- 1State Key Laboratory of Subtropical Silviculture, Zhejiang Agriculture and Forestry (A&F) University, Hangzhou, China
- 2Zhejiang Provincial Key Laboratory of Forest Aromatic Plants-based Healthcare Functions, Zhejiang Agriculture and Forestry (A&F) University, Hangzhou, China
- 3College of Life Sciences, Nanjing Agricultural University, Nanjing, Jiangsu, China
- 4Departments of Public Health Sciences and Statistics, Center for Statistical Genetics, Pennsylvania State University, Hershey, PA, United States
Auxin is essential for regulating plant growth and development as well as the response of plants to abiotic stresses. AUX/LAX proteins are auxin influx transporters belonging to the amino acid permease family of proton-driven transporters, and are involved in the transport of indole-3-acetic acid (IAA). However, how AUX/LAX genes respond to abiotic stresses in Chinese hickory is less studied. For the first time identification, structural characteristics as well as gene expression analysis of the AUX/LAX gene family in Chinese hickory were conducted by using techniques of gene cloning and real-time fluorescent quantitative PCR. Eight CcAUX/LAXs were identified in Chinese hickory, all of which had the conserved structural characteristics of AUX/LAXs. CcAUX/LAXs were most closely related to their homologous proteins in Populus trichocarpa , which was in consistence with their common taxonomic character of woody trees. CcAUX/LAXs exhibited different expression profiles in different tissues, indicating their varying roles during growth and development. A number of light-, hormone-, and abiotic stress responsive cis-acting regulatory elements were detected on the promoters of CcAUX/LAX genes. CcAUX/LAX genes responded differently to drought and salt stress treatments to varying degrees. Furthermore, CcAUX/LAX genes exhibited complex expression changes during Chinese hickory grafting. These findings not only provide a valuable resource for further functional validation of CcAUX/LAXs, but also contribute to a better understanding of their potential regulatory functions during grafting and abiotic stress treatments in Chinese hickory.
1. Introduction
Plants experience varying degrees of biotic and abiotic stresses throughout their life cycle in the natural environment, with abiotic stresses, including drought, high salinity, high temperature, low temperature, waterlogging, and so on, being the most common environmental factors in nature, all of which affect plant natural growth and development and can even result in plant damage or death (Ramegowda and Senthil-Kumar, 2015; Pandey et al., 2017; Samadi et al., 2021; Aliyari Rad et al., 2022; Dehghanian et al., 2022). As one of the most important plant hormones, auxin affects different aspects of plant growth and development through the establishment of auxin concentration gradient which is determined by the combined actions of its synthesis, metabolism, transportation, and signal transduction. Previous studies have found that auxin regulates the normal growth of plants, including apical dominance, phototropism and geotropism, inflorescence and frond development, lateral and adventitious root formation, vascular tissue differentiation, and fruit maturity (Korver et al., 2018; Gomes and Scortecci, 2021). Furthermore, auxin is essential for the temporal coordination of plants’ responses to abiotic stresses and many environmental stress responses are dependent on the dynamic distribution of auxin in different plant tissues (Peleg and Blumwald, 2011; Rahman, 2013; Min et al., 2014; Hadi et al., 2021).
Auxin is synthesized in active parts of plants, such as shoot apex, terminal buds, young leaves, developing seeds, meristems of main root tips, and developing lateral roots (Matthes et al., 2019), and then transported to distal target tissues either through the bulk flow in stem vascular tissues in a non-polar free diffusion way or through active transport mediated by transporters (Wulf et al., 2019). Auxin transporters are considered to be involved in the active transport process of auxin. Auxin transporters mainly include AUXIN RESISTENT1/LIKE AUX1 (AUX/LAX) influx carriers, PIN-FORMED (PIN) efflux carriers, and ATP binding cassette B/P- glycoprotein/Multi-drug-resistance (ABCB/MDR/PGP) efflux/condition carriers (Hammes et al., 2022). PIN proteins are the primary auxin efflux carriers in plants, transporting auxin between or within cells (Barbosa et al., 2018; Ung et al., 2022). ABCB proteins belong to the ABC transporter protein supergene family, and the majority of which have been identified as auxin transporters that functions in the long-distance transport of auxin and the outflow of auxin in the apical tissue (Cho and Cho, 2013; Mellor et al., 2022). AUX/LAXs are multimembrane-spanning proteins involve in the uptake of auxin and the flow of auxin between cells. AUX/LAXs are related to amino acid transporters and constitute a plant-specific subclass within the amino acid/auxin permease superfamily (Péret et al., 2012; Robert et al., 2015). Numerous studies are currently being conducted on the AUX/LAX gene family, and the functions of certain members in some species have been identified (Swarup et al., 2008; Péret et al., 2012; Chai et al., 2016).
In Arabidopsis, the AUX/LAX influx carriers include four members, AUX1, LAX1, LAX2 and LAX3. Although the AUX/LAX family is highly conservative in sequences and biochemical functions, however, each member exhibits distinct temporal and spatial expression patterns and works independently or cooperatively in a variety of developmental events (Péret et al., 2012; Swarup and Péret, 2012). AUX1 was the first discovered member of the AUX/LAX family that played an important role in the root gravity response, mutation of AUX1 result in a significant loss of root geotropism (Bennett et al., 1996). Moreover, AUX1 was found asymmetrically in the plasma membrane of root phloem cells, where it promoted the transport of auxin from the root to the top and bottom, then advance root hair growth (Swarup et al., 2001; Marchant et al., 2002; Kleine-Vehn et al., 2006). In wild cherry (Prunus avium L.), PaLAX1 accelerates the rate of auxin uptake into cells and alters the distribution of free endogenous auxin (Hoyerová et al., 2008). In Arabidopsis, AtLAX3 and AtAUX1 coordinate lateral root development by regulating the emergence and initiation of lateral root primordia (Marchant et al., 2002; Swarup et al., 2008). LAX2 was involved in leaf vein formation and xylem development (Péret et al., 2012). The AUX/LAX gene family influences phyllotactic patterns and is required for the establishment of embryonic root cell organization and plant embryogenesis in Arabidopsis (Bainbridge et al., 2008; Ugartechea-Chirino et al., 2010; Haskovec et al., 2019). In addition to the aforementioned functions, the auxin influx carrier is involved in processes such as seed germination, adventitious roots, and female gametophyte development (Panoli et al., 2015; Chai et al., 2016; Lee et al., 2019). Furthermore, auxin transport may function in the interaction of symbiosis and pathogenic plant microorganisms, influencing auxin penetration in host plant cells (Lee et al., 2011).
There is increasing evidence that AUX/LAX auxin transporters function in plants’ adaptation to changing environmental conditions. In Arabidopsis, an increase in the expression of AUX1 and auxin biosynthesis-related genes following ethylene treatment led to an increase in auxin accumulation, thereby regulating the root growth inhibition mediated by alkali stress (Li et al., 2015). AtAUX1 played an important role in arsenic-induced oxidative stress tolerance (Krishnamurthy and Rathinasabapathi, 2013). OsPIN3 was involved in auxin transport and drought stress response in rice, indicating that the polar auxin transport pathway is involved in regulating plant response to adversity stress (Zhang et al., 2012). Furthermore, drought stress altered the transcriptional expression profiles of AUX/LAX genes. It was found that five OsAUX/LAXs responded to drought and salinity, with most OsLAXs down-regulated and three up-regulated in some rice tissues and under drought, salt, and abscisic acid (ABA) treatments (Chai and Subudhi, 2016). Due to drought stress, in maize (Zea mays L.), most ZmLAX genes were up-regulated in stems but down-regulated in roots, implying dynamic growth hormone transport between stems and roots under abiotic stress (Yue et al., 2015). In soybean (Glycine max L.), most GmLAXs were down-regulated by drought and dehydration, while only six GmLAXs were down-regulated by salt stress (Chai et al., 2016). In Boehmeria nivea L., the BnAUX/LAXs responded to drought stress at the transcriptional level, with differential expression in leaves and roots (Bao et al., 2019). However, sorghum (Sorghum bicolor L.) LAXs exhibited irregular expression patterns under drought and salt stress (Chai et al., 2016). The above evidences reveal the responses of AUX/LAXs to abiotic stress, which provides a foundation for further investigation of the function of AUX/LAX genes.
Chinese hickory (Carya cathayensis Sarg.) is a commercially important tree species that produces nuts with a high nutritional value and has thus become one of the economic pillars for mountain farmers seeking to escape poverty (Cao et al., 2012; Ni and Shi, 2014; Yuan et al., 2018). However, during growth and development, Chinese hickory is vulnerable to environmental hazards such as drought, salinity, and high temperatures, resulting in slow growth, leaf loss, flower and fruit drop, and even tree death, resulting in reduced fruit quality and yield (Xi et al., 2016; Sharma et al., 2020; Zhang et al., 2021; Jiao et al., 2022). Grafting is widely used to improve plant adaptation to biotic or abiotic stresses (Assunção et al., 2019). In recent years, grafting has been regarded as one of the most promising techniques to solve the problems of Chinese hickory, including big tree size, sensitive to biotic and abiotic stresses, and long juvenile phase. However, the survival rate of grafting in Chinese hickory are still low because of the complex factors influencing the whole grafting process, which has restricted the development of Chinese hickory industry to a large extent. Consequently, revealing the regulatory mechanisms of grafting success would be of great importance to promote the development of Chinese hickory. The adhesion between rootstock and scion is the first step in successful grafting, followed by the formation of the cambium, and finally, the establishment of vascular connections (Kurotani and Notaguchi, 2021). The content of auxin in the healing area increased significantly after grafting and remained high levels throughout the healing process (Sharma and Zheng, 2019). Several studies about Chinese hickory grafting have identified the key stages important for survival (Liu et al., 2002), and have concluded that auxin plays an important role during the grafting success (Zheng et al., 2002; Qiu et al., 2016; Xu et al., 2017; Saravana Kumar et al., 2018; Yuan et al., 2018; Xu et al., 2020; Yang et al., 2021). Despite the significant advances in the studies of Chinese hickory and Chinese hickory grafting, little is known about the genetic roles of key genes, including auxin influx carriers under abiotic stress treatments and during grafting.
In the current study, the AUX/LAX family genes in Chinese hickory were cloned and structurally analyzed in terms of phylogeny analysis, gene structure analysis, and detection of cis-acting regulatory elements on their promoters. We explored the expression patterns of the CcAUX/LAX genes in response to salt, drought, and grafting treatments. The tissue-specific expression profiles of CcAUX/LAX genes, as well as their differential responses to salt and drought stress, provide the molecular basis for improving abiotic stress tolerance in Chinese hickory. Simultaneously, our study provided new insights into the expression of the CcAUX/LAX gene family during the grafting process and investigated the transport of auxin during the grafting mechanism, laying the groundwork for future research into the mechanism of auxin in the graft healing process.
