- 1The Key Laboratory for Quality Improvement of Agricultural Products of Zhejiang Province, Zhejiang A & F University, Hangzhou, China
- 2College of Advanced Agricultural Sciences, Zhejiang A & F University, Hangzhou, China
How to improve the yield of crops has always been the focus of breeding research. Due to the population growth and global climate change, the demand for food has increased sharply, which has brought great challenges to agricultural production. In order to make up for the limitation of global cultivated land area, it is necessary to further improve the output of crops. Photosynthesis is the main source of plant assimilate accumulation, which has a profound impact on the formation of its yield. This review focuses on the cultivation of high light efficiency plants, introduces the main technical means and research progress in improving the photosynthetic efficiency of plants, and discusses the main problems and difficulties faced by the cultivation of high light efficiency plants. At the same time, in view of the frequent occurrence of high-temperature disasters caused by global warming, which seriously threatened plant normal production, we reviewed the response mechanism of plants to heat stress, introduced the methods and strategies of how to cultivate heat tolerant crops, especially rice, and briefly reviewed the progress of heat tolerant research at present. Given big progress in these area, the era of cultivating smart rice with high light efficiency and heat tolerance has come of age.
Introduction
Due to the limited arable land worldwide, human beings have been committed to continuously improving the output of crops. Most of the yield accumulation of plants comes from the assimilation products of photosynthesis. The photosynthetic yield of any crop depends on the effective solar radiation, radiation capture efficiency and photosynthetic efficiency throughout the growing season (Jansson et al., 2018). Because of the continuous growth of the world’s population, it is expected that the global population will reach 9 billion by 2050, which means that the demand for food will rise sharply, bringing great challenges to agricultural production (Stratonovitch and Semenov, 2015). In order to meet the food requirement, the existing food production must increase by nearly 70% (Lobell et al., 2011) and increasing the yield accumulation of plants by various means will be the most important way.
High light efficiency refers to higher light utilization efficiency under low light and low CO2 concentration, or stronger photosynthesis than other plants under the same conditions. Although the current global CO2 concentration has increased, which seems beneficial to crop photosynthesis, this change is concentrated in the past few decades since 1975, and plants lack sufficient time to adapt to such rapid environmental changes. In addition, the decline of nitrogen uptake by plants under high CO2 concentration and the source-sink limitation also imply that the method of changing CO2 concentration to increase yield is not sustainable (Drake et al., 1997; Long et al., 2015; Zhu et al., 2021). Theoretically, the potential photosynthetic efficiencies of C3 and C4 plants were maximally able to reach 0.046 and 0.060, respectively. However, the current photosynthetic efficiencies of the two types of plants during the growing season only reach 47% and 57% of their theoretical values, respectively, and still have a large rising space (Zhu et al., 2010; Hein et al., 2021). In addition, the frequent occurrence of extreme high temperature caused by climate change in recent years has affected the normal production of a variety of crops, causing plant photosynthesis to be blocked and production to be reduced. It is estimated that the yield of rice will decrease by 3%-8% for every 1°C increase in the average temperature (Paul et al., 2020). This shows that, in addition to improve the photosynthetic efficiency of plants, it is also of great significance to ensure that plants can maintain a normal yield balance under the coming extreme high temperature. With the advent of post functional genomics in rice, breeding strategies that utilize beneficial alleles and eliminate deleterious alleles to optimize crop genome have broad prospects for designing future crops (Varshney et al., 2021; Yu et al., 2022), and the era of breeding smart rice with high light efficiency and heat resistance has come of age.
Research progress of high light efficiency
Photosynthetic products are synthesized in leaves, transported to stems and leaf sheaths and stored as NSCs (non-structural carbohydrates), and then transferred to grains during grain filling (Hein et al., 2021). There are many factors affecting plant photosynthesis. Besides the main environmental conditions such as temperature and light, the morphological development and genetic regulation of plants are also important factors limiting their own photosynthesis. The improvement of photosynthetic rate of crop leaves can be achieved by targeted control of single component processes, such as improving the affinity of key enzyme Rubisco (1,5-ribose diphosphate carboxylation oxygenase) for CO2 (Long, 1991), increasing leaf vein density (Feldman et al., 2017), and adjusting stomatal size and density (Xiong et al., 2022). Furthermore, it is also an effective way to improve photosynthetic efficiency to explore key genes affecting photosynthesis through molecular genetic means.
According to the different carbon fixation products, plants can be divided into C3 and C4 types. Some important food crops such as rice and wheat belong to C3 plants, and their photosynthetic efficiencies largely limited by Rubisco’s oxygenation activity and photorespiration (Atkinson et al., 2016; Singer et al., 2019). In contrast, C4 plants can improve their photosynthetic efficiency by about 30% compared with C3 plants due to their extremely low photorespiration level and CO2 concentration mechanism (CCM, Langdale, 2011). Studies have shown that only 10% increase in photosynthetic efficiency can increase crop yield by nearly 50% (Zhu et al., 2008). It can be seen that if C4 pathway can be introduced into C3 plants such as rice, it will make great contributions to improve grain yield.
In addition, with the development of high-throughput phenotyping technology, people can accurately evaluate the phenotype of a large number of plants within a short time, which provides great convenience for breeding (Araus et al., 2018). In general, the leaves of plants can display different reflectance values at specific wavelengths due to different growth conditions. Therefore, the reflection wavelength of different plant leaves can be obtained by using reflection spectrum technology, which is very effective for determining various leaf characteristics including leaf greenness, light utilization efficiency and leaf pigment content (Tayade et al., 2022). At the same time, in view of the subtle color change of leaves caused by chlorophyll decomposition, reflection spectrum technology is also widely used in determining the senescence rate of leaves (Hein et al., 2021). This not only provides a rapid and accurate method for evaluating the photosynthetic efficiency of different plants, but also provides technical support for cultivating and screening plants with high photosynthetic efficiency, so it has great application potential.
Design C4 crops
At present, people have made preliminary exploration in the design of C4 crops by means of genetic engineering (Ishimaru et al., 1998; Wang et al., 2012; Lian et al., 2014; Cheng et al., 2016; Kandoi et al., 2022). Many studies have shown that overexpression of key photosynthetic enzymes of C4 pathway helped to improve photosynthetic efficiency of C3 plants. However, this enhancement effect is not caused by the introduction of CCM, but by the increased chlorophyll synthesis caused by enhanced metabolism (Kandoi et al., 2022). Thus, the gained effect was quite limited. Given the effect of overexpression of individual photosynthetic enzymes on the rate of carbon assimilation, there are several attempts to combinatorically over-express multiple C4 photosynthetic enzymes to further enhance photosynthesis. However, the results showed that this approach not only did not concentrate CO2 near the chloroplast, but also produced a slight developmental delay (Häusler et al., 2001; Taniguchi et al., 2008; Yadav and Mishra, 2020). Since the current design for C4 crops still stays on single-cell methods such as overexpression of some photosynthetic genes, this limited improvement in photosynthetic efficiency can’t bring substantial improvement in yield (Cui, 2021).
The complex mechanism of C4 pathway is not only reflected in the types of photosynthetic enzymes. The spatial separation of C4 plant photosynthesis is the main reason for its formation of CCM (Furbank, 2017). Mesophyll cells tightly surround Bundle sheath (BS) cells in the center, making BS cells isolated from external O2 and reducing the occurrence of photorespiration. Moreover, BS cells are significantly larger than C3 plants and contain more chloroplasts, which greatly improves the photosynthetic efficiency of C4 plants (Yadav and Mishra, 2020).
