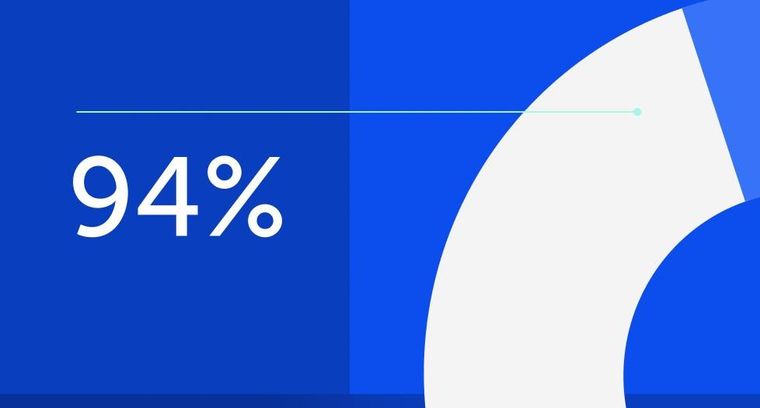
94% of researchers rate our articles as excellent or good
Learn more about the work of our research integrity team to safeguard the quality of each article we publish.
Find out more
ORIGINAL RESEARCH article
Front. Plant Sci., 28 October 2022
Sec. Plant Abiotic Stress
Volume 13 - 2022 | https://doi.org/10.3389/fpls.2022.1042855
This article is part of the Research TopicAdaptation Mechanisms of Grass and Forage Plants to Stressful Environments, Volume IIView all 12 articles
Climate change causes plants encountering several abiotic stresses simultaneously. Responses of plants to a single stress has been comprehensively studied, but it is hard to speculated infer the effects of stress combination based on these researches. Here, the response mechanism of bermudagrass to low temperature and salt treatment was investigated in this study. The results showed that low temperature (LT) treatment decreased the relative growth rate, chlorophyll fluorescence transient curve, biomass, and crude fat content of bermudagrass, whereas low temperature + salt (LT+S) treatment greatly undermined these declines. Furthermore, at 6 h and 17 d, the expression levels of glyoxalase I (GLYI), Cu-Zn/superoxide dismutase (Cu-Zn/SOD), peroxidase 2 (POD2), and oxidative enzyme 1(CAT1) in roots were considerably higher in the low temperature + salt treatment than in the low temperature treatment. Low temperature stress is more detrimental to bermudagrass, but mild salt addition can mitigate the damage by enhancing photosynthesis and improving the expression of antioxidant system genes (Cu-Zn/SOD, POD2 and CAT1) and glyoxalase system GLYI gene in roots. This study summarized the probable interaction mechanism of low temperature and salt stress on bermudagrass, which can provide beneficial reference for the growth of fodder in cold regions.
Bermudagrass, as a popular forage and warm season turfgrass, shows limited growth and begins to wilt when the daily mean temperature goes below 12°C, and then it enters the dormancy period when the temperature reaches 7-10°C (Munshaw et al., 2004; Huang et al., 2019). Low temperature restricts the establishment of bermudagrass in cold region. In addition, bermudagrass has advantages in the restoration of saline-alkali land due to resistance against salt stress (Xie et al., 2017). Therefore, bermudagrass often suffers from combination both of low temperature and salt stress.
Low temperature and salt stress lead to shared or specific physiological, metabolic and genes expression responses. It is well known that low temperature and salt stress restrain plant growth and reduce photosynthesis (Allen and Ort, 2001; Hasdai et al., 2006; Li et al., 2019; Zulfiqar and Ashraf, 2021b; Zulfiqar et al., 2022b). Plants experienced chloroplast structural damage, chlorophyll degradation, stomatal closure and enzyme activity decrease during both low temperature and salt stress (Meloni et al., 2003; Nowicka et al., 2018). Oxidative stress resulted from over accumulation of reactive oxygen species (ROS) and osmotic stress appear in plant response to both low temperature and salt stress (Hasanuzzaman et al., 2020a; Zulfiqar and Ashraf, 2021a; Zulfiqar and Ashraf, 2022a). Both oxidative and osmotic stress have negative effects on plant metabolism, molecular biosynthesis and cell viability (Mahajan and Tuteja, 2005; Liang et al., 2018; Zulfiqar et al., 2019; Munns et al., 2020; Zhao et al., 2021). Furthermore, both low temperature and salt stress cause alteration in ion homeostasis, which destroy the biological activity of membrane and some enzymes (Fürtauer et al., 2019; Shao et al., 2020). Differently, salt stress results in a toxic concentration of Na+ and inhibits absorption of K+ (Kim et al., 2007; Yang and Guo, 2018). Many specific ions transported genes are responsible for transportation of Na+ and K+, such as SOS (salt overly sensitive) family genes and HKT (high affinity transporter) (Li et al., 2019). Low temperature disturbs transmembrane H+ gradient by changing the activity of a H+ pumping protein, H+-ATPase (Ponce-Pineda et al., 2021).
Plants are equipped with fine tune resistant mechanisms to counter low temperature and salt stress (Banerjee et al., 2018). Osmoprotectants such as proline and soluble sugar are biosynthesized to remit molecular denaturation (Fürtauer et al., 2019; Zulfiqar et al., 2019; Munns et al., 2020). Plants have a complex antioxidant system, which includes enzymatic system as well as the glutathione-ascorbic acid (GSH-ASA) cycle (Bela et al., 2015). Antioxidant systems are activated to scavenge ROS. For example, antioxidant enzymes such as SOD (superoxide dismutase), POD (enzymes peroxidase) and CAT (oxidative enzyme) and their encoding genes are upregulated in the early stress response (Hasanuzzaman et al., 2020a; Hasanuzzaman et al., 2020b; Shao et al., 2020; Zulfiqar et al., 2020a; Zulfiqar and Ashraf, 2021a; Jabeen et al., 2022; Zulfiqar and Ashraf, 2022a). SOD has the ability to catalyze the conversion of superoxide anions to H2O2 and O2, which is the primary substance for scavenging free radicals in living things. H2O2 is scavenged by enzymes CAT and POD through synergistic action to maintain a stable level of free radicals in plants (Yan et al., 2010). In addition, glyoxalase (GLY) system, closely related to GSH metabolism, was reported to participate in stress responses in the past, such as salt and heavy metal stress (Yadav et al., 2005; Singla-Pareek et al., 2006; Roy et al., 2008; Singla-Pareek et al., 2008). GLY is a type of intracellular enzyme found in both prokaryotes and eukaryotes which is mostly sublocalized in the cytoplasm and organelles. GLY system has long been known in animals and is assumed to be engaged in a variety of tasks, including cell division, proliferation, and protection against oxoaldehyde toxicity (Thornalley, 1990). In plants, salt stress increased GlLYI activity, which is accompanied by MG detoxification and decrease in GSH concentration(Parvin et al., 2019).
