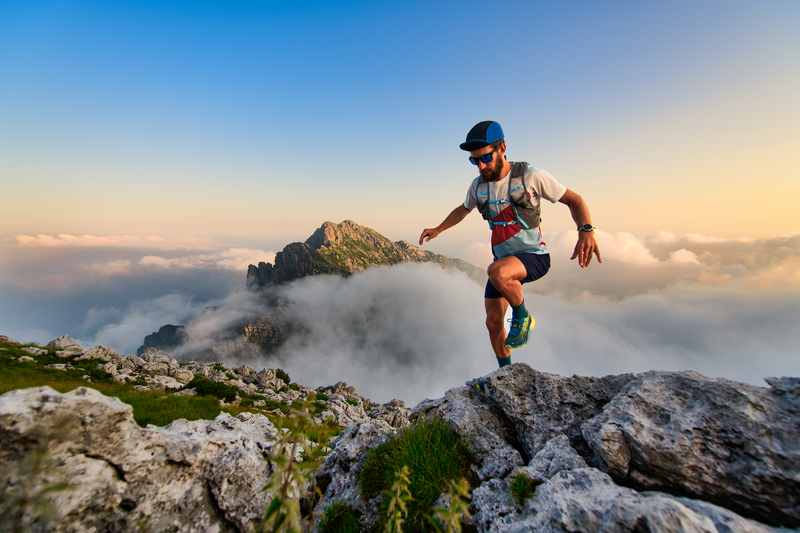
94% of researchers rate our articles as excellent or good
Learn more about the work of our research integrity team to safeguard the quality of each article we publish.
Find out more
ORIGINAL RESEARCH article
Front. Plant Sci. , 28 November 2022
Sec. Plant Pathogen Interactions
Volume 13 - 2022 | https://doi.org/10.3389/fpls.2022.1064628
This article is part of the Research Topic Systemic Resistance and Defense Priming Against Pathogens View all 9 articles
Chitin is a well-known elicitor of disease resistance and its recognition by plants is crucial to perceive fungal infections. Chitin can induce both a local immune response and a systemic disease resistance when provided as a supplement in soils. Unlike local immune responses, it is poorly explored how chitin-induced systemic disease resistance is developed. In this study, we report the systemic induction of disease resistance against the fungal pathogen Bipolaris oryzae by chitin supplementation of soils in rice. The transcriptome analysis uncovered genes related to cell-wall biogenesis, cytokinin signaling, regulation of phosphorylation, and defence priming in the development of chitin-induced systemic response. Alterations of cell-wall composition were observed in leaves of rice plants grown in chitin-supplemented soils, and the disease resistance against B. oryzae was increased in rice leaves treated with a cellulose biosynthesis inhibitor. The disruption of genes for lysin motif (LysM)-containing chitin receptors, OsCERK1 (Chitin elicitor receptor kinase 1) and OsCEBiP (Chitin elicitor-binding protein), compromised chitin-induced systemic disease resistance against B. oryzae and differential expression of chitin-induced genes found in wild-type rice plants. These findings suggest that chitin-induced systemic disease resistance in rice is caused by a perturbation of cell-wall biogenesis in leaves through long-distance signalling after local recognition of chitins by OsCERK1 and OsCEBiP.
Plants have developed two types of defence mechanisms, local immune response and systemic resistance, which are used to counteract threats from pathogens (Sun and Zhang, 2021). A local immune response is first induced upon pathogen approach and infection. Plants recognize microbe- or pathogen-associated molecular patterns (MAMPs/PAMPs) via a suite of pattern recognition receptors (PRRs) that induce pattern-triggered immunity (PTI), which causes the production of reactive oxygen species (ROS) and activates the expression of pathogenesis-related (PR) genes to defend against pathogen invasion (Bittel and Robatzek, 2007; Zipfel, 2008). However, pathogens counteract this initial defence barrier by secreting effector proteins into plant cells that disrupt PTI and allow infection to progress. In response, plants have evolved nucleotide-binding/leucine-rich repeat receptors (NLRs) to recognize pathogen effectors, which induce a robust defence response often accompanied by a localised hypersensitive response (HR) leading to cell death. This form of immunity is called effector-triggered immunity (ETI) (Jones and Dangl, 2006; Cui et al., 2015).
Local pathogen infection triggers systemic acquired resistance (SAR) that occurs in distant non-infected cells and is associated with salicylic acid (SA)–dependent gene expression and the biosynthesis of secondary metabolites (Hartmann and Zeier, 2019). For instance, the synthetic SA-analogue benzothiadiazole (BTH), a chemical activator of SAR, can induce systemic resistance in tobacco (Nicotiana tabacum), wheat (Triticum aestivum), Arabidopsis (Arabidopsis thaliana), and rice (Oryza sativa) (Friedrich et al., 1996; Görlach et al., 1996; Lawton et al., 1996; Shimono et al., 2007). Not all microbes are pathogens but some are beneficial ones, collectively called plant growth–promoting rhizobacteria (PGPR) and fungi (PGPF). Colonization of PGPR or PGPF in roots can trigger induced systemic resistance (ISR) via long-distance signalling (Pieterse et al., 2014). Unlike the SA-dependent SAR pathway, ISR results in systemic resistance via multiple signalling pathways involving the phytohormones SA, jasmonic acid (JA), and ethylene (ET) (2014; Pieterse et al., 1998). SAR and ISR engage different mechanisms but are both considered to elicit defence priming (Pieterse et al., 2014; Mauch-Mani et al., 2017).
Chitin, a polymer of β-1,4-linked N-acetylglucosamine, is a component of the fungal cell wall and arthropod exoskeletons (Pillai et al., 2009; Sharp, 2013). Plants have PRRs that recognize chitin as a MAMP/PAMP and initiate PTI (Gong et al., 2020). In rice, OsCERK1 (chitin elicitor receptor kinase 1) and OsCEBiP (chitin elicitor-binding protein) are members of the protein families lysin motif (LysM)-containing receptor-like kinase (RLK) and receptor-like protein (RLP) without a kinase domain, respectively; they form a heterodimeric chitin receptor complex (Kaku et al., 2006; Shimizu et al., 2010; Hayafune et al., 2014). OsCEBiP is the major chitin-binding protein in rice cultured cells (Kouzai et al., 2014b), with two OsCEBiP molecules binding to one chitin oligomer (CO) longer than hexamer (Hayafune et al., 2014). By contrast, OsCERK1 does not directly bind to CO (Shinya et al., 2012) but mediates chitin-induced PTI by binding to and phosphorylating downstream factors (Kawasaki et al., 2017). OsCERK1 forms a heterodimer with the LysM-RLK OsMYR1 (Myc factor receptor 1), which perceives short-chain COs secreted by arbuscular mycorrhizal (AM) fungi and competitively inhibits OsCEBiP-dependent immune signalling (He et al., 2019; Zhang et al., 2021). In Arabidopsis, the LysM-RLK AtCERK1 is required for CO perception (Miya et al., 2007; Wan et al., 2008) by forming a receptor complex with AtLYK4 (LysM-containing receptor-like kinase 4) and AtLYK5 in the chitin signalling pathways (Cao et al., 2014).
