- 1The Research Institute of Subtropical Forestry, Chinese Academy of Forestry, Hangzhou, China
- 2National Engineering Research Center of Tree breeding and Ecological remediation, College of Biological Sciences and Biotechnology, Beijing Forestry University, Beijing, China
- 3Chinese Academy of Sciences, Kunming Institute of Botany, Kunming, China
- 4Royal Botanic Gardens, Kew, Wakehurst, West Sussex, United Kingdom
Germplasm conservation strongly depends on the desiccation tolerance (DT) of seeds. Xerophytic seeds have strong desiccation resistance, which makes them excellent models to study DT. Although some experimental strategies have been applied previously, most methods are difficult to apply to xerophytic seeds. In this review, we attempted to synthesize current strategies for the study of seed DT and provide an in-depth look at Caragana korshinskii as an example. First, we analyze congenital advantages of xerophytes in the study of seed DT. Second, we summarize several strategies used to study DT and illustrate a suitable strategy for xerophytic species. Then, based on our previous studies work with C. korshinskii, a feasible technical strategy for DT re-establishment is provided and we provide illustrate some special molecular mechanisms seen in xerophytic seeds. Finally, several steps to unveil the DT mechanism of xerophytic seeds are suggested, and three scientific questions that the field should consider are listed. We hope to optimize and utilize this strategy for more xerophytic species to more systematically decipher the physiological and molecular processes of seed DT and provide more candidate genes for molecular breeding.
Introduction
The safe conservation of plant genetic resources is essential for breeding programs and is an underpinning technology to guarantee adequate food supplies for future human generations (Villalobos and Engelmann, 1995; Gonçalves et al., 2019). Such conservation, with the aim of sustainable utilization, can be realized through several forms of germplasm, such as seeds, living plants in germplasm nurseries, and plant tissue in vitro (Dinato et al., 2020). Among these, seed storage is the most effective and cost-efficient means of long-term ex-situ preservation of genetic diversity for both crops and wild plant diversity (Chapman et al., 2018; Wade et al., 2020).
Large-scale ex-situ seed banking was first implemented in the early 20th century to maintain and protect crop lines and over the last 50 years has been increasingly used to preserve wild plants diversity too (Chapman et al., 2018; Wade et al., 2020). As back-up sites for the global conservation system, seed vaults complement in situ conservation and play a functional and symbolic role for enhanced integration and cooperation to conserve crop and wild plant diversity (Li and Pritchard, 2009; Westengen et al., 2013). Hitherto, almost 57,000 taxa are preserved in more than 350 institutions around the world (Chapman et al., 2018), such as the Svalbard Global Seed Vault for crops in Norway (Asdal and Guarino, 2018), the Royal Botanic Gardens Kew’s Millennium Seed Bank for wild species in the United Kingdom (Liu et al., 2018), the National Plant Germplasm System for crops in the United States of America (Byrne et al., 2018), and the Germplasm Bank of Wild Species (GBoWS) in China (Li et al., 2010).
A critical early step in seed banking is drying the material to a moisture content low enough to avoid ice formation on storage at sub-zero temperatures. However, different species produce seeds with varying levels of desiccation tolerance (DT). When considering DT, seeds are divided into orthodox, intermediate and recalcitrant (Roberts, 1973; Ellis et al., 1990; Ballesteros et al., 2021). Orthodox seeds, include those of many crops, can tolerate desiccation to 3-7% moisture content and potentially survive ex situ storage for many years under conventional gene-bank conditions of -20°C (Stanwood, 1989; Sara et al., 2014; Colville and Pritchard, 2019). However, about 8% of the world’s flowering plants are estimate to have seeds that are intolerant to drying (Wyse and Dickie, 2017). Such recalcitrant seeds are often sensitive to drying below c. 35% moisture content and tend to be a feature of wet forest species, including cocoa, coconut, rubber tree, oaks and dipterocarps (Pammenter and Berjak, 1999; Black and Pritchard, 2002). In contrast, intermediate seeds have partial DT (e.g., to c. 10% moisture content) and may undergo a precipitous loss of viability when stored at -20°C. Examples include economically valuable species such as tea, oil palm, citrus and coffee (Dussert et al., 2018). This interplay between drying level and storage temperature applies beyond intermediate seeds. For example, desiccation and cryopreservation (often in liquid nitrogen at -196°C) is an applicable technology for germplasm conservation as long as the seeds are not ultradry, as deep cooling can then lead to structural instability (Li and Pritchard, 2009; Ballesteros et al., 2021). Alternatively, there is interest in storing seeds ultra-dry without cooling as this can reduce the cost of seed storage, e.g., in the tropics (Zheng et al., 1998), Thus seed DT is a complex function of the level of water loss, the kinetics of dehydration, the drying time duration and any interaction with storage temperature.
More generally in plant sciences, DT is widely defined as the ability of an organism to withstand or endure extreme dryness or dehydration-like conditions (Black and Pritchard, 2002; Oliver et al., 2020), and unveiling the molecular mechanism of DT offers the prospect by genetic engineering of identifying and using novel DT genes for crop improvement and enhancing the storability of recalcitrant seeded species.
Xerophytic seeds can germinate in arid and semi-arid regions, and even in deserts. They must evolutionarily develop resistance mechanisms to adapt or tolerate stress to survive in dry, sandy environments (Gong et al., 2015). Hence, they logically provide a potentially excellent experimental material to explore DT mechanisms. However, due to sampling collection difficulties, which required long experimental periods, and other reasons, these species have been mainly overlooked. Consequently, such mechanisms of stress tolerance and resilience during the early stage of germination in xerophytic seed plants are primarily uncharted.
This review article synthesizes current evidence in order to decipher the potential DT mechanisms of dry plant seeds. Firstly, we describe the favorable aspects of xerophytic species’ seeds in DT studies, particularly in relation to the re-establishment of DT in germinating seeds. After reviewing recent progress in this research area, we take Caragana korshinskii seeds as a specific example of the functional response to the re-establishment of DT. This strategy may be suitable for exploring the mechanisms of DT in xerophytic seeds.
Subsections relevant for the subject
Congenital advantages of xerophytes in the study of seed DT
Dryland ecosystems are ecologically distinct, increasing in global extent under shifting climates and have been recognized as degraded in over 50% of their range (Shackelford et al., 2021). Various ecosystem assessments have indicated this is a threatened habitat and intervention is needed (Louhaichi et al., 2014; Pérez et al., 2019; Miguel et al., 2020; Shackelford et al., 2021). For this, it is best to use native species, particularly shrubby xerophytes. In the drylands of northwestern China, several drought‐resistant plant species have been widely planted since the 1970s (Yu and Wang, 2018). The selected species are pioneer xerophytic plants, such as Haloxylon ammodendron (Fan L et al., 2018), Hedysarum scoparium (Su et al., 2016), Hippophae rhamnoides (Ghangal et al., 2012), Zygophyllum xanthoxylon (Shi and Zhang, 2015), Calligonum mongolicum (Fan B et al., 2018), and Caragana korshinskii (Yang et al., 2014). They have broad functionality relating to soil and water conservation, desertification prevention and control, and vegetation restoration in arid and semiarid regions (Yu and Wang, 2018). Xerophytic plants are often exposed to stressful conditions, such as water deficit, dry air, high irradiance, and extreme temperatures, including during the germination phase of the life cycle.
With the development of molecular biology and omics, i.e., transcriptome, and proteome and metabolome, drought-resistance in some model plants and crops has already been thoroughly elucidated (Zhu, 2016; Qi et al., 2018; Gong et al., 2020). Adopting these technical strategies, we can decipher the mechanism of DT of xerophytic plant seeds and identify high-quality candidate genes for improving DT. Opportunities to do this are greatest with representatives of the families for which there are the most published plant genomes, and the top three families are Poaceae, Brassicaceae, and Fabaceae (Sun et al., 2022). Caragana korshinskii is a member of the Fabaceae for which there have been around 50 sequenced genomes (Sun et al., 2022). Furthermore, several seeds of species in Fabaceae relate to the study of seed DT like Pisum sativum, Sesbania virgate, Medicago truncatula, and Vigna unguiculata (Buitink et al., 2003; Leprince et al., 2004; Faria et al., 2005; Boudet et al., 2006; Buitink et al., 2006; Terrasson et al., 2013; Masetto et al, 2014; Masetto et al, 2016; Silva et al., 2017; Wang et al., 2021). Among them, DT in seeds of M. truncatula is well studied (Buitink et al., 2003; Leprince et al., 2004; Faria et al., 2005; Boudet et al., 2006; Buitink et al., 2006; Terrasson et al., 2013). These all position Caragana as the species complementary to Medicago.
