- Science Innovation Team of Oats, Inner Mongolia Agricultural University, Hothot, China
Stem rust, caused by Puccinia graminis f. sp. avenae (Pga) is a key disease affecting oat production worldwide. Silicon (Si) plays an essential role in enhancing plant resistance against pathogens. However, the scientific evidence of Si-mediated stem rust resistance of oat from the photosynthetic perspective has not been reported. The specific objective of this research was to investigate the effects of Si application on disease inhibition, photosynthetic gas exchange parameters, light response parameters, photosynthetic pigments and chlorophyll fluorescence parameters under Pga infection. Our results illustrated that Si application significantly reduced rust severity while the other parameters like net photosynthetic rate (Pn), stomatal conductance (Gs), intercellular CO2 concentration (Ci) and transpiration rate (Tr) were significantly increased. Si application increased maximum photosynthetic rate (Pnmax) and light saturation point (LSP), while reduced the dark respiration rate (Rd) and light compensation point (LCP). The results also indicated that Si application significantly increased the activities of maximum fluorescence (Fm), variable fluorescence (Fv), maximum quantum yield of photosystem II (Fv/Fm), photochemical quenching (qP), photosynthetic performance index (PIABS), actual PSII quantum yield (ΦPSII), electron transfer rate (ETR), the absorbed light energy per unit reaction center (ABS/RC) and the dissipated energy per unit reaction center (DIo/RC), whereas it decreased the minimal fluorescence (Fo), non-photochemical quenching (NPQ), the absorbed light energy used for electron transfer per unit reaction center (ETo/RC) and the absorbed light energy used for reduction of QA per unit reaction center (TRo/RC). The contents of chlorophyll a, b and carotenoids were also increased due to the change in the activity of parameters due to Si application as mentioned above. In conclusion, the results of the current study suggests that Si imparts tolerance to the stem rust possibly by the underlying mechanisms of improving gas exchange performance, and efficiency of the photochemical compounds in oat leaves.
Introduction
Oat (Avena sativa L.) is a vital grain and forage crop, and is the sixth most important crop grown globally (Zhao et al., 2020). Oat is increasingly being used for human consumption as a beneficial health food, as it contains a variety of nutrient-rich substances, including β-glucans and vitamin E (Alminger and Eklund-Jonsson, 2008; Nazare et al., 2009; Whitehead et al., 2014). Like other agricultural crops, its production is also affected by various factors including biotic and abiotic stresses. It has been estimated that, about 25% of annual crop losses are caused by biotic stresses especially plant diseases (Lugtenberg, 2015).
Among various plant diseases, stem rust of oats is one of the most significant factors, limiting the high yield potential of the cultivars, Puccinia graminis f. sp. avenae (Pga) is the causative agent of oat stem rust, a major oat disease that can lead to total crop failure during severe epidemics. It is an economically important disease in the USA and Canada’s prairie provinces (Gold Steinberg et al., 2005). After infected with stem rust, the thousand-grain weight is reduced, the flour is black, and the stems are easy to break (Yuan et al., 2014). Therefore, effective prevention and control methods of stem rust are urgently needed.
Silicon (Si) has gained more attention due to its role in plant growth and imparting tolerance to various biotic and abiotic stresses (Epstein, 2009; Frew et al., 2018). Its content in the earth’s crust is about 28%, which makes it the second largest element in terms of abundance after oxygen. Due to its potential role in improving plants’ defenses against various diseases caused by fungi, bacteria and viruses, it has become a prime focus of research, especially with the increase in the pest and diseases incidence due to climate change (Debona et al., 2017; Luyckx et al., 2017).
The leaves of host plants are the major photosynthetic tissues and are the main targets of many pathogens. The pathogens infection directly reduces the photosynthetic performance of the leaves and eventually results in huge losses in terms of crop yield (Yang et al., 2014). Inhibition of photosynthesis by pathogens has been reported in many plants. Studies have shown that the photosynthetic activities of wheat are closely related to stripe rust, and the photosystem II (PSII) is highly susceptible to Puccinia striiformis f. sp. tritici (Pst) infection, they also found that wheat may effectively improve resistance to stripe rust by maintaining a higher PSII activity. This result provides a better understanding of wheat resistance mechanisms against stripe rust infections (Chen et al., 2015; Li et al., 2015). Some evidence suggests that, within given limits, Si may maintain the photosynthetic rate of plants upon pathogen infection (Aucique-Pe´rez et al., 2014; Domiciano et al., 2015; Tatagiba et al., 2015; Debona et al., 2017), but the underlying mechanisms remain unresolved. Hence, the current studies were aimed to explore Si’s protective role and underlying defense response mechanism in oat against Pga infection.
We hypothesized that Si-modulated operational ability of photosystem II may be a potential mechanism imparting stem rust resistance in oat. The objective of the current study was to analyze the photosynthetic characteristics and photosystem II functions in leaves to reveal the photosynthetic mechanism of Si induced resistance towards stem rust in oat, to test the original hypothesis.
Materials and methods
Materials, culture conditions and experimental design
Oat cultivar Bayou 1 (high susceptible) was used for inoculation with Pga (race TKR, was provided by the Institute of Plant Immunity, Shenyang Agricultural University). Twenty oat seeds were grown in a 12 cm diameter pot (12cm×15cm) with the peat soil matrix, seedlings were cultured in a greenhouse (20 ± 2°C with a photoperiod of 16 h light/8 h dark) at the Oat Research Center of Inner Mongolia Agricultural University.
Four treatments with three replications each were prepared for both plants: (1) CK (no silicon and no Pga inoculation); (2) +Si-P (1.5 mmol·L–1 silicon application, no Pga inoculation); (3) -Si+P (no silicon, Pga inoculation); (4) +Si+P (1.5 mmol·L–1 silicon application and Pga inoculation). Silicon (1.5 mmol·L–1) was added as potassium silicate (K2SiO3) solution, in the silicon-deficient treatment, potassium chloride (KCl, pH 5.5) was used to equal the potassium component of the Si treatment, and the nutrient solution used was configured according to Hoagland’s classic formula (Jiang et al., 2013). From the beginning of the emergence of oat seedlings, different treatments of nutrient solutions were used to irrigate the pots every 3 days, 150 mL per pot. Below the pot, a solution collector plate was added and the cultivation system was opened.
The inoculation was carried out when the oat seedlings grew to the two-leaf stage (one leaf and one sprout). The method of inoculation was carried out as described by Li et al. (2014). First, the leaves were sprayed with a 0.05% Tween-20 solution (Polyoxyethylene sorbaitan monolaurate, water soluble emulsifier, 0.05%) using a handheld atomizer to form a water film on the leaves. Then, flat toothpick (only by contact) was used to pick fresh urediniospores (0.01 g) and inoculated on the seedlings. Finally, the inoculated plants were kept in a mist chamber at 18 to 20°C for 16 h in darkness. Plants were transferred to a 16/8 h (light/dark) photoperiod, and a climatic chamber at 24 °C with 80 ± 5% humidity.
Photosynthetic gas exchange parameters
The leaf gas exchange parameters of all treatment combination were recorded at 0, 1, 3, 5, 7, 9 and 11days after inoculation. The readings of the variables were performed on the first fully expanded leaf (the intermediate section, the chlorotic area), three readings were taken per leaf in the pot.
The net photosynthetic rate (Pn), stomatal conductance (Gs), intercellular CO2 concentration (Ci) and transpiration rate (Tr) were measured at room temperature (25°C) and 60% relative humidity with a portable system (CIRAS-3, PP Systems, UK). The photosynthetic active radiation (PAR) in the leaf chamber, provided by the CIRAS-3 LED light source, was set to 1000 µmol·m–2s–1.
On day 11 after inoculation, under a fixed atmospheric CO2 concentration (Ca) of 380 μmol·mol-1, the net photosynthetic rate (Pn), stomatal conductance (Gs) and intercellular CO2 concentration (Ci) to photosynthetic active radiation (PAR) curves of the most fully expanded leaf was recorded after the PAR of LED light sourced couple to a leaf chamber were set to 2000, 1800, 1500, 1200, 1000, 800, 500, 200, 100, 50, 20 and 0 μmol·m−2s−1, respectively. The maximum photosynthetic rate (Pnmax) and dark respiration rate (Rd) were recorded. The light compensation point (LCP) was calculated when PAR was close to zero and the light saturation point (LSP) as the PAR value was obtained when photosynthesis reached Pnmax.
