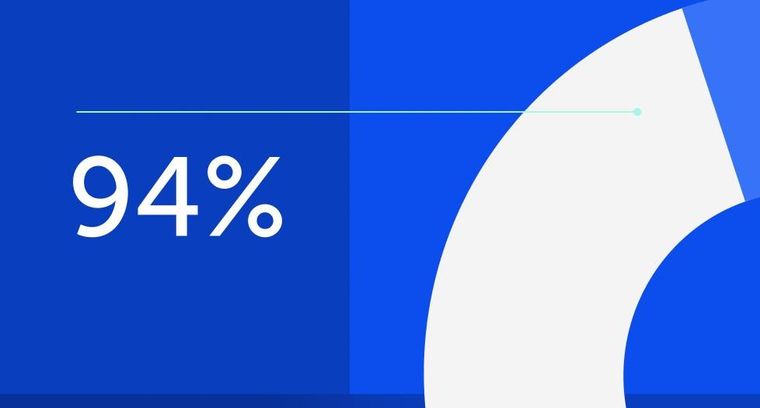
94% of researchers rate our articles as excellent or good
Learn more about the work of our research integrity team to safeguard the quality of each article we publish.
Find out more
SYSTEMATIC REVIEW article
Front. Plant Sci., 14 December 2022
Sec. Plant Systems and Synthetic Biology
Volume 13 - 2022 | https://doi.org/10.3389/fpls.2022.1064176
This article is part of the Research TopicMetabolic Engineering of Valuable Compounds in Photosynthetic OrganismsView all 11 articles
Plants produce energy-dense lipids from carbohydrates using energy acquired via photosynthesis, making plant oils an economically and sustainably attractive feedstock for conversion to biofuels and value-added bioproducts. A growing number of strategies have been developed and optimized in model plants, oilseed crops and high-biomass crops to enhance the accumulation of storage lipids (mostly triacylglycerols, TAGs) for bioenergy applications and to produce specialty lipids with increased uses and value for chemical feedstock and nutritional applications. Most successful metabolic engineering strategies involve heterologous expression of lipogenic factors that outperform those from other sources or exhibit specialized functionality. In this review, we summarize recent progress in engineering the accumulation of triacylglycerols containing - specialized fatty acids in various plant species and tissues. We also provide an inventory of specific lipogenic factors (including accession numbers) derived from a wide variety of organisms, along with their reported efficacy in supporting the accumulation of desired lipids. A review of previously obtained results serves as a foundation to guide future efforts to optimize combinations of factors to achieve further enhancements to the production and accumulation of desired lipids in a variety of plant tissues and species.
All organisms can convert carbohydrates into fatty acids (FAs), the building blocks of both phospholipids for membrane synthesis and triacylglycerols (TAGs) for carbon and energy storage. Some organisms including plants have evolved specialized lipogenic factors to accumulate large quantities of TAGs or produce specialty FAs. Bio-based TAGs, also known as storage lipids, contain more than twice the energy of carbohydrates, making them a sustainable energy-dense source of biofuels (Ohlrogge and Chapman, 2011; Singh et al., 2021). Specialty lipids containing high levels of specialty FAs can serve as feedstocks for jet fuel, nutraceuticals, and industrial products because of their distinct physical and functional properties (Dyer et al., 2008; Park et al., 2021). However, natural sources of these lipids are limited and therefore are not sufficient to meet growing demand. Plants use carbon and energy acquired from photosynthesis to synthesize FAs and accumulate TAGs and thus represent a renewable and economically viable platform for lipid production. General conservation of lipid synthesis across kingdoms makes it possible to engineer agronomic plants for the production and accumulation of desired lipids by inter-species heterologous expression of many lipogenic factors.
In plant cells, FAs are synthesized from acetyl-coenzyme A (CoA) in plastids (Figure 1; Ohlrogge and Browse, 1995; Li-Beisson et al., 2013). The heteromeric acetyl-CoA carboxylase (ACCase) catalyzes the conversion of acetyl-CoA to malonyl-CoA, the first committed step in FA synthesis. With acetyl-CoA serving as the starting unit, the acyl chain is extended by the FA synthase complex (FAS) through sequential condensation of two-carbon units from malonyl-acyl carrier protein (ACP). FAs reaching a certain chain length (typically C16 or C18) are released from ACP by fatty acyl thioesterases (FAT)A/B and exported from plastids. These FAs then enter the acyl-CoA pool in the endoplasmic reticulum (ER), where they are further modified and incorporated into glycerolipids (Figure 1). ER-localized FA elongase (FAE) can add additional two-carbon units to acyl-CoA to further elongate FAs. The acyl chains esterified to phosphatidylcholine (PC) undergo modifications to introduce double bond(s) and functional groups to FAs and the modified FAs re-enter the acyl-CoA pool through acyl-editing, a dynamic acyl exchange between PC and the acyl-CoA pool. TAGs can be assembled via sequential acylation of glycerol-3-phosphate (G3P) with acyl-CoA as the acyl donor catalyzed by glycerol-3-phosphate acyltransferase (GPAT), lysophosphatidyl acyltransferase (LPAT), phosphatidic acid phosphatase (PAP), and diacylglycerol:acyl-CoA acyltransferase (DGAT) (reviewed in Li-Beisson et al., 2013; Xu and Shanklin, 2016). Alternatively, PC can serve as the acyl donor for acylation of diacylglycerol (DAG) to form TAG by phospholipid:diacylglycerol acyltransferase (PDAT) (Dahlqvist et al., 2000). In addition, FAs esterified to PC may enter the TAG pool through the conversion of PC to DAG and subsequently to TAG by phosphatidylcholine:diacylglycerol cholinephosphotransferase (PDCT) or phospholipase C (PLC) (Wang, 2001; Lu et al., 2009). TAGs synthesized within the bilayer of the ER membrane are packaged into cytosolic lipid droplets (LDs) by coordination of LD-related proteins including SEIPIN, LDAP, LDIP, Oleosin, Caleosin, and Steroleosin (reviewed by Chapman et al., 2012 and Pyc et al., 2017).
Figure 1 Overview of FA synthesis and TAG assembly in plants. The conversion of acetyl-CoA to malonyl-CoA by ACCase is the first committed step in fatty acid synthesis in plastids. With acetyl-CoA serving as the starting unit, the acyl chain is extended by sequential condensation of two-carbon units from malonyl-ACP by FAS complex. FAs exported from plastids enter the acyl-CoA pool in the ER and can be incorporated into PC, where acyl groups are modified and re-enter the acyl-CoA pool thorough acyl editing. The Kennedy pathway incorporates acyl-CoA into glycerolipids via sequential acylation of G3P by GPAT, LPAT and DGAT. TAG can be synthesized through acyl-CoA-dependent (DGAT converting DAG and acyl-CoA to TAG) and acyl-CoA-independent (PDAT synthesizing TAG from DAG and PC) pathways. TAGs are packaged into LDs and protected by LD-associated proteins. ACCase, acetyl-CoA carboxylase; ACP, acyl carrier protein; CoA, coenzyme A; MCMT, malonyl-CoA: ACP malonyltransferase; FAS, fatty acid synthase; FATA/B, fatty acyl thioesterase A/B; ALT, acyl-lipid thioesterase; FAE, fatty acid elongase; GPAT, glycerol-3-phosphate acyltransferase; LPAT, lysophosphatidyl acyltransferase; PAP, phosphatidic acid phosphatase; DGAT, diacylglycerol:acyl-CoA acyltransferase; PDAT: phospholipid:diacylglycerol acyltransferase; PLC, phospholipase C; PDCT, phosphatidylcholine:diacylglycerol cholinephosphotransferase; LPCAT, lysophosphatidylcholine acyltransferase; PLA, phospholipase A; CPS, cyclopropane synthase; EPX, epoxygenase; FAD, fatty acid desaturase; FAH; fatty acyl hydroxylase; FADX, fatty acid conjugase; G3P, glycerol-3-phosphate; LPA, lysophosphatidic acid; PA, phosphatidic acid; DAG, diacylglycerol; PC, phosphatidylcholine; LPC, lysophosphatidylcholine; TAG, triacylglycerol.
In plants, most TAGs are synthesized and stored in seeds, serving as a major reserve of carbon and energy for seed germination and seedling establishment. In contrast, plant vegetative tissues usually contain trace amounts of TAGs despite their high capacity for FA synthesis. Studies of lipid engineering in plants increasingly focus on 1) producing value-added specialty lipids in seeds of oilseed crops (e.g., Camelina sativa and Brassica napus) by introducing specialized lipogenic factors to increase the value of seed oil (Xu et al., 2020; Yuan and Li, 2020) and 2) enhancing the accumulation of TAGs in vegetative tissues of high-biomass crops (e.g., Nicotiana tabacum, Sorghum bicolor, and Saccharum spp. Hybrids) by overexpressing TAG-enhancing lipogenic factors to increase the overall lipid yield on a per plant and unit land area basis (Vanhercke et al., 2019b; Park et al., 2021). Almost all these lipid engineering approaches require heterologous expression of lipogenic factors from other organisms. In this review, we present a survey of work evaluating the efficacy of lipogenic factors from various organisms in engineering lipids in selected target plant species. Specifically, we provide a list of lipogenic factors that exhibit specialized functional features in FA synthesis and modification (Section I and Table 1), glycerolipid assembly (Section II and Table 2) and LD biogenesis (Section III and Table 3) and highlight recent progress in optimizing combinations of lipogenic factors for enhanced production of desired lipids, and discuss challenges and future opportunities for lipid engineering in plants. It can be difficult to correlate the effects of expressed genes on lipid metabolism, especially those discussed in review articles, with their precise coding sequences. In this work we have included accession numbers wherever possible to address and remedy this issue.
Table 1 List of specialized lipogenic factors involved in FA synthesis and modification used for lipid engineering in plants.
Table 2 List of specialized lipogenic factors involved in glycerolipid assembly used for lipid engineering in plants.
Table 3 List of specialized lipogenic factors involved in LD biogenesis used for lipid engineering in plants.
Lipogenic factors involved in FA synthesis and modification determine the diversity of FAs with respect to their carbon chain lengths, degree of unsaturation, and addition of a variety of functional groups, which determine their physical properties and potential industrial uses. Typical FAs found conserved in the plant kingdom range from 16 to 18 carbons in length and contain 0 to 3 double bounds at Δ9, Δ12 and Δ15 positions (i.e., counting relative to the carboxyl group). In contrast to these “common” FAs, some FAs with shorter or longer chain lengths, additional double bonds, double bond(s) at different position(s), or functional groups at specific locations along the carbon chain are found in specific groups of plant species or non-plant organisms, and thus are referred to as specialty FAs. The structural properties of these specialty FAs make them promising feedstocks for biofuels, industrial products and nutraceuticals. To increase the value of plant lipids, engineering strategies involving the heterologous expression of lipogenic factors related to FA synthesis and modification have been developed to produce specialty FAs in both seed and vegetative tissues of domesticated plant species (Park et al., 2021).
