- 1National Engineering Laboratory for Endangered Medicinal Resource Development in Northwest China, Key Laboratory of Medicinal Resources and Natural Pharmaceutical Chemistry of Ministry of Education, College of Life Sciences, Shaanxi Normal University, Xi’an, China
- 2Department of Plant Pathology, Nanjing Agricultural University, Nanjing, China
- 3Fujian Key Laboratory for Monitoring and Integrated Management of Crop Pests, Institute of Plant Protection, Fujian Academy of Agricultural Sciences, Fuzhou, China
Ralstonia solanacearum causes devastating diseases in a wide range of economically important crops. It secretes a large number of virulence factors, also known as effectors, to promote its infection, and some of them are recognized when the host plant contains corresponding resistance genes. In this study we showed that a type III effector RipTPS from the avirulent R. solanacearum strain GMI1000 (RipTPSG) specifically induced cell death in Nicotiana tabacum, but not in Nicotiana benthamiana, whereas the RipTPS homolog in the virulent strain CQPS-1 (RipTPSC) induced cell death in neither N. tabacum nor N. benthamiana. These results indicated that RipTPSG is recognized in N. tabacum. Expression of RipTPSG induced upregulation of hypersensitive response (HR) -related genes in N. tabacum. The virulence of CQPS-1 was reduced when RipTPSG was genetically introduced into CQPS-1, further confirming that RipTPSG functions as an avirulence determinant. Protein sequence alignment indicated that there are only three amino acid polymorphisms between RipTPSG and RipTPSC. Site-directed mutagenesis analyses confirmed that the three amino acid residues are jointly required for the recognition of RipTPSG in N. tabacum. Expression of either RipTPSG or RipTPSC suppressed flg22-triggered reactive oxygen species (ROS) burst in N. benthamiana, suggesting that RipTPS contributes to pathogen virulence. Mutating the conserved residues in RipTPS’s trehalose-phosphate synthase (TPS) domain did not block its HR induction and defense suppression activity, indicating that the TPS activity is not required for RipTPS’s avirulence and virulence function.
Introduction
The bacterial pathogen Ralstonia solanacearum is a destructive phytopathogen that attacks many plants over a broad geographical range (Genin and Denny, 2012). It is a soil-borne bacterium which can infect more than 200 plant species, including many important crops such as potato, tobacco, tomato, and some ornamental plants (Niu et al., 2022). The extensive genetic diversity of strains causes various bacterial wilt diseases, leading to great economic losses worldwide every year (Qi et al., 2022). In-depth study of the molecular mechanism underlying the interaction between R. solanacearum and plants has important theoretical significance for formulating new disease control strategies.
Plant immunity relies on two levels of pathogen perception that trigger defense mechanisms, pattern-triggered immunity (PTI) and effector-triggered immunity (ETI) (Jones and Dangl, 2006). PTI is the first layer of plant immunity, which involves the recognition of conserved microbial elicitors termed pathogen-associated molecular patterns (PAMPs), such as flagellin, cold shock protein, and elongation factor Tu, by specific plasma membrane receptors (Jones and Dangl, 2006). The receptors then activate signaling cascades in the host cell to restrict pathogen growth (Segonzac et al., 2011). Reactive oxygen species (ROS) burst is a hallmark event of PTI signaling (Segonzac et al., 2011). For example, flg22, a conserved 22-amino-acid peptide from bacterial flagellin, is a PAMP which often triggers ROS burst in host plants (Nakano and Mukaihara, 2019b; Mittler et al., 2022). However, to overcome host PTI for successful infection, adapted pathogens have evolved the ability to inject effectors inside the host cell. In turn, to counter effector-mediated suppression of PTI, plant further evolves a second layer of immunity, ETI, which is based on internal recognition of effector proteins by cytoplasmic receptors. Compared with PTI, ETI initiates stronger and more prolonged defense responses (Jones and Dangl, 2006), which often results in programmed cell death called hypersensitive response (HR) to restrict bacterial multiplication at the infection site (Mukaihara et al., 2016). Although PTI and ETI are initiated by distinct activation mechanisms, they share similar contents of defense responses (Tsuda and Katagiri, 2010). PTI and ETI are mutually linked, and potentiate each other (Ngou et al., 2021; Yuan et al., 2021).
As one of the most destructive plant pathogens, R. solanacearum has gained increasing attention. Genome sequencing of several representative strains of R. solanacearum, such as GMI1000, has broadened our knowledge of the evolution and speciation of this pathogen and led to the increasing identification of molecular determinants involved in pathogenicity and host-range specificity. R. solanacearum employs a type III secretion system (T3SS) which secretes more than 70 type III effectors (T3Es) into plant cells to promote infection (Genin and Denny, 2012). These effectors are powerful weapons for bacterial pathogens, but may also lead to potential recognition of the pathogens by plant immune system (Sang et al., 2020). The T3Es recognized by plant resistance proteins to activate ETI are called avirulence determinants. They restrict pathogen virulence to specific plants and often determine the host-range specificity of a pathogen (Nakano and Mukaihara, 2019b).
GMI1000 is the first genome sequenced R. solanacearum strain and has been used as a model strain for R. solanacearum-related study (Poueymiro et al., 2009). To date, many T3Es of GMI1000 have been studied to analyze their avirulence or virulence functions (Sun et al., 2017; Morel et al., 2018; Sang et al., 2020; Xian et al., 2020; Yu et al., 2020; Cheng et al., 2021; Wang et al., 2021; Niu et al., 2022; Qi et al., 2022), although very few of them have been functionally characterized thoroughly in planta. Several of the reported effectors have been shown to elicit HR and act as avirulence factors in certain plants. For example, PopP1 and PopP2, two members of the YopJ/AvrRxv family, confer avirulence in Petunia as well as Nicotiana gultinosa (Lavie et al., 2002; Poueymiro et al., 2009) and Arabidopsis (Deslandes et al., 2003), respectively. AvrA is an avirulence determinant recognized by both Nicotiana tabacum and Nicotiana benthamiana (Poueymiro et al., 2009). RipAX2 triggers specific resistance and hence is specifically recognized by eggplant AG91-25 (Morel et al., 2018). In addition, it was indicated that RipE1 is an avirulence determinant recognized by N. benthamiana (Sang et al., 2020) and RipAW is recognized by both N. benthamiana and N. tabacum (Niu et al., 2022). Except for GMI1000, Rip36 and RipB from another R. solanacearum strain RS1000 have also been suggested as avirulent factors in S. torvum and N. benthamiana, respectively (Nahar et al., 2014; Nakano and Mukaihara, 2019b). Identification of the above virulent and avirulent factors provide extraordinary insights into the interaction between R. solanacearum and its host/nonhost plants. However, among the extensive repertoire of T3Es, only a small fraction has been studied in depth. Therefore, there is still a long way for us to unravel the story of the non-stop battle between R. solanacearum and plants.
Trehalose-6-Phosphate synthesized by trehalose-phosphate synthase (TPS) is a signaling metabolite and plays important roles in plant growth and flowering regulation (Schluepmann et al., 2012; Wahl et al., 2013). Interestingly, a role for trehalose metabolism is merging in pathogen-plant interaction (Tournu et al., 2013). For example, synthesis of the disaccharide trehalose by Pseudomonas aeruginosa strain PA14 is required for pathogenesis in Arabidopsis (Djonovic et al., 2013). In R. solanacearum species complex, RipTPS is a conserved T3E which has been reported to endow with a TPS enzymatic activity (Poueymiro et al., 2014). In that report, it was demonstrated that RipTPS could specifically elicit a hypersensitive-like response on N. tabacum, suggesting its role as a potential avirulent factor in tobacco. Unexpectedly, the TPS activity was not involved in RipTPS-elicited hypersensitive-like response (Poueymiro et al., 2014). It will be interesting to further characterize the function of RipTPS and the role of its TPS activity.
CQPS-1 is a R. solanacearum strain newly isolated from a highland and its genome has been sequenced (Liu et al., 2017). Most genes coding core T3Es were conserved in CQPS-1 compared with the model strain GMI1000. However, CQPS-1 can infect N. tabacum but GMI1000 cannot. Here, we found that only three amino acid polymorphisms existed between RipTPS in GMI1000 (RipTPSG) and CQPS-1 (RipTPSC) strains. The natural variation between these two RipTPS alleles will undoubtedly facilitate the function characterization of RipTPS. Therefore, using these materials, we provided strong evidence for the confirmation of RipTPSG as an avirulence determinant in N. tabacum, and proved that the three amino acid polymorphisms jointly determine the recognition of RipTPSG in N. tabacum. We also investigated whether RipTPS retained its ability to suppress plant immune responses by analyzing its effect on flg22-triggered ROS burst. Finally, roles of TPS activity in both RipTPSG-elicited HR in nonhost N. tabacum and RipTPSG-inhibited ROS burst induced by flg22 were evaluated through site mutagenesis analyses.
Materials and methods
Plant growth conditions and bacterial strains
Nicotiana benthamiana and Nicotiana tabacum plants were grown at 24°C in a walk-in chamber under long-day conditions (16 h light/8 h dark). Agrobacterium tumefaciens strain GV3101 was used to transiently express effectors in tobacco leaves. Escherichia coli strain DH5α was used for vector construction. They were cultured on Luria-Bertani (LB) agar plates or in LB liquid medium with proper antibiotics at 28°C and 37°C, respectively. Ralstonia solanacearum GMI1000 and CQPS-1 were grown on Bacto-agar and glucose (BG) medium at 28°C.