Chlorophyll a fluorescence parameters
After the photosynthetic gas exchange parameter measurements, a chlorophyll fluorescence experiment was carried out on the same leaves using a plant efficiency analyzer (Handy-PEA, Hansatech, UK). Leaves were dark-adapted for 30 min before measurements, during light illumination, chlorophyll a fluorescence intensity in dark- adapted leaves rose rapidly from an initial minimal level, Fo (O step) to the maximal level, Fm (P step), and two intermediate steps designated as J and I appeared at 2 and 30 ms, respectively. So, a fast rise of the chlorophyll fluorescence, transient with the notation OJIP, was recorded. The photosynthetic performance index based on the absorbed light energy (PIABS), the absorbed light energy per unit reaction center (ABS/RC), the absorbed light energy used for reduction of QA per unit reaction center (TRo/RC), the absorbed light energy used for electron transfer per unit reaction center (ETo/RC), and the dissipated energy per unit reaction center (DIo/RC) were obtained. The maximum quantum yield of PSII (Fv/Fm) was calculated according to the formula: Fv/Fm= [(Fm-Fo)/Fm].
Modulated chlorophyll fluorescence was measured with a FMS-2 pulse-modulated fluorometer (Hansatech, UK). The light-adapted leaves were continuously illuminated by actinic light at 800 μmol·m−2s−1 from the FMS-2 light source (PFD), steady-state fluorescence (Fs) was recorded after a 2 min illumination, and a saturation pulse (8000 μmol·m-2s-1; 0.8s) was applied to achieve the light-adapted maximum fluorescence (Fm’). The actinic light was then turned off, and the minimum fluorescence in the light-adapted state (Fo’) was determined by a 3 s illumination with far-red light. The following parameters were calculated (Maxwell and Johnson, 2000):
Actual PSII quantum yield, ΦPSII = (Fm’-Fs)/Fm’
Electron transport rate, ETR = ΦPSII×PFD×0.5×0.84
Photochemical quenching, qP= (Fm’- Fs)/(Fm’-Fo’)
Non-photochemical quenching, NPQ = (Fm/Fm’)-1
Photosynthetic pigment
The leaves of each plant per replication of each treatment were collected at 0, 1, 3, 5, 7, 9 and 11days after inoculation, 0.5 g of leaf tissue was frozen in liquid nitrogen, homogenized in 80% acetone with a small amount of SiO2 and centrifuged (3600 × g, 5 min). Contents of chlorophyll a (Chla), chlorophyll b (Chlb) and total carotenoids (Car) in the supernatant were then determined spectrophotometrically (UV2300IISpectrophotometer, CHINA), according to Lichtenthaler (Lichtenthaler, 1987).
Data analysis
In this study, the charts were made using Microsoft Excel 2016 software. All data were expressed as the mean ± SE. One-way ANOVA was performed to test the significance of the observed differences using SPSS (Inc., Chicago, USA). Differences between parameters were evaluated using Duncan’ s method, and P ≤ 0.05 was considered the statistically significant threshold.
Results
Phenotypes
Obviously, 15 days after inoculation with Pga, leaves developed many orange pustules without Si, and the color depth of pustules was significantly reduced under Si application (Figure 1).
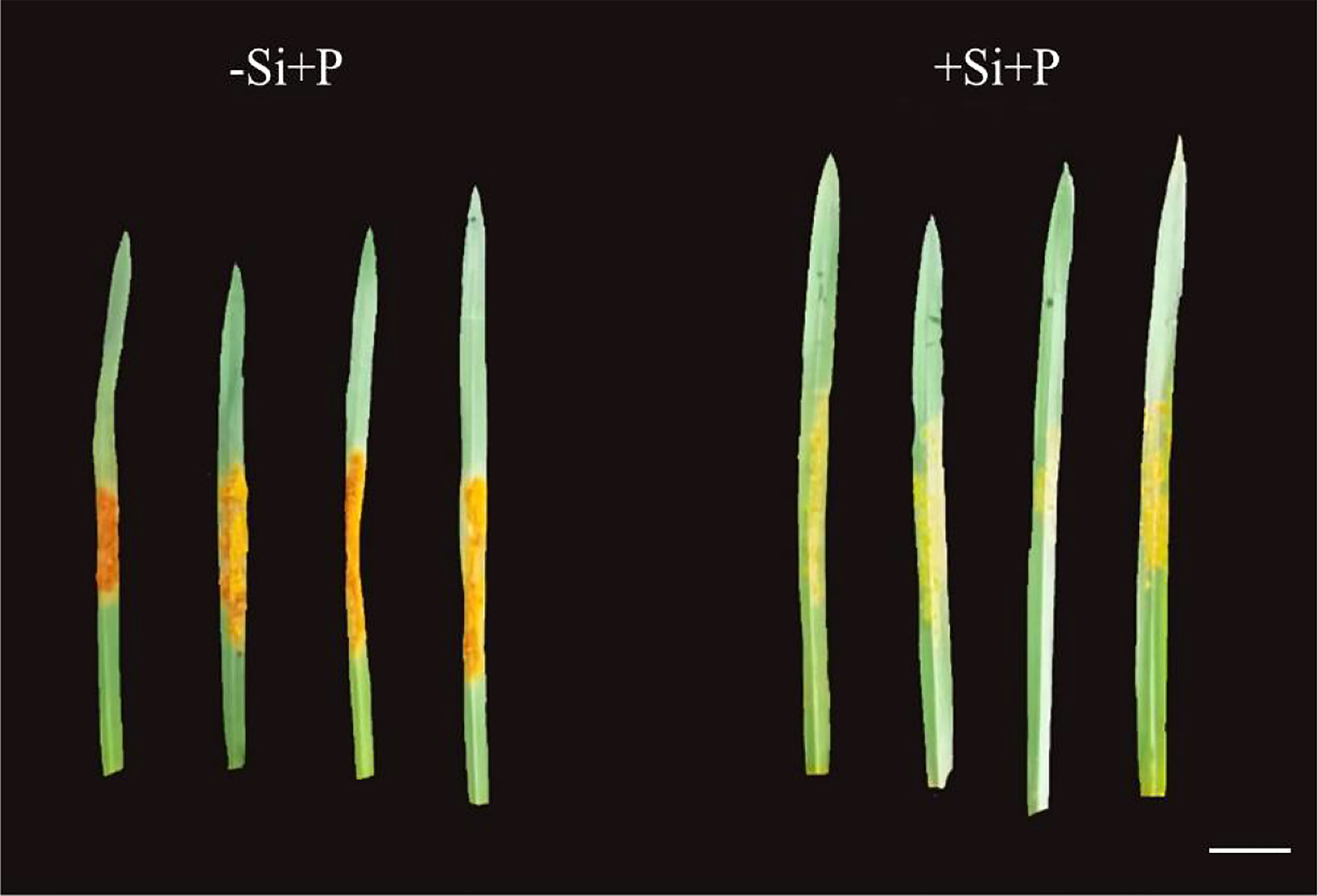
Figure 1 Effects of Si application and Pga inoculation on phenotype of oat, scale bar=1 cm. -Si+P, no Si application and Pga inoculation; +Si+P, 1.5 mmol·L–1 Si application and Pga inoculation.
Photosynthetic gas exchange parameters
The values of photosynthetic parameters showed no significant difference for non-inoculated oat seedlings (Figure 2). In the early stage of infection (0-3 days after inoculation), Pn (Figure 2A) and Tr (Figure 2B) increased rapidly, then began to decline gradually after reaching the peak and Ci (Figure 2D) exhibited the opposite tendency, while Gs (Figure 2C) showed a chaotic change pattern. Compared with -Si+P, Si application (+Si+P) increased Pn, Tr, Gs and Ci significantly (P ≤ 0.05).