Review of research strategies used by DT studies
According to genetic background of species, and the physiological change during seed development and germination, some strategies have been developed in previous studies to increase understanding of the mechanism(s) of seed DT and simultaneously have screened potential DT relative genes (Figure 1). Here, we introduce the principles and scope of application of these strategies.
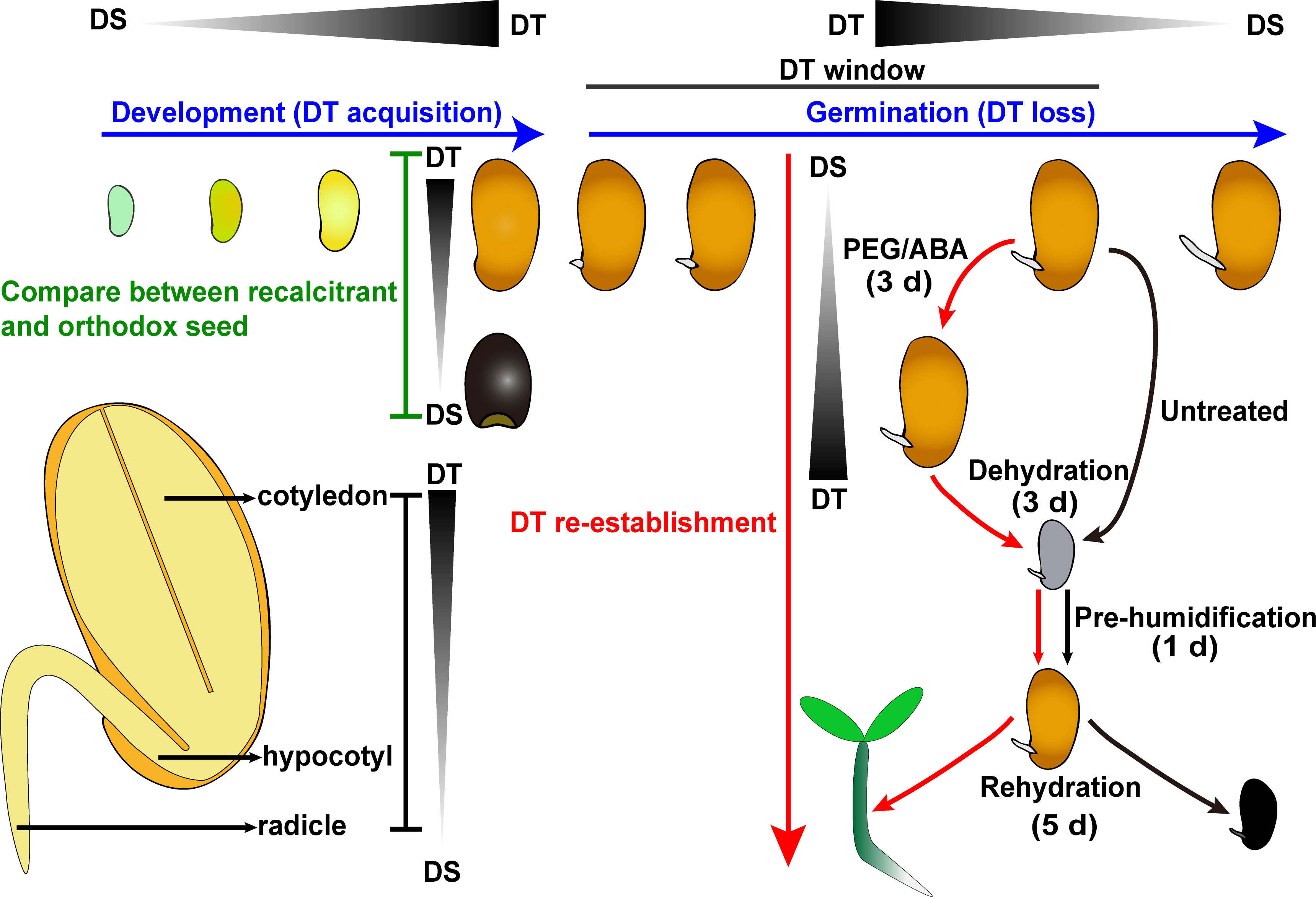
Figure 1 Schematic summary of the strategies used within DT studies in seed. Comparative analysis between orthodox and recalcitrant seeds is a handy strategy for depicting the character of DT (green words). Moreover, DT acquisition and loss accompanies the physiological processes of seed development and germination, respectively (blue words). When germinating seeds at the stage of radicle protrusion are fast dried (black arrows, 3 d), they do not survive, as shown by the lack of seedling growth after pre-humidification and rehydration. If drying treatment is preceded by PEG/ABA treatment (red arrows), after pre-humidification, seeds survive, as shown by further growth and seedling establishment. Emphasizing a PEG/ABA treatment can re-establish tolerance against desiccation treatment (red words). The detailed process of DT re-establishment of C. korshinskii seed is as follows: Seeds are germinated for 12 h, and then dried either directly or after 3 d of incubation in PEG 6000 solution of –1.7 Mpa at 10°C. After incubation, germinating seeds are rinsed thoroughly in distilled water and then dehydrated in silica gel for 3 d, pre-humidified for 1 d, and imbibed for 5 d. The total duration of the experiment is about 2 weeks. After PEG treatment, 81% of radicles recovered DT, which means that C. korshinskii seed radicle successfully re-establish DT (Peng et al., 2017). In addition, the radicles appear to be the most desiccation-sensitive part of germinating seeds, followed by the hypocotyls and cotyledons (the lower left part). A triangle with a gradient of black and grey indicates the DT transition. DT, desiccation tolerance; DS, desiccation-sensitive.
Comparing orthodox and recalcitrant seeds
Unlike orthodox types, recalcitrant seeds are highly sensitive to desiccation (Pammenter and Berjak, 1999; Dussert et al., 2018). On account of the DT differences, comparative proteomic and transcriptomic analysis between orthodox and recalcitrant seeds has proved to be a useful strategy for depicting the character of DT (Pukacka and Ratajczak, 2007; Delahaie et al., 2013; Ratajczak et al., 2013). The con-generic species Norway maple (Acer platanoides) and sycamore (A. pseudoplatanus), which can grow under similar climatic conditions, produce seeds with different tolerances to desiccation (Pukacka and Ratajczak, 2007). In these two species, changes in physiological or epigenetic processes mirror the response of the seeds to severe desiccation, such as changes in the antioxidant system and in the genomic 5-methylcytosine level (Pukacka et al., 2011; Ratajczak et al., 2013; Plitta-Michalak et al., 2018; Stolarska et al., 2020; Wojciechowska et al., 2020).
Inter-species comparison of seeds with different levels of DT at the point of natural dispersal provides a direct way of screening the frequency of DT in the plant kingdom (Pritchard et al., 2004). However, such an approach on species with diverse genetic background and phenotypic plasticity may be impacted by seed provenance and the maternal environment. For example, DT level varies significantly in seed lots of the recalcitrant-seeded species Aesculus hippocastanum when collected from sites across Europe (Daws et al., 2010). Thus, without knowing the precise stage of development in two species, inferences about physiological processes may be misleading and irrelevant genes inadvertently revealed (Table 1). Even if the genotype variation within the same genus is reduced to a minimum, i.e. relatedness is maximised, species-specific genes are still not guaranteed to be associated with DT. Thus, due to species-specific influences, comparing differences between recalcitrant and orthodox seeds cannot accurately elucidate the mechanism of DT (Lang et al., 2014).
