- 1Department of Fruit Tree Sciences, College of Horticulture, China Agricultural University, Beijing, China
- 2Peking University Institute of Advanced Agricultural Science, Shandong Laboratory for Advanced Agricultural Sciences, Weifang, China
- 3Department of Fruit Tree Sciences, Agricultural Research Organization, The Volcani Center, Bet Dagan, Israel
Fig fruits have significant health value and are culturally important. Under suitable climatic conditions, fig fruits undergo a superfast ripening process, nearly doubling in size, weight, and sugar content over three days in parallel with a sharp decrease in firmness. In this study, 119 FcAP2/ERF genes were identified in the fig genome, namely 95 ERFs, 20 AP2s, three RAVs, and one soloist. Most of the ERF subfamily members (76) contained no introns, whereas the majority of the AP2 subfamily members had at least two introns each. Three previously published transcriptome datasets were mined to discover expression patterns, encompassing the fruit peel and flesh of the ‘Purple Peel’ cultivar at six developmental stages; the fruit receptacle and flesh of the ‘Brown Turkey’ cultivar after ethephon treatment; and the receptacle and flesh of parthenocarpic and pollinated fruits of the ‘Brown Turkey’ cultivar. Eighty-three FcAP2/ERFs (68 ERFs, 13 AP2s, one RAV, and one soloist) were expressed in the combined transcriptome dataset. Most FcAP2/ERFs were significantly downregulated (|log2(fold change) | ≥ 1 and p-adjust < 0.05) during both normal fruit development and ethephon-induced accelerated ripening, suggesting a repressive role of these genes in fruit ripening. Five significantly downregulated ERFs also had repression domains in the C-terminal. Seven FcAP2/ERFs were identified as differentially expressed during ripening in all three transcriptome datasets. These genes were strong candidates for future functional genetic studies to elucidate the major FcAP2/ERF regulators of the superfast fig fruit ripening process.
Introduction
Transcription factors play important roles in plant signal transduction by activating or repressing the expression of target genes (Liu and Stewart, 2016). The APETALA2/ETHYLENE RESPONSIVE FACTOR (AP2/ERF) superfamily is a large class of transcription factors that are unique to plants (Licausi et al., 2013). All members of the AP2/ERF superfamily share a conserved AP2 domain, which has an amino acid (aa) length of approximately 60-70 and consists of a three-stranded β-sheet and one α-helix (Allen et al., 1998). AP2/ERFs can be divided into three subfamilies (ERF, AP2, and RAV) based on the number and aa sequences of AP2 domains present (Sakuma et al., 2002; Nakano et al., 2006). The ERF subfamily is the largest and is characterized by the presence of only one AP2 domain. The AP2 subfamily is characterized by two tandem AP2 domains, although a small number of proteins in the AP2 subfamily have only one AP2 domain. The RAV subfamily is much smaller than the other two subfamilies and is characterized by the presence of one AP2 domain and one B3 domain. In recent years, some AP2/ERF members have been assigned to another subfamily, soloist. Members of the soloist subfamily have significantly different aa sequences and gene structures than members of the ERF and AP2 subfamilies (Zhuang et al., 2011; Feng et al., 2020).
In addition to the AP2 domain, some AP2/ERFs also contain conserved activation or repression domains that affect downstream regulation of gene expression. The ERF-associated amphiphilic repression (EAR) motif (L/FDLNL/F(x)P) was the first repressor domain to be confirmed in the AP2/ERF family; it is present in the C-terminal of some AP2/ERF transcription factors (Ohta et al., 2001; Licausi et al., 2013). R/KLFGV is another repressor domain found in the B3 domain of members of the RAV subfamily (Hiratsu et al., 2003; Ikeda and Ohme-Takagi, 2009), and EDLL is a strong acidic-type activation domain (Tiwari et al., 2012).
AP2/ERF transcription factors form one of the largest transcription factor families in plants and are key components in downstream ethylene signal transduction (Franco-Zorrilla et al., 2014); they regulate plant growth, development, stress responses, and other biological processes. In recent years, many studies have shown that AP2/ERF superfamily members are extensively involved in fruit development and ripening by affecting ethylene synthesis, chlorophyll degradation, coloring, fruit softening, and flavor formation (Zhai et al., 2022).
AP2/ERFs are involved in fruit ripening through regulation of the ethylene biosynthesis-related genes 1-aminocyclopropane-1-carboxylate oxidase (ACO) in apple and pear and 1-aminocyclopropane-1-carboxylate synthase (ACS) in banana and apple (Xiao et al., 2013; Han et al., 2016; Li et al., 2016; Hao et al., 2018). AP2/ERFs also participate in chlorophyll degradation, acting as transcriptional activators through binding to the promoters of chlorophyll degradation-related genes in apple (Yin et al., 2016; Han et al., 2018). AP2/ERFs in pear reportedly function together with myeloblastosis (MYB) and basic helix-loop-helix (bHLH) transcription factors to influence anthocyanin accumulation (Yao et al., 2017; Ni et al., 2019; An et al., 2020). AP2/ERFs regulate fruit softening by changing the expression of cell wall-related genes, such as expansin, polygalacturonase, xyloglucan endotransglucosylase/hydrolases (XTHs), pectate lyase, and pectinesterase in banana; polygalacturonase in peach; polygalacturonase and pectinesterase in papaya; and XTH in persimmon and kiwifruit (Yin et al., 2010; Fan et al., 2016; Fu et al., 2016; Han et al., 2016; Wang et al., 2017; Wang et al., 2019). Moreover, AP2/ERFs are involved in the synthesis and accumulation of many specialized metabolites; for example, they regulate the expression of genes related to aroma formation, such as branched-chain amino acid transaminase and pyruvate decarboxylase in banana (Feng et al., 2016) and 2-methylene-furan-3-one reductase in strawberry (Zhang et al., 2018).
Fig (Ficus carica) originated in the Mediterranean area and was one of the earliest domesticated fruit trees. It is an important species, with both dry and fresh fruits eaten worldwide. Fig fruits have significant health value due to their antioxidant properties (Solomon et al., 2006). Fruit growth follows a sigmoidal curve, with stage I characterized by rapid increases in fruit size and weight, stage II having a long lag phase, and stage III characterized by superfast ripening over a very short duration, typically three to seven days. This is substantially shorter than the ripening phase of other common Mediterranean fruits, such as grapes, olives, and pomegranates. During stage III, fig fruit size and weight increase significantly and there is rapid sugar accumulation and fruit softening (Freiman et al., 2015; Kuang et al., 2022).
Fig was initially reported as a climacteric fruit (Marei and Crane, 1971), although in recent years the flesh and receptacle have been described as climacteric and non-climacteric, respectively (Freiman et al., 2015; Lama et al., 2019). Application of ethylene to fig fruits during stage II can accelerate fruit entry into stage III, promoting fig fruit ripening (Cui et al., 2021). Figs are dioecious, and the common female type can bear fruits by parthenocarpy or pollination (Flaishman et al., 2008). In contrast to parthenocarpic fruits, pollinated fruits are larger in diameter and weight, with improved firmness and a more commercially desirable appearance. During storage, senescence and spoilage are slower in pollinated fruits than in parthenocarpic fruits (Rosianski et al., 2016b).
Because fig fruits undergo superfast ripening that can be promoted by ethylene, it has been hypothesized that AP2/ERFs play important roles in fig fruit ripening. However, the AP2/ERF members present in fig and their expression patterns during fruit development have remained largely unknown. In this study, genome-wide identification of AP2/ERF genes was carried out in fig, and the gene structures, motif compositions, and chromosomal positions were determined. To investigate the relationship between fig fruit ripening and AP2/ERF expression, three transcriptomic datasets were used to analyze the expression patterns of AP2/ERF genes in fig fruits under several conditions: at different development stages; with and without ethephon treatment; and in parthenocarpic and pollinated fruits. This is the first genome-wide identification and expression pattern analysis of ethylene transcription factors in fig. This study revealed the most active AP2/ERF genes in fruit ripening, providing a critical reference for understanding the superfast ripening characteristics and quality formation of fig fruits. The results are valuable for future gene function mining studies and gene editing-assisted breeding.
Materials and methods
Physiological parameters of superfast fig fruit ripening
Five-year-old common figs (F. carica var. ‘Brown Turkey’) were used in this study. The trees had been planted from cuttings in the experimental station of China Agricultural University, Beijing, with 3 × 3 m spacing and a vertical trellis system. New shoots were managed with standard hedge training. Fruit ripened sequentially from the bottom to the top of each branch, meaning that fruits at similar heights were in the same developmental stages. At ~10 d before harvest, 32 fruits of different developmental stages were labeled, and the transverse diameter was measured with a vernier caliper every day. Three fruits were harvested every other day to measure fruit texture with a firmness meter (Mitutoyo GY-1 and 3, Japan) and soluble solid content with a refractometer (Atago PAL-1, Japan). There were three technical replicates of each measurement for each fruit.
Identification of AP2/ERF gene family members in F. carica
F. carica genomic data were downloaded from NCBI (DDBJ/EMBL/GenBank access code: VYVB01000000) (Usai et al., 2020). Arabidopsis thaliana AP2/ERF protein sequences were downloaded from TAIR (https://www.arabidopsis.org). Using AtAP2/ERFs as the query sequences, a preliminary search was performed for fig AP2/ERF genes using BLASTP through Tbtools (E-value threshold ≤ 1e-5) (Chen et al., 2020). The Hidden Markov Model (HMM) file for the AP2 domain (PF00847) was downloaded from the Pfam database (http://pfam.xfam.org/), and sequences containing the AP2 domain were retrieved from the fig genome database using HMMER 3.0 (Finn et al., 2011). The results of the two screening methods were combined and redundant gene sequences removed. NCBI Batch-CD analysis confirmed that all resulting gene sequences contained the AP2 domain.
Phylogenetic tree construction and AP2/ERF sequence analysis
All fig and Arabidopsis AP2/ERF protein sequences were aligned with ClustalW (Thompson et al., 2003), then a phylogenetic tree was constructed with MEGA11 using the maximum likelihood (ML) method with the following parameters: test of phylogeny, bootstrap method; number of bootstrap replicates, 1000; substitution type, amino acid; model/method, Jones-Taylor-Thornton (JTT) model; rates among sites, uniform rates; gaps/missing data treatment, use all sites; ML heuristic method, nearest-neighbor-interchange (NNI). Sequence length, molecular weight, and isoelectric point (pI) were computed with ProtParam (https://web.expasy.org/protparam/). The conserved motifs in AP2/ERF proteins were determined using MEME (http://meme.nbcr.net/meme/intro.html). Finally, gene structure was visualized with TBtools (Chen et al., 2020). The FcAP2/ERF promoters (the 2000-bp regions upstream of the start codon of each gene) were extracted from the fig genome and submitted to the PlantCare database (http://bioinformatics.psb.ugent.be/webtools/plantcare/html/) for identification of putative cis-regulatory elements.
Chromosomal location and gene duplication
Chromosomal locations of fig AP2/ERF genes were determined using TBtools (Chen et al., 2020). Genomic data were obtained from http://plants.ensembl.org/index.html for Vitis vinifera and Solanum lycopersicum and from BIG Data Center (https://bigd.big.ac.cn/gsa/) for Ficus hispida and Ficus microcarpa (BioProject Accession number GSA: PRJCA002187) (Zhang et al., 2020). Interspecific and intraspecific syntenic analyses were performed with the Multiple Collinearity Scan toolkit (Wang et al., 2012). KaKs Calculator 2.0 was used to calculate the nonsynonymous substitution rate (Ka) to synonymous substitution rate (Ks) ratios (Wang et al., 2010). The divergence times in millions of years ago (Mya) were calculated as follows (Lynch and Conery, 2000): T = Ks/(2 × 6.1 × 10-9) ×10-6.
AP2/ERF expression analysis in F. carica during fruit ripening
Expression levels of AP2/ERF genes were analyzed in the peel and flesh of ‘Purple Peel’ fig fruits during development by re-mining our previously sequenced and annotated transcriptome data (SRA accession: PRJNA723733) (Zhai et al., 2021). Briefly, six samples were taken during fruit development; samples 1 through 6 were taken at early stage I, mid stage I, early stage II, late stage II, mid stage III, and late stage III, respectively. Fruit peels (P) and flesh (F) were isolated and assayed separately at each timepoint (P1-P6 and F1-F6, respectively). There were three biological replicates for each sample. The RNA-Seq data generated from samples were matched to our laboratory’s previous transcriptome database using RSEM (RNA-Seq by Expectation Maximization) software package (Chai et al., 2017). Expression patterns were analyzed for AP2/ERF genes expressed at levels ≥ 20 fragments per kilobase of transcript per million mapped reads (FPKM) in at least one sample. If (sum_F)/(sum_P) was > 5 or < 0.2, a gene was defined as dominantly expressed in the flesh or peel, respectively. If (F4 + F5 + F6)/(F1 + F2 + F3) or (P4 + P5 + P6)/(P1 + P2 + P3) was > 2 or < 0.5, a gene was defined as positively or negatively correlated with fruit ripening, respectively.