At the moment, the global climate and environment are rapidly changing, and plants are being subjected to an increasing number of abiotic stresses (Zulfiqar and Hancock, 2020b; Zulfiqar et al., 2021c). However, plants are endowed with unique physiological responses under combined stress which are completely different from those under single stress (Bai et al., 2018; Henriet et al., 2019). Many studies have reported that the negative effects of stress interactions on crop productivity are much higher than when different stress components are applied alone (Mittler and Blumwald, 2010; Suzuki et al., 2014). The relative water content of tomato (Rong et al., 2017) and the biomass of Phragmites Karka (Abideen et al., 2022) were significantly decreased under compound stress. Drought and heat stress can lead to a significant reduction in Arabidopsis growth, but their combined stress has a more deleterious effect (Vile et al., 2012). However, other studies have reported the beneficial effects of the interaction of two different stresses applied simultaneously (Suzuki et al., 2014). For example, drought stress may offer protection against O3 damage in plants (Biswas and Jiang, 2011; Zhang et al., 2019) and resulted in reduced susceptibility to powdery mildew and Botrytis cinerea (Achuo et al., 2006). Combined stress of drought and salt can reduce boron toxicity in plants (Liu et al., 2018)
Previous research has typically focused on either low temperature or salt stress, with only a few studies have been done related to the combined effect of low temperature and salt stress on plants. Bermudagrass is a valuable forage grass and warm season turfgrass with a high salt tolerance. It is critical to investigate the physiological and molecular mechanisms of bermudagrass under the combined stress of low temperature and salt, in order to improve tolerance and lengthen the growth cycle. In this study, we used physiological and molecular methods to assess the effects of low temperature and salt stress on growth traits, photosynthesis, forage quality, and stress-related gene expression in this study. As a result, the goals of this study were to: (1) investigate the interaction of low temperature and salt on bermudagrass; and (2) elucidate the synergistic mechanism of low temperature and salt.
In this investigation, bermudagrass (Cynodon dactylon(L.) Pers.) “A12359” was used. On 6 July 2019, the bermudagrasses with uniformly cut roots were transplanted from the fields into plastic containers (4 cm in diameter and 21 cm deep) which filled with commercially available plant media, 36 pots in total were transplanted. The plants were kept in a controlled greenhouse with natural light (240 µmolm-2s-1), a 30/24°C average day/night temperature, and 50% relative humidity. The grasses were fertilized with 1/2 Hoagland nutrient solution (NH4H2PO4 (0.5 mM), KNO3 (2.5 mM), Ca (NO3)2.4H2O (2.5 mM), MgSO4.7H2O (1 mM), H3BO3 (1.43 mg), ZnSO4.7H2O (0.11 mg), CuSO4·5H2O (0.04 mg), MnCl2.4H2O (0.91 mg), H2MoO4 (0.05 mg), Fe-EDTA (0.04 mM)) twice a week. After three weeks, 24 potted plants were selected and clipped consistently to 14 cm, then placed to a plant incubator on 27 July 2019.
Control (CK), salt (S), low temperature (LT), low temperature +salt (LT+S) were the four experimental treatments. There were six pots for each treatment and a random block design was used to minimize the impact of environmental conditions. The LT and LT+S treatments were 15/10°C (day/night), while the others were 35/30°C (day/night). S and LT+S treatments were grown for 3 d with 1.0 percent salt solution, then 1.5 percent for 3 d, and finally 2.0 percent salt solution, until the material exhibited a substantial phenotype difference (17 d). Dilution of sea water yielded the saline solution. In the plant incubator, the plants were kept at 240µmolm-2s-1 photosynthetically active radiation, a 16-hour photoperiod, and 50% relative humidity. At 17 d following treatment (August 13, 2019), leaves and roots were harvested for physiological investigation, and gene expression levels in the leaves and roots were measured at 6 h and 17 d. These plants’ chlorophyll fluorescence and chlorophyll content were also measured at 3, 6 and 17 d of age.
Root length: All treated plants was measured with a ruler after roots had been washed and drained on absorbent paper.
Plant height: On d 3 (July 30, 2019), 6 (August 2, 2019) and 17 (August 13, 2019), the plant height was measured with a ruler.
Plant samples were heated in an oven at 105°C for 30 minutes before being dried to constant weight at 80°C and weighed on a 1/10000 precision balance.
We took 0.1 g of fresh leaves and cut them short (about 0.5 cm long), then placed them in a 15 ml centrifuge tube with 10 ml dimethyl sulfoxide and shaded them for 2-3 d. In a centrifuge tube, 1ml chlorophyll extract and 2 ml dimethyl sulfoxide were mixed and poured into a colorimetric dish. The absorbance is measured at 663 nm and 645 nm wavelengths using dimethyl sulfoxide as the blank. Three potted plants were collected per treatment.
A pulse-amplitude modulation fluorometer was used to create a fluorescence transient of chlorophyll a. (PAM2500, Heinz Walz GmbH). After 30 minutes of dark adaptation, the shoots (same position of plants in each treatment) were exposed to a red light of 3,000μmol photons m-2s-1. There were three replicates from different potted plants of each treatment. JIP-test was used as Table 1 to better analyze the OJIP curve.