2. Materials and methods
2.1. Plant materials, growth conditions and treatments
Seeds of Chinese hickory were collected in September, 2015, and infiltrated in carbendazim solution for about 1 hour. After infiltration, seeds were spread on the mixed medium containing 55% medium (Hangzhou Jinhai Agricultural Technology Co., LTD) and 45% yellow sandy soil, and covered with the same mixed medium for the thickness of about 5 cm. The seeds were then cultured for about 4 months at the temperature of ~28°C and humidity of 70%~80% until germination. After germination, the seeds were cultured on the seedbeds containing 10 cm river sand and 5 cm mixed medium (containing 40% peat soil, 30% wormcast and 30% silt soil) at the following conditions: temperature ~15°C, humidity 60~75%. The seedlings were watered every 4 days and disinfected every 20 days until reaching the height of ~10 cm. The seedlings were then transplanted into the cups with the size of 17 cm × 22 cm containing the medium 50% peat soil, 30% pastoral soil, 10% organic fertilizer, 5% epicarp, 3% perlite and 2% slow release fertilizer in May 2016. One seedling were planted in each cup and the seedlings were cultured in the greenhouse of Zhejiang Agricultural and Forestry University until April 2018 (two years old) at the following conditions: temperature 25~35°C, humidity 70%, photoperiod 12/12-hour light/dark. As a control for the abiotic stress treatment, Chinese hickory seedlings were irrigated with tap water. Chinese hickory seedlings were irrigated with 50 g·L-1 and 200 g·L-1 PEG6000 (polyethylene glycol) solutions until thoroughly watered, repeated every 3 days, and samples were collected 5 days, 10 days, and 15 days after treatment, respectively, under simulated drought stress. For salt stress treatment, the Chinese hickory seedlings were thoroughly watered with 50 mM and 150 mM NaCl solutions, repeated every 3 days, and samples were collected after 1 day, 3 days, and 10 days of treatment. The roots, stems, and leaves of Chinese hickory seedlings were collected at various stress levels and time intervals. For each tissue, collections from five different seedlings were mixed together and regarded as a single sample. For the tissue-specific expression analysis, we collected the tissues of Chinese hickory, stems, leaves, flowers and fruits. For each tissue, collections from five different seedlings were mixed together and regarded as a single sample. The grafting samples were the same as a previous work of our group (Xu et al., 2020). Graft unions of four replicates in each group were used for expression analysis of CcAUX/LAX genes. The samples were immediately immersed in liquid nitrogen and stored in a -80°C ultra-low temperature refrigerator.
2.2. Isolation and cloning of AUX/LAX genes in Chinese hickory
The sequences of AUX/LAX family genes in Chinese hickory were screened from previously published genome sequences (http://dx.doi.org/10.5524/100571) (Huang et al., 2019). The BLASTX algorithm was used to identify all unigenes, and overlapping sequences were assembled using the SeqMan software in the Lasergene software package. The eight assembled sequences were analyzed and found to be full-length AUX/LAX gene sequences from Chinese hickory. After identifying the full-length gene sequences, the genes were amplified with gene-specific primers (Supplementary Table 1) using the PrimeSTAR Max DNA Polymerase (TaKaRa, Dalian, China).
2.3. Phylogeny, gene structure, and MEME analysis
ClustalW was used to perform multiple sequence alignments of AUX/LAX proteins from Arabidopsis thaliana, Populus trichocarpa, Oryza sativa, Vitis vinifera, Eucalyptus grandis and Prunus persica. The phylogenetic tree was constructed using MEGA-X (version 10.0.1) with the neighbor-joining (N-J) method (1000 bootstrap replicates). To analyze gene structures, the Gene Structure Display Server (GSDS) online program (http://gsds.cbi.pku.edu.cn/) was used to compare the coding sequences of the CcAUX/LAX family genes with their corresponding genome sequences. TMHMM2 was used to analyze and visualize the transmembrane domains of CcAUX/LAXs. Protein secondary structure prediction with SOPMA (https://npsa-prabi.ibcp.fr/cgi-bin/npsa_automat.pl? page=npsa_sopma. html). ExPASy ProtParam (http://www.expasy.org/proteomics) was used to analyze the physiochemical parameters of CcAUX/LAXs, such as protein length, molecular weight (MW), and isoelectric point (pI). The Multiple Expectation Maximization for Motif Elicitation (MEME) Suite (https://meme-suite.org/tools/meme) was used to analyze the motifs of CcAUX/LAX family proteins, with the number of motifs set to eight (Bailey et al., 2009).
2.4. Analysis of cis−elements in CcAUX/LAX promoter region
The Chinese hickory Genome Database was used to search for the promoter sequence of the CcAUX/LAX genes based on the ORF sequence. For promoter analysis, the 2000 bp upstream the start codon (ATG) of CcAUX/LAX genes were retrieved from the Chinese hickory genome dataset and submitted to the PlantCARE database (Lescot et al., 2002) and affirmed by employing PLACE databases (Higo et al., 1999).
2.5. Subcellular localization analysis
The full-length coding sequences of CcLAX1, CcLAX2 and CcLAX4 were identified in Chinese hickory and constructed into the pCAMBIA1300 vector, which contains the CaMV 35S promoter and GFP gene, resulting in a 35S:: CcLAX: GFP fusion vector. The plasmid was then transformed into Agrobacterium strain GV3101 competent cells (WEIDI, AC1001). pm-rk was used as a plasma membrane marker. All strains were transformed into 5~6-week-old tobacco (Nicotiana benthamiana) leaves. After 48 hours of incubation in the dark, fluorescence images were acquired at 488 and 594 nm with a high-resolution laser confocal microscope (LSM880, Zeiss).
2.6. RNA isolation and real-time quantitative PCR analysis
Total RNA was isolated using the MiniBEST Universal RNA Extraction Kit (TaKaRa Bio, Code No. 9767) as specified by the manufacturer. The first-strand cDNA synthesis was carried out in accordance with the manufacturer’s instructions using a PrimeScript RT Master Mix (TaKaRa Bio, Code No. RR036A). The cDNA used for Real-Time Quantitative PCR analysis (qRT-PCR) was obtained by PrimeScriptTM RT Master Mix (TaKaRa Bio, Code No. RR036A). The qRT-PCR primer sequence is listed in Supplementary Table 2. The qRT-PCR experiments were carried out on a BioRad CFX96 real-time PCR instrument with TB Green Premix Ex Taq II (TaKaRa Bio, Code No. RR420A). The Chinese hickory ACTIN gene (CcActin) was used as a reference gene. All the expression analyses were repeated four times. Heat map was created by MeV software using average Ct values to show expression data.
2.7. Statistical analysis
The 2−ΔΔCT method described by Schmittgen and Livak (2008) was used to calculate relative gene expression levels. (Schmittgen and Livak, 2008). To describe the differences in expression of each CcAUX/LAX among different tissues, a one-way analysis of variance (ANOVA) was performed using IBM SPSS statistical software (version 22.0), and multiple comparisons were made using Duncan’s method at the 0.05 level of significance. The experiments were performed in three independent biological replicates.
3. Results
3.1. Identification of AUX/LAX family genes in Chinese hickory
The open reading frame (ORF) sequences of eight CcAUX/LAX genes were screened and identified based on Chinese hickory genome and transcriptome data (Qiu et al., 2016; Huang et al., 2019). To validate the accuracy of sequences, the full-length cDNA sequences of 8 CcAUX/LAX genes were cloned from Chinese hickory. The ORF of the CcAUX/LAX genes ranged from 1380 (CcLAX5) to 1479 (CcLAX3), and the number of corresponding amino acids varies from 459 aa to 494 aa (Table 1). The molecular weight of the proteins ranged from 52.20 kDa to 55.75 kDa, and the predicted isoelectric points varied from 8.29 (CcLAX6) to 9.01 (CcLAX8).
Prediction of the CcAUX/LAX transporter’s transmembrane structure revealed that all members of the family had 10 transmembrane structures (Supplementary Figure 1). The ratio of the secondary structure components of CcAUX/LAXs were analyzed by SOPMA, and the secondary structure composition of the eight proteins was relatively similar, with more random coils and α-helices (74.07%-78.7%), and less β-turns and extended chains (Table 2; Supplementary Figure 2).
3.2. Phylogenetic analysis of CcAUX/LAX family members
To better understand the evolutionary relationships of CcAUX/LAX genes with their homologous genes in other plant species, the protein sequences of AUX/LAXs in Chinese hickory and the other 6 species, including Arabidopsis thaliana, Populus trichocarpa, Oryza sativa, Vitis vinifera, Eucalyptus grandis and Prunus persica were compared on a phylogenetic tree, detailed information on the 36 AUX/LAX proteins is shown in Supplementary Table 3, which may help to predict the potential functions of CcAUX/LAXs. The 36 AUX/LAX proteins from seven different plant species were classified into Group I, II, III, IV, V and VI (Figure 1), with the number of members of 8, 6, 4, 3, 7 and 8, respectively. CcAUX/LAXs were mainly distributed in four groups of I, II, V and VI. There are 3 paralogous gene pairs in the Chinese hickory AUX/LAX family: CcLAX4/CcLAX8, CcLAX2/CcLAX6 and CcLAX1/CcLAX5. Furthermore, CcAUX/LAXs were found to be more closely related to the homologs in the four woody plants, but less related with the homologs in A. thaliana and O. sativa which were mainly distributed in groups III and IV. The phylogenetic relationships between AUX/LAXs suggested that they might perform different functions on the growth and development of different plant species.
