- Institute of Environment and Sustainable Development in Agriculture, Chinese Academy of Agricultural Sciences, Beijing, China
Introduction: Promoting crop growth and regulating denitrification process are two main ways to reduce soil N2O emissions in agricultural systems. However, how biochar and arbuscular mycorrhizal fungi (AMF) can regulate crop growth and denitrification in soils with different phosphorus (P) supplies to influence N2O emission remains largely unknown.
Method: Here, an eight-week greenhouse and one-year field experiments biochar and/or AMF (only in greenhouse experiment) additions under low and high P environments were conducted to characterize the effects on wheat (Triticum aestivum L.) growth and N2O emission.
Results: With low P supply, AMF addition decreased leaf Mn concentration (indicates carboxylate-releasing P-acquisition strategies), whereas biochar addition increased leaf Mn concentration, suggesting biochar and AMF addition regulated root morphological and physiological traits to capture P. Compared with low P supply, the high P significantly promoted wheat growth (by 16-34%), nutrient content (by 33-218%) and yield (by 33-41%), but suppressed soil N2O emissions (by 32-95%). Biochar and/or AMF addition exhibited either no or negative effects on wheat biomass and nutrient content in greenhouse, and biochar addition promoted wheat yield only under high P environment in field. However, biochar and/or AMF addition decreased soil N2O emissions by 24-93% and 32% in greenhouse and field experiments, respectively. This decrease was associated mainly with the diminished abundance of N2O-producing denitrifiers (nirK and nirS types, by 17-59%, respectively) and the increased abundance of N2O-consuming denitrifiers (nosZ type, by 35-65%), and also with the increased wheat nutrient content, yield and leaf Mn concentration.
Discussion: These findings suggest that strengthening the plant-soil-microbe interactions can mitigate soil N2O emissions via manipulating plant nutrient acquisition and soil denitrification.
1. Introduction
As a significant greenhouse gas, nitrous oxide (N2O) has the global warming potential 298 times higher than the equivalent mass of CO2 in the atmosphere (Philibert et al., 2013). Due to high rates of organic and inorganic nitrogen (N) fertilization in agricultural production, about 60% of global anthropogenic N2O emissions is derived from agricultural soils (Davidson, 2009). Among the three main processes (denitrification, nitrification and nitrifier-denitrification) that produce N2O in soils (Kool et al., 2011), denitrification is the primary source (Case et al., 2015). Generally, there are two major ways to decrease N2O emissions in agriculture (Jiang et al., 2020): (i) promote the crop growth and N uptake, thus reducing availability of N for N2O production, and (ii) alter the N cycling processes (e.g. denitrification) via regulating the abundance and activity of relevant microorganisms. Thus, linking plant nutrient-acquisition strategy and soil microbial community (composition and activity) can improve our understanding of mechanisms effective in suppressing N2O emissions under different management practices.
The denitrification rate and its contribution to N2O emissions are influenced strongly by soil conditions (e.g. soil pH, soil water content, availability of oxygen, C, N, P, etc.). The N2O:N2 product ratio in denitrification was correlated negatively with soil pH, labile C availability, and soil water-filled pore space, and positively with soil NO3- content (Senbayram et al., 2012). As a soil amendment, biochar application can modify these soil conditions. Biochar, being C-rich and porous, can improve soil aeration, reduce soil bulk density, enhance soil water holding capacity, and adsorb nutrients (such as P) from soil to change C, N and P availability (Zhang et al., 2016; Jiang et al., 2020; Sun et al., 2022). Biochar application can also increase pH of acid soils, but has no effect or can decrease pH of alkaline soils (Lehmann and Joseph, 2009; Dong et al., 2022). In addition, biochar can promote crop root activity and shoot growth, thus enhancing plant nutrient absorption and decreasing N content in soil (Liu et al., 2021; An et al., 2022). Thus, biochar application in agriculture is considered an efficient strategy to reduce N2O emissions via modulating denitrification and crop growth.
Different P conditions can influence soil N pools and cycling processes via regulating plant growth and microbial activity (Xiao et al., 2022a; Xiao et al., 2022b). In P-limited environments, P addition enhanced soil N immobilization and retained more N in the plant-soil system by promoting plant and microbial growth, thus suppressing N2O emission (Shen and Zhu, 2022; Wang et al., 2022). In soils with different P supplies, plants can adjust P-acquisition strategies by regulating root morphology (e.g. root diameter, specific root length) and the amount and composition of root exudates (Wang et al., 2021), which may influence the soil microbial community composition and activity and thus N2O emission (Coskun et al., 2017; Abalos et al., 2019). For instance, P enrichment may stimulate the activity of denitrifiers and nitrifiers (Ullah et al., 2016) or alter microbial community composition regarding the taxa involved in N2O production (Liu et al., 2012). In addition, P enrichment can increase water consumption by plants, which may lead to decreased soil moisture and diminished N2O emission (Chen et al., 2017). Thus, linking the plant nutrient acquistion stategy and soil functional microbiome may advance our understanding of the effects of different P supplies on plant growth and soil N2O emissions.
Arbuscular mycorrhizal fungi (AMF) benefit their host plant regarding nutrient uptake (primary P, N and Zn) from soil (Smith and Read, 2008). AMF inoculation can increase microbial biomass N and plant biomass, thus reducing the availability of N substrates (NH4+ and NO3-) in soil for N2O producers, thus decreasing N2O emissions (Bender et al., 2014; Storer et al., 2018; Shen and Zhu, 2021). AMF may also influence soil aggregation (Morris et al., 2019) and soil water relations (Augé, 2004), and increase oxygen diffusion towards the interiors of soil aggregates, thus affecting denitrification (Okiobe et al., 2022). To date, studies have showed that AMF can decrease the abundance of the nirK type denitrifiers (that produce N2O) and increase the abundance of nosZ type (that consume N2O), thus hampering denitrification (Bender et al., 2014; Gui et al., 2021; Zhao et al., 2021).
Based on the current knowledge, a reasonable hypothesis is that biochar, P and AMF may interact to suppress N2O production by modifying the plant growth and abundance of microorganisms associated with the N cycle. Plants usually increase root/shoot ratio, specific root length and carboxylate exudation and decrease root tissue density to enhance their capacity to acquire P in P-limited environments (Lambers et al., 2006; Shen et al., 2011). However, the large amounts of carboxylate exudation may stimulate the growth of microorganisms, including the functional microorganisms involved in denitrification (Coskun et al., 2017; Abalos et al., 2019). In the P-limited environments, AMF inoculation can reduce exudation from their host roots but improve host plant nutrient acquisition, whereas in P-rich environments AMF inoculation may inhibit plant growth (Smith et al., 2011; Ryan et al., 2012; Johnson et al., 2015; Zhang et al., 2021). On the other hand, biochar can adsorb P to decrease P availability in soil in both low- and high-P environments (Dai et al., 2016). Hence, biochar application may govern the AMF effects on plant growth via changing the P availability. Earlier studies have also shown that biochar can promote the AMF symbiosis via changing soil nutrient availability, altering the activity of specific microorganisms that have effects on mycorrhizae, and serving as a refuge for fungi to colonizing plants (Warnock et al., 2007; Lehmann et al., 2011). To date, how the interaction among biochar, P and AMF modifies plant growth and denitrification in soil, and thus influences N2O emission, remains obscure.
Wheat is one of the most widely cultivated crops in the world and is highly sensitive to soil P availability (Li et al., 2014), with AMF having negative effects on wheat growth at the high P supply (Efthymiou et al., 2018; Ryan and Graham, 2018). In the present study, we set up a fully factorial greenhouse experiment and a field experiment. Wheat was grown with or without biochar and/or AMF at the low and high P supply. The study was aimed at testing the following hypotheses: (i) high P supply can enhance plant growth and N uptake and reduce soil N2O emissions; (ii) AMF may increase crop growth and inhibit key denitrification microorganisms to decrease soil N2O emissions at the low P supply, whereas at the high P supply, AMF may inhibit wheat growth and diminish the mitigating effect on N2O emission; and (iii) biochar can suppress N2O emission via directly inhibiting the key denitrification genes, and via directly influence wheat growth or indirectly modifying the effect of AMF on wheat growth at differential P supply.
2. Materials and methods
2.1. Experimental treatments
2.1.1. Greenhouse experiment
A calcareous loamy soil (0-20 cm) was collected from Shangzhuang experimental station (40°08′ N, 116°10′ E) of China Agricultural University. The soil contained 17.8 g kg-1 organic matter, 870 mg kg-1 N, 2.9 mg kg-1 Olsen P, 156 mg kg-1 available K, and had pH value 7.8 (soil:water ratio was 1:5). The soil was air dried, sieved (2 mm) and sterilized by radiation with 60Co γ-rays at 10 kGy to eliminate indigenous AMF.
A greenhouse experiment was conducted with two phosphorus rates (40 and 300 mg P kg-1 soil as KH2PO4, denoted hereafter as P40 and P300, respectively), two mycorrhizal (AMF) treatments (with and without inoculation with Rhizophagus irregularis) and two biochar (BC) rates (with and without maize straw BC). There were five replicates per treatment. To achieve the same soil K addition in the two P treatments, K2SO4 was supplied at 327 mg K kg-1 soil in the P40 treatment. Furthermore, the mineral nutrients were added to all treatments (per kg soil) as follows: 200 mg of N (as KNO3), 50 mg of Mg (as MgSO4), 5 mg of Zn (as ZnSO4), and 2 mg of Cu (as CuSO4). The nutrients were uniformly mixed in soil before placing the soil in plastic pots (15 cm in diameter, 15 cm in height; 2 kg of soil pot-1). The glasshouse temperature range was 15–20°C with 10–12 h daylight throughout the wheat growth period.
Biochar was derived from maize straw residue at 450°C, and was provided by Mingchen Sanitation Equipment Co. LTD, Shandong Province, China. Biochar contained (per kg) 9.48 g total N, 1.34 g total P and 530 g carbon; pH was 9.7. Biochar was added at 3.5 g kg-1 soil (equivalent to 9 t ha-1) and was mixed thoroughly.