Na+ and K+: We weighed 0.1 g of the material into the desiccating tube, added 10 ml H2SO4, and placed it in the graphite digesting device. The amount of Na+ and K+ was determined using a flame spectrophotometer after the desiccated sample was diluted 100 times. For each treatment, three biological replicates collected from different potted plants.
For each treatment, three biological replicates were taken from different potted plants. Trizol reagent (Invitrogen, America) was used to isolated total RNA from about 0.1 g samples of leaves and roots. DNasel was used to remove contaminating genomic DNA from RNA. A UV spectrophotometry NanoDrop was used to examine the RNA concentration and purity (Thermo Fisher Scientific, Lenexa, Ks, USA). Using a Hifair III 1st Strand cDNA Synthesis SuperMix for qPCR with genome-DNA-removing enzyme, 2.5 ug RNA was reverse transcribed to cDNA (Yesen, Nanjing, China). The qPCR was carried out on a Quant Studio 6 detection system (ABI, Forster City, CA, USA) with a SYBR green PCR mix (Takara, RR420A, Shika, Japan). The following was the real-time PCR program: 95°C for 5 minutes; 40 cycles of 95°C for 10 seconds and 60°C for 30 seconds. Table 2 contains a list of primers. For gene expression level analysis, the bermudagrass Actin gene was used as an inner control, and the comparative Ct method was used.
All treatments were repeated three times. All the data was subjected to analysis of variance (AVOVA) with the Duncan’s multiple range tests means at a significant level of P<0.05 using the statistical package SPSS 16.0, Origin Pro 9.0 and Excel 2019 for Windows.
Bermudagrass growth was hindered under the three stress regimes. However, the development of bermudagrass under the LT+S treatment was superior to that under the LT treatment (Figure 1A). LT treatment and LT+S treatment drastically decreased the relative growth rate of shoot compared to control (Figure 1B). In comparison to the relative growth rate of shoots under 1.0 percent salt concentration, the relative growth rate of shoots under 1.5 percent and 2.0 percent salt concentration was further reduced with the continuous increase of salt concentration. However, there was no statistically significant difference in relative growth rate between combined stress and LT treatment (Figure 1B). When plants were subjected to salt and low temperature stress, the length of their roots exhibited an obvious elongation character (Figure 1C). Plant shoots dry weight decreased in all three stress treatments when compared to the control, and there was no difference between the LT and LT+S treatments (Figure 1D). The root dry weight increased significantly after salt treatment alone, and combined stress alleviates single low temperature damage (Figure 1E).
Figure 1 Bermudagrass phenotypic features under low temperature and salt stress. (A) Image of bermudagrass plants. (B) Relative growth rate of shoot. (C) Root length. (D) Shoot dry weight. (E) Root dry weight. Bermudagrass was transplanted into black plastic tubes after being clipped to a uniform length at the root. The plant height was reduced to the same height after a time of cultivation in a greenhouse. Duncan’ s multiple range tests show that different letters above the same columns imply statistically significant differences at P < 0.05.
The OJIP fluorescence transient curves were plotted in Figure 2 to show the effects of low temperature and salt on the photosynthetic efficiency of bermudagrass. The chlorophyll fluorescence transient response curve did not change significantly when treated with 1.0 percent salt compared to the control, but it was significantly reduced when treated with low temperature (Figure 2A). Furthermore, in the 2.0 percent range, the LT+S treatment curve rose with increasing salt concentration and was significantly higher than the LT treatment alone (Figure 2C). The values of basic fluorescence parameters were extracted from the recorded OJIP curve, and several structural and functional parameters were calculated and analyzed (Table 1). When salt content was less than 2.0 percent, area, φP0 and φE0 was greatly reduced under low temperature compared to the control, but significantly increased under LT+S stress compared to the LT treatment. All of these findings suggest that salt treatment can help plants recover from the effects of low temperature treatment. At salt concentrations of 1.0 percent and 1.5 percent, there was no difference in chlorophyll content between the LT+S treatment and the LT treatment, but at 2.0 percent, the chlorophyll content of the LT+S treatment was much lower than that of the LT treatment (Figure 2D). To summarize, combined stress affects photosynthetic performance by regulating PSII rather than chlorophyll content.
Figure 2 Effects of salt and cold treatment on photosynthetic parameters. (A–C). Transient changes in chlorophyll fluorescence in bermudagrass leaves under 1.0 percent (A), 1.5 percent (B) and 2.0 percent (C) salt treatment. (D) Chlorophyll content. Different letters above the same columns represent statistically significant differences at P < 0.05 (Duncan’ s multiple range test).
We measured the concentration of Na+ and K+ to further investigate the effects of salt and low temperature on bermudagrass. In leaves, the Na+ concentration increased significantly under salt stress compared with the control, and there was no difference between LT+S and S treatments (Figure 3A). However, K+ concentration in leaves increased remarkably under LT+S treatment, while there was no difference under low temperature and salt stress alone compared with the control (Figure 3C). In roots, Na+ and K+ concentration increased significantly under salt stress compared with the control, while them decreased significantly under LT+S treatment compared with the salt treatment (Figures 3B, 3D). Finally, compared with salt stress, the ratio of Na+/K+ in leaves was significantly decreased under combined stress and there was no difference in Na+/K+ ratio in roots under all stress conditions (Figures 3E, F).
Figure 3 The bar chart shows the average Na+ concentration (A, B), K+ concentration (C, D), and Na+/K+ ratio (E, F) of bermudagrass under each treatment, with three replicates per treatment. Different letters above the same columns indicate statistic significant difference at P < 0.05 (Duncan’ s multiple range test).
GLYI, Cu-Zn/SOD, POD2 and CAT1 expressions were measured to investigate the role of GLY and antioxidant system under low temperature and salt stress. GLYI was upregulated in both leaves and roots after 6 h of salt treatment, but it was no longer up-regulated after 17 d of salt treatment (Figures 4A, B). At 6 h or 17 d, LT treatment had no effect on GLYI in leaves but significantly reduced GLYI in roots (Figures 4A, B). GLYI expression in roots was significantly increased after 6 h and 17 d of LT+S treatment when compared to LT treatment (Figure 4B). Similarly, antioxidant-related genes (Cu-Zn/SOD, POD2, and CAT1) were downregulated in roots after 6 h and 17 d of LT treatment (Figures 4D, F, H). The combination of salt and LT, on the other hand, suppressed the expression of antioxidant-related genes. Cu-Zn/SOD and CAT1 expression levels in leaves exhibited no difference between control and low temperature treatment after 6 h, but were down-regulated after 17 d (Figures 4C, G). In comparison to the control treatment, the expression of POD2 in leaves rose considerably after 17 d of low temperature treatment (Figure 4E). When compared to low temperature treatment alone, combined stress does not further the expression of these genes. In conclusion, combined stress reduces low temperature stress in roots via regulating these genes.
