- 1Crop Science, Institute of Crop Science and Resource Conservation, University of Bonn, Bonn, Germany
- 2Water Engineering Department, School of Agriculture, Shiraz University, Shiraz, Iran
- 3Drought Research Center, Shiraz University, Shiraz, Iran
- 4Soil Science, Institute of Crop Science and Resource Conservation, University of Bonn, Bonn, Germany
- 5Organic Farming and Cropping Systems, University of Kassel, Witzenhausen, Germany
- 6Directorate, Leibniz Centre for Agricultural Landscape Research (ZALF), Müncheberg, Germany
- 7Crop Science, Thaer-Institute of Agricultural and Horticultural Sciences, Humboldt-University of Berlin, Berlin, Germany
- 8Institute of Bio-Geosciences (IBG-2, Plant Sciences), Forschungszentrum Jülich GmbH, Jülich, Germany
- 9Blaustein Institutes for Desert Research, Ben Gurion University of the Negev, Beer Sheva, Israel
- 10Plant Nutrition Group, Institute of Crop Science and Resource Conservation, University of Bonn, Bonn, Germany
- 11Institute for Bio- and Geosciences (IBG-3, Agrosphere), Forschungszentrum Jülich GmbH, Jülich, Germany
- 12School of BioSciences, Faculty of Science, University of Melbourne, Melbourne, VIC, Australia
- 13Crop Functional Genomics, Institute of Crop Science and Resource Conservation, University of Bonn, Bonn, Germany
- 14Emmy Noether Group Root Functional Biology, Institute of Crop Science and Resource Conservation, University of Bonn, Bonn, Germany
Plant root traits play a crucial role in resource acquisition and crop performance when soil nutrient availability is low. However, the respective trait responses are complex, particularly at the field scale, and poorly understood due to difficulties in root phenotyping monitoring, inaccurate sampling, and environmental conditions. Here, we conducted a systematic review and meta-analysis of 50 field studies to identify the effects of nitrogen (N), phosphorous (P), or potassium (K) deficiencies on the root systems of common crops. Root length and biomass were generally reduced, while root length per shoot biomass was enhanced under N and P deficiency. Root length decreased by 9% under N deficiency and by 14% under P deficiency, while root biomass was reduced by 7% in N-deficient and by 25% in P-deficient soils. Root length per shoot biomass increased by 33% in N deficient and 51% in P deficient soils. The root-to-shoot ratio was often enhanced (44%) under N-poor conditions, but no consistent response of the root-to-shoot ratio to P-deficiency was found. Only a few K-deficiency studies suited our approach and, in those cases, no differences in morphological traits were reported. We encountered the following drawbacks when performing this analysis: limited number of root traits investigated at field scale, differences in the timing and severity of nutrient deficiencies, missing data (e.g., soil nutrient status and time of stress), and the impact of other conditions in the field. Nevertheless, our analysis indicates that, in general, nutrient deficiencies increased the root-length-to-shoot-biomass ratios of crops, with impacts decreasing in the order deficient P > deficient N > deficient K. Our review resolved inconsistencies that were often found in the individual field experiments, and led to a better understanding of the physiological mechanisms underlying root plasticity in fields with low nutrient availability.
1. Introduction
Sustainable intensification of agriculture is one promising way to meet the expected global increase in demand for food, fiber, fodder, and biofuel (Godfray and Garnett, 2014). However, edaphic stresses such as drought, soil nutrient availability, high acidity, and high salinity severely limit worldwide production. Managing nutrient deficiencies may be difficult, considering that the global efficiency of fertilizer application is frequently not more than 50% for nitrogen (N), less than 10% for phosphorus (P), and about 40% for potassium (K) (Fageria, 2012). Excessive fertilization may, in turn, promote groundwater pollution and gaseous N emissions. Hereby, the European Commission targets a 20% reduction in fertilizer quantities and a 50% reduction in nutrient losses by 2030 (European Commission, 2020).
Studies focusing on roots and on their role in nutrient acquisition are crucial to lay the basis of management strategies to increase crop production while improving resource use efficiency (Gregory et al., 2013). Root systems are strongly influenced by a wide range of abiotic factors such as gravity, soil compactness, soil water content, soil texture, aeration, nutrient availability, pH, and temperature (Yapa et al., 1988; Bengough et al., 2011; Kopke et al., 2015; Schneider et al., 2017; Hartmann et al., 2018; Correa et al., 2019; Hadir et al., 2021). Biotic factors (e.g., bacteria, fungi, nematodes, etc.) can also affect biogeochemical processes and affect the root morphology in the soil (Larsen et al., 2015). And vice versa, the root exudates stimulate microbial flora activity by fostering enzyme production. The microorganism decompose the soil organic matter, and consequently, the amounts of nutrients (N, P) increase, affecting the morphological traits in roots (Barrios-Masias et al., 2019)
Root systems can exhibit a high degree of plasticity in response to physical, chemical and biological changes in the environment (Lynch, 1995; Ostonen et al., 2007; Rich and Watt, 2013; Correa et al., 2019). For example, as reviewed by Correa et al. (2019), roots showed a retarded development as sign of apparent plasticity1, including changes in architecture, as a response to severe stress (e.g. soil compaction). These architectural changes may in turn enhance the tolerance to variations in the environmental conditions (adaptive plasticity). Drew et al. (1973) showed that plants grown on nutrient-rich soil patches increased number and length of fine lateral roots, thus positively affecting the overall specific root length (SRL).
Gruber et al. (2013) grew Arabidopsis plants on agar at four deficiency levels for 12 nutrients and quantified seven root traits. Total root length increased by 48% under moderate N deficiency and decreased under most severe N deficiency. Furthermore, since the root biomass decreased comparatively less than the shoot, the root-to-shoot ratio gradually increased with decreasing N supply. In addition, N deficiency stimulated the growth of a more exploratory root system with long lateral roots. Foehse and Jungk (1983) reported that some N deficiency level stimulates root hair formation of spinach, tomato, and rape in pot experiments. Additionally, plants grown at low N displayed longer root hairs than plants grown at higher N concentrations. Moreover, when oilseed rape was grown in a split-pot system, root hairs did not form when all root system grown in media with poor N supply, whereas root hairs were formed when at least part of the roots (10%) was grown in N-rich media.
Crops cope with P deficiency by increasing root development in the P-rich zone (commonly in the topsoil) (Lynch and Brown, 2001; Rogers and Benfey, 2015), releasing carboxylates that capture iron and aluminium from the respective phosphates, thus rendering P more soluble (Hodge et al., 2009), as well as directing arbuscular mycorrhizal uptake pathways (Smith et al., 2018). Total root length generally decreases with P deficiency (Gruber et al., 2013; Haling et al., 2018), and the growth of primary and lateral roots is restrained when roots reach a low-P zone (Desnos, 2008). However, roots can also develop a shallower, horizontal, and highly branched root system (Lynch and Brown, 2001; Gruber et al., 2013; Müller et al., 2015). For example, beans develop more horizontal root angles under P-limited soil, resulting in a more extensive root area in the topsoil, where P was more concentrated than in the subsoil (Bonser et al., 1996). Another well-known mechanism to enhance the P acquisition in P-limited conditions is the increase in length and number of root hairs (Schmidt, 2001; Lambers et al., 2006).
In contrast to the numerous studies investigating root responses to N and P deficiencies, research on the effects of K deficiency in roots is scarcer. Notably, a study with Arabidopsis showed a decrease in root biomass (about 60%) and primary root length at the lowest supplied K concentration, while root-to-shoot ratios remained stable across different levels of K deficiency (Gruber et al., 2013).
The current understanding of root plasticity has been mostly derived from seedlings and pot experiments conducted in controlled environments such as greenhouses or phytochambers (López-Bucio et al., 2003; Rich and Watt, 2013). However, the root growth behaviors in those conditions are frequently different than those observed under field conditions due to several abiotic and biotic factors, which are more variable and differ significantly from those in the greenhouse (Rich and Watt, 2013; Watt et al., 2013; Heinze et al., 2016; Schittko et al., 2016; Rich et al., 2020). Plants growing in fields are usually grown in crop stands, thus interacting and competing with each other, changing their environment and that of their neighboring plants (Cahill et al., 2010; Faget et al., 2013; Weidlich et al., 2018). Thus, pot studies generally do not have the physical, chemical and microbial composition of field soils. This difference alters the growth rate and rooting depth of plants as compared to field studies (Eno and Popenoe, 1964; De Deyn et al., 2004; Passioura, 2006; Ruzicka et al., 2010; Ruzicka et al., 2012; Poorter et al., 2016; Howard et al., 2017). For instance, Mokany and Ash (2008) found a poor correlation of root biomass and root-to-shoot ratio in pot experiments vs. field conditions. Moreover, the root responses to any stress differ in pots compared to field, as shown in cassava, where the root weight and width were statistically similar under drought and irrigated conditions at field scale but different in the pot experiments (Kengkanna et al., 2019). Another limitation in pot studies is that the container shape affects root morphological characteristics. Roots of plants cultivated in smooth-sided containers can grow deformed or limit their growth because they cannot spread horizontally, as they would do in an open field, therefore, they expand vertically, wrapping up at the bottom of the pot (Amoroso et al., 2010; Oburger and Schmidt, 2016). Besides, the container influences the humidity, and ventilation of soil (Poorter et al., 2012). Consequently, transferring observations on root morphology or plasticity from pot experiments to real field conditions is usually impossible.
To overcome these limitations, we performed a systemic review and meta-analysis to analyze whether and how N, P, and K deficiencies impact root morphological traits of common arable crops under field conditions. We were particularly interested in root length, root biomass, root diameter, root hair formation and root/shoot performance indices such as root-to-shoot ratio, root length per unit of shoot biomass and specific root length.
2. Materials and methods
We used a systematic review and meta-analysis approach to show the evidence of the effects of nutrient deficiencies on roots. The approach was as follows.
2.1. Data sources and search strategy
We used the electronic databases Web of Science, Google scholar, and Wiley online library to search for articles published in peer-reviewed journals without any restriction in the year of publishing. The exact combinations used for searching keywords was:
● Root + deficiencies + nutrients + field
● Root + nitrogen + field
● Root + nitrogen + site
● Root + phosphorus + field
● Root + phosphorus + site
● Root + potassium + field
● Root + potassium + site
In addition, secondary literature cited in selected papers was also looked up and included if relevant. In total, we considered 50 studies in which root growth of common field crops under field conditions was evaluated. All the key contents about the considered studies are summarized in the Tables S1, S2, S3 of the Supplementary Material.
2.2. Selection criteria
The eligibility of the studies in this review was evaluated using the following criteria:
i. Investigation of roots, with observed data of at least one of the following traits: root growth, root length, root biomass, root-to-shoot ratio and/or root hair formation.
ii. Use of common agricultural crops.
iii. Reduction (or deficiency) of at least one of the three macro-nutrients N, P, or K, including a non-fertilized/insufficient control treatment.
iv. Experiments were conducted at a field-scale.
The exclusion criteria were:
i. Only qualitative data available.
ii. Forestry plants.
iii. Small-scale (e.g., pot or bucket experiments) or laboratory experiments (e.g., plants grown on agar).
2.3. Observed root traits
The following root traits were considered:
i. Root length and root length density (RLD)
ii. Root biomass
iii. Root mass density (RMD) or root weight density (RWD)
iv. Root length per shoot biomass
v. Root-to-shoot ratio
vi. Specific root length (SRL)
vii. Root diameter
viii. Root hair formation
ix. Speed of root growth
x. Root surface area
For definitions, please refer to the glossary provided by Freschet et al. (2021).
2.4. Data extraction
The extracted data for each study involved: i) name of the crop; ii) year of the study; iii) country of the experiment, iv) soil type; v) used method for root observation, vi) treatments; vii) effect on root morphology and distribution; and viii) effect on root length, root biomass, root diameter, shoot biomass and, root-to-shoot ratio, specific root length. Any other relevant information was also recorded and included in the text.
2.5. Estimation of the relative change of each trait due to nutrient deficiency
Besides an evaluation of the absolute trait values, the effect of the nutrient deficiency on each root trait was estimated using a relative change formula (Equation 1), where the value of the treatment without the specific nutrient was the comparison indicator.
Where X0 is the mean value of the trait (root length, biomass, etc.) without the nutrient application (e.g., 0 kg ha-1 of N) and X1 is the mean value of the trait with the nutrient addition (for example, application of 50 kg ha-1 of N). The relative change of root length, root biomass, root length per shoot biomass, root-to-shoot ratio, and diameter (if sufficient data was available) was calculated for each treatment and averaged for each study. Therefore, the mean of each study was considered as a single observation for the boxplots and the median estimation.
2.6. Statistical analysis
In order to compare the absolute values among the different studies, we normalized the absolute raw data with the following formula (Equation 2):
Where x is the absolute value of the root trait (root length, biomass, etc.) with respect to a specific nutrient availability level, Xmin is the lower bound in the values’ range (within the study and over all nutrient levels), and Xmax is the upper bound of the values’ range.
Then, we averaged the normalized data (grouped by deficiency or non-deficiency) to have two single observations per study (deficient and non-deficient). A normalized value close to 0 or 1 indicates that the value is similar to the study’s minimum or maximum values.