The AMF Rhizophagus irregularis was propagated on wheat plants growing in a 1:5 mixture (w/w) of river sand and zeolite in a greenhouse for 4 months. The inoculum used in the present study included substrate containing spores (150 g-1 potting substrate), mycelium and fine-root segments. The inoculum was added at 20 g kg-1 soil in the AMF treatment. The 10 mL of microbial wash filtrate from unsterilized soil was added to all pots to minimize differences in microbial communities among treatments (Martínez-García et al., 2017).
Wheat seeds (JiMai 22) were surface sterilized by stirring in 10% (v/v) hydrogen peroxide for 10 min and in 70% (v/v) ethanol for 3 min, followed by rinsing at least five times in deionized water. Six uniformly germinated seeds were sown into each pot and were thinned after emergence to four seedlings similar in size. The pots were watered daily and weighed every 2 days to adjust soil moisture to 18-20% (w/w).
2.1.2. Field experiment
A one-year field experiment with four-treatment was designed to test the effects of biochar and P addition mitigate N2O emissions. The study site is located in the Shunyi District, Beijing. The soil contained 9.68 g kg-1 organic matter,1.32 g kg-1 N, 6.48 mg kg-1 Olsen P, 73.6 mg kg-1 available K in 0-20 cm soil depth and a pH of 8.05. The field plots were conducted with a completely randomized block design with four treatments: BC0_P0 (0 t hm-2 biochar, 0 kg hm-2 P), BC0_P+ (52 kg hm-2 P), BC+_P0 (9 t hm-2 biochar), BC+_P+ (9 t hm-2 biochar, 52 kg.hm-2 P). Before sowing of winter wheat in 2020, the amount of biochar and P in four treatments were supplied every year. Sixteen plots were arranged with four replicates, and every plot size was 180 m2 (6 m × 30 m).
In field experiment, wheat (JiMai 22) was sown on 12 October 2020 and harvested on 6 June 2021. The P fertilizer input amount of each treatment was 0 (P0) and 52 kg P hm-2 (P+) (Ca(H2PO4)2), K 124 kg.hm-2 (K2SO4), N 112 kg hm-2 (KNO3), respectively. N fertilizer was uniformly applied at a 1:1 ratio according to topdressing (15 March 2021). Each treatment was irrigated after fertilization.
2.2. Gas collection and measurement
2.2.1. Greenhouse experiment
Seven weeks after sowing, nutrients solution with 200 mg kg-1 KNO3 was added in each pot, and the soil moisture adjusted to 30% (w/w), which was beneficial to denitrification process but had little effect on wheat growth during a week of sampling N2O. After 24 hours, N2O samples were collected from each pot by using a PVC cylindrical chamber (diameter of 16 cm and height of 50 cm), with a base having a 5 cm groove to which water was added to seal the system during sample collection. The gas in the chamber was sampled starting at 9:00 and then again starting at 15:00 each day for a week; during this period, the soil moisture was kept at 30% (w/w) by adding water. The four 50 mL gas samples were extracted by plastic syringes at 0, 10, 20 and 30 min in each of the two daily sampling periods.
2.2.2. Field experiment
N2O samples were collected by using a PVC box chamber (50cm×50cm×50cm) with a thickness of 1.5 mm and a base (50 cm × 50 cm × 15 cm). The gas was collected twice a week after fertilization, irrigation, rain and snow before the booting stage. Afterwards, the collection frequency decreased to once every 15 days during the fallow period. Gas samples were taken between 09:00 to 11:00 am. During this period, the four 200 mL gas samples were extracted by plastic syringes at 0, 10, 20 and 30 min for each plot.
2.2.3. N2O measurement
The measurement of N2O was done by gas chromatography (Agilent 7890A; Agilent, USA) with an electron capture detector (ECD) at 350°C (Liu et al., 2020). The hourly fluxes (F, μg m-2 h-1) of N2O were calculated according to Shi et al. (2019):
F= ρ×(V/A)×(ΔC/Δt)×(273/(273+T))
where ρ is the density of N2O under standard conditions, V is the chamber volume (m3), A is the area covered by chamber (m2), ΔC/Δt is the change in N2O concentration in the chamber over time, and T is the chamber air temperature.
The cumulative N2O emission (E, mg m-2) was calculated as follows:
E=(Fi+Fi+1)/2 ×24×10-3×T
where i is the ith measurement, 24 × 10-3 was used for unit conversion, and T was the number of days of sample collection.
The nosZ/(nirK+nirS) was the nosZ and (nirK+nirS) gene copy numbers raito. nosZ associated with N2O consumption in the process of denitrification, and (nirK+nirS) was significantly positively correlated with the denitrification rates (Xuan et al., 2022). The ratio is used to characterize the degree of N2O emission inhibition (Liu et al., 2020)
2.3. Plant harvest and soil sampling
2.3.1. Greenhouse experiment
Eight weeks after sowing (Feekes 3, tillering stage), shoots were cut at the soil surface, and roots were extracted from the soil. Shoots were rinsed in distilled water and then oven-dried at 75°C for 72 h, weighed and ground to fine powder. Nitrogen and phosphorus concentrations in shoots were determined after digestion with a mixture of 5 mL of concentrated H2SO4 and 8 mL of 30% v/v H2O2. Shoot N was analyzed by the Kjeldahl method and P by the molybdovanadophosphate method. Leaf Mn concentration was used to indicate carboxylate-releasing P-acquisition strategies (Lambers et al., 2015) and was determined directly by Atomic Absorption Spectroscopy (AAS, GBC 904 AvantaVer 1.33, Australia).
Roots were washed under running water and were scanned at the resolution of 400 dpi (EPSON 1680) (Epson, Long Beach, CA, USA). Root images were analyzed using a WinRHIZO image system (WinRHIZOPro2004b) (Null, Regent, Canada) to calculate root length and diameter (Wang et al., 2020). After scanning, a weighed subsample of the root system was cleared and stained with trypan blue to determine mycorrhizal colonization by the method of Kormanik and McGraw (1982). The roots were dried at 75°C for 72 h and weighed. Calculations of root tissue density and specific root length were done according to Li et al. (2013).
The soil samples were collected on the same day as the gas samples. The soil was sieved (2 mm) and separated into two subsamples: one was kept at -80°C for quantitative PCR assay and the other one was stored at 4°C for determining C, P and N content. Soil NO3--N and NH4+-N were extracted with 0.01 mol L-1 CaCl2 solution and determined by a AA3 flow analyzer (Braun and Lubbe, Norderstedt, Germany). Soil available P was measured by the Olsen method. Soil organic carbon was analyzed by wet digestion with 10% w/w H3PO4.
In order to test the effects of P, BC and AMF on denitrification, the copy numbers of the key genes of copper nitrite reductases (nirS, nirK) and nitrous oxide reductase (nosZ) were determined. DNA was extracted using a Fast DNA Spin Kit for Soils (MP Biomedicals, Solon, OH, USA) from 0.5 g of soil. The quantitative polymerase chain reaction (q-PCR) amplification primers for nirK, nirS and nosZ genes and the reaction conditions are shown in Table S1. The qPCR was performed using a CFX96 Optical Real-Time Detection System (Bio-Rad Laboratories, Hercules, CA, USA).
2.3.2. Field experiment
At wheat maturity, wheat yield was measured in each treatment plot. Soil samples (0–20 cm) were collected when soil N2O emissions tended to be stable after fertilization. Five soils from two diagonal lines through each plot were collected and pooled into one composite sample. The soil was sieved (2 mm) and was kept at -80°C, and then used to measure the functional genes of denitrification.
2.4. Statistical analyses
Two-way ANOVA was performed to test for effects of AMF and biochar addition on N2O emission, gene abundance, root traits and soil properties (P ≤0.05) at low or high P supply. Post-hoc Tukey HSD tests were performed to determine significant differences. If the two-way interaction AMF× BC was significant, the complete data was shown; if not, only the significant main effects were shown. Significant differences between the AMF and no AMF inoculation at each P supply or between BC and no BC addition at each P supply were based on the t-test (P ≤0.05). Pearson’s correlation analysis was used to test the relationships among the N2O emission, abundance of genes, root traits and soil properties. The random forest model in R studio software was used to analyze the comprehensive effects of genes, root traits and soil properties on the soil N2O emissions.
Two-way ANOVA was performed to test for effects of P and biochar addition on yield, N2O emission and gene abundance (P ≤0.05) in the field experiment. Post-hoc Tukey HSD tests were performed to determine significant differences. Pearson’s correlation analysis was used to test the relationships among the yield, N2O emission and gene abundance.
3. Results
3.1. Shoot growth properties
The interaction AMF × BC was not significant for shoot biomass and shoot N and P contents (Table 1). The shoot biomass was influenced significantly by mycorrhiza at both P rates, but the biochar main effect was a significant source of variation for shoot biomass at P300 only (Table 1 and Figure 1). The mycorrhizal main effect was a significant source of variation for shoot N content and shoot P content at both P40 and P300 (Table 1 and Figure 1). In the presence of mycorrhiza, shoot biomass and shoot N content were significantly lower than in the non-mycorrhizal treatment regardless of the P rate. By contrast, AMF addition was associated with a decrease in shoot P content at P40 and an increase at P300 (Figure 1). Compared to the low P supply, the high P significantly promoted shoot growth (by 16-34%), shoot N content (by 33-47%) and shoot P content (by 145-218%) (calculated from Figure 1). In the field experiment, the interaction BC×P was significant for wheat yield (Table 2). Compare to no P addition treatment, wheat yield increased significantly (by 33% and 41%, respectively) in P addition treatments.
