- 1School of Landscape and Ecological Engineering, Hebei University of Engineering, Handan, China
- 2State Key Laboratory of Crop Stress Biology for Arid Areas, College of Agronomy, Northwest A&F University, Yangling, China
Hexaploid wheat is a major food crop and is sensitive to heat stress. It is necessary to discover genes related to thermotolerance in wheat. Fes1s is a class of nucleotide exchange factor of heat shock protein 70s, proven to be participated in heat response in human, yeast, and Arabidopsis. However, little is known about Fes1s in hexaploid wheat. In this study, we identified nine Fes1s in hexaploid wheat (TaFes1s) and found that they present as three triads. A phylogenetic relationship analysis revealed that these Fes1s grouped into Fes1A, Fes1B and Fes1C subclades, and Fes1As and Fes1Bs were divergent in monocots, but possibly not in dicots. The sequences, gene structures and protein motifs of TaFes1s homoeologues within a triad were highly conserved. Through cis-elements analysis including heat shock elements, and miRNA targets prediction, we found that regulation of three TaFes1s homoeologues may be different, while the expression patterns of three homoeologues were similar. The expression levels of TaFes1As were higher than those of TaFes1Bs and TaFes1Cs, and based on these expressions, TaFes1As were chosen for functional characterization. Intriguingly, neither TaFes1A-5A nor TaFes1A-5D could not rescue the thermotolerance defect of Arabidopsis fes1a mutants at seedling stage, but in the transgenic plants seed germination was accelerated under normal and heat stress condition. The functional characterization indicated that roles of Fes1As would be different in Arabidopsis and hexaploid wheat, and function retention of TaFes1As may occur during wheat evolution. In conclusion, our study comprehensively characterized the distribution and expression of Fes1s in hexaploid wheat and found that two TaFes1As could accelerate seed germination under normal and heat stress condition.
Introduction
Hexaploid wheat (Triticum aestivum L.) is a staple crop that accounts for about 28% of global cereal production, and it is vulnerable to heat stress (Tack et al., 2015; Yadav et al., 2022). Heat stress significantly impedes the production and quality of wheat, and when above the optimum temperature, each increase of a single degree could reduce wheat yield by 5-10% (Liu et al., 2014; Lesk et al., 2016; Zhao et al., 2017). With global warming, the global mean temperature is predicted to increase 1.5°C in the next two decades, and together with more frequent extreme and short-term high temperature, heat stress will become a major abiotic factor that threatens wheat production (Liu et al., 2014; Tack et al., 2015; Zhao et al., 2017; Yadav et al., 2022).
As a sessile organism, wheat is vulnerable to numerous unfavorable environmental conditions, such as heat stress, thus, wheat has evolved complicated adaptive mechanisms at molecular level to cope with heat stress. Under heat stress, many proteins become misfolded and aggregate in cells disturbing plant growth and development, hence, the chaperone system involved in protein folding and quality control of unfolded proteins is activated (Wang et al., 2004; Buchberger et al., 2010). In this chaperone system, the heat shock protein 70 (HSP70) system plays a pivotal role, participating in protein synthesis, folding, transportation, translocation, activity regulation, and the prevention of aggregation (Wang et al., 2004; Buchberger et al., 2010; Rosenzweig et al., 2019). Characterization and expression of HSP70s in hexaploid wheat have been reported (Kumar et al., 2020; Lu et al., 2022).
The HSP70 chaperone system includes HSP70, co-chaperone HSP40 (DnaJ), and a nucleotide exchange factor (NEF). Each component has its own role: HSP70 acts as the chaperone interacting with its substrates; HSP40 directly interacts with HSP70 and mediates the substrates binding to HSP70 in synergism with ATP hydrolysis on HSP70, leading to the trapping of the substrate. NEF promotes the dissociation of ADP and the rebinding of ATP on HSP70, consequently resulting in substrate release from HSP70. Thus, binding and releasing of substrates in the HSP70 system are tightly related to the ADP/ATP exchange cycle, in which the dissociation of ADP is a rate-limiting step (Bukau and Horwich, 1998; Mayer and Bukau, 2005; Kampinga and Craig, 2010; Rosenzweig et al., 2019).
In eukaryotic cytosol, three classes of HSP70 NEFs are found, including Armadillo families (HSPBP1 in human, Fes1p in yeast), Bcl2-associated athanogene domain families, and HSP110 (Bracher and Verghese, 2015; Rosenzweig et al., 2019). HSPBP1 is thought to determinate the fate of substrate to degradation or not (Alberti et al., 2004). Fes1p is essential to the degradation of misfolded proteins in yeast (Gowda Naveen Kumar et al., 2013; Gowda et al., 2016). In Arabidopsis, three Fes1p homologues are characterized (AtFes1A, AtFes1B, AtFes1C); AtFes1A localizes in cytosol and interacts with HSP70, and the loss of AtFes1A leads to thermosensitivity of Arabidopsis plants (Zhang et al., 2010). AtFes1A is also required for the function of the Arabidopsis molecular chaperone system (Fu et al., 2015). In rice, three Fes1p homologues are identified (OsFes1A, OsFes1B, OsFes1C); in contrast to OsFes1A and OsFes1B which are located in cytosol and nucleus, OsFes1C is an ER-localized protein, and OsFes1C is involved in ER and salt stress (Qian et al., 2021). Overexpression of Seagrass Fes1 could improve the thermotolerance of transgenic Arabidopsis plants (Chen and Qiu, 2020). However, the distribution and roles of Fes1s in hexaploid wheat are still unknown.
Hexaploid wheat is derived from two major inter-specific hybridizations. The first hybridization occurs between two diploid species, T. urartu, and a possibly extinct Aegilops species closely related to Ae. speltoides. This hybridization forms a tetraploid wheat, wild emmer wheat (T. turgidum ssp. Dicoccoides). A second hybridization between wild emmer wheat and Ae. tauschii finally generates hexaploid wheat. Thus, the hexaploid wheat comprises three subgenomes (AA, BB, and DD), and many genes have copies/pairs distributed in these subgenomes, termed homoeologues (Dubcovsky and Dvorak, 2007; Marcussen et al., 2014; Levy and Feldman, 2022). The homoeologous genes has a single copy on each subgenome are regarded as a triad (Appels et al., 2018).
In this study, we first analyzed the distribution of Fes1s in hexaploid wheat (TaFes1s) and its progenitors. Then, the sequence characteristics and cis-elements of TaFes1s were identified, as well as their expression profiles in hexaploid wheat during growth and under stress condition. Finally, TaFes1A-5A and TaFes1A-5D were transformed into the Arabidopsis thermosensitive fes1a mutant, and we found that overexpression of both genes accelerated the germination of transgenic seeds under normal and heat conditions, rather than inhibiting the thermosensitivity of fes1a mutants. Our results indicate that the roles of Fes1As may be different in hexaploid wheat and Arabidopsis.
Materials and methods
Characterization of Fes1 genes in hexaploid wheat and its progenitors
Genome and protein sequences of hexaploid wheat (T. aestivum), T. urartu, Ae. tauschii, T. turgidum ssp. Dicoccoides were downloaded from Ensemblplants database, sequences of Ae. speltoides were downloaded from the e!DAL databse (Avni et al., 2022). Those sequences comprised the database in blastp program, and Fes1 protein sequences from Arabidopsis and rice were used as a query to search against the database above, with the following criteria: identity >50% and e value <1e-5. Besides, the Hmmsearch engine in the HMMER3.0 program was used to search the same database using the HMM profile of Fes1 domain (PF08609 in the Pfam database) as a query, with the threshold of 1e-5. The results of blastp and Hmmsearch were merged and manually corrected, and then subjected to NCBI CDD database to confirm the presence of the Fes1 domain. Those proteins containing Fes1 domain were characterized as Fes1 proteins.