Since natural polymeric chitin is difficult to use due to its intractability and insolubility (Pillai et al., 2009), water-soluble chitin forms such as COs have mainly been used in studies of plant immunity. We developed a method to produce chitin nanofiber (CNF) from original chitin polymers by simple physical treatment of crustacean exoskeletons (Ifuku and Saimoto, 2012). CNF can homogeneously disperse even in water and can be used as a solution of polymeric chitin. We previously reported that CNF, as well as a mixture of COs, elicits ROS production in Arabidopsis and rice and that spraying leaves with either COs or CNF enhances disease resistance against both the fungal pathogen Alternaria brassicicola and the bacterial pathogen Pseudomonas syringae pv. tomato DC3000 in Arabidopsis (Egusa et al., 2015). Moreover, CNF supplementation of soils induced systemic disease resistance in Arabidopsis, cabbage (Brassica oleracea var. capitata), and strawberry (Fragaria sp.) (Parada et al., 2018). In addition, treatment of rice roots with a CO solution induced systemic disease resistance for a day (Tanabe et al., 2006). The induction of ISR by the ectomycorrhizal fungus Laccaria bicolor on the nonmycorrhizal plant Arabidopsis was dependent on JA signalling and SA biosynthesis and signalling, and AtCERK1 was necessary for the effect of systemic resistance (Vishwanathan et al., 2020). Thus, although ISR induced by chitin or via chitin recognition has been studied, our knowledge about the molecular basis underlying the induction of systemic disease resistance by chitin, in particular signalling pathway, is lacking compared to our understanding of local immune responses to chitins.
In this study, the systemic disease resistance against Bipolaris oryzae, the causal agent of rice brown spot disease, was examined by performing a transcriptome analysis of rice plants treated with chitins. We exposed plants to both oligomeric chitin COs and polymeric chitin CNF to test the possibility of differential effects on chitin-induced disease resistance. Both chitins induced systemic disease resistance in leaves. Transcriptome analysis demonstrated that cell-wall biogenesis- and cytokinin-related genes are downregulated as a systemic response induced by chitins. We validated these results with a cellulose biosynthesis inhibitor, by monitoring cell-wall composition and quantifying phytohormone levels. Knockout mutants for OsCERK1 and OsCEBiP revealed that both LysM receptors are required for chitin-induced systemic disease resistance in response to B. oryzae in leaves.
Unless otherwise stated, the Nipponbare cultivar of Oryza sativa L. (japonica group) was used as wild-type rice. OsCEBiP or OsCERK1 transformant lines were generated in the Oryza sativa L. japonica ‘Nipponbare Kanto BL number 2’ background by Agrobacterium-mediated gene targeting based on homologous recombination, which was previously described (Kouzai et al., 2014a; Kouzai et al., 2014b). The knockout mutant and segregating wild-type siblings of oscebip line 169 and oscerk1 lines 19 and 53 were used in this study. The COs (the mixture of DP [degree of polymerization] 2-6 chitin oligomers; NA-COS-Y; Yaizu Suisankagaku Industry, Japan) solution and CNF dispersion in water were prepared as previously reported (Kaminaka et al., 2020). Rice seeds were soaked in distilled water (DW) for germination at 28°C for 3 or 4 days in the dark, and the germinated seeds were transplanted into sterilized culture soil (Bestmix No. 3; Nippon Rockwool, Japan) mixed with equal volume of 0.1 or 0.01% (w/v) CO solution or CNF dispersion in magenta boxes (GA-7; Sigma-Aldrich, USA). Plants were grown in a growth cabinet (BiOTRON; NK-systems, Japan) under controlled conditions (28°C 14-h-light/25°C 10-h-dark cycles) and fertilised once a week with a 1:1000 HYPONeX (6-10-5; HYPONeX, Japan) solution. The cell-wall biosynthesis inhibitor isoxaben (Santa Cruz Biotechnology, Germany) was resolved in dimethyl sulfoxide (DMSO), and a dilution in DW was sprayed onto leaves 5 h before sampling.
Bipolaris oryzae D6 (Kihara and Kumagai, 1994) was cultured on potato dextrose agar plates for 1 week at 25°C in the dark. The conidial suspension was prepared to a titre of 1×105 spores/mL in 0.25% (v/v) Tween 20. Fourth leaves from 3-week-old rice seedlings grown on normal or chitin-supplemented soils were detached and inoculated with a drop (5 µL) of spores on the leaf sheaths and incubated in the dark for 1 day and then in the light for 1 day at 25°C. Images of inoculated leaves were taken using a GT-S640 Scanner (EPSON, Japan), and each lesion diameter was measured by ImageJ (ver.1.53a). In this paper, scatter and box plots were generated using the R package ggplot2, and Tukey’s HSD analyses using the multcomp in R.
Approximately 500 mg of randomly selected leaves was excised from at least three individual 3-week-old rice seedlings grown on normal or chitin-supplemented soils. Samples were prepared with five biological replicates for each treatment. The leaves were placed in tubes and frozen in liquid nitrogen. The contents of each phytohormone were quantified using liquid chromatography–tandem mass spectrometry (LC-MS/MS) as previously described (Kanno et al., 2016).
Alcohol-insoluble residue (AIR) was prepared from excised third or fourth leaves of 3-week-old rice seedlings grown on normal or chitin-supplemented soils, according to Bacete et al. (2017). AIR fractions were subjected to FT-IR spectroscopy using an FT-IR spectrophotometer equipped with an attenuated total reflectance accessory (Spectrum 65; PerkinElmer Japan, Japan). The FT-IR spectra were collected in the wavenumber range from 600 to 4,000 cm−1 with 16 scans, and the average values of three AIR fractions obtained from independent plants were used.
Rice plants were grown as mentioned above except for the growth conditions (28°C 14-h-light/16°C 10-h-dark cycles). About 100 mg of randomly selected leaves or roots was excised from at least three individual 3-week-old rice seedlings grown on normal or chitin-supplemented soils. Samples were prepared from three biological replicates for each treatment. The leaves or roots were placed inside tubes with 5-mm stainless beads, frozen in liquid nitrogen, and pulverised for 30 s using ShakeMan 6 (Bio Medical Science, Japan). LBB solution [1 M LiCl, 100 mM Tris-HCl (pH 7.5), 1% SDS, 10 mM EDTA, 0.015% Antifoam A, 5 mM DTT, and 71.5 mM 2-ME, DNase/RNase-free water] was added to the samples and completely dissolved by vortexing. All samples were incubated for at least 5 min at room temperature with occasional inverting and mixing. After centrifugation at 20,630 g for 10 min at room temperature, the supernatant was transferred to new tubes and stored at –80°C. Sequencing libraries were produced according to the BrAD-seq protocol (Ichihashi et al., 2018). Sequencing was performed on a HiseqX instrument (Illumina) by Macrogen Japan. Raw reads were checked for quality, and adaptor sequences were trimmed using fastp (Chen et al., 2018). The resulting clean reads were mapped to the reference rice genome (MSU Rice Genome Annotation Project ver. 7.0; http://rice.plantbiology.msu.edu/) using STAR (Dobin et al., 2013), and reads were counted by featureCounts with the package Subread (Liao et al., 2014). Results of data analysis are summarised in Supplementary Table 1. The expression profiles were obtained by comparing control and chitin-treated plants using EdgeR in the R package (Robinson et al., 2010) with a trimmed mean of M values for normalisation. The list of differentially expressed genes (DEGs) was based on a false discovery rate (FDR) < 0.05. Venn diagrams and heatmaps were prepared at the Bioinformatics and Evolutionary Genomics webpage (http://bioinformatics.psb.ugent.be/webtools/Venn/) and ComplexHeatmap in R package (Gu et al., 2016), respectively. Gene Ontology (GO) enrichment analysis was conducted with the PANTHER (Mi et al., 2021) and REVIGO (Supek et al., 2011) tools, according to Bonnot et al. (2019). Co-expression analysis was performed using the ShinyGO (ver.0.61) website (http://bioinformatics.sdstate.edu/go/; Ge et al., 2020).