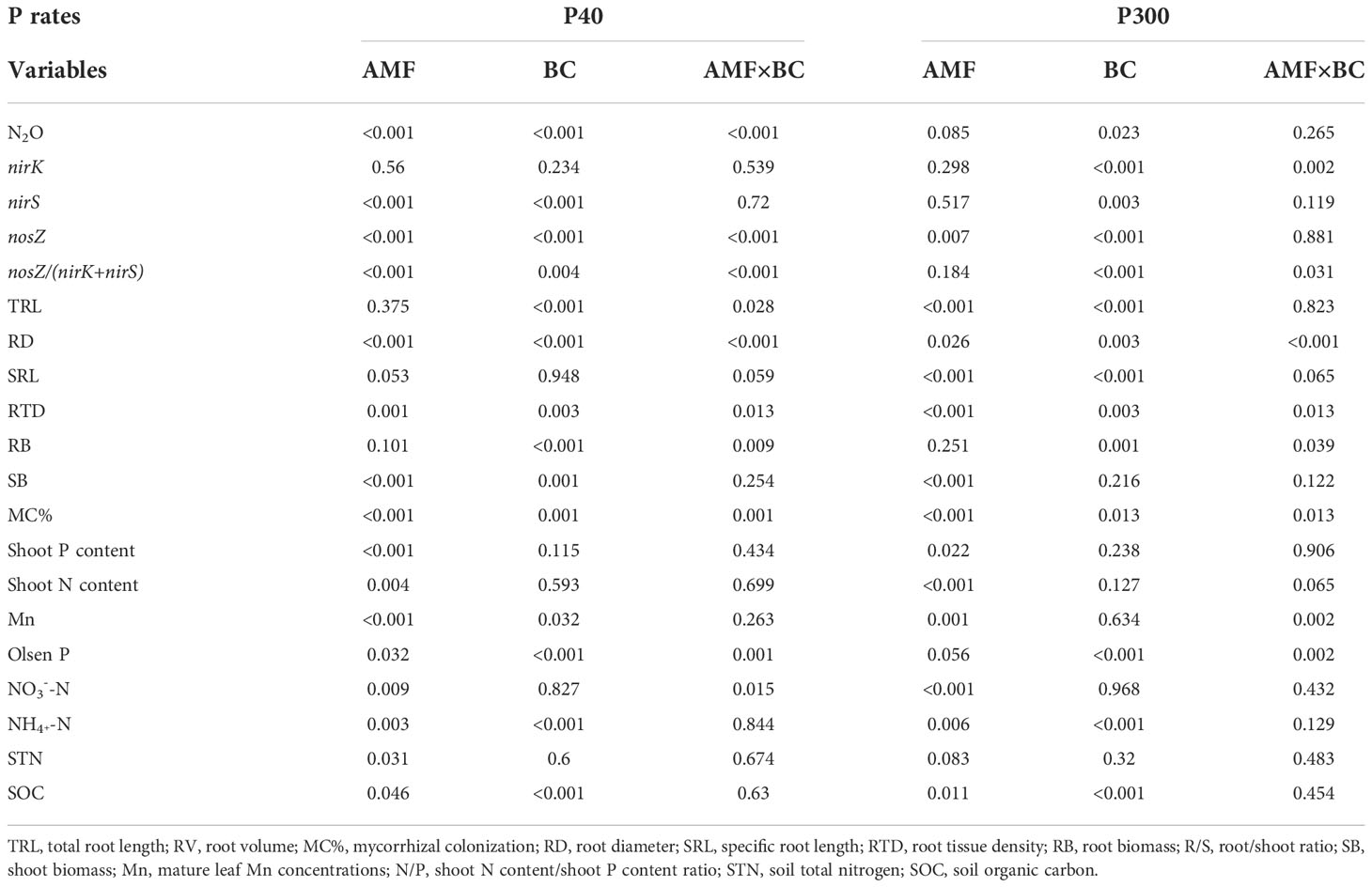
Table 1 The P values from 2-way ANOVA (AMF=arbuscular mycorrhizal inoculum and BC=biochar) at the two P rates (P40 and P300) regarding soil N2O emissions, gene abundance, and plant and soil properties in the greenhouse experiment.
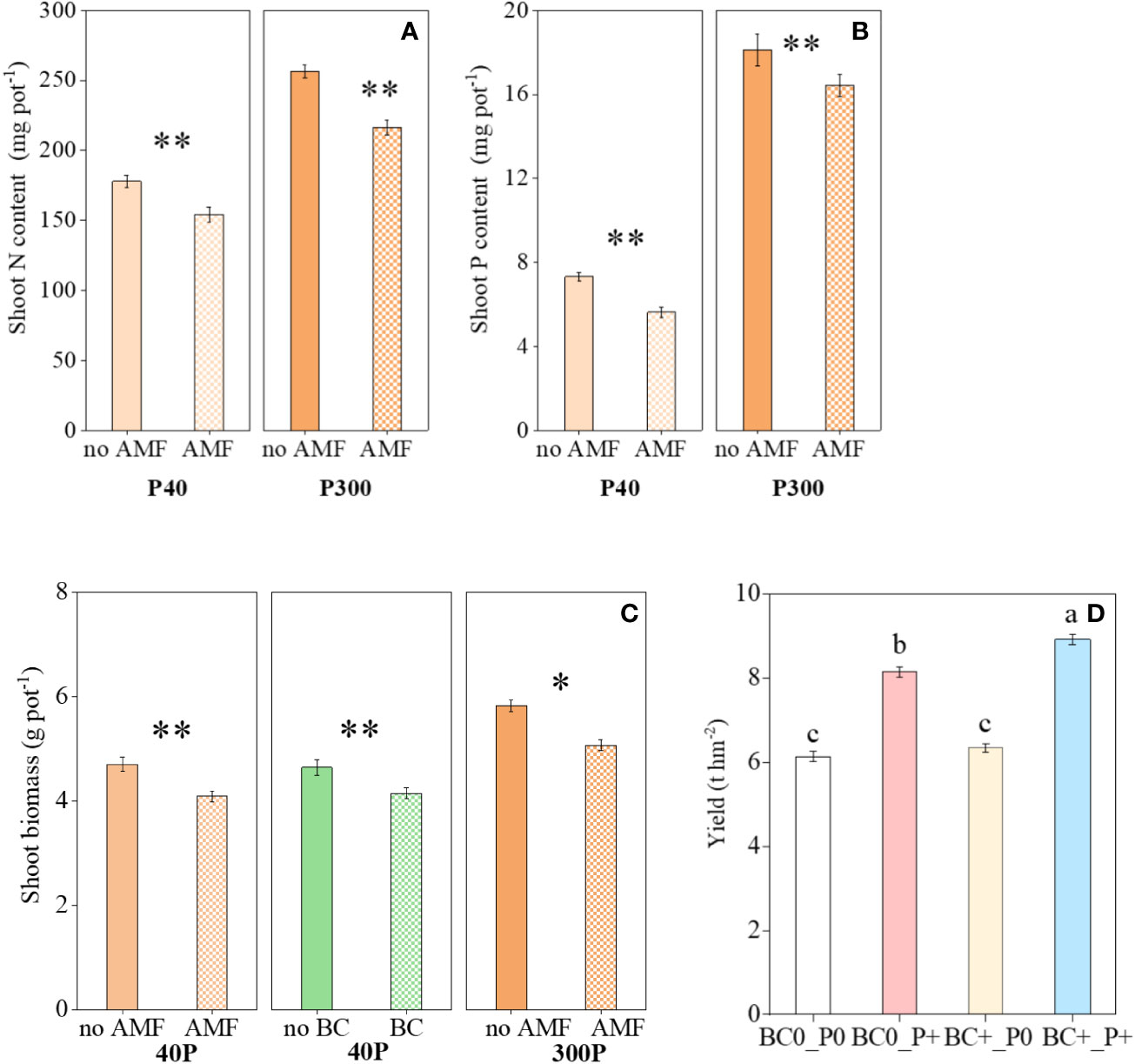
Figure 1 Variation in the (A) shoot N content, (B) shoot P content and (C) shoot biomass as influenced by P rates (40 and 300 mg kg−1 soil) and AMF and BC additions in the greenhouse experiment. Each value is the mean of five replicates (±SE). (D) Variation in wheat yield in field experiment. Each value is the mean of four replicates (±SE). *P ≤ 0.05 and **P < 0.01.
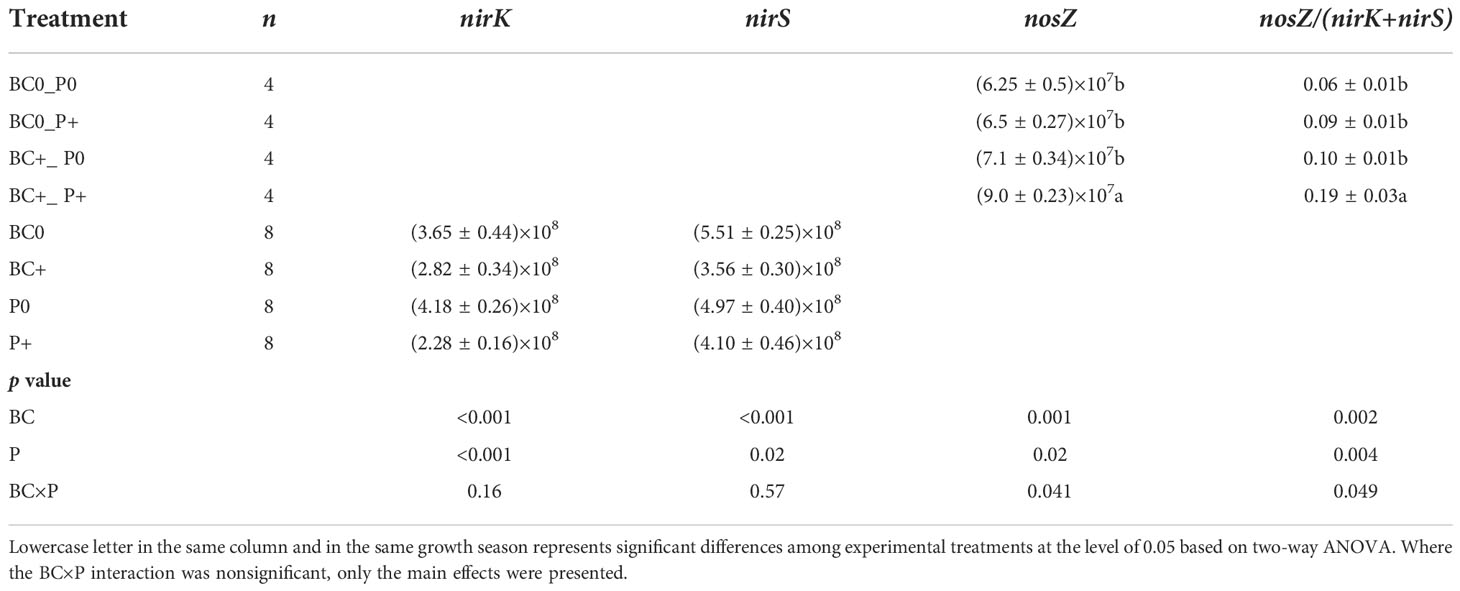
Table 2 Effects of P and biochar application on N2O emission, nirK, nirS and nosZ gene copy numbers and nosZ/(nirK+nirS) in field experiment.
