- 1Institute of Grassland Science, Key Laboratory of Vegetation Ecology of the Ministry of Education, Jilin Songnen Grassland Ecosystem National Observation and Research Station, Northeast Normal University, Changchun, Jilin, China
- 2State Environmental Protection Key Laboratory of Wetland Ecology and Vegetation Restoration, Northeast Normal University, Changchun, Jilin, China
- 3Key Laboratory of Geographical Processes and Ecological Security in Changbai Mountains, Ministry of Education, School of Geographical Sciences, Northeast Normal University, Changchun, Jilin, China
- 4College of Geography and Ocean Sciences, Yanbian University, Hunchun, China
- 5College of Wildlife and Protected Area, Northeast Forestry University, Harbin, China
In the context of global change, the frequency of precipitation pulses is expected to decrease while nitrogen (N) addition is expected to increase, which will have a crucial effect on soil C cycling processes as well as methane (CH4) fluxes. The interactive effects of precipitation pulses and N addition on ecosystem CH4 fluxes, however, remain largely unknown in grassland. In this study, a series of precipitation pulses (0, 5, 10, 20, and 50 mm) and long-term N addition (0 and 10 g N m-2 yr-1, 10 years) was simulated to investigate their effects on CH4 fluxes in a semi-arid grassland. The results showed that large precipitation pulses (10 mm, 20 mm, and 50 mm) had a negative pulsing effect on CH4 fluxes and relatively decreased the peak CH4 fluxes by 203-362% compared with 0 mm precipitation pulse. The large precipitation pulses significantly inhibited CH4 absorption and decreased the cumulative CH4 fluxes by 68-88%, but small precipitation pulses (5 mm) did not significantly alter it. For the first time, we found that precipitation pulse size increased cumulative CH4 fluxes quadratically in both control and N addition treatments. The increased soil moisture caused by precipitation pulses inhibited CH4 absorption by suppressing CH4 uptake and promoting CH4 release. Nitrogen addition significantly decreased the absorption of CH4 by increasing NH4+-N content and NO3–-N content and increased the production of CH4 by increasing aboveground biomass, ultimately suppressing CH4 uptake. Surprisingly, precipitation pulses and N addition did not interact to affect CH4 uptake because precipitation pulses and N addition had an offset effect on pH and affected CH4 fluxes through different pathways. In summary, precipitation pulses and N addition were able to suppress the absorption of CH4 from the atmosphere by soil, reducing the CH4 sink capacity of grassland ecosystems.
Introduction
Methane (CH4) is the second-largest greenhouse gas in the atmosphere, with a relative global warming potential over a 100-year horizon (GWP-100) of 27.9 times that of carbon dioxide (IPCC, 2021). Surprisingly, atmospheric CH4 concentrations increased as high as 1866.3 ± 3.3 ppb in 2019, 156% greater than pre-industrial levels (729.2 ± 9.4 ppb) and the largest over the past 800,000 (IPCC, 2021). These increased CH4 concentrations can raise the global surface temperature by impacting radiation processes (Milich, 1999; Boucher et al., 2009). It is estimated that atmospheric CH4 contributes to approximately 20% of global radiative forcing and is an essential contributor to global warming (Dalal and Allen, 2008; IPCC, 2021). Exchanges of CH4 between the atmosphere and soil involve complex biological processes that depend on the comprehensive performance of CH4 production by methanogens and consumption by methanotrophs (Le Mer and Roger, 2001; Freitag et al., 2010). More specifically, CH4 is generated through methanogenesis by methanogens under anaerobic conditions (Conrad et al., 2007; Juottonen, 2020), while it is oxidized and consumed under aerobic conditions by methanotrophs, a type of microbe that uses CH4 as their unique carbon (C) source (Freitag et al., 2010; Judd et al., 2016). Natural ecosystems contribute significantly to CH4 fluxes into the atmosphere and act as a source of CH4 (Dalal and Allen, 2008; Houweling et al., 2017; IPCC, 2021). Grassland is, however, recognized as a major natural sink of atmospheric CH4, consuming 3.03-3.73 Tg CH4 yr-1; this makes these environments crucial components in regulating the global CH4 budget and greenhouse effect as grassland plays an essential role in balancing atmospheric CH4 concentration (Zhuang et al., 2013; Yu et al., 2017). In the context of global climate change, the changes in precipitation pulses and N deposition significantly affect CH4 fluxes (Aronson and Helliker, 2010; Harms and Grimm, 2012; Petrakis et al., 2017; Deng et al., 2020). The interactive effects of precipitation pulses and N addition on CH4 fluxes, however, remain largely unknown in the grassland.
Since the 1870s, continued global warming has altered the global water cycles as well as precipitation regimes (Bichet et al., 2011; IPCC, 2021). The precipitation pulses and precipitation patterns have changed significantly (IPCC, 2021). Compared with precipitation pulses, scientists paid more attention to the effects of precipitation patterns on CH4 fluxes (Billings et al., 2000; Chen et al., 2013; Aronson et al., 2019; Yue et al., 2022). The response of CH4 fluxes to the precipitation pulses, however, is largely unknown. Precipitation pulses are an essential method of supplying supplementary water to the soil in natural terrestrial ecosystems, especially in arid and semi-arid regions (Noy-Meir, 1973). In the future, the occurrence of precipitation pulses is expected to decrease, while occurrences of heavy pulses are expected to increase on a global scale, altering soil biogeochemical cycling processes and ecosystem functions (Norton et al., 2008; Griffin-Nolan et al., 2021; IPCC, 2021). Ecologists have found that precipitation pulses cause an increase in soil water availability, ammonium nitrogen (NH4+-N), nitrate nitrogen (NO3–-N), dissolved organic carbon (DOC), and aboveground biomass (AGB) (Norton et al., 2008; Chen et al., 2009; Shen et al., 2015; Leitner et al., 2017; Zhao et al., 2017; Liu et al., 2019), decreases in soil temperature and soil O2 concentrations (Han et al., 2018; Niu et al., 2019), and shifts in redox conditions as well as the metabolic and community structures of soil microbes (Harms and Grimm, 2012; Wang et al., 2016; Petrakis et al., 2017). Precipitation pulses, therefore, could induce a pulse effect (also called the “Birch effect”) of greenhouse gas fluxes (Norton et al., 2008; Kim et al., 2012; Zhao et al., 2017). Although the pulse effects and driving mechanisms of precipitation pulses on carbon dioxide and nitrous oxide fluxes have been intensively studied, little is known about the response of CH4 fluxes to these changing precipitation pulses (Huxman et al., 2004; Norton et al., 2008; Chen et al., 2009; Kim et al., 2012). Previous studies have shown that small precipitation pulses do not significantly alter the CH4 fluxes in the forests or steppes examined because precipitation did not result in the substantial changes to soil water content required to affect CH4 production (Mariko et al., 2007; Ni et al., 2019). Higher precipitation pulses (31.8 mm and 200 mm) were able to stimulate an increase of up to 23,479% CH4 release in a temperate forested watershed and desert floodplain (Harms and Grimm, 2012; Petrakis et al., 2017). In contrast, more extreme precipitation pulses (203 mm and 208 mm) suppressed the absorption of CH4 in the grassland and even shifted the grassland ecosystem from a CH4 sink to a source (Zhao et al., 2017; Ren et al., 2019). This indicates that CH4 flux changes in response to precipitation pulses differ based on location, and much is still unknown about how different environments respond to precipitation pulses. How CH4 fluxes behave in response to a series of precipitation pulses also needs more study, especially examined in the context of long-term nitrogen addition.
Nitrogen (N), as an essential element, is the most limiting nutrient in arid and semi-arid grassland ecosystems (Wang et al., 2014a). Atmospheric N deposition in many parts of the world has substantially increased over the past decades (Galloway et al., 2004; Liu et al., 2013). The N enrichment on land surfaces can both alleviate N limitations and profoundly affect C cycling processes. Previous studies indicate that N addition could increase soil N availability, soil organic carbon, AGB, litter quality, and litter decomposition rates, decrease soil pH and cause acidification, and change the community structure and abundance of soil microbes and the CH4 release processes they mediated (Kruger and Frenzel, 2003; Treseder, 2008; Lu et al., 2014; Gong et al., 2015; Yang et al., 2017; Ji et al., 2019; Kong et al., 2019; Liu et al., 2021b). Various meta-analyses suggest that N addition could enhance the release of CH4 from the soil; in contrast, small amounts of N addition could stimulate CH4 uptake instead, while larger N addition tends to inhibit CH4 uptake from the atmosphere to the soil (Aronson and Helliker, 2010; Deng et al., 2020). The exact effect of long-term N addition on CH4 fluxes, therefore, is largely uncertain. Additionally, there may be complex interactions between N addition and precipitation pulses that affect CH4 fluxes. The CH4 fluxes response to the interaction effects on precipitation pulses and long-term N addition remains largely unknown.
In this study, the responses of CH4 fluxes to precipitation pulses of different sizes and long-term N addition were assessed in a semi-arid meadow steppe. The objectives were: (1) to assess the effects of precipitation pulses on the dynamic change and patterns on CH4 fluxes; (2) to examine the effects of long-term N addition on CH4 fluxes; and (3) to examine the interactive effects of precipitation pulses and N addition on CH4 fluxes. It was hypothesized that (1) the precipitation pulses would have a negative pulse effect on CH4 fluxes and shift the ecosystem from a CH4 sink to a source; (2) precipitation pulses and long-term N addition would both suppress CH4 uptake; and (3) their interaction would synergistically suppress CH4 uptake.
Materials and methods
Site description
The study site was located at the Jilin Songnen Grassland Ecosystem National Observation and Research Station at the Changling Horse Breeding Farm in western Jilin province in northeastern China (44°34′25″N, 123°31′6″E; 138-176 m above sea level). The study area has a semi-arid temperate continental monsoon climate. In the past 65 years (1953-2017), average annual air temperatures ranged from 3.40°C to 7.58°C, with an average value of 5.6°C (National Meteorological Information Center). The average annual precipitation is 445 mm, with more than 80% occurring during the growing season (1953-2017, National Meteorological Information Center). The percentage distributions of precipitation pulses of different sizes and their contributions to total precipitation during the growing season over the past 65 years are shown in Table S1. Although precipitation pulses smaller than 5 mm were frequent (63%), they only accounted for 13.01% of the total precipitation during the growing season. In contrast, extreme pulses above 50 mm only had a frequency of 1.38%, but their contribution to the total precipitation (13.40%) was comparable to pulses below 5 mm in size.
The vegetation of the studied meadow steppe is dominated by Leymus chinensis. The zonal soil at the study site is classified as Salic Solonetz (World Reference Base for Soil Resources) or an Aqui-Alkalic Halosol (Chinese soil classification) (Ren et al, 2019; Shi et al., 2019). The soil is saline-alkaline with a pH value of 8.0-10.0 (Cui et al., 2021). Table S2 shows the soil’s chemical and physical properties at 0-10 cm depth as measured in the control and long-term N addition plots before the precipitation pulse treatments were applied.
