- 1Department of Agronomy, Faculty of Agriculture and Environment, The Islamia University of Bahawalpur, Bahawalpur, Pakistan
- 2Key Laboratory of Vegetation Restoration and Management of Degraded Ecosystems, South China Botanical Garden, Chinese Academy of Sciences, Guangzhou, China
- 3Center of Plant Ecology, Core Botanical Gardens, Chinese Academy of Sciences, Guangzhou, China
- 4Department of Agronomy, University of Agriculture, Faisalabad, Pakistan
- 5Department of Botany, The Islamia University of Bahawalpur, Bahawalpur, Pakistan
- 6Tasmanian Institute of Agriculture, University of Tasmania, Hobart, TAS, Australia
- 7Department of Agricultural Engineering, Khwaja Fareed University of Engineering and Information Technology (KFUEIT), Rahim Yar Khan, Pakistan
- 8Department of Plant Breeding and Genetics, Ghazi University, Dera Ghazi Khan, Pakistan
- 9Department of Mechanical Engineering, NFC IEFR, Faisalabad, Pakistan
- 10Department of Mathematics, College of Science Al-Zulfi, Majmaah University, Al-Majmaah, Saudi Arabia
- 11Center of Research, Faculty of Engineering, Future University in Egypt, New Cairo, Egypt
In recent decades, environmental pollution with chromium (Cr) has gained significant attention. Although chromium (Cr) can exist in a variety of different oxidation states and is a polyvalent element, only trivalent chromium [Cr(III)] and hexavalent chromium [Cr(VI)] are found frequently in the natural environment. In the current review, we summarize the biogeochemical procedures that regulate Cr(VI) mobilization, accumulation, bioavailability, toxicity in soils, and probable risks to ecosystem are also highlighted. Plants growing in Cr(VI)-contaminated soils show reduced growth and development with lower agricultural production and quality. Furthermore, Cr(VI) exposure causes oxidative stress due to the production of free radicals which modifies plant morpho-physiological and biochemical processes at tissue and cellular levels. However, plants may develop extensive cellular and physiological defensive mechanisms in response to Cr(VI) toxicity to ensure their survival. To cope with Cr(VI) toxicity, plants either avoid absorbing Cr(VI) from the soil or turn on the detoxifying mechanism, which involves producing antioxidants (both enzymatic and non-enzymatic) for scavenging of reactive oxygen species (ROS). Moreover, this review also highlights recent knowledge of remediation approaches i.e., bioremediation/phytoremediation, or remediation by using microbes exogenous use of organic amendments (biochar, manure, and compost), and nano-remediation supplements, which significantly remediate Cr(VI)-contaminated soil/water and lessen possible health and environmental challenges. Future research needs and knowledge gaps are also covered. The review’s observations should aid in the development of creative and useful methods for limiting Cr(VI) bioavailability, toxicity and sustainably managing Cr(VI)-polluted soils/water, by clear understanding of mechanistic basis of Cr(VI) toxicity, signaling pathways, and tolerance mechanisms; hence reducing its hazards to the environment.
1. Introduction
Heavy metal contamination has disastrous impacts on terrestrial as well as aquatic life (Pushkar et al., 2021), and it has significantly disrupted the natural ecosystem (Zulfiqar et al., 2022). The unplanned urban and industrial development that disregards the value of a healthy environment is the main cause of environmental pollution (Dabir et al., 2019; Wei et al., 2022a). These actions have greatly increased the pollution from heavy metals, which upsets the natural balance (Posthuma et al., 2019; Qianqian et al., 2022). More than 1.7 million deaths were reported by World Health Organization (WHO) because of exposure to harmful contaminants, such as heavy metals (World Health Organization (WHO), 2017; Xu et al., 2018). The increase of heavy metal pollution in the environment increases the potential of human exposure to these heavy metals (Zulfiqar et al., 2019). Heavy metals may be harmful to living things due to their biodegradable properties (Qianqian et al., 2022). At different trophic levels, heavy metals frequently bioaccumulate and move within the ecosystem (Pushkar et al., 2021). Untreated trash can contain heavy metals that may leak into irrigation water/groundwater and easily absorbed by plants (Banerjee et al., 2019). Heavy metals can have fatal consequences on living things when they encounter them through water, air, food, etc. (Majumder et al., 2017; Yaashikaa et al., 2019). The degradation of heavy metals is a serious problem that requires immediate action.
In the earth’s mantle, chromium (Cr) is 17th the most plenteous element, and the valence state of Cr regulates its toxicity in plants. Cr is widely used in a various industry, including the Cr plating, tanneries, mining, steel, and chemical industry (Shahid et al., 2017; Pushkar et al., 2021). Cr has become more prevalent as an environmental pollutant due to its increased industrial uses (Pradhan et al., 2019; Wei et al., 2022a). Cr is a pervasive contaminant with significant environmental hazards, particularly for soil-plant ecosystem (Ao et al., 2022; Kapoor et al., 2022). It is a metallic compound that belongs to category VI-B in the periodic table with an atomic number of 24. It is a shiny, hard, and steel-gray mineral with maximum melting point (Owlad et al., 2009). The annual world mine production of Cr in thousand metric tons is mentioned in Figure 1. The trivalent and hexavalent Cr appears being the most persistent among the numerous chromium oxidation states (III to +VI) (Chug et al., 2016). Hexavalent Cr is known to be a dangerous metal relative to the trivalent form because of its carcinogenic, mutagenic, and oxidizing properties (Wei et al., 2022a). Compounds of Cr(VI) are thousand times more cytostatic and carcinogenic than Cr(III) (Mamais et al., 2016). Furthermore, as opposed to further forms, Cr(VI) is highly soluble and bioavailable, obtaining more consideration (Xiao W. et al., 2017). There is no known biological function of Cr in plants (Srivastava et al., 2021). The soil properties, such as soil texture, pH, organic matter (OM) composition, electrical conductivity (EC), sulphide ions, iron (Fe) and manganese (Mn) oxides, microbial activity, and soil moisture content, as well as the plant physiology, such as root surface area, rate of root exudation, rate of transpiration, and plant type all influence the biogeochemical behavior of Cr in soil-plant systems (Shahid et al., 2017; Xiao et al., 2021; Ao et al., 2022). Plants lack specialized transporters and channels for absorbing Cr because it is a non-essential element for them (Adhikari et al., 2020). As a result, certain carriers of the necessary ions for plant metabolism, such as Fe for Cr(III) and phosphate and sulphate for Cr(VI), are used by plants to accumulate Cr (Anjum et al., 2016a). The oxidative stress caused due to Cr toxicity may lead to reduce membrane stability due to the over-accumulation of reactive oxygen species (ROS) that may also damage the morpho-physiological attributes in the plants (Eleftheriou et al., 2015; Azeez et al., 2021). Due to oxidative reactions such as mutilation of DNA and RNA, inhibition of enzymes, lipid peroxidation, and protein oxidation, ROS can induce cell death when produced in high concentrations (Srivastava et al., 2021). The functioning and regulation of many proteins are reportedly suppressed by Cr toxicity (Dotaniya et al., 2014; Handa et al., 2018a), and plant tissues exhibit chromosomal abnormalities as a result (Shahid et al., 2017). Numerous techniques, including solvent extraction, adsorption, chemical reduction, bio-remediation, and others, have been thoroughly investigated and evaluated, to remove hazardous form Cr(VI) to non-toxic Cr(III) form from polluted soil, water, and air (Azeez et al., 2021). Moreover, plants have evolved a variety of sophisticated adaptation methods, such as chelation by organic compounds followed by sequestration within vacuoles, to deal with high amounts of ROS produced under biotic and abiotic challenges (Azeez et al., 2021; Pushkar et al., 2021). To combat the elevated amounts of Cr-mediated ROS, plants also have a secondary mechanism for generating antioxidant enzymes (Srivastava et al., 2021; Ao et al., 2022). Understanding the biogeochemistry of Cr in soil-plant environments and the effects that high levels of Cr will have on the ecosystem is crucial.
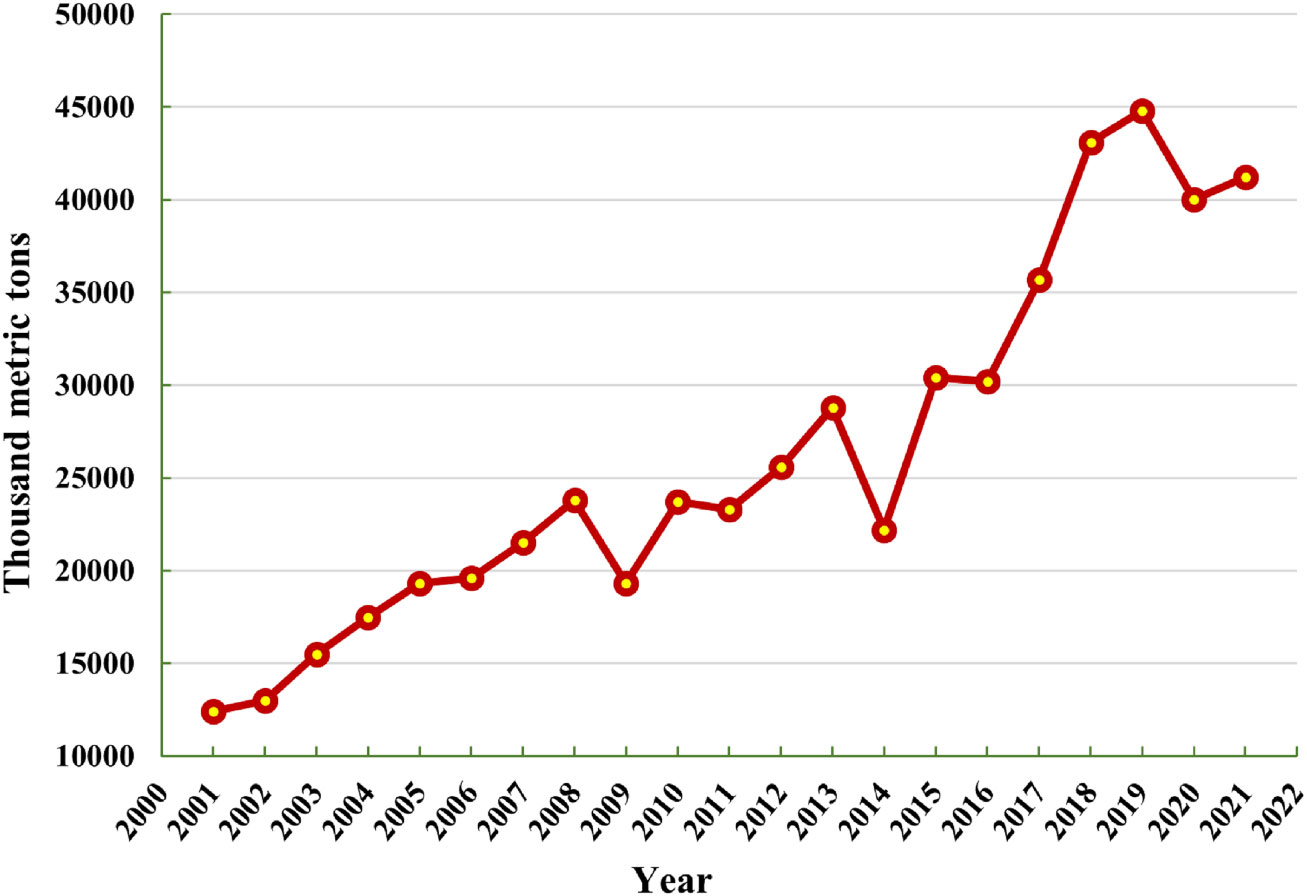
Figure 1 Annual world mine production of Cr in thousand metric tons (source, U.S.G.S. (United States Geological Survey), 2021).
The effects of Cr toxicity on agricultural productivity, lipid peroxidation, ROS production, and potential remediation procedures have been described in a number of previous research (Shanker et al., 2005; Shahid et al., 2017; Azeez et al., 2021; Srivastava et al., 2021; Ao et al., 2022). This review provides an overview of the most recent research on the mechanisms underlying transport of Cr, accumulation, toxicity, and detoxification in soil-plant systems. The toxic effects of Cr on key metabolic functions of plants leading to growth and yield impairment are reported. The mechanisms of Cr(VI) immobilization and reduction by organic amendments i.e., biochar, compost, and organic manure are also discussed Cr(VI). Additionally, in this review the recent remediation techniques are also highlighted, such as bioremediation, which includes phytoremediation, remediation using microbes, and supplements for nano-remediation. These techniques significantly reclaim Cr-contaminated soil and water while reducing potential health and environmental risks. To define future research goals and needs, research gaps in the biogeochemical behavior of Cr in soil-plant systems and difficulties in using in-situ remediation materials for Cr(VI)-contaminated soils are also integrated.
2. Chemical properties of chromium
The element Cr is relatively active. Instead of reacting with water, it reacts with many acids. At room temperature, it reacts with oxygen to create chromium oxide (Cr2O3). A thin layer of chromium oxide coats the metal’s surface, preventing further corrosion (rusting). The atomic number of Cr is 24 and has 51.996 g mol-1 molecular weight. Moreover, the electronegativity of Cr is 1.6, density 7.19 g cm-3 at 20°C, ionic radius 0.061 nm for Cr(III) and 0.044nm for Cr(VI), melting point 1907°C and boiling point is 2672°C. Cr is hard, brittle, and lustrous. It can be highly polished and is a silver-gray color.
3. Sources of chromium in environment
Cr is one of the heavy metals whose concentration is continuously rising because of industrial expansion and combustion processes, particularly the rise of the metal, chemical, and tanning sectors (Sharma et al., 2020). Industrial processes like leather tanning, Cr plating, pigment production, wood preservation, and the use of Cr as a corrosion-inhibitor in cooling towers are examples of anthropogenic sources (Shanker et al., 2005). Natural sources include the leaching of Cr during weathering of ultramafic rocks is another (Ao et al., 2022). Other environmental sources of Cr include power plants using liquid fuels, brown, and hard coal, industrial and municipal trash, and rocks eroded by water and air (Shahid et al., 2017). Cr pollution is not a concern on a global basis, but it may cause excessive concentrations of this pollutant to circulate in the biogeochemical cycle locally due to metal permeability into soil, water, or the atmosphere (Yang et al., 2022).
4. Chromium dynamics in soil
The average soil concentration of Cr is about 40 mg kg-1 (Isak et al., 2013). Cr exhibits a wide range of potential states of oxidation, the +3 state is vigorously persistent; the +3 and +6 forms are frequently seen in Cr groups, while the +1, +4, and +5 states are uncommon. Cr FeCr2O4 chromate, which contains about 70% of pure Cr2O3, is the main mineral possessing this element (Lakshmi and Sundaramoorthy, 2010). Natural Cr exists in most soils as relatively inert forms of Cr(III) that must be liberated over time by acid discharge (Chandra et al., 2010). The manganese (Mn) oxides present in soils will oxidize Cr(III) into Cr(VI), but a minute proportion of Cr(III) in soils is typically found in oxidizable forms (Mishra et al., 2009). Within the soil, Cr is perfectly integrated, however effectively bound to organic materials on Fe and Mn oxides and hydroxides (Balamurugan et al., 2014).
5. Factors affecting chromium dynamics
The disruption of the equilibrium state between species is significantly impacted by several chemical events that Cr conversion can cause in soils, including hydrolysis, oxidation, precipitation, and reduction (Di-Palma et al., 2015). Shift of redox state (Eh), soil pH, cation exchange capacity (CEC), biological conditions, microbial environments, and competitive cations have a significant impact on these complex interactions (Taghipour and Jalali, 2016). Cr speciation is particularly vulnerable to the values of soil Eh (Xiao et al., 2019). The dominant factor influencing soil Eh may be biochemical properties of metals, specifically those with different types of metal oxidation conditions in the soil (Bell et al., 2022). In addition to Cr(III) immobilization and precipitation, altered soil types cause hazardous Cr(VI) to be converted to less harmful Cr(III) (Pakade et al., 2019). Generally, in oxygen-rich conditions, Cr(VI) species dominate and exists as HCrO4-, Cr2O72- and CrO42-; these have higher bioavailability, solubility and propensity for transport (Ball and Izbicki, 2004; Larsen et al., 2016), in an acidic environment, Cr(VI) does have significant Eh (1.38 V), indicating its significant oxidizing propensity (Shadreck, 2013). By influencing its chemical speciation, soil pH significantly influences Cr geochemical activity (Amin and Kassem, 2012). Soil pH determines the chemical form of Cr in soil solution and controls the balance between solubility, adsorption and desorption of Cr in soil (Ertani et al., 2017). A decrease in soil pH causes the mobilization and release of Cr(III), while an increase in soil pH leads to formation of Cr(VI) in soil (Dias-Ferreira et al., 2015). Only at pH 5.5, Cr(III) have quite a poor stability (Kabata-Pendias, 2010). Cr(III) almost fully precipitates above the pH, and thus its compounds are known to be extremely stable in soil. In contrast, Cr(VI) is highly volatile in soil, and is present in acidic and alkaline pH environments (Kabata-Pendias, 2010). Apart from directly affecting the Cr speciation, pH also influences the chemical and mineralogical properties of soil such as CEC surface charge and Eh, thereby regulating the transport, solid phase fractionation and redox behavior of Cr (Xu et al., 2020). Soil Organic matter plays an important role in determination of Cr bioavailability in soil through oxidation/reduction and adsorption/desorption (Ao et al., 2022). It binds metals in soil and performs as a transporter of Cr and several other heavy metals, reflecting soil and deposits as metals and OM storage association (Eckbo et al., 2022). Soil OM controls the Cr bioavailability and speciation through three key mechanisms (adsorption, direct and indirect reduction) (Xia et al., 2019). (1) Soil OM has a higher CEC and can form simple organic molecules and humic substances with Cr ions in soil (Schaumann and Mouvenchery, 2018). (2) Dissolved organic carbon acts as an electron donor for the reduction of Cr(VI) to Cr(III) (Li et al., 2020). (3) Soil OM drives microbial growth and creates reducing conditions that indirectly stimulate the biological reduction of Cr(VI) in the soil (Wang et al., 2019). X-ray absorption near edge structure spectroscopy revealed that increasing soil OM favors the redox transformation of Cr(VI), resulting in prevalence of reduction product Cr(III) (Jardine et al., 2013). Microorganism multiplication in soils with high OM levels creates a lowered state and modifies the soil Eh to decrease harmful Cr(VI) organisms to less harmful. Numerous organic modifications (plant tissue, black carbon, compost, farm-yard manure, and poultry manure) are widely utilized in remedial and soil restoration procedures (Kanchinadham et al., 2015).
6. Cr uptake and translocation in plants
In plants, the mechanism of Cr uptake is yet to be discovered. Cr is a non-essential mineral with no specialized mechanism for absorption and is also reliant on Cr speciation (Adhikari et al., 2020). The contact between roots and soil is the first interaction for uptake of Cr by plants and the uptake by plant rootsis based on plant type and Cr speciation [Cr(III) and Cr(VI)] (Shahid et al., 2017). In addition, soil pH, Cr content, salinity, and the availability of dissolved salts also influence Cr uptake in aqueous media (Babula et al., 2008). Furthermore, studies have shown that the creation of Cr-organic ligand complexes improves Cr absorption in plants (Hao et al., 2022). In various plant species, uptake of Cr takes place via the same carriers as for essential ions for plant metabolism (Ding et al., 2019). In plant species, the oxidation state of the Cr ions, and the concentration of Cr in the growth media influence the distribution and translocation of Cr within plants (Shahid et al., 2017). Plants can take up both Cr(III) and Cr(VI) through epidermal root cells, but there are significant differences in the pathways and efficiency of their entry into cells. Cr(VI) is more easily taken up by plants as compared to Cr(III) due to higher water solubility and higher transmembrane efficiency (Aharchaou et al., 2017). The uptake of Cr(III) is a passive process with no use of energy, most Cr(III) is taken up by roots through the same carriers as for essential elements (Singh et al., 2013). However, the routes of Cr(III) entry into cell are not well established. The uptake of Cr(VI) is an active process and relies on phosphate or sulfate carriers owing to similarity in structure (Ding et al., 2019). Cr mobility in plant roots is low as compared to other heavy metals (Sharma et al., 2020). Thus, the concentration of Cr in the roots can be 100 times more than that of the shoots (Gupta and Sinha, 2006). Cr may be sequestered in the vacuoles of root cells as a protective strategy, resulting in increased Cr accumulation in roots (Mangabeira et al., 2011). As a result of this mechanism, plants have some inherent tolerance to Cr toxicity (Srivastava et al., 2021). Furthermore, Cr translocation from the roots to the aerial shoots is quite limited, and it is highly dependent on the chemical form of Cr within the tissue (Shahid et al., 2017). Cr(VI) is changed to Cr(III) in plant tissues, which tends to adhere to cell walls, preventing Cr from being transported further into plant tissues (Kabata-Pendias and Szteke, 2015).
Cr(III)Cr(VI)The activation of ferric reductase enzymes in roots leads to active transport of Cr(VI) and results in its rapid conversion to Cr(III) (Zayed et al., 1998). This transformed Cr(III) attaches to the cell wall, preventing it from transporting through the various plant tissues (Shanker et al., 2009). Increased MSN1 (a potential yeast transcriptional activator) production resulted in increased Cr and S absorption and tolerance in transgenic tobacco (Nicotiana tabacum) (Shahid et al., 2017). In the transgenic Indian mustard plant, Cr(VI) stress promotes the expression of SHST1 gene, a high affinity sulfate transporter located on the plasma membrane that mediates Cr(VI) uptake by roots (Lindblom et al., 2006). Studies on sulfate transporters confirmed that Sultr1;2 gene knockout in Arabidopsis thaliana inhibits Cr(VI) uptake rate, whereas its over expression in rice significantly increases the Cr(VI) uptake by roots (Xu et al., 2021).
7. Effect of chromium toxicity in plants
Cr may enhance plant development at low concentrations and hinder plant growth at higher concentrations, according to some research, even though there is no concrete proof to substantiate its positive participation in plant metabolism (Ao et al., 2022). In plants, higher concentration of Cr significantly affects various biochemical and morphological parameters i.e., reducing seed germination, plant biomass, photosynthetic efficiency, root damage, and eventually causes plant mortality (Zayed and Terry, 2003; Zaheer et al., 2015; Figure 2; Table 1). Excess amounts of Cr can cause stunted growth of the plant (Faisal and Hasnain, 2005a). Essential nutrients and Cr interaction can disturb the uptake pattern of various essential nutrients calcium (Ca2+) and magnesium (Mg2+) in the plant because of the interaction of Cr with soil (Zupančič et al., 2004). Moreover, agricultural soils with high levels of Cr contamination adversely affect the crop yield (Kanwal et al., 2014; Adrees et al., 2015a). Throughout the growth cycle, plants are sensitive to Cr toxicity, and detailed information about the toxic effect of Cr on morpho-physiological and biochemical parameters and toxicity mechanisms is highlighted below.
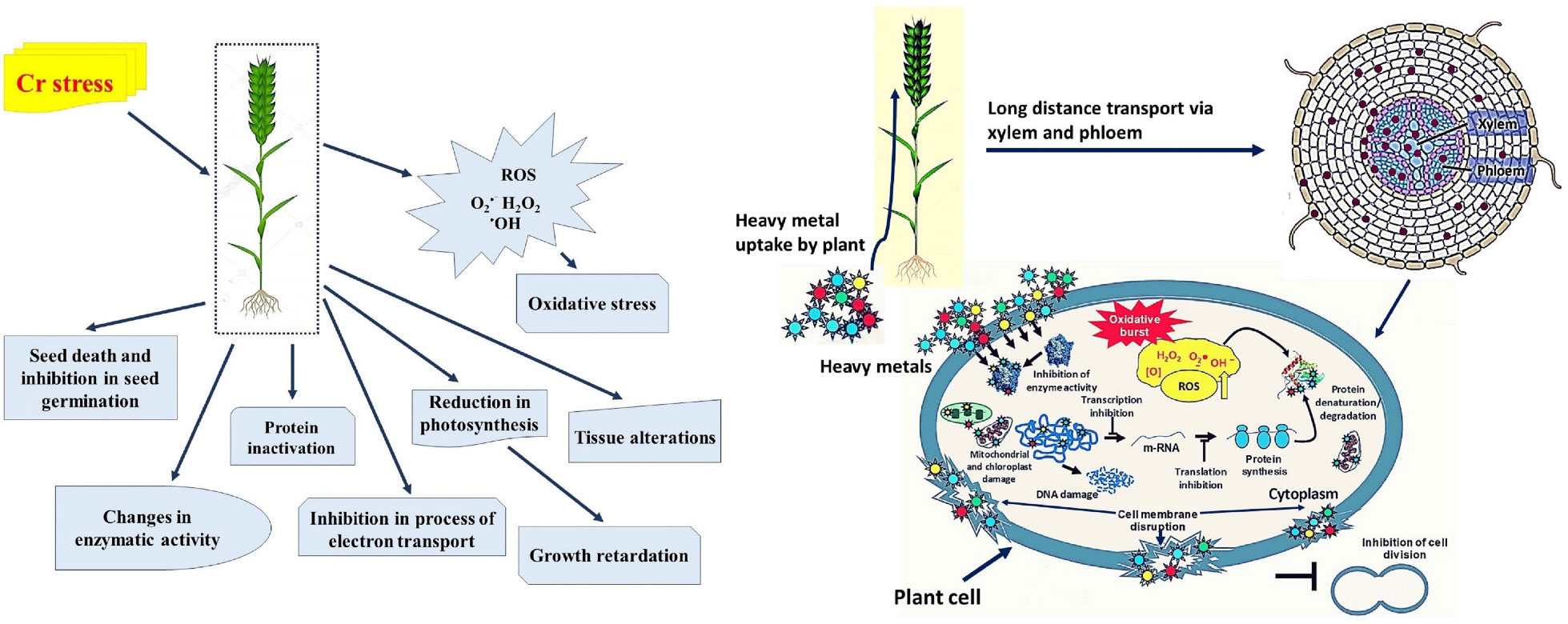
Figure 2 Schematic representation from the sequestration of chromium (Cr) into a plant cell to the plant’s death, through a series of events. Cr toxicity decelerates photosynthesis by preventing seedling establishment and root growth, which in turn slows down essential nutrient and water uptake. Moreover, toxicity of Cr alters photosynthetic pigments content in plant leaves, and these alterations typically result in chlorosis and necrosis of the leaves. In addition, to decreasing membrane integrity, high Cr stress also causes the loss of osmolytes and cell turgor pressure, which causes stomatal closure impacting overall osmoregulation. Additionally, Cr toxicity disrupts the equilibrium between the generation of reactive oxygen species (ROS) and the antioxidant defense system, which causes ROS to build up and cause oxidative damage to cellular organelles. DNA damage, protein and lipid synthesis, lipid peroxidation, enzyme activity, and impaired cell division are all affected by the formation of ROS, which ultimately leads to plants death (Shanker et al., 2005; Rizvi et al., 2020).
