitrification activity
To obtain more insight into the nitrification inhibitory spectrum of SA, the PNA of soil samples was examined during the incubation process. As can be seen from Figure 2, the PNA in the (NH4)2SO4 control was 2.2, 1.2 and 0.9 mg NO3–-N kg-1 h-1 in the calcareous, paddy and red soil, respectively. All SA treatments showed no significant effect on PNA in the calcareous soil, but a 55% reduction was found in the DCD treatment compared with the (NH4)2SO4 control. In the paddy soil, PNA decreased by 20-49% in soil samples treated with SA and with DCD as compared to the control, but with no significant difference between the SA and DCD treatments. In the red soil, SA treatments showed a lower PNA than in the other two soils, but no significant effect in the DCD treatment could be observed, indicating that the inhibition of SA was superior to DCD in red soil.
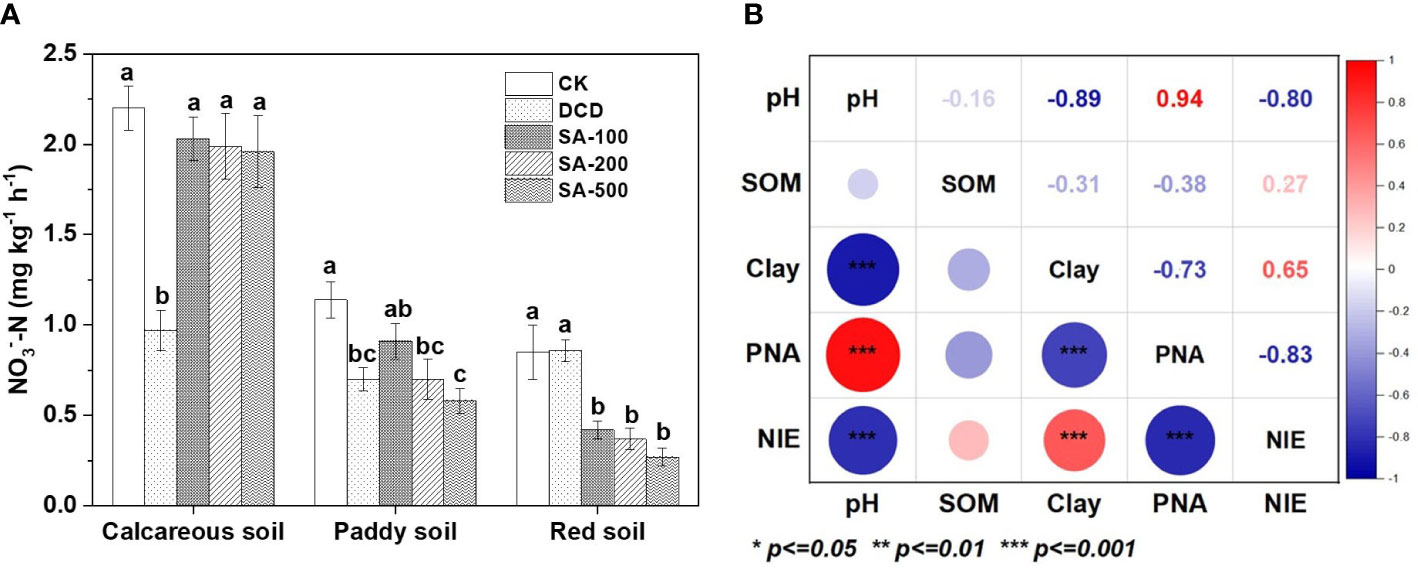
Figure 2 The potential nitrification activity (PNA) affected by different treatments in three soils at the end of incubation (A), and the Pearson correlation test between soil properties (pH, soil organic matter (SOM), and clay percentage), PNA, and nitrification inhibitory efficacy (NIE, %) (B). the Pearson correlation test between soil properties (pH, soil organic matter (SOM), and clay percentage), PNA, and nitrification inhibitory efficacy (NIE, %)(B). Values are means ± SE (n=3). Different letters indicate significant differences at P < 0.05 (LSD test) among treatments at each soil type. The sizes of the circles and the shades of color represent the degree of relevance. The numbers are the correlation coefficients.
Soil PNA had a significantly positive association with soil pH (r = 0.94, P < 0.001), but it had a negative correlation with soil clay percentage (r = -0.73, P < 0.001) and nitrification inhibitory efficacy (%, NIE, r = -0.83, P < 0.001). This negative correlation between NIE and PNA further verifies the nitrification inhibitory function of SA. NIE by SA was shown to be negatively associated with soil pH (r = -0.80, P < 0.001), and positively correlated with clay percentage (r = 0.65, P < 0.001). There was no significant correlation between PNA, NIE, and clay percentage with soil SOM (Figure 2B). This indicates that the differences in inhibition of nitrification by SA among the three soils might be attributed to the physicochemical properties of soil pH and clay percentage.
N2O emissions
The trends for soil N2O emission fluxes varied with soil type and treatment. Emissions in the (NH4)2SO4 control (CK) followed the order: calcareous soil > paddy soil > red soil. For the calcareous soil, N fertilizer addition produced an N2O emission peak of 661.4 μg N d-1 kg-1 soil at day 4 (Figure 3A). DCD strongly inhibited N2O emission, with an 82.2% lower accumulation than that in CK. Although SA treatments delayed the peak time for N2O generation, it didn’t significantly affect the cumulative N2O emissions (Figure 3B). In the paddy soil, the low-, medium and high-dose SA treatments not only reduced the peak N2O value (Figure 3C), but also suppressed the cumulative emission by 69.1%, 69.6% and 79.4% compared to the CK, respectively, which was significantly stronger than DCD’s 46.2% reduction (Figure 3D). Similarly, compared to the CK, low-, medium- and high-dose SA substantially inhibited N2O emission during the entire incubation period in red soil, resulting in a 40.8%, 41.3% and 46.4% reduction in cumulative N2O emissions, respectively, while DCD had no significant effect (Figures 3E, F).