Experimental design
In 2010, an area of 100 m × 100 m was fenced off in the L. chinensis meadow steppe, prohibiting grazing and mowing. In 2011, a long-term N addition experimental platform was established in the fenced area. Five blocks were set up in the experimental region, each with an area of 20 m × 10 m. Each block was then divided into two plots, each with an area of 10 m × 10 m. One plot was randomly assigned to N addition (10 g N m-2 yr-1) in each block, and the other unfertilized plot served as the control. In the long-term N addition plots, urea was applied every year in May and July at 5 g N m-2. In early May 2020, the intact soil columns were collected by the soil column collector (external diameter = 30.3 cm, height = 50 cm). The collector rotated and cut into 40 cm of the soil, and then the intact soil columns were collected and placed in the pots (internal diameter = 30.3 cm, height = 45 cm). Ten intact soil mesocosms (height = 40 cm) were collected from each plot (n = 100). Half of the soil mesocosms (n = 50) were used for CH4 flux measurement, and the other half (n = 50) were used for soil sampling. The collected soil mesocosms were placed in five blocks in the rainout shelter at the research station, with each block containing 20 mesocosms (ten control (CK) and ten N addition (NA) mesocosms). The mesocosms were buried 40 cm underground to reduce environmental interference and were watered to restore plant growth. From the middle of June to July 10th, the mesocosms were watered once a week with 1.44 L water (equal to a 20 mm precipitation pulse) to keep consistent soil water status. At the beginning of both May and July, urea was added at 5 g N m-2 to the surface of the N addition mesocosms. The precipitation pulses were conducted on July 31st. Based on the 65 years of historical precipitation in the study site (Table S1), five precipitation pulse sizes (0 mm, 5 mm, 10 mm, 20 mm, and 50 mm) were designed and applied randomly to a pair of the control (P0, P5, P10, P20, and P50) and N addition mesocosms (NP0, NP5, NP10, NP20, and NP50) in each block. The water was evenly sprayed into the P0 (0 L), P5 (0.36 L), P10 (0.72 L), P20 (1.44 L), and P50 (3.6 L) treatments using the sprayer in both control and N addition mesocosms. The CH4 fluxes and soil samples were collected immediately after watering (0 h), as well as at 2, 4, 8, 12, 24, 72, 144, 288, and 432 h after the precipitation pulse treatments.
Measurement of CH4 fluxes
In June 2020, the open base collar (20 cm × 20 cm × 10 cm high), with a U-shaped groove (2.5 cm in width) around the upper edge, was permanently inserted into the soil of each mesocosm before the precipitation pulse experiment. The open-bottom chamber was tightly fitted to the collar during the gas sampling and sealed with water. The gas samples were collected from inside the chambers using a 100 mL plastic syringe fitted with three-way stopcocks at 0 min, 30 min, and 60 min after the chamber closure. The collected gas samples were immediately transferred to vacuumed gas sampling bags (LB-301, Dalian Delin Gas Packing Co., Ltd, Dalian, China). The concentrations of CH4 were analyzed within one week using the N2O/CH4 analyzer (Model 913-1054, Los Gatos Research Inc., Mountain View, CA, USA).
Soil sampling and measurements
During each gas sampling occasion, soil temperature was measured and soil samples were collected. At the end of the precipitation pulses, aboveground vegetation in the pots was clipped to estimate aboveground biomass. Soil temperature was measured at a 5 cm layer of each soil mesocosm using a thermocouple penetration probe (Li 6400-09 TC, Li-cor Biosciences, Lincoln, NE, USA). Soil samples were taken at 0-10 cm depth of soil mesocosm using a stainless-steel corer (inner diameter of 3.3 cm). The soil samples were placed in sterile bags, transported to the laboratory with a cooler box, and stored at 4°C for subsequent analysis. The fresh soil samples were sieved using 2 mm mesh and divided into two subsamples in the laboratory. Fresh soil subsamples were analyzed for ammonium nitrogen (NH4+-N), nitrate nitrogen (NO3–-N), dissolved organic carbon (DOC), and microbial biomass carbon (MBC). The other subsamples were air-dried to determine pH and total carbon content (TC). The soil temperature, soil moisture, NH4+-N content, NO3–-N content, and pH value were measured on each sampling campaign, and the DOC, MBC, and TC were measured at the end (432 h) of precipitation pulse treatments.
The soil moisture was determined by the oven-drying method. The pH values of the soils were measured in a 1:2.5 (soil: water ratio) suspension with a PHS-3E glass pH electrode (PHS-3E, Shanghai Precision & Scientific Instrument Co., Ltd, Shanghai, China). Soil NH4+-N and NO3–-N content were extracted using 2 mol L-1 KCl solution by shaking for 1 h before being analyzed using a Lachat flow-injection auto-analyzer (Futura Flow Analyser, Alliance Instruments, Frepillon, France). TC was analyzed with an elemental analyzer (Vario Max CN, Elementar, Hanau, Germany). MBC was determined by the chloroform fumigation-extraction method (Vance et al., 1987). Extractable organic C in the fumigated and unfumigated samples was measured using an elemental analyzer. The MBC was calculated as the differences in DOC in the soil extracts between the fumigated and unfumigated samples. The amount of organic carbon in the un-fumigated soil extracts was used as DOC (Zhao et al., 2016). The AGB was determined by oven-drying, and harvested biomass was oven-dried at 65°C to a constant mass when weighed.
Statistical analysis
The CH4 fluxes were calculated from the change in CH4 concentrations with time (Eq. S1). Cumulative CH4 fluxes were linearly and sequentially accumulated from the fluxes between every two adjacent measurement intervals (Eq. S2). The impact-treatment is the relative effects of precipitation pulses of different sizes on CH4 fluxes (average or cumulative) from 0 mm pulse (Eq. S3).
Two-way ANOVA was conducted to examine the effects of precipitation pulses, N addition, and their interactions on CH4 fluxes as well as biotic and abiotic factors. Multiple comparisons were determined using Tukey’s HSD test at a probability level of 95% (P < 0.05). The correlations between CH4 fluxes (average and cumulative) and average soil moisture were tested using a linear model. Binary linear functions were used to test the dependence of CH4 fluxes on soil moisture and soil temperature in each treatment. A quadratic equation was developed to describe the relationship between CH4 fluxes (average and cumulative) and precipitation pulse sizes. The structural equation model (SEM) was performed to analyze the direct and indirect effects of precipitation pulse and N addition on cumulative CH4 fluxes using the lavaan package (Rosseel, 2012). The chi-square (χ2) test (P > 0.05), comparative fit index (CFI) > 0.9, and standardized root mean-square-residual (SRMR) value < 0.08 were used to indicate if the SEM models fit well. Statistical analyses were conducted using R Statistical Software (Version 4.1.2, R Corporation, Vienna, Austria) and IBM SPSS Statistics (IBM SPSS Statistics 25.0, IBM Corporation, Chicago, IL, USA). Results were presented as mean ± 1 standard error (SE). The graphics were drawn using OriginPro 2018 software (OriginPro 2018, OriginLab Corporation, Northampton, MA, USA).
Results
Effects of precipitation pulses and N addition on biotic and abiotic factors
The precipitation pulse (PP) treatments caused an immediate increase in soil moisture that was related to the PP sizes (Figure 1A). Soil moisture peaked at around 2-4 h and then decreased until the end of precipitation pulse treatments. The PP treatments of 5 mm, 10 mm, 20 mm, and 50 mm significantly enhanced the average soil moisture (P = 0.000, df = 4; Figure 1B), which quadratically increased with PP size in both control and N addition treatments (all P = 0.000, df = 24; Figure S1). N addition (NA) as well as the interaction of PP and NA, however, did not substantially alter the average soil moisture (Figure 1B). Soil temperatures had significant temporal dynamics in the P0 and NP0 treatments, showing a unimodal trend (Figure 1C). PP, NA, and their interaction, however, had no significant effect on the temporal dynamics and average soil temperature (Figure 1C, D).
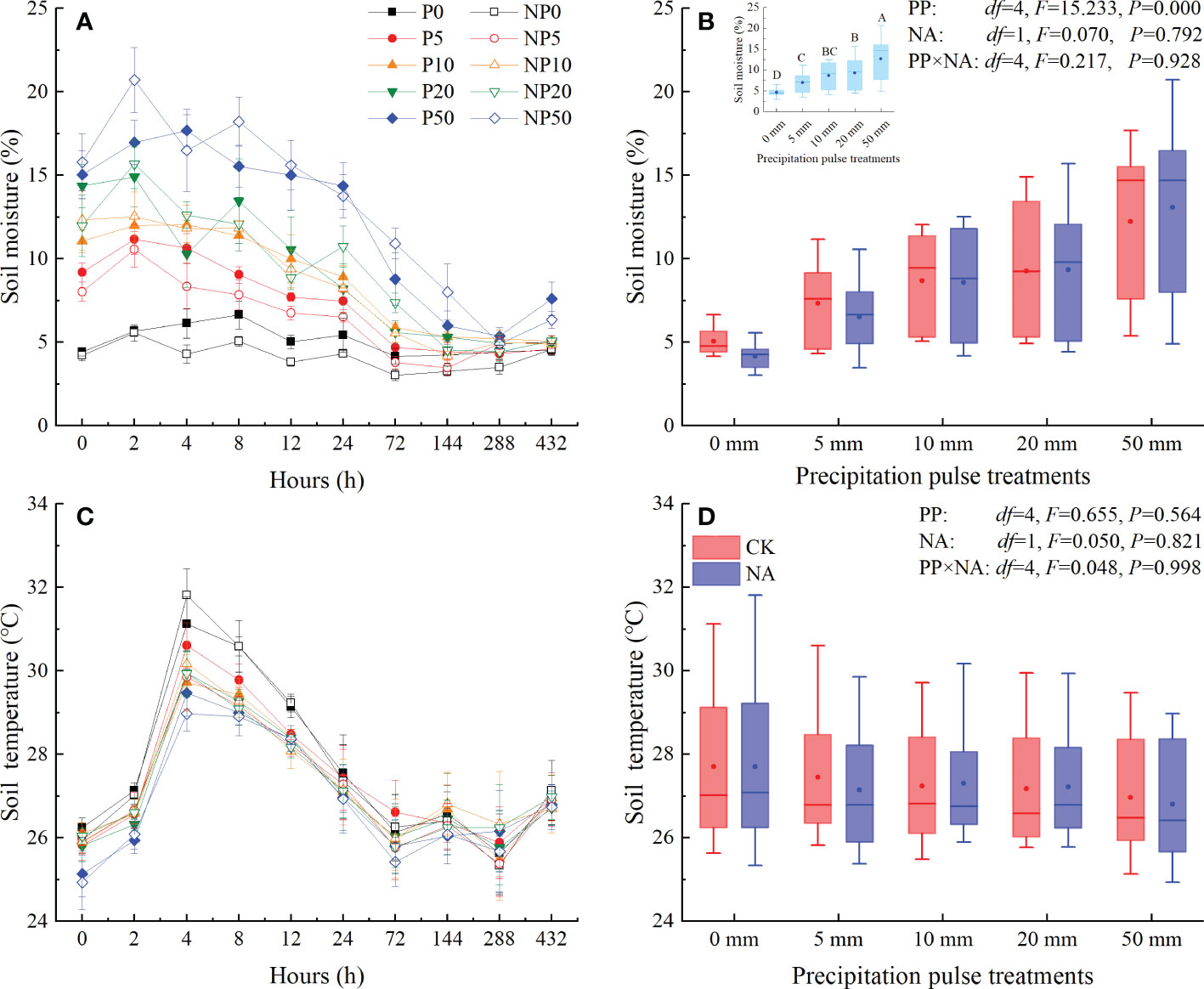
Figure 1 Responses of soil moisture at 0-10 cm depth ((A): temporal dynamic; (B): average soil moisture) and soil temperature at 5 cm depth ((C): temporal dynamic; (D)) average soil temperature) to precipitation pulses and N addition treatments. The inserted graph in panel B (light blue column) shows the differences in average soil moisture among the precipitation pulses. Boxplots show the median (lines within the box) and interquartile range (box boundaries). Whiskers extend to the most extreme data point within 1.5 × (75-25%) data range. The solid point represents the mean value. Different capital letters (above each boxplot) denote significant differences among the precipitation pulses.
