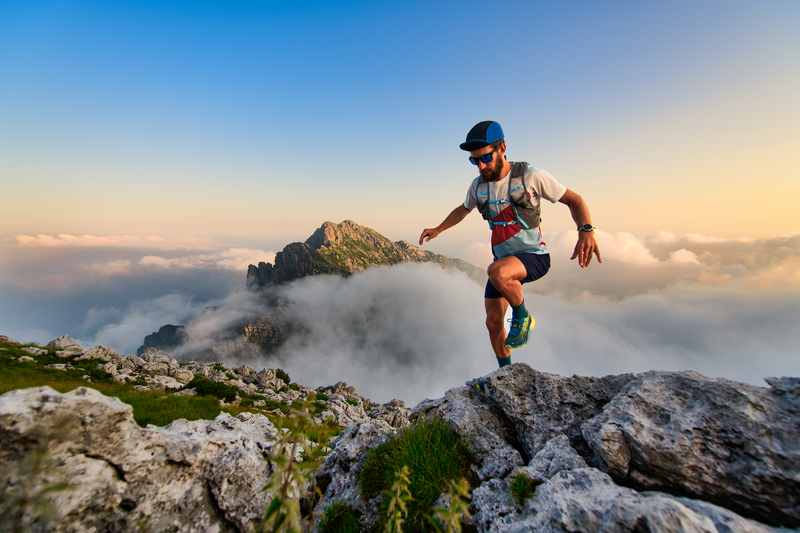
95% of researchers rate our articles as excellent or good
Learn more about the work of our research integrity team to safeguard the quality of each article we publish.
Find out more
REVIEW article
Front. Plant Sci. , 06 December 2022
Sec. Plant Symbiotic Interactions
Volume 13 - 2022 | https://doi.org/10.3389/fpls.2022.1046685
This article is part of the Research Topic Through Thick and Thin - The Army of Secondary Metabolites in Plant-Fungi Interactions View all 8 articles
Volatile organic compounds (VOCs), a bouquet of chemical compounds released by all life forms, play essential roles in trophic interactions. VOCs can facilitate a large number of interactions with different organisms belowground. VOCs-regulated plant-plant or plant-insect interaction both below and aboveground has been reported extensively. Nevertheless, there is little information about the role of VOCs derived from soilborne pathogenic fungi and beneficial fungi, particularly mycorrhizae, in influencing plant performance. In this review, we show how plant VOCs regulate plant-soilborne pathogenic fungi and beneficial fungi (mycorrhizae) interactions. How fungal VOCs mediate plant–soilborne pathogenic and beneficial fungi interactions are presented and the most common methods to collect and analyze belowground volatiles are evaluated. Furthermore, we suggest a promising method for future research on belowground VOCs.
Volatile organic compounds (VOCs) are a mixture of low molecular-weight compounds originating from different types of organisms (Maffei et al., 2011). Under biotic (insects, beneficial fungi, pathogenic fungi, bacteria) and abiotic (heat, drought, UV radiation) stresses, plants often release complex VOC bouquets. Plant VOCs are essential in communication between plants and other organisms (Dudareva et al., 2006), which has been demonstrated in the laboratory and in agricultural systems (Kessler and Baldwin, 2001; Baldwin et al., 2002; Turlings and Erb, 2018). In previous research, volatiles emitted from microorganisms such as bacteria and fungi have been investigated less than VOCs emitted from plants (Effmert et al., 2012; Junker and Tholl, 2013; Weisskopf, 2013; Penuelas et al., 2014).
Microbial VOCs are released by microorganisms such as bacteria and beneficial and pathogenic fungi (Korpi et al., 2009; Thorn and Greenman, 2012). Volatile organic compound profiles can be substantially altered by pathogen-derived VOCs, and can therefore function as biomarkers for detection, differentiation, and characterization or even forecast of early infections (Li et al., 2019; Hamow et al., 2021). More than 100 bacteria and fungi produce soil microbial VOCs (Effmert et al., 2012), and approximately 250 fungal VOCs have been described (Morath et al., 2012; Roze et al., 2012). Plants can perceive microbial VOCs from a distance and prime plant responses to microorganisms (Bailly and Weisskopf, 2012; Effmert et al., 2012; Bitas et al., 2013; Schmidt et al., 2015). Microbial VOCs can potentially mediate plant–microbe interactions (Moisan et al., 2020a; Moisan et al., 2020b; Xu et al., 2021). Microbial VOCs can diffuse through the soil environment and potentially affect plant growth and defense (Piechulla et al., 2017; Tyagi et al., 2018). Bacterial VOCs can increase plant growth and trigger systemic resistance and also influence motility and antibiotic resistance in other bacteria (Ryu et al., 2003; Ryu et al., 2004; Lee et al., 2012; D’Alessandro et al., 2014; Park et al., 2015). Similarly, VOCs emitted by pathogenic and beneficial microorganisms can promote plant growth (Velásquez et al., 2020b), and microbial volatiles can improve plant tolerance and sustain plant growth (Liu and Zhang, 2015; Jalali et al., 2017; Camarena-Pozos et al., 2019).
Volatile organic compounds can facilitate many interactions between below- and aboveground organisms (Schulz and Dickschat, 2007; Das et al., 2012; Junker and Tholl, 2013). Compared with aboveground VOCs, belowground VOCs are challenging to evaluate because of the nonhomogeneous soil environment. The difficulty results in technical limitations in collecting volatiles (Tholl et al., 2021). Since the first investigation of Baldwin and Schultz (1983), VOC-regulated plant–plant or plant–insect interactions both below and aboveground have been investigated extensively (Bruce et al., 2005; Baldwin et al., 2006; Kegge and Pierik, 2010; Clavijo McCormick et al., 2012; Effah et al., 2019; Effah et al., 2022). However, much less known about roles of VOCs originating from soilborne pathogenic and beneficial fungi, particularly mycorrhizae, in affecting plant performance. In addition, how exposure to fungal VOCs affects plant resistance or tolerance to aboveground and belowground herbivory has not been addressed. This review shows (1) how plant VOCs mediate plant–soilborne pathogenic and beneficial fungi (mycorrhizae) interactions; (2) how fungal VOCs modulate plant–soilborne pathogenic and beneficial fungi interactions and (3) we describe the most common methods to collect and analyze belowground volatiles and a promising method for future research on belowground VOCs is introduced.
Because of negative effects of chemical use in plant protection, analyzing production patterns of VOCs in root tissues is increasingly important because of potential VOC roles in belowground biotic interactions, particularly those with fungal pathogens. Numbers of root VOCs that have been identified and investigated have increased in recent years. In 2015, relatively few root volatiles (39 compounds) were known in maize, barley, bean (Vicia faba), and Arabidopsis thaliana (reviewed by Schenkel et al., 2015). However, hundreds more volatile compounds emitted by roots of diverse plant species have since been reported (Cordovez et al., 2017; Schenkel et al., 2018; Moisan et al., 2019). With the model plant A. thaliana, the focus has been on different functions of root volatiles in invasive and noninvasive conditions (Casarrubia et al., 2016; Cordovez et al., 2017; Schenkel et al., 2018; Moisan et al., 2019). Volatile organic compounds of many other plants have also been investigated. Volatile organic compounds in Solanaceae, including pepper (Capsicum annuum) (Kihika et al., 2017) and tomato (Kihika et al., 2017; Kirwa et al., 2018); Brassicaceae, including Brassica rapa (Moisan et al., 2020); and the cucurbit family, including cucumbers Cucumis metuliferus CM3 and line Xintaimici in relation to Meloidogyne incognita (Xie et al., 2022), as well as those in non-cultivated plants, including spotted knapweed Centaurea stoebe (Gfeller et al., 2019), have been scanned and investigated for antifungal activity or ability to increase plant defense against pathogens and herbivores. These root VOCs have been grouped into 15 biosynthetic origins/chemical classes in Figure 1 (Table S1). Because of limited pesticide use for the control of fungal pathogens, some antifungal VOCs may be promising control agents. However, antimicrobial activity of VOCs can vary with origin, dose, and application form, and possible phytotoxic effects and effects on human health of antifungal VOCs need to be investigated in order to develop effective and safe biocontrol strategies (Kaddes et al., 2019a).
Figure 1 Diversity of plant root VOCs, 155 volatile compounds from different plants collected from 2016 to 2022.
Volatile organic compounds are classified into different chemical groups depending on plant species, genotype, sex, development stage (Table 1 and Table S1) (Schenkel et al., 2015; Delory et al., 2016a; Delory et al., 2016b; Kihika et al., 2017; Kindlovits et al., 2018; Murungi et al., 2018; Xie et al., 2022). One of the most common groups is terpenoids, which include the sesquiterpenes (E)‐β‐caryophyllene, daucadiene, (E)‐α‐bergamotene, humulene, (E)‐β‐farnesene, and three putative petasitene isomers (petasitene 1–3) and the monoterpenes α‐pinene and β‐myrcene (Gfeller et al., 2019; Gulati et al., 2020). In Achillea collina, active volatile constituents included alismol, (E)-β-farnesene, β-sesquiphellandrene and neryl esters, heptadecen-7-one, albene and β-pinene, linoleic acid, 2,4,6-decatrienoic acid piperideide, sterols, and some triterpenes (Kindlovits et al., 2018). Other major groups of root volatiles include aldehydes, alcohols, n-alkanes, and ketones. Following strong mechanical injury in barley plants at each developmental stage, the four main volatile aldehydes were characterized and included hexanal, (E)-hex-2-enal, (E)-non-2-enal, and (E,Z)-nona-2,6-dienal (Delory et al., 2016a). The volatile organic compounds released by roots vary depending on the biotic stress agent that is causing damage to the plant. Tomato roots infected by Fusarium oxysporum emit VOCs such as benzonitrile, benzothiazol, dimethyl trisulfide, and formic acid, which have antifungal activities, and a terpene-like compound, which activates antagonistic response; whereas healthy tomato plants release n-alkanes, beclomethasone dipropionate, p-cymene, decanal, and 3-carene, which are compounds without antimicrobial activity or special role (Gulati et al., 2020). Belowground VOCs can affect root-associated microbes, including belowground fungal pathogens. Antimicrobial VOCs biosynthesized in natural hosts are typically at low levels, but the substantial antagonistic activity is promising. Contact-independent antagonisms by VOCs indicate potential for a single application with uniform exposure. Such exposure decreases the likelihood of unaffected host microbial refugia being re-colonized by pathogens after dissipation or degradation of an inhibitory compound (Gabriel et al., 2018). Black pepper-associated bacterium Pseudomonas putida BP25 was isolated from a root endosphere, and endophytic colonization by PpBP25 protected black pepper, ginger, and Arabidopsis against Phytophthora capsici, Pythium myriotylum, Giberella moniliformis, Rhizoctonia solani, Athelia rolfsii, Colletotrichum gloeosporioides, and the plant parasitic nematode Radopholus similis because of the release of volatile substances such as pyrazine derivatives (Sheoran et al., 2015). Pyrazine derivates such as 2,5-dimethyl pyrazine, 2-methyl pyrazine, dimethyl trisulfide, 2-ethyl 5-methyl pyrazine, and 2-ethyl 3,6-dimethyl pyrazine have in vitro inhibitory activity against the oomycete pathogens Ph. capsici and P. myriotylum; the fungal pathogens R. solani, C. gloeosporioides, A. rolfsii, G. moniliformis, and Magnaporthe oryzae; the bacterial pathogen Ralstonia pseudosolanacearum; and the plant parasitic nematode Radopholus similis. (Kihika et al., 2017; Murungi et al., 2018) In addition to pyrazine derivatives, the VOC dimethyltrisulfide of BP25 exhibits soil fumigant activity against Ph. capsici; R. solani, A. rolfsii, C. gloeosporioides, and G. moniliformis; and R. similis (Agisha et al., 2019). Thus, P. putida BP25 and its VOCs have promise as applications for eco-friendly disease management in sustainable agriculture. Botrytis cinerea, a necrotrophic fungus with a wide range of hosts, is extremely sensitive to monoterpenes, such as (+)-limonene, in in vitro applications (Simas et al., 2017), which inhibit fungal mycelial growth and spore germination. The eight-carbon oxylipin 1-octen-3-ol is the primary factor suppressing conidia germination and mycelial growth of Aspergillus nidulans (Herrero-Garcia et al., 2011).