In this section, we describe efforts to evaluate enzymes in the FA biosynthesis and modification pathway that are responsible for producing the following well-studied types of specialty FAs. Medium-chain FAs result from the action of FA thioesterases that release acyl chains from acyl carrier protein (ACP). Hydroxy, epoxy and conjugated FAs arise from the action of enzymes that evolved from the Δ12-oleic FA desaturase 2 (FAD2) class of integral membrane desaturases (Shanklin and Cahoon, 1998) which act primarily on oleic acid esterified to PC. Omega-7 monounsaturated FAs with a double bound at the ω7 position (i.e., counting relative to the methyl end of FAs) can be produced by Δ9-acyl-ACP or Δ9-acyl-CoA desaturase with high specificity for 16:0-ACP or 16:0-CoA, respectively (Bondaruk et al., 2007; Nguyen et al., 2010). Very-long-chain PUFAs arise from the action of multiple desaturases and elongases, and their engineering represents a tour-de-force in heterologous expression and pathway optimization (Napier et al., 2019). The last example is the addition of a cyclopropyl group across the double bond in oleic acid by cyclopropane synthase, a class of enzymes present in plants and prokaryotes (Bao et al., 2002; Bao et al., 2003). In section II and III, we summarize the approaches to incorporate these specialized FAs into TAGs and subsequently package them into LDs.
Medium-chain FAs (MCFAs) include FAs of 8-14 carbons in lengths, generated by the hydrolysis of FA from acyl carrier protein between the C8 and C14 stages of elongation via variants of FATB with defined chain length specificities (Figure 1). Lipids containing MCFAs are naturally produced in palm kernel (Elaeis guineensis), coconut (Cocos nucifera), and cuphea genus (Cuphea pulcherrima, Cuphea viscosissima, Cuphea palustris, Cuphea hookeriana), and these plants derived MCFAs serve as potential feedstocks for jet fuel and industrial products such as cosmetics and detergents (Dyer et al., 2008; Kallio et al., 2014; Park et al., 2021). To engineer the production of MCFAs in oilseed crops, FATB variants that specifically hydrolyze C8-C14 FAs from acyl-ACPs were isolated from California bay (Umbellularia californica) and Cuphea and expressed in Arabidopsis thaliana, Camelina sativa, and Brassica napus. Heterologous expression of U. californica FATB1 produced MCFAs primarily consisting of lauric acid (C12:0) up to 21%, 37%, and 50% of seed oil in Camelina, Arabidopsis, and B. napus, respectively (Eccleston et al., 1996; Voelker et al., 1996; Tjellström et al., 2013; Kim et al., 2015b). FATB variants from different Cuphea species showed different efficiencies and substrate chain length specificities when expressed in oilseed plants (Table 1). Expression of FATB2 from C. hookeriana could produce MCFAs ranging from C8 to C14 with capric acid (C10:0) as the most abundant species accounted for approximate 12%, 22%, and 40% of total seed lipids in Camelina, Arabidopsis, and B. napus, respectively (Dehesh et al., 1996; Tjellström et al., 2013; Kim et al., 2015b). FATB1 from C. viscosissima produced 15% MCFAs with chain lengths varying from C8 to C14 in seed oil when expressed in Camelina, whereas FATB2 from C. palustris produced only myristic acid (C14:0) to 24% and 39% of seed oil in Camelina and Arabidopsis, respectively (Tjellström et al., 2013; Kim et al., 2015b). Relatively low levels of MCFAs (1.2%-7.5% of seed oil) were detected in seeds of Arabidopsis and Camelina expressing FATB1, FATB3 or FATB4 from C. pulcherrima, compared with FATBs from other Cuphea species. Based on data collected from Camelina, UcFATB1 and CpFATB2 seem to be the most effective FATB variants in producing MCFAs with UcFATB1 preferentially generating lauric acid (C12:0) and CpFATB2 exclusively producing myristic acid (C14:0). Furthermore, co-expression of ChFATB2 or CpFATB1 with a Cuphea medium-chain-specific 3-ketoacyl-ACP synthase (KAS4) that catalyzes the condensation of acyl-ACP with malonyl-ACP increased MCFA content by up to 40% in B. napus seed oil as compared with that of plants expressing FATB alone (Dehesh et al., 1998). Disruption of acyl-ACP synthetase (AAE15/16) that re-activates FAs released from acyl-ACP in Arabidopsis overexpressing Cuphea FATB further enhanced MCFA accumulation in seeds (Tjellström et al., 2013). In addition to the FAT-type thioesterases containing two “hotdog” folds (Mayer and Shanklin, 2005), another acyl-ACP thioesterase family, acyl-lipid thioesterase (ALT) with a single “hotdog” fold, is generally present in all classes of plants (Kalinger et al., 2020). Overexpression of Arabidopsis ALT1 or ALT4 in Camelina seeds and Nicotiana benthamiana leaves yielded C6–C14 MCFA at a relatively lower level (as much as 3.5% of seed oil) compared to the effective FATB isoforms (Table 1; Kalinger et al., 2021).
The hydroxylation of FAs is mediated by the action of FA hydroxylase (FAH), the first functionally divergent FAD2 homolog to be identified (Figure 1). That they are mechanistically related is evidenced by reports that as few as four substitutions between desaturase and hydroxylase can interconvert their functionality (Broun et al., 1998; Broadwater et al., 2002). FAs with hydroxyl groups attached to the acyl chain are useful feedstocks for the formulation of plastics and lubricants (Dyer et al., 2008). The major natural source of hydroxy FAs for industrial uses is castor bean (Ricinus communis), which accumulates 90% hydroxy FAs (mostly ricinoleic acid, C18:1-OH) in its seed oil. The enzyme responsible for synthesizing hydroxy FAs in castor is FAH12 (van de Loo et al., 1995). Heterologous expression of RcFAH12 in Arabidopsis led to accumulation of hydroxy FAs consisting of primarily ricinoleic acid accounted for up to 19% of seed oil in wild type, fad2/fae1, fae1, fad3, or fad3/fae1 backgrounds (Broun and Somerville, 1997; Smith et al., 2003). Similarly, wild-type Camelina expressing RcFAH12 produced approximately 15% hydroxy FAs in seed oil (Aryal and Lu, 2018). Expression of Hiptage benghalensis hydroxylases HbFAH12-1 and HbFAH12-2 in Arabidopsis fad2/fae1 mutant yielded up to 21% and 18% hydroxy FA, respectively, in seed oil (Zhou et al., 2013). In contrast to the plant derived FAH12, a FAH homolog isolated from a fungal pathogen, Claviceps purpurea, produced 25% hydroxy FAs in seed oil when expressed in the Arabidopsis fad2/fae1 mutant (Meesapyodsuk and Qiu, 2008). While RcFAH12 can effectively produce hydroxy FAs in target plants and most plant engineering strategies to date have used RcFAH12 to synthesize hydroxy FAs, searching for a more effective FAH from other species to further enhance the accumulation of hydroxy FAs in bioengineered crops might be productive. Lesquerella (Physaria fendleri), a Brassicaceae species closely related to Arabidopsis and Camelina, produces about 50% of lesquerolic acid (C20:1-OH), an elongated form of ricinoleic acid, in its seed oil (Horn et al., 2016). Thus, Lesquerella represents a promising alternative industrial oilseed for HFA production, and specialized HFA-related factors in Lesquerella represent a promising source for engineering HFA accumulation in other crops (Horn et al., 2016; Chen et al., 2021). A recent study demonstrated the production of erythro-9,10-dihydroxystearate, a vicinal diol by an acyl-ACP desaturase variant via dioxygenase chemistry (Whittle et al., 2020). The identification of additional genes that are more efficient at vicinal diol production may facilitate large scale production of these compounds that are difficult to synthesize chemically.
An epoxy group with its oxygen bridging between adjacent carbons of fatty acyl chains conveys unique chemical reactivity useful for the production of plastics, polymers, coatings, and glues. Epoxy FAs are enriched in seed oils of certain plant species belonging to the Asteraceae and Euphorbiaceae families (Cahoon et al., 2002). Interestingly, the biosynthesis of epoxy FAs in different plant species is catalyzed by different classes of epoxygenase (EPX) enzymes. Those responsible for epoxy FA biosynthesis in Asteraceae species such as Crepis palaestina, Stokesia laevis, and Vernonia galamensis are divergent forms of the FAD2 desaturase, whereas epoxygenases in Euphorbiaceae species such as Euphorbia lagascae are cytochrome P450 enzymes (Bafor et al., 1993; Liu et al., 1998; Cahoon et al., 2002). Despite the distinction of these two classes of EPX, heterologous expression of these enzymes in plants resulted in accumulation of similar levels of epoxy FAs (mostly vernolic acid, C18:1- Δ12-epoxy FA) in seed oils (Table 1). Expression of the FAD2-like EPX coding genes from C. palaestina and S. laevis led to accumulation of approximately 2.4%-8% epoxy FAs in seed oils of Arabidopsis and soybean, and the cytochrome P450-type EPX from E. lagascae produced about 8% epoxy FAs in soybean somatic embryos (Singh et al., 2001; Cahoon et al., 2002; Hatanaka et al., 2004; Li et al., 2010). Providing the exogenous CpEPX with more linoleic acid (C18:2) substrate by disrupting FAD3 and FAE1 in Arabidopsis increased the levels of epoxy FAs to 8.6% of seed oil (Zhou et al., 2006). Previous studies suggested that heterologous expression of either type of EPX can reduce the accumulation of linoleic acid in target plants probably caused by decreased activity of the endogenous FAD2 enzyme, and co-expression of EPX with a typical FAD2 dramatically enhanced the production of epoxy FAs to 21% of seed oil in Arabidopsis fad3/fae1 mutant (Singh et al., 2001; Cahoon et al., 2002; Zhou et al., 2006). In addition, epoxy FAs can also be engineered in non-seed tissues. Expression of ElEPX in tobacco (Nicotiana tabacum) calli produced epoxy FAs accounted for 15% of total lipids (Cahoon et al., 2002). Transient expression of SlEPX in petunia (Petunia hybrida) leaves or a FAD2-like EPX from V. galamensis in N. benthamiana leaves resulted in accumulation of 0.5% or 8.7% epoxy FAs in total leaf lipids, respectively (Li et al., 2010; Sun et al., 2022). Moreover, co-expression of VgEPX with VgFAD2 increased the level of epoxy FAs to 13.1% of total lipids in N. benthamiana leaves (Sun et al., 2022). Collectively, divergent classes of EPX from different plant species seem to be equally effective in producing epoxy FAs in seeds and providing more linoleic acid by overexpressing a “typical” FAD2 or disrupting FAD3 and FAE1 is critical for further increasing epoxy FA levels.
Omega-7 unsaturated FAs (ω-7 FAs) are potential feedstocks for the production of octene, a high-demand industrial product used for polyethylene production (Nguyen et al., 2010). Some plants (e.g., milkweed [Asclepias syriaca] and cat’s claw vine [Doxantha unguis-cati]) can naturally produce ω-7 FAs (e.g., palmitoleic acid 16:1Δ9 and cis-vaccenic acid 18:1Δ11) by Δ9-acyl-ACP desaturase (AAD) with high specificity for 16:0-ACP (Cahoon et al., 1997; Cahoon et al., 1998). Heterologous expression of the milkweed 16:0-ACP desaturase in Arabidopsis failed to produce detectable ω-7 FA, while the Doxantha 16:0-ACP desaturase produced approximately 28% and 9% ω-7 FAs in Arabidopsis and Brassica seed oil, respectively (Bondaruk et al., 2007). An AAD variant with high specificity for converting 16:0-ACP to 16:1 Δ9-ACP was selected from a pool of randomized mutants of castor AAD (Cahoon and Shanklin, 2000), and expression of this engineered enzyme (Com25) in Arabidopsis seeds resulted in accumulation of ω-7 FAs to 14% of seed oil (Nguyen et al., 2010). Increasing the level of 16:0-ACP by silencing the 16:0-ACP elongase, β-ketoacyl-ACP synthase II (KASII/FAB1), in fae1 mutant overexpressing Com25 further increased the content of ω-7 FAs to 56% of seed oil. Co-expressing Com25 with two fungal Δ9-16:0-CoA desaturases from Stagonospora nodorum (SnD9D) and Aspergillus nidulans (AnD9D) in fab1/fae1 mutant increased ω-7 FA content to 71% of seed oil by desaturating saturated FAs after transfer from the plastid to the ER (Nguyen et al., 2010). A similar strategy co-expressing Com25 and a Δ9-16:0-CoA desaturase from Caenorhabditis elegans (FAT5) in Camelina seeds with 16:0-ACP substrate pools increased by silencing genes encoding KASII/FAB1, FAE1, and16:0-ACP thioesterase (FATB) increased ω-7 FAs to 60-65% of seed oil (Nguyen et al., 2015).