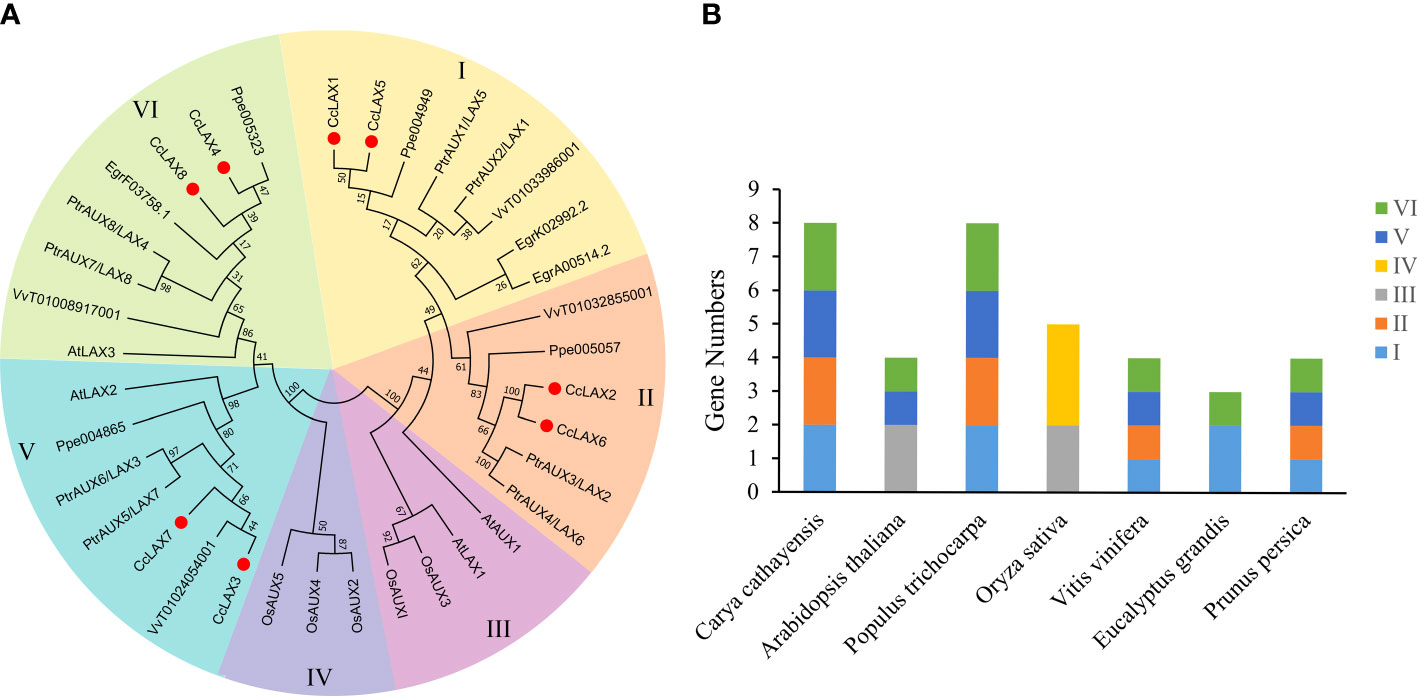
Figure 1 Phylogenetic analysis of CcAUX/LAX family genes with other AUX/LAX genes from 6 species. (A) The phylogenetic tree.The unrooted phylogenetic tree was built in MEGA6 using the neighbor-joining (N-J) method, and the bootstrap values were calculated using 1000 iterations. Different colored backgrounds indicate different groups. (B) The number and distribution of AUX/LAXs in different species. At, Arabidopsis thaliana; Pt, Populus trichocarpa; Os, Oryza sativa; Vv, Vitis vinifera; Eg, Eucalyptus grandis; Pp, Prunus persica.
3.3. Motif and gene structure analysis of the CcAUX/LAX genes
To understand the possible structural evolution of CcAUX/LAXs, the conserved motifs of the AUX/LAX sequences were evaluated using the MEME Suite web program. The results revealed that the majority of CcAUX/LAXs contained eight motifs, but motif 7 was missing for CcLAX5 (Figures 2A, B; Supplementary Figure 2). The structures of the CcAUX/LAX genes were further evaluated, and the variation of the intron-exon structures of the CcAUX/LAX was analyzed using GSDS online software. The results revealed that the gene structure differences among members of this family were relatively negligible, and their exon lengths were comparable. CcLAX5 had 7 exons and 6 introns, while the other genes have 8 exons and 7 introns (Figure 2C). These results indicated that CcAUX/LAXs had highly conservative characteristics during evolution.
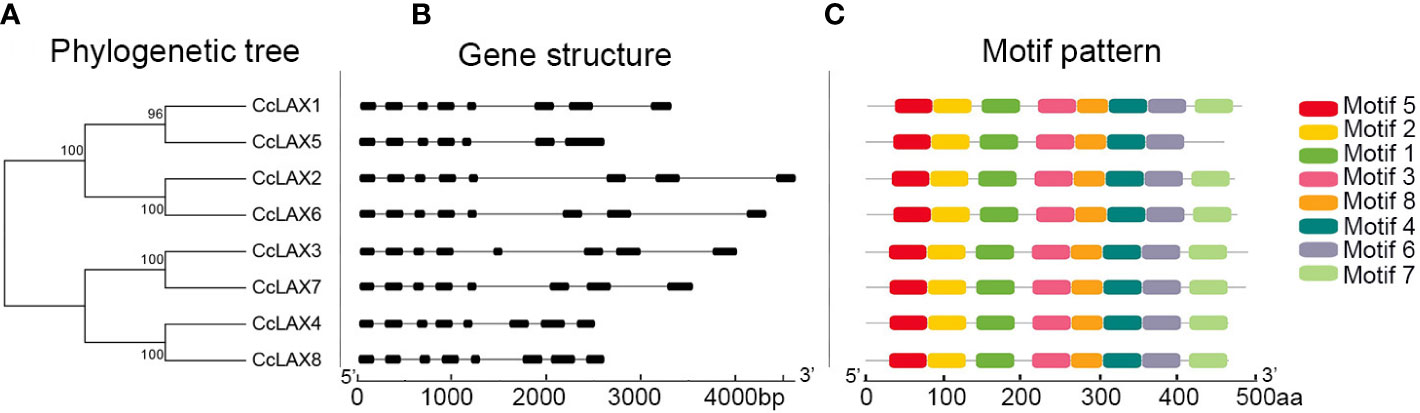
Figure 2 Phylogenetic analysis, gene structure and motif pattern in AUX/LAX genes from Chinese hickory. (A) The phylogenetic tree was constructed based on the full-length sequences of CcAUX/LAX proteins using MEGA7 software. (B) The exons are represented by black boxes, and the introns are represented by lines. (C) Motifs of the AUX/LAX proteins were analyzed by the Multiple Expectation Maximization for Motif Elicitation (MEME) web server, different colored boxes mean different motifs.
3.4. Cis−acting regulatory elements in the promoters of CcAUX/LAXs
The cis-regulatory elements upstream the 2000 bp of the start codon of the CcAUX/LAX genes were analyzed using PlantCARE online software. Aside from promoter core elements like the TATA-box and CAAT-box, several light-responsive elements, hormone-responsive elements, and abiotic stress-responsive elements were detected. The hormone and stress-related responsive elements were shown in Figure 3. The hormone responsive elements included ABRE (ACGTG, abscisic acid response), CGTCA-motif (CGTCA, methyl jasmonate response), ERE-motif (ATTTTAAA, ethylene response), P-box (CCTTTTG, gibberellin response), GARE-motif (TCTGTTG, gibberellin response), TCA-element (CCATCTTTTT, salicylic acid response) and TGA-element (AACGAC, auxin response), while abiotic stress response elements included CCAAT-box (CAACGG, MYB binding site), LTR (CCGAAA, involved in low temperature response), MBS (CAACTG, involved in drought response), TC-rich repeats (GTTTTCTTAC, involved in defense and abiotic stress) and W-box (TTGACC, WRKY binding site).
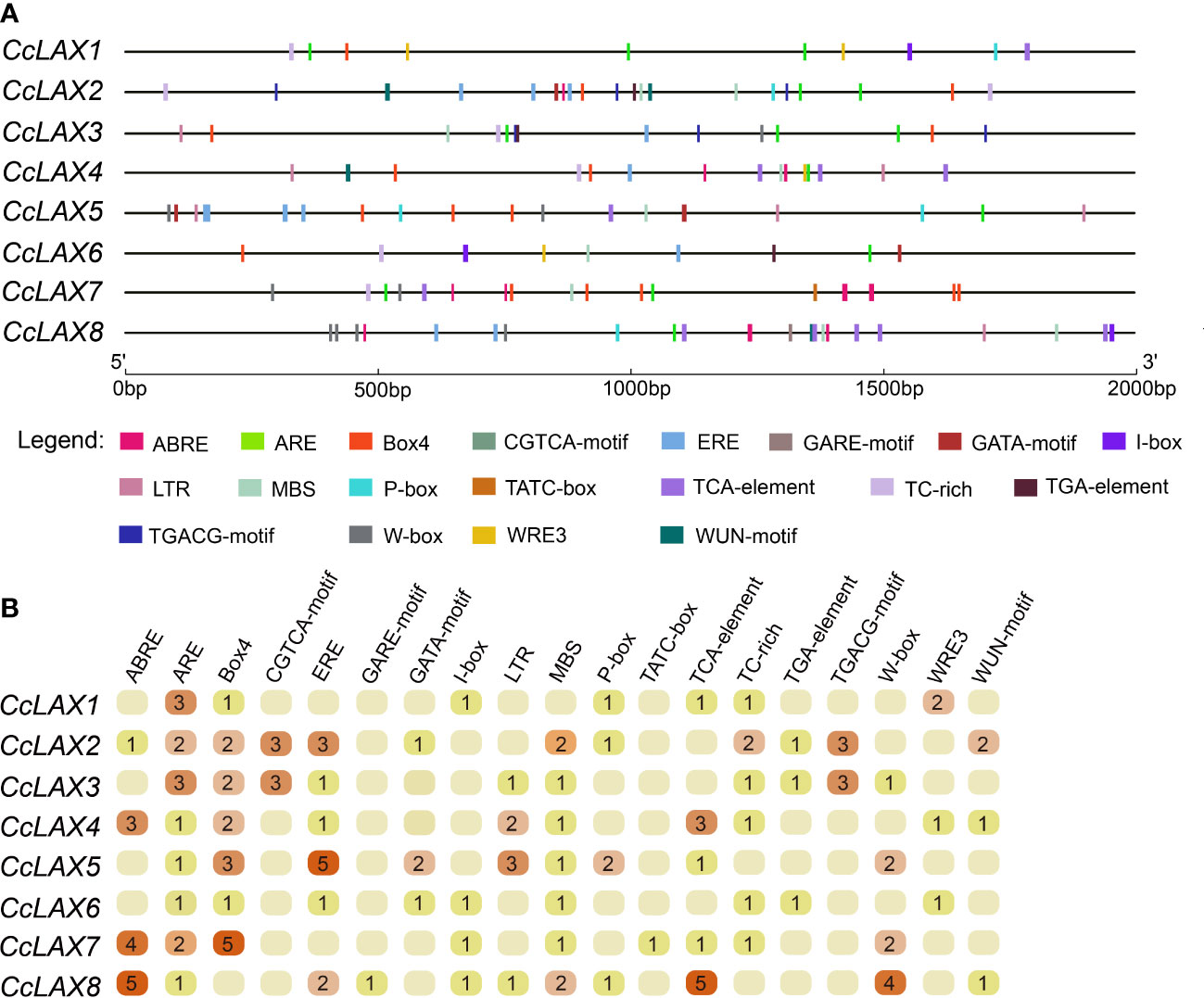
Figure 3 The cis-element prediction of CcAUX/LAX promoters. (A) The distribution of the 12 cis-acting elements in the 2000 bp promoter region is shown. The different types of cis-acting elements are represented by different shapes. ABRE, cis-acting element involved in the abscisic acid response. CGTCA-motif, MeJA-response element. ERE motif, cis-acting response involved in the ethylene response. P-box and GARE-motif, elements involved in the gibberellin response. TCA-element, cis-acting element associated with the salicylic acid response. TGA element, cis-acting element involved in the auxin response. CCAAT-box, abiotic stress response elements. LTR, cis-acting element involved in the low temperature response, MBS, MYB binding site involved in drought-inducibility. TC-rich, involved in defense and abiotic stress. W-box, WRKY binding site. (B) Number of cis-acting elements on the promoters of different CcAUX/LAXs.