The expression patterns of AP2/ERF genes in the fig fruit flesh and peel in response to ethephon treatment were analyzed by re-mining our previously sequenced and annotated transcriptome data (SRA accession: PRJNA606407) (Cui et al., 2021). Briefly, ‘Brown Turkey’ fig fruits in stage II were injected with 1 mL of 250 mg/L ethephon from the ostiole. Control and ethephon-treated fruits were collected at two, four, and six days after treatment (DAT), and fruit flesh and receptacle (R) transcriptomes were analyzed. There were three biological replicates of each sample. Annotation was conducted as described above for ‘Purple Peel’ samples. Gene expression patterns were analyzed for AP2/ERFs with values ≥ 20 transcripts per million (TPM) in at least one sample. Differentially expressed FcAP2/ERFs were classified as those with |log2(fold change) | ≥ 1 in an ethephon-treated sample compared to the control sample at the same timepoint in the same tissue.
AP2/ERF expression was also analyzed in pollinated and parthenocarpic ‘Brown Turkey’ fig fruits at two stages of development through re-mining transcriptome data submitted by the Flaishman group (SRA accession: PRJNA322124) (Rosianski et al., 2016a). Briefly, parthenocarpic (Par) and pollinated (Pol) fruits were collected at 60% and 100% ripeness (Par/Pol_60 and Par/Pol_100, respectively); RNA was extracted from the flesh and receptacles for sequencing and annotation. The raw sequencing reads were downloaded and mapped to the fig genome (DDBJ/EMBL/GenBank access code: VYVB01000000) using a series of plug-ins in TBtools, namely FastQC, Trimmomatic, and Kallisto (Chen et al., 2020). After obtaining a gene expression matrix, expression patterns were analyzed for AP2/ERFs that had FPKM values ≥ 20 in at least one sample. Differentially expressed AP2/ERFs were classified as those with |log2(fold change) | ≥ 1 in Par/Pol_100 compared to Par/Pol_60.
Quantitative reverse transcription (qRT)-PCR validation of AP2/ERF gene expression during fruit development
Total RNA was extracted from ‘Purple Peel’ fruits at six developmental stages as described in our previous publications (Cao et al., 2016; Chai et al., 2017). cDNA was prepared with the PrimeScript RT Reagent Kit (Takara, Dalian, China) following the manufacturer’s instructions. AP2/ERF genes with relatively high expression levels were used for qRT-PCR validation, including RAV and ERF members, as well as genes with repression domains. Primer pairs for eight AP2/ERF genes were designed with Primer3 (https://bioinfo.ut.ee/primer3/). qRT-PCR was performed on an ABI QuantStudio 6 using TB Green® Premix Ex Taq (RR420Q, Takara) with three technical replicates for each sample. The reaction conditions were as follows: 95°C for 30 s; 40 cycles of 95°C for 5 min and 60°C for 34 s. The 2−△△CT method (Livak and Schmittgen, 2001) was used to determine relative gene expression using elongation factor (c59932_g1) as the internal control.
Gene co-expression and protein interaction network analyses
Gene co-expression analysis and protein interaction network analysis were performed with Majorbio (https://cloud.majorbio.com). Spearmanʼs correlation coefficient was used to calculated gene co-expression. STRING (https://www.string-db.org) was used to generate the protein-protein interaction network based on the interaction network of homologs in A. thaliana. Connections were visualized in Cytoscape (Kohl et al., 2011).
Results
Superfast fig fruit ripening
Fig fruits showed very quick changes in major quality parameters during the last 10 d before they reached full ripeness. During the last four days, the transverse diameter and weight of fruits in stage III increased by an average of 4.49 mm and 10.24 g, respectively. The average increases in transverse diameter and weight per day for the last four days were 10.85% and 27.41%, respectively. This was a significant change compared to the average increases of 2.65% and 8.00% per day observed in late stage II. In addition, during the last four days, the soluble solid content increased by an average of 2.11°Brix (22.23% average increase per day) and the hardness decreased by an average of 2.65 kg/cm2 per day (32.33% average decrease per day). In contrast, there were only 11.80% average increases in soluble solid content and 8.60% average decreases in hardness per day in late stage II. Over two days (day 7 to day 9), the fruits reached commercial maturity; they were fully ripe one day later (Figure 1).
F. carica AP2/ERF family member identification and gene structure
Putative fig AP2/ERF genes identified through Arabidopsis homologous gene alignment and Markov Model predictions were merged to remove redundant sequences. After NCBI Batch-CD analysis was performed to remove erroneous sequences, there were 119 unique candidate AP2/ERF genes in the fig genome. The aa sequence lengths of the encoded proteins ranged from 90-737, the protein molecular weights ranged from 10.0-80.4 kDa, and pI values ranged from 4.25-11.48 (Figure 2 and Supplementary Table 1). Phylogenetic and sequence domain analysis revealed the presence of one soloist gene, 95 ERFs, 20 AP2s, and three RAV genes. Based on homology, the 95 ERFs were further divided into 15 subclasses (Ia, Ib, II, III, IV, Va, Vb, VIa, VIb, VIIa, VIIb, VIII, IX, Xa, and Xb) based on the classification of homologous genes in Arabidopsis (Nakano et al., 2006). Of the 20 AP2s, 14 contained two AP2 domains and six contained one AP2 domain (Figures 2 and 3).
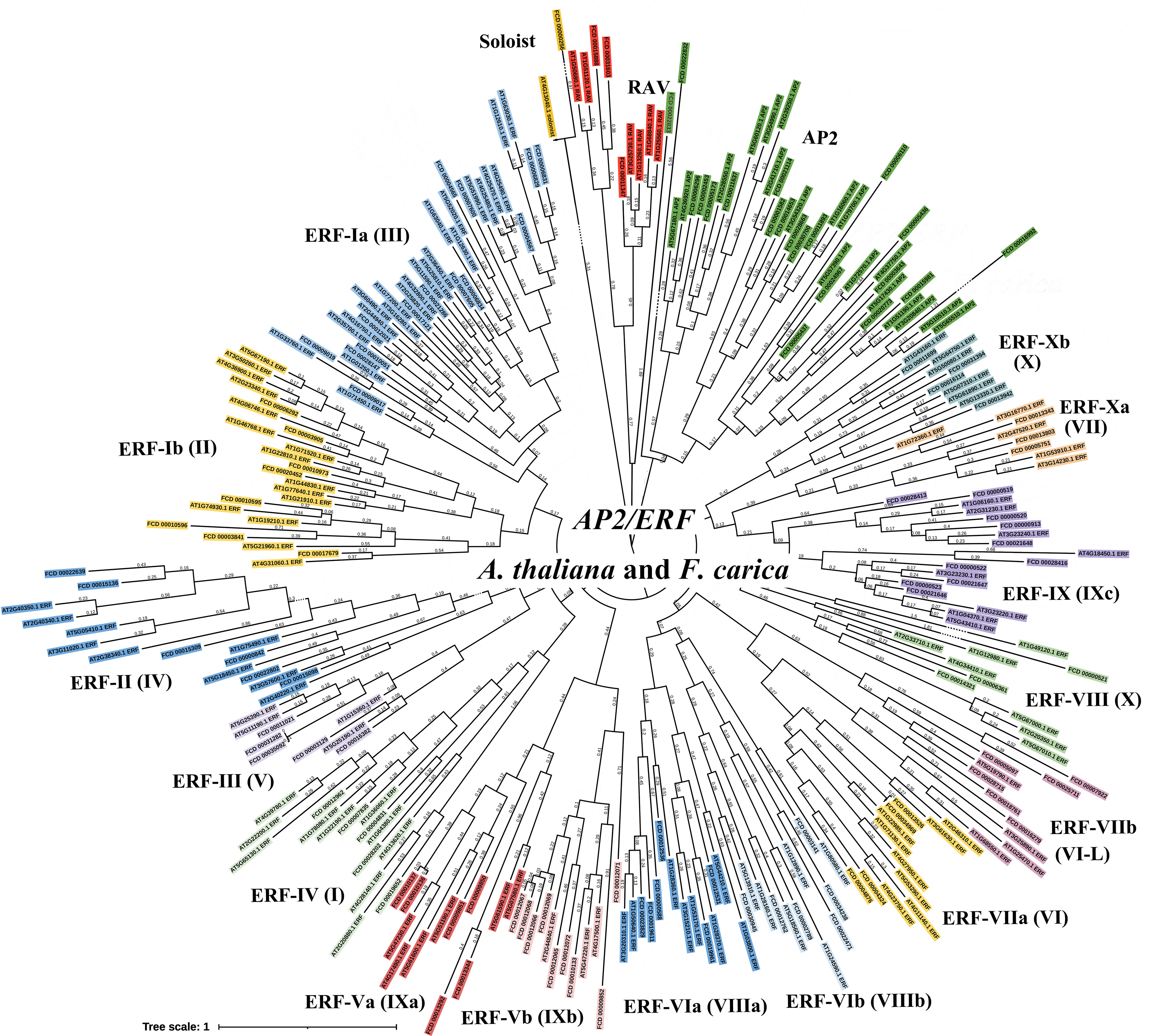
Figure 2 Phylogenetic tree showing the relationships between the 119 AP2/ERFs in F. carica and homologs in A. thaliana. There were 1000 bootstrap replicates.
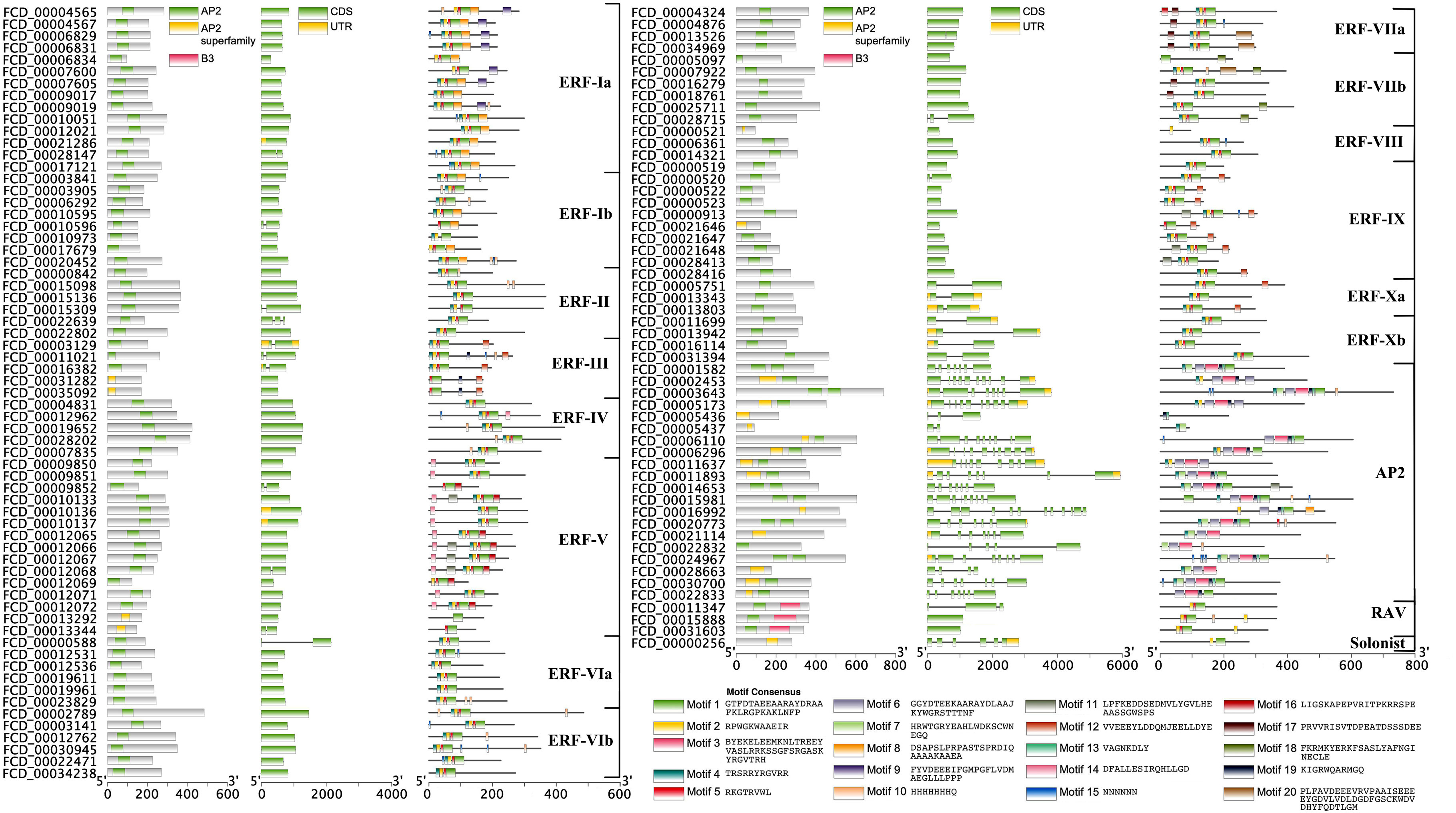
Figure 3 Analyses of domains (left), gene structure (middle), and motifs (right) in F. carica AP2/ERFs.