The FAD2 desaturases that produce conjugated FAs by converting Δ9 and Δ12 double bonds to Δ11 and Δ13 double bonds are designated as FA conjugases (FADX) (Figure 1; Cahoon et al., 1999; Dyer et al., 2002). The higher oxidation rates of conjugated FAs relative to typical polyunsaturated FAs make them useful as drying agents in paints and inks. Conjugated FAs can also serve as health supplements as they have been reported to have fat-reducing and anticancer effects in animals (Lee et al., 2002; Dyer et al., 2008; Yuan et al., 2014). Natural sources of conjugated FAs include tung tree (Vernicia fordii), Momordica charantia, Impatiens balsamina, and Calendula officinalis, and genes encoding FADX enzymes have been isolated from these plant species and evaluated for their efficacy in producing conjugated FAs in model plants and oilseed crops. Ectopic expression of FADX coding genes from I. balsamina, M. charantia, and C. officinalis in somatic soybean (Glycine max) embryos resulted in production of conjugated FAs to approximately 5%, 18%, and 22% of total FAs, respectively (Cahoon et al., 1999). For engineering approaches carried out in Arabidopsis seeds, mutants with FA desaturase 3 (FAD3) and FAE1 disrupted are used to provide more substrates (linoleic acid) for FADX. Arabidopsis fad3/fae1 mutants expressing FADX genes from V. fordii, M. charantia, and C. officinalis accumulated approximately 6%, 13%, and 15% conjugated FAs in seed oil, respectively (Cahoon et al., 1999; Cahoon et al., 2006). Recent attempts to engineer conjugated FAs in plant vegetative tissues by expressing VfFADX in Arabidopsis successfully produced conjugated FAs (eleostearic acid, 18:3, Δ9c, Δ11t, Δ13t) to 2% of total neutral lipids in leaves (Yurchenko et al., 2017). Among all FADX enzymes tested so far, CoFADX seems to be the most effective enzyme for producing high levels of conjugated FAs in both Arabidopsis and soybean, but different FADX orthologs produce different types of conjugated FAs. Conjugated FAs produced by CoFADX comprise exclusively calendic acid (18:3, Δ8t, Δ10t, Δ12c), while eleostearic acid is the primary conjugated FAs detected in transgenic plants expressing VfFADX or McFADX. In future efforts to engineer conjugated FAs, it will be important to select a FADX that produces high levels and desired types of conjugated FAs.
Very-long-chain polyunsaturated FAs (VLCPUFA) such as eicosapentaenoic acid (EPA) and docosahexaenoic acid (DHA) are FAs with 20 or 22 carbons and 4 to 6 double bonds. EPA and DHA are valuable nutraceuticals because of their beneficial roles in fetal neuronal system development and cardiovascular diseases prevention (Tocher et al., 2019). Marine fish oils are a major source of EPA, DHA and other ω3-VLCPUFAs. However, the marine-sourced EPA and DHA are insufficient to meet the increasing demand for these FAs in human diet (Tocher et al., 2019). To develop sustainable alternative sources of EPA and DHA, in the past two decades, enormous research effort has been directed at engineering ω3-VLCPUFAs in plant oils, and significant progress has been achieved in producing EPA and DHA in oilseed crops (Napier et al., 2019). There are two pathways that can produce VLCPUFAs from linoleic acids: 1) the conventional pathway including Δ6-desaturase (DES), Δ6-elongase (ELO), Δ5-DES, Δ5-ELO, and Δ4-DES; and 2) the alternative pathway using Δ9-ELO, Δ8-DES, Δ5-DES, Δ5-ELO, and Δ4-DES. Both pathways have been introduced into plants and successfully produced EPA and DHA in both seed and vegetative tissues (Qi et al., 2004; Wu et al., 2005; Wood et al., 2009; Petrie et al., 2010). It was later found that, besides the minimum five enzymes required for DHA biosynthesis in plants, introduction of a highly active Δ12-DES from Lachancea kluyveri and a Δ15/ω3-DES with broad substrate specificity from Pichia pastoris could effectively increase the ratio of ω3/ω6 FAs and therefore the level of DHA in seed oil (Petrie et al., 2012; Petrie et al., 2014). To optimize the engineering strategy to produce high levels of EPA and DHA in plants, DES and ELO enzymes from a wide variety of species including algae, fungi, oomycetes, mosses, animals, and flowering plants were introduced into plants to test their efficacy in producing EPA and DHA in various plant species (Abbadi et al., 2004; Qi et al., 2004; Wu et al., 2005; Wood et al., 2009; Petrie et al., 2010; Petrie et al., 2012; Petrie et al., 2014; Han et al., 2020; Han et al., 2022). The optimal enzyme combination that achieved the highest levels of EPA and DHA (over 20%) in seed oil reported to date consists of a Δ12-DES from Phytophthora sojae, Δ15/ω3-DES from Phytophthora infestans, Δ6-DES from Ostreococcus tauri, Δ6-ELO from Physcomitrella patens, Δ5-DES from Thraustochytrium sp., Δ5-ELO from O. tauri, and Δ4-DES from Ostreococcus RCC809 (Han et al., 2020; Han et al., 2022).
Cyclopropane FAs (CPAs), such as dihydrosterculic acid (9, 10-methylene octadecanoic acid) and lactobacillic acid (11, 12 methylene octadecanoic acid), are specialized FAs that contain a cyclopropane group (three-carbon carbocyclic ring) within the carbon chain. They are found in bacteria and certain plant species such as Litchi chinensis. The highly strained and reactive carbocyclic ring of CPA readily opens to form methyl-branched fatty acids, which exhibit unique physical and chemical properties such as low melting temperatures, resistance to oxidation, and propensity for self-polymerization, making CPAs suitable for application in lubricants, paints, and coatings (Carlsson et al., 2011). Ectopic expression of genes encoding cyclopropane synthase (CPS), the enzyme catalyzes the conversion of monounsaturated FAs to CPAs (Figure 1), successfully produced CPAs in plants that normally lack these compounds. Intriguingly, the CPS gene from Escherichia coli is more effective than CPS homologs isolated from plant species for synthesizing CPAs in plants (Table 1). Engineering of CPAs in Arabidopsis and Camelina seeds were carried out in mutant lines with reduced FA desaturase 2 (FAD2) and FA elongase 1 (FAE1) that accumulate increased levels of oleoyl substrates for CPA production. Expression of CPS genes cloned from Sterculia foetida and cotton (Gossypium hirsutum) in Arabidopsis fad2/fae1 mutant lines led to the accumulation of CPA to between 0.05% and 1% of total seed FAs, respectively (Yu et al., 2011). In contrast, the expression of E. coli CPS resulted in nearly 10% CPA accumulation in seed oil in Arabidopsis and Camelina (Yu et al., 2014; Yu et al., 2018). Recently, researchers tested the ability of CPS enzymes to synthesize CPAs in plant vegetative tissues by transiently expressing cotton or E. coli CPS genes in N. benthamiana leaves and found that GhCPS1 produced up to 1% CPA of total leaf FAs while EcCPS expression led to the accumulation of CPA up to 3.7% of total lipids (Okada et al., 2020). The levels of CPAs in leaf lipids can be further elevated to 4.8% and 11.8% by silencing the expression of endogenous NbFAD2 in leaves expressing GhCPS1 and EcCPS, respectively (Okada et al., 2020). Therefore, future strategies to engineer CPA accumulation in plant seed and vegetative tissues will exploit EcCPS rather than plant CPS variants.
TAGs, also known as storage lipids, are the most abundant form of vegetable oils. They primarily accumulate in seeds to provide energy for seed germination and establishment. In plant vegetative tissues such as leaves, TAGs are barely detectable and serve primarily as transient intermediates for FAs removed from membrane lipids prior to their degradation (Xu and Shanklin, 2016). Accumulation of free FAs in cells and specialty FAs in membrane lipids can result in negative effects on plant growth. One reason for this is that they elicit feedback inhibition of FA synthesis via biotin attachment domain-containing protein (BADC), a negative regulator of ACCase (Yang et al., 2015; Salie et al., 2016; Zale et al., 2016; Keereetaweep et al., 2018; Yu et al., 2021). Channeling FA flux toward the TAG pool can reduce the accumulation of free FAs and remove specialty FAs from membrane lipids, thereby mitigating their negative growth effects and further enhancing the accumulation of lipids with desired acyl composition. Therefore, Lipogenic factors involved in glycerolipid assembly capable of accommodating specialty FA substrates represent critical targets for enhancing specialty FA-containing TAG accumulation in plants. In the following subsections, we summarize previous efforts to assess the efficacies of TAG-assembly-related enzymes for optimizing the accumulation of desired lipids.
Incorporation of specialty FA into TAG requires specialized enzymes that can recognize the specialized FA for catalyzing multiple steps of TAG assembly (Figure 1). In the glycerolipid biosynthesis pathway, The ER-localized LPAT catalyzes the transfer of FAs from the acyl-CoA pool to LPA to form PA, which serves as a key intermediate for channeling FAs into TAGs and membrane lipids. Lysophosphatidylcholine acyltransferase (LPCAT) incorporates FAs into PC. PDCT and PLC catalyze the conversion of PC to DAG and thereby allow FAs esterified to PC to enter the DAG pool, which are subsequently converted to TAG by DGAT and PDAT. Below we describe some variants of these enzymes that are specialized for incorporating different types of specialty FAs into TAGs.
Divergent LPATs from specialty FA-accumulating organisms have evolved specialized substrate specificities for incorporating specialty FAs into TAGs (Table 2). For instance, LPAT variants from organisms naturally accumulating MCFAs (e.g., C. nucifera, C. viscosissima, and C. pulcherrima) preferentially incorporate MCFAs to the sn-2 position of LPA, and when combined with FATBs, enabled efficient deposition of MCFAs at the sn-2 position of TAG and further increased total MCFA contents (Knutzon et al., 1999; Kim et al., 2015a; Kim et al., 2015b). Notably, expression of CnLPAT, CvLPAT2, and CpuLPAT2a resulted in the deposition of capric acid (C10:0) at the TAG sn-2 position, whereas CpuLPATB expression led to accumulation of myristic acid (C14:0) instead of capric acid (C10:0) at the sn-2 position of TAG, suggesting distinct substrate specificities of divergent forms of LPATs for different MCFAs (Knutzon et al., 1999; Kim et al., 2015a; Kim et al., 2015b). RcLPAT2 isolated from castor and VfLPAT2 from tung tree producing conjugated FAs improved the accumulation of hydroxy FAs and conjugated FAs, respectively, in Arabidopsis seeds (Shockey et al., 2019). Co-expression of the LPAT from epoxy FA-rich V. galamensis with VgEPX increased the level of epoxy FAs from 8.7% (VgEPX alone) to 16.7% of total lipids in N. benthamiana leaves (Sun et al., 2022). For CPA engineering, co-expression of SfLPAT2 from CPA-enriched S. foetida with EcCPS in the fad2/fae1 mutant resulted in the accumulation of CPA at both sn-1 and sn-2 positions of PC and further increased CPA contents to 35% and 18% of seed oils in Arabidopsis and Camelina, respectively (Yu et al., 2014; Yu et al., 2018).