Phylogenetic analysis of Fes1 proteins
All the Fes1 proteins were aligned in the MAFFT program with the “L-INS-i” algorithm, and then the aligned sequences were submitted to IQ-tree to construct a maximum likelihood tree with 1000 bootstrap replicates with the command “-m MFP -b 1000 -redo -nt AUTO” (Nguyen et al., 2015). A substitution model of JTT+G4 was selected based on the Bayesian information criterion in the ModelFinder (Kalyaanamoorthy et al., 2017). The tree file was visualized via Figtree v1.4.4.
Gene structures, protein motifs, cis-elements, miRNA targets analysis of Fes1s in hexaploid wheat
For convenience, we renamed the Fes1 genes in hexaploid wheat as TaFes1X-YZ, in which Ta means T. aestivum, X represents the phylogenetic clade and YZ refers to the chromosome localization. For example, TaFes1A-5A means this wheat Fes1 gene was grouped in Fes1A clade and localized in chromosome 5A. Gene structures of TaFes1s were obtained from the genome annotation GFF3 files. Protein sequences of TaFes1s were submitted to the MEME to identify a maximum of 12 motifs ranging from 6 to 200 aa, other parameters in MEME were set as default. Finally, the gene structures and protein motifs were illustrated by TBtools (Chen et al., 2020).
The 2-kb genomic sequence from the transcription initiation site of each TaFes1 was extracted and searched for cis-elements in the PlantCARE database (Lescot et al., 2002). Heat shock elements (HSEs) were identified as described (Zhao et al., 2020; Lu et al., 2022). Briefly, the pentanucleotide motifs 5’-NGAAN-3’ and 5’-NTTCN-3’ were regarded as a unit in which N represent any nucleotide, and the unit numbers must be continuous at least three, these HSEs were regarded as typical HSEs; for varied HSEs, only one mismatch was allowed but mismatch was not permitted to be occurred on the “G” of 5-NGAAN-3 (“C” for 5’-NTTCN-3’) in the first and last unit if the unit number was three. To predict the TaFes1s targeted by microRNAs (miRNAs), all the TaFes1s transcripts were subjected to psRNATarget tool (Dai et al., 2018) to searched against the published wheat miRNAs with default parameters.
Expression analysis of TaFes1s
The transcription abundances of TaFes1s in wheat varieties “Azhurnaya” and “Chinese Spring” under normal conditions were obtained from a previous study (Ramírez-González et al., 2018), expression profiles of TaFes1s under heat and drought stress were obtained from other literatures (Liu et al., 2015; Wang et al., 2019; Ma et al., 2021).
Two TaFes1s were selected for qPCR validation (Supplementary Table 1). One week old seedlings of “Chinese Spring” and “TAM107” were subjected to drought stress (mimic by 20% PEG6000), heat stress (40°C), and combined heat and drought stress for 1 and 6 hours as described (Liu et al., 2015). RNA was isolated from leaves and about 1 μg total RNA was reverse-transcribed using the PrimeScript™ RT reagent Kit with gDNA Eraser (Perfect Real Time) (Takara, Dalian, China), and qPCR was performed on a Thermo Fisher Scientific QuantStudio 3 Real-Time PCR System using the TB Green® Premix Ex Taq™ II (Tli RNaseH Plus) kit (Takara, Dalian, China). RNA isolation and qPCR experiments were performed by three independent biological replicates.
Gene cloning, plasmid recombination, plant transformation
The CDSs of TaFes1A-5A and TaFes1A-5D were individually amplified (Supplementary Table 1) and transformed into pBIB-35S-Hygromycin-gene-GFP to construct a recombined vector via Gateway cloning technology, respectively. The recombined vector was confirmed by sequencing. Then the recombined vector was transformed into Agrobacterium GV3101. Finally, the recombined vector was transformed into Arabidopsis fes1a mutant by the floral dipping method.
Plant growth and heat stress treatment
Surface sterilized seeds of three independent T3 homozygous transgenic lines were planted on 1/2 MS media and kept at 4°C for three days. Then the plates were transferred to growth chamber (35/19°C, 16/8h) to evaluate the thermotolerance of seeds at germination stage. The germination rate was calculated as the ratio of germinated seeds to total seeds under both normal and heat stress condition (22/19°C, 16/8h). All plates were photographed every day. All experiments were repeated at least three independent times.
Results
Evolution of Fes1s in several monocots and dicots species
Using the blastp and Hmmsearch engine, three Fes1s were identified in each diploid species (T. urartu, Ae. tauschii, Ae. speltoides), and six and nine Fes1s were characterized in wild emmer wheat and hexaploid wheat, respectively; thus, the copy number of Fes1s in hexaploid wheat and its progenitors was consistent with the ploidy level. Using Arabidopsis and rice Fes1s as marker, the Fes1s in monocots were evenly and clearly classified into Fes1A, Fes1B, and Fes1C (Figure 1). Interestingly, the Arabidopsis Fes1s were all grouped in Clade I in which the Fes1s of monocots were present. To detect the relationship of Fes1s, we characterized the Fes1As in more monocots and dicots species. Based on the phylogenetic tree constructed by maximum likelihood (Supplementary Figure 1), except for the Fes1s in Arabidopsis, Fes1Cs evolved into a separate clade in both monocots and dicots, and Fes1As and Fes1Bs were also divided into different clades in monocots, while Fes1As and Fes1Bs remained in a clade and possibly were not divergent in dicots.
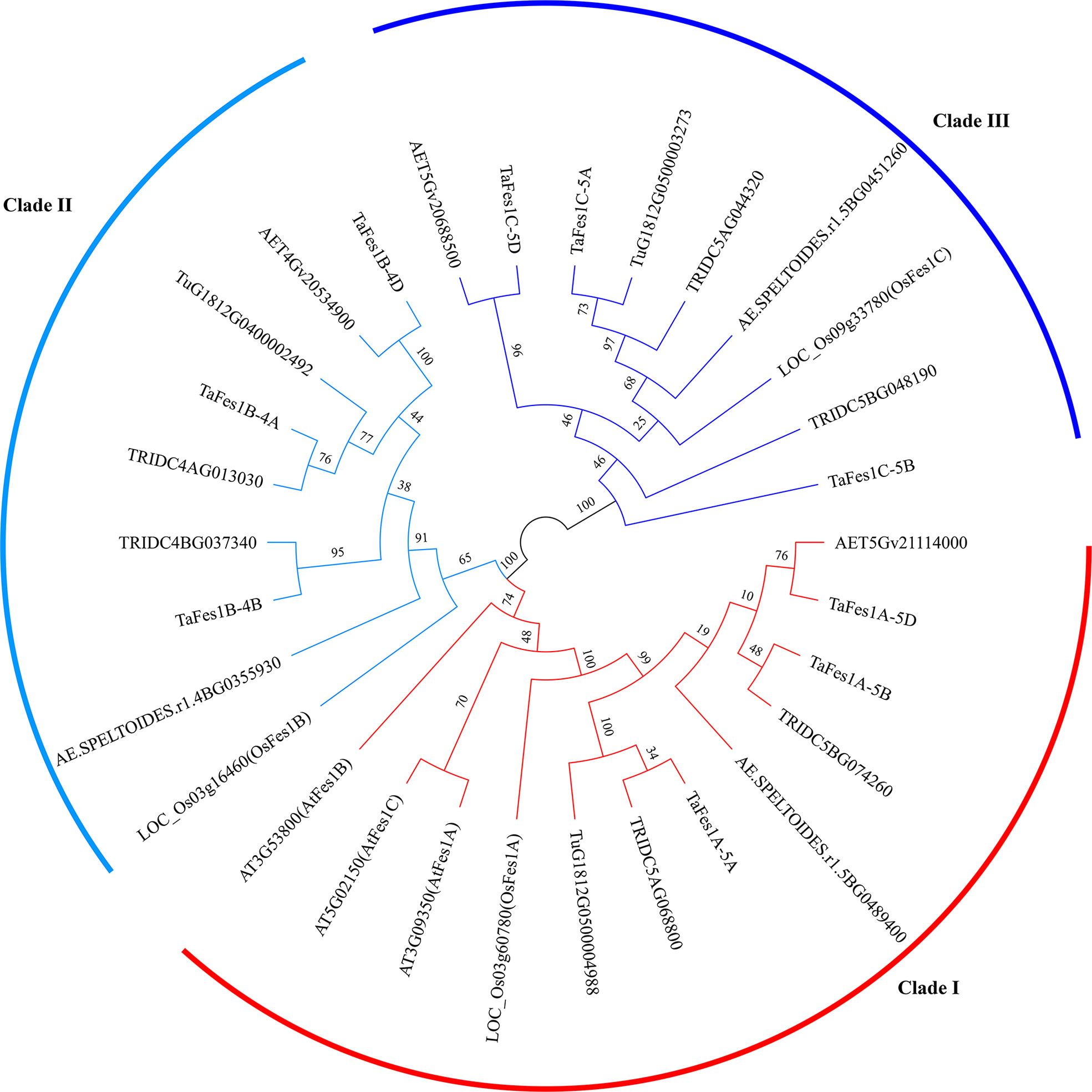
Figure 1 Phylogenetic relationship of Fes1s in hexaploid wheat and its progenitors. The maximum likelihood tree is constructed by IQ-tree software and evaluated by 1000 bootstrap replicates. Numbers near branches represent the percentage of 1000 bootstrap values. Different clades of Fes1s are represent by different colors. Species names are embedded in the Fes1s geneIDs: AET, Ae. tauschii; Tu, T. urartu; AE.SPELTOIDES, Ae. speltoides; TRIDC, T. turgidum ssp. Dicoccoides; Ta, T. aestivum; LOC, Oryza sativa ssp. Japonica; AT, Arabidopsis thaliana.