Gene structure analysis showed that there were 76 AP2/ERF genes without introns, including 74 ERFs and 2 RAVs. Only 21 out of the 95 ERFs contained introns, of which two contained two introns and the rest contained only one intron. Interestingly, the AP2 subfamily generally had more introns—all 20 AP2s contained introns and 16 (80%) contained more than five introns. FCD _00016992 contained the most introns (11) and exons (12) (Figure 3). Motif analysis demonstrated that most ERF subfamily members contained motifs 1, 2, 4, and 5; the 4-2-5-1 series was a feature of most ERF members. The majority of AP2 subfamily members contained motifs 3 and 6, which were unique to the AP2 subfamily. All three RAVs contained motifs 1, 2, and 5, and the soloist (FCD _00000256) contained only motifs 1 and 2 (Figure 3).
There were additional domains present in only a few of the AP2/ERFs. Four ERFs had EDLL activation domains in the C-terminal. Six ERFs and two AP2s contained the EAR repression domain. Two RAVs contained the R/KLFGV repression domain in the C-terminal. Significantly, both the middle and the C-terminal of one ERF (FCD_00012531) contained the EAR repression domain (Supplementary Figure 1).
Chromosome distribution, collinearity, and synteny analysis
Of the 119 AP2/ERF genes identified, 116 were unevenly distributed across 13 chromosomes (Supplementary Figure 2). Three genes (one RAV and two ERFs) could not be located on any of the chromosomes (Supplementary Table 2). Fourteen AP2/ERF genes were located on the longest chromosome (chromosome 5), whereas there were only two on chromosome 6. Chromosomes 3 and 11 each contained two RAVs. Chromosomes 6, 9, and 12 had only ERF members (Supplementary Figure 2). Tandem duplication had occurred in AP2/ERF gene clusters located on chromosomes 4, 8, 9, 10, and 12. Phylogenetic analysis also showed clustering of tandem duplicates on those chromosomes (Figure 3 and Supplementary Figure 2).
A total of 215 collinear blocks were identified from analysis of collinearity among AP2/ERF genes in the fig genome. Twenty-one FcAP2/ERF genes, comprising four AP2 and 17 ERF members, were unevenly distributed in 18 of these blocks (Figure 4A). Blocks 75 and 119 contained three and two AP2/ERF genes, respectively. The other blocks contained only one AP2/ERF gene each (Supplementary Table 3). Analysis of the Ka/Ks ratios revealed 32 pairs of paralogous FcAP2/ERF genes: 21 derived from segmental duplication and 11 from tandem duplication (Supplementary Table 4). The Ka/Ks ratios of the 32 gene pairs ranged from 0.05 to 0.57, suggesting that purifying selection was the primary force operating on these duplicate genes. The duplication events from which the 32 gene pairs were derived occurred between 1.81 and 379.01 Mya (Supplementary Table 4).
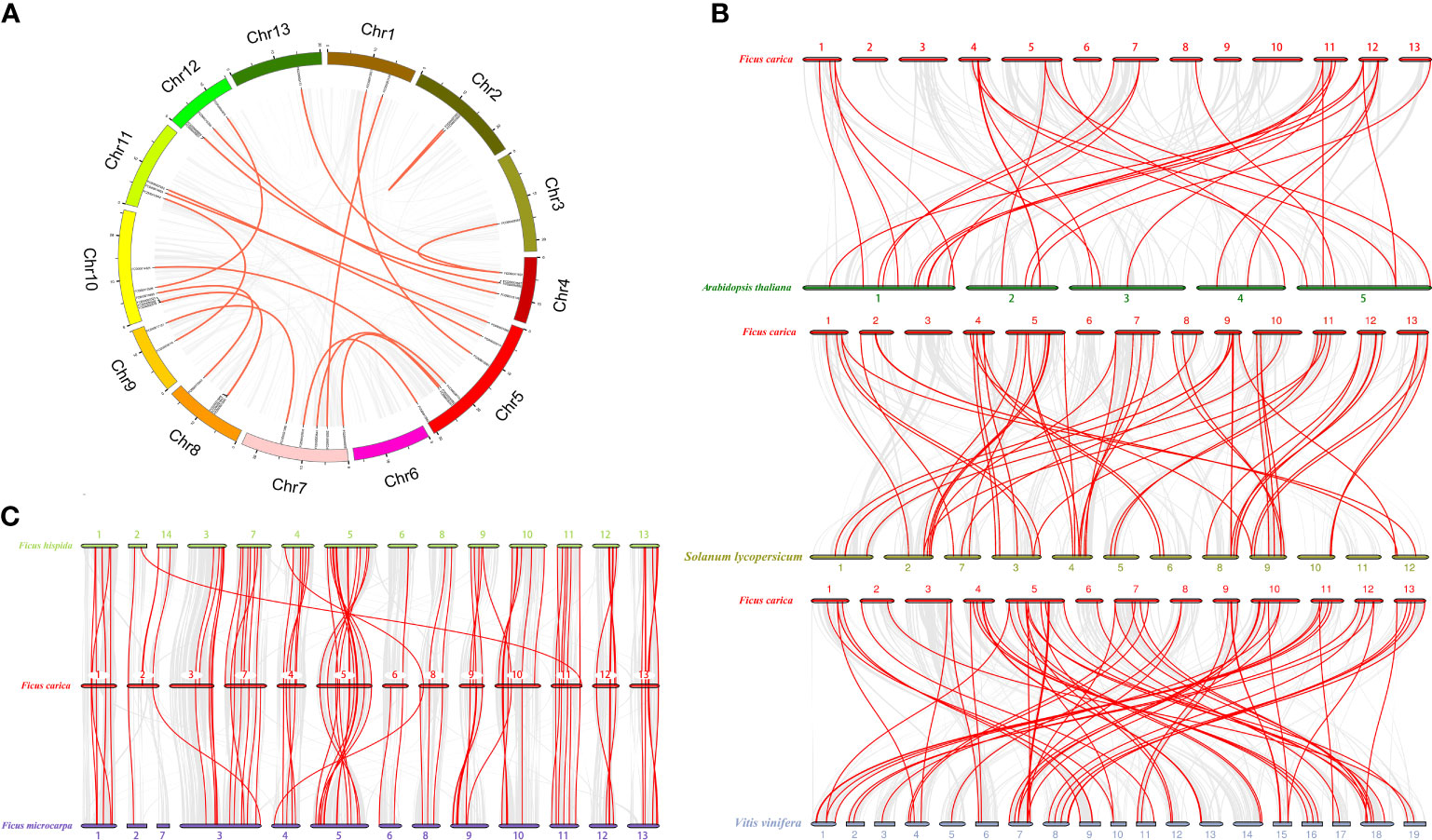
Figure 4 Collinearity and synteny analyses of FcAP2/ERF genes. (A) Collinearity of FcAP2/ERF genes. (B) Synteny analysis of AP2/ERF genes in F. carica and three other plant species. (C) Synteny analysis of AP2/ERF genes in three Ficus species (F. carica, F. hispida, and F. macrocarpa). Gray lines indicate all syntenic gene pairs within the genomes; red lines indicate syntenic AP2/ERF gene pairs.
Syntenic analyses of AP2/ERF genes in fig, A. thaliana, V. vinifera, S. lycopersicum, F. hispida, and F. macrocarpa demonstrated that there was relatively high conservation of synteny between F. carica and F. hispida (83 orthologous gene pairs) (Figures 4B, C). There were 32, 58, 71, and 72 orthologous pairs between fig and A. thaliana, V. vinifera, S. lycopersicum, and F. macrocarpa, respectively (Supplementary Table 5). Sixteen FcAP2/ERFs were found in syntenic regions between all five species: one RAV (FCD_00011347), one AP2 (FCD_00006296), and 14 ERFs (Supplementary Figure 3A), suggesting that these genes were highly evolutionarily conserved. Sixty-four FcAP2/ERFs (two RAVs, 16 AP2s, and 46 ERFs) were also found in syntenic relationships with both F. hispida and F. macrocarpa (Supplementary Figure 3B).
Expression patterns during fruit ripening
Gene expression levels were next analyzed for AP2/ERFs using previously generated ‘Purple Peel’ transcriptomes. There were 83 FcAP2/ERFs (namely 68 ERFs, 13 AP2s, one RAV, and one soloist gene) expressed in the flesh and peel samples at six developmental timepoints (Figure 5 and Supplementary Figure 4). Genes were divided into three groups based on expression level: group A (containing genes with maximum FPKM values from 300-1800), group B (maximum FPKM from 20-300) and group C (maximum FPKM ≤ 20). There were 19, 32, and 32 AP2/ERF genes in groups A, B, and C, respectively. Group A consisted of one RAV and 18 ERF genes; group B contained one soloist, three AP2, and 28 ERF genes; and group C contained 10 AP2s and 22 ERFs. Most of the AP2 genes had relatively low FPKM values and were therefore in group C (Figure 5B and Supplementary Figure 4).
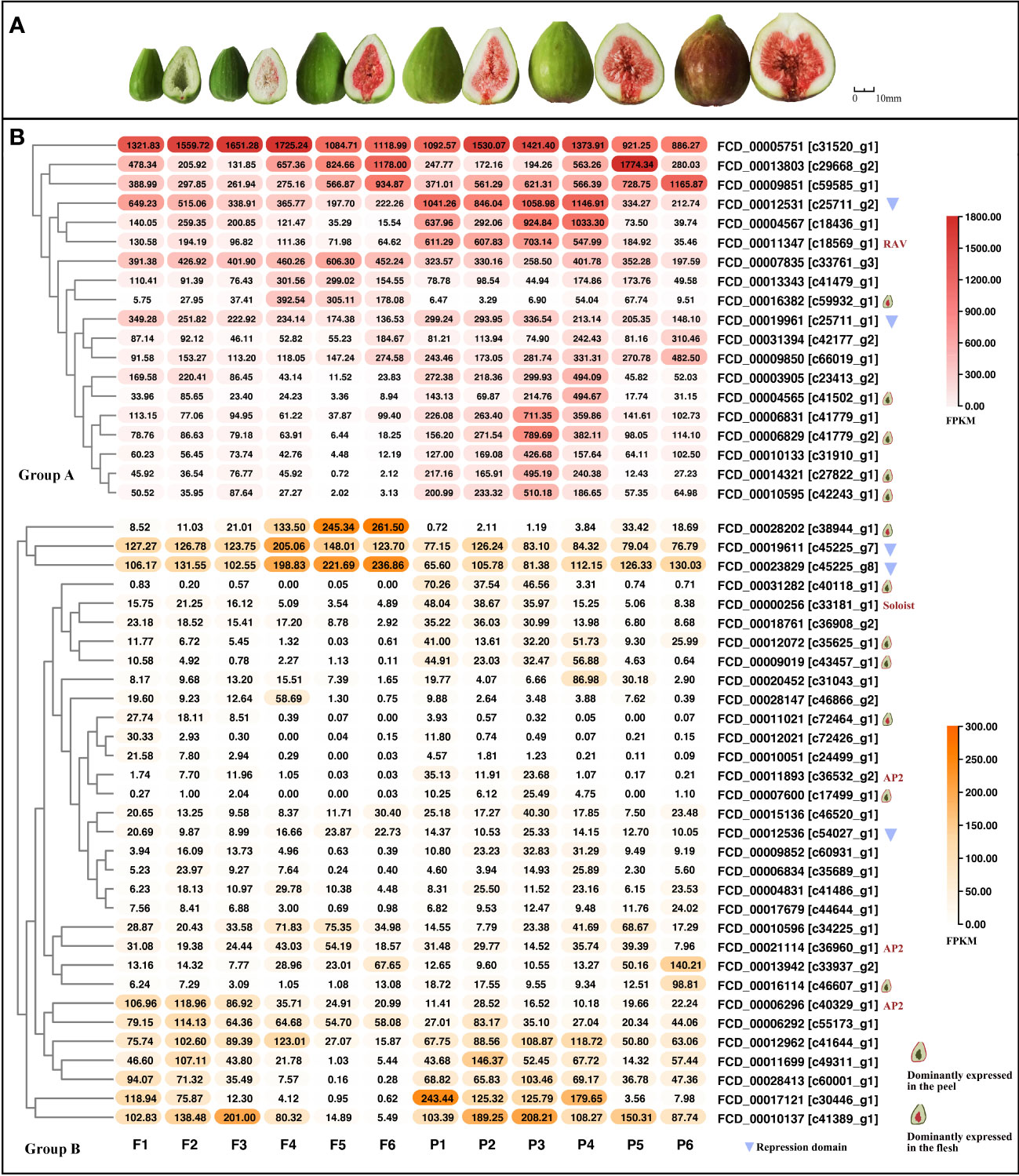
Figure 5 Expression patterns of FcAP2/ERF genes during fig fruit development. FcAP2/ERF genes were divided into three groups based on expression levels: group A (maximum FPKM between 300 and 1800), group B (maximum FPKM between 20 and 300) and group C (maximum FPKM ≤ 20). (A) Six stages of fig fruit development. (B) Expression patterns of genes in groups A and (B) Members of group C are shown in Supplementary Figure 3.