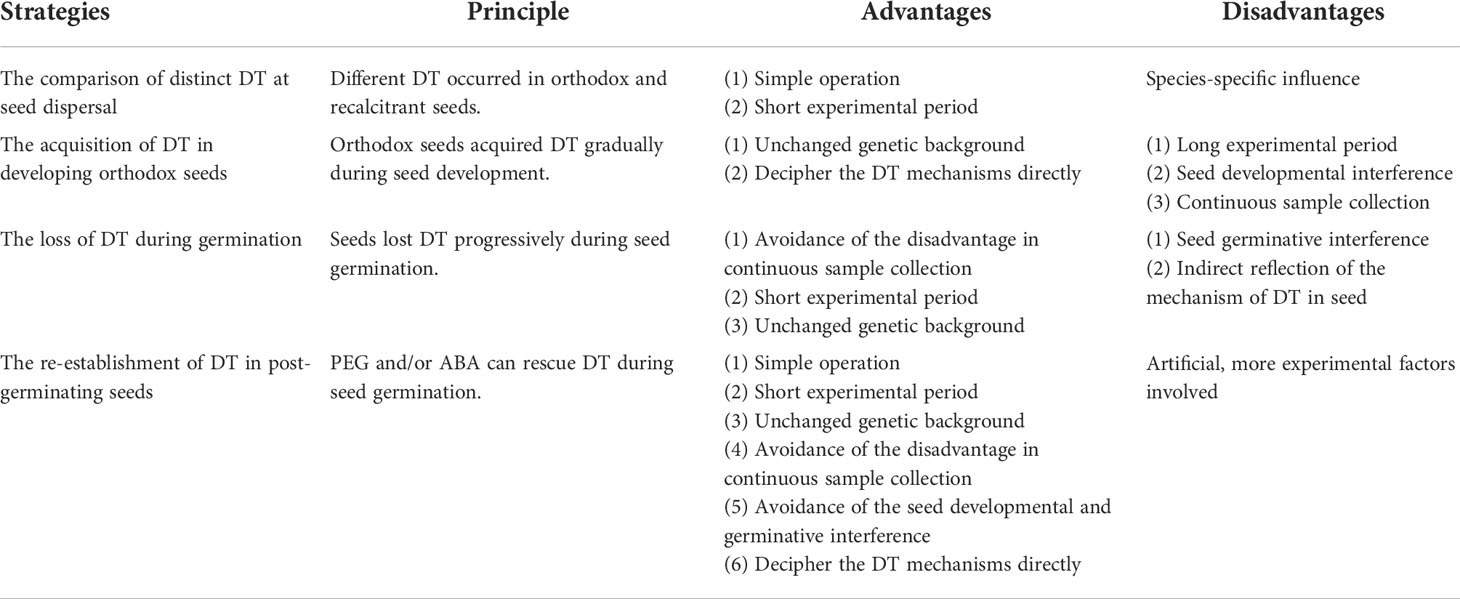
Table 1 Principle, advantages, and disadvantages of strategies for studying the mechanism of DT in seeds.
DT acquisition during orthodox seed maturation
During the development of orthodox seeds, water loss occurs gradually near the end of the accumulation of storage compounds, while within a similar time window, DT is acquired gradually (Li et al., 2011; Huang and Song, 2013; Lang et al., 2014; Peng et al., 2021). The transition of developing seeds from the phase of reserve accumulation to maturation drying is associated with distinct gene expression and metabolic switches, which are strongly coupled with longevity and impact seed storage (Fait et al., 2006; Angelovici et al., 2010; Chatelain et al., 2012; Verdier et al., 2013). In this phase, the synthesis of storage and protection proteins, such as late embryogenesis abundant (LEA) proteins, heat shock proteins (HSPs), and proteins involved in stress defense (antioxidant system and secondary metabolites), serve as shields to prevent cellular collapse during low water activities (Tunnacliffe and Wise, 2007; Huang et al., 2012; Almoguera et al., 2015; Lang et al., 2017).
Non-reducing sugars, such as sucrose and oligosaccharides are crucial protective compounds in the response of seeds to desiccation stress. They play a prominent role in protecting the tissues via water replacement and vitrification during seed dehydration (Black et al., 1999; Li et al., 2011; Lang et al., 2017; Leprince et al., 2017; Jing et al., 2018). The efficacy of these defense networks and their targets during desiccation and rehydration are well documented in plants (Hoekstra et al., 2001; Zhang and Bartels, 2018; Oliver et al., 2020). Furthermore, down-regulation of genes involved in the cell cycle, DNA processing, and primary metabolism free up more energy for resistance to dehydration stress (Buitink et al., 2006; Franchi et al., 2011; Maia et al., 2011; Oliver et al., 2020). With the arrest of growth, the production of reactive oxygen species (ROS) is decreased to a minimum, which minimizes damage to the cells.
These observations support the idea that physiological and molecular processes in seed DT seem to be able to provide suitable strategies for studying DT in seeds. However, the emergence of DT acquisition overlaps with seed maturation. Hence it is challenging to unequivocally separate genes conferred by the emergence of DT acquisition from those conferred by seed development. For example, the LAFL (LEAFY COTYLEDON, ABSCISIC ACID INSENSITIVE, FUSCA, and LEC) transcription factor network not only regulates seed development but also diverse seed-specific processes, including deposition of storage reserves (starch, storage proteins, and lipids), acquisition of DT, developmental arrest of the embryo, and dormancy (Jia et al., 2014). It is difficult to identify whether a transcription factor is involved in DT acquisition per se during seed development. In addition, genes associated with morphological changes of seeds during development also obstruct the screening of genes associated with DT. In addition, the experimental period of this system is determined by the period of seed development, which can be uncertain and time-consuming to characterize in full (Table 1).
DT loss during seed germination
It is well established that orthodox seeds lose their DT and become sensitive to extreme drying during the early stages of visible germination (Koster et al., 2003; Bewley et al., 2013). The loss of DT is correlated with the start of cell division soon after radicle emergence. The switch from DT to being desiccation-sensitive (DS) coincides with radicle cells containing double-strand DNA entering the G2 phase of the cell cycle (Saracco et al., 1995; Faria et al., 2005). In contrast to the seed developmental stage, many transcription factors (MYB, AP2, and NAC), and gene encoding methyltransferase and histone, are differentially expressed (Tian et al., 2016). And the DT relative genes such as LEA and HSP are down-regulated suggesting the loss of these proteins alters the DT of a germinating seedling (Mtwisha et al., 2007; Ginsawaeng et al., 2021).
Although using this system to explore the loss of DT generally shortens the experimental period, it has two limitations. Firstly, the physiological changes in seed germination would interfere DT evaluation. Secondly, this system is the reverse process of DT acquisition in orthodox seeds, being a loss of function rather than a gain of function process. Intriguingly, there is the potential to reverse the loss of DT as a result of the onset of visible germination. This physiological response provides a valuable means of dissecting the molecular, biochemical and temporal foundation of DT re-establishment in the germinating seed.
DT re-establishment in germinating seeds
Germinating orthodox seeds have a window to tolerate re-drying, which can be prolonged by applying mild osmotic stress (e.g., using polyethylene glycol [PEG] solution) and/or using plant hormone (abscisic acid [ABA]) before relatively fast-drying is applied. DT has been successfully re-established for seeds of 12 species (Table 2), including those of herbaceous plants (e.g., Arabidopsis thaliana, Cucumis sativus, Impatiens walleriana, Medicago truncatula, Pisum sativum, Vigna unguiculata, and Xerophyta viscosa) and woody plants (e.g., Cedrela fissilis, Caragana korshinskii, Peltophorum dubium, Sesbania virgata, and Tabebuia impetiginosa) (Bruggink and van der Toorn, 1995; Buitink et al., 2000; Leprince et al., 2000; Buitink et al., 2003; Leprince et al., 2004; Faria et al., 2005; Avelange-Macherel et al., 2006; Boudet et al., 2006; Buitink et al., 2006; Vieira et al., 2010; Maia et al., 2011; Terrasson et al., 2013; Lyall et al., 2014; Maia et al., 2014; Masetto et al., 2014; Costa et al., 2015a; Costa et al., 2015b; Masetto et al., 2016; Costa et al., 2016; Guimarães et al., 2016; Lang et al., 2017; Peng et al., 2017; Silva et al., 2017; Peng et al., 2018; Peng et al., 2021; Wang et al., 2021).
In the PEG or ABA-induced DT re-establishment system, germinating seeds return to the dormant or quiescent state (i.e. metabolically inactive) to exclude interference by seed developmental and germinative morphological alteration (Maia et al., 2011; Costa et al., 2015a; Dekkers et al., 2015; Peng et al., 2018). This technological process only takes approximately 2 weeks to apply as it comprises five steps (germination, PEG or ABA treatment, dehydration, pre-humidification, and rehydration) (Figure 1). Because there are well defined control points during this germination-based system, it is convenient for detailed studies on seed DT in a broad range of species, including xerophytes.
The DT re-establishment system is artificial processing rather than natural. Therefore, more experimental factors influence the efficiency and stability of the system. It may lead to the progress of re-establishment DT is not plain sailing (Table 1) (Maia et al., 2011; Peng et al., 2017).
Progress in re-establishment of seed DT
Long after the germination process has been triggered during imbibition of non-dormant seeds, the seeds will completely lose their DT and irreversibly become sensitive to desiccation. Hence, DT re-establishment is heavily dependent on timing within the germinative window (Leprince et al., 2000; Buitink et al., 2003; Vieira et al., 2010; Maia et al., 2011). A. thaliana seeds can be triggered to re-establish DT, and four clearly distinct germination stages have been defined (Maia et al., 2011). In the first three stages, seeds are able to withstand desiccation after a PEG treatment (-2.5 MPa). However, this ability is largely lost at stage IV when the first root hair is visible (Maia et al., 2011; Maia et al., 2014). Similar to A. thaliana seeds, after PEG treatment (-1.7 MPa) most seeds of 2.7 mm long radicles in M. truncatula survived and developed into normal germinating seeds after rehydration (Buitink et al., 2003).