7.1. Germination and stand establishment
Considering that seed germination is the first physiological activity that Cr affects, a seed’s capacity to germinate in a medium containing Cr would be an indication of how tolerable it is to this metal (Shanker et al., 2005; Rath and Das, 2021). Symptoms of Cr phytotoxicity comprise the early development of seedling or impediment of seed germination, suppressed root growth, and leaf chlorosis. Cr prominently reduced the seed germination of different plants such as vegetables cauliflower (Brassica oleracea L.), citrullus (Citrullus vulgaris), and crops, wheat (Triticum aestivum L.), barley (Hordeum vulgare L.), and maize (Zea mays L.) (Shahid et al., 2017; Ao et al., 2022). It was noted that higher toxicity of Cr in soil reduced the germination rate of jungle rice (Echinochloa colona), bush bean (Phaseolus vulgaris), alfalfa (Medicago sativa), and sugarcane (Saccharum officinarum) by 25%, 48%, 23%, and 57%, respectively as compared with control (Shanker et al., 2005).
According to several investigations, with an increase in Cr concentration in the external medium i.e., soil/nutrients solution, the DNA content of bean seedlings gradually improved and as a result, the DNA content followed a trajectory that was the opposite of the radical expansion (DalCorso, 2012). Higher concentrations of Cr significantly minimized the bean roots by interfering the cell division process in roots (Zeid, 2001; Singh et al., 2013). During seed germination, accumulated reserve materials like proteins and starch are hydrolyzed to produce precursors like sugars and amino acids for the development of embryo axis as well as substrates for different metabolic processes (Ao et al., 2022). Additionally, when the Cr content gradually increased, the activity of the α- and β-amylases of the developing seeds decreased, which may be responsible for the inhibition of seed germination (Oliveira, 2012). Seed germination of black gram (Vigna mungo) was reduced to 50.70% with the presence of Cr(VI) contents (300 µM) in nutrient solution (Rath and Das, 2021). Singh and Sharma (2017), observed that chickpea (Cicer arietinum) and green bean (Phaseolus aureus) seed germination was decreased by 42.60 and 53.53%, respectively, when Cr was present at higher concentrations (100 mg/L). More than 90% of the 45 tomatoes (Solanum lycopersicum) genotypes displayed reduced and delayed germination within 14 days under 78 mg/L Cr(VI) stress, according to a recent study by Hafiz and Ma (2021).
Higher ROS production from Cr treatment may have facilitated the breakdown of stored nutrients in seeds cotyledon, which ultimately leads to changing the characteristics of cell membranes, hence results in reduced seedling germination (Shafiq et al., 2008; Shah et al., 2010). The significant reduction in seedling length under Cr stress might be due to the reduced water potential and secondary stress-causing obstructed nutrient absorption (John et al., 2009). Because there are fewer meristematic cells in root tips than in cotyledons and shoot apex, Cr treatment also results in diminished seedling growth, particularly of roots (Rath and Das, 2021). The hydrolytic enzymes’ activity is impacted by Cr stress, depriving the radical and plumule of seed and ultimately slowing seedling growth (Stambulska et al., 2018). According to Sundaramoorthy et al. (2009), hexavalent Cr concentration even results in chromosomal abnormalities in the roots of seedling, which stimulate c-mitosis and result in extremely reduced root growth. The amylase activity of seeds under Cr stress may be inhibited, which would lead to a reduction in the transfer of carbohydrates to the germ (Stambulska et al., 2018). Additionally, Cr treatment stimulates protease activity, which results in a lower rate of seed germination or possibly seed death (Khan et al., 2020; Ao et al., 2022).
7.2. Uptake and interaction with other mineral elements
By altering the soil’s nutritional composition and controlling plant nutrient absorption, distribution, and transport, Cr have a significant impact on the metabolism of minerals and causes phytotoxicity in soil-plant systems (Chen et al., 2018). Cr can alter the mineral nutrition of plants in a complex way because of its structural resemblance to some critical elements (Ding et al., 2019). Researchers have focused most of their emphasis on how Cr affects the absorption and accumulation of other inorganic nutrients. Different processes are used by plants to absorb Cr (Ao et al., 2022). Both forms, i.e., Cr(III) and Cr(VI), have the potential to obstruct the uptake of several other ionically related ions, including Fe and S. Both Cr(III) and Cr(VI) have been reported to interfere with macronutrient elements (Ca, K, Mg, N, P, and S) and trace elements (Cu, Fe, Mn, Si, and Zn) through competitive uptake, even though the methods and pathways by which plants absorb Cr(III) and Cr(VI) differ (Ding et al., 2019; Askari et al., 2021; Ashraf et al., 2022a). Complex barriers caused by Cr prevent plants from absorbing essential minerals. According to Shahid et al. (2017), the existence of Cr and critical nutrients in soil and plant cells may be the cause of their antagonistic interactions and competitive absorption. Recent studies reported that excessive Cr toxicity minimizes adsorption sites and forms insoluble/low-bioavailable compounds in rhizosphere soil, which prevents the accumulation of vital nutrients including Ca, Cu, Fe, Mg, P, S, and Zn (De-Oliveira et al., 2016; Chen et al., 2018). The absorption of essential nutrients (such as N, P, K) in paddy irrigation reduced with an elevation in the level of Cr(VI) (Sundaramoorthy et al., 2010). In addition to Cr toxicity, a reduction in Fe content in leaf tissue indicates Cr(VI) involvement in Fe supply, leading to instability in Fe metabolism instability (Gopal et al., 2009).
This reduced uptake of nutrients might be occurred because of the decrease in root development and restriction of root penetration under Cr stress, or because of the reduction in translocation of essential elements (Shahzad et al., 2018; Sharma et al., 2020). Therefore, Cr(VI) competitive binding to common carriers may decrease the absorption of several nutrients. The suppression of plasma membrane H+ ATPase could be a possible explanation for the lower absorption of many of these elements in Cr stressed plants (Shanker et al., 2005). Additionally, the significant Cr buildup in the plant cell wall may harm the plasmodesmata, which serve as crucial channels for the transport of mineral nutrients, resulting in an imbalance in their metabolism (Kitagawa et al., 2015).
7.3. Plant water relations
The detrimental consequences of Cr concentrations cannot be precisely predicted in soil and surface water (Waseem et al., 2014). Plant roots serve the primary purposes of absorbing inorganic and organic nutrients, and water, protecting and anchoring the plant body to the ground, storing nutrients, and promoting vegetative reproduction (Rucińska-Sobkowiak, 2016). These organs typically contain higher Cr concentrations than in the above-ground plant and are known to be the first points of contact with harmful metals like Cr ions (Shanker et al., 2005; Burkhead et al., 2009). Accumulation of Cr ions in tissues may influence soil water absorption and tends to lower the water content in plant roots (Kumar et al., 2016). The direct involvement of Cr ions with the guard cells or the early effects of Cr buildup on plant parts (such as stems and roots) are what induce stomata to close (Ahmed et al., 2016). It is believed that Cr’s effects on water supply in soils, root development, reduced water absorption, and other harmful effects are distinct from its influence on the connection between plants and soil water (Chow et al., 2018). The osmotic ability of soil solution in Cr-enriched soils may be less than that of root cell sap (Vernay et al., 2007). In these circumstances, osmotic pressure, and soil solution will significantly restrict plant water absorption levels (Vernay et al., 2007; Rucińska-Sobkowiak, 2016). When the toxic metal i.e., Cr concentration hits the 10-3 M threshold level, osmotic pressure is thought to exist (Levitt, 1972). Adjustments to endogenous factors, such as root structure and morphology, are more likely to influence plant water absorption indirectly (Kumar et al., 2016). After being exposed to Cr, green amaranth (Amaranthus viridis) showed a substantial decrease in total root area (Sampanpanish et al., 2006). Reduced root hair surface, primary root elongation, increased root dieback, and poor secondary development is abnormalities in Cr-stressed plants that affected how water and plants interacted in the soil (Shanker et al., 2005; Chow et al., 2018). In epidermal and cortical cells of bush bean plants unveiled to Cr, there was impaired turgor and plasmolysis (Vazques et al., 1987). According to Gopal et al. (2009), Cr(VI) inhibits the physiological water supply, as evidenced by a drop in leaf water capability and elevation in diffusional stiffness in spinach leaves, implying that they are growing under water stress. Cr-induced structural changes reduce plant ability to acquire water in the soil and cause insufficient root-soil interaction (Ertani et al., 2017). A broad range of water-related changes is brought about by Cr exposure throughout the entire plant. Reduced water absorption and restriction of short-distance water transport in the apoplast and symplast pathways are effects of Cr toxicity in roots (Srivastava et al., 2021). Additionally, the apoplast’s resistance to water flow is increased by the thickening of the cell wall brought on by Cr ions or other incrusting substances within cell walls (Bhalerao and Sharma, 2015). The inhibition of aquaporin functions and variations in protein expression is most likely to blame for the impaired water transport through the membrane (Ullah et al., 2019a). Such changes affect the flow of water via the vascular system and reduce root sap exudation (Chen et al., 2010). Long-distance water transfer is reluctant, which causes a reduction in leaf water and, as a result, a water deficit in leaves (Shahid et al., 2017). Events that enhance plants’ capacity to retain water include a quick fall in root vacuolization, osmotic ability, and alternation in the tissues of stems and leaves (Srivastava et al., 2021; Ao et al., 2022).
7.4. Plant root and shoot growth
Cr has a significant impact on root growth and development in addition to seed germination (Shahid et al., 2017). The roots, which are a major organ for nutrient uptake and are consequently linked to Cr uptake, act as a major source of Cr toxicity in plants (Srivastava et al., 2021). A considerable reduction in root length of sour orange (Citrus aurantium) seedlings was discovered while conducting an experiment in a greenhouse experiment, under doses of 200 mg/kg Cr(III), (Shiyab, 2019). In water lettuce (Pistia stratiotes), Cr promotes root length, width, and laminal length at low concentrations (0.25 mg L-1) when compared to controls, but at higher concentrations (2.5 mg L-1), the root length was observed to be reduced (Kakkalameli et al., 2018). Similarly, it was observed that Cr toxicity minimized the shoot length of oats (Avena sativa) by 41% as compared to control (Shanker et al., 2005). The growth of lateral roots and the quantity of secondary roots are further effects of Cr (Mallick et al., 2010; Srivastava et al., 2021). Root cell division may have decreased because of the Cr-induced reduction in root length. Cr(VI) prevents plants from absorbing nutrients and water, which shortens roots and reduces cell division (Shahid et al., 2017). Treatment with Cr(VI) in maize (Zea mays) resulted in shorter and fewer root hairs, as well as a brownish color (Mallick et al., 2010). Even various studies claimed that the cell cycle extended when exposed to Cr toxicity (Sundaramoorthy et al., 2010). According to Zou et al. (2006), green amaranth (Amaranthus viridis) root tip cells had their mitotic index reduced because of exposure to Cr.
Another growth metric that is frequently impacted by Cr exposure is plant stem growth (Ding et al., 2019). The shoot length of sunflower (Helianthus annus) was observed to decrease when Cr(VI) content increased (Fozia et al., 2008). Similarly, when the soil’s Cr(III) concentration was raised in sour orange (Citrus aurantium) the shoot length decreased by 90.4% at 200 mg kg-1 of Cr (Shiyab, 2019). After being exposed to 600 mg kg-1 Cr(III), tea (Camellia sinensis) developed a short stem that grew slowly (Tang et al., 2012). According to Lukina et al. (2016), Cr(VI) toxicity (1000 mg kg-1) in 32 species had a negative impact on 94% of the species’ stem growth. The Cr-reduced root growth and development, which results in decreased water and nutrient transfer to the above-ground plant components, may be the cause of the decreased stem growth and height (Srivastava et al., 2021). Additionally, increased Cr transport to shoot tissues may directly interact with delicate plant tissues (leaves) and functions (photosynthesis), affecting shoot cellular metabolism and resulting in a shorter plant (Sharma et al., 2020).
7.5. Oxidative damages
In general, trace metal stress plants by oxidizing them either directly or indirectly by producing reactive oxygen species (ROS) (Qianqian et al., 2022). Cr toxicity causes oxidative damage in plants through overproduction of ROS such as O2-, H2O2, and OH- (Shahid et al., 2017; Basit et al., 2022). The process of reducing Cr(VI) to lower oxidation states is the root cause of Cr toxicity, where only ROS are produced (Shanker et al., 2005; Shahzad et al., 2018). Wakeel et al. (2020) reported that when Cr(VI) is radical reduced, the unstable intermediates i.e., Cr(IV) and Cr(V), which contribute to the generation of ROS, are created. Various plant organelles, such as mitochondria, peroxisomes, and chloroplasts, create these ROS as by-products of diverse metabolic activities (Srivastava et al., 2021). The primary causes of ROS generation in plant organelles i.e., mitochondria and chloroplasts are the inhibition of CO2 fixation and excessive decrease of the electron transport chain (Ao et al., 2022). Furthermore, the production of ROS is caused by the leakage of electrons from O2 caused by electron transport activity in mitochondria, peroxisomes, and chloroplasts (Anjum et al., 2016b). Cr toxicity in plants tends to share electrons, sulfhydryl groups in proteins establish covalent interactions with redox-inactive minerals (Anjum et al., 2017). Numerous studies have been reported showing a dramatic escalation in ROS (Sharma et al., 2019) with an increase in malondialdehyde (MDA) content with Cr toxicity (Adrees et al., 2015b). A pivotal part as signaling pathways molecules and mediators of responses to cellular metabolic disturbance, environmental stimuli, pathogen infection, various developmental stimuli, and a variety of biological and physiological responses are played by plants under normal circumstances when appropriate concentrations of ROS are present (Waszczak et al., 2018). However, the overproduction of ROS in plants results in disruption of cell homeostasis, cell membrane or protein fragmentation, DNA strand breaks, deactivation and degradation of genetic material, and harm to photosynthetic pigments (Srivastava et al., 2021; Ao et al., 2022). Similar findings were observed by Ullah et al. (2019b), who reported that increased ROS generation in plants with Cr toxicity results in oxidative damage, inflicting damage to DNA, lipids, pigments, and proteins, and stimulating the lipid peroxidation functions. These effects inhibit plant growth by preventing cell division or inducing cell death, which lowers biomass production (Wakeel et al., 2020). According to Shahid et al. (2014), the duration of exposure, Cr content, plant species, stage of development, level of stress, and particular organs all affect how hazardous Cr-induced ROS are for plants
7.6. Antioxidant defense system
Complex defense approaches, including non-enzymatic and enzymatic antioxidants, have evolved to prevent oxidative damage to plant cells (Semchuk et al., 2009; Ashraf et al., 2021; Zulfiqar et al., 2021). As with many other metals, excess Cr can promote the development of ROS and generally increases the activity of anti-oxidative enzymes (Table 2). Activities of enzymatic antioxidants such as catalase (CAT), glutathione peroxidase (GPX), ascorbate peroxidase (APX), peroxidase (POD), glutathione reductase (GR), superoxide dismutase (SOD), dehydroascorbate reductase (DHAR), glutathione-S-transferases (GST), monodehydroascorbate reductase (MDHAR), and non-enzymatic antioxidants such as glutathione (reduced form, GSH, and oxidized type, GSSG), ascorbic acid (AsA), and phenolic metabolites were significantly increased under Cr toxicity to minimized the counter effects of ROS production in plant metabolic processes (Shahid et al., 2017; Jan et al., 2020). Cr exposure was found to increase the content of GSH and AsA, while the concentration of phenolic contents was depleted (Panda, 2007). Moreover, non-enzymatic antioxidants that control the levels of ROS in cells, such as tocopherols, carotenoids, GSH, proline, and AsA are regarded as moderators of oxidative damage (Adrees et al., 2015a). Antioxidant capabilities can also be found in other low-molecular-weight substances such as tocopherols, carotenoids, and phenols (Akyol et al., 2020; Ao et al., 2022). However, their antioxidants’ activity and availability are dependent on secondary metabolites’ capacity to synthesize specific compounds, which varies widely among different plant species (Akyol et al., 2020).
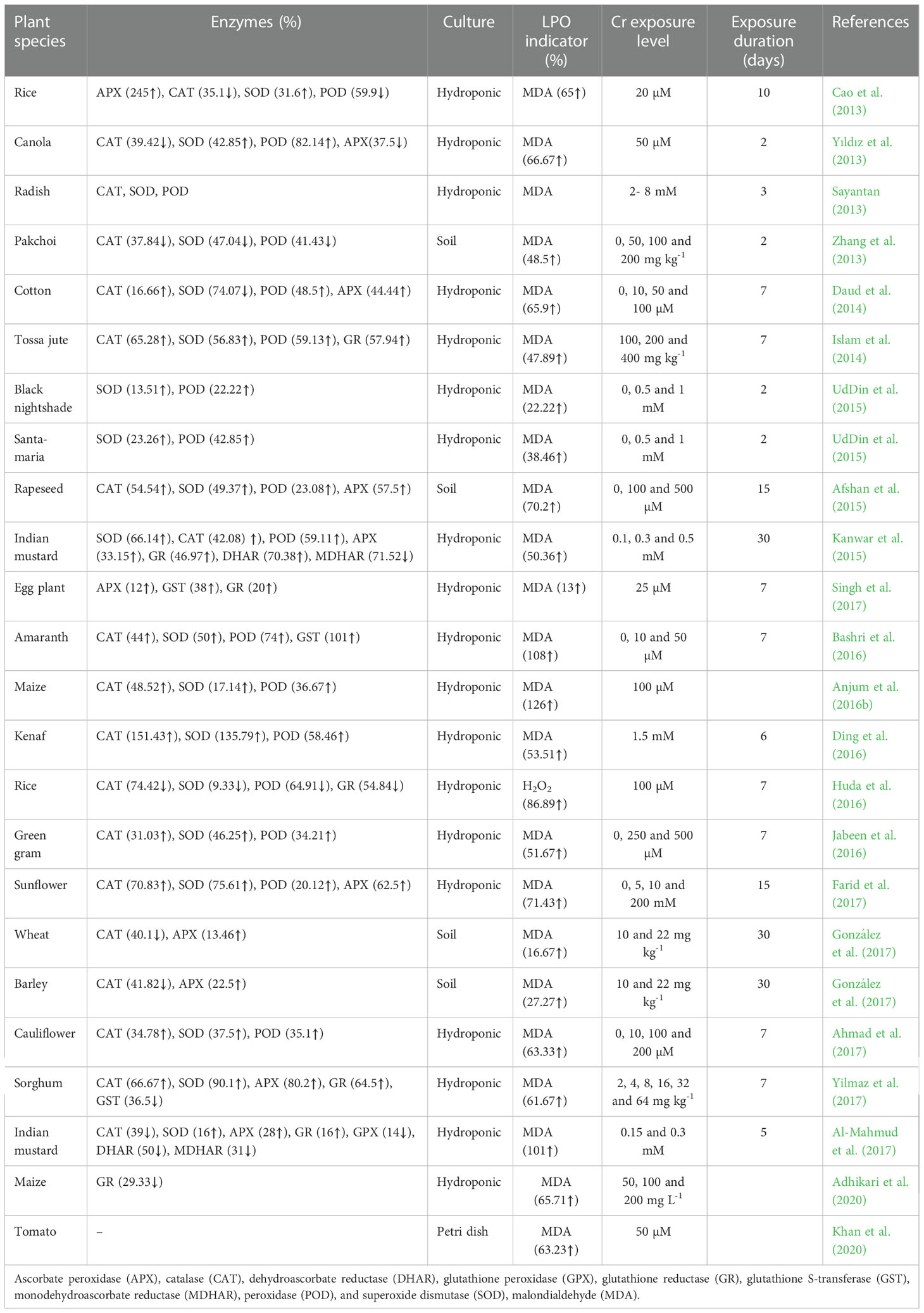
Table 2 Effects of chromium stress on activities of different antioxidant enzymes and lipid peroxidation in different plants.
Plant roots with high levels of Cr(III) content, SOD increased primarily, while the quality of H2O2 displayed a discontinuous pattern for the various Cr(III) absorption, which was assumed because of heterogeneity in the activity of various peroxidases (Kováčik et al., 2013). Plant resistance may have surpassed the innate immune level for high doses of Cr in this case, resulting in the observed declines in enzyme activity. With increasing Cr(III) content, there was an increase in proline content. Usman et al. (2020), reported that giant milkweed (Calotropis procera) treated with Cr(VI) (20 mg L-1) showed enhanced activity of CAT, GR, and SOD with SOD activity being the greatest (up to 12.2 U mg-1). The formation of reducing agents (GSH and AsA metabolites) that catalyze the dismutation of H2O2 to O2- and H2O is aided by the synergistic effects of GR, CAT, and APX and which all play critical roles in scavenging ROS (Bashir et al., 2020). When Cr metal binds to proteins, whether in the catalytic domain or elsewhere, it inhibits enzyme reactants by attaching unique functional groups to proteins, resulting in enzymatic function modifications (Gupta et al., 2010). In addition, from the enzyme, dislocation of essential cations the equilibrium of ROS in cells is disrupted by binding sites, and consequently, ROS is produced in dramatic amounts (Shahzad et al., 2016). The oxidation number of glutathione (GSH) and its constituents appear to bind and utilize Cr metal, which is important for reducing ROS (Lee et al., 2003). In addition, NADPH oxidase contributes to oxidative damage as it is associated with Cr (Pourrut et al., 2013). NADPH oxidases can consume cytosolic NADPH in the existence of Cr metal and generate free radical O2; it is quickly converted to H2O2 through SOD enzyme (Shahid et al., 2017). In the presence of NADPH oxidase, Cr-generated free radicals are external to the plasma membrane, where the pH is generally lower than on the interior side of the membrane (Sagi and Fluhr, 2006). The transporter membrane promotes Cr ingestion and affects the plasma membrane’s ability to produce ROS (Maiti et al., 2012). However, the underlying molecular mechanisms of scavenging ROS by antioxidants and non-enzymatic antioxidants are yet unknown and need more research.