Given the crucial role of PC in acyl editing and TAG biosynthesis, specialized LPCAT, the enzyme catalyzing the conversion of lysophosphatidylcholine (LPC) and acyl-CoA to PC, may contribute to the incorporation of specialty FAs into TAGs. Indeed, the specialized LPCAT from V. galamensis, an oleaginous plant containing high levels of epoxy FAs in its seed oil, greatly enhanced the accumulation of epoxy FAs from 8.7% to as much as 19.4% of total lipids when co-expressed with VgEPX in N. benthamiana leaves (Sun et al., 2022). Studies of transgenic Arabidopsis and Camelina engineered to produce CPA and hydroxy FAs revealed that PCs containing these specialty FAs were not efficiently converted to DAGs and TAGs, identifying bottlenecks for the accumulation of specialty FAs (Bates and Browse, 2011; Yu et al., 2018). To address these bottlenecks, specialized enzymes that convert CPA-containing or hydroxy-containing PCs to DAGs including PDCT and PLC were used to further enhance the production of specialty FAs (Figure 1; Table 2). In efforts to engineer hydroxy FAs in model and crop plants, a PDCT (RcPDCT) and a PLC (RcPLCL1) were isolated from castor and tested in transgenic plants expressing RcFAH12. It was shown that both RcPDCT and RcPLCL1 could enrich hydroxy FA in DAG and TAG and increase total hydroxy FA contents from 10-15% to approximately 20% of seed oil (Hu et al., 2012; Aryal and Lu, 2018). Co-expression of LcPDCT from CPA-enriched L. chinensis with EcCPS enhanced the deposition of CPA in DAG and TAG and led to a 50% increase of CPA in seed oil compared with that of plants expressing EcCPS alone (Yu et al., 2019).
Several studies have reported that specialized DGATs and PDATs with high specificities for specialty FAs are necessary for addressing bottlenecks for the accumulation of specialty FAs in target plants (Park et al., 2021; Lunn et al., 2022). In one of such study, a DGAT from C. pulcherrima, namely CpuDGAT1 was identified, which showed a higher enzyme activity toward MCFA substrates relative to typical FAs. Expression of CpuDGAT1 in Camelina seeds containing MCFA produced by the exogenous CvFATB1 enriched MCFA (capric acid, 10:0) in TAG and increased capric acid content from 8% to 14.5% of seed oil (Iskandarov et al., 2017). Two DGATs from V. galamensis were tested in petunia leaves and soybean seeds for their ability to enhance epoxy FA accumulation. When co-expressed with the epoxygenase gene from Stokesia laevis (SlEPX), both VgDGAT1 and VgDGAT2 further increased epoxy FA contents in petunia leaves and soybean seeds, and VgDGAT2 seemed to have a greater impact on epoxy FA accumulation than VgDGAT1 (Li et al., 2010). To engineer conjugated FAs in Arabidopsis, VfDGAT2, a DGAT gene isolated from tung tree was co-expressed with VfFADX. While no significant increase in eleostearic acid was detected in seeds co-expressing VfDGAT2 and VfFADX relative to that in seeds expressing VfFADX alone, introducing VfDGAT2 into Arabidopsis leaves expressing VfFADX resulted in redirection of eleostearic acids from phospholipids to TAGs, an increase in eleostearic acid contents, and mitigation of negative growth effects (Yurchenko et al., 2017; Shockey et al., 2019). For hydroxy FA engineering, specialized DGAT and PDAT isolated from R. communis were combined with RcFAH12 individually to produce TAGs with high levels of hydroxy FAs, and both RcDGAT2 and RcPDAT1A enhanced the incorporation of hydroxy FAs into TAG and increased the content of hydroxy FAs to 30% and 27% of seed oil, respectively (Burgal et al., 2008; van Erp et al., 2011; Shockey et al., 2019).
To further enhance the production of specialty TAGs, enzymes involved in different steps of TAG assembly were combined to maximize the incorporation of the specialty FAs into TAGs (Table 2). Combining CvLPAT2 and CpuDGAT1 from MCFA-enriched Cuphea species greatly enriched capric acid (C10:0) accumulation in TAG and increased capric acid content to 23.7% of seed oil, which is higher than that in Camelina expressing these enzymes individually (Iskandarov et al., 2017). For hydroxy FA engineering, RcLPAT2 and RcDGAT2 isolated from castor synergistically increased the level of hydroxy FA to up to 30% of seed oil, but adding RcGPAT9, a specialized GPAT that incorporates hydroxy FAs to the sn-1 position of G3P, to this combination did not further boost the accumulation of hydroxy FAs (Shockey et al., 2019). In a similar study, Lunn et al. (2019) successfully enriched tri-hydroxy-TAG, increased hydroxy FA to 34% of seed oil, and restored seed oil content to wild-type levels by co-expressing RcGPAT9, RcLPAT2, RcPDAT1A in an RcFAH12 transformed Arabidopsis fae1 mutant (Table 2). Another combination including RcLPCAT, RcPDCT, RcPDAT1-2, RcDGAT2 produced about 31% hydroxy FA in Arabidopsis fae1 mutant seeds expressing RcFAH12 and increased both seed size and oil per seed (Park et al., 2022).
Acetyl-TAGs (acTAGs) are specialty TAGs with an acetate esterified to the sn-3 position in place of a long-chain fatty acid. Oils containing acTAGs exhibit reduced viscosity and therefore have high value in a wide variety of industrial applications such emulsifiers and lubricants. Specialized DGATs (DAcT) responsible for acTAG biosynthesis were isolated from Euonymus alatus and Euonymus fortune, plants that naturally produce acTAGs in their seeds. Heterologous expression of EaDAcT resulted in accumulation of 40% and 52% of acTAG in seeds of Arabidopsis and Camelina, respectively (Durrett et al., 2010). EfDAcT, functioning more efficiently than EaDAcT, produced an average of 72% acTAG in transgenic Camelina seeds (Alkotami et al., 2021). Interestingly, co-expression of CnLPAT from MCFA-containing coconut and EaDAcT from acTAG-enriched E. alatus in camelina plants expressing UcFATB1 produced acTAGs with MCFAs, which have yet to be found in nature, suggesting a potential synthetic-biology strategy for creating novel lipid structures in plants (Bansal et al., 2018).
DGAT enzymes catalyze the final committed step of TAG biosynthesis, and an efficient DGAT is key to enhancing TAG accumulation in plants (Figure 1). Zienkiewicz et al. (2017) screened six out of 12DGATs from Nannochloropsis oceanica, a microalga that produces high amounts of TAGs, and identified DGAT5 (DGTT5) as the most efficient isoform for restoring TAG synthesis in a TAG synthesis-deficient mutant of yeast. Transient expression of NoDGTT5 in N. benthamiana leaves led to a 2-fold increase in TAG, and stable expression of NoDGTT5 in Arabidopsis increased leaf TAG contents by 6-fold and boosted seed oil content by 50% (Zienkiewicz et al., 2017). In another study of DGATs from microalga, DGAT1 from Chlorella ellipsoidea increased the oil content by 8–37% and by 12–18% in seeds of Arabidopsis and B. napus, respectively (Guo et al., 2017). In addition, mouse (Mus musculus) DGAT2, the predominant DGAT responsible for TAG biosynthesis in mouse, when transiently expressed in N. benthamiana leaves, produced over 20-fold more TAG than that of control leaves (Cai et al., 2019). Recently, a study of Cyperus esculentus, a unique plant accumulating large amounts of TAG in its underground tubers, revealed that its heterologous expression in N. tabacum increased the TAG content to 5.5% of leaf dry weight (DW), which is 7.2-fold and 1.7-fold higher than that in wild-type leaves and leaves expressing AtDGAT1, respectively (Gao et al., 2021). Moreover, CeDGAT2-2 expression resulted in a substantial increase in the proportion of oleic acid in N. tabacum leaves (Gao et al., 2021). In another study, heterologous expression of Arabidopsis DGAT1 reportedly led to a 7-fold increase in TAG contents in N. tabacum leaves (Bouvier-Navé et al., 2000). That the DGATs tested in different studies were driven by different promoters, expressed either transiently or stably, and tested in different plant tissues and species, precludes us from making meaningful comparisons for assessing the relative efficacy of DGATs from different sources. Thus, it would be useful to evaluate all promising DGATs under same conditions and in same tissues and target organisms.
It has been demonstrated in Arabidopsis that FA degradation proceeds via a TAG intermediate (Fan et al., 2014). Emerging evidence indicates that proper and efficient packaging of TAGs into LDs is critical for increasing the capacity of lipid accumulation in plant cells, and some lipogenic factors involved in this process have been included in metabolic engineering strategies to enhance lipid production in plants (Table 3). In this section, we describe some attempts to enhance specialty lipid accumulation in plants with the use of LD-related factors and list other LD-related factors that could be engineered for specialty lipid accumulation in plants.
Oleosins (OLE), the predominant LD coat proteins specific to plants, have been used in several studies to engineer LDs for increased lipid accumulation in plant cells. The L-oleosin from sesame (Sesamum indicum) and especially its modified version (cysteine- [Cys]-oleosin) have been combined with other lipogenic factors to engineer storage lipids in vegetative tissues of Arabidopsis, N. tabacum, Solanum tuberosum, and Sorghum bicolor (Winichayakul et al., 2013; Vanhercke et al., 2014; Vanhercke et al., 2017; Liu et al., 2017; Vanhercke et al., 2019a). Expression of the castor RcOLE in RcFAH12-expressing Arabidopsis further increased hydroxy FA from 18% to 22% of seed oil (Lu et al., 2006).
SEIPIN, a key protein that orchestrates the machinery of LD biogenesis at the ER, can promote LD biogenesis and increase TAG contents in plants (Cai et al., 2015). Overexpression of AtSEIPIN1 in Arabidopsis seeds engineered to synthesize hydroxy FAs increased the hydroxy FA and total lipid contents, representing a potential new target for engineering specialty FAs in plants (Lunn et al., 2018). Interestingly, some LD proteins without apparent homologs in plants still exhibit conserved functional features as part of the LD biogenesis machinery when ectopically expressed in plants and thus can be used as tools to manipulate LD formation in plants. For instance, ectopic expression of the mouse fat storage-inducing transmembrane protein 2 (FIT2), an ER-localized protein that facilitates the portioning of TAGs from the ER into nascent LDs, in Arabidopsis and N. benthamiana led to increased numbers and sizes of LDs and enhanced lipid accumulation in both leaves and seeds (Cai et al., 2017). In another similar study, mouse fat-specific protein 27 (FSP27), a vertebrate-specific protein that mediates LD fusion, was found to promote LD fusion, and enhance the accumulation of LDs and TAGs when expressed in Arabidopsis and N. benthamiana (Price et al., 2020). It is an open question whether proteins related to LD formation have evolved specificities for packaging selected specialty TAGs into LDs. Future efforts to elucidate the roles of LD-related proteins in specialty FA accumulation will shed new light on metabolic engineering of desirable lipids in plants.