3.5. Tissue−specific expression profiles and subcellular localization of CcAUX/LAX genes
To examine the potential roles of the AUX/LAX genes in different tissues of Chinese hickory such as roots, stems, leaves, flowers, and fruits, RT-qPCR assay was performed (Figure 4). Since the CcLAX4/8 sequences were highly conservative, it is difficult to design specific expression primers to distinguish them from each other, the expression levels of these genes were the combined expression of two gene copies. The results showed that CcLAX1 had the highest expression in flowers and lowest expression in fruits compared to other tissues, while expression of CcLAX2 was higher in roots and fruits, but almost undetectable in leaves and flowers. Expression of CcLAX6 was un-significantly changed between roots, stems, leaves, and flowers, but was the highest in fruits. CcLAX5, CcLAX3, CcLAX7, and CcLAX8 were expressed more abundantly in roots than in other tissues. These finding suggested that the CcAUX/LAX genes expressed differently in different tissues, implying that they might play different roles in the growth and development stages of Chinese hickory. The subcellular localization detected in tobacco leaf epidermal cells indicated that CcLAX1, CcLAX2 and CcLAX4 were membrane-localized (Figure 5).
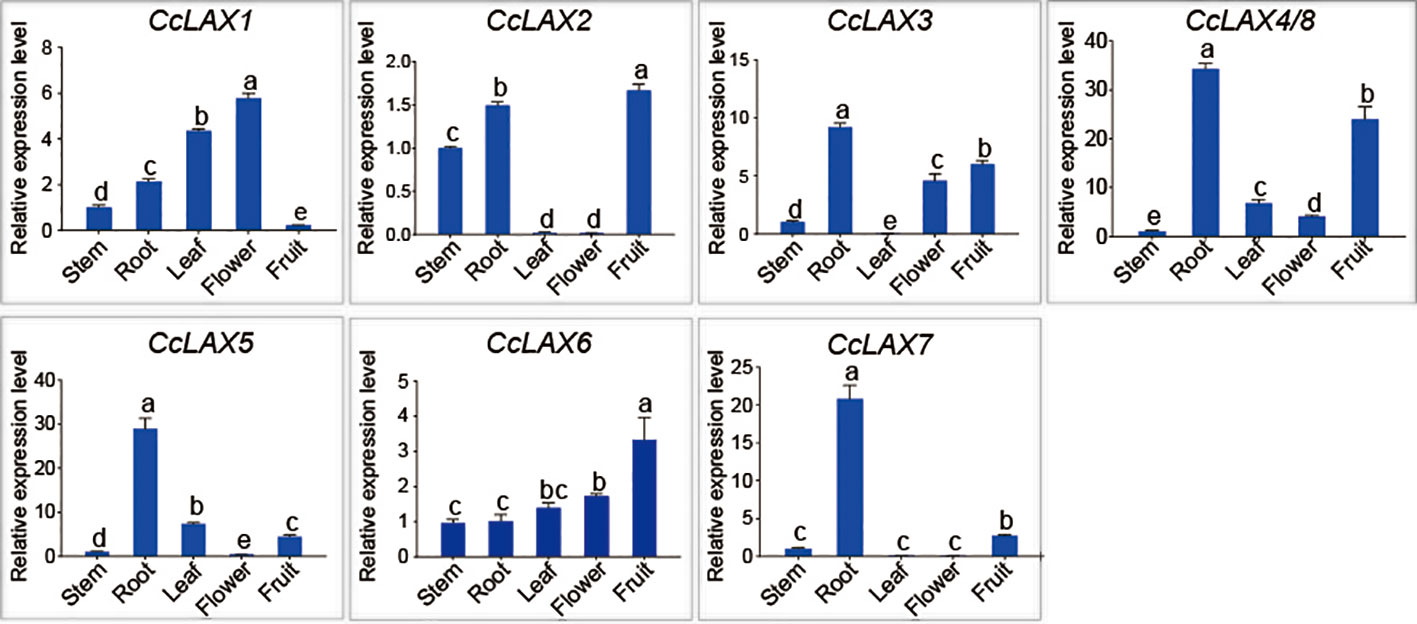
Figure 4 Expression patterns of CcAUX/LAX genes in Chinese hickory tissues (stem, root, leaf, flower, fruit). The CcActin gene was used as the internal reference gene for normalization. The relative expression levels of each CcAUX/LAX gene in stems were standardized as 1. Different letters represent a significant level of expression (P < 0.05) in different tissues of Chinese hickory. Multiple comparison test was performed by one-way analysis of variance and Duncan test.
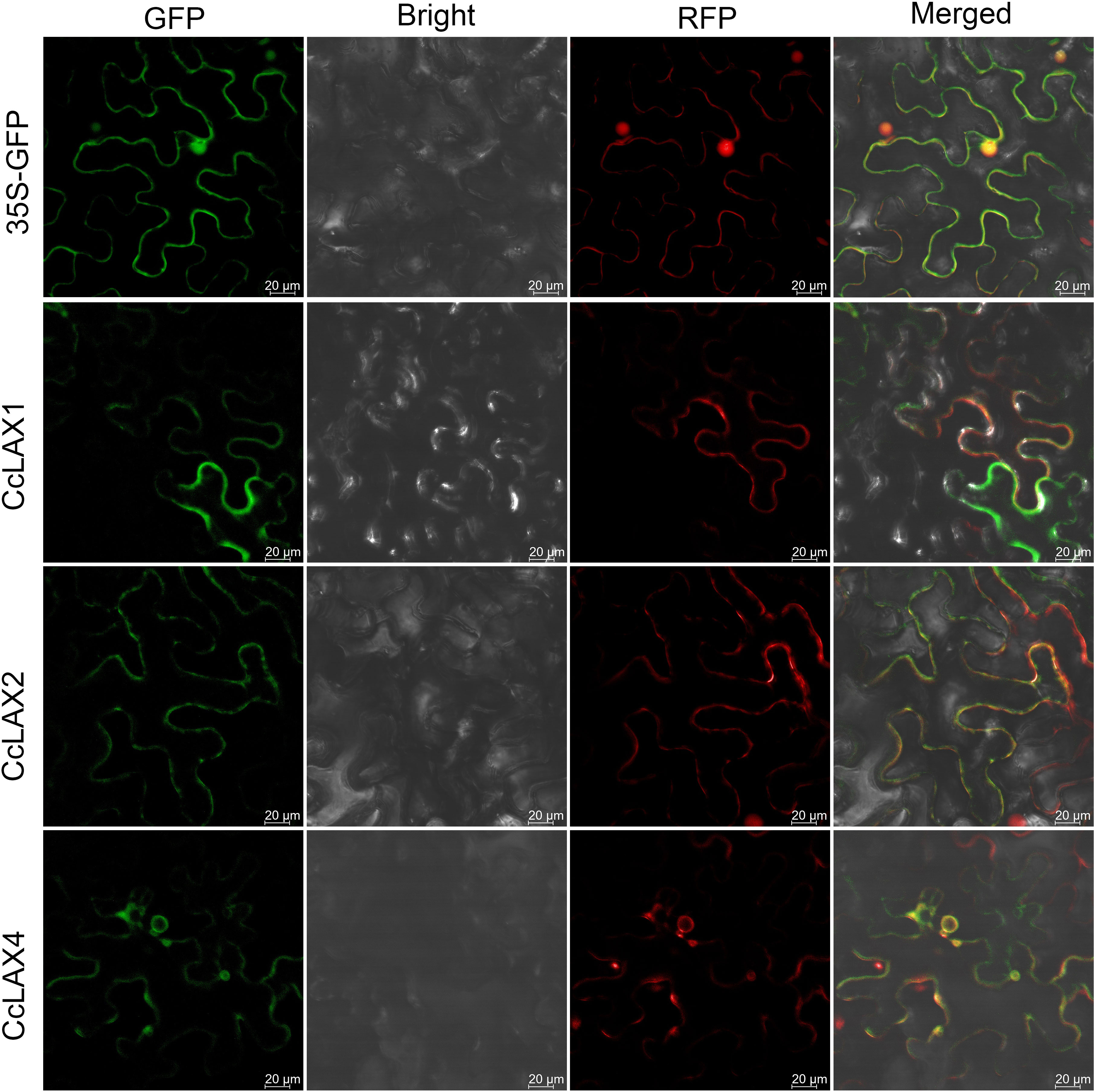
Figure 5 Subcellular localization of CcLAX1, CcLAX2 and CcLAX4 proteins. Left to right: green fluorescence (GFP), bright field (Bright), red fluorescence (RFP), and merged microscope images (Merged). Bar = 20μm.
3.6. Expression analysis of CcAUX/LAXs under drought and salt stresses
Auxin has been implicated in stress responses in numerous studies, and a significant number of auxin transporter genes have been linked to abiotic stress responses. To explore the potential function of CcAUX/LAX genes under environmental stress, qRT-PCR was used to assess the expression patterns of CcAUX/LAX genes in Chinese hickory roots, stems, and leaves after mild and severe salt stress and drought stress treatments (Figure 6). The expressions of CcAUX/LAXs were altered to varying degrees in the current study after drought and salt treatments. Under mild and severe drought stress, the expressions of some genes in roots were lower than those in leaves. For instance, the expressions of CcLAX5, CcLAX3 and CcLAX2 were down-regulated in roots, whereas increased in leaves (Figure 6A). After mild drought stress treatment, the expression level of CcLAX2 in roots was greatly increased, while no significant difference was observed under severe drought stress. At the early stage of drought treatment, CcLAX6 expression was up-regulated in roots, but there was no obvious change in leaves and stem segments. Furthermore, there was no statistically significant difference in the expression of the CcLAX4 and CcLAX8 genes after drought stress treatment.