Another feature is that different seed parts display variable levels of DT (Figure 1). The radicles appearing to be the most desiccation-sensitive part of germinating seeds, followed by the hypocotyl and cotyledon tissues (Buitink et al., 2003; Maia et al., 2011; Peng et al., 2017). In M. truncatula and C. korshinskii, radicles as experimental materials used for DT re-establishment (Buitink et al., 2003; Peng et al., 2017). In addition to the tissue part of seeds, osmotic potential, temperature, and treatment time are factors that also influence the re-establishment of DT in germinating seeds at PEG concentrations varying from -1 to -2.5 MPa (Maia et al., 2011; Peng et al., 2017; Wang et al., 2021).
The availability of a robust system of modulating the re-establishment of physiological DT in seeds facilitates multi-omics studies on discovering genes and pathways linked to how seeds survive drying. Transcriptome profiling and proteomic analyses have uncovered key metabolic and regulatory processes during DT re-established by PEG treatment in M. truncatula (Buitink et al., 2003; Boudet et al., 2006) and A. thaliana seeds (Maia et al., 2011). Transcripts of germinating seeds after PEG treatment are dominated by those encoding LEA, seed storage proteins, and dormancy-related proteins (Buitink et al., 2003; Boudet et al., 2006; Maia et al., 2011). In parallel, there is massive repression of genes belonging to many other classes, such as those involved in photosynthesis, cell wall modification, and energy metabolism (Buitink et al., 2003; Boudet et al., 2006; Maia et al., 2011). It also appears that mainly starch and lipid reserves, and to a lesser extent oligosaccharides, are mobilized at different time points to provide sucrose for osmotic adjustment and protection (Buitink et al., 2003). Overall, these changes facilitate a programmed reversion from a metabolic active state to a quiescent state.
According to the gene expression profile, the up-regulated genes in PEG treated seeds are related to the acquisition of DT while the down-regulated genes are related to imbibition. The down-regulated genes in PEG treated seeds are up-regulated upon imbibition. It appears that germinating seeds after PEG treatment indeed revert to a developmental DT stage (Buitink et al., 2003; Maia et al., 2011). From a regulatory point of view, several transcription factors modulate the regulatory network relative to LEA, HSPs, and seed longevity. Of note, a marked enrichment of transcription factors to the promoters of the most highly up-regulated DT-associated genes has been reported (Maia et al., 2011; Terrasson et al., 2013).
Besides PEG treatment, DT in germinating seeds can also be rescued by treatment with ABA (Maia et al., 2014; Costa et al., 2015a). In agreement with the role of PEG, the main physiological and molecular processes that occur during incubation in ABA reveal growth arrest and a partial return to the quiescent stage (Maia et al., 2014; Costa et al., 2015a). During ABA-induced DT re-establishment, the expression of a large number of genes encoding abiotic stress response TFs, such as ABI5, APETALA 2/ethylene-responsive element- binding factor (AP2/ERF) family, NAM/ATF1/CUC2 (NAC) class and WRKY TFs, ABRE-binding factor 1 (ABF1), etc. are induced (Costa et al., 2015a). The re-establishment of DT depends on the modulation of ABA sensitivity rather than enhanced ABA content, emphasizing ABA receptors play a pivotal role in the re-establishment of DT in germinating seeds (Maia et al., 2014; Costa et al., 2015a).
It is evident that comprehensive omics data is shedding light on the molecular and physiological processes associated with the re-establishment of DT in seeds. However, the main experimental findings on the mechanisms of DT re-establishment in seeds are rather limited to the model plants A. thaliana and M. truncatula. Their seeds exhibit much weaker DT compared to xerophytic seeds and there are no unexpected genes or mechanisms that have been functionally characterized. Therefore, it is important to widen the search for functional DT re-establishment to species that grow in harsh environments, e.g., xerophytic seeds with exceptional DT. In this way, it may be possible to identify new mechanisms of DT establishment in nature.
DT re-establishment in germinating seeds of caragana korshinskii kom.
Caragana korshinskii Kom. is a perennial sandy grassland and desert deciduous shrub species with strong drought resistance, distributed in the northwest of China and Mongolia (Lai et al., 2015). Its seeds are non-dormant and germinate rapidly after imbibition (Abudureheman et al., 2014; Lai et al., 2015). Post-radicle emergence, DT decreased with mitosis in both C. korshinskii and M. truncatula, but the rate of DT loss during visible germination in C. korshinskii is significantly slower (Faria et al., 2005; Peng et al., 2017), i.e. the window is wider. These characteristics indicate that C. korshinskii can be a particularly suitable species to explore DT mechanisms.
The broad changes in the transcriptome in radicles of C. korshinskii show the complexity of the response of these seeds to PEG-induced re-establishment of DT, including the enhancement of many genes encoding stress-related proteins (HSPs and LEAs), raffinose family oligosaccharide biosynthesis-related enzymes (RFOs), and TFs (WRKY, NAC, and ERF) (Peng et al., 2017). In addition, ROS accumulates in the radicle tip of germinating C. korshinskii seeds during DT re-establishment. Given the dual function of ROS, the accurate execution of signaling functions of ROS prevents the accumulation of oxidative damage while enabling the re-establishment of DT in emerging radicles (Mittler, 2017; Peng et al., 2017).
In addition to PEG or ABA, hydrogen peroxide can act as an independent inducer of DT re-establishment (Peng et al., 2017). Although there are apparent similarities between the DT re-establishment following PEG or H2O2 treatments in C. korshinskii, a key difference exists regarding the phenylpropanoid–flavonoid pathway that avoid exogenous ROS damage. This pathway is activated by H2O2 treatment but is insensitive to PEG treatment (Peng et al., 2017). Consequently, these comprehensive mechanisms induced by hydrogen peroxide provide novel information on DT re-establishment, which is beneficial for the understanding the role of ROS in seed DT in seeds of xerophytic species and potentially other species.
In addition to the best-known proteins and genes relative to DT in the model plant, the proteomic analysis of C. korshinskii seeds showed the greatest increase in abundance of metallothionein (MT), a change not previously observed in other species (Peng et al., 2018; Peng et al., 2021). During seed DT re-establishment, in addition to metal-binding capacity, in vivo and in vitro evidences illustrate CkMT4 might supply Cu2+/Zn2+ to SOD under high redox potential provided by PEG treatment for excess ROS scavenging (Peng et al., 2018; Peng et al., 2021). CkMT4 acts as a candidate gene for improving survival of germinating seeds under dehydration (Peng et al., 2021). Studies with C. korshinskii that identify putative key genes for adaptation may also help disentangle the evolutionary complexity of seed DT (Peng et al., 2021; Nonogaki et al., 2022).
Discussion
The germinating seeds of C. korshinskii display stronger DT than model plants (Buitink et al., 2003; Peng et al., 2017). Building on the above findings, it appears that the xerophytic seeds represented by C. korshinskii have potential research value for studying DT. However, to our knowledge, little studies focus on DT of xerophytic seeds, except C. korshinskii. The research value and limited research of xerophytic seeds are out-off-balance. To bridge the gap, a future research program is necessary to explore the full potential of their stress tolerance. The DT re-establishment system provides a powerful tool for revealing the mechanisms of DT and for screening novel pivotal genes associated with DT (Peng et al., 2018; Peng et al., 2021). As a follow-up, we suggest programming the following steps to unveil the DT mechanism of xerophytic seeds and building the gene library related to DT.
Firstly, in addition to C. korshinskii, more xerophytic species should be applied to re-establish DT. Secondly, employ multi-omics methods to associate physiological processes with molecular mechanisms. Crosswise comparison of the DT re-establishment in numerous different species will reveal universal and unique regulation mechanism. Then seed scientists will screen the novel DT relative genes and decipher the functional characterization of these genes. In the last step, construct a gene library from the xerophytic plant to provide efficient candidate genes for improving DT in seeds.
