- State Key Laboratory of Cotton Biology, Key Laboratory of Plant Stress Biology, School of Life Sciences, Henan University, Kaifeng, China
Over the course of evolution, plants have developed plasticity to acclimate to environmental stresses such as drought and salt stress. These plant adaptation measures involve the activation of cascades of molecular networks involved in stress perception, signal transduction and the expression of stress related genes. Here, we investigated the role of the plasma membrane-localized transporter of auxin PINFORMED1 (PIN1) in the regulation of pavement cells (PCs) and guard cells (GCs) development under drought and salt stress conditions. The results showed that drought and salt stress treatment affected the development of PCs and GCs. Further analysis identified the different regulation mechanisms of PIN1 in regulating the developmental patterns of PCs and GCs under drought and salt stress conditions. Drought and salt stress also regulated the expression dynamics of PIN1 in pif1/3/4/5 quadruple mutants. Collectively, we revealed that PIN1 plays a crucial role in regulating plant epidermal cells development under drought and salt stress conditions, thus contributing to developmental rebustness and plasticity.
Introduction
As a result of global warming and possible climate anomalies, plants often encounter a high level of biotic and abiotic stresses, which engage them in challenging moments that affect their survival and yield (Ramegowda and Senthil-Kumar, 2015; Wang et al., 2021b). During such conditions, plants tend to fine-tune their developmental process and environmental response via changes in several transcriptional and metabolic programs (Sun et al., 2007; Sun et al., 2010; Meng et al., 2016; Bawa et al., 2019; Guo et al., 2019; Tang et al., 2019; Sun et al., 2020; Li et al., 2021; Zhou et al., 2021; Liu et al., 2022b; Mostofa et al., 2022; Wu et al., 2022a; Yeshi et al., 2022). For example, drought and salt stress regulate plant growth and development (Li et al., 2016; Devkar et al., 2020; Zelm et al., 2020; Zhang et al., 2020; Karimi et al., 2021; Zhang et al., 2022), and a large number of plants respond to drought stress via reducing water loss through stomatal closure (Daszkowska-Golec and Szarejko, 2013; Pirasteh-Anosheh et al., 2016) as well as decreasing rate of leaf expansion under salt stress condition (Rasool et al., 2013; Muchate et al., 2016; Ullah et al., 2020). Drought and salt stress induce osmotic stress, limiting plant normal functions and developmental process, and tolerance to these stresses by plants is of major concern to current agriculture development. Several studies have identified the effects of drought and salt stress on the development and function of plant leaves (Sinha et al., 2016; Ji et al., 2018; Yang and Guo, 2018; López-Serrano et al., 2019; Devkar et al., 2020; Chowdhury et al., 2021). The plant cell wall promotes or impedes plant growth development depending on its mechanical properties, as it often responds to external stimuli, which regulate cell or tissue morphology (Cosgrove, 2017; Sampathkumar, 2020). Many studies have established the link between the development of leaf epidermal cells and leaf morphology (Ferreira et al., 2017; Fujiwara et al., 2018; Zhu et al., 2019). Leaf epidermis plays several key functions including regulating the exchange of gases, water, and nutrients with the surroundings (Zuch et al., 2021). However, the development and function of leaf epidermal cells, such as pavement cells (PCs) and guard cells (GCs), are sometimes disrupted by external environmental factors (Kebbas et al., 2015; Dong et al., 2018; Liu et al., 2022b).
The role of phytohormone’s response to drought and salt stress has been well studied (Ullah et al., 2018; Jalil and Ansari, 2019; Yu et al., 2020; Rehman et al., 2021), among which auxin is a critical hormone (Pandey et al., 2019; Ribba et al., 2020) in the regulation of plant leaf formation (Reinhardt et al., 2003). Interestingly, in the plant epidermal cells, the plasma membrane-localized transporter of auxin PINFORMED1 (PIN1) is the main efflux transporter of auxin that controls the formation and development of leaves and flowers (Reinhardt et al., 2003; Heisler et al., 2005; Heisler et al., 2010). Also, auxin is involved in the regulation of cell polarization, such as the polar distribution of the auxin efflux PIN1 proteins to the plasma membrane and the regulation of root hair initiation areas in the root epidermal cells (Fischer et al., 2006; Dhonukshe et al., 2008); hence it is not surprising that PIN1 regulates the development of leaves in response to auxin signaling (Li et al., 2011; Adamowski and Friml, 2015; Xiong and Jiao, 2019). While the plasticity of plant morphological traits, such as leaf vein pattern development has been linked with varying auxin distribution resulting from changes in PIN1 protein localization (Dhakal et al., 2021), no mechanism has yet been proposed for the role of PIN1 in PCs and GCs development under drought and salt stress conditions. To study the mechanism of epidermal cells development under drought and salt stress, we previously identified the role of PHYTOCHROME INTERACTING FACTOR (PIF) 1, PIF3, PIF4, and PIF5 genes in PCs and GCs development under drought and salt stress conditions using single-cell RNA-seq analysis (Wu et al., 2022b). However, the mechanism underlying the role of PIN1 in PCs and GCs development under drought and salt stress conditions required further exploration. In the current study, we investigated the role of PIN1 in epidermal cells’ response to drought and salt stress. These results demonstrate that PIN1 is critical in regulating the developmental response of PCs and GCs to drought and salt stress.
Materials and methods
Plant materials and growth conditions
Wild type (WT) Arabidopsis (Arabidopsis thaliana) Columbia ecotype (Col-0) was used in this study. The pif1 pif3 pif4 pif5 quadruple mutant (pif1-1, pif3-7, pif4-2, pif5-3), PIN1pro:GUS, pin1-5, and 35S::PIN1 were obtained from the Arabidopsis Biological Resource Center (ABRC). All mutants and WT Arabidopsis were grown in an artificial climate chamber under the growth conditions of 21-23 ℃, 100 μmol photons m-2s-1 (normal light treatment), 16 h light/8 h dark and 60%-70% humidity. For NaCl and Mannitol treatments, the seedlings were grown on 1/2MS medium plates containing 100 mM NaCl or 150 mM Mannitol for 1-7 days.
GUS staining and histological analysis
Histochemical GUS staining was performed as previously described (Liu et al., 2022b). Samples were fixed in 90% acetone at –20°C, rinsed four times with 0.1M sodium phosphate buffer (pH 7.4), and then incubated in X-Gluc solution [0.1M sodium phosphate (pH 7.4), 3 mM potassium ferricyanide, 0.5 mM potassium ferrocyanide, 0.5 g l–1 5-bromo-4-chloro-3-indolyl-β-d-glucuronide cyclohexilammonium salt] at 37°C. After staining, samples were incubated in methanol to remove chlorophyll and then mounted in the clearing solution (a mixture of chloral hydrate, water, and glycerol in a ratio of 8:2:1). Observation was performed using a stereomicroscope (MZ16F, Leica Microsystems, Germany) or a microscope equipped with Nomarski optics (BX51, Olympus Co., Tokyo, Japan).
Confocal microscopy
The seedlings were stained with 10 g/mL Propidium (PI) (Sigma) for 1 min before imaging. For confocal microscopy, fluorescence in roots was detected using a confocal laser scanning microscope (Zeiss, LSM980). PI signal was visualized using wavelengths of 610 to 630 nm.
Identification of the genes highly expressed in the corresponding cell type
The average expression and dispersion were briefly calculated for all genes, which were subsequently placed into 9 bins based on expression. Principal component analysis (PCA) was performed to reduce the dimensionality on the log-transformed gene-barcode matrices of the most variable genes. Cells were clustered via a graph-based approach and visualized in two dimensions using t-distributed stochastic neighbor embedding (tSNE). A likelihood ratio test, which simultaneously tests for changes in mean expression and percentage of cells expressing a gene, was used to identify significantly differentially expressed genes (DEGs) between clusters. We used the FindAllMarkers function (test.use = bimod, logfc.thresold = 0, min.pct = 0.25) in Seurat to identify DEGs of each cluster. For a given cluster, FindAllMarkers identified positive markers compared with all other cells.
Total RNA extraction and qPCR analysis
Total RNA was extracted with FastPure Plant Total RNA Extraction kit (Cat. No. DC104, Vazyme; Nanjing, China). Total RNA was treated with DNaseI (Vazyme; Nanjing, China) for 30 min to remove the remaining DNA; then the cDNA was synthesized with HiScript II One-Step RT-PCR Kit (Cat. No. P611, Vazyme; Nanjing, China); qRT-PCR was performed with the corresponding primers (Supplemental Table 1). qPCR run was performed on a CFX 96 (Bio-Rad, Herculesm, CA, USA) with the following cycle parameter: 95°C for 30 s, 35 cycles of 95°C for 30 s, 55–56°C for 15 s and 72°C for 15s.
Results
Analysis of the expression of PIN1 in different cell types by scRNA-seq
To determine the possible regulators of PCs and GCs development under drought and salt stress, we first determined the cell types based on previously produced scRNA-seq data (Wu et al., 2022b). The following cell types, PC, guard mother cell (GMC), GC, meristemoid mother cell (MMC), early stage meristemoid (EM), late stage meristemoid (LM), young guard cell (YGC), and mesophyll cell (MPC) were identified based on the known marker genes for the corresponding cell type. A cell cluster without a known marker gene was annotated as unknown (u.k.). We screened the DEGs in the corresponding cell types. During the analysis of the expression patterns of the DEGs, we found that PIN1 was highly expressed in PC and stomatal lineage cell populations, such as MPC, LM, and EM (Figure 1), indicating PIN1’s role in the development of PCs and GCs.