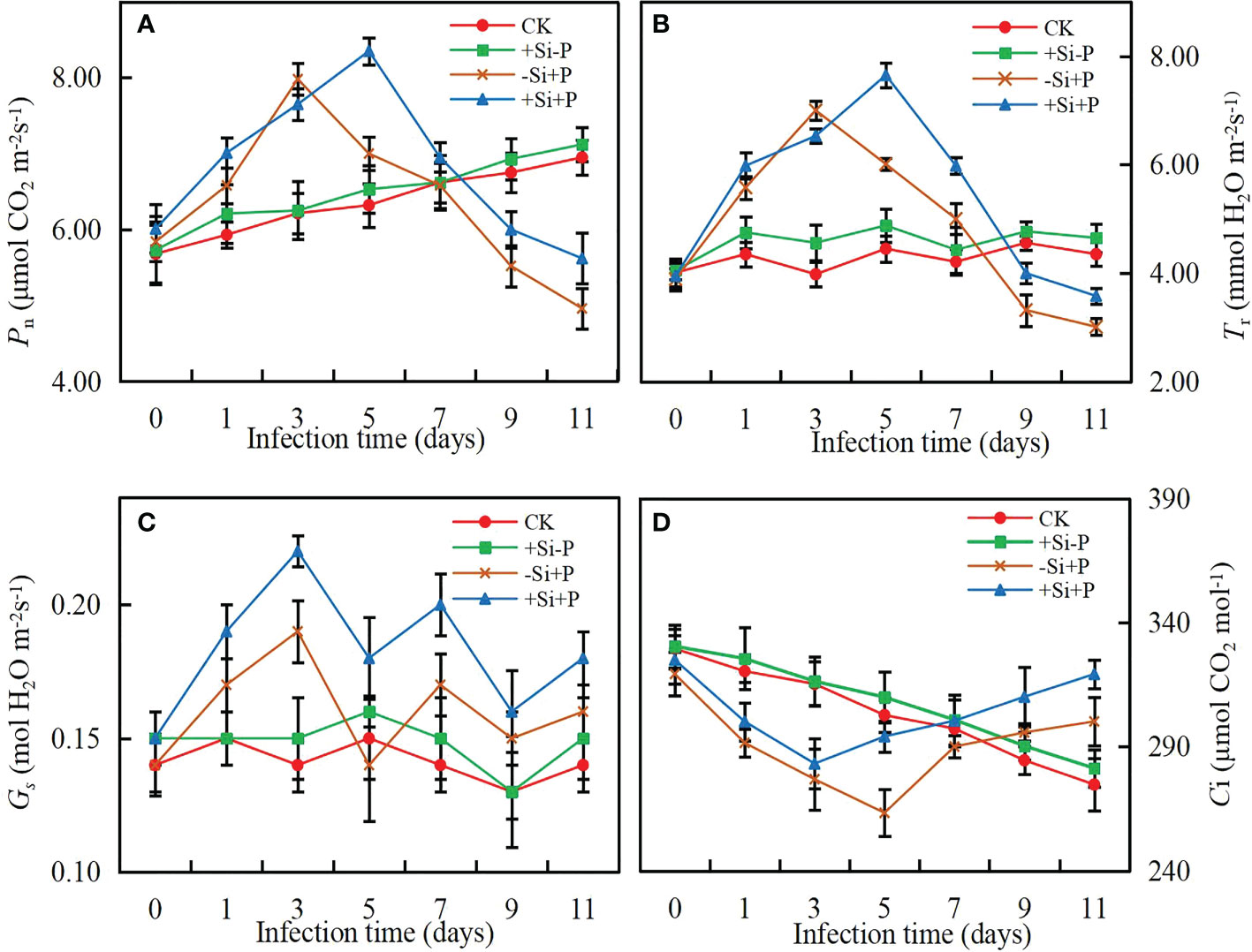
Figure 2 Effects of Si application and Pga inoculation on photosynthetic parameters of oat leaves. (A), net photosynthetic rate (Pn); (B), stomatal conductance (Gs); (C), transpiration rate (Tr); (D), intercellular CO2 concentration (Ci). CK, no Si application and no Pga inoculation; +Si-P, 1.5 mmol·L–1 Si application and no Pga inoculation; -Si+P, no Si application and Pga inoculation; +Si+P, 1.5 mmol·L–1 Si application and Pga inoculation.
Without Pga inoculation, Pn (Figure 3A) and Gs (Figure 3B) gradually increased with PAR, while Ci (Figure 3C) gradually decreased, and finally remained stable. Under -Si+P treatment, Pn began to decrease gradually when it reached the highest point, while remained stable under +Si+P treatment.
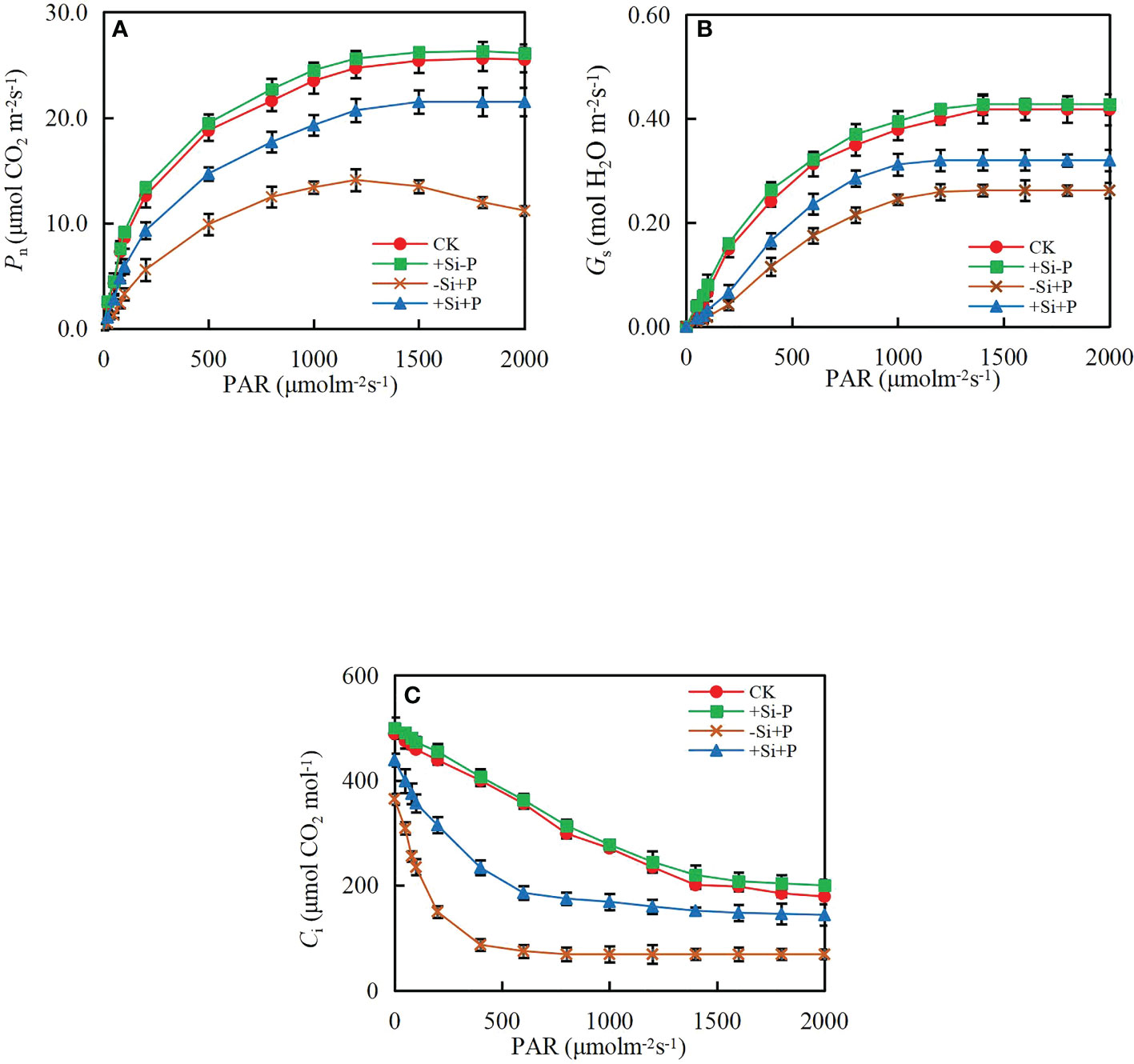
Figure 3 Response of (A) net photosynthetic rate (Pn), (B) stomatal conductance (Gs) and (C) intercellular CO2 concentration (Ci) to photosynthetic photon flux density (PAR) of oat leaves. CK, no Si application and no Pga inoculation; +Si-P, 1.5 mmol·L–1 Si application and no Pga inoculation; -Si+P, no Si application and Pga inoculation; +Si+P, 1.5 mmol·L–1 Si application and Pga inoculation.
By fitting the Pn/PAR curve of oat leaves, various light response parameters under different treatments were obtained (Figure 4). In non-inoculated oat seedlings, Si had no effect on light response parameters. Compared with CK, Pnmax (Figure 4A) and LSP (Figure 4B) were significantly decreased by 44.42 and 47.14%, respectively, while LCP (Figure 4C) and Rd (Figure 4D) were significantly increased by 119.30 and 35.19% under -Si+P treatment, respectively; Compared with -Si+P, Si application (+Si+P) significantly increased Pnmax and LSP by 47.26 and 15.30%, respectively, while decreased LCP and Rd by 25.28 and 11.64%, respectively (P ≤ 0.05).