AP2/ERFs with FPKM values ≥ 20 in at least one of the 12 samples (two tissues at six timepoints) were further analyzed. Genes were categorized as being dominantly expressed in either the flesh or peel if they had a greater than five-fold difference in the sum of FPKM values for all samples from either the flesh or the peel tissue, respectively. There were nine FcAP2/ERFs dominantly expressed in the peel and three in the flesh (Figure 5B and Supplementary Table 6). FCD_00007600 [c17499_g1] was the most dominantly expressed ERF in the flesh; when the FPKM values were summed across all samples, it was expressed 14.28 times more highly in the flesh than in the peel. Furthermore, this gene was conserved among the three Ficus species studied here. FCD_00031282 [c40118_g1] was the most dominantly expressed ERF in the peel, with the summed expression being 96.44 times higher in the peel than in the flesh (in which the FPKM was < 1 at all six stages) (Supplementary Table 6). This ERF was shown to have homologs in S. lycopersicum and F. hispida (Supplementary Table 5).
Nine ERFs were found to be positively correlated with fruit ripening; in contrast, 27 AP2/ERFs (one RAV, one AP2, one soloist, and 24 ERFs) were negatively correlated with fruit ripening (Figure 6A). Of the genes significantly correlated with fruit ripening, eight ERFs were dominantly expressed in the peel, all of which were negatively correlated with fruit ripening. Three ERFs were dominantly expressed in the flesh and had similar expression patterns; one was positively correlated and two were negatively correlated with fruit ripening. Although there were large differences in the expression levels of these genes between the flesh and peel, the expression patterns were comparable (Figure 6A). The FPKM values of FCD_00013803 [c29668_g2] and FCD_00012531 [c25711_g2] were expressed at extremely high levels. The former was positively correlated with ripening and was conserved among the three Ficus species, whereas the latter was negatively correlated with ripening and was conserved among all five species used in the syntenic analysis (Figure 6A Supplementary Figure 3). The Arabidopsis homolog of FCD_00013803, AtERF73, functions as a transcriptional activator in the hypoxia response and in root development (Seok et al., 2014). FCD_00012531 contained two EAR repression motifs (Supplementary Figure 1). Tobacco (Nicotiana tabacum) contains a homolog of FCD 00012531, NtERF4, which also has an EAR repression motif and acts as a transcriptional repressor (Ohta et al., 2000).
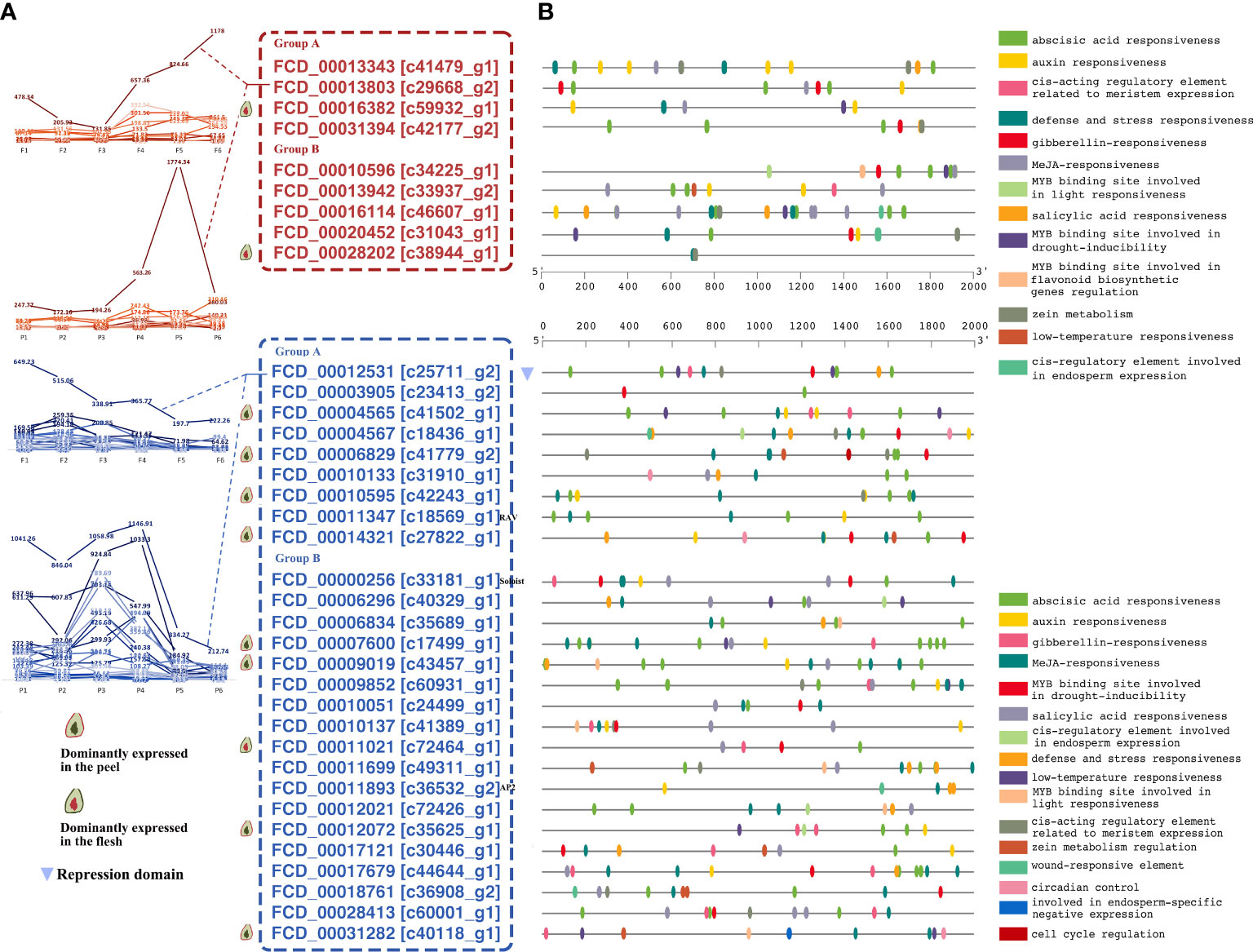
Figure 6 FcAP2/ERF genes significantly correlated with fruit ripening and analysis of their promoter regions. The thresholds used were FPKM ≥ 20 in at least one sample and the sum of expression values on days four through six divided by the sum of expression values on days one through three > 2 or < 0.5. (A) FcAP2/ERF genes positively or negatively correlated with fruit ripening. (B) Promoter region analysis of FcAP2/ERF genes significantly correlated with fruit ripening.
Analysis of the promoter regions showed that there were no significant differences in the type or number of promoter elements between genes positively and negatively correlated with fruit ripening. The main elements in the promoters of FcAP2/ERFs were hormone-related and abscisic acid (ABA) response-related elements (Figure 6B), demonstrating the importance of ABA and ethylene in fig fruit ripening and suggesting crosstalk between the two pathways. Four hormone-related elements, namely responsiveness to ABA, auxin, gibberellin, and methyl jasmonate (MeJA), were present in the promoter region of FCD_00013803[c29668_g2], which was significantly positively correlated with ripening. The promoter of FCD_00012531[c25711_g2], which was significantly negatively correlated with ripening, contained elements related to both hormones (ABA and gibberellin) and stress resistance (e.g., low temperature and defense responses) (Figure 6B).
Eight FcAP2/ERF genes were selected for qRT-PCR verification of the ‘Purple Peel’ fruit ripening RNA-Seq results (Supplementary Table 7). The expression patterns observed via qRT-PCR were similar to those seen in the RNA-Seq results (R2 = 0.72) (Supplementary Figure 5).
Ethephon treatment altered AP2/ERF gene expression patterns in fruits
Treatment with ethephon caused fig fruits in stage II to ripen six days earlier than control fruits. Fruits treated with ethephon were larger and softer and pigmented faster. After removing lowly-expressed genes, 34 expressed FcAP2/ERF genes were identified, one RAV (FCD_00011347 [c18569_g1]) and 33 ERFs, with ≥ two-fold changes in expression in the flesh or receptacle after ethephon treatment (Figure 7 and Supplementary Table 8).
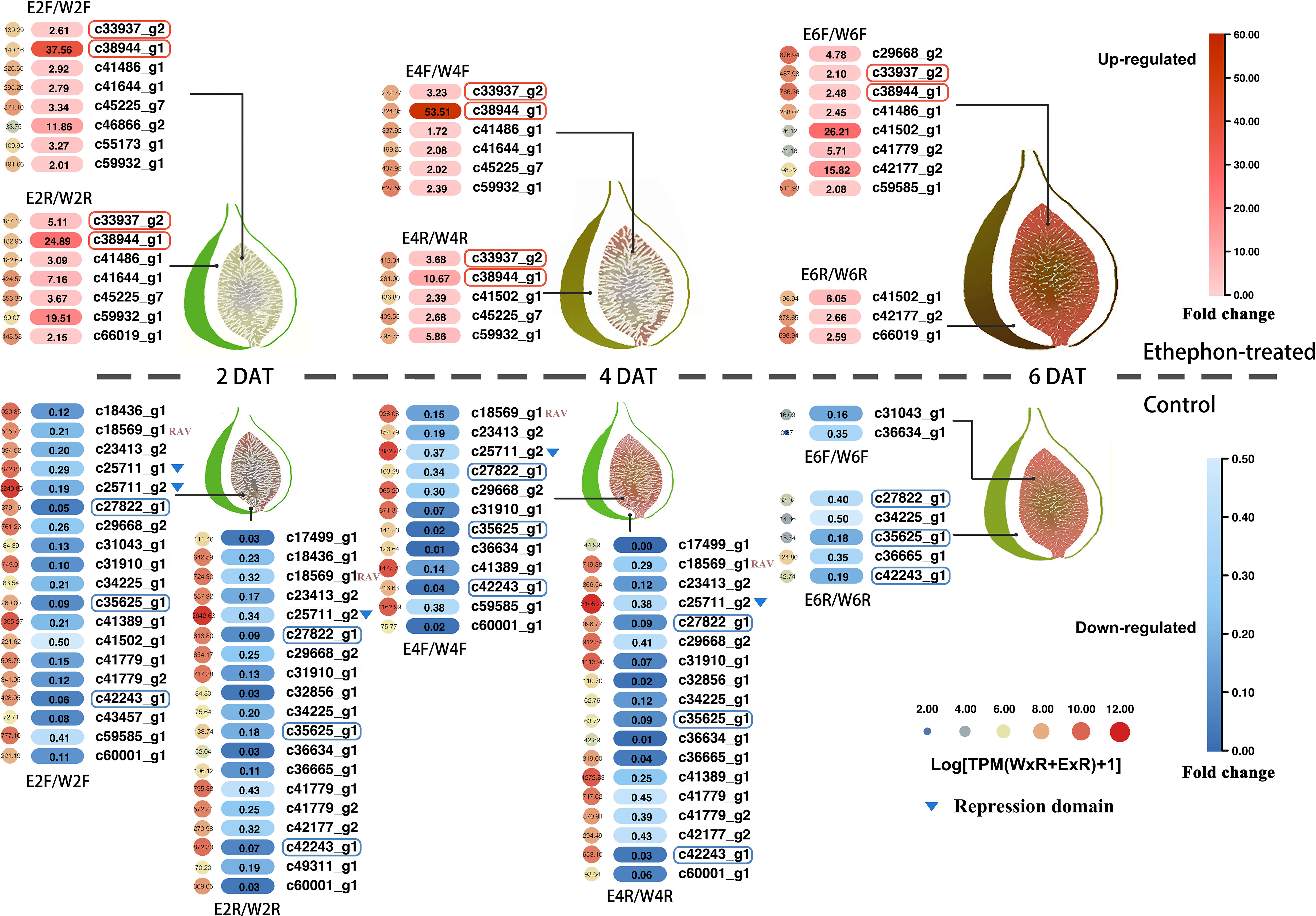
Figure 7 Changes in FcAP2/ERF gene expression in fig fruit flesh and receptacle tissues after ethephon treatment. Color intensity indicates the ratio of expression in ethephon-treated tissues compared to control tissues. Genes circled in red or blue were differentially expressed in all five comparisons of treated to control tissues.