7.7. Photosynthetic activity and yield formation
Phytotoxicity of Cr adversely affects various metabolic processes i.e., CO2 fixations, electron transfer, photophosphorylation, and enzyme concentration, which directly impairs photosynthesis (Anjum et al., 2017; Sharma et al., 2020; Ashraf et al., 2022b). Taken to be critical indices that measure plant photosynthesis under Cr stress are photosynthetic rate, photosynthetic pigments, and photochemical efficiency (Ma et al., 2016). Cr is a potent inhibitor of plant photosynthesis, according to numerous studies (Shanker et al., 2005; Shahzad et al., 2016; Bashir et al., 2020). According to Mathur et al. (2016), Cr toxicity prevents CO2 fixation, electron transfer, enzyme activity, and photophosphorylation in plants. This destroys the photosynthetic apparatus, specifically light-harvesting complex II, PSI, and PSII, and prevents the production of Calvin cycle enzymes (responsible for ATP production) (Sinha et al., 2018). In a study, Anjum et al. (2016a) found that maize plants exposed to Cr stress had significantly lower the levels of net photosynthesis, chlorophyll contents, gas exchange capacity, transpiration rate, water use efficiency, and stomatal conductance. The degradation of photosynthetic pigments caused by exposure to the high concentration of Cr leads to reduction in light-harvesting capacity (Handa et al., 2018b; Srivastava et al., 2021). Net photosynthetic rate (Pn) and chlorophyll content in wheat (Triticum aestivum) were decreased as Cr exposure period gradually increased (Srivastava et al., 2021). Cr prevents mitochondrial electron transport in higher plants, which increases the production of ROS and causes chloroplast modifications, pigment changes, and oxidative stress (Sharma et al., 2016). One of the crucial plant parts involved in photosynthesis is the leaf and total leaf area (Srivastava et al., 2021). In rice (Oryza sativa) the Cr(VI) toxicity reduced the number of leaves per plant by 50% while significantly affecting the overall leaf area and photosynthesis activity of plant (Sundaramoorthy et al., 2010). Under 3.4 mM Cr(VI) toxicity in nutritional media, smooth mesquite (Prosopis laevigatar) was shown to have fewer leaves that significantly affect the chlorophyll content and photosynthesis activity of plant (Buendía-González et al., 2010). Furthermore, it was shown that Cr toxicity significantly decreased the leaf’s net photosynthetic rate, transpiration rate, stomatal conductance, and intercellular CO2 concentration, of sunflower with reductions of 36%, 71%, 57%, and 25%, respectively (Sharma et al., 2020).The first requirement for large plant yields is high plant biomass (Shahid et al., 2017). Cr is known to have negative impacts on several physiological and metabolic processes, which compromises plant production and yield equally (Ali et al., 2015). Various studies highlighted that Cr phototoxicity results to minimize plant biomass and yield of melon (Cucumis melo) (Akinci and Akinci, 2010), wheat (Triticum aestivum) (Adrees et al., 2015a), french bean (Phaseolus vulgaris) (Sharma et al., 2016), okra (Hibiscus esculentus) (Amin et al., 2013), turnip mustard (Brassica campestris) (Qing et al., 2015), Arabidopsis (Arabidopsis thaliana) (Ding et al., 2019), common duckweed (Lemna minor) (Reale et al., 2016), wheat (Triticum aestivum) (Ali et al., 2015), barley (Hordeum vulgare) (Ali S. et al., 2013) maize (Zea mays) (Anjum et al., 2017), cotton (Gossypium hirsutum) (Farooq et al., 2016), makoi (Solanum nigrum) (UdDin et al., 2015). In plants, higher concentration of Cr significantly affects various biochemical and morphological parameters i.e., minimized nutrient and water uptake, reduction in cell division, nutrients imbalance (translocation and uptake), the inefficiency of inorganic nutrient uptake by plant, higher oxidative stress, and ROS formation, oxidative stress damage to sensitive cell organelles such as chlorophyll, mitochondria, lipids, proteins, and reduction in photosynthesis activity that results to minimize the growth, biomass, yield of plant (Shanker et al., 2005; Shahid et al., 2017; Ao et al., 2022). At the cellular, molecular, organ, and plant levels, each of these elements, alone or in combination, have an impact on plant growth, development, and yield (Shahid et al., 2017). However, the type of plant and chemical speciation of Cr will determine which of these factors will be more severely impacted. The impact of Cr on plant development, however, differs depending on the variety of plants. In general, transgenic and hyperaccumulator plants have a lot of potential for Cr tolerance and selective accumulation (Sarangi et al., 2009).
7.8. Enzymatic activity
Cr stress can stimulate potentially three forms of metabolic changes in plants: (i) modification in the synthesis of organic pigments facilitates the growth and development of plants (e.g., anthocyanin, and chlorophyll (Shanker et al., 2005; Shahid et al., 2017); (ii) enhanced the synthesis of metabolites (e.g., ascorbic acid, and glutathione) as a direct reaction to Cr stress that will affect the plants (Srivastava et al., 2021); and (iii) modifications in the metabolic-pool to channelize the synthesis of new biochemically associated metabolites that will confer tolerance or resistance to Cr stress (e.g., histidine and phytochelatins) (Shanker et al., 2005; Ao et al., 2022). Initially at germination stage, toxicity of Cr significantly reduced the activity of gibberellin (GA) and enhanced the activity of abscisic acid (ABA) (major factor of seed dormancy), which lead to seed imbibition and reduced germination rate (Seneviratne et al., 2019). Similarly, according to Yan et al. (2014) hydrolyzing enzymes secreted by the aleurone layer of seeds are crucial for seed germination. By releasing food reserves from the endosperm, enzymes i.e., acid phosphatases (ACPs), α-amylases, and proteases promote effective seedling establishment and growth (see section 5.1). Acid phosphatase, α-amylase, and alkaline phosphatase activity were decreased in the endosperm of cereals i.e., wheat, oat, barley, and maize seeds when Cr was present (Seneviratne et al., 2019). In addition, the enzymes involved in the assimilation of important nutrient nitrogen i.e., nitrogenase, nitrate reductase, nitrite reductase, glutamine synthetase, glutamate synthase, glutamate dehydrogenase were significantly reduced with the contamination of Cr in plants (Sangwan et al., 2014). Deficiency of nutrients in plants due to Cr toxicity results into degradation of various amines, alkaloids, pigments, vitamins, coenzymes, nucleic acids, and nucleotides as nutrients are structural component of these organelles (Shanker et al., 2005; Sangwan et al., 2014). Similarly, the activities of enzymes involved in photosynthesis NADP-malic enzyme (NADP-ME), pyruvate, phosphate dikinase (PPDK), and Phosphoenolpyruvate carboxylase (PEPC), plant respiration i.e., α-ketoglutarate dehydrogenase and isocitrate dehydrogenase, and gene transcription i.e., RNA polymerase are significantly reduced in various plants due to phototoxicity of Cr.
8. Remediation of Cr contaminated soils
The concentration of metals in polluted soils is affected by multiple chemical and biological attributes (Alengebawy et al., 2021). Soils preserve heavy metals by adsorbent, crystallization, and chelation; nevertheless, such interactions restrict their mobility and bioavailability (Yan et al., 2020). However, the implementation of chemical processes, such as organic and inorganic modifications in field can complement this natural attenuation process (Mench et al., 2006; Shahid et al., 2017). These technologies generally minimized the availability of Cr, boost the fertility of the soil, and increase plant growth (Gavrilescu, 2022). Organic amendments (compost) possess a significant proportion of humified organic material and may restrict the availability of Cr in the soil, even though they allow vegetation to be regenerated (Lwin et al., 2018). On the other hand, phosphate fertilizers are useful in metal inactivation through the creation of stable mineral phosphate within the inorganic amendments (Ahmad et al., 2019). Biological options, particularly phytoremediation, have been considered reliable, ecologically acceptable, and cost-effective replacement to physicochemical approaches for the restoration of depleted environments. Various physicochemical activities that can be used to eliminate Cr-polluted environments include ionization, precipitation, reverse osmosis, evaporation, and chemical reduction (Roy and Bharadvaja, 2021). Moreover, there are numerous issues linked with these processes, like permeate flux, inflated prices, high energy consumption, and low extraction efficiency shows that these are less significant in industry. In general, the main considerations in choosing an acceptable treatment to eliminate metals are technological applicability, eco-friendly, and cost-effectiveness (Acheampong et al., 2010).
8.1. Phytoremediation
Phytoremediation is a process in which plants are used for remediation of polluted soils and considered an eco-friendly and green approach (Ali H. et al., 2013; Srivastava et al., 2021). There are various strategies associated with phytoremediation techniques including phytoextraction, rhizofiltration, phytovolatilization, biotransformation, rhizdegradation, phytostabilization, and phytorestoration (Yan et al., 2020). Phytoextraction is focused on the ‘hyperaccumulation’ process, and phytostabilization is focused on the surface complexation mechanism and both are involved in metal affinity phenomena (Xu et al., 2012). Phytoextraction and phytostabilization are two of those practically and economically viable solutions for treating metal-polluted soils (Kuiper et al., 2004). Biotransformation is another term for phyto-transformation. That is the separation of pollutants absorbed by plants via internal metabolic pathways or the segmentation of pollutants just outside of the plant because of plant-generated chemicals (such as enzymes). Plant absorption and metabolism are the primary components, which result in plant deterioration. The uptake of contaminants by plant roots and its conversion to a gaseous state, and release into the atmosphere is referred as phytovolatilization. Volatilization through leaves (ITRC, 2009) is the phytovolatilization process. Degradation by plant rhizospheric microorganisms is the method referred as rhizodegradation (Mench et al., 2009). This ecologically accepted technology is successfully used to fix soils that are polluted by various contaminants. Furthermore, phytoremediation is increasingly used as a technical alternative to treat contaminated water in various forms of wetland treatment (Zhang et al., 2010). In crux, phytoremediation is a feasible, socially, and economically suitable, and eco-friendly solution for the soils polluted with Cr. Nonetheless, to counteract the health risks due to Cr concentration in edible parts of food crops, the proportion of Cr in edible parts of food crops should be closely scrutinized.
8.2. Microbe-assisted remediation
Several methods of metal remediation have been used to address the harmful impacts of metal contamination, including physical, chemical, and biological processes, to inactive specific hazardous metals from the atmosphere (Marques et al., 2011). Microbial remediation has gained significant attention among different biological remediation methods because of its cost-effectiveness, higher efficacy, and non-expendable technologies (Malaviya and Singh, 2014; Fernandez et al., 2018). Some of the microbes that tolerate Cr establish ability to minimize the toxicity of Cr(VI) concentration from the atmosphere and thus play a prominent role in the remediation of Cr(VI) (Table 3; Figure 3). Many investigations on the collection and profiling of distinct Cr-lowering microbial strains of bacteria have been published in last few years (Pseudomonas spp., Bacillus spp., Enterobacter spp., Acinetobacter spp,.), fungi (Aspergillus spp., Penicillium spp., Rhizopus spp.), and yeast (Candida spp., Saccharomyces spp.) (Chen et al., 2016).
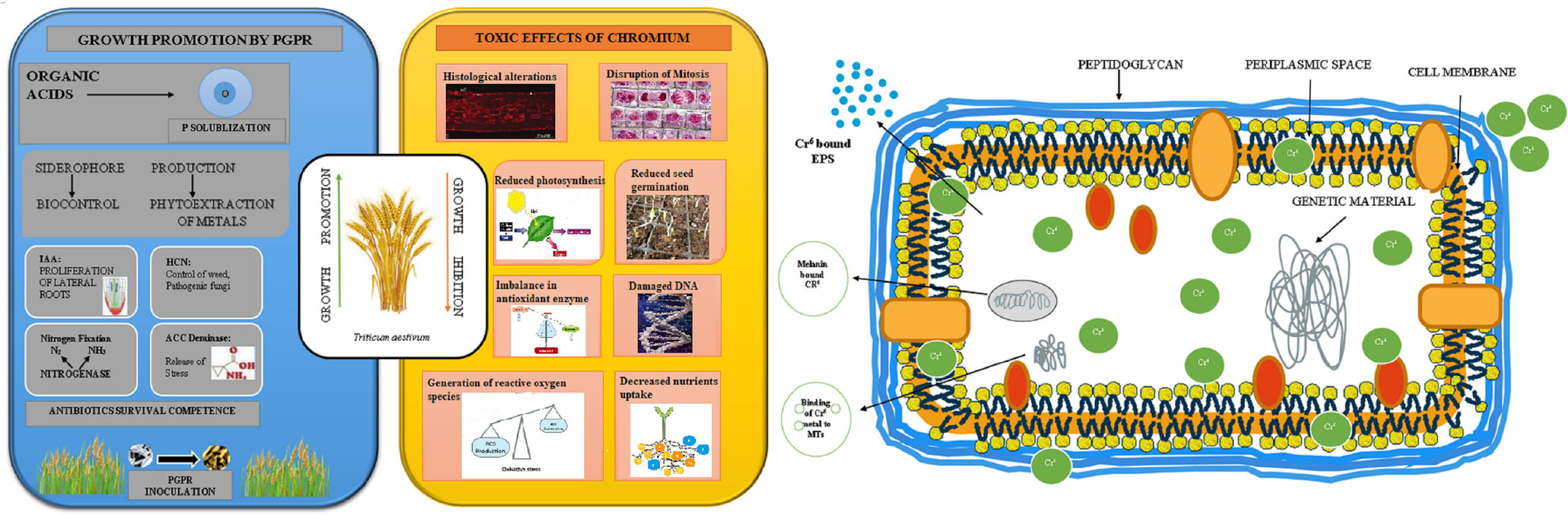
Figure 3 A schematic illustrating how rhizobacteria that encourage plant growth might boost growth and reduce the damaging effects of chromium (Cr) on plant. The removal/detoxification of Cr ions by active biomolecules i.e., secretion of melanin, metallothionein (MTs), and polymeric substances (EPS), released by rhizobacteria strains under Cr stress (Rizvi et al., 2020; Ao et al., 2022).
The use of plant growth-promoting bacteria (PGPB) in plants, is also regarded as a significant and environmentally acceptable method for the removal of heavy metals from soil (Fahad et al., 2014). These bacteria encourage plants to endure extreme stress and improve plant nutrition to stimulate plant growth (N, P, Fe) and release different metabolites related to stress, such as the production of phytohormones, solubilization of phosphates, and production of siderophores (Dodd and Perez-Alfocea, 2012). Several studies have documented the use of plant growth promotion (PGP) rhizobacteria for heavy metal bioremediation, like Bacillus sp., Pseudomonas sp., etc. (Ndeddy-Aka and Babalola, 2016). Microorganisms have been found to reduce hexavalent Cr through various means, either by using hexavalent Cr as the final acceptor of electrons or by releasing some dissolving enzymes (Table 4; Ahemad, 2015). In an experiment, Karthik and Arulselvi (2017) evaluate the effect of Cr(VI) on the plant growth-promoting properties of potential rhizobacterial strain isolated from the rhizosphere of a common bean (Phaseolus vulgaris). The strain AR8 was chosen from 36 rhizobacterial strains when compared to uninoculated Cr(VI) treated plants, the inoculation of Cellulosimicrobium funkei strain AR8 significantly improved the root length of test crops in both the presence and absence of Cr(VI). Strain AR8 could be used for growth stimulation as well as for the removal of Cr in Cr-contaminated soil because of these exceptional characteristics (Figure 3).
According to Caravelli et al. (2008) the Sphaerotilus natans CSCr-3, a filamentous bacterium obtained from activated sludge, reduced Cr concentration up to 1.5 mM in the presence of a carbon source. This removal efficiency is significant because S. natans was originally recognized for its biosorption capability. Under alkaline medium, another bacterium, Ochrobactrum sp., was able to decrease Cr(VI). This isolate substantially tolerated and reduced Cr(VI) up to 15.4 mM. The inclusion of glucose generated a significant improvement in Cr(VI)-reduction, while the availability of sulphate or nitrate had no effect (He et al., 2009). Five Cr resistant bacterial strains with auxin biosynthesis abilities were used by Arshad and Ahmed (2017). Halomonas venusta APA and Arthrobacter mysorens AHA were determined to be the most effective isolates in terms of phytostimulatory effects on green gram (Vigna radiata). A huge proportion of microbial variants have been recorded for remediation of Cr(VI) using biosorption and bioaccumulation methods, such as Paecilomyces lilacinus (Sharma and Adholeya, 2011), Aspergillus niger (Srivastava and Thakur, 2006a, b), Bacillus cereus IST105 (Naik et al., 2012), Zobellella denitrificans (He et al., 2016), and Bacillus mycoides 200AsB1 (Wang et al., 2016). In conclusion, the use of a suitable microbial inoculum might become useful in effectively altering the soil infected with Cr.
8.3. Chemical remediation
In-situ or ex-situ complex formation through chelating substances has been used for metal extraction (Di-Palma et al., 2005; Finzgar and Lestan, 2007). The efficacy of extraction depends upon the availability of readily exchangeable ions in the soil matrix capable of forming strong complexes with minimum specific chelating agents (Di-Palma, 2009). For removal of maximum amounts of metals found in polluted soils, phytoextraction may be used, with some portion of the soil metal content freely available to plants. There are various synthetic chelating components, such as EDTA (ethylene diamine tetra acetic acid), diethylene trinitrile pentaacetic acid (DTPA), nitrile triacetic acid (NTA), pyridine-2,6-dicarboxylic acid (PDA), trans-l,2-diaminocyclohexane-N,N,N0,N0-tetraacetate (CDTA), or ethylenediamine disuccinate (EDDS) used for remediation of soil polluted with organic and inorganic contaminants. To increase the accessibility of metals in soil and the transference of metals from root to shoot, several ideas have been proposed (Meers et al., 2005). Application of chelating agents substantially improved the Cr uptake in above-ground biomass of many crops (Table 5). Patra et al. (2018) revealed that in Cr(VI) polluted soil, application of chelators including EDTA, DTPA, citric acid, and salicylic acid, along with metal ions, enhanced the growth of lemongrass (Cymbopogon citratus) and enhanced Cr bioavailability. Chigbo and Batty (2013) analyzed that the application of EDTA and citric acid reduced alfalfa (Medicago sativa) shoot dry matter by 55%, decreasing the soil Cr removal efficiency. The removal of Cr increased to 54.28% when the polluted soil was pre-treated with 0.01M EDTA-2Na (Xu Y. et al., 2019). Mohanty and Patra (2012) revealed the total accumulation rate for Cr was improved with the application of DTPA to rice (Oryza sativa) and wheat (Triticum aestivum), While the use of EDDHA was proven to be useful in accelerating the process of Cr accumulation in green gram (Vigna radiata) seedlings. The role of chelating substances in reducing the harmful impact of Cr(VI) is demonstrated in this study. The chelating agents in the culture medium augmented with Cr(VI) improved the bioavailability of Cr in plants. In another study, EDTA application in Cr contaminated soil resulted in higher endogenous levels of Cr(III) in plants. Moreover, EDTA addition improved the growth by regulating Cr species, ion homeostasis and accumulation of secondary metabolites in castor bean (Ricinus communis L.) (Qureshi et al., 2020).
8.4. Remediation by nanoparticles
Nano-remediation is an eco-friendly and cost-effective method of detoxifying heavy metals in soil and other environments using nanoparticles (NPs) (Ahmed et al., 2021a; Wei et al., 2022a; Table 6). By absorbing heavy metals, lowering the hazardous valence to a stable metallic state, and accelerating the reaction, this unique remediation strategy has been demonstrated to be efficient in the removal of toxic heavy metals (Mondal et al., 2020). Synthesis of nZVI NPs in colloidal solution using green tea extract having an average particle diameter of 5-10 nm with polyphenol coating (which served as a capping and reducing agent) was significantly effective in remediating Cr(VI) from groundwater passing through porous soil beds (Mystrioti et al., 2014). Synthesis of NPs by using various rose apple (Syzgium jambos L.), candlenut tree (Aleurites moluccanus L.), and oolong-tea leaves extracts were significantly remediate Cr(VI) from aqueous medium up to 90% at initial 5 minutes, due to its maximum NPs antioxidant property, but complete removal took after 60 minutes (Xiao et al., 2016). The removal effectiveness of Cr(VI) was greatly influenced by factors i.e., Cr(VI) initial concentration, NPs dosage, solution pH, and temperature. For a constant concentration of Cr, the availability of active sites rises with increasing NPs dosage, which improves the removal rate (Xiao Z. et al., 2017). Various probable processes for effective decontamination of inorganic pollutants by NPs have already been hypothesized throughout the application, including precipitation, adsorption, complexation, and reduction (Mondal et al., 2020; Ahmed et al., 2021b). The most well-known method for eliminating Cr is called as reduction, which is followed by adsorption. According to Li and Zhang (2007), whenever the trace-metal ions already had a greater negatively standard-redox strength (E0) as compared to, or were like Fe0 (-0.41 V), the method for decontamination of Cr via green-synthesized iron-NPs was largely regulated by surface complexation/adsorption. However, whenever the Cr ions already had substantially higher positive E0 as compared to Fe0, precipitation and reduction of Cr ions predominate (Lin et al., 2019). When the Cr cations had somewhat more positive E0 as compared to Fe0, both reduction and adsorption happened (Ahmed et al., 2021b). Other possibilities included co-precipitation and Fe-hydroxide oxidation (Sebastian et al., 2018; Figure 4). In addition, bimetallic Fe-NPs and Fe-oxide remove pollutants through catalytic degradation and adsorption respectively (Ahmed et al., 2021b). However, there are significant limitations and knowledge gaps that must be addressed to ensure social acceptance and safe usage of green synthesized NPs for toxic heavy metals remediation. As a result, more field studies are required to assess the application’s safety, reliability, efficacy, fate, intrinsic toxicity of NPs, and long-term impacts of NPs on Cr bioavailability and absorption in contaminated soils. To attain its promised implications in the environmental sector, future research should focus on doze optimization and safe targeted delivery of NPs.
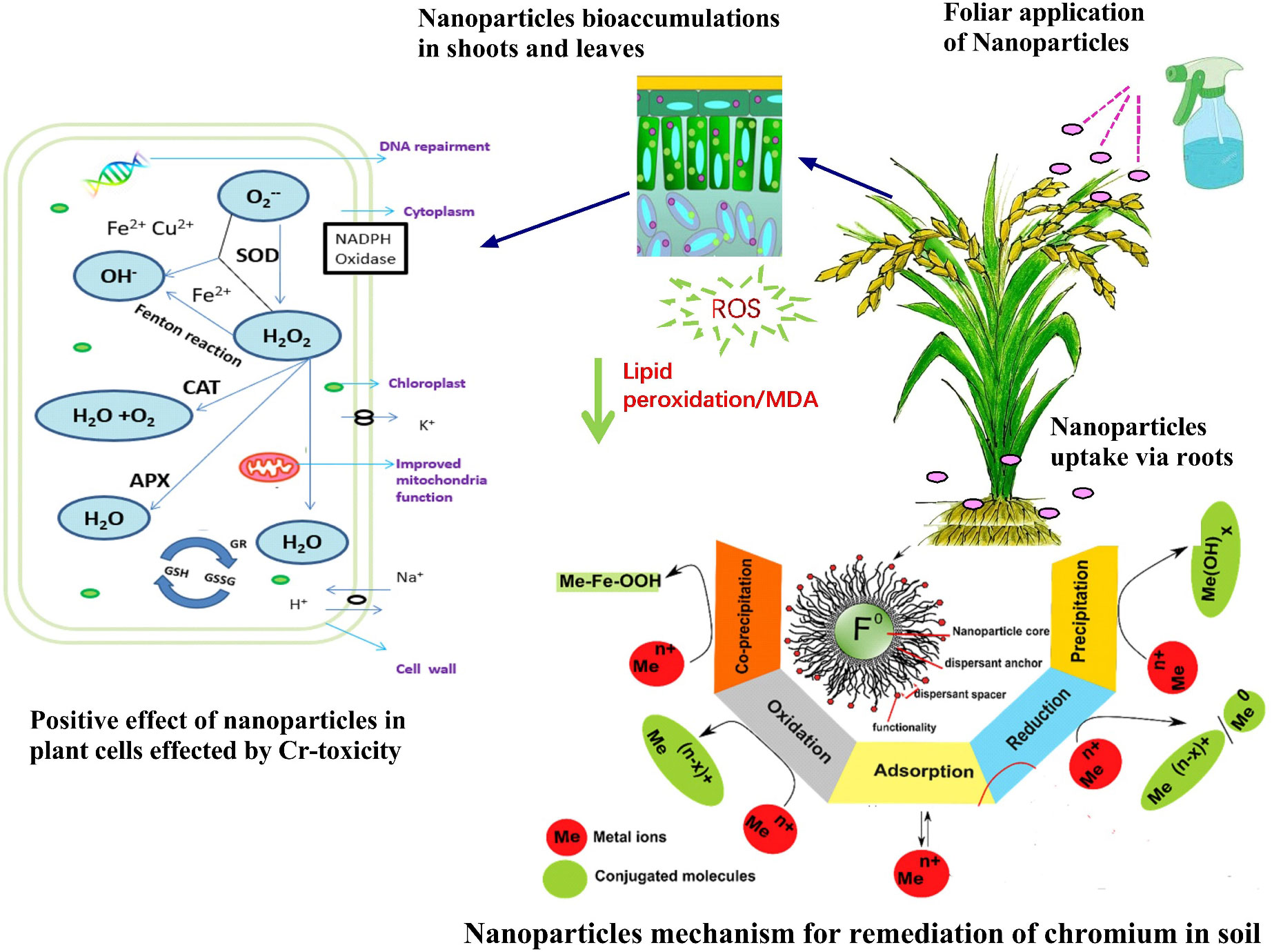
Figure 4 Nanoparticles (NPs) application reduced oxidative stress in plant species. Under chromium (Cr) toxicity, cellular respiration produces O2.- that is converted into hydrogen peroxide by the activity of superoxide (SOD). H2O2 is then converted into O2 and H2O by the combined activities of ascorbate peroxidase (APX), catalase (CAT), glutathione reductase (GR), and glutathione peroxidase (GPX). NPs minimizes the accumulation of O2.- and H2O2. The reactive oxygen species (ROS) which causes lipid peroxidation, enzyme inactivation, and cell death. The activity of ROS was significantly minimized by NPs due to improved production of antioxidants i.e., CAT, SOD, and POD (Ali et al., 2021). Mechanism representations i.e., precipitation, reduction, adsorption, oxidation, and coprecipitation for the decontamination of toxic trace-metals i.e., Cr in soil/aqueous medium by NPs with a core-shell structure (Yang et al., 2019).