We then performed a t-test (t.test function of the stats R package) to compare the normalized data (one record per study if available) from deficient and non-deficient treatments and evaluate its statistical significance. The statistical analysis and all plots were created using the software R (version 4.0.2).
2.7. Considered studies
We found 32 studies that met the criteria of our search in the electronic databases and additional 18 publications cited within those studies. In total, 50 studies were analyzed in this work. We recognized that the keywords “field” and “site” were not often used in the titles or as keywords in our target studies, and thus additional papers were included through the references provided in the initially found manuscripts.
In the studies considered, the crops were grown in the USA, China, Australia, UK, Brazil, New Zealand, Iran, Costa Rica, Honduras, Canada, Mozambique, Colombia, Japan, Denmark, Germany and Belgium. Moreover, 29 out of 50 studies used fibrous root types (monocots) in their research, while the remaining 21 evaluated taproot root types (dicots). The studied crops are shown in Table 1.
3. Results
3.1. Relative change in root morphological traits under N, P, and K deficiency
Our meta-analysis revealed that root length and biomass, in most cases, decreased with increasing N, P, and K deficiency. Root length per shoot biomass and root-to-shoot ratio increased when plants were grown under N and P-deficient conditions. The specific root length was similar in nutrient-deficient and non-deficient treatments. The relative changes in root length, root biomass, root length per shoot biomass, root-to shoot ratio and specific root length under N, P and K deficiency are shown in Figure 1.
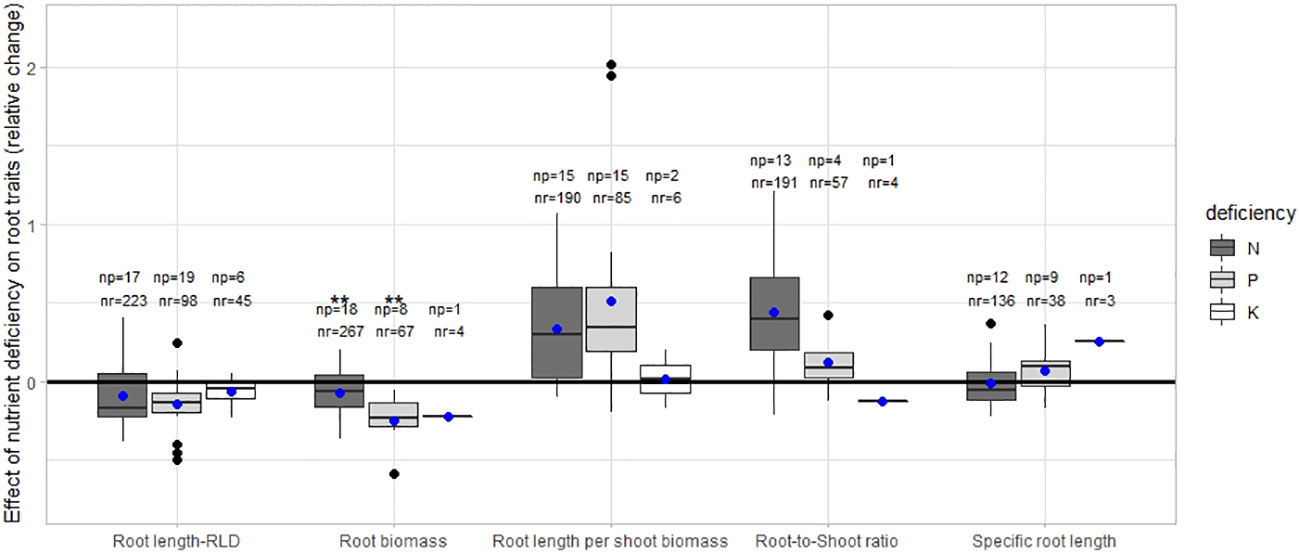
Figure 1 The relative change of the root traits under deficiency [(X0-X1)/X1] where X0 is the value in the treatment without any addition of the nutrient and X1 is the value of the treatment with the nutrient application. np stands for the number of publications/studies considered in the calculation, and nr for the total number of observations within these publications. The line within the boxes refers to the median. ** stands for significant differences at a 0.95 confidence level. Blue dots represent the mean.
The magnitude (median) of the relative changes of the different root traits was similar among dicot and monocot plants under N and P deficiency (Supplementary Figure S1). The relative change of root-to-shoot ratio was greater under P deficiency than P-added in monocots plants.
3.2. Nitrogen
The normalized root length, root biomass, root length per shoot biomass, and root-to-shoot ratio showed significant differences for N-deficient and non-deficient conditions (Figure 2). The normalized specific root length was similar in both treatments.
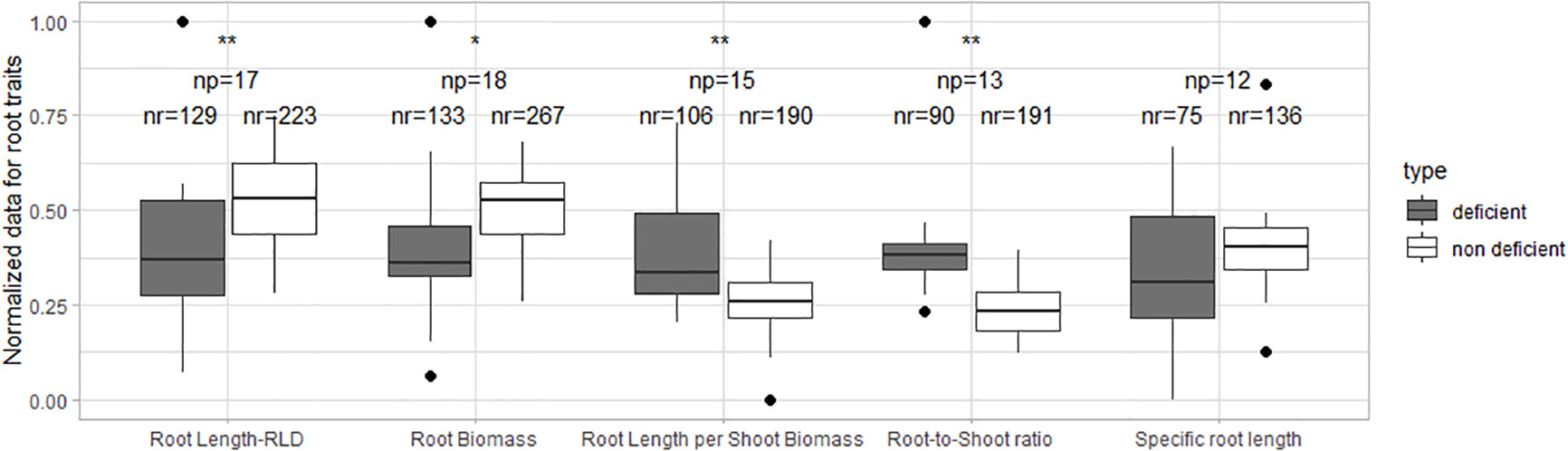
Figure 2 Boxplot of the normalized root data under N deficiency and N non-deficiency. A t-test was performed; * stands for significant differences at a 0.9 confidence level and ** at a 0.95 confidence level. np stands for the number of publications/studies considered in the calculation, and nr is the total number of observations within these publications (np).
3.2.1. Root length, root length density, and root surface area
Table 2 shows an overview of the studies that report the effects of N deficiency on the total root length or RLD. It also shows the crop, soil type, factors investigated in each study, and the overall impact. Most of the observations revealed that absolute root length and RLD were lower under conditions of N deficiency than under sufficient N supply, particularly at N02 (Barber and Mackay, 1986; Anderson, 1987; Anderson, 1988; Barraclough et al., 1989; Xue et al., 2014; Chen et al., 2020; Hadir et al., 2021; Mehrabi et al., 2021; Fang et al., 2022). This was observed for maize (Anderson, 1987; Anderson, 1988; Fang et al., 2022), winter wheat (Barraclough et al., 1989; Xue et al., 2014; Mehrabi et al., 2021), cotton and sugar beet (Chen et al., 2020; Hadir et al., 2021).
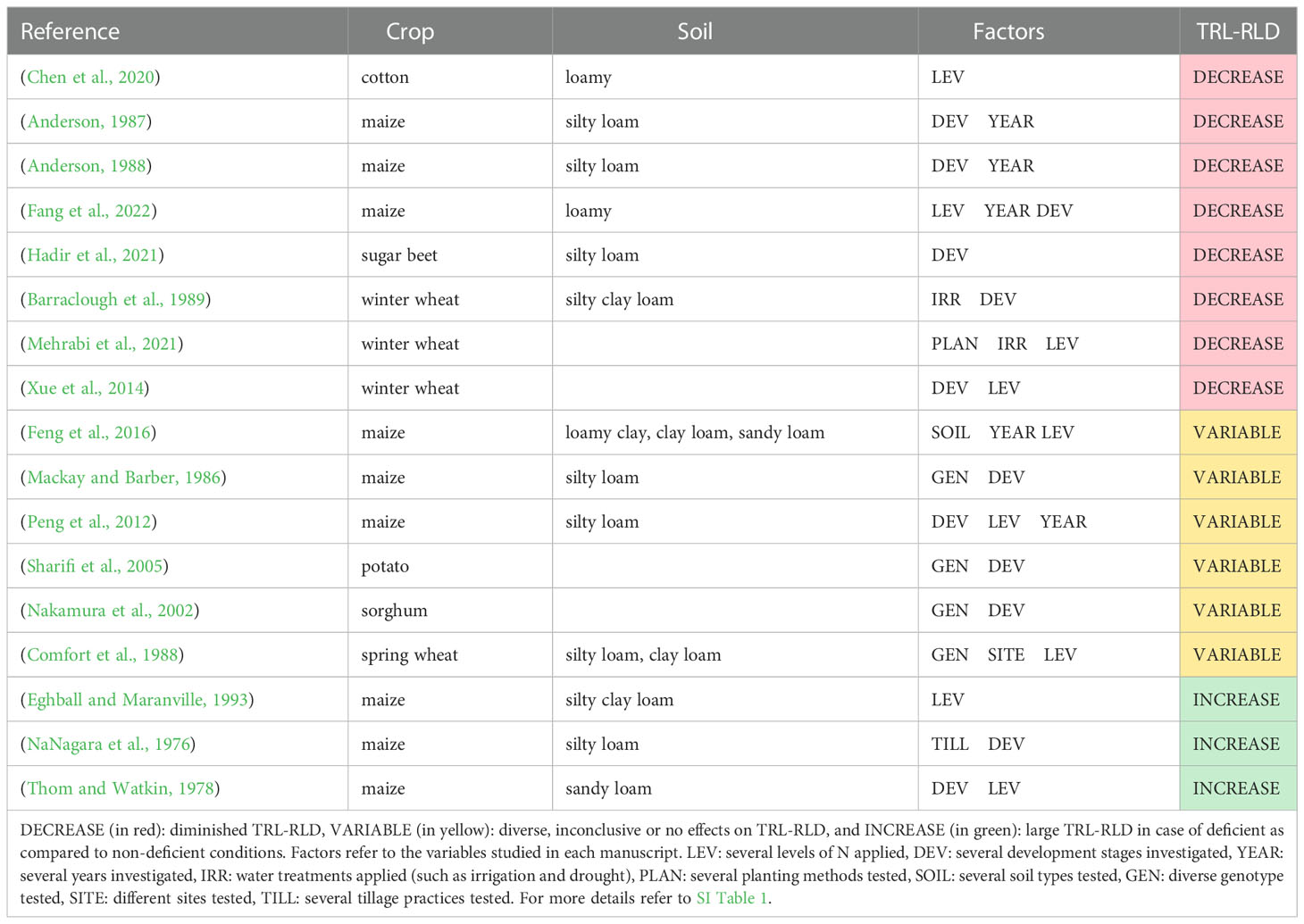
Table 2 Studies that report effects of N deficiency on total root length and/or root length density (TRL-RLD) at field scale.
Some studies reported variable effects on root length and RLD depending on the other studied factors. In this line, Mackay and Barber (1986); Sharifi et al. (2005) and Nakamura et al. (2002) reported a genotype effect of N deficiency on maize, potato and sorghum root morphology. Feng et al. (2016) and Comfort et al. (1988) observed a weak parabolic relationship between N supply and root length (in maize and spring wheat). Peng et al. (2012) outlined that the effect of N deficiency on maize root length was related to the crop’s developmental stage. N deficiency (N0) stimulated root growth in early maize growth stages, and the total root length peaked before the tasseling, followed by an early decline compared with other treatments with increasing N supply in all three years studied.
In contrast, other studies found an increase in root length in N0 treatments. NaNagara et al. (1976); Thom and Watkin (1978), and Eghball and Maranville (1993) observed increased root lengths under zero N supply treatment compared with N-fertilized treatments of maize. Moreover, NaNagara et al. (1976) found that the effects of N fertilization on root length interacted with the tillage regime and development stage.