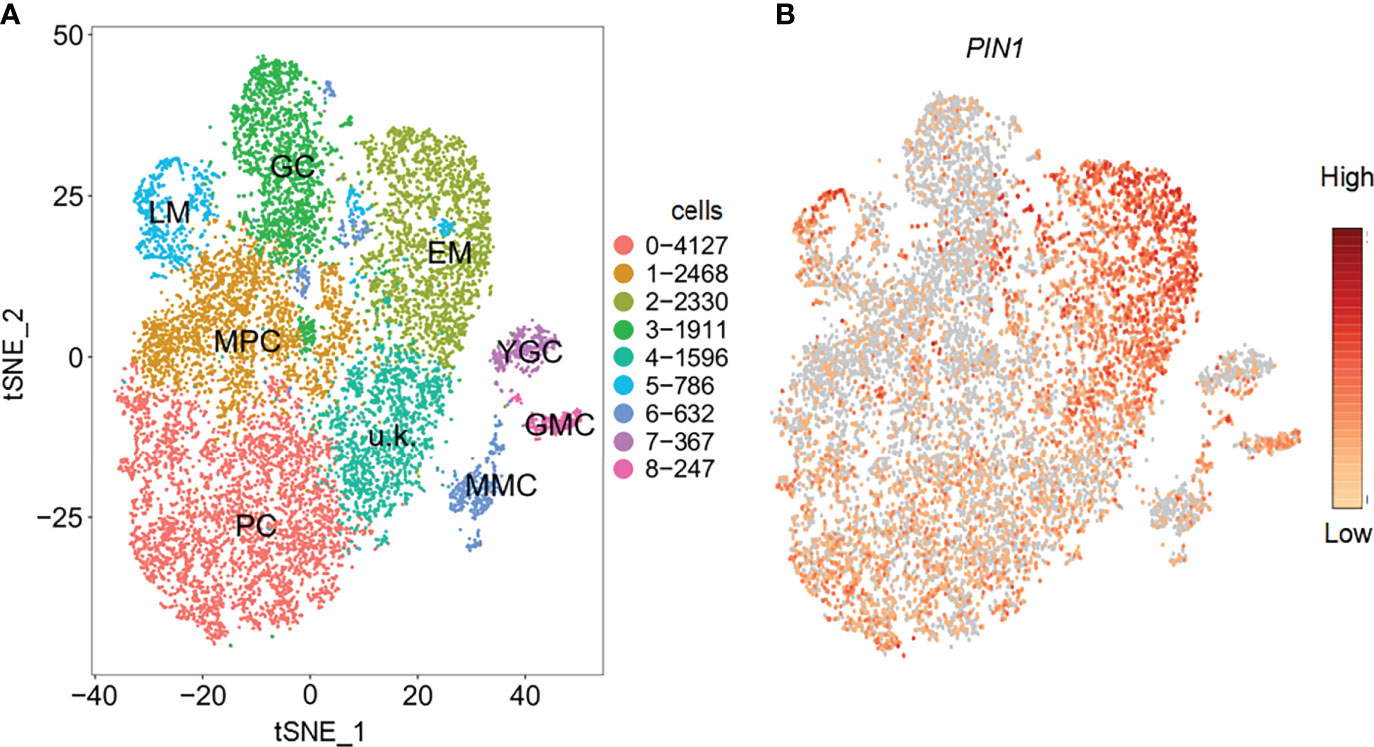
Figure 1 Identification of cell types with representative marker genes. (A) Distribution of cell types on tSNE plot; corresponding cell types are annotated on the plot. (B) Feature plot showing the expression pattern of PIN1. PC, pavement cell; GMC, guard mother cell; GC, guard cell; MMC, meristemoid mother cell; EM, early stage meristemoid; LM, late stage meristemoid; YGC, young guard cell; MPC, mesophyll cell; u.k., unknown.
Detection of the specific expression of pif1/3/4/5 in epidermal cells
In order to analyze the temporal and spatial expression dynamics of PIN1 under normal, drought, and salt treatment conditions, we used transgenic plants expressing PIN1pro:GUS and observed the expression changes of PIN1 at day 1, 3, 5 and 6 by GUS staining. As shown in Figure 2A, under normal growth conditions, there was no significant change in the expression level of PIN1pro:GUS in whole cotyledons from day 1 to day 6. Under NaCl treatment conditions, compared with the control, the GUS signal of PIN1pro: GUS in whole cotyledon was significantly decreased on day 5, and there was no significant change on the sixth day. Under mannitol treatment conditions, the GUS signal of PIN1pro:GUS increased gradually with treatment time. Compared with the control group, the GUS signal of PIN1pro:GUS in the cotyledons of day 5 was significantly decreased, and the GUS signal of PIN1pro:GUS in the cotyledons of day 6 was not significantly changed, while the GUS signal of PIN1pro:GUS was significantly enhanced in the veins of the cotyledons (Figure 2A). These results indicated that NaCl and mannitol treatment inhibited the expression of PIN1pro:GUS in cotyledons on day 5 before germination and promoted the expression of PIN1pro:GUS in vein (Figure 2A).
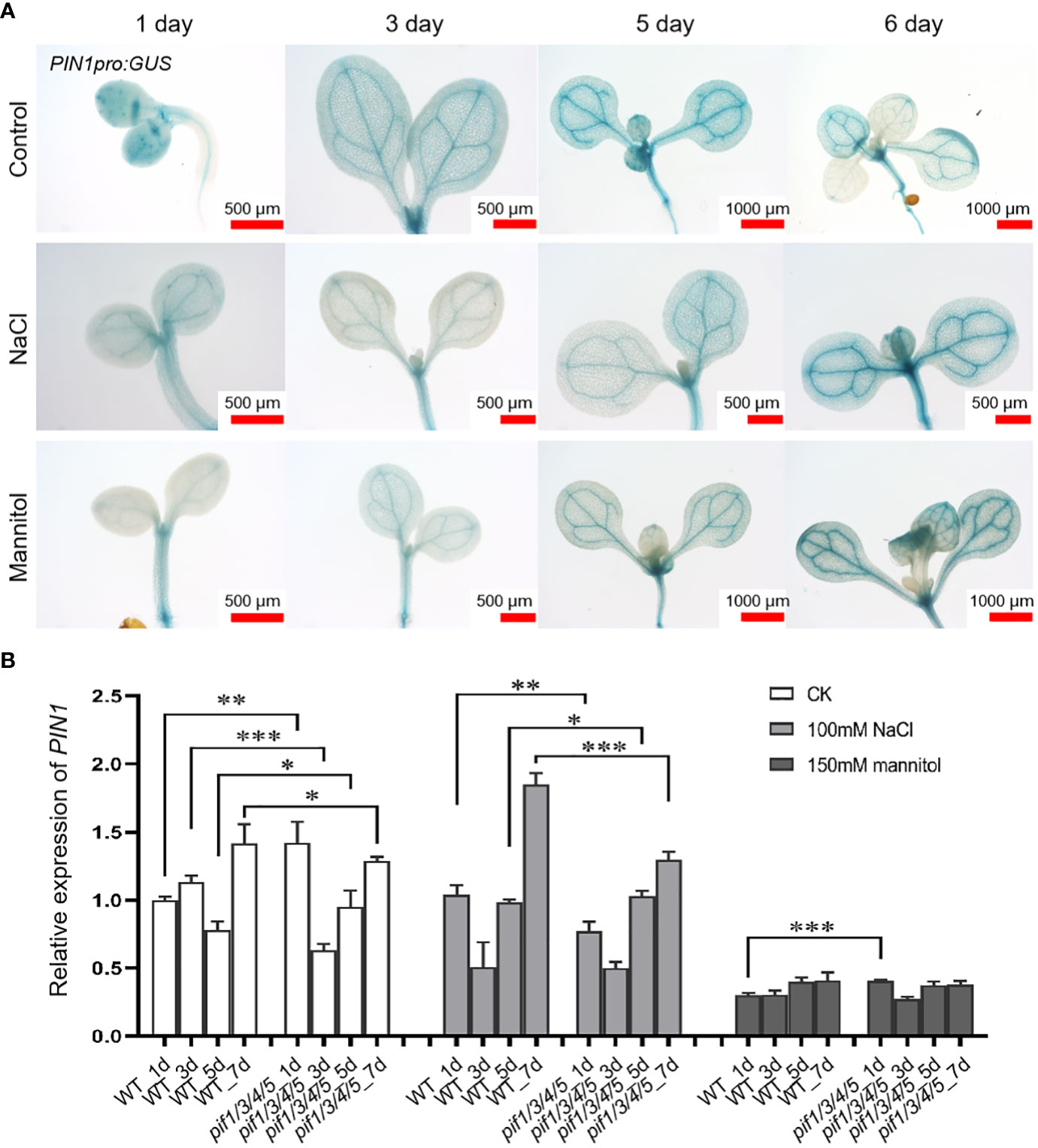
Figure 2 Analysis of the expression of PIN1pro: GUS and PIN1 gene after being treated with NaCl and mannitol. (A) Analysis of the expression of PIN1pro: GUS in whole cotyledons (up panel) during the development of seedlings grown under control, NaCl, and mannitol conditions, respectively. The seedlings of PIN1pro:GUS were grown in 1/2 MS plates plus 100 mM NaCl or 150 mM mannitol for 1, 3, 5, and 6 days, respectively; the seedlings of PIN1pro:GUS grown in normal 1/2 MS plates were used as controls. Then the seedlings were harvested and used for detecting the GUS activities by GUS staining, as described in Materials and Methods. (B) qPCR analysis of the relative expression of PIN1 under control, NaCl, and mannitol conditions, respectively. Relative expression indicates the mean value ( ± SD) of three independent experiments. The black stars represent student’s t-test of pif1/3/4/5 vs WT. *p < 0.05; **p < 0.01; ***p < 0.001.
The expression of PIN1 in pif1/3/4/5 quadruple mutant under NaCl and mannitol treatment conditions was analyzed by q-PCR. Compared with the control group, NaCl treatment significantly down-regulated the expression of PIN1 in pif1/3/4/5 quadruple mutant, but there was no significant change in WT plants (Figure 2B). At the same time, mannitol treatment also reduced PIN1 expression in WT and pif1/3/4/5 quadruple mutant compared with the control group (Figure 2B). Under mannitol treatment conditions, the levels of PIN1 in pif1/3/4/5 quadruple mutant on day 1 were significantly higher than WT (Figure 2B). Over time, the levels of PIN1 in pif1/3/4/5 quadruple mutant were gradually lower than WT (Figure 2B).
Drought and salt stress affect the development of leaf morphology
In order to analyze the potential role of PIN1 in regulating plant leaves under drought and salt stress, we analyzed the development of seedlings of pin1-5 mutant and 35S::PIN1, the WT was used as control. As shown in Figure 3A, compared with WT, pin1-5 mutant seedlings under normal growth conditions had significantly shorter petioles and a higher leaf length–width (L–W) ratio. Under normal conditions, the leaf development of 35S::PIN1 seedlings was slow in the early stage and faster in the late stage. Between days 7 and 14 after treatment, the leaf area of WT seedlings increased 2 times; the pin1-5 mutant increased 1.2 times, while the 35S::PIN1 increased 4.5 times. Under NaCl treatment conditions, petiole length of WT was significantly shortened, and L-W ratio of leaves was also decreased (Figures 3A, B). Compared with the WT, under drought and NaCl treatment conditions, the leaf area and petiole length of pin1-5 mutant was significantly shortened, while the ratio of L–W was significantly increased. The petiole length of the 35S::PIN1 was significantly lower than that of WT, while the L–W ratio was significantly higher than that of WT after 7 days of stress treatment (Figure 3B).