8.5. Use of organic amendments for remediation
Organic material is facilitated in soil deposition of Cr, according to Branzini and Zubillaga (2012). We postulated that soil comprising most of the humidified organic material had a lesser Cr accessibility, which would minimize Cr deposition in plants. The use of organic modifications in Cr polluted soils and their impact on reducing Cr absorption in plants have been reported in several studies.
8.5.1. Biochar
Biochar is produced in a low oxygen atmosphere through the combustion of carbonaceous material collected from a range of sources (Tomczyk et al., 2020). Biochar has a higher porosity, extensive functional groups containing oxygen over its microscopic layer, and acts as an adsorbent to sequester heavy metals in soil (Xu and Fang, 2015). It has a larger surface area, a higher negative and stronger surface charge, biochar has higher absorption properties as compared to raw organic soil materials. Thus, biochar application enhances water holding capacity, reduce nutrient losses, and improve soil structure. In addition, biochar-containing soils have resemblance to organic pollutants (Yu et al., 2009; Haider et al., 2022a). Integrating biochar with other soil amendments before tillage activity, such as manure fertilizer, compost, or lime, will enhance sustainability by cutting down the amount of tillage practices needed (Haider et al., 2022b; Haider et al., 2022c). Biochar improves nutrient uptake by preventing their loss by leaching (Major, 2009). The incorporation of biochar minimizes the availability of Cr and its accumulation and toxicity in plants (Table 7). Muhammad et al. (2017) studied the use of wheat straw biochar significantly increased the yield of paddy rice, total organic carbon, and nitrogen and minimized nutrient leaching. Toxic metal concentrations like Cr Chinese cabbage (Brassica rapa subsp. pekinensis) (Xu W. et al., 2019), fenugreek (Trigonella foenum-graecum) (Raj et al., 2021), lettuce (Lactuca sativa) (Nigussie et al., 2012), radish (Nabavinia et al., 2015), maize (Abbas et al., 2020), rice (Khan S. et al., 2013), barley (Rajput et al., 2021), mustard (Choppala et al., 2015) were substantially reduced by biochar application. In recent days, application of nZVI iron nanoparticles loaded maize straw pyrolyzed biochar significantly effect to minimize the toxicity of Cr in aqueous medium (Wei et al., 2022b). However, pyrolysis temperature, type of feedstock soil type, and the influence of biochar on metal immobilization and assimilation vary within crops (Woldetsadik et al., 2016; Nkoh et al., 2022).
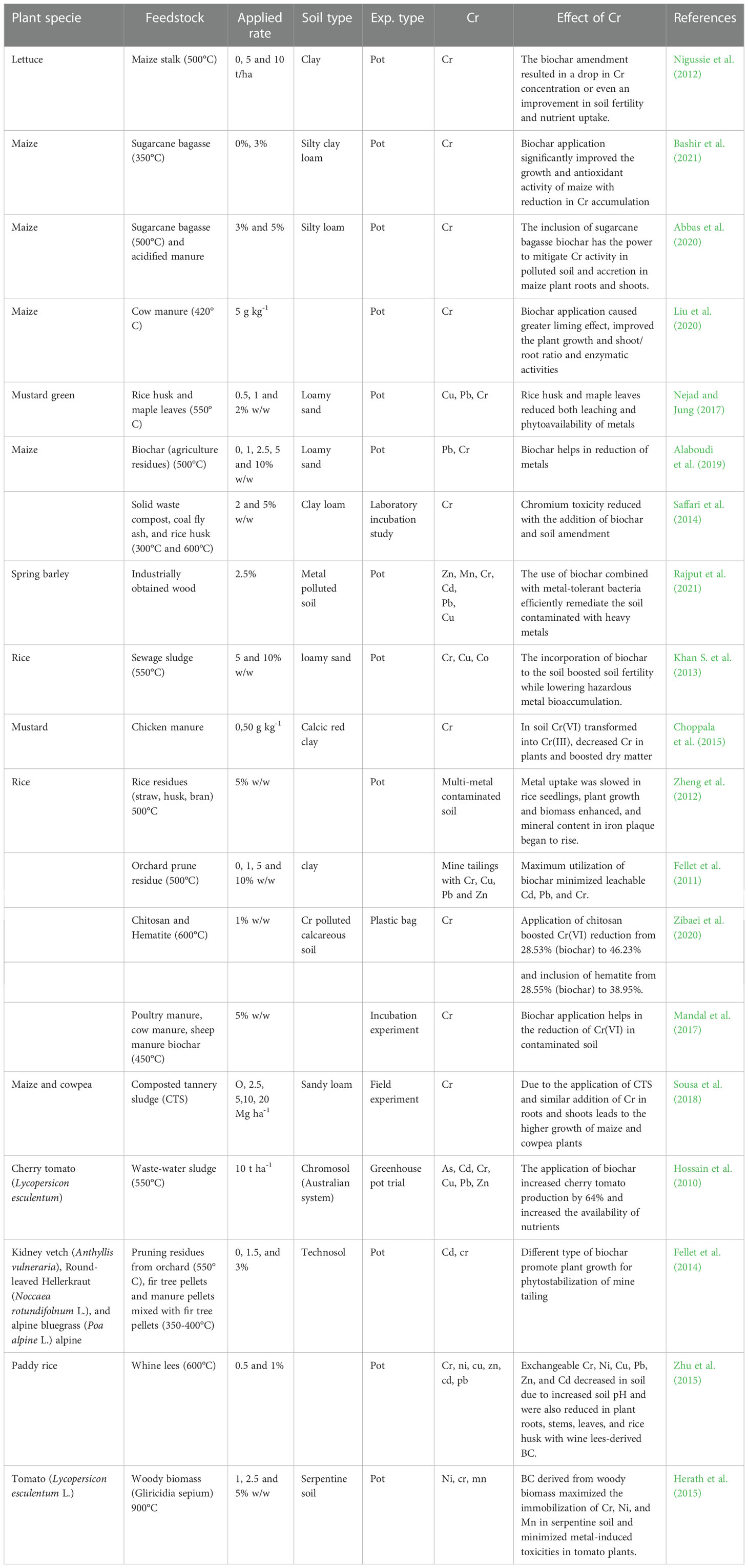
Table 7 Effects of biochar application on crops growth and Cr uptake, grown on Cr-contaminated soils.
8.5.2. Compost
Compost is a well decomposed organic material produced under anaerobic conditions (Stanislawska-Glubiak et al., 2015). Furthermore, supplying nutrients, the addition of organic composts in large amounts supplies nutrients and serves as a soil stabilizer to boost the soil physical properties. Organic composts have insignificant number of contaminants and metals and used in polluted soils to minimize the availability of metals (Park et al., 2011). Despite an increase in their overall content, vermicomposting most likely eliminates heavy metals by forming organic complexes. Vermicompost has greatly reduced the availability of metals to plants and is easily accessible at low costs and is thus known as a good replacement for minimizing the availability of the metal (Matos and Arruda, 2003). Additionally, the application of compost to achieve better crop quality in Cr-polluted soils is beneficial (Table 8). Besides, compost application to two ornamental plants lemon balm (Melissa officinalis) and begonia (Begonia semperflorens) reduced the accumulation of Cr in plant tissue (Rendina et al., 2011). Application of compost decreased the solubility of Cr in soil and rice plant assimilation. Moreover, h the addition of vermicompost significantly improved the growth and yield traits including chlorophyll contents, plant height, and number of tillers, straw yield, grain yield, and harvest index (Koka et al., 2019).
8.5.3. Manures
Organic manures improve soil fertility and microbial productivity, leading to a substantial improvement in soil health. The influence of organic changes on metals(loids) functionality and bioavailability is determined by the strength of the organic matter, microbial population, and influence on chemical and physical properties of soil, or even the specific kind of soil and metals(loids) associated (Angelova et al., 2013). Farmyard manure (FYM) is the primary source of organic manure in the cropping system. FYM has a favorable impact on agricultural yields, enhancing the physical, chemical, and biological parameters of soil (Alam et al., 2014). The application of FYM in the soil to minimize Cr toxicity in Cr polluted soils for crop plants could be a useful approach (Singh et al., 2007). The preference for manure is a vital step in achieving the good efficacy of maize crop phytoextraction. Various organic manures, when applied to the soil, reduce the bioavailability and uptake of Cr (Naser et al., 2017). The rate of Cr reduction in soil was enhanced by organic amendments examined with mustard plants. Banks et al. (2006) studied the effect of growing plant and supplemental OM (cow manure) on Cr transported in soil. As organic matter level increases, chromate leaching decreased, followed by persistence on cation exchange sites or precipitation.
8.6. Genetic mechanisms to control Cr toxicity in plants
A significant problem is avoiding and reducing the harmful effects of heavy metals contamination in soil (Zeeshan et al., 2021). Genetic engineering can significantly improve a plant’s ability to transform, translocate, and lessen the adverse impacts of heavy metals (Raza et al., 2021). Omic tools have gained a lot of interest recently for their use in plant development and programs to mitigate agricultural production challenges, specially to mitigate heavy metal stress (Khan et al., 2021). To identify target genes, proteins, and metabolites linked to Cr detoxification and stress tolerance responses in plants, genomics, proteomics, and metabolomics have become effective methods (Chaudhary et al., 2019). It is possible to modify the Cr stress-responsive genes, proteins, and metabolites to either increase plant tolerance to Cr stress or decrease Cr accumulation (Thakur et al., 2019). Tools for genetic engineering that are particularly effective at changing the genes involved in the acquisition, transport, and accumulation of Cr inside the plant are necessary for this type of manipulation (Khan et al., 2021). The main goal of genetic engineering is the creation of tolerant varieties using either a transgenic approach or genome editing (Raza et al., 2021). Anwar and Kim (2020) reported that through genome editing active participation in the control of plant metabolism, essential genes important for increased metal tolerance have been developed into transgenics, which provide insights into how to understand and improve the tolerance capacity of plants. A successful method for creating resistant cultivars is to transfer candidate genes from plants with a high tendency for HM hyper-accumulation (Rahman et al., 2022).
The best way to reduce metal toxicity within cellular locations is to use transgenic plants with altered efficiencies for metal transport into vacuoles (Khan et al., 2021). Heavy metals (HM) transporter genes are thought to be potential candidates for genetic engineering to improve metal tolerance in plants (Zhang et al., 2018). OsMTP1 in cultivated tobacco (Nicotiana tabacum) and PgIREG1 in Arabidopsis are two examples of metal transporter genes that have been genetically modified (Merlot et al., 2014; Das et al., 2016). Other metal transporter genes include those that encode metal chelators, metallothioneins (MTs) (Peng et al., 2017), and genes associated with antioxidant machinery (Peng et al., 2017; Raza et al., 2021). The use of transgenic techniques to increase resistance to metal oxidation has also been documented. Transgenic hyperaccumulators may be created by manipulating the antioxidant system to maintain redox equilibrium to avoid the destruction of biomolecules such as DNA, proteins, and lipids and to maintain the structural and functional stability of cellular structures of plant under Cr stress (Du et al., 2019). Transgenic plants that overexpress antioxidant genes for SOD, CAT, and APX with reduced ROS generation under Cr stress have been created to prevent metal toxicity-induced oxidative stress (Gao et al., 2016). Additionally, enhanced antioxidant systems in transgenic lines are associated with higher growth performance in terms of photosynthesis, mineral uptake, maintenance of redox homeostasis, and enzyme activity (Khan et al., 2021). Although transgenic lines created for over-expression traits do not always show the expected benefits, they can nevertheless have positive consequences by influencing the alternative tolerance mechanisms.
The phytochelatins (PCs), which contain hazardous metal ions and are enzymatically generated from GSH, amino acids, organic acids, or MTs, are another crucial area for improving the Cr stress tolerance in plants (Yadav, 2020). It should be noted that only MTs have coding genes, but the production of other compounds (such as GSH, amino acids, and organic acids) is controlled by the actions of the enzymes involved. Better physiological and biochemical characteristics, including membrane function and antioxidant activity, are displayed by transformed plants (Khan et al., 2021). According to Ai et al. (2018), overexpression of MYB1 from grown radish improved PC and anthocyanin synthesis, giving transgenic Petunia higher resistance against several metal toxicities, including Cr. Improved growth and stomatal density were seen in MYB1 over-expressing lines mainly due to the maintenance of relative water content (RWC), chlorophyll, and antioxidant activity. Therefore, it can be concluded that transgenic research aimed at creating cultivars with improved metal tolerance will have a considerable impact on crop production in the future (Ai et al., 2018).
The engineering of transcription factors (TFs) that control the synthesis of important metabolic chemicals also has an impact on the Cr stress tolerance in addition to the previously described essential regulators of metal tolerance. Many TF gene families play a vital role in the ability of HMs to withstand stress, including R2R3-type MYB, ZAT6, Zinc-Finger type, bZIP, GeBP-LIKE 4 (GPL4), and NAC (Khan et al., 2021; Raza et al., 2021). It was noted that transgenic rice that overexpresses OsMYB-R1 has a noticeable increase in lateral roots, which was assumed to be related to improved tolerance to Cr (Tiwari et al., 2020). Further supporting the role of lateral roots in Cr tolerance is the correlation between the increase in lateral roots and a corresponding increase in auxin accumulation in transgenic lines as compared to wild type plants. Along with that, it was also thought that the OsMYB-R1 over-expressing lines had significantly higher antioxidant activity and proline accumulation, which were likely mediated by salicylic acid (SA) signaling and contributed to the transgenic rice’s ability to tolerate Cr (Tiwari et al., 2020). As a result, TFs are essential molecular regulators that help plants tolerate Cr stress and lessen the negative effects of exposure to metals, which supports plant growth and development. However, the identification and functional confirmation of several additional TFs from diverse TF families, many of which are still mostly unknown, could, therefore, be helpful in creating enhanced plant types with high HM tolerance.
9. Conclusion and future perspectives
This paper presents new perspectives on Cr toxicity in plants and provides a review of related research on Cr toxicity in the environment, mainly in water and soil. Cr exists primarily in three oxidative states: Cr (0), Cr(III), and Cr(VI) which are the most stable form of Cr. Cr (0) is the metallic kind, the kind of Cr(III), and Cr(VI) is the most preponderant in soil and water. The current review looked at the various negative impacts of Cr exposure in plants, both morphologically and physiologically. Cr can cause a variety of hazardous consequences in plants, including changes in the germination process and root, stem, and leaf growth, as well as detrimental impacts on morphological and physiological systems like photosynthesis, water relations, and mineral nutrition. The hazardous qualities of Cr(VI) stem from its action as an oxidizing agent and the generation of free radicals during the reduction of Cr(VI) to Cr(III) that happens within the cell. Apart from generating reactive oxygen species (ROS), Cr(III) in the contrary can induce hazardous effects when present in large amounts because of its propensity to coordinate diverse chemical molecules, resulting in inhibition of metalloenzyme systems. Several approaches for viable alleviation of Cr-induced phytotoxicity have been used to combat this threat. Bioremediation, which involves phytoremediation (phytoextraction, phytodegradation, phytovolatilization, rhizosphere destruction, rhizofiltration, phytostabilization, and phytorestoration), and microbial treatment are the most common solutions (bacteria and fungi). Exogenous use of chelates, organic amendments (biochar, manure, and compost), and nano-remediation supplements are some more current Cr decontamination approaches. The findings of this review support the development of innovative and useful methods to limit the bioavailability and toxicity of chromium and the sustainable management of chromium-contaminated soil/water, thus benefiting the environment and public health. Harmful threats must be mitigated.
Chromium contamination in soil continues to increase with the increase in global production and use of the metal, which could endanger the lives of animals, plants, and humans. To better understand the ecological harm caused by Cr and practical remediation methods, this study concentrates on the biogeochemical behavior of Cr in soil-plant systems and the application of organic and inorganic amendments to reclaim Cr(VI)-contaminated soils. According to recent studies, there are significant differences in various chemical forms of Cr in terms of its solubility, mobility, adsorption/desorption, toxicity, bioavailability, and transformation. Chromium uptake and transport in soil plant systems is largely influenced by soil physicochemical characteristics (soil pH, EC, CEC, OM, manganese and iron oxides, microorganisms, etc.). When Cr enters plant cells through the pathways of necessary nutrients like Fe, sulphate, and phosphate, it might result in physiological and molecular alterations. Cr buildup affects nutrient intake, photosynthesis, growth, and development, and seed germination. High Cr concentrations can cause oxidative stress in plants and alter the structure of cell nuclei and chloroplasts. Overproduction of ROS could disrupt cell homoeostasis, stop cell division, harm DNA, and even cause cell death. Organic and inorganic reductants have been widely employed for the in-situ remediation of Cr(VI)-contaminated soil to lessen the hazard of Cr(VI) to soil-plant systems. Chemical, physical, and microbiological methods, as well as phytoremediation, have all been developed as countermeasures for Cr polluted soil cleanup over the previous few decades. It is especially helpful to use microorganisms to eliminate Cr from the environment. Numerous advantages of microbial remediation include lower costs and more public acceptance. Phytoremediation is a useful alternative that does away with the requirement for moving and excavating soil. However, compared to the total area of contamination, the area completely decontaminated by bacteria, and phytoremediation is substantially smaller. This study demonstrates that many environmental Cr-related concerns remain poorly understood even though several studies have been done in recent years. These include the distribution patterns of Cr in plants, the soil-plant uptake of Cr, the geochemical behavior of Cr in soil, and the process of Cr buildup. Furthermore, there is still disagreement regarding the potential environmental risks associated with the use of organic and inorganic reductants for the remediation of Cr(VI)-contaminated soils. This is because there is little knowledge about these risks. Therefore, the need for new Cr-pollution reduction strategies is urgent. With improvements in our understanding of the reciprocal interactions between the immune and neurological systems, the microbiome is increasingly seen as a crucial component of both human and animal health. We must be knowledgeable about the numerous chemical, physical, and biological remediation techniques and their corresponding benefits and drawbacks if we are to successfully combat the global threat of Cr pollution and toxicity. More research is needed to understand localization and partitioning of chromium in plant cells, determination of ROS producing and scavenging pathways, and analyzing how Ca2+ homeostasis regulates these interactions to elucidate complete Cr metabolic and detoxification mechanisms. Current research focuses on the efficiency of reduction and stabilization of reducing agents, but very little attention has been paid to the long-term stability of reduced Cr(III) in amended soils. Due to the complexity and diversity of soil systems, immobilized Cr(III) can be re-oxidized to Cr(VI) and remobilized. Therefore, it is necessary to investigate the long-term stability of chromium (III) in amended soils. Since reducing agents, especially nanomaterials can affect physical and chemical properties of soil, their potential impact on soil properties and biodiversity should also be considered to assess their ecological risks. Nevertheless, as we continue to grasp the molecular processes underlying Cr toxicity, we will be able to develop novel, more potent treatment approaches to reverse the harm exposure to this metal causes to human health. The review’s observations should aid in the development of creative and useful methods for limiting Cr bioavailability and toxicity and sustainably managing Cr-polluted soils/water, hence reducing its dangers to the environment and public health.
Author contributions
UZ: Conceptualization, Data collection and analysis, Writing – original draft. MA: Validation and Formal analysis. MM: Visualization, Formal analysis. SH: Writing – review and editing, Methodology and Supervision. BS: Contribution to study design, Software. BA: Visualization, Formal analysis. MI, SA and IK: Software, Formal analyses. FH, MW, MT and SE: Writing–review and editing, and Resource. All authors contributed to the article and approved the submitted version.
Conflict of interest
The authors declare that the research was conducted in the absence of any commercial or financial relationships that could be construed as a potential conflict of interest.
Publisher’s note
All claims expressed in this article are solely those of the authors and do not necessarily represent those of their affiliated organizations, or those of the publisher, the editors and the reviewers. Any product that may be evaluated in this article, or claim that may be made by its manufacturer, is not guaranteed or endorsed by the publisher.
References
Abbas, A., Azeem, M., Naveed, M., Latif, A., Bashir, S., Ali, A., et al. (2020). Synergistic use of biochar and acidified manure for improving growth of maize in chromium contaminated soil. Int. J. Phytoremed. 22, 52–61. doi: 10.1080/15226514.2019.1644286
Acheampong, M. A., Meulepas., R. J. W., Lens, P. N. L. (2010). Removal of heavy metals and cyanide from gold mine wastewater. J. Chem. Technol. Biotechnol. 85, 590–613. doi: 10.1002/jctb.2358
Adejumo, S. A., Togun, A. O., Adediran, J. A., Ogundiran, M. B. (2011). In-situ remediation of heavy metal contaminated soil using Mexican sunflower (Tithonia diversifolia) and cassava waste composts. World J. Agric. Sci. 7, 224–233. doi: 10.4236/ajps.2013.412302
Adhikari, A., Adhikari, S., Ghosh, S., Azahar, I., Shaw, A. K., Roy, D., et al. (2020). Imbalance of redox homeostasis and antioxidant defense status in maize under chromium (VI) stress. Environ. Exp. Bot. 169, 103873. doi: 10.1016/j.envexpbot.2019.103873
Adrees, M., Ali, S., Iqbal, M., Bharwana, S. A., Siddiqi, Z., Farid, M., et al. (2015b). Mannitol alleviates chromium toxicity in wheat plants in relation to growth, yield, stimulation of anti-oxidative enzymes, oxidative stress and cr uptake in sand and soil media. Ecotoxicol Environ. Saf. 122, 1–8. doi: 10.1016/j.ecoenv.2015.07.003
Adrees, M., Ali, S., Rizwan, M., Ibrahim, M., Abbas, F., Farid, M., et al. (2015a). The effect of excess copper on growth and physiology of important food crops: A review. Environ. Sci. pollut. Res. 22, 8148–8162. doi: 10.1007/s11356-015-4496-5
Afshan, S., Ali, S., Bharwana, S. A., Rizwan, M., Farid, M., Abbas, F., et al. (2015). Citric acid enhances the phytoextraction of chromium, plant growth, and photosynthesis by alleviating the oxidative damages in Brassica napus L. Environ. Sci. pollut. Res. 22, 11679–11689. doi: 10.1007/s11356-015-4396-8
Aharchaou, I., Rosabal, M., Liu, F., Battaglia, E., Vignati, D., Fortin, C. (2017). Bioaccumulation and subcellular partitioning of Cr(III) and Cr(VI) in the freshwater green alga Chlamydomonas reinhardtii. Aquat Toxicol. 182, 49–57. doi: 10.1016/j.aquatox.2016.11.004
Ahemad, M. (2015). Enhancing phytoremediation of chromium-stressed soils through plant-growth-promoting bacteria. J. Genet. Eng. Biotechnol. 13, 51–58. doi: 10.1016/j.jgeb.2015.02.001
Ahmad, R., Ali, S., Hannan, F., Rizwan, M., Iqbal, M., Hassan, Z., et al. (2017). Promotive role of 5-aminolevulinic acid on chromium-induced morphological, photosynthetic, and oxidative changes in cauliflower (Brassica oleracea botrytis L.). Environ. Sci. pollut. Res. 24, 8814–8824. doi: 10.1007/s11356-017-8603-7
Ahmad, K., Ejaz, A., Azam, M., Khan, Z. I., Ashraf, M., Al-Qurainy, F., et al. (2011). Lead, cadmium and chromium contents of canola irrigated with sewage water. Pak J. Bot. 43, 1403–1410.
Ahmad, M., Ghoneim, A., Al-Oud, S. S., Alotaibi, K. D., Nadeem, M. (2019). Acidulated activation of phosphate rock enhances release, lateral transport and uptake of phosphorus and trace metals upon direct-soil application. Soil Sci. Plant Nutt. 65, 183–195. doi: 10.1080/00380768.2019.1570333
Ahmad, S., Mfarrej, M. F. B., El-Esawi, M. A., Waseem, M., Alatawi, A., Nafees, M., et al. (2022). Chromium-resistant staphylococcus aureus alleviates chromium toxicity by developing synergistic relationships with zinc oxide nanoparticles in wheat. Ecotoxicol Environ. Saf. 230, 113142. doi: 10.1016/j.ecoenv.2021.113142
Ahmed, F., Hossain, M., Abdullah, A. T., Akbor, M., Ahsan, M. (2016). Public health risk assessment of chromium intake from vegetable grown in the wastewater irrigated site in Bangladesh. Pollut. 2, 425–432. doi: 10.7508/PJ.2016.04.005
Ahmed, T., Noman, M., Ijaz, M., Ali, S., Rizwan, M., Ijaz, U., et al. (2021a). Current trends and future prospective in nanoremediation of heavy metals contaminated soils: A way forward towards sustainable agriculture. Ecotoxicol Environ. Saf. 227, 112888. doi: 10.1016/j.ecoenv.2021.112888
Ahmed, T., Noman, M., Manzoor, N., Shahid, M., Hussaini, K. M., Rizwan, M., et al. (2021b). Green magnesium oxide nanoparticles-based modulation of cellular oxidative repair mechanisms to reduce arsenic uptake and translocation in rice (Oryza sativa L.) plants. Environ. pollut. 288, 117785. doi: 10.1016/j.envpol.2021.117785
Ai, T. N., Naing, A. H., Yun, B. W., Kim, C. K. (2018). Overexpression of RsMYB1 enhances anthocyanin accumulation and heavy metal stress tolerance in transgenic petunia. Front. Plant Sci. 9, 1388. doi: 10.3389/fpls.2018.01388
Akyol, T. Y., Yilmaz, O., Uzilday, B., Uzilday, R. O., Turkan, I. (2020). Plant response to salinity: an analysis of ROS formation, signaling, and antioxidant defense. Turk J. Bot. 44, 1–13. doi: 10.3906/bot-1911-15
Alaboudi, K. A., Ahmed, B., Brodie, G. (2019). Effect of biochar on Pb, cd and cr availability and maize growth in artificial contaminated soil. Annl Agric. Sci. 64, 95–102. doi: 10.1016/j.aoas.2019.04.002
Alam, M., Hussain, Z., Khan, A., Khan, M. A., Rab, A., Asif, M., et al. (2020). The effects of organic amendments on heavy metals bioavailability in mine impacted soil and associated human health risk. Sci. Hortic. 262, 109067. doi: 10.1016/j.scienta.2019.109067
Alam, M. S., Mishra, A. K., Singh, K., Singh, S. K., David, A. (2014). Response of sulphur and FYM on soil physico-chemical properties and growth, yield and quality of mustard (Brassica nigra L). J. Agric. Physic. 14, 156–160.