LD-associated lipases hydrolyze TAGs to release FAs, which are subsequently catabolized via β-oxidation in the peroxisomes to produce acetyl-CoA (Eastmond and Graham, 2001). SUGAR DEPENDENT 1 (SDP1) is a primary TAG lipase responsible for TAG degradation in plants (Eastmond, 2006; Kelly et al., 2013b). The suppression of SDP1 during seed development resulted in increased production of seed oil in Arabidopsis (van Erp et al., 2014), B. napus (Kelly et al., 2013a), Jatropha curcas (Kim et al., 2014), and soybean (Kanai et al., 2019; Aznar-Moreno et al., 2022). SDP1 from Physaria fendleri has been shown to preferentially hydrolyze TAGs containing hydroxy FAs and suppression of its expression increased total FA content by 14-19%, primarily contributing to the significantly increased hydroxy FA (Azeez et al., 2022). Recent studies identified additional proteins involved in the mobilization of LDs in plants including UBX-domain containing protein 10 (PUX10), CELL DIVISION CYCLE 48, (CDC48A), Comparative Gene Identification-58 (CGI58), ATP-binding cassette transporter-like protein (PXA1), and AT-hook motif containing nuclear localized transcriptional repressor (AHL4) (Zolman et al., 2001; James et al., 2010; Park et al., 2013; Deruyffelaere et al., 2018; Kretzschmar et al., 2018; Cai et al., 2020). Future work to tune the expression of these factors may contribute further to enhancing the accumulation of lipids, including specialty FAs, in plants.
Extensive efforts and substantial progress have been made in the past two decades to design and test metabolic engineering strategies for producing desirable lipids in plants for bioenergy, industrial, and nutraceutical purposes. These studies have generated a broad array of lipogenic factors for engineering different types of lipids in various plant species. Selecting lipogenic factors that outperform their alternatives when expressed in a target crop is key to optimizing the design of engineering approaches for maximized production of selected lipids. Notably, the optimal lipogenic factors for plant lipid engineering may be sourced outside of the plant kingdom. For instance, the CPS from E. coli and the FAH from a fungal pathogen (C. purpurea) were shown to be more effective in producing CPA or hydroxy FA in plants than the plant-sourced ones (Meesapyodsuk and Qiu, 2008; Yu et al., 2014). In organisms producing high levels of specialty FAs, besides the enzymes responsible for FA synthesis and modification, other lipogenic factors function in glycerolipid assembly and LD formation may have evolved specialized features to accommodate these specialty FAs by depositing them in TAGs and subsequently packaging them in LDs. Therefore, future efforts to enhance specialty lipid accumulation in agronomic crops may be enhanced by introducing multiple specialized lipogenic factors involved in all key steps in lipid synthesis and packaging.
As our understanding of the structural basis of specialized lipogenic factors increases, future research of metabolic engineering will benefit from designing novel lipogenic factors that can outperform naturally occurring ones or produce novel lipids that have not been previously identified in nature based on sequence comparison, computational protein design or directed evolution. Deployment of new computational tools such as AlphaFold to these efforts will likely enhance their success (Mirdita et al., 2022). The feasibility of the former approach has been validated in several studies. In attempts to generate novel DGAT enzymes with improved efficiencies in TAG production, mutant variants of soybean and hazelnut (Corylus americana) DGAT1s produced higher levels of TAGs when expressed in plants compared to the wild-type versions (Roesler et al., 2016; Hatanaka et al., 2022). The structural details of acyl-ACP desaturases guided the generation of a mutant Δ9-acyl-ACP with amino acid substitutions in the substrate binding pocket, which was combined with other lipogenic factors to engineer the specialty ω7 monounsaturated FAs in seed oil (Cahoon and Shanklin, 2000; Nguyen et al., 2010) (Whittle and Shanklin, 2001). Similarly, expression of the native M. charantia FADX in Arabidopsis fad3/fae1 mutant yielded 10% α-eleostearic acid, while the mutagenized McFADX (G111V) or McFADX (G111V/D115E) resulted in a doubling of conjugated FA accumulation to approximately 20% of seed oil. Like the native McFADX, the mutant McFADX (G111V) produced predominantly α-eleostearic acid and little punicic acid, whereas the McFADX (G111V/D115E) double mutant produced nearly equal amounts of α-eleostearic acid and punicic acid (Rawat et al., 2012). In addition, variants of the castor stearoyl-ACP desaturase (T117R/D280K) generated by site-directed mutation can synthesize a novel FA, erythro-9,10-dihydroxystearate, with vicinal hydroxyl groups at C9 and C10 positions (Whittle et al., 2020). Improved mechanistic understanding will facilitate the development of novel improved lipogenic factors via site-directed mutagenesis i.e., rational, structure-based design in combination with computational modeling (Guy et al., 2022) that can be optimized by design-build-test-learn cycles for plant lipid engineering.
Whereas the majority of plant lipid metabolic engineering has focused on seeds, there is growing interest in engineering lipids in plant vegetative tissues because of their high biomass and high capacity for FA synthesis. Most of the FA flux in plant vegetative tissues is for phospholipids to support membrane synthesis, while TAGs serve as an intermediate for FA degradation and are present only at a minimal level in vegetative tissues (Fan et al., 2014). A variety of lipogenic factors and combinations thereof, have been evaluated for their efficacies in enhancing storage lipid accumulation in vegetative tissues of a small number of plant species (Vanhercke et al., 2019b). So far, the most successful approach, characterized as the “push, pull, and protect” strategy, include 1) seed-specific transcription factors such as WRINKLED1 and LEAFY COTYLEDON2 (LEC2) to push the carbon flux toward FA synthesis, 2) acyltransferases such as DGAT and PDAT to pull FAs into the TAG pool, and 3) LD proteins such as oleosin to package TAGs into LDs and protect them from degradation (Vanhercke et al., 2014; Zale et al., 2016; Vanhercke et al., 2017; Alameldin et al., 2017; Liu et al., 2017; Vanhercke et al., 2019a). A major challenge for enhancing TAG accumulation in non-seed tissues is the impairment of growth associated with TAG accumulation, which may result from the accumulation of cytotoxic free FAs, toxic effects of expression of seed-specific transcription factors, and/or the enlarged TAG pool redirecting carbon flux away from other metabolic pathways (Yang et al., 2015; Zale et al., 2016; Vanhercke et al., 2019a; Mitchell et al., 2020). Future efforts to develop improved strategies for mitigated growth impairment and further enhancement of vegetative TAG production will focus on the identification of alternative lipogenic factors that can more efficiently incorporate FAs to TAGs and have reduced negative impacts on plant growth. Additional promising approaches include restricting the expression of lipogenic factors to certain tissues or growth stages using inducible or tissue-specific promoters (Andrianov et al., 2010; Kim et al., 2015c; Liang et al., 2022) , or the expression of factors such as purple acid phosphatase2 (Cai et al., 2022). Despite the challenges, vegetative biomass represents a sustainable and economical platform for lipid accumulation and the success in engineering TAG accumulation therein will facilitate increased yields per unit land area of high-value lipids containing specialty FAs in vegetative tissues by introducing additional specialized lipogenic factors.
The original contributions presented in the study are included in the article/supplementary material. Further inquiries can be directed to the corresponding authors.
X-HY, YC, and JS conceived the study; YC and X-HY drafted the manuscript; JS revised the manuscript. All authors contributed to the article and approved the submitted version.
Funding support was provided by the DOE Center for Advanced Bioenergy and Bioproducts Innovation (the U.S. Department of Energy, Office of Science, Office of Biological and Environmental Research under Award Number DE-SC0018420) and Genomic Science Program (the U.S. Department of Energy, Office of Science, Office of Biological and Environmental Research, grant no. DE-SC0021369). JS was funded in part by the Physical Biosciences Program, within the US Department of Energy (DOE), Division of Chemical Sciences, Geosciences and Biosciences (grant KC0304000).
The authors declare that the research was conducted in the absence of any commercial or financial relationships that could be construed as a potential conflict of interest.
All claims expressed in this article are solely those of the authors and do not necessarily represent those of their affiliated organizations, or those of the publisher, the editors and the reviewers. Any product that may be evaluated in this article, or claim that may be made by its manufacturer, is not guaranteed or endorsed by the publisher.
Any opinions, findings, and conclusions or recommendations expressed in this publication are those of the author(s) and do not necessarily reflect the views of the U.S. Department of Energy.
Abbadi, A., Domergue, F., Bauer, J., Napier, J. A., Welti, R., Zähringer, U., et al. (2004). Biosynthesis of very-long-chain polyunsaturated fatty acids in transgenic oilseeds: constraints on their accumulation. Plant Cell 16, 2734–2748.
Alameldin, H., Izadi-Darbandi, A., Smith, S. A., Balan, V., Jones, A. D., Sticklen, M. (2017). Production of seed-like storage lipids and increase in oil bodies in corn (Maize; zea mays l.) vegetative biomass. Ind. Crops Products. 108, 526–534.
Alkotami, L., Kornacki, C., Campbell, S., McIntosh, G., Wilson, C., Tran, T. N. T., et al. (2021). Expression of a high-activity diacylglycerol acetyltransferase results in enhanced synthesis of acetyl-TAG in camelina seed oil. Plant J. 106, 953–964.
Andrianov, V., Borisjuk, N., Pogrebnyak, N., Brinker, A., Dixon, J., Spitsin, S., et al. (2010). Tobacco as a production platform for biofuel: overexpression of arabidopsis DGAT and LEC2 genes increases accumulation and shifts the composition of lipids in green biomass. Plant Biotechnol. J. 8, 277–287.
Aryal, N., Lu, C. (2018). A phospholipase c-like protein from ricinus communis increases hydroxy fatty acids accumulation in transgenic seeds of camelina sativa. Front. Plant Sci. 9, 1576.
Azeez, A., Parchuri, P., Bates, P. D. (2022). Suppression of physaria fendleri SDP1 increased seed oil and hydroxy fatty acid content while maintaining oil biosynthesis through triacylglycerol remodeling. Front. Plant Sci. 13, 931310.
Aznar-Moreno, J. A., Mukherjee, T., Morley, S. A., Duressa, D., Kambhampati, S., Chu, K. L., et al. (2022). Suppression of SDP1 improves soybean seed composition by increasing oil and reducing undigestible oligosaccharides. Front. Plant Sci. 13, 863254.
Bafor, M., Smith, M. A., Jonsson, L., Stobart, K., Stymne, S. (1993). Biosynthesis of vernoleate (cis-12-epoxyoctadeca-cis-9-enoate) in microsomal preparations from developing endosperm of euphorbia lagascae. Arch. Biochem. Biophys. 303, 145–151.
Bansal, S., Kim, H. J., Na, G., Hamilton, M. E., Cahoon, E. B., Lu, C., et al. (2018). Towards the synthetic design of camelina oil enriched in tailored acetyl-triacylglycerols with medium-chain fatty acids. J. Exp. Bot. 69, 4395–4402.
Bao, X., Katz, S., Pollard, M., Ohlrogge, J. (2002). Carbocyclic fatty acids in plants: biochemical and molecular genetic characterization of cyclopropane fatty acid synthesis of sterculiafoetida. Proc. Natl. Acad. Sci. U.S.A. 99, 7172–7177.