On the other hand, the unique Kranz structure of C4 plants’ leaves is another important feature that distinguishes C4 plants from C3 plants (Figure 1, Lundgren et al., 2014). The leaves of C4 plants have developed and more BS cells and more dense leaf vein tissues, and the connection between BS cells and mesophyll cells is also closer, which promotes the exchange of materials between them. Compared with C3 plants, C4 plants have higher material transport efficiency (Wang et al., 2011; Danila et al., 2016; Cui, 2021). Kranz structure contains many different forms, but almost all evolved from C3 plants (Sage et al., 2014). Some genera such as Flaveria and Steinchisma contain both C3 and C4 species or some C3-C4 evolutionary intermediates. Recently, many more C3-C4 intermediates have been found (Brown et al., 2005; Marshall et al., 2007; Keerberg et al., 2014; Khoshravesh et al., 2016). It can be seen that some C3-C4 groups led by Flaveria are important resources for studying the evolutionary mechanism of C4 plants, which provides a valuable biological basis for early attempts to C3 to C4 engineering and for studying the evolutionary mechanism of Kranz anatomical structure (Cui, 2021).
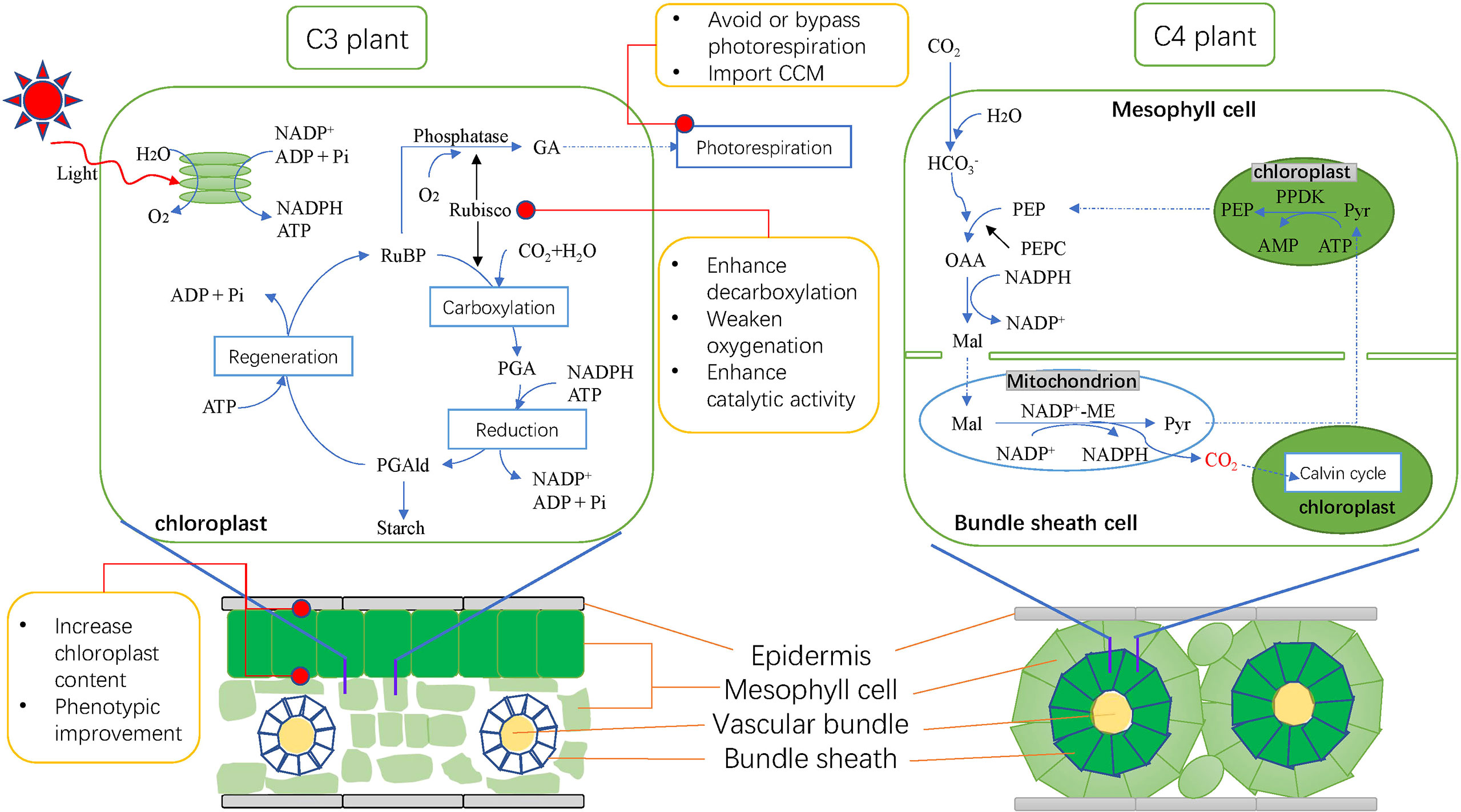
Figure 1 Photosynthetic pathway, leaf structure of C3 and C4 plants and main improvement methods for C3 plants at present. C3 plants on the left and C4 plants on the right. Blue solid line represents the photosynthetic reaction; blue dotted line represents the transport of substances; black line represents the catalysis of the enzyme; red dots represent major improvement objectives. RuBP, ribulose-1,5-bisphosphate; Rubisco, RuBP carboxylase/oxygenase; PGA, 3-phosphoglycerate; PGAld, 3-phosphoglyceraldehyde; GA, glycollic acid; PEP, Phosphoenolpyruvate; PEPC, Phosphoenolpyruvate carboxylase; OAA, oxaloacetic acid; Mal, malic acid; Pyr, pyruvic acid; NADP-ME, NADP-malic enzyme; PPDK, pyruvate phosphate dikinase; CCM, carbon concentration mechanism.
Methods of cultivating high light efficiency plants
Enhancing the carboxylation of Rubisco
Studies have shown that up to 1/2 of Rubisco’s reactions during photosynthesis are oxygenation reactions (Tcherkez et al., 2006). Therefore, this enzyme is regarded as one of the most important targets to improve the photosynthetic efficiency of C3 plants. Some studies suggest that CCM of cyanobacteria can be introduced into C3 plants (Zarzycki et al., 2013; Hanson et al., 2016). This special CCM can achieve high CO2 concentration around Rubisco only through the carboxy matrix in the cell (Lin et al., 2014). Compared to the general mechanism by which C4 plants achieve CO2 enrichment through spatial separation of photosynthesis, this CCM mechanism for cyanobacteria is clearly much simpler and thus very promising for C3 crop improvement. Recently, it has been found that by introducing five photosynthetic enzymes from Zea mays into specific cells of rice leaves, the minimum C4 cycle can be constructed to achieve the purpose of CO2 concentration and have little impact on rice. This also provides another strong evidence for the feasibility of introducing CCM into rice (Ermakova et al., 2021).
Enhancing the catalytic activity of Rubisco
In addition, the low catalytic activity of Rubisco is also one of the main reasons for the low photosynthetic efficiency of C3 plants. Different from the efficient catalytic ability of other enzymes, Rubisco’s catalytic rate is very slow, and each active site can only catalyze about 3.7 enzymatic reactions per second, while most other enzymes can catalyze more than 100 reactions (Parry et al., 2013). This makes plants need to synthesize Rubisco in large quantities to compensate for their low catalytic capacity. It is estimated that plants need to consume up to 50% of leaf soluble protein and 25% of leaf nitrogen to maintain sufficient photosynthetic rate, which greatly limits the growth of plants (Lin et al., 2014). Therefore, improving the catalytic capacity of Rubisco is also an inevitable problem in cultivating high light efficiency plants.