The Fes1s in hexaploid wheat present as three triads, and only occurred on chromosome group 4 and 5 (Table 1). All TaFes1s on chromosome 5 were distributed in distal telomeric regions (R3), and those on chromosome 4 were found in the interstitial regions (R2a and R2b). The protein length of TaFes1As were the shortest.
Gene structures, protein motifs analysis of TaFes1s
Gene structures and protein motifs could explain the phylogenetic relationship of a gene family to some extent (Zhu et al., 2020). Further analysis found that the gene structures of each TaFes1 homoeologue were conserved in a triad but different among triads (Figure 2A). The intron numbers were five, four, and seven for homoeologues in TaFes1As, TaFes1Bs, and TaFes1Cs, respectively. However, the splice variants were somehow not consistent with the intron numbers. All TaFes1As and TaFes1Cs generated two and one variants. While one homoeologue of TaFes1Bs, TaFes1B-4B, had 4 variants and the other two had 2 variants.
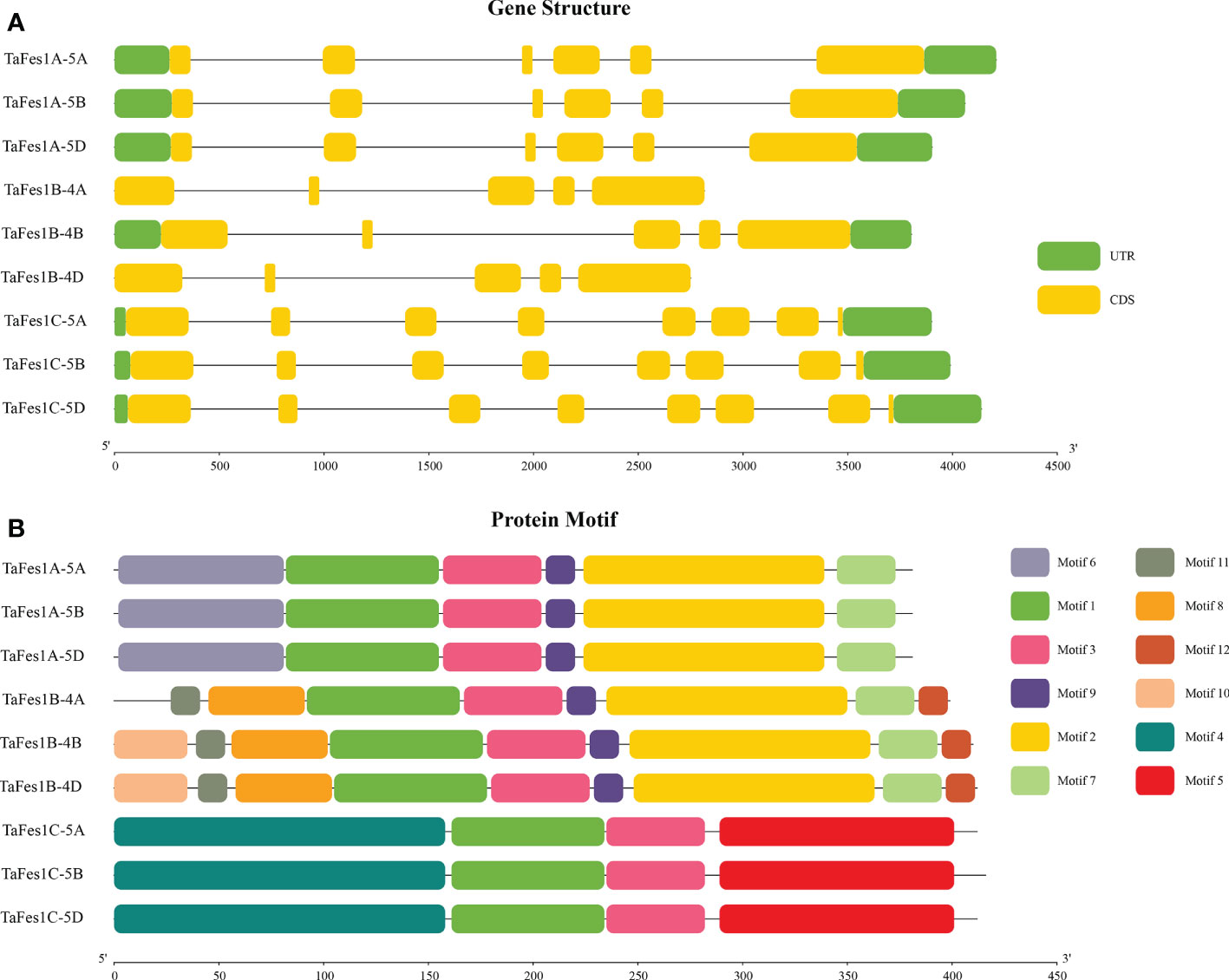
Figure 2 Gene structures and protein motifs of TaFes1s. (A) Gene structures of TaFes1s. Green boxes represent untranslated regions (UTR), yellow boxes refer to coding sequences (CDS), lines mean introns. The scale in bottom indicates gene length in base pairs unit. (B) Protein motifs of TaFes1s. Motifs are identified by MEME. Different motifs are shown in different colors, lines are protein sequences which none conserved motif is characterized. The scale in bottom indicates protein length in amino acid unit.
The protein motifs of each homoeologue were also conserved in a triad but different among triads (Figure 2B, Supplementary Table 2). Six, eight to nine, and four motifs were identified in TaFes1As, TaFes1Bs, and TaFes1Cs, respectively. Among all the twelve identified motifs, only Motifs 1 and 3 were shared by all TaFes1s. Besides, Motifs 9, 2, and 7 were common amongst TaFes1As and TaFes1Bs. This was consistent with the finding in the phylogenetic tree that Fes1As and Fes1Bs were more closely related. In addition, triad-specific motifs were also characterized, and they were Motif 6 in TaFes1As, Motifs 11, 8, 12 and 10 in TaFes1Bs; and Motifs 4 and 5 in TaFes1Cs. The gene structures and protein motif analyses indicate that the function of TaFes1s may be conserved within triads but distinct among triads.