Sequence analysis
The sequence alignment was performed using SeqHunter software (Ye et al., 2010).
Agrobacterium-mediated transient expression
The agrobacterial cells with corresponding constructs were suspended in an infiltration buffer containing 150 μM acetosyringone, 10 mM MgCl2 and 10 mM MES (pH 5.6), and were incubated at 28°C for 2 hours after being adjusted to an OD600 of 0.5. Then the bacterial suspensions were infiltrated into the fully expanded leaves of 5-week-old tobacco with a needless syringe.
RNA extraction and quantitative PCR
Total RNA was extracted using the RNAsimple Total RNA Extraction Kit (TIANGEN). The RNA sample was then reverse transcribed in a 20-μL volume using the HiScript II Q Select RT SuperMix for qPCR kit (Vazyme). SYBR Green quantitative PCR was performed to determine the relative expression levels of HR-related genes NtHIN1 and NtHsr203J, and NtEF1α was selected as an internal control. Quantification of the relative changes in gene transcript levels was performed using the 2−ΔΔCt method (Livak and Schmittgen, 2001). The primers used for amplification were listed in Table S1.
Measurement of ROS burst
The ROS production level was measured as previously described (Segonzac et al., 2011) with slight modifications. Briefly, leaf disks were taken from 5-week-old N. benthamiana plants, and then floated overnight in 200 μL sterile distilled water. Before measurement, water was replaced with 100 μL reaction solution containing 17 μg/mL luminol, 10 μg/mL horseradish peroxidase and 100 nM flg22. The ROS production level was monitored using a Glomax-96 Microplate Luminometer (Promega).
Generation of transgenic CQPS-1 expressing RipTPSG
R. solanacearum RipTPSG was cloned into pHM1 vector with EcoRI/HindIII. The recombinant plasmid was introduced into CQPS-1 by electroporation.
Site-directed mutagenesis of RipTPSG and generation of RipTPSG knockout mutants
R. solanacearum RipTPSG was cloned into pENTR vector. PCR-based site-directed mutagenesis was performed to mutate the aspartic 23 of RipTPSG into glycine, serine 290 into arginine, alanine 483 into valine, tyrosine 154 into valine, tryptophan 163 into serine, and aspartic 208 into glycine, respectively. The LR reaction was conducted to clone RipTPSGD23G, RipTPSGS290R, RipTPSGA438V, RipTPSGY154V, RipTPSGW163S, and RipTPSGD208G into pGWB505, respectively. All the primers used for this experiment were listed in Table S1.
The upstream and downstream fragments of RipTPSG were separately amplified from GMI1000 genomic DNA and fused using overlap PCR, and the resulting fragment was inserted into the XbaI/BamHI sites of pK18mobsacB (Schafer et al., 1994). Then the recombinant pK18mobsacB vector was transformed into R. solanacearum GMI1000, and the RipTPS deletion mutants were generated by homologous recombination-based procedures. Primers used for generation and identification of RipTPSG knockout mutants were listed in Table S1.
R. solanacearum infection assay
For leaf inoculation, R. solanacearum strains were grown in liquid BG medium at 28°C overnight. Bacterial cells were collected by centrifugation and washed with sterile water and adjusted to a final OD600 of 0.001. N. tabacum leaves were infiltrated with the bacterial solution. The bacterial titers were measured three days after inoculation.
For R. solanacearum soil drenching inoculation, 30-day-old N. tabacum plants were used. R. solanacearum was grown overnight at 28°C in BG liquid medium till OD600 to 2.0, then centrifuged and suspended in distilled water. Plants grown in pots were inoculated via soil drenching with a bacterial suspension (OD600 = 0.1). Scoring of visual disease symptoms on the basis of a scale ranging from ‘0’ (no symptoms) to ‘4’ (complete wilting) was performed as previously described (Vailleau et al., 2007).
Statistical analysis
All experiments were performed with at least three biological replicates, and data were analyzed using SPSS 20.0 software. Student’s t-test was performed along with analysis of variance to compare the differences between treatments.
Results
RipTPSG induces HR on N. tabacum, but RipTPSC cannot
R. solanacearum strain GMI1000 cannot infect N. tabacum, indicating GMI1000 is recognized in N. tabacum. To identify the potential avirulence determinants in GMI1000 that are specifically recognized in N. tabacum, we screened thirteen type III effectors from GMI1000 by observing their ability to induce HR in N. tabacum. This assay led to the identification of an effector RipTPS, which only induced HR in N. tabacum, but not in N. benthamiana (Figure 1A). This result is consistent with a previous report (Poueymiro et al., 2014), indicating that RipTPS in GMI1000 (RipTPSG) may be recognized in N. tabacum.
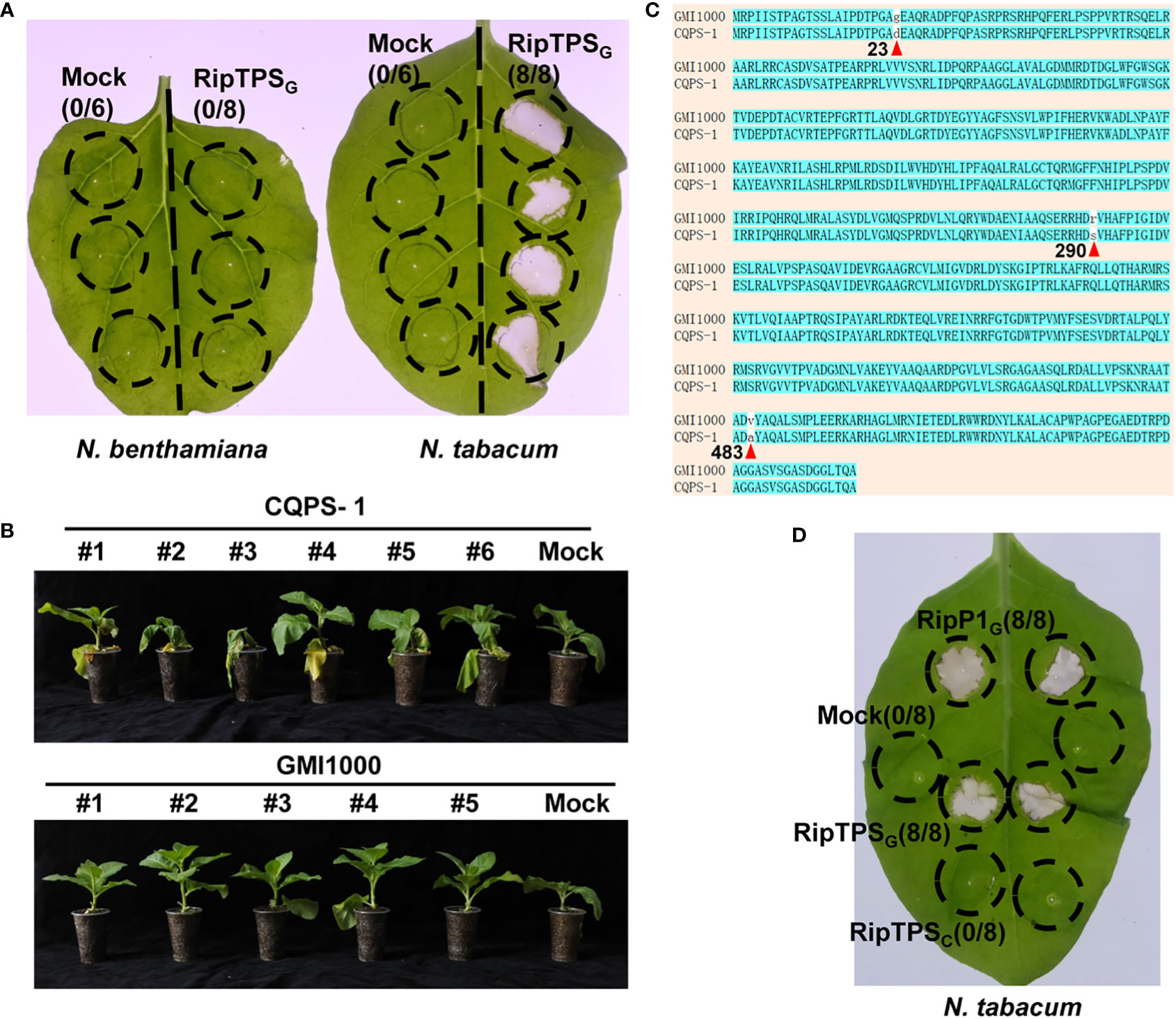
Figure 1 RipTPSG (RipTPS from GMI1000), but not RipTPSC (RipTPS from CQPS-1), elicits hypersensitive response (HR)-like phenotype in Nicotiana tabacum. (A) RipTPSG elicits an HR-like phenotype in N. tabacum, but not in Nicotiana benthamiana. (B) Phenotypes of N. tabacum infected with R. solanacearum strain GMI1000 or CQPS-1 11 days after root inoculation. (C) Pairwise sequence alignment of RipTPS proteins derived from avirulent GMI1000 and virulent CQPS-1. Positions of the three amino acid polymorphisms are indicated by red triangles and the corresponding numbers below the amino acids. (D) RipTPSG elicits an HR-like phenotype in N. tabacum, but RipTPSC cannot. For A and D, leaves of N. benthamiana or N. tabacum were infiltrated with Agrobacterium tumefaciens GV3101 carrying LTI6b (Mock, a negative control), RipP1G (RipP1 from GMI1000, a positive control), RipTPSG or RipTPSC. Photographs were taken 24 hours after infiltration. Circles indicate the infiltrated area on the leaf panels. The fraction in brackets represents the number of HR over the total number of the infiltrated leaves.