Bypassing endogenous photorespiration
Photorespiration has become the most important factor limiting the photosynthetic yield of plants, which can cause 20-50% yield loss of C3 crops (Wang et al., 2020; Nayak et al., 2022). It is one of the effective measures to avoid the loss of fixed carbon by designing and installing the metabolic bypass of photorespiration to inhibit or bypass the endogenous photorespiration. E. coli can use glycolate as the sole carbon source and can release CO2 while metabolizing (Pellicer et al., 1996). By introducing the glycolic acid metabolism pathway of E. coli into crops such as Arabidopsis and potato, the researchers made the plants express some or all glycolic acid metabolism related genes, reduce or bypass their own photorespiration, and significantly improve plant photosynthesis (Kebeish et al., 2007; Nolke et al., 2014). The same method was applied to the improvement of cucumber, and it was found that the partial or complete introduction of exogenous glycolic acid pathway increased the biomass by 44.9% and 59%, respectively (Chen et al., 2019).
Leaf phenotypic improvement
Besides endogenous genetic regulation, leaf phenotype is also an important factor affecting photosynthetic efficiency. More dense leaf veins and smaller and many more stomata contribute to gas exchange and material transport, which is an effective method to improve photosynthetic efficiency (Feldman et al., 2017; Xiong et al., 2022). Furthermore, the size, curl, inclination angle and other structures of leaves all affect the photosynthetic efficiency of plants. Through the comparison between different rice varieties, it was found that the leaves of rice with high photosynthetic efficiency often have the anatomical characteristics of larger mesophyll cells, more chloroplasts, fewer mesophyll cells between adjacent two leaf veins, and larger chloroplast surface area (Mathan et al., 2021). The content of chlorophyll and other photosynthetic pigments is directly related to the photosynthetic potential and primary productivity. It is generally considered that high chlorophyll content is an ideal phenotype. However, some studies have shown that higher chlorophyll may affect the available light of lower leaves and thus affect the photosynthesis of the whole plant. Reducing the antenna size to appropriately reduce the chlorophyll content, on the contrary, contributes to the improvement of PSII efficiency and nitrogen use efficiency (Song et al., 2017; Leister, 2022).
Prolonging the photosynthetic period of leaves
In rice grains, nearly 80% of the reserve substances come from photosynthesis of leaves after heading (Da Costa et al., 2022). For example, hybrid maize, because of its longer period of photosynthetic activity, still maintains a higher chlorophyll content during senescence relative to normal maize, thereby increasing grain yield (Wu et al., 2016b). This indicated that it was a feasible way to achieve high yield by prolonging the photosynthetic function period of leaves and preventing premature senescence of leaves. Improving the synthesis capacity of chlorophyll, slowing down the senescence and degradation speed of chloroplast, and improving the antioxidant capacity of chloroplast are the main means to ensure the photosynthetic efficiency (Khan et al., 2020; Tang et al., 2020; Yu et al., 2020; Pang et al., 2022). It was found that leaf senescence may be induced by hexose accumulation. By applying different concentrations of sucrose to young and mature leaves, it was found that the electrolyte leakage and malondialdehyde level of leaves increased under high sucrose concentration, resulting in the decrease of leaf photosynthesis (Asim et al., 2022).
Improve the heat tolerance of plants
In the 21st century, due to the intensification of the greenhouse effect and global warming, extreme weather occurs frequently in the world, of which extreme high temperature has the most serious impact on plants (Zhao et al., 2022). Taking rice as an example, high temperature will not only affect the normal synthesis and processing of protein, change the activities of various enzymes, lead to disorder of energy metabolism and excessive accumulation of reactive oxygen species, but also cause poor development of flower organs, affect normal pollination and fertilization, lead to poor grain development and shortened filling time, thus reduce the quality and nutritional value of grains (Wu et al., 2016a; Prasertthai et al., 2022). A series of negative effects caused serious damage to plant yield (King et al., 2015). It is estimated that the yield of rice will decrease by 3%-8% for every 1°C increase in temperature (Paul et al., 2020). With the continuous change of the global climate, it can be expected that extreme high temperatures will occur more frequently. Therefore, improving the heat tolerance of plants to improve the stable yield is one of the bases to ensure the cultivation of high light efficiency crops.
Plant response to heat stress (HS)
Signal transmission mechanism in plants
Plants need to convert external temperature changes into internal molecular signals to induce their own heat shock response (HSR). In higher plants, photoreceptors including phytochrome and cryptochrome may be the potential temperature sensors of plants (Miyazaki et al., 2015; Jung et al., 2016; Legris et al., 2016; Fujii et al., 2017). These photoreceptors change their activity through temperature changes, participate in the regulation of various temperature signaling pathways or directly regulate the temperature sensitive response of plants Li et al., 2022. In addition, some transcription factors and regulatory elements also have the characteristics that their activity changes with temperature, and also have the potential as temperature sensors. These features include changes in DNA/chromatin structure, variable splicing of mRNA, changes in RNA secondary structure, etc., which have also been reported (Nusinow et al., 2011; Vu et al., 2019; Perrella et al., 2022). However, most of the temperature receptors reported and identified at present are to regulate the morphological change or developmental transition process of plants under warm-temperature environment, and only one case of temperature receptors tolerant to extreme high temperature has been reported (Zhang et al., 2022).
Ca2+signaling is considered to be the fastest signal response in plants (Kan and Lin, 2021). HS affects the fluidity of plasma membrane and causes a large amount of internal flow of Ca2+ in a short time. It can reach the peak in a short period of 15s, thus rapidly transmitting the external temperature signal (Saidi et al., 2010). The elevated Ca2+ level in the cytoplasm induces various stress responses to tolerant high temperature by activating or inhibiting the activities of Ca2+/CAM related kinases, phosphatases and transcription factors. In addition, reactive oxygen species (ROS) have also recently been reported to be involved in the heat shock response of plants, which activates the plant’s overall defense mechanism by stimulating stressed tissues to generate a series of responses while transmitting stress signals from stressed to non-stressed areas (Zandalinas et al., 2020).
Heat shock protein
When HS occurs, the normal function of the endoplasmic reticulum is affected, resulting in a large accumulation of unfolded and misfolded proteins, resulting in cytotoxicity. Meanwhile, plants will activate the transcription of heat shock factor (HSF) and induce a large number of heat shock proteins (HSPs). This kind of special proteins with molecular chaperone activity can maintain the thermal stability of other proteins and ensure that other proteins perform correct post-translational folding. Some HSPs also play a role in the degradation of abnormal proteins and non-functional proteins (Taipale et al., 2010; Usman et al., 2017; Kang et al., 2022). HSP is considered to be one of the most important defense responses of plants under HS, which can significantly improve the survival rate of plants. Moreover, many HSPs are not only induced by high temperature conditions, but also activated by various other stresses such as hypoxia, drought and heavy metal ions, thus becoming an important part of plant tolerance under various abiotic stresses.