3.2.2. Root biomass
Table 3 summarizes the main effects of N deficiency on root biomass. Most of the observations show a decrease in the total root biomass in the N0 treatment, regardless the crop (Welbank and Williams, 1968; Myers, 1980; Barraclough et al., 1989; Nakamura et al., 2002; Sharifi et al., 2005; Otto et al., 2014; Xue et al., 2014; Schneider et al., 2017; Mehrabi et al., 2021; Fang et al., 2022), crop developmental stage (Myers, 1980; Barraclough et al., 1989; Nakamura et al., 2002; Sharifi et al., 2005; Xue et al., 2014; (Welbank and Williams, 1968), genotype (Schneider et al., 2017; Fang et al., 2022), or irrigation regimens (Mehrabi et al., 2021).
Some studies found variable effects on root biomass depending on the other studied factors. Anderson (1987, 1988) reported that tillage treatments and year of cultivation affected maize root morphology differently under N deficiency. In the 3-year field experiment in three different soils types (loamy clay, clay loam, and sandy loam) conducted by Feng et al. (2016), less maize root biomass was found in N0 treatment, except in the loamy clay in one out of the three years. In another maize study, at early and grain-filling stages, plants grown under N0 conditions presented higher root dry weight than those submitted to N168 and N672 treatments (Thom and Watkin, 1978). In winter wheat, Wang et al. (2014) found that the effect of N on root weight density depended on soil water conditions. Root biomass under N deficiency reacted differently depending on the development stages (Hadir et al., 2021), level of deficiency (Chen et al., 2020), and tillage (Sainju et al., 2005).
In contrast, only one study (Eghball and Maranville, 1993) reported increased maize root biomass under N deficiency and no interactions with the maize genotype. Dry maize root weight at N0 was higher than at N60, N120, and N180.
3.2.3. Root-to-shoot ratio
Table 4 describes the effects of N deficiency on two ratios: root-to-shoot and root length per shoot biomass, including the soil type and variables investigated in each study. Most of the studies reported an increase in the root-to-shoot ratio upon N deprivation (Welbank and Williams, 1968; Welbank and Williams, 1968; Myers, 1980; Anderson, 1988; Eghball and Maranville, 1993; Sharifi et al., 2005; Wang et al., 2005; Farrior et al., 2013; Xue et al., 2014; Hadir et al., 2020), indicating a greater investment of assimilates into the belowground crop parts under low N conditions (Figure 2).
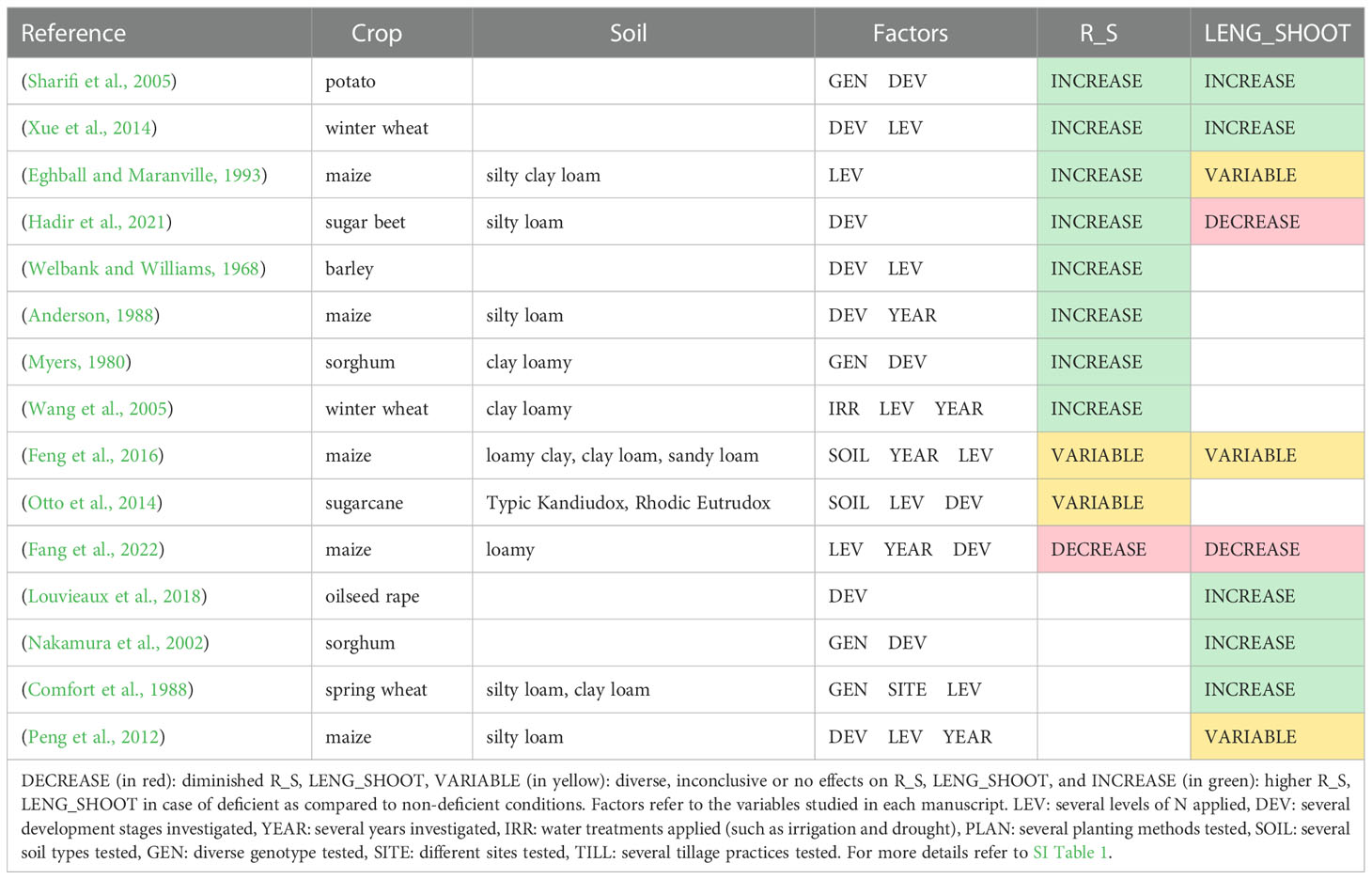
Table 4 Studies that report effects of N deficiency on the root-to-shoot ratio (R_S) and root length per shoot biomass (LENG_SHOOT) at the field scale.
Two studies reported variable effects on the root-to-shoot ratio depending on the other studied factors. Feng et al. (2016) reported that the root-to-shoot ratio of maize at silking was higher in N0, except in the loamy clay soil in one out of the three years of the study. In sugarcane, N deficiency led to a decrease in root-to-shoot ratio at the beginning of the production cycle at one out of two experimental sites. In later growth stages, the root-to-shoot ratio was similar between the treatments (Otto et al., 2014).
3.2.4. Root diameter, root diameter distribution, and specific root length
All the studies that investigated the effect of N deficiency on root diameter and specific root length are listed in Table 5. Only a few studies reported observations of root radius, root diameter, root diameter distribution, or specific root length, and a predominant effect of N treatments on these traits cannot be identified. An increase in maize average root diameter in N0 as compared to N180 was observed in a long-term experiment (Anderson, 1987). In contrast, Sharifi et al. (2005) reported no effect of low N conditions on root diameter for potato. Otherwise, a decrease in average root diameter at N0 was reported for maize (Eghball and Maranville, 1993) and sugar beet experiment (Hadir et al., 2021).
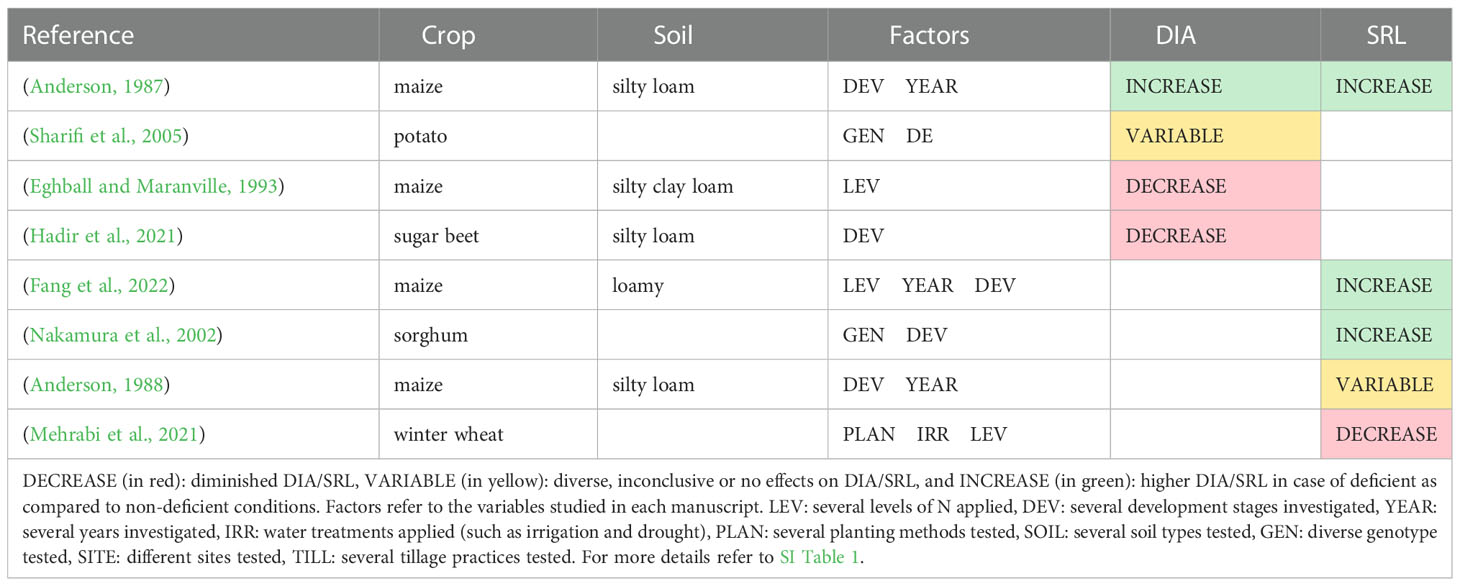
Table 5 Studies that report effects of N deficiency on the root diameter (DIA) and specific root length (SRL) at the field scale.
Higher values of specific root length at N0 were found in maize (Anderson, 1987; Fang et al., 2022) and sorghum (Nakamura et al., 2002). In contrast, Mehrabi et al. (2021) reported a smaller SRL when N was not applied.
3.2.5. Other effects on root morphology
Barber and Mackay (1986) conducted a field experiment with two different maize genotypes in two different soils. The percentage of roots with root hairs was not affected by the amounts of applied N (N0 and N227), but N0 led to a decrease in both root number and root hair length in all maize genotypes.
Schneider et al. (2021) found that maize lines with few-thick nodal roots had smaller total axial root lengths in N0, while lines with many-thin developed a greater total axial root length in N0. The phenotype of fewer, thicker nodal roots was associated with deeper root distribution and resulted in an increased shoot growth under N deficiency.
Maize showed a decrease in the speed of root growth rate (30-49% less) in the topsoil (0-25cm) but an increase (50-60% more) in the subsoil (26-80cm) in treatment N0 compared with N227 at the early growth stage (Barber and Mackay, 1986).
3.3. Phosphorus
A summary of the experimental setup and main effects of P deficiency in root morphology and topology is provided in Table S2.
Normalized data of root length, root biomass, and root length per shoot biomass differ significantly between P-deficient and non-deficient treatments (Figure 3). The differences in root-to-shoot ratio and specific root length were non-significant.
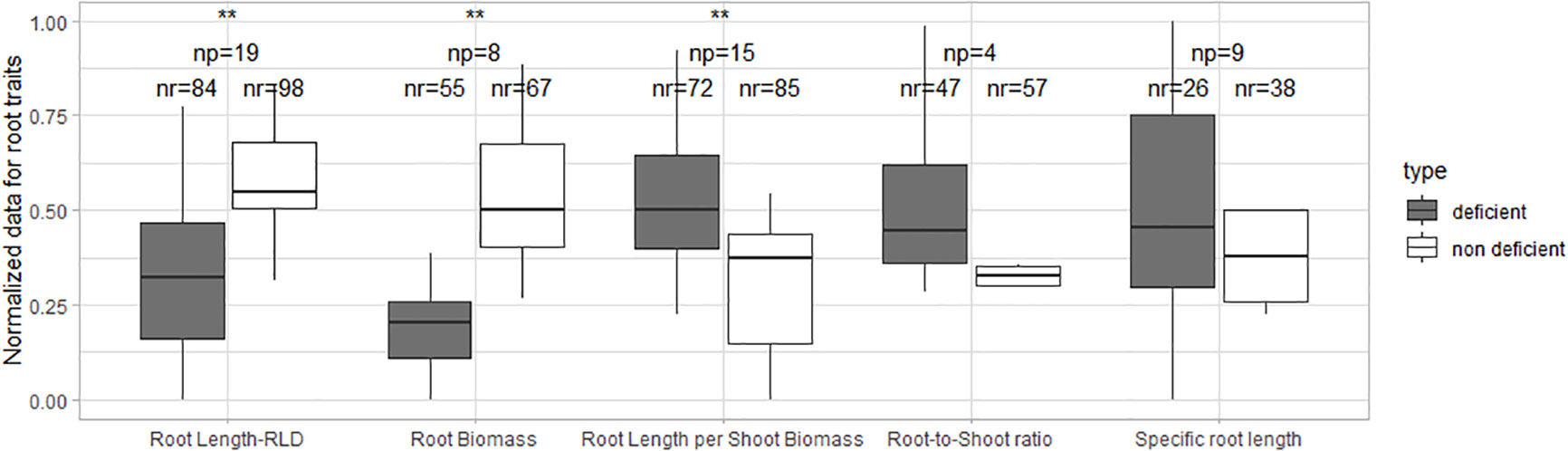
Figure 3 Boxplot of the normalized root data under P deficiency and P non-deficiency. A t-test was performed; ** stands for significant differences at a 0.95 confidence level. np stands for the number of publications/studies considered in the calculation, and nr is the total number of observations within these publications (np).