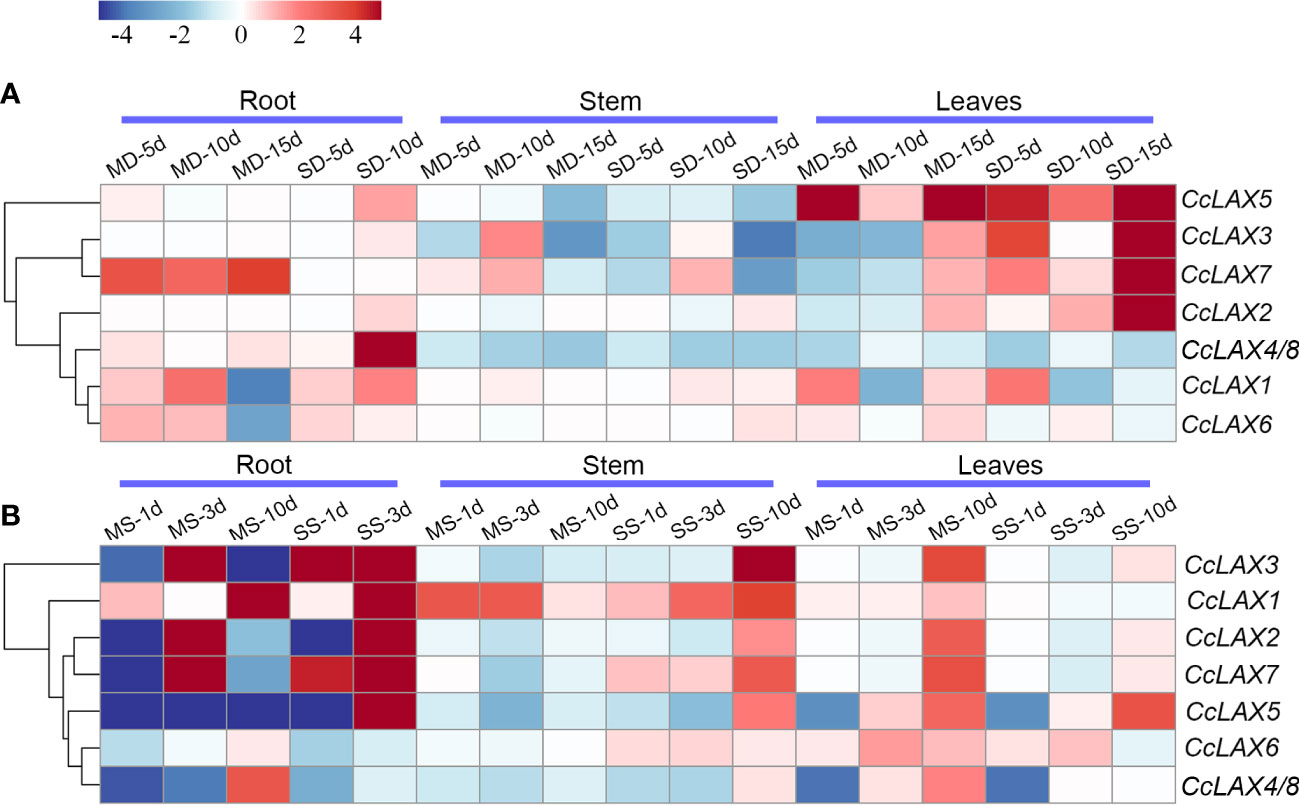
Figure 6 Expression patterns of putative CcAUX/LAX genes in Chinese hickory under drought and salt treatments. (A) Expression profiles of CcAUX/LAX family genes in roots, stems and leaves under mild drought stress (50g·L-1 PEG) and severe drought stress (200g·L-1 PEG) treatments for 5 days, 10 days and 15 days, respectively (B) Expression profiles of CcAUX/LAX family genes in roots, stems and leaves under mild salt stress (50 mM NaCl) and severe salt stress (150 mM NaCl) treatments for 1 days, 3 days and 10 days, respectively. MD-5d, mild drought stress treatment for 5 days; MD-10d, mild drought stress treatment for 10 days; MD-15d, mild drought stress treatment for 15 days; MS-1d, mild salt stress treatment for 1 days; MS-3d, mild salt stress treatment for 3 days; MS-10d, mild salt stress treatment for 10 days.
Under salt stress treatment, most of the genes were highly expressed in roots, but low expression was found in stems and leaves (Figure 6B). The results showed that the expression levels of CcLAX1, CcLAX2, CcLAX3, CcLAX5 and CcLAX7 in roots were the highest after 3 days of severe salt stress treatment. CcLAX3 was up-regulated in roots after both mild and severe salt stress treatments. The expression of CcLAX6 was down-regulated in roots and up-regulated in leaves after salt stress treatment. Interestingly, a few genes had higher levels of expression after drought stress application, whilst their transcript levels were decreased under salt stress treatment. Likewise, few had higher expression levels under salt stress, while low expression was found under drought stress, indicating their different roles under different circumstances.
3.7. Expression profiles of CcAUX/LAX genes during grafting
According to our previous study, we found that the critical period for grafting healing of Chinese hickory was 3, 7, and 14 days after grafting respectively (2020; Qiu et al., 2016; Xu et al., 2017; Saravana Kumar et al., 2018). 30 days after grafting was selected to represent reconnection between the rootstock and the scion (Xu et al., 2020). To understand the possible role of CcAUX/LAXs in the grafting process of Chinese hickory, the expression patterns of CcAUX/LAXs at different time points after grafting in scions and rootstocks were analyzed. The results revealed that CcAUX/LAX genes had different expression patterns during different stages after grafting (Figure 7). Interestingly, a few genes showed differential expression patterns during the grafting process. The expression levels of CcLAX5 and CcLAX6 were reduced in both rootstocks and scions after grafting, but the reduction was not significant. Most genes were expressed at a higher level in the scion than in the rootstock. CcLAX1, CcLAX3, CcLAX4 and CcLAX8 were highly expressed in the scion and had higher levels of expression at 3, 7, 14, and 30 days after grafting, implying that they might play crucial role during grafting healing.
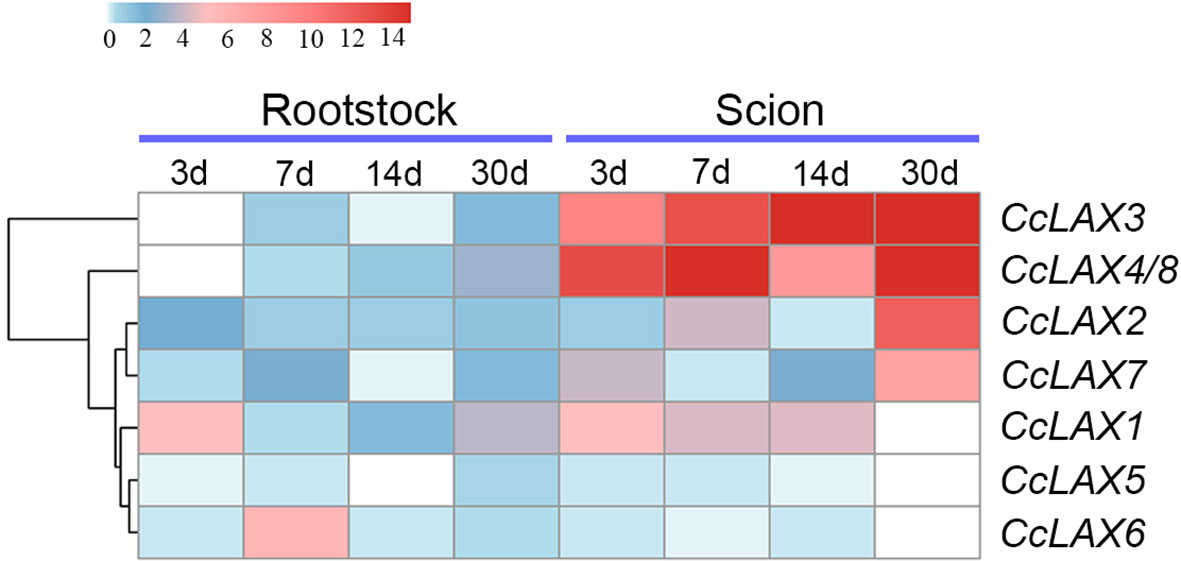
Figure 7 Heatmap of CcAUX/LAX gene expressions at different grafting stages. Red and blue represent relatively high and low expression compared to the control, respectively. The values used in the heat map were the mean of three replicates, and normalized to the control sample. The control samples were scions and rootstocks collected at day 0 of grafting.
IAA (indole-3-acetic acid) is an important plant hormone that regulates various aspects of plant growth and development, NPA (N-1-naphthylphthalic acid) is an inhibitor that inhibits or blocks the polar transport of auxin. A previous study found that IAA application significantly increased the rate of successful grafting (80%), whereas NPA application decreased the rate of successful grafting (24%), when compared to non-applied samples (32%) in Chinese hickory (Saravana Kumar et al., 2018). The expression patterns of the CcAUX/LAX genes under IAA and NPA treatments were examined to further reveal their potential role in the grafting process (Figure 8). After IAA treatment, the expression of CcLAX2 increased in rootstocks at 7 days and 14 days after grafting, and tended to be stable at 30 days after grafting. In contrast, in the scion, the expression of CcLAX2 gene was down-regulated. However, the expression pattern of CcLAX5 was opposite to CcLAX2, with decreased expression in rootstocks after IAA treatment. The expression levels of CcLAX3, CcLAX6 and CcLAX4/8 genes were up-regulated in rootstocks after NPA and IAA treatment, and the up-regulation of gene expression was greater at 3 and 14 days after NPA treatment. In the scions, hormone treatment did not affect the expression of CcLAX3, CcLAX6 and CcLAX4/8 genes in the pre-grafting period, while gene expression was down-regulated in the post-grafting periods. It was also observed that the expression levels of most genes tended to be stable after 30 days of grafting. In addition, different genes had great differences in the degree of responses to IAA and NPA treatments.
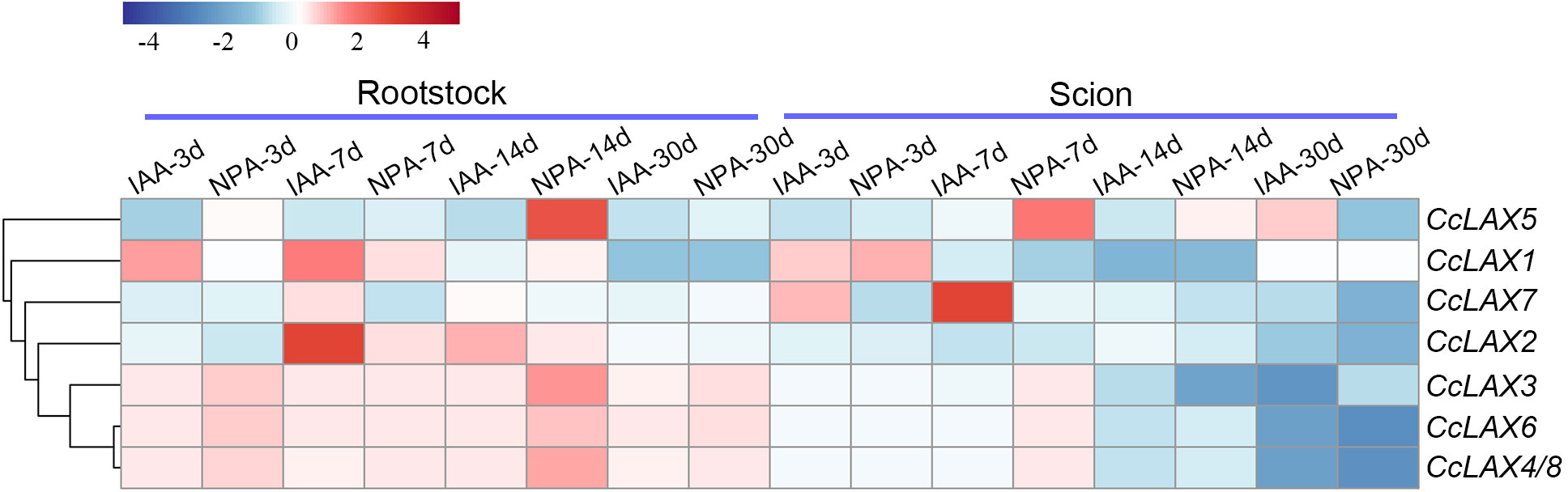
Figure 8 Expression patterns of CcAUX/LAX genes in response to IAA and NPA during grafting. Red and blue represent relatively high and low expression compared to the control, respectively. The values used in the heat map were the mean of four replicates, and normalized to the control sample. The control samples were scion and rootstock collected at day 0 of grafting.