CQPS-1 is another R. solanacearum strain whose type III secretion system cluster is conserved compared with GMI1000 (Liu et al., 2017). In contrast to GMI1000, CQPS-1 can infect N. tabacum (Liu et al., 2017, Figure 1B). Here, pairwise sequence alignment of RipTPS derived from avirulent GMI1000 and virulent CQPS-1 strains showed that there are only three amino acid polymorphisms between them (Figure 1C). Interestingly, the RipTPS from CQPS-1 (RipTPSC) cannot induce HR in N. tabacum (Figure 1D), further suggesting that RipTPSG is recognized in N. tabacum.
To determine whether RipTPSG triggers ETI-like response, expression of two HR-related genes NtHIN1 and NtHsr203J was measured. The result showed that RipTPSG dramatically enhanced relative expression of NtHIN1 and NtHsr203J in N. tabacum compared with LTI6b control which is a membrane-localized protein unrelated to plant immunity, whereas their induction was significantly compromised in RipTPSC (Figure 2). Together, these results indicate that RipTPSG is an avirulence protein recognized in N. tabacum.
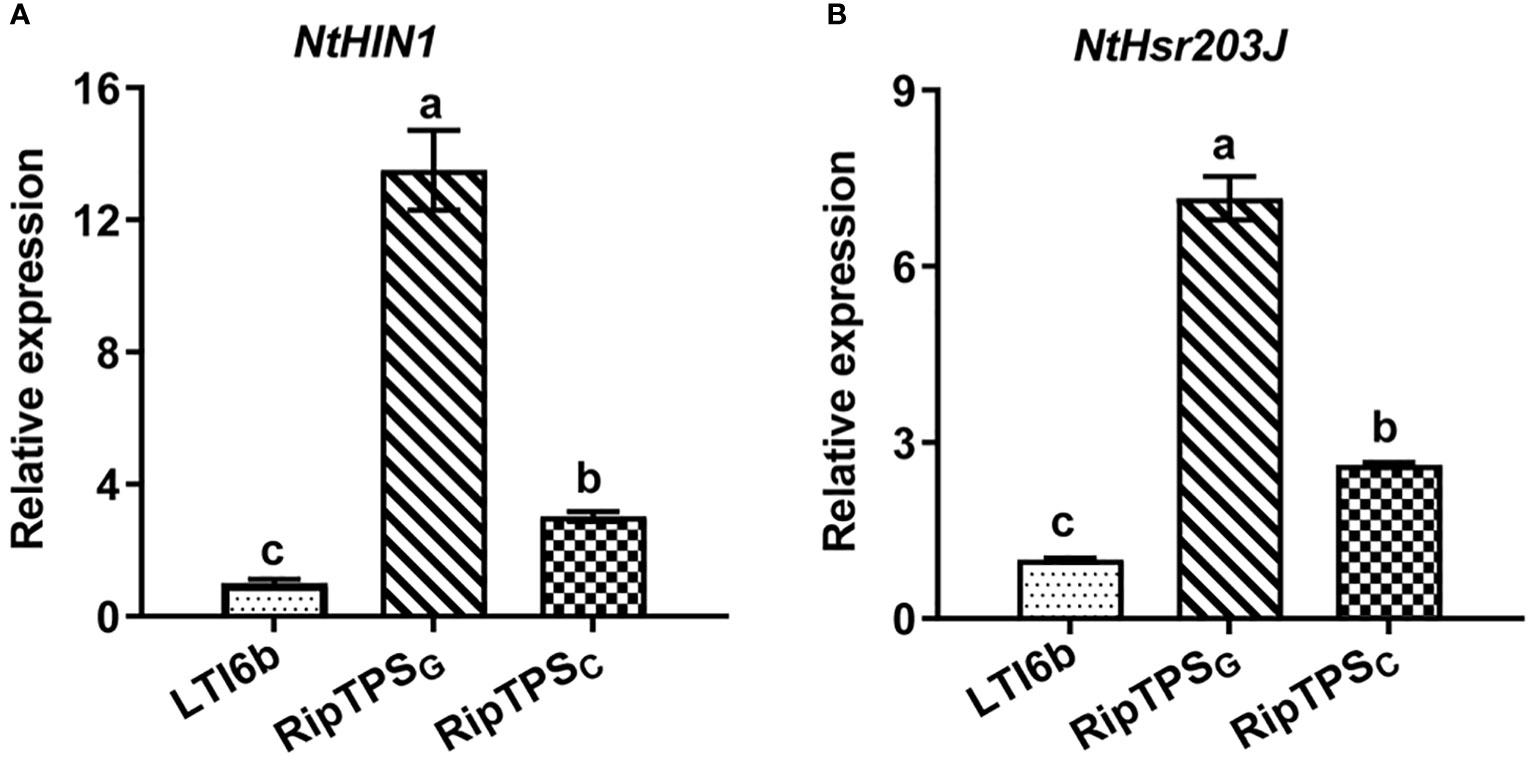
Figure 2 RipTPSG upregulates expression of HR-related genes in N. tabacum. Leaves of N. tabacum were infiltrated with A tumefaciens GV3101 carrying LTI6b (Control), RipTPSG or RipTPSC. The expression of NtHIN1 (A) and NtHsr203J (B) was quantified by qRT-PCR at 19 hpi. Data are means ± standard errors (SE, n = 9). The different letters indicate significant differences at p ≤ 0.01.
Expression of RipTPSG in CQPS-1 reduces its virulence on N. tabacum
To genetically investigate the function of RipTPSG as an avirulence determinant, we expressed RipTPSG in CQPS-1 and investigated whether it can affect virulence of CQPS-1 on N. tabacum. Results showed that, compared to the empty vector control, expressing RipTPSG significantly decreased CQPS-1’s population in the inoculated N. tabacum leaves (Figure 3A). In addition, expressing RipTPSG in CQPS-1 largely reduced the disease index (Figure 3B) and consequently enhanced the survival percent (Figure 3C) of N. tabacum. These results indicate that expression of RipTPSG significantly reduced virulence of CQPS-1 on N. tabacum, confirming RipTPSG as an avirulence determinant in N. tabacum.
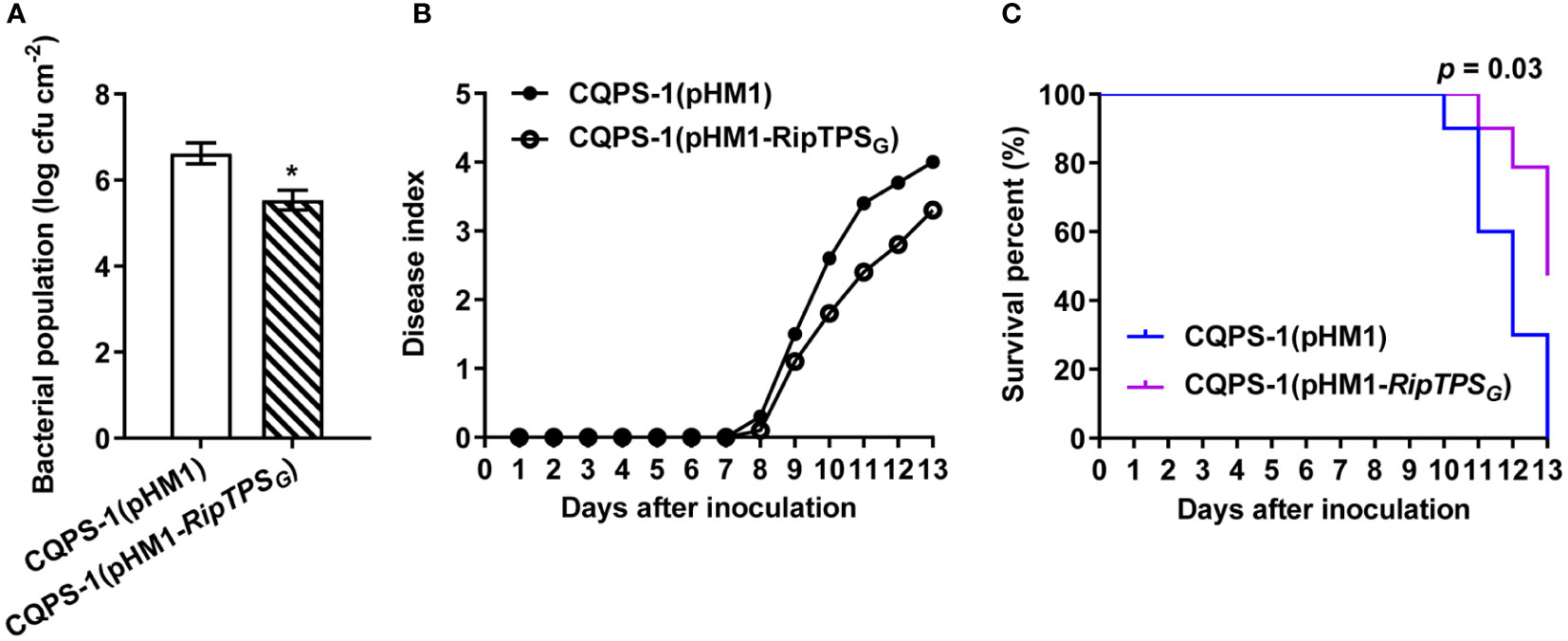
Figure 3 Expression of RipTPSG in CQPS-1 reduces pathogen virulence in N. tabacum. (A) Titers of R. solanacearum strain CQPS-1. CQPS-1 cells expressing the empty vector (pHM1) and RipTPSG (pHM1-RipTPSG) were separately inoculated on 30-day-old N. tabacum leaves and bacterial population was quantified 2 days after inoculation. * indicates significant difference at p ≤ 0.05. (B) Disease index of 30-day-old N. tabacum after soil drenching inoculation of CQPS-1(pHM1) or CQPS-1(pHM1-RipTPSG). Fifteen plants were observed per genotype per biological replicate. (C) Survival analysis of the data in (B). Statistical analysis was performed using a Log-rank (Mantel-Cox) test (n = 45), and the corresponding p value is shown in the graph.