Differentially expressed genes were then analyzed in the flesh (F) and receptacle (R) of ethephon-treated fruits (E) compared to the controls (W) on each sampling day (two, four, and six) for a total of six comparisons: E2F vs. W2F; E2R vs. W2R; E4F vs. W4F; E4R vs. W4R; E6F vs. W6F; and E6R vs. W6R. There were eight, seven, six, five, eight, and three AP2/ERF genes upregulated and 19, 19, 12, 18, two, and five AP2/ERF genes downregulated in E2F, E2R, E4F, E4R, E6F, and E6R, respectively. There were more FcAP2/ERFs downregulated than upregulated at days two and four after ethephon treatment (Figure 7). Two ERF genes (FCD_00013942 [c33937_g2] and FCD_00028202 [c38944_g1]) were upregulated in E2F, E2R, E4F, E4R, and E6F. The gene c38944_g1 was most highly upregulated in E2F and E4F, at 37.56 and 53.51 times, respectively (Figure 7). Three ERF genes (FCD_00014321 [c27822_g1], FCD_00012072 [c35625_g1], and FCD_00010595 [c42243_g1]) were downregulated in E2F, E2R, E4F, E4R, and E6R. Two ERF genes (FCD_00019961 [c25711_g1] and FCD_00012531 [c25711_g2]), which contained EAR repression domains, were downregulated in E2F, and the latter was also downregulated in E4F, E2R, and E4R. The RAV gene (FCD_00011347 [c18569_g1]) was downregulated in response to ethephon in E2F, E2R, E4F, and E4R, suggesting a repressive role of these genes in fruit ripening (Figure 7).
AP2/ERF expression patterns during pollinated and parthenocarpic fruit ripening
Gene expression was next compared between pollinated and parthenocarpic fruits in a total of four conditions: flesh and receptacle samples each from fruits at 60% and 100% ripeness (Figure 8). Fifteen differentially expressed FcAP2/ERF genes were identified using threshold values of TPM ≥ 20 in at least one of the eight samples and |log2(fold change) | ≥ 1 in fruits at 100% ripeness compared to 60% ripeness. One RAV gene (FCD_00011347 [c18569_g1]) and 11 ERFs differentially expressed between 100% and 60% ripeness were also differentially expressed in response to ethephon treatment (Figure 8). Interestingly, most FcAP2/ERFs were upregulated at 100% compared to 60% ripeness. There were only two downregulated ERFs, FCD_00004567 [c18436_g1] and FCD_00012962 [c41644_g1], in 100% vs. 60% ripe parthenocarpic fruit receptacles; these were also downregulated during ‘Purple Peel’ fig fruit development and were conserved among all five plant species included in the syntenic analysis (Figure 8 and Supplementary Figure 3). The RAV gene (FCD_00011347 [c18569_g1]) was upregulated in the flesh of 100% ripe compared to 60% ripe pollinated fruits. FCD_00031394 [c42177_g2] was upregulated in 100% ripe compared to 60% ripe fruits, in both parthenocarpic and pollinated fruit flesh and receptacles. In the ethephon treatment data, FCD_00031394 [c42177_g2] was also found to be upregulated during ripening and at the late ripening stage (E6F vs. W6F and E6R vs. W6R) (Figures 6 and 7). Thus, c42177_g2 may be a positive regulator of fruit ripening.
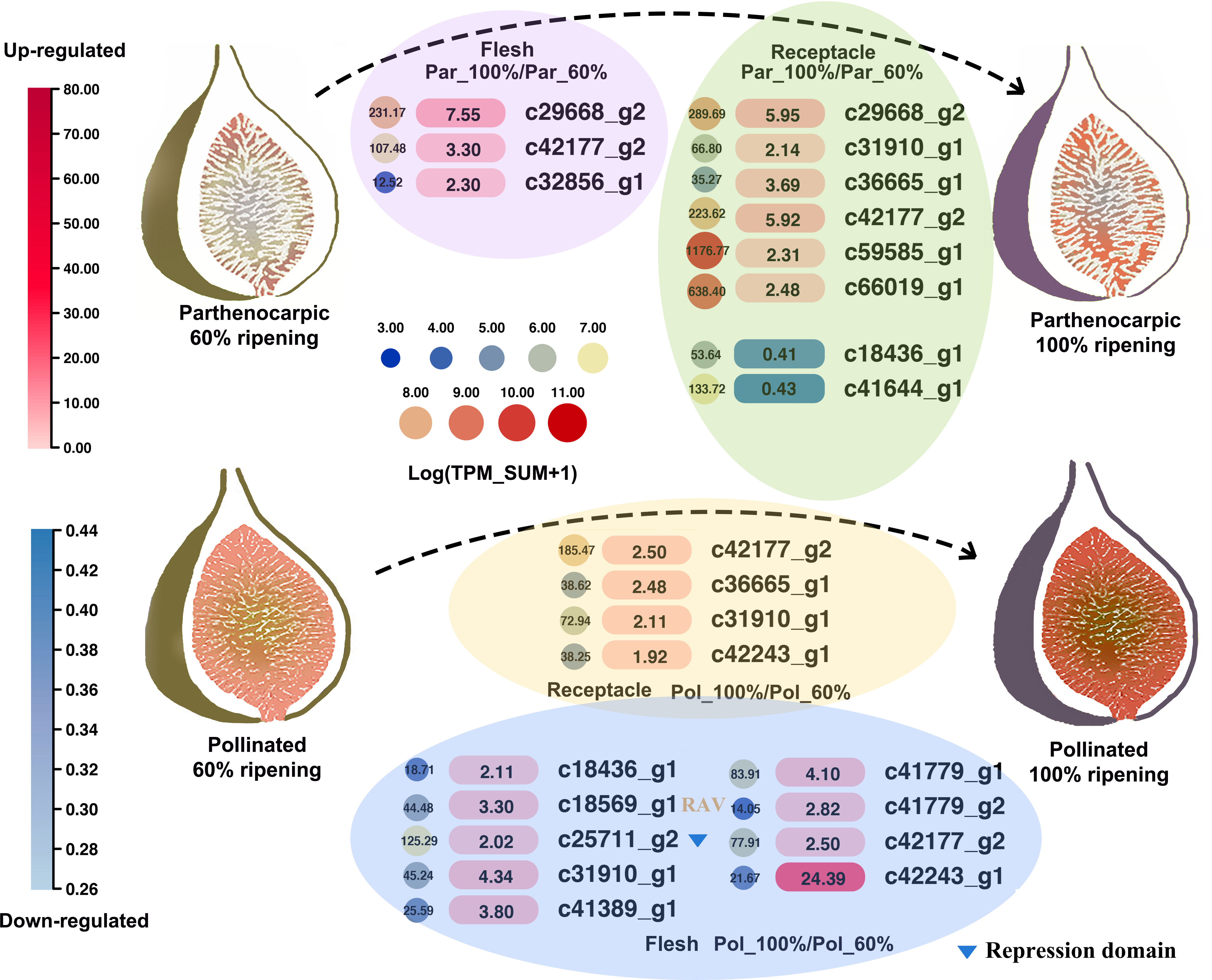
Figure 8 Changes in FcAP2/ERF gene expression between fig fruit flesh and receptacles at 60% and 100% ripeness in pollinated and parthenocarpic fruits. Color intensity indicates the ratio of expression in 100% compared to 60% ripe tissues.
Potential key FcAP2/ERFs in superfast fruit ripening
To identify FcAP2/ERFs associated with superfast fig fruit ripening, multiple datasets were integrated and genes that were consistently differentially regulated at the superfast ripening stage were screened. For each condition, comparisons were made by calculating the ratio of gene expression in the riper compared to the less ripe fig fruit. Specifically, the samples compared were F6/F5 and R6/R5 in ‘Purple Peel’ and E4/W4, E6/W6, Pol_100/Pol_60, and Par_100/Par_60 in the flesh and receptacles of ‘Brown Turkey’. Six ERFs (from the ERF-I, -V, and -X subgroups) and one RAV were identified as differentially regulated in all datasets (Figure 9A).
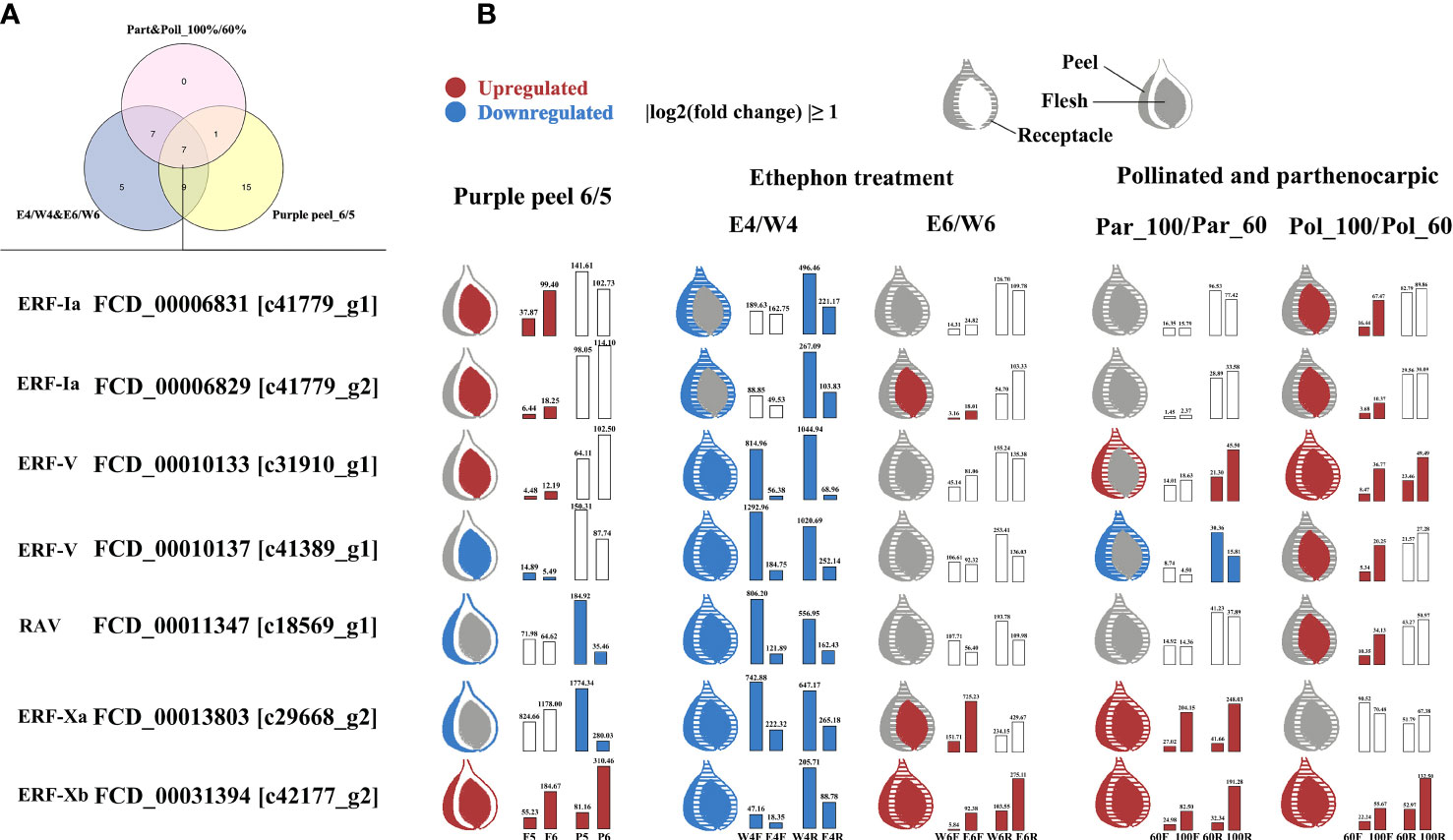
Figure 9 Summary of expression patterns for seven key FcAP2/ERF genes identified as differentially expressed in three transcriptomic datasets. (A) The intersection of FcAP2/ERFs differentially expressed in all three transcriptomic datasets. (B) Fruit ripening comparisons. The bar graph shows gene expression values in FPKM or TPM.