Cis-elements and miRNA targets analysis of TaFes1s
Cis-elements are important regulatory factors participating in the transcriptional regulation of genes during plant growth, development and stress response. The upstream 2-kb sequences of the nine TaFes1s were extracted for cis-elements analysis. At least 57 elements were identified and assigned into three groups: Hormone signaling, Abiotic/Biotic stress, and Others (Table 2). The five most common (total number in all Fes1s) were: TGACG-motif (MeJA responsiveness), CGTCA-motif (MeJA responsiveness), ABRE (Abscisic acid responsiveness), TCA-element (salicylic acid responsiveness), and AuxRR-core (Auxin responsiveness) in “Hormone signaling” group; STRE, G-box, as-1, ARE, and Sp1 in “Abiotic/Biotic stress” group; CAAT-box, TATA-box, MYB, MYC, CCGTCC motif in “Other” groups. Among the 57 elements, only six (CAAT-box, MYB, STRE, as-1, CGTCA-motif, TGACG-motif) were present in all TaFes1s. Intriguingly, the TATA-Box was absent in TaFes1B-4A. In each triad, each homoeologue except TaFes1B-4D contained specific elements (Figure 3), indicating that the expression profiles of TaFes1 homoeologues may be different.
However, none typical HSE elements were identified in all TaFes1s via PlantCARE database. This was somehow confused because that Fes1s were the nucleotide exchange factors of the important heat responsive genes, HSP70s, TaFes1s were possibly heat responsive. Using our previous procedure, we first analyzed HSEs in the upstream 2 kb sequences of TaFes1s. Consistently, none typical HSEs were characterized in all TaFes1s, and four varied HSEs were identified in four TaFes1s (Table 3). Longer promoter sequences were further extracted and analyzed, and this resulted in 13 more HSEs being identified.
MiRNAs are a widespread class of non-coding regulatory endogenous RNA with 20-22 nucleotides in length, and they act as critical posttranscriptional regulators in gene expression, being involved in plant growth and stress response (Khraiwesh et al., 2012; Pagano et al., 2021). The putative regulatory association between TaFes1s and miRNAs was predicted by psRNAtarget tool, and seven TaFes1s were predicted to regulated by eight miRNAs (Figure 4). Only one miRNA silenced TaFes1s via translation inhibition, and others acted through transcript cleavage. Three TaFes1s (TaFes1A-5A, TaFes1B-4A, TaFes1C-5D) were targeted by one miRNA, three TaFes1s (TaFes1A-5D, TaFes1C-5A, TaFes1C-5B) were targeted by two miRNAs, and one TaFes1 (TaFes1A-5A) was targeted by three miRNAs. None of the three TaFes1s homoeologues were targeted by the same miRNA, indicating that the regulation of TaFes1 homoeologues by miRNAs would be different. This result provides useful information for further investigation of posttranscriptional regulation of TaFes1s.
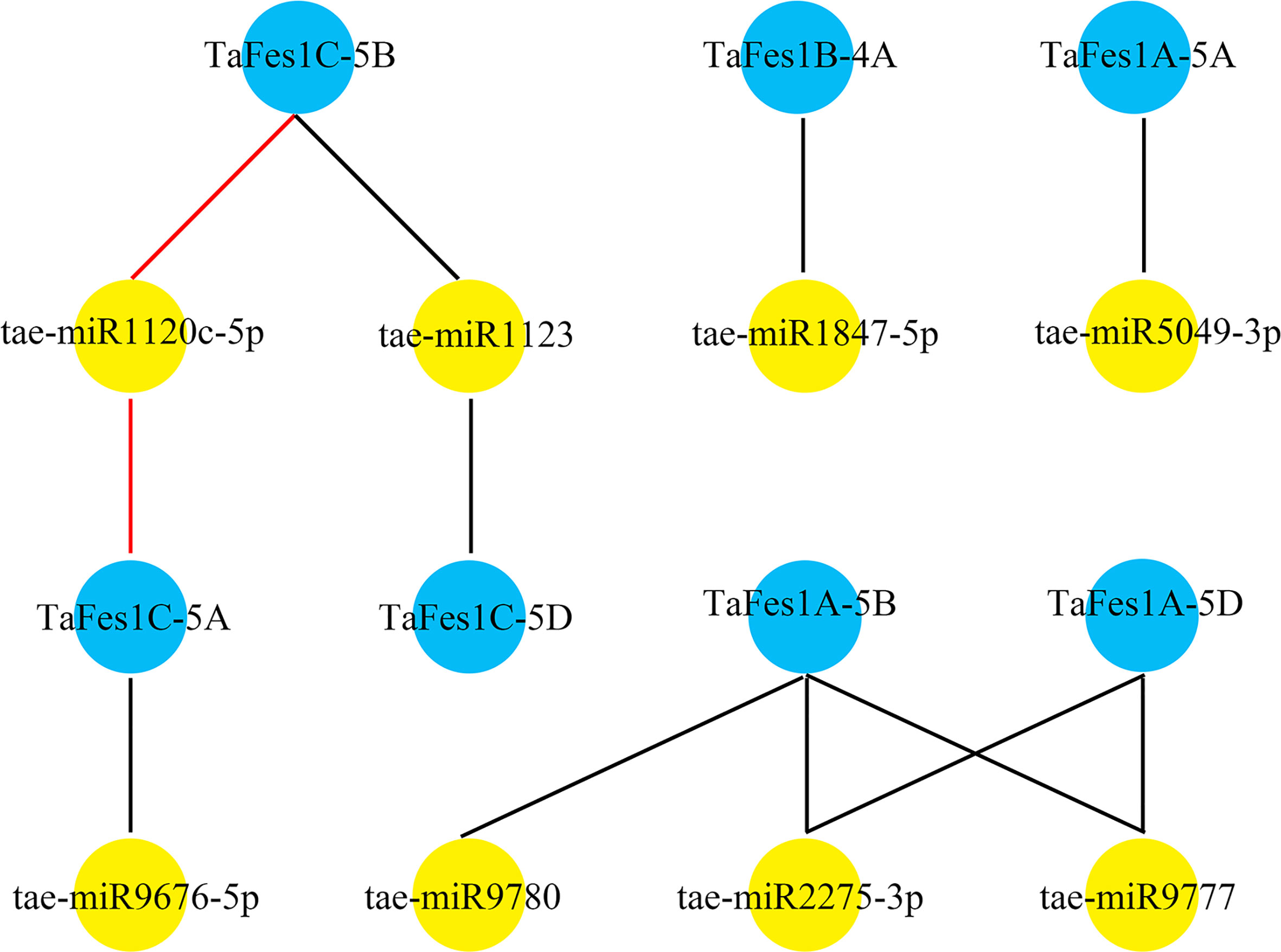
Figure 4 Potential regulation association between miRNAs and TaFes1s. MiRNAs are represented by yellow circles and TaFes1s are represent by blue circles. Dark lines link miRNAs and TaFes1s indicate the regulation relationship are transcription cleavage, and red lines are translation inhibition.
Expression profiles of TaFes1s under normal and abiotic stress condition
The transcription profiles of genes could provide important clues for illustrating the gene function, thus, the expression profiles of TaFes1s during wheat growth, development, heat stress, drought stress, and combined heat and drought stress were analyzed. Under normal condition, compared with TaFes1Bs and TaFes1Cs, all three TaFes1As were more highly and constitutively expressed across different tissues in both “Azhurnaya” and “Chinese Spring”, the three TaFes1Bs were likely preferred to be transcribed more in grain, and the three TaFes1Cs were constitutively but relatively lowly expressed (Figure 5A; Supplementary Figure 2). In wheat thermotolerant variety “TAM107” (Figure 5B), all TaFes1s were transcribed more in heat, and combined stress conditions, three TaFes1As were sharply responded to short time stress (H_1h and DH_1h), while three TaFes1Bs were stably highly expressed (H_1h, H_6h, DH_1h, and DH_1h). In wheat thermosensitive variety “Chinese Spring”, besides the fact that TaFes1C-5B were relatively lowly expressed, other TaFes1s showed similar expression patterns in grains (Figure 5C). In flag leaves, TaFes1As were transcribed most highly, and mainly under 30 minutes and 1 hour of heat stress. Finally, the expression patterns of TaFes1A-5A and TaFes1A-5D were checked by qPCR experiments and this confirmed that both genes mainly responded to short time stresses (Figures 5D, E).