Besides extensive research on DT in xerophytic seeds, the deciphering of the cellular or molecular process for C. korshinskii is just beginning and more subtle regulatory mechanic studies should be carried out. Below is a list of three selected questions that we believe require further investigation:
a. What is the role of post-translational modification in the re-establishment of DT? Post-translational modification, as the frontier of signaling pathway regulatory studies, has been intensely studied in botany-related fields, especially plant stress resistance (Xu and Xue, 2019; Ding et al., 2020; Chen et al., 2021). During DT re-establishment in germinating seeds of C. korshinskii, the genes related to the degradation of the ubiquitin pathway exhibit a higher level of expression, which indicates ubiquitination may be involved in this process (Peng et al., 2017). We believe that this case also requires methylation, acetylation or phosphorylation, etc. Therefore, extensive epigenetic studies are needed to identify the molecule modification and explain their functionalities.
b. Can the control of cell division under mild osmotic stress be optimized in relation to critical thresholds for germination (Maleki et al., 2022)? Plant growth and resistance involve a balance between metabolites and energy distribution relating to cell expansion and division. During DT re-establishment, seeds abandon the germination process for enhanced resilience (Maia et al., 2014; Costa et al., 2015a; Peng et al., 2018). Under this scenario, the direction of energetically expensive processes undergoes a sharp change. A switch should be presented that drastically alters the seeds’ behavior at particularly water potentials whereby the outcome of the physiological stage can be directed from germinal to quiescent. Considering the intimate connection between inhibition of cell division and the quiescent phase, it is obvious that the expression and regulatory networks of genes related to cell division need to be further analyzed.
c. How to maintain the healthy state of DNA on the process of DT re-establishment in germinating seeds? In the process of DT re-establishment, PEG treatment alleviates DNA damage induced by dehydration (Faria et al., 2005). The ROS scavenging system may prevent DNA from oxidative damage (Leprince et al., 2000; Buitink et al., 2006; Maia et al., 2011; Lang et al., 2017; Peng et al., 2017; Peng et al., 2018; Peng et al., 2021). In seeds with DT, some DNA damage that occurs during dehydration or dry storage may be repaired (Osborne, 2000). We thus provided a hypothesis that DNA protection and DNA repair systems could be activated during DT re-establishment. Evolutionary processes gave rise to DNA repair tools that are efficient in repairing damaged DNA (Britt, 1999; Que et al., 2019). Nevertheless, little work has been done to identify how DNA repair systems reduce the degree of DNA degradation during DT re-establishment. It will be interesting to focus on dissecting DNA protection and repair pathways in DT re-establishment.
In conclusion, this article aims to direct more attention towards the value of scientific research using xerophytic seeds and focusing on their underlying genes that permit the DT. We estimate that many efficient genes will be identified by disentangling the physiologic process of DT in xerophytic seeds, providing candidate genes for improving DT in intermediate and recalcitrant seeds through genetic engineering, which may be beneficial for germplasm conservation. The re-establishment of DT in diversified xerophytic seeds will assist in developing the comprehensive exploration of DT. This challenging program will require more researchers to devote themselves to analyzing the mechanism of DT in xerophytic seeds in order to accelerate the process of DT seed breeding.
Author contributions
LP drafted the manuscript, Figure 1 and Tables 1, 2. HX and HWP edited them. All authors contributed to the article and approved the submitted version.
Funding
This work was supported by the National Natural Science Foundation of China (31971646), and the Fundamental Research Funds for the Central Non-profit Research of Chinese Academy of Forestry (CAFYBB2020QB002).
Conflict of interest
The authors declare that the research was conducted in the absence of any commercial or financial relationships that could be construed as a potential conflict of interest.
Publisher’s note
All claims expressed in this article are solely those of the authors and do not necessarily represent those of their affiliated organizations, or those of the publisher, the editors and the reviewers. Any product that may be evaluated in this article, or claim that may be made by its manufacturer, is not guaranteed or endorsed by the publisher.
References
Abudureheman, B., Liu, H., Zhang, D., Guan, K. (2014). Identification of physical dormancy and dormancy release patterns in several species (Fabaceae) of the cold desert, north-west China. Seed Sci. Res. 24, 133–145. doi: 10.1017/s0960258514000063
Almoguera, C., Personat, J. M., Prieto-Dapena, P., Jordano, J. (2015). Heat shock transcription factors involved in seed desiccation tolerance and longevity retard vegetative senescence in transgenic tobacco. Planta 242 (2), 461–475. doi: 10.1007/s00425-015-2336-y
Angelovici, R., Galili, G., Fernie, A. R., Fait, A. (2010). Seed desiccation: bridge between maturation germination. Trends Plant Sci. 15 (4), 211–218. doi: 10.1016/j.tplants.2010.01.003
Asdal, Å., Guarino, L. (2018). The Svalbard global seed vault: 10 years-1 million samples. biopreserv. Biobank 16 (5), 391–392. doi: 10.1089/bio.2018.0025
Avelange-Macherel, M. H., Ly-Vu, B., Delaunay, J., Richomme, P., Leprince, O. (2006). NMR metabolite profiling analysis reveals changes in phospholipid metabolism associated with the re-establishment of desiccation tolerance upon osmotic stress in germinated radicles of cucumber. Plant Cell Environ. 29 (4), 471–482. doi: 10.1111/j.1365-3040.2005.01424.x
Ballesteros, D., Fanega-Sleziak, N., Davies, R. M. (2021). Cryopreservation of seeds and seed embryos in orthodox-, intermediate-, and recalcitrant-seeded species. Methods Mol. Biol. 2180, 663–682. doi: 10.1007/978-1-0716-0783-1_36
Bewley, J. D., Bradford, K. J., Hilhorst, H. W. M., Nonogaki, H. (2013). Seeds: Physiology of development, germination and dormancy 3rd ed (New York, USA: Springer-Verlag). doi: 10.1093/oxfordjournals.pcp.a075190
Black, M., Corbineau, F., Gee, H., Come, D. (1999). Water content, raffinose, and dehydrins in the induction of desiccation tolerance in immature wheat embryos. Plant Physiol. 120 (2), 463–472. doi: 10.1104/pp.120.2.463
Black, M., Pritchard, H. W. (2002). Desiccation and survival in plants: drying without dying (Wallingford, UK: CABI Publishing. Bruggink). doi: 10.1079/9780851995342.0003
Boudet, J., Buitink, J., Hoekstra, F. A., Rogniaux, H., Larré, C., Satour, P., et al. (2006). Comparative analysis of the heat stable proteome of radicles of Medicago truncatula seeds during germination identifies late embryogenesis abundant proteins associated with desiccation tolerance. Plant Physiol. 140 (4), 1418–1436. doi: 10.1104/pp.105.074039
Britt, A. B. (1999). Molecular genetics of DNA repair in higher plants. Trends Plant Sci. 4 (1), 20. doi: 10.1016/s1360-1385(98)01355-7
Bruggink, T., van der Toorn, P. (1995). Induction of desiccation tolerance in germinated seeds. Seed Sci. Res. 5 (1), 1–4. doi: 10.1017/s096025850000252x
Buitink, J., Leger, J. J., Guisle, I., Vu, B. L., Wuillème, S., Lamirault, G., et al. (2006). Transcriptome profiling uncovers metabolic and regulatory processes occurring during the transition from desiccation-sensitive to desiccation-tolerant stages in Medicago truncatula seeds. Plant J. 47 (5), 735–750. doi: 10.1111/j.1365-313x.2006.02822.x