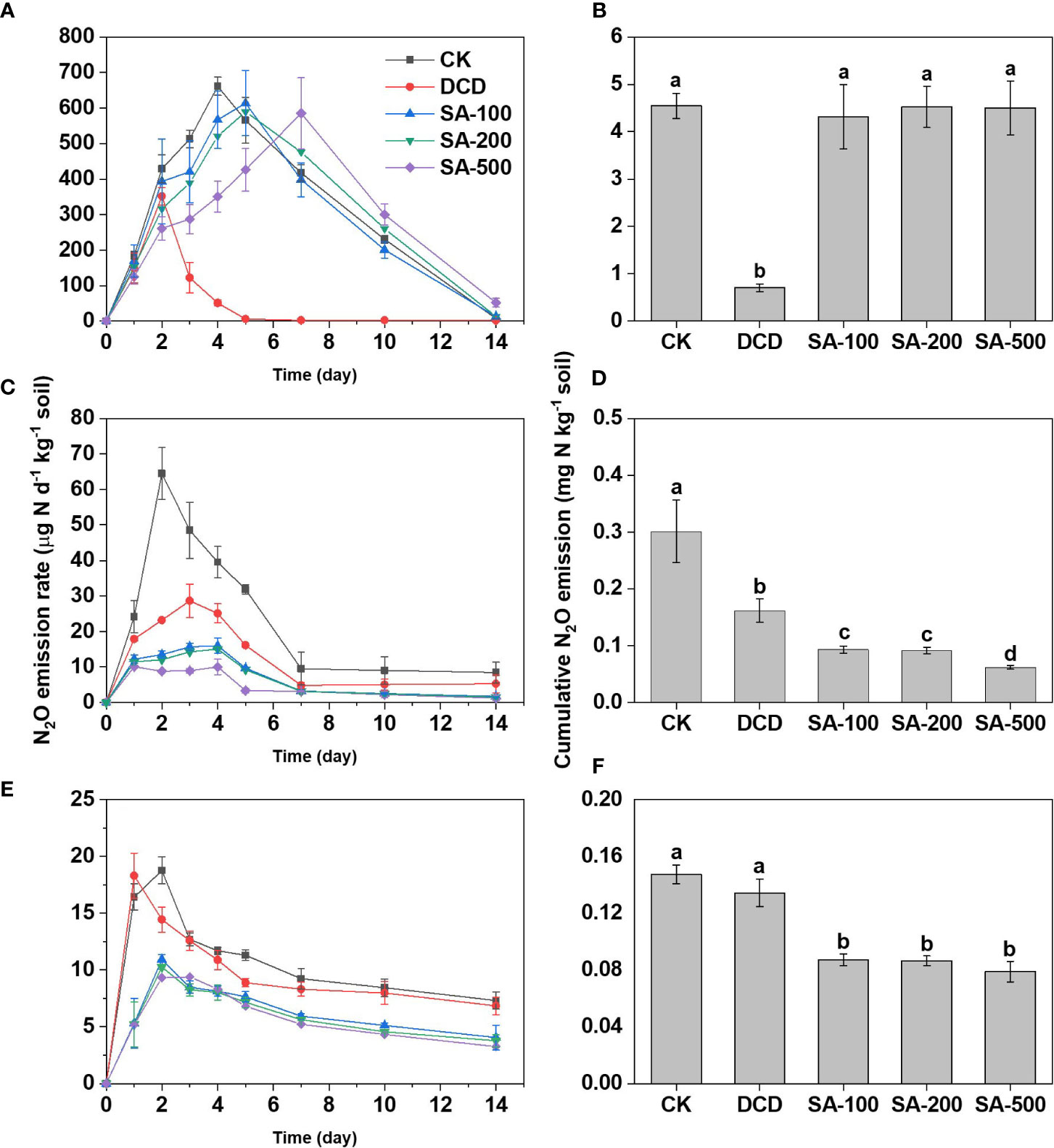
Figure 3 The dynamic change of N2O flux and cumulative emissions in calcareous soil (A, B), paddy soil (C, D), and red soil (E, F) during the 14-d incubation. Values are means ± SE (n=3). Different letters indicate significant differences at P < 0.05 (LSD test) among treatments.
Abundance of AOB and AOA
As compared with the control, no significant inhibition of different doses of SA addition was observed on the abundances of AOA and AOB in the calcareous soil both at day 7 and day 14, with the exception of high-dose SA for AOB at day 14 (Figure 4B). DCD treatment significantly (P < 0.05) decreased the abundance of AOB, by 63.1% and 82.7% at day 7 and 14 compared to the control, respectively, but it showed no significant inhibition on AOA abundance (Figure 4A).
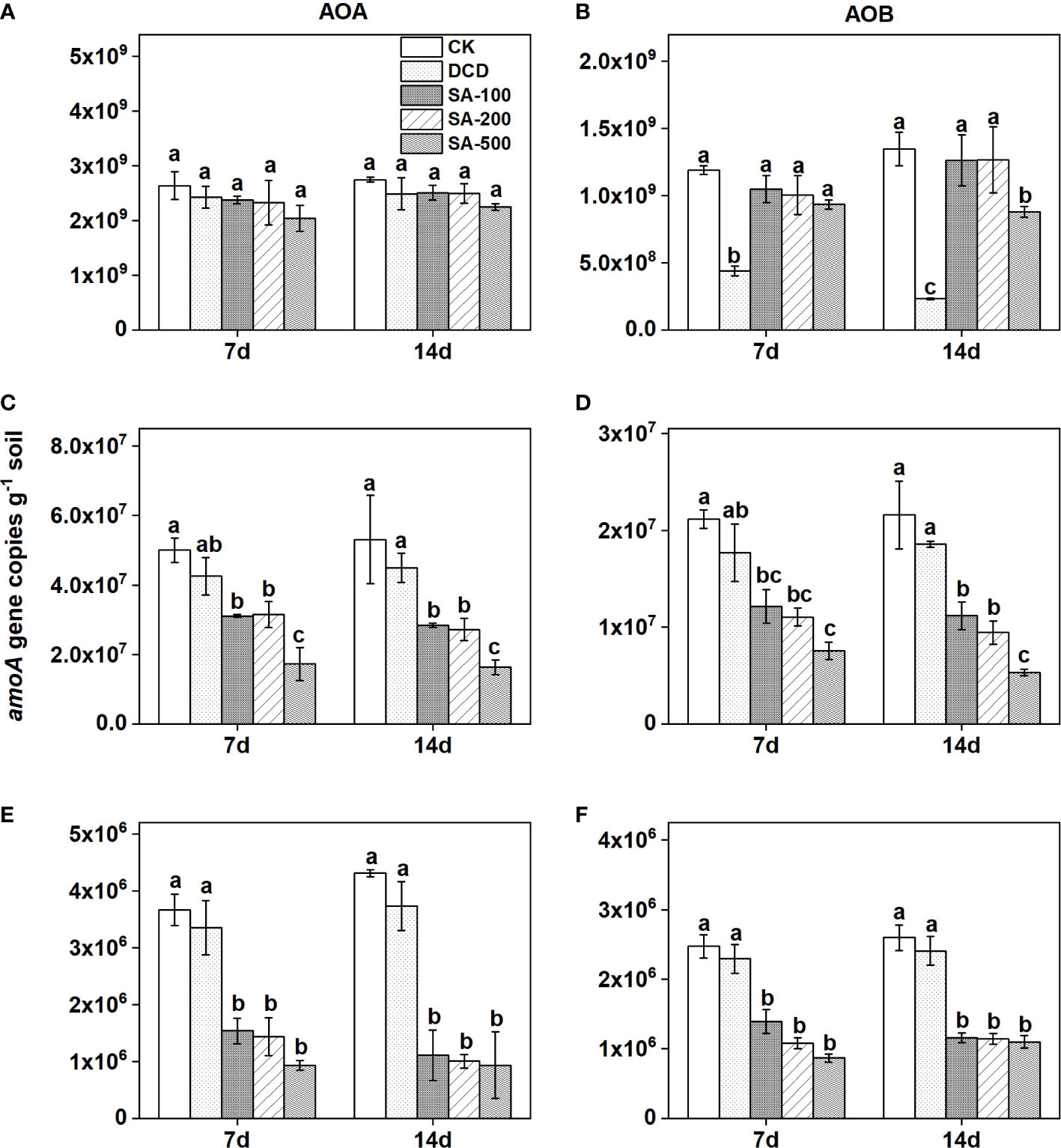
Figure 4 The amoA gene copy numbers of ammonia-oxidizing archaea (AOA) and bacteria (AOB) in calcareous soil (A, B), paddy soil (C, D), and red soil (E, F) under different treatments at day 7 and day 14 days. Values are means ± SE (n=3). Different letters indicate significant differences at P < 0.05 (LSD test) among treatments.
In the paddy soil, AOA abundance significantly (P < 0.05) decreased, by 37.8%, 37.0%, and 65.5% at day 7, and by 46.5%, 48.7%, and 69.2% at day 14 in the presence of low-, medium-, and high-dose SA as compared with the control, respectively, but no significant inhibition of DCD could be found (Figure 4C). The abundance of AOB was also lower, by 16.4% at day 7 and by 14.1% at day 14, in the DCD treatment than in the control, and three SA addition treatments significantly reduced AOB abundance (P < 0.05), by 42.8-64.3% at day 7 and by 48.2-75.3% at day 14, relative to that in the control (Figure 4D).