3.3.1. Root length and root length density
Table 6 summarizes the studies that report the effects of P deficiency on the total root length or RLD, describing the crop, soil type, factors investigated in each study, and the overall impact. Most studies reported a decrease in root length or root length density under P deficiency (Figure 3). This was the case for maize (Sheng et al., 2012; Zhang et al., 2012; Deng et al., 2014), oilseed rape (Duan et al., 2020), sugar beet (Hadir et al., 2020), soybean (Otani and Ae, 1996; Ao et al., 2010), common beans (Ho et al., 2005; Ochoa et al., 2006; Miguel et al., 2015), wheat (Teng et al., 2013), as well as for buckwheat, castor, peanut and sorghum (Otani and Ae, 1996). Although all these authors reported a decrease in root length under P deficiency conditions (P0), there are some particularities. For example, in two studies, several (six and eight) P levels were tested. In both cases, root length and/or RLD increased with P-fertilizer rate at first, peaked, and then either declined again in the case of wheat (Teng et al., 2013) or reached a plateau in the case of maize (Deng et al., 2014).
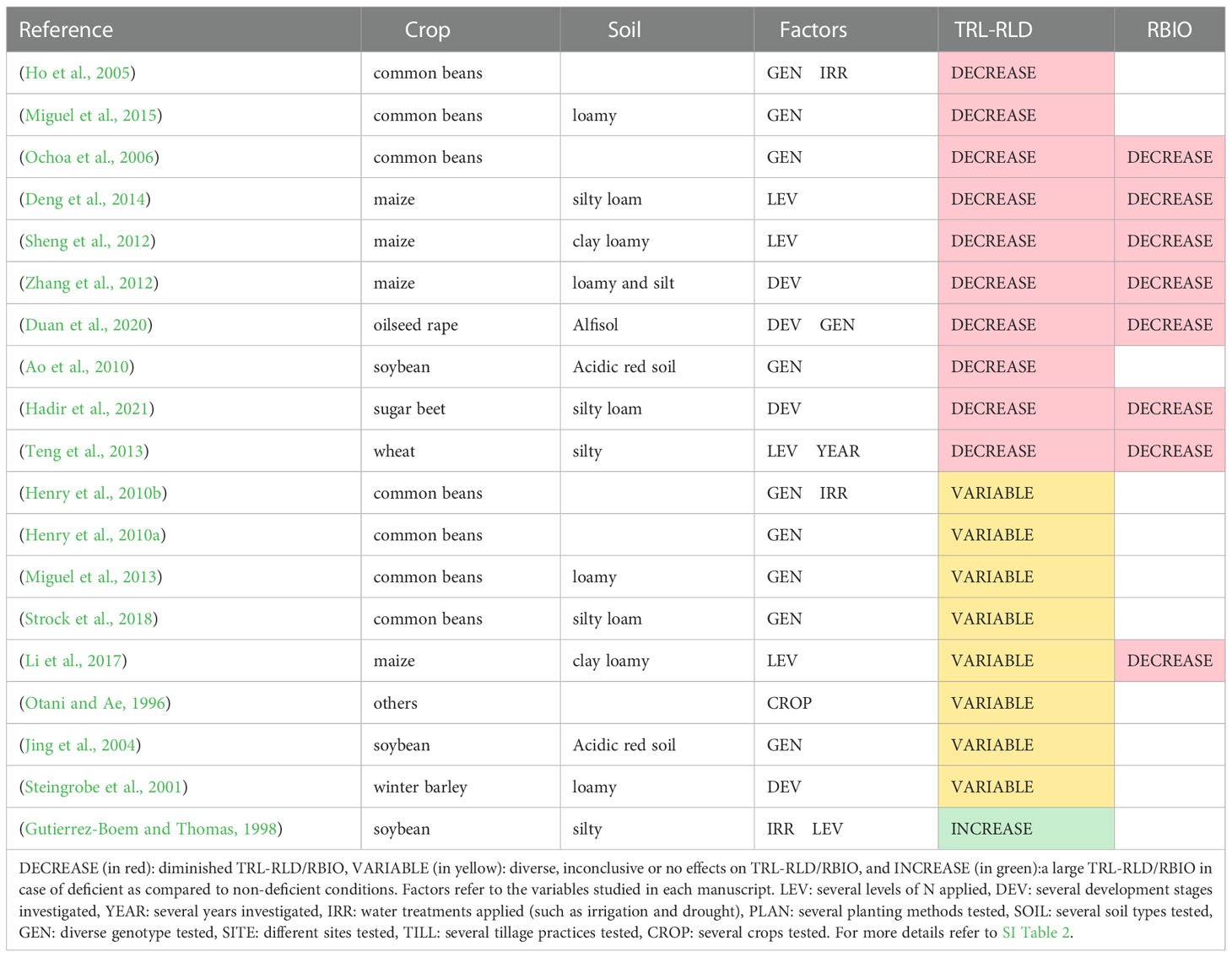
Table 6 Studies that report effects of P deficiency on total root length and/or root length density (TRL-RLD) and root biomass (RBIO) at field scale.
Some studies described that root length was not affected only by P deficiency but also by interactions with other factors. For instance, a genotype effect was found for common beans (Henry et al., 2010a; Henry et al., 2010b; Miguel et al., 2013; Strock et al., 2018) and soybean (Jing et al., 2004). In maize, the level of P deficiency caused diverse effects in RLD (Li et al., 2017). Moreover, the root length of winter barley reacted differently along the development stages at P0 (Steingrobe et al., 2001).
On the contrary, only one study in soybean reported an increase in the root length density in P0, particularly at the topsoil. Nevertheless, no differences were observed in the subsoil (Gutierrez-Boem and Thomas, 1998).
3.3.2. Root biomass
In most studies, deficiency of P supply decreased absolute root biomass (Figure 1), as found in maize (Sheng et al., 2012; Zhang et al., 2012; Deng et al., 2014; Li et al., 2017), oilseed rape (Duan et al., 2020), sugar beet (Hadir et al., 2021), wheat (Teng et al., 2013) and common bean (Ochoa et al., 2006). However, a P oversupply could also decrease the root biomass. For instance, in the studies with maize (Deng et al., 2014; Li et al., 2017) and winter wheat (Teng et al., 2013) where several P levels were tested, root dry weight initially increased with increasing soil P supply, reaching its peak and then gradually declined in case of oversupply of P.
3.3.3. Root-to-shoot ratio
Few studies reported the effect of P deficiency on the root-to-shoot ratio (Table 7); therefore, it is not possible to conclude about the effect of P deficiency on this trait. An increase in root-to-shoot in P0 compared to high P treatments was found for wheat (Teng et al., 2013) and maize (Deng et al., 2014). In oilseed rape, the root-to-shoot ratio was higher or smaller depending on the genotype under P stress (Duan et al., 2020). Only one study (in sugar beet) reported a decrease in the root-to-shoot ratio under P deficiency (Hadir et al., 2021).
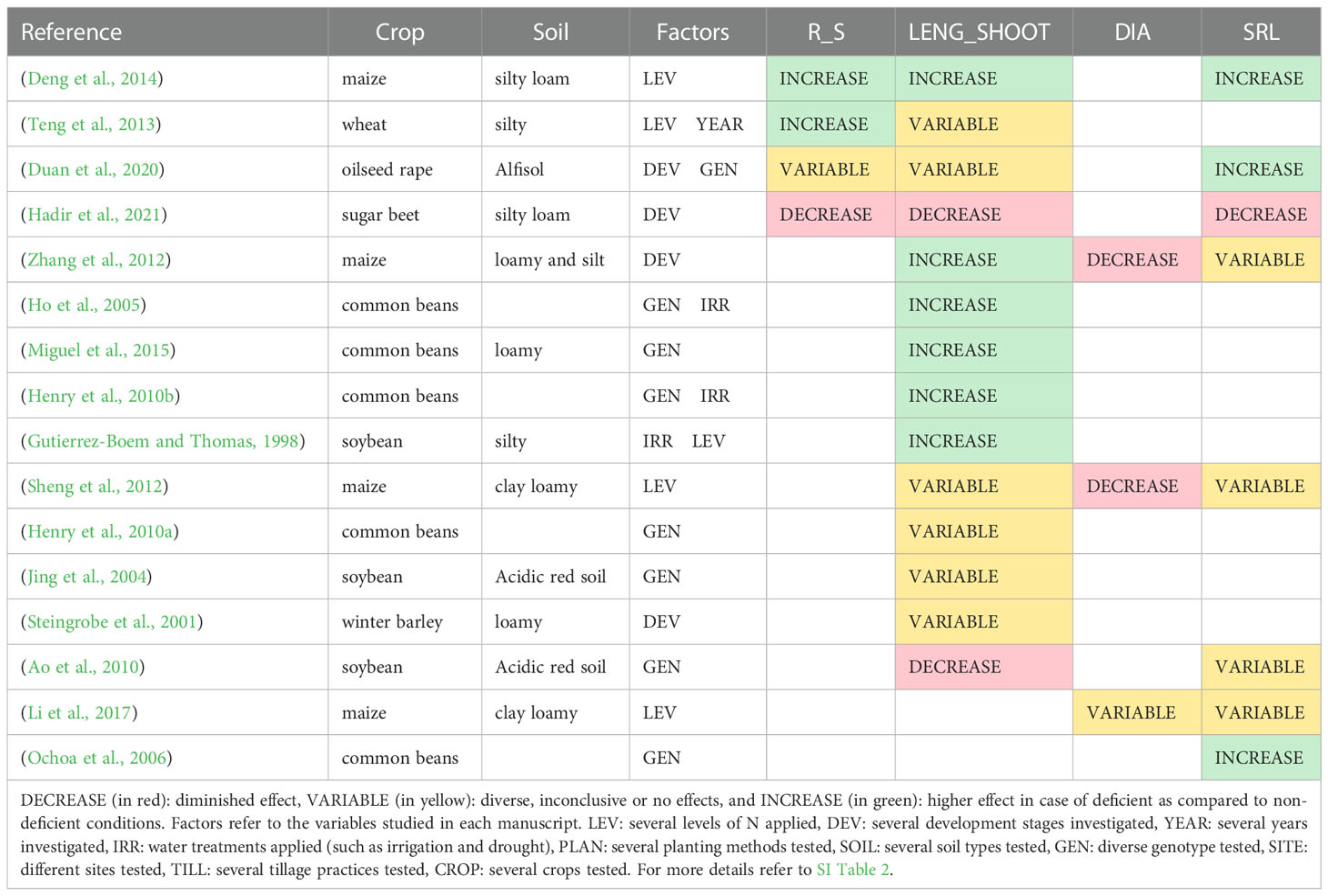
Table 7 Studies that report effects of P deficiency on the root-to-shoot ratio (R_S), root length per shoot biomass (LEGN_SHOOT), root diameter (DIA) and specific root length (SRL) at the field scale.
3.3.4. Root diameter, root diameter distribution, and specific root length
Few studies reported the effect of P deficiency on root diameter distribution (Table 7). In maize, a decrease in root diameter was observed in P0 compared to the plants that received P fertilizer at the vegetative stage, jointing, and silking (Sheng et al., 2012; Zhang et al., 2012). On the other hand, Li et al. (2017) found no differences in the maize mean root diameter among the tested P treatments.
A P deficiency led to a higher specific root length in oilseed rape (Duan et al., 2020), in maize (Deng et al., 2014), and in common bean (Ochoa et al., 2006) (Table 7). However, in maize, some specificities were found; for instance, Li et al. (2017) observed a higher SRL in P0 compared with P35 but lower compared with P18. On the contrary, Sheng et al. (2012) reported lower maize SRL in P0 compared with P18 but higher in P35, and Zhang et al. (2012) observed a higher SRL in P0, except before flowering. In soybean, the SRL increased in one genotype under low P and decreased in the other (Ao et al., 2010). Furthermore, in sugar beet, the SRL was smaller in the P0 treatment in a long-term field experiment (Hadir et al., 2021).
3.3.5. Other effects on root morphology
Zhu et al. (2010) found that genotypes with long root hairs under low P availability had significantly higher plant growth, P uptake, specific P absorption rates, and lower metabolic cost-benefit ratios than short-haired genotypes. In this work, root hairs were also longer in the low P treatment.
An increment in relative basal root fraction in common beans at low P was observed by Ho et al. (2005).