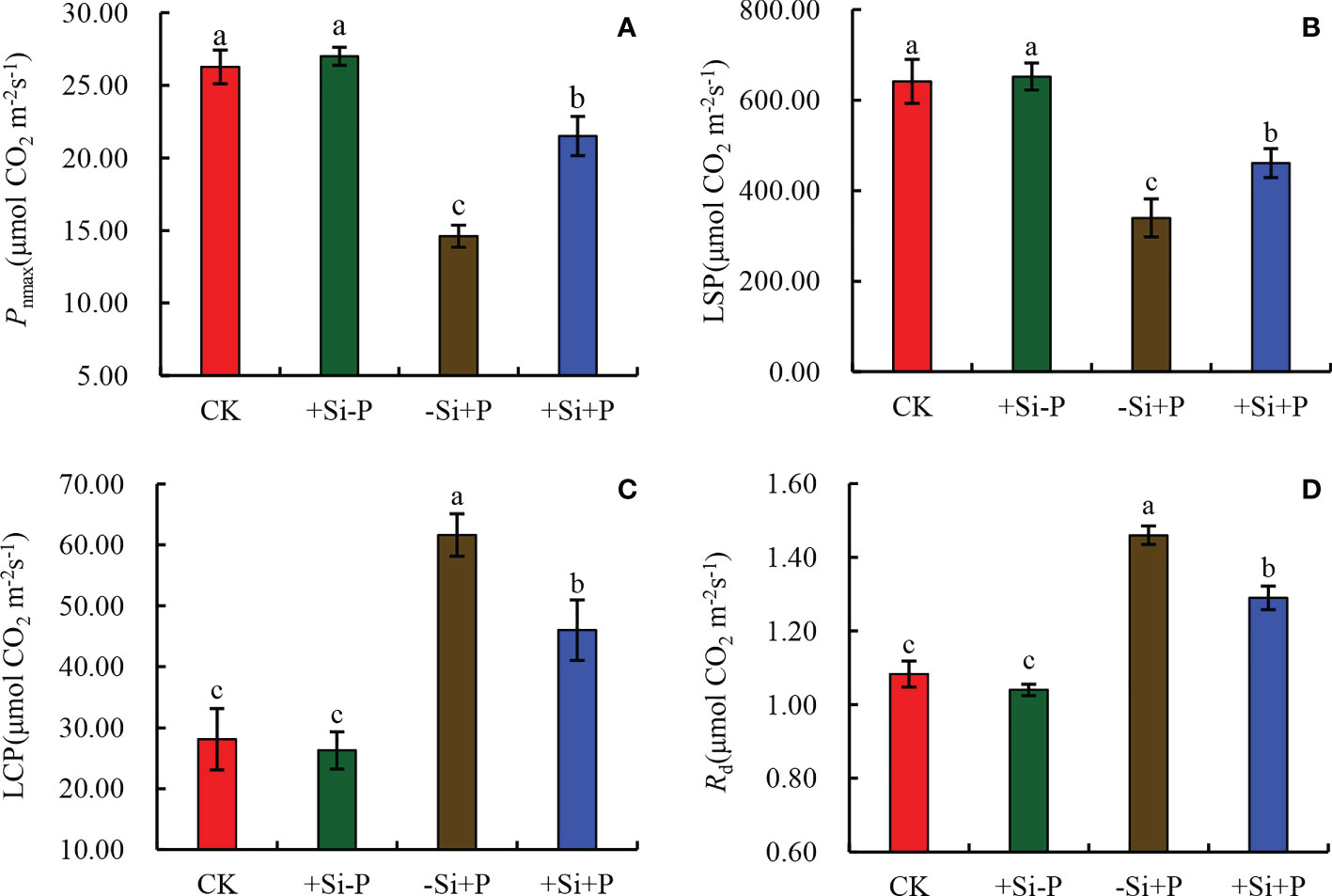
Figure 4 Effects of Si application and Pga inoculation on light response parameters of oat leaves. (A), maximum photosynthetic rate (Pnmax); (B), light saturation point (LSP); (C), light compensation point (LCP); (D), dark respiration rate (Rd). CK, no Si application and no Pga inoculation; +Si-P, 1.5 mmol·L–1 Si application and no Pga inoculation; -Si+P, no Si application and Pga inoculation; +Si+P, 1.5 mmol·L–1 Si application and Pga inoculation. Data are expressed as mean ± SE (n=3). According to Duncan’s multiple comparison tests among treatments, different letters on bars show significant differences at 0.05 level of probability.
Photosynthetic pigments
In non-inoculated oat seedlings, Si application did not have any effects on the content of pigments (Chla, Chlb, Car and Chla+Chlb) in oat leaves (Figure 5). Pigments content began to decrease significantly at 3 d after Pga inoculation, and compared with -Si+P, Si application (+Si+P) significantly increased Chla (up to 20.00%) (Figure 5A), Chlb (up to 20.00%) (Figure 5B), Car (up to 26.67%) (Figure 5D) and Chla+Chlb (up to 20.00%) (Figure 5C), respectively (P ≤ 0.05).
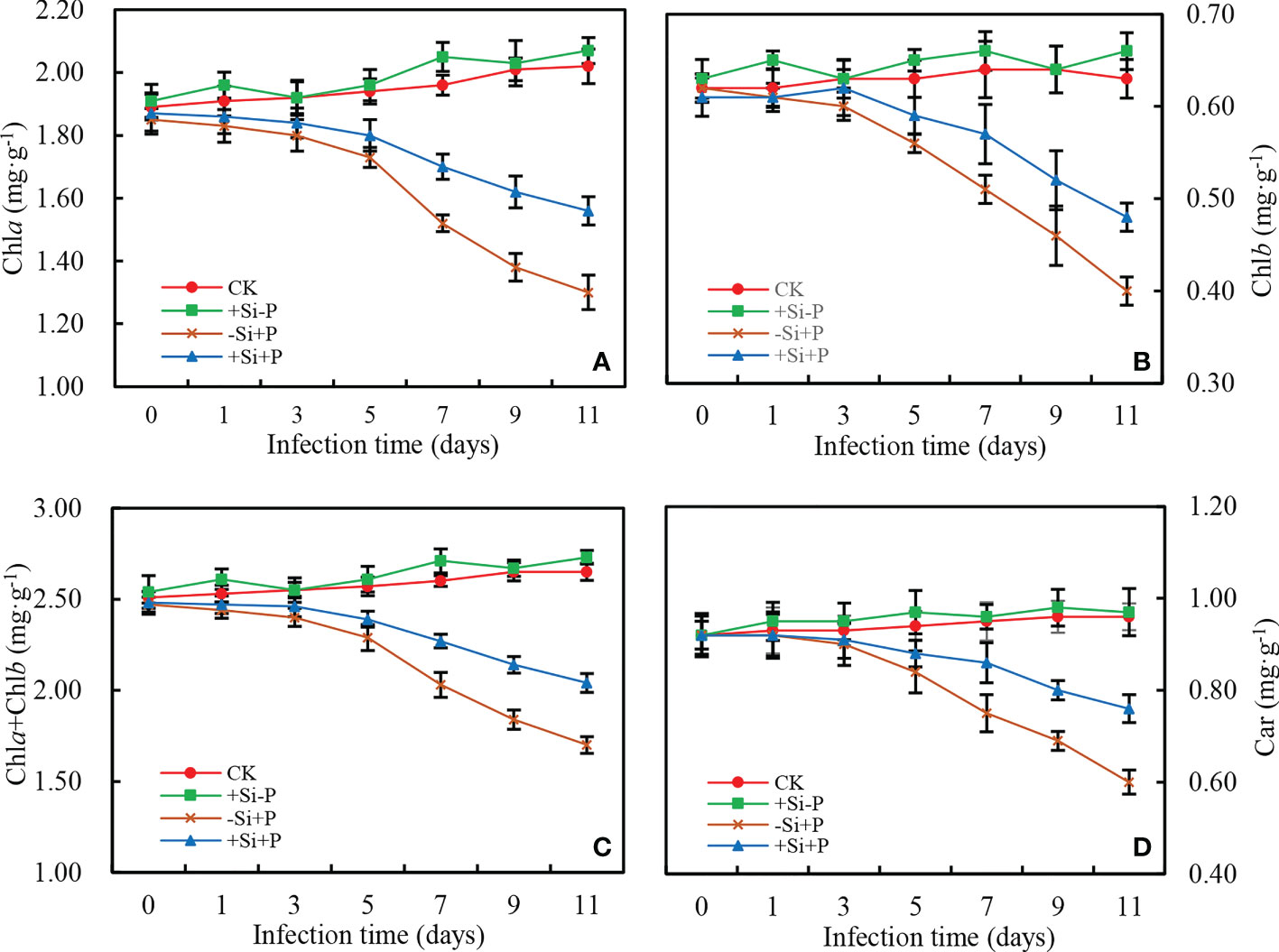
Figure 5 Effects of Si application and Pga inoculation on photosynthetic pigment content of oat leaves. (A), chlorophyll a (Chla); (B), chlorophyll b (Chlb); (C), chlorophyll a+b (Chla+Chlb); (D), total carotenoids (Car). CK, no Si application and no Pga inoculation; +Si-P, 1.5 mmol·L–1 Si application and no Pga inoculation; -Si+P, no Si application and Pga inoculation; +Si+P, 1.5 mmol·L–1 Si application and Pga inoculation.