Buitink, J., Leprince, O., Hoekstra, F. A. (2000). Dehydration-induced redistribution of amphiphilic molecules between cytoplasm and lipids is associated with desiccation tolerance in seeds. Plant Physiol. 124 (3), 1413–1426. doi: 10.1104/pp.124.3.1413
Buitink, J., Ly Vu, B., Satour, P., Leprince, O. (2003). The re-establishment of desiccation tolerance in germinated radicles of Medicago truncatula gaertn seeds. Seed Sci. Res. 13, 273–286. doi: 10.1079/ssr2003145
Byrne, P. F., Volk, G. M., Candice, G., Gore, M. A., Simon, P. W., Stephen, S. (2018). Sustaining the future of plant breeding: the critical role of the usda-ars national plant germplasm system. Crop Sci. 58 (2), 1–18. doi: 10.2135/cropsci2017.05.0303
Chapman, T., Miles, S., Trivedi, C. (2018). Capturing, protecting and restoring plant diversity in the UK: RBG kew and the millennium seed bank. Plant Divers. 41 (2), 124–131. doi: 10.1016/j.pld.2018.06.001
Chatelain, E., Hundertmark, M., Leprince, O., Le Gall, S., Satour, P., Deligny-Penninck, S., et al. (2012). Temporal profiling of the heat-stable proteome during late maturation of Medicago truncatula seeds identifies a restricted subset of late embryogenesis abundant proteins associated with longevity. Plant Cell Environ. 35 (8), 1440–1455. doi: 10.1111/j.1365-3040.2012.02501.x
Chen, X., Ding, Y., Yang, Y., Song, C., Wang, B., Yang, S., et al. (2021). Protein kinases in plant responses to drought, salt, and cold stress. J. Integr. Plant Biol. 63 (1), 53–78. doi: 10.1111/jipb.13061
Colville, L., Pritchard, H. W. (2019). Seed life span and food security. New Phytol. 224 (2), 557–562. doi: 10.1111/nph.16006
Costa, M. C. D., Faria, J. M. R., José, A. C., Ligterink, W., Hilhorst, H. W. M. (2016). Desiccation tolerance and longevity of germinated Sesbania virgata (cav.) pers.seeds. J. Seed Sci. 38 (1), 50–56. doi: 10.1590/2317-1545v38n3161129
Costa, M. C., Nijveen, H., Ligterink, W., Buitink, J., Hilhorst, H. W. (2015b). Time-series analysis of the transcriptome of the re-establishment of desiccation tolerance by ABA in germinated arabidopsis thaliana seeds. Genom. Data 5, 154–156. doi: 10.1016/j.gdata.2015.06.003
Costa, M. C., Righetti, K., Nijveen, H., Yazdanpanah, F., Ligterink, W., Buitink, J., et al. (2015a). A gene co-expression network predicts functional genes controlling the re-establishment of desiccation tolerance in germinated arabidopsis thaliana seeds. Planta 242 (2), 435–449. doi: 10.1007/s00425-015-2283-7
Daws, M. I., Lydall, E., Chmielarz, P., Leprince, O., Matthews, S., Thanos, C. A., et al. (2010). Developmental heat sum influences recalcitrant seed traits in Aesculus hippocastanum across Europe. New Phytol. 162 (1), 157–166. doi: 10.1111/j.1469-8137.2004.01012.x
Dekkers, B. J., Costa, M. C., Maia, J., Bentsink, L., Ligterink, W., Hilhorst, H. W. (2015). Acquisition and loss of desiccation tolerance in seeds: from experimental model to biological relevance. Planta 241 (3), 563–577. doi: 10.1007/s00425-014-2240-x
Delahaie, J., Hundertmark, M., Bove, J., Leprince, O., Rogniaux, H., Buitink, J. (2013). LEA polypeptide profiling of recalcitrant and orthodox legume seeds reveals ABI3-regulated LEA protein abundance linked to desiccation tolerance. J. Exp. Bot. 64 (14), 4559–4573. doi: 10.1093/jxb/ert274
Dinato, N. B., Santos, I., Vigna, B., Ferreira de Paula, A., Favero, A. P. (2020). Pollen cryopreservation for plant breeding and genetic resources conservation. Cryo Lett. 41 (3), 115–127. doi: 10.1270/jsbbr.1.97
Ding, Y., Shi, Y., Yang, S. (2020). Molecular regulation of plant responses to environmental temperatures. Mol. Plant 13 (4), 544–564. doi: 10.1016/j.molp.2020.02.004
Dussert, S., Serret, J., Bastos-Siqueira, A., Morcillo, F., Déchamp, E., Rofidal, V., et al. (2018). Integrative analysis of the late maturation programme and desiccation tolerance mechanisms in intermediate coffee seeds. J. Exp. Bot. 69 (7), 1583–1597. doi: 10.1093/jxb/erx492
Ellis, R. H., Hong, T. D., Roberts, E. H. (1990). An intermediate category of seed storage behaviour? I. Coffee. J. Exp. Bot. 41, 1167–1174. doi: 10.1093/jxb/41.9.1167
Fait, A., Angelovici, R., Less, H., Ohad, I., Urbanczyk-Wochniak, E., Fernie, A. R., et al. (2006). Arabidopsis seed development and germination is associated with temporally distinct metabolic switches. Plant Physiol. 142 (3), 839–854. doi: 10.1104/pp.106.086694
Fan, L., Wang, G., Hu, W., Pantha, P., Tran, K. N., Zhang, H., et al. (2018). Transcriptomic view of survival during early seedling growth of the extremophyte Haloxylon ammodendron. Plant Physiol. Biochem. 132, 475–489. doi: 10.1016/j.plaphy.2018.09.024
Fan, B., Zhou, Y., Ma, Q., Yu, Q., Zhao, C., Sun, K. (2018). The bet-hedging strategies for seedling emergence of Calligonum mongolicum to adapt to the extreme desert environments in northwestern China. Front. Plant Sci. 9. doi: 10.3389/fpls.2018.01167
Faria, J. M., Buitink, J., van Lammeren, A. A., Hilhorst, H. W. (2005). Changes in DNA and microtubules during loss and re-establishment of desiccation tolerance in germinating Medicago truncatula seeds. J. Exp. Bot. 56 (418), 2119–2130. doi: 10.1093/jxb/eri210
Franchi, G. G., Piotto, B., Nepi, M., Baskin, C. C., Baskin, J. M., Pacini, E. (2011). Pollen and seed desiccation tolerance in relation to degree of developmental arrest, dispersal, and survival. J. Exp. Bot. 62 (15), 5267–5281. doi: 10.1093/jxb/err154
Ghangal, R., Raghuvanshi, S., Sharma, P. C. (2012). Expressed sequence tag based identification and expression analysis of some cold inducible elements in seabuckthorn (Hippophae rhamnoides l.). Plant Physiol. Biochem. 51, 123–128. doi: 10.1016/j.plaphy.2011.10.005
Ginsawaeng, O., Gorka, M., Erban, A., Heise, C., Brueckner, F., Hoefgen, R., et al. (2021). Characterization of the heat-stable proteome during seed germination in arabidopsis with special focus on LEA proteins. Int. J. Mol. Sci. 22 (15), 8172. doi: 10.3390/ijms22158172
Gonçalves, A. R., Chaves, L. J., de Campos Telles, M. P. (2019). Genetic variability and effective population size in Hymenaea stigonocarpa (Fabaceae) germplasm collection: Tools for breeding programs and genetic conservation. Genetica 147, 5–6. doi: 10.1007/s10709-019-00076-0
Gong, C., Wang, J., Hu, C., Wang, J., Ning, P., Bai, J. (2015). Interactive response of photosynthetic characteristics in Haloxylon ammodendron and Hedysarum scoparium exposed to soil water and air vapor pressure deficits. J. Environ. Sci. (China) 34, 184–196. doi: 10.1016/j.jes.2015.03.012
Gong, Z., Xiong, L., Shi, H., Yang, S., Herrera-Estrella, L. R., Xu, G., et al. (2020). Plant abiotic stress response and nutrient use efficiency. Sci. China Life Sci. 63 (5), 635–674. doi: 10.1002/9780813809380.ch7
Guimarães, C. C., Maia, J., Faria, J. M. R., Hilhorst, H. W. M., Ligterink, W., Pereira, W. V. S., et al. (2016). Changes in gene expression and soluble carbohydrate contents during the imbibition and re-induction of desiccation tolerance in Peltophorum dubium seeds. Seed Sci. Technol. 44 (1), 1–13. doi: 10.15258/sst.2016.44.1.20
Hoekstra, F. A., Golovina, E. A., Buitink, J. (2001). Mechanisms of plant desiccation tolerance. Trends Plant Sci. 6 (9), 431–438. doi: 10.1002/9780470376881.ch3
Huang, H., Møller, I. M., Song, S. Q. (2012). Proteomics of desiccation tolerance during development and germination of maize embryos. J. Proteomics 75 (4), 1247–1262. doi: 10.1016/j.jprot.2011.10.036
Huang, H., Song, S. (2013). Change in desiccation tolerance of maize embryos during development and germination at different water potential PEG-6000 in relation to oxidative process. Plant Physiol. Biochem. 68, 61–70. doi: 10.1016/j.plaphy.2013.02.029