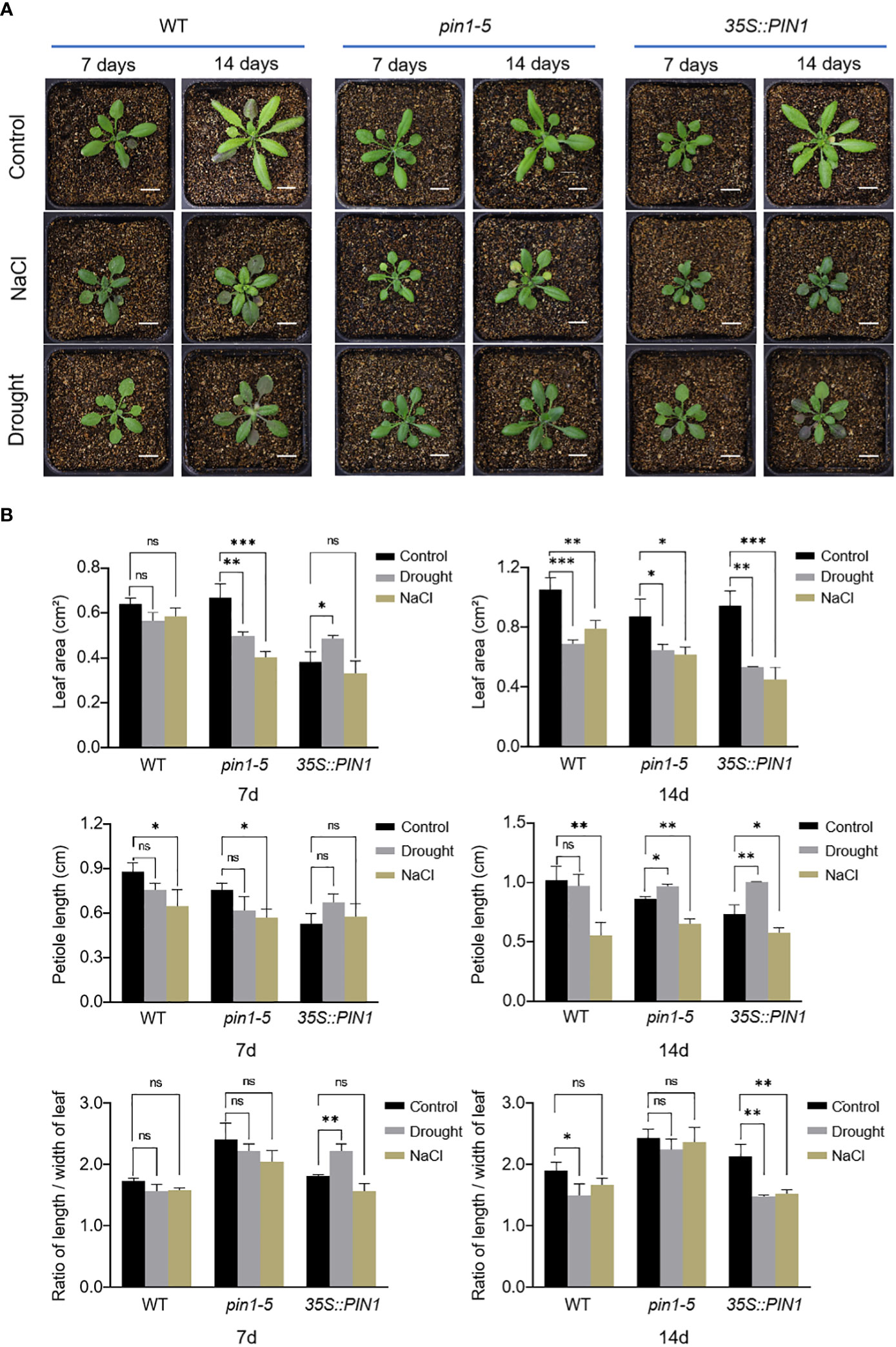
Figure 3 The leaves’ shape changes in response to NaCl and drought stresses. (A) pin1-5, 35S::PIN1 and WT were grown in soil for drought and NaCl treatment for 7 days and 14 days, respectively; the growth phenotypes were recorded by a camera; untreated seedlings were used as controls. Scale bar: 1 cm. (B) Statistical analysis of leaf area, petiole length and ratio of length/width of leaf in pin1-5, 35S::PIN1 and WT seeding. The data were analyzed by one-way ANOVA following Brown–Forsythe test. ns: p > 0.05; *p < 0.05; **p < 0.01; ***p < 0.001.
PIN1 is involved in regulating the developmental patterns of GC and PC
To understand the role of PIN1 in the development of PC and GC, we analyzed the developmental dynamics of PC and GC in seedlings of pin1-5 mutant, 35S::PIN1 and WT during early seedling development. As shown in Figures 4A, C, compared with the WT, the growth rate that was characterized by the roots length of pin1-5 mutant seedlings was faster than that of WT, but the subsequent growth was slower under normal conditions. The germination and subsequent growth of 35S::PIN1 seedlings were slower than that of WT. Figure 2B shows the development of PCs and GCs along with the growth time from day 1 to day 7. Statistical analysis showed that in WT, the size of PCs increased rapidly from day 1 to day 7, leading to a gradual decrease in the intensity of PCs (Figures 4D, E). Compared with WT, the size of PCs of pin1-5 mutant showed a similar growth trend to WT from day 1 to day 5, but after day 5, the size of PCs was smaller than WT, resulting in a slightly higher intensity of PCs in pin1-5 mutant than WT (Figures 4D, E). The trend of the size of PCs in 35S::PIN1 was similar to that of pin1-5 mutant, and the overall size of PCs was smaller than in WT, so the intensity of PCs per unit area was higher than in WT (Figures 4D, E). The intensity of GCs per unit area in WT increased rapidly until day 4 before decreasing (Figure 4F). Compared with WT, the intensity of GCs per unit area of pin1-5 mutant was slightly lower, while the intensity of GCs per unit area of 35S::PIN1 was higher (Figure 4F).
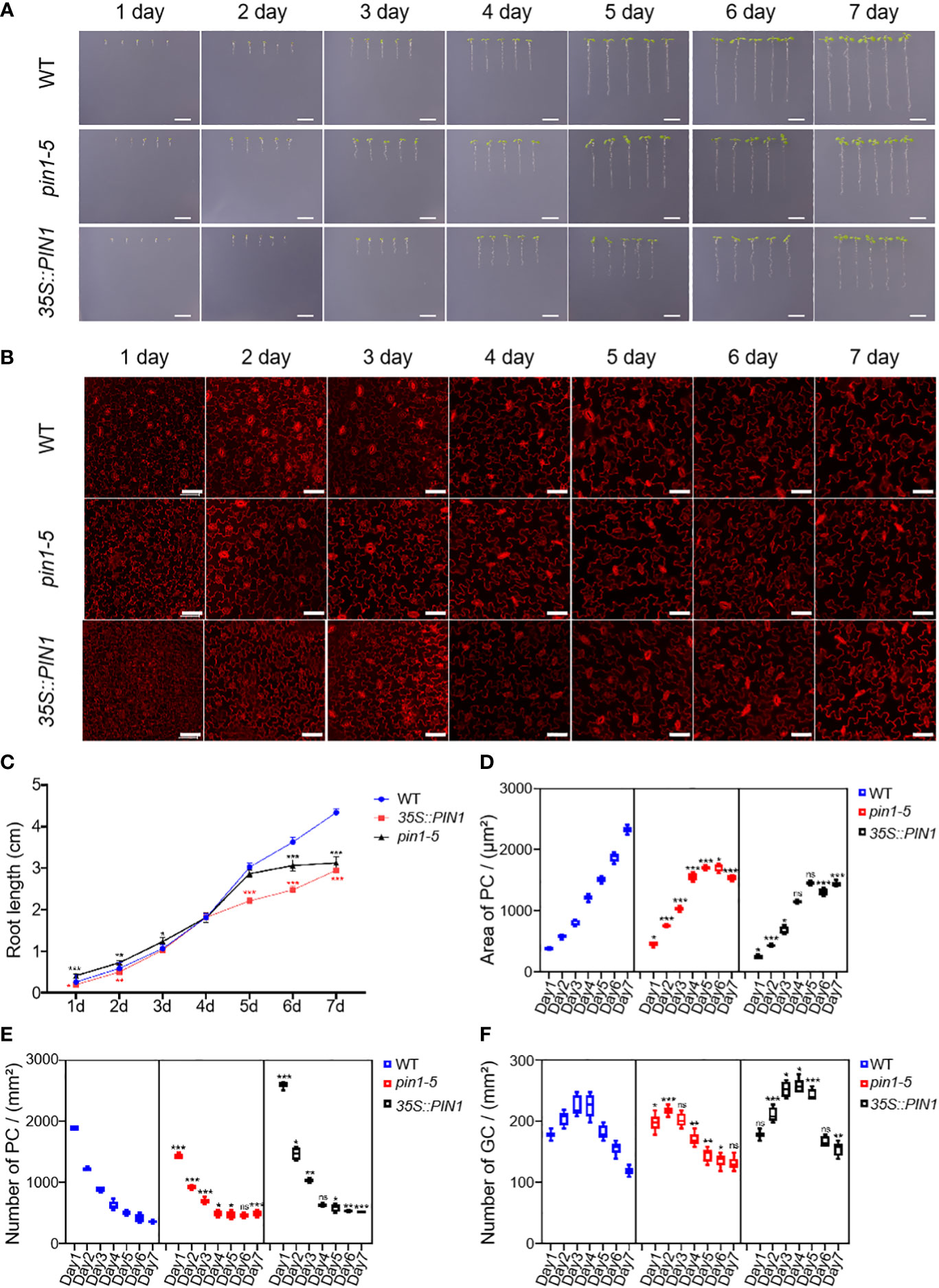
Figure 4 Pavement cells (PCs) and guard cells (GCs) development analysis of pin1-5, 35S::PIN1, and WT under normal conditions. Analysis of the growth of pin1-5, 35S::PIN1 and WT under control conditions. (A) The seeding of pin1-5, 35S::PIN1 and WT were grown on 1/2MS medium for 7 days. The seedlings born 1-7 were photographed continuously by a camera to record their developmental and growth phenotypes. Untreated seedlings were used as controls. Scale bar: 1 cm. (B) The development of PC and GC was measured under the following conditions: pin1-5, 35S::PIN1 and WT seedlings were cultured in 1/2 MS medium for 1-7 days, then harvested and stained with propyl chloride (PI) for 30 min to stain the membrane. After PI staining, PC and GC′s were detected under a confocal laser microscope. Scale bar: 50 μm. (C) Statistical analysis of the root length of seedlings of pin1-5, 35S::PIN1 and WT (n = 5). (D) Statistical analysis of the size of PCs in the lower epidermis of cotyledons of seedlings of pin1-5, 35S::PIN1 and WT (n = 5). (E) Statistical analysis of the intensity of PCs in the lower epidermis of cotyledons of seedlings of pin1-5, 35S::PIN1 and WT (n = 5). (F) Statistical analysis of the intensity of GCs in the lower epidermis of cotyledons of seedlings of pin1-5, 35S::PIN1 and WT (n = 5). The data were analyzed by one-way ANOVA following Brown–Forsythe test. ns: p > 0.05; *p < 0.05; **p < 0.01; ***p < 0.001.