HS memory
Due to climate change, high temperature usually occurs temporarily and repeatedly. In order to cope with this situation, plants have the ability to maintain and acquire heat tolerance at the end of HS events, so that plants can respond to repeated HS events more quickly, which is called HS memory (Charng et al., 2006). HS memory includes two types, namely type I and type II (Oberkofler et al., 2021). Both types guarantee that the genes corresponding to them are able to be continuously induced or show an enhancement of re-induction at the end of HS. This physiological response is induced by some key HSFs (such as HSFA2) and involves histone methylation around memory genes and the maintenance of low nucleosome occupancy, thus ensuring its efficient induction (Perrella et al., 2022). HS memory can last up to 5-6 days in Arabidopsis, which significantly improves the tolerance of plants to high temperature (Liu et al., 2018).
Breeding heat tolerant rice by genetic methods
Directional genetic improvement of rice by molecular biology has significant advantages over traditional breeding methods, which can greatly shorten the breeding period and ensure the accurate improvement of target traits. With the help of heat-tolerant germplasm resources including N22, various means were used to explore the molecular mechanism of rice HSR and identify relevant genes/QTLs, so as to realize the direct regulation of rice heat response pathway. At present, a large number of heat resistance related genes have been identified, which has provided some help for heat resistance breeding of rice. However, the number of successfully cloned genes is very limited (Table 1) (Rabara et al., 2021). Therefore, it is still the focus of rice heat tolerance research to successfully clone the identified heat tolerance genes and make them be used in breeding practice.
In recent years, with the development of molecular biology, some regulatory elements led by E3 ubiquitin ligase and microRNA have been revealed to participate in the regulation of heat tolerance in rice and other plants (Ning et al., 2011; Song et al., 2013; Cui et al., 2017). Different from the genes/QTLs that directly affect the phenotype of plant traits, these stress response factors cause the decrease of gene expression or the change of protein abundance through regulating various metabolic pathways, and ultimately affect heat tolerance of plants. This undoubtedly provides a new channel for breeding rice with heat tolerance. However, although many heat tolerance related regulatory loci and QTLs have been reported, these studies cannot further improve rice yield (Khan et al., 2019; Rabara et al., 2021). In other words, these studies can only guarantee the stability of rice yield under various high temperature conditions. Due to the rapid growth of the global population, the current grain production has been difficult to meet the future demand. It is difficult to achieve the breeding goal of increasing production simply by improving heat resistance. A few studies have identified some genes/QTLs that simultaneously affect rice heat tolerance and yield, but few of them have been successfully cloned (Wang et al., 2016b; El-Esawi and Alayafi, 2019; Lo et al., 2020). The ultimate goal of heat-resistant breeding is to make the rice yield meet the increasing food demand under future climate conditions. Therefore, it is necessary to take yield improvement into account when studying heat resistance.
Improve breeding efficiency
Although the current use of marker-assisted selection (MAS) to aggregate the main effect QTL or gene of the target trait has significantly improved the breeding efficiency compared with the traditional methods, heat tolerance of rice is a complex trait controlled by multi minor-effect gene loci. Thus, the aggregation of several major-effect genes alone does not result in a very ideal phenotype, which limits the further improvement of breeding efficiency (Spindel et al., 2015).
Genome selection (GS) refers to a method of selection using high-density molecular genetic markers covering the whole genome to calculate genome estimated breeding value (Meuwissen et al., 2001). This method is still a kind of MAS method in essence, but it has many advantages compared with general MAS breeding (Varshney et al., 2020). First, GS allows early selection without detecting for major effect genes affecting the target trait and frees the reliance of traditional breeding on phenotypic information with increased accuracy. Moreover, GS has successfully realized the identification and selection of many small effect genes, which is more conducive to the improvement of complex traits (Newell and Jannink, 2014). Finally, compared with the traditional MAS method, GS is more effective in improving traits with low heritability and difficult to measure (Yu et al., 2022). It can be seen that if GS can be applied to improve heat tolerance of rice, it will reduce or even get rid of the phenotypic identification, improve the accuracy of selection, further improve the breeding efficiency, and have a broad application prospect.
Summary
Photosynthesis, as a direct source of plant yield, has received extensive attention. Many studies hope to further enhance the accumulation of plant assimilates by improving photosynthetic efficiency. The photosynthesis of plants is affected by both itself and the environment. Due to the limitation of nitrogen assimilation and source sink, the improvement of plant photosynthetic efficiency by changing the environmental CO2 concentration is very limited. It is expected that future research will still focus on the genetic improvement of plants. The improvement of photosynthetic pathway or components and the introduction of C4 pathway are the main research focuses at present. The former has been reported in a large number, but the latter is still at an early stage of exploration. In order to realize plant C3-C4 engineering, the spatial separation of photosynthesis and Kranz structure are the two key problems to be solved, and the utilization of some C3-C4 intermediates point out the promising direction. In addition, with the global warming and the frequent occurrence of extreme weather, high temperature has increasingly become one of the important factors limiting plant yield. HS almost affects all physiological processes of plants, including photosynthesis, and even causes plant death in serious cases. Therefore, cultivating heat tolerant rice under the current climate conditions has become one of the important objectives of breeding. At present, the related genes successfully cloned are limited, which makes it difficult to cultivate heat-resistant rice. Therefore, it will be one of the key problems for future research to apply heat-resistant genes to breeding practice. Moreover, because most of the current studies have little relationship with rice yield traits, different genes/QTLs affecting yield and heat tolerance have to be considered simultaneously in the breeding process, which undoubtedly increases the burden of breeding work and extends the breeding period. It is expected that how to strengthen the relationship between heat tolerance and rice yield will be an important issue to be solved in the future to improve breeding efficiency.
Author contributions
QS and YX jointly wrote this mini review, XQ helped to revise the manuscript, and JY conceived and guided the writing of the manuscript.
Funding
This work was financially supported by grants from the National Natural Science Foundation of China (32171931) and the open project from State Key Laboratory of Rice Biology (160102) to JY.
Conflict of interest
The authors declare that the research was conducted in the absence of any commercial or financial relationships that could be construed as a potential conflict of interest.
Publisher’s note
All claims expressed in this article are solely those of the authors and do not necessarily represent those of their affiliated organizations, or those of the publisher, the editors and the reviewers. Any product that may be evaluated in this article, or claim that may be made by its manufacturer, is not guaranteed or endorsed by the publisher.