The PP treatments significantly affected the temporal dynamics of NH4+-N content (Figure 2A), NO3–-N content (Figure 2C), and pH value (Figure 2E). The peaks of NH4+-N content, NO3–-N content, and pH mainly occurred at 0-8 h, 2-12 h, and 24-144 h after the PP treatments, respectively. All sizes of PP significantly increased average NO3–-N content (P = 0.000, df = 4; Figure 2D) but not average NH4+-N content (Figure 2B). The 20 mm and 50 mm PP treatments significantly increased the pH, while the 5 mm PP treatment significantly decreased the pH (P = 0.000, df = 4; Figure 2F). NA significantly increased NH4+-N content (P = 0.000, df = 1; Figure 2B) and NO3–-N content (P = 0.000, df = 1; Figure 2D), but significantly decreased the pH (P = 0.000, df = 1; Figure 2F). The interaction of PP and NA significantly affected the pH (P = 0.000, df = 4; Figure 2F) but had no significant effects on NH4+-N content (Figure 2B) or NO3–-N content (Figure 2D).
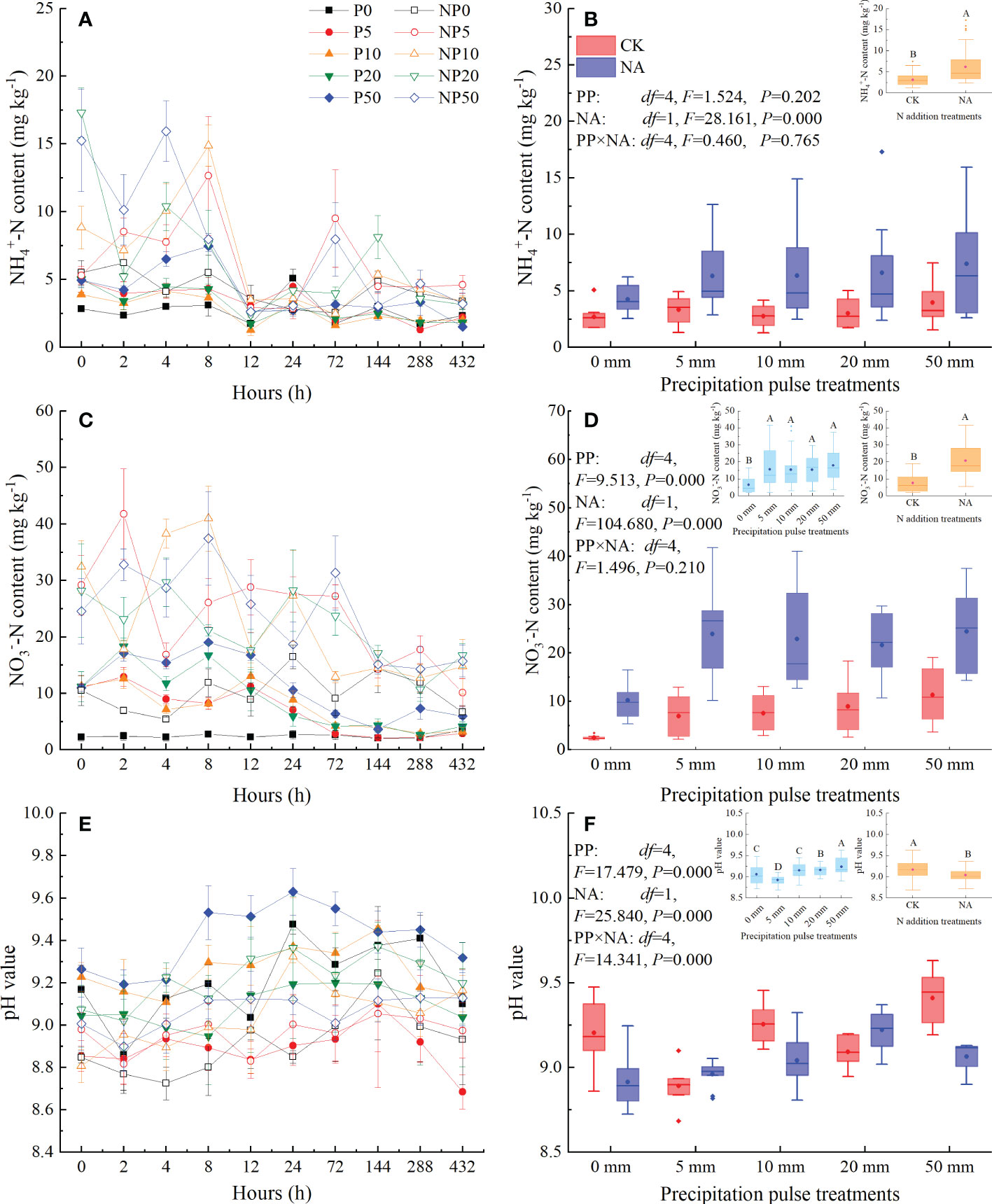
Figure 2 Responses of NH4+-N content ((A): temporal dynamic; (B): average NH4+-N content), NO3−-N content ((C): temporal dynamic; (D): average NO3−-N content), and pH value ((E): temporal dynamic; (F): average pH value) at 0-10 cm depth to precipitation pulses and N addition treatments. The inserted graphs (orange column) in panels B, D, and F show the differences in average NH4+-N content, average NO3−-N content, and average pH value between the control and N addition treatments. The inserted graphs (light blue column) in panels D and F show the differences in average NO3−-N content and average pH value among the precipitation pulses. Boxplots show the median (lines within the box) and interquartile range (box boundaries). Whiskers extend to the most extreme data point within 1.5 × (75-25%) data range. The solid point represents the mean value. Different capital letters (above each boxplot) denote significant differences among the precipitation pulses or between the control and N addition treatments.
At the end of precipitation treatment, PP treatments significantly affected the DOC content (P = 0.005, df = 4; Figure 3A), MBC content (P = 0.004, df = 4; Figure 3B), and AGB (P = 0.026, df = 4; Figure 3D), but not the TC (Figure 3C). Compared with the 0 mm PP treatment, 50 mm PP treatment significantly decreased DOC content (Figure 3A), while the 10 mm, 20 mm, and 50 mm PP treatments significantly increased MBC content and AGB (Figure 3B, D). NA significantly increased AGB (P = 0.000, df = 1; Figure 3D), but did not substantially alter DOC content (marginal effect, P = 0.085, df = 1; Figure 3A), MBC (Figure 3B), and TC (Figure 3C). The interaction of PP and NA had no significant effect on the DOC, MBC, TC, or AGB (Figure 3).
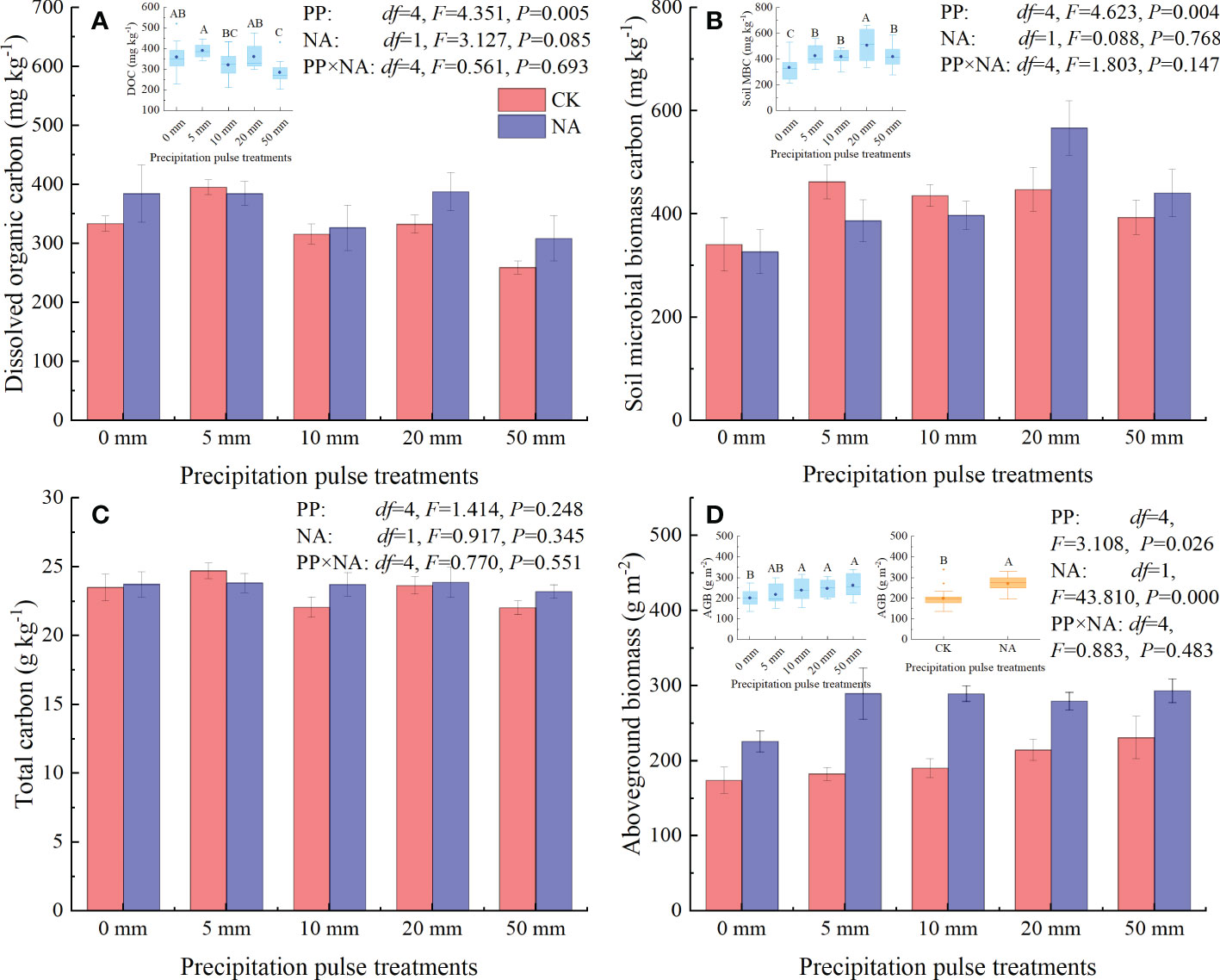
Figure 3 Responses of dissolved organic carbon (DOC, A), soil microbial biomass carbon (MBC, B), total carbon (TC, C), and aboveground biomass (AGB, D) to precipitation pulses and N addition treatments. The inserted graphs (light blue column) in panels A, B, D show the differences in DOC, MBC, and AGB among the precipitation pulses. The inserted graphs (orange column) in panel D show the differences in AGB between the control and N addition treatments. Boxplots show the median (lines within the box) and interquartile range (box boundaries). Whiskers extend to the most extreme data point within 1.5 × (75-25%) data range. The solid point represents the mean value. Different capital letters (above each boxplot) denote significant differences among the precipitation pulses treatments or between the control and N addition treatments.
Effects of precipitation pulses and N addition on CH4 fluxes
The studied grassland acted as a sink for CH4 in the P0 and NP0 treatments, with fluxes ranging from -8.22 to -3.78 μg m-2 h-1 and -6.91 to -2.57 μg m-2 h-1, respectively (Figure 4A). The 5 mm PP treatment slightly suppressed CH4 uptake and average CH4 fluxes, but not at statistically significant levels (Figures 4A, B). The 10 mm, 20 mm, and 50 mm PP treatments, however, substantially changed the temporal dynamics and the source-sink relationship of CH4 fluxes (Figure 4A). The 10 mm PP treatment shifted CH4 fluxes from sinks to sources within 2 to 4 h, while 20 mm and 50 mm PP treatments immediately triggered the release of CH4 (Figure 4A). The CH4 fluxes primarily peaked at 12 h and relatively decreased the CH4 uptake by 203-362% and 243-333% compared with P0 and NP0 treatments, respectively (Figure 4A). After that, CH4 releases decreased and absorption resumed at 144 h until the end of PP treatments (Figure 4A).