Similar to the paddy soil, the AOA and AOB abundance were both significantly inhibited by all SA treatments in the red soil (Figures 4E, F). Meanwhile, AOA was shown to be more sensitive to SA than AOB. As compared with the control, the inhibition of AOA and AOB abundance by low-, medium-, and high-dose SA treatments reached 58.0-74.6% and 43.7-64.8% at day 7, and reached 74.3%-78.3% and 55.4%-60.2% at day 14, respectively. However, the amoA gene copies of AOA remained unchanged in the treatment of DCD (Figure 4E).
Significant and positive correlations were observed between AOB abundance and NO3–N contents in red soil (R2 = 0.44, P < 0.05), paddy soil (R2 = 0.54, P < 0.01), and calcareous soil (R2 = 0.68, P < 0.001) at day 14, while AOA abundance was positively associated with soil NO3–-N in paddy soil (R2 = 0.38, P < 0.01) and red soil (R2 = 0.59, P < 0.001), but not in calcareous soil (Figure S1).
The relationships between soil properties, abundance of ammonia oxidizers, and N2O emissions
Structural equation modeling (SEM) was applied to explore the microbial mechanisms underlying the mitigation of N2O emissions by SA in the paddy soil and red soil (Figure 5). The final model explained 75% and 85% of the variation in the N2O emissions in the paddy soil and red soil, respectively. SA application significantly reduced AOA gene abundance (explaining % = 85%, 80%) by altering NH4+ concentration (-0.44***, -0.61***) and DOC content (-0.59***, -0.48***), and reduced AOB (explaining % = 72%, 86%) by changing DOC (-0.84***, -0.50***) in paddy soil and red soil, respectively. Furthermore, the N2O emissions were positively correlated with AOA abundance (0.59***) and with AOB abundance (0.38*) in red soil (Figure 5B). In paddy soil, a positive association was also found between N2O emissions and AOA abundance (0.47*), and AOB abundance (0.46*) (Figure 5A). SEM results indicate that SA application mitigates N2O emissions by directly changing the soil-environmental factors of NH4+ and DOC content, and by indirectly altering AOA and AOB abundance.
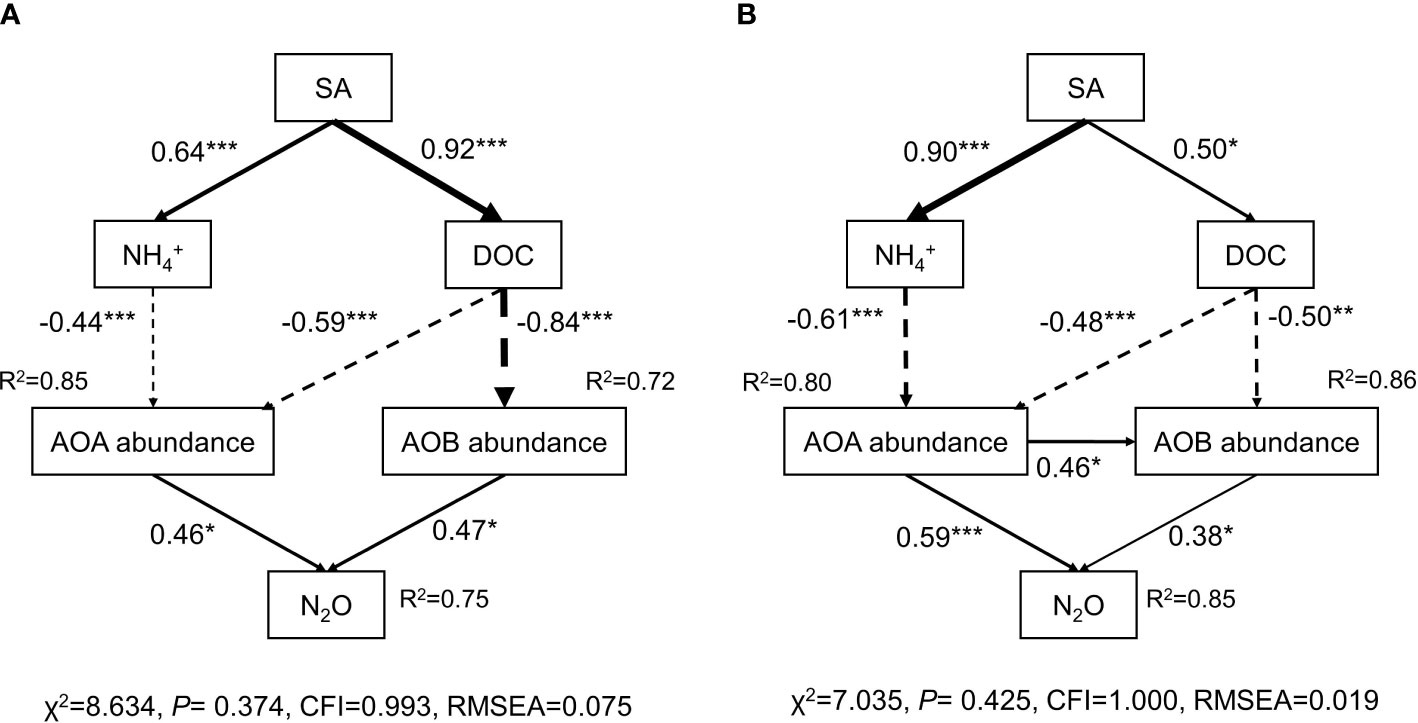
Figure 5 The structural equation model (SEM) explaining the mechanisms driving N2O emission as SA induces changes in soil properties and influences ammonia oxidizer communities in paddy and red soils. The arrow width indicates the strength of the standardized path coefficients. The solid line represents positive effects and the dashed line represents negative effects. Numbers on the arrows indicate significant standardized path coefficients (*p < 0.05; **p < 0.01; ***p < 0.001). R2 indicates the proportion of the variables explained by the factors.
Discussion
Factors influencing SA efficacy of nitrification inhibition
The efficacy of SA is mainly dependent on soil type. The differences among the three soils examined in our study may be explained by the contrasting physico-chemical properties of the soils. One of the most important among these is soil pH. The strongest and most sustained inhibitory effect of SA was found in the acidic red soil, which is consistent with previous results on other BNIs under low pH conditions, such as 1,9-decanediol, MHPP, and LN (Lu et al., 2019; Lan et al., 2022). However, SA lost its inhibitory activity in the alkaline calcareous soil. Interestingly, this pattern is opposite to that of the SNI DCD, which was more effective at suppressing nitrification in alkaline calcareous soil, with no effect in acidic red soil (Table 2). Several other studies have found that the efficacy of the SNIs DCD and DMPP is generally higher under more alkaline conditions (Shi et al., 2016; Bachtsevani et al., 2021; Cui et al., 2021).