Steingrobe et al. (2001) grew winter barley in plots that had received 0 and 44 kg P ha-1 over 14 years. The authors observed a faster root production (root dry weight increment per shoot increment) of winter barley in treatments with P0 compared with P44 in all the vegetative stages.
3.4. Potassium
Only six studies that investigated the effect of K deficiency on root growth were identified. A summary of their setup and major findings are described in Supplementary Table 3.
Normalized data of root length and root length per shoot biomass did not show significant differences in these traits between K-deficient and non-deficient treatments (Figure 4). Studies of K deficiency did not provide enough data on root biomass, root-to-shoot ratio, and specific root length to perform statistical analysis. However, some effects are described in the sections below.
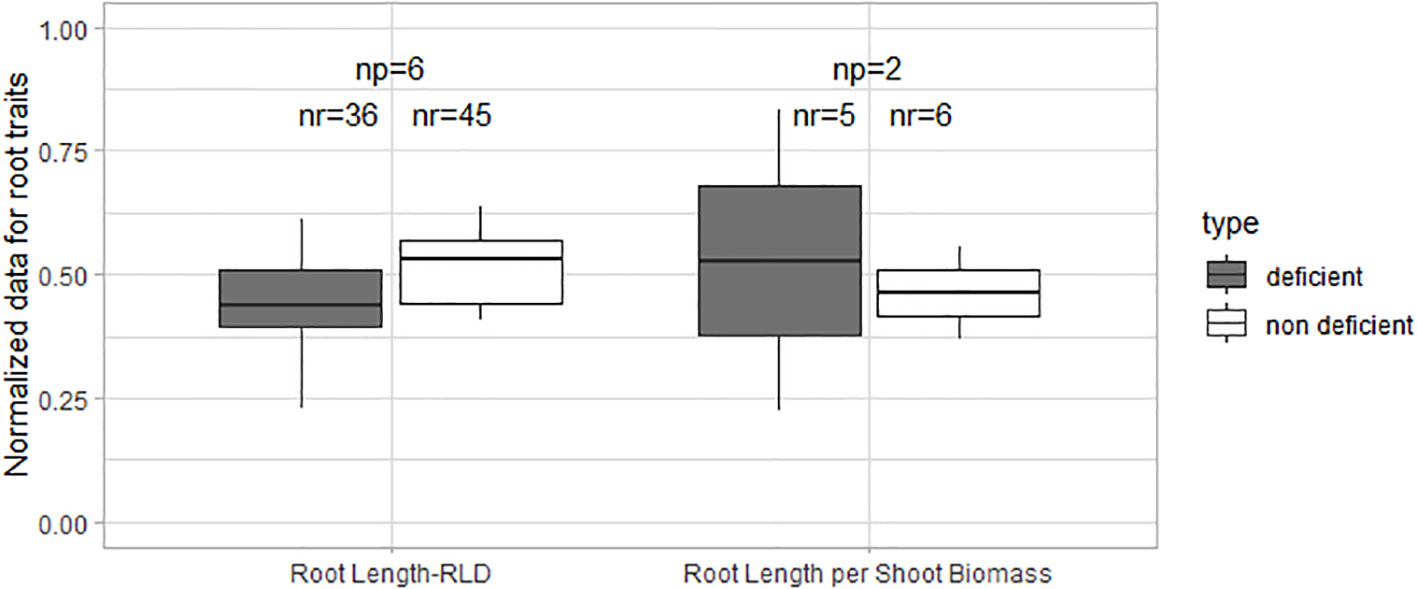
Figure 4 Boxplot of the normalized root data under K deficiency and K non-deficiency. A t-test was performed; no significant differences were found. np stands for the number of publications/studies considered in the calculation and nr the total number of observations within these publications (np).
3.4.1. Root length and root length density
Most studies reported smaller (but not significant) root lengths or RLD under low K conditions (Table 8). For example, in cotton (Mullins et al., 1994), in sugar beet (Hadir et al., 2020), millet (Valadabadi and Farahani, 2009; Zhao et al., 2016), and maize (Zhao et al., 2016).
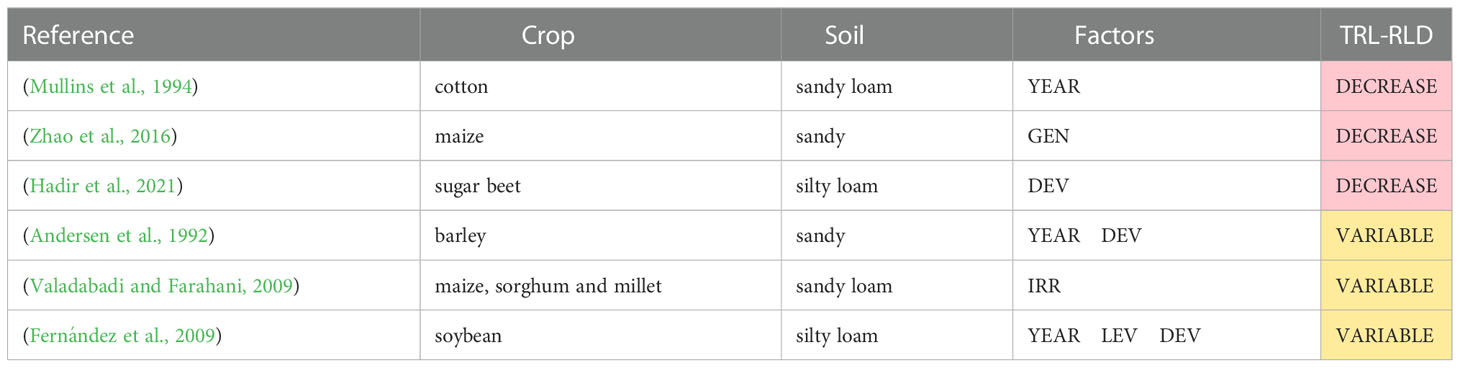
Table 8 Studies that report effects of K deficiency on total root length and/or root length density (TRL-RLD) at field scale.
Some studies found variable effects on root length and RLD depending on the other studied factors. In barley, Andersen et al. (1992) did not detect significant differences between the medium and high K treatments (K50 and K200) in one year, while in the other year, the root density in the subsoil layers significantly increased by application of high K amounts (K200). In soybean, Fernández et al. (2009) found longer root lengths under low K conditions compared with medium and high K treatments in one of the two years of the experiment, andthe root length was smaller in low K treatments in the second year.
3.4.2. Root biomass, root-to-shoot ratio, and root diameter
K0 led to a decrease in sugar beet root biomass and root-to-shoot ratio in a long-term field experiment (Hadir et al., 2021).
In soybean, a decrease in the average root diameter in low K conditions was observed throughout the growing period (Fernández et al., 2009) and at the seedling and shooting stages (Zhao et al., 2016). The average root diameter was similar in booting and tasseling in the study of Zhao et al. (2016).
3.5. Summary of the effects of nutrient deficiencies on root morphological traits
Figure 5 summarizes the effects of nutrient deficiencies on five root traits evaluated in this study based on the relative change and normalized values of root traits. Also, the factors that influence contradictory effects in field experiments are listed (Figure 5).
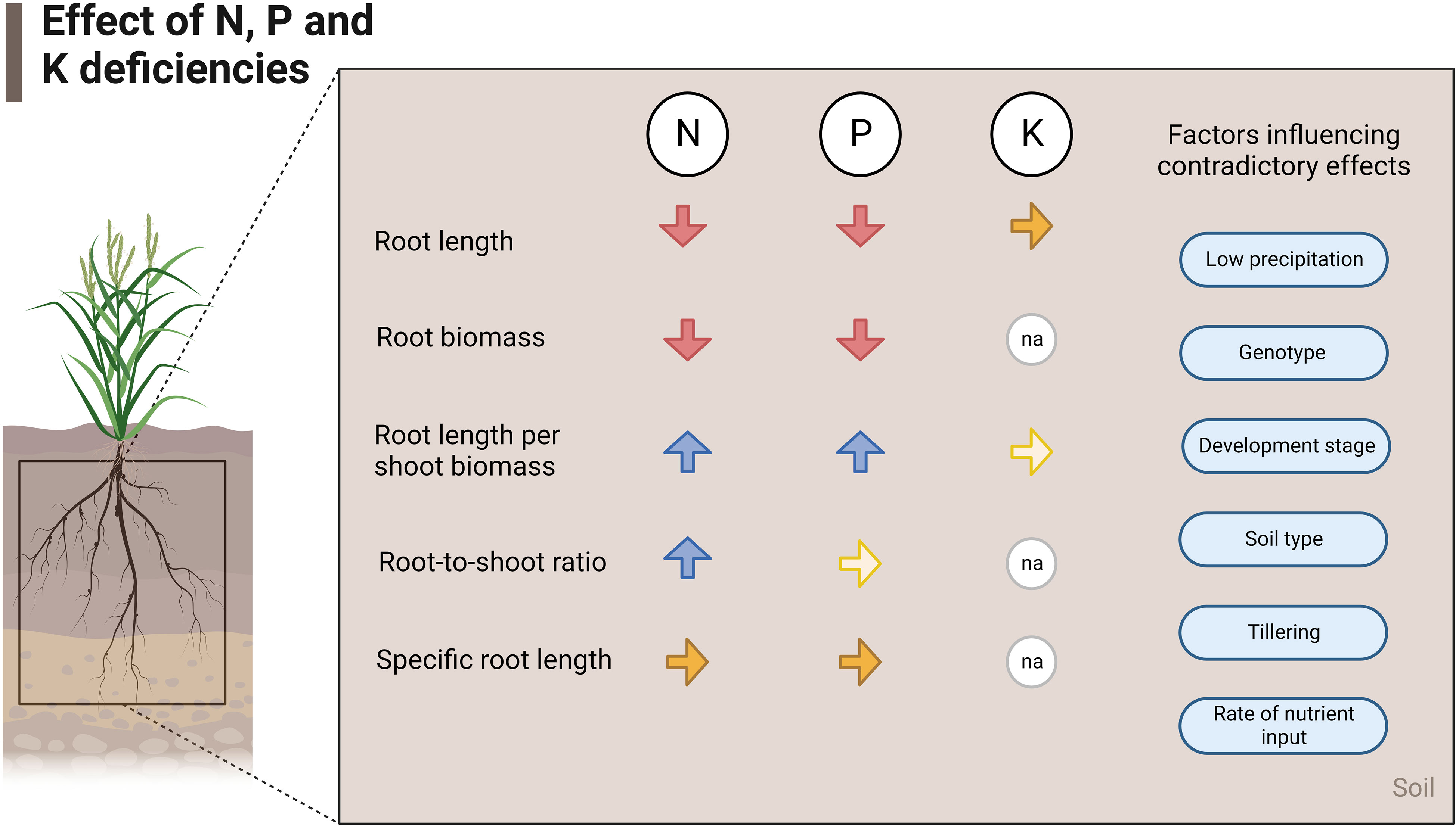
Figure 5 Effects of N, P, and K deficiencies at field scale. The red arrows show a decrease, the blue arrows show an increase and the yellow arrows show similarity in that trait in case of deficiency of the respective nutrient. Lighter-colored arrows stand for few studies found investigating that specific parameter (2-4 studies), and na stands for not applicable.
N and P deficiencies in field crop production frequently lead to the reduction of absolute root length, RLD, and absolute root biomass but to an increase of root length per shoot biomass (Figures 1, 5). Moreover, the root-to-shoot ratio increased under low N conditions. Few studies investigated the effects of low P on root-to-shoot, and no statistical differences were found in the normalized data between P deficient and non-deficient treatments. Specific root length was also statistically similar under N and P-deficiency and non-deficiency treatments. The lack of studies on the effects of K deficiency on root morphology limited the assessment of all the traits covered in this review. However, the available data showed that root length and root length per shoot biomass were similar in control and K-sufficient treatments.
4. Discussion
The spatial-temporal fluctuations and occurrences of nutrients in the soil are monitored by sensory mechanisms at root tips. This information triggers chemical signals which may shape root growth (Asim et al., 2020). The decrease in root length and root biomass upon N and P deficiency (see also Figures 1-3) seems to be a general property of root morphological plasticity. The low N and P availability negatively affects the above-ground part of the plant, including the leaf area and the photosynthetic capacity per unit of leaf area, consequently leading to a decrease in carbohydrates to be invested in root growth (Postma et al., 2014). Initially, a reduction in photosynthesis might be offset by an increase in the allocation of photosynthates to roots in order to maintain root growth. However, this resource relocation leads to a more pronounced shoot growth reduction, possibly limiting light capture and photosynthesis even more. Eventually, the smaller plants cannot sustain proper root and shoot growth, and absolute root length and biomass decrease.