Fourth leaves from 3-week-old rice seedlings grown on soil without chitin supplementation were excised into nine leaf discs 0.5 mm in size and floated overnight at 22°C in a well filled with sterilized DW (sDW). COs or CNF elicitation solutions were prepared by suspending COs or CNF in sDW to a final concentration of 0.01% (w/v). Peroxidase from a horseradish root (HRP: Oriental Yeast, Japan) stock solution (500×HRP) and luminol L-012 (L-012; Wako, Tokyo, Japan) stock solution (20 mM) were prepared as previously described (Parada et al., 2018). Before elicitation, the sDW was carefully removed from each well without tissue damage or desiccation. The elicitation solution was immediately added to each well after removing sDW, and chemiluminescence was measured with a microplate reader (ARVO X3; PerkinElmer Japan, Japan) for 40 min.
We examined the level of systemic disease resistance in chitin-treated rice plants using the rice brown spot fungus B. oryzae. Supplementation of soils with COs and CNF solution/dispersion induced disease resistance compared to untreated control plants, as determined by the size of lesions on leaves; both chitin forms had comparable effects (Figure 1). Mounting an immune response is often accompanied by growth inhibition, a trade-off between immunity and growth (Huot et al., 2014). Supplementation of soils with 0.1% (w/v) CNF hinders the development of cabbage and strawberry plants (Parada et al., 2018). However, 0.1% CNF added to soils did not affect leaf or stem growth in rice seedlings (Supplementary Figure 1). These results revealed that both COs and CNF can systemically induce disease resistance without compromising growth in rice.
Figure 1 Chitins induce a systemic disease resistance in rice. Induced systemic disease resistance against Bipolaris oryzae by chitins, as measured by lesion diameter (in centimetres) of leaves two days after inoculation. Three-week-old rice seedlings grown on soil mixed with distilled water (DW; Control) and 0.1% or 0.01% (w/v) chitin oligomers (COs) and chitin nanofiber (CNF) were inoculated with B. oryzae. Different letters indicate significant differences by Tukey’s test (p < 0.05, n > 14). Representative results from three independent experiments are shown.
To explore the molecular mechanisms underlying the induction of systemic disease resistance by chitins, we performed an RNA-seq analysis of rice leaves and roots grown in soils mixed with COs or CNF. We identified 81 and 230 DEGs in COs- and CNF-treated rice leaves, respectively (FDR < 0.05; numbered in both MSU-DB and RAP-DB) compared to control leaves (Supplementary Figure 2; Supplementary Tables 2, 3). Of these 297 non-redundant DEGs, only 14 genes were shared between COs and CNF treatments (Supplementary Figure 2). The 297 DEGs consisted of 157 upregulated (LogFC > 0) and 140 downregulated (LogFC < 0) genes by chitin treatments and showed similar trends in their expression patterns in COs- and CNF-treated leaves (Figure 2A). A GO enrichment analysis of DEGs indicated an enrichment for categories “regulation of protein serine/threonine phosphatase activity (GO:0080163)”, “glutathione metabolic process (GO:0006749)”, and “cellular modified amino acid metabolic process (GO:0006575)” among upregulated genes (Figure 2B), while downregulated genes were associated with “sulfate assimilation (GO:0000103)”, “response to cytokinin (GO:0009735)”, “cytokinesis (GO: 0000910)”, and “cell wall biogenesis (GO:0042546)” (Figure 2C). A co-expression analysis conducted using ShinyGO (Ge et al., 2020) determined that the expression of 41 genes upregulated by chitins was strongly and significantly (2.18 × 10–48) correlated with BTH-induced genes (Supplementary Figure 3; Table 1; Shimono et al., 2007).
Figure 2 Transcriptome analysis of rice leaves grown on chitin-supplemented soils. (A) Heatmap representation of gene expression levels of differentially expressed genes (DEGs) in response to COs and CNF (left). LogFC is shown between −2 and 2, with outside values indicated as 2 or −2. Red, upregulated genes; blue, downregulated genes. DEGs in each treatment are indicated on the right. Red, DEG; ivory, not differentially expressed. (B, C) Results of Gene Ontology (GO) enrichment analysis summarised as plot data for upregulated DEGs in chitin-treated samples (B) and downregulated DEGs (C).
Table 1 Expression levels and annotation of genes upregulated by chitin treatment correlated with BTH-induced genes.
We conducted a similar analysis on root samples (Supplementary Figure 4; Supplementary Tables 4, 5). Roots exhibited a much smaller number of DEGs compared to that of leaves upon chitin treatment (Supplementary Figures 2, 4). GO enrichment analysis revealed that upregulated genes in response to COs and CNFs are involved in “cellular response to nitrate (GO:0071249)”, “nitrogen cycle metabolic process (GO:0071941)”, and “nitrate assimilation (GO:0042128)” (Supplementary Figure 4C). These results demonstrated that both COs and CNF induce the expression of genes involved in cytokinin signalling, cell-wall biogenesis, and disease resistance induced by BTH in leaves, while the chitin supplementation in soils affected the different genes in roots.
Phytohormones plays important roles in ISR (Pieterse et al., 2014; Hartmann and Zeier, 2019). We thus measured endogenous levels of phytohormones (auxin [IAA], gibberellins [GA1], abscisic acid [ABA], JA, jasmonyl isoleucine [JA-Ile], trans-zeatin [tZ], isopentyladenine [iP], and SA) in the leaves of rice seedlings grown on soils supplemented with chitins (Table 2). Of all phytohormones tested, only the contents for the active cytokinin tZ significantly (P = 9.71 × 10–4) decreased in CNF-treated samples compared to control seedlings. This finding was congruent with our RNA-seq analysis showing that the GO term “response to cytokinin” was enriched in chitin-suppressed genes in leaves (Supplementary Figure 5).
The plant cell wall offers a passive physical defence barrier to prevent pathogen access to plant cells; in agreement, alteration of cell-wall composition is associated with disease resistance (Bacete et al., 2018). Modification of cell-wall composition caused by genetic inactivation or overexpression of cell-wall-related genes in Arabidopsis resulted in enhanced disease resistance or susceptibility against various pathogens (Bacete et al., 2018; Molina et al., 2021). Since the GO enrichment analysis suggested an alteration of cell-wall composition in leaves by chitin supplementation of soils (Figure 2C), we purified the cell-wall fraction from leaves of control (DW) and chitin-treated rice seedlings and measured its absorbance using FT-IR spectroscopy. We selected the wavenumber range of 800–1700 cm−1 as in Molina et al. (2021), which can be assigned to main cell-wall components (Alonso-Simón et al., 2011). As shown in Figure 3A, we observed different FT-IR spectra in seedlings grown on chitin-supplemented soils compared to control, indicating that chitin supplementation of soils results in an alteration of cell-wall composition in rice leaves.