Alengebawy, A., Abdelkhalek, S. T., Qureshi, S. R., Wang, M. Q. (2021). Heavy metals and pesticides toxicity in agricultural soil and plants: Ecological risks and human health implications. Toxics 3, 42. doi: 10.3390/toxics9030042
Ali, S. S., Al-Tohamy, R., Koutra, E., Moawad, M. S., Kornaros, M., Mustafa, A. M., et al. (2021). Nanobiotechnological advancements in agriculture and food industry: Applications, nanotoxicity, and future perspectives. Sci. Tot Environ. 792, 148359. doi: 10.1016/j.scitotenv.2021.148359
Ali, S., Chaudhary, A., Rizwan, M., Anwar, H. T., Adrees, M., Farid, M., et al. (2015). Alleviation of chromium toxicity by glycinebetaine is related to elevated antioxidant enzymes and suppressed chromium uptake and oxidative stress in wheat (Triticum aestivum L.). Environ. Sci. pollut. Res. 22, 10669–10678. doi: 10.1007/s11356-015-4193-4
Ali, S., Farooq, M. A., Yasmeen, T., Hussain, S., Arif, M. S., Abbas, F., et al. (2013). The influence of silicon on barley growth, photosynthesis, and ultra-structure under chromium stress. Ecotoxicol Environ. Saf. 89, 66–72. doi: 10.1016/j.ecoenv.2012.11.015
Ali, H., Khan, E., Sajad, M. A. (2013). Phytoremediation of heavy metals-concepts and applications. Chemosphere 91, 869–881. doi: 10.1016/j.chemosphere.2013.01.075
Al-Mahmud, J., Hasanuzzaman, M., Nahar, K., Rahman, A., Hossain, M. S., Fujita, M. (2017). Maleic acid assisted improvement of metal chelation and antioxidant metabolism confers chromium tolerance in Brassica juncea L. Ecotoxicol Environ. Saf. 144, 216–226. doi: 10.1016/j.ecoenv.2017.06.010
Amin, H., Arain, B. A., Amin, F., Surhio, M. A. (2013). Phytotoxicity of chromium on germination, growth, and biochemical attributes of Hibiscus esculentus L. Am. J. Plant Sci. 2013, 2431–2439. doi: 10.1016/s0269-7491(99)00238-9
Amin, A. S., Kassem, M. A. (2012). Chromium speciation in environmental samples using a solid phase spectrophotometric method. Spectrochim Acta Part A Mol. Biomol Spectrosc. 96, 541–547. doi: 10.1016/j.saa.2012.05.020
Angelova, V. R., Akova, V. I., Artinova, N. S., Ivanov, K. I. (2013). The effect of organic amendments on soil chemical characteristics. Bulg J. Agric. Sci. 19, 958–971.
Angelova, V., Ivanova, R., Pevicharova, G., Ivanov, K. (2010). “Effect of organic amendments on heavy metals uptake by potato plants,” in 19th world congress of soil science, soil solutions for a changing world (Brisbane, Australia: DVD) 16.
Anjum, S. A., Ashraf, U., Imran, K., Tanveer, M., Shahid, M., Shakoor, A., et al. (2017). Phyto-toxicity of chromium in maize: oxidative damage, osmolyte accumulation, anti-oxidative defense, and chromium uptake. Pedosphere 27, 262–273. doi: 10.1016/S1002-0160(17)60315-1
Anjum, S. A., Ashraf, U., Khan, I., Saleem, M. F., Wang, L. C. (2016a). Chromium toxicity induced alterations in growth, photosynthesis, gas exchange attributes and yield formation in maize. Pak J. Agric. Sci. 53, 751–757. doi: 10.21162/pakjas/16.3824
Anjum, S. A., Ashraf, U., Khan, I., Tanveer, M., Saleem, M. F., Wang, L. (2016b). Aluminum and chromium toxicity in maize: implications for agronomic attributes, net photosynthesis, physio-biochemical oscillations, and metal accumulation in different plant parts. Water Air Soil pollut. 227, 326. doi: 10.1007/s11270-016-3013-x
Anwar, A., Kim, J. K. (2020). Transgenic breeding approaches for improving abiotic stress tolerance: recent progress and future perspectives. Int. J. Mol. Sci. 21, 2695. doi: 10.3390/ijms21082695
Ao, M., Chen, X., Deng, T., Sun, S., Tang, Y., Morel, J. L., et al. (2022). Chromium biogeochemical behaviour in soil-plant systems and remediation strategies: A critical review. J. Hazard Mat. 424, 127233. doi: 10.1016/j.jhazmat.2021.127233
Arshad, Q., Ahmed, A. (2017). Chromium-resistant PGPB: Growth stimulatory impact on Vigna radiata L. under chromium stress. Rom Biotechnol. Lett. 22, 12988.
Ashraf, M. A., Rasheed, R., Hussain, I., Hafeez, A., Adrees, M., ur Rehman, M. Z., et al. (2022a). Effect of different seed priming agents on chromium accumulation, oxidative defense, glyoxalase system and mineral nutrition in canola (Brassica napus L.) cultivars. Environ. pollut. 309, 119769. doi: 10.1016/j.envpol.2022.119769
Ashraf, M. A., Rasheed, R., Hussain, I., Iqbal, M., Farooq, M. U., Saleem, M. H., et al. (2022b). Taurine modulates dynamics of oxidative defense, secondary metabolism, and nutrient relation to mitigate boron and chromium toxicity in Triticum aestivum L. plants. Environ. Sci. pollut. Res., 1–22. doi: 10.1007/s11356-022-19066-5
Ashraf, M. A., Rasheed, R., Zafar, S., Iqbal, M., Saqib, Z. A. (2021). Menadione sodium bisulfite neutralizes chromium phytotoxic effects in okra by regulating cytosolutes, lipid peroxidation, antioxidant system and metal uptake. Int. J. Phytoremed. 23, 736–746. doi: 10.1080/15226514.2020.1854171
Askari, S. H., Ashraf, M. A., Ali, S., Rizwan, M., Rasheed, R. (2021). Menadione sodium bisulfite alleviated chromium effects on wheat by regulating oxidative defense, chromium speciation, and ion homeostasis. Environ. Sci. pollut. Res. 28, 36205–36225. doi: 10.1007/s11356-021-13221-0
Azeez, N. A., Dash, S. S., Gummadi, S. N., Deepa, V. S. (2021). Nano-remediation of toxic heavy metal contamination: Hexavalent chromium [Cr(VI)]. Chemosphere 266, 129204. doi: 10.1016/j.chemosphere.2020.129204
Babula, P., Adam, V., Opatrilova, R., Zehnalek, J., Havel, L., Kizek, R. (2008). Uncommon heavy metals, metalloids, and their plant toxicity: A review. Environ. Chem. Lett. 6, 189–213. doi: 10.1007/s10311-008-0159-9
Balamurugan, D., Udayasooriyan, C., Kumar, V. K., Jayabala, K. R. M., Natesan, R. (2014). Removal of hexavalent chromium (Cr VI) from spiked soil using Na y (Nano sodium) zeolite supported zero valent iron nanoparticles. Environ. Ecol. Res. 2, 291–300. doi: 10.13189/eer.2014.020802
Ball, J. W., Izbicki, J. (2004). Occurrence of hexavalent chromium in ground water in the western Mojave desert, California. Appl. Geochem. 19, 1123–1135. doi: 10.1016/j.apgeochem.2004.01.011
Banerjee, S., Kamila, B., Barman, S., Joshi, S. R., Mandal, T., Halder, G. (2019). Interlining Cr(VI) remediation mechanism by a novel bacterium pseudomonas brenneri isolated from coalmine wastewater. J. Environ. Manage. 233, 271–282. doi: 10.1016/j.jenvman.2018.12.048
Banks, M. K., Schwab, A. P., Henderson, C. (2006). Leaching and reduction of chromium in soil as affected by soil organic content and plants. Chemosphere 62, 255–264. doi: 10.1016/j.chemosphere.2005.05.020
Bashir, M. A., Naveed, M., Ahmad, Z., Gao, B., Mustafa, A., Núnez-Delgado, A. (2020). Combined application of biochar and sulfur regulated growth, physiological, antioxidant responses and cr removal capacity of maize (Zea mays L.) in tannery polluted soils. J. Environ. Manage. 259, 110051. doi: 10.1016/j.jenvman.2019.110051
Bashir, M. A., Wang, X., Naveed, M., Mustafa, A., Ashraf, S., Samreen, T., et al. (2021). Biochar mediated-alleviation of chromium stress and growth improvement of different maize cultivars in tannery polluted soils. Int. J. Environ. Res. Public Health 18, 4461. doi: 10.3390/ijerph18094461
Bashri, G., Parihar, P., Singh, R., Singh, S., Singh, V. P., Prasad, S. M. (2016). Physiological and biochemical characterization of two amaranthus species under cr (VI) stress differing in cr (VI) tolerance. Plant Physiol. Biochem. 108, 12–23. doi: 10.1016/j.plaphy.2016.06.030
Basit, F., Bhat, J. A., Dong, Z., Mou, Q., Zhu, X., Wang, Y., et al. (2022). Chromium toxicity induced oxidative damage in two rice cultivars and its mitigation through external supplementation of brassinosteroids and spermine. Chemosphere. 302, 134423. doi: 10.1016/j.chemosphere.2022.13442
Bell, J., Ma, X., McDonald, T. J., Huang, C. H., Sharma, V. K. (2022). Overlooked role of chromium (V) and chromium (IV) in chromium redox reactions of environmental importance. ACS ES&T Water 2, 932–942. doi: 10.1021/acsestwater.1c00409
Bhalerao, S. A., Sharma, A. S. (2015). Chromium: As an environmental pollutant. Int. J. Curr. Microbiol. Appl. Sci. 4, 732–746. doi: 10.1016/j.jenvman.2021.112174
Bhattacharya, A., Gupta, A. (2013). Evaluation of Acinetobacter sp. B9 for cr (VI) resistance and detoxification with potential application in bioremediation of heavymetals- rich industrial wastewater. Environ. Sci. pollut. Res. 20, 6628–6637. doi: 10.1007/s11356-013-1728-4
Bolan, N. S., Adriano, D. C., Natesan, R., Koo, B. J. (2003). Effects of organic amendments on the reduction and phytoavailability of chromate in mineral soil. J. Environ. Qual. 32, 120–128. doi: 10.2134/jeq2003.1200
Branzini, A., Zubillaga, M. S. (2012). Comparative use of soil organic and inorganic amendments in heavy metals stabilization. Appl. Environ. Soil Sci. 2012, 721032. doi: 10.1155/2012/721032
Buendía-González, L., Orozco-Villafuerte, J., Cruz-Sosa, F., Barrera-Díaz, C., Vernon-Carter, E. (2010). Prosopis laevigata a potential chromium (VI) and cadmium (II) hyperaccumulator desert plant. Bioresour Technol. 101, 5862–5867. doi: 10.1016/j.biortech.2010.03.027
Burkhead, J. L., Gogolin-Reynolds, K. A., Abdel-Ghany, S. E., Cohu, C. M., Pilon, M. (2009). Copper homeostasis. New Phytol. 182, 799–816. doi: 10.1111/j.1469-8137.2009.02846.x
Cao, F., Wang, N., Zhang, M., Dai, H., Dawood, M., Zhang, G., et al. (2013). Comparative study of alleviating effects of GSH, Se, and zn under combined contamination of cadmium and chromium in rice (Oryza sativa). Biometals 26, 297–308. doi: 10.1007/s10534-013-9611-9
Caravelli, A. H., Giannuzzi, L., Zaritzky, N. E. (2008). Reduction of hexavalent chromium by Spaerotilus natans a filamentous microorganism present in activated sludges. J. Hazard Mater. 156, 214–222. doi: 10.1016/j.jhazmat.2007.12.014
Chandra, P. R., Abdussalam, A. K., Nabeesa, S. (2010). Distribution of bio-accumulated cd and cr in two vigna species and the associated histological variations. J. Stress Physiol. Biochem. 6, 4–12.
Chaudhary, J., Khatri, P., Singla, P., Kumawat, S., Kumari, A. R. V., Vikram, A., et al. (2019). Advances in omics approaches for abiotic stress tolerance in tomato. Biology 8, 90. doi: 10.3390/biology8040090
Chatterjee, J., Chatterjee, C.. (2000). Phytotoxicity of cobalt, chromium and copper in cauliflower. Environmental pollution, 109, 69–74. doi: 10.1016/s0269-7491(99)00238-9
Chen, H., Dou, J., Xu, H. (2018). Remediation of Cr(VI)-contaminated soil with cocomposting of three different biomass solid wastes. J. Soils Sediments 18, 897–905. doi: 10.1007/s11368-017-1811-4
Chen, Z., Song, S., Wen, Y. (2016). Reduction of cr (VI) into cr (III) by organelles of Chlorella vulgaris in aqueous solution: an organelle-level attempt. Sci. Total Environ. 572, 361–368. doi: 10.1016/j.scitotenv.2016.07.217
Chen, J. C., Wang, K. S., Chen, H., Lu, C. Y., Huang, L. C., Li, H. C., et al. (2010). Phytoremediation of cr (III) by Ipomonea aquatica (water spinach) from water in the presence of EDTA and chloride: Effects of cr speciation. Bioresour Technol. 101, 3033–3039. doi: 10.1016/j.biortech.2009.12.041
Chigbo, C., Batty, L. (2013). Effect of EDTA and citric acid on phytoremediation of cr-b [a] p-co-contaminated soil. Environ. Sci. pollut. Res. 20, 8955–8963. doi: 10.1007/s11356-013-1883-7
Chiu, C. C., Cheng, C. J., Lin, T. H., Juang, K. W., Lee, D. Y. (2009). The effectiveness of four organic matter amendments for decreasing resin-extractable cr (VI) in cr (VI)-contaminated soils. J. Hazard Mater. 161, 1239–1244. doi: 10.1016/j.jhazmat.2008.04.081
Choppala, G., Bolan, N., Kunhikrishnan, A., Skinner, W., Seshadri, B. (2015). Concomitant reduction and immobilization of chromium in relation to its bioavailability in soils. Environ. Sci. pollut. Res. 22, 8969–8978. doi: 10.1007/s11356-013-1653-6
Chow, Y. N., Lee, L. K., Zakaria, N. A., Foo, K. Y. (2018). Phytotoxic effects of trivalent chromium-enriched water irrigation in Vigna unguiculata seedling. J. Clean Prod. 202, 101–108. doi: 10.1016/j.jclepro.2018.07.144
Chug, R., Gour, V. S., Mathur, S., Kothari, S. L. (2016). Optimization of extracellular polymeric substances production using Azotobacter beijreinckii and Bacillus subtilis and its application in chromium (VI) removal. Bioresour Technol. 214, 604–608. doi: 10.1016/j.biortech.2016.05.010
Dabir, A., Heidari, P., Ghorbani, H., Ebrahimi, A. (2019). Cadmium and lead removal by new bacterial isolates from coal and aluminum mines. Int. J. Environ. Sci. Technol. 16, 8297–8304. doi: 10.1007/s13762-019-02303-9
DalCorso, G. (2012). “Heavy metal toxicity in plants,” in Plants and heavy metals. springer briefs in molecular science. Ed. Furini, A. (Dordrecht: Springer), 1–25.
Danish, S., Kiran, S., Fahad, S., Ahmad, N., Ali, M. A., Tahir, F. A., et al. (2019). Alleviation of chromium toxicity in maize by fe fortification and chromium tolerant ACC deaminase producing plant growth promoting rhizobacteria. Ecotoxicol. Environ. Saf. 185, 109706. doi: 10.1016/j.ecoenv.2019.109706
Das, N., Bhattacharya, S., Maiti, M. K. (2016). Enhanced cadmium accumulation and tolerance in transgenic tobacco overexpressing rice metal tolerance protein gene OsMTP1 is promising for phytoremediation. Plant Physiol. Biochem. 105, 297–309. doi: 10.1016/j.plaphy.2016.04.049
Daud, M. K., Mei, L., Variath, M. T., Ali, S., Li, C., Rafiq, M. T., et al (2014). Chromium (VI) uptake and tolerance potential in cotton cultivars: Effect on their root physiology, ultramorphology, and oxidative metabolism. BioMed. Res. Int., 1–12. doi: 10.1155/2014/975946
Deepali, G. K. K. (2009). Chromium uptake efficiency of Spinacea olaracea from contaminated soil. J. Appl. Sci. Environ. Manage. 13, 71–72.
De-Oliveira, L. M., Gress, J., De, J., Rathinasabapathi, B., Marchi, G., Chen, Y., et al. (2016). Sulfate and chromate increased each other’s uptake and translocation in as hyperaccumulator Pteris vittata. Chemosphere 147, 36–43. doi: 10.1016/j.chemosphere.2015.12.088
Dias-Ferreira, C., Kirkelund, G. M., Ottosen, L. M. (2015). Ammonium citrate as enhancement for electrodialytic soil remediation and investigation of soil solution during the process. Chemosphere 119, 889–895. doi: 10.1016/j
Ding, G., Jin, Z., Han, Y., Sun, P., Li, G., Li, W. (2019). Mitigation of chromium toxicity in Arabidopsis thaliana by sulfur supplementation. Ecotoxicol Environ. Saf. 182, 109379. doi: 10.1016/j.ecoenv.2019.109379
Ding, H., Wang, G., Lou, L., Lv, J. (2016). Physiological responses and tolerance of kenaf (Hibiscus cannabinus L.) exposed to chromium. Ecotoxicol Environ. Saf. 133, 509–518. doi: 10.1016/j.ecoenv.2016.08.007
Di-Palma, L. (2009). Influence of indigeneous and added iron on copper extraction from soil. J. Hazard Mater 170, 96–102. doi: 10.1016/j.jhazmat.2009.04.128
Di-Palma, L., Ferrantelli, P., Medici, F. (2005). Heavy metals extraction from contaminated soil: recovery of the flushing solution. J. Environ. Manage. 77, 205–211. doi: 10.1016/j.jenvman.2005.02.018
Di-Palma, L., Gueye, M., Petrucci, E. (2015). Hexavalent chromium reduction in contaminated soil: a comparison between ferrous sulphate and nanoscale zerovalent iron. J. Hazard Mater. 281, 70–76. doi: 10.1016/j.jhazmat.2014.07.058
Dodd, I. C., Perez-Alfocea, F. (2012). Microbial amelioration of crop salinity stress. J. Exp. Bot. 63, 3415–3428. doi: 10.1093/jxb/ers033
Dotaniya, M. L., Das, H., Meena, V. D. (2014). Assessment of chromium efficacy on germination, root elongation, and coleoptile growth of wheat (Triticum aestivum L.) at different growth periods. Environ. Monit Assess. 186, 2957–2963. doi: 10.1007/s10661-013-3593-5
Du, B., Zhao, W., An, Y., Li, Y., Zhang, X., Song, L., et al. (2019). Overexpression of an alfalfa glutathione s-transferase gene improved the saline-alkali tolerance of transgenic tobacco. Biol. Open 8, bio043505. doi: 10.1242/bio.043505
Ebrahimi, M. (2014). The effect of EDTA addition on the phytoremediation efficiency of Pb and cr by Echinochloa crus galii (L.) beave and associated potential leaching risk. Soil Sediment Contam Int. J. 23, 245–256. doi: 10.1080/15320383.2014.815153
Ebrahimi, M. (2015). Effect of EDTA treatment method on leaching of Pb and cr by Phragmites australis (Cav.) trin. ex steudel (common reed). Casp J. Environ. Sci. 13, 153–166.