4. Discussion
Auxin plays an active role in many plant developmental processes, as well as in coordinating plant responses to biotic and abiotic stresses (Petrášek and Friml, 2009; Swarup and Péret, 2012; Kazan, 2013; Sharma and Zheng, 2019). AUX/LAX is an auxin influx transporter protein, which is involved in the establishment of auxin concentration gradient and the regulation of plant grafting and abiotic stress. In this study, eight CcAUX/LAX genes in Chinese hickory were identified according to the published genome. And the expression profiles were determined during drought and salt stress as well as grafting to elucidate the function of CcAUX/LAXs.
4.1. Characterization analysis of CcAUX/LAX genes in Chinese hickory
Characterization analysis of CcAUX/LAX genes (8 members) in Chinese hickory showed that the number of LAX genes in Chinese hickory was consistent with that in P. trichocarpa, but higher than Arabidopsis (4 members), O. sativa (5 members), V. vinifera (3 members) and P. persica (5 members). Phylogenetic analysis of 36 AUX/LAX proteins in 6 plants species was carried out to compare with CcAUX/LAXs. On the phylogenetic tree, CcAUX/LAX were close to PtLAX, indicating that they may come from a common ancestor. Moreover, the result showed that three paralogous homologous gene pairs (CcLAX4/CcLAX8, CcLAX2/CcLAX6 and CcLAX1/CcLAX5) were found in the CcAUX/LAX gene family (Figure 1), which was the same as poplars (Carraro et al., 2012). These results suggested that the expanded CcAUX/LAX gene family may be due to genome-wide replication during the evolution of woody plants. The protein similarity and physicochemical properties showed that the protein similarity of CcAUX/LAX family reached 72.12%-94.08% (Supplementary Table 4), with the similar physicochemical properties. The gene structure of CcAUX/LAXs exhibited a much conserved exon-intron organization with eight exons and seven introns excepted the CcLAX5, which was similar to the gene structures of AUX/LAXs in other plants, with 6-8 exons in Arabidopsis, 2-7 exons in rice, and 8 exons in soybean. Furthermore, CcAUX/LAXs contained 10 transmembrane helices (Table 1, Supplementary Figure 1), and the subcellular localization assays indicated that CcLAX1, CcLAX2 and CcLAX4 proteins were localized to the plasma membrane (Figure 5), which was consistent with a previous study (Bao et al., 2019). These suggested that the protein structure of CcAUX/LAXs remained virtually unchanged during evolution, possibly due to its functional importance.
4.2. Tissue-specific expression analysis of CcAUX/LAX genes
Despite significant gene and protein structural conservation, CcAUX/LAXs expression at the transcriptional level varies considerably between tissues/organ (Figure 4). Tissue-specific expression profiling indicates that some duplicated gene pairs may play redundant roles in some tissues, such as CcLAX3 and CcLAX7 in roots and CcLAX2 and CcLAX6 in fruits. In Arabidopsis, AtLAX3 regulates lateral root development, and the present study also found that CcLAX3 and CcLAX7 gene pairs are highly expressed in roots. It is likely that the LAX3 gene had retained its original function during evolution (Swarup and Péret, 2012). The role of PaLAX1 in inflorescence development has been demonstrated, and overexpression of PaLAX1 in ataux1 mutants results in the production of multiple inflorescences (Hoyerová et al., 2008). The expression level of CcLAX1 gene in flowers was higher than in other tissues, indicating that CcLAX1 might be involved in inflorescence development. Furthermore, most CcAUX/LAX genes were expressed at higher levels in roots than in other tissues, while lower expression levels were found in leaves and stems. This may indicate that multiple LAX coordinately participate in the regulation of Chinese hickory root development. Therefore, further detailed analysis of the cell type-specific expression patterns of CcAUX/LAXs in different tissues/organ and during different developmental processes will help to identify their specific gene functions.
4.3. Expression patterns of CcAUX/LAX genes under salt and drought stresses
Under abiotic stresses such as drought and salinity, plants usually activate various mechanisms to resist the adverse environment (Chai, et al., 2016). Auxin is one of the most essential plant hormones that regulating plant mediating a variety of environmental stress responses (Melnyk et al., 2015; Yu et al., 2017). Recent studies showed that some auxin transporter genes have been involved in abiotic stress responses (Krishnamurthy and Rathinasabapathi, 2013; Rahman, 2013).In Glycine max, most LAX genes responded to a variety of plant hormone stimulation and abiotic stress (Chai et al., 2016). The majority of ClLAX, ClPIN, and ClABCB genes in stem and root tissues responded to cold, drought, and high salinity (Yu et al., 2017). The majority of ZmPIN and ZmLAX genes in Zea mays were up-regulated in shoots but down-regulated in roots due to drought stress (Yue et al., 2015). In the present study, the expression of CcLAX1, CcLAX6 and CcLAX7 was up-regulated in the roots of Chinese hickory under mild drought stress, while the expression of other genes had no significantly changed or down-regulated. In addition, the expression of most CcAUX/LAX genes were down-regulated at the early stage of salt stress treatment, while the expression of CcLAX2, CcLAX3 and CcLAX7 were significantly up-regulated in the roots after 3 days of mild and severe salt stress treatments. Besides, most of the CcAUX/LAX expression in stems did not change significantly under drought stress, which was similar in the salt stress treatment. This may be due to the low expression of CcAUX/LAX in stems. The expression of CcAUX/LAX after drought and salt stress is irregular, which may be due to the coordinated expression among members involved in auxin uptake or transport, thus regulating the response of Chinese hickory to drought and salt stress.
4.4. CcAUX/LAX genes are potentially involved in the grafting healing process
Grafting is a common asexual technique for propagation in Chinese hickory, which can successfully address the issues of tall trees, a long juvenile period, and a low level of improved cultivation. A detailed analysis of morphological characteristics during Chinese hickory grafting revealed that 3, 7, and 14 DAG (days after grafting) are critical time points during the grafting process, showing the development of the necrotic layer, healing tissue proliferation, and differentiation of new vascular tissue in grafts, respectively (Qiu et al., 2016). Based on the transcriptome data of Chinese hickory grafting, auxin-related unigenes were identified as differentially expressed genes (DEGs) during the grafting process (Qiu et al., 2016). Further investigation revealed that the expression of Aux/IAA, GH3, and ABCB family genes changed dramatically during graft healing (Yuan et al., 2018; Xu et al., 2020; Yang et al., 2021). The AUX/LAX transporter is an auxin transporter that aids in the transport of auxin between cells and the formation of vascular bundles. In order to determine the role of CcAUX/LAX gene in the grafting process, the expression of CcAUX/LAX gene was studied. All CcAUX/LAX were in a low expression state in the rootstock, while CcLAX3 and CcLAX4/8 were consistently high expression in the scion. This may be because the auxin synthesized in the scion shoots is transported to the grafting healing site by them to promote the connection of vascular tissues, thus promoting the survival of the grafting. During graft healing, auxin plays a role by regulating cell differentiation and vascular bundles. Previous studies revealed that NPA is an auxin transport inhibitor that reduces polar auxin transport (Teale and Palme, 2018). In our study, NPA treatment significantly reduced the expression of several CcAUX/LAX genes (such CcLAX1, CcLAX2 and CcLAX7) compared to IAA treatment, implying that obstructing auxin transport might impact the AUX/LAX-mediated auxin signaling pathway. However, after NPA treatment, a few genes, including CcLAX3, CcLAX6, and CcLAX4/8, showed increased expression, most likely due to increased expression of some AUX/LAX transporter proteins to maintain auxin homeostasis when auxin transport was inhibited. In contrast, IAA treatment did not increase the expression of CcAUX/LAX gene, probably due to the exogenous growth hormone treatment affected the endogenous growth hormone transport. A previous study by our group found that IAA treatment increased while NPA treatment significantly reduced the survival rate of Chinese hickory grafting. Variations in the expressions of the CcAUX/LAX genes after IAA and NPA treatment could explain some of our previous findings (Xu et al., 2017). Furthermore, the differential expression patterns of CcAUX/LAX family genes suggested that auxin transport during grafting in Chinese hickory might be regulated in a complex manner.
5. Conclusion
To investigate the potential roles of auxin influx transporters in response to drought, salt stress and grafting process of Chinese hickory, eight CcAUX/LAX genes were identified in Chinese hickory for the first time, which were most closely related to the homologous genes in poplar. CcAUX/LAXs were located on the cell membrane and displayed different expression levels in different tissues, indicating their varying roles during growth and development. Moreover, cis-acting elements related to phytohormones and stress responses were detected on the promoters of CcAUX/LAXs. The expression levels of CcAUX/LAXs were up-regulated or down-regulated to varying degrees during drought and salt stress treatments, indicating their involvement in the response process of plants to abiotic stresses. In addition, the significant changes in the expressions of CcAUX/LAXs during grafting and in response to IAA and NPA treatments during grafting may partly explain the mechanism of auxin in regulating Chinese hickory grafting. This study will lay a foundation for further understanding the regulatory mechanisms of auxin transporters during grafting and in response to abiotic stresses in Chinese hickory. In future, further functional analysis and regulatory networks of these genes will be carried out to explore the molecular mechanisms of CcAUX/LAXs in response to drought, salt stress and grafting.
Data availability statement
The original contributions presented in the study are included in the article/Supplementary Material. Further inquiries can be directed to the corresponding authors.
Author contributions
BZ, YH, HY and RW conceived and designed the concept of manuscript. YY, JW, YX, ST, FS and DX performed the experiments. YY, XX, DX, QH, JW and YX analyzed the data. FA, YH, HY, DY and XW did the formal analysis. YY, FA, and HY drafted the manuscript, BZ, FA, XW, AS, LZ, DY, HY, YH, and RW revised and finalized the manuscript. All authors contributed to the article and approved the submitted version.
Funding
This study was supported by National Natural Science Foundation of China (31901346, 32071807, 31971695); Key Project of Zhejiang Provincial Natural Science Foundation (LZ18C160001); National Key Research and Development Program of China (2018YFD1000604); Independent Research Project of State Key Laboratory of Subtropical Silviculture, Zhejiang A&F University (ZY20180208, ZY20180308); Open Foundation of State Key Laboratory of Subtropical Silviculture, Zhejiang A&F University (KF202007, KF201903, KF201708); Overseas Expertise Introduction Project for Discipline Innovation (111 Project D18008); Key Research and Development Program of Zhejiang Province (2018C02004); National High Technology Research and Development Program of China (863 Program) (2013AA102605); Fruit Innovation Team Project of Zhejiang Province (2016C02052-12); Key Agricultural New Varieties Breeding Projects founded by Zhejiang Province Science and Technology Department (2021C02066-12, 2016C02052-13); Zhejiang Provincial Natural Science Foundation for Distinguished Young Scholar (LR13C160001); Open Foundation of First-class Discipline of Forestry, Zhejiang Province (201703); The First-class General Financial Grant from the China Postdoctoral Science Foundation (2017M610377).