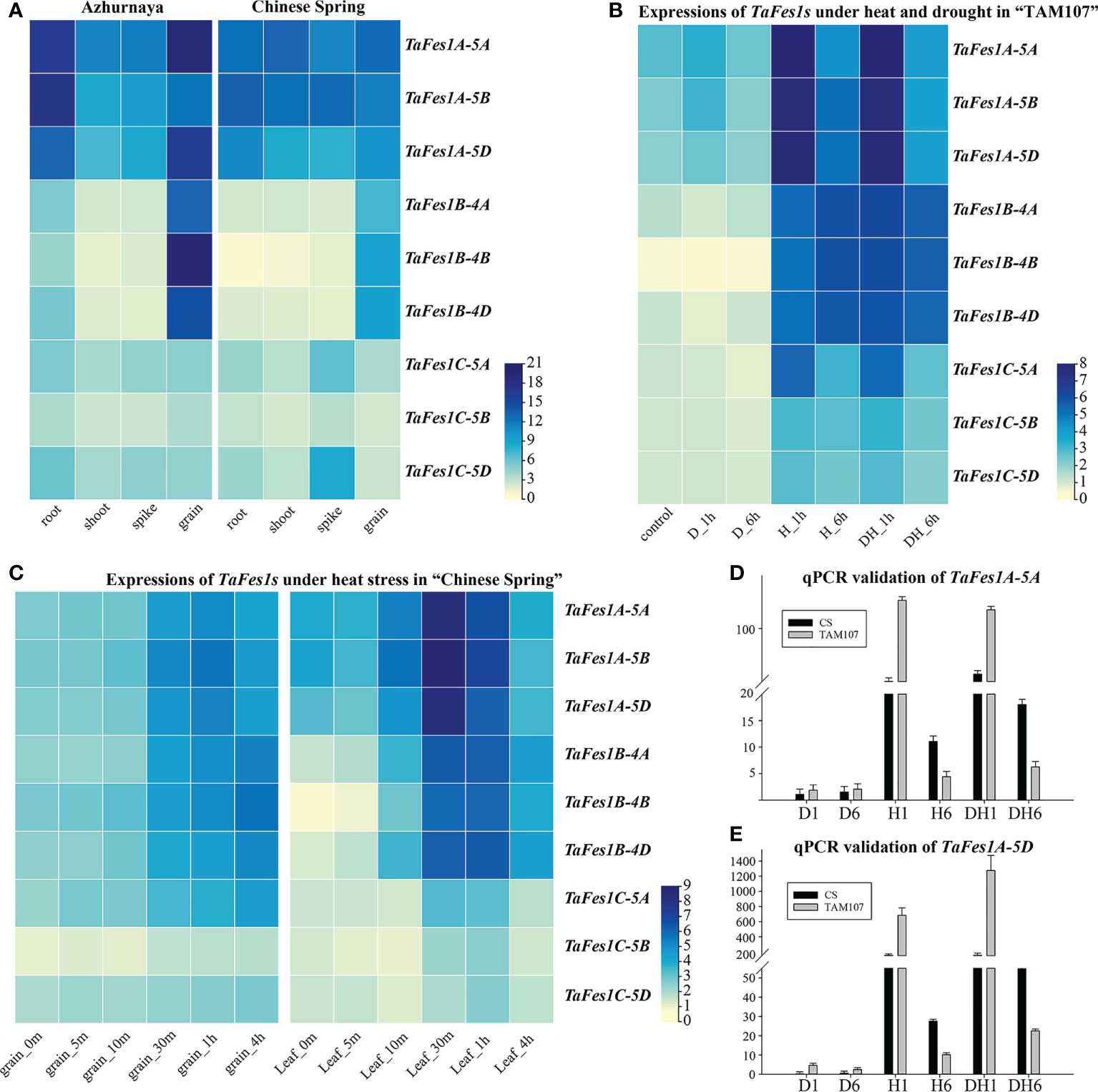
Figure 5 Expression profiles of TaFes1s in hexaploid wheat under normal and stress conditions. (A) Expression profiles of TaFes1s in wheat cultivar “Azhurnaya” and “Chinese Spring”, expression abundance is showed in tpm unit. (B) Expression profiles of TaFes1s in leaves of wheat cultivar “TAM107” at seedlings stage under heat and drought conditions. Expression abundance is shown as log2tpm. D_1h, drought stress for 1 hour, D_6h, drought stress for 6 hour, H_1h, heat stress for 1 hour, H_6h, heat stress for 6 hour, DH_1h, drought and heat stress for 1 hour, DH_6h, drought and heat stress for 6 hour. (C) Expression profiles of TaFes1s in wheat cultivar “Chinese Spring” at 15 days after anthesis under heat stress. Expression abundance is shown as log2tpm. The qPCR validation of TaFes1A-5A (D) and TaFes1A-5D (E) in wheat cultivar “Chinese Spring” and “TAM107” under heat and drought stress, where the expression level is check by three independent biological replicates and shown as 2-ΔΔCt. CS, Chinese Spring.
Roles of TaFes1A-5A and TaFes1A-5D in transgenic Arabidopsis
Based on the expression profiles of TaFes1s under normal and stress condition, we further selected TaFes1As for functional characterization. TaFes1A-5A and TaFes1A-5D were successfully cloned and transformed into an Arabidopsis thermosensitive fes1a mutant. For each gene, three independent T3 homozygous transgenic lines were finally obtained for further analysis.
It has been reported that the fes1a mutant was thermosensitive at seedling stage (Zhang et al., 2010), in this study, the mutant also showed defect in basal and acquired thermotolerance. However, overexpression of TaFes1A-5A and TaFes1A-5D could not complement thermal defect of fes1a mutants. Intriguingly, under normal condition, the seeds of transgenic lines germinated about 1 day earlier than wild type and fes1a mutant, and the wild type and fes1a mutant showed no difference (Figures 6A–D; Supplementary Figure 3). Under heat stress, the germination of transgenic seeds occurred significantly earlier than that of wild type and fes1a mutant. About 20% of transgenic seeds were germinated at day 4 of heat stress, and this ratio increased to 50% at day 5, while seeds of wild type and fes1a mutant started germination at this point (Figure 6E–H; Supplementary Figure 3). Thus, TaFes1A-5A and TaFes1A-5D could accelerate germination under both normal and heat stress conditions, but they could not rescue the thermotolerance defect of fes1a mutant at seedling stage.