These seven consistently differentially regulated FcAP2/ERFs were all downregulated four days after ethephon treatment (Figure 9B). FCD_00006829 [c41779_g2] and FCD_00031394 [c42177_g2] were upregulated in the flesh, peel, and receptacle during fig fruit ripening at levels between 1.02 times (receptacles of Pol_100 vs. Pol_60) and 15.82 times (E6F vs. W6F). These two ERFs may therefore be activators of fig fruit ripening. FCD_00010137 [c41389_g1] and FCD_00011347 [c18569_g1] were downregulated in the flesh, peel, and receptacle during ‘Purple Peel’ fruit ripening and in ethephon-treated and parthenocarpic ‘Brown Turkey’ fruits; these genes may therefore be repressors of fruit ripening. The level of downregulation was between 0.14 times (E4F vs. W4F) and 0.96 times (flesh of Par_100 vs. Par_60). Pollination also altered expression of the two ERFs; they were upregulated in the flesh and receptacle from 60% to 100% ripeness in pollinated figs (Figure 9B).
Arabidopsis homologs of the seven consistently differentially expressed FcAP2/ERFs were identified and interacting proteins were screened (Supplementary Table 9). The most striking result was observed for the homolog of FcRAV (FCD_00011347 [c18569_g1]), which was considered to be a repressor because it was downregulated during fruit ripening in ‘Purple Peel’ and after ethephon treatment in ‘Brown Turkey’ (Figures 6 and 7). The homolog, AT1G13260, interacted with Topless-Related proteins (TPRs), Highly ABA-Induced (HAI1), and Sucrose Nonfermenting 1-Related Protein Kinases (SNRKs). TPRs always act as transcriptional co-repressors (Xu et al., 2021), suggesting that the RAV gene in fig may inhibit fruit ripening by recruiting transcriptional co-repressors. HAI1 and SNRKs are involved in the ABA signaling network (Chong et al., 2019; Shang et al., 2022), indicating that the RAV gene may be regulated by both ethylene and ABA.
Discussion
In this study, genome-wide identification and gene structure analyses were carried out for AP2/ERF gene family members in F. carica for the first time. Due to the extensive involvement of AP2/ERFs in fruit ripening, three transcriptomic datasets related to fig fruit ripening were used to identify FcAP2/ERF genes expressed in fig fruits and to measure their expression patterns. Our findings provide new insights into the expression patterns and possible functions of FcAP2/ERFs and establish promising candidate fruit ripening-related FcAP2/ERFs for further study.
Evolution of the AP2/ERF family
Gene duplication plays an important role in plant evolution. Homologous genes are generated by mechanisms such as tandem and segmental duplication, which form the basis for the emergence of new genes and novel functions (Birchler and Yang, 2022). The 119 FcAP2/ERF genes identified in the fig genome were found to have been derived from tandem and segmental duplication events. Furthermore, syntenic analysis with A. thaliana, V. vinifera, S. lycopersicum, F. hispida, and F. macrocarpa showed that 16 of the FcAP2/ERF genes had homologs in all five species (Supplementary Figure 3). These 16 FcAP2/ERF genes therefore appeared to be evolutionarily conserved and may have existed in a common ancestor. Although the origin of the AP2/ERF family in plants is uncertain, it has been speculated that it resulted from the transfer of an HNH-AP2 endonuclease gene from bacteria or viruses into plants (Magnani et al., 2004).
Significantly, FcAP2 subfamily members contained far more introns than other AP2/ERF subfamilies in fig. Eukaryotic genes can be classified as intron-less (no introns), intron-poor (three or fewer introns) or intron-rich (more than three introns) (Liu et al., 2021). FcAP2 subfamily members were found to be intron-rich (Figure 3). Studies have shown that intron loss is accelerated after gene fragment duplication (Lin et al., 2006). Intron-less and intron-poor genes were also shown to have evolved more recently and to be more functionally constrained than intron-rich genes (Liu et al., 2021). Therefore, it has been hypothesized that FcERFs and FcRAVs, which have fewer introns, evolved later than the AP2 subfamily, and may in fact have been derived from the AP2 subfamily to perform additional biological functions. This hypothesis was supported by the low expression levels of most FcAP2 genes observed in this study (Figure 5 and Supplementary Figure 4).
Repressors in the AP2/ERF family
Analysis of two transcriptomic datasets related to fruit ripening showed that there were more downregulated than upregulated FcAP2/ERFs during fruit ripening (Figures 6 and 7), suggesting that those genes may serve as repressors. The EAR motif is the most prevalent repression motif that has been identified in plants (Kagale and Rozwadowski, 2011). Gene structure analysis showed that FCD_00019611 and FCD_00012531, which were downregulated during fruit ripening (Figure 6), contained EAR motifs (Supplementary Figure 1). They were homologs of the Arabidopsis repressor genes AtERF3 and AtERF4 (Supplementary Figure 1), and therefore likely play transcriptional inhibitory roles in fig fruit development.
Interaction network analyses of AtERF3 and AtERF4 provided information about the possible functions of their fig homologs. AtERF3 and AtERF4 were experimentally proven to interact with SAP18. In addition, AtERF3, HD1, and SAP18 can interact with each other (Supplementary Figure 6). In yeast and mammalian systems, transcriptional downregulation involves core histone deacetylation, which results in compact nucleosome structure and thus suppresses gene expression. This process is mediated by a complex containing HDA1, SAP18, SAP30, and other proteins (Knoepfler and Eisenman, 1999). AtSAP18 has been shown to act as a linker, connecting the HDA complex to transcriptional repressors that are bound to chromatin in a sequence-specific manner, leading to transcriptional repression (Song and Galbraith, 2006). We therefore hypothesize that FcERFs with the EAR motif mediates transcriptional repression, possibly via histone deacetylation, in fig fruits.
Plant hormones and AP2/ERFs
Plant hormones are well-known regulators of fruit ripening. In this study, many hormone-related elements were identified in the promoters of FcAP2/ERF genes (Figure 6). Our previous studies showed that plant hormones interfered with the expression of FcAP2/ERFs in fig fruits. After gibberellin treatment, members of the FcAP2/ERF family showed differing expression patterns (Chai et al., 2018). After cytokinin treatment, most AP2/ERFs were downregulated in fig flesh but upregulated in the receptacles (Chai et al., 2019). After ethephon treatment, most AP2/ERFs were downregulated in both flesh and receptacles (Cui et al., 2021). Moreover, ABA, auxin, MeJA, and brassinolide (BR) also mediate changes in plant growth and development through AP2/ERFs (Hu et al., 2004; Zhu et al., 2010; Gao et al., 2019; Lin et al., 2020). In turn, AP2/ERFs affect plant hormone synthesis. The most well characterized of these processes are AP2/ERF mediation of ethylene and ABA synthesis (Zhang et al., 2013; Sun et al., 2021), but jasmonate and auxin can also be regulated by AP2/ERFs (Blencowe et al., 2006; Tan et al., 2018; Xie et al., 2019). The involvement of AP2/ERFs in hormone signaling and synthesis adds to the complexity of the known plant hormone regulatory network. This elaborate regulatory mechanism improves the adaptability of plants to the environment and necessitates further exploration of the functions of important FcAP2/ERFs.
AP2/ERFs are associated with fig fruit ripening
Figs undergo a very rapid ripening process, during which a synchronous peak in respiration rate and ethylene release has been reported (Marei and Crane, 1971). Ethylene has long been used in horticultural crop production to promote fruit ripening and to improve quality attributes such as pigmentation. In fig, ethylene treatment at stage II stimulates fruit growth and ripening; ethylene-treated figs ripen between four and 11 days earlier than untreated controls (Marei and Crane, 1971; Cui et al., 2021). Ethylene treatment promotes the synthesis of ribosomes, ribonucleic acids, and proteins in fig fruits (Marei and Crane, 1971) and induces upregulation of genes that are involved in biosynthesis of and responses to ethylene (Lama et al., 2018). By joint screening of three transcriptomic datasets derived from developing and ripening fig fruits, nine candidate FcAP2/ERF genes were identified based on expression levels and patterns consistent with fruit ripening.
The poor storability and short shelf lives of fig fruits are the main limitations in the development of the fresh fig industry. Rapid decreases in fruit hardness and loss of texture in the postharvest stage are tightly connected with the superfast ripening process. ERF subfamily genes have been shown to regulate fruit softening by changing the expression of cell wall-related genes, such as ERF.B3 of Solanum lycopersicum, ERF9 of Actinidia chinensis, ERF11 of Musa nana, ERF9 of Chaenomeles sinensis, ERF8/16/19 of Diospyros kaki, and ERF2 of Amygdalus persica (Gao et al., 2020). In previous studies, we identified genes associated with fig fruit softening, including polygalacturonase, pectinesterase inhibitor, pectate lyase, and expansin (Cui et al., 2019; Cui et al., 2021). AP2/ERF-binding motifs were found to be abundant in the fig pectate lyase promoter (Supplementary Figure 7), further in-depth studies are being conducted at present. With the rapid development and adoption of gene editing technologies in crop sciences, precise control of fruit softening without alteration of other important fruit quality attributes could be achieved by manipulating the expression of genes that specifically affect fruit softening. Understanding the functions of key AP2/ERFs in superfast fig fruit ripening will allow for selection of appropriate genes to achieve this goal.
Conclusion
A total of 119 AP2/ERF genes were identified in the F. carica genome, namely 95 ERFs, 20 AP2s, three RAVs and one soloist. The evolutionary and expression pattern analyses conducted here provide valuable information about the evolution, characteristics, and fruit ripening-related functions of FcAP2/ERF genes. Multi-omics data allowed for screening of potential key FcAP2/ERF genes involved in fruit ripening. The results of this study provide valuable findings for further investigation and contribute to understanding of the roles of AP2/ERF genes in fig fruit development.
Data availability statement
Publicly available datasets were analyzed in this study. This data can be found here: The RNA-Seq data used in this study derived from NCBI (SRA accession: PRJNA723733 [six stages], PRJNA606407 [ethephon treatment], and PRJNA322124 [pollinated and parthenocarpic]).
Author contributions
YC, YZ, JH, and MS prepared the data. YC and YZ completed the analysis and conducted the experiments. YC, YZ, JH, MS, MF, and HM prepared the manuscript. All authors have read and approved the manuscript for publication.
Funding
This work was supported by the key research project for fig development of Weiyuan County and National Natural Science Foundation of China project NSFC [31372007].
Conflict of interest
The authors declare that the research was conducted in the absence of any commercial or financial relationships that could be construed as a potential conflict of interest.
Publisher’s note
All claims expressed in this article are solely those of the authors and do not necessarily represent those of their affiliated organizations, or those of the publisher, the editors and the reviewers. Any product that may be evaluated in this article, or claim that may be made by its manufacturer, is not guaranteed or endorsed by the publisher.
Supplementary material
The Supplementary Material for this article can be found online at: https://www.frontiersin.org/articles/10.3389/fpls.2022.1040796/full#supplementary-material
Supplementary Table 1 | Amino acid (aa) length, molecular weight (MW), and isoelectric point (pI) values for the proteins encoded by the 119 FcAP2/ERF genes.
Supplementary Table 2 | The FcAP2/ERF genes that failed to align to any of the chromosomes.
Supplementary Table 3 | Detailed information about intergenomic collinearity in the fig genome.
Supplementary Table 4 | Divergence between paralogous FcAP2/ERF gene pairs in F. carica. S, segmental duplication; T, tandem duplication. Members of the AP2 family are shown in bold.
Supplementary Table 5 | Synteny analysis of AP2/ERF gene pairs in F. carica and five other plant species.
Supplementary Table 6 | FcAP2/ERF genes with a greater than five-fold change in the sum of expression values between the flesh and peel.
Supplementary Table 7 | Primers used for quantitative reverse transcription (qRT)-PCR.