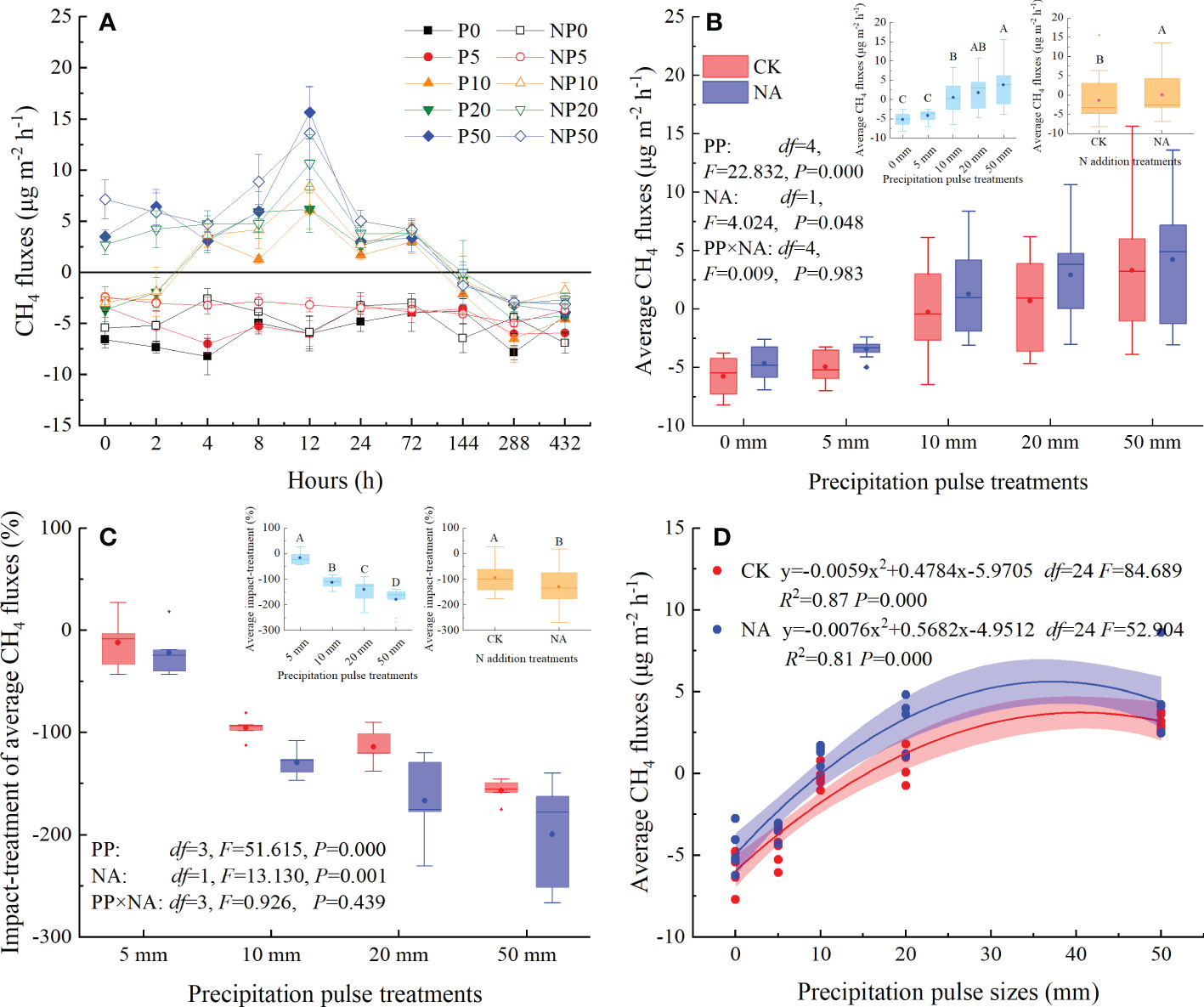
Figure 4 Responses of CH4 fluxes ((A): temporal dynamic; (B): average flux; (C): impact-treatment of average CH4 flux) to precipitation pulses and N addition treatment, and the relationship between average CH4 fluxes and precipitation pulse sizes (D). The inserted graphs (light blue column) in panels B, C show the differences in average CH4 fluxes and impact-treatment of average CH4 flux among the precipitation pulses. The inserted graphs (orange column) in panels B, C show the differences in the average CH4 fluxes between the control and the N addition treatments. Boxplots show the median (lines within the box) and interquartile range (box boundaries). Whiskers extend to the most extreme data point within 1.5 × (75-25%) data range. The solid point represents the mean value. Different capital letters (above each boxplot) denote significant differences among the precipitation pulses or between the control and N addition treatments.
The PP significantly affected the average CH4 fluxes (P = 0.000, df = 4; Figure 4B). More specifically, the 10 mm, 20 mm, and 50 mm PP treatments significantly enhanced the average CH4 fluxes (109-171%), but the 5 mm PP treatment had no significant effect (Figure 4B). The relative effect of PP treatment on CH4 fluxes increased significantly with increasing PP sizes (P = 0.000, df = 3; Figure 4C). The relationship between the average CH4 fluxes and PP sizes could be fitted by a quadratic equation, explaining the 87% (P = 0.000, df = 24) and 81% (P = 0.000, df = 24) variation in the average CH4 fluxes in the control and N addition treatments, respectively (Figure 4D). N addition significantly enhanced the average CH4 fluxes (P = 0.048, df = 1; Figure 4B) and the impact-treatment of average CH4 fluxes (P = 0.001, df = 1; Figure 4C). The interaction of PP and NA, however, had no significant influence on average CH4 fluxes (Figure 4B) or the impact-treatment of average CH4 fluxes (Figure 4C).
Cumulative CH4 fluxes showed a decreasing trend following the 0 mm and 5 mm PP treatments, as CH4 continued to be absorbed from the atmosphere by the soil (Figures 4A, 5A). The 10 mm, 20 mm, and 50 mm PP treatments significantly increased the cumulative CH4 fluxes, reaching the highest cumulative fluxes at 144 h (Figure 5A). After that, cumulative CH4 fluxes were reduced and the areas were converted into CH4 sinks. During the experimental period, cumulative CH4 fluxes ranged from -0.17 to -0.03 mg CH4 pot-1 and -0.16 to -0.02 mg CH4 pot-1 in the control and N addition treatments, respectively (Figure 5A).
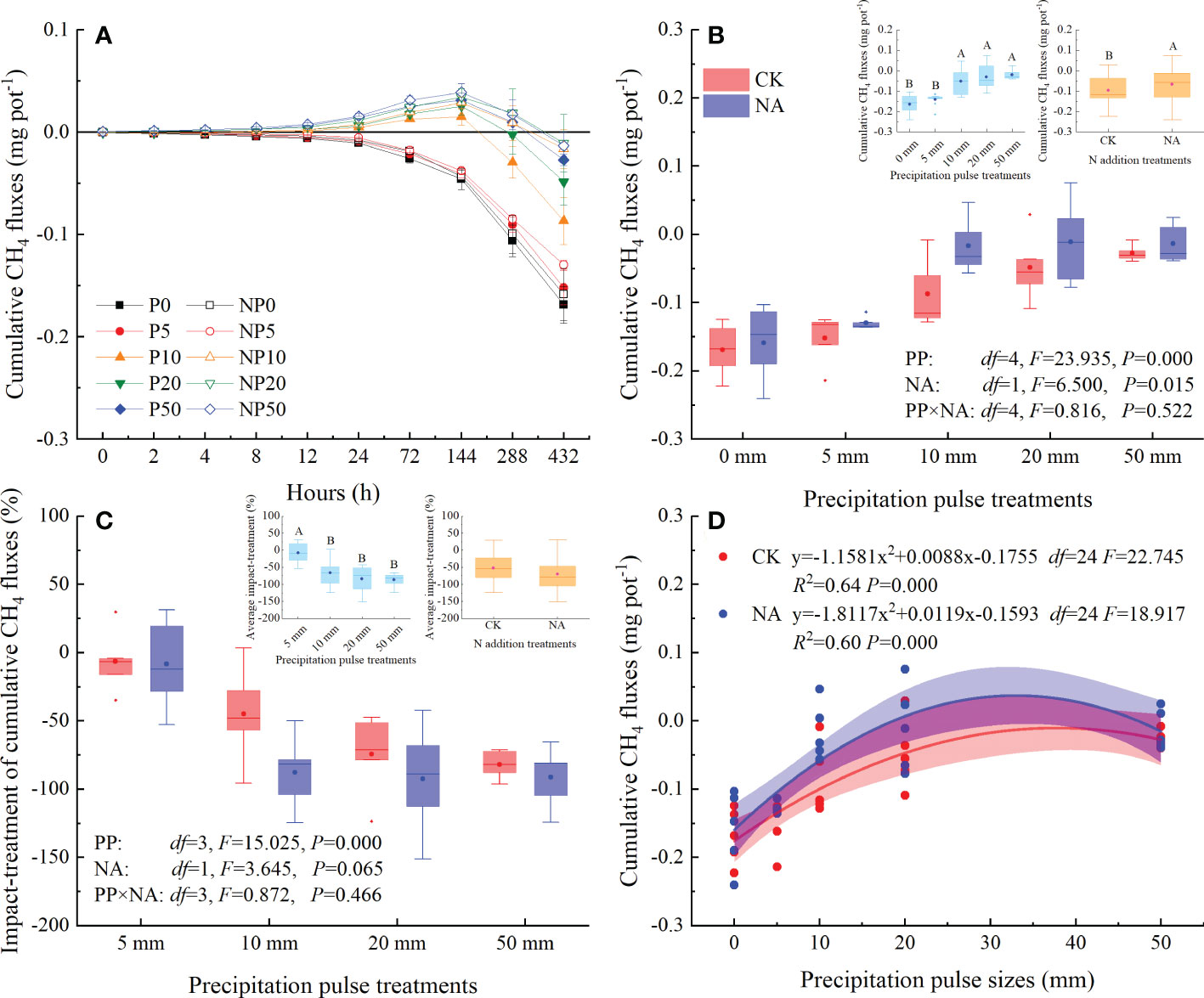
Figure 5 Responses of the cumulative CH4 fluxes ((A): temporal dynamic; (B): average cumulative fluxes; (C): impact-treatment of cumulative CH4 flux) to precipitation pulses and N addition, and the relationship between cumulative CH4 fluxes and precipitation pulse sizes (D). The inserted graphs (light blue column) in panels B, C show the differences in cumulative CH4 fluxes and impact-treatment of cumulative CH4 flux among the precipitation pulses. The inserted graph (orange column) in panel B shows the differences in cumulative CH4 fluxes between the control and N addition treatments. Boxplots show the median (lines within the box) and interquartile range (box boundaries). Whiskers extend to the most extreme data point within 1.5 × (75-25%) data range. The solid point represents the mean value. Different capital letters (above each boxplot) denote significant differences among the precipitation pulses or between the control and N addition treatments.
The PP treatments significantly affected the cumulative CH4 fluxes (P = 0.000, df = 4; Figure 5B) and the impact-treatment of cumulative CH4 fluxes (P = 0.000, df = 3; Figure 5C). The 10 mm, 20 mm, and 50 mm PP treatments significantly reduced the absorption of CH4 (68-88%), with a higher relative effect on cumulative CH4 fluxes; in contrast, the 5 mm PP treatment did not have any effect (Figure 5B, 5C). There was a significant quadratic relationship between the cumulative CH4 fluxes and PP sizes, which could explain the 64% (P = 0.000, df = 24) and 60% (P = 0.000, df = 24) variation in the cumulative CH4 fluxes in the control and N addition treatments, respectively (Figure 5D). NA significantly increased the cumulative CH4 fluxes (P = 0.015, df = 1; Figure 5B), but had a marginal effect on the impact-treatment of cumulative CH4 fluxes (P = 0.065, df = 1; Figure 5C). The interaction of PP and NA, however, did not significantly affect the cumulative CH4 fluxes (Figure 5B) and the impact-treatment of cumulative CH4 fluxes (Figure 5C).