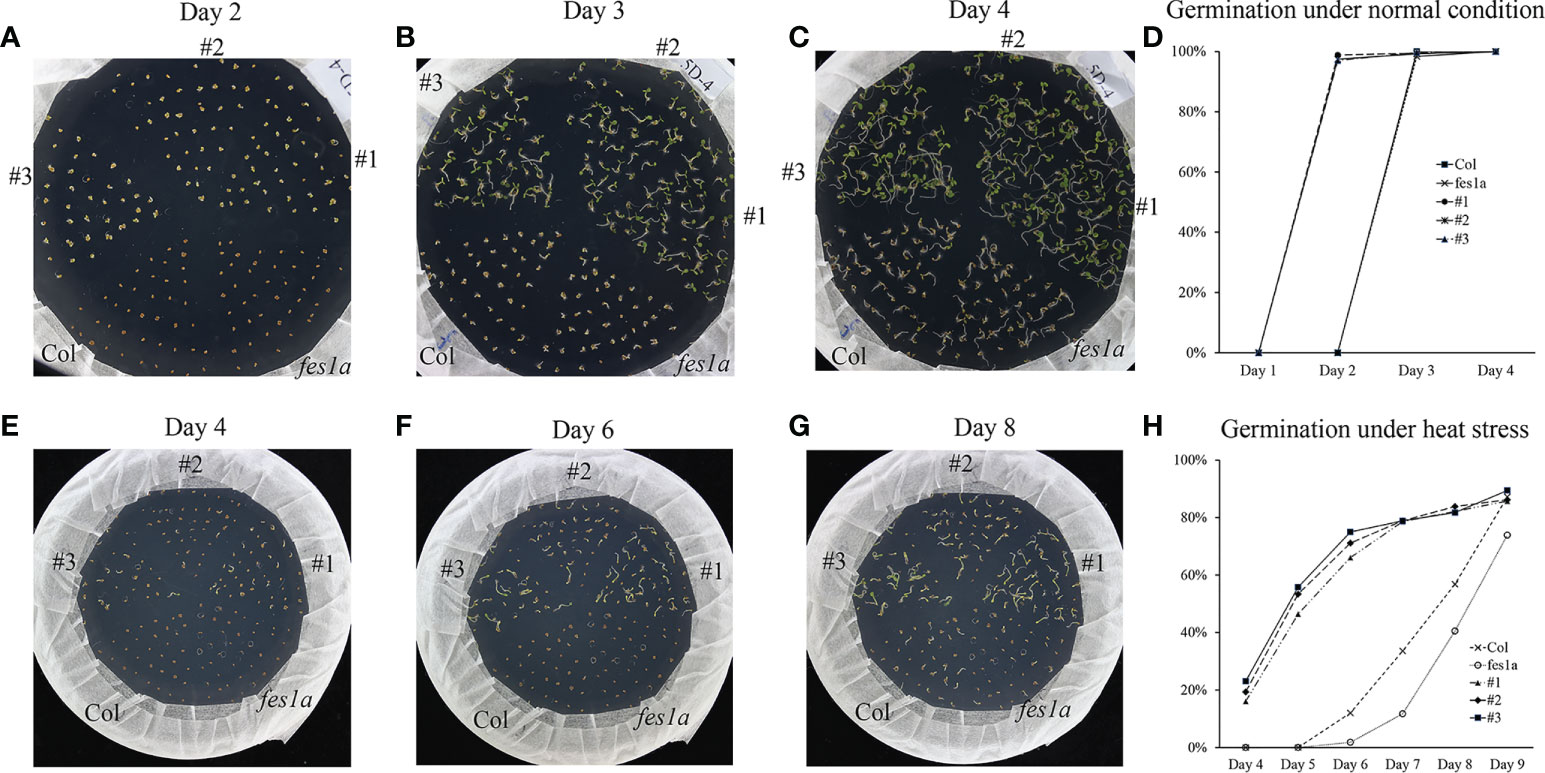
Figure 6 The seeds germination of TaFes1A-5D transgenic lines under normal and heat conditions. (A–C). Dynamic germination of transgenic lines, wild type, and mutant seeds under normal condition (22/19°C, 16/8h). (D) Germination statistics calculated from (A–C) under normal condition. (E–G). Dynamic germination of transgenic lines, wild type, and mutant seeds under heat stress condition (35/19°C, 16/8h). (H) Germination statistics calculated from (E–G) under normal condition.
Discussion
The roles of Fes1s are largely known in humans and yeast, participating in protein quality control (Alberti et al., 2004; Gowda Naveen Kumar et al., 2013; Gowda et al., 2016; Rosenzweig et al., 2019). However, in plants, little is known about these genes. In Arabidopsis, three Fes1s were identified and attention has been paid to AtFes1A. Loss of AtFes1A resulted in thermotolerance defect, while the single knockout of a TFIIIB-related factor gene or a Bcl2-associated athanogene domain gene could suppress this defect (Zhang et al., 2010; Fu et al., 2015; Fu et al., 2019; Fu et al., 2020). The roles of Fes1C in ER and salt stress were also characterized in rice (Qian et al., 2021). While distribution, expression and roles of wheat Fes1s are still unknown.
In this study, we first characterized nine Fes1s in hexaploid wheat, and they are clearly classified into Fes1A, Fes1B, and Fes1C clades, presenting as triads. Further phylogenetic analysis found that except Fes1s in Arabidopsis, Fes1As and Fes1Bs were divergent in monocots but not in dicots, and Fes1Cs evolved into different subclades in monocots and dicots, indicating that Fes1Cs may have evolved later than Fes1As and Fes1Bs. Our results display the evolutionary relationship of Fes1s in monocots and dicots. However, the Fes1s in Arabidopsis always grouped together, and this is very interesting but based on our results we could not explain any further, additional functional analysis will be needed to determine whether their roles are conserved or not. In our functional characterization we found that two TaFes1As could not rescue the thermotolerance defect of Arabidopsis fes1a mutant at seedling stage, and this result may imply that the roles of Fes1As orthologs in these two species were different.
Next, we analyzed the sequence characteristics of TaFes1s and found that gene structures and protein motifs were highly conserved within each clade but distinct among clades. Similar degrees of sequence conservation were found in other chaperone gene families, such as wheat HSP90s (Wang et al., 2011; Lu et al., 2020), and HSP101s (Erdayani et al., 2020), and this conservation may indicate their similar biological roles. Consistently and finally, the functional characterization of TaFes1A-5A and TaFes1A-5D has revealed that both genes played similar roles in seed germination under normal and heat conditions in Arabidopsis.
The fates of homoeologous genes in polyploidy plants usually evolved into functional diversification, gene silencing, and the retention of original or similar functions. About 35.8% of the total genes in hexaploid wheat can be termed as triads (Appels et al., 2018). At transcriptional level about 30% of these triads showed biased expression patterns among each homoeologous gene under normal condition (Ramírez-González et al., 2018), and this ratio increased to about 64% under heat and drought conditions (Liu et al., 2015). The silencing of the homoeologues was usually found in different tissues and developmental stages (Bottley et al., 2006; Bottley and Koebner, 2008). The functional diversification of wheat homoeologous genes was observed in wheat LEAFY HULL STERILE1 (Shitsukawa et al., 2007) and Grain Weight 8 (Ma et al., 2019). The functional retention of wheat homoeologous genes was characterized in TaEXPA1 (Hu et al., 2013b) and SEPALLATA (Shitsukawa et al., 2007). Sequence similarity and expression partitioning were important factors helping to deduce the functional conversation of homoeologous genes. Highly conserved sequences and expressions of wheat SEPALLATA led to the functional conservation of three homoeologs, and large differences in sequences and expressions of wheat LEAFY HULL STERILE1 caused the three homoeologues to function differentially (Shitsukawa et al., 2007). However, using sequences and expressions for deduction maybe also easily misleading. The coding sequence of TaEXPA1 homoeologues were high similar, and epigenetic modification led to expression divergence, but the three homoeologues displayed functional retention in Arabidopsis (Hu et al., 2013a; Hu et al., 2013b). Highly similar sequences and different expression patterns were also observed in wheat Grain Weight 8 homoeologues, but these homoeologues were associated with different traits (Ma et al., 2019). Thus, understanding the functions of homoeologues requires extensive experiments.
Data availability statement
The original contributions presented in the study are included in the article/Supplementary Material. Further inquiries can be directed to the corresponding author.
Author contributions
YL conceived and designed the study. YL performed the analysis, drafted and revised the manuscript. MH and XL performed functional characterization. JW and RM contributed to the transgenic materials. AZ performed QPCR analysis. All authors contributed to the article and approved the submitted version.
Funding
This study was mainly supported by the Natural Science Foundation of Hebei Province, China (C2020402026), and S&T Program of Hebei, China (22326303D).