Bao, X., Thelen, J. J., Bonaventure, G., Ohlrogge, J. B. (2003). Characterization of cyclopropane fatty-acid synthase from sterculia foetida. J. Biol. Chem. 278, 12846–12853.
Bates, P. D., Browse, J. (2011). The pathway of triacylglycerol synthesis through phosphatidylcholine in arabidopsis produces a bottleneck for the accumulation of unusual fatty acids in transgenic seeds. Plant J. 68, 387–399.
Bondaruk, M., Johnson, S., Degafu, A., Boora, P., Bilodeau, P., Morris, J., et al. (2007). Expression of a cDNA encoding palmitoyl-acyl carrier protein desaturase from cat’s claw (Doxantha unguis-cati l.) in arabidopsis thaliana and brassica napus leads to accumulation of unusual unsaturated fatty acids and increased stearic acid content in the seed oil. Plant Breed. 126, 186–194.
Bouvier-Navé, P., Benveniste, P., Oelkers, P., Sturley, S. L., Schaller, H. (2000). Expression in yeast and tobacco of plant cDNAs encoding acyl CoA:diacylglycerol acyltransferase. Eur. J. Biochem. 267, 85–96.
Broadwater, J. A., Whittle, E., Shanklin, J. (2002). Desaturation and hydroxylation. residues 148 and 324 of arabidopsis FAD2 in addition to substrate chain length exert a major influence in partitioning of catalytic specificity. J. Biol. Chem. 277, 15613–15620.
Broun, P., Shanklin, J., Whittle, E., Somerville, C. (1998). Catalytic plasticity of fatty acid modification enzymes underlying chemical diversity of plant lipids. Science 282, 1315–1317.
Broun, P., Somerville, C. (1997). Accumulation of ricinoleic lesquerolic and densipolic acids in seeds of transgenic arabidopsis plants that express a fatty acyl hydroxylase cDNA from castor bean. Plant Physiol. 113, 933–942.
Burgal, J., Shockey, J., Lu, C., Dyer, J., Larson, T., Graham, I., et al. (2008). Metabolic engineering of hydroxy fatty acid production in plants: RcDGAT2 drives dramatic increases in ricinoleate levels in seed oil. Plant Biotechnol. J. 6, 819–831.
Cahoon, E. B., Carlson, T. J., Ripp, K. G., Schweiger, B. J., Cook, G. A., Hall, S. E., et al. (1999). Biosynthetic origin of conjugated double bonds: production of fatty acid components of high-value drying oils in transgenic soybean embryos. Proc. Natl. Acad. Sci. U.S.A. 96, 12935–12940.
Cahoon, E. B., Coughlan, S. J., Shanklin, J. (1997). Characterization of a structurally and functionally diverged acyl-acyl carrier protein desaturase from milkweed seed. Plant Mol. Biol. 33, 1105–1110.
Cahoon, E. B., Dietrich, C. R., Meyer, K., Damude, H. G., Dyer, J. M., Kinney, A. J. (2006). Conjugated fatty acids accumulate to high levels in phospholipids of metabolically engineered soybean and arabidopsis seeds. Phytochemistry 67, 1166–1176.
Cahoon, E. B., Ripp, K. G., Hall, S. E., McGonigle, B. (2002). Transgenic production of epoxy fatty acids by expression of a cytochrome P450 enzyme from euphorbia lagascae seed. Plant Physiol. 128, 615–624.
Cahoon, E. B., Shah, S., Shanklin, J., Browse, J. (1998). A determinant of substrate specificity predicted from the acyl-acyl carrier protein desaturase of developing cat’s claw seed. Plant Physiol. 117, 593–598.
Cahoon, E. B., Shanklin, J. (2000). Substrate-dependent mutant complementation to select fatty acid desaturase variants for metabolic engineering of plant seed oils. Proc. Natl. Acad. Sci. U.S.A. 97, 12350–12355.
Cai, Y., Goodman, J. M., Pyc, M., Mullen, R. T., Dyer, J. M., Chapman, K. D. (2015). Arabidopsis SEIPIN proteins modulate triacylglycerol accumulation and influence lipid droplet proliferation. Plant Cell 27, 2616–2636.
Cai, G., Kim, S. C., Li, J., Zhou, Y., Wang, X. (2020). Transcriptional regulation of lipid catabolism during seedling establishment. Mol. Plant 13, 984–1000.
Cai, Y., McClinchie, E., Price, A., Nguyen, T. N., Gidda, S. K., Watt, S. C., et al. (2017). Mouse fat storage-inducing transmembrane protein 2 (FIT2) promotes lipid droplet accumulation in plants. Plant Biotechnol. J. 15, 824–836.
Cai, Y., Whitehead, P., Chappell, J., Chapman, K. D. (2019). Mouse lipogenic proteins promote the co-accumulation of triacylglycerols and sesquiterpenes in plant cells. Planta 250, 79–94.
Cai, Y., Zhai, Z., Keereetaweep, J., Liu, H., Shi, H., Schwender, J., et al (2022). Purple acid phosphatase 2 increases fatty acid synthesis and offsets yield drag associated with triacylglycerol hyperaccumulation in vegetative tissues. New Phytologist (CABBI and BES). doi: 10.1111/nph.18392
Carlsson, A. S., Yilmaz, J. L., Green, A. G., Stymne, S., Hofvander, P. (2011). Replacing fossil oil with fresh oil - with what and for what? Eur. J. Lipid Sci. Technol. 113, 812–831.
Chapman, K. D., Dyer, J. M., Mullen, R. T. (2012). Biogenesis and functions of lipid droplets in plants: Thematic review series: Lipid droplet synthesis and metabolism: from yeast to man. J. Lipid Res. 53, 215–226.
Chen, G. Q., Johnson, K., Nazarenus, T. J., Ponciano, G., Morales, E., Cahoon, E. B. (2021). Genetic engineering of lesquerella with increased ricinoleic acid content in seed oil. Plants (Basel). 10 (6), 1093.
Dahlqvist, A., Stahl, U., Lenman, M., Banas, A., Lee, M., Sandager, L., et al. (2000). Phospholipid:diacylglycerol acyltransferase: an enzyme that catalyzes the acyl-CoA-independent formation of triacylglycerol in yeast and plants. Proc. Natl. Acad. Sci. U.S.A. 97, 6487–6492.
Dehesh, K., Edwards, P., Fillatti, J., Slabaugh, M., Byrne, J. (1998). KAS IV: a 3-ketoacyl-ACP synthase from cuphea sp. is. medium. chain. specific. condensing. enzyme. Plant J. 15, 383–390.
Dehesh, K., Jones, A., Knutzon, D. S., Voelker, T. A. (1996). Production of high levels of 8:0 and 10:0 fatty acids in transgenic canola by overexpression of ch FatB2 a thioesterase cDNA from cuphea hookeriana. Plant J. 9, 167–172.
Deruyffelaere, C., Purkrtova, Z., Bouchez, I., Collet, B., Cacas, J. L., Chardot, T., et al. (2018). PUX10 is a CDC48A adaptor protein that regulates the extraction of ubiquitinated oleosins from seed lipid droplets in arabidopsis. Plant Cell 30, 2116–2136.
Durrett, T. P., McClosky, D. D., Tumaney, A. W., Elzinga, D. A., Ohlrogge, J., Pollard, M. (2010). A distinct DGAT with sn-3 acetyltransferase activity that synthesizes unusual reduced-viscosity oils in euonymus and transgenic seeds. Proc. Natl. Acad. Sci. U.S.A. 107, 9464–9469.
Dyer, J. M., Chapital, D. C., Kuan, J. C., Mullen, R. T., Turner, C., McKeon, T. A., et al. (2002). Molecular analysis of a bifunctional fatty acid conjugase/desaturase from tung. implications for the evolution of plant fatty acid diversity. Plant Physiol. 130, 2027–2038.
Dyer, J. M., Stymne, S., Green, A. G., Carlsson, A. S. (2008). High-value oils from plants. Plant J. 54, 640–655.
Eastmond, P. J. (2006). SUGAR-DEPENDENT1 encodes a patatin domain triacylglycerol lipase that initiates storage oil breakdown in germinating arabidopsis seeds. Plant Cell 18, 665–675.
Eastmond, P. J., Graham, I. A. (2001). Re-examining the role of the glyoxylate cycle in oilseeds. Trends Plant Sci. 6, 72–78.
Eccleston, V. S., Cranmer, A. M., Voelker, T. A., Ohlrogge, J. B. (1996). Medium-chain fatty acid biosynthesis and utilization in brassica napus plants expressing lauroyl-acyl carrier protein thioesterase. Planta 198, 46–53.
Fan, J., Yan, C., Roston, R., Shanklin, J., Xu, C. (2014). Arabidopsis lipins PDAT1 acyltransferase and SDP1 triacylglycerol lipase synergistically direct fatty acids toward β-oxidation thereby maintaining membrane lipid homeostasis. Plant Cell 26, 4119–4134.
Gao, Y., Sun, Y., Gao, H., Chen, Y., Wang, X., Xue, J., et al. (2021). Ectopic overexpression of a type-II DGAT (CeDGAT2-2) derived from oil-rich tuber of cyperus esculentus enhances accumulation of oil and oleic acid in tobacco leaves. Biotechnol. Biofuels 14, 76.
Guo, X., Fan, C., Chen, Y., Wang, J., Yin, W., Wang, R. R., et al. (2017). Identification and characterization of an efficient acyl-CoA: diacylglycerol acyltransferase 1 (DGAT1) gene from the microalga chlorella ellipsoidea. BMC Plant Biol. 17, 48.
Guy, J. E., Cai, Y., Baer, M. D., Whittle, E., Chai, J., Yu, X. H., et al. (2022). Regioselectivity mechanism of the thunbergia alata Δ6-16:0-acyl carrier protein desaturase. Plant Physiol. 188, 1537–1549.
Han, L., Silvestre, S., Sayanova, O., Haslam, R. P., Napier, J. A. (2022). Using field evaluation and systematic iteration to rationalize the accumulation of omega-3 long-chain polyunsaturated fatty acids in transgenic camelina sativa. Plant Biotechnol. J 20 (9), 1833–1852.
Han, L., Usher, S., Sandgrind, S., Hassall, K., Sayanova, O., Michaelson, L. V., et al. (2020). High level accumulation of EPA and DHA in field-grown transgenic camelina - a multi-territory evaluation of TAG accumulation and heterogeneity. Plant Biotechnol. J. 18, 2280–2291.
Hatanaka, T., Shimizu, R., Hildebrand, D. (2004). Expression of a stokesia laevis epoxygenase gene. Phytochemistry 65, 2189–2196.
Hatanaka, T., Tomita, Y., Matsuoka, D., Sasayama, D., Fukayama, H., Azuma, T., et al. (2022). Different acyl-CoA:diacylglycerol acyltransferases vary widely in function and a targeted amino acid substitution enhances oil accumulation. J. Exp. Bot. 73, 3030–3043.
Horn, P. J., Liu, J., Cocuron, J. C., McGlew, K., Thrower, N. A., Larson, M., et al. (2016). Identification of multiple lipid genes with modifications in expression and sequence associated with the evolution of hydroxy fatty acid accumulation in physaria fendleri. Plant J. 86, 322–348.
Hu, Z., Ren, Z., Lu, C. (2012). The phosphatidylcholine diacylglycerol cholinephosphotransferase is required for efficient hydroxy fatty acid accumulation in transgenic arabidopsis. Plant Physiol. 158, 1944–1954.