Figure 4 Glyoxalase and antioxidant enzyme related genes transcriptional level in bermudagrass under low temperature and salt stress. (A, C, E, G) represents genes transcriptional level in leaves and Figure (B, D, F–H) represents genes transcriptional level in roots. Different letters above the same columns indicate statistic significant difference at P < 0.05 (Duncan’ s multiple range test).
We measured the quality indexes to further investigate the effects of salt and low temperature on bermudagrass. The effect of salt treatment alone on the nutritive value of forage was insignificant. The crude fat content was significantly lower in the LT and LT+S treatments compared to the control, but significantly higher in the LT+S treatment compared to the LT treatment alone, which was consistent with the fluorescence curve results (Figure 5A). Unlike crude fat, crude protein was significantly increased under LT+S conditions (Figure 5B). Only the LT+S treatment significantly reduced crude fiber (Figure 5C). It’s worth mentioning that under the combined stress condition, the forage quality of the bermudagrass did not degrade any more compared to the low temperature treatment, but the crude fat rose.
This article looked into the defensive mechanism of bermudagrass under low temperatures and salt stress. Plants response to stress is a complex process including morphology, physiology, and biochemistry (Huang et al., 2019). Low temperature-treated bermudagrass showed reduced relative growth rate in shoots, shoot dry weight, and increased root length in previous research (Esmaili and Salehi, 2012), which matched our findings (Figures 1B, E). From the standpoint of plant growth phenotype, bermudagrass under the LT+S treatment is superior to that of low temperature treatment, the root dry weight reflects the same situation. Relative growth rate, shoot dry weight and root length under low temperature and low temperature + salt treatment shows no discernible differences (Figures 1A-E). All of this suggests that the combined stress did not produce more substantial harm to the plants; rather, a moderate amount of salt may have mitigated the damage caused by low temperatures. Maintaining proper balance of Na+/K+ and higher K+ concentration is considered as an important mechanism for plants to response to salt stress (Evelin et al., 2009). Lower Na+/K+ is a marker of salinity tolerance for plants (van Zelm et al., 2020). In salt-tolerant plants, K+ efflux can be significantly inhibited to maintain stable Na+/K+ and reduce salt stress injury (Yang and Guo, 2018). In our study, Na+/K+ remained stable and K+ concentration increased in root under salt stress (Figures 3D, F), so it can be inferred that bermudagrass A12359 has a certain salinity tolerance, which is the reason that it can alleviate the damage of low temperature to bermudagrass at the high salt concentration (2.0%).
Low temperature stress affects a variety of physiological processes in plants, the most susceptible of which is photosynthesis (Stitt and Hurry, 2002). We looked at photosynthetic indexes at the third, sixth and seventeenth d to learn more about how bermudagrass protects itself under low temperatures and different salt concentrations. The PSII reaction center becomes sensitive under stress, and the OJIP fluorescence transient curves and chlorophyll fluorescence characteristics can accurately reflect PSII’s physiological status (Chen et al., 2021). The electron transport activities (PSI and PSII) of the chloroplast thylakoid membrane were found to be dramatically reduced at low temperatures, with PSII being more vulnerable to cold pressure than PSI (Shi et al., 2016). The chlorophyll fluorescence transient response curve decreased under the three stress conditions as processing time at low temperature and salt concentration increased, compared to control, in our studies, but it decreased more noticeably under the LT treatment, and the fluorescence curve is higher under the LT+S treatment than the LT treatments when the salt concentration reaches 1.5 percent and 2.0 percent (Figures 2B, C).The findings revealed that an optimal salt concentration could help to relieve photosynthetic physiology in cold-stressed plants (Figures 2A, C). Low temperature stress causes a drop in E0, which is mostly influenced by alterations in the PSII receptor side (Van Heerden et al., 2003). φE0 represents the reaction center of absorbed light quantum yield for electron transfer, and the higher the value, the more stressed the plants are. In this study, φE0 significantly decreased under LT stress while improving under LT+S treatment, and the same changes were observed in area and φP0. All of these results suggest that when LT+S was used instead of LT stress, electron transfer efficiency improved, and the effect of low temperature stress alone on plant photosynthesis was reduced.
Plants respond to salt, severe temperatures, and other factors through the GLY system, which consists of GlyI, GlyII, and GSH (coenzyme). The detoxification of methylglyoxal, a by-product of carbohydrate metabolism, is its primary physiological role (Roy et al., 2008). In this study, since the expression levels of GLYI and antioxidant oxidases related genes change early under stress conditions (Wang et al., 2020; Zhang et al., 2021), the gene expression levels were measured once after 6 h of treatment, and then again at the end of the experiment (17d), so as to observe the gene expression changes. Under salt, mannitol and heavy metal stress, GLYI expression in mustard was dramatically increased (Veena et al., 1999). GLYI was considerably elevated in both roots and leaves after 6 h of salt treatment in our experiment (Figures 4A, B), which is consistent with earlier research. Low temperature causes mechanical constraints-membrane damage, whereas salinity causes malignancy by disrupting the ion and osmotic balance of cells (Mahajan and Tuteja, 2005). Osmotic stress can particularly promote the production of GLYI, at which point the GLY system is triggered to repair the damage (Inoue et al., 1998). Low temperature stress was found to increase the levels of reactive oxygen species (ROS) and lower enzyme antioxidant activity in plants (Aroca et al., 2005; Sasaki et al., 2007), affecting the antioxidant system. In our study, antioxidant enzyme genes (Cu-Zn/SOD, POD2, and CAT1) in roots were significantly down-regulated under low temperature stress (Figures 4D, F, H). When exposed to low temperature stress alone, the expression of GLYI in leaves does not rise and even decreases dramatically in roots, and several indicators reveal that the injury to plants is highest at this time. However, when compared to low temperature treatment, the expression level of GLYI was higher during LT+S stress, indicating that the GLY system was activated again, reducing the damage caused by low temperature. Furthermore, the GLY system raises the level of free reduced glutathione, which is necessary for the removal of harmful reactive oxygen species (e.g., H2O2) and organic peroxides that accumulate in stressed plants, as well as the maintenance of other antioxidants (Frendo et al., 2013). Despite the roles of antioxidant enzymes and GLY in stress are clearly, we indicated that these genes may involve in stress acclimation to several different environmental adversity.