Chlorophyll fluorescence parameters
As observed in the study, under Pga infection conditions, Si application (+Si+P) lead to the decrease of Fo (Figure 6B) and the increase of Fm (Figure 6C) and changed the trend of OJIP curve (Figure 6A).
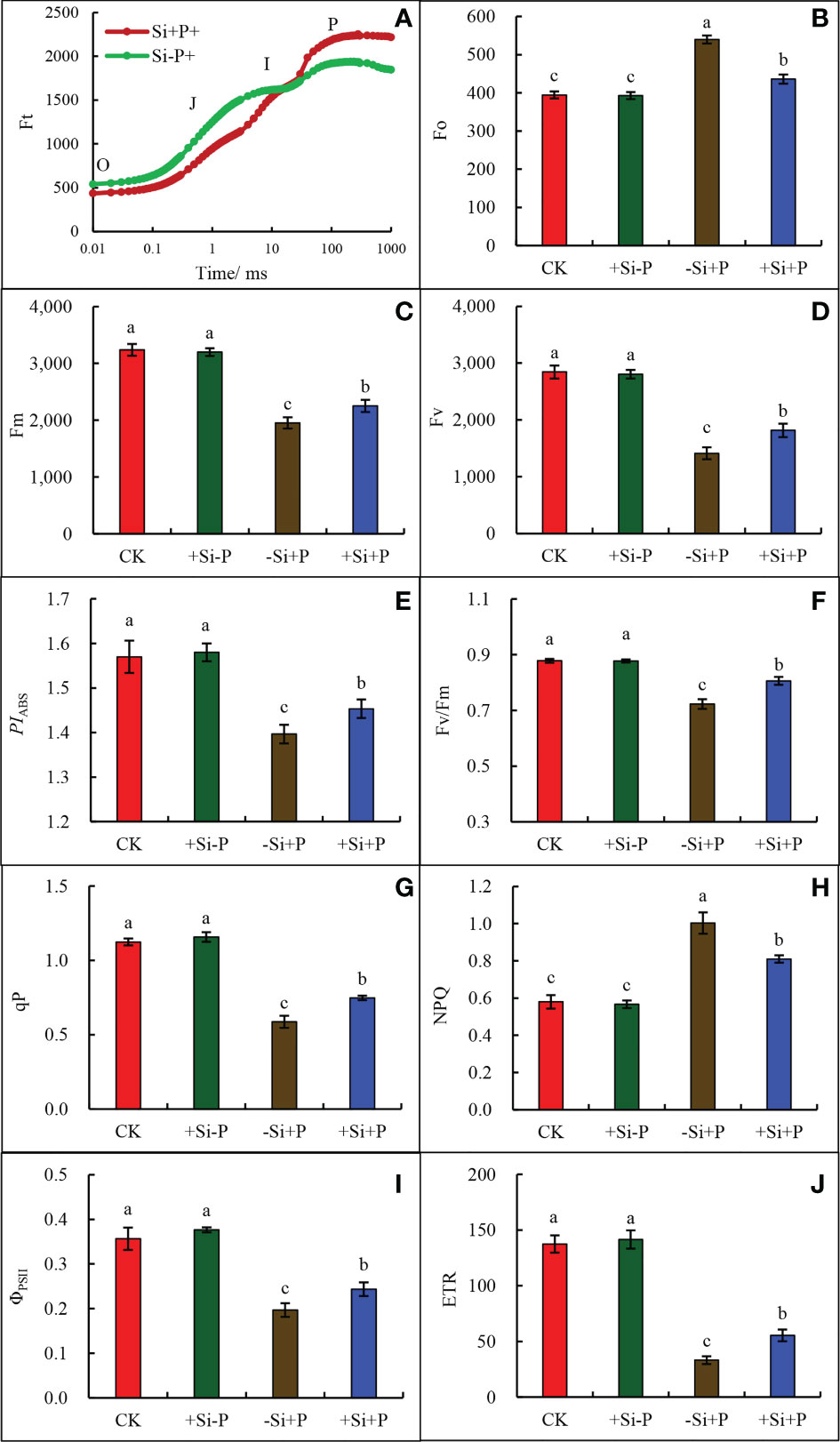
Figure 6 Effects of Si application and Pga inoculation on OJIP curves and Chlorophyll fluorescence parameters of oat leaves. (A), OJIP curve; (B), chlorophyll b (Fo); (C), maximum fluorescence (Fm); (D), variable fluorescence (Fv); (E), photosynthetic performance index (PIABS); (F), maximum quantum yield of PSII (Fv/Fm); (G), Photochemical quenching (qP); (H), non-photochemical quenching (NPQ); (I), actual PSII quantum yield (ΦPSII); (J) electron transport rate (ETR). CK, no Si application and no Pga inoculation; +Si-P, 1.5 mmol·L–1 Si application and no Pga inoculation; -Si+P, no Si application and Pga inoculation; +Si+P, 1.5 mmol·L–1 Si application and Pga inoculation. Data are expressed as mean ± SE (n=3). According to Duncan’s multiple comparison tests among treatments, different letters on bars show significant differences at 0.05 level of probability.
Compared with CK, Pga inoculation (-Si+P) significantly reduced Fm (Figure 6C), Fv (Figure 6D ), PIABS (Figure 6E), Fv/Fm (Figure 6F), qP (Figure 6G), ΦPSII (Figure 6I) and ETR (Figure 6J), which were reduced by 39.7, 50.4, 10.8,18.2, 47.3, 44.4 and 75.9% respectively, while Fo (Figure 6B) and NPQ (Figure 6H) were increased by 36.8 and 71.4%, respectively.
Compared with -Si+P, Si application (+Si+P) increased Fm, Fv, Fv/Fm, PIABS, ETR, qP and ΦPSII by 15.4, 28.6, 12.5, 3.6, 67.0, 27.1 and 20.0%, respectively, while Fo and NPQ were significantly decreased by19.2 and 19.0% (P ≤ 0.05).
Compared with CK, Pga inoculation (-Si+P) significantly reduced ABS/RC (Figure 7A) and DIo/RC (Figure 7B) by 49.5 and 53.3%, respectively; while increased ETo/RC (Figure 7C) and TRo/RC (Figure 7D) by 98.7 and 54.6%, respectively. Compared with -Si+P, Si application (+Si+P) significantly increased ABS/RC and DIo/RC by 35.2 and 55.6%, respectively, while reduced ETo/RC and TRo/RC by 35.8 and 20.2%, respectively (P ≤ 0.05).