These different reactivities of BNIs and SNIs under different pH regimes may be related to their interactions with ammonia oxidizer targets. Soil pH is a critical factor driving the niche partitioning of AOB and AOA (Zhang et al., 2012). Since AOA are the dominant nitrifiers in acidic red soil (Prosser et al., 2020), the strong ability of the BNIs SA and 1,9-decanediol to inhibit AOA and AOB further explains their effectiveness in acidic soil. In contrast, alkaline soils are generally considered as favorable habitats for the growth of AOB (Jia and Conrad, 2009). It is reasonable for the SNI DCD to exhibit superior inhibition in AOB-dominated alkaline soils, owing to the greater sensitivity of AOB than AOA (Shen et al., 2013). A possible reason for the loss of SA inhibition in alkaline calcareous soil is that the para-hydroxy group of SA, flanked by two methoxy groups and key to the nitrification-inhibiting effect, may react with hydroxides under alkaline conditions and become inactive in a phenoxide state (Friedman and Jürgens, 2000; Chethan and Malleshi, 2007). Thus, this leads to no effect of SA on AOB and AOA. Additionally, the degradation rates of SA under alkaline conditions might be also responsible for the disappearance of inhibition of SA, which is worthy of future determination of the SA’s dynamic concentrations in soils.
In addition, soil texture, specifically soil clay, has been shown to play a key role in affecting both efficacy and persistence of nitrification inhibitors (NIs). Generally, the sorption of NIs to the soil clays and immobilization by non-target microorganisms is linked to a decrease in the efficacy of NIs (Barth et al., 2001; Guardia et al., 2018). However, several other studies showed that the effect of clay on NIs efficacy was not only dependent on the clay proportion but also on the clay type, and the affinity of NIs to the clay (Jacinthe and Pichtel, 1992; McGeough et al., 2016). In this study, the inhibitory effect of SA was generally higher in paddy soil and red soil with higher clay content (Figure 2B). This is in good accordance with previous findings on the BNI 1,9-decanediol (Lu et al., 2019) and the SNI nitrapyrin combined with DMPP/DCD in several types of soil (Cui et al., 2021). It may be argued that nitrification and BNI (SA) degradation rates are likely both relatively lower in red (loamy clay) than in calcareous soil (sandy loam), hence leading to greater co-location of SA, NH4+, and ammonia-oxidizing microorganisms in time and space. Although the SNIs DCD and DMPP alone generally show reduced efficacy in soils with high clay and silt contents (Barth et al., 2008; Shi et al., 2016), they may have a stronger inhibitory effect in silt clay and clay soils in long-term incubations (Singh et al., 2008; Cui et al., 2021).
Although previous studies have found that BNIs display higher efficacy in mildly acidic soils (Lu et al., 2019; Subbarao et al., 2021; Lan et al., 2022), the present study shows that BNI efficacy might be negatively correlated with soil pH and positively correlated with soil clay content, which seems to be different from the general observation for commercial SNIs (McGeough et al., 2016; Shi et al., 2016; Bachtsevani et al., 2021; Cui et al., 2021). This finding may help identify the range of possible applications of BNIs in agricultural N management. However, it should be noted that this finding was just limited to three types of soils in China. The involving factors of SA’s inhibition merit validation in other soil types with long-term incubation periods. Continued investigations into the interactions of different soil-environmental factors (eg., factor combination pH value/clay content) in comprehensively determining the degree of inhibition of BNIs are also needed.
Effect of SA on N2O emission and possible mechanisms
This is the first study to examine the effect of the BNI SA, derived from rice roots, on soil N2O emissions. Similar to effects seen with the BNI 1,9-decanediol (Lu et al., 2019), a strong reduction in N2O emission was found upon SA application in both red and paddy soils, and the reduction increased with increase in soil acidity. However, SA had no significant effect on N2O emission in the alkaline calcareous soil, coincident with its limited effect on the dominant AOB populations and in agreement with the inactivation of its para-hydroxyl group under alkaline conditions (Chethan and Malleshi, 2007). In AOA-dominated acidic soils, however, SA can significantly reduce N2O emissions, whereas DCD shows no effect (Figure 4C), underscoring the more dominant role in AOA in producing N2O emissions in acidic soils. Although our 24h closure method cannot rule out the possibility that the available oxygen in the headspace is insufficient, our estimate provides a direct linkage between N2O flux and soil physicochemical properties, and abundance of ammonia oxidizers treated with SA.
It is well established that ammonia oxidizers play a critical role in N2O emissions (Prosser et al., 2020). In the current study, SA reduced N2O emissions from red and paddy soils by both inhibiting AOA and AOB abundance. These dual inhibition on AOA and AOB are consistent with other identified BNIs (Lu et al., 2019; Sarr et al., 2020; Lan et al., 2022). Moreover, the inhibition of AOA by SA in red soil was higher than that of AOB (Figures 4E, F), indicating a higher affinity of SA for AOA than AOB, which is supported by SEM analysis, showing a stronger positive relationship between AOA and N2O than AOB (Figure 5). A previous study highlighted that AOA were more sensitive to the aromatic SNI nitrapyrin than the linear SNIs allylthiourea and DCD (Shen et al., 2013). The chemical structure of nitrification inhibitors may influence the inhibitory mechanism of ammonia monooxygenase, which may be due to the different enzyme’s active site (Wright et al., 2020) and suggests that SA may have a stronger affinity for the enzyme active sites of AOA than AOB, due to its aromatic chemical structure. In addition to the ammonia-oxidizing microorganisms, BNIs and SNIs may possess non-target effects on the rest of the soil microbiota. A recent study by Wang et al. (2021) showed that BNI sorgoleone not only inhibited the growth of a wide range of different bacterial taxa (Flavobacterium, Variovorax, Acinetobacter), but also stimulated the growth of certain taxa (Nocardia and Methylobacillus).
We found that application of the BNI SA significantly altered soil NH4+ and DOC, and subsequently AOA and AOB gene abundance (Figure 5). SEM analysis indicates that AOA abundance, not AOB, decreases significantly under increasing soil NH4+ content due to BNI SA application in paddy and red soils. This is in good agreement with studies where NH4+ substrate concentration was shown to be one of the main factors determining the abundance of AOA (Prosser and Nicol, 2012; Tao et al., 2021b). The AOA microbial community are more active under low-ammonium and other oligotrophic environments (Di et al., 2009; Verhamme et al., 2011), possibly due to higher affinities for ammonia monooxygenase of AOA (Hatzenpichler, 2012). On the contrary, higher NH4+ concentrations may inhibit the AOA abundance and activity (Verhamme et al., 2011). It should also be noted that AOA are enhanced by organic N fertilization and slow-release fertilizers, but not inorganic N fertilizers (Guo et al., 2017; Hink et al., 2018; Prosser et al., 2020). We verify that the NH4+ concentration is the key factor influencing the AOA growth in weakly acidic and acidic soils.
The SEM model further revealed that soil DOC is another factor regulating AOA and AOB abundance, since soil DOC had significantly negative correlation with AOA and AOB abundance, respectively (Figure 5). This is in line with other studies by Song et al. (2016) and Zhang et al. (2021), who found soil DOC content was a key factor altering the growth and community structure of ammonia oxidizer in acidic soils. Although ammonia oxidizers were traditionally believed to be strict autotrophs that are not affected by DOC, members of AOA and AOB that are more versatile could use carbon sources in a heterotrophic mode as well (Schmidt, 2009; Walker et al., 2010). In addition, soil DOC is a readily available substrate for heterotrophic microbes. These heterotrophs might produce antimicrobial compounds to suppress AOA and AOB communities, thereby competing for the ecological niche of ammonia oxidizers and shaping the larger microbial network by competitive exclusion (Jacoby and Kopriva, 2019; Wang et al., 2021). Although the mechanisms by which DOC affects ammonia oxidizers have remained still unclear, our findings show that the effect of the BNI SA on DOC is critical for the changes seen in the abundance of ammonia oxidizers, and subsequently mitigating N2O emissions, in weakly acidic and acidic soils. Since soil pH and clay content have great effects on nitrification (Figure 2B), it is also advisable to further analyze the influence of factor combination pH value/clay content on N2O flux in order to clarify the inhibitory process of SA more comprehensively.