Dependences of temporal dynamics of CH4 fluxes on soil moisture and soil temperature
The binary linear model showed that the temporal dynamics of CH4 fluxes were mainly driven by soil moisture and soil temperature after the PP treatments (Table 1). Only the P0 and NP0 treatments had no significant relationship between CH4 fluxes and soil moisture and soil temperature (Table 1). The CH4 fluxes were significantly positively correlated with soil moisture and soil temperature in the NP5 treatment (P < 0.01, df = 49), but were significantly negatively correlated with soil moisture in the P5 treatment (P < 0.05, df = 42). The CH4 fluxes from control and N addition treatments were both significantly positively correlated with soil moisture and soil temperature in the 10 mm (both P < 0.01), 20 mm (both P < 0.01), and 50 mm (both P < 0.001) PP treatments. The degree of fitting increased with increasing PP sizes, explaining the 10-34% and 18-28% change in the temporal dynamics of CH4 fluxes in the control and N addition treatments, respectively (Table 1).
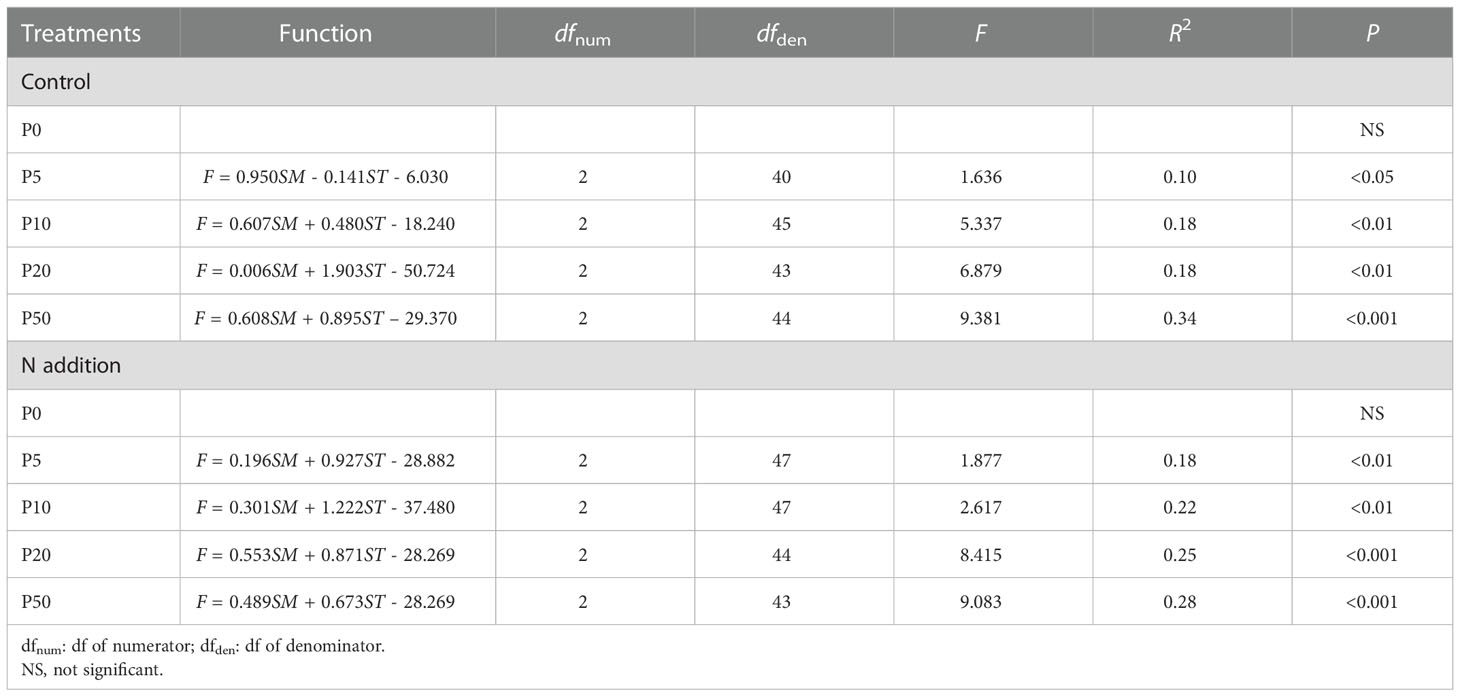
Table 1 Dependency of CH4 fluxes (F) on soil moisture (SM, 0-10 cm depth) and soil temperature (ST, 5 cm depth) after precipitation pulses and long-term N addition treatments.
Relationships between cumulative CH4 fluxes with biotic and abiotic factors
Structural equation model (SEM) analysis indicated that N addition significantly increased the NO3–-N content (P < 0.001), thereby decreasing the soil pH (P < 0.01; Figure 6). The pH change directly increased the cumulative CH4 fluxes (P < 0.05) and indirectly enhanced the MBC (P < 0.01) and DOC (P < 0.001) through decreased TC (P < 0.01). The MBC and DOC significantly increased the cumulative CH4 fluxes (both P < 0.05), while the TC had a marginal effect on cumulative CH4 fluxes (P < 0.1). The precipitation pulses significantly increased the soil moisture (P < 0.001), which directly increased the cumulative CH4 fluxes (P < 0.01). Additionally, soil moisture positively affected NO3–-N content (P < 0.001), pH (P < 0.05), and MBC (P < 0.001), but a negative effect on DOC (P < 0.01) and TC (P > 0.05); this, in turn altered the cumulative CH4 fluxes. All factors jointly explained the 41% variation in cumulative CH4 fluxes that were observed (Figure 6).
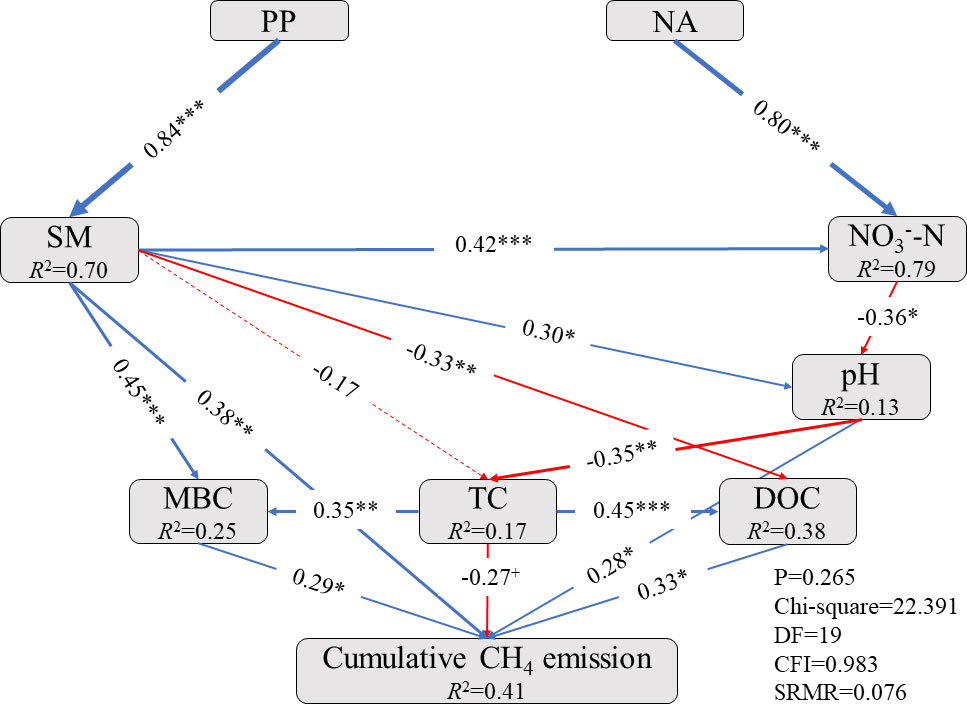
Figure 6 Structural equation model (SEM) performed to examine the direct and indirect effects of precipitation pulses and long-term N addition on cumulative CH4 fluxes. The blue arrows indicated positive effects, while the red arrows indicated negative effects. The solid arrows indicated significant paths (P < 0.05). Conversely, the dotted lines indicated insignificant paths (P > 0.05). Arrow width represented the strength of the relationship. Values associated with solid arrows represent standardized path coefficients. R2 values represent the proportion of the variance explained for each endogenous variable. Significance levels are as follows: +, P < 0.1; *, P < 0.05; **, P < 0.01; ***, P < 0.001. Goodness-of-fit statistics are shown below the model. PP, precipitation pulse; NA, N addition; SM, soil moisture; NO3–-N, NO3–-N content; pH, pH value; MBC, microbial biomass carbon; TC, total carbon; DOC, dissolved organic carbon.
Discussion
The suppression effect of precipitation pulses on cumulative CH4 fluxes
The effects of precipitation pulses on CH4 fluxes are very complex. Previous studies have shown that precipitation pulses could stimulate (Harms and Grimm, 2012; Petrakis et al., 2017), suppress (Zhao et al., 2017; Ren et al., 2019), or not significantly affect CH4 fluxes (Mariko et al., 2007; Ni et al., 2019). The effects mainly depended on the precipitation pulse size, ecosystem type, and soil moisture status (Harms and Grimm, 2012; Kim et al., 2012; Petrakis et al., 2017; Zhao et al., 2017; Ni et al., 2019). Without manipulated precipitation, the studied grassland acted as a net sink of CH4 (Figures 4A, 5A), which is consistent with the findings of previous studies (Dalal and Allen, 2008; Wang et al., 2014b; Yu et al., 2017; Zhao et al., 2017). The average rate of CH4 absorption of the studied grassland (-5.23 ± 0.36 μg m-2 h-1, Figure 4B) was, however, much lower than that of the semi-arid grassland from northeast China (-74.31 ± 62.51 μg m-2 h-1) as well as the Qinghai-Tibetan Plateau (-31.29 ± 21.78 μg m-2 h-1) (Wang et al., 2014b). As expected, the large (10 mm, 20 mm, and 50 mm) precipitation pulses had a negative pulsing effect on CH4 fluxes and significantly suppressed CH4 absorption, but the small (5 mm) precipitation pulse did not significantly alter it (Figure 5B).
Without water supplementation (0 mm PP treatment), the soil was arid and soil moisture was relatively stable (Figure 1A). Low soil moisture limited the activity of methanotrophs due to water stress, and the absorption rate of CH4 was, therefore, very low (Le Mer and Roger, 2001; Borken et al., 2006; Aronson et al., 2019). Although the 5 mm PP treatment substantially increased average soil moisture (Figure 1B), it did not significantly alter the temporal dynamics or cumulative CH4 fluxes (Figures 4A, 5B). Ni et al. (2019) also confirmed that short-term precipitation pulses did not alter CH4 fluxes in a forest ecosystem, suggesting that small precipitation changes do not alter O2 in soil pore spaces enough to affect CH4 fluxes. As expected, the large precipitation pulses (10 mm, 20 mm, and 50 mm) altered the source-sink relationship of CH4, converting CH4 fluxes of the studied grassland from a sink to a source (Figures 4A, 5A), which were consistent with an in situ field study (Ren et al., 2019). The temporal dynamics of CH4 fluxes were controlled by the soil moisture and soil temperature following the precipitation pulses (Table 1). While the precipitation pulses significantly increased soil moisture, they did not alter soil temperature (Figure 1). The changes in soil moisture dynamics caused by precipitation pulses, therefore, may be responsible for the changes in CH4 fluxes. In this study, there were several potential mechanisms by which precipitation pulses could have triggered the CH4 source-sink conversion. Firstly, the infiltration of soil water caused by the precipitation pulses could displace CH4 trapped in the soil pore space and release it to the atmosphere, especially in the case of the 50 mm PP treatment. Secondly, a large precipitation pulse could increase the soil water availability and alleviate water limitation, decreasing the redox potential and availability of O2 in favor of anaerobic processes, thereby promoting methanogenic activity and suppressing CH4 oxidation (Harms and Grimm, 2012; Kim et al., 2012). Thirdly, the increased availability of water could stimulate microbial, specifically methanogen, biomass (Huang et al., 2015; Venturini et al., 2022). Fourthly, the availability of substrates before PP treatments was accumulated through microbial metabolism, soil aggregates shattering, and organisms death, which would be rapidly utilized by methanogen under these increased soil moisture conditions (Unger et al., 2010; Harms and Grimm, 2012; Kim et al., 2012; Lado-Monserrat et al., 2014). Though precipitation pulses break the balance between the CH4 production and consumption and change the source-sink relationship of CH4 fluxes through physical and biological processes (Figure 4A), they cannot permanently change the nature of grassland as a CH4 sink (Figure 5B). In the context of global change, however, it appears that the sink strength of grassland ecosystems will decrease with the increase of heavy precipitation pulses in the future (Zhao et al., 2017; Ren et al., 2019).