References
Araus, J. L., Kefauver, S. C., Zaman-Allah, M., Olsen, M. S., Cairns, J. E. (2018). Translating high-throughput phenotyping into genetic gain. Trends Plant Sci. 23 (5), 451–466. doi: 10.1016/j.tplants.2018.02.001
Asim, M., Hussain, Q., Wang, X., Sun, Y., Liu, H., Khan, R., et al. (2022). Mathematical modeling reveals that sucrose regulates leaf senescence via dynamic sugar signaling pathways. Int. J. Mol. Sci. 23 (12), 6498. doi: 10.3390/ijms23126498
Atkinson, N., Feike, D., Mackinder, L. C., Meyer, M. T., Griffiths, H., Jonikas, M. C., et al. (2016). Introducing an algal carbon-concentrating mechanism into higher plants: location and incorporation of key components. Plant Biotechnol. J. 14, 1302–1315. doi: 10.1111/pbi.12497
Brown, N. J., Parsley, K., Hibberd, J. M. (2005). The future of C4 research–maize, flaveria or cleome? Trends Plant Sci. 10, 215–221. doi: 10.1016/j.tplants.2005.03.003
Cao, Z., Tang, H., Cai, Y., Zeng, B., Zhao, J., Tang, X., et al. (2022). Natural variation of HTH5 from wild rice, oryza rufipogon griff., is involved in conferring high-temperature tolerance at the heading stage. Plant Biotechnol. J. 20 (8), 1591–1605. doi: 10.1111/pbi.13835
Charng, Y. Y., Liu, H. C., Liu, N. Y., Hsu, F. C., Ko, S. S. (2006). Arabidopsis Hsa32, a novel heat shock protein, is essential for acquired thermotolerance during long recovery after acclimation. Plant Physiol. 140, 1297–1305. doi: 10.1104/pp.105.074898
Chen, F., Dong, G., Wang, F., Shi, Y., Zhu, J., Zhang, Y., et al. (2021). A β-ketoacyl carrier protein reductase confers heat tolerance via the regulation of fatty acid biosynthesis and stress signaling in rice. New Phytol. 232 (2), 655–672. doi: 10.1111/nph.17619
Cheng, G., Wang, L., Lan, H. (2016). Cloning of PEPC-1 from a C4 halophyte suaeda aralocaspica without kranz anatomy and its recombinant enzymatic activity in responses to abiotic stresses. Enzyme Microb. Technol. 83, 57–67. doi: 10.1016/j.enzmictec.2015.11.006
Chen, Z. F., Kang, X. P., Nie, H. M., Zheng, S. W., Zhang, T. L., Zhou, D., et al. (2019). Introduction of exogenous glycolate catabolic pathway can strongly enhances photosynthesis and biomass yield of cucumber grown in a low-CO2 environment. Front. Plant Sci. 10. doi: 10.3389/fpls.2019.00702
Cui, H. (2021). Challenges and approaches to crop improvement through C3-to-C4 engineering. Front. Plant Sci. 12. doi: 10.3389/fpls.2021.715391
Cui, J., You, C., Chen, X. (2017). The evolution of microRNAs in plants. Curr. Opin. Plant Biol. 35, 61–67. doi: 10.1016/j.pbi.2016.11.006
Da Costa, M. V. J., Ramegowda, V., Ramakrishnan, P., Nataraja, K. N., Sheshshayee, M. S. (2022). Comparative metabolite profiling of rice contrasts reveal combined drought and heat stress signatures in flag leaf and spikelets. Plant Sci. 320, 111262. doi: 10.1016/j.plantsci.2022.111262
Danila, F. R., Quick, W. P., White, R. G., Furbank, R. T., von Caemmerer, S. (2016). The metabolite pathway between bundle sheath and mesophyll: Quantification of plasmodesmata in leaves of C3 and C4 monocots. Plant Cell 28, 1461–1471. doi: 10.1105/tpc.16.00155
Drake, B. G., Gonzalez-Meler, M. A., Long, S. P. (1997). MORE EFFICIENT PLANTS a consequence of rising atmospheric CO2? Annu. Rev. Plant Biol. 48, 609–639. doi: 10.1146/annurev.arplant.48.1.609
El-Esawi, M. A., Alayafi, A. A. (2019). Overexpression of rice Rab7 gene improves drought and heat tolerance and increases grain yield in rice (Oryza sativa l.). Genes 10 (1), 56. doi: 10.3390/genes10010056
Ermakova, M., Arrivault, S., Giuliani, R., Danila, F., Alonso-Cantabrana, H., Vlad, D., et al. (2021). Installation of C4 photosynthetic pathway enzymes in rice using a single construct. Plant Biotechnol. J. 19 (3), 575–588. doi: 10.1111/pbi.13487
Feldman, A. B., Leung, H., Baraoidan, M., Elmido-Mabilangan, A., Canicosa, I., Quick, W. P., et al. (2017). Increasing leaf vein density via mutagenesis in rice results in an enhanced rate of photosynthesis, smaller cell sizes and can reduce interveinal mesophyll cell number. Front. Plant Sci. 8. doi: 10.3389/fpls.2017.01883
Fujii, Y., Tanaka, H., Konno, N., Ogasawara, Y., Hamashima, N., Tamura, S., et al. (2017). Phototropin perceives temperature based on the lifetime of its photoactivated state. Proc. Natl. Acad. Sci. U.S.A. 114, 9206–9211. doi: 10.1073/pnas.1704462114
Furbank, R. T. (2017). Walking the C4 pathway: Past, present, and future. J. Exp. Bot. 68, 4057–4066. doi: 10.1093/jxb/erx006
Hanson, M. R., Lin, M. T., Carmo-Silva, A. E., Parry, M. A. (2016). Towards engineering carboxysomes into C3 plants. Plant J. 87, 38–50. doi: 10.1111/tpj.13139
Häusler, R. E., Rademacher, T., Li, J., Lipka, V., Fischer, K. L., Schubert, S., et al. (2001). Single and double overexpression of C(4)-cycle genes had differential effects on the pattern of endogenous enzymes, attenuation of photorespiration and on contents of UV protectants in transgenic potato and tobacco plants. J. Exp. Bot. 52, 1785–1803. doi: 10.1093/jexbot/52.362.1785
Hein, N. T., Ciampitti, I. A., Jagadish, S. V. K. (2021). Bottlenecks and opportunities in field-based high-throughput phenotyping for heat and drought stress. J. Exp. Bot. 72, 5102–5116. doi: 10.1093/jxb/erab021
He, Y., Zhang, X., Shi, Y., Xu, X., Li, L., Wu, J. L. (2021). PREMATURE SENESCENCE LEAF 50 promotes heat stress tolerance in rice (Oryza sativa l.). Rice (New York N.Y.) 14 (1), 53. doi: 10.1186/s12284-021-00493-w
Ishimaru, K., Ohkawa, Y., Ishige, T., Tobias, D. J., Ohsugi, R. (1998). Elevated pyruvate, orthophosphate dikinase (PPDK) activity alters carbon metabolism in C3 transgenic potatoes with a C4 maize PPDK gene. Physiol. Plant 103, 340–346. doi: 10.1034/j.1399-3054.1998.1030306.x
Jansson, C., Vogel, J., Hazen, S., Brutnell, T., Mockler, T. (2018). Climate-smart crops with enhanced photosynthesis. J. Exp. Bot. 69, 3801–3809. doi: 10.1093/jxb/ery213
Jung, J., Domijan, M., Klose, C., Biswas, S., Ezer, D., Gao, M., et al. (2016). Phytochromes function as thermosensors in arabidopsis. Science 354, 886–889. doi: 10.1126/science.aaf6005
Kandoi, D., Ruhil, K., Govindjee, G., Tripathy, B. C. (2022). Overexpression of cytoplasmic C4 flaveria bidentis carbonic anhydrase in C3 arabidopsis thaliana increases amino acids, photosynthetic potential, and biomass. Plant Biotechnol. J 20(8), 1518–1532. doi: 10.1111/pbi.13830