3.2. Root morphological and physiological traits
Root biomass and root tissue density were significantly influenced by the interaction AMF×BC at P40 and P300 (Table 1). At the low P supply, compared to the control, root biomass decreased significantly (by 30% to 48%) in the other treatments (Figures 2A, B). Regarding root tissue density, contrasting results were obtained at two P supplies. Compared to the other treatments, root tissue density was more than 2-fold lower in the combined AMF and BC treatment at P40 and in the control at P300 (Figure 2B). Root tissue density had a significantly negative correlation with root diameter in both P40 and P300 (Table S2).
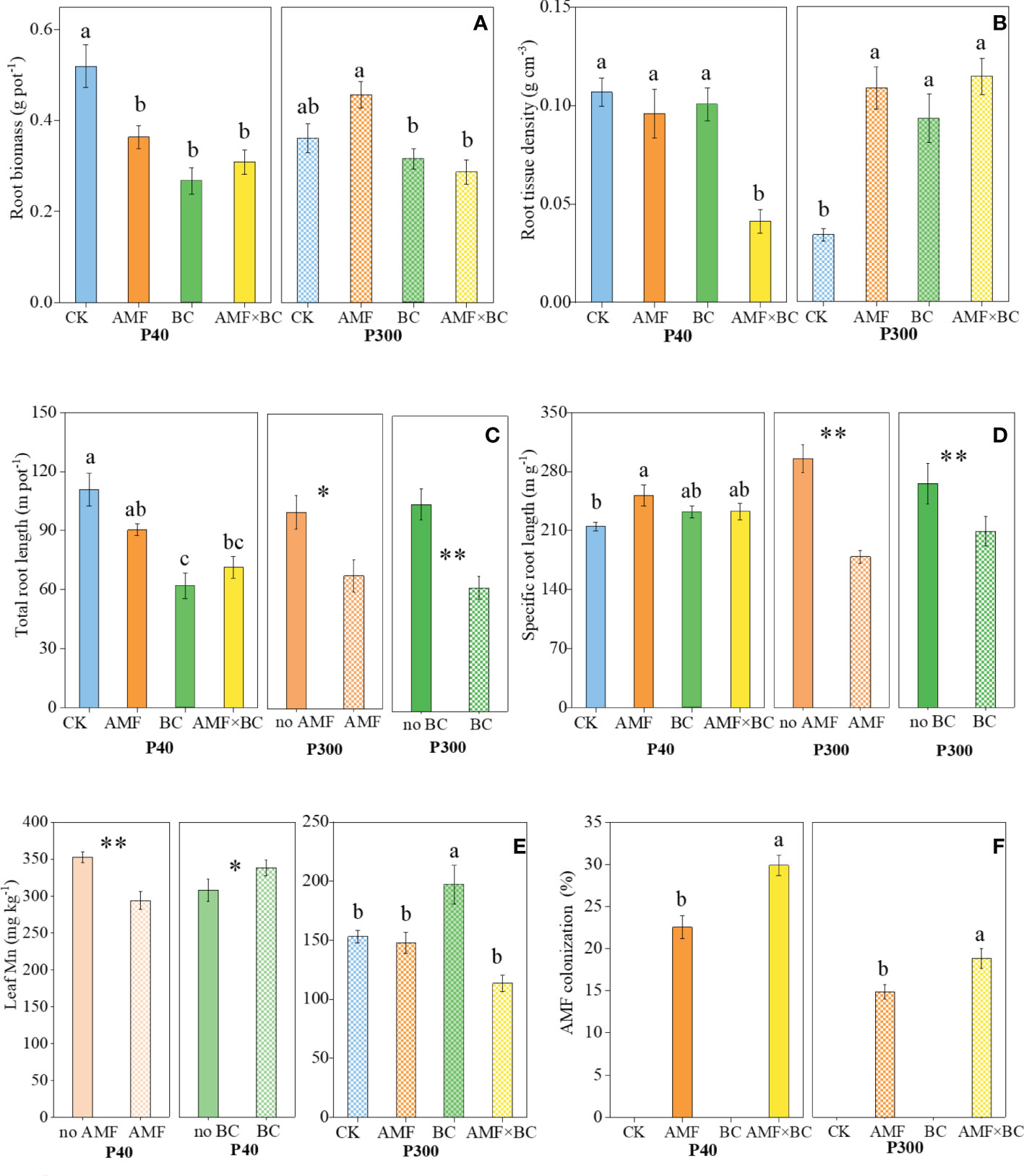
Figure 2 Variation in the root biomass and morphological traits (A) root biomass, (B) root tissue density, (C) total root length, (D) specific root length, (E) leaf Mn concentrations, and (F) AMF colonization as influenced by P rates (40 and 300 mg kg−1 soil) and AMF and BC additions. Each value is the mean of five replicates (±SE). For a given P rate, different letters in each graph denote significant difference among treatments (P ≤ 0.05). *P ≤ 0.05 and **P < 0.01.
The AMF×BC interaction was a significant source of variation for total root length and specific root length at P40 only (Table 1). In the combined AMF and BC treatment, total root length was 36% lower, and specific root length was 8.4% higher, than the control at P40 (Figure 2C). At the high P supply, both main effects significantly influenced total root length and specific root length (Table 1). The total root length and specific root length decreased significantly (by 32% and 65%, respectively) with mycorrhizal inoculation. Biochar addition decreased total root length by 40% and specific root length by 22% (Figures 2C, D).
Leaf Mn concentration was significantly influenced by the interaction AMF×BC at P300 only (Table 1). Compared to the control, Mn concentration was decreased significantly in the combined AMF and BC treatment at P300 (Figure 2E). At the low P supply, both main effects significantly influenced Mn concentration (Figure 2E) that increased by 10% with biochar addition and decreased by 17% with mycorrhizal inoculation.
3.3. AMF colonization
The mycorrhizal colonization was significantly influenced by the AMF × BC interaction (Table 1). No root colonization was detected in the treatments without AMF addition. AMF colonization tended to be higher at P40 than P300. In the given P treatment (Figure 2F) biochar addition increased AMF colonization by 27-33% compared to the AMF treatment (Figure 2F).
3.4. Soil N2O emissions
The soil N2O emissions was significantly influenced by the AMF×BC interaction at P40 only (Table 1). At the low P supply, compared to the control, the soil N2O emissions decreased significantly (by 88%, 76% and 93%) in, respectively, the AMF, BC and the combined AMF and BC treatments (Figure 3A).
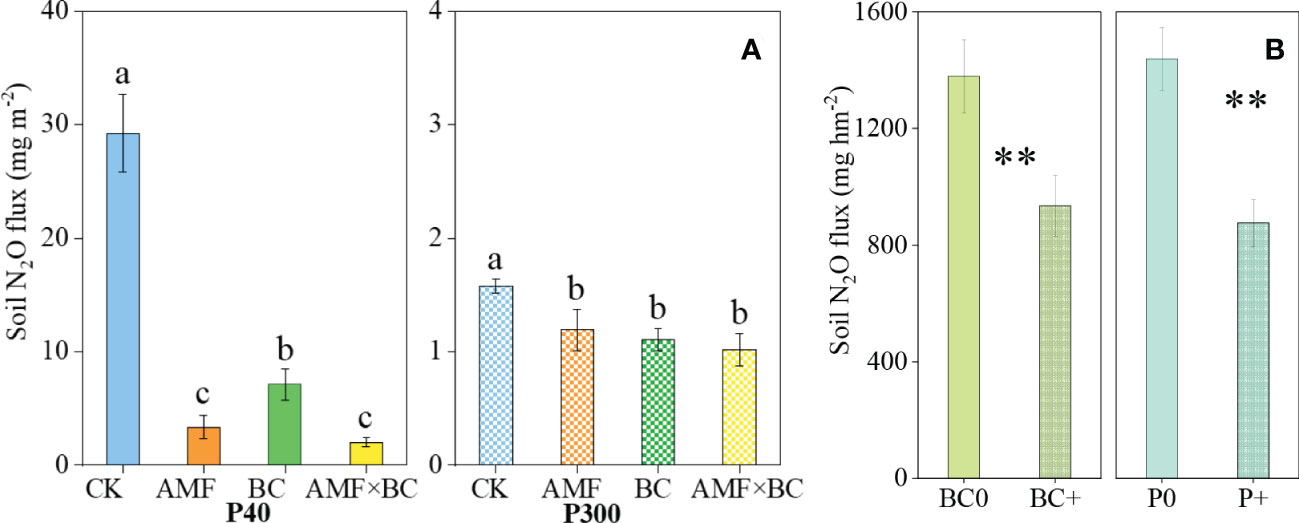
Figure 3 (A) Variation in the cumulative emissions of N2O as influenced by P rates (40 and 300 mg kg−1 soil) and AMF and BC additions in the greenhouse experiment. Each value is the mean of five replicates (±SE). For a given P rate, different letters in each graph denote significant difference among treatments. (B) Variation in the cumulative emissions of N2O as influenced by P and BC additions in field experiment. Each value is the mean of four replicates (±SE). **P < 0.01.
The biochar main effect was a significant source of variation for soil N2O emissions at P300. Compared to control, the soil N2O emissions decreased significantly (by 30% and 24%, respectively) with biochar addition and mycorrhizal inoculation. Compared to the low P supply, the high P significantly suppressed soil N2O emissions by 50-95% (calculated from Figure 3A).
In the field experiment, the soil N2O emissions was significantly influenced by P and biochar addition (Table 2). Compared to no biochar and no P environments, biochar and P addition reduced soil N2O emissions by 32% and 39%, respectively (Figure 3B).
3.5. Soil properties
The AMF×BC interaction was a significant source of variation for soil NO3--N at P40 only (Table 1). The mycorrhizal main effect was a significant source of variation for soil NO3--N at P300. At the low P supply, the soil NO3–N was 48% higher with mycorrhizal inoculation than the control, but decreased by 27% in the presence of mycorrhiza compared to the non-mycorrhizal treatment at P300 (Figure S1A).