Iskandarov, U., Silva, J. E., Kim, H. J., Andersson, M., Cahoon, R. E., Mockaitis, K., et al. (2017). A specialized diacylglycerol acyltransferase contributes to the extreme medium-chain fatty acid content of. Plant Physiol. 174, 97–109.
James, C. N., Horn, P. J., Case, C. R., Gidda, S. K., Zhang, D., Mullen, R. T., et al. (2010). Disruption of the arabidopsis CGI-58 homologue produces chanarin-dorfman-like lipid droplet accumulation in plants. Proc. Natl. Acad. Sci. U.S.A. 107, 17833–17838.
Kalinger, R. S., Pulsifer, I. P., Hepworth, S. R., Rowland, O. (2020). Fatty acyl synthetases and thioesterases in plant lipid metabolism: Diverse functions and biotechnological applications. Lipids 55, 435–455.
Kalinger, R. S., Williams, D., Ahmadi Pirshahid, A., Pulsifer, I. P., Rowland, O. (2021). Production of C6-C14 medium-chain fatty acids in seeds and leaves via overexpression of single hotdog-fold acyl-lipid thioesterases. Lipids 56, 327–344.
Kallio, P., Pásztor, A., Akhtar, M. K., Jones, P. R. (2014). Renewable jet fuel. Curr. Opin. Biotechnol. 26, 50–55.
Kanai, M., Yamada, T., Hayashi, M., Mano, S., Nishimura, M. (2019). Soybean (Glycine max l.) triacylglycerol lipase GmSDP1 regulates the quality and quantity of seed oil. Sci. Rep. 9, 8924.
Keereetaweep, J., Liu, H., Zhai, Z., Shanklin, J. (2018). Biotin attachment domain-containing proteins irreversibly inhibit acetyl CoA carboxylase. Plant Physiol. 177, 208–215.
Kelly, A. A., Shaw, E., Powers, S. J., Kurup, S., Eastmond, P. J. (2013a). Suppression of the SUGAR-DEPENDENT1 triacylglycerol lipase family during seed development enhances oil yield in oilseed rape (Brassica napus l.). Plant Biotechnol. J. 11, 355–361.
Kelly, A. A., van Erp, H., Quettier, A. L., Shaw, E., Menard, G., Kurup, S., et al. (2013b). The sugar-dependent1 lipase limits triacylglycerol accumulation in vegetative tissues of arabidopsis. Plant Physiol. 162, 1282–1289.
Kim, H. J., Silva, J. E., Iskandarov, U., Andersson, M., Cahoon, R. E., Mockaitis, K., et al. (2015a). Structurally divergent lysophosphatidic acid acyltransferases with high selectivity for saturated medium chain fatty acids from cuphea seeds. Plant J. 84, 1021–1033.
Kim, H. J., Silva, J. E., Vu, H. S., Mockaitis, K., Nam, J. W., Cahoon, E. B. (2015b). Toward production of jet fuel functionality in oilseeds: identification of FatB acyl-acyl carrier protein thioesterases and evaluation of combinatorial expression strategies in camelina seeds. J. Exp. Bot. 66, 4251–4265.
Kim, H. U., Lee, K. R., Jung, S. J., Shin, H. A., Go, Y. S., Suh, M. C., et al. (2015c). Senescence-inducible LEC2 enhances triacylglycerol accumulation in leaves without negatively affecting plant growth. Plant Biotechnol. J. 13, 1346–1359.
Kim, M. J., Yang, S. W., Mao, H. Z., Veena, S. P., Yin, J. L., Chua, N. H. (2014). Gene silencing of sugar-dependent 1 (JcSDP1) encoding a patatin-domain triacylglycerol lipase enhances seed oil accumulation in jatropha curcas. Biotechnol. Biofuels 7, 36.
Knutzon, D. S., Hayes, T. R., Wyrick, A., Xiong, H., Maelor Davies, H., Voelker, T. A. (1999). Lysophosphatidic acid acyltransferase from coconut endosperm mediates the insertion of laurate at the sn-2 position of triacylglycerols in lauric rapeseed oil and can increase total laurate levels. Plant Physiol. 120, 739–746.
Kretzschmar, F. K., Mengel, L. A., Müller, A. O., Schmitt, K., Blersch, K. F., Valerius, O., et al. (2018). PUX10 is a lipid droplet-localized scaffold protein that interacts with CELL DIVISION CYCLE48 and is involved in the degradation of lipid droplet proteins. Plant Cell 30, 2137–2160.
Lee, J. S., Takai, J., Takahasi, K., Endo, Y., Fujimoto, K., Koike, S., et al. (2002). Effect of dietary tung oil on the growth and lipid metabolism of laying hens. J. Nutr. Sci. Vitaminol. (Tokyo). 48, 142–148.
Liang, Y., Yu, X-H., Anaokar, S., Shi, H., Dahl, W.S., Cai, Y., et al (2002). Engineering triacylglycerol accumulation in duckweed (Lemna japonica). Plant Biotechnology Journal Plant Biotechnol J. doi: 10.1111/pbi.13943
Li-Beisson, Y., Shorrosh, B., Beisson, F., Andersson, M. X., Arondel, V., Bates, P. D., et al. (2013). Acyl-lipid metabolism. Arabidopsis. Book. 11, e0161.
Liu, Q., Guo, Q., Akbar, S., Zhi, Y., El Tahchy, A., Mitchell, M., et al. (2017). Genetic enhancement of oil content in potato tuber (Solanum tuberosum l.) through an integrated metabolic engineering strategy. Plant Biotechnol. J. 15, 56–67.
Liu, L., Hammond, E. G., Nikolau, B. J. (1998). In vivo studies of the biosynthesis of vernolic acid in the seed of vernonia galamensis. Lipids 33, 1217–1221.
Li, R., Yu, K., Hatanaka, T., Hildebrand, D. F. (2010). Vernonia DGATs increase accumulation of epoxy fatty acids in oil. Plant Biotechnol. J. 8, 184–195.
Lu, C., Fulda, M., Wallis, J. G., Browse, J. (2006). A high-throughput screen for genes from castor that boost hydroxy fatty acid accumulation in seed oils of transgenic arabidopsis. Plant J. 45, 847–856.
Lunn, D., Wallis, J. G., Browse, J. (2018). Overexpression of Seipin1 increases oil in hydroxy fatty acid-accumulating seeds. Plant Cell Physiol. 59, 205–214.
Lunn, D., Wallis, J. G., Browse, J. (2019). Tri-Hydroxy-Triacylglycerol is efficiently produced by position-specific castor acyltransferases. Plant Physiol. 179, 1050–1063.
Lunn, D., Wallis, J. G., Browse, J. (2022). A multigene approach secures hydroxy fatty acid production in arabidopsis. J. Exp. Bot. 73, 2875–2888.
Lu, C., Xin, Z., Ren, Z., Miquel, M., Browse, J. (2009). An enzyme regulating triacylglycerol composition is encoded by the ROD1 gene of arabidopsis. Proc. Natl. Acad. Sci. U.S.A. 106, 18837–18842.
Mayer, K. M., Shanklin, J. (2005). A structural model of the plant acyl-acyl carrier protein thioesterase FatB comprises two helix/4-stranded sheet domains the n-terminal domain containing residues that affect specificity and the c-terminal domain containing catalytic residues. J. Biol. Chem. 280, 3621–3627.
Meesapyodsuk, D., Qiu, X. (2008). An oleate hydroxylase from the fungus claviceps purpurea: cloning functional analysis and expression in arabidopsis. Plant Physiol. 147, 1325–1333.
Mirdita, M., Schütze, K., Moriwaki, Y., Heo, L., Ovchinnikov, S., Steinegger, M. (2022). ColabFold: making protein folding accessible to all. Nat. Methods 19, 679–682.
Mitchell, M. C., Pritchard, J., Okada, S., Zhang, J., Venables, I., Vanhercke, T., et al. (2020). Increasing growth and yield by altering carbon metabolism in a transgenic leaf oil crop. Plant Biotechnol. J 18 (10), 2042–2052.
Napier, J. A., Haslam, R. P., Tsalavouta, M., Sayanova, O. (2019). The challenges of delivering genetically modified crops with nutritional enhancement traits. Nat. Plants 5, 563–567.
Nguyen, H. T., Mishra, G., Whittle, E., Pidkowich, M. S., Bevan, S. A., Merlo, A. O., et al. (2010). Metabolic engineering of seeds can achieve levels of omega-7 fatty acids comparable with the highest levels found in natural plant sources. Plant Physiol. 154, 1897–1904.
Nguyen, H. T., Park, H., Koster, K. L., Cahoon, R. E., Shanklin, J., Clemente, T. E., et al. (2015). Redirection of metabolic flux for high levels of omega-7 monounsaturated fatty acid accumulation in camelina seeds. Plant Biotechnol. J. 13, 38–50.
Ohlrogge, J., Chapman, K. (2011). The seeds of green energy: expanding the contribution of plant oils as biofuels. Biochemist. 33, 34–38.
Okada, S., Taylor, M., Zhou, X. R., Naim, F., Marshall, D., Blanksby, S. J., et al. (2020). Producing cyclopropane fatty acid in plant leafy biomass. Front. Plant Sci. 11, 30.
Park, S., Gidda, S. K., James, C. N., Horn, P. J., Khuu, N., Seay, D. C., et al. (2013). The α/β hydrolase CGI-58 and peroxisomal transport protein PXA1 coregulate lipid homeostasis and signaling in arabidopsis. Plant Cell 25, 1726–1739.
Park, M. E., Lee, K. R., Chen, G. Q., Kim, H. U. (2022). Enhanced production of hydroxy fatty acids in arabidopsis seed through modification of multiple gene expression. Biotechnol. Biofuels Bioprod. 15, 66.
Park, K., Sanjaya, S. A., Quach, T., Cahoon, E. B. (2021). Toward sustainable production of value-added bioenergy and industrial oils in oilseed and biomass feedstocks. GCB. Bioenergy 13, 1610–1623.
Petrie, J. R., Shrestha, P., Belide, S., Kennedy, Y., Lester, G., Liu, Q., et al. (2014). Metabolic engineering camelina sativa with fish oil-like levels of DHA. PloS One 9, e85061.
Petrie, J. R., Shrestha, P., Liu, Q., Mansour, M. P., Wood, C. C., Zhou, X. R., et al. (2010). Rapid expression of transgenes driven by seed-specific constructs in leaf tissue: DHA production. Plant Methods 6, 8.
Petrie, J. R., Shrestha, P., Zhou, X. R., Mansour, M. P., Liu, Q., Belide, S., et al. (2012). Metabolic engineering plant seeds with fish oil-like levels of DHA. PloS One 7, e49165.
Price, A. M., Doner, N. M., Gidda, S. K., Jambunathan, S., James, C. N., Schami, A., et al. (2020). Mouse fat-specific protein 27 (FSP27) expressed in plant cells localizes to lipid droplets and promotes lipid droplet accumulation and fusion. Biochimie 169, 41–53.
Pyc, M., Cai, Y., Greer, M. S., Yurchenko, O., Chapman, K. D., Dyer, J. M., et al. (2017). Turning over a new leaf in lipid droplet biology. Trends Plant Sci. 22, 596–609.
Qi, B., Fraser, T., Mugford, S., Dobson, G., Sayanova, O., Butler, J., et al. (2004). Production of very long chain polyunsaturated omega-3 and omega-6 fatty acids in plants. Nat. Biotechnol. 22, 739–745.