Eckbo, C., Okkenhaug, G., Hale, S. E. (2022). The effects of soil organic matter on leaching of hexavalent chromium from concrete waste: Batch and column experiments. J. Environ. Manage. 309, 114708. doi: 10.1016/j.jenvman.2022.114708
Eleftheriou, E., Adamakis, I. D., Panteris, E., Fatsiou, M. (2015). Chromium-induced ultrastructural changes and oxidative stress in roots of Arabidopsis thaliana. Int. J. Mol. Sci. 16, 15852–15871. doi: 10.3390/ijms160715852
Ertani, A., Mietto, A., Borin, M., Nardi, S. (2017). Chromium in agricultural soils and crops: a review. Water Air Soil pollut. 228, 190. doi: 10.1007/s11270-017-3356-y
Essahale, A., Malki, M., Marín, I., Moumni, M. (2012). Hexavalent chromium reduction and accumulation by acinetobacter AB1 isolated from fez tanneries in Morocco. Indian J. Microbiol. 52, 48–53. doi: 10.1007/s12088-011-0187-1
Fahad, S., Hussain, S., Bano, A., Saud, S., Hassan, S., Shan, D., et al. (2014). Potential role of phytohormones and plant growth-promoting rhizobacteria in abiotic stresses: consequences for changing environment. Environ. Sci. Poll Res. 22, 4907–4921. doi: 10.1007/s11356-014-3754-2
Faisal, M., Hasnain, S. (2005a). Bacterial cr (VI) reduction concurrently improves sunflower (Helianthus annuus L.) growth. Biotech. Let. 27, 943–947. doi: 10.1007/s10529-005-7188-2
Faisal, M., Hasnain, S. (2005b). Chromate resistant bacillus cereus augments sunflower growth by reducing toxicity of cr (VI). J. Plant Biol. 48, 187–194. doi: 10.1007/BF03030407
Faisal, M., Hasnain, S. (2006). Growth stimulatory effect of ochrobactrum intermedium and bacillus cereus on Vigna radiata plants. Let Appl. Microbiol. 43, 461–466. doi: 10.1111/j.1472-765X.2006.01977.x
Farid, M., Ali, S., Akram, N. A., Rizwan, M., Abbas, F., Bukhari, S. A. H., et al. (2017). Phyto-management of cr-contaminated soils by sunflower hybrids: Physiological and biochemical response and metal extractability under cr stress. Environ. Sci. pollut. Res. 24, 16845–16859. doi: 10.1007/s11356-017-9247-3
Farooq, M., Ali, S., Hameed, A., Bharwana, S., Rizwan, M., Ishaque, W., et al. (2016). Cadmium stress in cotton seedlings: physiological, photosynthesis and oxidative damages alleviated by glycinebetaine. S Afr J. Bot. 104, 61–68. doi: 10.1016/j.sajb.2015.11.006
Fatima, H., Ahmed, A. (2018). Micro-remediation of chromium contaminated soil. Peer J. 6, 1–13. doi: 10.7717/peerj.6076
Fazlzadeh, M., Rahmani, K., Zarei, A., Abdoallahzadeh, H., Nasiri, F., Khosravi, R. (2017). A novel green synthesis of zero valent iron nanoparticles (nZVI ) using three plant extracts and their efficient application for removal of Cr(VI) from aqueous solutions. Adv. Powder Technol. 28, 122–130. doi: 10.1016/j.apt.2016.09.003
Fellet, G., Marchiol, L., Delle, V. G., Peressotti, A. (2011). Application of biochar on mine tailings: Effects and perspectives for land reclamation. Chemosphere 83, 1262–1267. doi: 10.1016/j.chemosphere.2011.03.053
Fellet, G., Marmiroli, M., Marchiol, L. (2014). Elements uptake by metal accumulator species grown on mine tailings amended with three types of biochar. Sci. Total Environ. 468, 598–608. doi: 10.1016/j.scitotenv.2013.08.072
Fernandez, P. M., Vinarta, S. C., Bernal, A. R., Cruz, E. L., Figueroa, L. I. C. (2018). Bioremediation strategies for chromium removal: current research, scale-up approach and future perspectives. Chemosphere 208, 139–148. doi: 10.1016/j.chemosphere.2018.05.166
Finzgar, N., Lestan, D. (2007). Multi-step leaching of Pb and zn contaminated soils with EDTA. Chemosphere 66, 824–832. doi: 10.1016/j.chemosphere.2006.06.029
Firdaus-E-Bareen, Tahira, S. A. (2010). Efficiency of seven different cultivated plant species for phytoextraction of toxic metals from tannery effluent contaminated soil using EDTA. Soil Sediment Contam. 19, 160–173. doi: 10.1080/15320380903548474
Fozia, A., Muhammad, A. Z., Muhammad, A., Zafar, M. K. (2008). Effect of chromium on growth attributes in sunflower (Helianthus annuus L.). J. Environ. Sci. 20, 1475–1480. doi: 10.1016/S1001-0742(08)62552-8
Gao, X., Ai, W. L., Gong, H., Cui, L. J., Chen, B. X., Luo, H. Y., et al. (2016). Transgenic NfFeSOD Sedum alfredii plants exhibited profound growth impairments and better relative tolerance to long-term abiotic stresses. Plant Biotechnol. Rep. 10, 117–128. doi: 10.1007/s11816-016-0391-x
Gavrilescu, M. (2022). Enhancing phytoremediation of soils polluted with heavy metals. Curr. Opin. Biotechnol. 74, 21–31. doi: 10.1016/j.copbio.2021.10.024
González, A., Gil-Díaz, M. M., Pinilla, P., Lobo, M. C. (2017). Impact of cr and zn on growth, biochemical and physiological parameters, and metal accumulation by wheat and barley plants. Water Air Soil pollut. 228, 1–17. doi: 10.1007/s11270-017-3507-1
Gopal, R., Rizvi, A. H., Nautiyal, N. (2009). Chromium alters iron nutrition and water relations of spinach. J. Plant Nutr. 32, 1551–1559. doi: 10.1080/01904160903094313
Gul, S., Naz, A., Fareed, I., Irshad, M. (2015). Reducing heavy metals extraction from contaminated soils using organic and inorganic amendments-a review. Pol. J. Environ. Stud. 24, 1423–1426. doi: 10.15244/pjoes/26970
Gupta, D., Huang, H., Yang, X., Razafindrabe, B., Inouhe, M. (2010). The detoxification of lead in Sedum alfredii h. @ is not related to phytochelatins but the glutathione. J. Hazard Mater 177, 437–444. doi: 10.1016/j.jhazmat.2009.12.052
Gupta, A., Meyer, J. M., Goel, R. (2002). Development of heavy metal-resistant mutants of phosphate solubilizing pseudomonas sp. NBRI 4014 and their characterization. Curr. Microbiol. 45, 323–327. doi: 10.1007/s00284-002-3762-1
Gupta, A. K., Sinha, S. (2006). Chemical fractionation and heavy metal accumulation in the plant of Sesamum indicum (L.) var. T55 grown on soil amended with tannery sludge: Selection of single extractants. Chemosphere. 64, 161–173. doi: 10.1016/j.chemosphere.2005.10.016
Gu, Y., Xu, W., Liu, Y., Zeng, G., Huang, J., Tan, X., et al. (2015). Mechanism of cr (VI) reduction by Aspergillus niger: enzymatic characteristic, oxidative stress response, and reduction product. Environ. Sci. pollut. Res. 22, 6271–6279. doi: 10.1007/s11356-014-3856-x
Hafiz, M. F., Ma, L. (2021). Effect of chromium on seed germination, early seedling growth and chromium accumulation in tomato genotypes. Acta Physiol. Plant 43, 100. doi: 10.1007/s11738-021-03267-5
Haider, F. U., Farooq, M., Naveed, M., Cheema, S. A., Ain, U.-N., Salim, M. A., et al. (2022b). Influence of biochar and microorganism co-application on stabilization of cadmium (Cd) and improved maize growth in cd-contaminated soil. Front. Plant Sci. 13, 983830. 10.3389/fpls.2022.983830
Haider, F. U., Wang, X., Farooq, M., Hussain, S., Cheema, S. A., Ain, U.-N., et al. (2022c). Biochar application for the remediation of trace metals in contaminated soils: Implications for stress tolerance and crop production. Ecotoxicol. Environ. Saf. 230, 113165. doi: 10.1016/j.ecoenv.2022.113165
Haider, F. U., Wang, X., Zulfiqar, U., Farooq, M., Hussain, S., Mehmood, T., et al. (2022a). Biochar application for remediation of organic toxic pollutants in contaminated soils; an update. Ecotoxicol Environ. Saf. 248, 114322. doi: 10.1016/j.ecoenv.2022.114322
Handa, N., Kohli, S. K., Sharma, A., Thukral, A. K., Bhardwaj, R., Alyemeni, M. N., et al. (2018b). Selenium ameliorates chromium toxicity through modifications in pigment system, antioxidative capacity, osmotic system, and metal chelators in Brassica juncea seedlings. South Afr. J. Bot. 119, 1–10. doi: 10.1016/j.sajb.2018.08.003
Handa, N., Kohli, S. K., Thukral, A. K., Bhardwaj, R., Alyemeni, M. N., Wijaya, L., et al. (2018a). Protective role of selenium against chromium stress involving metabolites and essential elements in Brassica juncea L. seedlings. 3 Biotech. 8, 1–14. doi: 10.1007/s13205-018-1087-4
Han, F. X., Sridhar, B. M., Monts, D. L., Su, Y. (2004). Phytoavailability and toxicity of trivalent and hexavalent chromium to Brassica juncea. New Phytol. 162, 489–499. doi: 10.1111/j.1469-8137.2004.01027.x
Hao, Y., Ma, H., Wang, Q., Zhu, C., He, A. (2022). Complexation behaviour and removal of organic-cr (III) complexes from the environment: A review. Ecotoxicol Environ. Saf. 240, 113676. doi: 10.1016/j.ecoenv.2022.113676
Hattab, N., Motelica-Heino, M., Faure, O., Bouchardon, J. L. (2015). Effect of fresh and mature organic amendments on the phytoremediation of technosols contaminated with high concentrations of trace elements. J. Environ. Manage. 159, 37–47. doi: 10.1016/j.jenvman.2015.05.012
He, Z., Gao, F., Sha, T., Hu, Y., He, C. (2009). Isolation and characterization of a cr (VI) reduction Ochrobactrum sp. strain CSCr-3 from chromium landfill. J. Hazard Mater. 163, 869–873. doi: 10.1016/j.jhazmat.2008.07.041
He, Z., Hu, Y., Yin, Z., Hu, Y., Zhong, H. (2016). Microbial diversity of chromium-contaminated soils and characterization of six chromium-removing bacteria. Environ. Manage. 57, 1319–1328. doi: 10.1007/s00267-016-0675-5
Herath, I., Kumarathilaka, P., Navaratne, A., Rajakaruna, N., Vithanage, M. (2015). Immobilization and phytotoxicity reduction of heavy metals in serpentine soil using biochar. J. Soils Sediments 15, 126–138. doi: 10.1007/s11368-014-0967-4
Hossain, M. K., Strezov, V., Chan, K. Y., Nelson, P. F. (2010). Agronomic properties of wastewater sludge biochar and bioavailability of metals in production of cherry tomato (Lycopersicon esculentum). Chemosphere 78, 1167–1171. doi: 10.1016/j.chemosphere.2010.01.009
Huda, A. K. M. N., Hossain, M., Mukta, R. H., Khatun, M. R., Haque, M. A. (2021). EDTA-enhanced cr detoxification and its potential toxicity in rice (Oryza sativa L.). Plant Stress 2, 100014. doi: 10.1016/j.stress.2021.100014
Huda, A. N., Swaraz, A. M., Reza, M. A., Haque, M. A., Kabir, A. H. (2016). Remediation of chromium toxicity through exogenous salicylic acid in rice (Oryza sativa L.). Water Air Soil pollut. 227, 1–11. doi: 10.1007/s11270-016-2985-x
Isak, R. S., Parveen, R. S., Rafique, A. S., Alamgir, A. S. (2013). Phytotoxic effects of heavy metals (Cr, cd, Mn and zn) on wheat (Triticum aestivum L.) seed germination and seedlings growth in black cotton soil of nanded, India. Res. J. Chem. Sci. 3, 14–23.
Islam, M. K., Khanam, S., Lee, S. Y., Alam, I., Huhl, M. R. (2014). The interaction of arsenic (As) and chromium (Cr) influences growth and antioxidant status in tossa jute (Corchorus olitorius). Plant Omics 7, 499–509.
Islam, F., Yasmeen, T., Arif, M. S., Riaz, M., Shahzad, S. M., Imran, Q., et al. (2016). Combined ability of chromium (Cr) tolerant plant growth promoting bacteria (PGPB) and salicylic acid (SA) in attenuation of chromium stress in maize plants. Plant Physiol. Biochem. 108, 456–467. doi: 10.1016/j.plaphy.2016.08.014
ITRC (2009). Phytotechnology technical and regulatory guidance and decision trees. Washington, D.C.: Interstate Technology & Regulatory Council, Phytotechnologies Team.
Jabeen, N., Abbas, Z., Iqbal, M., Rizwan, M., Jabbar, A., Farid, M., et al. (2016). Glycinebetaine mediates chromium tolerance in mung bean through lowering of cr uptake and improved antioxidant system. Arch. Agron. Soil Sci. 62, 648–662. doi: 10.1080/03650340.2015.1082032
Jamil, S., Abhilash, P. C., Singh, N., Sharma, P. N. (2009). Jatropha curcas: a potential crop for phytoremediation of coal fly ash. J. Hazard Mater. 172, 269–275. doi: 10.1016/j.jhazmat.2009.07.004
Jan, S., Noman, A., Kaya, C., Ashraf, M., Alyemeni, M. N., Ahmad, P. (2020). 24-epibrassinolide alleviates the injurious effects of cr (VI) toxicity in tomato plants: Insights into growth, physio-biochemical attributes, antioxidant activity and regulation of ascorbate–glutathione and glyoxalase cycles. J. Plant Growth Regul. 39, 1587–1604. doi: 10.1007/s00344-020-10169-2
January, M. C., Cutright, T. J., Van-Keulen, H., Wei, R. (2008). Hydroponic phytoremediation of cd, cr, Ni, as, and fe: Can Helianthus annuus hyperaccumulate multiple heavy metals? Chemosphere. 70, 531–537. doi: 10.1016/j.chemosphere.2007.06.066
Jardine, P. M., Stewart, M. A., Barnett, M. O., Basta, N. T., Brooks, S. C., Fendorf, S., et al. (2013). Influence of soil geochemical and physical properties on chromium (VI) sorption and bioaccessibility. Environ. Sci. Technol. 47 (19), 11241–11248. doi: 10.1021/es401611h
Jean, L., Bordas, F., Gautier-Moussard, C., Vernay, P., Hitmi, A., Bollinger, J. C. (2008). Effect of citric acid and EDTA on chromium and nickel uptake and translocation by Datura innoxia. Environ. pollut. 153, 555–563. doi: 10.1016/j.envpol.2007.09.013
Jin, X., Liu, Y., Tan, J., Owens, G., Chen, Z. (2017). Removal of Cr(VI) from aqueous solutions via reduction and absorption by green synthesized iron nanoparticles. J. Clean Prod. 176, 929–936. doi: 10.1016/j.jclepro.2017.12.026
John, R. P., Ahmad, K., Gadgil, S. (2009). Heavy metal toxicity: Effect on plant growth, biochemical parameters and metal accumulation by Brassica juncea L. Int. J. Plant Prod. 3, 65–76. doi: 10.22069/IJPP.2012.653
Juwarkar, A. A., Yadav, S. K., Kumar, P., Singh, S. K. (2008). Effect of biosludge and biofertilizer amendment on growth of Jatropha curcas in heavy metal contaminated soils. Environ. Monit Assess. 145, 7–15. doi: 10.1007/s10661-007-0012-9
Kabata-Pendias, A., Szteke, B. (2015). Trace elements in abiotic and biotic environments Vol. 2015 (Boca Raton, FL, USA: CRC Press).
Kakkalameli, S. B., Daphedar, A., Hulakoti, N., Patil, B. N., Taranath, T. C. (2018). Azollafifiliculoides lam as a phytotool for remediation of heavy metals from sewage. Int. J. Pharm. 8, 282–287.
Kanchinadham, S. B. K., Narasimman, L., Pedaballe, V., Kalyanaraman, C. (2015). Diffusion and leachability index studies on stabilization of chromium contaminated soil using fly ash. J. Hazard Mater. 297, 52–58. doi: 10.1016/j.jhazmat.2015.04.045
Kanwal, U., Ali, S., Shakoor, M. B., Farid, M., Hussain, S., Yasmeen, T., et al. (2014). EDTA ameliorates phytoextraction of lead and plant growth by reducing morphological and biochemical injuries in Brassica napus L. under lead stress. Environ. Sci. pollut. Res. 21, 9899–9910. doi: 10.1007/s11356-014-3001-x
Kanwar, M. K., Poonam, P. S., Bhardwaj, R. (2015). Involvement of asada-halliwell pathway during phytoremediation of chromium (VI) in Brassica juncea L. plants. Int. J. Phytoremed. 17, 1237–1243. doi: 10.1080/15226514.2015.1058326
Kapoor, R. T., Bani, Mfarrej, M. F., Alam, P., Rinklebe, J., Ahmad, P. (2022). Accumulation of chromium in plants and its repercussion in animals and humans. Environ. pollut. 301, 119044. doi: 10.1016/j.envpol.2022.119044
Karthik, C., Arulselvi, P. I. (2017). Biotoxic effect of chromium (VI) on plant growth-promoting traits of novel Cellulosimicrobium funkei strain AR8 isolated from Phaseolus vulgari rhizosphere. Geomicrobiol J. 34, 434–442. doi: 10.1080/01490451.2016.1219429
Karthik, C., Elangovan, N., Kumar, T. S., Govindharaju, S., Barathi, S., Oves, M., et al. (2017). Characterization of multifarious plant growth promoting traits of rhizobacterial strain AR6 under chromium (VI) stress. Microbiol. Res. 204, 65–71. doi: 10.1016/j.micres.2017.07.008
Karuppiah, P., Rajaram, S. (2011). Exploring the potential of chromium reducing bacillus sp. and there plant growth promoting activities. J. Microbiol. Res. 1, 17–23. doi: 10.5923/j.microbiology.20110101.04
Khan, M. N., Alamri, S., Al-Amri, A. A., Alsubaie, Q. S., AlMunqedi, B., Ali, H. M., et al. (2020). Effect of nitric oxide on seed germination and seedling development of tomato under chromium toxicity. J. Plant Growth Regul. 2, 1–13. doi: 10.1007/s00344-020-10212-2
Khan, M. Y., Asghar, H. N., Jamshaid, M. U., Akhtar, M. J., Zahir, Z. A. (2013). Effect of microbial inoculation on wheat growth and phytostabilization of chromium contaminated soil. Pak J. Bot. 45, 27–34.
Khan, M. J., Azeem, M. T., Jan, M. T., Perveen, S. (2012). Effect of amendments on chemical immobilization of heavy metals in sugar mill contaminated soils. Soil Environ. 31, 55–66.
Khan, S., Chao, C., Waqas, M., Arp, H. P. H., Zhu, Y. G. (2013). Sewage sludge biochar influence upon rice (Oryza sativa L.) yield, metal bioaccumulation and greenhouse gas emissions from acidic paddy soil. Environ. Sci. Tech. 47, 8624–8632. doi: 10.1021/es400554x
Khan, M. I. R., Chopra, P., Chhillar, H., Ahanger, M. A., Hussain, S. J., Maheshwari, C. (2021). Regulatory hubs and strategies for improving heavy metal tolerance in plants: Chemical messengers, omics and genetic engineering. Plant Physiol. Biochem. 164, 260–278. doi: 10.1016/j.plaphy.2021.05.006
Kitagawa, M., Paultre, D., Rademaker, H. (2015). Intercellular communication via plasmodesmata. New Phytol. 205, 970–972. doi: 10.1111/nph.13254
Koka, R. K., Sharma, P. K., Behera, J., Chalageri, G. (2019). Remediation of chromium toxicity by FYM and vermicompost in rice (Oryza sativa). Int. J. Curr. Microbiol. App Sci. 8, 1906–1922. doi: 10.20546/ijcmas.2019.802.222
Kováčik, J., Babula, P., Klejdus, B., Hedbavny, J. (2013). Chromium uptake and consequences for metabolism and oxidative stress in chamomile plants. J. Agric. Food Chem. 61, 7864–77873. doi: 10.1021/jf401575a
Kuiper, I., Lagendijk, E. L., Bloemberg, G. V., Lugtenberg, B. J. J. (2004). Rhizoremediation: a beneficial plant-microbe interaction. Mol. Plant Microb. Interact. 17, 6–15. doi: 10.1094/MPMI.2004.17.1.6
Kumar, V., Omar., R. A., Umrao, P. D., Kaistha, S. D. (2020a). Cr (VI) toxicity inhibits microbe enhanced plant growth promotion without affecting bioremediation potential. J. Appl. Biol. Biotechnol. 8, 28–34. doi: 10.7324/JABB.2020.80205
Kumar, V., Sharma, P. K., Jatav, H. S., Singh, S. K., Rai, A., Kant, S., et al. (2020b). Organic amendments application increases yield and nutrient uptake of mustard (Brassica juncea) grown in chromium contaminated soils. Commun. Soil Sci. Plant Anal. 51, 149–159. doi: 10.1080/00103624.2019.1695831
Kumar, V., Suryakant, P., Kumar, S., Kumar, N. (2016). Effect of chromium toxicity on plants: A review. Agriways 4, 107–120.
Lakshmi, S., Sundaramoorthy, P. (2010). Effect of chromium on germination and seedling growth of vegetable crops. Asian J. Sci. Technol. 1, 28–31.
Larsen, K. K., Wielandt, D., Schiller, M., Bizzarro, M. (2016). Chromatographic speciation of Cr(III)-species, inter-species equilibrium isotope fractionation and improved chemical purification strategies for high-precision isotope analysis. J. Chromatogr A. 1443, 162–174. doi: 10.1016/j.chroma.2016.03.040
Latha, S., Vinothini, G., Dhanasekaran, D. (2015). Chromium [Cr (VI)] biosorption property of the newly isolated actinobacterial probiont Streptomyces werraensis LD22. 3. Biotech 5, 423–432. doi: 10.1007/s13205-014-0237-6
Lee, S., Moon, J. S., Ko, T. S., Petros, D., Goldsbrough, P. B., Korban, S. S. (2003). Overexpression of arabidopsis phytochelatin synthase paradoxically leads to hypersensitivity to cadmium stress. Plant Physiol. 131, 656–663. doi: 10.1104/pp.014118
Levitt, J. (1972). “Salts and other stress,” in Responses of plants to environmental stresses (New York: Academic Press), 489–530.
Lin, Y., Jin, X., Owens, G., Chen, Z. (2019). Simultaneous removal of mixed contaminants triclosan and copper by green synthesized bimetallic iron/nickel nanoparticles. Sci. Tot Environ. 695, 133878. doi: 10.1016/j.scitotenv.2019.133878
Li, J., Fan, M., Li, M., Liu, X. (2020). Cr (VI) removal from groundwater using double surfactant-modified nanoscale zero-valent iron (nZVI): Effects of materials in different status. Sci. Total Environ. 717, 1317112. doi: 10.1016/j.scitotenv.2020.137112
Lindblom, S. D., Abdel-Ghany, S., Hanson, B. R., Hwang, S., Terry, N., Pilon-Smits, E. A., et al (2006). Constitutive expression of a high‐affinity sulfate transporter in indian mustard affects metal tolerance and accumulation. J. Environ. Qual. 35, 726–733. doi: 10.2134/jeq2005.0119
Liu, S., Pu, S., Dend, D., Huang, H., Yan, C., Ma, H., et al. (2020). Comparable effects of manure and its biochar on reducing soil cr bioavailability and narrowing the rhizosphere extent of enzyme activities. Environ. Int. 134, 105277. doi: 10.1016/j.envint.2019.105277
Li, X. Q., Zhang, W. X. (2007). Sequestration of metal cations with zerovalent iron nanoparticles a study with high resolution X-ray photoelectron spectroscopy (HR-XPS). J. Phys. Chem. C. 111, 6939–6946. doi: 10.1021/jp0702189
Long, B., Ye, B., Liu, Q., Zhang, S., Ye, J., Zou, L., et al. (2018). Characterization of Penicillium oxalicum SL2 isolated from indoor air and its application to the removal of hexavalent chromium. PloS One 13, 0191484. doi: 10.1371/journal.pone.0191484
Lotlikar, N. P., Damare, S. R., Meena, R. M., Linsy, P., Brenda, M. (2018). Potential of marine-derived fungi to remove hexavalent chromium pollutant from culture broth. Ind. J. Microbiol. 58, 182–192. doi: 10.1007/s12088-018-0719-z
Lukina, A., Boutin, C., Rowland, O., Carpenter, D. (2016). Evaluating trivalent chromium toxicity on wild terrestrial and wetland plants. Chemosphere 162, 355–364. doi: 10.1016/j.chemosphere.2016.07.055
Lwin, C.S., Seo, B.H., Kim, H.U., Owens, G., Kim, K.R. (2018). Application of soil amendments to contaminated soils for heavy metal immobilization and improved soil quality—A critical review. Soil science and plant nutrition, 64(2), pp.156–167. doi: 10.1080/00380768.2018.1440938
Madhavi, V., Prasad, T. N., Reddy, A. V., Ravindra, R. B., Madhavi, G. (2013). Application of phytogenic zerovalent iron nanoparticles in the adsorption of hexavalent chromium. Spectrochim Acta A. 116, 17–25. doi: 10.1016/j.saa.2013.06.045
Mahmoud, M. S., Mohamed, S. A. (2017). Calcium alginate as an eco-friendly supporting material for baker's yeast strain in chromium bioremediation. HBRC J. 13, 245–254. doi: 10.1016/j.hbrcj.2015.06.003
Maiti, S., Ghosh, N., Mandal, C., Das, K., Dey, N., Adak, M. K. (2012). Responses of the maize plant to chromium stress with reference to antioxidation activity. Braz. J. Plant Physiol. 24, 203–212. doi: 10.1590/S1677-04202012000300007
Major, J. (2009). Biochar application to a Colombia savanna oxisol: fate and effect on soil fertility, crop production, nutrient leching and soil hydrology (New York, NY, USA: Dept. Crop Soil Sci. Cornell University).
Majumder, R., Sheikh, L., Naskar, A., Mukherjee, M. (2017). Depletion of cr (VI) from aqueous solution by heat dried biomass of a newly isolated fungus Arthrinium malaysianum: A mechanistic approach. Sci Rep 7, 1–15. doi: 10.1038/s41598-017-10160-0
Malaviya, P., Singh, A. (2014). Bioremediation of chromium solutions and chromium containing wastewaters. Crit. Rev. Microbiol. 30, 1–27. doi: 10.3109/1040841X.2014.974501
Mallick, S., Sinam, G., Mishra, R. K., Sinha, S. (2010). Interactive effects of cr and fe treatments on plants growth, nutrition, and oxidative status in Zea mays L. Ecotoxicol Environ. Saf. 73, 987–995. doi: 10.1016/j.ecoenv.2010.03.004
Ma, J., Lv, C., Xu, M., Chen, G., Lv, C., Gao, Z. (2016). Photosynthesis performance, antioxidant enzymes, and ultrastructural analyses of rice seedlings under chromium stress. Environ. Sci. pollut. Res. 23, 1768–1778. doi: 10.1007/s11356-015-5439-x
Mamais, D., Noutsopoulos, C., Kavallari., I., Nyktari, E., Kaldis, A., Panousi, E., et al. (2016). Biological groundwater treatment for chromium removal at low hexavalent chromium concentrations. Chemosphere 152, 238–244. doi: 10.1016/j.chemosphere.2016.02.124
Mandal, S., Sarkar, B., Bolan, N., Ok., Y. S., Naidu, R. (2017). Enhancement of chromate reduction in soils by surface modified biochar. J. Environ. Manage. 186, 277–284. doi: 10.1016/j.jenvman.2016.05.034
Mangabeira, P. A., Ferreira, A. S., De-Almeida, A. A. F., Fernandes, V. F., Lucena, E., Souza, V. L., et al. (2011). Compartmentalization and ultrastructural alterations induced by chromium in aquatic macrophytes. Biometals. 24, 1017–1026. doi: 10.1007/s10534-011-9459-9
Maqbool, Z., Asghar, H. N., Shahzad, T., Hussain, S., Riaz, M., Ali, S., et al. (2015). Isolating, screening and applying chromium reducing bacteria to promote growth and yield of okra (Hibiscus esculentus L.) in chromium contaminated soils. Ecotoxicol Environ. Saf. 114, 343–349. doi: 10.1016/j.ecoenv.2014.07.007
Marques, A. P., Rangel, A. O., Castro, P. M. (2011). Remediation of heavy metal contaminated soils: an overview of site remediation techniques. Crit. Rev. Env. Sci. Technol. 41, 879–914. doi: 10.1080/10643380903299517
Mathur, S., Kalaji, H. M., Jajoo, A. (2016). Investigation of deleterious effects of chromium phytotoxicity and photosynthesis in wheat plant. Photosynthetica 54, 185–192. doi: 10.1007/s11099-016-0198-6
Matos, G. D., Arruda, M. A. Z. (2003). Vermicompost as natural adsorbent for removing metal ions from laboratory effluents. Proc. Biochem. 39, 81–88. doi: 10.1016/S0032-9592(02)00315-1
Mazhar, R., Ilyas, N., Arshad, M., Khalid, A., Hussain, M. (2020). Isolation of heavy metal-tolerant PGPR strains and amelioration of chromium effect in wheat in combination with biochar. Iran J. Sci. Technol. Trans. A Sci. 44, 1–12. doi: 10.1007/s40995-019-00800-7
Meers, E., Ruttens, A., Hopgood, M. J., Samson, D., Tack, F. M. G. (2005). Comparison of EDTA and EDDS as potential soil amendments for enhanced phytoextraction of heavy metals. Chemosphere 58, 1011–1022. doi: 10.1016/j.chemosphere.2004.09.047
Meers, E., Tack, F. M. G., Verloo, M. G. (2008). Degradability of ethylenediaminedisuccinic acid (EDDS) in metal contaminated soils: implications for its use soil remediation. Chemosphere 70, 358–363. doi: 10.1016/j.chemosphere.2007.07.044
Mench, M., Schwitzgnibel, J. P., Schroeder, P., Bert, V., Gawronski, S., Gupta, S. (2009). Assessment of successful experiments and limitations of phytotechnologies: contaminant uptake, detoxification and sequestration, and consequences for food safety. Environ. Sci. pollut. Res. 16, 876–900. doi: 10.1007/s11356-009-0252-z
Mench, M., Vangronsveld, J., Beckx, C., Ruttens, A. (2006). Progress in assisted natural remediation of an arsenic contaminated agricultural soil. Environ. pollut. 144, 51–61. doi: 10.1016/j.envpol.2006.01.011
Merlot, S., Hannibal, L., Martins, S., Martinelli, L., Amir, H., Lebrun, M., et al. (2014). The metal transporter PgIREG1 from the hyperaccumulator Psychotria gabriellae is a candidate gene for nickel tolerance and accumulation. J. Exp. Bot. 65, 1551–1564. doi: 10.1093/jxb/eru025
Mishra, K., Gupta, K., Rai, U. N. (2009). Bioconcentration and phytotoxicity of chromium in Eichhornia crassipes. J. Environ. Biol. 30, 521–526.