For the first time it was demonstrated that cumulative CH4 fluxes increased quadratically with precipitation pulse size in both the control and N addition treatments (Figure 5D). These results further suggest that precipitation pulses suppress CH4 uptake by controlling soil moisture (Figures 5B, 7B, S1). Average soil moisture in the 0-10 cm layer quadratically increased with precipitation pulse size after precipitation treatments (all P = 0.000, df = 24; Figure S1). Contrary to expectations, the optimal relationship between soil moisture and precipitation pulse size was not linear. Extreme precipitation pulses (20 mm and 50 mm) caused soil moisture to penetrate deeper and significantly increased average soil moisture in the 10-30 cm soil layer (P = 0.000, df = 4; Figure S2). The 10 mm, 20 mm, and 50 mm precipitation pulses significantly increased aboveground biomass (Figure 3D), which then also consumed a large amount of soil water. The increased aboveground biomass can also increase soil water by reducing soil evaporation, but the effect is largely unknown. Soil moisture infiltration and plant growth together decreased soil moisture in the 0-10 cm soil layer when larger precipitation pulse treatments were applied, resulting in a quadratic increase in average soil moisture with precipitation pulse size. Cumulative CH4 fluxes increased linearly with average soil moisture due to the different responses of gas diffusion and activity of microbe to the increased soil moisture (Figure 7). The small precipitation pulses did not alter soil water contents and O2 concentrations enough to affect the CH4 oxidation environment (Ni et al., 2019). The large precipitation pulses significantly inhibited the soil gases diffusion participating in CH4 oxidation and suppressed CH4 oxidation (Liu et al., 2008; Zhao et al., 2017). At the same time, the large precipitation pulses decreased the redox potential, created a saturated soil condition and lasted for a few days, which were conducive to methanogenesis (Harms and Grimm, 2012; Decock and Six, 2013; Petrakis et al., 2017). This study saw the release of CH4 from the soil to the atmosphere under these conditions (Figure 4A), confirming that large precipitation pulses (10 mm, 20 mm, and 50 mm) stimulated the activity of methanogens (Ren et al., 2019). The decreased soil gases diffusion participating in CH4 oxidation and increased activity of methanogens, therefore, led to a decrease in CH4 uptake with increasing soil moisture. Ecologists have confirmed that CH4 uptake decrease with increasing soil moisture in grassland ecosystems (Jiang et al., 2010; Shrestha et al., 2012; Liu et al., 2021a; Wu et al., 2021). In conclusion, precipitation pulses suppressed CH4 uptake by increasing soil moisture and exhibited a quadratic relationship with the cumulative CH4 fluxes.
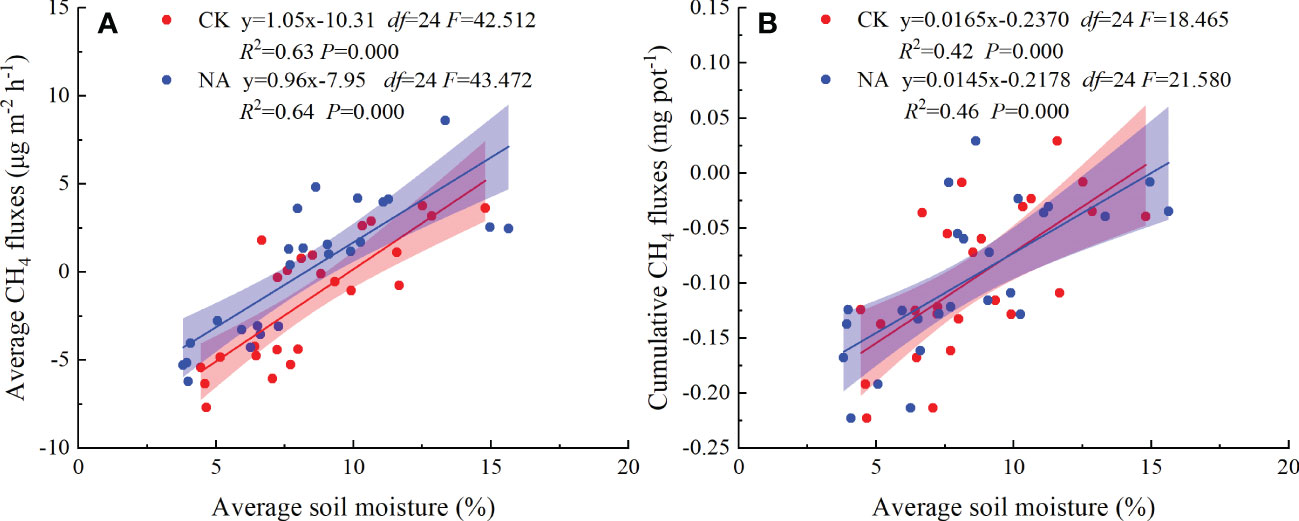
Figure 7 The relationship between CH4 fluxes ((A): average fluxes; (B): cumulative fluxes) and average soil moisture after the precipitation pulses and long-term N addition treatments.
The suppression effect of long-term N addition on cumulative CH4 fluxes
Effects of N addition on CH4 fluxes have been extensively studied in many ecosystems (Aronson and Helliker, 2010; Deng et al., 2020; Wu et al., 2022). As expected, N addition significantly suppressed the absorption of CH4 in the studied grassland ecosystem (Figures 4B, 5B), consistent with the results of the meta-analyses (Aronson and Helliker, 2010). In the present study, N addition significantly increased the NH4+-N content (Figure 2B) and NO3–N content (Figure 2D), along with aboveground biomass (Figure 3D), while significantly decreasing the pH value (Figure 2E). N addition, therefore, could decrease the absorption of CH4 or increase the production of CH4 through changing amounts of NH4+-N, NO3–-N, and AGB, as well as altering pH value, suppressing CH4 uptake (Fang et al., 2014; Yang et al., 2017; Chen et al., 2019; Kong et al., 2019; Ren et al., 2019).
There are multiple underlying mechanisms for N addition suppressing CH4 uptake. First, the increased NH4+-N competes with CH4 for methane monooxygenase (MMO), which decreased the combination point of MMO to CH4, thereby reducing the oxidation of CH4 (Schnell and King, 1994). Second, NH4+-N is oxidized to hydroxylamine (NH2OH) and nitrite (NO2--N) by CH4 monooxygenase or ammonia-oxidizing microorganisms, which has a toxic effect on methanotrophs (Bodelier, 2011). Third, the NH4+-N and NO3–-N content in the N addition treatment were 1.96 and 2.77 times greater than that of the unfertilized treatment, respectively (Figure 2D), resulting in osmotic stress and suppressing the activity of methanotrophs (Bodelier and Laanbroek, 2004; Saari et al., 2004; Yang et al., 2017). Four, the increased AGB could allocate more C to promote root exudates, which would improve substrate availability for methanogens (Waldo et al., 2019). Additionally, N addition could enhance litter mass input to soil and nutrient return from litter decomposition by increasing AGB, thereby alleviating the C limitation on methanogens (Gong et al., 2015; Ren et al., 2019). As a result, methanogens enhanced the CH4 production, which in turn offset the absorption of CH4 and suppressed CH4 uptake (Ren et al., 2019). It was found that cumulative CH4 fluxes were marginally significant positively correlated with the changes in average NH4+-N (P = 0.114, df = 49; Figure S3A) and NO3–-N content (P= 0.077, df = 49; Figure S3B), as well as AGB (P= 0.074, df = 49; Figure S3C). This implies that N addition could decrease the absorption of CH4 by increasing NH4+-N content and NO3–-N content, and/or increase the production of CH4 by increasing AGB, ultimately suppressing CH4 uptake.
N addition significantly reduced soil pH value by 0.13 units (Figure 2F), half of the average global level for terrestrial ecosystems (Tian and Niu, 2015). A significant positive correlation between cumulative CH4 fluxes and pH value was detected (P= 0.042, df=49; Figure S3D), consistent with the findings of Ren et al. (2019). Contrary to expectations, the decreased pH enhanced CH4 uptake in saline-alkaline soils, possibly due to reduced pH alleviating the physiological stress of saline-alkaline conditions on methanotrophs. In summary, N addition suppressed CH4 uptake not by reducing pH value, but by increasing NH4+-N content, NO3–-N content, and AGB.
No interactive effect of precipitation pulses and N addition on cumulative CH4 fluxes
Both the precipitation pulses and N addition significantly suppressed CH4 uptake (Figures 4B, 5B). In contrast, the precipitation pulses and N addition together had no interactive effect on CH4 uptake (Figures 4B, 5B). Several potential mechanisms could explain this result. First, cumulative CH4 emissions after precipitation pulses were significantly affected by soil moisture (P < 0.001, df = 49), pH (P < 0.001, df = 49), and DOC (P < 0.05, df = 49; Figure S4). There were no significant interactive effects between precipitation pulses and N addition on soil moisture (Figure 1B) and DOC (Figure 3A). The precipitation pulses and N addition had an interactive effect on the pH (Figure 2F). The pH value, however, had opposite responses to precipitation pulses and N addition (Figure 2F), as precipitation pulses significantly increased the pH, whereas N addition significantly decreased it (Figure 2F). Precipitation pulses and N addition, therefore, could not interactively affect CH4 uptake by interactively affecting soil moisture, pH, and DOC. Second, precipitation pulses suppressed CH4 uptake by increasing soil moisture, whereas N addition suppressed CH4 uptake by increasing NH4+-N, NO3–-N, and AGB. Precipitation pulses and N addition inhibited the absorption of CH4 through different pathways. Third, the structural equation model showed that pH value was a key factor in precipitation pulses, and N addition interactively affected CH4 fluxes. Precipitation pulses significantly increased pH by increasing soil moisture, while N addition decreased pH through increasing NO3–-N content (Figure 6). The precipitation pulses and N addition together, therefore, had opposite effects on pH. Additionally, N addition decreased cumulative CH4 fluxes by decreasing pH (total correlation coefficient: 0.100), whereas the precipitation pulses increased cumulative CH4 fluxes by increasing soil moisture (Figure 6). Precipitation pulses and N addition together, therefore, had an offset effect on cumulative CH4 fluxes, rather than a synergistic suppressing effect. In summary, there were no interactive effects of precipitation pulses and N addition on cumulative CH4 fluxes.
Conclusions
This study evaluated the effects of precipitation pulses, N addition, and their interactions on CH4 fluxes as well as examined their driving mechanisms in a semi-arid meadow steppe in Northeast China. Both precipitation pulses and N addition significantly suppressed CH4 uptake. Precipitation pulses significantly altered the temporal dynamics of soil moisture, resulting in a negative pulse effect on CH4 fluxes and shifting the grassland ecosystem from a CH4 sink to a source. The cumulative CH4 fluxes increased quadratically with precipitation pulse sizes in both control and N addition treatments. N addition possibly decreases the absorption of CH4 by increasing NH4+-N content and NO3–-N content, or increases the production of CH4 by increasing aboveground biomass, ultimately inhibiting CH4 uptake. The plants could influence the response of CH4 fluxes to precipitation pulses and N addition by regulating water and substrate availability. Surprisingly, precipitation pulses and N addition had no interactive effects on CH4 fluxes because precipitation pulses and N addition had an offset effect on the key factor (pH) and affected CH4 fluxes through different pathways. The interactive effects between precipitation and N addition on CH4 fluxes should be further investigated in the future.