Drought and salt stress regulate the developmental patterns of GCs and PCs through PIN1
To analyze whether PIN1 was also involved in regulating the differentiation and development of PCs and GCs under NaCl and drought conditions, we analyzed the growth, development and differentiation dynamics of WT, pin1-5 mutant and 35S::PIN1 seedlings. As shown in Figures 5A, C, pin1-5 mutant and 35S::PIN1 seedlings’ growth was slightly slower than WT from day 1 to day 7 under NaCl treatment conditions. Figure 5B shows the developmental dynamics of PCs and GCs in WT, pin1-5 mutant and 35S::PIN1 seedlings from day 1 to day 7 under NaCl treatment conditions. Statistical analysis showed that under NaCl treatment conditions, the size of PCs in WT seedlings increased gradually from day 1 to day 6 (Figure 5D), and the intensity of PCs in WT decreased continuously from day 1 to day 7 (Figure 5E). The changing trend of size and intensity of PCs in seedlings of pin1-5 mutant was similar to that of WT (Figures 5D, E). However, the size of PCs in 35S::PIN1 seedlings increased slowly from day 1 to day 7, and was lower than WT (Figures 5D, E). The intensity of PCs in 35S::PIN1 seedlings was always higher than WT (Figures 5D, E).
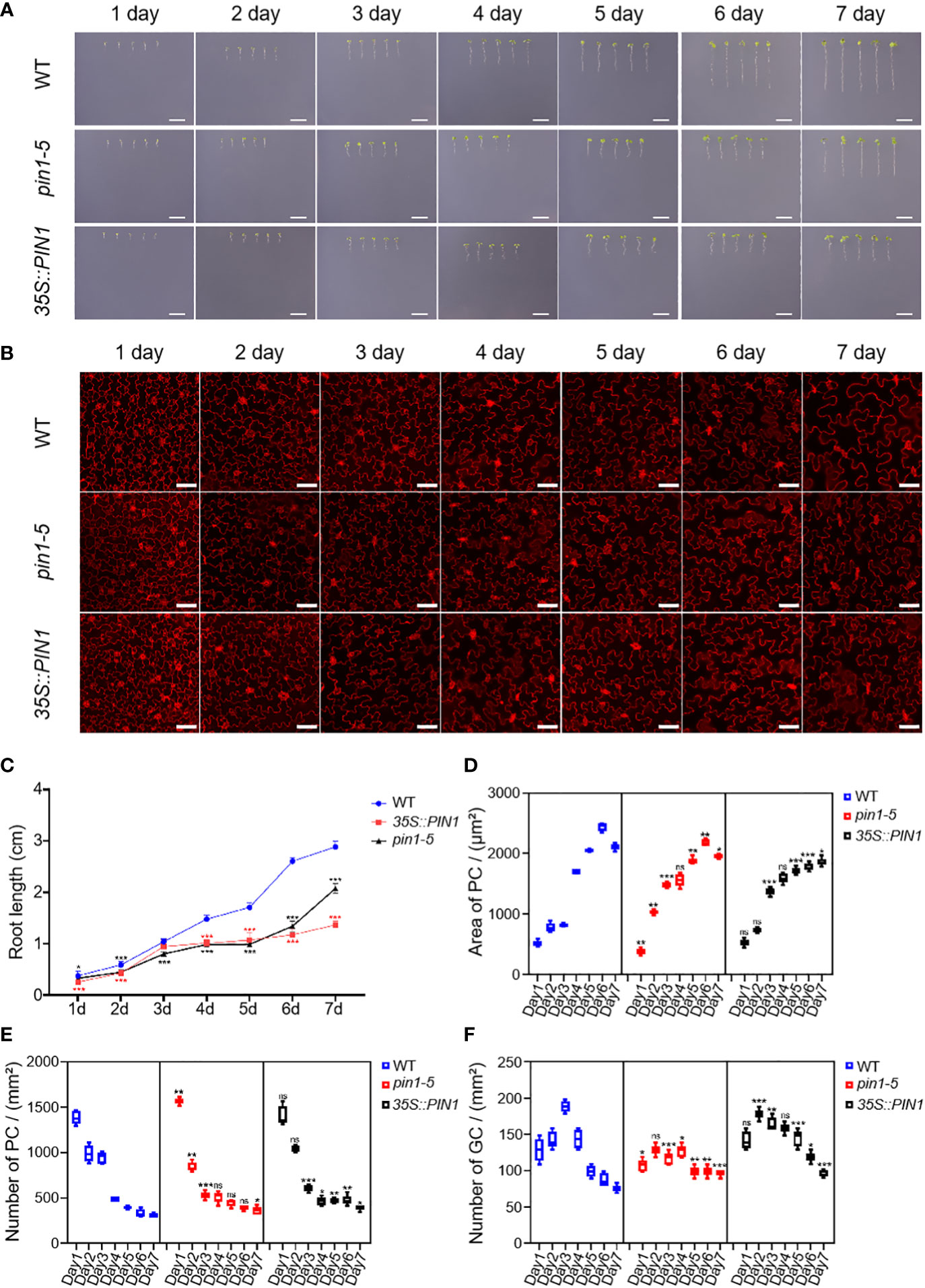
Figure 5 Analysis of PC and GC development in pin1-5, 35S::PIN1 and WT under NaCl conditions. Analysis of the growth of pin1-5, 35S::PIN1 and WT under control conditions. (A) The seeding of pin1-5, 35S::PIN1 and WT were grown in 1/2 MS plates plus 100 mM NaCl for 7 days. The seedlings grown for 1~7 days were photographed continuously by a camera to record their developmental and growth phenotypes, and untreated seedlings were used as controls. Scale bar: 1 cm. (B) The development of PC and GC was measured under the following conditions: pin1-5, 35S::PIN1 and WT seedlings were grown in 1/2 MS plates plus 100 mM NaCl for 1~7 days, then harvested and stained with PI for 30 min to stain the membrane. After PI staining, PC and GC′s were detected under a confocal laser microscope. Scale bar: 50 μm. (C) Statistical analysis of the root length of seedlings of pin1-5, 35S::PIN1 and WT (n = 5). (D) Statistical analysis of the size of PCs in the lower epidermis of cotyledons of seedlings of pin1-5, 35S::PIN1 and WT (n = 5). (E) Statistical analysis of the intensity of PCs in the lower epidermis of cotyledons of seedlings of pin1-5, 35S::PIN1 and WT (n = 5). (F) Statistical analysis of the intensity of GCs in the lower epidermis of cotyledons of seedlings of pin1-5, 35S::PIN1 and WT (n = 5). The data were analyzed by one-way ANOVA following Brown–Forsythe test. ns: p > 0.05; *p < 0.05; **p < 0.01; ***p < 0.001.
Analysis of the intensity of GCs showed that under NaCl treatment conditions, the intensity of GCs in WT increased rapidly from day 1 to day 3 and gradually decreased from day 3 until day 7 (Figure 5F). Compared with WT, the intensity of GCs in pin1-5 mutant seedlings had little change and began to stabilize after day 4. The intensity of GCs in 35S::PIN1 seedlings showed a rapid increase from day 1 to day 2 and then gradually decreased from day 2 to day 7 (Figure 5F).
Compared with the control group, the growth of WT, pin1-5 mutant, and 35S::PIN1 seedlings was significantly inhibited under mannitol treatment conditions, and the growth rate of pin1-5 mutant and 35S::PIN1 seedlings was significantly slower than that of WT (Figures 6A, C). Compared with WT, under mannitol treatment conditions, the germination rate of pin1-5 mutant seedlings was faster, but the subsequent growth rate was slower. Both germination and growth of the 35S::PIN1 were inhibited (Figure 6A). Under mannitol treatment conditions, the size of PCs in WT seedlings increased gradually from day 1 to day 6 and stabilized on day 7 (Figure 6D). The intensity of PCs showed a gradual decline from day 1 to day 7 (Figure 6E). Compared with WT, the size of PCs in seedlings of 35S::PIN1 increased gradually from day 1 to day 7 under mannitol treatment conditions, and the changing trend of the size of PCs in seedlings of pin1-5 mutant was similar to WT (Figure 6D). The intensity of PCs in seedlings of WT, pin1-5 mutant, and 35S::PIN1 showed an opposite trend with the area of PCs (Figures 6D, E). Overall, the intensity of PCs in seedlings of 35S::PIN1 was significantly higher than WT, while the intensity of PCs in seedlings of pin1-5 was lower than WT (Figure 6E).