Kang, Y., Lee, K., Hoshikawa, K., Kang, M., Jang, S. (2022). Molecular bases of heat stress responses in vegetable crops with focusing on heat shock factors and heat shock proteins. Front. Plant Sci. 13. doi: 10.3389/fpls.2022.837152
Kan, Y., Lin, H. X. (2021). Molecular regulation and genetic control of rice thermal response. Crop J. 9, 497–505. doi: 10.1016/j.cj.2021.02.008
Kan, Y., Mu, X. R., Zhang, H., Gao, J., Shan, J. X., Ye, W. W., et al. (2022). TT2 controls rice thermotolerance through SCT1-dependent alteration of wax biosynthesis. Nat. Plants 8 (1), 53–67. doi: 10.1038/s41477-021-01039-0
Kebeish, R., Niessen, M., Thiruveedhi, K., Bari, R., Hirsch, H. J., Rosenkranz, R., et al. (2007). Chloroplastic photorespiratory bypass increases photosynthesis and biomass production in arabidopsis thaliana. Nat. Biotechnol. 25, 593–599. doi: 10.1038/nbt1299
Keerberg, O., Parnik, T., Ivanova, H., Bassuner, B., Bauwe, H. (2014). C2 photosynthesis generates about 3-fold elevated leaf CO2 levels in the C3-C4 intermediate species flaveria pubescens. J. Exp. Bot. 65, 3649–3656. doi: 10.1093/jxb/eru239
Khan, S., Anwar, S., Ashraf, M. Y., Khaliq, B., Sun, M., Hussain, S., et al. (2019). Mechanisms and adaptation strategies to improve heat tolerance in rice. A review. Plants (Basel Switzerland) 8 (11), 508. doi: 10.3390/plants8110508
Khan, A., Jalil, S., Cao, H., Tsago, Y., Sunusi, M., Chen, Z., et al. (2020). The purple leaf (pl6) mutation regulates leaf color by altering the anthocyanin and chlorophyll contents in rice. Plants (Basel) 9, 1477. doi: 10.3390/plants9111477
Khoshravesh, R., Stinson, C. R., Stata, M., Busch, F. A., Sage, R. F., Ludwig, M., et al. (2016). C3-C4 intermediacy in grasses: Organelle enrichment and distribution, glycine decarboxylase expression, and the rise of C2 photosynthesis. J. Exp. Bot. 67, 3065–3078. doi: 10.1093/jxb/erw150
King, A. D., Donat, M. G., Fischer, E. M., Hawkins, E., Alexander, L. V., Karoly, D. J., et al. (2015). The timing of anthropogenic emergence in simulated climate extremes. Environ. Res. Lett. 10 (9), 094015. doi: 10.1088/1748-9326/10/9/094015
Langdale, J. A. (2011). C4 cycles: past, present, and future research on C4 photosynthesis. Plant Cell 23, 3879–3892. doi: 10.1105/tpc.111.092098
Legris, M., Klose, C., Burgie, E. S., Rojas, C. C. R., Neme, M., Hiltbrunner, A., et al. (2016). Phytochrome b integrates light and temperature signals in arabidopsis. Science 354, 897–900. doi: 10.1126/science.aaf5656
Leister, D. (2022). Enhancing the light reactions of photosynthesis: strategies, controversies and perspectives. Mol. Plant S1674-2052 (22), 00268–00264. doi: 10.1016/j.molp.2022.08.005
Lian, L., Wang, X., Zhu, Y., He, W., Cai, Q., Xie, H., et al. (2014). Physiological and photosynthetic characteristics of indica Hang2 expressing the sugarcane PEPC gene. Mol. Biol. Rep. 41, 2189–2197. doi: 10.1007/s11033-014-3070-4
Li, X. M., Chao, D. Y., Wu, Y., Huang, X., Chen, K., Cui, L. G., et al. (2015). Natural alleles of a proteasome α2 subunit gene contribute to thermotolerance and adaptation of African rice. Nat. Genet. 47 (7), 827–833. doi: 10.1038/ng.3305
Li, X., Liang, T., Liu, H. (2022). How plants coordinate their development in response to light and temperature signals. Plant Cell 34, 955–966. doi: 10.1093/plcell/koab302
Lin, M. T., Occhialini, A., Andralojc, P. J., Parry, M. A., Hanson, M. R. (2014). A faster rubisco with potential to increase photosynthesis in crops. Nature 513, 547–550. doi: 10.1038/nature13776
Liu, H. C., Lamke, J., Lin, S. Y., Hung, M. J., Liu, K. M., Charng, Y. Y., et al. (2018). Distinct heat shock factors and chromatin modifications mediate the organ-autonomous transcriptional memory of heat stress. Plant J. 95, 401–413. doi: 10.1111/tpj.13958
Lobell, D. B., Schlenker, W., Costa-Roberts, J. (2011). Climate trends and global crop production since 1980. Science 333 (6042), 616–620. doi: 10.1126/science.1204531
Lo, S. F., Cheng, M. L., Hsing, Y. C., Chen, Y. S., Lee, K. W., Hong, Y. F., et al. (2020). Rice big grain 1 promotes cell division to enhance organ development, stress tolerance and grain yield. Plant Biotechnol. J. 18 (9), 1969–1983. doi: 10.1111/pbi.13357
Long, S. P. (1991). Modification of the response of photosynthetic productivity to rising temperature by atmospheric CO2 concentrations: Has its importance been underestimated? Plant Cell Environ. 14, 729–739. doi: 10.1111/j.1365-3040.1991.tb01439.x
Long, S. P., Marshall-Colon, A., Zhu, X. G. (2015). Meeting the global food demand of the future by engineering crop photosynthesis and yield potential. Cell 161, 56–66. doi: 10.1016/j.cell.2015.03.019
Lundgren, M. R., Osborne, C. P., Christin, P. A. (2014). Deconstructing kranz anatomy to understand C4 evolution. J. Exp. Bot. 65, 3357–3369. doi: 10.1093/jxb/eru186
Marshall, D. M., Muhaidat, R., Brown, N. J., Liu, Z., Stanley, S., Griffiths, H., et al. (2007). Cleome, a genus closely related to arabidopsis, contains species spanning a developmental progression from C(3) to C(4) photosynthesis. Plant J. 51, 886–896. doi: 10.1111/j.1365-313X.2007.03188.x
Mathan, J., Singh, A., Jathar, V., Ranjan, A. (2021). High photosynthesis rate in two wild rice species is driven by leaf anatomy mediating high rubisco activity and electron transport rate. J. Exp. Bot. 72, 7119–7135. doi: 10.1093/jxb/erab313
Meuwissen, T. H. E., Hayes, B. J., Goddard, M. E. (2001). Prediction of total genetic value using genome-wide dense marker maps. Genetics 157, 1819–1829. doi: 10.1093/genetics/157.4.1819
Miyazaki, Y., Takase, T., Kiyosue, T. (2015). ZEITLUPE positively regulates hypocotyl elongation at warm temperature under light in arabidopsis thaliana. Plant Signal Behav. 10, e998540. doi: 10.1080/15592324.2014.998540
Nayak, L., Panda, D., Dash, G. K., Lal, M. K., Swain, P., Baig, M. J., et al. (2022). A chloroplast glycolate catabolic pathway bypassing the endogenous photorespiratory cycle enhances photosynthesis, biomass and yield in rice (Oryza sativa l.). Plant Sci. 314, 111103. doi: 10.1016/j.plantsci.2021.111103
Newell, M. A., Jannink, J. L. (2014). Genomic selection in plant breeding. Methods Mol. Biol. 1145, 117–130. doi: 10.1007/978-1-4939-0446-4_10