Jia, H., Suzuki, M., McCarty, D. R. (2014). Regulation of the seed to seedling developmental phase transition by the LAFL and VAL transcription factor networks. Dev. Biol. 3 (1), 135–145. doi: 10.1002/wdev.126
Jing, Y., Lang, S., Wang, D., Xue, H., Wang, X. F. (2018). Functional characterization of galactinol synthase and raffinose synthase in desiccation tolerance acquisition in developing arabidopsis seeds. J. Plant Physiol. 230, 109–121. doi: 10.1016/j.jplph.2018.10.011
Koster, K. L., Reisdorph, N., Ramsay, J. L. (2003). Changing desiccation tolerance of pea embryo protoplasts during germination. J. Exp. Bot. 54 (387), 1607–1614. doi: 10.1093/jxb/erg170
Lai, L., Tian, Y., Wang, Y., Zhao, X., Jiang, L., Baskin, J. M., et al. (2015). Distribution of three congeneric shrub species along an aridity gradient is related to seed germination and seedling emergence. AoB Plants 7, 71. doi: 10.1093/aobpla/plv071
Lang, S., Liu, X., Ma, G., Lan, Q., Wang, X. (2014). Identification of desiccation tolerance transcripts potentially involved in rape (Brassica napus l.) seeds development and germination. plant physiol. Biochem 83, 316–326. doi: 10.1016/j.plaphy.2014.08.001
Lang, S., Liu, X., Xue, H., Li, X., Wang, X. (2017). Functional characterization of BnHSFA4a as a heat shock transcription factor in controlling the re-establishment of desiccation tolerance in seeds. J. Exp. Bot. 68 (9), 2361–2375. doi: 10.1093/jxb/erx097
Leprince, O., Harren, F. J., Buitink, J., Alberda, M., Hoekstra, F. A. (2000). Metabolic dysfunction and unabated respiration precede the loss of membrane integrity during dehydration of germinating radicles. Plant Physiol. 122 (2), 597–608. doi: 10.1104/pp.122.2.597
Leprince, O., Pellizzaro, A., Berriri, S., Buitink, J. (2017). Late seed maturation: drying without dying. J. Exp. Bot. 68 (4), 827–841. doi: 10.1093/jxb/erw363
Leprince, O., Satour, P., Vu, B. L., Buitink, J. (2004). The role of sugars and hexose phosphorylation in regulating the re-establishment of desiccation tolerance in germinated radicles of Cucumis sativa and Medicago truncatula. Physiol. Plant 122 (2), 200–209. doi: 10.1111/j.1399-3054.2004.00386.x
Li, D. Z., Pritchard, H. W. (2009). The science and economics of ex situ plant conservation. Trends Plant Sci. 14 (11), 614–621. doi: 10.1016/j.tplants.2009.09.005
Liu, U., Breman, E., Cossu, T. A., Kenney, S. (2018). The conservation value of germplasm stored at the millennium seed bank, royal botanic gardens, KEW, UK. Biodivers. Conserv. 27 (6), 1347–1386. doi: 10.1007/s10531-018-1497-y
Li, D., Yang, X., Wang, Y., Cai, J. (2010). The germplasm bank of wild species, southwest China. Bull. Chin. Acad. Sci. 24 (4), 4. doi: 10.17520/biods.2021341
Li, X., Zhuo, J., Jing, Y., Liu, X., Wang, X. (2011). Expression of a GALACTINOL SYNTHASE gene is positively associated with desiccation tolerance of Brassica napus seeds during development. J. Plant Physiol. 168 (15), 1761–1770. doi: 10.1016/j.jplph.2011.04.006
Louhaichi, M., Clifton, K., Hassan, S. (2014). Direct seeding of Salsola vermiculata for rehabilitation of degraded arid and semi-arid rangelands. Range Manage. Agrofor. 35 (2), 182–187. doi: 10.1002/2688-8319.12078/v2/review1
Lyall, R., Ingle, R. A., Illing, N. (2014). The window of desiccation tolerance shown by early-stage germinating seedlings remains open in the resurrection plant, Xerophyta viscosa. PloS One 9 (3), e93093. doi: 10.1371/journal.pone.0093093
Maia, J., Dekkers, B. J., Dolle, M. J., Ligterink, W., Hilhorst, H. W. (2014). Abscisic acid (ABA) sensitivity regulates desiccation tolerance in germinated arabidopsis seeds. New Phytol. 203 (1), 81–93. doi: 10.1111/nph.12785
Maia, J., Dekkers, B. J., Provart, N. J., Ligterink, W., Hilhorst, H. W. (2011). The re-establishment of desiccation tolerance in germinated Arabidopsis thaliana seeds and its associated transcriptome. PloS One 6 (12), e29123. doi: 10.1371/journal.pone.0029123
Maleki, K., Solani, E., Seal, C. E., Pritchard, H. W., Lamichhane, J. R. (2022). The seed germination spectrum of 528 plant species: a global meta-regression in relation to temperature and water potential. bioRxiv. doi: 10.1101/2022.08.24.504107
Masetto, T. E., Faria, J. M., Fraiz, A. C. (2014). Re-induction of desiccation tolerance after germination of Cedrela fissilis vell. seeds. An. Acad. Bras. Cienc. 86 (3), 1273–1286. doi: 10.1590/0001-3765201420130164
Masetto, T. E., Fraiz, A. C. R., Faria, J. M. R. (2016). Cell changes during the re-induction of desiccation tolerance in germinated seeds of sesbania virgata. J. Seed Sci. 38 (3), 254–258. doi: 10.1590/2317-1545v38n3161129
Miguel, M. F., Butterfield, H. S., Lortie, C. J. (2020). A meta-analysis contrasting active versus passive restoration practices in dryland agricultural ecosystems. PeerJ, e10428. doi: 10.7717/peerj.10428
Mittler, R. (2017). ROS are good. Trends Plant Sci. 22 (1), 11–19. doi: 10.1016/j.tplants.2016.08.002
Mtwisha, L., Farrant, J. M., Brandt, W., Hlongwane, C., Lindsey, G. G. (2007). ASP53, a thermostable protein from Acacia erioloba seeds that protects target proteins against thermal denaturation. Funct. Plant Biol. 34 (2), 139–149. doi: 10.1071/FP06135
Nonogaki, M., Yamazaki, S., Nishiyama, E., Ohshima, K., Nonogaki, H. (2022). Seed traits and phylogenomics: Prospects for the 21st century. Seed Sci. Res., 1–7. doi: 10.1017/S0960258522000046
Oliver, M. J., Farrant, J. M., Hilhorst, H., Mundree, S., Williams, B., Bewley, J. D. (2020). Desiccation tolerance: avoiding cellular damage during drying and rehydration. Annu. Rev. Plant Biol. 71, 435–460. doi: 10.1146/annurev-arplant-071219-105542
Osborne, D. J. (2000). Hazards of a germinating seed: available water and the maintenance of genomic integrity. Isr. J. Plant Sci. 48 (3), 173–179. doi: 10.1560/jnxx-m1th-2um3-5uf6
Pammenter, N. W., Berjak, P. (1999). A review of recalcitrant seed physiology in relation to desiccation-tolerance mechanisms. Seed Sci. Res. 9 (01), 13–37. doi: 10.1017/s0960258599000033
Peng, L., Lang, S., Wang, Y., Pritchard, H. W., Wang, X. (2017). Modulating role of ROS in re-establishing desiccation tolerance in germinating seeds of Caragana korshinskii kom. J. Exp. Bot. 68 (13), 3585–3601. doi: 10.1093/jxb/erx172
Peng, L., Sun, Q., Xue, H., Wang, X. (2018). ). iTRAQ-based quantitative proteomic analysis reveals pathways associated with re-establishing desiccation tolerance in germinating seeds of Caragana korshinskii kom. J. Proteomics 179, 1–16. doi: 10.1016/j.jprot.2018.01.009
Peng, L., Wu, H., Huang, X., Zeng, M., Deng, S., Xue, H., et al. (2021). Functional characterization of an unobtrusive protein, CkMT4, in re-establishing desiccation tolerance in germinating seeds. Int. J. Biol. Macromol. 173, 180–192. doi: 10.1016/j.ijbiomac.2021.01.011
Pérez, D. R., González, F., Ceballos, C., Oneto, M. E., Aronson, J. (2019). Direct seeding and outplantings in drylands of Argentinean patagonia: Estimated costs, and prospects for large-scale restoration and rehabilitation. Restor. Ecol. 27, 1105–1116. doi: 10.1111/rec.12961
Plitta-Michalak, B. P., Naskret-Barciszewska, M. Z., Kotlarski, S., Tomaszewski, D., Tylkowski, T., Barciszewski, J., et al. (2018). Changes in genomic 5-methylcytosine level mirror the response of orthodox (Acer platanoides l.) and recalcitrant (Acer pseudoplatanus l.) seeds to severe desiccation. Tree Physiol. 38 (4), 617–629. doi: 10.1007/s11738-999-0064-2
Pritchard, H. W., Wood, C. B., Hodges, S., Vautier, H. J. (2004). 100-seed test for desiccation tolerance and germination: a case study on eight tropical palm species. Seed Sci. Technol. 32 (2), 393–403. doi: 10.15258/sst.2004.32.2.11
Pukacka, S., Ratajczak, E. (2007). Ascorbate and glutathione metabolism during development and desiccation of orthodox and recalcitrant seeds of the genus acer. Funct. Plant Biol. 34 (7), 601–613. doi: 10.1071/fp07013