Two organic esters (methyl pro-2-enoate and methyl propanoate) suppress mycelial growth of the fungi Fusarium culmorum and Cochliobolus sativus when in direct contact, whereas with indirect contact, the VOCs cause a decrease in the outflow of K+ ions into the intracellular medium and an increase in the permeability of pathogenic spore membranes (Kaddes et al., 2019b). Because activity of proton pumps must guarantee the efflux of H+ ions into the intracellular medium to retain electrical charges on either side of the membrane at equilibration in order to adjust for K+ imbalance, dramatic changes can occur in the pH of the intracellular medium and prohibit spore germination (Kaddes et al., 2019b). Therefore, roles of root volatiles in regulating belowground microbiomes via effects on microbial communities and attraction of beneficial microbial species have been the focus of research. Despite the bright prospects to control fungal diseases, applications of belowground volatiles in sustainable agriculture need to be investigated further (Sharifi et al., 2022).
Pathogenic fungi obtain nutrients by either feeding on living host plant cells (biotrophic pathogens) or killing cells (necrotrophic pathogens). Notably, volatile compounds emitted by pathogenic fungi are different from those emitted by plant roots (Schenkel et al., 2015; Gulati et al., 2020). Among pathogen genera, fungal volatile compounds have been characterized for many species (Fiers et al., 2013; Casarrubia et al., 2016; Werner et al., 2016; Cordovez et al., 2017; Cordovez et al., 2018; Martín-Sánchez et al., 2020; Moisan et al., 2020). Pathogen-produced VOCs have low chemical diversity and are most likely used as info-chemicals or chemical stimuli to attract or repel interacting organisms (Gulati et al., 2020). Pathogenic fungi and the volatiles emitted by such fungi negatively affect plant growth. Fungal volatile compounds such as 1-octen-3-ol, 2-phenylethanol, 3-methyl-1-butanol, 1-hexanol, 3-octanol, 3-octanone, and trans-2-octenal (Table 2) are classified as phytotoxic (Werner et al., 2016). The compound 1-octen-3-ol represses root growth and causes cotyledon bleaching of A. thaliana seedlings at low concentrations via H2O2 production (Splivallo et al., 2007) and impairs seed germination (Lee et al., 2014). Volatiles emitted by the belowground fungal pathogens Serratia plymuthica and F. culmorum affect root and shoot growth of maize by limiting the availability of micronutrients such as Fe, Zn, Cu, and Mo (Table 2) in roots (Martín-Sánchez et al., 2020). The fungus F. acuminatum releases volatiles into soil that prohibit growth and decrease shoot length and root surface area and biomass in tomato (Gulati et al., 2020). The fungi F. culmorum and Cochliobolus sativus produce VOCs that decrease leaf surface area and mean root length in barley (Fiers et al., 2013). Volatiles emitted by the pathogenic fungi R. solani and F. oxysporum f. sp. raphani decrease the root growth rate of B. rapa seedlings (Moisan et al., 2021).
In addition to negative effects on plants, many pathogenic fungal VOCs are growth manipulators, because the VOCs affect plant architecture and increase growth (Werner et al., 2016). Many of those VOCs are alcohols, pyrones, phenols, sesquiterpenes, ketones, and aldehydes, which can affect plant growth and architecture (Casarrubia et al., 2016; Cordovez et al., 2018; Fincheira and Quiroz, 2018; Moisan et al., 2019). Volatile organic compounds emitted by the fungal root pathogen R. solani promote in vitro growth of early developmental stages of A. thaliana by altering root architecture and increasing root biomass (Cordovez et al., 2017) and increase root growth of B. rapa (Moisan et al., 2020a). Increases in growth can benefit pathogens by enlarging the habitat for pathogenic colonization of surfaces and survival (Cordovez et al., 2017; Moisan et al., 2019; Moisan et al., 2020). Fungal volatiles emitted by R. solani and Phoma leveillei stimulate not only plant growth but also flowering by accelerating plant bolting and bud and flower production, which improves reproductive success (Moisan et al., 2019). The ability of fungal pathogens to modulate plant growth via VOCs is likely widespread, because VOCs act as an “alert” signal to plants, which accelerate growth by upregulating genes involved in auxin or cytokinin signaling while downregulating genes involved in ethylene or jasmonic acid signaling (Sanchez-Lopez et al., 2016; Cordovez et al., 2017; Martínez-Medina et al., 2017b; Li and Kang, 2018).
Fungal VOCs increase plant protection by inducing host defense systems and resistance against pathogens via different mechanisms (Werner et al., 2016). One important mechanism is to change the balance of K+ ions flow and disturb the pH gradient, which inhibits fungal mycelial growth and spore germination (Kaddes et al., 2019b). Naphthalene and monoterpenes (p-cymene, 3-carene) produced in tomato roots in response to F. oxysporum have antibacterial effects (Gulati et al., 2020). Plant VOC emission profiles can also change after infection with a fungal pathogen, leading to chemical protection in plants and preventing further fungal pathogen colonization or attracting specific beneficial microorganisms with antifungal properties (Schulz-Bohm et al., 2017; Gulati et al., 2020).
Notably, soilborne pathogenic fungi can improve plant resistance to above- and belowground herbivory. Resistance to the cabbage root fly Delia radicum (Diptera: Anthomyiidae) and the large cabbage white butterfly Pieris brassicae (Lepidoptera: Pieridae) increase with exposure to volatiles of the pathogenic fungi R. solani, F. oxysporum, Ulocladium atrum, and P. leveillei (Moisan et al., 2019). Fungal VOCs also negatively affected D. radicum development rate and P. brassicae caterpillar performance (Cordovez et al., 2017; Moisan et al., 2019; Moisan et al., 2020b). Exposure of roots to fungal VOCs can alter primary and lateral root architecture, which leads to changes in plant chemistry and morphological characteristics and negatively affects the performance of root herbivores by delaying insect growth and accumulation of body mass (Ditengou et al., 2015; Casarrubia et al., 2016). Fungal VOCs can also promote the accumulation of glucosinolates in leaves or main roots, which diminishes leaf caterpillar performance or slows larval development (Aziz et al., 2016). In addition, volatiles produced by soilborne fungi can affect nematode development and behavior. Volatiles emitted by some F. oxysporum strains inhibit egg hatch in the root-knot nematode Meloidogyne incognita and slow development of the cyst nematode Heterodera schachtii (Terra et al., 2018; Moisan et al., 2021). Thus, volatiles from soilborne fungi not only negatively affect or modulate plant growth but also diffuse through the soil matrix to help plants attract disease antagonists or natural enemies for defense.
Arbuscular mycorrhizal fungi (AMF) in the phylum Glomeromycotina are ubiquitous soil microorganisms and obligate root symbionts inhabiting almost all terrestrial ecosystems. The AMF establish symbiotic associations with approximately 80% of vascular plants and with approximately 90% of agricultural plants (Smith and Read, 2008). In the mutualistic association, the fungal partner receives up to 20% of total photosynthates (Allen et al., 2003) and lipids (Bravo et al., 2017) from the host, whereas the plant increases mineral nutrient and water uptake through mycorrhizal hyphae networks (Smith and Read, 2008). Arbuscular mycorrhizal fungi account for 5% to 36% of total soil biomass and 9% to 55% of soil microbial biomass (Olson et al., 1999), and 1 gram of soil contains 10 to 100 m of mycorrhizal hyphae (Gilbert and Johnson, 2017). Mycorrhizal fungal symbionts are important in environmental ecology and agricultural ecosystems, because AMF are ubiquitous and are involved in nutrient cycles (Azcon-Aguilar and Miguel-Barea, 2015).
Establishing arbuscular mycorrhizae (AM) involves a sequence of genetically controlled phases that commences with pre-symbiotic molecular crosstalk resulting in reciprocal perception, followed by host root cells proceeding with considerable functional and structural alterations to accommodate the fungi (Choi et al., 2018). Level of nutrient availability is determinant in establishment and development of mycorrhizal symbiosis. In the mutualistic association, fungi and plants perceive one another via interacting molecular signals (Bonfante and Genre, 2015). Before physical contact, strigolactones released from host roots in response to inorganic phosphorus starvation induce AM spore germination, hyphae production, and branching to physically contact roots (Ho-Plágaro and García-Garrido, 2022). Moreover, flavonoids, 2-hydroxy fatty acids, polyamines, and cutin monomers are among the active plant compounds influencing hyphal elongation or branching (Becard et al., 1992; Ghachtouli et al., 1995; Akiyama et al., 2005; Nagahashi and Douds, 2011; Wang et al., 2012; Gutjahr and Parniske, 2013). The AM hyphopodium penetrates into roots and forms arbuscles where nutrients and photosynthates are exchanged (Nadal and Paszkowski, 2013). The AMF develop extensive extraradical hyphal networks in soil, which affect other organisms and root physiology as well as pattern of root exudation.
Although important advances in understanding the molecular regulation of AM symbiosis have been achieved (Ho-Plágaro and García-Garrido, 2022), there is little information on VOCs during mycorrhization. Sun et al. (2015) showed that germinating spores of the AMF Gigaspora margarita emit unidentified volatiles, which increase density and number of lateral roots in A. thaliana (non-host plant for AMF) and Lotus japonicus (Table 2). Fungi also modulate host root orientation by releasing sporal VOCs that alter the branch angle of lateral roots, thereby increasing the chances of AM hyphae contacting roots in the rhizosphere (Sun et al., 2015). Because auxins regulate the branch angle of lateral roots, VOCs can trigger the auxin signaling pathway in plants (Roychoudhry et al., 2013). Expression profiles of genes associated with AM establishment and lateral root formation in L. japonicus indicate that the gene LjCCD7, an important component of the strigolactone synthesis pathway, is stimulated by fungal VOC signals (Sun et al., 2015). Mycorrhizal VOCs increase strigolactone biosynthesis and root proliferation, and secretion of such VOCs in the rhizosphere facilitates AM hyphal identification of roots and increases root colonization. Plant hormones and root volatiles and exudates are important factors modulating interactions between host plants and AMF. Exogenous abscisic acid (ABA) application to mother spores of Rhizophagus irregularis substantially increases daughter spore production, and hairy root volatiles considerably increase pre-symbiotic sporulation (Liu et al., 2019). Plants can distinguish between symbiotic and pathogenic interactions in the early stages of colonization and respond by releasing different root VOCs depending on whether the colonization is beneficial or pathogenic (Dreher et al., 2019). Indeed, 93 VOCs exhibit differential responses in Medicago truncatula roots treated by the root pathogen Aphanomyces euteiches or the mycorrhizal fungus R. irregularis. Several VOCs are released specifically in response to R. irregularis, such as limonene, which could be a result of the action of specific receptors on plasma membranes (Dreher et al., 2019). Therefore, specific receptors for AMF activate the common symbiotic signaling pathway for symbiotic interactions (Parniske, 2008).
Ectomycorrhizal (EM) symbiosis is another very common mycorrhiza–plant interaction. Many ectomycorrhizal ascomycetes and basidiomycetes form symbioses with approximately 6000 tree species, including beeches, dipterocarps, eucalypts, oaks, pines, and poplars (Brundrett, 2002; Van Der Heijden et al., 2015). In contrast to arbuscular mycorrhizae, ectomycorrhizae support hosts by generating hyphal networks (known as the Hartig net) that surround epidermal cells of emerging lateral roots (Smith and Read, 2010; Tedersoo et al., 2010). A complex signaling dialogue between host plant and fungus is necessary to establish EM. Menotta et al. (2004) showed that 29 volatile compounds, including alcohols, aldehydes, and ketones, are produced during the interaction between the host plant Tilia americana and Tuber borchii at the pre-symbiotic stage of EM establishment. Terpenoids such as 1-pentanol, 2,3-dimethyldecane, and p-isopropylbenzaldehyde—which are the most diffusible compounds in soil (Hiltpold and Turlings, 2008)—are involved in the interaction and therefore may be good candidates for belowground signaling during plant–EM interactions. Indeed, Ditengou et al. (2015) found that those volatiles have roles in the pre-symbiotic communication between roots of a Populus host and the fungus Laccaria bicolor. The sequiterpene thujopsene generated by the fungus increases Populus lateral root formation and root hair length in the pre-symbiotic phase and thus facilitates EM establishment. In addition, thujopsene induces the formation of superoxide anion radicals in the meristematic zone of root tips, whereas the prohibition of fungal sesquiterpene synthesis by lovastatin decreases lateral root formation. Recently, the EM fungus Tricholoma vaccinum was found to release geosmin (Abdulsalam et al., 2021), which improves sporulation (Bentley and Meganathan, 1981) and spore germination in AMF (Carpenter-Boggs et al., 1995). Therefore, geosmin may be also important in EM formation.