The interaction AMF×BC interaction was not significant for the soil NH4+-N (Table 1). The soil NH4+-N was significantly influenced by mycorrhiza and biochar addition at both P rates. Biochar addition was associated with an increase in the soil NH4+-N at P40 and P300. Mycorrhizal inoculation was associated an increase at P40 and a decrease at P300 (Figure S1B).
3.6. Abundance of nitrification and denitrification genes in the soil
The abundance of nirS gene was significantly influenced by mycorrhizal addition at P40 only, but the biochar main effect was a significant source of variation for nirS gene abundance at both P rates (Table 1). At the low P supply, nirS gene abundance decreased significantly (by 25%-26%) with biochar addition or mycorrhizal inoculation, but abundance of nirS gene increased by 19% with biochar addition at P300 compared to the no biochar treatment (Figure 4A). The AMF×BC interaction was a significant source of variation for abundance of nirK gene at P300 only (Table 1). Compared to the control at P300, the nirK gene copy number decreased significantly (by 35% to 59%) in the other treatments (Figure 4B). In the field experiment, P addition decreased significantly the abundance of nirS and nirK genes by 17% and 45% compared to no P addition, respectively (Table 2).
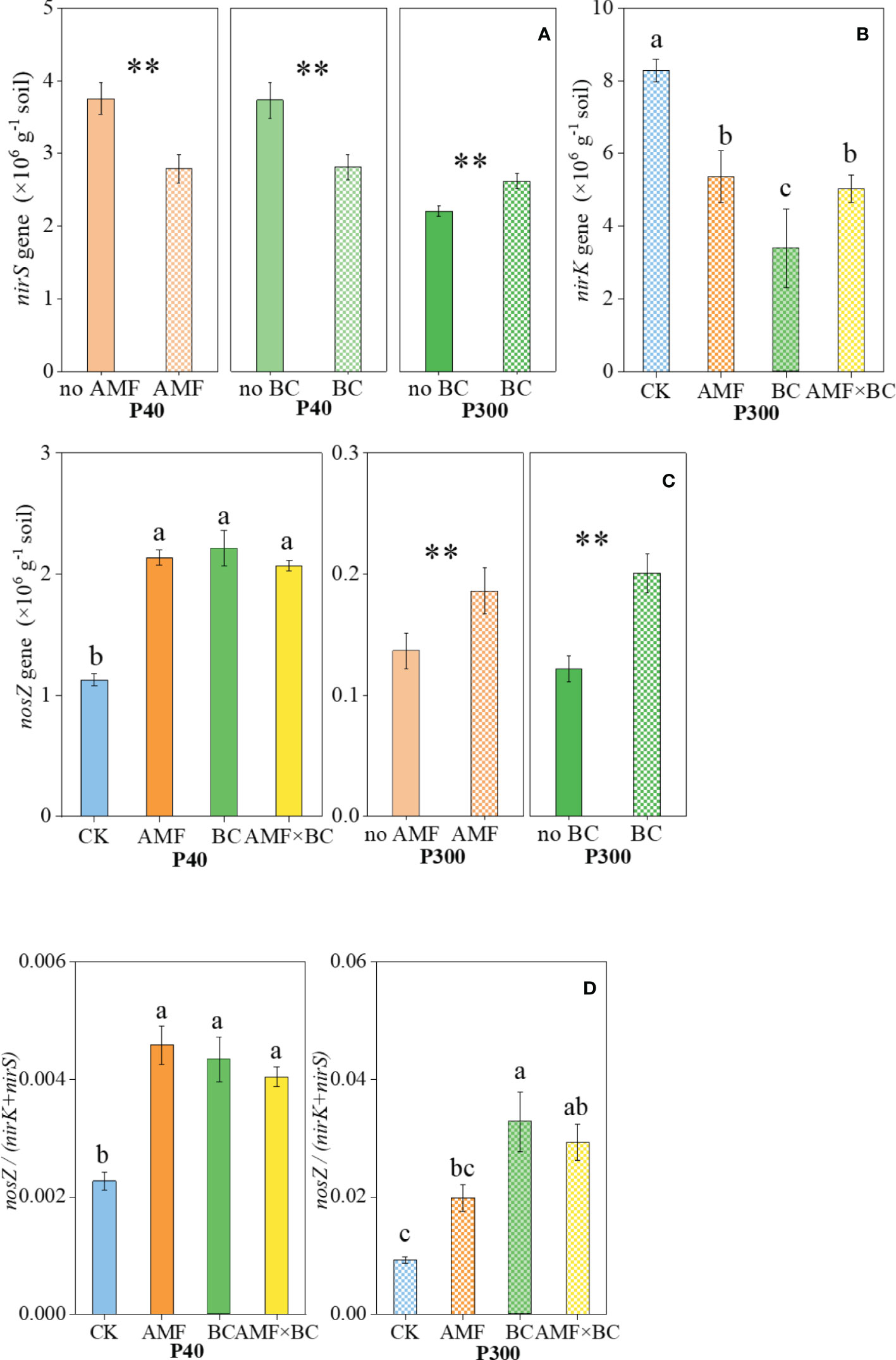
Figure 4 Variation in the (A) nirS, (B) nirK, and (C) nosZ gene copy numbers and (D) the ratio of nosZ/(nirK+nirS) as influenced by P rates (40 and 300 mg kg−1 soil) and AMF and BC additions. Each value is the mean of five replicates (±SE). For a given P rate, different letters in each graph denote significant difference among treatments (P ≤ 0.05). **P < 0.01. Note an order of magnitude difference in the scale on the Y-axis.
The nosZ gene abundance was significantly influenced by the AMF×BC interaction at P40 only, whereas at 300 P both main effects significantly influenced nosZ genes (Table 1). Compared to the control at P40, the nosZ gene abundance increased significantly (by 84% to 96%) in the other treatments. at the high P supply, the number of nosZ gene copies increased significantly (by 37% and 65% with biochar addition or mycorrhizal inoculation, respectively) (Figure 4C).
The nosZ/(nirK+nirS) ratio was significantly influenced by the interaction AMF×BC at P40 and P300 (Table 1). Compared to the control, the nosZ/(nirK+nirS) ratio increased significantly (by 115% to 256% at P40 and 78% to 102% at P300) in the other treatments (Figure 4D). The nosZ/(nirK+nirS) ratio at high P level was 4.06 to 7.56 folds higher than at low P level (calculated from Figure 4).
In the field experiment, the nosZ gene and the nosZ/(nirK+nirS) ratio were significantly influenced by the BC×P interaction. Compared to the BC0P0 treatment, the nosZ gene and nosZ/(nirK+nirS) ratio increased significantly by 43% and 226% in the BC+P+ treatment. The nosZ gene and nosZ/(nirK+nirS) ratio increased significantly by 35% and 172% with P addition compared to no P addition treatment (Table 2).
3.7. Factors affecting soil N2O emissions
At the low P supply, N2O emission was correlated positively with shoot growth, root growth and nirS gene abundance. The nosZ gene copy number, nosZ/(nirK+nirS) ratio, soil NO3–N, NH4+-N, SOC and AMF colonization were correlated negatively with N2O emission. At the high P supply, the nosZ abundance and nosZ/(nirK+nirS) ratio were correlated negatively with N2O emission, whereas shoot growth, root morphology, nirK copy number and soil NO3–N were correlated positively with N2O emission (Figure S2A). In the field experiment, N2O emission was correlated positively with nirK and nirS genes, and was correlated negatively with wheat yield, nosZ and nosZ/(nirK+nirS) ratio (Figure S2B).
When the data from the two P treatments of greenhouse experiment were combined across 25 variables, the abundances of nirk and nirS genes were correlated positively with N2O emission, but nosZ/(nirk+nirS) ratio showed a negative correlation with N2O emission. Root growth was correlated with N2O positively, and shoot growth and AMF colonization were correlated negatively. The mature leaf Mn content was correlated positively with N2O. Additionally, Olsen P and soil NH4+-N showed a negative correlation with N2O (Figure 5A).
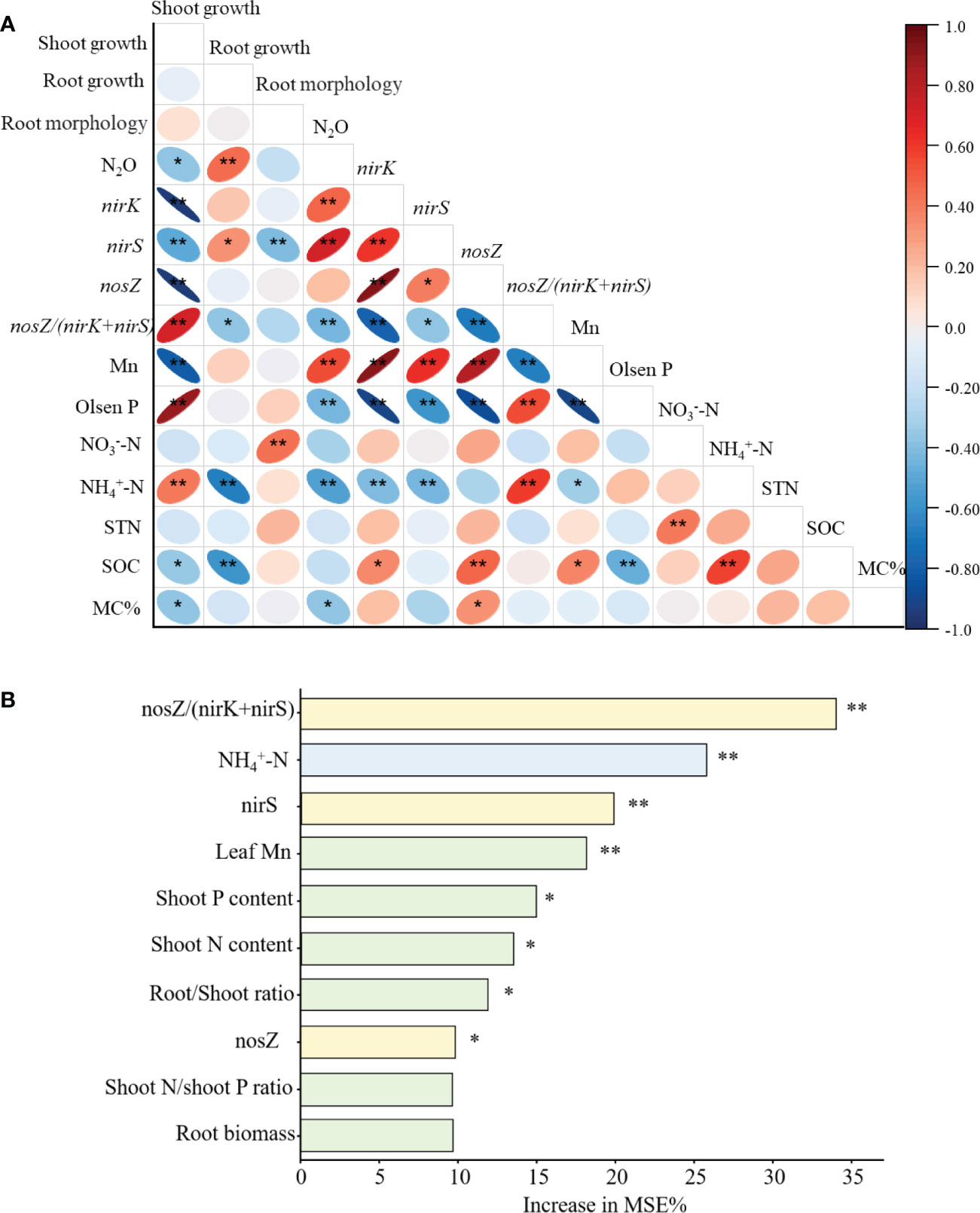
Figure 5 (A) Heat map of Pearson’s correlation coefficients with original data among N2O emission, gene copies, plant traits and soil properties. (B) Random Forest for importance of the specific variables to N2O emission. The variables were sorted in the decreasing order of the importance value. **P < 0.01 and *P < 0.05.