Pukacka, S., Ratajczak, E., Kalemba, E. (2011). The protective role of selenium in recalcitrant Acer saccharium l. seeds subjected to desiccation. J. Plant Physiol. 168 (3), 220–225. doi: 10.1016/j.jplph.2010.07.021
Qi, J., Song, C. P., Wang, B., Zhou, J., Kangasjärvi, J., Zhu, J. K., et al. (2018). Reactive oxygen species signaling and stomatal movement in plant responses to drought stress and pathogen attack. J. Integr. Plant Biol. 60 (9), 805–826. doi: 10.1111/jipb.12654
Que, Q., Chen, Z., Kelliher, T., Skibbe, D., Dong, S., Chilton, M. D. (2019). Plant DNA repair pathways and their applications in genome engineering. Methods Mol. Biol. 1917, 3–24. doi: 10.1007/978-1-4939-8991-1_1
Ratajczak, E., Str Her, E., Oelze, M. L., Kalemba, E. M., Pukacka, S. A., Dietz, K. J. (2013). The involvement of the mitochondrial peroxiredoxin PRXIIF in defining physiological differences between orthodox and recalcitrant seeds of two acer species. Funct. Plant Biol. 40 (10), 1005–1017. doi: 10.1071/fp13002
Roberts, E. H. (1973). Predicting the storage life of seeds. Proceedings 1, 499–514. doi: 10.15258/sst.2008.36.2.11
Saracco, F., Bino, R. J., Bergervoet, J., Lanteri, S. (1995). Influence of priming-induced nuclear replication activity on storability of pepper (Capsicum annuum l.) seed. Seed Sci. Res. 5 (1), 25–29. doi: 10.1017/s0960258500002555
Sara, L., Jakob, S., Saumya, S. (2014). The state of food and agriculture 2014 (Rome, Italy: FAO). doi: 10.18356/579516b5-en
Shackelford, N., Paterno, G. B., Winkler, D. E., Erickson, T. E., Leger, E. A., Svejcar, L. N., et al. (2021). Drivers of seedling establishment success in dryland restoration efforts. Nat. Ecol. Evol. 5 (9), 1283–1290. doi: 10.1038/s41559-021-01510-3
Shi, X. J., Zhang, M. L. (2015). Phylogeographical structure inferred from cpDNA sequence variation of Zygophyllum xanthoxylon across north-west China. J. Plant Res. 128 (2), 269–282. doi: 10.1007/s10265-014-0699-y
Silva, A. C., Davide, L. C., Braz, G. T., Maia, J., Silva, E. (2017). Re-induction of desiccation tolerance in germinated cowpea seeds. S. Afr. J. Bot. 113, 34–39. doi: 10.1016/j.sajb.2017.07.011
Stolarska, E., Bilska, K., Wojciechowska, N., Bagniewska-Zadworna, A., Rey, P., Kalemba, E. M. (2020). Integration of MsrB1 and MsrB2 in the redox network during the development of orthodox and recalcitrant acer seeds. Antioxidants 9 (12), 1250. doi: 10.3390/antiox9121250
Su, L., Lan, Q., Pritchard, H. W., Xue, H., Wang, X. (2016). Reactive oxygen species induced by cold stratification promote germination of Hedysarum scoparium seeds. Plant Physiol. Biochem. 109, 406–415. doi: 10.1016/j.plaphy.2016.10.025
Sun, Y., Shang, L., Zhu, Q. H., Fan, L., Guo, L. (2022). Twenty years of plant genome sequencing: Achievements and challenges. Trends Plant Sci. 27 (4), 391–401. doi: 10.1016/j.tplants.2021.10.006
Terrasson, E., Buitink, J., Righetti, K., Ly Vu, B., Pelletier, S., Zinsmeister, J., et al. (2013). An emerging picture of the seed desiccome: confirmed regulators and newcomers identified using transcriptome comparison. Front. Plant Sci. 4. doi: 10.3389/fpls.2013.00497
Tian, X., Li, S., Liu, Y., Liu, X. (2016). Transcriptomic profiling reveals metabolic and regulatory pathways in the desiccation tolerance of mungbean (Vigna radiata [L.] r. wilczek). Front. Plant Sci. 7. doi: 10.3389/fpls.2016.01921
Tunnacliffe, A., Wise, M. J. (2007). The continuing conundrum of the LEA proteins. Sci. Nat. 94 (10), 791–812. doi: 10.1007/s00114-007-0254-y
Verdier, J., Lalanne, D., Pelletier, S., Torres-Jerez, I., Righetti, K., Bandyopadhyay, K., et al. (2013). A regulatory network-based approach dissects late maturation processes related to the acquisition of desiccation tolerance and longevity of Medicago truncatula seeds. Plant Physiol. 163 (2), 757–774. doi: 10.1104/pp.113.222380
Vieira, C. V., Da Silva, E. A. A., de Alvarenga, A. A., de Castro, E. M., Toorop, P. E. (2010). Stress-associated factors increase after desiccation of germinated seeds of tabebuia impetiginosa mart. Plant Growth Regul. 62, 257–263. doi: 10.1007/s10725-010-9496-3
Villalobos, V. M., Engelmann, F. (1995). Ex situ conservation of plant germplasm using biotechnology. World J. Microbiol. Biotechnol. 11 (4), 375–382. doi: 10.1007/bf00364612
Wade, R., Augyte, S., Harden, M., Nuzhdin, S., Yarish, C., Alberto, F. (2020). Macroalgal germplasm banking for conservation, food security, and industry. PloS Biol. 18 (2). doi: 10.1371/journal.pbio.3000641
Wang, W. Q., Wang, Y., Song, X. J., Zhang, Q., Cheng, H. Y., Liu, J., et al. (2021). Proteomic analysis of desiccation tolerance and its re-establishment in different embryo axis tissues of germinated pea seeds. J. Proteome Res. 20 (5), 2352–2363. doi: 10.1021/acs.jproteome.0c00860.s009
Westengen, O. T., Jeppson, S., Guarino, L. (2013). Global ex-situ crop diversity conservation and the Svalbard global seed vault: assessing the current status. PloS One 8 (5), e64146. doi: 10.1371/journal.pone.0064146
Wojciechowska, N., Alipour, S., Stolarska, E., Bilska, K., Rey, P., Kalemba, E. M. (2020). Peptide-bound methionine sulfoxide (MetO) levels and MsrB2 abundance are differentially regulated during the desiccation phase in contrasted acer seeds. Antioxidants 9 (5), 391. doi: 10.3390/antiox9050391
Wyse, S. B., Dickie, J. B. (2017). Predicting the global incidence of seed desiccation desiccation sensitivity. J. Ecol. Environ. 105, 1082–1093. doi: 10.1111/1365-2745.12725
Xu, F. Q., Xue, H. W. (2019). The ubiquitin-proteasome system in plant responses to environments. Plant Cell Environ. 42 (10), 2931–2944. doi: 10.1111/pce.13633
Yang, Q., Yin, J., Li, G., Qi, L., Yang, F., Wang, R., et al. (2014). Reference gene selection for qRT-PCR in Caragana korshinskii kom. under different stress conditions. Mol. Biol. Rep. 41 (4), 2325–2334. doi: 10.1007/s11033-014-3086-9
Yu, K. L., Wang, G. H. (2018). Long-term impacts of shrub plantations in a desert-oasis ecotone: Accumulation of soil nutrients, salinity, and development of herbaceour layer. Land Degrad. Dev. 29 (8), 2681–2693. doi: 10.1002/ldr.3009
Zhang, Q., Bartels, D. (2018). Molecular responses to dehydration and desiccation in desiccation-tolerant angiosperm plants. J. Exp. Bot. 69 (13), 3211–3222. doi: 10.1093/jxb/erx489
Zheng, G. H., Jing, X. M., Tao, K. L. (1998). Ultradry seed storage cuts cost of gene bank. Nature 393 (6682), 223–224. doi: 10.1038/30383
Keywords: caragana korshinskii, desiccation tolerance, germplasm conservation, seed, xerophytic woody species
Citation: Peng L, Huang X, Qi M, Pritchard HW and Xue H (2022) Mechanistic insights derived from re-establishment of desiccation tolerance in germinating xerophytic seeds: Caragana korshinskii as an example. Front. Plant Sci. 13:1029997. doi: 10.3389/fpls.2022.1029997
Received: 28 August 2022; Accepted: 27 September 2022;
Published: 07 November 2022.
Edited by:
Melvin John Oliver, University of Missouri, United StatesReviewed by:
Julia Buitink, INRA Centre Angers-Nantes Pays de la Loire, FranceThomas Boothby, University of Wyoming, United States
Wilco Ligterink, KeyGene, Netherlands
Copyright © 2022 Peng, Huang, Qi, Pritchard and Xue. This is an open-access article distributed under the terms of the Creative Commons Attribution License (CC BY). The use, distribution or reproduction in other forums is permitted, provided the original author(s) and the copyright owner(s) are credited and that the original publication in this journal is cited, in accordance with accepted academic practice. No use, distribution or reproduction is permitted which does not comply with these terms.
*Correspondence: Hua Xue, eHVlaHVhMjAxM0BiamZ1LmVkdS5jbg==
†ORCID: Long Peng, orcid.org/0000-0002-8345-6278
Hugh W. Pritchard, orcid.org/0000-0002-2487-6475
Hua Xue, orcid.org/0000-0001-8416-6232