Ning, Y., Jantasuriyarat, C., Zhao, Q., Zhang, H., Chen, S., Liu, J., et al. (2011). The SINA E3 ligase OsDIS1 negatively regulates drought response in rice. Plant Physiol. 157, 242–255. doi: 10.1104/pp.111.180893
Nolke, G., Houdelet, M., Kreuzaler, F., Peterhansel, C., Schillberg, S. (2014). The expression of a recombinant glycolate dehydrogenase polyprotein in potato (Solanum tuberosum) plastids strongly enhances photosynthesis and tuber yield. Plant Biotechnol. J. 12, 734–742. doi: 10.1111/pbi.12178
Nusinow, D. A., Helfer, A., Hamilton, E. E., King, J. J., Imaizumi, T., Schultz, T. F., et al. (2011). The ELF4-ELF3-LUX complex links the circadian clock to diurnal control of hypocotyl growth. Nature 475(7356), 398–402. doi: 10.1038/nature10182
Oberkofler, V., Pratx, L., Baurle, I. (2021). Epigenetic regulation of abiotic stress memory: Maintaining the good things while they last. Curr. Opin. Plant Biol. 61, 102007. doi: 10.1016/j.pbi.2021.102007
Pang, C., Zhang, W., Peng, M., Zhao, X., Shi, R., Wu, X., et al. (2022). Fine mapping and characterization of a major gene responsible for chlorophyll biosynthesis in brassica napus l. Biomolecules 12 (3), 402. doi: 10.3390/biom12030402
Park, J. R., Yang, W. T., Kim, D. H., Kim, K. M. (2020). Identification of a novel gene, osbht, in response to high temperature tolerance at booting stage in rice. Int. J. Mol. Sci. 21 (16), 5862. doi: 10.3390/ijms21165862
Parry, M. A., Andralojc, P. J., Scales, J. C., Salvucci, M. E., Carmo-Silva, A. E., Alonso, H., et al. (2013). Rubisco activity and regulation as targets for crop improvement. J. Exp. Bot. 64, 717–730. doi: 10.1093/jxb/ers336
Paul, P., Dhatt, B. K., Sandhu, J., Hussain, W., Irvin, L., Morota, G., et al. (2020). Divergent phenotypic response of rice accessions to transient heat stress during early seed development. Plant Direct 4, e00196. doi: 10.1002/pld3.196
Pellicer, M. T., Badía, J., Aguilar, J., Baldomà, L. (1996). Glc locus of escherichia coli: characterization of genes encoding the subunits of glycolate oxidase and the glc regulator protein. J. bacteriol. 178, 2051–2059. doi: 10.1128/jb.178.7.2051-2059.1996
Perrella, G., Baurle, I., van Zanten, M. (2022). Epigenetic regulation of thermomorphogenesis and heat stress tolerance. New Phytol. 234, 1144–1160. doi: 10.1111/nph.17970
Prasertthai, P., Paethaisong, W., Theerakulpisut, P., Dongsansuk, A. (2022). High temperature alters leaf lipid membrane composition associated with photochemistry of PSII and membrane thermostability in rice seedlings. Plants (Basel) 11 (11), 1454. doi: 10.3390/plants11111454
Rabara, R. C., Msanne, J., Basu, S., Ferrer, M. C., and Roychoudhury, A. (2021). Coping with inclement weather conditions due to high temperature and water deficit in rice: An insight from genetic and biochemical perspectives. Physiologia plantarum 172, 487–504. doi: 10.1111/ppl.13272
Sage, R. F., Khoshravesh, R., Sage, T. L. (2014). From proto-kranz to C4 kranz: building the bridge to C4 photosynthesis. J. Exp. Bot. 65, 3341–3356. doi: 10.1093/jxb/eru180
Saidi, Y., Peter, M., Finka, A., Cicekli, C., Vigh, L., Goloubinoff, P. (2010). Membrane lipid composition affects plant heat sensing and modulates Ca(2+)-dependent heat shock response. Plant Signal Behav. 5, 1530–1533. doi: 10.4161/psb.5.12.13163
Singer, S. D., Soolanayakanahally, R. Y., Foroud, N. A., Kroebel, R. (2019). Biotechnological strategies for improved photosynthesis in a future of elevated atmospheric CO2. Planta 251, 24. doi: 10.1007/s00425-019-03301-4
Song, J. B., Gao, S., Sun, D., Li, H., Shu, X. X., Yang, Z. M. (2013). miR394 and LCR are involved in arabidopsis salt and drought stress responses in an abscisic acid-dependent manner.pdf. BMC Plant Biol. 13, 210. doi: 10.1186/1471-2229-13-210
Song, Q., Wang, Y., Qu, M., Ort, D. R., Zhu, X. G. (2017). The impact of modifying photosystem antenna size on canopy photosynthetic efficiency-development of a new canopy photosynthesis model scaling from metabolism to canopy level processes. Plant Cell Environ. 40 (12), 2946–2957. doi: 10.1111/pce.13041
Spindel, J., Begum, H., Akdemir, D., Virk, P., Collard, B., Redoña, E, et al. (2015). Genomic selection and association mapping in rice (Oryza sativa): effect of trait genetic architecture, training population composition, marker number and statistical model on accuracy of rice genomic selection in elite, tropical rice breeding lines. PLoS genetics 11 (2), e1004982. doi: 10.1371/journal.pgen.1004982
Stratonovitch, P., Semenov, M. A. (2015). Heat tolerance around flowering in wheat identified as a key trait for increased yield potential in Europe under climate change. J. Exp. Bot. 66 (12), 3599–3609. doi: 10.1093/jxb/erv070
Taipale, M., Jarosz, D. F., Lindquist, S. (2010). HSP90 at the hub of protein homeostasis: emerging mechanistic insights. Nat. Rev. Mol. Cell Biol. 11, 515–528. doi: 10.1038/nrm2918
Tang, Y., Fang, Z., Liu, M., Zhao, D., Tao, J. (2020). Color characteristics, pigment accumulation and biosynthetic analyses of leaf color variation in herbaceous peony (Paeonia lactiflora pall.). 3 Biotech. 10, 76. doi: 10.1007/s13205-020-2063-3
Taniguchi, Y., Ohkawa, H., Masumoto, C., Fukuda, T., Tamai, T., Lee, K., et al. (2008). Overproduction of C4 photosynthetic enzymes in transgenic rice plants: An approach to introduce the C4-like photosynthetic pathway into rice. J. Exp. Bot. 59, 1799–1809. doi: 10.1093/jxb/ern016
Tayade, R., Yoon, J., Lay, L., Khan, A. L., Yoon, Y., Kim, Y. (2022). Utilization of spectral indices for high-throughput phenotyping. Plants 11 (13), 1712. doi: 10.3390/plants11131712
Tcherkez, G. G., Farquhar, G. D., Andrews, T. J. (2006). Despite slow catalysis and confused substrate specificity, all ribulose bisphosphate carboxylases may be nearly perfectly optimized. Proc. Natl. Acad. Sci. U.S.A. 103, 7246–7251. doi: 10.1073/pnas.0600605103
Usman, M. G., Rafii, M. Y., Martini, M. Y., Yusuff, O. A., Ismail, M. R., Miah, G. (2017). Molecular analysis of Hsp70 mechanisms in plants and their function in response to stress. Biotechnol. Genet. Eng. Rev. 33, 26–39. doi: 10.1080/02648725.2017.1340546
Varshney, R. K., Bohra, A., Yu, J., Graner, A., Zhang, Q., Sorrells, M. E. (2021). Designing future crops: Genomics-assisted breeding comes of age. Trends Plant Sci. 26 (6), 631–649. doi: 10.1016/j.tplants.2021.03.010