Figure 3 Perturbation of cell-wall biogenesis upon chitin treatments and induced systemic disease resistance in rice leaves. (A) Supplementation of soils with chitins systematically induced alterations of cell-wall composition in rice leaves. Each line represents the differential Fourier-transform infrared (FT-IR) spectra between control plants and seedlings grown on COs- or CNF-containing soils (n = 3). (B) A cellulose biosynthesis inhibitor induces disease resistance in rice leaves. The leaves of 3-week-old rice seedlings grown on soil were sprayed with control (DW), DMSO, or isoxaben (1, 0.1, and 0.01 µM, respectively) five hours before B oryzae inoculation. Lesion diameter (cm) of leaves two days after inoculation are shown. Representative results from three independent experiments are shown. Different letters indicate significant differences by Tukey’s test (p < 0.05, n >14).
Defects in cell-wall biosynthesis are associated with disease resistance against Plectosphaerella cucumerina, Botrytis cinerea, and Ralstonia solanacearum in Arabidopsis (Hernández-Blanco et al., 2007). However, an effect of cell-wall biosynthesis inhibition on disease resistance has not been reported in rice. We examined disease resistance against B. oryzae in rice leaves treated with cellulose biosynthesis inhibitor isoxaben (Heim et al., 1990; Tateno et al., 2016). Isoxaben treatment significantly enhanced disease resistance against B. oryzae, compared to control and DMSO-treated seedlings (p < 0.005), indicating that, as in Arabidopsis, alteration of cell-wall composition increases resistance against pathogens in rice (Figure 3B).
To assess whether OsCERK1 or OsCEBiP contributes to the systemic disease resistance in chitin-treated rice seedlings, we tested induction of disease resistance against B.oryzae in their respective knockout mutants and corresponding wild-type segregants (Kouzai et al., 2014a; Kouzai et al., 2014b), which we used as wild-type plants in the following experiments. Both wild-type siblings and wild-type plants exhibited a systemic induction of disease resistance against B. oryzae upon chitin treatments, whereas neither knockout mutant did (Figure 4).
Figure 4 Chitins induce systemic disease resistance in LysM-receptor mutants. Lesion diameters upon B oryzae inoculation in wild-type plants (WT), wild-type siblings (ws), and knockout (ko) mutants of OsCERK1 or OsCEBiP, conducted as in Figure 1. Seedlings were grown on 0.01% (w/v) COs- or CNF-containing soils. Representative results from three independent experiments are shown. Asterisks indicate significant differences by Student’s test (***p < 0.001, n.s., not significant, n > 9).
We also tested chitin-induced local immune response in all genotypes. COs induce ROS production, a typical response of PTI, in Arabidopsis and rice (Kaku et al., 2006; Miya et al., 2007). Furthermore, polymeric chitin in the form of CNF induces ROS production in Arabidopsis seedlings, rice cultured cells, and cabbage and strawberry leaf discs (Egusa et al., 2015; Parada et al., 2018). ROS production was induced by both COs and CNF in rice leaves, and compromised in the oscerk1 mutant compared to its wild-type siblings (Supplementary Figure 6A). However, the level of ROS production was comparable between the oscebip mutant and its wild-type siblings (Supplementary Figure 6B). Taken together, these findings indicate that both OsCERK1 and OsCEBiP are required for chitin-induced systemic disease resistance in rice, but OsCEBiP did not appear to be essential for a local immune response in leaves.
To investigate the effects of the oscerk1 or oscebip mutants on chitin-induced gene expression, we performed an RNA-seq analysis on the leaves of oscerk1 and oscebip mutants grown on soils mixed with chitins. Using the 297 DEGs in the wild type in response to chitin treatment as reference (Figure 2A), we established that the expression patterns in the oscebip mutant background were drastically different from those observed in the wild type and the oscerk1 mutant (Figure 5A; Supplementary Tables 6-9). Next, we determined DEGs specific to the knockout mutants by comparing expression levels between control and chitin-treated seedlings and identified 1744 DEGs in oscebip and 1495 DEGs in oscerk1, of which 535 genes were common to both receptor mutants with both chitin treatments (Figure 5B). In fact, only 32 of the 297 chitin-induced DEGs in the wild type were differentially expressed in both knockout mutants, and 162 genes were specifically induced by chitin treatment in the wild type (Figure 5B). A GO enrichment analysis of these 162 genes identified the terms “mitotic cytokinesis (GO:0000281)” and “plant-type cell wall organization or biogenesis (GO:0071669)” as enriched (Figure 5C), which corresponded to the GO terms obtained in the DEGs downregulated by chitin supplementation (Figure 2C).
Figure 5 Transcriptome analysis of leaves from LysM-receptor mutants grown on chitin-supplemented soils. (A) Heatmap representation of expression levels of genes identified in the wild type (WT) as being differentially expressed in leaves upon chitin treatment and listed in Supplementary Table 2 and 3 in the leaves of the WT and oscebip or oscerk1 mutants. LogFC is shown between −2 and 2, with outside values indicated as 2 or −2. Red, upregulated; blue, downregulated. (B) Venn diagram showing the overlap between chitin-induced DEGs in the WT and genes that were DEGs in the knockout mutants (blue: in the WT, red: in oscebip mutants, green: in oscerk1 mutants). (C) Results of GO enrichment analysis of the genes not differentially expressed in oscebip and oscerk1 mutants defined above [162 genes; in the WT-specific group of (B)].
This study aimed to elucidate the molecular mechanism underlying the systemic resistance induced by chitins in rice. To this end, we used two types of chitins, COs (DP2-6) and polymeric chitin CNF, and determined their effects on systemic disease resistance and the transcriptome using knockout mutants of the well-characterized LysM-containing chitin receptors, OsCERK1 and OsCEBiP. Supplementation of soils with COs or CNF significantly induced systemic disease resistance against B. oryzae in rice leaves of wild-type plants and wild-type siblings of the knockout mutants (Figures 1, 4), while both oscerk1 and oscebip mutants compromised chitin-induced systemic disease resistance (Figure 4). Unlike that, OsCERK1, but not OsCEBiP, is required to elicit ROS production induced by both COs and CNF in rice leaves (Supplementary Figure 6). These results indicated that OsCERK1 and OsCEBiP regulate chitin-induced systemic disease resistance, although a chitin-induced local immune response in leaves likely requires another chitin-binding protein(s). When chitins are supplemented in soils, chitin perception would be expected to take place in roots and then initiate a long-distance signalling from roots to shoots to induce systemic disease resistance in leaves. Since both OsCERK1 and OsCEBiP are required for chitin-induced systemic disease resistance, these LysM receptors should function as chitin receptors in roots, but OsCEBiP is not required for ROS production in leaves (Supplementary Figure 6). However, the oscebip mutant also compromises elicitor activity in rice suspension cultured cells (Kouzai et al., 2014b). This discrepancy may be explained by the different materials used for analysis and ROS measurements: photosynthetic (leaves) versus non-photosynthetic (cell suspensions).