The extensive hyphal network that develops during mycorrhizal colonization alters root morphology and architecture (Schellenbaum et al., 1991; Norman et al., 1996) and increases soil biological activity by what is called the “mycorrhizosphere effect” (Linderman, 1988). Thus, the mycorrhizosphere effect affects soil microbial communities. Mycorrhization causes changes in components of root exudates and therefore shapes soil microbial communities (Badri and Vivanco, 2009). The antifungal activities of mycorrhizal root exudates also promote disease resistance. Zhang et al. (2012) showed that Glomus versiforme changes the exudation pattern of cotton roots and contributes to bioactive effects on Verticillium dahliae conidial germination. Similarly, direct antibiotic activity of exudates originating from tomato roots colonized by AMF toward F. oxysporum f. sp. lycopersici has been observed, with nonvolatile citrate and chlorogenic acid as the antifungal substances (Hage-Ahmed et al., 2013). Zhang et al. (2018) demonstrated that R. irregularis symbionts emit undefined volatile compounds that directly suppress growth and extension of fungal pathogens such as F. oxysporum, F. graminearum, V. dahliae, and Rhizoctonia solani. Furthermore, Tricholoma vaccinum (EM fungus) produces VOCs that include the monoterpene limonene and the sesquiterpene β-barbatene, which have antimicrobial properties (Abdulsalam et al., 2021). Notably, plants in natural communities participate in shared or common mycorrhizal networks that enlarge areas accessed by root systems and allow linkages with other plants (Johnson and Gilbert, 2015). In forests, trillions of mycorrhizal rootlets from various forest trees are interconnected by hyphae of different EM fungal species to form extraradicular mycorrhizal networks or wood-wide webs (Selosse et al., 2006; Klein et al., 2016). Molecules likely transported by mycorrhizal networks include small RNAs, hormones or hormone metabolites, ions, peptides, allelochemicals, and particularly defense signals that prime plant resistance to pathogens and herbivores (Johnson and Gilbert, 2015; Hettenhausen et al., 2017; Song et al., 2019; Alaux et al., 2020).
Remarkably, mycorrhizal symbiosis changes plant hormonal homeostasis (Ho-Plágaro and García-Garrido, 2022), because phytohormones are involved in transient plant defense responses essential for homeostatic establishment between AMF and host. Plant hormones can also regulate VOC production in plants. Ethylene, influenced by mycorrhizal colonization (Ludwig-Müller, 2010), not only functions as a phytohormone to modulate volatile biosynthesis but is also emitted into the rhizosphere as a VOC (Chen et al., 2020). Ethylene released from roots or in soil treatments increases the diversity of soil microbes by increasing the number of keystone taxa, such as Pseudolabrys spp. (Alphaproteobacteria), Dokdonella spp. (Gammaproteobacteria), and Catenulispora spp. (Actinobacteria), which leads to changes in soil microbiomes because of changes in production of antibiotics or microbial growth stimulators (Chen et al., 2020). Ethylene also modulates VOC biosynthesis and emissions in plants. For example, ethylene inhibits VOC biosynthesis in potato (Dawood et al., 2014) and rice (Mujiono et al., 2020) subjected to flooding stress. Activation of the salicylic acid (SA) signaling pathway modifies root exudate profiles, which affects soil microbiomes (Martínez-Medina et al., 2017a; Jacoby et al., 2020). Arbuscular mycorrhizal colonization can decrease SA contents in plants, whereas the colonization rate can be inhibited by constitutive SA biosynthesis (Medina et al., 2003). Plant roots can emit large quantities of SA. The SA is dispersed over several centimeters and is transformed into its volatile derivatives, which then alter the structure of microbial communities (Dehimeche et al., 2021; Kong et al., 2021). Notably, Pons et al. (2020) found that phytohormones including cytokinin (isopentenyl adenosine), an auxin (indole-acetic acid), gibberellin (gibberellin A4), and ethylene are also produced by the AM fungus Rhizophagus irregularis. Similarly, the EM fungus Tricholoma vaccinum emits ethylene and excretes ABA, SA, jasmonates, and indole-3-acetid acid (Abdulsalam et al., 2021). Thus, root VOC emissions are affected by the mycorrhizosphere effect and mycorrhiza-induced changes in phytohormone homeostasis during colonization from the first stage to later stages. In addition, during colonization, mycorrhizal volatiles are released with broad-spectrum and long-term fungistatic efficacy (Zhang et al., 2018). Overall, common mycorrhizal networks (AMF) and wood-wide webs (EM fungi) shape microbiomes in the mycorrhizosphere.
Arsenic (As) is a prevalent toxic element in natural surroundings and is also used in various industries. The enormous anthropogenic discharge has led to As accumulation in the environment, particularly in waters and soils, seriously threatening crop cultivation and human health in recent decades (Zhao and Wang, 2020). Notably, Li et al. (2021) identified an AM association associated with As volatilization. In in vitro cultivation with intact Medicago sativa plants colonized by R. irregularis, the AM symbiosis methylates and volatilizes inorganic As to a variety of organic forms, including dimethylarsinic acid, dimethylarsine, and trimethylarsine, modulated by RiMT-11 (a gene of R. irregularis encoding the methyltransferase type 11 protein). As a result, the AM symbiosis increased host plant tolerance to As stress. In the process of detoxification, methylation can produce trivalent methylarsenites as intermediate products, which are even more toxic than inorganic As (Li et al., 2021). The methyltransferase evolved to generate extremely toxic trivalent As species that acted as antibiotics to destroy competitors in the primitive anaerobic earth (Chen et al., 2017). As atmospheric oxygen levels increased, the trivalent forms were oxidized to pentavalent methylarsenicals, which converted methylation into a process of detoxification. Because AMF are very ancient symbiotic fungi, whether As methylation capability originally evolved to benefit both plants and AMF by eliminating pathogenic microbes remains a topic of great interest.
Mycorrhizae affect concentrations and composition of root VOCs in various plant species such as Sorghum bicolor (Sun and Tang, 2013), Medicago truncatula (Dreher et al., 2019), and Vitis vinifera (Velásquez et al., 2020a). In addition, mycorrhizal effects on VOC production differ depending on the AMF species (Sun and Tang, 2013; Volpe et al., 2018) and the plant species (Meier and Hunter, 2019). The mycorrhizal fungus genus Glomus may increase biotic stress tolerance in various plants attacked by herbivores (Dowarah et al., 2021).
Methyl salicylate synthesized from SA modulates plant defenses to environmental stresses and disease resistance by stimulating defensive compound production or by activating SA-signaling defense (Raskin, 1992). Mycorrhiza-induced increases in methyl salicylate may be important, because it is a volatile that is an elicitor linked with induced resistance to plant diseases and has been used in plant protection (Tang et al., 2015; Kalaivani et al., 2016). Methyl salicylate can control some aphid species and prevents attacks on host plants (Sasso et al., 2007; Babikova et al., 2014). Under drought stress and aphid infestation, increases in methyl salicylate levels in mycorrhizal plants led to greater attraction of the aphid parasitoid Aphidius ervi than that in nonmycorrhizal ones (Volpe et al., 2018).
In addition to methyl salicylate, mycorrhizal colonization increases other volatiles associated with plant defenses against pathogen/herbivore attack or drought stress, including benzaldehyde, geraniol, (E)–2–hexenal, and 3–hexenal in grapevine plants (Velásquez et al., 2020b). Application of C6–volatiles, such as (E)– 2–hexenal, leads to increased resistance against the necrotrophic fungus B. cinerea (gray mold) in Arabidopsis by suppressing fungal development and triggering the plant defense response (Shiojiri et al., 2006). Benzaldehyde is a VOC with nematicidal and antifungal properties (Shaukat et al., 2005), and geraniol is associated with plant defense, triggering apoptosis–like cell death, with fragmentation of nuclei and DNA (Izumi et al., 1999). Geraniol may be in high concentrations in various plant organs because it is a precursor for diverse monoterpenes (D’Onofrio et al., 2016), whereas benzenic compounds are the major components of some essential oils and are linked to plant defense and reproduction (Dudareva et al., 2004; Carvajal et al., 2016).
D-Limonene, p-xylene, and 1,3-diethylbenzene increase in Elymus nutans plants colonized with AMF (Zhang et al., 2022). Under insect attack, 1,3-diethylbenzene and D-limonene can be generated to strengthen plant defenses and repel insects (Agut et al., 2015; Kigathi et al., 2019; Mitra et al., 2021). The compound p-xylene is primarily an attractant to natural enemies of herbivorous insects (Li et al., 2022). Levels of monoterpenes increase substantially in Funneliformis mosseae-colonized roots of grapevine (Velásquez et al., 2020a). Terpenoids are important in above- and belowground tritrophic interactions, because terpenoids attract parasitoids and predators of herbivorous insects (Palma et al., 2012; Penuelas et al., 2014). Notably, there are increases in monoterpene alcohols associated with plant defense, such as p-mentha-1.8-dien-7-ol, myrtenol, and p-cymen-7-ol. Monoterpenes of p-menthane are widely dispersed in plants and are major components of various essential oils and plant extracts with biological activity in plant defense (Lange, 2015). Terpenoids are also associated with function and formation of mycorrhizal symbiosis. Terpenoid accumulation is observed in AM roots of many plants, particularly in root cortical cells with collapsed arbuscules (Akiyama and Hayashi, 2002; Fester et al., 2002). In addition, mycorrhization causes a sharp increase in transcripts of two early enzymes (DXS and DXR) of the 2-methyl-D-erythritol-4-phosphate pathway in host roots (Walter et al., 2000; Hans et al., 2004). Nevertheless, mechanisms associated with fluxes of volatile terpenoids with different roles in mycorrhizal symbiosis remain unknown (Kapoor et al., 2017).
Volatile organic compounds are typically released as a blend of compounds that is diluted in the environment around a plant. In recent years, evidence increasingly shows that belowground plant VOCs have important roles in trophic interactions. Although advances have been made in techniques to sample aboveground VOCs (Raguso and Pellmyr, 1998; Tholl et al., 2006; Penuelas et al., 2014; Materic et al., 2015), sampling belowground volatiles is more difficult because of the nonhomogeneous trapping environment (van Dam et al., 2016; Tholl et al., 2021; Sharifi et al., 2022).
Understanding the roles of belowground VOCs in belowground communication networks is attracting increased attention (van Doan et al., 2021). It is necessary to invest in advanced methodology and instrumentation to capture and fully analyze belowground VOCs (Sharifi et al., 2022). Currently, most research on root VOCs uses ground root material with the caveat that the total profile of volatiles in root tissue was analyzed rather than emissions of volatiles (Gfeller et al., 2019). However, the approach can detect chemicals that may be not induced by major root damage (Tholl et al., 2021). In this section, we describe the most challenges in sampling belowground volatiles and the recent progress in sampling and analyzing belowground VOCs; we further describe a promising experimental design for future research on belowground VOCs.
Compared with volatiles in relatively homogeneous aboveground environments, belowground VOCs are a mixture of volatiles from plant roots, bacteria, fungi, parasites, herbivores, and predators (Delory et al., 2016a; van Dam et al., 2016). Therefore, it is difficult to evaluate the exact effects of specific original VOCs on trophic interactions. It may be necessary to distinguish among origins of VOCs when the goal is to assess effects of fungal VOCs on plant–root–insect interactions. Effects of fungal VOCs may be synergistic or antagonistic, depending on whether VOCs originate from roots, insects, or other soilborne organisms. In addition, belowground VOCs are not only diluted by the surrounding environment (Turlings et al., 2012; Tang et al., 2019; Ehlers et al., 2020; Erktan et al., 2020; Wester-Larsen et al., 2020) but also depend on microbes such as those producing and consuming VOCs (Raza et al., 2016; Bier et al., 2017; Schenkel et al., 2018; Abis et al., 2020; Gutiérrez-Santa et al., 2020). Therefore, a sampling method suitable to collect targeted belowground VOCs is required that can overcome the challenges of a nonhomogeneous environment (van Dam et al., 2016; Tholl et al., 2021).