Acknowledgments
We are very grateful to Prof. Jian Liu of Shandong Normal University for kindly providing Arabidopsis fes1a mutant as a gift.
Conflict of interest
The authors declare that the research was conducted in the absence of any commercial or financial relationships that could be construed as a potential conflict of interest.
Publisher’s note
All claims expressed in this article are solely those of the authors and do not necessarily represent those of their affiliated organizations, or those of the publisher, the editors and the reviewers. Any product that may be evaluated in this article, or claim that may be made by its manufacturer, is not guaranteed or endorsed by the publisher.
Supplementary material
The Supplementary Material for this article can be found online at: https://www.frontiersin.org/articles/10.3389/fpls.2022.1037989/full#supplementary-material
References
Alberti, S., Böhse, K., Arndt, V., Schmitz, A., Höhfeld, J. (2004). The cochaperone HspBP1 inhibits the CHIP ubiquitin ligase and stimulates the maturation of the cystic fibrosis transmembrane conductance regulator. Mol. Biol. Cell 15 (9), 4003–4010. doi: 10.1091/mbc.e04-04-0293
Appels, R., Eversole, K., Feuillet, C., Keller, B., Rogers, J., Stein, N., et al. (2018). Shifting the limits in wheat research and breeding using a fully annotated reference genome. Science 361 (6403), eaar7191. doi: 10.1126/science.aar7191
Avni, R., Lux, T., Minz-Dub, A., Millet, E., Sela, H., Distelfeld, A., et al. (2022). Genome sequences of three aegilops species of the section sitopsis reveal phylogenetic relationships and provide resources for wheat improvement. Plant J. 110 (1), 179–192. doi: 10.1111/tpj.15664
Bottley, A., Koebner, R. M. D. (2008). Variation for homoeologous gene silencing in hexaploid wheat. Plant J. 56 (2), 297–302. doi: 10.1111/j.1365-313X.2008.03586.x
Bottley, A., Xia, G. M., Koebner, R. M. D. (2006). Homoeologous gene silencing in hexaploid wheat. Plant J. 47 (6), 897–906. doi: 10.1111/j.1365-313X.2006.02841.x
Bracher, A., Verghese, J. (2015). The nucleotide exchange factors of Hsp70 molecular chaperones. Front. Mol. Biosci. 2. doi: 10.3389/fmolb.2015.00010
Buchberger, A., Bukau, B., Sommer, T. (2010). Protein quality control in the cytosol and the endoplasmic reticulum: Brothers in arms. Mol. Cell 40 (2), 238–252. doi: 10.1016/j.molcel.2010.10.001
Bukau, B., Horwich, A. L. (1998). The Hsp70 and Hsp60 chaperone machines. Cell 92 (3), 351–366. doi: 10.1016/S0092-8674(00)80928-9
Chen, C., Chen, H., Zhang, Y., Thomas, H. R., Frank, M. H., He, Y., et al. (2020). TBtools: An integrative toolkit developed for interactive analyses of big biological data. Mol. Plant 13 (8), 1194–1202. doi: 10.1016/j.molp.2020.06.009
Chen, S., Qiu, G. (2020). Overexpression of seagrass nucleotide exchange factor gene ZjFes1 enhances heat tolerance in transgenic arabidopsis. Plant Signaling Behav. 15 (2), 1709719. doi: 10.1080/15592324.2019.1709719
Dai, X., Zhuang, Z., Zhao, P. X. (2018). psRNATarget: a plant small RNA target analysis server, (2017 release). Nucleic Acids Res. 46 (W1), W49–W54. doi: 10.1093/nar/gky316
Dubcovsky, J., Dvorak, J. (2007). Genome plasticity a key factor in the success of polyploid wheat under domestication. Science 316 (5833), 1862–1866. doi: 10.1126/science.1143986
Erdayani, E., Nagarajan, R., Grant, N. P., Gill, K. S. (2020). Genome-wide analysis of the HSP101/CLPB gene family for heat tolerance in hexaploid wheat. Sci. Rep. 10 (1), 3948. doi: 10.1038/s41598-020-60673-4
Fu, C., Hou, Y., Ge, J., Zhang, L., Liu, X., Huo, P., et al. (2019). Increased fes1a thermotolerance is induced by BAG6 knockout. Plant Mol. Biol. 100 (1), 73–82. doi: 10.1007/s11103-019-00844-8
Fu, C., Liu, X., Li, X., Huo, P., Ge, J., Hou, Y., et al. (2020). BRF negatively regulates thermotolerance defect of fes1a in arabidopsis. Front. Plant Sci. 11. doi: 10.3389/fpls.2020.00171
Fu, C., Zhang, J., Liu, X., Yang, W., Yu, H., Liu, J. (2015). AtFes1A is essential for highly efficient molecular chaperone function in arabidopsis. J. Plant Biol. 58 (6), 366–373. doi: 10.1007/s12374-015-0181-y
Gowda, N. K. C., Kaimal, J. M., Masser, A. E., Kang, W., Friedländer, M. R., Andréasson, C. (2016). Cytosolic splice isoform of Hsp70 nucleotide exchange factor Fes1 is required for the degradation of misfolded proteins in yeast. Mol. Biol. Cell 27 (8), 1210–1219. doi: 10.1091/mbc.E15-10-0697
Gowda Naveen Kumar, C., Kandasamy, G., Froehlich Marceli, S., Dohmen, R. J., Andréasson, C. (2013). Hsp70 nucleotide exchange factor Fes1 is essential for ubiquitin-dependent degradation of misfolded cytosolic proteins. Proc. Natl. Acad. Sci. 110 (15), 5975–5980. doi: 10.1073/pnas.1216778110
Hu, Z., Han, Z., Song, N., Chai, L., Yao, Y., Peng, H., et al. (2013a). Epigenetic modification contributes to the expression divergence of three TaEXPA1 homoeologs in hexaploid wheat (Triticum aestivum). New Phytol. 197 (4), 1344–1352. doi: 10.1111/nph.12131
Hu, Z., Song, N., Xing, J., Chen, Y., Han, Z., Yao, Y., et al. (2013b). Overexpression of three TaEXPA1 homoeologous genes with distinct expression divergence in hexaploid wheat exhibit functional retention in arabidopsis. PLos One 8 (5), e63667. doi: 10.1371/journal.pone.0063667
Kalyaanamoorthy, S., Minh, B. Q., Wong, T. K. F., von Haeseler, A., Jermiin, L. S. (2017). ModelFinder: fast model selection for accurate phylogenetic estimates. Nat. Methods 14 (6), 587–589. doi: 10.1038/nmeth.4285
Kampinga, H. H., Craig, E. A. (2010). The HSP70 chaperone machinery: J proteins as drivers of functional specificity. Nat. Rev. Mol. Cell Biol. 11 (8), 579–592. doi: 10.1038/nrm2941
Khraiwesh, B., Zhu, J.-K., Zhu, J. (2012). Role of miRNAs and siRNAs in biotic and abiotic stress responses of plants. Biochim. Biophys. Acta (BBA) - Gene Regul. Mech. 1819 (2), 137–148. doi: 10.1016/j.bbagrm.2011.05.001
Kumar, A., Sharma, S., Chunduri, V., Kaur, A., Kaur, S., Malhotra, N., et al. (2020). Genome-wide identification and characterization of heat shock protein family reveals role in development and stress conditions in Triticum aestivum l. Sci. Rep. 10 (1), 7858. doi: 10.1038/s41598-020-64746-2
Lescot, M., Déhais, P., Thijs, G., Marchal, K., Moreau, Y., Van de Peer, Y., et al. (2002). PlantCARE, a database of plant cis-acting regulatory elements and a portal to tools for in silico analysis of promoter sequences. Nucleic Acids Res. 30 (1), 325–327. doi: 10.1093/nar/30.1.325