Noteworthy, the above-mentioned general trend has exceptions. Some studies reported plants with longer roots in low nutrient conditions. In principle, the increase in root length could be a temporary effect in the early development stages (Peng et al., 2012; Xue et al., 2014). On the other hand, it could be that early investment in root growth under low nutrient conditions represents an advantageous strategy to cope with nutrient deprivation, e.g., as a tool to forage into the subsoil (Jia et al., 2022). Several reports indicate that the contribution of subsoil nutrients to overall uptake can be quite variable (Kautz et al., 2013) and also depend on other factors. Those include penetration resistance (Schneider et al., 2017), water distribution in the soil, as well as the availability of other nutrients, e.g., N abundancy when P is deficient (Bauke et al., 2017)
An apparent effect of N and P deficiencies on root morphology is the higher ratio between root length and shoot biomass. This may be explained by the enormous negative impact of N and P starvation on above-ground biomass, estimated at about 34% of shoot biomass decrease when N or P is deficient. Indeed, the root length also decreases due to the N and P deficiency, but not as much as the shoot biomass. Our review shows a decrease in root length of about 20% for N deficiency and 15% for P deficiency (Figure 1), which is lower than the decrease in above-ground biomass.
Most of the root-to-shoot ratios (Figure 2) increased under N deficiency. It is well known that the root-to-shoot ratio increases under N deficiency due to the concept of functional equilibrium. Competition for carbohydrates and nitrogenous compounds regulates root-to-shoot ratios. For example, when plants are changed from a non-N environment to an N environment with sufficient N supply, the shoot increases its growth in the short term, switching to a lower root-to-shoot ratio and delaying the root growth (Ågren and Ingestad, 2006). On the other hand, when the plant is transferred from a high N level to a zero N level, a non-equilibrium scenario appears; in the beginning, the ratio does not change much as long as free nitrate is available in the tissue, but when the internal nitrate content is depleted, the redistribution of organic-N determines the growth rate (Brouwer, 1983). In that scenario, root growth increases gradually more than shoot growth (Brouwer, 1983). In the end, shoot growth decreases when all the compounds are in N equilibrium. When the plants grow in a prolonged N-deficiency environment, the response to a renewed supply of N decreases (Brouwer, 1983).
Greenhouse (Horst et al., 1996; Shen et al., 2018) and lab studies (Rychter and Randall, 1994; Mollier and Pellerin, 1999; Ciereszko et al., 2011) have shown that the root-to-shoot ratio increases in low P conditions. However, our study could not confirm this finding, possibly due to the sample size (only three studies) which was too small to compare the effect between different conditions.
Specific root length was not affected by N or P deficiency consistently. For example, Ostonen et al. (2007) found a higher SRL in treatments with low nutrient levels. However, this finding was related only to the finest roots, and our review lacks the differentiation of root types. Poorter and Ryser (2015) have analyzed the response of specific leaf area (SLA) to light constraints and the specific root length (SRL) to nutrient availability constraints, as a similar response to constraints above and below ground crop parts, respectively. The changes in SRL were not as significant as SLA changes. However, by separating the root types by function (primary roots from lateral roots), the authors found that low nutrient levels positively affect the SRL of the lateral roots, which are supposedly most active in resource acquisition.
Due to a lack of data, our study can only conclude one consistent result with respect to the effect of K, which is the reduction in root length under K deficiency conditions. This observation can be explained, as in the case of N and P deficiency, with the lower availability of assimilates when K availability is reduced.
Interestingly, the root types monocot and dicot do not only share similar root morphology responses to N and P deficiency but also do so in similar magnitude (see Supplementary Figure S1), despite the differences in their root systems. However, some discrepancies exist in the relative change of root-to-shoot under P deficiency, which was be similar in dicot plants but greater in monocot plants compared with P-added soils. Under K deficiency, the data collected did not support a firm conclusion about the root morphology; however, the decrease in root length differed in magnitude between monocot (approx. 10%) and dicot (approx. 2%). It is similar to the study of Samal et al. (2010), who found a contrast in the magnitude of decrease among some crops tested under K deficiency. Therefore, despite the differences in the root architecture among crops and root types, it is highly likely that the fundamental regulators and sensing mechanisms are similar among monocot and dicot species.
To the best of our knowledge, this study considered all retrievable publications investigating root morphology in common crops at the field scale. Publications involved many soil types, weather conditions, management strategies, and genotypes. Furthermore, we showed findings contradictory to pot experiments and revealed the strengths of field-scale studies. Moreover, due to the meta-analysis of individual observations in each publication, we were able to quantify and statistically support the decrease in root length and biomass and the increase in root length per shoot biomass in low N and P environments. Our study had some limitations, though. None of the studies provided data on all the parameters we investigated. However, some studies had the data needed (such as root biomass, shoot biomass and root length) to calculate root-to-shoot data, root length per shoot biomass, and specific root length. We could calculate these ratios for a better comprehension of the deficiency response.
Nevertheless, the most critical limitation was the incompleteness of information about soil properties and nutrient concentration in the soils and crops in many studies. In this regard, our approach was to classify soil as “deficient” when the nutrient was not applied (0 kg ha-1), which is not necessarily true depending on the soil nutrient content and the needs of a specific crop. Hence, the unfertilized treatment may or may not lead to nutrient deficiency.
Furthermore, our study did not address relevant interactions that may have an impact on the root morphology in the field, for instance, drought, soil temperature, and soil pH. They remain as open questions for further studies. Additionally, studies did not report about root-soil contact and interaction of roots with the rhizosphere microbiome and potential consequences for plant nutrient acquisition (Wendel et al., 2022) which remains a research gap.
5. Conclusions
Our study contributes to the knowledge about root adaptation to nutrient-deficient soils. We detected common mechanisms for how root morphology responds to N, P, and K deficiency, even though roots experience multiple interactions simultaneously in the field. Our main findings point out a decrease in root length and biomass but an increase in root length per shoot biomass and root-to-shoot ratio. These findings are particularly interesting for modelling of root growth and agroecosystem, which requires data about the changes in root traits under different nutrient conditions. Future work must now focus on elucidating interactions of nutrient-driven changes in root architectures with other environmental parameters, such as drought, temperature, the soil microbiome, or soil type. Particular focus could be lain on root nutrient plasticity at field scale, since its assessment with high temporal and spatial resolution is nowadays possible with the emerging non-invasive technologies for root phenotyping.
Data availability statement
The original contributions presented in the study are included in the article/Supplementary Material. Further inquiries can be directed to the corresponding author.
Author contributions
GL, SS, JP contributed to the conception and design of the study. ASt, GL, SA, SS contributed to the search in scientific databases. GL organized the database, extracted the information, and made calculations and statistical analyses. GL, SS wrote the first draft of the manuscript. JP, WA, GS wrote sections of the manuscript. MA, FE, TG, MG, TK, SR, ASc, MW, PY contributed to improving and correcting the text and figures/tables. All authors contributed to the manuscript revision, read and approved the submitted version.
Funding
The presented study has been funded by the Deutsche Forschungsgemeinschaft (DFG, German Research Foundation) under Germany’s Excellence Strategy-EXC 2070-390732324 (PhenoRob) and by the German Federal Ministry of Education and Research (BMBF) in the framework of the funding measure ‘Soil as a Sustainable Resource for the Bio economy - BonaRes’, project BonaRes (Module A): BonaRes Center for Soil Research, subproject ‘Sustainable Subsoil Management - Soil3’ (grant 031B0151A).
Acknowledgments
Prof MW holds the Adrienne Clarke Chair of Botany, which is supported through the University of Melbourne Botany Foundation. We thank Marília Kamleitner, Department of Plant Nutrition, Institute of Crop Science and Resource Conservation, University of Bonn, for critically reading this manuscript. Figure 5 was created with BioRender.com.
Conflict of interest
Authors JP and AS were employed by company Forschungszentrum Jülich GmbH.
The remaining authors declare that the research was conducted in the absence of any commercial or financial relationships that could be construed as a potential conflict of interest.
Publisher’s note
All claims expressed in this article are solely those of the authors and do not necessarily represent those of their affiliated organizations, or those of the publisher, the editors and the reviewers. Any product that may be evaluated in this article, or claim that may be made by its manufacturer, is not guaranteed or endorsed by the publisher.
Supplementary material
The Supplementary Material for this article can be found online at: https://www.frontiersin.org/articles/10.3389/fpls.2022.1067498/full#supplementary-material
Footnotes
- ^ Plasticity is defined as the reorganization of the root architecture in response to one or several external disturbances that affect and impact the root morphology (Correa et al., 2019).
- ^ Treatment description: N0 stands for no N fertilizer applied, N(N supply level) stands for the amount of N applied in kg ha-1 (e.g. N150: 150 kg N ha-1 were applied). This is similar for P and K nutrients (e.g. P0, P44, K0, K30).
References
Ågren, G., Ingestad, T. (2006). Root: Shoot ratio as a balance between nitrogen productivity and photosynthesis. Plant Cell Environ. 10, 579–586. doi: 10.1111/1365-3040.ep11604105
Amoroso, G., Piero, F., Riccardo, P., Ferrini, F., Fini, A., Faoro, M. (2010). Effect of container design on plant growth and root deformation of littleleaf linden and field elm. HORTSCIENCE 45, 1824–1829. doi: 10.21273/HORTSCI.45.12.1824
Andersen, M. N., Jensen, C. R., Lösch, R. (1992). The interaction effects of potassium and drought in field-grown barley. i. yield, water-use efficiency and growth. Acta Agricult. Scand. Section B - Soil Plant Sci. 42, 34–44. doi: 10.1080/09064719209410197
Anderson, E. L. (1987). Corn root growth and distribution as influenced by tillage and nitrogen fertilization. Agron. J. 79, 544–549. doi: 10.2134/agronj1987.00021962007900030029x
Anderson, E. L. (1988). Tillage and n fertilization effects on maize root growth and root:shoot ratio. Plant Soil 108, 245–251. doi: 10.1007/BF02375655
Ao, J., Fu, J., Tian, J., Yan, X., Liao, H. (2010). Genetic variability for root morph-architecture traits and root growth dynamics as related to phosphorus efficiency in soybean. Funct. Plant Biol. 37, 304. doi: 10.1071/FP09215
Asim, M., Ullah, Z., Xu, F., An, L., Aluko, O. O., Wang, Q., et al. (2020). Nitrate signaling, functions, and regulation of root system architecture: Insights from arabidopsis thaliana. Genes (Basel) 11, E633. doi: 10.3390/genes11060633
Barber, S., Mackay, A. (1986). Root growth and phosphorus and potassium uptake by two corn genotypes in the field. Fertilizer Res. 10, 217–230. doi: 10.1007/BF01049351
Barraclough, P. B., Kuhlmann, H., Weir, A. H. (1989). The effects of prolonged drought and nitrogen fertilizer on root and shoot growth and water uptake by winter wheat. J. Agron. Crop Sci. 163, 352–360. doi: 10.1111/j.1439-037X.1989.tb00778.x
Barrios-Masias, F. H., Lazcano, C., Hernandez-Espinoza, L. H. (2019). “Advances in understanding vegetable physiology: root systems as the next frontier in improving sustainable vegetable production,” in Burleigh dodds series in agricultural science. Ed. Hochmuth, G. (Burleigh Dodds Science Publishing), 3–34. doi: 10.19103/AS.2019.0045.01
Bauke, S. L., Landl, M., Koch, M., Hofmann, D., Nagel, K. A., Siebers, N., et al. (2017). Macropore effects on phosphorus acquisition by wheat roots – a rhizotron study. Plant Soil 416, 67–82. doi: 10.1007/s11104-017-3194-0
Bengough, A. G., McKenzie, B. M., Hallett, P. D., Valentine, T. A. (2011). Root elongation, water stress, and mechanical impedance: a review of limiting stresses and beneficial root tip traits. J. Exp. Bot. 62, 59–68. doi: 10.1093/jxb/erq350
Bonser, A. M., Lynch, J., Snapp, S. (1996). Effect of phosphorus deficiency on growth angle of basal roots in phaseolus vulgaris. New Phytol. 132, 281–288. doi: 10.1111/j.1469-8137.1996.tb01847.x
Brouwer, R. (1983). Functional equilibrium: sense or nonsense? NJAS 31, 335–348. doi: 10.18174/njas.v31i4.16938
Cahill, J. F., McNickle, G. G., Haag, J. J., Lamb, E. G., Nyanumba, S. M., St. Clair, C. C. (2010). Plants integrate information about nutrients and neighbors. Science 328, 1657–1657. doi: 10.1126/science.1189736
Chen, J., Liu, L., Wang, Z., Zhang, Y., Sun, H., Song, S., et al. (2020). Nitrogen fertilization increases root growth and coordinates the root–shoot relationship in cotton. Front. Plant Sci. 11. doi: 10.3389/fpls.2020.00880
Ciereszko, I., Szczygła, A., Żebrowska, E. (2011). Phosphate deficiency affects acid phosphatase activity and growth of two wheat varieties. J. Plant Nutr. 34, 815–829. doi: 10.1080/01904167.2011.544351
Comfort, S. D., Malzer, G. L., Busch, R. H. (1988). Nitrogen fertilization of spring wheat genotypes: Influence on root growth and soil water depletion. Agron. J. 80, 114–120. doi: 10.2134/agronj1988.00021962008000010025x
Correa, J., Postma, J. A., Watt, M., Wojciechowski, T. (2019). Soil compaction and the architectural plasticity of root systems. J. Exp. Bot. 70, 6019–6034. doi: 10.1093/jxb/erz383
De Deyn, G. B., Raaijmakers, C. E., van der Putten, W. H. (2004). Plant community development is affected by nutrients and soil biota. J. Ecol. 92, 824–834. doi: 10.1111/j.0022-0477.2004.00924.x
Deng, Y., Chen, K., Teng, W., Zhan, A., Tong, Y., Feng, G., et al. (2014). Is the inherent potential of maize roots efficient for soil phosphorus acquisition? PloS One 9, e90287. doi: 10.1371/journal.pone.0090287
Desnos, T. (2008). Root branching responses to phosphate and nitrate. Curr. Opin. Plant Biol. 11, 82–87. doi: 10.1016/j.pbi.2007.10.003
Drew, M. C., Saker, L. R., Ashley, T. W. (1973). Nutrient supply and the growth of the seminal root system in barley. New Phytol. 75, 479–490.