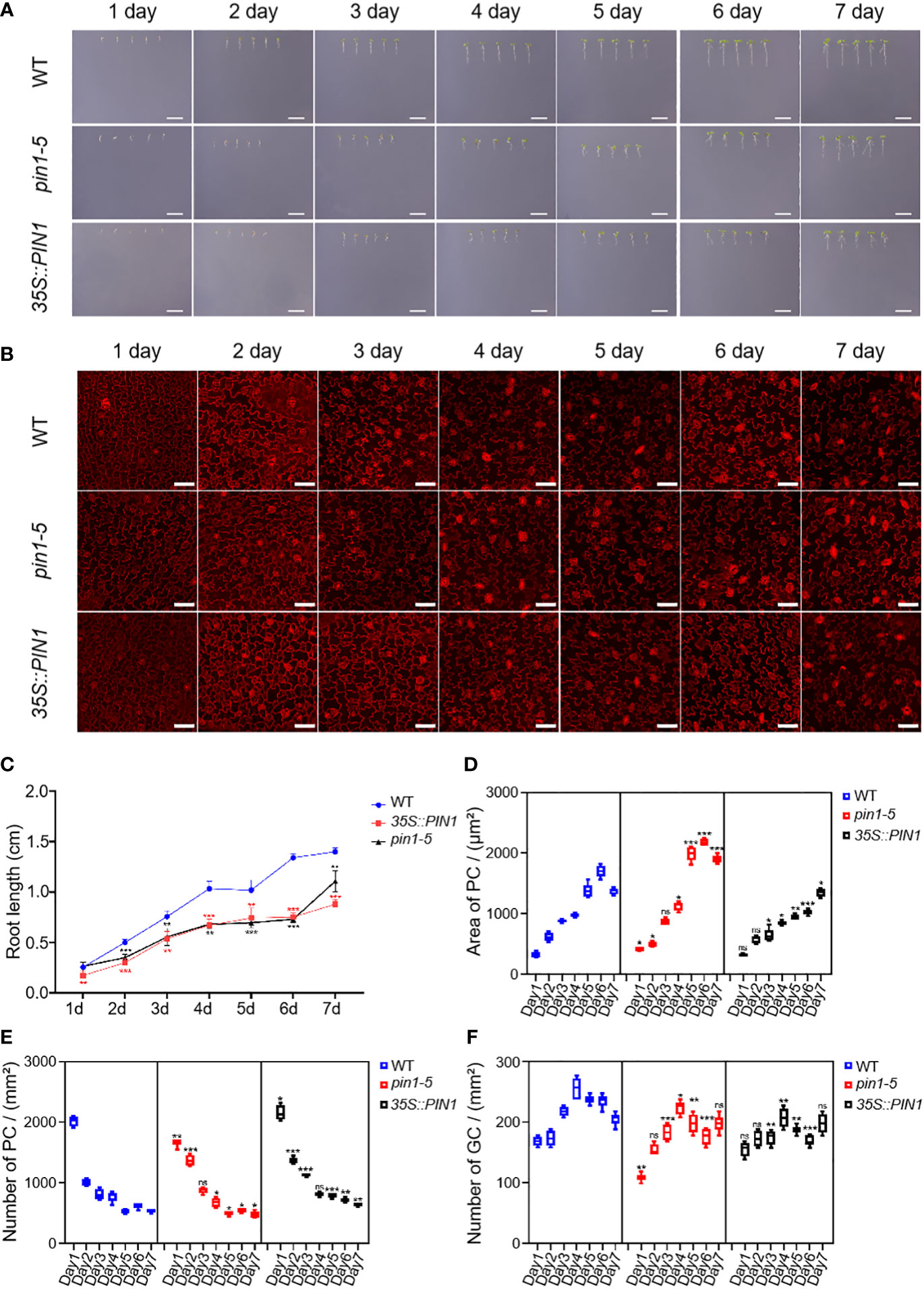
Figure 6 Analysis of PC and GC development in pin1-5, 35S::PIN1 and WT under drought conditions. Analysis of the growth of pin1-5, 35S::PIN1 and WT under control conditions. (A) The seeding of pin1-5, 35S::PIN1 and WT were grown in 1/2 MS plates plus 150 mM mannitol for 7 days. The seedlings born 1-7 were photographed continuously by a camera to record their developmental and growth phenotypes, and untreated seedlings were used as controls. Scale bar: 1 cm. (B) The development of PC and GC was measured under the following conditions: pin1-5, 35S::PIN1 and WT seedlings were grown in 1/2 MS plates plus 150 mM mannitol for 1–7 days, then harvested and stained with PI for 30 min to stain the membrane. After PI staining, PC and GC′s were detected under a confocal laser microscope. Scale bar: 50 μm. (C) Statistical analysis of the root length of seedlings of pin1-5, 35S::PIN1 and WT (n = 5). (D) Statistical analysis of the size of PCs in the lower epidermis of cotyledons of seedlings of pin1-5, 35S::PIN1 and WT (n = 5). (E) Statistical analysis of the intensity of PCs in the lower epidermis of cotyledons of seedlings of pin1-5, 35S::PIN1 and WT (n = 5). (F) Statistical analysis of the intensity of GCs in the lower epidermis of cotyledons of seedlings of pin1-5, 35S::PIN1 and WT (n = 5). The data were analyzed by one-way ANOVA following Brown–Forsythe test. ns: p > 0.05; *p < 0.05; **p < 0.01; ***p < 0.001.
From day 1 to day 4, the intensity of GCs in WT seedlings gradually increased and began to decrease after day 4 (Figure 6F). Compared with WT, under mannitol treatment conditions, the intensity of GCs in pin1-5 mutant and 35S::PIN1 seedlings showed the same trend, gradually increasing from day 1 to day 4 (Figure 6E). In general, the intensity of GCs in pin1-5 mutant and 35S::PIN1 seedlings was significantly lower than that in WT (Figure 6E).
Considering the defects in stomatal development caused by NaCl and mannitol treatment, it may be that these treatments influenced cotyledon development and altered the expression of ROP1, ROP2, RIC1, RIC4, ERH3 and CLASP. To analyze the effects of drought and salt stress on cotyledon development, we analyzed the cotyledon development of WT, pin1-5 mutant and 35S::PIN1 seedlings under control, NaCl and mannitol treatment conditions (Figure 7A). As shown in Figure 7A, compared with WT, 35S::PIN1 seedlings under normal growth conditions had significantly reduced aspect ratio relative to wild-type cotyledons, whereas the pin1-5 mutant remained similar to the wild type. Under NaCl conditions, the cotyledon aspect ratio of WT increased (Figures 7A, B). Compared with WT, the cotyledon aspect ratio of pin1-5 mutant and 35S::PIN1 did not change significantly under mannitol treatment conditions (Figures 7A, B). However, under NaCl treatment conditions, the cotyledon aspect ratio of pin1-5 mutant and 35S::PIN1 was significantly reduced compared with WT. To examine whether PIN could regulate the expression of ROP1, ROP2, RIC1, RIC4, ERH3 and CLASP, qPCR analysis was performed. Compared with the control group, NaCl treatment resulted in significantly up-regulated expressions of ROP1, ROP2, RIC4 and ERH3 in WT but not in pin1-5 mutant seedlings (Figures 7C, D, F, G). Meanwhile, mannitol treatment enhanced the expression of ROP1, ROP2, RIC4, and ERH3 in WT and pin1-5 mutant compared with the control group (Figures 7C, D, F, G). The levels of ROP1, ROP2, RIC4 and ERH3 in the pin1-5 mutant were also higher than WT under mannitol treatment conditions (Figures 7C, D, F, G). For RIC1, compared with the control group, its expression was significantly decreased under NaCl and mannitol treatment conditions (Figure 7E). As for the expression of CLASP, it was found that under NaCl and mannitol treatment conditions, its expression in WT, pin1-5 mutant, and 35S::PIN1 was significantly increased (Figure 7H).
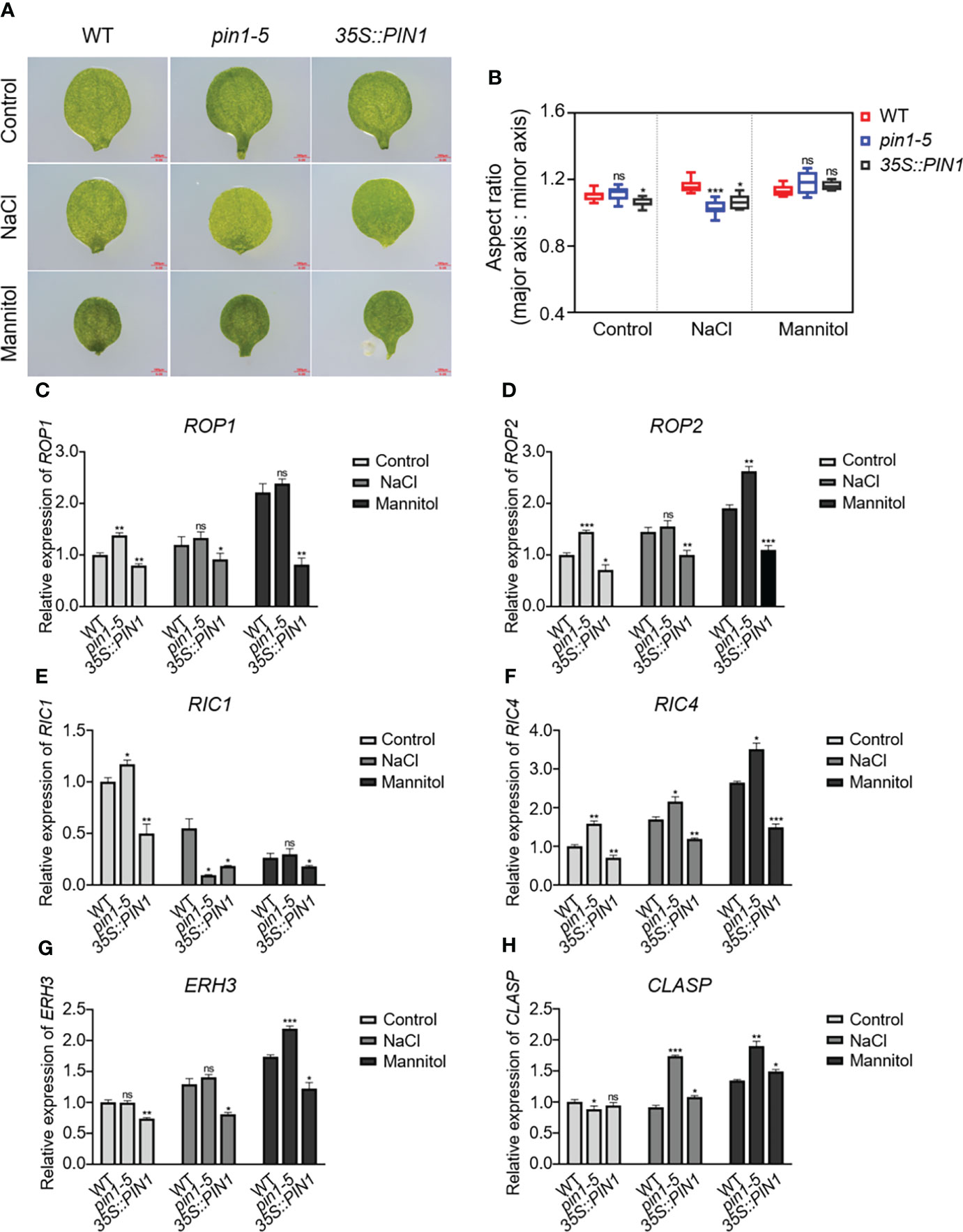
Figure 7 Effects of PIN1 on cotyledon morphological development. (A) The wild-type (WT) seedlings, pin1-5 and 35S::PIN1, were grown in 1/2 MS plates, 1/2 MS plates plus 100 mM NaCl, and 1/2 MS plates plus 150 mM mannitol for 7 days, and the development of cotyledons was observed on micrographs. Scale bars, 0.5 mm. n ≥ 8 cotyledons per genotype. (B) Boxplots represent the aspect ratio of WT and mutant cotyledons. (C-H) qPCR analysis of the relative expression of ROP1, ROP2, RIC1, RIC4, ERH3, and CLASP under control, NaCl, and mannitol conditions, respectively. Relative expression indicates the mean value ( ± SD) of three independent experiments. The data were analyzed by one-way ANOVA following Brown–Forsythe test. ns: p > 0.05; *p < 0.05; **p < 0.01; ***p < 0.001.