Crude protein and crude fiber are two important indexes for measuring the nutritional value of herbage, as well as important contents for improving herbage quality. The first selection factors for high quality forage are high crude protein content and low crude fiber content (Barth, 2012). Previous research has shown that plants in low temperature environments reduce structural carbohydrate content while increasing soluble carbohydrate and protein content to minimize plant harm (Vo and Johnson, 2001). In our experiment, compared to the control, bermudagrass protein content increased significantly under LT and LT+S treatment (Figure 5B). This phenomenon could be explained by the fact that the LT treatment was set at 10 -15°C and the cells were subjected to low temperature stress but did not reach the point where the fluidity of the cell membrane becomes weak and rigidity increases. At this point, the cell membrane’s phase transition reduces the ability of the membrane protein to bind to phospholipids and causes it to become free protein, resulting in an increase in protein content in the plant. Crude fat is a nutrient with a high calorific value that can enhance feed palatability (Li et al., 2019). According to the test results, the crude fat content was lowest under low temperature treatment and significantly higher under low temperature + salt treatment (Figure 5A), proving once again that appropriate salt can mitigate the damage caused by low temperature.
Figure 5 The bar chart shows the average of crude fat (A), crude protein (B), and crude fiber (C) content of bermudagrass under each treatment, with three replicates per treatment. Different letters above the same columns indicate statistic significant difference at P < 0.05 (Ducan’ s multiple range test).
At present, plants are subjected to more and more abiotic stresses (Zulfiqar and Hancock, 2020b; Zulfiqar et al., 2021c), and the physiological responses under combined stress and single stress are completely different. Therefore, the changes of phenotype, photosynthesis, Na+, K+, gene expression and forage quality under single salt, low temperature stress and combined stress were analyzed in this study to explore the interaction between low temperature and salinity on bermudagrass. It was found that low temperatures cause more damage to bermudagrass, but moderate salt addition can mitigate the damage by enhancing photosynthesis, improving the expression of antioxidant system genes (Cu-Zn/SOD, POD2 and CAT1) and glyoxalase system GLYI gene in roots and thus improves forage quality. This provides putative pathways improving turfgrass and forage tolerance to combination stress.
The original contributions presented in the study are included in the article/supplementary material. Further inquiries can be directed to the corresponding author.
All authors contributed largely to the work presented in this article. Conceived and designed the experiments: JF. Performed the experiments XZ, GW, XL, YX. Analyzed the data: XZ, YY. Language modification: EA. Wrote the paper: XZ, YY. All authors contributed to the article and approved the submitted version.
This work was supported by National Key R&D Program of China (2019YFD0900702) and Agricultural Variety Improvement Project of Shandong Province (2019LZGC010).
The authors declare that the research was conducted in the absence of any commercial or financial relationships that could be construed as a potential conflict of interest.
All claims expressed in this article are solely those of the authors and do not necessarily represent those of their affiliated organizations, or those of the publisher, the editors and the reviewers. Any product that may be evaluated in this article, or claim that may be made by its manufacturer, is not guaranteed or endorsed by the publisher.
CK, control treatment; S, salt treatment; LT, low temperature treatment; LT+S, low temperature + salt treatment; GLY, glyoxalase; SOD, superoxide dismutase; POD, peroxidase; CAT, oxidative enzyme; MG, methylglyoxal; GSH, glutathione; ASA, ascorbic acid; SOS, salt overly sensitive; HKT, high affinity transporter.
Abideen, Z., Koyro, H. W., Hussain, T., Rasheed, A., Alwahibi, M. S., Elshikh, M. S., et al. (2022). Biomass production and predicted ethanol yield are linked with optimum photosynthesis in phragmites karka under salinity and drought conditions. Plants (Basel) 11 (13), 1657. doi: 10.3390/plants11131657
Achuo, E. A., Prinsen, E., Höfte, M. (2006). Influence of drought, salt stress and abscisic acid on the resistance of tomato to botrytis cinerea and oidium neolycopersici. Plant Pathol. 55, 178–186. doi: 10.1111/j.1365-3059.2006.01340.x
Allen, D. J., Ort, D. R. (2001). Impacts of chilling temperatures on photosynthesis in warm-climate plants. Trends Plant Sci. 6 (1), 36–42. doi: 10.1016/s1360-1385(00)01808-2
Aroca, R., Amodeo, G., Fernández-Illescas, S., Herman, E. M., Chaumont, F., Chrispeels, M. J. (2005). The role of aquaporins and membrane damage in chilling and hydrogen peroxide induced changes in the hydraulic conductance of maize roots. Plant Physiol. 137 (1), 341–353. doi: 10.1104/pp.104.051045