Duan, X., Jin, K., Ding, G., Wang, C., Cai, H., Wang, S., et al. (2020). The impact of different morphological and biochemical root traits on phosphorus acquisition and seed yield of brassica napus. Field Crops Res. 258, 107960. doi: 10.1016/j.fcr.2020.107960
Eghball, B., Maranville, J. W. (1993). Root development and nitrogen influx of corn genotypes grown under combined drought and nitrogen stresses. Agron. J. 85, 147–152. doi: 10.2134/agronj1993.00021962008500010027x
Eno, C. F., Popenoe, H. (1964). Gamma radiation compared with steam and methyl bromide as a soil sterilizing agent. Soil Sci. Soc. America J. 28, 533–535. doi: 10.2136/sssaj1964.03615995002800040024x
European Commission (2020) A farm to fork strategy. for a fair, healthy and environmentally-friendly food system. Available at: https://eur-lex.europa.eu/resource.html?uri=cellar:ea0f9f73-9ab2-11ea-9d2d-01aa75ed71a1.0001.02/DOC_1&format=PDF (Accessed September 15, 2022).
Faget, M., Nagel, K. A., Walter, A., Herrera, J. M., Jahnke, S., Schurr, U., et al. (2013). Root–root interactions: extending our perspective to be more inclusive of the range of theories in ecology and agriculture using in-vivo analyses. Ann. Bot. 112, 253–266. doi: 10.1093/aob/mcs296
Fang, H., Li, Y., Gu, X., Chen, P., Li, Y. (2022). Root characteristics, utilization of water and nitrogen, and yield of maize under biodegradable film mulching and nitrogen application. Agric. Water Manage. 262, 107392. doi: 10.1016/j.agwat.2021.107392
Farrior, C. E., Tilman, D., Dybzinski, R., Reich, P. B., Levin, S. A., Pacala, S. W. (2013). Resource limitation in a competitive context determines complex plant responses to experimental resource additions. Ecology 94, 2505–2517. doi: 10.1890/12-1548.1
Feng, G., Zhang, Y., Chen, Y., Li, Q., Chen, F., Gao, Q., et al. (2016). Effects of nitrogen application on root length and grain yield of rain-fed maize under different soil types. Agron. J. 108, 1656–1665. doi: 10.2134/agronj2015.0367
Fernández, F. G., Brouder, S. M., Volenec, J. J., Beyrouty, C. A., Hoyum, R. (2009). Root and shoot growth, seed composition, and yield components of no-till rainfed soybean under variable potassium. Plant Soil 322, 125–138. doi: 10.1007/s11104-009-9900-9
Foehse, D., Jungk, A. (1983). Influence of phosphate and nitrate supply on root hair formation of rape, spinach and tomato plants. Plant Soil 74, 359–368. doi: 10.1007/BF02181353
Freschet, G. T., Pagès, L., Iversen, C. M., Comas, L. H., Rewald, B., Roumet, C., et al. (2021). A starting guide to root ecology: strengthening ecological concepts and standardising root classification, sampling, processing and trait measurements. New Phytol. 232, 973–1122. doi: 10.1111/nph.17572
Godfray, H. C. J., Garnett, T. (2014). Food security and sustainable intensification. Philos. Trans. R. Soc. B: Biol. Sci. 369, 20120273. doi: 10.1098/rstb.2012.0273
Gregory, P. J., Atkinson, C. J., Bengough, A. G., Else, M. A., Fernández-Fernández, F., Harrison, R. J., et al. (2013). Contributions of roots and rootstocks to sustainable, intensified crop production. J. Exp. Bot. 64, 1209–1222. doi: 10.1093/jxb/ers385
Gruber, B. D., Giehl, R. F. H., Friedel, S., von Wirén, N. (2013). Plasticity of the arabidopsis root system under nutrient deficiencies. Plant Physiol. 163, 161–179. doi: 10.1104/pp.113.218453
Gutierrez-Boem, F. H., Thomas, G. W. (1998). Phosphorus nutrition and water deficits in field-grown soybeans. Plant and Soil. 207, 87–96.
Hadir, S., Gaiser, T., Hüging, H., Athmann, M., Pfarr, D., Kemper, R., et al. (2020). Sugar beet shoot and root phenotypic plasticity to nitrogen, phosphorus, potassium and lime omission. Agriculture 11, 21. doi: 10.3390/agriculture11010021
Hadir, S., Gaiser, T., Hüging, H., Athmann, M., Pfarr, D., Kemper, R., et al. (2021). Sugar beet shoot and root phenotypic plasticity to nitrogen, phosphorus, potassium and lime omission. Agriculture 11, 21. doi: 10.3390/agriculture11010021
Haling, R. E., Brown, L. K., Stefanski, A., Kidd, D. R., Ryan, M. H., Sandral, G. A., et al. (2018). Differences in nutrient foraging among trifolium subterraneum cultivars deliver improved p-acquisition efficiency. Plant Soil 424, 539–554. doi: 10.1007/s11104-017-3511-7
Hartmann, A., Šimůnek, J., Aidoo, M. K., Seidel, S. J., Lazarovitch, N. (2018). Implementation and application of a root growth module in HYDRUS. Vadose Zone J. 17, 170040. doi: 10.2136/vzj2017.02.0040
Heinze, J., Sitte, M., Schindhelm, A., Wright, J., Joshi, J. (2016). Plant-soil feedbacks: a comparative study on the relative importance of soil feedbacks in the greenhouse versus the field. Oecologia 181, 559–569. doi: 10.1007/s00442-016-3591-8
Henry, A., Chaves, N. F., Kleinman, P. J. A., Lynch, J. P. (2010a). Will nutrient-efficient genotypes mine the soil? effects of genetic differences in root architecture in common bean (Phaseolus vulgaris l.) on soil phosphorus depletion in a low-input agro-ecosystem in central America. Field Crops Res. 115, 67–78. doi: 10.1016/j.fcr.2009.10.004
Henry, A., Rosas, J. C., Beaver, J. S., Lynch, J. P. (2010b). Multiple stress response and belowground competition in multilines of common bean (Phaseolus vulgaris l.). Field Crops Res. 117, 209–218. doi: 10.1016/j.fcr.2010.03.004
Hodge, A., Berta, G., Doussan, C., Merchan, F., Crespi, M. (2009). Plant root growth, architecture and function. Plant Soil 321, 153–187. doi: 10.1007/s11104-009-9929-9
Ho, M. D., Rosas, J. C., Brown, K. M., Lynch, J. P. (2005). Root architectural tradeoffs for water and phosphorus acquisition. Funct. Plant Biol. 32, 737. doi: 10.1071/FP05043
Horst, W. J., Abdou, M., Wiesler, F. (1996). Differences between wheat cultivars in acquisition and utilization of phosphorus. Z. Pflanzenernaehr. Bodenk. 159, 155–161. doi: 10.1002/jpln.1996.3581590209
Howard, M. M., Bell, T. H., Kao-Kniffin, J. (2017). Soil microbiome transfer method affects microbiome composition, including dominant microorganisms, in a novel environment. FEMS Microbiol. Lett. 364, 1–8. doi: 10.1093/femsle/fnx092
Jia, Z., Giehl, R. F. H., von Wirén, N. (2022). Nutrient–hormone relations: Driving root plasticity in plants. Mol. Plant 15, 86–103. doi: 10.1016/j.molp.2021.12.004
Jing, Z., Jiabing, F., Honq, A., Yong, H., Hai, N., Yueming, H., et al. (2004). Characterization of root architecture in an applied core collection for phosphorus efficiency of soybean germplasm. Chinese Science Bulletin 49, 10. doi: 10.1360/04wc0142
Kautz, T., Amelung, W., Ewert, F., Gaiser, T., Jahn, R., Javaux, M., et al. (2013). Nutrient acquisition from arable subsoils in temperate climates: A review. Soil Biol. Biochem. 57, 1003–1022. doi: 10.1016/j.soilbio.2012.09.014
Kengkanna, J., Jakaew, P., Amawan, S., Busener, N., Bucksch, A., Saengwilai, P. (2019). Phenotypic variation of cassava root traits and their responses to drought. Appl. Plant Sci. 7, e01238. doi: 10.1002/aps3.1238
Kopke, U., Athmann, M., Han, E., Kautz, T. (2015). Optimising cropping techniques for nutrient and environmental management in organic agriculture. Sustain. Agric. Res. 4, 15–25. doi: 10.22004/ag.econ.230376
Lambers, H., Shane, M. W., Cramer, M. D., Pearse, S. J., Veneklaas, E. J. (2006). Root structure and functioning for efficient acquisition of phosphorus: Matching morphological and physiological traits. Ann. Bot. 98, 693–713. doi: 10.1093/aob/mcl114
Larsen, J., Jaramillo-López, P., Nájera-Rincon, M., González-Esquivel, C. E. (2015). Biotic interactions in the rhizosphere in relation to plant and soil nutrient dynamics. J. Soil Sci. Plant Nutr. 15, 449–463. doi: 10.4067/S0718-95162015005000039
Li, H., Mollier, A., Ziadi, N., Shi, Y., Parent, L.-É., Morel, C. (2017). The long-term effects of tillage practice and phosphorus fertilization on the distribution and morphology of corn root. Plant Soil 412, 97–114. doi: 10.1007/s11104-016-2925-y
López-Bucio, J., Cruz-Ramırez, A., Herrera-Estrella, L. (2003). The role of nutrient availability in regulating root architecture. Curr. Opin. Plant Biol. 6, 280–287. doi: 10.1016/S1369-5266(03)00035-9
Louvieaux, J., De Gernier, H., Hermans, C. (2018). “Exploiting genetic variability of root morphology as a lever to improve nitrogen use efficiency in oilseed rape,” in Engineering nitrogen utilization in crop plants. Eds. Shrawat, A., Zayed, A., Lightfoot, D. A. (Cham: Springer International Publishing), 185–206). doi: 10.1007/978-3-319-92958-3_11
Lynch, J. (1995). Root architecture and plant productivity. Plant Physiol. 109, 7–13. doi: 10.1104/pp.109.1.7
Lynch, J. P., Brown, K. M. (2001). Topsoil foraging – an architectural adaptation of plants to low phosphorus availability. Plant Soil 237, 225–237. doi: 10.1023/A:1013324727040
Mackay, A. D., Barber, S. A. (1986). Effect of nitrogen on root growth of two corn genotypes in the field. Agron. J. 78, 699–703. doi: 10.2134/agronj1986.00021962007800040028x
Mehrabi, F., Sepaskhah, A. R., Ahmadi, S. H. (2021). Winter wheat root distribution with irrigation, planting methods, and nitrogen application. Nutr. Cycl Agroecosyst 119, 231–245. doi: 10.1007/s10705-021-10120-1
Miguel, M. A., Postma, J. A., Lynch, J. P. (2015). Phene synergism between root hair length and basal root growth angle for phosphorus acquisition. Plant Physiol. 167, 1430–1439. doi: 10.1104/pp.15.00145
Miguel, M. A., Widrig, A., Vieira, R. F., Brown, K. M., Lynch, J. P. (2013). Basal root whorl number: a modulator of phosphorus acquisition in common bean (Phaseolus vulgaris). Ann. Bot. 112, 973–982. doi: 10.1093/aob/mct164
Mokany, K., Ash, J. (2008). Are traits measured on pot grown plants representative of those in natural communities? J. Vegetation Sci. 19, 119–126. doi: 10.3170/2007-8-18340
Mollier, A., Pellerin, S. (1999). Maize root system growth and development as influenced by phosphorus deficiency. J. Exp. Bot. 50, 487–497. doi: 10.1093/jxb/50.333.487
Müller, J., Toev, T., Heisters, M., Teller, J., Moore, K. L., Hause, G., et al. (2015). Iron-dependent callose deposition adjusts root meristem maintenance to phosphate availability. Dev. Cell 33, 216–230. doi: 10.1016/j.devcel.2015.02.007
Mullins, G. L., Reeves, D. W., Burmester, C. H., Bryant, H. H. (1994). In-row subsoiling and potassium placement effects on root growth and potassium content of cotton. Agron. J. 86, 136–139. doi: 10.2134/agronj1994.00021962008600010025x
Myers, R. J. K. (1980). The root system of a grain sorghum crop. Field Crops Res. 3, 53–64. doi: 10.1016/0378-4290(80)90007-6
Nakamura, T., Adu-Gyamfi, J. J., Yamamoto, A., Ishikawa, S., Nakano, H., Ito, O. (2002). Varietal differences in root growth as related to nitrogen uptake by sorghum plants in low-nitrogen environment. Food Secur. Nutrient Stressed Environ.: Exploiting Plants’ Genet. Capabilities 117–24. doi: 10.1007/978-94-017-1570-6_12
NaNagara, T., Phillips, R. E., Leggett, J. E. (1976). Diffusion and mass flow of nitrate-nitrogen into corn roots grown under field conditions 1. Agron. J. 68, 67–72. doi: 10.2134/agronj1976.00021962006800010018x