Data availability statement
The original contributions presented in the study are included in the article/Supplementary Material. Further inquiries can be directed to the corresponding authors.
Author contributions
WG: Conceptualization, Investigation, Formal analysis, Visualization, Writing – original draft, Writing – review and editing. XY: Conceptualization, Investigation, Formal analysis, Visualization, Writing – original draft, Writing – review and editing. YZ: Formal analysis, Writing – review and editing. TZ: Conceptualization, Investigation, Formal analysis, Visualization, Writing – original draft. BS: Formal analysis, Writing – original draft, Writing – review and editing. TY: Formal analysis, Visualization, Writing – review and editing. JM: Conceptualization, Formal analysis, Writing – review and editing. WX: Formal analysis, Visualization, Writing – original draft. YW: Formal analysis, Visualization, Writing – review and editing. WS: Conceptualization, Funding acquisition, Supervision, Writing – original draft, Writing – review and editing. All authors contributed to the article and approved the submitted version.
Funding
This study was financially supported by the National Natural Science Foundation of China (32001183, 31870456, 32071627, 32001182), the Program of Introducing Talents of Discipline to Universities (B16011), China Postdoctoral Science Foundation (2021M700743), the Science and Technology Project of the Jilin Provincial Education Department (JJKH20221170KJ), and the Fundamental Research Funds for the Central Universities (2412022XK005, 2412020QD019).
Acknowledgments
We greatly thank Shicheng Jiang, Xiuquan Yue, and Yanan Li for their help in laboratory analyses.
Conflict of interest
The authors declare that the research was conducted in the absence of any commercial or financial relationships that could be construed as a potential conflict of interest.
Publisher’s note
All claims expressed in this article are solely those of the authors and do not necessarily represent those of their affiliated organizations, or those of the publisher, the editors and the reviewers. Any product that may be evaluated in this article, or claim that may be made by its manufacturer, is not guaranteed or endorsed by the publisher.
Supplementary material
The Supplementary Material for this article can be found online at: https://www.frontiersin.org/articles/10.3389/fpls.2022.1071511/full#supplementary-material
References
Aronson, E. L., Goulden, M. L., Allison, S. D. (2019). Greenhouse gas fluxes under drought and nitrogen addition in a southern California grassland. Soil Biol. Biochem. 131, 19–27. doi: 10.1016/j.soilbio.2018.12.010
Aronson, E. L., Helliker, B. R. (2010). Methane flux in non-wetland soils in response to nitrogen addition: a meta-analysis. Ecology 91, 3242–3251. doi: 10.1890/09-2185.1
Bichet, A., Wild, M., Folini, D., Schär, C. (2011). Global precipitation response to changing forcings since 1870. Atmo. Chem. Phys. 11, 9961–9970. doi: 10.5194/acp-11-9961-2011
Billings, S. A., Richter, D. D., Yarie, J. (2000). Sensitivity of soil methane fluxes to reduced precipitation in boreal forest soils. Soil Biol. Biochem. 32, 1431–1441. doi: 10.1016/s0038-0717(00)00061-4
Bodelier, P. L. E. (2011). Interactions between nitrogenous fertilizers and methane cycling in wetland and upland soils. Curr. Opin. Env. Sust. 3, 379–388. doi: 10.1016/j.cosust.2011.06.002
Bodelier, P. L. E., Laanbroek, H. J. (2004). Nitrogen as a regulatory factor of methane oxidation in soils and sediments. FEMS Microbiol. Ecol. 47, 265–277. doi: 10.1016/S0168-6496(03)00304-0
Borken, W., Davidson, E. A., Savage, K., Sundquist, E. T., Steudler, P. (2006). Effect of summer throughfall exclusion, summer drought, and winter snow cover on methane fluxes in a temperate forest soil. Soil Biol. Biochem. 38, 1388–1395. doi: 10.1016/j.soilbio.2005.10.011
Boucher, O., Friedlingstein, P., Collins, B., Shine, K. P. (2009). The indirect global warming potential and global temperature change potential due to methane oxidation. Environ. Res. Lett. 4, 44007. doi: 10.1088/1748-9326/4/4/044007
Chen, S., Hao, T., Goulding, K., Misselbrook, T., Liu, X. (2019). Impact of 13-years of nitrogen addition on nitrous oxide and methane fluxes and ecosystem respiration in a temperate grassland. Environ. pollut. 252, 675–681. doi: 10.1016/j.envpol.2019.03.069
Chen, S., Lin, G., Huang, J., Jenerette, G. D. (2009). Dependence of carbon sequestration on the differential responses of ecosystem photosynthesis and respiration to rain pulses in a semiarid steppe. Glob. Change Biol. 15, 2450–2461. doi: 10.1111/j.1365-2486.2009.01879.x
Chen, W., Zheng, X., Chen, Q., Wolf, B., Butterbach-Bahl, K., Brueggemann, N., et al. (2013). Effects of increasing precipitation and nitrogen deposition on CH4 and N2O fluxes and ecosystem respiration in a degraded steppe in inner Mongolia, China. Geoderma 192, 335–340. doi: 10.1016/j.geoderma.2012.08.018
Conrad, R., Chan, O. C., Claus, P., Casper, P. (2007). Characterization of methanogenic archaea and stable isotope fractionation during methane production in the profundal sediment of an oligotrophic lake (Lake stechlin, Germany). Limnol. Oceanogr. 52, 1393–1406. doi: 10.4319/lo.2007.52.4.1393
Cui, H., Sun, W., Delgado-Baquerizo, M., Song, W., Ma, J. Y., Wang, K., et al. (2021). Cascading effects of n fertilization activate biologically driven mechanisms promoting P availability in a semi-arid grassland ecosystem. Funct. Ecol. 35, 1001–1011. doi: 10.1111/1365-2435.13773
Dalal, R. C., Allen, D. E. (2008). Greenhouse gas fluxes from natural ecosystems. Aust. J. Bot. 56, 369–407. doi: 10.1071/bt07128
Decock, C., Six, J. (2013). An assessment of N-cycling and sources of N2O during a simulated rain event using natural abundance 15N. Agric. Ecosyst. Environ. 165, 141–150. doi: 10.1016/j.agee.2012.11.012
Deng, L., Huang, C., Dong-Gill, K., Shangguan, Z., Wang, K., Song, X., et al. (2020). Soil GHG fluxes are altered by N deposition: New data indicate lower N stimulation of the N2O flux and greater stimulation of the calculated C pools. Glob. Change Biol. 26, 2613–2619. doi: 10.1111/gcb.14970
Fang, H., Cheng, S., Yu, G., Cooch, J., Wang, Y., Xu, M., et al. (2014). Low-level nitrogen deposition significantly inhibits methane uptake from an alpine meadow soil on the Qinghai-Tibetan Plateau. Geoderma 213, 444–452. doi: 10.1016/j.geoderma.2013.08.006
Freitag, T. E., Toet, S., Ineson, P., Prosser, J. I. (2010). Links between methane flux and transcriptional activities of methanogens and methane oxidizers in a blanket peat bog. FEMS Microbiol. Ecol. 73, 157–165. doi: 10.1111/j.1574-6941.2010.00871.x
Galloway, J. N., Dentener, F. J., Capone, D. G., Boyer, E. W., Howarth, R. W., Seitzinger, S. P., et al. (2004). Nitrogen cycles: past, present, and future. Biogeochemistry 70, 153–226. doi: 10.1007/s10533-004-0370-0
Gong, S. W., Guo, R., Zhang, T., Guo, J. X. (2015). Warming and nitrogen addition increase litter decomposition in a temperate meadow ecosystem. PloS One 10, e0116013. doi: 10.1371/journal.pone.0116013
Griffin-Nolan, R. J., Slette, I. J., Knapp, A. K. (2021). Deconstructing precipitation variability: Rainfall event size and timing uniquely alter ecosystem dynamics. J. Ecol. 109, 3356–3369. doi: 10.1111/1365-2745.13724
Han, G. X., Sun, B. Y., Chu, X. J., Xing, Q. H., Song, W. M., Xia, J. Y. (2018). Precipitation events reduce soil respiration in a coastal wetland based on four-year continuous field measurements. Agr. Forest Meteorol. 256-257, 292–303. doi: 10.1016/j.agrformet.2018.03.018
Harms, T. K., Grimm, N. B. (2012). Responses of trace gases to hydrologic pulses in desert floodplains. J. Geophys. Res-Biogeo. 117, G01035. doi: 10.1029/2011jg001775
Houweling, S., Bergamaschi, P., Chevallier, F., Heimann, M., Kaminski, T., Krol, M., et al. (2017). Global inverse modeling of CH4 sources and sinks: an overview of methods. Atmo. Chem. Phys. 17, 235–256. doi: 10.5194/acp-17-235-2017
Huang, G., Li, Y., Su, Y. G. (2015). Effects of increasing precipitation on soil microbial community composition and soil respiration in a temperate desert, northwestern China. Soil Biol. Biochem. 83, 52–56. doi: 10.1016/j.soilbio.2015.01.007
Huxman, T. E., Snyder, K. A., Tissue, D., Leffler, A. J., Ogle, K., Pockman, W. T., et al. (2004). Precipitation pulses and carbon fluxes in semiarid and arid ecosystems. Oecologia 141, 254–268. doi: 10.1007/s00442-004-1682-4
IPCC (2021). Climate change 2021: The physical science basis. Contribution of working group I to the sixth assessment report of the intergovernmental panel on climate change (Cambridge, UK: Cambridge University Press).