Bai, Y., Kissoudis, C., Yan, Z., Visser, R., van der Linden, G. (2018). Plant behaviour under combined stress: tomato responses to combined salinity and pathogen stress. Plant J. 93 (4), 781–793. doi: 10.1111/tpj.13800
Banerjee, A., Tripathi, D. K., Roychoudhury, A. (2018). Hydrogen sulphide trapeze: Environmental stress amelioration and phytohormone crosstalk. Plant Physiol. Biochem. 132, 46–53. doi: 10.1016/j.plaphy.2018.08.028
Barth, S. (2012). Breeding strategies for forage and grass improvement. Ann. Bot. 110 (6), 1261–1262. doi: 10.1093/aob/mcs219
Bela, K., Horváth, E., Gallé, Á., Szabados, L., Tari, I., Csiszár, J. (2015). Plant glutathione peroxidases: emerging role of the antioxidant enzymes in plant development and stress responses. J. Plant Physiol. 176, 192–201. doi: 10.1016/j.jplph.2014.12.014
Biswas, D. K., Jiang, G. M. (2011). Differential drought-induced modulation of ozone tolerance in winter wheat species. J. Exp. Bot. 62 (12), 4153–4162. doi: 10.1093/jxb/err104
Chen, X., Zhou, Y., Cong, Y., Zhu, P., Xing, J., Cui, J., et al. (2021). Ascorbic acid-induced photosynthetic adaptability of processing tomatoes to salt stress probed by fast OJIP fluorescence rise. Front. Plant Sci. 12. doi: 10.3389/fpls.2021.594400
Esmaili, S., Salehi, H. (2012). Effects of temperature and photoperiod on postponing bermudagrass (cynodon dactylon [l.] pers.) turf dormancy. J. Plant Physiol. 169 (9), 851–858. doi: 10.1016/j.jplph.2012.01.022
Evelin, H., Kapoor, R., Giri, B. (2009). Arbuscular mycorrhizal fungi in alleviation of salt stress: a review. Ann. Bot. 104, 1263–1280. doi: 10.1093/aob/mcp251
Frendo, P., Baldacci-Cresp, F., Benyamina, S. M., Puppo, A. (2013). Glutathione and plant response to the biotic environment. Free Radic. Biol. Med. 65, 724–730. doi: 10.1016/j.freeradbiomed.2013.07.035
Fürtauer, L., Weiszmann, J., Weckwerth, W., Nägele, T. (2019). Dynamics of plant metabolism during cold acclimation. Int. J. Mol. Sci. 20 (21), 5411. doi: 10.3390/ijms20215411
Hasanuzzaman, M., Bhuyan, M., Zulfiqar, F., Raza, A., Mohsin, S. M., Mahmud, J. A., et al. (2020a). Reactive oxygen species and antioxidant defense in plants under abiotic stress: Revisiting the crucial role of a universal defense regulator. Antioxid. (Basel) 9 (8), 681. doi: 10.3390/antiox9080681
Hasanuzzaman, M., Bhuyan, M., Parvin, K., Bhuiyan, T. F., Anee, T. I., Nahar, K., et al. (2020b). Regulation of ROS metabolism in plants under environmental stress: A review of recent experimental evidence. Int. J. Mol. Sci. 21 (22), 8695. doi: 10.3390/ijms21228695
Hasdai, M., Weiss, B., Levi, A., Samach, A., Porat, R. (2006). Differential responses of arabidopsis ecotypes to cold, chilling and freezing temperatures. Ann. Appl. Biol. 148 (2), 113–120. doi: 10.1111/j.1744-7348.2006.00044.x
Henriet, C., Aimé, D., Térézol, M., Kilandamoko, A., Rossin, N., Combes-Soia, L., et al. (2019). Water stress combined with sulfur deficiency in pea affects yield components but mitigates the effect of deficiency on seed globulin composition. J. Exp. Bot. 70 (16), 4287–4304. doi: 10.1093/jxb/erz114
Huang, S., Jiang, S., Liang, J., Chen, M., Shi, Y. (2019). Current knowledge of bermudagrass responses to abiotic stresses. Breed Sci. 69 (2), 215–226. doi: 10.1270/jsbbs.18164
Inoue, Y., Tsujimoto, Y., Kimura, A. (1998). Expression of the glyoxalase I gene of saccharomyces cerevisiae is regulated by high osmolarity glycerol mitogen-activated protein kinase pathway in osmotic stress response. J. Biol. Chem. 273 (5), 2977–2983. doi: 10.1074/jbc.273.5.2977
Jabeen, R., Iqbal, A., Deeba, F., Zulfiqar, F., Mustafa, G., Nawaz, H., et al. (2022). Isolation and characterization of peroxidase P7-like gene and rab-GDI like gene from potential medicinal plants: A step toward understanding cell defense signaling. Front. Plant Sci. 13. doi: 10.3389/fpls.2022.975852
Kim, B. G., Waadt, R., Yong, H. C., Pandey, G. K., Sheng, L. (2007). The calcium sensor cbl10 mediates salt tolerance by regulating ion homeostasis in arabidopsis. Plant J. 52 (3), 473–484. doi: 10.1111/j.1365-313X.2007.03249.x
Liang, W., Ma, X., Wan, P., Liu, L. (2018). Plant salt-tolerance mechanism: A review. Biochem. Biophys. Res. Commun. 495 (1), 286–291. doi: 10.1016/j.bbrc.2017.11.043
Li, N., Du, C., Ma, B., Gao, Z., Wu, Z., Zheng, L., et al. (2019). Functional analysis of ion transport properties and salt tolerance mechanisms of RtHKT1 from the recretohalophyte reaumuria trigyna. Plant Cell Physiol. 60 (1), 85–106. doi: 10.1093/pcp/pcy187
Liu, C., Dai, Z., Xia, J., Chang, C., Sun, H. (2018). Combined effect of salt and drought on boron toxicity in puccinellia tenuiflora. Ecotoxicol. Environ. Saf. 157, 395–402. doi: 10.1016/j.ecoenv.2018.03.061
Li, S., Wang, N., Ji, D., Zhang, W., Wang, Y., Yu, Y., et al. (2019). A GmSIN1/GmNCED3s/GmRbohBs feed-forward loop acts as a signal amplifier that regulates root growth in soybean exposed to salt stress. Plant Cell 31 (9), 2107–2130. doi: 10.1105/tpc.18.00662
Mahajan, S., Tuteja, N. (2005). Cold, salinity and drought stresses: an overview. Arch. Biochem. Biophys. 444 (2), 139–158. doi: 10.1016/j.abb.2005.10.018