The three amino acid residues jointly determine the recognition of RipTPSG in N. tabacum
Since RipTPSG and RipTPSC differs in only three amino acid residues, it’s reasonable to speculate that these three residues are important for the recognition of RipTPSG in N. tabacum. The three residues are D23, S290 and A483 in RipTPSG. The corresponding three residues in RipTPSC are G23, R290 and V483, respectively (Figure 1C). To evaluate the involvement of the three polymorphic amino acid sites in RipTPSG avirulence activity, we generated a series of RipTPSG mutants, including all three single-residue mutants and all three double-residue mutants. In these mutants, we mutated the residues in RipTPSG to the corresponding ones in RipTPSC. RipTPSC can be considered as the three-residue mutant of RipTPSG. After transiently expressing these mutated genes, we found that all the single-residue and double-residue mutants still elicited strong HR (Figure 4). Only when all three residues were mutated (RipTPSC), RipTPSG-elicited HR was abolished. These results indicate that the three amino acid polymorphisms jointly determine the recognition of RipTPSG in N. tabacum.
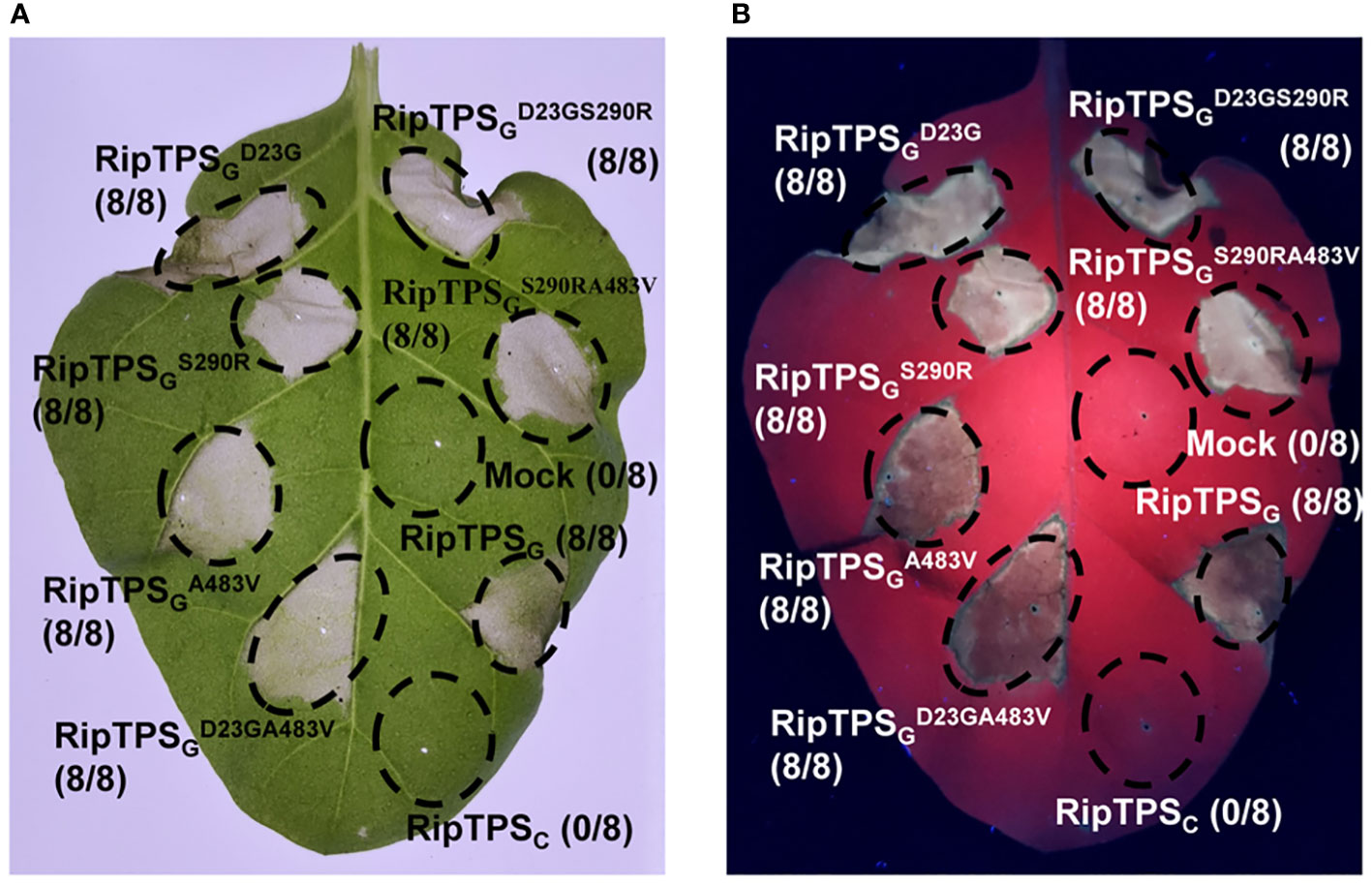
Figure 4 Three amino acid residues are jointly required for RipTPSG-induced HR in N. tabacum. Leaves of N. tabacum were infiltrated with GV3101 carrying LTI6b (Mock, Control), RipTPSG, RipTPSC, or RipTPSG mutants. Photographs were taken 36 hours after infiltration under white light (A) or ultraviolet light (B). Circles indicate the infiltrated area on the leaf panels. The fraction in brackets represents the number of HR over the total number of infiltrated leaves.
Both RipTPSG and RipTPSC suppress flg22-induced ROS burst in N. benthamiana
R. solanacearum usually secretes effectors to interfere with host immunity and promote its infection. To investigate whether RipTPSG affects plant basal defense, we expressed RipTPSG in N. benthamiana and measured ROS production induced by the bacterial PAMP flg22, which is a major epitope peptide of bacterial flagellin. We found that a peak value greater than 3.3×104 relative luminescence units (RLU) was reached in control plants (Mock, Figure 5A). However, in RipTPSG-expressing plants, the peak value was less than 2.0×104 RLU. Compared with the LTI6b control, RipTPSG expression significantly inhibited the total ROS accumulation during a 30 min period (Figure 5B). These results suggest that RipTPSG interferes with PAMP-triggered ROS accumulation. Similar effect was also observed for RipTPSC (Figures 5C, D), indicating that the three amino acid polymorphisms do not affect its function in suppression of basal defense response.