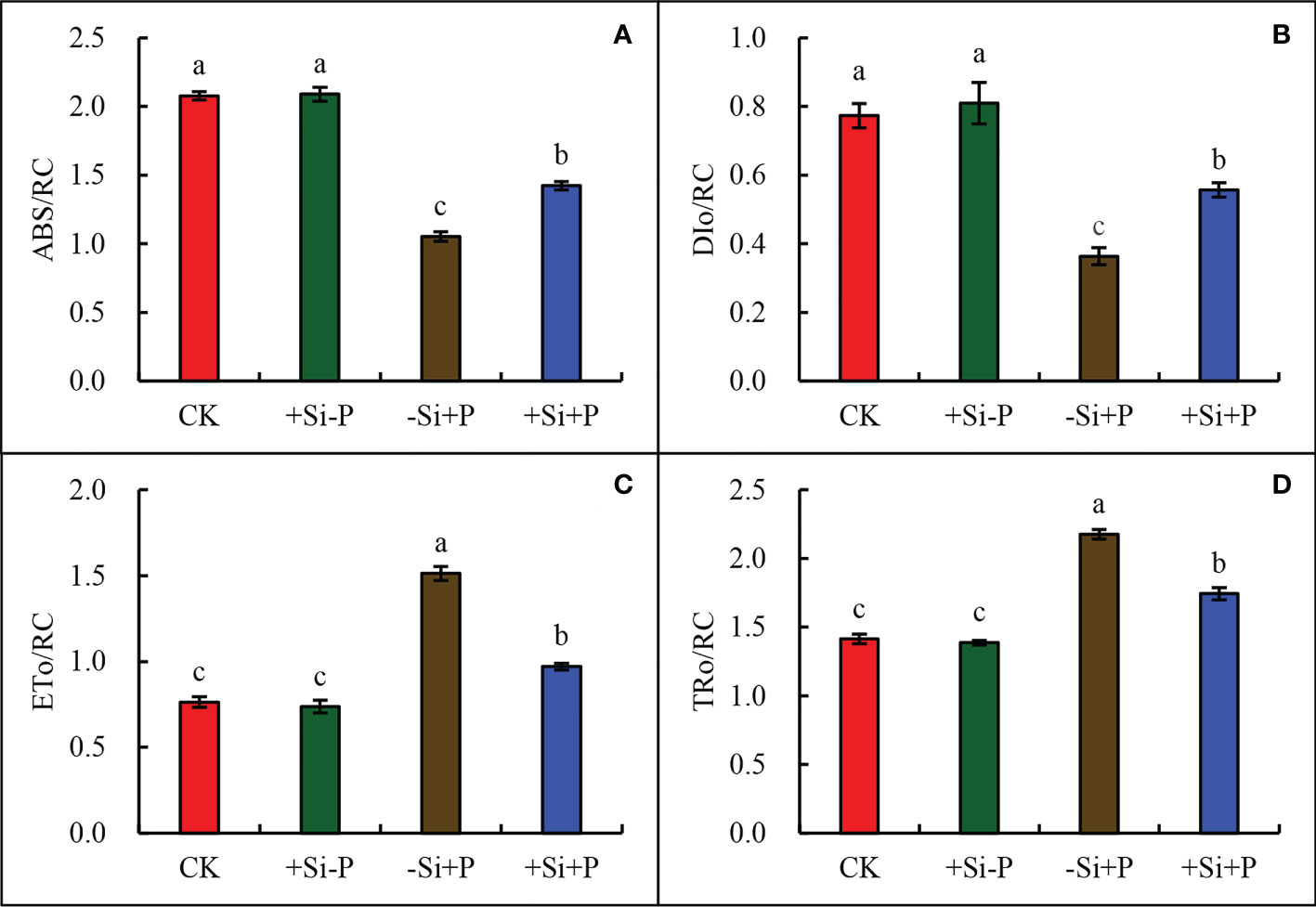
Figure 7 Effect of Si application and Pga inoculation on ABS/RC (A), DIo/RC (B), ETo/RC (C), and TRo/RC (D) of oat leaves. CK, no Si application and no Pga inoculation; +Si-P, 1.5 mmol·L–1 Si application and no Pga inoculation; -Si+P, no Si application and Pga inoculation; +Si+P, 1.5 mmol·L–1 Si application and Pga inoculation. Data are expressed as mean ± SE (n=3). According to Duncan’s multiple comparison tests among treatments, different letters on bars show significant differences at 0.05 level of probability.
Discussion
The present study showed that the leaf photosynthetic capacity sensitively responded to Pga infection and Si application in oat. The results demonstrated that leaf disease symptoms were remarkably reduced in oat leaves supplied with Si (Figure 1). Similarly, wheat could be reduced the rice blast intensity by keeping a high foliar Si concentration (Xavier-Filha et al., 2011). This also supports previous reports (Camargo et al., 2020) and several other plants against foliar pathogens (Fauteux et al., 2005; Domiciano et al., 2010; Resende et al., 2012).
Our study provides new information from a photosynthesis perspective regarding the effect of Si on improving oat tolerance to stem rust. Previous studies have shown that there are two aspects that characterize the possible mechanisms of Si imparting plant tolerance to various diseases. One is Si polymerization and deposition in epidermal cell walls below the cuticle, forming a cuticle-Si double layer in leaves to prevent fungal invasion (Kim et al., 2002; Cai et al., 2008; Hayasaka et al., 2008; Samuels et al., 2010). Another view is that Si can induce the defense responses of plants by increasing the activity of defense-related enzymes such as peroxidase, polyphenoloxidase, phenylalanine ammonia-lyase, etc., and promoting the synthesis of antifungal compounds such as phenolics and phytoalexins (Fawe et al., 1998; Rodrigues et al., 2004; Re´mus-Borel et al., 2005). Traditionally, these positive effects of Si have been associated with alleviating biotic stresses, improving resistance to lodging, and increasing leaves erectness, which allows better light transmittance through plant canopies and enhances whole-plant photosynthesis (Tamai and Ma, 2008).
Necrotrophic pathogens produce many hydrolases that degrade plant cell walls and ultimately have a profound effect on photosynthesis (Scholes and Rolfe, 2009). In our study, due to infection with stem rust, the photosynthetic activity was remarkably impaired, as also noticed on sugarcane leaves infected with brown rust (Camargo et al., 2020). Studies have found that Si increased rice resistance against brown spots was related to the promotion of the primary metabolism of photorespiration (Vivancos et al., 2015).
For the oat-Pga interaction, the reduction of symptoms on the leaves of plants treated with Si improved their gas exchange performance and reduction in the dysfunction at the photochemical level (Aucique-Pérez et al., 2014). Meanwhile, the results of the present study bring new evidence that the photosynthetic machinery of Pga infected oat leaves can be significantly protected when supplied with Si; such protection was related to some preservation of the photosynthetic performance, it is shown as the higher ability to use the incident light {higher values for PIABS (Figure 6E), ABS/RC (Figure 7A), DIo/RC (Figure 7B) and LSP (Figure 4B)}, as well as the partial preservation of chlorophylls and carotenoids contents (Figure 5). Kretschmer et al. (2020) also found that the F. oxysporum generally had negative effects on chlorophyll and carotenoid content in tomato leaves, which was in agreement with our view. Lee et al. (2015) observed that carotenoid or chlorophyll biosynthesis silencing at the phytoene desaturase or Mg-chetalase H steps during wheat infection by the hemibiotrophic fungus Zymoseptoria tritici, resulted in a faster appearance of HR symptoms.
Sampol et al. (2003) first reported that Gs was the main limiting factor on Pn in response to grapevine leaves after infected by the grapevine fan leaf virus (GFLV). To a certain extent, adding Si could indirectly have helped preserve the photosynthetic apparatus’s functionality and the gas exchange capacity upon fungal infection by decreasing rust severity, as noted by the significantly higher values of Pn (Figure 2A), Tr (Figure 2B), Gs (Figure 2C), and Ci (Figure 2D) in the Si application plants under the Pga infection. Regardless of whether Si is applied or not, infected plants may also negatively affect the process of CO2 fixation and decrease their capacity to use solar energy for photosynthesis. This result is shown by non-stomatal limitations, as indicated by declines in Pn but not Ci, despite the decreases in Gs significantly. Si application can enhance plant cell wall, and cell wall thickness is one of the main factors determining the structural components of Gs because higher thickness increases the pathway of CO2 from the intercellular spaces to the chloroplast membrane (Yamamoto et al., 2012).
The fluorescence signal rose from the initial fluorescence level (Fo) (Figure 6B) to the maximum level (Fm) (Figure 6C) with well-defined intermediate J and I step, showing a typical polyphase behavior (OJIP curve) (Figure 6A). These results demonstrated that all samples were photosynthetically active when supplied with Si in response to Pga. Measurements of chlorophyll fluorescence parameters provided important information for PSII activity and changes in photosynthetic metabolism ability of infected leaves (Schnabel et al., 1998). In the current data that we referred to, there is a very little useful information available on the relationship between Si and PSII photochemical efficiency of infected leaves. Our results showed that Pga infection significantly reduced chlorophyll fluorescence parameters, including Fm (Figure 6C), PIABS (Figure 6E), Fv/Fm (Figure 6F), qP (Figure 6G), and ETR (Figure 6J), but Si application significantly increased these parameters in the infected plants. Bassanezi et al. (2002) found that electron transport capacity such as ETR, ETo/RC, generation of ATP and NADPH did not change apparently in the healthy areas of diseased leaves, but chlorophyll fluorescence emission decreased in visibly lesioned areas of bean rust, angular leaf spot, or anthracnose. Rahoutei et al. (2010) pointed out that in both symptomatic and asymptomatic leaves of Nicotiana benthamiana Gray plants, infected with pepper mild mottle virus (PMMoV) and Paprika mild mottle virus (PaMMoV), the ETR in PSII deceased. In addition, the results of the current study showed that Pga infection significantly increased ETo/RC (Figure 6J) while it was significantly reduced after the application of Si. Bonfig et al. (2006) reported that non-photochemical quenching (NPQ) in Arabidopsis leaves was decreased after being infected with either a virulent or an avirulent strain of Pseudomonas syringae. However, the NPQ in oat leaves was found to be increased significantly after the infection of Pga (Figure 6H), which demands further studies to elucidate the cause of this.