Meloni, D. A., Oliva, M. A., Martinez, C. A., Cambraia, J. (2003). Photosynthesis and activity of superoxide dismutase, peroxidase and glutathione reductase in cotton under salt stress. Environ. Exp. Bot. 49 (1), 69–76. doi: 10.1016/s0098-8472(02)00058-8
Mittler, R., Blumwald, E. (2010). Genetic engineering for modern agriculture: challenges and perspectives. Annu. Rev. Plant Biol. 61, 443–462. doi: 10.1146/annurev-arplant-042809-112116
Munns, R., Passioura, J. B., Colmer, T. D., Byrt, C. S. (2020). Osmotic adjustment and energy limitations to plant growth in saline soil. New Phytol. 225 (3), 1091–1096. doi: 10.1111/nph.15862
Munshaw, G. C., Zhang, X., Ervin, E. H. (2004). Effect of salinity on bermudagrass cold hardiness. HortScience 39, 420–423. doi: 10.1023/B:EJPP.0000021072.89968.de
Nowicka, B., Ciura, J., Szymańska, R., Kruk, J. (2018). Improving photosynthesis, plant productivity and abiotic stress tolerance - current trends and future perspectives. J. Plant Physiol. 231, 415–433. doi: 10.1016/j.jplph.2018.10.022
Parvin, K., Hasanuzzaman, M., Bhuyan, M., Nahar, K., Mohsin, S. M., Fujita, M. (2019). Comparative physiological and biochemical changes in tomato (Solanum lycopersicum l.) under salt stress and recovery: Role of antioxidant defense and glyoxalase systems. Antioxid. (Basel) 8 (9), 350. doi: 10.3390/antiox8090350
Ponce-Pineda, I. G., Carmona-Salazar, L., Saucedo-García, M., Cano-Ramírez, D., Morales-Cedillo, F., Peña-Moral, A., et al. (2021). MPK6 kinase regulates plasma membrane h+-ATPase activity in cold acclimation. Int. J. Mol. Sci. 22 (12), 6338. doi: 10.3390/ijms22126338
Rong, Z., Yu, X., Ottosen, C. O., Rosenqvist, E., Zhen, W. (2017). Drought stress had a predominant effect over heat stress on three tomato cultivars subjected to combined stress. BMC Plant Biol. 17 (1), 24. doi: 10.1186/s12870-017-0974-x
Roy, S. D., Saxena, M., Bhomkar, P. S., Pooggin, M., Hohn, T., Bhalla-Sarin, N. (2008). Generation of marker free salt tolerant transgenic plants of arabidopsis thaliana using the gly i gene and cre gene under inducible promoters. Plant Cell Tissue Organ Culture 95 (1), 1–11. doi: 10.1007/s11240-008-9402-0
Sasaki, K., Kim, M. H., Imai, R. (2007). Arabidopsis COLD SHOCK DOMAIN PROTEIN2 is a RNA chaperone that is regulated by cold and developmental signals. Biochem. Biophys. Res. Commun. 364 (3), 633–638. doi: 10.1016/j.bbrc.2007.10.059
Shao, A., Sun, Z., Fan, S., Xu, X., Wang, W., Amombo, E., et al. (2020). Moderately low nitrogen application mitigate the negative effects of salt stress on annual ryegrass seedlings. PeerJ 8, e10427. doi: 10.7717/peerj.10427
Shi, W., Wei, X., Chen, G. X. (2016). Effects of low temperature on photosynthetic characteristics in the super-high-yield hybrid rice 'liangyoupeijiu' at the seedling stage. Genet. Mol. Res. 15 (4). doi: 10.4238/gmr15049021
Singla-Pareek, S. L., Yadav, S. K., Pareek, A., Reddy, M. K., Sopory, S. K. (2008). Enhancing salt tolerance in a crop plant by overexpression of glyoxalase II. Transgenic Res. 17 (2), 171–180. doi: 10.1007/s11248-007-9082-2
Singla-Pareek, S. L., Yadav, S. K., Pareek, A., Sopory, M. K. R. K. (2006). Transgenic tobacco overexpressing glyoxalase pathway enzymes grow and set viable seeds in zinc-spiked soils. Plant Physiol. 140 (2), 613–623. doi: 10.1104/pp.105.073734
Stitt, M., Hurry, V. (2002). A plant for all seasons: alterations in photosynthetic carbon metabolism during cold acclimation in arabidopsis. Curr. Opin. Plant Biol. 5 (3), 199–206. doi: 10.1016/s1369-5266(02)00258-3
Suzuki, N., Rivero, R. M., Shulaev, V., Blumwald, E., Mittler, R. (2014). Abiotic and biotic stress combinations. New Phytol. 203 (1), 32–43. doi: 10.1111/nph.12797
Thornalley, P. J. (1990). The glyoxalase system: new developments towards functional characterization of a metabolic pathway fundamental to biological life. Biochem. J. 269 (1), 1–11. doi: 10.1042/bj2690001
Van Heerden, P. D., Tsimilli-Michael, M., Krüger, G. H., Strasser, R. J. (2003). Dark chilling effects on soybean genotypes during vegetative development: parallel studies of CO2 assimilation, chlorophyll a fluorescence kinetics O-J-I-P and nitrogen fixation. Physiol. Plant 117 (4), 476–491. doi: 10.1034/j.1399-3054.2003.00056.x
van Zelm, E., Zhang, Y., Testerink, C. (2020). Salt tolerance mechanisms of plants. Annu. Rev. Plant Biol. 71, 403–433. doi: 10.1146/annurev-arplant-050718-100005
Veena, Reddy, V. S., Sopory, S. K. (1999). Glyoxalase i from brassica juncea: molecular cloning, regulation and its over-expression confer tolerance in transgenic tobacco under stress. Plant J. 17 (4), 385–395. doi: 10.1046/j.1365-313x.1999.00390.x
Vile, D., Pervent, M., Belluau, M., Vasseur, F., Bresson, J., Muller, B., et al. (2012). Arabidopsis growth under prolonged high temperature and water deficit: independent or interactive effects? Plant Cell Environ. 35 (4), 702–718. doi: 10.1111/j.1365-3040.2011.02445.x
Vo, S., Johnson, E. A. (2001). Alpine plant life: Functional plant ecology of high mountain ecosystems. MT Res. Dev. 21 (2), 202–202. doi: 10.1659/0276-4741(2001)021[0202:APLFPE]2.0.CO;2