Potential applications of SA
Given the different responses of BNIs and SNIs to soil pH and texture, SA and the previously identified 1,9-decanediol present favorable alternatives to the commercially available SNIs DCD and DMPP, especially in acidic soils, which accounts for thirty percent of the earth’s ice-free lands (von Uexküll and Mutert, 1995). On the international fertilizer market, the commercial SNIs DCD and DMPP have found application in alkaline sandy loam with fast nitrification rates where AOB dominates (Jia and Conrad, 2009; Shi et al., 2016), but there has been a lack of environmentally friendly NIs suitable for acidic soils where AOA play a more significant role (Li et al., 2018; Subbarao et al., 2021). Due to spatiotemporal co-location of NH4+ and ammonia oxidizers, and strong dual inhibition of AOA and AOB, the BNI SA from rice roots has good potential as an application along with N fertilizer in acidic clay soils, if aims are to reduce N loss and alleviate soil acidification (He et al., 2012). In addition to rice and other cereal or vegetable cropping systems, plant-derived SA may also be applied to organic produce of high economic value, such as tea and blueberries, which prefer growth in acidic and NH4+-dominated soils (Britto and Kronzucker, 2002).
Of importance is also the realization that complete inhibition of soil nitrate production in soils may not be a desirable outcome, as full plant adaptation to fully reduced soil N is rare (Kronzucker et al., 1997; Kronzucker et al., 1998) and most crops suffer toxicity on pure NH4+ soil substrates (Britto and Kronzucker, 2002; Britto and Kronzucker, 2013; Li et al., 2019); BNIs, if judiciously applied, will allow for the establishment of mixed-N substrates that will allow for some nitrification to proceed (Kirk and Kronzucker, 2005), favoring plant growth and yield (Kronzucker et al., 1999; Kronzucker et al., 2000; Chen et al., 2021; Subbarao and Searchinger, 2021), while, however, greatly reducing N losses from agro-ecosystems (Coskun et al., 2017a; Coskun et al., 2017b) and the associated harmful environmental effects. Achieving such balance and avoiding the establishment of fully reduced soil environments, currently espoused by some workers in the field (Subbarao and Searchinger, 2021), must be the goal of the design of application protocols of BNIs such as SA.
The limitations of the informative value of the two-week incubation experiments of three soils without plants should be highlighted. In addition, concentrations of 100 to 500 mg SA kg-1 soil used in our incubation experiment are higher than SNIs and may be unacceptable for economic reasons. Therefore, great efforts must be made to reduce its applied amount while increasing the effectiveness of SA, which will contribute to promoting the actual usefulness of SA in agricultural practice. For example, the examination of BNIs synergisms, and combinations with suitable solvents or new materials will need to be taken into account to improve their stability (Lu et al., 2022). In addition to direct exogenous BNIs applications along with N fertilizers, the newly uncovered plant BNI traits could also be feasibly introduced into other crops and forage grasses as a genetic “green” mitigation strategy (Subbarao et al., 2017). By genetically exploiting the capability of crop varieties possessing high SA secretion ability from roots such as the rice genotypes identified in this study, reductions of soil nitrification and N2O emissions may become feasible without additional cost to or difficulties with application logistics for farmers. Deploying BNI-enabled crops could be a powerful nature-based solution to reducing N losses while maintaining yields (Subbarao et al., 2021).
Conclusions
We provide evidence for inhibition of nitrification and N2O emissions by SA derived from rice roots in acidic red soil and weakly acidic paddy soil with relatively low pH and high clay percentage, and show that this may be attributable to a two-pronged inhibition of the growth of AOA and AOB microbes in soil. In contrast, our results show that SA possesses limited inhibition in an alkaline calcareous soil. The present findings reveal that soil NH4+ and DOC content are the key factors leading to the SA inhibition of AOA and AOB abundance, controlling subsequent N2O emissions, in paddy and red soils, and point at the possibilities of the design of novel fertilizer formulations that incorporate SA especially for acidic and AOA-dominated soils. Future studies will need to verify the inhibitory efficacy of SA and the control factors using more soil types in a longer time scale, as well as estimate the effects of SA on plant growth and diverse soil microbiota in the fields.
Data availability statement
The original contributions presented in the study are included in the article/Supplementary Material. Further inquiries can be directed to the corresponding author.
Author contributions
YL and WS designed the experiments and wrote the original draft manuscript. YL, YH, NL, WZ conducted the laboratory analysis. HK, GD and WS critically reviewed and edited the preliminary draft. All authors contributed to the article and approved the submitted version.
Funding
This work was supported by funded by grants from the Strategic Priority Research Program of the Chinese Academy of Sciences (XDA28020301), the National Natural Science Foundation of China (32072670, 32030099), the National Key Research and Development Program of China (2021YFD1700801), the Distinguished Young Scholar Program of Jiangsu Province (BK20190108, BK20200050) and Enterprise Cooperation Projects (Am20210407RD).
Conflict of interest
Author GD was employed by Amway China Botanical R&D Center.
The remaining authors declare that the research was conducted in the absence of any commercial or financial relationships that could be construed as a potential conflict of interest.
The authors declare that this study received funding from Amway China Botanical R&D Center. The funder had the following involvement in the study: reviewing the article.
Publisher’s note
All claims expressed in this article are solely those of the authors and do not necessarily represent those of their affiliated organizations, or those of the publisher, the editors and the reviewers. Any product that may be evaluated in this article, or claim that may be made by its manufacturer, is not guaranteed or endorsed by the publisher.