The method used to sample belowground VOCs strongly depends on the research purpose. However, there are two main approaches to analyze root volatiles. Volatile organic compounds are measured either directly in the soil matrix or after root excavation, extraction, and analysis under lab conditions. In this review, the aim was to explain the most applicable methods to directly collect belowground volatiles in the soil matrix (Gfeller et al., 2019; van Doan et al., 2021; Tholl et al., 2021), and describe a detailed comparison between the approaches (Table 3). There are two approaches to measure VOCs, with one method a dynamic “push–pull” system and the other a passive method using solid-phase microextraction (SPME) (Tholl et al., 2021).
Table 3 Advantages and disadvantages of dynamic and passive methods to collect volatile organic compounds (VOCs) in belowground environments.
The technique using a “push–pull” system collects all belowground VOCs, including those emitted from roots, soilborne organisms, and soil matrix), by using clean-air flow through the belowground system, with VOCs trapped by a Super-Q filter (Figure 2) (Hiltpold et al., 2011; van Doan et al., 2021). Briefly, spherical pots are connected to multiple air delivery systems, and volatiles are trapped on Super-Q filters (25 mg of Super-Q adsorbent, 80–100 mesh; Alltech Assoc., Deerfield, IL, USA). Cleaned humidified air is pushed through the system at a rate of 1 L min-1 and pulled through Super-Q traps at a rate of 0.7 L min-1. After collection, Super-Q filters are rinsed with 150 μL of dichloromethane. N-octane and nonyl-acetate (Sigma, Buchs, Switzerland) are added as internal standards (200 ng in 10 μL of dichloromethane). Root volatiles are analyzed by a gas chromatograph coupled to a mass spectrometer (GC-MS, Agilent 7820A GC coupled to an Agilent 5977E MS; Agilent Technologies, Santa Clara, CA, USA). Putative volatile identification is obtained by comparing mass spectra with those of the NIST05 Mass Spectra Library (van Doan et al., 2021). Other sorbent materials are also frequently used in dynamic approaches to trap VOCs and are summarized by Tholl et al. (2006); however, Tenax TA and Carbopack B are used even in passive methods to sample belowground VOCs (Martín-Sánchez et al., 2020).
Figure 2 Volatile organic compound (VOC) emissions from roots, soilborne organisms, and soil matrix are collected by a push–pull system. The VOCs are trapped by a Super-Q trap.
Of possible passive sampling methods, a less complex system is one in which an SPME fiber (coated with 100-μm polydimethylsiloxane; Supelco, Bellefonte, PA, USA) is inserted into a gap of a pot and exposed to belowground VOCs for 60 min at room temperature and then transferred to another pot for 60 min to collect VOCs (Figure 3). An incubated fiber is immediately analyzed by GC-MS using an Agilent 7820A GC interfaced with an Agilent 5977E MSD. Volatile organic compounds are tentatively identified by comparing mass spectra to library entries of the National Institute of Standards and Technology (NIST 14) and an external standard library (Gfeller et al., 2019). The advantage of such a system is that all emerging VOCs from the belowground mixture are collected, and thus, most VOCs are generated by targeted organisms. However, using SPME is at best a semi-quantitative approach, and depending on VOC composition, different SPME fibers should be tested because of differences in fiber affinity for classes of VOC compounds. In addition, extraction times and temperatures are important and need to be optimized, because high temperatures and long extraction times cause desorption of VOCs that have relatively low fiber affinity or low boiling point from an SPME fiber during extraction.
Figure 3 Volatile organic compound (VOC) emissions from roots, soilborne organisms, and soil matrix are collected by a passive system. VOCs are trapped by a solid phase micro extraction (SPME) fiber. Polytetrafluoroethylene (PTFE).
To understand the mechanisms of belowground VOCs in ecosystems, we need to measure the belowground VOCs directly measured in a certain time. Danner et al. (2012; 2015) directly measured VOCs released from root herbivore damage in cuvettes on the top of the soil at the stem and root interface. Acton et al. (2018) measured VOCs by using airflow generated in a root glass chamber filled with a potting substrate. All belowground VOCs emitted to the environment in a certain time can be measured by proton transfer reaction mass spectrometry (PTR-MS) (Majchrzak et al., 2018; Tholl et al., 2021; Sharifi et al., 2022).
The PTR-MS method also has disadvantages, because it characterizes only mass-to-charge ratio (m/z) of VOCs and not the exact molecular identity. In addition, one molecular formula may represent different structures, which cannot be discriminated by PTR-MS. Some small-chain alkanes are also not detected by the technique. Therefore, the PTR-MS method is generally used simultaneously with GC-MS to determine chemical identities of volatiles from the m/z data (Sharifi et al., 2022).
The challenge with passive and dynamic methods is in collecting the many different original belowground VOCs and establishing emission origins. To meet the challenge, a new experimental setup and methods can be optimized to minimize the disadvantages of the two approaches (Figure 4). An experimental system can be set up in which both sampling approaches are used simultaneously, and different treatments or events (blank pot with soil only, healthy plant, plant exposed to fungi, plant exposed to belowground herbivore, plant exposed to fungi and belowground herbivore) are used to compare differences in VOCs. After comparison and subtraction of VOC patterns of different events, emission origins and abundance of VOCs can be established. Sharifi et al. (2022) presented an in-situ design suitable for sampling belowground VOCs that used a perforated polytetrafluoroethylene (PTFE) tube exposed to communities of plant roots and soil microorganisms. The tube is placed in a pot before sowing seeds to avoid disturbing the soil and rhizosphere when belowground VOCs are collected. Belowground VOCs are collected by an SPME syringe extracted via a network of tubing. To separate the original VOCs, an experimental setup is suggested according to Sharifi et al. (2022) that can continuously sample different treatments or events by SPME fibers inserted into PTFE tube systems. After collection of VOCs, SPME fibers are analyzed in a cyclic manner by GC-MS or GCxGC-TOF-MS. The tube systems can also be sampled by a dynamic approach in which different treatments are connected by motor rotation switch valves to a PTR-MS. With this approach, SPME collections obtained by frequent static sampling cycles can provide a good approximation of real-time resolution in emissions of individual VOCs, in addition to VOC composition and abundance. The PTR-MS can characterize actual real-time continual emissions of different events. After subtraction and comparison of different events, VOC origins and emissions can be characterized on the basis of the combined sampling and analysis methods to yield a highly accurate approximation of VOC patterns and emission origins (Figure 4).
Figure 4 Schematic illustration of an in-situ design to collect and analyze belowground volatile organic compounds (VOCs) by a combined technique. Original VOCs are distinguished by subtracting chromatographic peaks of certain events.
Volatile organic compounds emitted by plant roots and pathogenic and beneficial fungi, particularly mycorrhizal fungi, can shape trophic interactions in belowground systems. Fungal VOCs mediate plant growth, metabolites, and consequences of interactions between insects, pathogens, and plants. In this review, an approach using combined methods is proposed to collect VOCs and analyze the effect of each originated VOC in real-time. With the approach, the effect of each originated VOC on belowground trophic interactions can be precisely evaluated. Because of the essential roles of VOCs in inter- and intraspecific communication, using VOCs of certain fungal species may be a promising and sustainable way to reduce the incidence of diseases derived from soilborne phytopathogens. In addition, using fungal VOCs to increase plant tolerance against abiotic stresses is an area for future research with great potential. Despite various reports on interactions between belowground VOCs derived from fungi and plants and root VOCs and fungi that result in benefits for one or both partners, the actual mechanisms involved remain unknown. Therefore, the molecular mechanisms responsible for volatile production by VOC producers (plants and fungi, including fungal symbionts), perception by VOC receivers, and genetic reprogramming of VOC receivers need to be investigated further. Moreover, there are few reports on VOCs during mycorrhization, which should be a research area with great potential interest because of the importance of AMF in agriculture and ecosystems. In addition, most knowledge on VOC emissions by fungi is based on single strains under laboratory conditions, which can differ from rhizospheric conditions with complex microbial communities. Therefore, to facilitate practical VOC application, inoculated strains should be integrated into complex rhizosphere communities in order to mimic the natural conditions in soil.
ND: conceptualization, outline, writing abstract, mycorrhiza section, and conclusion, edit, and revisions. HV: conceptualization, outline, writing VOC pathogen section, and format. CD: conceptualization, outline, and writing introduction and method section. KH: writing methods and revisions. KL: conceptualization, outline, and revisions. KP: conceptualization, outline, revisions, edit, and corresponding author. All authors contributed to the article and approved the submitted version.
This work was supported by the project “Investigation of using beneficial microbes to control some main pathogens on Solanaceae family”, Ministry of Science and Technology of Vietnam. This work was also funded by grant RRF-2.3.1-21-2022-00007, and by the industrial research and development projects in Hungarian–Vietnamese cooperation, grant number 2019-2.1.12-TÉT_VN-2020-00001.
The authors declare that the research was conducted in the absence of any commercial or financial relationships that could be construed as a potential conflict of interest.
All claims expressed in this article are solely those of the authors and do not necessarily represent those of their affiliated organizations, or those of the publisher, the editors and the reviewers. Any product that may be evaluated in this article, or claim that may be made by its manufacturer, is not guaranteed or endorsed by the publisher.
The Supplementary Material for this article can be found online at: https://www.frontiersin.org/articles/10.3389/fpls.2022.1046685/full#supplementary-material
Abdulsalam, O., Wagner, K., Wirth, S., Kunert, M., David, A., Kallenbach, M., et al. (2021). Phytohormones and volatile organic compounds, like geosmin, in the ectomycorrhiza of tricholoma vaccinum and Norway spruce (Picea abies). Mycorrhiza 31 (2), 173–188. doi: 10.1007/s00572-020-01005-2
Abis, L., Loubet, B., Ciuraru, R., Lafouge, F., Houot, S., Nowak, V., et al. (2020). Reduced microbial diversity induces larger volatile organic compound emissions from soils. Sci. Rep. 10, 6104. doi: 10.1038/s41598-020-63091-8
Acton, W. J. F., Jud, W., Ghirardo, A., Wohlfahrt, G., Hewitt, C. N., Taylor, et al. (2018). The effect of ozone fumigation on the biogenic volatile organic compounds (BVOCs) emitted from brassica napus above- and below-ground. PloS One 13, e0208825. doi: 10.1371/journal.pone.0208825
Agisha, V. N., Kumar, A., Eapen, S. J., Sheoran, N., Suseelabhai, R. (2019). Broad-spectrum antimicrobial activity of volatile organic compounds from endophytic Pseudomonas putida BP25 against diverse plant pathogens. Biocontrol Sci. Technol., 1–21. doi: 10.1080/09583157.2019.1657067
Agut, B., Gamir, J., Jaques, J. A., Flors, V. (2015). Tetranychus urticae-triggered responses promote genotype-dependent conspecific repellence or attractiveness in citrus. N. Phytol. 207, 790–804. doi: 10.1111/nph.13357
Akiyama, K., Hayashi, H. (2002). Arbuscular mycorrhizal fungus-promoted accumulation of two new triterpenoids in cucumber roots. Biosci. Biotechnol. Biochem. 66, 762–769. doi: 10.1271/bbb.66.762
Akiyama, K., Matsuzaki, K., Hayashi, H. (2005). Plant sesquiterpenes induce hyphal branching in arbuscular mycorrhizal fungi. Nature 435, 824e827. doi: 10.1038/Nature03608
Alaux, P. L., Naveau, F., Declerck, S., Cranenbrouck, S. (2020). Common mycorrhizal network induced JA/ET genes expression in healthy potato plants connected to potato plants infected by Phytophthora infestans. Front. Plant Sci. 11, 602. doi: 10.3389/fpls.2020.00602
Allen, M. F., Swenson, W., Querejeta, J. I., Egerton-Warburton, L. M., Treseder, K. K. (2003). Ecology of mycorrhizae: A conceptual framework for complex interactions among plants and fungi. Annu. Rev. Phytopathol. 41, 271–303. doi: 10.1146/annurev.phyto.41.052002.095518
Azcon-Aguilar, C., Miguel-Barea, J. (2015). Nutrient cycling in the mycorrhizosphere. J. Soil Sci. Plant Nutr. 15, 372–396. doi: 10.4067/S0718-95162015005000035
Aziz, M., Nadipalli, R. K., Xie, X., Sun, Y., Surowiec, K., Zhang, J. L., et al. (2016). Augmenting sulfur metabolism and herbivore defense in arabidopsis by bacterial volatile signaling. Front. Plant Sci. 7. doi: 10.3389/fpls.2016.00458
Babikova, Z., Gilbert, L., Bruce, T., Dewhirst, S. Y., Pickett, J. A., Johnson, et al. (2014). Arbuscular mycorrhizal fungi and aphids interact by changing host plant quality and volatile emission. Funct. Ecol. 28, 375–385. doi: 10.1111/1365-2435.12181
Badri, D. V., Vivanco, J. M. (2009). Regulation and function of root exudates. Plant Cell Environ. 32 (6), 666–681. doi: 10.1111/j.1365-3040.2009.01926.x
Bailly, A., Weisskopf, L. (2012). The modulating effect of bacterial volatiles on plant growth: current knowledge and future challenges. Plant Signal. Behav. 7, 79–85. doi: 10.4161/psb.7.1.18418
Baldwin, I. T., Schultz, J. C. (1983). Rapid changes in tree leaf chemistry induced by damage: evidence for communication between plants. Science (New York, N.Y.) 221: 277–279.