Discussion
Drought and salt stresses affect the developmental pattern of leaf epidermal cells
Plants have developed several mechanisms to respond and adapt to different types of abiotic stress enacted by external environmental factors as part of their evolution. How and where plants sense changes in water levels in the root has been well studied. It has been demonstrated that the PLASMA MEMBRANE INTRINSIC PROTEINS (PIPs), a subfamily of plasma membrane-localized aquaporin channels, enhance water movement in plant roots (Dietrich, 2018). Similarly, in salt stress, phosphatidic acid is a minor membrane phospholipid required for plant growth response to salt stress. Phosphatidic acid binds to PINOID (PID) to promote PID-dependent PIN phosphorylation under salt stress (Wang et al., 2019). But the mechanisms underlying how leaf epidermal cells respond to drought and salt stress require further attention. Leaf development can be controlled by multiple regulatory networks. Environmental factors such as drought and salt stress (Chun et al., 2018; Gambetta et al., 2020) affect plants critical developmental processes, such as leaf development. These unfavorable factors invoke changes in plant growth patterns, regulating cell form and function, which plays a role in plant growth style. Although we previously analyzed the role of PHYTOCHROME INTERACTING FACTOR (PIF) 1, PIF3, PIF4, and PIF5 in PCs and GCs development under drought and salt stress (Liu et al., 2022b), no mechanism has yet been proposed for the role of PIN1 in PCs and GCs development under drought and salt stress conditions. Here, we investigated the role of PIN1 in drought and salt stress regulation of the developmental patterns of PCs and GCs in Arabidopsis leaves. The data showed that drought and salt stress highly regulated plant leaf morphology via decreased leaf area and petiole length in WT, pin1-5 mutant and 35S::PIN1 seedlings (Figure 3). In agreement with these findings, several other studies reported on how drought and salt regulate plant physiology and morphology (Bartels and Sunkar, 2005; Ma and Qin, 2014). The functioning and development of these leaves’ epidermal cells largely depend on each other. More often, the growth and development of the leaf depend on the conditions of these epidermal cells (Bar and Ori, 2014), suggesting that PCs and GCs developmental dynamics are critical for plant response to drought and salt stress conditions.
PIN1 regulates the development of leaf morphology and epidermal cells under drought and salt stress conditions
Besides biochemical manipulations, mechanical stress induced at the tissue scale influences microtubules to align with the maximal direction of stress, hence regulating cell and tissue level morphogenesis (Hamant et al., 2008; Hervieux et al., 2016; Takatani et al., 2020). Signals from environmental stress induce the expression of temporary regulatory networks, which promotes an overall defense process in plants. A large number of studies have uncovered major genes that regulate leaf epidermal cells development in plants subjected to drought and salt stress (Fricke et al., 2006; Maricle et al., 2009; Taleisnik et al., 2009; Garrido et al., 2014; Zhang et al., 2018; Liu et al., 2022b). This study investigated the role of PIN1 in the development of plant epidermal cells under drought and salt stress. Firstly, we analyzed the developmental dynamics of pin1-5 mutant, 35S::PIN1 and WT during early seedling development. We observed that the intensity of PCs of pin1-5 mutant seedlings and 35S::PIN1 seedlings was higher than in WT (Figure 4), while the intensity of GCs of 35S::PIN1 seedlings was higher than in pin1-5 mutant and WT seedlings (Figure 4). Our previous findings demonstrated that drought and salt stress regulate the developmental patterns of GCs and PCs (Liu et al., 2022b). Meanwhile, how drought and salt stress regulate the differentiation and developmental patterns of GCs and PCs through PIN1 signaling is not determined yet. In Figure 5, we observed that NaCl treatment inhibited the growth of 35S::PIN1 and pin1-5 mutant seedlings. However, the PC size and number per unit area of 35S::PIN1 seedlings increased more than WT and pin1-5 mutant seedlings. For GC development under NaCl treatment conditions, we observed that, compared with WT, The intensity of GCs in 35S::PIN1 seedlings showed a rapid increase from day 1 to day 2 and then gradually decreased from day 2 to day 7 (Figure 3), indicating that even during growth inhibition by abiotic stress, plants adaptations to cope with and adapt to these stresses have evolved over time, thus why we observed changes in the same organ responding to drought and salt stress, which also suggest that the plasma membrane-localized transporter of auxin PIN1 does not only control the formation and development of flowers and regulation of root hair initiation but also regulate PCs and GCs differentiation and development under salt stress tolerance.
Under mannitol treatment, compared with the control group, the growth of WT, pin1-5 mutant and 35S::PIN1 seedlings was significantly inhibited. However, compared with WT and pin1-5 mutant, the size of PCs of 35S::PIN1 seedlings increased. Although the intensity of PCs in WT, pin1-5 mutant and 35S::PIN1 seedlings showed an opposite trend with the area of PCs, the overall intensity of PCs in 35S::PIN1 seedlings was significantly higher than in WT and pin1-5 mutant (Figure 6). However, the intensity of GCs in pin1-5 mutant and 35S::PIN1 seedlings was slightly increased, which highlights the involvement of PIN1 in PCs and GCs differentiation and development under drought stress tolerance.
Several genes involved in signaling and regulatory pathways or enzymes known to alleviate plant stress have been reported (Wang et al., 2003; Kim et al., 2010; Huang et al., 2012; Chen et al., 2021; Wang et al., 2021a; Liu et al., 2022a; Liu et al., 2022b). For example, several genes have been involved in the signaling network mediating cell fate determination of the epidermis and stomatal functioning (Pillitteri and Torii, 2012; Mckown and Bergmann, 2020). In addition, since PIN1 expression and distribution in plants is regulated by the auxin signaling pathway (Chen et al., 2011; Omelyanchuk et al., 2016; Guillory and Bonhomme, 2021), it is possible that PIN1 may regulate PCs and GCs development under drought and salt stress via auxin signaling. In Figure 1, using scRNA-seq analysis, we identified that PIN1 was highly expressed in PCs, suggesting a possible function of PIN1 in regulating PCs and GCs development. For further confirmation, we analyzed the temporal and spatial expression dynamics of PIN1 in pif1/3/4/5 quadruple mutant under normal, drought, and salt treatment conditions using GUS staining and qPCR analysis (Figures 2A, B). The results showed that the expression level of PIN1 in pif1/3/4/5 quadruple mutant changed under NaCl and mannitol treatments. Compared with the mock, the expression level of PIN1 in pif1/3/4/5 quadruple mutant was down regulated under NaCl and mannitol treatments (Figure 2B). Together, the results demonstrate how NaCl and mannitol treatments regulate the expression of PIN1 in pif1/3/4/5 quadruple mutant. Because cotyledon development in plants may be regulated by stomatal functioning, we analyzed the effects of drought and salt stress on cotyledon development. As shown in Figure 7, we observed that NaCl and mannitol treatments significantly regulated the cotyledon aspect ratio of pin1-5 mutant and 35S::PIN1 seedlings compared to that of WT. We also determined whether PIN could regulate the expression of ROP1, ROP2, RIC1, RIC4, ERH3 and CLASP using qPCR analysis. We found that compared to the control group, NaCl and mannitol treatments upregulated the expression of ROP1, ROP2, RIC4 and ERH3. Compared with that of WT, the expression of ROP1, ROP2, RIC4 and ERH3 in the seedlings of pin1-5 mutant was increased, but decreased in 35S::PIN1 seedlings, suggesting that PIN1 may be involved in regulating the cotyledon development by mediating the expression of these genes under drought and salt stress conditions. Collectively, these results suggest that temporally regulatory networks are crucial in leaf epidermal cell development and differentiation under drought and salt stress, among which PIN1 is a critical regulator.
Conclusion
In summary, PIN1 is critical in developing leaf epidermal cells under drought and salt stress conditions. PIN1 regulated plant morphology under drought and salt stress. PIN1 was constitutively involved in drought and salt stress regulation of PC and GC development. The determination of possible regulators of PC and GC development under drought and salt stress showed that PIN1 was highly expressed in PC and stomatal lineage cell populations, highlighting PIN1 as a critical regulator of PC and GC development under drought and salt stress. The gene expression analysis showed PIN1 expression dynamics in pif1/3/4/5 quadruple mutant under drought and salt stress. Collectively, this work sheds light on the role of PIN1 in developing PCs and GCs under drought and salt stress, highlighting this gene as a promising candidate for breeding stress-tolerant crops.
Data availability statement
The original contributions presented in the study are included in the article/Supplementary material. Further inquiries can be directed to the corresponding author.
Author contributions
Conceptualization of the project: XS. Performance of specific experiments: RW, ZL, YZ, HL, SS, YL, AQ, XY, ZZ, JY, MH, and GB. Data analysis and writing of the first draft: XS and GB. Supervision and validation of the manuscript: XS. All authors approved the final version.
Acknowledgments
We are grateful to the Arabidopsis Biological Resource Center (ABRC) for the Arabidopsis seeds. This research was supported by the National Natural Science Foundation of China (31670233).
Conflict of interest
The authors declare that the research was conducted in the absence of any commercial or financial relationships that could be construed as a potential conflict of interest.
Publisher’s note
All claims expressed in this article are solely those of the authors and do not necessarily represent those of their affiliated organizations, or those of the publisher, the editors and the reviewers. Any product that may be evaluated in this article, or claim that may be made by its manufacturer, is not guaranteed or endorsed by the publisher.