Most important variables to influence soil N2O emissions based on the random forest model were ranked in order of importance (Figure 5B). The nosZ/(nirK+nirS) ratio, soil NH4+-N, nirS abundance and mature leaf Mn concentration were the significant factors associated with N2O emission. Shoot P and N content, root/shoot ratio, and nosZ copy numbers were the additional significant factors influencing N2O emission (Figure 5B).
In summary, the addition of biochar and/or AMF had a negative effect on the total root length for both P40 and P300. The AMF addition decreased leaf Mn concentration, whereas biochar addition increased leaf Mn concentration at P40, suggesting biochar and AMF addition promote P uptake. Compared with P40, the high P significantly promoted wheat growth (16-34%), nutrient content (33-218%) and yield (33-41%), but inhibited soil N2O emissions (32-95%). However, biochar and/or AMF addition reduced soil N2O emissions by 24-93% and 32%, respectively, in greenhouse and field experiments. This decrease was associated mainly with the diminished abundance of nirK and nirS (17-59%) and the increased nosZ (35-65%) in the greenhouse and field experiments, respectively.
4. Discussion
4.1. The growth of plants in different environments
In the present study, the results showed that the wheat root and shoot biomass growth and yield were all higher at the high than low P supply regardless of the biochar or AMF additions (Figure 1), suggesting the inhibiting effect of low P on plant growth. In general, AMF has a negative effect on plant growth in P-rich soils, whereas it promotes nutrient acquisition in the low-P soils (Johnson et al., 2015). However, in the greenhouse experiment, AMF additions (AMF added alone or in combination with BC) reduced biomass and nutrient content at both low and high P rates (Figure 1 and Table 1). Compared to the control, AMF addition significantly decreased shoot biomass and P content at the low and high P supply (Figure 1). AMF inoculation could inhibit the root nutrients absorption in the high nutrient environment, while maintaining the contribution of AMF absorption pathway, finally, this negative effect influenced the crop growth (Smith et al., 2011). The root length decreased significantly by mycorrhizal inoculation in the present study (Figure 2), hinting a trade-off between AMF and root length in absorption of available P (Li et al., 2019; Zhang et al., 2023). We also found that leaf Mn concentration was significantly reduced by AMF inoculation as well, especially in low P environment (Figure 2E). Leaf Mn concentration has been proposed as a signature for the strength of carboxylates exudation in the rhizosphere (Lambers et al., 2021). It means that mycorrhizal inoculation reduced the rhizosphere carboxylate releasing of wheat root. Similar trend was also found in the previous work on Kennedia species that the AMF inoculation reduced exudation of organic carboxylates up to 50% (Ryan et al., 2012). However, the reduction in the release of carboxylate may decrease the ability of plants to utilize insoluble phosphorus (Lambers et al., 2008). As a thin-root species, wheat mainly depends more on the root system in P acquisition compared with thick-root species (Wen et al., 2019). Therefore, AMF inoculation may suppress wheat growth through inhibiting the root growth and exudation, and then bring a negative effect on wheat growth.
In the different P environments, adding biochar alone had no significant effect on shoot N and P content in wheat shoots in the greenhouse (Figure 1), but biochar and P addition could promote wheat yield in the field (Table 2). Biochar addition could significantly reduce root biomass, total root length (Figures 2A, B), which suggested that biochar addition can diminish carbon partitioning to roots. Our findings in previous field experiment also indicated that biochar addition decreased assimilate partitioning to rice roots at elongation stage (Liu et al., 2021). That might have been attributed to biochar addition improving root activity (Cao et al., 2019). For instance, our results showed that biochar increasing leaf Mn concentration (Figure 2E). This indicated that the addition of biochar could increase the release of carboxylate. We also found that biochar addition can enhance the AMF colonization (Figure 2F), which may be caused by biochar can change soil nutrient availability (such as P available, Figure S1) and be a refuge for colonizing fungi (Warnock et al., 2007).
Meanwhile, our results showed a negative relationship between root tissue density and root diameter in both low and high P environments (Table S2). This may be because the increase in root diameter was mainly driven by the high thickness or proportion of root cortex (Kong et al., 2019). Due to the cortex density was significantly lower than stele density in root structure, suggesting that an increase of cortex thickness meant a decrease in root tissue density (Kong et al., 2019). Meanwhile, the higher proportion or thickness of cortex can provide more niche space for AMF colonization and facilitate higher mycorrhizal colonization intensity (Kong et al., 2017). In the present study, AMF colonization was the highest, while RTD decreased under AMF and biochar combined treatment at the low P supply (Figures 2B, F), reflecting a integrated variation of root morphology and AMF in plant P-acquisition strategy.
4.2. N2O emission and driving factors
In both greenhouse and field experiments, N2O emission flux was much higher at the low P supply compared to the high P supply (Figure 3), which was consistent with the results reported elsewhere (Shen et al., 2021; Shen and Zhu, 2022). The suppression of soil N2O emissions by P addition can partly be attributed to the improved shoot N content and thus decreased availability of N substrates (Xiao et al., 2022a). In the present study, the shoot N content and yield at the high P supply was indeed significantly higher at high compared with low P supply (Figure 1), providing a support for the above viewpoint.
In addition, compared to low P supply, the abundance of nirK and nirS genes associated with N2O production decreased by 17% to 59%, but the nosZ genes associated with N2O consumption (N2O→N2) in denitrification were increased by 34% to 1340% at the high P supply, which result in the nosZ/(nirK+nirS) ratio at the high P supply was 2.72 to 7.56 times greater than that at the low P supply (Figure 4 and Table 2), suggesting a strong influence of P addition on N cycling genes involved in denitrification (Xiao et al., 2022b). In the present study, among the top five factors affecting the N2O emission, nosZ/(nirK+nirS), nirS, leaf Mn concentration and stem P content were closely related to soil Olsen P across all the treatments (Figure 5B). Therefore, these findings suggest that the variation of soil P environment dominated N2O emission by altering proportion of functional microbes in the total microbiome, nutrient acquisition strategy, and plant growth.
As Figure 3 shown, AMF addition significantly decreased N2O emissions, especially at the low P supply. There was no evidence of a positive effect of AMF on crop growth in the present study (Figure 1); therefore, the suppressed effect of AMF on N2O emission may be not via promoting plant N retention and reducing availability of soil N for N2O production. Nevertheless, we found that AMF decreased the copy number of nirK genes and increased nosZ genes, thus reducing N2O emission by regulating the denitrification process (Figure 4). Zhao et al. (2021) and Storer et al. (2018) both reported that AMF could directly influence denitrification and reduce N2O emission, which was consistent with the results in this study.
Biochar application had no significant positive effect on the shoot N and P content regardless of the P supply in greenhouse experiment (Table 1). However, a decrease in N2O emission by biochar addition was likely associated with a decreased copy number of nirS genes at the low P supply and nirK genes at the high P supply, and with the increased abundance of the nosZ gene to modify the denitrification process (Figure 4). For instance, N2O emission had a negative correlation with nosZ gene and positive correlation with nirK genes in the field experiment (Figure S2B), which was consistent with our previous research in the field (Liu et al., 2020). In addition, biochar application could significantly reduce P availability in soil, especially in low P environment, which might bring about P deficiency stress, and then reduced activity of denitrifying microorganisms led to decreased soil N2O emissions (Wang et al., 2022).
Because biochar enhanced AMF colonization (Figure 2F), AMF and biochar showed a significant interaction on N2O emission at the low P supply (Table 1). The inhibition effect of AMF plus biochar application on N2O emission was stronger than that of biochar application alone (Figure 3A). This may be attributed mainly to a decreased copy number of nirS genes in the either AMF or BC treatment. In summary, the effect of AMF and biochar on suppressing N2O emission was mainly via regulating denitrification process rather than reducing substrates for denitrification by promoting plant growth.
5. Conclusion
The eight-week greenhouse and one-year field experiments verified that the high P supply suppressed soil N2O emissions via promoting plant growth, root nutrient acquisition capacity and yield, and also through regulating denitrification process, especially increasing the nosZ/(nirK+nirS) ratio compared to the low P supply. The soil N2O emissions was mitigate after AMF and/or biochar addition regardless of P supply in greenhouse or in field experiments, but the effect of under high P supply which might be attributed mainly to the decreased copy numbers of genes associated with N2O production (nirK and nirS) and the increased copy number of the nosZ gene associated with N2O consumption in the process of denitrification. Our findings highlight that strong interaction among plant, soil microbiome and soil properties in regulating denitrification.