Oburger, E., Schmidt, H. (2016). New methods to unravel rhizosphere processes. Trends Plant Sci. 21, 243–255. doi: 10.1016/j.tplants.2015.12.005
Ochoa, I. E., Blair, M. W., Lynch, J. P. (2006). QTL analysis of adventitious root formation in common bean under contrasting phosphorus availability. Crop Sci. 46, 1609–1621. doi: 10.2135/cropsci2005.12-0446
Ostonen, I., Püttsepp, Ü., Biel, C., Alberton, O., Bakker, M. R., Lõhmus, K., et al. (2007). Specific root length as an indicator of environmental change. Plant Biosyst. - Int. J. Dealing all Aspects Plant Biol. 141, 426–442. doi: 10.1080/11263500701626069
Otani, T., Ae, N. (1996). Sensitivity of phosphorus uptake to changes in root length and soil volume. Agron. J. 88, 371–375. doi: 10.2134/agronj1996.00021962008800030002x
Otto, R., Franco, H. C. J., Faroni, C. E., Vitti, A. C., Oliveira, E. C. A., Sermarini, R. A., et al. (2014). The role of nitrogen fertilizers in sugarcane root biomass under field conditions. AS 05, 1527–1538. doi: 10.4236/as.2014.514164
Passioura, J. B. (2006). The perils of pot experiments. Funct. Plant Biol. 33, 1075–1079. doi: 10.1071/FP06223
Peng, Y., Li, X., Li, C. (2012). Temporal and spatial profiling of root growth revealed novel response of maize roots under various nitrogen supplies in the field. PloS One 7, e37726. doi: 10.1371/journal.pone.0037726
Poorter, H., Fiorani, F., Pieruschka, R., Wojciechowski, T., van der Putten, W. H., Kleyer, M., et al. (2016). Pampered inside, pestered outside? differences and similarities between plants growing in controlled conditions and in the field. New Phytol. 212, 838–855. doi: 10.1111/nph.14243
Poorter, H., Hler, B., van Dusschoten, D., Climent, J., Postma, J. A. (2012). Pot size matters: a meta-analysis of the effects of rooting volume on plant growth. Funct. Plant Biol. 39, 839–850. doi: 10.1071/FP12049
Poorter, H., Ryser, P. (2015). The limits to leaf and root plasticity: what is so special about specific root length? New Phytol. 206, 1188–1190. doi: 10.1111/nph.13438
Postma, J. A., Schurr, U., Fiorani, F. (2014). Dynamic root growth and architecture responses to limiting nutrient availability: linking physiological models and experimentation. Biotechnol. Adv. 32, 53–65. doi: 10.1016/j.biotechadv.2013.08.019
Rich, S. M., Christopher, J., Richards, R., Watt, M. (2020). Root phenotypes of young wheat plants grown in controlled environments show inconsistent correlation with mature root traits in the field. J. Exp. Bot. 71, 4751–4762. doi: 10.1093/jxb/eraa201
Rich, S. M., Watt, M. (2013). Soil conditions and cereal root system architecture: review and considerations for linking Darwin and weaver. J. Exp. Bot. 64, 1193–1208. doi: 10.1093/jxb/ert043
Rogers, E. D., Benfey, P. N. (2015). Regulation of plant root system architecture: implications for crop advancement. Curr. Opin. Biotechnol. 32, 93–98. doi: 10.1016/j.copbio.2014.11.015
Ruzicka, D. R., Barrios-Masias, F. H., Hausmann, N. T., Jackson, L. E., Schachtman, D. P. (2010). Tomato root transcriptome response to a nitrogen-enriched soil patch. BMC Plant Biol. 10, 75. doi: 10.1186/1471-2229-10-75
Ruzicka, D. R., Hausmann, N. T., Barrios-Masias, F. H., Jackson, L. E., Schachtman, D. P. (2012). Transcriptomic and metabolic responses of mycorrhizal roots to nitrogen patches under field conditions. Plant Soil 350, 145–162. doi: 10.1007/s11104-011-0890-z
Rychter, A. M., Randall, D. D. (1994). The effect of phosphate deficiency on carbohydrate metabolism in bean roots. Physiol. Plant 91, 383–388. doi: 10.1111/j.1399-3054.1994.tb02964.x
Sainju, U. M., Singh, B. P., Whitehead, W. F. (2005). Tillage, cover crops, and nitrogen fertilization effects on cotton and sorghum root biomass, carbon, and nitrogen. Agron. J. 97, 1279–1290. doi: 10.2134/agronj2004.0213
Samal, D., Kovar, J. L., Steingrobe, B., Sadana, U. S., Bhadoria, P. S., Claassen, N. (2010). Potassium uptake efficiency and dynamics in the rhizosphere of maize (Zea mays l.), wheat (Triticum aestivum l.), and sugar beet (Beta vulgaris l.) evaluated with a mechanistic model. Plant Soil 332, 105–121. doi: 10.1007/s11104-009-0277-6
Schittko, C., Runge, C., Strupp, M., Wolff, S., Wurst, S. (2016). No evidence that plant–soil feedback effects of native and invasive plant species under glasshouse conditions are reflected in the field. J. Ecol. 104, 1243–1249. doi: 10.1111/1365-2745.12603
Schmidt, W. (2001). From faith to fate: Ethylene signaling in morphogenic responses to p and fe deficiency. J. Plant Nutr. Soil Sci. 164, 147–154. doi: 10.1002/1522-2624(200104)164:2<147::AID-JPLN147>3.0.CO;2-B
Schneider, F., Don, A., Hennings, I., Schmittmann, O., Seidel, S. (2017). The effect of deep tillage on crop yield – what do we really know? Soil Tillage Res. 174, 193–204. doi: 10.1016/j.still.2017.07.005
Schneider, H., Yang, J., Brown, K., Lynch, J. (2021). Nodal root diameter and node number in maize (zea mays l.) interact to influence plant growth under nitrogen stress. Plant Direct 5 (3), e00310. doi: 10.1002/pld3.310
Sharifi, M., Zebarth, B. J., Hajabbasi, M. A., Kalbasi, M. (2005). Dry matter and nitrogen accumulation and root morphological characteristics of two clonal selections of ‘Russet norkotah’ potato as affected by nitrogen fertilization. J. Plant Nutr. 28, 2243–2253. doi: 10.1080/01904160500323552
Sheng, M., Lalande, R., Hamel, C., Ziadi, N., Shi, Y. (2012). Growth of corn roots and associated arbuscular mycorrhizae are affected by long-term tillage and phosphorus fertilization. Agron. J. 104, 1672–1678. doi: 10.2134/agronj2012.0153
Shen, Q., Wen, Z., Dong, Y., Li, H., Miao, Y., Shen, J. (2018). The responses of root morphology and phosphorus-mobilizing exudations in wheat to increasing shoot phosphorus concentration. AoB Plants 10, ply054. doi: 10.1093/aobpla/ply054
Smith, S., Anderson, I., Smith, F. (2018). Mycorrhizal associations and phosphorus acquisition: From cells to ecosystems. Phosphorus Metabolism in Plants. 48, 409–439. doi: 10.1002/9781119312994.apr0529
Steingrobe, B., Schmid, H., Claassen, N. (2001). Root production and root mortality of winter barley and its implication with regard to phosphate acquisition. Plant and Soil 237, 239–248.
Strock, C. F., Morrow de la Riva, L., Lynch, J. P. (2018). Reduction in root secondary growth as a strategy for phosphorus acquisition. Plant Physiol. 176, 691–703. doi: 10.1104/pp.17.01583
Teng, W., Deng, Y., Chen, X.-P., Xu, X.-F., Chen, R.-Y., Lv, Y., et al. (2013). Characterization of root response to phosphorus supply from morphology to gene analysis in field-grown wheat. J. Exp. Bot. 64, 1403–1411. doi: 10.1093/jxb/ert023
Thom, E. R., Watkin, B. R. (1978). Effect of rate and time of fertiliser nitrogen application on total plant, shoot, and root yields of maize ( Zea mays l.). New Z. J. Exp. Agric. 6, 29–38. doi: 10.1080/03015521.1978.10426010
Valadabadi, S. A., Farahani, H. A. (2009). Studying the interactive effect of potassium application and individual field crops on root penetration under drought condition. J. Agric. Biotechnol. Sustain. Dev. 2, 5.
Wang, C., Liu, W., Li, Q., Ma, D., Lu, H., Feng, W., et al. (2014). Effects of different irrigation and nitrogen regimes on root growth and its correlation with above-ground plant parts in high-yielding wheat under field conditions. Field Crops Res. 165, 138–149. doi: 10.1016/j.fcr.2014.04.011
Wang, Y., Mi, G., Chen, F., Zhang, J., Zhang, F. (2005). Response of root morphology to nitrate supply and its contribution to nitrogen accumulation in maize. J. Plant Nutr. 27, 2189–2202. doi: 10.1081/PLN-200034683
Watt, M., Moosavi, S., Cunningham, S. C., Kirkegaard, J. A., Rebetzke, G. J., Richards, R. A. (2013). A rapid, controlled-environment seedling root screen for wheat correlates well with rooting depths at vegetative, but not reproductive, stages at two field sites. Ann. Bot. 112, 447–455. doi: 10.1093/aob/mct122
Weidlich, E. W. A., Temperton, V. M., Faget, M. (2018). Neighbourhood stories: role of neighbour identity, spatial location and order of arrival in legume and non-legume initial interactions. Plant Soil 424, 171–182. doi: 10.1007/s11104-017-3398-3
Welbank, P. J., Williams, E. D. (1968). Root growth of a barley crop estimated by sampling with portable powered soil-coring equipment. J. Appl. Ecol. 5, 477. doi: 10.2307/2401574
Wendel, A. S., Bauke, S. L., Amelung, W., Knief, C. (2022). Root-rhizosphere-soil interactions in biopores. Plant Soil 475, 253–277. doi: 10.1007/s11104-022-05406-4
Xue, Y.-F., Zhang, W., Liu, D.-Y., Yue, S.-C., Cui, Z.-L., Chen, X.-P., et al. (2014). Effects of nitrogen management on root morphology and zinc translocation from root to shoot of winter wheat in the field. Field Crops Res. 161, 38–45. doi: 10.1016/j.fcr.2014.01.009
Yapa, L. G. G., Fritton, D. D., Willatt, S. T. (1988). Effect of soil strength on root growth under different water conditions. Plant Soil 109, 9–16. doi: 10.1007/BF02197574
Zhang, Y., Yu, P., Peng, Y.-F., Li, X.-X., Chen, F.-J., Li, C.-J. (2012). Fine root patterning and balanced inorganic phosphorus distribution in the soil indicate distinctive adaptation of maize plants to phosphorus deficiency. Pedosphere 22, 870–877. doi: 10.1016/S1002-0160(12)60073-3
Zhao, X., Yu, H., Wen, J., Wang, X., Du, Q., Wang, J., et al. (2016). Response of root morphology, physiology and endogenous hormones in maize (Zea mays l.) to potassium deficiency. J. Integr. Agric. 15, 785–794. doi: 10.1016/S2095-3119(15)61246-1
Keywords: nutrient limitation, root plasticity, nitrogen, phosphorous, potassium, root morphology, fertilizer
Citation: Lopez G, Ahmadi SH, Amelung W, Athmann M, Ewert F, Gaiser T, Gocke MI, Kautz T, Postma J, Rachmilevitch S, Schaaf G, Schnepf A, Stoschus A, Watt M, Yu P and Seidel SJ (2023) Nutrient deficiency effects on root architecture and root-to-shoot ratio in arable crops. Front. Plant Sci. 13:1067498. doi: 10.3389/fpls.2022.1067498
Received: 11 October 2022; Accepted: 12 December 2022;
Published: 04 January 2023.
Edited by:
Saoirse Tracy, University College Dublin, IrelandReviewed by:
Felipe H. Barrios Masias, University of Nevada, Reno, United StatesKailou Liu, Jiangxi Institute of Red Soil, China
Copyright © 2023 Lopez, Ahmadi, Amelung, Athmann, Ewert, Gaiser, Gocke, Kautz, Postma, Rachmilevitch, Schaaf, Schnepf, Stoschus, Watt, Yu and Seidel. This is an open-access article distributed under the terms of the Creative Commons Attribution License (CC BY). The use, distribution or reproduction in other forums is permitted, provided the original author(s) and the copyright owner(s) are credited and that the original publication in this journal is cited, in accordance with accepted academic practice. No use, distribution or reproduction is permitted which does not comply with these terms.
*Correspondence: Gina Lopez, Z2luYS5sb3BlekB1bmktYm9ubi5kZQ==; Sabine Julia Seidel, c2FiaW5lLnNlaWRlbEB1bmktYm9ubi5kZQ==