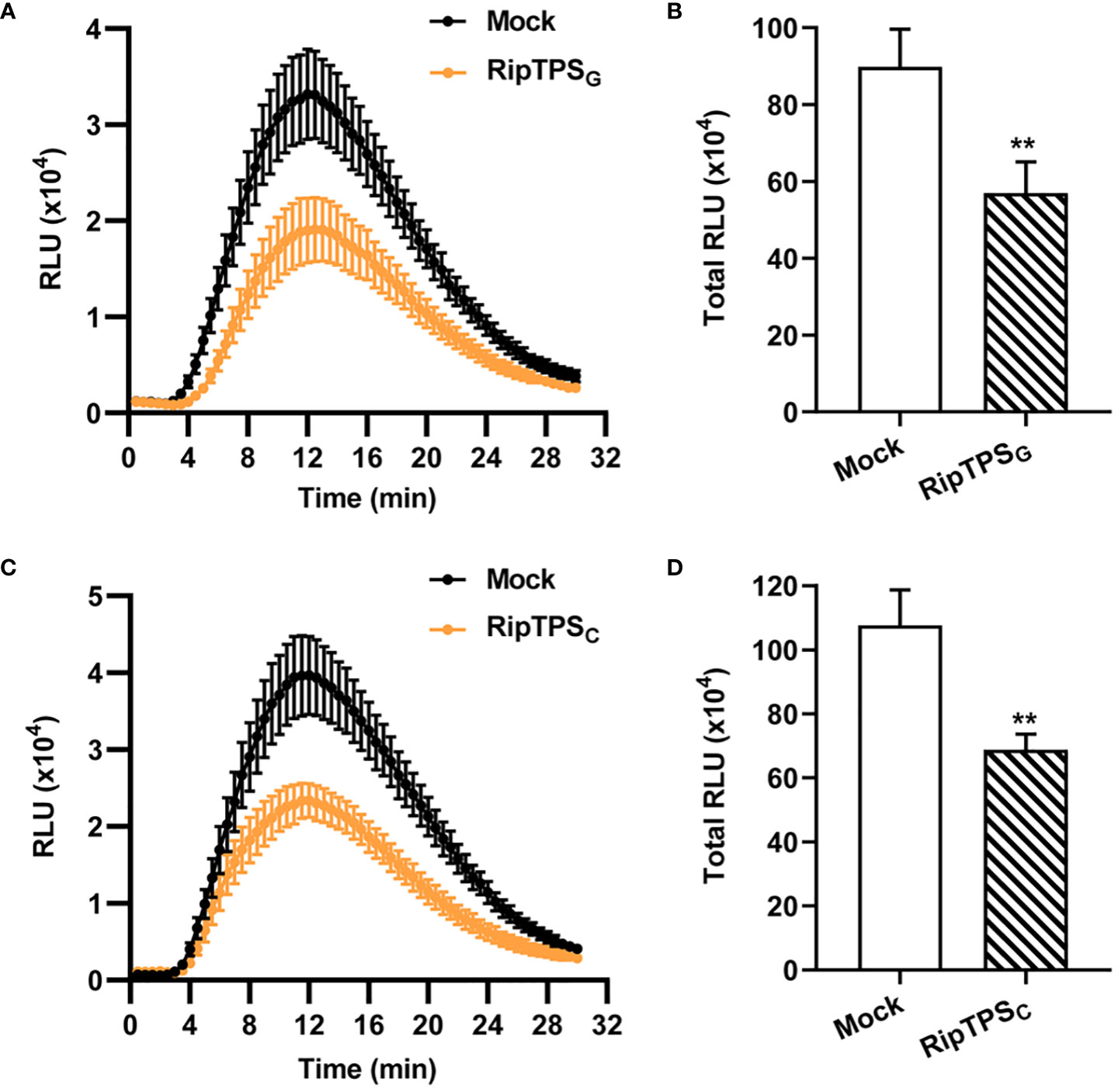
Figure 5 Expression of RipTPSG or RipTPSC suppresses flg22-induced reactive oxygen species (ROS) burst in N. benthamiana. (A, C) Time course curve of ROS production in leaves of N. benthamiana transiently expressing LTI6b (Mock, Control), RipTPSG (A) or RipTPSC (C). Two days after infiltration, leaf discs were treated with 100 nM flg22 elicitor, and ROS production was measured as photon counts for 30 min. (B, D) Total ROS accumulation during 30 min in N. benthamiana leaves of (A, C), respectively. RLU: relative luminescence units. Data are means ± SE from 14 independent leaf discs. ** indicates significant difference at p ≤ 0.01.
RipTPS’s role of suppressing flg22-induced ROS burst in N. benthamiana is independent of its TPS enzymatic activity
Poueymiro et al. (2014) revealed that RipTPSG directs the production of plant signal metabolite trehalose-6-phosphate. They identified three residues essential for its trehalose-6-synthase enzymatic activity and showed that enzymatic activity is not required for RipTPS-elicited HR in N. tabacum. Here, we also showed that the three catalytic mutants of RipTPSG (RipTPSGY154V, RipTPSGW163S and RipTPSGD208G) elicited HR in N. tabacum as well as RipTPSG (Figure 6A), confirming the finding of Poueymiro et al. (2014). Since trehalose-6-phosphate is an essential signal molecule in plants, we wonder whether RipTPS’s TPS activity plays a role in its suppression of plant basal defense. We compared the effect of RipTPSG and its three catalytic mutants on flg22-induced ROS burst in N. benthamiana and found that all three mutants showed the similar activity to RipTPSG (Figures 6B–E). This result indicates that the trehalose-6-synthase enzymatic activity is not required for RipTPS’s function in suppression of PAMP-triggered ROS production.
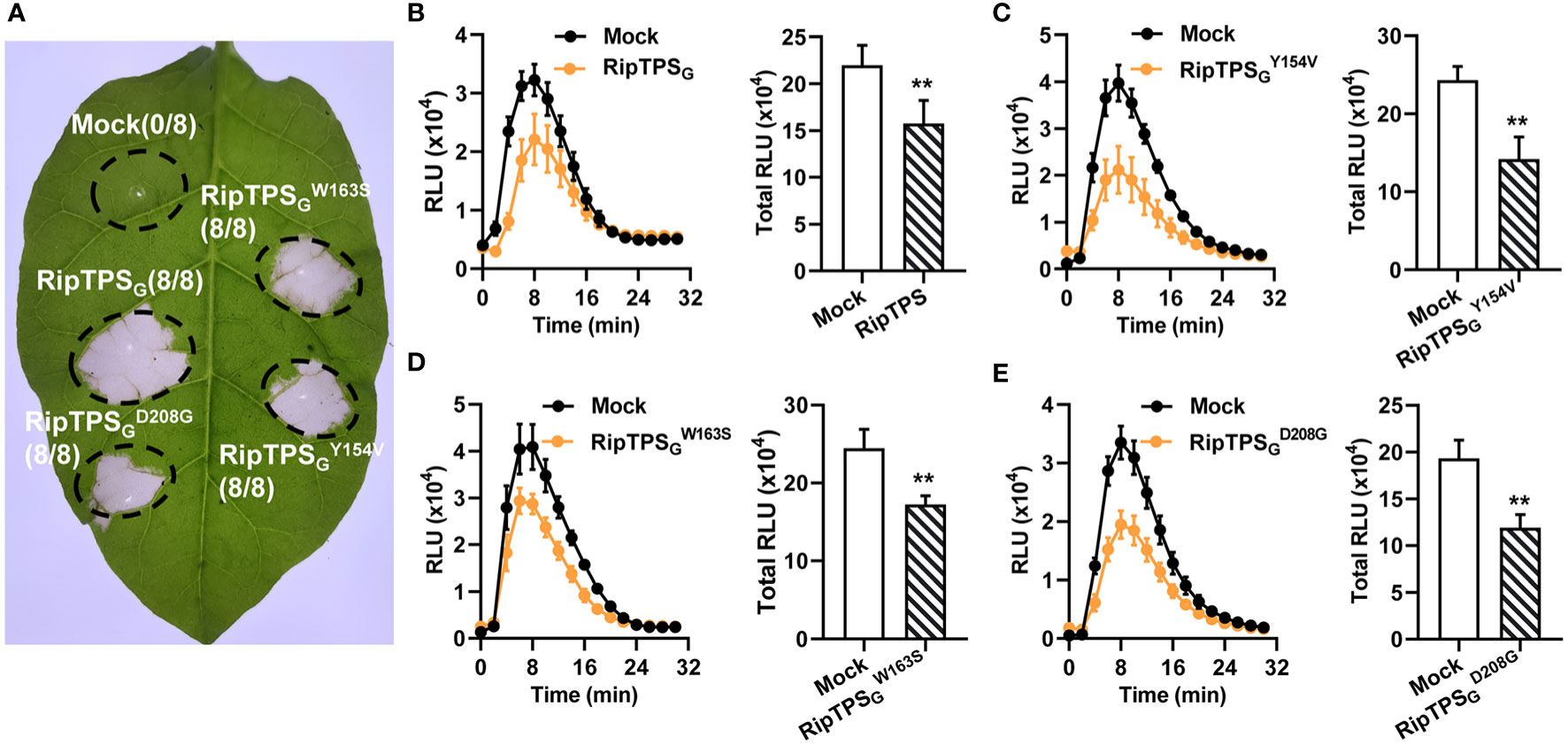
Figure 6 TPS activity is required for neither RipTPSG-elicited HR in N. tabacum nor suppression of flg22-induced ROS burst in N. benthamiana. (A) RipTPSG-elicited HR in N. tabacum is independent of its TPS activity. Leaves of N. tabacum were infiltrated with GV3101 carrying LTI6b (Mock), RipTPSG, or RipTPSG mutants compromised in its enzymatic activity (RipTPSGY154V, RipTPSGW163s and RipTPSGD208G). Photographs were taken 24 hours after infiltration. Circles indicate the infiltrated area on the leaf panels. The fraction in brackets represents the number of HR over the total number of infiltrated leaves. (B–E) Suppression of flg22-induced ROS burst by RipTPSG in N. benthamiana is independent of TPS activity. Both of time course curve of ROS production and total ROS accumulation in leaves of N. benthamiana transiently expressing LTI6b (Mock, Control), RipTPSG, or RipTPSG mutants were showed. Two days after infiltration, leaf discs were treated with 100 nM flg22, and ROS production was measured as photon counts for 30 min. RLU: relative luminescence units. Data are means ± SE from 14 independent leaf discs. ** indicates significant difference at p ≤ 0.01.
Deletion of RipTPSG does not affect GMI1000-induced HR in N. tabacum
To further reveal the role of RipTPS in GMI1000-induced HR in N. tabacum, we deleted RipTPS in GMI1000 (Figure 7A). The RipTPS deletion mutants were infiltrated into N. tabacum leaves, and the HR induction was observed. The result demonstrated that two independent RipTPS deletion mutants still induced strong HR, which is similar to the wild-type GMI1000 (Figure 7B), indicating deletion of RipTPS alone is not sufficient to abolish GMI1000-induced HR in N. tabacum.