Jiang, C. M., Yu, G. R., Fang, H. J., Cao, G. M., Li, Y. N. (2010). Short-term effect of increasing nitrogen deposition on CO2, CH4 and N2O fluxes in an alpine meadow on the Qinghai-Tibetan Plateua, China. Atmos. Environ. 44, 2920–2926. doi: 10.1016/j.atmosenv.2010.03.030
Ji, Y., Conrad, R., Xu, H. (2019). Responses of archaeal, bacterial, and functional microbial communities to growth season and nitrogen fertilization in rice fields. Biol. Fert. Soils 56, 81–95. doi: 10.1007/s00374-019-01404-4
Judd, C. R., Koyama, A., Simmons, M. P., Brewer, P., von Fischer, J. C. (2016). Co-Variation in methanotroph community composition and activity in three temperate grassland soils. Soil Biol. Biochem. 95, 78–86. doi: 10.1016/j.soilbio.2015.12.014
Juottonen, H. (2020). Disentangling the effects of methanogen community and environment on peatland greenhouse gas production by a reciprocal transplant experiment. Funct. Ecol. 34, 1268–1279. doi: 10.1111/1365-2435.13536
Kim, D. G., Vargas, R., Bond-Lamberty, B., Turetsky, M. R. (2012). Effects of soil rewetting and thawing on soil gas fluxes: a review of current literature and suggestions for future research. Biogeosciences 9, 2459–2483. doi: 10.5194/bg-9-2459-2012
Kong, D., Li, S., Jin, Y., Wu, S., Chen, J., Hu, T., et al. (2019). Linking methane emissions to methanogenic and methanotrophic communities under different fertilization strategies in rice paddies. Geoderma 347, 233–243. doi: 10.1016/j.geoderma.2019.04.008
Kruger, M., Frenzel, P. (2003). Effects of N-fertilisation on CH4 oxidation and production, and consequences for CH4 emissions from microcosms and rice fields. Glob. Change Biol. 9, 773–784. doi: 10.1046/j.1365-2486.2003.00576.x
Lado-Monserrat, L., Lull, C., Bautista, I., Lidon, A., Herrera, R. (2014). Soil moisture increment as a controlling variable of the “Birch effect”. Interactions with the pre-wetting soil moisture and litter addition. Plant Soil 379, 21–34. doi: 10.1007/s11104-014-2037-5
Leitner, S., Homyak, P. M., Blankinship, J. C., Eberwein, J., Jenerette, G. D., Zechmeister-Boltenstern, S., et al. (2017). Linking NO and N2O emission pulses with the mobilization of mineral and organic N upon rewetting dry soils. Soil Biol. Biochem. 115, 461–466. doi: 10.1016/j.soilbio.2017.09.005
Le Mer, J., Roger, P. (2001). Production, oxidation, emission and consumption of methane by soils: A review. Eur. J. Soil Biol. 37, 25–50. doi: 10.1016/S1164-5563(01)01067-6
Liu, C., Holst, J., Brueggemann, N., Butterbach-Bahl, K., Yao, Z., Han, S., et al. (2008). Effects of irrigation on nitrous oxide, methane and carbon dioxide fluxes in an Inner Mongolian steppe. Adv. Atmos. Sci. 25, 748–756. doi: 10.1007/s00376-008-0748-3
Liu, Y., Liu, S., Miao, R., Liu, Y., Wang, D., Zhao, C. (2019). Seasonal variations in the response of soil CO2 efflux to precipitation pulse under mild drought in a temperate oak (Quercus variabilis) forest. Agr. For. Meteorol. 271, 240–250. doi: 10.1016/j.agrformet.2019.03.009
Liu, Y. W., Wei, D., Tenzintarchen, Zhao, J. X., Geng, X. D., Dai, D. X., et al. (2021b). Nitrogen addition alters C-N cycling in alpine rangelands: Evidence from a 4-year in situ field experiment. Catena 203, 105366. doi: 10.1016/j.catena.2021.105366
Liu, W., Yuan, W., Xu, S., Shao, C., Hou, L., Xu, W., et al. (2021a). Spatiotemporal patterns and drivers of methane uptake across a climate transect in Inner Mongolian steppe. Sci. Total Environ. 757, 143768. doi: 10.1016/j.scitotenv.2020.143768
Liu, X. J., Zhang, Y., Han, W. X., Tang, A. H., Shen, J. L., Cui, Z. L., et al. (2013). Enhanced nitrogen deposition over China. Nature 494, 459–462. doi: 10.1038/nature11917
Lu, X., Mao, Q., Gilliam, F. S., Luo, Y., Mo, J. (2014). Nitrogen deposition contributes to soil acidification in tropical ecosystems. Glob. Change Biol. 20, 3790–3801. doi: 10.1111/gcb.12665
Mariko, S., Urano, T., Asanuma, J. (2007). Effects of irrigation on CO2 and CH4 fluxes from Mongolian steppe soil. J. Hydrol. 333, 118–123. doi: 10.1016/j.jhydrol.2006.07.027
Milich, L. (1999). The role of methane in global warming: where might mitigation strategies be focused? Glob. Environ. Change 9, 179–201. doi: 10.1016/S0959-3780(98)00037-5
Ni, X., Liao, S., Wu, F., Groffman, P. M. (2019). Short-term precipitation pulses stimulate soil CO2 emission but do not alter CH4 and N2O fluxes in a northern hardwood forest. Soil Biol. Biochem. 130, 8–11. doi: 10.1016/j.soilbio.2018.11.021
Niu, F., Chen, J., Xiong, P., Wang, Z., Zhang, H., Xu, B. (2019). Responses of soil respiration to rainfall pulses in a natural grassland community on the semi-arid Loess Plateau of China. Catena 178, 199–208. doi: 10.1016/j.catena.2019.03.020
Norton, U., Mosier, A. R., Morgan, J. A., Derner, J. D., Ingram, L. J., Stahl, P. D. (2008). Moisture pulses, trace gas emissions and soil C and N in cheatgrass and native grass-dominated sagebrush-steppe in Wyoming, USA. Soil Biol. Biochem. 40, 1421–1431. doi: 10.1016/j.soilbio.2007.12.021
Noy-Meir, I. (1973). Desert ecosystems: environment and producers. Annu. Rev. Ecol. Evol. S. 4, 25–51. doi: 10.1146/annurev.es.04.110173.000325
Petrakis, S., Seyfferth, A., Kan, J., Inamdar, S., Vargas, R. (2017). Influence of experimental extreme water pulses on greenhouse gas emissions from soils. Biogeochemistry 133, 147–164. doi: 10.1007/s10533-017-0320-2
Ren, R., Xu, W., Zhao, M., Sun, W. (2019). Grazing offsets the stimulating effects of nitrogen addition on soil CH4 emissions in a meadow steppe in northeast China. PloS One 14, e0225862. doi: 10.1371/journal.pone.0225862
Rosseel, Y. (2012). Lavaan: An R package for structural equation modeling. J. Stat. Softw 48, 1–36. doi: 10.18637/jss.v048.i02
Saari, A., Rinnan, R., Martikainen, P. J. (2004). Methane oxidation in boreal forest soils: Kinetics and sensitivity to pH and ammonium. Soil Biol. Biochem. 36, 1037–1046. doi: 10.1016/j.soilbio.2004.01.018
Schnell, S., King, G. M. (1994). Mechanistic analysis of ammonium inhibition of atmospheric methane consumption in forest soils. Appl. Environ. Microb. 60, 3514–3521. doi: 10.1128/aem.60.10.3514-3521.1994
Shen, Z. X., Li, Y. L., Fu, G. (2015). Response of soil respiration to short-term experimental warming and precipitation pulses over the growing season in an alpine meadow on the northern Tibet. Appl. Soil Ecol. 90, 35–40. doi: 10.1016/j.apsoil.2015.01.015
Shi, B., Xu, W., Zhu, Y., Wang, C., Loik, M. E., Sun, W. (2019). Heterogeneity of grassland soil respiration: Antagonistic effects of grazing and nitrogen addition. Agr. For. Meteorol. 268, 215–223. doi: 10.1016/j.agrformet.2019.01.028
Shrestha, P. M., Kammann, C., Lenhart, K., Dam, B., Liesack, W. (2012). Linking activity, composition and seasonal dynamics of atmospheric methane oxidizers in a meadow soil. ISME J. 6, 1115–1126. doi: 10.1038/ismej.2011.179
Tian, D. S., Niu, S. L. (2015). A global analysis of soil acidification caused by nitrogen addition. Environ. Res. Lett. 10, 24019. doi: 10.1088/1748-9326/10/2/024019
Treseder, K. K. (2008). Nitrogen additions and microbial biomass: a meta-analysis of ecosystem studies. Ecol. Lett. 11, 1111–1120. doi: 10.1111/j.1461-0248.2008.01230.x
Unger, S., Maguas, C., Pereira, J. S., David, T. S., Werner, C. (2010). The influence of precipitation pulses on soil respiration - assessing the “Birch effect” by stable carbon isotopes. Soil Biol. Biochem. 42, 1800–1810. doi: 10.1016/j.soilbio.2010.06.019
Vance, E. D., Brookes, P. C., Jenkinson, D. S. (1987). An extraction method for measuring soil microbial biomass C. Soil Biol. Biochem. 19, 703–707. doi: 10.1016/0038-0717(87)90052-6
Venturini, A. M., Dias, N. M. S., Gontijo, J. B., Yoshiura, C. A., Paula, F. S., Meyer, K. M., et al. (2022). Increased soil moisture intensifies the impacts of forest-to-pasture conversion on methane emissions and methane-cycling communities in the Eastern Amazon. Environ. Res. 212, 113139. doi: 10.1016/j.envres.2022.113139
Waldo, N. B., Hunt, B. K., Fadely, E. C., Moran, J. J., Neumann, R. B. (2019). Plant root exudates increase methane emissions through direct and indirect pathways. Biogeochemistry 145, 213–234. doi: 10.1007/s10533-019-00600-6
Wang, Y., Chen, H., Zhu, Q., Peng, C., Wu, N., Yang, G., et al. (2014b). Soil methane uptake by grasslands and forests in China. Soil Biol. Biochem. 74, 70–81. doi: 10.1016/j.soilbio.2014.02.023
Wang, Q., He, N., Liu, Y., Li, M., Xu, L. (2016). Strong pulse effects of precipitation events on soil microbial respiration in temperate forests. Geoderma 275, 67–73. doi: 10.1016/j.geoderma.2016.04.016
Wang, C., Wang, X. B., Liu, D. W., Wu, H. H., Lu, X. T., Fang, Y. T., et al. (2014a). Aridity threshold in controlling ecosystem nitrogen cycling in arid and semi-arid grasslands. Nat. Commun. 5, 4799. doi: 10.1038/ncomms5799
Wu, J. J., Cheng, X. L., Xing, W., Liu, G. H. (2022). Soil-atmosphere exchange of CH4 in response to nitrogen addition in diverse upland and wetland ecosystems: A meta-analysis. Soil Biol. Biochem. 164, 108467. doi: 10.1016/j.soilbio.2021.108467
Wu, J. Q., Wang, H. Y., Li, G., Wu, J. H., Gong, Y., Wei, X. X., et al. (2021). Responses of CH4 flux and microbial diversity to changes in rainfall amount and frequencies in a wet meadow in the Tibetan Plateau. Catena 202, 105253. doi: 10.1016/j.catena.2021.105253
Yang, X., Wang, C., Xu, K. (2017). Response of soil CH4 fluxes to stimulated nitrogen deposition in a temperate deciduous forest in northern China: A 5-year nitrogen addition experiment. Eur. J. Soil Biol. 82, 43–49. doi: 10.1016/j.ejsobi.2017.08.004
Yue, P., Zuo, X., Li, K., Li, X., Wang, S., Misselbrook, T. (2022). Precipitation changes regulate the annual methane uptake in a temperate desert steppe. Sci. Total Environ. 804, 150172. doi: 10.1016/j.scitotenv.2021.150172
Yu, L., Huang, Y., Zhang, W., Li, T., Sun, W. (2017). Methane uptake in global forest and grassland soils from 1981 to 2010. Sci. Total Environ. 607-608, 1163–1172. doi: 10.1016/j.scitotenv.2017.07.082
Zhao, H., Li, T., Li, L., Hao, Y. (2017). A stable CH4 sink responding to extreme precipitation events in a fenced semiarid steppe. J. Soil Sediment 17, 2731–2741. doi: 10.1007/s11368-017-1798-x
Zhao, C., Miao, Y., Yu, C., Zhu, L., Wang, F., Jiang, L., et al. (2016). Soil microbial community composition and respiration along an experimental precipitation gradient in a semiarid steppe. Sci. Rep. 6, 24317. doi: 10.1038/srep24317
Keywords: precipitation pulse, long-term N addition, methane, suppression effect, meadow steppe
Citation: Gao W, Yang X, Zhang Y, Zhao T, Shi B, Yang T, Ma J, Xu W, Wu Y and Sun W (2023) Suppression of methane uptake by precipitation pulses and long-term nitrogen addition in a semi-arid meadow steppe in northeast China. Front. Plant Sci. 13:1071511. doi: 10.3389/fpls.2022.1071511
Received: 16 October 2022; Accepted: 30 December 2022;
Published: 16 January 2023.
Edited by:
Omer Yetemen, Istanbul Technical University, TürkiyeReviewed by:
Mianhai Zheng, South China Botanical Garden, Chinese Academy of Sciences (CAS), ChinaXiang Liu, Lanzhou University, China
Copyright © 2023 Gao, Yang, Zhang, Zhao, Shi, Yang, Ma, Xu, Wu and Sun. This is an open-access article distributed under the terms of the Creative Commons Attribution License (CC BY). The use, distribution or reproduction in other forums is permitted, provided the original author(s) and the copyright owner(s) are credited and that the original publication in this journal is cited, in accordance with accepted academic practice. No use, distribution or reproduction is permitted which does not comply with these terms.
*Correspondence: Jianying Ma, bWFqeTY1MkBuZW51LmVkdS5jbg==; Wei Sun, c3Vud2VpQG5lbnUuZWR1LmNu
†These authors have contributed equally to this work