Baldwin, I. T., Halitschke, R., Paschold, A., Von Dahl, C. C., Preston, C. A. (2006). Volatile signaling in plant–plant interactions: “Talking trees” in the genomics era. Science 311, 812–815. doi: 10.1126/science.1118446
Baldwin, I. T., Kessler, A., Halitschke, R. (2002). Volatile signaling in plant–plant–herbivore interactions: what is real? Curr. Opin. Plant Biol. 5, 351–354. doi: 10.1016/S1369-5266(02)00263-7
Becard, G., Douds, D. D., Pfeffer, P. E. (1992). Extensive in vitro hyphal growth of vesicular-arbuscular mycorrhizal fungi in the presence of CO(2) and flavonols. Appl. Environ. Microbiol. 58, 821e825. doi: 10.1128/aem.58.3.821-825.1992
Bentley, R., Meganathan, R. (1981). Geosmin and methylisoborneol biosynthesis in streptomycetes. evidence for an isoprenoid pathway and its absence in non-differentiating isolates. FEBS Lett. 125 (2), 220–222. doi: 10.1016/0014-5793(81)80723-5
Bier, M. C., Medeiros, A. B., Soccol, C. R. (2017). Biotransformation of limonene by an endophytic fungus using synthetic and orange residue-based media. Fungal Biol. 121, 137–144. doi: 10.1016/j.funbio.2016.11.003
Bitas, V., Kim, H. S., Bennett, J. W., Kang, S. (2013). Sniffing on microbes: diverse roles of microbial volatile organic compounds in plant health. Mol. Plant Microbe Interact. 26, 835–843. doi: 10.1094/MPMI-10-12-0249-CR
Bonfante, P., Genre, A. (2015). Arbuscular mycorrhizal dialogues: do you speak ‘plantish’ or ‘fungish’? Trends Plant Sci. 20, 150–154. doi: 10.1016/j.tplants.2014.12.00
Bravo, A., Brands, M., Wewer, V., Dörmann, P., Harrison, M. J. (2017). Arbuscular mycorrhiza-specific enzymes FatM and RAM2 fine-tune lipid biosynthesis to promote development of arbuscular mycorrhiza. New Phytol. 214 (4), 1631–1645. doi: 10.1111/nph.14533
Bruce, T. J., Wadhams, L. J., Woodcock, C.M. (2005). Insect host location: A volatile situation. Trends Plant Sci. 10, 269–274. doi: 10.1016/j.tplants.2005.04.003
Brundrett, M. C. (2002). Coevolution of roots and mycorrhizas of land plants. New Phytol. 154, 275–304. doi: 10.1046/j.1469-8137.2002.00397.x
Camarena-Pozos, D. A., Flores-Núñez, V. M., López, M. G., López-Bucio, J., Partida-Martínez, L. P. (2019). Smells from the desert: Microbial volatiles that affect plant growth and development of native and non-native plant species. Plant Cell Environ. 42 (4), 1368–1380. doi: 10.1111/pce.13476
Carpenter-Boggs, L., Loynachan, T. E., Stahl, P. D. (1995). Spore germination of Gigaspora margarita stimulated by volatiles of soilisolated actinomycetes. Soil Biol. Biochem. 27, 1445–1451. doi: 10.1016/0038-0717(95)00075-P
Carvajal, M. A., Vergara, A. P., Santander, R., Osorio, M. E. (2016). Chemical composition and anti-phytopathogenic activity of the essential oil of Beilschmiedia miersii. Nat. Prod. Commun. 11, 1367–1372, doi: 10.1177/1934578x1601100945.
Casarrubia, S., Sapienza, S., Fritz, H., Daghino, S., Rosenkranz, M., Schnitzler, J. P., et al. (2016). Ecologically different fungi affect arabidopsis development: Contribution of soluble and volatile compounds. PloS One 11 (12), e0168236. doi: 10.1371/journal.pone.0168236
Chen, Y., Bonkowski, M., Shen, Y., Griffiths, B. S., Jiang, Y., Wang, X., et al. (2020). Root ethylene mediates rhizosphere microbial community reconstruction when chemically detecting cyanide produced by neighbouring plants. Microbiome 8, 4. doi: 10.1186/s40168-019-0775-6
Chen, S. C., Sun, G. X., Rosen, B. P., Zhang, S. Y., Deng, Y., Zhu, B. K., et al. (2017). Recurrent horizontal transfer of arsenite methyltransferase genes facilitated adaptation of life to arsenic. Sci. Rep. 7, 7741. doi: 10.1038/s41598-017-08313-2
Choi, J., Summers, W., Paszkowski, U. (2018). Mechanisms underlying establishment of arbuscular mycorrhizal symbioses. Annu. Rev. Phytopathol. 56, 135–160. doi: 10.1146/annurev-phyto-080516-035521
Clavijo McCormick, A., Unsicker, S. B., Gershenzon, J. (2012). The specificity of herbivore-induced plant volatiles in attracting herbivore enemies. Trends Plant Sci. 17, 303–310. doi: 10.1016/j.tplants.2012.03.012
Cordovez, V., Mommer, L., Moisan, K., Lucas-Barbosa, D., Pierik, R., Mumm, R., et al. (2017). Plant phenotypic and transcriptional changes induced by volatiles from the fungal root pathogen Rhizoctonia solani. Front. Plant Sci. 8. doi: 10.3389/fpls.2017.01262
Cordovez, V., Schop, S., Hordijk, K., de Boulois, H. D., Coppens, F., Hanssen, I., et al. (2018). Priming of plant growth promotion by volatiles of root-associated microbacterium spp. Appl. Environ. Microbiol. 84, e01865-18. doi: 10.1128/AEM.01865-18
D’Alessandro, M., Erb, M., Ton, J., Brandenburg, A., Karlen, D., Zopfi, J. (2014). Volatiles produced by soil-borne endophytic bacteria increase plant pathogen resistance and affect tritrophic interactions. Plant Cell Environ. 37, 813–826. doi: 10.1111/pce.12220
Danner, H., Brown, P., Cator, E. A., Harren, F. M., van Dam, N. M., Cristescu, S. M. (2015). Aboveground and belowground herbivores synergistically induce volatile organic sulfur compound emissions from shoots but not from roots. J. Chem. Ecol. 41, 631–640. doi: 10.1007/s10886-015-0601-y
Danner, H., Samudrala, D., Cristescu, S. M., Van Dam, N. M. (2012). Tracing hidden herbivores: time-resolved non-invasive analysis of belowground volatiles by proton-transfer-reaction mass spectrometry (PTR-MS). J. Chem. Ecol. 38, 785–794. doi: 10.1007/s10886-012-0129-3
Das, A., Lee, S. H., Hyun, T. K., Kim, S. W., Kim, J. Y. (2012). Plant volatiles as method of communication. Plant Biotechnol. Rep. 7, 9–26. doi: 10.1007/s11816-012-0236-1
Dawood, T., Rieu, I., Wolters-Arts, M., Debrksen, E. B., Mariani, C., Visser, E. J. (2014). Rapid flooding-induced adventitious root development from preformed primordia in Solanum dulcamara. AoB Plants 6, plt058. doi: 10.1093/aobpla/plt058
Dehimeche, N., Buatois, B., Bertin, N., Staudt, M. (2021). Insights into the intraspecific variability of the above and belowground emissions of volatile organic compounds in tomato. Molecules 26, 237. doi: 10.3390/molecules26010237
Delory, B. M., Delaplace, P., du Jardin, P., Fauconnier, M. L. (2016a). Barley (Hordeum distichon l.) roots synthesise volatile aldehydes with a strong age-dependent pattern and release (E)-non-2-enal and (E, z)-nona-2,6-dienal after mechanical injury. Plant Physiol. Biochem. 104, 134–145. doi: 10.1016/j.plaphy.2016.03.028
Delory, B. M., Delaplace, P., Fauconnier, M. L., du Jardin, P. (2016b). Root-emitted volatile organic compounds: can they mediate belowground plant-plant interactions? Plant Soil 402, 1–26. doi: 10.1007/s11104-016-2823-3
Ditengou, F. A., Müller, A., Rosenkranz, M., Felten, J., Lasok, H., van Doorn, M. M., et al. (2015). Volatile signalling by sesquiterpenes from ectomycorrhizal fungi reprogrammes root architecture. Nat. Commun. 6 (1), 6279. doi: 10.1038/ncomms7279
D’Onofrio, C., Matarese, F., Cuzzola, A. (2016). Study of the terpene profile at harvest and during berry development of Vitis vinifera l. aromatic varieties aleatico, brachetto, malvasia di candia aromatica and moscato bianco. J. Sci. Food Agric. 97, 2898–2907. doi: 10.1002/jsfa.8126
Dowarah, B., Gill, S. S., Agarwala, N. (2021). Arbuscular mycorrhizal fungi in conferring tolerance to biotic stresses in plants. J. Plant Growth Regul. 110, 999. doi: 10.1007/s00344-021-10392-5
Dreher, D., Baldermann, S., Schreiner, M., Hause, B. (2019). An arbuscular mycorrhizal fungus and a root pathogen induce different volatiles emitted by Medicago truncatula roots. J. Advanced Res. 19, 85–90. doi: 10.1016/j.jare.2019.03.002
Dudareva, N., Negre, F., Nagegowda, D. A., Orlova, I. (2006). Plant volatiles: recent advances and future perspectives. Crit. Rev. Plant Sci 25, 417–440. doi: 10.1080/07352680600899973
Dudareva, N., Pichersky, E., Gershenzon, J. (2004). Biochemistry of plant volatiles. Plant Physiol. 135, 1893–1902. doi: 10.1038/s41598-022-18479-z
Effah, E., Svendsen, L., Barrett, D. P., McCormick, A. C. (2022). Exploring plant volatile-mediated interactions between native and introduced plants and insects. Sci Rep 12, 15450. doi: 10.1038/s41598-022-18479-z
Effah, E., Holopainen, J. K., McCormick, A. C. (2019). Potential roles of volatile organic compounds in plant competition. Perspect. Plant Ecol. Evol. Syst. 38, 58–63. doi: 10.1016/j.ppees.2019.04.003
Effmert, U., Kalderas, J., Warnke, R., Piechulla, B. (2012). Volatile mediated interactions between bacteria and fungi in the soil. J. Chem. Ecol. 38, 665–703. doi: 10.1007/s10886-012-0135-5
Ehlers, B. K., Berg, M. P., Staudt, M., Holmstrup, M., Glasius, M., Ellers, J., et al. (2020). Plant secondary compounds in soil and their role in belowground species interactions. Trends Ecol. Evol. 35, 716–730. doi: 10.1016/j.tree.2020.04.001
Erktan, A., Or, D., Scheu, S. (2020). The physical structure of soil: determinant and consequence of trophic interactions. Soil Biol. Biochem. 148, 107876. doi: 10.1016/j.soilbio.2020.107876
Fester, T., Hause, B., Schmidt, D., Halfmann, K., Schmidt, J., Wray, V., et al. (2002). Occurrence and localization of apocarotenoids in arbuscular mycorrhizal plant roots. Plant Cell Physiol. 43, 256–265. doi: 10.1093/pcp/pcf029
Fiers, M., Lognay, G., Fauconnier, M. L., Jijakli, M. H. (2013). Volatile compound-mediated interactions between barley and pathogenic fungi in the soil. PloS One 8 (6), e66805. doi: 10.1371/journal.pone.0066805
Fincheira, P., Quiroz, A. (2018). Microbial volatiles as plant growth inducers. Microbiol. Res. 208, 63–75. doi: 10.1016/j.micres.2018.01.002
Gabriel, K. T., Sexton, D. J., Cornelison, C. T. (2018). Biomimicry of volatile-based microbial control for managing emerging fungal pathogens. J. Appl. Microbiol. 124, 1024–1031. doi: 10.1111/jam.13667
García-Gómez, P., Bahaji, A., Gámez-Arcas, S., Muñoz, F. José, Sánchez-López, Ángela María, Almagro, G., et al. (2020). Volatiles from the fungal phytopathogen penicillium aurantiogriseum modulate root metabolism and architecture through proteome resetting. Plant Cell environ. 43 (10), 2551–2570. doi: 10.1111/pce.13817
Gfeller, V., Huber, M., Förster, C., Huang, W., Köllner, T. G., Erb., M. (2019). Root volatiles in plant–plant interactions I: High root sesquiterpene release is associated with increased germination and growth of plant neighbours. Plant Cell Environ. 42, 1950–1963. doi: 10.1111/pce.13532
Ghachtouli, N. E., Paynot, M., Martin-Tanguy, J., Morandi, D., Gianinazzi, S. (1995). Effect of polyamines and polyamine biosynthesis inhibitors on spore germination and hyphal growth of Glomus mosseae. Mycol. Res. 100, 597e600. doi: 10.1016/S0953-7562(96)80014-1
Gilbert, L., Johnson, D. (2017). “Plant–plant communication through common mycorrhizal networks,” in Advances in botanical research, vol. 82. (London, UK: Elsevier), 83–97.