Supplementary Table 8 | Detailed information about differentially expressed FcAP2/ERF genes in fig flesh and receptacle tissues in response to ethephon treatment.
Supplementary Table 9 | Interactions between nine key FcAP2/ERF homologs in A. thaliana.
Supplementary Figure 1 | EDLL activation domains, EAR repression domains, and R/KLFGV domains in FcAP2/ERFs.
Supplementary Figure 2 | Chromosomal distribution of FcAP2/ERFs. Red lines indicate AP2/ERF gene pairs derived from tandem duplication. The number after each chromosome name indicates the number of FcAP2/ERF genes mapped to that chromosome.
Supplementary Figure 3 | Veen analysis of syntenic AP2/ERFs between F. carica and five other plant species. (A) FcAP2/ERF genes that showed syntenic relationships with all five plant species. (B) FcAP2/ERF genes that showed syntenic relationships with the other two Ficus species, F. hispida and F. macrocarpa.
Supplementary Figure 4 | Expression patterns of lowly-expressed (group C) genes at six fruit developmental stages.
Supplementary Figure 5 | Quantitative reverse transcription (qRT)-PCR validation of RNA-Seq results at six fruit developmental stages.
Supplementary Figure 6 | Interaction networks of FCD_00019611 and FCD_00012531 based on those of A. thaliana homologs.
Supplementary Figure 7 | AP2/ERF binding element in the promoter of pectate lyase.
References
Allen, M. D., Yamasaki, K., Ohme-Takagi, M., Tateno, M., Suzuki, M. (1998). A novel mode of DNA recognition by a β-sheet revealed by the solution structure of the GCC-box binding domain in complex with DNA. EMBO J. 17 (18), 5484–5496. doi: 10.1093/emboj/17.18.5484
An, J. P., Zhang, X. W., Bi, S. Q., You, C. X., Wang, X. F., Hao, Y. J. (2020). The ERF transcription factor MdERF38 promotes drought stress-induced anthocyanin biosynthesis in apple. Plant J. 101 (3), 573–589. doi: 10.1111/tpj.14555
Birchler, J. A., Yang, H. (2022). The multiple fates of gene duplications: deletion, hypofunctionalization, subfunctionalization, neofunctionalization, dosage balance constraints, and neutral variation. Plant Cell. 34 (7), 2466–2474. doi: 10.1093/plcell/koac076
Blencowe, B. J. (2006). Alternative splicing: new insights from global analyses. Cell. 126 (1), 37–47. doi: 10.1016/j.cell.2006.06.023
Cao, L., Xu, X., Chen, S., Ma, H. (2016). Cloning and expression analysis of Ficus carica anthocyanidin synthase 1 gene. Sci. Hortic. 211, 369–375. doi: 10.1016/j.scienta.2016.09.015
Chai, L., Chai, P., Chen, S., Flaishman, M. A., Ma, H. (2018). Transcriptome analysis unravels spatiotemporal modulation of phytohormone-pathway expression underlying gibberellin-induced parthenocarpic fruit set in San Pedro-type fig (Ficus carica l.). BMC Plant Biol. 18 (1), 100. doi: 10.1186/s12870-018-1318-1
Chai, P., Dong, S., Chai, L., Chen, S., Flaishman, M., Ma, H. (2019). Cytokinin-induced parthenocarpy of San Pedro type fig (Ficus carica l.) main crop: explained by phytohormone assay and transcriptomic network comparison. Plant Mol. Biol. 99 (4-5), 329–346. doi: 10.1007/s11103-019-00820-2
Chai, L., Wang, Z., Chai, P., Chen, S., Ma, H. (2017). Transcriptome analysis of San Pedro-type fig (Ficus carica l.) parthenocarpic breba and non-parthenocarpic main crop reveals divergent phytohormone-related gene expression. Tree Genet. Genomes. 13 (4), 83. doi: 10.1007/s11295-017-1166-4
Chen, C., Chen, H., Zhang, Y., Thomas, H. R., Frank, M. H., He, Y., et al. (2020). TBtools: an integrative toolkit developed for interactive analyses of big biological data. Mol. Plant 13 (8), 1194–1202. doi: 10.1016/j.molp.2020.06.009
Chong, G. L., Foo, M. H., Lin, W. D., Wong, M. M., Verslues, P. E. (2019). Highly ABA-induced 1 (HAI1)-interacting protein HIN1 and drought acclimation-enhanced splicing efficiency at intron retention sites. Proc. Natl. Acad. Sci. U S A. 116 (44), 22376–22385. doi: 10.1073/pnas.1906244116
Cui, Y., Wang, Z., Chen, S., Vainstein, A., Ma, H. (2019). Proteome and transcriptome analyses reveal key molecular differences between quality parameters of commercial-ripe and tree-ripe fig (Ficus carica l.). BMC Plant Biol. 19 (1), 1–16. doi: 10.1186/s12870-019-1742-x
Cui, Y., Zhai, Y., Flaishman, M., Li, J., Chen, S., Zheng, C., et al. (2021). Ethephon induces coordinated ripening acceleration and divergent coloration responses in fig (Ficus carica l.) flesh and receptacles. Plant Mol. Biol. 105 (4), 347–364. doi: 10.1007/s11103-020-01092-x
Fan, Z. Q., Kuang, J. F., Fu, C. C., Shan, W., Han, Y. C., Xiao, Y. Y., et al. (2016). The banana transcriptional repressor MaDEAR1 negatively regulates cell wall-modifying genes involved in fruit ripening. Front. Plant Sci. 7. doi: 10.3389/fpls.2016.01021
Feng, B. H., Han, Y. C., Xiao, Y. Y., Kuang, J. F., Fan, Z. Q., Chen, J. Y., et al. (2016). The banana fruit dof transcription factor MaDof23 acts as a repressor and interacts with MaERF9 in regulating ripening-related genes. J. Exp. Bot. 67 (8), 2263–2275. doi: 10.1093/jxb/erw032
Feng, K., Hou, X. L., Xing, G. M., Liu, J. X., Duan, A. Q., Xu, Z. S., et al. (2020). Advances in AP2/ERF super-family transcription factors in plant. Crit. Rev. Biotechnol. 40 (6), 750–776. doi: 10.1080/07388551.2020.1768509
Finn, R. D., Clements, J., Eddy, S. R. (2011). HMMER web server: interactive sequence similarity searching. Nucleic Acids Res. 39, W29–W37. doi: 10.1093/nar/gkr367
Flaishman, M. A., Rodov, V., Stover, E. (2008). The fig: botany, horticulture, and breeding. Hortic. Rev. 34 (0), 113–196. doi: 10.1002/9780470380147.ch2
Franco-Zorrilla, J. M., López-Vidriero, I., Carrasco, J. L., Godoy, M., Vera, P., Solano, R. (2014). DNA-Binding specificities of plant transcription factors and their potential to define target genes. Proc. Natl. Acad. Sci. U S A. 111 (6), 2367–2372. doi: 10.1073/pnas.1316278111
Freiman, Z. E., Rosianskey, Y., Dasmohapatra, R., Kamara, I., Flaishman, M. A. (2015). The ambiguous ripening nature of the fig (Ficus carica l.) fruit: a gene-expression study of potential ripening regulators and ethylene-related genes. J. Exp. Bot. 66 (11), 3309–3324. doi: 10.1093/jxb/erv140
Fu, C. C., Han, Y. C., Qi, X. Y., Shan, W., Chen, J. Y., Lu, W. J., et al. (2016). Papaya CpERF9 acts as a transcriptional repressor of cell-wall-modifying genes CpPME1/2 and CpPG5 involved in fruit ripening. Plant Cell Rep. 35 (11), 2341–2352. doi: 10.1007/s00299-016-2038-3
Gao, Y., Liu, Y., Liang, Y., Lu, J., Jiang, C., Fei, Z., et al. (2019). Rosa Hybrida RhERF1 and RhERF4 mediate ethylene- and auxin-regulated petal abscission by influencing pectin degradation. Plant J. 99 (6), 1159–1171. doi: 10.1111/tpj.14412
Gao, J., Zhang, Y., Li, Z., Liu, M. (2020). Role of ethylene response factors (ERFs) in fruit ripening. Food Qual. Safety. 4 (1), 15–20. doi: 10.1093/fqsafe/fyz042
Han, Z., Hu, Y., Lv, Y., Rose, J. K., Sun, Y., Shen, F., et al. (2018). Natural variation underlies differences in ETHYLENE RESPONSE FACTOR17 activity in fruit peel degreening. Plant Physiol. 176 (3), 2292–2304. doi: 10.1104/pp.17.01320
Han, Y. C., Kuang, J. F., Chen, J. Y., Liu, X. C., Xiao, Y. Y., Fu, C. C., et al. (2016). Banana transcription factor MaERF11 recruits histone deacetylase MaHDA1 and represses the expression of MaACO1 and expansins during fruit ripening. Plant Physiol. 171 (2), 1070–1084. doi: 10.1104/pp.16.00301
Hao, P. P., Wang, G. M., Cheng, H. Y., Ke, Y. Q., Qi, K. J., Gu, C., et al. (2018). Transcriptome analysis unravels an ethylene response factor involved in regulating fruit ripening in pear. Physiol. Plant 163, 124–135. doi: 10.1111/ppl.12671
Hiratsu, K., Matsui, K., Koyama, T., Ohme-Takagi, M. (2003). Dominant repression of target genes by chimeric repressors that include the EAR motif, a repression domain, in arabidopsis. Plant J. 34 (5), 733–739. doi: 10.1046/j.1365-313x.2003.01759.x
Hu, Y. X., Wang, Y. X., Liu, X. F., Li, J. Y. (2004). Arabidopsis RAV1 is down-regulated by brassinosteroid and may act as a negative regulator during plant development. Cell Res. 14 (1), 8–15. doi: 10.1038/sj.cr.7290197
Ikeda, M., Ohme-Takagi, M. (2009). A novel group of transcriptional repressors in arabidopsis. Plant Cell Physiol. 50 (5), 970–975. doi: 10.1093/pcp/pcp048
Kagale, S., Rozwadowski, K. (2011). EAR motif-mediated transcriptional repression in plants: an underlying mechanism for epigenetic regulation of gene expression. Epigenetics. 6 (2), 141–146. doi: 10.4161/epi.6.2.13627
Knoepfler, P. S., Eisenman, R. N. (1999). Sin meets NuRD and other tails of repression. Cell. 99 (5), 447–450. doi: 10.1016/s0092-8674(00)81531-7
Kohl, M., Wiese, S., Warscheid, B. (2011). Cytoscape: software for visualization and analysis of biological networks. Methods Mol. Biol. 696, 291–303. doi: 10.1007/978-1-60761-987-1_18
Kuang, L., Chen, S., Guo, Y., Scheuring, D., Flaishman, M. A., Ma, H. (2022). Proteome analysis of vacuoles isolated from fig (Ficus carica l.) flesh during fruit development. Plant Cell Physiol. 63 (6), 785–801. doi: 10.1093/pcp/pcac039
Lama, K., Yadav, S., Rosianski, Y., Shaya, F., Lichter, A., Chai, L., et al. (2019). The distinct ripening processes in the reproductive and non-reproductive parts of the fig syconium are driven by ABA. J. Exp. Bot. 70 (1), 115–131. doi: 10.1093/jxb/ery333
Licausi, F., Ohme-Takagi, M., Perata, P. (2013). APETALA2/Ethylene responsive factor (AP2/ERF) transcription factors: mediators of stress responses and developmental programs. New Phytol. 199 (3), 639–649. doi: 10.1111/nph.12291
Li, T., Jiang, Z., Zhang, L., Tan, D., Wei, Y., Yuan, H., et al. (2016). Apple (Malus domestica) MdERF2 negatively affects ethylene biosynthesis during fruit ripening by suppressing MdACS1 transcription. Plant J. 88, 735–748. doi: 10.1111/tpj.13289
Lin, T., Du, J., Zheng, X., Zhou, P., Li, P., Lu, X. (2020). Comparative transcriptome analysis of MeJA-responsive AP2/ERF transcription factors involved in notoginsenosides biosynthesis. 3 Biotech. 10 (7), 290. doi: 10.1007/s13205-020-02246-w
Lin, H., Zhu, W., Silva, J. C., Gu, X., Buell, C. R. (2006). Intron gain and loss in segmentally duplicated genes in rice. Genome Biol. 7 (5), 1–11. doi: 10.1186/gb-2006-7-5-r41