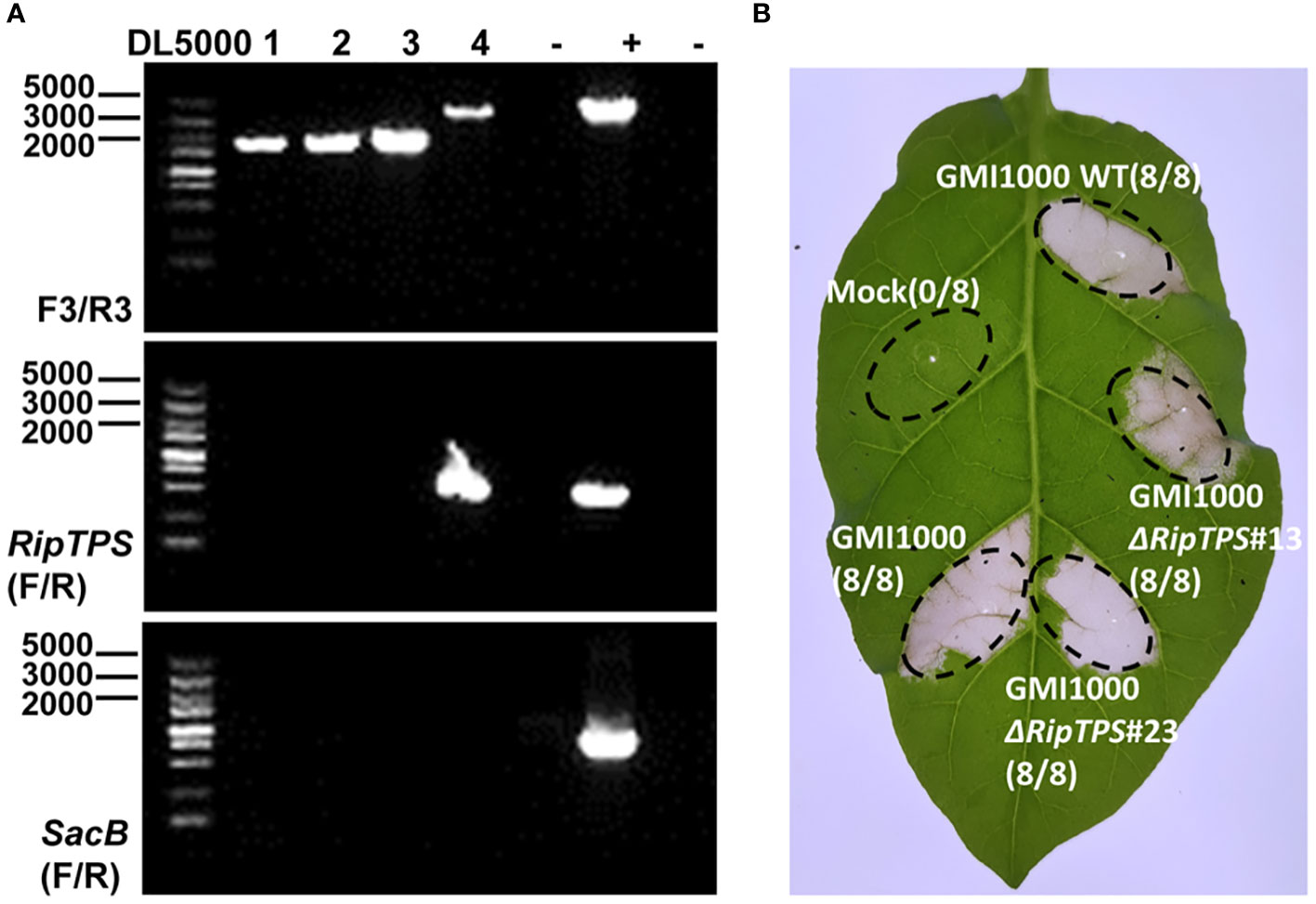
Figure 7 Deletion of RipTPS in GMI1000 does not affect its HR induction phenotype in N. tabacum. (A) Identification of RipTPS deletion mutants. The primer pairs F3/R3, RipTPS(F/R) and SacB(F/R) were used for confirmation of double exchange. After double exchange, the RipTPS gene was deleted. DL5000: DNA ladder. (B) HR induced by wild-type GMI1000 and RipTPS deletion mutants. Wild-type GMI1000 or RipTPS deletion mutant cells with an OD600 of 0.1 were infiltrated into 40-day-old N. tabacum leaves, respectively. Photographs were taken 48 hours after inoculation. Water was used as mock. Circles indicate the infiltrated area on the leaf panels. The fraction in brackets represents the number of HR over the total number of infiltrated leaves. GMI1000ΔRipTPS#13 and GMI1000ΔRipTPS#23 are two independent RipTPS deletion mutants.
Discussion
RipTPSG acts as a host-specificity avirulence factor
R. solanacearum is an aggressive pathogen with a large repertoire of T3Es (Genin and Denny, 2012). Functional characterization of these effectors is critical for understanding the mechanisms of host specificity and pathogenicity in these economically important pathogens (Kim et al., 2021; Yang et al., 2022). However, to date, only few R. solanacearum T3Es were characterized for their roles in interaction between R. solanacearum and its host/nonhost plants. The model R. solanacearum strain GMI1000 cannot infect either N. benthamiana or N. tabacum, but its close relative CQPS-1 infects both N. benthamiana and N. tabacum. This feature makes these two R. solanacearun strains good models to study host-R. solanacearum recognition. We analyzed HR induced by R. solanacearum T3Es in N. tabacum and N. benthamiana, and identified RipTPSG which induced HR in N. tabacum but not in N. benthamiana, indicating that RipTPSG is recognized in N. tabacum. This result was supported by a previous report (Poueymiro et al., 2014).
To further demonstrate whether RipTPSG is an avirulence determinant, we took advantage of the R. solanacearum CQPS-1 whose genome has also been sequenced. In contrast to GMI1000, CQPS-1 contains conserved T3SS gene clusters, but is a virulent R. solanacearum strain on tobacco (Liu et al., 2017). In the present study, we found that there are only three amino acid polymorphisms between RipTPS proteins of the two strains (Figure 1). We then took advantage of this natural variation and obtained the following evidence: (1) RipTPSG elicited HR on N. tabacum, but RipTPSC cannot (Figure 1); (2) RipTPSG dramatically enhanced expression of HR-related genes NtHIN1 and NtHsr203J in N. tabacum (Takahashi et al., 2004), which was significantly compromised when RipTPSG was replaced by RipTPSC (Figure 2); (3) The RipTPSG expression reduced virulence of CQPS-1 on N. tabacum (Figure 3). These results demonstrate that RipTPSG is an avirulence determinant that triggers defense in N. tabacum, while RipTPSC acts as a virulence T3E. However, expression of RipTPSC still slightly enhanced expression of the HR marker genes (Figure 2). Similar weak responses of N. benthamiana to the virulent alleles has also been reported previously, especially in the overexpression assays (Westerink et al., 2004; Bos et al., 2006).
Compared with other bacterial plant pathogens, R. solanacearum has a large repertoire of secreted effectors (Yu et al., 2020), and some of these effectors are functionally redundant (Cunnac et al., 2004; Macho et al., 2010) and balanced for a successful infection (Sang et al., 2020). Deleting RipTPS alone in GMI1000 did not significantly affect R. solanacearum-induced HR in N. tatacum (Figure 7), indicating other avirulence genes exist in GMI1000. Indeed, AvrA (Poueymiro et al., 2009) and RipAW (Niu et al., 2022) from GMI1000 have been reported to act as avirulence factors in N. tabacum. Differently, AvrA and RipAW are recognized by both N. benthamiana and N. tabacum (Poueymiro et al., 2009; Niu et al., 2022). Therefore, RipTPS acts as an avirulence determinant specifically recognized in N. tabacum.
The three amino acid polymorphisms determine the recognition ofRipTPSG in N. tabacum
During the coevolution of phytopathogens and plants, pathogens must overcome ETI for further infection through evolution of pathogen effectors that escape or suppress ETI (Jones and Dangl, 2006). Sang et al. (2020) showed that R. solanacearum effector RipAY directly inhibited another effector RipE1-triggered ETI in N. benthamiana to counteract its perception by plant immune system. Many plant pathogens have also developed strategies to escape host recognition by point mutations, gene deletions, transposon insertions or deliberate mistranslation (Westerink et al., 2004; Vargas-Rodriguez et al., 2021). Escaping recognition by the strategy of point mutations of avirulence factor has been reported in several phytopathogens such as Cladosporium fulvum (Westerink et al., 2004) and Phytophthora infestans (Bos et al., 2006). Here, only three polymorphic amino acid residues are present in RipTPS between avirulent strain GMI1000 and virulent strain CQPS-1. Site-directed mutagenesis analyses showed that neither the single nor the double amino acid substitution abolished RipTPSG-elicted HR. RipTPSG-triggered HR was abolished only when all its three polymorphic residues were substituted into the corresponding residues in RipTPSC (Figure 4). This finding suggests that all three amino acid residues are required for the recognition of RipTPSG in N. tabacum, and RipTPSC evades the recognition through simultaneously mutating these three residues. Poueymiro et al. (2014) pointed out that the C-terminal half (amino acid 336-557) of RipTPSG alone could trigger the HR-like response. Our result indicated that the N-terminal region of RipTPS also contributes to its recognition in N. tabacum, since both D23 and S290 residues are required for RipTPSG-induced HR in our result (Figure 4). These three residues may affect three-dimensional structure of RipTPS protein or interactions between RipTPS and other plant proteins.