In addition, the experimental results showed that the ETR (Figure 6J) reduction is much lower than Pnmax (Figure 4A), coupled with the lower LSP (Figure 4B), which are expected to generate a photoinhibition event. It explains the performance of the Pn/PAR (Figure 3A) response curve reasonably in this study, especially for the infected plants without Si application treatment. In fact, the diseased plants are prone to suffer from photoinactivation, which may lead to oxidative damage and losses of PSII functionality, ultimately leading to increased Fo (Figure 6B) values (Baker, 2008; Rolfe and Scholes, 2012).
Conclusions
The results from the present study indicated that Si application in oat could promote plant growth and enhance plant tolerance to stem rust and improve its photosynthetic performance. During Pga infection with Si addition, adequate Gs and Pn values were maintained, which helped to protect the photosynthetic system against chronic photoinhibition. Under Pga inoculation, Si application increased pigment content and made it more efficient in the process of light energy dissipation such as Fv/Fm, PIABS, ABC/RC and DIo/RC. Our findings also suggest that gas exchange properties and photochemical functions are involved in the Si-mediated amelioration of oats to stem rust.
Data availability statement
The original contributions presented in the study are included in the article/supplementary material. Further inquiries can be directed to the corresponding author.
Author contributions
JL conceived and supervised the experiments. YL performed the experiments, contributed to data analysis, and wrote the paper. BZ, JM, and PL gave valuable advice for the modifications of the paper. All authors contributed to the article and approved the submitted version.
Funding
This study was supported by National Key R&D Program of China (2018YFE0107900) and National Modern Agricultural Industry Technology System (CARS-07).
Conflict of interest
The authors declare that the research was conducted in the absence of any commercial or financial relationships that could be construed as a potential conflict of interest.
Publisher’s note
All claims expressed in this article are solely those of the authors and do not necessarily represent those of their affiliated organizations, or those of the publisher, the editors and the reviewers. Any product that may be evaluated in this article, or claim that may be made by its manufacturer, is not guaranteed or endorsed by the publisher.
References
Alminger, M., Eklund-Jonsson, C. (2008). Whole-grain cereal products basedon a high fibre barley or oat genotype lower post-prandial glucose and insulin responses in healthy humans. Eur. J. Nutr. 47 (6), 294–300. doi: 10.1007/s00394-008-0724-9
Aucique-Pe´rez, C. E., Rodrigues, F. A., Moreira, W. R., DaMatta, F. M. (2014). Leaf gas exchange and chlorophyll a fluorescence in wheat plants supplied with silicon and infected with pyricularia oryzae. Phytopathology 104, 143–149. doi: 10.1094/PHYTO-06-13-0163-R
Baker, N. R. (2008). Chlorophyll fluorescence: A probe of photosynthesis in vivo. Annu. Rev. Plant Biol. 59, 89–113. doi: 10.1146/annurev.arplant.59.032607.092759
Bassanezi, R. B., Amorim, L., Filho, A. B., Berger, R. D. (2002). Gas exchange and emission of chlorophyll fluorescence during the monocycle of rust, angular leaf spot and anthracnose on bean leaves as a function of their trophic characteristics. J. Phytopathol. 150, 37–47. doi: 10.1046/j.1439-0434.2002.00714.x
Bonfig, K. B., Schreiber, U., Gabler, A., Roitsch, T., Berge, S. (2006). Infection with virulent and avirulent p. syringae strains differentially affects photosynthesis and sink metabolism in arabidopsis leaves. Planta 225, 1–12. doi: 10.1007/s00425-006-0303-3
Cai, K. Z., Gao, D., Luo, S. M., Zeng, R. S., Yang, J. Y., Zhu, X. Y. (2008). Physiological and cytological mechanisms of silicon-induced resistance in rice against blast disease. Physiol. Plantarum 134, 324–333. doi: 10.1111/j.1399-3054.2008.01140.x
Camargo, M. S., Coutinho, I. D., Lourenço, S. A., Soares, M. K. M., Colnago, L. A., da-Glória, B. A., et al. (2020). Potential prophylactic role of silicon against brown rust (Puccinia melanocephala) in sugarcane. Eur. J. Plant Pathol. 157, 77–88. doi: 10.1007/s10658-020-01982-2
Chen, Y. E., Cui, J. M., Su, Y. Q., Yuan, S., Yuan, M., Zhang, H. Y. (2015). Influence of stripe rust infection on the photosynthetic characteristics and antioxidant system of susceptible and resistant wheat cultivars at the adult plant stage. Front. Plant Sci. 6. doi: 10.3389/fpls.2015.00779
Debona, D., Rodrigues, F. A., Datnoff, L. E. (2017). Silicon’s role in abiotic and biotic plant stresses. Annu. Rev. Phytopathol. 55, 85–107. doi: 10.1146/annurev-phyto-080516-035312
Domiciano, G. P., Cacique, I. S., Freitas, C. C., Filippi, M. C. C., DaMatta, F. M., Vale, F. X. R., et al. (2015). Alterations in gas exchange and oxidative metabolism in rice leaves infected by pyricularia oryzae are attenuated by silicon. Phytopathology 105, 738–747. doi: 10.1094/PHYTO-10-14-0280-R
Domiciano, G. P., Rodrigues, F. A., Vale, F. X. R., Xavier, M. S., Moreira, W. R., Andrade, C. C. L., et al. (2010). Wheat resistance to spot blotch potentiated by silicon. J. Phytopathol. 158, 334–343. doi: 10.1111/j.1439-0434.2009.01623.x
Epstein, E. (2009). Silicon: Its manifold roles in plants. Ann. Appl. Biol. 155, 155–160. doi: 10.1111/j.1744-7348.2009.00343.x
Fauteux, F., Remus-Borel, W., Menzies, J. G., Bélanger, R. R. (2005). Silicon and plant disease resistance against pathogenic fungi. FEMS Microbiol. Lett. 249, 1–6. doi: 10.1016/j.femsle.2005.06.034
Fawe, A., Abou-Zaid, M., Menzies, J. G., Be´langer, R. R. (1998). Silicon-mediated accumulation of flavonoid phytoalexins in cucumber. Phytopathology 88, 396–401. doi: 10.1094/PHYTO.1998.88.5.396
Frew, A., Weston, L. A., Reynolds, O. L., Gurr, G. M. (2018). The role of silicon in plant biology: A paradigm shift in research approach. Ann. botany 121, 1265–1273. doi: 10.1093/aob/mcy009
Gold Steinberg, J., Mitchell Fetch, J., Fetch, T. G., Jr. (2005). Evaluation of avena spp. accessions for resistance to oat stem rust. Plant Dis. 89, 521–525. doi: 10.1094/PD-89-0521
Hayasaka, T., Fujii, H., Ishiguro, K. (2008). The role of silicon inpreventing appressorial penetration by the rice blast fungus. Phytopathology 98, 1038–1044. doi: 10.1094/PHYTO-98-9-1038
Jiang, J. F., Li, J. G., Yuan, H. D. (2013). Effect of calcium nutrition on resistance of tomato against bacterial wilt induced by ralstoniasolanacearum. Eur. J. Plant Pathol. 136, 547–555. doi: 10.1007/s10658-013-0186-7
Kim, S. G., Kim, K. W., Park, E. W., Choi, D. (2002). Silicon-induced cell wall fortification of rice leaves: A possible cellular mechanism of enhanced host resistance to blast. Phytopathology 92, 1095–1103. doi: 10.1094/PHYTO.2002.92.10.1095
Kretschmer, M., Damoo, D., Djamei, A., Kronstad, J. (2020). Chloroplastsand plant immunity: where are the fungal effectors? Pathogens 9, 19. doi: 10.3390/pathogens9010019