Data availability statement
The original contributions presented in the study are included in the article/Supplementary Material. Further inquiries can be directed to the corresponding author.
Author contributions
ZH: conceptualization, writing-original draft, review & editing. ZD: data analysis. SH: data analysis. AZ: conceptualization and writing-review & editing. All authors contributed to the article and approved the submitted version.
Funding
This study was supported by the National Natural Science Foundation of China (31601834) and Central Public-interest Scientific Institution Basal Research Fund (No.BSRF202209).
Acknowledgments
We thank to Zed Rengel from University of Western Australia for helping us to revise the English language problem.
Conflict of interest
The authors declare that the research was conducted in the absence of any commercial or financial relationships that could be construed as a potential conflict of interest.
The handling editor KX declared a past collaboration with the author AZ.
Publisher’s note
All claims expressed in this article are solely those of the authors and do not necessarily represent those of their affiliated organizations, or those of the publisher, the editors and the reviewers. Any product that may be evaluated in this article, or claim that may be made by its manufacturer, is not guaranteed or endorsed by the publisher.
Supplementary material
The Supplementary Material for this article can be found online at: https://www.frontiersin.org/articles/10.3389/fpls.2022.1069627/full#supplementary-material
Supplementary Table 1 | Primers used in qPCR. Note: R=A or G; S=C or G.
Supplementary Table 2 | Pearson’s correlation with original data among N2O emission, genes, plant traits and soil properties under 40P treatment (upper-right part) and 300P treatment (lower-left part). Note: GR: nosZ/(nirK+nirS). SB, shoot biomass; RB, root biomass; TRL, total root length; RD, root diameter; RV, root volume; SRL, specific root length; RTD, root tissue density; R/S, root/shoot ratio; P con, Phosphorus concentration; P acc, Phosphorus accumulation; N con, nitrogen concentration; N acc, nitrogen accumulation; N/P, shoot N/shoot P ratio; C%, shoot C content; Mn, leaf Mn concentrations; OP, Olsen phosphorus; STN, shoot total nitrogen content; SOC, soil organic carbon; MC, mycorrhizal colonization.
Supplementary Figure 1 | Variation in the soil NO3–N and NH4+-N, Olsen P, soil organic carbon, and root diameter as influenced by P rates (40 and 300 mg kg−1 soil) and AMF and BC additions in the greenhouse experiment. Each value is the mean of five replicates ( ± SE). ** P<0.01 and * P<0.05.
Supplementary Figure 2 | (A) Heat map of Pearson’s correlation coefficients among N2O emission, gene copies, plant traits and soil properties under P300 treatment (upper-right part) and P40 treatment (lower-left part) under greenhouse experiment. Each value is the mean of five replicates( ± SE) (B) Heat map of Pearson’s correlation coefficients with original data among yield, N2O emission, gene copies in one-year field experiment. Each value is the mean of four replicates ( ± SE). Note: shoot growth as a principal component includes shoot biomass, shoot phosphorus concentration and content, and shoot nitrogen concentration and content. Root growth as a principal component includes root biomass, total root length and root/shoot ratio. Root morphology as a principal component includes root diameter, specific root length and root tissue density. Mn, mature leaf Mn concentrations; STN, soil total nitrogen content; SOC, soil organic carbon; MC%, mycorrhizal colonization. ** P<0.01 and * P<0.05.
References
Abalos, D., van Groenigen, J. W., Philippot, L., Lubbers, I. M., De Deyn, G. B. (2019). Plant trait-based approaches to improve nitrogen cycling in agroecosystems. J. Appl. Ecol. 56 (11), 2454–2466. doi: 10.1111/1365-2664.13489
An, N., Zhang, L., Liu, Y., Shen, S., Li, N., Wu, Z. C., et al. (2022). Biochar application with reduced chemical fertilizers improves soil pore structure and rice productivity. Chemosphere 298, 134304. doi: 10.1016/j.chemosphere.2022.134304
Auge, R. M. (2004). Arbuscular mycorrhizae and soil/plant water relations. Can. J. Soil Sci. 84 (4), 373–381. doi: 10.4141/S04-002
Bender, S. F., Plantenga, F., Neftel, A., Jocher, M., Oberholzer, H. R., Kohl, L., et al. (2014). Symbiotic relationships between soil fungi and plants reduce N2O emissions from soil. Isme J. 8 (6), 1336–1345. doi: 10.1038/ismej.2013.224
Cao, H., Ning, L., Xun, M., Feng, F., Li, P., Yue, S., et al. (2019). Biochar can increase nitrogen use efficiency of malus hupehensis by modulating nitrate reduction of soil and root. Appl. Soil Ecol. 135, 25–32. doi: 10.1016/j.apsoil.2018.11.002
Case, S. D. C., McNamara, N. P., Reay, D. S., Stott, A. W., Grant, H. K., Whitaker, J. (2015). Biochar suppresses N2O emissions while maintaining n availability in a sandy loam soil. Soil Biol. Biochem. 81, 178–185. doi: 10.1016/j.soilbio.2014.11.012
Chen, H., Zhang, W., Gurmesa, G. A., Zhu, X., Li, D., Mo, J. (2017). Phosphorus addition affects soil nitrogen dynamics in a nitrogen-saturated and two nitrogen-limited forests. Eur. J. Soil Sci. 68 (4), 472–479. doi: 10.1111/ejss.12428
Coskun, D., Britto, D. T., Shi, W., Kronzucker, H. J. (2017). How plant root exudates shape the nitrogen cycle. Trends Plant Sci. 22, 661–673. doi: 10.1016/j.tplants.2017.05.004
Dai, L., Li, H., Tan, F., Zhu, N., He, M., Hu, G. (2016). Biochar: a potential route for recycling of phosphorus in agricultural residues. GCB Bioenergy 8, 852–858. doi: 10.1111/gcbb.12365
Davidson, E. A. (2009). The contribution of manure and fertilizer nitrogen to atmospheric nitrous oxide since 1860. Nat. Geosci. 2, 659–662. doi: 10.1038/ngeo608
Dong, Z., Li, H., Xiao, J., Sun, J., Liu, R., Zhang, A. (2022). Soil multifunctionality of paddy field is explained by soil pH rather than microbial diversity after 8-years of repeated applications of biochar and nitrogen fertilizer. Sci. Total Environ. 853, 158620. doi: 10.1016/j.scitotenv.2022.158620
Efthymiou, A., Jensen, B., Jakobsen, I. (2018). The roles of mycorrhiza and penicillium inoculants in phosphorus uptake by biochar-amended wheat. Soil Biol. Biochem. 127, 168–177. doi: 10.1016/j.soilbio.2018.09.027
Gui, H., Gao, Y., Wang, Z., Shi, L., Yan, K., Xu, J. (2021). Arbuscular mycorrhizal fungi potentially regulate N2O emissions from agricultural soils via altered expression of denitrification genes. Sci. Total Environ. 774, 145133. doi: 10.1016/j.scitotenv.2021.145133
Jiang, Z., Lian, F., Wang, Z., Xing, B. (2020). The role of biochars in sustainable crop production and soil resiliency. J. Exp. Bot. 71, 520–542. doi: 10.1093/jxb/erz301
Johnson, N. C., Wilson, G. W., Wilson, J. A., Miller, R. M., Bowker, M. A. (2015). Mycorrhizal phenotypes and the l aw of the m inimum. New Phytol. 205, 1473–1484. doi: 10.1111/nph.13172
Kong, D., Wang, J., Wu, H., Valverde-Barrantes, O. J., Wang, R., Zeng, H., et al. (2019). Nonlinearity of root trait relationships and the root economics spectrum. Nat. Commun. 10, 2203. doi: 10.1038/s41467-019-10245-6
Kong, D., Wang, J., Zeng, H., Liu, M., Miao, Y., Wu, H., et al. (2017). The nutrient absorption–transportation hypothesis: optimizing structural traits in absorptive roots. New Phytol. 213, 1569–1572. doi: 10.1111/nph.14344
Kool, D. M., Dolfing, J., Wrage, N., Van Groenigen, J. W. (2011). Nitrifier denitrification as a distinct and significant source of nitrous oxide from soil. Soil Biol. Biochem. 43, 174–178. doi: 10.1016/j.soilbio.2010.09.030
Kormanik, P. P., McGraw, A. (1982). Quantification of vesicular-arbuscular mycorrhizae in plant roots. In: Schenck, NC (ed) Methods and Principles of Mycorrhiza Research. American Phytopathological Society, St Paul, pp 37–45.
Lambers, H., Hayes, P. E., Laliberte, E., Oliveira, R. S., Turner, B. L. (2015). Leaf manganese accumulation and phosphorus-acquisition efficiency. Trends Plant Sci. 20, 83–90. doi: 10.1016/j.tplants.2014.10.007
Lambers, H., Raven, J. A., Shaver, G. R., Smith, S. E. (2008). Plant nutrient-acquisition strategies change with soil age. Trends Ecol. Evol. 23, 95–103. doi: 10.1016/j.tree.2007.10.008
Lambers, H., Shane, M. W., Cramer, M. D., Pearse, S. J., Veneklaas, E. J. (2006). Root structure and functioning for efficient acquisition of phosphorus: matching morphological and physiological traits. Ann. Bot. 98, 693–713. doi: 10.1093/aob/mcl114
Lambers, H., Wright, I. J., Guilherme Pereira, C., Bellingham, P. J., Bentley, L. P., Boonman, A., et al. (2021). Leaf manganese concentrations as a tool to assess belowground plant functioning in phosphorus-impoverished environments. Plant Soil 461, 43–61. doi: 10.1007/s11104-020-04690-2
Lehmann, J., Joseph, S. (2009). “Biochar for environmental management: an introduction,” in Biochar for environmental management. science and technology. Eds. Lehmann, J., Joseph, S. (London: Earthscan), pp 1–pp12.