RipTPS exhibited defense suppression activity in N. benthamiana
ROS play a crucial role in biotic stress sensing and activation of stress-response networks (Mittler et al., 2022). ROS burst is a hallmark event for plant basal defense (Segonzac et al., 2011; Nakano and Mukaihara, 2019a). Here, we showed that expression of RipTPSG suppressed flg22-induced ROS burst in N. benthamiana (Figure 5), indicating that RipTPSG interferes with plant immune response in N. benthamaina. Similar phenomenon was also observed in other T3Es, such as PopP2 and AvrA which are known as avirulence determinants but contribute to pathogen virulence in susceptible host (Macho et al., 2010). Interestingly, expression of RipTPSC also suppressed flg22-induced ROS burst in N. benthamiana (Figure 5), indicating that RipTPSC retains its ability to suppress plant defense response and the three amino acid polymorphisms do not affect RipTPS’s virulence activity.
TPS activity is involved in neither RipTPSG’s avirulence nor virulence functions
The most striking feature of RipTPS is its inherently trehalose-6-synthase enzymatic activity as its name suggests (Poueymiro et al., 2014). Trehalose, a non-reducing sugar, has been found in multiple microbes ranging from bacteria to yeast and in plants. In plants, trehalose not only serves as a reserve carbohydrate and structural components of cells but also acts as a signaling molecule, and it plays important physiological roles in plant growth and stress resistance (Wahl et al., 2013; Sarkar and Sadhukhan, 2022; Shao et al., 2022). Interestingly, in some phytopathogens, trehalose metabolism has emerged as an essential player in virulence-associated phenotypes and proficient initial plant infection (Tournu et al., 2013). For instance, trehalose biosynthesis promotes Pseudomonas aeruginosa virulence in plants by promoting the acquisition of nitrogen-containing nutrients (Djonovic et al., 2013). However, we demonstrated that the TPS enzymatic activity was not required for its avirulence function, which is consistent with the result of Poueymiro et al. (2014). We also showed that the TPS enzymatic activity was not required for its function in suppression of PAMP-induced ROS production (Figure 6). Further studies are needed to elucidate the role of the TPS activity of RipTPS in R. solanacearum-plant interactions.
In summary, in this study we demonstrated that RipTPS from R. solanacearum strain GMI1000 is an avirulence determinant recognized in N. tabacum, whereas the RipTPS homolog from another strain CQPS-1 can escape this recognition. The three amino acid residues of RipTPS are jointly required for the recognition of RipTPSG in N. tabacum. Both RipTPSG and RipTPSC retain their ability to suppress plant defense responses. The TPS activity of RipTPS is not required for its avirulence function and also virulence activity of suppressing PAMP-induced ROS burst. It will be a promising direction to identify the corresponding resistance gene recognizing RipTPSG in N. tabacum and also to elucidate the role of TPS activity of RipTPS in R. solanacearum-plant interactions in the future.
Data availability statement
The original contributions presented in the study are included in the article/Supplementary Material. Further inquiries can be directed to the corresponding author.
Author contributions
YA and MZ conceived and designed the experiments. JC, ZX, XO, and PC performed the experiments. YA, RW, PL and MZ analyzed the data. YA, JC and MZ drafted and modified the manuscript. All authors contributed to the article and approved the submitted version.
Funding
This research was supported by the National Natural Science Foundation of China (32072399, 32272641), the Fundamental Research Funds for the Central Universities (GK202201017), the Program of Fujian Key Laboratory for Monitoring and Integrated Management of Crop Pests (MIMCP-202203), and the Joint Research Project of FAAS (DWHZ2021-13).
Conflict of interest
The authors declare that the research was conducted in the absence of any commercial or financial relationships that could be construed as a potential conflict of interest.
Publisher’s note
All claims expressed in this article are solely those of the authors and do not necessarily represent those of their affiliated organizations, or those of the publisher, the editors and the reviewers. Any product that may be evaluated in this article, or claim that may be made by its manufacturer, is not guaranteed or endorsed by the publisher.
Supplementary material
The Supplementary Material for this article can be found online at: https://www.frontiersin.org/articles/10.3389/fpls.2022.1040826/full#supplementary-material
References
Bos, J. I. B., Kanneganti, T. D., Young, C., Cakir, C., Huitema, E., Win, J., et al. (2006). The c-terminal half of Phytophthora infestans RXLR effector AVR3a is sufficient to trigger R3a-mediated hypersensitivity and suppress INF1-induced cell death in Nicotiana benthamiana. Plant J. 48, 165–176. doi: 10.1111/j.1365-313X.2006.02866.x
Cheng, D., Zhou, D., Wang, Y. D., Wang, B. S., He, Q., Song, B. T., et al. (2021). Ralstonia solanacearum type III effector RipV2 encoding a novel E3 ubiquitin ligase (NEL) is required for full virulence by suppressing plant PAMP-triggered immunity. Biochem. Bioph. Res. Co. 550, 120–126. doi: 10.1016/j.bbrc.2021.02.082
Cunnac, S., Occhialini, A., Barberis, P., Boucher, C., Genin, S. (2004). Inventory and functional analysis of the large hrp regulon in Ralstonia solanacearum: identification of novel effector proteins translocated to plant host cells through the type III secretion system. Mol. Microbiol. 53, 115–128. doi: 10.1111/j.1365-2958.2004.04118.x
Deslandes, L., Olivier, J., Peeters, N., Feng, D. X., Khounlotham, M., Boucher, C., et al. (2003). Physical interaction between RRS1-r, a protein conferring resistance to bacterial wilt, and PopP2, a type III effector targeted to the plant nucleus. Proc. Natl. Acad. Sci. U.S.A. 100, 8024–8029. doi: 10.1073/pnas.1230660100
Djonovic, S., Urbach, J. M., Drenkard, E., Bush, J., Feinbaum, R., Ausubel, J. L., et al. (2013). Trehalose biosynthesis promotes Pseudomonas aeruginosa pathogenicity in plants. PloS Pathog. 9, e1003217. doi: 10.1371/journal.ppat.1003217
Genin, S., Denny, T. P. (2012). Pathogenomics of the Ralstonia solanacearum species complex. Annu. Rev. Phytopathol. 50, 67–89. doi: 10.1146/annurev-phyto-081211-173000
Jones, J. D. G., Dangl, J. L. (2006). The plant immune system. Nature 444, 323–329. doi: 10.1146/annurev-phyto-081211-173000
Kim, B., You, H., Cecile, S. (2021). The c-terminal domain of the Ralstonia solanacearum type III effector RipY is required for recognition in Nicotiana benthamiana. Mol. Plant Microbe Interact. 34, S.