Lee, W. S., Devonshire, B. J., Hammond-Kosack, K. E., Rudd, J. J., Kanyuka, K. (2015). Deregulation of plant cell death through disruption of chloroplast functionality affects asexual sporulation of zymoseptoria tritici on wheat. Mol. Plant-Microbe Interact. 28, 590–604. doi: 10.1094/MPMI-10-14-0346-R
Lichtenthaler, H. K. (1987). Chlorophyll and carotenoids: pigments of photosyntheticbiomembranes. Methods Enzymol. 148, 350–382. doi: 10.1016/0076-6879(87)48036-1
Li, X., Liu, T. G., Chen, W. Q., Zhong, S. F., Zhang, H. Y., Tang, Z. X., et al. (2015). Wheat WCBP1 encodes a putative copper-binding protein involved in stripe rust resistance and inhibition of leaf senescence. BMC Plant Biol. 15, 239–254. doi: 10.1186/s12870-015-0612-4
Li, T. Y., Wu, L. X., Wang, H. (2014). Physiological race and virulence analysis of oat stem rust fungus in my country. J. Triticeae Crops 34, 552–556. doi: 10.7606/j.issn.1009-1041.2014.04.19
Lugtenberg, B. (2015). “Principles of plant-microbe interactions,” in Microbes for sustainable agriculture (Life of Microbes in the Rhizosphere: Springer Nature), 17–24. doi: 10.1007/978-3-319-08575-3_3
Luyckx, M., Hausman, J. F., Lutts, S., Guerriero, G. (2017). Silicon and plants: Current knowledge and technological perspectives. Front. Plant Sci. 8. doi: 10.3389/fpls.2017.00411
Maxwell, K., Johnson, G. N. (2000). Chlorophyll fluorescence-a prac-tical guide. J. Exp. Bot. 51, 659–668. doi: 10.1093/jexbot/51.345.659
Nazare, J. A., Normand, S., Oste Triantafyllou, A., Brac de la Perri`ere, A., Desage, M., Laville, M. (2009). Modulation of the postprandial phase by β-glucan in overweight subjects: effects on glucose and insulin kinetics. Mol. Nutr. Food Res. 53, 361–369. doi: 10.1002/mnfr.200800023
Rahoutei, J., Garcı á-Luque, I., Baro´n, M. (2010). Inhibition of photosynthesis by viral infection: effect on PSII structure and function. Physiol. Plantarum 110, 286–292. doi: 10.1034/j.1399-3054.2000.110220.x
Re´mus-Borel, W., Menzies, J. G., Be´langer, R. R. (2005). Silicon induce santifungal compounds in powdery mildew-infected wheat. Physiol. Mol. Plant Pathol. 66, 108–115. doi: 10.1016/j.pmpp.2005.05.006
Resende, R. S., Rodrigues, F. A., Cavatte, P. C., Martins, S. C. V., Moreira, W. R., Chaves, A. R. M., et al. (2012). Leaf gas exchange and oxidative stress in sorghum plants supplied with silicon and infected by colletotrichum sublineolum. Phytopathology 102, 892–898. doi: 10.1094/PHYTO-01-12-0014-R
Rodrigues, F. A., McNally, D. J., Datnoff, L. E., Jones, J. B., Labbe, C., Benhamou, N., et al. (2004). Silicon enhancesthe accumulation of diterpenoid phytoalexins in rice: A potential mechanism for blast resistance. Phytopathology 94, 177–183. doi: 10.1094/PHYTO.2004.94.2.177
Rolfe, S. A., Scholes, J. D. (2012). Chlorophyll fluorescence imaging of plant-pathogen interactions. Protoplasma 247, 163–175. doi: 10.1007/s00709-010-0203-z
Sampol, B., Bota, J., Riera, D., Medrano, H., Flexas, J. (2003). Analysis of the virus-induced inhibition of photosynthesis in malmsey grapevines. New Phytol. 160, 403–412. doi: 10.1046/j.1469-8137.2003.00882.x
Samuels, A. L., Glass, A. D. M., Ehret, D. L., Menzies, J. G. (2010). Mobility anddeposition of silicon in cucumber plants. Plant Cell Environ. 14, 485–492. doi: 10.1111/j.1365-3040.1991.tb01518.x
Schnabel, G., Strittmatter, G., Noga, G. (1998). Changes in photosynthetic electron transport in potato cultivars with different field resistance after infection with phytophthora infestans. J. Phytopathol. 146, 205–210. doi: 10.1111/j.1439-0434.1998.tb04681.x
Scholes, J. D., Rolfe, S. A. (2009). Chlorophyll fluorescence imaging as a tool for understanding the impact of fungal diseases on plant performance. Funct. Plant Biol. 36, 80–892. doi: 10.1071/FP09145
Tamai, K., Ma, J. F. (2008). Reexamination of silicon effects on rice growth and production under field conditions using a low silicon mutant. Plant Soil 307, 21–27. doi: 10.1007/s11104-008-9571-y
Tatagiba, S. D., DaMatta, F. M., Rodrigues, F. A. (2015). Leaf gas exchange and chlorophyll a fluorescence imaging of rice leaves infected with monographella albescens. Phytopathology 105, 180–188. doi: 10.1094/PHYTO-04-14-0097-R
Vivancos, J., Labbé, C., Menzies, J. G., Bélanger, R. R. (2015). Silicon-mediated resistance of arabidopsis against powdery mildew involves mechanisms other than the salicylic acid (SA)-dependent defence pathway. Mol. Plant Pathol. 16, 572–582. doi: 10.1111/mpp.12213
Whitehead, A., Beck, E. J., Tosh, S., Wolever, T. M. S. (2014). Cholesterol-lowering effects of oat β-glucan: A meta-analysis of randomized controlled trials. Am. J. Clin. Nutr. 100, 1413–1421. doi: 10.3945/ajcn.114.086108
Xavier-Filha, M. S., Rodrigues, F. A., Domiciano, G. P., Oliveira, H. V., Silveira, P. R., Moreira, W. R. (2011). Wheat resistance to leaf blast mediated by silicon. Australas. Plant Pathol. 40, 28–38. doi: 10.1007/s13313-010-0010-1
Yamamoto, T., Nakamura, A., Iwai, H., Ishii, T., Ma, J. F., Yokoyama, R., et al. (2012). Effect of silicon deficiency on secondary cell wall synthesis in rice leaf. J. Plant Res. 125, 771–779. doi: 10.1007/s10265-012-0489-3
Yang, C., Zhang, Z. S., Gao, H. Y., Liu, M. J., Fan, X. L. (2014). Mechanisms by which the infection of sclerotinia sclerotiorum (Lib.) de bary affects the photosynthetic performance in tobacco leaves. BMC Plant Biol. 14. doi: 10.1186/s12870-014-0240-4
Yuan, J. H., Cao, L. X., Zhang, L. J. (2014). Evaluation of 100 oat germplasm for stem rust resistance. J. Henan Agric. Sci. 43, 89–92. doi: 10.15933/j.cnki.1004-3268.2014.01.023
Zhao, B. P., Ma, B. L., Hu, Y. G., Liu, J. H. (2020). Source-sink adjustment: A mechanistic understanding of the timing and severity of drought stress on photosynthesis and grain yields of two contrasting oat (Avena sativa l.) genotypes. J. Plant Growth Regul. 40, 263–276. doi: 10.1007/s00344-020-10093-5
Keywords: oat, stem rust, silicon, photosynthesis, photosystem II
Citation: Li Y, Liu J, Lv P, Mi J and Zhao B (2022) Silicon improves the photosynthetic performance of oat leaves infected with Puccinia graminis f. sp. avenae. Front. Plant Sci. 13:1037136. doi: 10.3389/fpls.2022.1037136
Received: 05 September 2022; Accepted: 07 November 2022;
Published: 23 November 2022.
Edited by:
Shiwen Wang, Northwest A&F University, ChinaReviewed by:
Renato De Mello Prado, São Paulo State University, BrazilLina Yin, Northwest A&F University, China
Copyright © 2022 Li, Liu, Lv, Mi and Zhao. This is an open-access article distributed under the terms of the Creative Commons Attribution License (CC BY). The use, distribution or reproduction in other forums is permitted, provided the original author(s) and the copyright owner(s) are credited and that the original publication in this journal is cited, in accordance with accepted academic practice. No use, distribution or reproduction is permitted which does not comply with these terms.
*Correspondence: Jinghui Liu, Y2F1bGpoQGFsaXl1bi5jb20=