Wang, W., Shao, A., Amombo, E., Fan, S., Xu, X., Fu, J. (2020). Transcriptome-wide identification of MAPKKK genes in bermudagrass (Cynodon dactylon l.) and their potential roles in low temperature stress responses. PeerJ 8, e10159. doi: 10.7717/peerj.10159
Xie, Y., Han, S., Li, X., Amombo, E., Fu, J. (2017). Amelioration of salt stress on bermudagrass by the fungus aspergillus aculeatus. Mol. Plant Microbe Interact. 30 (3), 245–254. doi: 10.1094/MPMI-12-16-0263-R
Yadav, S. K., Singla-Pareek, S. L., Ray, M., Reddy, M. K., Sopory, S. K. (2005). Methylglyoxal levels in plants under salinity stress are dependent on glyoxalase i and glutathione. Biochem. Biophys. Res. Commun. 337 (1), 61–67. doi: 10.1016/j.bbrc.2005.08.263
Yang, Y., Guo, Y. (2018). Elucidating the molecular mechanisms mediating plant salt-stress responses. New Phytol. 217 (2), 523–539. doi: 10.1111/nph.14920
Yan, F., Mu, Y., Yan, G., Liu, J., Shen, J., Luo, G. (2010). Antioxidant enzyme mimics with synergism. Mini Rev. Med. Chem. 10 (4), 342–356. doi: 10.2174/138955710791330972
Yusuf, M. A., Kumar, D., Rajwanshi, R., Strasser, R. J., Tsimilli-Michael, M., Govindjee, et al. (2010). Overexpression of gamma-tocopherol methyl transferase gene in transgenic brassica juncea plants alleviates abiotic stress: physiological and chlorophyll a fluorescence measurements. Biochim. Biophys. Acta 1797 (8), 1428–1438. doi: 10.1016/j.bbabio.2010.02.002
Zhang, P., Duo, T., Wang, F., Zhang, X., Yang, Z., Hu, G. (2021). De novo transcriptome in roots of switchgrass (Panicum virgatum l.) reveals gene expression dynamic and act network under alkaline salt stress. BMC Genomics 22 (1), 82. doi: 10.1186/s12864-021-07368-w
Zhang, J., Gao, F., Jia, H., Hu, J., Feng, Z. (2019). Molecular response of poplar to single and combined ozone and drought. Sci. Total Environ. 655, 1364–1375. doi: 10.1016/j.scitotenv.2018.11.195
Zhao, S., Zhang, Q., Liu, M., Zhou, H., Ma, C., Wang, P. (2021). Regulation of plant responses to salt stress. Int. J. Mol. Sci. 22 (9), 4609. doi: 10.3390/ijms22094609
Zulfiqar, F., Akram, N. A., Ashraf, M. (2019). Osmoprotection in plants under abiotic stresses: new insights into a classical phenomenon. Planta 251 (1), 3. doi: 10.1007/s00425-019-03293-1
Zulfiqar, F., Ashraf, M. (2021a). Bioregulators: unlocking their potential role in regulation of the plant oxidative defense system. Plant Mol. Biol. 105 (1-2), 11–41. doi: 10.1007/s11103-020-01077-w
Zulfiqar, F., Ashraf, M. (2021b). Nanoparticles potentially mediate salt stress tolerance in plants. PPB 160, 257–268. doi: 10.1016/j.plaphy.2021.01.028
Zulfiqar, F., Ashraf, M. (2022a). Antioxidants as modulators of arsenic-induced oxidative stress tolerance in plants: An overview. J. Hazard Mater 427, 127891. doi: 10.1016/j.jhazmat.2021.127891
Zulfiqar, F., Casadesús, A., Brockman, H., Munné-Bosch, S. (2020a). An overview of plant-based natural biostimulants for sustainable horticulture with a particular focus on moringa leaf extracts. Plant Sci. 295, 110194. doi: 10.1016/j.plantsci.2019.110194
Zulfiqar, F., Hancock, J. T. (2020b). Hydrogen sulfide in horticulture: Emerging roles in the era of climate change. PPB 155, 667–675. doi: 10.1016/j.plaphy.2020.08.010
Zulfiqar, F., Nafees, M., Chen, J., Darras, A., Ferrante, A., Hancock, J. T., et al. (2022b). Chemical priming enhances plant tolerance to salt stress. Front. Plant Sci. 13. doi: 10.3389/fpls.2022.946922
Keywords: bermudagrass, low temperature, salt, photosynthesis, antioxidant, glyoxalase
Citation: Zhou X, Yin Y, Wang G, Amombo E, Li X, Xue Y and Fu J (2022) Mitigation of salt stress on low temperature in bermudagrass: resistance and forage quality. Front. Plant Sci. 13:1042855. doi: 10.3389/fpls.2022.1042855
Received: 13 September 2022; Accepted: 11 October 2022;
Published: 28 October 2022.
Edited by:
Jin-Lin Zhang, Lanzhou University, ChinaReviewed by:
Peng Zhou, Shanghai Jiao Tong University, ChinaCopyright © 2022 Zhou, Yin, Wang, Amombo, Li, Xue and Fu. This is an open-access article distributed under the terms of the Creative Commons Attribution License (CC BY). The use, distribution or reproduction in other forums is permitted, provided the original author(s) and the copyright owner(s) are credited and that the original publication in this journal is cited, in accordance with accepted academic practice. No use, distribution or reproduction is permitted which does not comply with these terms.
*Correspondence: Jinmin Fu, dHVyZmNuQHFxLmNvbQ==
†These authors have contributed equally to this work
Disclaimer: All claims expressed in this article are solely those of the authors and do not necessarily represent those of their affiliated organizations, or those of the publisher, the editors and the reviewers. Any product that may be evaluated in this article or claim that may be made by its manufacturer is not guaranteed or endorsed by the publisher.
Research integrity at Frontiers
Learn more about the work of our research integrity team to safeguard the quality of each article we publish.