CNF supplementation of soils resulted in more DEGs than COs supplementation in rice leaves, (Figure 2), as was previously observed with the transcriptomes of soybean (Glycine max) roots grown in soils mixed with COs or CNF (Kaminaka et al., 2020). In addition, the RNA-seq analysis demonstrated that the expression patterns of DEGs in rice leaves are similar between soils supplemented with COs and CNF, with some differences as well (Figure 2). CNF can be degraded into oligomeric chitins by chitinase more rapidly than non-nanofibrillated chitin (Egusa et al., 2015). Thus, CNF may be perceived as these degraded forms of oligomeric chitins rather than as the form of CNF. However, previous reports indicated that AtCERK1 also binds to polymeric chitin, which plays an essential role in chitin signalling (Petutschnig et al., 2010; Wan et al., 2012). Therefore, oligomeric and polymeric chitins may have specific roles in local immune responses and systemic disease resistance.
RNA-seq analysis of the leaves of rice seedlings grown on soils supplemented with chitins suggested the involvement of cell-wall biogenesis, cytokinin signalling, and regulation of phosphorylation in the systemic response induced by chitins (Figure 2). Cell-wall biogenesis may play a key role in chitin-induced systemic response, as this function would require the chitin receptors OsCERK1 and OsCEBiP (Figure 5B, C). This finding was also supported by the evidence that chitin supplementation of soils disturbs cell-wall composition, as evidenced by FT-IR spectrometry (Figure 3A). The leaves of chitin-treated rice seedlings showed spectra quite similar to those of the cellulose-deficient mutant procuste 1-8 (prc1-8) and the pectin-deficient mutant quasimodo 1-1 (qua1-1) of Arabidopsis (Mouille et al., 2003). We observed the same lower absorbance from 1170 to 1050 cm–1 in chitin-treated rice seedlings that was attributed to cellulose and xyloglucans observed in the Arabidopsis powdery mildew resistant 5 (pmr5) and pmr6 mutants, which exhibit enhanced resistance to powdery mildew (Vogel et al., 2004). In addition, the cellulose biosynthesis inhibitor isoxaben significantly induced disease resistance in leaves (Figure 3B). Isoxaben targets cellulose synthase (CESA) subunits in Arabidopsis (Scheible et al., 2001; Desprez et al., 2002), which is in line with the strong reduction in the expression of genes encoding CESA or cellulose synthase-like (CSLA) among CNF-induced DEGs compared to control seedlings (Supplementary Figure 5). Cell-wall-derived oligosaccharides released from hemicellulose activate the immune response via OsCERK1 during infection by the fungal pathogen Magnaporthe oryzae in rice (Yang et al., 2021). Thus, damage-associated molecular pattern (DAMP)-triggered immunity caused by cell-wall-derived molecules, particularly cellulose, might be involved in chitin-induced systemic resistance in rice.
We measured a significant reduction in cytokinin levels in leaves of rice seedlings grown on CNF-supplemented soils (Table 2). Isoxaben treatment reduces the contents of the active cytokinin tZ as well as iP types in Arabidopsis (Gigli-Bisceglia et al., 2018). The expression levels of several genes encoding type-A response regulators, which regulate cytokinin signalling, were lower in rice leaves upon supplementation of soils with both chitins (Supplementary Figure 5). Loss of function of type-A ARR6 (Arabidopsis Response Regulator 6) induces disease resistance to P. cucumerina BMM and Hyaloperonospora parasitica Noco2 and is accompanied by an alteration in cell-wall composition (Bacete et al., 2020). These findings suggest that downregulation of genes involved in cytokinin signalling, which is associated with alterations of cell-wall components, participates in chitin-induced systemic disease resistance.
LysM-containing receptors perceive ligands for both immune responses and when establishing symbiosis. In rice, OsCERK1 is involved in recognizing both immune and symbiotic signals. For chitin-triggered immunity, OsCERK1 forms a receptor complex with OsCEBiP that binds to long-chain COs such as chitooctaose (CO8) (Shimizu et al., 2010; Hayafune et al., 2014). OsMYR1/OsLYK2, which directly binds to short-chain COs like chitotetraose (CO4) released by beneficial symbiont AM fungi, forms a heteromer with OsCERK1 to establish AM symbiosis (He et al., 2019). Disruption of OsCERK1 decreases the colonisation of AM fungi and the production of calcium spikes, whereas the oscebip mutant does not have any effect on symbiosis (Miyata et al., 2014; Carotenuto et al., 2017). OsMYR1 depletes OsCERK1 for OsCERK1-OsCEBiP formation and prevents immune signalling induced by CO8, while OsCEBiP inhibits OsCERK1-OsMYR1 binding in a CO8-dependent manner (Zhang et al., 2021). This competition between OsCERK1-OsCEBiP and OsCERK1-OsMYR1 might balance immunity and symbiosis (Zhang et al., 2021). Since the systemic induction of disease resistance by chitins appears similar to what takes place during ISR caused by beneficial fungi, chitin-induced systemic disease resistance may employ the recognition mechanism for chitins involved in local immune response via OsCERK1-OsCEBiP but not the OsCERK1-OsMYR1 receptor complex participating in AM symbiosis. In addition, the expression of DEGs upregulated by chitins in leaves displayed a strong positive correlation with the genes induced by BTH, an SA analogue that induces defence priming (Shimono et al., 2007) (Table 1). Our previous study reported that CNF supplementation of soils induces defence priming in cabbage and strawberry (Parada et al., 2018). Taken together with the evidence that fungal ISR caused by L. bicolor in Arabidopsis occurs via AtCERK1 (Vishwanathan et al., 2020), chitin-induced systemic disease resistance may mimic ISR induced by plant growth–promoting fungi.
In summary, chitins supplemented into soils systemically induce disease resistance against the fungal pathogen B. oryzae via recognition of chitins by the LysM receptors OsCERK1 and OsCEBiP in rice. Cell-wall biogenesis and cytokinin signalling are perturbed as a systemic response in leaves, and defence priming-related genes and phosphorylation-related genes are upregulated. These effects, together with another unknown process, eventually induce disease resistance (Figure 6). This study uncovers the molecular basis underlying chitin-induced systemic disease resistance. These findings may also contribute to elucidating the molecular basis of ISR, which is not well understood, and provides support for the application of chitins as a promising material in agriculture to confer disease resistance. However, it remains unknown how plants systemically induce disease resistance in response to chitins. In addition, both oligomeric and polymeric chitins caused similar effects on chitin-induced systemic disease resistance but differently affected local immune responses and global gene expression in leaves. Thus, it will be essential to expand our knowledge regarding chitin-induced disease resistance in rice, for example, by identifying the molecules involved in long-distance signalling and those derived from cell walls and by confirming the direct perception of CNF by LysM receptors.
Figure 6 Hypothetical model of chitin-induced systemic disease resistance in rice. Chitins supplemented in the soil induce disease resistance in leaves against the fungal pathogen B oryzae. Chitins are first recognized by the LysM receptors OsCERK1 and OsCEBiP in roots. Then, long-distance signalling initiated in the roots perturbs cell-wall biogenesis and upregulates defence priming-related genes in leaves, inducing disease resistance.
The datasets presented in this study can be found in online repositories. The names of the repository/repositories and accession number(s) can be found on: https://www.ddbj.nig.ac.jp/, DRA012267. The raw read data for RNA-seq were deposited in the DNA Data Bank of Japan under the accession number DRA012267.
YN, SI, AM, and HK conceived and designed the experiments. MT, KH, KN, SM, ME, YK, MS, and AM performed the experiments. MT, KH, and AM analysed the sequencing data. MT, ME, YN, SI, AM and HK wrote the manuscript. All authors contributed to the article and approved the submitted version.