Mohan, V., Devi, K. S., Srinivasan, R., Sushamani, K. (2014). In-vitro evaluation of chromium tolerant plant growth promoting bacteria from tannery sludge sample, dindugal, Tamil nadu, India. Int. J. Curr. Microbiol. App Sci. 3, 336–344.
Mohanty, M., Patra, H. K. (2012). Effect of chelate-assisted hexavalent chromium on physiological changes, biochemical alterations, and chromium bioavailability in crop plants–an in vitro phytoremediation approach. Bioremediat J. 16, 147–155. doi: 10.1080/10889868.2012.687414
Mondal, P., Anweshan, A., Purkait, M. K. (2020). Green synthesis and environmental application of iron-based nanomaterials and nanocomposite: A review. Chemosphere 259, 127509. doi: 10.1016/j.chemosphere.2020.127509
Muhammad, N., Aziz, R., Brookes, P. C., Xu, J. (2017). Impact of wheat straw biochar on yield of rice and some properties of psammaquent and plinthudult. J. Soil Sci. Plant Nutr. 17, 808–823. doi: 10.4067/S0718-95162017000300019
Mystrioti, C., Xenidis, A., Papassiopi, N. (2014). Reduction of hexavalent chromium with polyphenol-coated nano zero-valent iron: Column studies. Desal Water Treat. 56, 1162–1170. doi: 10.1080/19443994.2014.941298
Nabavinia, F., Emami, H., Astaraee, A., Lakzian, A. (2015). Effect of tannery wastes and biochar on soil chemical and physicochemical properties and growth traits of radish. Int. Agrophys. 29, 333–339. doi: 10.1515/intag-2015-0040
Naik, U. C., Srivastava, S., Thakur, I. S. (2012). Isolation and characterization of Bacillus cereus IST105 from electroplating effluent for detoxification of hexavalent chromium. Environ. Sci. pollut. Res. 19, 3005–3014. doi: 10.1007/s11356-012-0811-6
Naser, H. M., Rahman, M. Z., Sultana, S., Quddus, M. A., Haoque, M. A. (2017). Remediation of heavy metal polluted soil through organic amendments. Bangladesh J. Agric. Res. 42, 589–598. doi: 10.3329/bjar.v42i4.35786
Ndeddy-Aka, R. J., Babalola, O. O. (2016). Effect of bacterial inoculation of strains of Pseudomonas aeruginosa, Alcaligenes faecalis and Bacillus subtilis on germination, growth and heavy metal (Cd, cr, and Ni) uptake of Brassica juncea. Int. J. Phytoremed 18, 200–209. doi: 10.1080/15226514.2015.1073671
Nejad, Z. D., Jung, M. C. (2017). The effects of biochar and inorganic amendments on soil remediation in the presence of hyperaccumulator plant. Int. J. Energy Environ. Eng. 8, 317–329. doi: 10.1007/s40095-017-0250-8
Nigussie, A., Kissi, E., Misganaw, M., Ambaw, G. (2012). Effect of biochar application on soil properties and nutrient uptake of lettuces (Lactuca sativa) grown in chromium polluted soils. Am. Euros J. Agri Environ. Sci. .12, 369–376.
Nkoh, N. J., Ajibade, F. O., Atakpa, E. O., Abdulaha-Al, B. M., Mia, S., Odii, E. C., et al. (2022). Reduction of heavy metal uptake from polluted soils and associated health risks through biochar amendment: A critical synthesis. J. Hazard Mat Adv. 6, 100086. doi: 10.1016/j.hazadv.2022.100086
Oliveira, H. (2012). Chromium as an environmental pollutant: Insights on induced plant toxicity. J. Bot., 1–8. doi: 10.1155/2012/375843
Oliveira, M. L. J., Araujo, A. S. F. D., Melo, W. J. D. (2015). Chromium in soil organic matter and cowpea after four consecutive annual applications of composted tannery sludge. Rev. Bras. Ciênc Solo. 39, 297–302. doi: 10.1590/01000683rbcs20150158
Ontañon, O. M., González, P. S., Agostini, E. (2015). Biochemical and molecular mechanisms involved in simultaneous phenol and cr (VI) removal by Acinetobacter guillouiae SFC 500-1A. Environ. Sci. pollut. Res. 22, 13014–13023. doi: 10.1007/s11356-015-4571-y
Owlad, M., Aroua, M. K., Daud, W. A. W., Baroutian, S. (2009). Removal of hexavalent chromium-contaminated water and wastewater: A review. Water Air Soil pollut. 2001, 59–77. doi: 10.1007/s11270-008-9893-7
Pakade, V. E., Tavengwa, N. T., Madikizela, L. M. (2019). Recent advances in hexavalent chromium removal from aqueous solutions by adsorptive methods. RSC Adv. 9, 26142–26164. doi: 10.1039/C9RA05188K
Panda, S. K. (2007). Chromium-mediated oxidative stress and ultrastructural changes in root cells of developing rice seedlings. J. Plant Physiol. 164, 1419–1428. doi: 10.1016/j.jplph.2007.01.012
Park, J. H., Lamb, D., Paneerselvam, P., Choppala, G., Bolan, N., Chung, J. W. (2011). Role of organic amendments on enhanced bioremediation of heavy metal (loid) contaminated soils. J. Hazard Mater. 185, 549–574. doi: 10.1016/j.jhazmat.2010.09.082
Parmar, J. K., Patel, K. D. (2016). Effect of chromium and amendments on yield and heavy metal contents in different parts of wheat. Ecol. Environ. Conserv. 35, 387–393.
Patra, D. K., Pradhan, C., Patra, H. K. (2018). Chelate based phytoremediation study for attenuation of chromium toxicity stress using lemongrass: Cymbopogon flexuosus (nees ex steud.) w. Watson. Int. J. Phytorem. 20, 1324–1329. doi: 10.1080/15226514.2018.1488812
Pattnaik, S., Dash, D., Mohapatra, S., Pattnaik, M., Marandi, A. K., Das, S., et al. (2020). Improvement of rice plant productivity by native Cr(VI) reducing and plant growth promoting soil bacteria Enterobacter cloacae. Chemosphere 240, 124895. doi: 10.1016/j.chemosphere.2019.124895
Peng, J. S., Ding, G., Meng, S., Yi, H. Y., Gong, J. M. (2017). Enhanced metal tolerance correlates with heterotypic variation in SpMTL, a metallothionein-like protein from the hyperaccumulator Sedum plumbizincicola. Plant Cell Environ. 40, 1368–1378. doi: 10.1111/pce.12929
Polti, M. A., Amoroso, M. J., Abate, C. M. (2011). Intracellular chromium accumulation by Streptomyces sp. MC1. Water Air Soil pollut. 214, 49–57. doi: 10.1007/s11270-010-0401-5
Posthuma, L., Altenburger, R., Backhaus, T., Kortenkamp, A., Müller, C., Focks, A., et al. (2019). Improved component-based methods for mixture risk assessment are key to characterize complex chemical pollution in surface waters. Environ. Sci. Eur. 31, 1–11. doi: 10.1186/s12302-019-0246-5
Pourrut, B., Shahid, M., Douay, F., Dumat, C., Pinelli, E. (2013). “Molecular mechanisms involved in lead uptake, toxicity and detoxification in higher plants,” in Heavy metal stress in plants (Berlin/Heidelberg, Germany: Springer), 121–147.
Pradhan, D., Sukla, L. B., Mishra, B. B., Devi, N. (2019). Biosorption for removal of hexavalent chromium using microalgae Scenedesmus sp. J. Clean Prod. 209, 617–629. doi: 10.1016/j.jclepro.2018.10.288
Pushkar, B., Sevak, P., Parab, S., Nilkanth, N. (2021). Chromium pollution and its bioremediation mechanisms in bacteria: A review. J. Environ. Manage. 287, 112279. doi: 10.1016/j.jenvman.2021.112279
Qianqian, M., Haider, F. U., Farooq, M., Adeel, M., Shakoor, N., Jun, W., et al. (2022). Selenium treated foliage and biochar treated soil for improved lettuce (Lactuca sativa L.) growth in cd-polluted soil. J. Cleaner Prod. 335, 130267. doi: 10.1016/j.jclepro.2021.130267
Qing, X., Zhao, X., Hu, C., Wang, P., Zhang, Y., Zhang, X., et al. (2015). Selenium alleviates chromium toxicity by preventing oxidative stress in cabbage (Brassica campestris L. sp. pekinensis) leaves. Ecotoxicol Environ. Saf. 114, 179–189. doi: 10.1016/j.ecoenv.2015.01.026
Qureshi, F. F., Ashraf, M. A., Rasheed, R., Ali, S., Hussain, I., Ahmed, A., et al. (2020). Organic chelates decrease phytotoxic effects and enhance chromium uptake by regulating chromium-speciation in castor bean (Ricinus communis L.). Sci. Total Environ. 716, 137061. doi: 10.1016/j.scitotenv.2020.137061
Radziemska, M., Wyszkowski, M., Bęś, A., Mazur, Z., Jeznach, J., Brtnický, M. (2019). The applicability of compost, zeolite and calcium oxide in assisted remediation of acidic soil contaminated with cr (III) and cr (VI). Environ. Sci. pollut. Res. 26, 21351–21362. doi: 10.1007/s11356-019-05221-y
Rahman, S. U., Nawaz, M. F., Gul, S., Yasin, G., Hussain, B., Li, Y., et al. (2022). State-of-the-art OMICS strategies against toxic effects of heavy metals in plants: A review. Ecotoxicol Environ. Saf. 242, 113952. doi: 10.1016/j.ecoenv.2022.113952
Rai, S. B., Khalid, A., Qadeer, S., Mahmood, S., Aziz, I. (2016). Reduction in phytotoxicity of chromium using ACC-deaminase containing bacteria. Soil Environ. 35, 155–160.
Rajkumar, M., Nagendran, R., Lee, K. J., Lee, W. H., Kim, S. Z. (2006). Influence of plant growth promoting bacteria and Cr6+ on the growth of Indian mustard. Chemosphere 62, 741–748. doi: 10.1016/j.chemosphere.2005.04.117
Rajput, V. D., Gorovtsov, A. V., Fedorenko, G. M., Minkina, T. M., Fedorenko, A. G., Lysenko, V. S., et al. (2021). The influence of application of biochar and metal-tolerant bacteria in polluted soil on morpho-physiological and anatomical parameters of spring barley. Environ. Geochem Health 43, 1477–1489. doi: 10.1007/s10653-019-00505-1
Raj, A., Yadav, A., Arya, S., Sirohi, R., Kumar, S., Rawat, A. P., et al. (2021). Preparation, characterization and agri applications of biochar produced by pyrolysis of sewage sludge at different temperatures. Sci. Tot Environ. 795, 148722. doi: 10.1016/j.scitotenv.2021.148722
Ramírez, V., Baez, A., López, P., Bustillos, M. D. R., Villalobos, M. A., Carreño, R., et al. (2019). Chromium hyper-tolerant bacillus sp. MH778713 assists phytoremediation of heavy metals by mesquite trees (Prosopis laevigata). Front. Microbiol. 10, 1833. doi: 10.3389/fmicb.2019.01833
Rath, A., Das, A. B. (2021). Chromium stress induced oxidative burst in Vigna mungo (L.) hepper: physio-molecular and antioxidative enzymes regulation in cellular homeostasis. Physiol. Mol. Biol. Plants 27, 265–279. doi: 10.1007/s12298-021-00941-3
Raza, A., Habib, M., Charagh, S., Kakavand, S. N. (2021). “Genetic engineering of plants to tolerate toxic metals and metalloids,” in Handbook of bioremediation Academic Press, 411–436. doi: 10.1016/b978-0-12-819382-2.00026-0
Reale, L., Ferranti, F., Mantilacci, S., Corboli, M., Aversa, S., Landucci, F., et al. (2016). Cyto-histological and morpho-physiological responses of common duckweed (Lemna minor L.) to chromium. Chemosphere 145, 98–105. doi: 10.1016/j.chemosphere.2015.11.047
Rendina, A., Josefina, B. M., de-Fabrizio, I. A. (2011). Changes in the speciation, partitioning and phytoavailability of chromium induced by organic soil amendments. Chem. Speciat Bioavailab. 23, 53–60. doi: 10.3184/095422911X12971977268431
Rizvi, A., Zaidi, A., Ameen, F., Ahmed, B., AlKahtani, Muneera, D. F., et al. (2020). Heavy metal induced stress on wheat: phytotoxicity and microbiological management. RSC Adv. 10, 38379–38403. doi: 10.1039/D0RA05610C
Rossi, A. D., Rigon, M. R., Zaparoli, M., Braido, R. D., Colla, L. M., Dotto, G. L., et al. (2018). Chromium (VI) biosorption by saccharomyces cerevisiae subjected to chemical and thermal treatments. Environ. Sci. pollut. Res. 25, 19179–19186. doi: 10.1007/s11356-018-2377-4
Roy, A., Bharadvaja, N. (2021). Efficient removal of heavy metals from artificial wastewater using biochar. Environ. Nanotechnol Monit Manag 16, 100602. doi: 10.1016/j.enmm.2021.100602
Rucińska-Sobkowiak, R. (2016). Water relations in plants subjected to heavy metal stresses. Acta Physiol. Plant 38, 257. doi: 10.1007/s11738-016-2277-5
Saffari, M., Karimian, N., Ronaghi, A., Yasrebi, J., Ghasemi-Faseai, R. (2014). Reduction of chromium toxicity by applying various soil amendments in artificially contaminated soil. J. Adv. Environ. Health Res. 2, 251–262. doi: 10.22102/jaehr.2014.40176
Sagi, M., Fluhr, R. (2006). Production of reactive oxygen species by plant NADPH oxidases. Plant Physiol. 141, 336–340. doi: 10.1104/pp.106.078089
Saif, S., Khan, M. S. (2017). Assessment of heavy metals toxicity on plant growth promoting rhizobacteria and seedling characteristics of Pseudomonas putida SFB3 inoculated greengram. Acta Sci. Agric. 1, 47–56.
Sampanpanish, P., Pongsapich, W., Khaodhiar, S., Khan, E. (2006). Chromium removal from soil by phytoremediation with weed plant species in Thailand. Water Air Soil pollut. 6, 191–206. doi: 10.1007/s11267-005-9006-1
Samuel, J., Paul, M. L., Pulimi, M., Nirmala, M. J., Chandrasekaran, N., Mukherjee, A. (2012). Hexavalent chromium bioremoval through adaptation and consortia development from sukinda chromite mine isolates. Ind. Eng. Chem. Res. 51, 3740–3749. doi: 10.1021/ie201796s
Sangwan, P., Kumar, V., Joshi, U. N. (2014). Effect of chromium(vi) toxicity on enzymes of nitrogen metabolism in clusterbean (Cyamopsis tetragonoloba L.). Enzyme Res. 1–9. doi: 10.1155/2014/784036
Sarangi, B. K., Kalve, S., Pandey, R. A., Chakrabarti, T. (2009). Transgenic plants for phytoremediation of arsenic and chromium to enhance tolerance and hyperaccumulation. Transgenic Plant J. 3, 57–86.
Sayantan, D. (2013). Amendment in phosphorus levels moderate the chromium toxicity in Raphanus sativus L. as assayed by antioxidant enzymes activities. Ecotoxicol Environ. Saf. 95, 161–170. doi: 10.1016/j.ecoenv.2013.05.037
Schaumann, G. E., Mouvenchery, Y. K. (2018). Considerations on cross-linking by bivalent cations in soil organic matter with low exchange capacity. J. Plant Nutr. Soil Sci. 181 (3), 441–452. doi: 10.1002/jpln.201700246
Sebastian, A., Nangia, A., Prasad, M. N. V. (2018). A green synthetic route to phenolics fabricated magnetite nanoparticles from coconut husk extract: implications to treat metal contaminated water and heavy metal stress in Oryza sativa L. J. Clean Prod. 174, 355–366. doi: 10.1016/j.jclepro.2017.10.343
Sehrish, A. K., Aziz, R., Hussain, M. M., Rafiq, M. T., Rizwan, M., Muhammad, N., et al. (2019). Effect of poultry litter biochar on chromium (Cr) bioavailability and accumulation in spinach (Spinacia oleracea) grown in cr-polluted soil. Arabian J. Geosci. 12, 57. doi: 10.1007/s12517-018-4213-z
Seleiman, M. F., Ali, S., Refay, Y., Rizwan, M., Alhammad, B. A., El-Hendawy, S. E. (2020). Chromium resistant microbes and melatonin reduced cr uptake and toxicity, improved physio-biochemical traits and yield of wheat in contaminated soil. Chemosphere 250, 126239. doi: 10.1016/j.chemosphere.2020.12623
Semchuk, N. M., Lushchak, V., Falk, J., Krupinska, K., Lushchak, V. I. (2009). Inactivation of genes, encoding tocopherol biosynthetic pathway enzymes, results in oxidative stress in outdoor grown Arabidopsis thaliana. Plant Physiol. Biochem. 47, 384–390. doi: 10.1016/j.plaphy.2009.01.009
Seneviratne, M., Rajakaruna, N., Rizwan, M., Madawala, H. M. S. P., Ok, Y. S., Vithanage, M. (2019). Heavy metal-induced oxidative stress on seed germination and seedling development: a critical review. Environ. Geochem Health 41, 1813–1831. doi: 10.1007/s10653-017-0005-8
Shadreck, I. (2013). Chromium, an essential nutrient and pollutant: a review. Afr J. Pure Appl. Chem. 7, 310–317. doi: 10.5897/AJPAC2013.0517
Shafiq, M., Iqbal, M. Z., Mohammad, A. (2008). Effect of lead and cadmium on germination and seedling growth of Leucaena leucocephala. J. Appl. Sci. Environ. Manage. 12, 61–66. doi: 10.4314/jasem.v12i3.55497
Shah, F. U. R., Ahmad, N., Masood, K. R., Peralta-Videa, J. R., Ahmad, F. U. D. (2010). Heavy metal toxicity in plants. Plant Adapt Phytorem., 71–97. doi: 10.1007/978-90-481-9370-7_4
Shahid, M., Pourrut, B., Dumat, C., Nadeem, M., Aslam, M., Pinelli, E. (2014). Heavy metal-induced reactive oxygen species: phytotoxicity and physicochemical changes in plants. Rev. Environ. Contam Toxicol. 232, 1–44. doi: 10.1007/978-3-319-06746-9_1
Shahid, M., Shamshad, S., Rafiq, M., Khalid, S., Bibi, I., Niazi, N. K., et al. (2017). Chromium speciation, bioavailability, uptake, toxicity and detoxification in soil-plant system: A review. Chemosphere 178, 513–533. doi: 10.1016/j.chemosphere.2017.03.074
Shahzad, B., Tanveer, M., Hassan, W., Shah, A. N., Anjum, S. A., Cheema, S. A., et al. (2016). Lithium toxicity in plants: Reasons, mechanisms and remediation possibilities–a review. Plant Physiol. Biochem. 107, 104–115. doi: 10.1016/j.plaphy.2016.05.034
Shahzad, B., Tanveer, M., Rehman, A., Cheema, S. A., Fahad, S., Rehman, S., et al. (2018). Nickel; whether toxic or essential for plants and environment-a review. Plant Physiol. Biochem. 132, 641–651. doi: 10.1016/j.plaphy.2018.10.014
Shanker, A. K., Cervantes, C., Loza-Tavera, H., Avudainayagam, S. (2005). Chromium toxicity in plants. Environ. Int. 31 (5), 0–753. doi: 10.1016/j.envint.2005.02.003
Shanker, A. K., Djanaguiraman, M., Venkateswarlu., B. (2009). Chromium interactions in plants: Current status and future strategies. Metallomics 1, 375–383. doi: 10.1039/b904571f
Sharma, S., Adholeya, A. (2011). Detoxification and accumulation of chromium from tannery effluent and spent chrome effluent by Paecilomyces lilacinus fungi. Int. Biodeterior Biodegrad. 65, 309–317. doi: 10.1016/j.ibiod.2010.12.003
Sharma, A., Kapoor, D., Wang, J., Shahzad, B., Kumar, V., Bali, A. S., et al. (2020). Chromium bioaccumulation and its impacts on plants: an overview. Plants 9, 100. doi: 10.3390/plants9010100
Sharma, P., Kumar, A., Bhardwaj, R. (2016). Plant steroidal hormone epibrassinolide regulate heavy metal stress tolerance in Oryza sativa L. by modulating antioxidant defense expression. Environ. Exp. Bot. 122, 1–9. doi: 10.1016/j.envexpbot.2015.08.005
Shiyab, S. (2019). Morphophysiological effects of chromium in sour orange (Citrus aurantium L.). HortSci. 54, 829–834. doi: 10.21273/HORTSCI13809-18
Shukla, O. P., Rai, U. N., Dubey, S. (2009). Involvement and interaction of microbial communities in the transformation and stabilization of chromium during the composting of tannery effluent treated biomass of Vallisneria spiralis L. Bioresour Technol. 100, 2198–2203. doi: 10.1016/j.biortech.2008.10.036
Silva, R. S., Antunes, J. E. L., Aquino, J. P. A. D., Sousa, R. S. D., Melo, W. J. D., Araujo, A. S. F. (2021). Plant growth-promoting rhizobacteria effect on maize growth and microbial biomass in a chromium-contaminated soil. Bragantia 80. doi: 10.1590/1678-4499.20200492
Singh, G., Brar, M. S., Malhi, S. S. (2007). Decontamination of chromium by farm yard manure application in spinach grown in two texturally different cr-contaminated soils. J. Plant Nutr. 30, 289–308. doi: 10.1080/01904160601118125
Singh, M., Kushwaha, B. K., Singh, S., Kumar, V., Singh, V. P., Prasad, S. M. (2017). Sulphur alters chromium (VI) toxicity in Solanum melongena seedlings: role of sulphur assimilation and sulphur-containing antioxidants. Plant Physiol. Biochem. 112, 183–192. doi: 10.1016/j.plaphy.2016.12.024
Singh, H. P., Mahajan, P., Kaur, S., Batish, D. R., Kohli, R. K. (2013). Chromium toxicity and tolerance in plants. Environ. Chem. Lett. 11 (3), 229–254. doi: 10.1007/s10311-013-0407-5
Singh, D., Sharma, N. (2017). Effect of chromium on seed germination and seedling growth of green gram (Phaseols aureus L.) and chickpea (Cicer arietinum L). Int. J. Appl. Nat. Sci. 6, 37–46.