Conflict of interest
The authors declare that the research was conducted in the absence of any commercial or financial relationships that could be construed as a potential conflict of interest.
Publisher’s note
All claims expressed in this article are solely those of the authors and do not necessarily represent those of their affiliated organizations, or those of the publisher, the editors and the reviewers. Any product that may be evaluated in this article, or claim that may be made by its manufacturer, is not guaranteed or endorsed by the publisher.
Supplementary material
The Supplementary Material for this article can be found online at: https://www.frontiersin.org/articles/10.3389/fpls.2022.1060965/full#supplementary-material
References
Aliyari Rad, S., Dehghanian, Z., Asgari Lajayer, B., Nobaharan, K., Astatkie, T. (2022). Mitochondrial respiration and energy production under some abiotic stresses. J. Plant Growth Regul. 41, 3285–3299. doi: 10.1007/s00344-021-10512-1
Assunção, M., Santos, C., Brazão, J., Fevereiro, P. (2019). Understanding the molecular mechanisms underlying graft success in grapevine. BMC Plant Biol. 19, 1–17. doi: 10.1186/s12870-019-1967-8
Bailey, T. L., Boden, M., Buske, F. A., Frith, M., Grant, C. E., Clementi, L., et al. (2009). MEME suite: Tools for motif discovery and searching. Nucleic Acids Res. 37, 202–208. doi: 10.1093/nar/gkp335
Bainbridge, K., Guyomarc’h, S., Bayer, E., Swarup, R., Bennett, M., Mandel, T., et al. (2008). Auxin influx carriers stabilize phyllotactic patterning. Genes Dev. 22, 810–823. doi: 10.1101/gad.462608
Bao, Y., Huang, X., Rehman, M., Wang, Y., Wang, B., Peng, D. (2019). Identification and expression analysis of the PIN and AUX/LAX gene families in ramie (Boehmeria nivea l. gaud). Agronomy 9, 435. doi: 10.3390/agronomy9080435
Barbosa, I. C. R., Hammes, U. Z., Schwechheimer, C. (2018). Activation and polarity control of PIN-FORMED auxin transporters by phosphorylation. Trends Plant Sci. 23, 523–538. doi: 10.1016/j.tplants.2018.03.009
Bennett, M. J., Marchant, A., Green, H. G., May, S. T., Ward, S. P., Millner, P. A., et al. (1996). Arabidopsis AUX1 gene: A permease-like regulator of root gravitropism. Science 273, 948–950. doi: 10.1126/science.273.5277.948
Cao, X., Ding, Z., Jiang, F. (2012). Natural product research : formerly natural product letters antitumor constituents from the leaves of Carya cathayensis. Nat. Prod. Res. 22, 2089–2094. doi: 10.1080/14786419.2011.628174
Carraro, N., Tisdale-orr, T. E., Clouse, R. M., Knöller, A. S., Spicer, R. (2012). Diversification and expression of the PIN, AUX/LAX, and ABCB families of putative auxin transporters in Populus. Front. Plant Sci. 3. doi: 10.3389/fpls.2012.00017
Chai, C., Subudhi, P. K. (2016). Comprehensive analysis and expression profiling of the OsLAX and OsABCB auxin transporter gene families in rice (Oryza sativa) under phytohormone stimuli and abiotic stresses. Front. Plant Sci. 7. doi: 10.3389/fpls.2016.00593
Chai, C., Wang, Y., Valliyodan, B., Nguyen, H. T. (2016). Comprehensive analysis of the soybean (Glycine max) GmLAX auxin transporter gene family. Front. Plant Sci. 7. doi: 10.3389/fpls.2016.00282
Cho, M., Cho, H. T. (2013). The function of ABCB transporters in auxin transport. Plant Signal. Behav. 8, 2–4. doi: 10.4161/psb.22990
Dehghanian, Z., Habibi, K., Dehghanian, M., Aliyar, S., Asgari Lajayer, B., Astatkie, T., et al. (2022). Reinforcing the bulwark: unravelling the efficient applications of plant phenolics and tannins against environmental stresses. Heliyon 8, e09094. doi: 10.1016/j.heliyon.2022.e09094
Gomes, G. L. B., Scortecci, K. C.. (2021). Auxin and its role in plant development: structure, signalling, regulation and response mechanisms. Plant Biol. 23, 894–904. doi: 10.1111/plb.13303
Hadi, S., Aida, A., Nader, K., Behnam, A., Mohammad, P.. (2021). Roles of Phytohormones in Plants under Abiotic Stresses. In: Handbook of Plant and Crop Physiology. doi: 10.1201/9781003093640
Hammes, U. Z., Murphy, A. S., Schwechheimer, C. (2022). Auxin transporters–a biochemical view. Cold Spring Harb. Perspect. Biol. 14, 1–23. doi: 10.1101/cshperspect.a039875
Haskovec, J., Jönsson, H., Kreusser, L. M., Markowich, P. (2019). Auxin transport model for leaf venation. Proc. R. Soc A Math. Phys. Eng. Sci. 475:1400–1401. doi: 10.1098/rspa.2019.0015
Higo, K., Ugawa, Y., Iwamoto, M., Korenaga, T.. (1999). Plant cis-acting regulatory DNA elements (PLACE) database: 1999. Nucleic Acids Res 27, 297–300. doi: 10.1093/nar/27.1.297
Hoyerová, K., Perry, L., Hand, P., Laňková, M., Kocábek, T., May, S., et al. (2008). Functional characterization of PaLAX1, a putative auxin permease, in heterologous plant systems. Plant Physiol. 146, 1128–1141. doi: 10.1104/pp.107.109371
Huang, Y., Xiao, L., Zhang, Z., Zhang, R., Wang, Z., Huang, C., et al. (2019). The genomes of pecan and Chinese hickory provide insights into Carya evolution and nut nutrition. Gigascience 8, 1–17. doi: 10.1093/gigascience/giz036
Jiao, Y., Zhang, J., Pan, C. (2022). Integrated physiological, proteomic, and metabolomic analyses of pecan cultivar ‘Pawnee’ adaptation to salt stress. Sci. Rep. 12, 1–14. doi: 10.1038/s41598-022-05866-9
Kazan, K. (2013). Auxin and the integration of environmental signals into plant root development. Ann. Bot. 112, 1655–1665. doi: 10.1093/aob/mct229
Kleine-Vehn, J., Dhonukshe, P., Swarup, R., Bennett, M., Friml, J. (2006). Subcellular trafficking of the Arabidopsis auxin influx carrier AUX1 uses a novel pathway distinct from PIN1. Plant Cell 18, 3171–3181. doi: 10.1105/tpc.106.042770
Korver, R. A., Koevoets, I. T., Testerink, C. (2018). Out of shape during stress: A key role for auxin. Trends Plant Sci. 23, 783–793. doi: 10.1016/j.tplants.2018.05.011
Krishnamurthy, A., Rathinasabapathi, B. (2013). Auxin and its transport play a role in plant tolerance to arsenite-induced oxidative stress in Arabidopsis thaliana. Plant Cell Environ. 36 1–12:1412–1413. doi: 10.1111/pce.12093
Kurotani, K., Notaguchi, M. (2021). Cell-to-cell connection in plant grafting — molecular insights into symplasmic reconstruction. Plant Cell phys. 62, 1362–1371. doi: 10.1093/pcp/pcab109
Lee, H. W., Cho, C., Pandey, S. K., Park, Y., Kim, M. J., Kim, J. (2019). LBD16 and LBD18 acting downstream of ARF7 and ARF19 are involved in adventitious root formation in Arabidopsis. BMC Plant Biol. 19, 1–11. doi: 10.1186/s12870-019-1659-4
Lee, C., Chronis, D., Kenning, C., Peret, B., Hewezi, T., Davis, E. L., et al. (2011). The novel cyst nematode effector protein 19C07 interacts with the Arabidopsis auxin influx transporter LAX3 to control feeding site development. Plant Physiol. 155, 866–880. doi: 10.1104/pp.110.167197
Lescot, M., Déhais, P., Thijs, G., Marchal, K., Moreau, Y., Van De Peer, Y., et al (2002). PlantCARE, a database of plant cis-acting regulatory elements and a portal to tools for in silico analysis of promoter sequences. Nucleic Acids Res 30, 325–327. doi: 10.1093/nar/30.1.325
Li, J., Xu, H.-H., Liu, W.-C., Zhang, X.-W., Lu, Y.-T. (2015). Ethylene inhibits root elongation during alkaline stress through auxin1 and associated changes in auxin accumulation. Plant Physiol. 168, 1777–1791. doi: 10.1104/pp.15.00523
Liu, C., Hong, J., Xia, G., Huang, J. (2002). Cytological observation on healing responses in grafting of Carya cathayensis. Sci. silvae Sin 45, 34–37. doi: 10.1007/978-1-4020-9623-5_5
Marchant, A., Bhalerao, R., Casimiro, I., Eklöf, J., Casero, P. J., Bennett, M., et al. (2002). AUX1 promotes lateral root formation by facilitating indole-3-acetic acid distribution between sink and source tissues in the Arabidopsis seedling. Plant Cell 14, 589–597. doi: 10.1105/tpc.010354.2
Matthes, M. S., Best, N. B., Robil, J. M., Malcomber, S., Gallavotti, A., McSteen, P. (2019). Auxin EvoDevo: Conservation and diversification of genes regulating auxin biosynthesis, transport, and signaling. Mol. Plant 12, 298–320. doi: 10.1016/j.molp.2018.12.012
Mellor, N. L., Voß, U., Ware, A., Janes, G., Barrack, D., Bishopp, A., et al. (2022). Systems approaches reveal that ABCB and PIN proteins mediate co-dependent auxin efflux. Plant Cell 34, 2309–2327. doi: 10.1093/plcell/koac086
Melnyk, C. W., Schuster, C., Leyser, O., Meyerowitz, E. M. (2015). A developmental framework for graft formation and vascular reconnection in Arabidopsis thaliana. Curr. Biol. 25, 1306–1318. doi: 10.1016/j.cub.2015.03.032
Min, L., Li, Y., Hu, Q., Zhu, L., Gao, W., Wu, Y., et al. (2014). Sugar and auxin signaling pathways respond to high-temperature stress during anther development as revealed by transcript profiling analysis in cotton. Plant Physiol. 164, 1293–1308. doi: 10.1104/pp.113.232314
Ni, L., Shi, W. (2014). Composition and free radical scavenging activity of kernel oil from Torreya grandis, Carya cathayensis and Myrica rubra. Int. J. Pharm. Res. 13, 221–226. doi: 10.9758/cpn.2013.11.3.168
Pandey, P., Irulappan, V., Bagavathiannan, M. V., Senthil-Kumar, M. (2017). Impact of combined abiotic and biotic stresses on plant growth and avenues for crop improvement by exploiting physio-morphological traits. Front. Plant Sci. 8. doi: 10.3389/fpls.2017.00537