Supplementary material
The Supplementary Material for this article can be found online at: https://www.frontiersin.org/articles/10.3389/fpls.2022.1043204/full#supplementary-material
References
Adamowski, M., Friml, J. (2015). PIN-dependent auxin transport: Action, regulation, and evolution. Plant Cell 27, 20–32. doi: 10.1105/tpc.114.134874
Bar, M., Ori, N. (2014). Leaf development and morphogenesis. Development 141, 4219–4230. doi: 10.1242/dev.106195
Bartels, D., Sunkar, R. (2005). Drought and salt tolerance in plants. Crit. Rev. Plant Sci. 24, 23–58. doi: 10.1080/07352680590910410
Bawa, G., Feng, L., Yan, L., Du, Y., Shang, J., Sun, X., et al. (2019). Pre-treatment of salicylic acid enhances resistance of soybean seedlings to fusarium solani. Plant Mol. Biol. 101, 315–323. doi: 10.1007/s11103-019-00906-x
Chen, X., Ding, Y., Yang, Y., Song, C., Wang, B., Yang, S., et al. (2021). Protein kinases in plant responses to drought, salt, and cold stress. J. Integr. Plant Biol. 63, 53–78. doi: 10.1111/jipb.13061
Chen, M., Liu, H., Jixiang, K., Yang, Y., Zhang, N., Li, R., et al. (2011). RopGEF7 regulates PLETHORA-dependent maintenance of the root stem cell niche in arabidopsis. Plant Cell 23, 2880–2894. doi: 10.1105/tpc.111.085514
Chowdhury, M. R., Ahamed, M. S., Mas-Ud, M. A., Islam, H., Fatamatuzzohora, M., Hossain, M. F., et al. (2021). Stomatal development and genetic expression in. Heliyon 7, e07889. doi: 10.1016/j.heliyon.2021.e07889
Chun, H. J., Baek, D., Cho, H. M., Jung, H. S., Jeong, M. S., Jung, W.-H., et al. (2018). Metabolic adjustment of arabidopsis root suspension cells during adaptation to salt stress and mitotic stress memory. Plant Cell Physiol. 60, 612–625. doi: 10.1093/pcp/pcy231
Cosgrove, D. J. (2017). Diffuse growth of plant cell walls. Plant Physiol. 176, 16–27. doi: 10.1104/pp.17.01541
Daszkowska-Golec, A., Szarejko, I. (2013). Open or close the gate – stomata action under the control of phytohormones in drought stress conditions. Front Plant Sci 4, 138. doi: 10.3389/fpls.2013.00138
Devkar, V., Thirumalaikumar, V. P., Xue, G. P., Vallarino, J. G., Turečková, V., Strnad, M., et al. (2020). Multifaceted regulatory function of tomato SlTAF1 in the response to salinity stress. New Phytol. 225, 1681–1698. doi: 10.1111/nph.16247
Dhakal, S., Reiter, J. W., Laroche, A., Schultz, E. A. (2021). Leaf vein pattern response to heat and drought requires genes that influence PINFORMED1 localization and is mimicked by ABA treatment. Environ. Exp. Bot. 185, 104426. doi: 10.1016/j.envexpbot.2021.104426
Dhonukshe, P., Tanaka, H., Goh, T., Ebine, K., Mähönen, A. P., Prasad, K., et al. (2008). Generation of cell polarity in plants links endocytosis, auxin distribution and cell fate decisions. Nature 456, 962–966. doi: 10.1038/nature07409
Dietrich, D. (2018). Hydrotropism - how roots search for water. J. Exp. Bot. 69, 2759–2771. doi: 10.1093/jxb/ery034
Dong, H., Bai, L., Zhang, Y., Zhang, G., Mao, Y., Min, L., et al. (2018). Modulation of guard cell turgor and drought tolerance by a peroxisomal acetate-malate shunt. Mol. Plant 11, 1278–1291. doi: 10.1016/j.molp.2018.07.008
Ferreira, C., Simioni, C., Schmidt, É.C., Ramlov, F., Maraschin, M., Bouzon, Z. L. (2017). The influence of salinity on growth, morphology, leaf ultrastructure, and cell viability of the seagrass halodule wrightii ascherson. Protoplasma 254, 1529–1537. doi: 10.1007/s00709-016-1041-4
Fischer, U., Ikeda, Y., Ljung, K., Serralbo, O., Singh, M., Heidstra, R., et al. (2006). Vectorial information for arabidopsis planar polarity is mediated by combined AUX1, EIN2, and GNOM activity. Curr. Biol. 16, 2143–2149. doi: 10.1016/j.cub.2006.08.091
Fricke, W., Akhiyarova, G., Wei, W., Alexandersson, E., Miller, A., Kjellbom, P. O., et al. (2006). The short-term growth response to salt of the developing barley leaf. J. Exp. Bot. 57, 1079–1095. doi: 10.1093/jxb/erj095
Fujiwara, M. T., Yasuzawa, M., Kojo, K. H., Niwa, Y., Abe, T., Yoshida, S., et al. (2018). The arabidopsis arc5 and arc6 mutations differentially affect plastid morphology in pavement and guard cells in the leaf epidermis. PloS One 13, e0192380. doi: 10.1371/journal.pone.0192380
Gambetta, G. A., Herrera, J. C., Dayer, S., Feng, Q., Hochberg, U., Castellarin, S. D. (2020). The physiology of drought stress in grapevine: Towards an integrative definition of drought tolerance. J. Exp. Bot. 71, 4658–4676. doi: 10.1093/jxb/eraa245
Garrido, Y., Tudela, J. A., Marín, A., Mestre, T., Martínez, V., Gil, M. I. (2014). Physiological, phytochemical and structural changes of multi-leaf lettuce caused by salt stress. J Sci Food Agric 94, 1592–1599. doi: 10.1002/jsfa.6462
Guillory, A., Bonhomme, S. (2021). Phytohormone biosynthesis and signaling pathways of mosses. Plant Mol. Biol. 107, 1–33. doi: 10.1007/s11103-021-01172-6
Guo, J., Zhou, Y., Li, J., Sun, Y., Shangguan, Y., Zhu, Z., et al. (2020). COE 1 and GUN1 regulate the adaptation of plants to high light stress. Biochem. Biophys. Res. Commun. 521, 184–189. doi: 10.1016/j.bbrc.2019.10.101
Hamant, O., Heisler, M. G., Jönsson, H., Krupinski, P., Uyttewaal, M., Bokov, P., et al. (2008). Developmental patterning by mechanical signals in arabidopsis. Science (New York, N.Y.) 322, 1650–1655. doi: 10.1126/science.1165594
Heisler, M. G., Hamant, O., Krupinski, P., Uyttewaal, M., Ohno, C., Jönsson, H., et al. (2010). Alignment between PIN1 polarity and microtubule orientation in the shoot apical meristem reveals a tight coupling between morphogenesis and auxin transport. PloS Biol. 8, e1000516. doi: 10.1371/journal.pbio.1000516
Heisler, M. G., Ohno, C., Das, P., Sieber, P., Reddy, G. V., Long, J. A., et al. (2005). Patterns of auxin transport and gene expression during primordium development revealed by live imaging of the arabidopsis inflorescence meristem. Curr. Biol. 15, 1899–1911. doi: 10.1016/j.cub.2005.09.052
Hervieux, N., Dumond, M., Sapala, A., Routier-Kierzkowska, A.-L., Kierzkowski, D., Roeder, A. h.K., et al. (2016). A mechanical feedback restricts sepal growth and shape in arabidopsis. Curr. Biol. 26, 1019–1028. doi: 10.1016/j.cub.2016.03.004
Huang, G. T., Ma, S. L., Bai, L. P., Zhang, L., Ma, H., Jia, P., et al. (2012). Signal transduction during cold, salt, and drought stresses in plants. Mol. Biol. Rep. 39, 969–987. doi: 10.1007/s11033-011-0823-1
Jalil, S., Ansari, M. I. (2019). Role of Phytohormones in Recuperating Salt Stress. Salt Stress, Microbes, and Plant Interactions: Mechanisms and Molecular Approaches Akhtar, M. S. (Singapore, Springer Singapore), 2, 91–104.
Ji, T., Li, S., Li, L., Huang, M., Wang, X., Wei, M., et al. (2018). Cucumber phospholipase d alpha gene overexpression in tobacco enhanced drought stress tolerance by regulating stomatal closure and lipid peroxidation. BMC Plant Biol. 18, 355. doi: 10.1186/s12870-018-1592-y
Karimi, S. M., Freund, M., Wager, B. M., Knoblauch, M., Fromm, J., M. Mueller, H., et al. (2021). Under salt stress guard cells rewire ion transport and abscisic acid signaling. The New phytologist 231, 1040–1055. doi: 10.1111/nph.17376
Kebbas, S., Lutts, S., Aid, F. (2015). Effect of drought stress on the photosynthesis of acacia tortilis subsp. raddiana at the young seedling stage. Photosynthetica 53, 288–298. doi: 10.1007/s11099-015-0113-6
Kim, D. Y., Jin, J. Y., Alejandro, S., Martinoia, E., Lee, Y. (2010). Overexpression of AtABCG36 improves drought and salt stress resistance in arabidopsis. Physiol. Plant 139, 170–180. doi: 10.1111/j.1399-3054.2010.01353.x
Li, S., Fan, C., Li, Y., Zhang, J., Sun, J., Chen, Y., et al. (2016). Effects of drought and salt-stresses on gene expression in caragana korshinskii seedlings revealed by RNA-seq. BMC Genomics 17, 200. doi: 10.1186/s12864-016-2562-0
Li, H., Lin, D., Dhonukshe, P., Nagawa, S., Chen, D., Friml, J., et al. (2011). Phosphorylation switch modulates the interdigitated pattern of PIN1 localization and cell expansion in arabidopsis leaf epidermis. Cell Res. 21, 970–978. doi: 10.1038/cr.2011.49
Liu, Z., Guo, C., Wu, R., Hu, Y., Zhou, Y., Wang, J., et al. (2022a). FLS2–RBOHD–PIF4 module regulates plant response to drought and salt stress 23, 1080. doi: 10.3390/ijms23031080
Liu, Z., Guo, C., Wu, R., Wang, J., Zhou, Y., Yu, X., et al. (2022b). Identification of the regulators of epidermis development under drought- and salt-stressed conditions by single-cell RNA-seq. Int. J. Mol. Sci. 23, 2759. doi: 10.3390/ijms23052759
Li, T., Wu, R., Liu, Z., Wang, J., Guo, C., Zhou, Y., et al. (2021). GUN4 affects the circadian clock and seedlings adaptation to changing light conditions. Int. J. Mol. Sci. 23, 194. doi: 10.3390/ijms23010194
López-Serrano, L., Canet-Sanchis, G., Vuletin Selak, G., Penella, C., San Bautista, A., López-Galarza, S., et al. (2019). Pepper rootstock and scion physiological responses under drought stress. Front Plant Sci 10, 38. doi: 10.3389/fpls.2019.00038
Ma, Y., Qin, F. (2014). “ABA regulation of plant responses to drought and salt stresses,” in Abscisic acid: Metabolism, transport and signaling. Ed. Zhang, D.-P. (Dordrecht: Springer Netherlands), 315–336.
Maricle, B. R., Koteyeva, N. K., Voznesenskaya, E. V., Thomasson, J. R., Edwards, G. E. (2009). Diversity in leaf anatomy, and stomatal distribution and conductance, between salt marsh and freshwater species in the C(4) genus spartina (Poaceae). New Phytol. 184, 216–233. doi: 10.1111/j.1469-8137.2009.02903.x
Mckown, K. H., Bergmann, D. C. (2020). Stomatal development in the grasses: lessons from models and crops (and crop models) 227, 1636–1648. doi: 10.1111/nph.16450
Meng, Y., Chen, F., Shuai, H., Luo, X., Ding, J., Tang, S., et al. (2016). Karrikins delay soybean seed germination by mediating abscisic acid and gibberellin biogenesis under shaded conditions. Sci. Rep. 6, 22073. doi: 10.1038/srep22073
Mostofa, M., Abdelrahman, M., Rahman, M., Cuong, T., Nguyen, K., Watanabe, Y., et al. (2022). Karrikin receptor KAI2 coordinates salt tolerance mechanisms in arabidopsis thaliana. Plant Cell Physiol. pcac121 doi: 10.1093/pcp/pcac121
Muchate, N. S., Nikalje, G. C., Rajurkar, N. S., Suprasanna, P., Nikam, T. D. (2016). Plant salt stress: Adaptive responses, tolerance mechanism and bioengineering for salt tolerance. Botanical Rev. 82, 371–406. doi: 10.1007/s12229-016-9173-y
Omelyanchuk, N., Kovrizhnykh, V., Oshchepkova, E. A., Pasternak, T., Palme, K., Mironova, V. (2016). A detailed expression map of the PIN1 auxin transporter in arabidopsis thaliana root. BMC Plant Biol. 16, 5. doi: 10.1186/s12870-015-0685-0
Pandey, V., Bhatt, I. D., Nandi, S. K. (2019). “Chapter 20 - role and regulation of auxin signaling in abiotic stress tolerance,” in Plant signaling molecules. Eds. Khan, M. I. R., Reddy, P. S., Ferrante, A., Khan, N. A. (Woodhead Publishing), 319–331.