Supplementary material
The Supplementary Material for this article can be found online at: https://www.frontiersin.org/articles/10.3389/fpls.2022.1099689/full#supplementary-material
References
Bachtsevani, E., Papazlatani, C. V., Rousidou, C., Lampronikou, E., Menkissoglu-Spiroudi, U., Nicol, G. W., et al. (2021). Effects of the nitrification inhibitor 3,4-dimethylpyrazole phosphate (DMPP) on the activity and diversity of the soil microbial community under contrasting soil pH. Biol. Fert. Soils. 57, 1117–1135. doi: 10.1007/s00374-021-01602-z
Barth, G., von Tucher, S., Schmidhalter, U. (2001). Influence of soil parameters on the effect of 3,4-dimethylpyrazole-phosphate as a nitrification inhibitor. Biol. Fert. Soils. 34, 98–102. doi: 10.1007/s003740100382
Barth, G., von Tucher, S., Schmidhalter, U. (2008). Effectiveness of 3,4-dimethylpyrazole phosphate as nitrification inhibitor in soil as influenced by inhibitor concentration, application form, and soil matric potential. Pedosphere 18, 378–385. doi: 10.1016/S1002-0160(08)60028-4
Britto, D. T., Kronzucker, H. J. (2002). NH4+ toxicity in higher plants: A critical review. J. Plant Physiol. 159, 567–584. doi: 10.1078/0176-1617-0774
Britto, D. T., Kronzucker, H. J. (2013). Ecological significance and complexity of n-source preference in plants. Ann. Bot. 6, 957–963. doi: 10.1093/aob/mct157
Byrnes, R. C., Nunez, J., Arenas, L., Rao, I., Trujillo, C., Alvarez, C., et al. (2017). Biological nitrification inhibition by Brachiaria grasses mitigates soil nitrous oxide emissions from bovine urine patches. Soil Biol. Biochem. 107, 156–163. doi: 10.1016/j.soilbio.2016.12.029
Chen, H. F., Zhang, Q., Wang, X. R., Zhang, J. H., Ismail, A. M., Zhang, Z. H. (2021). Nitrogen form-mediated ethylene signal regulates root-to-shoot k+ translocation via NRT1.5. Plant Cell Environ. 44, 3576–3588. doi: 10.1111/pce.14182
Chethan, S., Malleshi, N. G. (2007). Finger millet polyphenols: Optimization of extraction and the effect of pH on their stability. Food Chem. 105, 862–870. doi: 10.1016/j.foodchem.2007.02.012
Coskun, D., Britto, D. T., Shi, W. M., Kronzucker, H. J. (2017a). How plant root exudates shape the nitrogen cycle. Trends Plant Sci. 22, 661–673. doi: 10.1016/j.tplants.2017.05.004
Coskun, D., Britto, D. T., Shi, W. M., Kronzucker, H. J. (2017b). Nitrogen transformations in modern agriculture and the role of biological nitrification inhibition. Nat. Plants. 3, 1–10. 10.1038/nplants.2017.74
Cui, L., Li, D. P., Wu, Z. J., Xue, Y., Xiao, F. R., Zhang, L. L., et al. (2021). Effects of nitrification inhibitors on soil nitrification and ammonia volatilization in three soils with different pH. Agronomy 11, 1674. doi: 10.3390/agronomy11081674
Di, H. J., Cameron, K. C., Shen, J. P., Winefield, C. S., O'Callaghan, M., Bowatte, S., et al. (2009). Nitrification driven by bacteria and not archaea in nitrogen-rich grassland soils. Nat. Geosci. 2, 621–624. doi: 10.1038/ngeo613
Francis, C. A., Roberts, K. J., Beman, J. M., Santoro, A. E., Oakley, B. B. (2005). Ubiquity and diversity of ammonia oxidizing archaea in water columns and sediments of the ocean. Proc. Natl. Acad. Sci. U. S. A. 102, 14683–14688. doi: 10.1073/pnas.0506625102
Friedman, M., Jürgens, H. S. (2000). Effect of pH on the stability of plant phenolic compounds. J. Agric. Food Chem. 48, 2101–2010. doi: 10.1021/jf990489j
Glass, A. D., Britto, D. T., Kaiser, B. N., Kinghorn, J. R., Kronzucker, H. J., Kumar, A., et al. (2002). The regulation of nitrate and ammonium transport systems in plants. J. Exp. Bot. 53, 855–864. doi: 10.1093/jexbot/53.370.855
Guardia, G., Marsden, K. A., Vallejo, A., Jones, D. L., Chadwick, D. R. (2018). Determining the influence of environmental and edaphic factors on the fate of the nitrification inhibitors DCD and DMPP in soil. Sci. Total Environ. 624, 1202–1212. doi: 10.1016/j.scitotenv.2017.12.250
Guo, J. J., Ling, N., Chen, H., Zhu, C., Kong, Y. L., Wang, M., et al. (2017). Distinct drivers of activity, abundance, diversity and composition of ammonia-oxidizers: evidence from a long-term field experiment. Soil Biol. Biochem. 115, 403–414. doi: 10.1016/j.soilbio.2017.09.007
Hart, S. C., Stark, J. M., Davidson, E. A., Firestone, M. K. (1994). Nitrogenmineralization, immobilization. Soil Sci. Soc. Am. 985–1018.
Hatzenpichler, R. (2012). Diversity, physiology, and niche differentiation of ammonia-oxidizing archaea. Appl. Environ. Microbiol. 78, 7501–7510. doi: 10.1128/AEM.01960-12
He, J. Z., Hu, H. W., Zhang, L. M. (2012). Current insights into the autotrophic thaumarchaeal ammonia oxidation in acidic soils. Soil Biol. Biochem. 55, 146–154. doi: 10.1016/j.soilbio.2012.06.006
Hink, L., Gubry-Rangin, C., Nicol, G. W., Prosser, J. I. (2018). The consequences of niche and physiological differentiation of archaeal and bacterial ammonia oxidisers for nitrous oxide emissions. ISME J. 12, 1084–1093. doi: 10.1038/s41396-017-0025-5
Jacinthe, P. A., Pichtel, J. R. (1992). Interaction of nitrapyrin and dicyandiamide with soil humic compounds. Soil Sci. Soc Am. J. 56, 465–470. doi: 10.2136/sssaj1992.03615995005600020021x
Jacoby, R. P., Kopriva, S. (2019). Metabolic niches in the rhizosphere microbiome: new tools and approaches to analyse metabolic mechanisms of plant-microbe nutrient exchange. J. Exp. Bot. 70, 1087–1094. doi: 10.1093/jxb/ery438
Jia, Z., Conrad, R. (2009). Bacteria rather than archaea dominate microbial ammonia oxidation in an agricultural soil. Environ. Microbiol. 11, 1658–1671. doi: 10.1111/j.1462-2920.2009.01891.x
Kirk, G. J. D., Kronzucker, H. J. (2005). The potential for nitrification and nitrate uptake in the rhizospheres of wetland plants: a modelling study. Ann. Bot. 96, 639–646. doi: 10.1093/aob/mci216
Kronzucker, H. J., Kirk, G. J. D., Siddiqi, M. Y., Glass, A. D. M. (1998). Effects of hypoxia on 13NH4+ fluxes in rice roots: Kinetics and compartmental analysis. Plant Physiol. 116, 581–587. doi: 10.1104/pp.116.2.581
Kronzucker, H. J., Siddiqi, M. Y., Glass, A. D. M. (1997). Conifer root discrimination against soil nitrate and the ecology of forest succession. Nature 385, 59–61. doi: 10.1038/385059a0
Kronzucker, H. J., Siddiqi, M. Y., Glass, A. D. M., Kirk, G. J. D. (1999). Nitrate-ammonium synergism in rice: A subcellular analysis. Plant Physiol. 119, 1041–1046. doi: 10.1104/pp.119.3.1041
Kronzucker, H. J., Siddiqi, M. Y., Glass, A. D. M., Kirk, G. J. D. (2000). Comparative kinetic analysis of ammonium and nitrate acquisition by tropical lowland rice: Implications for rice cultivation and yield potential. New Phytol. 145, 471–476. doi: 10.1046/j.1469-8137.2000.00606.x