Liu, H., Lyu, H. M., Zhu, K., Van de Peer, Y., Max Cheng, Z. M. (2021). The emergence and evolution of intron-poor and intronless genes in intron-rich plant gene families. Plant J. 105 (4), 1072–1082. doi: 10.1111/tpj.15088
Liu, W., Stewart, C. N., Jr (2016). Plant synthetic promoters and transcription factors. Curr. Opin. Biotechnol. 37, 36–44. doi: 10.1016/j.copbio.2015.10.001
Livak, K. J., Schmittgen, T. D. (2001). Analysis of relative gene expression data using real-time quantitative PCR and the 2–ΔΔCT method. Methods. 25 (4), 402–408. doi: 10.1006/meth.2001.1262
Lynch, M., Conery, J. S. (2000). The evolutionary fate and consequences of duplicate genes. Sci. (New York N.Y.). 290 (5494), 1151–1155. doi: 10.1126/science.290.5494.1151
Magnani, E., Sjölander, K., Hake, S. (2004). From endonucleases to transcription factors: evolution of the AP2 DNA binding domain in plants. Plant Cell. 16 (9), 2265–2277. doi: 10.1105/tpc.104.023135
Marei, N., Crane, J. C. (1971). Growth and respiratory response of fig (Ficus carica l. cv. mission) fruits to ethylene. Plant Physiol. 48 (3), 249–254. doi: 10.1104/pp.48.3.249
Nakano, T., Suzuki, K., Fujimura, T., Shinshi, H. (2006). Genome-wide analysis of the ERF gene family in arabidopsis and rice. Plant Physiol. 140 (2), 411–432. doi: 10.1104/pp.105.073783
Ni, J., Bai, S., Zhao, Y., Qian, M., Tao, R., Yin, L., et al. (2019). Ethylene response factors Pp4ERF24 and Pp12ERF96 regulate blue light-induced anthocyanin biosynthesis in ‘Red zaosu’ pear fruits by interacting with MYB114. Plant Mol. Biol. 99 (1-2), 67–78. doi: 10.1007/s11103-018-0802-1
Ohta, M., Matsui, K., Hiratsu, K., Shinshi, H., Ohme-Takagi, M. (2001). Repression domains of class II ERF transcriptional repressors share an essential motif for active repression. Plant Cell. 13 (8), 1959–1968. doi: 10.1105/tpc.010127
Ohta, M., Ohme-Takagi, M., Shinshi, H. (2000). Three ethylene-responsive transcription factors in tobacco with distinct transactivation functions. Plant J. 22 (1), 29–38. doi: 10.1046/j.1365-313x.2000.00709.x
Rosianski, Y., Doron-Faigenboim, A., Freiman, Z. E., Lama, K., Milo-Cochavi, S., Dahan, Y., et al. (2016a). Tissue-specific transcriptome and hormonal regulation of pollinated and parthenocarpic fig (Ficus carica l.) fruit suggest that fruit ripening is coordinated by the reproductive part of the syconium. Front. Plant Sci. 7. doi: 10.3389/fpls.2016.01696
Rosianski, Y., Freiman, Z. E., Cochavi, S. M., Yablovitz, Z., Kerem, Z., Flaishman, M. A. (2016b). Advanced analysis of developmental and ripening characteristics of pollinated common-type fig (Ficus carica l.). Sci. Hortic. 198, 98–106. doi: 10.1016/j.scienta.2015.11.027
Sakuma, Y., Liu, Q., Dubouzet, J. G., Abe, H., Shinozaki, K., Yamaguchi-Shinozaki, K. (2002). DNA- binding specificity of the ERF/AP2 domain of arabidopsis DREBs, transcription factors involved in dehydration-and cold-inducible gene expression. Biochem. Biophys. Res. Commun. 290 (3), 998–1009. doi: 10.1006/bbrc.2001.6299
Seok, H. Y., Tarte, V. N., Lee, S. Y., Park, H. Y., Moon, Y. H. (2014). Arabidopsis HRE1α, a splicing variant of AtERF73/HRE1, functions as a nuclear transcription activator in hypoxia response and root development. Plant Cell Rep. 33 (8), 1255–1262. doi: 10.1007/s00299-014-1613-8
Shang, Y., Yang, D., Ha, Y., Hur, Y. S., Lee, M. M., Nam, K. H. (2022). Brassinosteroid-insensitive 1-associated receptor kinase 1 modulates abscisic acid signaling by inducing PYR1 monomerization and association with ABI1 in arabidopsis. Front. Plant Sci. 13. doi: 10.3389/fpls.2022.849467
Solomon, A., Golubowicz, S., Yablowicz, Z., Grossman, S., Bergman, M., Gottlieb, H. E., et al. (2006). Antioxidant activities and anthocyanin content of fresh fruits of common fig (Ficus carica l.). J. Agric. Food Chem. 54 (20), 7717–7723. doi: 10.1021/jf060497h
Song, C. P., Galbraith, D. W. (2006). AtSAP18, an orthologue of human SAP18, is involved in the regulation of salt stress and mediates transcriptional repression in arabidopsis. Plant Mol. Biol. 60 (2), 241–257. doi: 10.1007/s11103-005-3880-9
Sun, X., Wen, C., Xu, J., Wang, Y., Zhu, J., Zhang, Y. (2021). The apple columnar gene candidate MdCoL and the AP2/ERF factor MdDREB2 positively regulate ABA biosynthesis by activating the expression of MdNCED6/9. Tree Physiol. 41 (6), 1065–1076. doi: 10.1093/treephys/tpaa162
Tan, X. L., Fan, Z. Q., Shan, W., Yin, X. R., Kuang, J. F., Lu, W. J., et al. (2018). Association of BrERF72 with methyl jasmonate-induced leaf senescence of Chinese flowering cabbage through activating JA biosynthesis-related genes. Hortic. Res. 5, 22. doi: 10.1038/s41438-018-0028-z
Thompson, J. D., Gibson, T. J., Higgins, D. G. (2003). Multiple sequence alignment using ClustalW and ClustalX. Curr. Protoc. Bioinf. 1), 2–3. doi: 10.1002/0471250953.bi0203s00
Tiwari, S. B., Belachew, A., Ma, S. F., Young, M., Ade, J., Shen, Y., et al. (2012). The EDLL motif: a potent plant transcriptional activation domain from AP2/ERF transcription factors. Plant J. 70 (5), 855–865. doi: 10.1111/j.1365-313X.2012.04935.x
Usai, G., Mascagni, F., Giordani, T., Vangelisti, A., Bosi, E., Zuccolo, A., et al. (2020). Epigenetic patterns within the haplotype phased fig (Ficus carica l.) genome. Plant J. 102 (3), 600–614. doi: 10.1111/tpj.14635
Wang, Y., Tang, H., Debarry, J. D., Tan, X., Li, J., Wang, X., et al. (2012). MCScanX: a toolkit for detection and evolutionary analysis of gene synteny and collinearity. Nucleic Acids Res. 40 (7), e49. doi: 10.1093/nar/gkr1293
Wang, X., Zeng, W., Ding, Y., Wang, Y., Niu, L., Yao, J. L., et al. (2019). Peach ethylene response factor PpeERF2 represses the expression of ABA biosynthesis and cell wall degradation genes during fruit ripening. Plant Sci. 283, 116–126. doi: 10.1016/j.plantsci.2019.02.009
Wang, D., Zhang, Y., Zhang, Z., Zhu, J., Yu, J. (2010). KaKs_Calculator 2.0: a toolkit incorporating gamma-series methods and sliding window strategies. Genomics Proteomics Bioinf. 8 (1), 77–80. doi: 10.1016/S1672-0229(10)60008-3
Wang, M. M., Zhu, Q. G., Deng, C. L., Luo, Z. R., Sun, N. J., Grierson, D., et al. (2017). Hypoxia-responsive ERFs involved in postdeastringency softening of persimmon fruit. Plant Biotechnol. J. 15 (11), 1409–1419. doi: 10.1111/pbi.12725
Xiao, Y. Y., Chen, J. Y., Kuang, J. F., Shan, W., Xie, H., Jiang, Y. M., et al. (2013). Banana ethylene response factors are involved in fruit ripening through their interactions with ethylene biosynthesis genes. J. Exp. Bot. 64 (8), 2499–2510. doi: 10.1093/jxb/ert108
Xie, Z., Nolan, T. M., Jiang, H., Yin, Y. (2019). AP2/ERF transcription factor regulatory networks in hormone and abiotic stress responses in arabidopsis. Front. Plant Sci. 10. doi: 10.3389/fpls.2019.00228
Xu, F., Jia, M., Li, X., Tang, Y., Jiang, K., Bao, J., et al. (2021). Exportin-4 coordinates nuclear shuttling of TOPLESS family transcription corepressors to regulate plant immunity. Plant Cell. 33 (3), 697–713. doi: 10.1093/plcell/koaa047
Yao, G., Ming, M., Allan, A. C., Gu, C., Li, L., Wu, X., et al. (2017). Map-based cloning of the pear gene MYB114 identifies an interaction with other transcription factors to coordinately regulate fruit anthocyanin biosynthesis. Plant J. 92 (3), 437–451. doi: 10.1111/tpj.13666
Yin, X. R., Allan, A. C., Chen, K. S., Ferguson, I. B. (2010). Kiwifruit EIL and ERF genes involved in regulating fruit ripening. Plant Physiol. 153 (3), 1280–1292. doi: 10.1104/pp.110.157081
Yin, X. R., Xie, X. L., Xia, X. J., Yu, J. Q., Ferguson, I. B., Giovannoni, J. J., et al. (2016). Involvement of an ethylene response factor in chlorophyll degradation during citrus fruit degreening. Plant J. 86 (5), 403–412. doi: 10.1111/tpj.13178
Zhai, Y., Cui, Y., Song, M., Vainstein, A., Chen, S., Ma, H. (2021). Papain-like cysteine protease gene family in fig (Ficus carica l.): Genome-wide analysis and expression patterns. Front. Plant Sci. 12. doi: 10.3389/fpls.2021.681801
Zhai, Y., Fan, Z., Cui, Y., Gu, X., Chen, S., Ma, H. (2022). AP2/ERF in fruit ripening: Roles, interactions and expression regulation. Front. Plant Sci. 13. doi: 10.3389/fpls.2022.979348
Zhang, X., Wang, G., Zhang, S., Chen, S., Wang, Y., Wen, P., et al. (2020). Genomes of the banyan tree and pollinator wasp provide insights into fig-wasp coevolution. Cell. 183 (4), 875–889. e17. doi: 10.1016/j.cell.2020.09.043
Zhang, Y., Yin, X., Xiao, Y., Zhang, Z., Li, S., Liu, X., et al. (2018). An ETHYLENE RESPONSE FACTOR-MYB transcription complex regulates furaneol biosynthesis by activating QUINONE OXIDOREDUCTASE expression in strawberry. Plant Physiol. 178 (1), 189–201. doi: 10.1104/pp.18.00598
Zhang, H., Zhang, J., Quan, R., Pan, X., Wan, L., Huang, R. (2013). EAR motif mutation of rice OsERF3 alters the regulation of ethylene biosynthesis and drought tolerance. Planta. 237 (6), 1443–1451. doi: 10.1007/s00425-013-1852-x
Zhuang, J., Chen, J. M., Yao, Q. H., Xiong, F., Sun, C. C., Zhou, X. R., et al. (2011). Discovery and expression profile analysis of AP2/ERF family genes from triticum aestivum. Mol. Biol. Rep. 38 (2), 745–753. doi: 10.1007/s11033-010-0162-7
Keywords: ethylene response factors, expression pattern, gene structure, genome-wide identification, fruit development, transcriptome
Citation: Cui Y, Zhai Y, He J, Song M, Flaishman MA and Ma H (2022) AP2/ERF genes associated with superfast fig (Ficus carica L.) fruit ripening. Front. Plant Sci. 13:1040796. doi: 10.3389/fpls.2022.1040796
Received: 09 September 2022; Accepted: 19 October 2022;
Published: 31 October 2022.
Edited by:
Shenghui Jiang, Qingdao Agricultural University, ChinaReviewed by:
Mingjun Li, Northwest A&F University, ChinaIrfan Ali Sabir, Shanghai Jiao Tong University, China
Copyright © 2022 Cui, Zhai, He, Song, Flaishman and Ma. This is an open-access article distributed under the terms of the Creative Commons Attribution License (CC BY). The use, distribution or reproduction in other forums is permitted, provided the original author(s) and the copyright owner(s) are credited and that the original publication in this journal is cited, in accordance with accepted academic practice. No use, distribution or reproduction is permitted which does not comply with these terms.
*Correspondence: Huiqin Ma, aHFtYUBjYXUuZWR1LmNu