Varshney, R. K., Sinha, P., Singh, V. K., Kumar, A., Zhang, Q., Bennetzen, J. L. (2020). 5Gs for crop genetic improvement. Curr. Opin. Plant Biol. 56, 190–196. doi: 10.1016/j.pbi.2019.12.004
Vu, L. D., Gevaert, K., De Smet, I. (2019). Feeling the heat: Searching for plant thermosensors. Trends Plant Sci. 24 (3), 210–219. doi: 10.1016/j.tplants.2018.11.004
Wang, M., Lu, X., Xu, G., Yin, X., Cui, Y., Huang, L., et al. (2016b). OsSGL, a novel pleiotropic stress-related gene enhances grain length and yield in rice. Sci. Rep. 6, 38157. doi: 10.1038/srep38157
Wang, L., Peterson, R. B., Brutnell, T. P. (2011). Regulatory mechanisms underlying C4 photosynthesis. New Phytol. 190, 9–20. doi: 10.1111/j.1469-8137.2011.03649.x
Wang, D., Qin, B., Li, X., Tang, D., Zhang, Y., Cheng, Z., et al. (2016a). Nucleolar DEAD-box RNA helicase TOGR1 regulates thermotolerant growth as a pre-rRNA chaperone in rice. PloS Genet. 12 (2), e1005844. doi: 10.1371/journal.pgen.1005844
Wang, L. M., Shen, B. R., Li, B. D., Zhang, C. L., Lin, M., Tong, P. P., et al. (2020). A synthetic photorespiratory shortcut enhances photosynthesis to boost biomass and grain yield in rice. Mol. Plant 13, 1802–1815. doi: 10.1016/j.molp.2020.10.007
Wang, Y.-M., Xu, W.-G., Hu, L., Zhang, L., Li, Y., Du, X.-H. (2012). Expression of maize gene encoding C4-pyruvate orthophosphate dikinase (PPDK) and C4-phosphoenolpyruvate carboxylase (PEPC) in transgenic arabidopsis. Plant Mol. Biol. Rep. 30, 1367–1374. doi: 10.1007/s11105-012-0451-5
Wu, C., Cui, K., Wang, W., Li, Q., Fahad, S., Hu, Q., et al. (2016a). Heat-induced phytohormone changes are associated with disrupted early reproductive development and reduced yield in rice. Sci. Rep. 6, 34978. doi: 10.1038/srep34978
Wu, X., Ding, D., Shi, C., Xue, Y., Zhang, Z., Tang, G., et al. (2016b). microRNA-dependent gene regulatory networks in maize leaf senescence. BMC Plant Biol. 16, 73. doi: 10.1186/s12870-016-0755-y
Xia, S., Liu, H., Cui, Y., Yu, H., Rao, Y., Yan, Y., et al. (2022). UDP-N-acetylglucosamine pyrophosphorylase enhances rice survival at high temperature. New Phytol. 233 (1), 344–359. doi: 10.1111/nph.17768
Xiong, Z., Dun, Z., Wang, Y., Yang, D., Xiong, D., Cui, K., et al. (2022). Effect of stomatal morphology on leaf photosynthetic induction under fluctuating light in rice. Front. Plant Sci. 12. doi: 10.3389/fpls.2021.754790
Xu, Y., Zhang, L., Ou, S., Wang, R., Wang, Y., Chu, C., et al. (2020). Natural variations of SLG1 confer high-temperature tolerance in indica rice. Nat. Commun. 11 (1), 5441. doi: 10.1038/s41467-020-19320-9
Xu, P., Wu, T., Ali, A., Zhang, H., Liao, Y., Chen, X., et al. (2022). EARLY MORNING FLOWERING1 (EMF1) regulates the floret opening time by mediating lodicule cell wall formation in rice. Plant Biotechnol. J. 20 (8), 1441–1443. doi: 10.1111/pbi.13860
Yadav, S., Mishra, A. (2020). Ectopic expression of C4 photosynthetic pathway genes improves carbon assimilation and alleviate stress tolerance for future climate change. Physiol. Mol. Biol. Plants 26, 195–209. doi: 10.1007/s12298-019-00751-8
Yang, Y., Xu, J., Huang, L., Leng, Y., Dai, L., Rao, Y., et al. (2016). PGL, encoding chlorophyllide a oxygenase 1, impacts leaf senescence and indirectly affects grain yield and quality in rice. J. Exp. Bot. 67 (5), 1297–1310. doi: 10.1093/jxb/erv529
Yu, S., Ali, J., Zhou, S., Ren, G., Xie, H., Xu, J., et al. (2022). From green super rice to green agriculture: Reaping the promise of functional genomics research. Mol. Plant 15, 9–26. doi: 10.1016/j.molp.2021.12.001
Yu, K., Wang, J., Sun, C., Liu, X., Xu, H., Yang, Y., et al. (2020). High-density QTL mapping of leaf-related traits and chlorophyll content in three soybean RIL populations. BMC Plant Biol. 20, 470. doi: 10.1186/s12870-020-02684-x
Zandalinas, S. I., Fichman, Y., Devireddy, A. R., Sengupta, S., Azad, R. K., Mittler, R. (2020). Systemic signaling during abiotic stress combination in plants. Proc. Natl. Acad. Sci. U.S.A. 117, 13810–13820. doi: 10.1073/pnas.2005077117
Zarzycki, J., Axen, S. D., Kinney, J. N., Kerfeld, C. A. (2013). Cyanobacterial-based approaches to improving photosynthesis in plants. J. Exp. Bot. 64, 787–798. doi: 10.1093/jxb/ers294
Zhang, H., Zhou, J. F., Kan, Y., Shan, J. X., Ye, W. W., Dong, N. Q., et al. (2022). A genetic module at one locus in rice protects chloroplasts to enhance thermotolerance. Science 376, 1293–1300. doi: 10.1126/science.abo5721
Zhao, F., Lei, J., Wang, R., Zhang, Q., Qi, Y., Zhang, K., et al. (2022). Environmental determination of spring wheat yield in a climatic transition zone under global warming. Int. J. Biometeorol. 66, 481–491. doi: 10.1007/s00484-021-02196-9
Zhu, T., De Lima, C. F. F., De Smet, I. (2021). The heat is on: How crop growth, development and yield respond to high temperature. J. Exp. Bot 72 (21), 7359–7373. doi: 10.1093/jxb/erab308
Zhu, X. G., Long, S. P., Ort, D. R. (2008). What is the maximum efficiency with which photosynthesis can convert solar energy into biomass? Curr. Opin. Biotechnol. 19, 153–159. doi: 10.1016/j.copbio.2008.02.004
Keywords: smart rice, high light efficiency, C4 engineering, heat tolerance, breeding
Citation: Shen Q, Xie Y, Qiu X and Yu J (2022) The era of cultivating smart rice with high light efficiency and heat tolerance has come of age. Front. Plant Sci. 13:1021203. doi: 10.3389/fpls.2022.1021203
Received: 17 August 2022; Accepted: 16 September 2022;
Published: 07 October 2022.
Edited by:
Joseph Edwards, University of Texas at Austin, United StatesReviewed by:
Prachi Pandey, National Institute of Plant Genome Research (NIPGR), IndiaGuanjun Huang, Huazhong Agricultural University, China
Copyright © 2022 Shen, Xie, Qiu and Yu. This is an open-access article distributed under the terms of the Creative Commons Attribution License (CC BY). The use, distribution or reproduction in other forums is permitted, provided the original author(s) and the copyright owner(s) are credited and that the original publication in this journal is cited, in accordance with accepted academic practice. No use, distribution or reproduction is permitted which does not comply with these terms.
*Correspondence: Jinsheng Yu, amluc2h5dUB6YWZ1LmVkdS5jbg==
†These authors have contributed equally to this work