Lehmann, J., Rillig, M. C., Thies, J., Masiello, C. A., Hockaday, W. C., Crowley, D. (2011). Biochar effects on soil biota–a review. Soil Biol. Biochem. 43, 1812–1836. doi: 10.1016/j.soilbio.2011.04.022
Li, H., Ma, Q., Li, H., Zhang, F., Rengel, Z., Shen, J. (2013). Root morphological responses to localized nutrient supply differ among crop species with contrasting root traits. Plant Soil 376, 151–163. doi: 10.1007/s11104-013-1965-9
Liu, L., Gundersen, P., Zhang, T., Mo, J. (2012). Effects of phosphorus addition on soil microbial biomass and community composition in three forest types in tropical China. Soil Biol. Biochem. 44, 31–38. doi: 10.1016/j.soilbio.2011.08.017
Liu, B., Li, H., Li, H., Zhang, A., Rengel, Z. (2021). Long-term biochar application promotes rice productivity by regulating root dynamic development and reducing nitrogen leaching. GCB Bioenergy 13, 257–268. doi: 10.1111/gcbb.12766
Liu, H., Li, H., Zhang, A., Rahaman, M. A., Yang, Z. (2020). Inhibited effect of biochar application on N2O emissions is amount and time-dependent by regulating denitrification in a wheat-maize rotation system in north China. Sci. Total Environ. 721, 137636. doi: 10.1016/j.scitotenv.2020.137636
Li, H., Zhang, D., Wang, X., Li, H., Rengel, Z., Shen, J. (2019). Competition between Zea mays genotypes with different root morphological and physiological traits is dependent on phosphorus forms and supply patterns. Plant Soil 434, 125–137. doi: 10.1007/s11104-018-3616-7
Martínez-García, L. B., De Deyn, G. B., Pugnaire, F. I., Kothamasi, D., van der Heijden, M. G. (2017). Symbiotic soil fungi enhance ecosystem resilience to climate change. Global Change Biol. 23, 5228–5236. doi: 10.1111/gcb.13785
Morris, E. K., Morris, D. J. P., Vogt, S., Gleber, S. C., Bigalke, M., Wilcke, W., et al. (2019). Visualizing the dynamics of soil aggregation as affected by arbuscular mycorrhizal fungi. ISME J. 13, 1639–1646. doi: 10.1038/s41396-019-0369-0
Okiobe, S. T., Pirhofer-Walzl, K., Leifheit, E., Rillig, M. C., Veresoglou, S. D. (2022). Proximal and distal mechanisms through which arbuscular mycorrhizal associations alter terrestrial denitrification. Plant Soil. 476, 315–336. doi: 10.1007/s11104-022-05534-x
Philibert, A., Loyce, C., Makowski., D. (2013). Prediction of N2O emission from local information with random forest. Environ. pollut. 177, 156–163. doi: 10.1016/j.envpol.2013.02.019
Ryan, M. H., Graham, J. H. (2018). Little evidence that farmers should consider abundance or diversity of arbuscular mycorrhizal fungi when managing crops. New Phytol. 220, 1092–1107. doi: 10.1111/nph.15308
Ryan, M. H., Tibbett, M., Edmonds-tibbett, T., Suriyagoda, L. D. B., Lambers, H., Cawthray, G. R., et al. (2012). Carbon trading for phosphorus gain: the balance between rhizosphere carboxylates and arbuscular mycorrhizal symbiosis in plant phosphorus acquisition. Plant Cell Environ. 35, 2170–2180. doi: 10.1111/j.1365-3040.2012.02547.x
Senbayram, M., Chen, R., Budai, A., Bakken, L., Dittert, K. (2012). N2O emission and the N2O/(N2O+N2) product ratio of denitrification as controlled by available carbon substrates and nitrate concentrations. Agricult. Ecosyst. Environ. 147, 4–12. doi: 10.1016/j.agee.2011.06.022
Shen, Y., Xu, T., Chen, B., Zhu, B. (2021). Soil N2O emissions are more sensitive to phosphorus addition and plant presence than to nitrogen addition and arbuscular mycorrhizal fungal inoculation. Rhizosphere 19, 100414. doi: 10.1016/j.rhisph.2021.100414
Shen, J., Yuan, L., Zhang, J., Li, H., Bai, Z., Chen, X., et al. (2011). Phosphorus dynamics: From soil to plant. Plant Physiol. 156, 997–1005. doi: 10.1104/pp.111.175232
Shen, Y., Zhu, B. (2021). Arbuscular mycorrhizal fungi reduce soil nitrous oxide emission. Geoderma 402, 115179. doi: 10.1016/j.geoderma.2021.115179
Shen, Y., Zhu, B. (2022). Effects of nitrogen and phosphorus enrichment on soil N2O emission from natural ecosystems: A global meta-analysis. Environ. pollut. 301, 118993. doi: 10.1016/j.envpol.2022.118993
Shi, Y., Liu, X., Zhang, Q. (2019). Effects of combined biochar and organic fertilizer on nitrous oxide fluxes and the related nitrifier and denitrifier communities in a saline-alkali soil. Sci. Total Environ. 686, 199–211. doi: 10.1016/j.scitotenv.2019.05.394
Smith, S. E., Jakobsen, I., Grønlund, M., Smith, F. A. (2011). Roles of arbuscular mycorrhizas in plant phosphorus nutrition: interactions between pathways of phosphorus uptake in arbuscular mycorrhizal roots have important implications for understanding and manipulating plant phosphorus acquisition. Plant Physiol. 156, 1050–1057. doi: 10.1104/pp.111.174581
Storer, K., Coggan, A., Ineson, P., Hodge, A. (2018). Arbuscular mycorrhizal fungi reduce nitrous oxide emissions from N2O hotspots. New Phytol. 220, 1285–1295. doi: 10.1111/nph.14931
Sun, J., Li, H., Wang, Y., Du, Z., Rengel, Z., Zhang, A. (2022). Biochar and nitrogen fertilizer promote rice yield by altering soil enzyme activity and microbial community structure. GCB Bioenergy 14, 1266–1280. doi: 10.1111/gcbb.12995
Ullah, B., Shaaban, M., Hu, R., Zhao, J., Lin, S. (2016). Assessing soil nitrous oxide emission as affected by phosphorus and nitrogen addition under two moisture levels. J. Integr. Agric. 15, 2865–2872. doi: 10.1016/S2095-3119(16)61353-9
Wang, R., Bicharanloo, B., Hou, E., Jiang, Y., Dijkstra, F. A. (2022). Phosphorus supply increases nitrogen transformation rates and retention in soil: A global meta-analysis. Earth's Future 10, e2021EF002479. doi: 10.1029/2021EF002479
Wang, X. X., Li, H., Chu, Q., Feng, G., Kuyper, T. W., Rengel, Z. (2020). Mycorrhizal impacts on root trait plasticity of six maize varieties along a phosphorus supply gradient. Plant Soil 448, 71–86. doi: 10.1007/s11104-019-04396-0
Wang, X. X., Zhang, J., Wang, H., Rengel, Z., Li, H. (2021). Plasticity and co-variation of root traits govern differential phosphorus acquisition among 20 wheat genotypes. Oikos. doi: 10.1111/oik.08606
Warnock, D. D., Lehmann, J., Kuyper, T. W., Rillig, M. C. (2007). Mycorrhizal responses to biochar in soil–concepts and mechanisms. Plant Soil 300, 9–20. doi: 10.1007/s11104-007-9391-5
Wen, Z., Li, H., Shen, Q., Tang, X., Xiong, C., Li, H., et al. (2019). Tradeoffs among root morphology, exudation and mycorrhizal symbioses for phosphorus-acquisition strategies of 16 crop species. New Phytol. 223, 882–895. doi: 10.1111/nph.15833
Xiao, J., Dong, S., Shen, H., Li, S., Wessell, K., Liu, S., et al. (2022b). N addition overwhelmed the effects of p addition on the soil c, n, and p cycling genes in alpine meadow of the qinghai-Tibetan plateau. Front. Plant Sci. 13, 860590. doi: 10.3389/fpls.2022.860590
Xiao, J., Dong, S., Shen, H., Li, S., Zhi, Y., Mu, Z., et al. (2022a). Phosphorus addition promotes nitrogen retention in alpine grassland plants while increasing n deposition. Catena 210, 105887. doi: 10.1016/j.catena.2021.105887
Xuan, Y., Mai, Y., Xu, Y., Zheng, J., He, Z., Shu, L., et al. (2022). Enhanced microbial nitrification-denitrification processes in a subtropical metropolitan river network. Water Res. 222, 118857. doi: 10.1016/j.watres.2022.118857
Zhang, H., Chen, C., Gray, E. M., Boyd, S. E., Yang, H., Zhang, D. (2016). Roles of biochar in improving phosphorus availability in soils: A phosphate adsorbent and a source of available phosphorus. Geoderma 276, 1–6. doi: 10.1016/j.geoderma.2016.04.020
Zhang, L., Chu, Q., Zhou, J., Rengel, Z., Feng, G. (2021). Soil phosphorus availability determines the preference for direct or mycorrhizal phosphorus uptake pathway in maize. Geoderma 403, 115261. doi: 10.1016/j.geoderma.2021.115261
Zhang, A., Wang, X., Zhang., D., Dong, Z., Ji, H., Li, H. (2023). Localized nutrient supply promotes maize growth and nutrient acquisition by shaping root morphology and physiology and mycorrhizal symbiosis. Soil Tillage Res. 225, 105550. doi: 10.1016/j.still.2022.105550
Keywords: denitrification, root morphology, foraging strategy, nitrogen retention, plant-soil-microbe interaction, leaf Mn concentration
Citation: Hao Z, Dong Z, Han S and Zhang A (2022) Effects of biochar and arbuscular mycorrhizal fungi on winter wheat growth and soil N2O emissions in different phosphorus environments. Front. Plant Sci. 13:1069627. doi: 10.3389/fpls.2022.1069627
Received: 14 October 2022; Accepted: 23 November 2022;
Published: 14 December 2022.
Edited by:
Kaixiong Xing, Hainan Normal University, ChinaReviewed by:
Lei Jiang, Zhejiang A&F University, ChinaXiaoyan Tang, Sichuan Agricultural University, China
Xinxin Wang, Agricultural University of Hebei, China
Copyright © 2022 Hao, Dong, Han and Zhang. This is an open-access article distributed under the terms of the Creative Commons Attribution License (CC BY). The use, distribution or reproduction in other forums is permitted, provided the original author(s) and the copyright owner(s) are credited and that the original publication in this journal is cited, in accordance with accepted academic practice. No use, distribution or reproduction is permitted which does not comply with these terms.
*Correspondence: Aiping Zhang, emhhbmdhaXBpbmdAY2Fhcy5jbg==