Laffite, A., Florio, A., Andrianarisoa, K. S., Des Chatelliers, C. C., Schloter-Hai, B., Ndaw, S. M., et al. (2020). Biological inhibition of soil nitrification by forest tree species affects Nitrobacter populations. Environ. Microbiol. 22, 1141–1153. doi: 10.1111/1462-2920.1490
Lan, T., Huang, Y. X., Song, X., Deng, O. P., Zhou, W., Luo, L., et al. (2021). Biological nitrification inhibitor co-application with urease inhibitor or biochar yield different synergistic interaction effects on NH3 volatilization, n leaching, and n use efficiency in a calcareous soil under rice cropping. Environ. pollut. 293, 118499. doi: 10.1016/j.envpol.2021.118499
Lan, T., Li, M. X., He, X. Q., Deng, O. P., Zhou, W., Luo, L., et al. (2022). Effects of synthetic nitrification inhibitor (3,4-dimethylpyrazole phosphate; DMPP) and biological nitrification inhibitor (methyl 3-(4-hydroxyphenyl) propionate; MHPP) on the gross n nitrification rate and ammonia oxidizers in two contrasting soils. Biol. Fertil. Soils. 58, 333–344. doi: 10.1007/s00374-022-01628-x
Li, Y. Y., Chapman, S. J., Nicol, G. W., Yao, H. Y. (2018). Nitrification and nitrifiers in acidic soils. Soil Biol. Biochem. 116, 290–301. doi: 10.1016/j.soilbio.2017.10.023
Li, G. J., Zhang, L., Wang, M., Di, D. W., Kronzucker, H. J., Shi, W. M. (2019). The Arabidopsis AMOT1/EIN3 gene plays an important role in the amelioration of ammonium toxicity. J. Exp. Bot. 70, 1375–1388. doi: 10.1093/jxb/ery457
Lu, Y. F., Zhang, X. N., Jiang, J. F., Kronzucker, H. J., Shen, W. S., Shi, W. M. (2019). Effects of the biological nitrification inhibitor 1,9-decanediol on nitrification and ammonia oxidizers in three agricultural soils. Soil Biol. Biochem. 129, 48–59. doi: 10.1016/j.soilbio.2018.11.008
Lu, Y. F., Zhang, X. N., Ma, M. K., Zu, W. J., Kronzucker, H. J., Shi, W. M. (2022). Syringic acid from rice as a biological nitrification and urease inhibitor and its synergism with 1,9-decanediol. Biol. Fertil. Soils. 58, 277–289. doi: 10.1007/s00374-021-01584-y
Ma, Y., Jones, D. L., Wang, J. Y., Cardenas, L. M., Chadwick, D. R. (2021). Relative efficacy and stability of biological and synthetic nitrification inhibitors in a highly nitrifying soil: Evidence of apparent nitrification inhibition by linoleic acid and linolenic acid. Eur. J. Soil Sci. 72, 2356–2371. doi: 10.1111/ejss.13096
McGeough, K. L., Watson, C. J., Muller, C., Laughlin, R. J., Chadwick, D. R. (2016). Evidence that the efficacy of the nitrification inhibitor dicyandiamide (DCD) is affected by soil properties in UK soils. Soil Biol. Biochem. 94, 222–232. doi: 10.1016/j.soilbio.2015.11.017
Min, J., Sun, H. J., Kronzucker, H. J., Wang, Y., Shi, W. M. (2021b). Comprehensive assessment of the effects of nitrification inhibitor application on reactive nitrogen loss in intensive vegetable production systems. Agric. Ecosyst. Environ. 307, 107227. doi: 10.1016/j.agee.2020.107227
Min, J., Sun, H. J., Wang, Y., Pan, Y. F., Kronzucker, H. J., Zhao, D. Q., et al. (2021a). Mechanical side-deep fertilization mitigates ammonia volatilization and nitrogen runoff and increases profitability in rice production independent of fertilizer type and split ratio. J. Clean Prod. 316, 128370. doi: 10.1016/j.jclepro.2021.128370
Nardi, P., Akutsu, M., Pariasca-Tanaka, J., Wissuwa, M. (2013). Effect of methyl 3-4-hydroxyphenyl propionate, a sorghum root exudate, on n dynamic, potential nitrification activity and abundance of ammonia-oxidizing bacteria and archaea. Plant Soil. 367, 627–637. doi: 10.1007/s11104-012-1494-y
O'Sullivan, C. A., Fillery, I. R. P., Roper, M. M., Richards, R. A. (2016). Identification of several wheat landraces with biological nitrification inhibition capacity. Plant Soil. 404, 61–74. doi: 10.1007/s11104-016-2822-4
Prosser, J. I., Hink, L., Gubry-Rangin, C., Nicol, G. W. (2020). Nitrous oxide production by ammonia oxidizers: Physiological diversity, niche differentiation and potential mitigation strategies. Glob. Change Biol. 26, 103–118. doi: 10.1111/gcb.14877
Prosser, J. I., Nicol, G. W. (2012). Archaeal and bacterial ammonia-oxidisers in soil: the quest for niche specialisation and differentiation. Trends Microbiol. 20, 523–531. doi: 10.1016/j.tim.2012.08.001
Rotthauwe, J. H., Witzel, K. P., Liesack, W. (1997). The ammonia monooxygenase structural gene amoA as a functional marker: molecular fine-scale analysis of natural ammonia-oxidizing populations. Appl. Environ. Microbiol. 63, 4704–4712. doi: 10.1128/aem.63.12.4704-4712.1997
Santoro, A. E., Buchwald, C., Mcllvin, M. R., Casciotti, K. L. (2011). Isotopic signature of N2O produced by marine ammonia-oxidizing archaea. Science 333, 1282–1285. doi: 10.1126/science.1208239
Sarr, P. S., Ando, Y., Nakamura, S., Deshpande, S., Subbarao, G. V. (2020). Sorgoleone release from sorghum roots shapes the composition of nitrifying populations, total bacteria, and archaea and determines the level of nitrification. Biol. Fertil. Soils. 56, 145–166. doi: 10.1007/s00374-019-01405-3
Schmidt, I. (2009). Chemoorganoheterotrophic growth of Nitrosomonas europaea and Nitrosomonas eutropha. Curr. Microbiol. 59, 130–138. doi: 10.1007/s00284-009-9409-8
Shen, T. L., Stieglmeier, M., Dai, J. L., Urich, T., Schleper, C. (2013). Responses of the terrestrial ammonia-oxidizing archaeon ca. Nitrososphaera viennensis and the ammonia-oxidizing bacterium Nitrosospira multiformis to nitrification inhibitors. FEMS Microbiol. Lett. 344, 121–129. doi: 10.1111/1574-6968.12164
Shi, X. Z., Hu, H. W., Muller, C., He, J. Z., Chen, D. L., Suter, H. C. (2016). Effects of the nitrification inhibitor 3,4-dimethylpyrazole phosphate on nitrification and nitrifiers in two contrasting agricultural soils. Appl. Environ. Microbiol. 82, 5236–5248. doi: 10.1128/AEM.01031-16
Singh, J., Saggar, S., Giltrap, D. L., Bolan, N. S. (2008). Decomposition of dicyandiamide (DCD) in three contrasting soils and its effect on nitrous oxide emission, soil respiratory activity, and microbial biomass: An incubation study. Soil Res. 46, 517–525. doi: 10.1071/SR07204
Song, H., Cao, W. C., Huang, T., Wang, J. G., Dong, Z. R. (2016). Changing roles of ammonia-oxidizing bacteria and archaea in a continuously acidifying soil caused by over-fertilization with nitrogen. Environ. Sci. pollut. Res. 23, 11964–11974. doi: 10.1007/s11356-016-6396-8
Subbarao, G. V., Arangob, J., Masahiroc, K., Hooperd, A. M., Yoshihashia, T., Andoa, Y., et al. (2017). Genetic mitigation strategies to tackle agricultural GHG emissions: The case for biological nitrification inhibition technology. Plant Sci. 262, 165–168. doi: 10.1016/j.plantsci.2017.05.004
Subbarao, G. V., Kishii, M., Bozal-Leorri, A., Ortiz-Monasterio, I., Gao, X., Ibba, M. I., et al. (2021). Enlisting wild grass genes to combat nitrification in wheat farming: A nature-based solution. Proc. Natl. Acad. Sci. U.S.A. 118, e2106595118. doi: 10.1073/pnas.2106595118