Sinha, V., Pakshirajan, K., Chaturvedi, R. (2018). Chromium tolerance, bioaccumulation and localization in plants: an overview. J. Environ. Manage. 206, 715–730. doi: 10.1016/j.jenvman.2017.10.033
Sousa, R. S. D., Nunes, L. A. P. L., Lima, A. B. D., Melo, W. J. D., Antunes, J. E. L., Araujo, A. S. F. D. (2018). Chromium accumulation in maize and cowpea after successive applications of composted tannery sludge. Acta Sci. Agron. 40. doi: 10.4025/actasciagron.v40i1.35361
Srivastava, S., Thakur, I. S. (2006a). Biosorption potency of aspergillus niger for removal of chromium (VI). Curr. Microbiol. 53, 232–237. doi: 10.1007/s00284-006-0103-9
Srivastava, S., Thakur, I. S. (2006b). Isolation and process parameter optimization of aspergillus sp. for removal of chromium from tannery effluent. Bioresour Technol. 97, 1167–1173. doi: 10.1016/j.biortech.2005.05.012
Srivastava, D., Tiwari, M., Dutta, P., Singh, P., Chawda, K., Kumari, M., et al. (2021). Chromium stress in plants: Toxicity, tolerance, and phytoremediation. Sustainability 13, 4629. doi: 10.3390/su13094629
Stambulska, U. Y., Bayliak, M. M., Lushchak, V. I. (2018). Chromium (VI) toxicity in legume plants: modulation effects of rhizobial symbiosis. BioMed. Res. Int. 3, 1–13. doi: 10.1155/2018/8031213
Stanislawska-Glubiak, E., Korzeniowska, J., Kocon, A. (2015). Effect of peat on the accumulation and translocation of heavy metals by maize grown in contaminated soils. Environ. Sci. pollut. Res. 22, 4706–4714. doi: 10.1007/s11356-014-3706-x
Sundaramoorthy, P., Baskaran, L., Chidambaram, A. L. A., Sankar, G. (2009). Growth and physiological activity of green gram (Vigna radiata L.) under effluent stress. Iran J. Environ. Health Sci. Engg. 6, 17–22.
Sundaramoorthy, P., Chidambaram, A., Ganesh, K. S., Unnikannan, P., Baskaran, L. (2010). Chromium stress in paddy: (i) nutrient status of paddy under chromium stress; (ii) phytoremediation of chromium by aquatic and terrestrial weeds. C R Biol. 333, 597–607. doi: 10.1016/j.crvi.2010.03.002
Sunitha, R., Mahimairaja, S. (2014). Phytostabilization of chromium by organic amendments in sunflower (Helianthus annus L.) field soil. Nat. Environ. pollut. Technol. 13, 351.
Taghipour, M., Jalali, M. (2016). Influence of organic acids on kinetic release of chromium in soil contaminated with leather factory waste in the presence of some adsorbents. Chemosphere 155, 395–404. doi: 10.1016/j.chemosphere.2016.04.063
Tang, J., Xu, J., Wu, Y., Li, Y., Tang, Q. (2012). Effects of high concentration of chromium stress on physiological and bio-chemical characters and accumulation of chromium in tea plant (Camellia sinensis L.). Afr J. Biotechnol. 11, 2248–2255. doi: 10.5897/AJB11.2402
Tariq, M., Waseem, M., Rasool, M. H., Zahoor, M. A., Hussain, I. (2019). Isolation and molecular characterization of the indigenous Staphylococcus aureus strain K1 with the ability to reduce hexavalent chromium for its application in bioremediation of metal-contaminated sites. Peer J. 7, 7726. doi: 10.7717/peerj.7726
Thakur, S., Choudhary, S., Dubey, P., Bhardwaj, P. (2019). Comparative transcriptome profiling reveals the reprogramming of gene networks under arsenic stress in Indian mustard. Genome 62, 12. doi: 10.1139/gen-2018-0152
Tirry, N., Kouchou, A., El Omari, B., Ferioun, M., El Ghachtouli, N. (2021). Improved chromium tolerance of Medicago sativa by plant growth-promoting rhizobacteria (PGPR). J. Genet. Eng. Biotechnol. 19, 149. doi: 10.1186/s43141-021-00254-8
Tiwari, K. K., Dwivedi, S., Singh, N. K., Rai, U. N., Tripathi, R. D. (2009). Chromium (VI) induced phytotoxicity and oxidative stress in pea (Pisum sativum L.): Biochemical changes and translocation of essential nutrients. J. Environ. Biol. 30, 389–394.
Tiwari, P., Indoliya, Y., Chauhan, A.-S., Singh, P., Singh, P.-K., Singh, P.-C., et al. (2020). Auxin-salicylic acid cross-talk ameliorates OsMYB–R1 mediated defense towards heavy metal, drought and fungal stress. J. Hazard Mater 399, 122811. doi: 10.1016/j.jhazmat.2020.122811
Tomczyk, A., Sokołowska, Z., Boguta, P. (2020). Biochar physicochemical properties: pyrolysis temperature and feedstock kind effects. Rev. Environ. Sci. Bio/Technol. 19, 191–215. doi: 10.1007/s11157-020-09523-3
Turgut, C., Pepe, M. K., Cutright, T. J. (2004). The effect of EDTA and citric acid on phytoremediation of cd, cr, and Ni from soil using Helianthus annuus. Environ. pollut. 131, 147–154. doi: 10.1016/j.envpol.2004.01.017
Turgut, C., Pepe, M. K., Cutright, T. J. (2005). The effect of EDTA on helianthus annuus uptake, selectivity, and translocation of heavy metals when grown in Ohio, new Mexico and Colombia soils. Chemosphere 58, 1087–1095. doi: 10.1016/j.chemosphere.2004.09.073
UdDin, I., Bano, A., Masood, S. (2015). Chromium toxicity tolerance of Solanum nigrum L. and Parthenium hysterophorus L. plants with reference to ion pattern, antioxidation activity and root exudation. Ecotoxicol Environ. Saf. 113, 271–278. doi: 10.1016/j.ecoenv.2014.12.014
Ullah, R., Hadi, F., Ahmad, S., Jan, A. U., Rongliang, Q. (2019b). Phytoremediation of lead and chromium contaminated soil improves with the endogenous phenolics and proline production in parthenium, cannabis, euphorbia, and rumex species. Water Air Soil pollut. 230, 40. doi: 10.1007/s11270-019-4089-x
Ullah, A., Shahzad, B., Tanveer, M., Nadeem, F., Sharma, A., Lee, D. J., et al. (2019a). “Abiotic stress tolerance in plants through pre-sowing seed treatments with mineral elements and growth regulators,” in Priming and pretreatment of seeds and seedlings Singapore: Springer, 427–445.
Upadhyay, N., Vishwakarma, K., Singh, J., Mishra, M., Kumar, V., Rani, R., et al. (2017). Tolerance and reduction of chromium (VI) by Bacillus sp. MNU16 isolated from contaminated coal mining soil. Front. Plant Sci. 8, 778. doi: 10.3389/fpls.2017.00778
U.S.G.S. (United States Geological Survey) (2021). Available at: http://minerals.usgs.gov/minerals/pubs/commodity/chromium/.
Usman, K., Al-Jabri, H., Abu-Dieyeh, M. H., Alsafran, M. H. S. A. (2020). Comparative assessment of toxic metals bioaccumulation and the mechanisms of chromium (Cr) tolerance and uptake in Calotropis procera. Front. Plant Sci. 11. doi: 10.3389/fpls.2020.00883
Vazques, M. D., Poschenrieder, C. H., Barcelo, J. (1987). Chromium (VI) induced structural changes in bush bean plants. Ann. Bot. 59, 427–438. doi: 10.1093/oxfordjournals.aob.a087331
Vernay, P., Gauthier-Moussard, C., Hitmi, A. (2007). Interaction of bioaccumulation of heavy metal chromium with water relation, mineral nutrition and photosynthesis in developed leaves of Lolium perenne L. Chemosphere 68, 1563–1575. doi: 10.1016/j.chemosphere.2007.02.052
Wakeel, A., Xu, M., Gan, Y. (2020). Chromium-induced reactive oxygen species accumulation by altering the enzymatic antioxidant system and associated cytotoxic, genotoxic, ultrastructural, and photosynthetic changes in plants. Int. J. Mol. Sci. 21, 728. doi: 10.3390/ijms21030728
Wang, Y., Chen, S. Y., Yang, X., Wu, Y. X., Huang, X. F., He, E. K., et al. (2019). Enhanced removal of Cr(VI) in the Fe(III)/natural polyphenols system: role of the in situ generated Fe(II). J. Hazard Mater. 377, 321–329. doi: 10.1016/j.jhazmat.2019.05.083
Wang, S. S., Ye, S. L., Han, Y. H., Shi, X. X., Chen, D. L., Li, M. (2016). Biosorption and bioaccumulation of chromate from aqueous solution by a newly isolated bacillus mycoides strain 200AsB1. RSC Adv. 6, 101153–101161. doi: 10.1039/C6RA23879C
Wani, P. A., Khan, M. S. (2010). Bacillus species enhance growth parameters of chickpea (Cicer arietinum L.) in chromium stressed soils. Food Chem. Toxicol. 48, 3262–3267. doi: 10.1016/j.fct.2010.08.035
Wani, P. A., Khan, M. S., Zaidi, A. (2008). Chromium-reducing and plant growth-promoting mesorhizobium improves chickpea growth in chromium-amended soil. Biotechnol. Lett. 30, 159–163. doi: 10.1007/s10529-007-9515-2
Wani, P. A., Wani, J. A., Wahid, S. (2018). Recent advances in the mechanism of detoxification of genotoxic and cytotoxic Cr(VI) by microbes. J. Environ. Chem. Engg. 6, 3798–3807. doi: 10.1016/j.jece.2018.05.042
Waseem, A., Arshad, J., Iqbal, F., Sajjad, A., Mehmood, Z., Murtaza, G. (2014). Pollution status of Pakistan: A retrospective review on heavy metal contamination of water, soil, and vegetables. BioMed. Res. Int., 1–29. doi: 10.1155/2014/813206
Waszczak, C., Carmody, M., Kangasj¨arvi, J. (2018). Reactive oxygen species in plant signaling. Annu. Rev. Plant Biol. 69, 209–236. doi: 10.1146/annurev
Wei, Y., Chu, R., Zhang, Q., Usman, M., Haider, F. U., Cai, L. (2022b). Nano zero-valent iron loaded corn-straw biochar for efficient removal of hexavalent chromium: remediation performance and interfacial chemical behaviour. RSC Adv. 12, 26953. doi: 10.1039/D2RA04650D
Wei, Y., Fang, Z., Zheng, L., Tan, L., Tsang, E. P. (2016). Green synthesis of fe nanoparticles using citrus maxima peels aqueous extracts. Mater Lett. 185, 384–386. doi: 10.1016/j.matlet.2016.09.029
Wei, Y., Fang, Z., Zheng, L., Tsang, E. P. (2017). Biosynthesized iron nanoparticles in aqueous extracts of Eichhornia crassipes and its mechanism in the hexavalent chromium removal. Appl. Sur Sci. 399, 322–329. doi: 10.1016/j.apsusc.2016.12.090
Wei, Y., Usman, M., Farooq, M., Adeel, M., Haider, F. U., Pan, Z., et al. (2022a). Removing hexavalent chromium by nano zero-valent iron loaded on attapulgite. Water Air Soil pollut. 233. doi: 10.1007/s11270-022-05513-z
Weng, X., Jin, X., Lin, J., Naidu, R., Chen, Z. (2016). Removal of mixed contaminants Cr(VI) and Cu(II) by green synthesized iron based nanoparticles. Ecol. Engg. 97, 32–39. doi: 10.1016/j.ecoleng.2016.08.003
Woldetsadik, D., Drechsel, P., Keraita, B., Marschner, B., Itanna, F., Gebrekidan, H. (2016). Effects of biochar and alkaline amendments on cadmium immobilization, selected nutrient and cadmium concentrations of lettuce (Lactuca sativa) in two contrasting soils. Springerplus. 5, 1–16. doi: 10.1186/s40064-016-2019-6
World Health Organization (WHO), (2017). The cost of a polluted environment: 1.7 million child deaths a year, says WHO (World Health Organization).
Wyszkowski, M., Radziemska, M. (2010). Effects of chromium (III and VI) on spring barley and maize biomass yield and content of nitrogenous compounds. J. Toxicol. Environ. Health A. 73, 1274–1282. doi: 10.1080/15287394.2010.492016
Wyszkowski, M., Radziemska, M. (2013). Assessment of tri-and hexavalent chromium phytotoxicity on oats (Avena sativa L.) biomass and content of nitrogen compounds. Water Air Soil pollut. 224, 1–14. doi: 10.1007/s11270-013-1619-9
Xiao, L., Guan, D., Chen, Y., Dai, J., Ding, W., Peart, M. R., et al. (2019). Distribution and availability of heavy metals in soils near electroplating factories. Environ. Sci. pollut. Res. 26, 22596–22610. doi: 10.1007/s11356-019-04706-0
Xiao, W., Ye, X., Yang, X., Zhu, Z., Sun, C., Zhang, Q., et al. (2017). Isolation and characterization of chromium (VI)-reducing Bacillus sp. FY1 and Arthrobacter sp. WZ2 and their bioremediation potential. Biorem J. 21, 100–108. doi: 10.1080/10889868.2017.1282939
Xiao, W., Ye, X., Zhu, Z., Zhang, Q., Zhao, S., Chen, D., et al. (2021). Continuous flooding stimulates root iron plaque formation and reduces chromium accumulation in rice (Oryza sativa L.). Sci. Tot Environ. 788, 147786. doi: 10.1016/j.scitotenv.2021.147786
Xiao, Z., Yuan, M., Yang, B., Liu, Z., Huang, J., Sun, D. (2016). Plant-mediated synthesis of highly active iron nanoparticles for Cr(VI) removal: Investigation of the leading biomolecules. Chemosphere 150, 357–364. doi: 10.1016/j.chemosphere.2016.02.056
Xiao, Z., Zhang, H., Xu, Y., Yuan, M., Jing, X., Huang, J. (2017). Ultra-efficient removal of chromium from aqueous medium by biogenic iron based nanoparticles. Sep Purif Technol. 174, 466–473. doi: 10.1016/j.seppur.2016.10.047
Xia, S., Song, Z., Jeyakumar, P., Shaheen, S. M., Rinklebe, J., Ok, Y. S., et al. (2019). A critical review on bioremediation technologies for Cr(VI)- contaminated soils and wastewater. Crit. Rev. Environ. Sci. Technol. 49 (12), 1027–1078. doi: 10.1080/10643389.2018.1564526
Xu, H., Chen, Y., Huang, H., Liu, Y., Yang, Z. (2012). Removal of lead (II) and cadmium (II) from aqueous solutions using spent. Agaricus bisporus. Can. J. Chem. Eng. 91, 421–431. doi: 10.1002/cjce.21671
Xu, Y., Fang, Z. (2015). The research progress of remediating the heavy metal-contaminated soil with biochar. China J. Environ. Eng. 35, 156–159.
Xu, Y., Li, J., Xia, W., Sun, Y., Qian, G., Zhang, J. (2019). Enhanced remediation of arsenic and chromium co-contaminated soil by eletrokinetic-permeable reactive barriers with different reagents. Environ. Sci. pollut. Res. 26, 3392–3403. doi: 10.1007/s11356-018-3842-9
Xu, T., Nan, F., Jiang, X., Tang, Y., Zeng, Y., Zhang, W., et al. (2020). Effect of soil pH on the transport, fractionation, and oxidation of chromium (III). Ecotoxicol Environ. Saf. 195, 110459. doi: 10.1016/j.ecoenv.2020.110459
Xu, X., Nie, S., Ding, H., Fan, H. F. (2018). Environmental pollution and kidney diseases. Nat. Publ Gr. 14. doi: 10.1038/nrneph.2018.11
Xu, W., Shafi, M., Penttinen, P., Hou, S., Wang, X., Ma, J., et al. (2019). Bioavailability of heavy metals in contaminated soil as affected by different mass ratios of biochars. Environ. Technol., 1–9. doi: 10.1080/21622515.2019.1609096
Xu, Z. R., Cai, M. L., Chen, S. H., Huang, X. Y., Zhao, F. J., Wang, P., et al. (2021). High-affinity sulfate transporter Sultr1; 2 is a major transporter for Cr (VI) uptake in plants. Environmental Science & Technology 55 (3), 1576–1587. doi: 10.1021/acs.est.0c04384
Yaashikaa, P. R., Senthil, K. P., Mohan, B. V. P., Kanaka, D. R., Manivasagan, V., Saranya, K., et al. (2019). Modelling on the removal of cr (VI) ions from aquatic system using mixed biosorbent (Pseudomonas stutzeri and acid treated banyan tree bark). J. Mol. Liq. 276, 362–370. doi: 10.1016/j.molliq.2018.12.004
Yadav, S. K. (2020). Heavy metals toxicity in plants: an overview on the role of glutathione and phytochelatins in heavy metal stress tolerance of plants. South Afr J. Bot. 76 (2), 167–179. doi: 10.1016/j.sajb.2009.10.007
Yan, D., Duermeyer, L., Leoveanu, C., Nambara, E. (2014). The functions of the endosperm during seed germination. Plant Cell Physiol. 55, 1521–1533. doi: 10.1093/pcp/pcu089
Yang, J., Hou, B., Wang, J., Tian, B., Bi, J., Wang, N., et al. (2019). Nanomaterials for the removal of heavy metals from wastewater. Nanomaterials 9, 424–. doi: 10.3390/nano9030424
Yang, Y., Huang, J., Sun, Q., Wang, J., Huang, L., Fu, S., et al. (2022). microRNAs: Key players in plant response to metal toxicity. Int. J. Mol. Sci. 23, 8642. doi: 10.3390/ijms23158642
Yan, A., Wang, Y., Tan, S. N., Mohd, Y. M. L., Ghosh, S., Chen, Z. (2020). Phytoremediation: A promising approach for revegetation of heavy metal-polluted land. Front. Plant Sci. 11, 359. doi: 10.3389/fpls.2020.00359
Yilmaz, S. H., Kaplan, M., Temizgul, R., Yilmaz, S. (2017). Antioxidant enzyme response of sorghum plant upon exposure to aluminum, chromium and lead heavy metals. Turkish J. Biochem. 42, 503–512. doi: 10.1515/tjb-2016-0112
Yıldız, M., Terzi, H., Bingül, N. (2013). Protective role of hydrogen peroxide pretreatment on defense systems and BnMP1 gene expression in cr (VI)-stressed canola seedlings. Ecotoxicol. 22, 1303–1312. doi: 10.1007/s10646-013-1117-2
Yu, X. Y., Ying, G. G., Kookana, R. S. (2009). Reduced plant uptake of pesticides with biochar additions to soil. Chemosphere 76, 665–671. doi: 10.1016/j.chemosphere.2009.04.001
Zaheer, I. E., Ali, S., Rizwan, M., Farid, M., Shakoor, M. B., Gill, R. A., et al. (2015). Citric acid assisted phytoremediation of copper by Brassica napus L. Ecotoxicol Environ. Saf. 120, 310–317. doi: 10.1016/j.ecoenv.2015.06.020
Zayed, A., Lytle, C. M., Qian, J. H., Terry, N. (1998). Chromium accumulation, translocation and chemical speciation in vegetable crops. Planta 206, 293–299. doi: 10.1007/s004250050403
Zayed, A. M., Terry, N. (2003). Chromium in the environment: factors affecting biological remediation. Plant Soil. 249, 139–156. doi: 10.1023/A:1022504826342
Zeeshan, N., Nasir, A. A., Haider, F. U., Naveed, K., Naseer, S., Murtaza, G. (2021). Risk assessment of trace metals deposition and effects on the growth of Abelmochus esculentus L. by industrially polluted soils of faisalabad, Pakistan. Pak J. Agri Sci. 58, 881–889. doi: 10.21162/PAKJAS/21.409
Zeid, I. M. (2001). Responses of Phaseolus vulgaris to chromium and cobalt treatments. Biol. Plant 44, 111–115. doi: 10.1023/A:1017934708402
Zhang, S., Li, S., Ding, X., Li, F., Liu, C., Liao, X., et al. (2013). Silicon mediated the detoxification of cr on pakchoi (Brassica chinensis L.) in cr-contaminated soil. J. Food Agric. Environ. 11, 814–819. doi: 10.1016/j.proenv.2013.04.009
Zhang, X., Rui, H., Zhang, F., Hu, Z., Xia, Y., Shen, Z. (2018). Overexpression of a functional Vicia sativa PCS1 homolog increases cadmium tolerance and phytochelatins synthesis in arabidopsis. Front. Plant Sci. doi: 10.3389/fpls.2018.00107
Zhang, B. Y., Zheng, J. S., Sharp, R. G. (2010). Phytoremediation in engineered wetlands: Mechanisms and applications. Proc. Environ. Sci. 2, 1315–1325. doi: 10.1016/j.proenv.2010.10.142
Zheng, R. L., Cai, C., Liang, J. H., Huang, Q., Chen, Z., Huang, Y. Z., et al. (2012). The effects of biochars from rice residue on the formation of iron plaque and the accumulation of cd, zn, Pb, as in rice (Oryza sativa L.) seedlings. Chemosphere 89, 856–862. doi: 10.1016/j.chemosphere.2012.05.008
Zhou, B. J., Chen, T. H. (2016). Biodegradation of phenol with chromium (VI) reduction by the pseudomonas sp. strain JF122. Desalin. Water Treat 57, 3544–3551. doi: 10.1080/19443994.2014.987825
Zhu, Q., Wu, J., Wang, L., Yang, G., Zhang, X. (2015). Effect of biochar on heavy metal speciation of paddy soil. Water Air Soil pollut. 226, 1–10. doi: 10.1007/s11270-015-2680-3
Ziagova, M. G., Koukkou, A. I., Liakopoulou-Kyriakides, M. (2014). Optimization of cultural conditions of arthrobacter sp. Sphe3 for growth-associated chromate (VI) reduction in free and immobilized cell systems. Chemosphere 95, 535–540. doi: 10.1016/j.chemosphere.2013.09.112
Zibaei, Z., Ghasemi-Fasaei, R., Ronaghi, A., Zarei, M., Zeinali, S. (2020). Improvement of biochar capability in cr immobilization via modification with chitosan and hematite and inoculation with Pseudomonas putida. Commun. Soil Sci. Plant Anal. 51, 963–975. doi: 10.1080/00103624.2020.1744624
Zou, J., Wang, M., Jiang, W., Liu, D. (2006). Effects of hexavalent chromium (VI) on root growth and cell division in root tip cells of Amaranthus viridis L. Pak J. Bot. 38, 673.
Zulfiqar, U., Ayub, A., Hussain, S., Waraich, E. A., El-Esawi, M. A., Ishfaq, M., et al. (2021). Cadmium toxicity in plants: Recent progress on morpho-physiological effects and remediation strategies. J. Soil Sci. Plant Nutr. 22, 212–269. doi: 10.1007/s42729-021-00645-3
Zulfiqar, U., Farooq, M., Hussain, S., Maqsood, M., Hussain, M., Ishfaq, M., et al. (2019). Lead toxicity in plants: Impacts and remediation. J. Environ. Manage. 250, 109557. doi: 10.1016/j.jenvman.2019.109557
Zulfiqar, U., Jiang, W., Wang, X., Hussain, S., Ahmad, M., Maqsood, M. F., et al. (2022). Insights into the plant-microbe interaction, soil amendments and advanced genetic approaches for cadmium remediation: A review. insights into the plant-microbe interaction, soil amendments and advanced genetic approaches for cadmium remediation: A review. Front. Plant Sci. 13. doi: 10.3389/fpls.2022.773815
Keywords: chromium phytotoxicity, environment, contamination, plant physiology and growth, remediation
Citation: Zulfiqar U, Haider FU, Ahmad M, Hussain S, Maqsood MF, Ishfaq M, Shahzad B, Waqas MM, Ali B, Tayyab MN, Ahmad SA, Khan I and Eldin SM (2023) Chromium toxicity, speciation, and remediation strategies in soil-plant interface: A critical review. Front. Plant Sci. 13:1081624. doi: 10.3389/fpls.2022.1081624
Received: 27 October 2022; Accepted: 16 December 2022;
Published: 13 January 2023.
Edited by:
M. J. I. Shohag, University of Florida, United StatesReviewed by:
Riti Thapar Kapoor, Amity University Uttar Pradesh, IndiaMuhammad Arslan Ashraf, Government College University, Faisalabad, Pakistan
Asif Naeem, Nuclear Institute for Agriculture and Biology, Pakistan
Ashutosh Yadav, Indian Institute of Toxicology Research (CSIR), India
Qaisar Mahmood, COMSATS University, Pakistan
Copyright © 2023 Zulfiqar, Haider, Ahmad, Hussain, Maqsood, Ishfaq, Shahzad, Waqas, Ali, Tayyab, Ahmad, Khan and Eldin. This is an open-access article distributed under the terms of the Creative Commons Attribution License (CC BY). The use, distribution or reproduction in other forums is permitted, provided the original author(s) and the copyright owner(s) are credited and that the original publication in this journal is cited, in accordance with accepted academic practice. No use, distribution or reproduction is permitted which does not comply with these terms.
*Correspondence: Usman Zulfiqar, dXNtYW4uenVsZmlxYXJAaXViLmVkdS5waw==; Basharat Ali, YmFzaGFyYXQyMDE4QHlhaG9vLmNvbQ==; Ilyas Khan, aS5zYWlkQG11LmVkdS5zYQ==