This work was supported by JSPS KAKENHI Grant-in-Aid for Scientific Research (B) (Grant no. 19KT0010) and Takahashi Industrial and Economic Research Foundation.
We would like to thank Dr. Makoto Ueno (Shimane University) and Dr. Atsushi Ishihara (Tottori University) for providing the B. oryzae strain. We also thank Ms. Mei Yokomizo for technical assistance.
The authors declare that the research was conducted in the absence of any commercial or financial relationships that could be construed as a potential conflict of interest.
All claims expressed in this article are solely those of the authors and do not necessarily represent those of their affiliated organizations, or those of the publisher, the editors and the reviewers. Any product that may be evaluated in this article, or claim that may be made by its manufacturer, is not guaranteed or endorsed by the publisher.
The Supplementary Material for this article can be found online at: https://www.frontiersin.org/articles/10.3389/fpls.2022.1064628/full#supplementary-material
Alonso-Simón, A., García-Angulo, P., Mélida, H., Encina, A., Álvarez, J. M., Acebes, J. L. (2011). The use of FTIR spectroscopy to monitor modifications in plant cell wall architecture caused by cellulose biosynthesis inhibitors. Plant Signal. Behav. 6, 1104–1110. doi: 10.4161/psb.6.8.15793
Bacete, L., Mélida, H., López, G., Dabos, P., Tremousaygue, D., Denancé, N., et al. (2020). Arabidopsis response regulator 6 (ARR6) modulates plant cell-wall composition and disease resistance. Mol. Plant-Microbe Interact. 33, 767–780. doi: 10.1094/MPMI-12-19-0341-R
Bacete, L., Mélida, H., Miedes, E., Molina, A. (2018). Plant cell wall-mediated immunity: cell wall changes trigger disease resistance responses. Plant J. 93, 614–636. doi: 10.1111/tpj.13807
Bacete, L., Mélida, H., Pattathil, S., Hahn, M. G., Molina, A., Miedes, E. (2017). “Characterization of plant cell wall damage-associated molecular patterns regulating immune responses,” in Plant pattern recognition receptors, methods in molecular biology, vol. 1578 . Eds. Shan, L., He, P. (New York, NY: Humana Press), 13–23. doi: 10.1007/978-1-4939-6859-6_2
Bittel, P., Robatzek, S. (2007). Microbe-associated molecular patterns (MAMPs) probe plant immunity. Curr. Opin. Plant Biol. 10, 335–341. doi: 10.1016/j.pbi.2007.04.021
Bonnot, T., Gillard, M., Nagel, D. (2019). A simple protocol for informative visualization of enriched gene ontology terms. Bio-101 9, e3429. doi: 10.21769/BioProtoc.3429
Cao, Y., Liang, Y., Tanaka, K., Nguyen, C. T., Jedrzejczak, R. P., Joachimiak, A., et al. (2014). The kinase LYK5 is a major chitin receptor in arabidopsis and forms a chitin-induced complex with related kinase CERK1. Elife 3, 1–19. doi: 10.7554/eLife.03766
Carotenuto, G., Chabaud, M., Miyata, K., Capozzi, M., Takeda, N., Kaku, H., et al. (2017). The rice LysM receptor-like kinase OsCERK1 is required for the perception of short-chain chitin oligomers in arbuscular mycorrhizal signaling. New Phytol. 214, 1440–1446. doi: 10.1111/nph.14539
Chen, S., Zhou, Y., Chen, Y., Gu, J. (2018). Fastp: an ultra-fast all-in-one FASTQ preprocessor. Bioinformatics 34, i884–i890. doi: 10.1093/bioinformatics/bty560
Cui, H., Tsuda, K., Parker, J. E. (2015). Effector-triggered immunity: from pathogen perception to robust defense. Annu. Rev. Plant Biol. 66, 487–511. doi: 10.1146/annurev-arplant-050213-040012
Desprez, T., Vernhettes, S., Fagard, M., Refrégier, G., Desnos, T., Aletti, E., et al. (2002). Resistance against herbicide isoxaben and cellulose deficiency caused by distinct mutations in same cellulose synthase isoform CESA6. Plant Physiol. 128, 482–490. doi: 10.1104/pp.010822
Dobin, A., Davis, C. A., Schlesinger, F., Drenkow, J., Zaleski, C., Jha, S., et al. (2013). STAR: ultrafast universal RNA-seq aligner. Bioinformatics 29, 15–21. doi: 10.1093/bioinformatics/bts635
Egusa, M., Matsui, H., Urakami, T., Okuda, S., Ifuku, S., Nakagami, H., et al. (2015). Chitin nanofiber elucidates the elicitor activity of polymeric chitin in plants. Front. Plant Sci. 6. doi: 10.3389/fpls.2015.01098
Friedrich, L., Lawton, K., Ruess, W., Masner, P., Specker, N., Rella, M. G., et al. (1996). A benzothiadiazole derivative induces systemic acquired resistance in tobacco. Plant J. 10, 61–70. doi: 10.1046/j.1365-313X.1996.10010061.x
Ge, S. X., Jung, D., Yao, R., Jung, D., Yao, R. (2020). ShinyGO: a graphical gene-set enrichment tool for animals and plants. Bioinformatics 36, 2628–2629. doi: 10.1093/bioinformatics/btz931
Gigli-Bisceglia, N., Engelsdorf, T., Strnad, M., Vaahtera, L., Khan, G. A., Jamoune, A., et al. (2018). Cell wall integrity modulates arabidopsis thaliana cell cycle gene expression in a cytokinin- and nitrate reductase-dependent manner. Development 145, 1–14. doi: 10.1242/dev.166678
Gong, B.-Q., Wang, F.-Z., Li, J.-F. (2020). Hide-and-seek: chitin-triggered plant immunity and fungal counterstrategies. Trends Plant Sci. 25, 805–816. doi: 10.1016/j.tplants.2020.03.006
Görlach, J., Volrath, S., Knauf-Beiter, G., Hengy, G., Beckhove, U., Kogel, K. H., et al. (1996). Benzothiadiazole, a novel class of inducers of systemic acquired resistance, activates gene expression and disease resistance in wheat. Plant Cell 8, 629–643. doi: 10.1105/tpc.8.4.629
Gu, Z., Eils, R., Schlesner, M. (2016). Complex heatmaps reveal patterns and correlations in multidimensional genomic data. Bioinformatics 32, 2847–2849. doi: 10.1093/bioinformatics/btw313
Hartmann, M., Zeier, J. (2019). N-hydroxypipecolic acid and salicylic acid: a metabolic duo for systemic acquired resistance. Curr. Opin. Plant Biol. 50, 44–57. doi: 10.1016/j.pbi.2019.02.006
Hayafune, M., Berisio, R., Marchetti, R., Silipo, A., Kayama, M., Desaki, Y., et al. (2014). Chitin-induced activation of immune signaling by the rice receptor CEBiP relies on a unique sandwich-type dimerization. Proc. Natl. Acad. Sci. U.S.A. 111, E404–E413. doi: 10.1073/pnas.1312099111
Heim, D. R., Skomp, J. R., Tschabold, E. E., Larrinua, I. M. (1990). Isoxaben inhibits the synthesis of acid insoluble cell wall materials in arabidopsis thaliana. Plant Physiol. 93, 695–700. doi: 10.1104/pp.93.2.695