Pillitteri, L. J., Torii, K. U. (2012). Mechanisms of stomatal development. Annual review of plant biology 63, 591–614. doi: 10.1146/annurev-arplant-042811-105451
Pirasteh-Anosheh, H., Saed-Moucheshi, A., Pakniyat, H., Pessarakli, M. (2016). Stomatal responses to drought stress. Water Stress and Crop Plants 24–40. doi: 10.1002/9781119054450.ch3
Ramegowda, V., Senthil-Kumar, M. (2015). The interactive effects of simultaneous biotic and abiotic stresses on plants: Mechanistic understanding from drought and pathogen combination. J. Plant Physiol. 176, 47–54. doi: 10.1016/j.jplph.2014.11.008
Rasool, S., Hameed, A., Azooz, M., Muneeb, U., Siddiqi, T., Ahmad, P. (2013). Salt stress: Causes, types and responses of plants. In book: Ecophysiology and Responses of Plants under Salt Stress. Eds. Ahmad, P., Azooz, M.M., Prasad, M.N.V. (New York, NY, Springer New York) 1–24.
Rehman, A., Almas, H., Qayyum, A., Li, H., Peng, Z., Qin, G., et al. (2021). Role of phytohormones in drought stress. Plant Growth Regulators for Climate-Smart Agriculture 81–98. doi: 10.1201/9781003109013-6
Reinhardt, D., Pesce, E. R., Stieger, P., Mandel, T., Baltensperger, K., Bennett, M., et al. (2003). Regulation of phyllotaxis by polar auxin transport. Nature 426, 255–260. doi: 10.1038/nature02081
Ribba, T., Garrido-Vargas, F., O’brien, J. A. (2020). Auxin-mediated responses under salt stress: from developmental regulation to biotechnological applications. J. Exp. Bot. 71, 3843–3853. doi: 10.1093/jxb/eraa241
Sampathkumar, A. (2020). Mechanical feedback-loop regulation of morphogenesis in plants. Development (Cambridge, England) 147, dev177964. doi: 10.1242/dev.177964
Sinha, R., Gupta, A., Senthil-Kumar, M. (2016). Understanding the impact of drought on foliar and xylem invading bacterial pathogen stress in chickpea. Front Plant Sci 7, 902. doi: 10.3389/fpls.2016.00902
Sun, X., Fu, T., Chen, N., Guo, J., Ma, J., Zou, M., et al. (2010). The stromal chloroplast Deg7 protease participates in the repair of photosystem II after photoinhibition in arabidopsis. Plant Physiol. 152, 1263–1273. doi: 10.1104/pp.109.150722
Sun, Y., Liu, Z., Guo, J., Zhu, Z., Zhou, Y., Guo, C., et al. (2020). WRKY33-PIF4 loop is required for the regulation of H2O2 homeostasis. Biochem. Biophys. Res. Commun. 527, 922–928. doi: 10.1016/j.bbrc.2020.05.041
Sun, X., Peng, L., Guo, J., Chi, W., Ma, J., Lu, C., et al. (2007). Formation of DEG5 and DEG8 complexes and their involvement in the degradation of photodamaged photosystem II reaction center D1 protein in arabidopsis. Plant Cell 19, 1347–1361. doi: 10.1105/tpc.106.049510
Takatani, S., Verger, S., Okamoto, T., Takahashi, T., Hamant, O., Motose, H. (2020). Microtubule response to tensile stress is curbed by NEK6 to buffer growth variation in the arabidopsis hypocotyl. Curr. Biol. 30, 1491–1503.e1492. doi: 10.1016/j.cub.2020.02.024
Taleisnik, E., Rodríguez, A. A., Bustos, D., Erdei, L., Ortega, L., Senn, M. E. (2009). Leaf expansion in grasses under salt stress. J. Plant Physiol. 166, 1123–1140. doi: 10.1016/j.jplph.2009.03.015
Tang, Z.-Q., Shang, J., Zhang, L., Du, J.-B., Yang, H., Zeng, S.-H., et al. (2019). Characterization of synergy between cucumber mosaic virus and alternaria alternata in nicotiana tabacum. Physiol. Mol. Plant Pathol. 108, 101404. doi: 10.1016/j.pmpp.2019.03.001
Ullah, N., Basit, A., Ahmad, I., Ullah, I., Shah, S. T., Mohamed, H. I., et al. (2020). Mitigation the adverse effect of salinity stress on the performance of the tomato crop by exogenous application of chitosan. Bull. Natl. Res. Centre 44, 181. doi: 10.1186/s42269-020-00435-4
Ullah, A., Manghwar, H., Shaban, M., Khan, A. H., Akbar, A., Ali, U., et al. (2018). Phytohormones enhanced drought tolerance in plants: a coping strategy. Environ. Sci. pollut. Res. Int. 25, 33103–33118. doi: 10.1007/s11356-018-3364-5
Wang, Y., Huan, Q., Li, K., Qian, W. (2021b). Single-cell transcriptome atlas of the leaf and root of rice seedlings. J. Genet. Genomics 48, 881–898. doi: 10.1016/j.jgg.2021.06.001
Wang, X., Niu, Y., Zheng, Y. (2021a). Multiple functions of MYB transcription factors in abiotic stress responses. International journal of molecular sciences 22 (11), 6125. doi: 10.3390/ijms22116125
Wang, P., Shen, L., Guo, J., Jing, W., Qu, Y., Li, W., et al. (2019). Phosphatidic acid directly regulates PINOID-dependent phosphorylation and activation of the PIN-FORMED2 auxin efflux transporter in response to salt stress. Plant Cell 31, 250–271. doi: 10.1105/tpc.18.00528
Wang, W., Vinocur, B., Altman, A. (2003). Plant responses to drought, salinity and extreme temperatures: Towards genetic engineering for stress tolerance. Planta 218, 1–14. doi: 10.1007/s00425-003-1105-5
Wu, R., Liu, Z., Wang, J., Guo, C., Zhou, Y., Bawa, G., et al. (2022a). COE2 is required for the root foraging response to nitrogen limitation. Int. J. Mol. Sci. 23, 861. doi: 10.3390/ijms23020861
Wu, R., Liu, Z., Wang, J., Li, W., Qin, A., Yu, X., et al. (2022b). Identification of two bZIP transcription factors that regulate development of pavement and trichome cells in arabidopsis thaliana by single-cell RNA-sequencing. bioRxiv. 2022.2004.2012.488054. doi: 10.1101/2022.04.12.488054
Xiong, Y., Jiao, Y. (2019). The diverse roles of auxin in regulating leaf development. Plants 8, 243. doi: 10.3390/plants8070243
Yang, Y., Guo, Y. (2018). Elucidating the molecular mechanisms mediating plant salt-stress responses. The New phytologist 217, 523–539. doi: 10.1111/nph.14920
Yeshi, K., Crayn, D., Ritmejerytė, E., Wangchuk, P. (2022). Plant secondary metabolites produced in response to abiotic stresses has potential application in pharmaceutical product development. Molecules (Basel, Switzerland), 27 (1), 313. doi: 10.3390/molecules27010313
Yu, Z., Duan, X., Luo, L., Dai, S., Ding, Z., Xia, G. (2020). How plant hormones mediate salt stress responses. Trends Plant Sci. 25, 1117–1130. doi: 10.1016/j.tplants.2020.06.008
van Zelm, E., Zhang, Y., Testerink, C. (2020). Salt tolerance mechanisms of plants. Annual review of plant biology 71, 403–433. doi: 10.1146/annurev-arplant-050718-100005
Zhang, H., Sun, X., Dai, M. (2022). Improving crop drought resistance with plant growth regulators and rhizobacteria: Mechanisms, applications, and perspectives. Plant Commun. 3, 100228. doi: 10.1016/j.xplc.2021.100228
Zhang, H., Xiang, Y., He, N., Liu, X., Liu, H., Fang, L., et al. (2020). Enhanced vitamin c production mediated by an ABA-induced PTP-like nucleotidase improves plant drought tolerance in arabidopsis and maize. Mol. Plant 13, 760–776. doi: 10.1016/j.molp.2020.02.005
Zhang, J., Zhang, H., Srivastava, A. K., Pan, Y., Bai, J., Fang, J., et al. (2018). Knockdown of rice MicroRNA166 confers drought resistance by causing leaf rolling and altering stem xylem development. Plant Physiol. 176, 2082–2094. doi: 10.1104/pp.17.01432
Zhou, W., Yang, Y., Zheng, C., Luo, X., Chandrasekaran, U., Yin, H., et al. (2021). Flooding represses soybean seed germination by mediating anaerobic respiration, glycometabolism and phytohormones biosynthesis. Environ. Exp. Bot. 188, 104491. doi: 10.1016/j.envexpbot.2021.104491
Zhu, X., Wang, L., Yang, R., Han, Y., Hao, J., Liu, C., et al. (2019). Effects of exogenous putrescine on the ultrastructure of and calcium ion flow rate in lettuce leaf epidermal cells under drought stress. Horticul. Environment Biotechnol. 60, 479–490. doi: 10.1007/s13580-019-00151-7
Keywords: abiotic stress, arabidopsis, epidermal cells, leaf development, PINFORMED1
Citation: Bawa G, Liu Z, Wu R, Zhou Y, Liu H, Sun S, Liu Y, Qin A, Yu X, Zhao Z, Yang J, Hu M and Sun X (2022) PIN1 regulates epidermal cells development under drought and salt stress using single-cell analysis. Front. Plant Sci. 13:1043204. doi: 10.3389/fpls.2022.1043204
Received: 13 September 2022; Accepted: 18 October 2022;
Published: 14 November 2022.
Edited by:
Mohammad Golam Mostofa, Michigan State University, United StatesReviewed by:
Shaojun Dai, Shanghai Normal University, ChinaMaksymilian Zienkiewicz, University of Warsaw, Poland
Copyright © 2022 Bawa, Liu, Wu, Zhou, Liu, Sun, Liu, Qin, Yu, Zhao, Yang, Hu and Sun. This is an open-access article distributed under the terms of the Creative Commons Attribution License (CC BY). The use, distribution or reproduction in other forums is permitted, provided the original author(s) and the copyright owner(s) are credited and that the original publication in this journal is cited, in accordance with accepted academic practice. No use, distribution or reproduction is permitted which does not comply with these terms.
*Correspondence: Xuwu Sun, c3VueHV3dXNzZEBzaW5hLmNvbQ==
†These authors have contributed equally to this work