Panoli, A., Martin, M. V., Alandete-Saez, M., Simon, M., Neff, C., Swarup, R., et al. (2015). Auxin import and local auxin biosynthesis are required for mitotic divisions, cell expansion and cell specification during female gametophyte development in Arabidopsis thaliana. PloS One 10, e0126164. doi: 10.1371/journal.pone.0126164
Peleg, Z., Blumwald, E. (2011). Hormone balance and abiotic stress tolerance in crop plants. Curr. Opin. Plant Biol. 14, 290–295. doi: 10.1016/j.pbi.2011.02.001
Péret, B., Swarup, K., Ferguson, A., Seth, M., Yang, Y., Dhondt, S., et al. (2012). AUX/LAX genes encode a family of auxin influx transporters that perform distinct functions during Arabidopsis development. Plant Cell 24, 2874–2885. doi: 10.1105/tpc.112.097766
Petrášek, J., Friml, J. (2009). Auxin transport routes in plant development. Development 136, 2675–2688. doi: 10.1242/dev.030353
Qiu, L., Jiang, B., Fang, J., Shen, Y., Fang, Z., Saravana Kumar, R. M., et al. (2016). Analysis of transcriptome in hickory (Carya cathayensis), and uncover the dynamics in the hormonal signaling pathway during graft process. BMC Genomics 17, 1–13. doi: 10.1186/s12864-016-3182-4
Rahman, A. (2013). Auxin : a regulator of cold stress response. Physiol. Plant 147, 28–35. doi: 10.1111/j.1399-3054.2012.01617.x
Ramegowda, V., Senthil-Kumar, M. (2015). The interactive effects of simultaneous biotic and abiotic stresses on plants: Mechanistic understanding from drought and pathogen combination. J. Plant Physiol. 176, 47–54. doi: 10.1016/j.jplph.2014.11.008
Robert, H. S., Grunewald, W., Sauer, M., Cannoot, B., Soriano, M., Swarup, R., et al. (2015). Plant embryogenesis requires aux/lax-mediated auxin influx. Dev. 142, 702–711. doi: 10.1242/dev.115832
Samadi, S., Asgari Lajayer, B., Moghiseh, E., Rodríguez-Couto, S. (2021). Effect of carbon nanomaterials on cell toxicity, biomass production, nutritional and active compound accumulation in plants. Environ. Technol. Innov. 21, 101323. doi: 10.1016/j.eti.2020.101323
Saravana Kumar, R. M., Gao, L. X., Yuan, H. W., Xu, D.B., Liang, Z., Tao, S. C., et al. (2018). Auxin enhances grafting success in Carya cathayensis (Chinese hickory). Planta 247, 761–772. doi: 10.1007/s00425-017-2824-3
Schmittgen, T. D., Livak, K. J. (2008). Analyzing real-time PCR data by the comparative CT method. Nat. Protoc. 3, 1101–1108. doi: 10.1038/nprot.2008.73
Sharma, A., Wang, J., Xu, D., Tao, S., Chong, S., Yan, D., et al. (2020). Melatonin regulates the functional components of photosynthesis, antioxidant system, gene expression, and metabolic pathways to induce drought resistance in grafted Carya cathayensis plants. Sci. Total Environ. 713, 136675. doi: 10.1016/j.scitotenv.2020.136675
Sharma, A., Zheng, B. (2019). Molecular responses during plant grafting and its regulation by auxins, cytokinins, and gibberellins. Biomolecules 9, 397. doi: 10.3390/biom9090397
Swarup, K., Benková, E., Swarup, R., Casimiro, I., Péret, B., Yang, Y., et al. (2008). The auxin influx carrier LAX3 promotes lateral root emergence. Nat. Cell Biol. 10, 1475–1476. doi: 10.1038/ncb1754
Swarup, R., Friml, J., Marchant, A., Ljung, K., Sandberg, G., Palme, K., et al. (2001). Localization of the auxin permease AUX1 suggests two functionally distinct hormone transport pathways operate in the Arabidopsis root apex. Genes Dev. 15, 2648–2653. doi: 10.1101/gad.210501
Swarup, R., Péret, B. (2012). AUX/LAX family of auxin influx carriers — an overview. Front. Plant Sci. 3:1475–1476. doi: 10.3389/fpls.2012.00225
Teale, W., Palme, K. (2018). Naphthylphthalamic acid and the mechanism of polar auxin transport. J. Exp. Bot. 69, 303–312. doi: 10.1093/jxb/erx323
Ugartechea-Chirino, Y., Swarup, R., Swarup, K., Péret, B., Whitworth, M., Bennett, M., et al. (2010). The AUX1 LAX family of auxin influx carriers is required for the establishment of embryonic root cell organization in Arabidopsis thaliana. Ann. Bot. 105, 277–289. doi: 10.1093/aob/mcp287
Ung, K. L., Winkler, M., Schulz, L., Kolb, M., Janacek, D. P., Dedic, E., et al. (2022). Structures and mechanism of the plant PIN-FORMED auxin transporter. Nature 609, 605–610. doi: 10.1038/s41586-022-04883-y
Wu, W., Bi, X. L., Cao, J. Q., Zhang, K. Q., Zhao, Y. Q. (2012). New antitumor compounds from carya cathayensis. Bioorg. Med. Chem. Lett. 22, 1895–1898. doi: 10.1016/j.bmcl.2012.01.062
Wulf, K. E., Reid, J. B., Foo, E. (2019). Auxin transport and stem vascular reconnection - has our thinking become canalized? Ann. Bot. 123, 429–439. doi: 10.1093/aob/mcy180
Xi, Z., Lu, D., Liu, L., Ge, H. (2016). Detection of drought-induced hickory disturbances in western Lin an county, China, using multitemporal landsat imagery. Remote Sens. 8:1493–1494. doi: 10.3390/rs8040345
Xu, D., Yang, Y., Tao, S., Wang, Y., Yuan, H., Sharma, A., et al. (2020). Identification and expression analysis of auxin-responsive GH3 family genes in Chinese hickory (Carya cathayensis) during grafting. Mol. Biol. Rep. 47, 4495–4506. doi: 10.1007/s11033-020-05529-w
Xu, D., Yuan, H., Tong, Y., Zhao, L., Qiu, L., Guo, W., et al. (2017). Comparative proteomic analysis of the graft unions in hickory (Carya cathayensis) provides insights into response mechanisms to grafting process. Front. Plant Sci. 8. doi: 10.3389/fpls.2017.00676
Yang, Y., Huang, Q., Wang, X., Mei, J., Sharma, A., Tripathi, D. K., et al. (2021). Genome-wide identification and expression profiles of ABCB gene family in Chinese hickory (Carya cathayensis sarg.) during grafting. Plant Physiol. Biochem. 168, 477–487. doi: 10.1016/j.plaphy.2021.10.029
Yuan, H., Zhao, L., Chen, J., Yang, Y., Xu, D., Tao, S., et al. (2018). Identification and expression profiling of the Aux/IAA gene family in Chinese hickory (Carya cathayensis sarg.) during the grafting process. Plant Physiol. Biochem. 127, 55–63. doi: 10.1016/j.plaphy.2018.03.010
Yu, C., Dong, W., Zhan, Y., Huang, Z.-A., Li, Z., Kim, I. S., et al. (2017). Genome-wide identification and expression analysis of ClLAX, ClPIN and ClABCB genes families in Citrullus lanatus under various abiotic stresses and grafting. BMC Genet. 18, 1–15. doi: 10.1186/s12863-017-0500-z
Yue, R., Tie, S., Sun, T., Zhang, L., Yang, Y., Qi, J., et al. (2015). Genome-wide identification and expression profiling analysis of ZmPIN,ZmPILS,ZmLAX and ZmABCB auxin transporter gene families in maize (Zea mays l.) under various abiotic stresses. PloS One 10, 1–23. doi: 10.1371/journal.pone.0118751
Zhang, C., Dong, W., Huang, Z. A., Cho, M., Yu, Q., Wu, C., et al. (2018). Genome-wide identification and expression analysis of the CaLAX and CaPIN gene families in pepper (Capsicum annuum l.) under various abiotic stresses and hormone treatments. Genome 61, 121–130. doi: 10.1139/gen-2017-0163
Zhang, J., Jiao, Y., Sharma, A., Shen, D., Wei, B., Hong, C., et al. (2021). Transcriptomic analysis reveals potential pathways associated with salt resistance in pecan (Carya illinoensis K. Koch). J. Biotechnol. 330, 17–26. doi: 10.1016/j.jbiotec.2021.02.001
Zhang, Q., Li, J., Zhang, W., Yan, S., Wang, R., Zhao, J., et al. (2012). The putative auxin efflux carrier OsPIN3t is involved in the drought stress response and drought tolerance. Plant J. 72, 805–816. doi: 10.1111/j.1365-313X.2012.05121.x
Zhao, H., Ma, T., Wang, X., Deng, Y., Ma, H., Zhang, R., et al. (2015). OsAUX1 controls lateral root initiation in rice (Oryza sativa l.). Plant Cell Environ. 38, 2208–2222. doi: 10.1111/pce.12467
Keywords: auxin, AUX/LAX, Carya cathayensis, drought, grafting, salt
Citation: Yang Y, Wang J, Xu Y, Abbas F, Xu D, Tao S, Xie X, Song F, Huang Q, Sharma A, Zheng L, Yan D, Wang X, Zheng B, Yuan H, Wu R and He Y (2023) Genome-wide identification and expression analysis of AUX/LAX family genes in Chinese hickory (Carya cathayensis Sarg.) Under various abiotic stresses and grafting. Front. Plant Sci. 13:1060965. doi: 10.3389/fpls.2022.1060965
Received: 04 October 2022; Accepted: 09 December 2022;
Published: 05 January 2023.
Edited by:
Tanveer Alam Khan, Leibniz Institute of Plant Genetics and Crop Plant Research (IPK), GermanyReviewed by:
Aqeel Ahmad, University of Florida, United StatesBehnam Asgari Lajayer, University of Tabriz, Iran
Om Prakash Narayan, Tufts University, United States
Copyright © 2023 Yang, Wang, Xu, Abbas, Xu, Tao, Xie, Song, Huang, Sharma, Zheng, Yan, Wang, Zheng, Yuan, Wu and He. This is an open-access article distributed under the terms of the Creative Commons Attribution License (CC BY). The use, distribution or reproduction in other forums is permitted, provided the original author(s) and the copyright owner(s) are credited and that the original publication in this journal is cited, in accordance with accepted academic practice. No use, distribution or reproduction is permitted which does not comply with these terms.
*Correspondence: Yi He, aGV5aUB6anUuZWR1LmNu; Rongling Wu, cnd1QHBocy5wc3UuZWR1; Huwei Yuan, aHd5dWFuQHphZnUuZWR1LmNu
†These authors have contributed equally to this work