Hernández-Blanco, C., Feng, D. X., Hu, J., Sánchez-Vallet, A., Deslandes, L., Llorente, F., et al. (2007). Impairment of cellulose synthases required for arabidopsis secondary cell wall formation enhances disease resistance. Plant Cell 19, 890–903. doi: 10.1105/tpc.106.048058
He, J., Zhang, C., Dai, H., Liu, H., Zhang, X., Yang, J., et al. (2019). A LysM receptor heteromer mediates perception of arbuscular mycorrhizal symbiotic signal in rice. Mol. Plant 12, 1561–1576. doi: 10.1016/j.molp.2019.10.015
Huot, B., Yao, J., Montgomery, B. L., He, S. Y. (2014). Growth-defense tradeoffs in plants: a balancing act to optimize fitness. Mol. Plant 7, 1267–1287. doi: 10.1093/mp/ssu049
Ichihashi, Y., Fukushima, A., Shibata, A., Shirasu, K. (2018). “High impact gene discovery: simple strand-specific mRNA library construction and differential regulatory analysis based on gene co-expression network,” in Plant transcription factors. methods in molecular biology, vol. 1830 . Ed. Yamaguchi, N. (New York, NY: Humana Press), 163–189. doi: 10.1007/978-1-4939-8657-6_11
Ifuku, S., Saimoto, H. (2012). Chitin nanofibers: preparations, modifications, and applications. Nanoscale 4, 3308–3318. doi: 10.1039/C2NR30383C
Jones, J. D. G., Dangl, J. L. (2006). The plant immune system. Nature 444, 323–329. doi: 10.1038/nature05286
Kaku, H., Nishizawa, Y., Ishii-Minami, N., Akimoto-Tomiyama, C., Dohmae, N., Takio, K., et al. (2006). Plant cells recognize chitin fragments for defense signaling through a plasma membrane receptor. Proc. Natl. Acad. Sci. U.S.A. 103, 11086–11091. doi: 10.1073/pnas.0508882103
Kaminaka, H., Miura, C., Isowa, Y., Tominaga, T., Gonnami, M., Egusa, M., et al. (2020). Nanofibrillation is an effective method to produce chitin derivatives for induction of plant responses in soybean. Plants 9, 810. doi: 10.3390/plants9070810
Kanno, Y., Oikawa, T., Chiba, Y., Ishimaru, Y., Shimizu, T., Sano, N., et al. (2016). AtSWEET13 and AtSWEET14 regulate gibberellin-mediated physiological processes. Nat. Commun. 7, 13245. doi: 10.1038/ncomms13245
Kawasaki, T., Yamada, K., Yoshimura, S., Yamaguchi, K. (2017). Chitin receptor-mediated activation of MAP kinases and ROS production in rice and arabidopsis. Plant Signal. Behav. 12, e1361076. doi: 10.1080/15592324.2017.1361076
Kihara, J., Kumagai, T. (1994). Ecotypes of the fungus bipolaris oryzae with various responses of the mycochrome system. Physiol. Plant 92, 689–695. doi: 10.1111/j.1399-3054.1994.tb03041.x
Kouzai, Y., Mochizuki, S., Nakajima, K., Desaki, Y., Hayafune, M., Miyazaki, H., et al. (2014a). Targeted gene disruption of OsCERK1 reveals its indispensable role in chitin perception and involvement in the peptidoglycan response and immunity in rice. Mol. Plant-Microbe Interact. 27, 975–982. doi: 10.1094/MPMI-03-14-0068-R
Kouzai, Y., Nakajima, K., Hayafune, M., Ozawa, K., Kaku, H., Shibuya, N., et al. (2014b). CEBiP is the major chitin oligomer-binding protein in rice and plays a main role in the perception of chitin oligomers. Plant Mol. Biol. 84, 519–528. doi: 10.1007/s11103-013-0149-6
Lawton, K. A., Friedrich, L., Hunt, M., Weymann, K., Delaney, T., Kessmann, H., et al. (1996). Benzothiadiazole induces disease resistance in arabidopsis by activation of the systemic acquired resistance signal transduction pathway. Plant J. 10, 71–82. doi: 10.1046/j.1365-313X.1996.10010071.x
Liao, Y., Smyth, G. K., Shi, W. (2014). featureCounts: an efficient general purpose program for assigning sequence reads to genomic features. Bioinformatics 30, 923–930. doi: 10.1093/bioinformatics/btt656
Mauch-Mani, B., Baccelli, I., Luna, E., Flors, V. (2017). Defense priming: an adaptive part of induced resistance. Annu. Rev. Plant Biol. 68, 485–512. doi: 10.1146/annurev-arplant-042916-041132
Mi, H., Ebert, D., Muruganujan, A., Mills, C., Albou, L.-P., Mushayamaha, T., et al. (2021). PANTHER version 16: a revised family classification, tree-based classification tool, enhancer regions and extensive API. Nucleic Acids Res. 49, D394–D403. doi: 10.1093/nar/gkaa1106
Miya, A., Albert, P., Shinya, T., Desaki, Y., Ichimura, K., Shirasu, K., et al. (2007). CERK1, a LysM receptor kinase, is essential for chitin elicitor signaling in arabidopsis. Proc. Natl. Acad. Sci. U.S.A. 104, 19613–19618. doi: 10.1073/pnas.0705147104
Miyata, K., Kozaki, T., Kouzai, Y., Ozawa, K., Ishii, K., Asamizu, E., et al. (2014). The bifunctional plant receptor, OsCERK1, regulates both chitin-triggered immunity and arbuscular mycorrhizal symbiosis in rice. Plant Cell Physiol. 55, 1864–1872. doi: 10.1093/pcp/pcu129
Molina, A., Miedes, E., Bacete, L., Rodríguez, T., Mélida, H., Denancé, N., et al. (2021). Arabidopsis cell wall composition determines disease resistance specificity and fitness. Proc. Natl. Acad. Sci. U.S.A. 118, e2010243118. doi: 10.1073/pnas.2010243118
Mouille, G., Robin, S., Lecomte, M., Pagant, S., Höfte, H. (2003). Classification and identification of arabidopsis cell wall mutants using Fourier-transform InfraRed (FT-IR) microspectroscopy. Plant J. 35, 393–404. doi: 10.1046/j.1365-313X.2003.01807.x
Parada, R. Y., Egusa, M., Aklog, Y. F., Miura, C., Ifuku, S., Kaminaka, H. (2018). Optimization of nanofibrillation degree of chitin for induction of plant disease resistance: Elicitor activity and systemic resistance induced by chitin nanofiber in cabbage and strawberry. Int. J. Biol. Macromol. 118, 2185–2192. doi: 10.1016/j.ijbiomac.2018.07.089
Petutschnig, E. K., Jones, A. M. E., Serazetdinova, L., Lipka, U., Lipka, V. (2010). The lysin motif receptor-like kinase (LysM-RLK) CERK1 is a major chitin-binding protein in arabidopsis thaliana and subject to chitin-induced phosphorylation. J. Biol. Chem. 285, 28902–28911. doi: 10.1074/jbc.M110.116657
Pieterse, C. M. J., van Wees, S. C. M., van Pelt, J. A., Knoester, M., Laan, R., Gerrits, H., et al. (1998). A novel signaling pathway controlling induced systemic resistance in arabidopsis. Plant Cell 10, 1571–1580. doi: 10.1105/tpc.10.9.1571