Subbarao, G. V., Nakahara, K., Hurtado, M. P., Ono, H., Moreta, D. E., Salcedo, A. F., et al. (2009). Evidence for biological nitrification inhibition in Brachiaria pastures. Proc. Natl. Acad. Sci. U.S.A. 106, 17302–17307. doi: 10.1073/pnas.0903694106
Subbarao, G. V., Nakahara, K., Ishikawa, T., Ono, H., Yoshida, M., Yoshihashi, T., et al. (2013). Biological nitrification inhibition (BNI) activity in sorghum and its characterization. Plant Soil. 366, 243–259. doi: 10.1007/s11104-012-1419-9
Subbarao, G. V., Nakahara, K., Ishikawa, T., Yoshihashi, T., Ito, O., Ono, H., et al. (2008). Free fatty acids from the pasture grass Brachiaria humidicola and one of their methyl esters as inhibitors of nitrification. Plant Soil. 313, 89–99. doi: 10.1007/s11104-008-9682-5
Subbarao, G. V., Sahrawat, K. L., Nakahara, K., Ishikawa, T., Kishii, M., Rao, I. M., et al. (2012). Biological nitrification inhibition–a novel strategy to regulate nitrification in agricultural systems. Adv. Agron. 114, 249–302. doi: 10.1016/B978-0-12-394275-3.00001-8
Subbarao, G. V., Searchinger, T. D. (2021). A “more ammonium solution“ to mitigate nitrogen pollution and boost crop yields. Proc. Natl. Acad. Sci. U.S.A. 118, e2107576118. doi: 10.1073/pnas.2107576118
Sun, L., Lu, Y. F., Yu, F. W., Kronzucker, H. J., Shi, W. M. (2016). Biological nitrification inhibition by rice root exudates and its relationship with nitrogen-use efficiency. New Phytol. 212, 646–656. doi: 10.1111/nph.14057
Tao, R., Li, J., Hu, B. W., Chu, G. X. (2021a). Mitigating N2O emission by synthetic inhibitors mixed with urea and cattle manure application via inhibiting ammonia-oxidizing bacteria, but not archaea, in a calcareous soil. Environ. pollut. 273, 116478. doi: 10.1016/j.envpol.2021.116478
Tao, R., Zhao, X. R., Wu, X. L., Hu, B. W., Vanyanbah, K. B., Li, J., et al. (2021b). Nitrapyrin coupled with organic amendment mitigates N2O emissions by inhibiting different ammonia oxidizers in alkaline and acidic soils. Appl. Soil Ecol. 166, 104062. doi: 10.1016/j.apsoil.2021.104062
Verhamme, D. T., Prosser, J. I., Nicol, G. W. (2011). Ammonia concentration determines differential growth of ammonia-oxidising archaea and bacteria in soil microcosms. ISME J. 5, 1067–1071. doi: 10.1038/ismej.2010.191
Villegas, D., Arevalo, A., Nunez, J., Mazabe, J., Subbarao, G., Rao, I., et al. (2020). Biological nitrification inhibition (BNI): Phenotyping of a core germplasm collection of the tropical forage grass Megathyrsus maximus under greenhouse conditions. Front. Plant Sci. 11, 820. doi: 10.3389/fpls.2020.00820
von Uexküll, H. R., Mutert, E. (1995). Global extent, development and economic-impact of acid soils. Plant Soil 171, 1–15. doi: 10.1007/BF00009558
Walker, C. B., de la Torre, J. R., Klotz, M. G., Urakawa, H., Pinela, N., Arp, D. J., et al. (2010). Nitrosopumilus maritimus genome reveals unique mechanisms for nitrification and autotrophy in globally distributed marine crenarchaea. Proc. Natl. Acad. Sci. U.S.A. 107, 8818–8823. doi: 10.1073/pnas.0913533107
Wang, P., Chai, Y. N., Roston, R., Dayan, F. E., Schachtman, D. P. (2021). The sorghum bicolor root exudate sorgoleone shapes bacterial communities and delays network formation. Msystems 6, e00749-20. doi: 10.1128/mSystems.00749-20
Wang, B. Z., Zhao, J., Guo, Z. Y., Ma, J., Xu, H., Jia, Z. J. (2015). Differential contributions of ammonia oxidizers and nitrite oxidizers to nitrification in four paddy soils. ISME J. 9, 1062–1075. doi: 10.1038/ismej.2014.194
Wright, C. L., Schatteman, A., Crombie, A., Murrell, J., Lehtovirta-Morley, L. (2020). Inhibition of ammonia monooxygenase from ammonia-oxidizing archaea by linear and aromatic alkynes. Appl. Environ. Microbiol. 86, e02388–e02319. doi: 10.1128/AEM.02388-19
Yao, Y. L., Zeng, K., Song, Y. Z. (2020). Biological nitrification inhibitor for reducing N2O and NH3 emissions simultaneously under root zone fertilization in a Chinese rice field. Environ. pollut. 264, 114821. doi: 10.1016/j.envpol.2020.114821
Zaman, M., Saggar, S., Blennerhassett, J. D., Singh, J. (2009). Effect of urease and nitrification inhibitors on n transformation, gaseous emissions of ammonia and nitrous oxide, pasture yield and n uptake in grazed pasture system. Soil Biol. Biochem. 41, 1270–1280. doi: 10.1016/j.soilbio.2009.03.011
Zhang, M., Fan, C. H., Li, Q. L., Li, B., Zhu, Y. Y., Xiong, Z. Q. (2015). A 2-yr field assessment of the effects of chemical and biological nitrification inhibitors on nitrous oxide emissions and nitrogen use efficiency in an intensively managed vegetable cropping system. Agric. Ecosyst. Environ. 201, 43–50. doi: 10.1016/j.agee.2014.12.003
Zhang, J. Q., He, P., Liu, Y. H., Du, W., Jing, H. C., Nie, C. (2021). Soil properties and microbial abundance explain variations in N2O fluxes from temperate steppe soil treated with nitrogen and water in inner Mongolia, China. Appl. Soil Ecol. 165, 103984. doi: 10.1016/j.apsoil.2021.103984
Zhang, L. M., Hu, H. W., Shen, J. P., He, J. ,. Z. (2012). Ammonia-oxidizing archaea have more important role than ammonia-oxidizing bacteria in ammonia oxidation of strongly acidic soils. ISME J. 6, 1032–1045. doi: 10.1038/ismej.2011.168
Zhang, X. N., Lu, Y. F., Yang, T., Kronzucker, H. J., Shi, W. M. (2019). Influencing the release of the biological nitrification inhibitor 1,9-decanediol from rice (Oryza sativa l.) roots. Plant Soil 436, 253–265. doi: 10.1007/s11104-019-03933-1
Keywords: biological nitrification inhibitor, syringic acid, ammonia-oxidizing bacteria, ammonia-oxidizing archaea, nitrous oxide
Citation: Lu Y, Hua Y, Lv N, Zu W, Kronzucker HJ, Dong G and Shi W (2022) Syringic acid from rice roots inhibits soil nitrification and N2O emission under red and paddy soils but not a calcareous soil. Front. Plant Sci. 13:1099689. doi: 10.3389/fpls.2022.1099689
Received: 16 November 2022; Accepted: 30 November 2022;
Published: 20 December 2022.
Edited by:
Zhenhua Zhang, Hunan Agricultural University, ChinaReviewed by:
Lin Zhang, China Agricultural University, ChinaXiangbi Chen, Key Laboratory of Agro-ecological Processes in Subtropical Region, Chinese Academy of Sciences (CAS), China
Copyright © 2022 Lu, Hua, Lv, Zu, Kronzucker, Dong and Shi. This is an open-access article distributed under the terms of the Creative Commons Attribution License (CC BY). The use, distribution or reproduction in other forums is permitted, provided the original author(s) and the copyright owner(s) are credited and that the original publication in this journal is cited, in accordance with accepted academic practice. No use, distribution or reproduction is permitted which does not comply with these terms.
*Correspondence: Weiming Shi, d21zaGlAaXNzYXMuYWMuY24=