Gulati, S., Ballhausen, M. B., Kulkarni, P., Grosch, R., Garbeva, P. (2020). A non-invasive soil-based setup to study tomato root volatiles released by healthy and infected roots. Sci. Rep. 10, 12704. doi: 10.1038/s41598-020-69468-z
Gutiérrez-Santa, A. A., Carrillo-Cerda, H. A., Rodriguez-Campos, J., Velázquez-Fernández, J. B., Patrón-Soberano, O. A., Contreras-Ramos, S. M. (2020). Dynamics of volatilomes emitted during cross-talking of plantgrowth-promoting bacteria and the phytopathogen, Fusarium solani. World J. Microbiol. Biotechnol. 36, 152. doi: 10.1007/s11274-020-02928-w
Gutjahr, C., Parniske, M. (2013). Cell and developmental biology of arbuscular mycorrhiza symbiosis. Annu. Rev. Cell Dev. Biol. 29, 593e617. doi: 10.1146/annurev-cellbio-101512-122413
Hage-Ahmed, K., Moyses, A., Voglgruber, A., Hadacek, F., Steinkellner, S. (2013). Alterations in root exudation of intercropped tomato mediated by the arbuscular mycorrhizal fungus Glomus mosseae and the soilborne pathogen Fusarium oxysporum f.sp. lycopersici. J. Phytopathol. 161, 763–773. doi: 10.1111/jph.12130
Hamow, K. A., Ambrózy, Z., Puskás, K., Majláth, I., Cséplő, M., Mátyus, R., et al. (2021). Emission of novel volatile biomarkers for wheat powdery mildew. Sci. Total Environ. 781, 146767. doi: 10.1016/j.scitotenv.2021.146767
Herrero-Garcia, E., Garzia, A., Cordobés, S., Espeso, E. A., Ugalde, U. (2011). 8-Carbon oxylipins inhibit germination and growth, and stimulate aerial conidiation in Aspergillus nidulans. Fungal Biol. 115, 4-5. 393–400. doi: 10.1016/j.funbio.2011.02.005
Hans, J., Hause, B., Strack, D., Walter, M. H. (2004). Cloning, characterization, and immunolocalization of a mycorrhiza-inducible 1-deoxy-dxylulose 5-phosphate reductoisomerase in arbuscule-containing cells of maize. Plant Physiol. 134, 614–624. doi: 10.1104/pp.103.032342
Hettenhausen, C., Li, J., Zhuang, H., Sun, H., Xu, Y., Qi, J., et al. (2017). Stem parasitic plant Cuscuta australis (dodder) transfers herbivory-induced signals among plants. Proc. Natl. Acad. Sci. U S A. 114, E6703–E6709. doi: 10.1073/pnas.1704536114
Hiltpold, I., Turlings, T. C. J. (2008). Belowground chemical signaling in maize: when simplicity rhymes with efficiency. J. Chem. Ecol. 34, 628–635.
Hiltpold, I., Erb, M., Robert, C. A. M., Turlings, T. C. J. (2011). Systemic root signalling in a belowground, volatile-mediated tritrophic interaction. Plant Cell Environ. 34, 1267–1275. doi: 10.1111/j.1365-3040.2011.02327.x
Ho-Plágaro, T., García-Garrido, J. M. (2022). Molecular regulation of arbuscular mycorrhizal symbiosis. Int. J. Mol. Sci. 23, 5960. doi: 10.3390/ijms23115960
Izumi, S., Takashima, O., Hirata, T. (1999). Geraniol is a potent inducer of apoptosis-like cell death in the cultured shoot primordia of Matricaria chamomilla. Biochem. Biophys. Res. Commun. 259, 519–522. doi: 10.1006/bbrc.1999.0813
Jacoby, R. P., Chen, L., Schwier, M., Koprivova, A., Kopriva, S. (2020). Recent advances in the role of plant metabolites in shaping the root microbiome. F1000Research 9, 151. doi: 10.12688/f1000research.21796.1
Jalali, F., Zafari, D., Salari, H. (2017). Volatile organic compounds of some Trichoderma spp. increase growth and induce salt tolerance in Arabidopsis thaliana. Fungal Ecol. 29, 67–75. doi: 10.1016/j.funeco.2017.06.007
Johnson, D., Gilbert, L. (2015). Interplant signalling through hyphal networks. New Phytol. 205, 1448–1453. doi: 10.1111/nph.13115
Junker, R. R., Tholl, D. (2013). Volatile organic compound mediated interactions at the plant-microbe interface. J. Chem. Ecol. 39, 810–825. doi: 10.1007/s10886-013-0325-9
Ju, Y., Yue, X., Zhao, X., Zhao, H., Fang, Y. (2018). Physiological, micro-morphological and metabolomic analysis of grapevine (Vitis vinifera l.) leaf of plants under water stress. Plant Physiol. Biochem. 130, 501–510. doi: 10.1016/j.plaphy.2018.07.036
Kaddes, A., Fauconnier, M. L., Sassi, K., Nasraoui, B., Jijakli, M. H. (2019a). Endophytic fungal volatile compounds as solution for sustainable agriculture. Molecules 24 (6), 1065. doi: 10.3390/molecules24061065
Kaddes, A., Fauconnier, M. L., Sassi, K., Nasraoui, B., Jijakli, M. H. (2019b). Antifungal properties of two volatile organic compounds on barley pathogens and introduction to their mechanism of action. Int. J. Environ. Res. Public Health 16, 2866. doi: 10.3390/ijerph16162866
Kalaivani, K., Kalaiselvi, M. M., Senthil-Nathan, S. (2016). Effect of methyl salicylate (MeSA), an elicitor on growth, physiology and pathology of resistant and susceptible rice varieties. Sci. Rep. 6, 1–11. doi: 10.1038/srep34498
Kapoor, R., Anand, G., Gupta, P., Mandal, S. (2017). Insight into the mechanisms of enhanced production of valuable terpenoids by arbuscular mycorrhiza. Phytochem Rev 16, 677–692. doi: 10.1007/s11101-016-9486-9
Kegge, W., Pierik, R. (2010). Biogenic volatile organic compounds and plant competition. Trends Plant Sci. 15, 126–132. doi: 10.1016/j.tplants.2009.11.007
Kessler, A., Baldwin, I. T. (2001). Defensive function of herbivore-induced plant volatile emissions in nature. Science 291, 2141–2144. doi: 10.1126/science.291.5511.2141
Kigathi, R. N., Weisser, W. W., Reichelt, M., Gershenzon, J., Unsicker, S. B. (2019). Plant volatile emission depends on the species composition of the neighboring plant community. BMC Plant Biol. 19, 58. doi: 10.1186/s12870-018-1541-9
Kihika, R., Murungi, L. K., Coyne, D., Ng’ang’a, M., Hassanali, A., Teal, P. E. A., et al. (2017). Parasitic nematode Meloidogyne incognita interactions with different capsicum annum cultivars reveal the chemical constituents modulating root herbivory. Sci. Rep. 7 (1), 2903. doi: 10.1038/s41598-017-02379-8
Kindlovits, S., Sárosi, S., Inotai, K., Petrović, G., Stojanović, G., Németh, et al. (2018). Phytochemical characteristics of root volatiles and extracts of achillea collina becker genotypes. J. Essential Oil Res. 30 (5), 330–340. doi: 10.1080/10412905.2018.1470581
Kirwa, H. K., Murungi, L. K., Beck, J. J., Torto, B. (2018). Elicitation of differential responses in the root-knot nematode Meloidogyne incognita to tomato root exudate cytokinin, flavonoids, and alkaloids. J. Agric. Food Chem. 66 (43), 11291–11300 . doi: 10.1021/acs.jafc.8b05101
Klein, T., Siegwolf, R. T. W., Körner, C. (2016). Belowground carbon trade among tall trees in a temperate forest. Science 352, 342–344. doi: 10.1126/science.aad6188
Kong, H. G., Song, G. C., Sim, H. J., Ryu, C. M. (2021). Achieving similar root microbiota composition in neighbouring plants through airborne signalling. ISME J. 15, 397–408. doi: 10.1038/s41396-020-00759-z
Korpi, A., Jarnberg, J., Pasanen, A. L. (2009). Microbial volatile organic compounds. Crit. Rev. Toxicol. 39, 139–193. doi: 10.1080/10408440802291497
Lackus, N. D., Lackner, S., Gershenzon, J., Unsicker, S. B., Köllner, T. G. (2018). The occurrence and formation of monoterpenes in herbivore-damaged poplar roots. Sci. Rep. 8, 17936. doi: 10.1038/s41598-018-36302-6
Lange, B. M. (2015). Biosynthesis and biotechnology of high-value pmenthane monoterpenes, including menthol, carvone, and limonene. Adv. Biochem. Eng. Biotechnol. 148, 319–353. doi: 10.1007/10_2014_289
Lee, B., Farag, M. A., Park, H. B., Kloepper, J. W., Lee, S. H., Ryu, C. M. (2012). Induced resistance by a long-chain bacterial volatile: Elicitation of plant systemic defense by a C13 volatile produced by Paenibacillus polymyxa. PloS One 7, e48744. doi: 10.1371/journal.pone.0048744
Lee, S., Hung, R., Schink, A., Mauro, J., Bennett, J. W. (2014). Arabidopsis thaliana for testing the phytotoxicity of volatile organic compounds. Plant Growth Regul. 74, 177–186. doi: 10.1007/s10725-014-9909-9
Liang, H., Zhang, X., Jun, R. A. O., Huanwen, C. H. E. N. (2008). Microbial volatile organic compounds: generation pathways and mass spectrometric detection. J. Chin. Biotechnol. 28, 124–133.
Li, J., Chen, B., Zhang, X., Hao, Z., Zhang, X., Zhu, Y. (2021). Arsenic transformation and volatilization by arbuscular mycorrhizal symbiosis under axenic conditions. J. Hazard Mater. 413, 125390. doi: 10.1016/j.jhazmat.2021.125390
Li, N., Kang, S. (2018). Do volatile compounds produced by Fusarium oxysporum and Verticillium dahliae affect stress tolerance in plants? Mycology 9, 166–175. doi: 10.1080/21501203.2018.1448009
Linderman, R. G. (1988). Mycorrhizal interactions with the rhizosphere microflora – the mycorrhizosphere effect. Phytopathology 78, 366–371.
Li, Z., Paul, R., Tis, T. B., Saville, A. C., Hansel, J. C., Yu, T., et al. (2019). Non-invasive plant disease diagnostics enabled by smartphone-based fingerprinting of leaf volatiles. Nat. Plants 5, 856–866. doi: 10.1038/s41477-019-0476-y
Liu, X., Feng, Z., Zhu, H. (2019). Exogenous abscisic acid and root volatiles increase sporulation of Rhizophagus irregularis DAOM 197198 in asymbiotic and pre-symbiotic status. Mycorrhiza 29, 581–589. doi: 10.1007/s00572-019-00916-z
Liu, X. M., Zhang, H. (2015). The effects of bacterial volatile emissions on plant abiotic stress tolerance. Front. Plant Sci. 6. doi: 10.3389/fpls.2015.00774
Li, M., Xia, S., Zhang, T., Williams, R. L., Xiao, H. (2022). Volatiles from cotton plants infested by agrotis segetum (Lep.: Noctuidae) attract the larval parasitoid microplitis mediator (Hym.: Braconidae). Plants (Basel) 11, 863. doi: 10.3390/plants11070863
Ludwig-Müller, J. (2010). “Hormonal responses in host plants triggered by arbuscular mycorrhizal fungi,” in Arbuscular mycorrhizas: Physiology and function. Eds. Koltai, H., Kapulnik, Y. (Dordrecht: Springer).