Rawat, R., Yu, X. H., Sweet, M., Shanklin, J. (2012). Conjugated fatty acid synthesis: residues 111 and 115 influence product partitioning of momordica charantia conjugase. J. Biol. Chem. 287, 16230–16237.
Roesler, K., Shen, B., Bermudez, E., Li, C., Hunt, J., Damude, H. G., et al. (2016). An improved variant of soybean type 1 diacylglycerol acyltransferase increases the oil content and decreases the soluble carbohydrate content of soybeans. Plant Physiol. 171, 878–893.
Salie, M. J., Zhang, N., Lancikova, V., Xu, D., Thelen, J. J. (2016). A family of negative regulators targets the committed step of de Novo fatty acid biosynthesis. Plant Cell 28, 2312–2325.
Shanklin, J., Cahoon, E. B. (1998). DESATURATION AND RELATED MODIFICATIONS OF FATTY ACIDS1. Annu. Rev. Plant Physiol. Plant Mol. Biol. 49, 611–641.
Shockey, J., Lager, I., Stymne, S., Kotapati, H. K., Sheffield, J., Mason, C., et al. (2019). Specialized lysophosphatidic acid acyltransferases contribute to unusual fatty acid accumulation in exotic euphorbiaceae seed oils. Planta 249, 1285–1299.
Singh, R., Arora, A., Singh, V. (2021). Biodiesel from oil produced in vegetative tissues of biomass - a review. Bioresour. Technol. 326, 124772.
Singh, S., Thomaeus, S., Lee, M., Stymne, S., Green, A. (2001). Transgenic expression of a delta 12-epoxygenase gene in arabidopsis seeds inhibits accumulation of linoleic acid. Planta 212, 872–879.
Smith, M. A., Moon, H., Chowrira, G., Kunst, L. (2003). Heterologous expression of a fatty acid hydroxylase gene in developing seeds of arabidopsis thaliana. Planta 217, 507–516.
Sun, Y., Liu, B., Xue, J., Wang, X., Cui, H., Li, R., et al. (2022). Critical metabolic pathways and genes cooperate for epoxy fatty acid-enriched oil production in developing seeds of vernonia galamensis an industrial oleaginous plant. Biotechnol. Biofuels Bioprod. 15, 21.
Tjellström, H., Strawsine, M., Silva, J., Cahoon, E. B., Ohlrogge, J. B. (2013). Disruption of plastid acyl:acyl carrier protein synthetases increases medium chain fatty acid accumulation in seeds of transgenic arabidopsis. FEBS Lett. 587, 936–942.
Tocher, D. R., Betancor, M. B., Sprague, M., Olsen, R. E., Napier, J. A. (2019). Omega-3 long-chain polyunsaturated fatty acids EPA and DHA: Bridging the gap between supply and demand. Nutrients 11 (1), 89.
van de Loo, F. J., Broun, P., Turner, S., Somerville, C. (1995). An oleate 12-hydroxylase from ricinus communis l. is. Fatty acyl. desaturase. homolog. Proc. Natl. Acad. Sci. U.S.A. 92, 6743–6747.
van Erp, H., Bates, P. D., Burgal, J., Shockey, J., Browse, J. (2011). Castor phospholipid:diacylglycerol acyltransferase facilitates efficient metabolism of hydroxy fatty acids in transgenic arabidopsis. Plant Physiol. 155, 683–693.
van Erp, H., Kelly, A. A., Menard, G., Eastmond, P. J. (2014). Multigene engineering of triacylglycerol metabolism boosts seed oil content in arabidopsis. Plant Physiol. 165, 30–36.
Vanhercke, T., Belide, S., Taylor, M. C., El Tahchy, A., Okada, S., Rolland, V., et al. (2019a). Up-regulation of lipid biosynthesis increases the oil content in leaves of sorghum bicolor. Plant Biotechnol. J. 17, 220–232.
Vanhercke, T., Divi, U. K., El Tahchy, A., Liu, Q., Mitchell, M., Taylor, M. C., et al. (2017). Step changes in leaf oil accumulation via iterative metabolic engineering. Metab. Eng. 39, 237–246.
Vanhercke, T., Dyer, J. M., Mullen, R. T., Kilaru, A., Rahman, M. M., Petrie, J. R., et al. (2019b). Metabolic engineering for enhanced oil in biomass. Prog. Lipid Res. 74, 103–129.
Vanhercke, T., El Tahchy, A., Liu, Q., Zhou, X. R., Shrestha, P., Divi, U. K., et al. (2014). Metabolic engineering of biomass for high energy density: oilseed-like triacylglycerol yields from plant leaves. Plant Biotechnol. J. 12, 231–239.
Voelker, T. A., Hayes, T. R., Cranmer, A. M., Turner, J. C., Davies, H. M. (1996). Genetic engineering of a quantitative trait: metabolic and genetic parameters influencing the accumulation of laurate in rapeseed. Plant J. 9, 229–241.
Whittle, E. J., Cai, Y., Keereetaweep, J., Chai, J., Buist, P. H., Shanklin, J. (2020). Castor stearoyl-ACP desaturase can synthesize a vicinal diol by dioxygenase chemistry. Plant Physiol. 182, 730–738.
Whittle, E., Shanklin, J. (2001). Engineering delta 9-16:0-acyl carrier protein (ACP) desaturase specificity based on combinatorial saturation mutagenesis and logical redesign of the castor delta 9-18:0-ACP desaturase. J. Biol. Chem. 276, 21500–21505.
Winichayakul, S., Scott, R. W., Roldan, M., Hatier, J. H., Livingston, S., Cookson, R., et al. (2013). In vivo packaging of triacylglycerols enhances arabidopsis leaf biomass and energy density. Plant Physiol. 162, 626–639.
Wood, C. C., Petrie, J. R., Shrestha, P., Mansour, M. P., Nichols, P. D., Green, A. G., et al. (2009). A leaf-based assay using interchangeable design principles to rapidly assemble multistep recombinant pathways. Plant Biotechnol. J. 7, 914–924.
Wu, G., Truksa, M., Datla, N., Vrinten, P., Bauer, J., Zank, T., et al. (2005). Stepwise engineering to produce high yields of very long-chain polyunsaturated fatty acids in plants. Nat. Biotechnol. 23, 1013–1017.
Xu, Y., Mietkiewska, E., Shah, S., Weselake, R. J., Chen, G. (2020). Punicic acid production in brassica napus. Metab. Eng. 62, 20–29.
Xu, C., Shanklin, J. (2016). Triacylglycerol metabolism function and accumulation in plant vegetative tissues. Annu. Rev. Plant Biol. 67, 179–206.
Yang, Y., Munz, J., Cass, C., Zienkiewicz, A., Kong, Q., Ma, W., et al. (2015). Ectopic expression of WRINKLED1 affects fatty acid homeostasis in brachypodium distachyon vegetative tissues. Plant Physiol. 169, 1836–1847.
Yuan, G. F., Chen, X. E., Li, D. (2014). Conjugated linolenic acids and their bioactivities: a review. Food Funct. 5, 1360–1368.
Yu, X. H., Cahoon, R. E., Horn, P. J., Shi, H., Prakash, R. R., Cai, Y., et al. (2018). Identification of bottlenecks in the accumulation of cyclic fatty acids in camelina seed oil. Plant Biotechnol. J. 16, 926–938.
Yu, X. H., Cai, Y., Chai, J., Schwender, J., Shanklin, J. (2019). Expression of a lychee PHOSPHATIDYLCHOLINE : DIACYLGLYCEROL CHOLINEPHOSPHOTRANSFERASE with an escherichia coli CYCLOPROPANE SYNTHASE enhances cyclopropane fatty acid accumulation in camelina seeds. Plant Physiol. 180, 1351–1361.
Yu, X. H., Cai, Y., Keereetaweep, J., Wei, K., Chai, J., Deng, E., et al. (2021). Biotin attachment domain-containing proteins mediate hydroxy fatty acid-dependent inhibition of acetyl CoA carboxylase. Plant Physiol. 185, 892–901.
Yu, X. H., Prakash, R. R., Sweet, M., Shanklin, J. (2014). Coexpressing escherichia coli cyclopropane synthase with sterculia foetida lysophosphatidic acid acyltransferase enhances cyclopropane fatty acid accumulation. Plant Physiol. 164, 455–465.
Yu, X. H., Rawat, R., Shanklin, J. (2011). Characterization and analysis of the cotton cyclopropane fatty acid synthase family and their contribution to cyclopropane fatty acid synthesis. BMC Plant Biol. 11, 97.
Yurchenko, O., Shockey, J. M., Gidda, S. K., Silver, M. I., Chapman, K. D., Mullen, R. T., et al. (2017). Engineering the production of conjugated fatty acids in arabidopsis thaliana leaves. Plant Biotechnol. J. 15, 1010–1023.
Zale, J., Jung, J. H., Kim, J. Y., Pathak, B., Karan, R., Liu, H., et al. (2016). Metabolic engineering of sugarcane to accumulate energy-dense triacylglycerols in vegetative biomass. Plant Biotechnol. J. 14, 661–669.
Zhou, X. R., Singh, S. P., Green, A. G. (2013). Characterisation of the FAD2 gene family from hiptage benghalensis: a ricinoleic acid accumulating plant. Phytochemistry 92, 42–48.
Zhou, X. R., Singh, S., Liu, Q., Green, A. (2006). Combined transgenic expression of Δ12-desaturase and Δ12-epoxygenase in high linoleic acid seeds leads to increased accumulation of vernolic acid. Funct. Plant Biol. 33, 585–592.
Zienkiewicz, K., Zienkiewicz, A., Poliner, E., Du, Z. Y., Vollheyde, K., Herrfurth, C., et al. (2017). Nannochloropsis a rich source of diacylglycerol acyltransferases for engineering of triacylglycerol content in different hosts. Biotechnol. Biofuels 10, 8.
Keywords: lipid engineering, fatty acid, triacylglycerol, lipid droplet, specialty fatty acid, specialty lipid, lipogenic factor
Citation: Cai Y, Yu X-H and Shanklin J (2022) A toolkit for plant lipid engineering: Surveying the efficacies of lipogenic factors for accumulating specialty lipids. Front. Plant Sci. 13:1064176. doi: 10.3389/fpls.2022.1064176
Received: 07 October 2022; Accepted: 28 November 2022;
Published: 14 December 2022.
Edited by:
Maria F. Drincovich, Centro de Estudios Fotosintéticos y Bioquímicos (CEFOBI), ArgentinaReviewed by:
Agnieszka Zienkiewicz, Nicolaus Copernicus University in Toruń, PolandCopyright © 2022 Cai, Yu and Shanklin. This is an open-access article distributed under the terms of the Creative Commons Attribution License (CC BY). The use, distribution or reproduction in other forums is permitted, provided the original author(s) and the copyright owner(s) are credited and that the original publication in this journal is cited, in accordance with accepted academic practice. No use, distribution or reproduction is permitted which does not comply with these terms.
*Correspondence: Xiao-Hong Yu, eGh5dUBibmwuZ292; John Shanklin, c2hhbmtsaW5AYm5sLmdvdg==
Disclaimer: All claims expressed in this article are solely those of the authors and do not necessarily represent those of their affiliated organizations, or those of the publisher, the editors and the reviewers. Any product that may be evaluated in this article or claim that may be made by its manufacturer is not guaranteed or endorsed by the publisher.
Research integrity at Frontiers
Learn more about the work of our research integrity team to safeguard the quality of each article we publish.