Lavie, M., Shillington, E., Eguiluz, C., Grimsley, N., Boucher, C. (2002). PopP1, a new member of the YopJ/AvrRxv family of type III effector proteins, acts as a host-specificity factor and modulates aggressiveness of Ralstonia solanacearum. Mol. Plant Microbe Interact. 15, 1058–1068. doi: 10.1094/Mpmi.2002.15.10.1058
Liu, Y., Tang, Y. M., Qin, X. Y., Yang, L., Jiang, G. F., Li, S. L., et al. (2017). Genome sequencing of Ralstonia solanacearum CQPS-1, a phylotype I strain collected from a highland area with continuous cropping of tobacco. Front. Microbiol. 8. doi: 10.3389/Fmicb.2017.00974
Livak, K. J., Schmittgen, T. D. (2001). Analysis of relative gene expression data using real-time quantitative PCR and the 2–ΔΔCt method. Methods 25, 402–408. doi: 10.1006/meth.2001.1262
Macho, A. P., Guidot, A., Barberis, P., Beuzon, C. R., Genin, S. (2010). A competitive index assay identifies several Ralstonia solanacearum type III effector mutant strains with reduced fitness in host plants. Mol. Plant Microbe Interact. 23, 1197–1205. doi: 10.1094/Mpmi-23-9-1197
Mittler, R., Zandalinas, S. I., Fichman, Y., Van Breusegem, F. (2022). Reactive oxygen species signalling in plant stress responses. Nat. Rev. Mol. Cell Biol 23, 663–669. doi: 10.1038/s41580-022-00499-2
Morel, A., Guinard, J., Lonjon, F., Sujeeun, L., Barberis, P., Genin, S., et al. (2018). The eggplant AG91-25 recognizes the type III-secreted effector RipAX2 to trigger resistance to bacterial wilt (Ralstonia solanacearum species complex). Mol. Plant Pathol. 19, 2459–2472. doi: 10.1111/mpp.12724
Mukaihara, T., Hatanaka, T., Nakano, M., Oda, K. (2016). Ralstonia solanacearum type III effector RipAY is a glutathione-degrading enzyme that is activated by plant cytosolic thioredoxins and suppresses plant immunity. mBio 7, e00359-16. doi: 10.1128/mBio.00359-16
Nahar, K., Matsumoto, I., Taguchi, F., Inagaki, Y., Yamamoto, M., Toyoda, K., et al. (2014). Ralstonia solanacearum type III secretion system effector Rip36 induces a hypersensitive response in the nonhost wild eggplant Solanum torvum. Mol. Plant Pathol. 15, 297–303. doi: 10.1111/mpp.12079
Nakano, M., Mukaihara, T. (2019a). Comprehensive identification of PTI suppressors in type III effector repertoire reveals that Ralstonia solanacearum activates jasmonate signaling at two different steps. Int. J. Mol. Sci. 20, 5992. doi: 10.3390/Ijms20235992
Nakano, M., Mukaihara, T. (2019b). The type III effector RipB from Ralstonia solanacearum RS1000 acts as a major avirulence factor in Nicotiana benthamiana and other Nicotiana species. Mol. Plant Pathol. 20, 1237–1251. doi: 10.1111/mpp.12824
Ngou, B. P. M., Ahn, H., Ding, P. T., Jones, J. D. G. (2021). Mutual potentiation of plant immunity by cell-surface and intracellular receptors. Nature 592, 110–115. doi: 10.1038/s41586-021-03315-7
Niu, Y., Fu, S. Y., Chen, G., Wang, H. J., Wang, Y. S., Hu, J. X., et al. (2022). Different epitopes of Ralstonia solanacearum effector RipAW are recognized by two Nicotiana species and trigger immune responses. Mol. Plant Pathol. 23, 188–203. doi: 10.1111/mpp.13153
Poueymiro, M., Cazale, A. C., Francois, J. M., Parrou, J. L., Peeters, N., Genin, S. (2014). A Ralstonia solanacearum type III effector directs the production of the plant signal metabolite trehalose-6-phosphate. mBio 5, e02065-14. doi: 10.1128/mBio.02065-14
Poueymiro, M., Cunnac, S., Barberis, P., Deslandes, L., Peeters, N., Cazale-Noel, A. C., et al. (2009). Two type III secretion system effectors from Ralstonia solanacearum GMI1000 determine host-range specificity on tobacco. Mol. Plant Microbe Interact. 22, 538–550. doi: 10.1094/Mpmi-22-5-0538
Qi, P. P., Huang, M. L., Hu, X. H., Zhang, Y., Wang, Y., Li, P. Y., et al. (2022). A Ralstonia solanacearum effector targets TGA transcription factors to subvert salicylic acid signaling. Plant Cell 34, 1666–1683. doi: 10.1093/plcell/koac015
Sang, Y. Y., Yu, W. J., Zhuang, H. Y., Wei, Y. L., Derevnina, L., Yu, G., et al. (2020). Intra-strain elicitation and suppression of plant immunity by Ralstonia solanacearum type-III effectors in Nicotiana benthamiana. Plant Commun. 1, 100025. doi: 10.1016/J.Xplc.2020.100025
Sarkar, A. K., Sadhukhan, S. (2022). Imperative role of trehalose metabolism and trehalose-6-phosphate signaling on salt stress responses in plants. Physiol. Plant 174, e13647. doi: 10.1111/ppl.13647
Schafer, A., Tauch, A., Jager, W., Kalinowski, J., Thierbach, G., Puhler, A. (1994). Small mobilizable multi-purpose cloning vectors derived from the Escherichia coli plasmids pK18 and pK19: selection of defined deletions in the chromosome of Corynebacterium glutamicum. Gene 145, 69–73. doi: 10.1016/0378-1119(94)90324-7
Schluepmann, H., Berke, L., Sanchez-Perez, G. F. (2012). Metabolism control over growth: a case for trehalose-6-phosphate in plants. J. Exp. Bot. 63, 3379–3390. doi: 10.1093/jxb/err311
Segonzac, C., Feike, D., Gimenez-Ibanez, S., Hann, D. R., Zipfel, C., Rathjen, J. P. (2011). Hierarchy and roles of pathogen-associated molecular pattern-induced responses in Nicotiana benthamiana. Plant Physiol. 156, 687–699. doi: 10.1104/pp.110.171249
Shao, J. H., Wu, W. X., Rasul, F., Munir, H., Huang, K., Awan, M. I., et al. (2022). Trehalose induced drought tolerance in plants: physiological and molecular responses. Not. Bot. Horti Agrobo. 50, 12584. doi: 10.15835/Nbha50112584
Sun, Y. H., Li, P., Deng, M. Y., Shen, D., Dai, G. Y., Yao, N., et al. (2017). The Ralstonia solanacearum effector RipAK suppresses plant hypersensitive response by inhibiting the activity of host catalases. Cell. Microbiol 19, e12736. doi: 10.1111/cmi.12736
Takahashi, Y., Uehara, Y., Berberich, T., Ito, A., Saitoh, H., Miyazaki, A., et al. (2004). A subset of hypersensitive response marker genes, including HSR203J, is the downstream target of a spermine signal transduction pathway in tobacco. Plant J. 40, 586–595. doi: 10.1111/j.1365-313X.2004.02234.x
Tournu, H., Fiori, A., Van Dijck, P. (2013). Relevance of trehalose in pathogenicity: some general rules, yet many exceptions. PloS Pathog. 9, e1003447. doi: 10.1371/journal
Tsuda, K., Katagiri, F. (2010). Comparing signaling mechanisms engaged in pattern-triggered and effector-triggered immunity. Curr. Opin. Plant Biol. 13, 459–465. doi: 10.1016/j.pbi.2010.04.006
Vailleau, F., Sartorel, E., Jardinaud, M.F., Chardon, F., Genin, S., Huguet, T., et al. (2007). Characterization of the interaction between the bacterial wilt pathogen Ralstonia solanacearum and the model legume plant Medicago truncatula. Mol. Plant Microbe Interact. 20, 159–167. doi: 10.1094/Mpmi-20-2-0159
Vargas-Rodriguez, O., Badran, A. H., Hoffman, K. S., Chen, M. Y., Crnkovi, A., Ding, Y. S., et al. (2021). Bacterial translation machinery for deliberate mistranslation of the genetic code. Proc. Natl. Acad. Sci. U.S.A. 118, e2110797118. doi: 10.1073/pnas.2110797118
Wahl, V., Ponnu, J., Schlereth, A., Arrivault, S., Langenecker, T., Franke, A., et al. (2013). Regulation of flowering by trehalose-6-phosphate signaling in Arabidopsis thaliana. Science 339, 704–707. doi: 10.1126/science.1230406
Wang, Y. R., Zhao, A. C., Morcillo, R. J. L., Yu, G., Xue, H., Rufian, J. S., et al. (2021). A bacterial effector protein uncovers a plant metabolic pathway involved in tolerance to bacterial wilt disease. Mol. Plant 14, 1281–1296. doi: 10.1016/j.molp.2021.04.014
Westerink, N., Brandwagt, B. F., De Wit, P. J. G. M., Joosten, M. (2004). Cladosporium fulvum circumvents the second functional resistance gene homologue at the cf-4 locus (Hcr9-4E) by secretion of a stable avr4E isoform. Mol. Microbiol. 54, 533–545. doi: 10.1111/j.1365-2958.2004.04288.x
Xian, L., Yu, G., Wei, Y. L., Rufian, J. S., Li, Y. S., Zhuang, H. Y., et al. (2020). A bacterial effector protein hijacks plant metabolism to support pathogen nutrition. Cell Host Microbe 28, 548–557. doi: 10.1016/j.chom.2020.07.003
Yang, L., Wei, Z. L., Valls, M., Ding, W. (2022). Metabolic profiling of resistant and susceptible tobaccos response incited by Ralstonia pseudosolanacearum causing bacterial wilt. Front. Plant Sci. 12. doi: 10.3389/Fpls.2021.780429
Ye, W. W., Wang, Y. C., Dou, D. L. (2010). SeqHunter: a bioinformatics toolbox for local blast and sequence analysis. China J. Bioinform. 8, 364–367, 377.
Yuan, M. H., Jiang, Z. Y., Bi, G. Z., Nomura, K. Y., Liu, M. H., Wang, Y. P., et al. (2021). Pattern-recognition receptors are required for NLR-mediated plant immunity. Nature 592, 105–109. doi: 10.1038/s41586-021-03316-6
Keywords: RipTPS, avirulence, virulence, Ralstonia solanacearum, plant immunity
Citation: An Y, Chen J, Xu Z, Ouyang X, Cao P, Wang R, Liu P and Zhang M (2022) Three amino acid residues are required for the recognition of Ralstonia solanacearum RipTPS in Nicotiana tabacum. Front. Plant Sci. 13:1040826. doi: 10.3389/fpls.2022.1040826
Received: 09 September 2022; Accepted: 27 September 2022;
Published: 13 October 2022.
Edited by:
Yuheng Yang, Southwest University, ChinaReviewed by:
Yuee Tian, Henan University of Science and Technology, ChinaQiong Zhang, University of California, Berkeley, United States
Furong Liu, University of California, Berkeley, United States
Copyright © 2022 An, Chen, Xu, Ouyang, Cao, Wang, Liu and Zhang. This is an open-access article distributed under the terms of the Creative Commons Attribution License (CC BY). The use, distribution or reproduction in other forums is permitted, provided the original author(s) and the copyright owner(s) are credited and that the original publication in this journal is cited, in accordance with accepted academic practice. No use, distribution or reproduction is permitted which does not comply with these terms.
*Correspondence: Meixiang Zhang, bWVpeGlhbmd6aGFuZ0Bzbm51LmVkdS5jbg==
†These authors have contributed equally to this work