Maffei, M. E., Gertsch, J., Appendino, G. (2011). Plant volatiles: production, function and pharmacology. Nat. Prod. Rep. 28, 1359–1380. doi: 10.1039/c1np00021g
Majchrzak, T., Wojnowski, W., Lubinska, M., Szczygeł, Różańska, A., Namieśnik, J., et al. (2018). PTR-MS and GC-MS as complementary techniques for analysis of volatiles. A tutorial Rev. Analytica Chimica Acta. 1035, 1–13. doi: 10.1016/j.aca.2018.06.056
Martínez-Medina, A., Appels, F. V. W., van Wees, S. C. M. (2017b). Impact of salicylic acid- and jasmonic acid-regulated defences on root colonization by Trichoderma harzianum T-78. Plant Signaling Behav. 12, e1345404. doi: 10.1080/15592324.2017.1345404
Martínez-Medina, A., Van Wees, S. C. M., Pieterse, C. M. J. (2017a). Airborne signals from trichoderma fungi stimulate iron uptake responses in roots resulting in priming of jasmonic acid-dependent defences in shoots of arabidopsis thaliana and Solanum lycopersicum: fungal volatiles trigger iron uptake responses and ISR. Plant Cell Environ. 40, 2691–2705. doi: 10.1111/pce.13016
Martín-Sánchez, L., Cristiana, A., Paolina, G., Gianpiero, V. (2020). Investigating the effect of belowground microbial volatiles on plant nutrient status: perspective and limitations. J. Plant Interact. 2020 15 (1), 188–195. doi: 10.1080/17429145.2020.1776408
Materic, D., Bruhn, D., Turner, C., Morgan, G., Mason, N., Gauci, V. (2015). Methods in plant foliar volatile organic compounds research. Appl. Plant Sci. 3, 1500044. doi: 10.3732/apps.1500044
Medina, J. H., Gagnon, H., Piché, Y., Ocampo, J. A., Garrido, J., Vierheilig, H. (2003). Root colonization by arbuscular mycorrhizal fungi is affected by the salicylic acid content of the plant. Plant Sci. 164, 993–998. doi: 10.1016/S0168-9452(03)00083-9
Meier, A. R., Hunter, M. D. (2019). Mycorrhizae alter constitutive and herbivore-induced volatile emissions by milkweeds. J. Chem. Ecol. 45, 610–625. doi: 10.1007/s10886-019-01080-6
Menotta, M., Gioacchini, A., Amicucci, A., Buffalini, M., Sisti, D., Stocchi, V., et al. (2004). Headspace solidphase microextraction with gas chromatography and mass spectrometry in the investigation of volatile organic compounds in an ectomycorrhizae synthesis system. Rapid Commun. Mass Spectrom 18, 206–210. doi: 10.1002/rcm.1314
Mitra, P., Das, S., Debnath, R., Mobarak, S. H., Barik, A. (2021). Identification of Lathyrus sativus plant volatiles causing behavioral preference of aphis craccivora. Pest Manage. Sci. 77, 285–299. doi: 10.1002/ps.6018
Moisan, K., Aragón, M., Gort, G., Dicke, M., Cordovez, V., Raaijmakers, et al. (2020b). Fungal volatiles influence plant defence against aboveground and belowground herbivory. Funct. Ecol. 34, 2259–2269. doi: 10.1111/1365-2435.13633
Moisan, K., Cordovez, V., van de Zande, E. M., Raaijmakers, J. M., Dicke, M., Lucas-Barbosa, D. (2019). Volatiles of pathogenic and non-pathogenic soil-borne fungi affect plant development and resistance to insects. Oecologia 190, 589–604. doi: 10.1007/s00442-019-04433-w
Moisan, K., Dicke, M., Raaijmakers, J. M., Rachmawati, E., Cordovez, V. (2021). Volatiles from the fungus Fusarium oxysporum affect interactions of brassica rapa plants with root herbivores. Ecol. Entomol. 46, 240–248. doi: 10.1111/een.12956
Moisan, K., Raaijmakers, J. M., Dicke, M., Lucas-Barbosa, D., Cordovez, V. (2020a). Volatiles from soil-borne fungi affect directional growth of roots. Plant Cell Environ. 202144, 339–345. doi: 10.1111/pce.13890
Morath, S. U., Hung, R., Bennett, J. W. (2012). Fungal volatile organic compounds: a review with emphasis on their biotechnological potential. Fungal Biol. Rev. 26, 73–83. doi: 10.1016/j.fbr.2012.07.001
Mujiono, K., Tohi, T., Sobhy, I. S., Hojo, Y., Ho, N. T., Shinya, T., et al. (2020). Ethylene functions as a suppressor of volatile production in rice. J. Exp. Bot. 71, 6491–6511. doi: 10.1093/jxb/eraa341
Murungi, L. K., Kirwa, H., Coyne, D., Teal, P. E. A., Beck, J. J., Torto, B. (2018). Identification of key root volatiles signaling preference of tomato over spinach by the root knot nematode Meloidogyne incognita. J. Agric. Food Chem. 66 (28), 7328–7336. doi: 10.1021/acs.jafc.8b03257
Nadal, M., Paszkowski, U. (2013). Polyphony in the rhizosphere: presymbiotic communication in arbuscular mycorrhizal symbiosis. Curr. Opin. Plant Biol. 16, 473e479. doi: 10.1016/j.pbi.2013.06.005
Nagahashi, G., Douds, D. D. (2011). The effects of hydroxy fatty acids on the hyphal branching of germinated spores of AM fungi. Fungal Biol. UK 115, 351e358. doi: 10.1016/j.funbio.2010.01.006
Norman, J. R., Atkinson, D., Hooker, J. E. (1996). Arbuscular mycorrhizal fungal-induced alteration to root architecture in strawberry and induced resistance to the root pathogen Phytophthora fragariae. Plant Soil 185 (2), 191–198. doi: 10.1007/BF02257524
Olson, P. A., Thingstrub, I., Jakobsen, I., Baath, E. (1999). Estimation of the biomass of arbuscular mycorrhizal fungi in a linseed field. Soil Biol. Biochem. 31 (13), 1879–1887. doi: 10.1016/S0038-0717(99)00119-4
Palma, R., Mutis, A., Manosalva, L., Ceballos, R., Quiroz, A. (2012). Behavioral and electrophysiological responses of Hylastinus obscurus to volatiles released from the roots of Trifolium pratense l. J. Soil Sci. Plant Nutr. 12, 183–193. doi: 10.4067/S0718-95162012000100015
Park, Y. S., Dutta, S., Ann, M., Raaijmakers, J. M., Park, K. (2015). Promotion of plant growth by Pseudomonas fluorescens strain SS101 via novel volatile organic compounds. Biochem. Biophys. Res. Commun. 461, 361–365. doi: 10.1016/j.bbrc.2015.04.039
Parniske, M. (2008). Arbuscular mycorrhiza: the mother of plant root endosymbioses. Nat. Rev. Microbiol. 6, 763–775. doi: 10.1038/nrmicro1987
Penuelas, J., Asensio, D., Tholl, D., Wenke, K., Rosenkranz, M., Piechulla, B. (2014). Biogenic volatile emissions from the soil. Plant Cell Environ. 37, 1866–1891. doi: 10.1111/pce.12340
Piechulla, B., Lemfack, M. C., Kai, M. (2017). Effects of discrete bioactive microbial volatiles on plants and fungi. Plant Cell Environ. 40, 2042–2067. doi: 10.1111/pce.13011
Pons, S., Fournier, S., Chervin, C., Be´card, G., Rochange, S., Frey, et al. (2020). Phytohormone production by the arbuscular mycorrhizal fungus Rhizophagus irregularis. PloS One 15 (10), e0240886. doi: 10.1371/journal.pone.0240886
Raguso, R. A., Pellmyr, O. (1998). Dynamic headspace analysis of floral volatiles: a comparison of methods. Oikos 81, 238–254. doi: 10.2307/3547045
Raskin, I. (1992). Role of salicylic acid in plants. Annu. Rev. Plant Biol. 43, 439–463. doi: 10.1146/annurev.pp.43.060192.002255
Raza, W., Ling, N., Liu, D., Wei, Z., Huang, Q., Shen, Q. (2016). Volatile organic compounds produced by pseudomonas fluorescens WR-1 restrict the growth and virulence traits of ralstonia solanacearum. Microbiol. Res. 192, 103–113. doi: 10.1016/j.micres.2016.05.014
Roychoudhry, S., Del Bianco, M., Kieffer, M. (2013). Auxin controls gravitropic setpoint angle in higher plant lateral branches. Curr. Biol. 23, 1497–1504. doi: 10.1016/j.cub.2013.06.034
Roze, L. V., Beaudry, R. M., Linz, J. E. (2012). Analysis of volatile compounds emitted by filamentous fungi using solid-phase microextraction-gas chromatography/mass spectrometry. Methods Mol. Biol. 944, 133–142. doi: 10.1007/978-1-62703-122-6_9
Ryu, C. M., Farag, M. A., Hu, C. H., Reddy, M. S., Kloepper, J. W., Pare, et al. (2004). Bacterial volatiles induce systemic resistance in arabidopsis. Plant Physiol. 134, 1017–1026. doi: 10.1104/pp.103.026583
Ryu, C. M., Farag, M. A., Hu, C. H., Reddy, M. S., Wei, H. X., Pare, P. W. (2003). Bacterial volatiles promote growth in Arabidopsis. Proc. Natl. Acad. Sci. U.S.A. 100, 4927–4932. doi: 10.1073/pnas.0730845100
Sanchez-Lopez, A. M., Baslam, M., Diego, N. D., Munoz, F. J., Bahaji, A., Almagro, G., et al. (2016). Volatile compounds emitted by diverse phytopathogenic microorganisms promote plant growth and flowering through cytokinin action. Plant Cell Environ. 39, 2592–2608. doi: 10.1111/pce.12759
Sasso, R., Iodice, L., Digilio, M. C., Carretta, A., Ariati, L., Guerrieri, E. (2007). Host-locating response by the aphid parasitoid aphidius ervi to tomato plant volatiles. J. Plant Interact. 2, 175–183. doi: 10.1080/17429140701591951
Schellenbaum, L., Berta, G., Ravolanirina, F., Tisserant, B., Gianinazzi, S., Fitter, A. H. (1991). Influence of endomycorrhizal infection on root morphology in a micropropagated woody plant species (Vitis vinifera l.). Biol. J. Linne Soc. 68 (2), 135–141. doi: 10.1093/oxfordjournals.aob.a088231
Schenkel, D., Lemfack, M. C., Piechulla, B., Splivallo, R. (2015). A meta-analysis approach for assessing the diversity and specificity of belowground root and microbial volatiles. Front. Plant Sci. 6. doi: 10.3389/fpls.2015.00707
Schenkel, D., Maciá-Vicente, J. G., Bissell, A., Splivallo, R. (2018). Fungi indirectly affect plant root architecture by modulating soil volatile organic compounds. Front. Microbiol. 9. doi: 10.3389/fmicb.2018.01847
Schmidt, R., Cordovez, V., De Boer, W., Raaijmakers, J., Garbeva, P. (2015). Volatile affairs in microbial interactions. ISME J. 9, 2329–2335. doi: 10.1038/ismej.2015.42
Schmidt, R. D., Etalo, W., Jager, V., Gerards, S., Zweers, H., Boer, W., et al. (2016). Microbial small talk: volatiles in fungal–bacterial interactions. Front. Microbiol. 6. doi: 10.3389/fmicb.2015.01495
Schulz-Bohm, G. K. S., Hundscheid, M., Melenhorst, J., De Boer, W., Garbeva, P. (2017). Calling from distance: attraction of soil bacteria by plant root volatiles. ISME J 12 (5), 1252–1262. doi: 10.1038/s41396-017-0035-3
Schulz, S., Dickschat, J. S. (2007). Bacterial volatiles: the smell of small organisms. Nat. Prod. Rep. 24, 814–842. doi: 10.1039/b507392h
Selosse, M. A., Richard, F., He, X., Simard, S. W. (2006). Mycorrhizal networks: des liaisons dangereuses? Trends Ecol. Evol. 21, 621–628. doi: 10.1016/j.tree.2006.07.003
Sharifi, R., Jeon, J. S., Ryu, C. M. (2022). Belowground plant–microbe communications via volatile compounds. J. Exp. Bot. 73 (2), 463–486. doi: 10.1093/jxb/erab465
Shaukat, S. S., Siddqui, J. A., Munir, N. (2005). Nematicidal, antifungal and phytotoxic responses of Conyza canadensis. Plant Pathol. J. 4, 61–68. doi: 10.3923/ppj.2005.61.68