Pieterse, C. M. J., Zamioudis, C., Berendsen, R. L., Weller, D. M., Van Wees, S. C. M., Bakker, P. A. H. M. (2014). Induced systemic resistance by beneficial microbes. Annu. Rev. Phytopathol. 52, 347–375. doi: 10.1146/annurev-phyto-082712-102340
Pillai, C. K. S., Paul, W., Sharma, C. P. (2009). Chitin and chitosan polymers: chemistry, solubility and fiber formation. Prog. Polym. Sci. 34, 641–678. doi: 10.1016/j.progpolymsci.2009.04.001
Robinson, M. D., McCarthy, D. J., Smyth, G. K. (2010). edgeR: a bioconductor package for differential expression analysis of digital gene expression data. Bioinformatics 26, 139–140. doi: 10.1093/bioinformatics/btp616
Scheible, W. R., Eshed, R., Richmond, T., Delmer, D., Somerville, C. (2001). Modifications of cellulose synthase confer resistance to isoxaben and thiazolidinone herbicides in arabidopsis Ixr1 mutants. Proc. Natl. Acad. Sci. U.S.A. 98, 10079–10084. doi: 10.1073/pnas.191361598
Sharp, R. G. (2013). A review of the applications of chitin and its derivatives in agriculture to modify plant-microbial interactions and improve crop yields. Agronomy 3, 757–793. doi: 10.3390/agronomy3040757
Shimizu, T., Nakano, T., Takamizawa, D., Desaki, Y., Ishii-Minami, N., Nishizawa, Y., et al. (2010). Two LysM receptor molecules, CEBiP and OsCERK1, cooperatively regulate chitin elicitor signaling in rice. Plant J. 64, 204–214. doi: 10.1111/j.1365-313X.2010.04324.x
Shimono, M., Sugano, S., Nakayama, A., Jiang, C.-J., Ono, K., Toki, S., et al. (2007). Rice WRKY45 plays a crucial role in benzothiadiazole-inducible blast resistance. Plant Cell 19, 2064–2076. doi: 10.1105/tpc.106.046250
Shinya, T., Motoyama, N., Ikeda, A., Wada, M., Kamiya, K., Hayafune, M., et al. (2012). Functional characterization of CEBiP and CERK1 homologs in arabidopsis and rice reveals the presence of different chitin receptor systems in plants. Plant Cell Physiol. 53, 1696–1706. doi: 10.1093/pcp/pcs113
Sun, T., Zhang, Y. (2021). Short- and long-distance signaling in plant defense. Plant J. 105, 505–517. doi: 10.1111/tpj.15068
Supek, F., Bošnjak, M., Škunca, N., Šmuc, T. (2011). REVIGO summarizes and visualizes long lists of gene ontology terms. PloS One 6, e21800. doi: 10.1371/journal.pone.0021800
Tanabe, S., Okada, M., Jikumaru, Y., Yamane, H., Kaku, H., Shibuya, N., et al. (2006). Induction of resistance against rice blast fungus in rice plants treated with a potent elicitor, n-acetylchitooligosaccharide. Biosci. Biotechnol. Biochem. 70, 1599–1605. doi: 10.1271/bbb.50677
Tateno, M., Brabham, C., DeBolt, S. (2016). Cellulose biosynthesis inhibitors – a multifunctional toolbox. J. Exp. Bot. 67, 533–542. doi: 10.1093/jxb/erv489
Vishwanathan, K., Zienkiewicz, K., Liu, Y., Janz, D., Feussner, I., Polle, A., et al. (2020). Ectomycorrhizal fungi induce systemic resistance against insects on a nonmycorrhizal plant in a CERK1-dependent manner. New Phytol. 228, 728–740. doi: 10.1111/nph.16715
Vogel, J. P., Raab, T. K., Somerville, C. R., Somerville, S. C. (2004). Mutations in PMR5 result in powdery mildew resistance and altered cell wall composition. Plant J. 40, 968–978. doi: 10.1111/j.1365-313X.2004.02264.x
Wan, J., Tanaka, K., Zhang, X.-C., Son, G. H., Brechenmacher, L., Nguyen, T. H. N., et al. (2012). LYK4, a lysin motif receptor-like kinase, is important for chitin signaling and plant innate immunity in arabidopsis. Plant Physiol. 160, 396–406. doi: 10.1104/pp.112.201699
Wan, J., Zhang, X. C., Neece, D., Ramonell, K. M., Clough, S., Kim, S. Y., et al. (2008). A LysM receptor-like kinase plays a critical role in chitin signaling and fungal resistance in arabidopsis. Plant Cell 20, 471–481. doi: 10.1105/tpc.107.056754
Yang, C., Liu, R., Pang, J., Ren, B., Zhou, H., Wang, G., et al. (2021). Poaceae-specific cell wall-derived oligosaccharides activate plant immunity via OsCERK1 during magnaporthe oryzae infection in rice. Nat. Commun. 12, 2178. doi: 10.1038/s41467-021-22456-x
Zhang, C., He, J., Dai, H., Wang, G., Zhang, X., Wang, C., et al. (2021). Discriminating symbiosis and immunity signals by receptor competition in rice. Proc. Natl. Acad. Sci. U.S.A. 118, e2023738118. doi: 10.1073/pnas.2023738118
Keywords: rice (Oryza sativa), chitin, systemic signalling, disease resistance, Bipolaris oryzae, CERK1, CEBiP, cell-wall biogenesis
Citation: Takagi M, Hotamori K, Naito K, Matsukawa S, Egusa M, Nishizawa Y, Kanno Y, Seo M, Ifuku S, Mine A and Kaminaka H (2022) Chitin-induced systemic disease resistance in rice requires both OsCERK1 and OsCEBiP and is mediated via perturbation of cell-wall biogenesis in leaves. Front. Plant Sci. 13:1064628. doi: 10.3389/fpls.2022.1064628
Received: 08 October 2022; Accepted: 09 November 2022;
Published: 28 November 2022.
Edited by:
Ho Won Jung, Dong-A University, South KoreaReviewed by:
Sung Un Huh, Kunsan National University, South KoreaCopyright © 2022 Takagi, Hotamori, Naito, Matsukawa, Egusa, Nishizawa, Kanno, Seo, Ifuku, Mine and Kaminaka. This is an open-access article distributed under the terms of the Creative Commons Attribution License (CC BY). The use, distribution or reproduction in other forums is permitted, provided the original author(s) and the copyright owner(s) are credited and that the original publication in this journal is cited, in accordance with accepted academic practice. No use, distribution or reproduction is permitted which does not comply with these terms.
*Correspondence: Hironori Kaminaka, a2FtaW5ha2FAdG90dG9yaS11LmFjLmpw
†ORCID: Mitsunori Seo, orcid.org/0000-0001-8325-7984
Shinsuke Ifuku, orcid.org/0000-0002-5001-6006
Akira Mine, orcid.org/0000-0002-4822-4009
Hironori Kaminaka, orcid.org/0000-0002-3685-8688
Disclaimer: All claims expressed in this article are solely those of the authors and do not necessarily represent those of their affiliated organizations, or those of the publisher, the editors and the reviewers. Any product that may be evaluated in this article or claim that may be made by its manufacturer is not guaranteed or endorsed by the publisher.
Research integrity at Frontiers
Learn more about the work of our research integrity team to safeguard the quality of each article we publish.