Lesk, C., Rowhani, P., Ramankutty, N. (2016). Influence of extreme weather disasters on global crop production. Nature 529 (7584), 84–87. doi: 10.1038/nature16467
Levy, A. A., Feldman, M. (2022). Evolution and origin of bread wheat. Plant Cell 34 (7), 2549–2567. doi: 10.1093/plcell/koac130
Liu, B., Liu, L., Tian, L., Cao, W., Zhu, Y., Asseng, S. (2014). Post-heading heat stress and yield impact in winter wheat of China. Global Change Biol. 20 (2), 372–381. doi: 10.1111/gcb.12442
Liu, Z., Xin, M., Qin, J., Peng, H., Ni, Z., Yao, Y., et al. (2015). Temporal transcriptome profiling reveals expression partitioning of homeologous genes contributing to heat and drought acclimation in wheat (Triticum aestivum l.). BMC Plant Biol. 15 (1), 152. doi: 10.1186/s12870-015-0511-8
Lu, Y., Zhao, P., Zhang, A., Ma, L., Xu, S., Wang, X. (2020). Alternative splicing diversified the heat response and evolutionary strategy of conserved heat shock protein 90s in hexaploid wheat (Triticum aestivum l.). Front. Genet. 11. doi: 10.3389/fgene.2020.577897
Lu, Y., Zhao, P., Zhang, A., Wang, J., Ha, M. (2022). Genome-wide analysis of HSP70s in hexaploid wheat: Tandem duplication, heat response, and regulation. Cells 11 (5), 818. doi: 10.3390/cells11050818
Ma, L., Hao, C., Liu, H., Hou, J., Li, T., Zhang, X. (2019). Diversity and sub-functionalization of TaGW8 homoeologs hold potential for genetic yield improvement in wheat. Crop J. 7 (6), 830–844. doi: 10.1016/j.cj.2019.09.006
Marcussen, T., Sandve, S. R., Heier, L., Spannagl, M., Pfeifer, M., The International Wheat Genome Sequencing Consortium, et al. (2014). Ancient hybridizations among the ancestral genomes of bread wheat. Science 345 (6194), 1250092. doi: 10.1126/science.1250092
Ma, S., Wang, M., Wu, J., Guo, W., Chen, Y., Li, G., et al. (2021). WheatOmics: A platform combining multiple omics data to accelerate functional genomics studies in wheat. Mol. Plant 14 (12), 1965–1968. doi: 10.1016/j.molp.2021.10.006
Mayer, M. P., Bukau, B. (2005). Hsp70 chaperones: Cellular functions and molecular mechanism. Cell. Mol. Life Sci. 62 (6), 670. doi: 10.1007/s00018-004-4464-6
Nguyen, L.-T., Schmidt, H. A., von Haeseler, A., Minh, B. Q. (2015). IQ-TREE: A fast and effective stochastic algorithm for estimating maximum-likelihood phylogenies. Mol. Biol. Evol. 32 (1), 268–274. doi: 10.1093/molbev/msu300
Pagano, L., Rossi, R., Paesano, L., Marmiroli, N., Marmiroli, M. (2021). miRNA regulation and stress adaptation in plants. Environ. Exp. Bot. 184, 104369. doi: 10.1016/j.envexpbot.2020.104369
Qian, D., Xiong, S., Li, M., Tian, L., Qing Qu, L. (2021). OsFes1C, a potential nucleotide exchange factor for OsBiP1, is involved in the ER and salt stress responses. Plant Physiol. 187 (1), 396–408. doi: 10.1093/plphys/kiab263
Ramírez-González, R. H., Borrill, P., Lang, D., Harrington, S. A., Brinton, J., Venturini, L., et al. (2018). The transcriptional landscape of polyploid wheat. Science 361 (6403), eaar6089. doi: 10.1126/science.aar6089
Rosenzweig, R., Nillegoda, N. B., Mayer, M. P., Bukau, B. (2019). The Hsp70 chaperone network. Nat. Rev. Mol. Cell Biol. 20 (11), 665–680. doi: 10.1038/s41580-019-0133-3
Shitsukawa, N., Tahira, C., Kassai, K.-i., Hirabayashi, C., Shimizu, T., Takumi, S., et al. (2007). Genetic and epigenetic alteration among three homoeologous genes of a class e MADS box gene in hexaploid wheat. Plant Cell 19 (6), 1723–1737. doi: 10.1105/tpc.107.051813
Tack, J., Barkley, A., Nalley, L. L. (2015). Effect of warming temperatures on US wheat yields. Proc. Natl. Acad. Sci. 112 (22), 6931–6936. doi: 10.1073/pnas.1415181112
Wang, X., Chen, S., Shi, X., Liu, D., Zhao, P., Lu, Y., et al. (2019). Hybrid sequencing reveals insight into heat sensing and signaling of bread wheat. Plant J. 98 (6), 1015–1032. doi: 10.1111/tpj.14299
Wang, W., Vinocur, B., Shoseyov, O., Altman, A. (2004). Role of plant heat-shock proteins and molecular chaperones in the abiotic stress response. Trends Plant Sci. 9 (5), 244–252. doi: 10.1016/j.tplants.2004.03.006
Wang, G.-F., Wei, X., Fan, R., Zhou, H., Wang, X., Yu, C., et al. (2011). Molecular analysis of common wheat genes encoding three types of cytosolic heat shock protein 90 (Hsp90): functional involvement of cytosolic Hsp90s in the control of wheat seedling growth and disease resistance. New Phytol. 191 (2), 418–431. doi: 10.1111/j.1469-8137.2011.03715.x
Yadav, M. R., Choudhary, M., Singh, J., Lal, M. K., Jha, P. K., Udawat, P., et al. (2022). Impacts, tolerance, adaptation, and mitigation of heat stress on wheat under changing climates. Int. J. Mol. Sci. 23 (5), 2838. doi: 10.3390/ijms23052838
Zhang, J.-X., Wang, C., Yang, C.-Y., Wang, J.-Y., Chen, L., Bao, X.-M., et al. (2010). The role of arabidopsis AtFes1A in cytosolic Hsp70 stability and abiotic stress tolerance. Plant J. 62 (4), 539–548. doi: 10.1111/j.1365-313X.2010.04173.x
Zhao, P., Javed, S., Shi, X., Wu, B., Zhang, D., Xu, S., et al. (2020). Varying architecture of heat shock elements contributes to distinct magnitudes of target gene expression and diverged biological pathways in heat stress response of bread wheat. Front. Genet. 11. doi: 10.3389/fgene.2020.00030
Zhao, C., Liu, B., Piao, S., Wang, X., Lobell, D. B., Huang, Y., et al. (2017). Temperature increase reduces global yields of major crops in four independent estimates. Proc. Natl. Acad. Sci. 114 (35), 9326–9331. doi: 10.1073/pnas.1701762114
Keywords: hexaploid wheat, Fes1, thermotolerance, evolution, expression
Citation: Lu Y, Ha M, Li X, Wang J, Mo R and Zhang A (2022) Distribution, expression of hexaploid wheat Fes1s and functional characterization of two TaFes1As in Arabidopsis. Front. Plant Sci. 13:1037989. doi: 10.3389/fpls.2022.1037989
Received: 06 September 2022; Accepted: 03 October 2022;
Published: 17 October 2022.
Edited by:
Xiaoyu Weng, University of Texas at Austin, United StatesReviewed by:
Amita Pandey, Shriram Institute for Industrial Research, IndiaJianbo Li, Chinese Academy of Forestry, China
Copyright © 2022 Lu, Ha, Li, Wang, Mo and Zhang. This is an open-access article distributed under the terms of the Creative Commons Attribution License (CC BY). The use, distribution or reproduction in other forums is permitted, provided the original author(s) and the copyright owner(s) are credited and that the original publication in this journal is cited, in accordance with accepted academic practice. No use, distribution or reproduction is permitted which does not comply with these terms.
*Correspondence: Yunze Lu, eXVuemVsdUBoZWJldS5lZHUuY24=