Sheoran, N., Nadakkakath, A. V., Munjal, V., Kundu, A., Subaharan, K., Venugopal, V., et al. (2015). Genetic analysis of plant endophytic pseudomonas putida BP25 and chemo-profiling of its antimicrobial volatile organic compounds. Microbiol. Res. 173, 66–78. doi: 10.1016/j.micres.2015.02.001
Shiojiri, K., Kishimoto, K., Ozawa, R., Kugimiya, S., Urashimo, S., Arimura, G., et al. (2006). Changing green leaf volatile biosynthesis in plants: an approach for improving plant resistance against both herbivores and pathogens. Proc. Natl. Acad. Sci. U.S.A. 103, 16672–16676. doi: 10.1073/pnas.0607780103
Simas, D. L. R., Silésia, H. B. M., de Amorim, F. R. V., Goulart, C. S., Alviano, D. S., Alviano, A. J. R., et al. (2017). Citrus species essential oils and their components can inhibit or stimulate fungal growth in fruit. Industrial Crops and Products 98, 108–115. doi: 10.1016/j.indcrop.2017.01.026
Song, Y., Wang, M., Zeng, R., Groten, K., Baldwin, I. T. (2019). Priming and filtering of antiherbivore defences among Nicotiana attenuata plants connected by mycorrhizal networks. Plant Cell Environ. 42, 2945–2961. doi: 10.1111/pce.13626
Splivallo, R., Novero, M., Bertea, C. M., Bossi, S., Bonfante, P. (2007). Truffle volatiles inhibit growth and induce an oxidative burst in Arabidopsis thaliana. New Phytol. 175, 417–424. doi: 10.1111/j.14698137.2007.02141.x
Sun, X. G., Bonfante, P., Tang, M. (2015). Effect of volatiles versus exudates released by germinating spores of Gigaspora margarita on lateral root formation. Plant Physiol. Biochem. 97, 1–10. doi: 10.1016/j.plaphy.2015.09.010
Sun, X. G., Tang, M. (2013). Effect of arbuscular mycorrhizal fungi inoculation on root traits and root volatile organic compound emissions of sorghum bicolor. South Afr. J. Bot. 88, 373–379. doi: 10.1016/j.sajb.2013.09.007
Tang, F., Fu, Y. Y., Ye, J. R. (2015). The effect of methyl salicylate on the induction of direct and indirect plant defense mechanisms in poplar (Populus× euramericana ‘Nanlin 895’). J. Plant Interact. 10, 93–100. doi: 10.1080/17429145.2015.1020024
Tang, J., Schurgers, G., Rinnan, R. (2019). Process understanding of soil BVOC fluxes in natural ecosystems: A review. Rev. Geophysics 57, 966–986. doi: 10.1029/2018RG000634
Tedersoo, L., May, T. W., Smith, M. E. (2010). Ectomycorrhizal lifestyle in fungi: global diversity, distribution, and evolution of phylogenetic lineages. Mycorrhiza 20(4), 217–263. doi: 10.1007/s00572-009-0274-x
Terra, W. C., Campos, V. P., Martins, S. J., Costa, L. S. A. S., da Silva, J. C. P., Barros, A. F., et al. (2018). Volatile organic molecules from Fusarium oxysporum strain 21 with nematicidal activity against Meloidogyne incognita. Crop Prot. 106, 125–131. doi: 10.1016/j.cropro.2017.12.022
Tholl, D., Boland, W., Hansel, A., Loreto, F., Röse, U. S. R., Schnitzler, J. P. (2006). Practical approaches to plant volatile analysis. Plant J. 45, 540–560. doi: 10.1111/j.1365-313X.2005.02612.x
Tholl, D., Hossain, O., Weinhold, A., Röse, U. S. R., Wei, Q. (2021). Trends and applications in plant volatile sampling and analysis. Plant J. 106, 314–325. doi: 10.1111/tpj.15176
Thorn, R. M. S., Greenman, J. (2012). Microbial volatile compounds in health and disease conditions. J. Breath Res. 6, 24001. doi: 10.1088/1752-7155/6/2/024001
Turlings, T. C. J., Erb, M. (2018). Tri-trophic interactions mediated by herbivore-induced plant volatiles: mechanisms, ecological relevance, and application potential. Annu. Rev. Entomol. 63, 433–452. doi: 10.1146/annurev-ento-020117-043507
Turlings, T. C. J., Hiltpold, I., Rasmann, S. (2012). The importance of rootproduced volatiles as foraging cues for entomopathogenic nematodes. Plant Soil 358, 51–60. doi: 10.1007/s11104-012-1295-3
Tyagi, S., Mulla, S. I., Lee, K. J., Chae, J. C., Shukla, P. (2018). VOCs-mediated hormonal signaling and crosstalk with plant growth promoting microbes. Crit. Rev. Biotechnol. 38 (8), 1277–1296. doi: 10.1080/07388551.2018.1472551
van Dam, N. M., Weinhold, A., Garbeva, P. (2016). “Calling in the dark: The role of volatiles for communication in the rhizosphere,” in Deciphering chemical language of plant communication. Eds. Blande, J. D., Glinwood, R. (Cham: Springer International Publishing), 175–210.
Van Der Heijden, M. G. A., Martin, F., Selosse, M. A., Sanders, I. R. (2015). Mycorrhizal ecology and evolution: the past, the present, and the future. New Phytol. 205, 1406–1423. doi: 10.1111/nph.13288
van Doan, C., Züst, T., Maurer, C., Zhang, X., Machado, R. A. R., Mateo, P., et al. (2021). Herbivore-induced plant volatiles mediate defense regulation in maize leaves but not in maize roots. Plant Cell Environ. 44 (8), 2672–2686. doi: 10.1111/pce.14052
Velásquez, A., Valenzuela, M., Carvajal, M., Fiaschi, G., Avio, L., Giovannetti, M., et al. (2020b). The arbuscular mycorrhizal fungus Funneliformis mosseae induces changes and increases the concentration of volatile organic compounds in Vitis vinifera cv. sangiovese leaf tissue. Plant Physiol. Biochem. 155, 437–443. doi: 10.1016/j.plaphy.2020.06.048
Velásquez, A., Vega-Celedón, P., Fiaschi, G., Agnolucci, M., Avio, L., Giovannetti, M., et al. (2020a). Responses of Vitis vinifera cv. Cabernet sauvignon roots to the arbuscular mycorrhizal fungus Funneliformis mosseae and the plant growth-promoting rhizobacterium Ensifer meliloti include changes in volatile organic compounds. Mycorrhiza 30 (1), 161–170. doi: 10.1007/s00572-020-00933-3
Volpe, V., Chitarra, W., Cascone, P., Volpe, M. G., Bartolini, P., Moneti, G., et al. (2018). The association with two different arbuscular mycorrhizal fungi differently affects water stress tolerance in tomato. Front. Plant Sci. 9. doi: 10.3389/fpls.2018.01480
Walter, M. H., Fester, T., Strack, D. (2000). Arbuscular mycorrhizal fungi induce the non-mevalonate methylerythritol phosphate pathway of isoprenoid biosynthesis correlated with accumulation of the ‘yellow pigment’ and other apocarotenoids. Plant J. 21, 571–578. doi: 10.1046/j.1365-313x.2000.00708.x
Wang, E., Schornack, S., Marsh, J. F., Gobbato, E., Schwessinger, B., Eastmond, P., et al. (2012). A common signaling process that promotes mycorrhizal and oomycete colonization of plants. Curr Biol. 22 (23), 2242–6. doi: 10.1016/j.cub.2012.09.043
Weisskopf, L. (2013). “The potential of bacterial volatiles for crop protection against phytophathogenic fungi,” in Microbial pathogens and strategies for combating them: Science, technology and education. Ed. Méndez-Vilas, A. (Badajoz: Formatex Research Center), 1352–1363.
Werner, S., Andrea, P., Nicole, B. (2016). Belowground communication: impacts of volatile organic compounds (VOCs) from soil fungi on other soil-inhabiting organisms. Appl. Microbiol. Biotechnol. 100 (20). doi: 10.1007/s00253-016-7792-1
Wester-Larsen, L., Kramshøj, M., Albers, C. N., Rinnan, R. (2020). Biogenic volatile organic compounds in Arctic soil: a field study of concentrations and variability with vegetation cover. J. Geophysical Research: Biogeosciences 125, e2019JG005551. doi: 10.1029/2019JG005551
Xie, X., Ling, J., Mao, Z., Li, Y., Zhao, J., Yang, Y., et al. (2022). Negative regulation of root-knot nematode parasitic behavior by root-derived volatiles of wild relatives of Cucumis metuliferus CM3. Horticulture Res. 9, uhac051. doi: 10.1093/hr/uhac051
Xu, Y., Tong, Z., Zhang, X., Zhang, X., Luo, Z., Shao, W., et al. (2021). Plant volatile organic compound (E)-2-hexenal facilitates botrytis cinerea infection of fruits by inducing sulfate assimilation. New Phytol. 231, 432–446. doi: 10.1111/nph.17378
Zhang, Q., Gao, X., Ren, Y., Ding, X., Qiu, J., Li, N., et al. (2018). Improvement of Verticillium wilt resistance by applying arbuscular mycorrhizal fungi to a cotton variety with high symbiotic efficiency under field conditions. Int. J. Mol. Sci. 19 (1), 241. doi: 10.3390/ijms19010241
Zhang, G., Raza, W., Wang, X., Ran, W., Shen, Q. (2012). Systemic modification of cotton root exudates induced by arbuscular mycorrhizal fungi and Bacillus vallismortis HJ-5 and their effects on Verticillium wilt disease. Appl. Soil Ecol. 61, 85–91. doi: 10.1016/j.apsoil.2012.02.003
Zhang, W., Yu, L., Han, B., Liu, K., Shao, X. (2022). Mycorrhizal inoculation enhances nutrient absorption and induces insect-resistant defense of Elymus nutans. Front. Plant Sci. 13. doi: 10.3389/fpls.2022.898969
Keywords: belowground volatile organic compounds, arbuscular mycorrhizal symbiosis, ectomycorrhiza, pathogenic fungi, belowground plant-fungi interactions, belowground VOC research methods
Citation: Duc NH, Vo HTN, van Doan C, Hamow KÁ, Le KH and Posta K (2022) Volatile organic compounds shape belowground plant–fungi interactions. Front. Plant Sci. 13:1046685. doi: 10.3389/fpls.2022.1046685
Received: 16 September 2022; Accepted: 09 November 2022;
Published: 06 December 2022.
Edited by:
Pierre-Emmanuel Courty, INRA Centre Dijon Bourgogne Franche-Comté, FranceReviewed by:
Marta Berrocal-Lobo, Polytechnic University of Madrid, SpainCopyright © 2022 Duc, Vo, van Doan, Hamow, Le and Posta. This is an open-access article distributed under the terms of the Creative Commons Attribution License (CC BY). The use, distribution or reproduction in other forums is permitted, provided the original author(s) and the copyright owner(s) are credited and that the original publication in this journal is cited, in accordance with accepted academic practice. No use, distribution or reproduction is permitted which does not comply with these terms.
*Correspondence: Katalin Posta, UG9zdGEuS2F0YWxpbkB1bmktbWF0ZS5odQ==
†These authors have contributed equally to this work
Disclaimer: All claims expressed in this article are solely those of the authors and do not necessarily represent those of their affiliated organizations, or those of the publisher, the editors and the reviewers. Any product that may be evaluated in this article or claim that may be made by its manufacturer is not guaranteed or endorsed by the publisher.
Research integrity at Frontiers
Learn more about the work of our research integrity team to safeguard the quality of each article we publish.